- 1Department of Biologic and Materials Sciences & Prosthodontics, School of Dentistry, University of Michigan, Ann Arbor, MI, United States
- 2Sensory Cells and Circuits Section, National Center for Complementary and Integrative Health, Bethesda, MD, United States
Somatosensory innervation of the oral cavity enables the detection of a range of environmental stimuli including minute and noxious mechanical forces. The trigeminal sensory neurons underlie sensation originating from the tooth. Prior work has provided important physiological and molecular characterization of dental pulp sensory innervation. Clinical dental experiences have informed our conception of the consequence of activating these neurons. However, the biological role of sensory innervation within the tooth is yet to be defined. Recent transcriptomic data, combined with mouse genetic tools, have the capacity to provide important cell-type resolution for the physiological and behavioral function of pulp-innervating sensory neurons. Importantly, these tools can be applied to determine the neuronal origin of acute dental pain that coincides with tooth damage as well as pain stemming from tissue inflammation (i.e., pulpitis) toward developing treatment strategies aimed at relieving these distinct forms of pain.
1 Introduction
Teeth have served mammals across their evolution, appearing in their fossil record as early as 140 million years ago (1–3). Most modern species of mammals have retained teeth to facilitate survival through the acquisition and processing of food (mastication) as well as for hunting and defense (1, 4, 5). While specific tooth morphology varies considerably across species, external hard enamel and dentin, along with dense internal sensory innervation, are common anatomical features (5).
Sensory innervation via the somatosensory nervous system enables us to perceive our external world by detecting environmental stimuli. Initial neuronal signals are generated at the peripheral terminals of primary somatosensory neurons (6, 7). These neurons transmit information encoded in neural patterns of impulses from the periphery to the central nervous system. Peripheral somatosensory innervation of the oral cavity, including the teeth, originates from sensory neurons with somas located in the trigeminal ganglia at the base of the skull (Note: sensory neurons in the dorsal root ganglia innervate the neck, trunk, and extremities).
Research over the past half-century has utilized neuroscience techniques to explore the role of tooth-pulp- and dentin-innervating sensory neurons (hereafter referred to as intradental neurons) in oral health and disease. This review will delve into the current understanding of intradental innervation, drawing from historical studies, insights from recent single-cell sequencing and transcriptomic methods, and proposed theories of intradental sensory transduction. To begin, we will summarize the tooth anatomy including our current understanding of the intradental sensory apparatus.
2 Tooth anatomy and intradental innervation
The tooth consists of four major tissues organized into layers (Figure 1A): (1) a central pulp that houses living cellular components including vasculature and dense nerve networks, (2) partially mineralized, porous dentin comprising the bulk of the crown and root tooth structure that contains nerve endings, (3) a hard mineralized enamel cap that protects the superficial crown and (4) a mineralized cementum layer that covers the root tooth surface and serves as an attachment to the surrounding alveolar bone via the periodontal ligament.
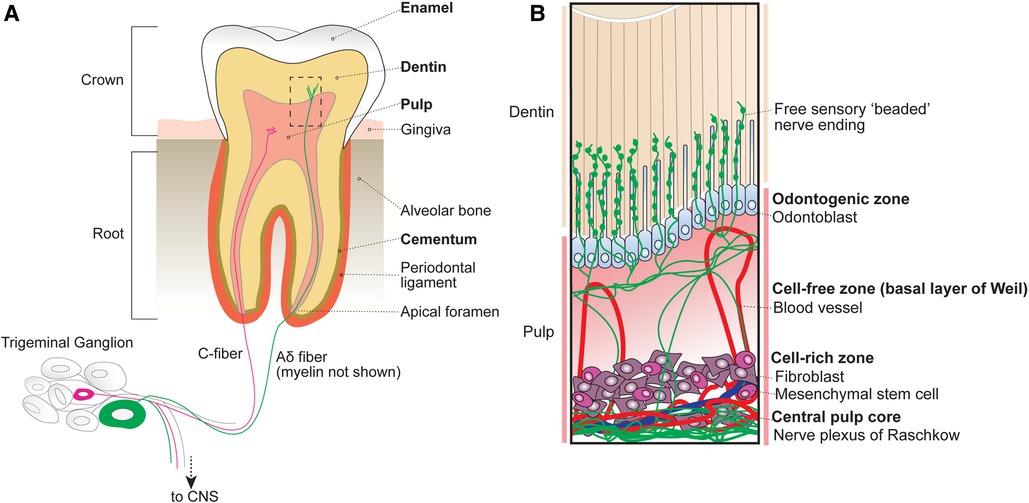
Figure 1. Anatomy of the tooth organ. (A) The tooth receives sensory innervation from peripheral sensory neurons whose cell bodies reside in the trigeminal ganglion at the base of the skull. Sensory afferents as well as blood vessels enter the tooth structure at the root apices via small openings known as apical foramen. Surrounding the pulp are several layers of mineralized dentin that support the tooth structure by dissipating external forces. After entering the root, sensory neurons either terminate within the central pulp of the tooth or extend into the inner dentin. The outermost portion of the exposed tooth surface (crown) is covered by protective enamel, which is the hardest substance produced in the human body. The dentin of the tooth roots is encapsulated by mineralized cementum that anchors the tooth within the alveolar bone via the periodontal ligament. Unlike the dentin and cementum layers that can be regenerated across life, enamel is finite. (B) Inset from box shown in A depicting the organization of cellular components within the tubular dentin and pulp. The outermost layer of the pulp contains the odontogenic zone, which is comprised of specialized dentin-maintaining polarized cells known as odontoblasts that extend processes into the overlying fluid-filled dentinal tubules. Free sensory endings radiate outwards from nerve bundles at the central pulp core (plexus of Raschkow), towards the occlusal pulp-dentin interface, with some endings extending at or beyond adjacent odontoblast processes. Tubular free nerve endings contain “beaded” swellings filled with synaptic machinery suggesting release of local signals, although their function is not understood. Beneath the pulpal odontogenic zone lies a cell-free zone (also known as the basal layer of Weil), which is largely absent of cellular components. The cell-free zone separates the odontogenic zone from the more central cell-rich zone. Here, mesenchymal stem cells function to replenish odontoblasts as well as local fibroblasts that function to form connective tissues within the pulp. Within the cell-rich zone lies the innermost central pulp core, where the nerve plexus of Raschkow as well as majority of pulp vasculature can be found.
2.1 Pulp
At the innermost part of the tooth lies the dental pulp, which houses living cellular components and unmineralized connective tissues. Based on cellular composition, the dental pulp is divided into 4 main zones from superficial to deep (Figure 1B): (1) odontogenic zone, (2) cell-free zone (basal layer of Weil), (3) cell-rich zone, and (4) central pulp core (8).
The peripheral odontogenic zone consists of specialized cells, odontoblasts, forming a barrier at the pulp-dentin interface. Odontoblasts actively secrete type I collagen and mineralizing agents to form and generate the overlying dentin (9, 10). Intriguingly, odontoblasts also extend a cellular process into the overlying dentin (11, 12). Below the odontogenic zone is the cell-free zone (also known as the basal layer of Weil), which is acellular aside from vasculature and free nerve endings (13). Beneath the cell-free zone lies the cell-rich zone, which houses the majority of pulpal cells including mesenchymal stem cells that replenish surrounding local fibroblasts, peripheral odontoblasts, and locally produced immune cells (14). At the centermost region of the pulp lies the pulp core, which is largely composed of fibroblasts, hosts most of the vasculature that maintains the pulp tissue, and the nerve plexus (8).
The central tooth pulp houses the majority of intradental innervation. Sensory innervation is supplied to the teeth via the superior and inferior alveolar nerve from the maxillary and mandibular divisions of the trigeminal ganglion, respectively (8, 12). Free nerve endings traverse from the center of the pulp into dentin from nerve bundles known as the Raschkow nerve plexus. Historically, sensory neurons have been classified based on morphological and electrophysiological characteristics, broadly dividing them into 3 categories (6): c-fibers (small-diameter, unmyelinated, slow conduction velocity), Aδ (medium-diameter, myelinated, intermediate conduction velocity), or Aβ (large-diameter, myelinated, fast conduction velocity) (Figure 2A). In humans, both large myelinated and small unmyelinated nerve endings enter the tooth root at a small opening known as the apical foramen (12, 15). Consequently, it has long been assumed that intradental sensory neurons include unmyelinated c-fibers, as well as larger, myelinated Aδ and/or Aβ trigeminal sensory neurons.
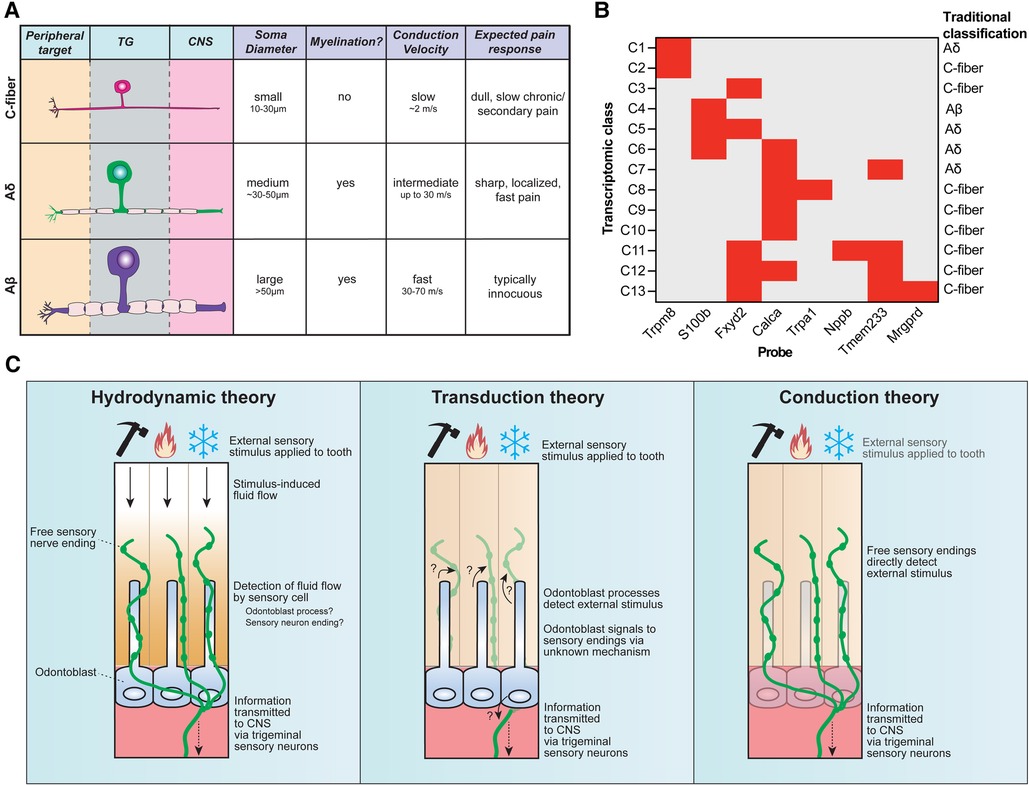
Figure 2. Sensory neuron diversity and proposed mechanisms of intradental sensitivity. (A) The traditional classification scheme of somatosensory neurons categorizes trigeminal sensory neurons into three main groups: c-fibers (small somal size, unmyelinated axons, and slow conduction velocity), Aδ (medium somal size, lightly myelinated axons, and intermediate conduction velocity), and Aβ (large somal size, myelinated axons, and fast conduction velocity). Stereotypical pain responses associated with each neuron class are also indicated. (B) Recent advances in single-cell RNA sequencing reveal that trigeminal sensory neurons consist of 13 distinct transcriptomic classes. Staining for 8 diagnostic markers via multiplexed in-situ hybridization enables class assignment of trigeminal neurons in tissue sections or whole mount ganglia (66). Graph depicts diagnostic in situ probes with corresponding transcriptomic class assignments, as well as how this relates to traditional neuron classes. (C) Three major theories have been proposed to underlie intradental sensitivity. (Left) According to the hydrodynamic theory, intradental sensory cells detect fluid movements within the dentin and/or pulp induced by external stimuli to give rise to tooth sensation. Tubular free sensory nerve endings and odontoblast processes are candidate sensory cells to detect stimulus-induced fluid flow. (Center) The transduction theory proposes that odontoblasts, not sensory neurons, function as the primary intradental sensory cells to detect external stimuli. (Right) The conduction theory proposes that tubular free sensory endings directly function as primary detectors of external stimuli. All 3 theories converge on the transduction of trigeminal sensory neurons to relay information to the central nervous system.
Tracing of the superior cervical ganglia in large animal models suggests at least a portion of unmyelinated intradental neurons are of sympathetic origin (16). These sympathetic free nerve endings primarily reside along the pulpal blood vessels with highest densities near the pulp horns below the odontoblast layer (17). However, some unmyelinated axons also originate from the trigeminal ganglion, as inferior alveolar nerve degeneration results in a partial reduction of unmyelinated axons at the apical foramen, in addition to complete loss of myelinated intradental neurons (12, 18–20).
Retrograde tracing experiments have shown that in rats, intradental molar neurons are primarily myelinated and medium to large in diameter, indicative of Aδ/Aβ sensory neurons (21, 22). More recently, retrograde tracing revealed that each mouse molar receives unique innervation by a population of approximately 50 large diameter, myelinated neurons (23). Myelinated Aδ/Aβ sensory neurons lose their myelin sheath within the pulp proper before projecting into overlying dentin (24), which may account for prior overestimates of intradental c-fiber contribution. However, the presence of c-fiber conduction velocities in larger animal models suggests that this class may also contribute to intradental sensation (25–28). Whether the lack of evidence supporting intradental c-fibers in rodent models reveals inherent species variability vs. a conserved minor sensory role of c-fibers in tooth sensation remains to be determined.
2.2 Dentin
Surrounding the pulp is the dentin, which makes up the bulk of the tooth. Dentin is a layered, mineralized collagenous matrix that provides overall support while effectively cushioning external forces to protect the tooth structure (29). Underlying odontoblasts at the pulp-dentin barrier function to maintain and generate dentin throughout the life of the tooth. Generally, dentin can be divided into several layers based on degrees of hardness and histological composition (10). Intertubular dentin is deeper, most abundant, and features a fluid-filled tubule network that radiates outward from the tooth pulp towards the occlusal tooth surface (5, 30). An outer, less mineralized layer of atubular mantle dentin covers the intertubular dentin. This, in turn, extends to an even more compliant, thin enamel-dentin junction layer (∼25 µm–100 µm thick) that dissipates external forces minimizing the risk and spread of tooth fractures (5, 31).
Within the intertubular dentin, both odontoblasts and myelinated sensory neurons (suggesting Aδ/Aβ-type) extend processes outward from the pulp-dentin border to penetrate approximately one-third of the tubule length (12, 32, 33). This has led to speculation that odontoblasts may function as primary sensory cells that communicate with adjacent free nerve endings to trigger sensation and/or pain (See Section 5 for further discussion on proposed mechanisms of tooth sensitivity). However, electron microscopy studies demonstrate that the dentinal odontoblast process is devoid of pre-synaptic fusion machinery, indicating direct communication within the dentin from the odontoblast process to adjacent free nerve endings is unlikely (12). On the contrary, free nerve endings within the dentin tubules exhibit enlarged swellings along their tips (termed “beaded endings”) that house signaling machinery (including clear and dense core vesicles that express synaptophysin, smooth endoplasmic reticulum, and mitochondria) (8, 12, 34). The function of these beaded endings requires further investigation, but hints at the possibility that tubular free nerve endings could locally communicate with adjacent odontoblast processes.
Anterograde tracing of trigeminal sensory neurons reveals that dentin innervation is not homogenous, with maximal innervation density at the coronal pulp horns and sparse presence in root dentin (12, 33). The receptive fields and branching patterns of individual intradental neurons has been proposed, but not shown. Applying sparse labeling techniques to trace individual neuron branching patterns holds promise to elucidate how intradental sensory neurons individually and might collectively function in the dentin to contribute to tooth sensation.
2.3 Enamel
Enamel is the hardest substance in the human body, providing protection to the inner tissues of the tooth organ. Enamel is acellular and composed of highly mineralized hydroxyapatite crystals forming an outermost cap of the tooth crown (30, 35). The enamel layer forms in early tooth development prior to eruption but, unlike dentin, does not regenerate throughout life (36). Despite its hardness, enamel is susceptible to brittle fracture from extreme forces (37, 38) or erosion by bacterial or dietary acids (39, 40). Enamel thickness varies based on species, diet, and tooth type with thicker enamel corresponding to animals who do not regenerate their teeth [e.g., human enamel can be up to 2.5 mm thick at the molar cusp, while mouse enamel is approximately 50–60 μm (41)] (5). Given that enamel is finite and cannot be regenerated, sensory mechanisms safeguarding tooth structural integrity would be presumed to benefit tooth survival and longevity.
2.4 Root
The tooth roots are embedded within the alveolar bone anchored by the periodontal ligament. As the anatomical crown is encapsulated by a hard enamel cap, the root dentin is covered by a thin mineralized cementum layer. Despite a comparable mineralization and hardness to dentin, cementum has a lower elastic modulus (5, 42) as it is less prone to impact via direct forces. Unlike enamel, cementum can regenerate via cementoblasts lining the periodontal ligament, allowing self-repair following minor trauma or infection (5, 43, 44). As previously mentioned, apical foramina at the root tips allow for innervation and vasculature to penetrate the tooth interior. Minimal, if any, innervation is observed extending into root dentin and cementum layers (33).
In summary, tooth structure features conserved anatomical specializations including enamel, dentin, and specialized patterns of sensory innervation. Given the tooth's irreplicable nature and essential role in diet and defense, we propose these specializations enable their use as masticatory and defensive tools and contribute to structural protection to preserve the tooth organ.
3 Molecular and transcriptomic classification of intradental neurons
The traditional classification of neurons (c-fiber, Aδ, Aβ) has been ubiquitously used in somatosensory neuroscience research for decades (Figure 2A) (6, 45). However, an increasing body of evidence underscores that this system fails to capture the rich molecular heterogeneity that exist within these classes. Work in the last decades has sought to molecularly characterize intradental neurons by evaluating candidate gene expression for various sensory/nociceptive markers, neuropeptides, and ion channels (6). However, establishing a consensus on the molecular expression of intradental neurons and their homogeneity remains challenging due to considerable variability across reports. Discrepancies may be ascribed to differences in model systems (e.g., cultured vs. native intradental neurons, or varying animal models), antibodies/probe variability, and potential bias by evaluating only a panel of candidate molecules.
A substantial body of evidence suggests that at least a portion of intradental neurons exhibit markers for nociceptors. Specifically, multiple reports demonstrate that intradental neurons express calcitonin gene-related peptide (CGRP, Calca) (21, 23, 34, 46–49), a neuropeptide that contributes to neurogenic inflammation (50). Furthermore, focus has been placed on evaluating the expression of transient receptor potential (TRP) channels in intradental neurons based on their canonical roles in thermal nociception and/or inflammatory pain (51, 52). Among TRP channels, Trpv1 has been particularly attractive as this thermosensitive channel could represent a potential therapeutic target for pain and inflammation (53, 54). However, while immunohistochemical staining of human coronal dental pulp demonstrates co-expression of TRPV1 in a subset of CGRP+ intradental nerve fibers (55), reported TRPV1 expression in intradental neurons has varied widely (8%–87%) across multiple studies (23, 46, 56–61).
More recent efforts to sequence the transcriptomes of trigeminal sensory neurons have helped refine their classification. Single-cell transcriptomic profiling has identified around a dozen major transcriptomic classes of sensory neurons (62–65) which can be assigned to cells in tissue sections and whole-mount trigeminal ganglia using in-situ hybridization (ISH) staining (Figure 2B) (66). Retrograde labeling of trigeminal sensory neurons innervating mouse molars in conjunction with in situ classification has recently allowed the determination of the transcriptomic diversity of neurons innervating molar teeth, revealing each tooth receives contributions from a population of specialized trigeminal neurons that express nociceptive markers as well as genes associated with fast-conducting neurons (S100b) reflecting either Aδ or Aβ (23). Intradental neuron expression of S100b and Calca (encodes CGRP) or S100b and nociceptive voltage-gated sodium channel Nav1.8 (Scn10a) aligns with previous reports (58, 61), and designates the majority as large diameter, C6 cells (23) which would be traditionally classified as Aδ mechano-nociceptors. Importantly, this study indicated that few intradental neurons likely represent the small-diameter c-type classes (C2, C7-10) that express Trpv1, Trpm8, and Trpa1 (23). While this does not rule out that TRP channels may still be present and play a role in intradental neuron physiology, it does fall in line with those previous reports that indicate lower expression levels of these channels. Of note, intradental neurons demonstrated enriched expression of nociceptive marker 5-hydroxytryptamine (serotonin) receptor 3A (Htr3a) and the touch receptor Piezo2 (23). These channels may contribute to intradental neuron physiology and warrant further investigation with tractable genetic models.
Similarly, a subsequent transcriptomic profiling of acutely cultured mouse molar neurons showed the majority feature enriched expression of S100b, Calca, and Piezo2 (67). Intriguingly, this study identified additional populations of intradental neurons which showed enriched expression of Trpv1 and Trpa1 (67). While these neurons were assigned to C7/C8/C10 classes based on the presence of TRP channels, they also featured high expression of S100b associated with myelinated neurons not c-fibers.
4 The function of intradental sensory neurons
Given that intradental neurons richly innervate the inner dentin and tooth pulp, this begs the question as to how they contribute to sensation. Patients typically describe conscious tooth sensation as painful, and many studies assume that pain is the only output from intradental neurons (68). Certainly, infections that produce inflammation of the pulp are associated with dramatic inflammatory pain (69, 70). However, considering that pain typically serves a protective role to prevent tissue injury and encourage healing, the utility of inflammatory pain from the tooth is ambiguous since it often requires clinical interventions for resolution in human patients. Nociceptive pain from operative dental procedures results from the removal of tooth structure. Here, accompanying pain serves as a protective signal indicating direct tissue damage. Given that dental drilling represents an artificially amplified stimulus, pain may also represent an exaggerated output of intradental neurons. Consequently, natural factors damaging the tooth might produce different, less severe sensation.
Supporting this notion, psychophysical studies using electrical stimulation on healthy human teeth indicate more diverse perceptual outputs from intradental neurons (71). Lower intensity pulses elicited pre-pain tingling sensations that transition into intense pain when delivered at higher frequencies (8, 68, 71, 72). Pulse intensities that would be predicted to activate myelinated fibers produce pre-pain sensation and also trigger a jaw-opening reflex (73). This indirectly suggests that myelinated intradental neurons likely underlie both pre-pain and pain sensations, and may also initiate protective reflex(es) to preserve the tooth organ. The detection of Piezo2 in intradental neurons through recent transcriptomic analyses hints that these neurons could detect lower intensity forces, as this ion channel underlies discriminative touch in the skin (8, 23, 67, 72, 74–76). While human patients are capable of sensing innocuous touch vibration applied to the teeth (77), it has not been demonstrated whether intradental vs. surrounding tissue innervation is responsible for vibration sensation.
Single unit recordings of the inferior alveolar nerve of large animal models (primarily cats and dogs conducted in the 1970s and 1980s) support innervation of the dentin by myelinated intradental neurons (25–28, 78, 79). Activation of intradental neurons in large animal models using an electric pulp tester evoked responses from fibers with conduction velocities in the Aδ-range (25–27, 78, 79). Corroborating these findings, studies performed using mechanical probing, cold application, scraping, and air-blast of exposed dentin similarly triggered neuronal responses with conduction velocities in the Aδ range (27, 80–82). While intradental neurons responded in these extreme manipulations where the dentin was exposed, their response profile within the context of intact enamel, behavioral outputs, as well as associated perceptions remain largely unexplored.
Though less consistently reported, a handful of animal studies have found responses with c-fiber conduction velocities to noxious stimuli (temperature, mechanical) and inflammatory mediators applied to teeth (25–28). For instance, mechanical probing of exposed tooth pulp, but not dentin, activates fibers with slower conduction velocities, suggesting distinct pulpal localization of c-fibers (83). Broadly, c-fiber activation is thought to present as persistent, dull pain—similar to inflammatory pain (84, 85). This opens a possibility that activation of Aδ vs. c-fibers may give rise to differing types of intradental sensation. In this model, dentinal Aδ fibers would transduce superficial stimuli, while pulpal c-fibers would respond when stimuli penetrate deeper into the pulp or in the context of pulpal infection. In support of this model for functional divergence, one study using intact cat canine teeth found intense heating induces a biphasic response marked by acute Aδ-range action potentials followed by a prolonged activation of c-fiber spikes (80). Furthermore, application of the inflammatory mediator bradykinin to exposed pulp elicits action potentials from c-fibers (27) as well as dull pain sensations in humans (86). Taken together, this body of work strongly suggests that an elusive subpopulation of intradental neurons represents c-fibers that trigger pulpal pain but lack an obvious function outside of the context of extreme stimulation or infection.
Outside of their direct sensory roles, intradental neurons may also contribute to local tissue responses. The distribution and patterning of free sensory nerve endings within the pulp-dentin complex of a fully developed tooth is not static, but can dynamically remodel in response to injury or infection. CGRP+ sensory endings exhibit branch sprouting surrounding sites of pulp damage, although prolonged and extensive damage leads to eventual pruning and denervation at the damage site (48, 87). Denervation by resection of the inferior alveolar nerve is shown to accelerate necrosis induced by pulp damage (88), suggesting that sensory fibers may contribute to pulp-dentin regeneration in response to injury. Indeed, inflammation-induced CGRP release in the pulp has been shown to regulate capillary blood flow and induce tissue regeneration (89). Further research is necessary to determine if intradental sensory neurons respond to inflammation by releasing CGRP or other neuronal factors in order to promote dentin repair.
5 Proposed mechanisms of tooth sensitivity
In most peripheral tissues, free sensory nerve endings are directly activated by sensory stimuli such as mechanical forces or temperature (90). However, the tooth organ is structurally distinct given its encapsulated hard surface, multiple layers of mineralization, and fluid-filled dentin tubules within which the specialized sensory endings and odontoblast processes permeate. This specialized anatomy opens a possibility that sensory detection within the teeth may differ compared to other peripheral targets. Several theories have been proposed regarding tooth sensation: (1) hydrodynamic theory—sensory neurons are indirectly activated by external stimuli through induced fluid movement within the tubules, (2) transduction theory—odontoblasts act as primary sensory cells signaling to sensory neurons, and (3) conduction theory—sensory neuron endings within the inner pulp and dentin tubules are directly activated by sensory stimuli (Figure 2C). It is important to note that these theories are not mutually exclusive and elements of each may function together to give rise to intradental sensation. In this section, we will briefly reflect on current evidence regarding these theories of dental sensitivity.
5.1 Hydrodynamic theory
Investigations into the functionality of intradental neurons have largely been guided by the hydrodynamic theory, first proposed by Brannstrom (91). This theory asserts that sensory cells are activated by fluid flow within the dentinal tubules as a consequence of external stimuli. Here, fluid movements are proposed to amplify external stimuli facilitating their detection by inner sensory cells.
Evidence supporting the hydrodynamic theory stems from both clinical observations and in vivo functional studies. Both have shown that sensations elicited from direct dentin stimulation are heightened when the dentinal tubules are exposed and thought to increase tubular fluid flow (8, 28, 80, 92–94). Supporting this idea, re-blocking tubules with composite reduces the sensitivity (95). However, the reduced sensitivity of dentin following composite application is confounded by disruption of the native tooth composition and structure. Additionally, these observations do not rule out sensory stimuli are directly activating sensory endings (see below, conduction theory).
Studies have sought to estimate fluid flow through the tubules (in terms of both flow rate and direction) in response to external stimuli. Interestingly, most external stimuli tested induce measurable fluid flow within dentin and pulp (96–99). Several independent reports estimate similar pulpal flow rates (in the range of 3.5–22.2 × 103 picolitres(pl)/second), showing fluid flow rate is most concentrated at the region of stimulation and that most mechanical stimuli give rise to inward (or “pulpward”) flow (96–99). Thermal stimuli may induce bidirectional fluid movement within enamel and/or dentin tubules due to thermal contraction/expansion (100, 101). Measurements estimating induced intradental fluid flow in response to thermal stimulation corroborate computational modeling results that cold stimuli induce outward flow, while heating induces pulpward fluid flow (96, 102, 103). Intradental neurons demonstrate lower response threshold to outward fluid flow based on single unit recordings. Conversely, inward pulpward flow requiring a much higher flow rate to elicit a response (104–106). In support of this idea, human patients report heightened sensitivity in response to outward pressure applied to exposed dentin (107). The mechanism or the utility of this preferential response is not clear. Notably, the hydrodynamic theory predates the cloning and study of thermosensitive ion channels that respond directly to temperature (108, 109) and most of these have yet to be explored functionally, in vivo within the context of intradental sensation.
Overall, the hydrodynamic theory posits that fluid flow induces the activation of intradental sensory cells, necessitating the activation of a molecular mechanotransducer. Because external stimuli always impact internal fluid flow, it will remain challenging to determine the physical initiator of intradental sensation. Assaying qualitative differences from multiple modalities of tooth stimulation (hot vs. cold vs. touch) could be informative considering the hydrodynamic theory posits that all would converge on fluid flow. Furthermore, the sensory cell responsible for detecting fluid movement within the tubules is debated, with proposed candidates being tubular odontoblast processes or free nerve endings (transduction vs. conduction theory). Piezo2 emerges as a potential molecular candidate as it may be expressed in both odontoblasts and intradental neurons, but further investigation in vivo is required (66, 67, 110).
5.2 Transduction theory
The transduction theory suggests that odontoblasts function as the primary sensory cells that signal to free nerve endings. Odontoblasts could represent excitable cells in vivo based on their expression of ion channel receptors and electrophysiological responses in culture (111–122). However, as with any cells, in vitro culture may significantly alter the expression pattern and excitability of odontoblasts and may not provide an accurate representation of their native state. The reported variability in Trp channel expression by odontoblasts lends further credence to this concern (111). While this has been a more active area of research in recent decades, direct functional evidence is limited due to the technical challenges of assaying native odontoblasts. Recently, TRPC5 in odontoblasts was proposed as the direct cold sensor, contributing to dentinal cold sensitivity (113). Trpc5 expression appears limited to root-adjacent odontoblasts (113), where sensory innervation into dentinal tubules is notably sparse (12, 33). Odontoblast morphology has also been shown to drastically differ in coronal vs. root dentin (11), further suggesting potential functional heterogeneity in odontoblast populations based on anatomical location.
It remains an open question as to how odontoblasts would communicate with sensory neurons. While nerve endings and odontoblast processes are adjacently oriented within a single tubule, electron microscopy studies suggest they do not directly contact or connect via gap junctions (12, 32). Electron microscopy demonstrate tubular odontoblast processes are devoid of axoplasmic organelles or signaling components (12). Thus, if odontoblasts do represent sensory cells, their ability to relay this information to sensory nerves must be non-traditional. One model proposes that, when excited, odontoblasts release an extravesicular signaling molecule to bind receptors on adjacent free nerve endings. Extracellular ATP (eATP) remains a candidate given a body of in vitro evidence using co-cultured odontoblasts and trigeminal sensory neurons. P2X3 immunostaining has been demonstrated in a subset of human dental pulp afferent fibers in addition to odontoblasts (123). A similar relationship between epithelial cells and sensory neurons has been reported in the skin. Epithelial keratinocytes function as nonneuronal sensory cells that detect touch and temperature to release ATP onto its cognate receptor P2X4 expressed on nearby free sensory endings (124, 125). Further in vivo evidence is necessary to demonstrate that intradental sensory neurons are responsive to ATP.
5.3 Conduction theory
The conduction theory proposes that free sensory endings directly detect sensory stimuli applied to the tooth, then transduce electrical signals to the nervous system. Indeed, somatosensory neurons express all relevant molecular machinery to detect external stimuli and their heterogeneity and anatomical localizations reflect this specialization. Supporting a model that the neurons initiate signaling within the tooth, dentinal sensory endings have been shown to contain axoplasmic organelles that are reminiscent of vesicles found in presynaptic neurons. Activated sensory neurons may signal to odontoblasts to initiate protective dentin production. Interestingly, a recent study of the inferior alveolar nerve found that chronic constriction injury (CCI), which has been shown to induce chronic neuronal activation (126), gave rise to significant pulp calcification. Further investigation is required to determine whether intradental neurons indeed function as the primary detectors of sensory stimuli as opposed to odontoblasts. Nevertheless, intradental neurons play an indispensable role in transmitting sensory input from the teeth to the central nervous system.
The encapsulated nature of the tooth structure has made it challenging to parse out the extent these three theories contribute to overall tooth sensitivity. Future studies using targeted genetic approaches such as cell-specific ablation in conjunction with functional assays will shed light on the cellular mechanism of intradental sensitivity.
6 Concluding remarks
This review presents an overview of tooth structure and innervation toward understanding tooth sensation. The distinctive structure of the tooth organ features elements that are conserved among mammals and lead to the protection of these essential organs. Enamel covers the outer tooth and myelinated sensory neurons extend from the pulp and penetrate the dentin tubules. The tooth pulp is densely innervated by sensory neurons which have been further described based on their transcriptomic profiles. Most intradental innervation originates from myelinated trigeminal neurons that terminate in the inner dentin. Functional studies and clinical observations investigating sensory innervation in both animal models and humans have demonstrated pain is a predictable consequence of extreme scenarios of intradental sensory neuron activation (e.g., when tooth structure is damaged and/or dentin is exposed). The contribution of sensory neurons to sensation in an intact tooth has not been defined.
Future research is needed to determine the cellular and molecular origin of tooth sensation as well as how molecularly-defined intradental neurons respond to a range of tooth stimulations and produce nociceptive vs. inflammatory tooth pain. Given the advances of genetic tractability using murine models in recent decades, mouse studies show promise for elucidating the identity of intradental sensory neurons. However, when interpreting rodent data, it is critical to note that studies on molars, which are structurally similar to human teeth, provide the most relevant comparisons considering these do not regenerate throughout life. Ultimately, these insights will contribute to the development of targeted clinical anesthetics and treatments for dental pain
Author contributions
ER: Conceptualization, Investigation, Visualization, Writing – original draft, Writing – review & editing. MN: Visualization, Writing – review & editing. JE: Conceptualization, Funding acquisition, Supervision, Visualization, Writing – original draft, Writing – review & editing.
Funding
The author(s) declare that financial support was received for the research, authorship, and/or publication of this article.
This work is supported by the National Institute of Dental and Craniofacial Research through awards K22 DE029779 (JE) and T32 DE007057 (ER).
Acknowledgments
We thank Charlotte Mistretta and Robert Bradley for critically reading the manuscript and helpful discussions.
Conflict of interest
The authors declare that the research was conducted in the absence of any commercial or financial relationships that could be construed as a potential conflict of interest.
Publisher's note
All claims expressed in this article are solely those of the authors and do not necessarily represent those of their affiliated organizations, or those of the publisher, the editors and the reviewers. Any product that may be evaluated in this article, or claim that may be made by its manufacturer, is not guaranteed or endorsed by the publisher.
References
1. Yamanaka A. Evolution and development of the mammalian multicuspid teeth. J Oral Biosci. (2022) 64:165–75. doi: 10.1016/j.job.2022.03.007
2. Kermack KA, Lees PM, Mussett F, Abercrombie M. Aegialodon dawsoni, a new trituberculosectorial tooth from the lower wealden. Proc R Soc Lond B Biol Sci. (1997) 162:535–54. doi: 10.1098/rspb.1965.0055
3. Sigogneau-Russell D, Hooker JJ, Ensom PC. The oldest tribosphenic mammal from Laurasia (Purbeck Limestone Group, Berriasian, Cretaceous, UK) and its bearing on the ‘dual origin’ of Tribosphenida. C R Acad Sci II A. (2001) 333:141–7. doi: 10.1016/S1251-8050(01)01612-3
4. Jheon AH, Seidel K, Biehs B, Klein OD. From molecules to mastication: the development and evolution of teeth. Wiley Interdiscip Rev Dev Biol. (2013) 2:165–82. doi: 10.1002/wdev.63
5. Berkovitz B, Shellis P. Chapter 2—Mammalian tooth structure and function. In: Berkovitz B, Shellis P, editors. The Teeth of Mammalian Vertebrates. Academic Press (2018). p. 25–46. doi: 10.1016/B978-0-12-802818-6.00002-8
6. Le Pichon CE, Chesler AT. The functional and anatomical dissection of somatosensory subpopulations using mouse genetics. Front Neuroanat. (2014) 8. doi: 10.3389/fnana.2014.00021
7. Chesler AT, Szczot M, Bharucha-Goebel D, Čeko M, Donkervoort S, Laubacher C, et al. The role of PIEZO2 in human mechanosensation. N Engl J Med. (2016) 375:1355–64. doi: 10.1056/NEJMoa1602812
8. Byers MR, Narhi MV, Henry MA. Nerve supply of the pulp-dentine complex and responses to injury. In: Hargreaves KM, Goodis HE, Tay F, editors. Seltzer and Benders; Dental Pulp. Hanover Park, IL: Quintessence Publishing Co, Inc. (2012). p. 133–58.
9. Bleicher F. Odontoblast physiology. Exp Cell Res. (2014) 325:65–71. doi: 10.1016/j.yexcr.2013.12.012
10. Arana-Chavez VE, Massa LF. Odontoblasts: the cells forming and maintaining dentine. Int J Biochem Cell Biol. (2004) 36:1367–73. doi: 10.1016/j.biocel.2004.01.006
11. Byers MR, Sugaya A. Odontoblast processes in dentin revealed by fluorescent di-I. J Histochem Cytochem. (1995) 43:159–68. doi: 10.1177/43.2.7529786
12. Byers MR. Dental sensory receptors. In: Smythies JR, Bradley RJ, editors. International Review of Neurobiology, vol. 25. Academic Press (1984) p. 39–94.
13. Ghannam MG, Alameddine H, Bordoni B. Anatomy, head and neck, pulp (tooth). In: StatPearls. Treasure Island, FL, StatPearls Publishing (2022).
14. Shi S, Gronthos S. Perivascular niche of postnatal mesenchymal stem cells in human bone marrow and dental pulp. J Bone Miner Res. (2003) 18:696–704. doi: 10.1359/jbmr.2003.18.4.696
15. Graf W, Bjorlin G. Diameters of nerve fibers in human tooth pulps. J Am Dent Assoc. (1951) 43:186–93. doi: 10.14219/jada.archive.1951.0180
16. Christensen K. Sympathetic nerve fibers in the alveolar nerves and nerves of the dental pulp. J Dent Res. (1940) 19:227–42. doi: 10.1177/00220345400190030301
17. Avery JK, Cox CF, Chiego DJ. Presence and location of adrenergic nerve endings in the dental pulps of mouse molars. Anat Rec. (1980) 198:59–71. doi: 10.1002/ar.1091980105
18. Fehér E, Csányi K, Vajda J. Ultrastructure and degeneration analysis of the nerve fibres of the tooth pulp in the cat. Arch Oral Biol. (1977) 22:699–704. doi: 10.1016/0003-9969(77)90100-5
19. Fried K, Hildebrand C. Degeneration of unmyelinated axons in the dental root canal induced by 6-OH-dopamine. Experientia. (1978) 34:1354–5. doi: 10.1007/BF01981467
20. Arwill T, Edwall L, Lilja J, Olgart L, Svensson SE. Ultrastructure of nerves in the dentinal-pulp border zone after sensory and autonomic nerve transection in the cat. Acta Odontol Scand. (1973) 31:273–81. doi: 10.3109/00016357309002514
21. Fried K, Arvidsson J, Robertson B, Brodin E, Theodorsson E. Combined retrograde tracing and enzyme/immunohistochemistry of trigeminal ganglion cell bodies innervating tooth pulps in the rat. Neuroscience. (1989) 33:101–9. doi: 10.1016/0306-4522(89)90314-X
22. Paik SK, Park KP, Lee SK, Ma SK, Cho YS, Kim YK, et al. Light and electron microscopic analysis of the somata and parent axons innervating the rat upper molar and lower incisor pulp. Neuroscience. (2009) 162:1279–86. doi: 10.1016/j.neuroscience.2009.05.046
23. Emrick JJ, von Buchholtz LJ, Ryba NJP. Transcriptomic classification of neurons innervating teeth. J Dent Res. (2020) 99:1478–85. doi: 10.1177/0022034520941837
24. Fried K, Aldskogius H, Hildebrand C. Proportion of unmyelinated axons in rat molar and incisor tooth pulps following neonatal capsaicin treatment and/or sympathectomy. Brain Res. (1988) 463:118–23. doi: 10.1016/0006-8993(88)90533-1
25. Cadden SW, Lisney SJW, Matthews B. Thresholds to electrical stimulation of nerves in cat canine tooth-pulp with aβ-, aδ- and C-fibre conduction velocities. Brain Res. (1983) 261:31–41. doi: 10.1016/0006-8993(83)91280-5
26. Virtanen A, Närhi M, Huopaniemi T, Hirvonen T. Thresholds of intradental A- and C-nerve fibres in the cat to electrical current pulses of different duration. Acta Physiol Scand. (1983) 119:393–8. doi: 10.1111/j.1748-1716.1983.tb07355.x
27. Närhi M, Jyväsjärvi E, Virtanen A, Huopaniemi T, Ngassapa D, Hirvonen T. Role of intradental A- and C-type nerve fibres in dental pain mechanisms. Proc Finn Dent Soc. (1992) 88(Suppl 1):507–16.
28. Närhi M, Jyväsjärvi E, Hirvonen T, Huopaniemi T. Activation of heat-sensitive nerve fibres in the dental pulp of the cat. Pain. (1982) 14:317–26. doi: 10.1016/0304-3959(82)90140-3
29. Wang RZ, Weiner S. Strain-structure relations in human teeth using moiré fringes. J Biomech. (1998) 31:135–41. doi: 10.1016/S0021-9290(97)00131-0
30. Goldberg M, Kulkarni AB, Young M, Boskey A. Dentin: structure, composition and mineralization. Front Biosci (Elite Ed). (2011) 3:711–35. doi: 10.2741/e281
31. Imbeni V, Kruzic JJ, Marshall GW, Marshall SJ, Ritchie RO. The dentin–enamel junction and the fracture of human teeth. Nat Mater. (2005) 4:229–32. doi: 10.1038/nmat1323
32. Byers MR, Neuhaus SJ, Gehrig JD. Dental sensory receptor structure in human teeth. PAIN. (1982) 13:221–35. doi: 10.1016/0304-3959(82)90012-4
33. Byers MR, Calkins DF. Trigeminal sensory nerve patterns in dentine and their responses to attrition in rat molars. Arch Oral Biol. (2021) 129:105197. doi: 10.1016/j.archoralbio.2021.105197
34. Byers MR, Cornel LM. Multiple complex somatosensory systems in mature rat molars defined by immunohistochemistry. Arch Oral Biol. (2018) 85:84–97. doi: 10.1016/j.archoralbio.2017.09.007
35. Lacruz RS, Habelitz S, Wright JT, Paine ML. Dental enamel formation and implications for oral health and disease. Physiol Rev. (2017) 97:939–93. doi: 10.1152/physrev.00030.2016
36. Abou Neel EA, Aljabo A, Strange A, Ibrahim S, Coathup M, Young AM, et al. Demineralization–remineralization dynamics in teeth and bone. Int J Nanomed. (2016) 11:4743–63. doi: 10.2147/IJN.S107624
37. Rensberger JM. Mechanical adaptation in enamel. In: Tooth Enamel Microstructure. CRC Press (1997).
38. Maas MC, Dumont ER. Built to last: the structure, function, and evolution of primate dental enamel. Evol Anthropol. (1999) 8:133–52. doi: 10.1002/(SICI)1520-6505(1999)8:4%3C133::AID-EVAN4%3E3.0.CO;2-F
39. Järvinen VK, Rytömaa II, Heinonen OP. Risk factors in dental erosion. J Dent Res. (1991) 70:942–7. doi: 10.1177/00220345910700060601
40. Schlueter N, Amaechi BT, Bartlett D, Buzalaf MAR, Carvalho TS, Ganss C, et al. Terminology of erosive tooth wear: consensus report of a workshop organized by the ORCA and the cariology research group of the IADR. Caries Res. (2019) 54:2–6. doi: 10.1159/000503308
41. Gibson CW, Li Y, Suggs C, Kuehl MA, Pugach MK, Kulkarni AB, et al. Rescue of the murine amelogenin null phenotype with two amelogenin transgenes. Eur J Oral Sci. (2011) 119(Suppl 1):70–4. doi: 10.1111/j.1600-0722.2011.00882.x
42. Angker L, Swain MV. Nanoindentation: application to dental hard tissue investigations. J Mater Res. (2006) 21:1893–905. doi: 10.1557/jmr.2006.0257
43. Nuñez J, Vignoletti F, Caffesse RG, Sanz M. Cellular therapy in periodontal regeneration. Periodontol 2000. (2019) 79:107–16. doi: 10.1111/prd.12250
44. Bosshardt DD, Selvig KA. Dental cementum: the dynamic tissue covering of the root. Periodontol 2000. (1997) 13:41–75. doi: 10.1111/j.1600-0757.1997.tb00095.x
45. Julius D, Basbaum AI. Molecular mechanisms of nociception. Nature. (2001) 413:203–10. doi: 10.1038/35093019
46. Gibbs JL, Melnyk JL, Basbaum AI. Differential TRPV1 and TRPV2 channel expression in dental pulp. J Dent Res. (2011) 90:765–70. doi: 10.1177/0022034511402206
47. Michot B, Lee CS, Gibbs JL. TRPM8 and TRPA1 do not contribute to dental pulp sensitivity to cold. Sci Rep. (2018) 8:13198. doi: 10.1038/s41598-018-31487-2
48. Erdogan O, Michot B, Xia J, Alabdulaaly L, Yesares Rubi P, Ha V, et al. Neuronal–immune axis alters pain and sensory afferent damage during dental pulp injury. PAIN. (2024) 165(2):392–403. doi: 10.1097/j.pain.0000000000003029
49. Moore ER, Michot B, Erdogan O, Ba A, Gibbs JL, Yang Y. CGRP and SHH mediate the dental pulp cell response to neuron stimulation. J Dent Res. (2022) 101:1119–26. doi: 10.1177/00220345221086858
50. Roosterman D, Goerge T, Schneider SW, Bunnett NW, Steinhoff M. Neuronal control of skin function: the skin as a neuroimmunoendocrine organ. Physiol Rev. (2006) 86:1309–79. doi: 10.1152/physrev.00026.2005
51. Julius D. TRP channels and pain. Annu Rev Cell Dev Biol. (2013) 29:355–84. doi: 10.1146/annurev-cellbio-101011-155833
52. Parenti A, De Logu F, Geppetti P, Benemei S. What is the evidence for the role of TRP channels in inflammatory and immune cells? Br J Pharmacol. (2016) 173:953–69. doi: 10.1111/bph.13392
53. Hargreaves KM, Ruparel S. Role of oxidized lipids and TRP channels in orofacial pain and inflammation. J Dent Res. (2016) 95:1117–23. doi: 10.1177/0022034516653751
54. Green DP, Ruparel S, Roman L, Henry MA, Hargreaves KM. Role of endogenous TRPV1 agonists in a postburn pain model of partial-thickness injury. Pain. (2013) 154:2512–20. doi: 10.1016/j.pain.2013.07.040
55. Fehrenbacher JC, Sun XX, Locke EE, Henry MA, Hargreaves KM. Capsaicin-evoked iCGRP release from human dental pulp: a model system for the study of peripheral neuropeptide secretion in normal healthy tissue. Pain. (2009) 144:253–61. doi: 10.1016/j.pain.2009.03.027
56. Chaudhary P, Martenson ME, Baumann TK. Vanilloid receptor expression and capsaicin excitation of rat dental primary afferent neurons. J Dent Res. (2001) 80:1518–23. doi: 10.1177/00220345010800060801
57. Stenholm E, Bongenhielm U, Ahlquist M, Fried K. VRl- and VRL-l-like immunoreactivity in normal and injured trigeminal dental primary sensory neurons of the rat. Acta Odontol Scand. (2002) 60:72–9. doi: 10.1080/000163502753509455
58. Park C-K, Kim MS, Fang Z, Li HY, Jung SJ, Choi S-Y, et al. Functional expression of thermo-transient receptor potential channels in dental primary afferent neurons: implication for tooth pain. J Biol Chem. (2006) 281:17304–11. doi: 10.1074/jbc.M511072200
59. Chung M-K, Lee J, Duraes G, Ro JY. Lipopolysaccharide-induced pulpitis up-regulates TRPV1 in trigeminal ganglia. J Dent Res. (2011) 90:1103–7. doi: 10.1177/0022034511413284
60. Kim HY, Chung G, Jo HJ, Kim YS, Bae YC, Jung SJ, et al. Characterization of dental nociceptive neurons. J Dent Res. (2011) 90:771–6. doi: 10.1177/0022034511399906
61. Won J, Vang H, Lee PR, Kim YH, Kim HW, Kang Y, et al. Piezo2 expression in mechanosensitive dental primary afferent neurons. J Dent Res. (2017) 96:931–7. doi: 10.1177/0022034517702342
62. Usoskin D, Furlan A, Islam S, Abdo H, Lönnerberg P, Lou D, et al. Unbiased classification of sensory neuron types by large-scale single-cell RNA sequencing. Nat Neurosci. (2015) 18:145–53. doi: 10.1038/nn.3881
63. Nguyen MQ, Wu Y, Bonilla LS, von Buchholtz LJ, Ryba NJP. Diversity amongst trigeminal neurons revealed by high throughput single cell sequencing. PLoS One. (2017) 12:e0185543. doi: 10.1371/journal.pone.0185543
64. Nguyen MQ, Le Pichon CE, Ryba N. Stereotyped transcriptomic transformation of somatosensory neurons in response to injury. Elife. (2019) 8:e49679. doi: 10.7554/eLife.49679
65. Sharma N, Flaherty K, Lezgiyeva K, Wagner DE, Klein AM, Ginty DD. The emergence of transcriptional identity in somatosensory neurons. Nature. (2020) 577:392–8. doi: 10.1038/s41586-019-1900-1
66. von Buchholtz LJ, Lam RM, Emrick JJ, Chesler AT, Ryba NJP. Assigning transcriptomic class in the trigeminal ganglion using multiplex in situ hybridization and machine learning. Pain. (2020) 161:2212–24. doi: 10.1097/j.pain.0000000000001911
67. Lee PR, Kim J, Rossi HL, Chung S, Han SY, Kim J, et al. Transcriptional profiling of dental sensory and proprioceptive trigeminal neurons using single-cell RNA sequencing. Int J Oral Sci. (2023) 15:1–14. doi: 10.1038/s41368-022-00210-3
68. Fried K, Sessle BJ, Devor M. The paradox of pain from the tooth-pulp: low-threshold “algoneurons”? Pain. (2011) 152:2685–9. doi: 10.1016/j.pain.2011.08.004
69. Bender IB. Pulpal pain diagnosis–a review. J Endod. (2000) 26:175–9. doi: 10.1097/00004770-200003000-00012
70. Levin LG, Law AS, Holland GR, Abbott PV, Roda RS. Identify and define all diagnostic terms for pulpal health and disease states. J Endod. (2009) 35:1645–57. doi: 10.1016/j.joen.2009.09.032
71. Virtanen ASJ, Huopaniemi T, Närhi MVO, Pertovaara A, Wallgren K. The effect of temporal parameters on subjective sensations evoked by electrical tooth stimulation. Pain. (1987) 30:361–71. doi: 10.1016/0304-3959(87)90024-8
72. Dong WK, Shiwaku T, Kawakami Y, Chudler EH. Static and dynamic responses of periodontal ligament mechanoreceptors and intradental mechanoreceptors. J Neurophysiol. (1993) 69:1567–82. doi: 10.1152/jn.1993.69.5.1567
73. Matthews B, Baxter J, Watts S. Sensory and reflex responses to tooth pulp stimulation in man. Brain Res. (1976) 113:83–94. doi: 10.1016/0006-8993(76)90008-1
74. Ranade SS, Woo S-H, Dubin AE, Moshourab RA, Wetzel C, Petrus M, et al. Piezo2 is the major transducer of mechanical forces for touch sensation in mice. Nature. (2014) 516:121–5. doi: 10.1038/nature13980
75. Kubo K, Shibukawa Y, Shintani M, Suzuki T, Ichinohe T, Kaneko Y. Cortical representation area of human dental pulp. J Dent Res. (2008) 87:358–62. doi: 10.1177/154405910808700409
76. Paphangkorakit J, Osborn JW. The effect of pressure on a maximum incisal bite force in man. Arch Oral Biol. (1997) 42:11–7. doi: 10.1016/S0003-9969(96)00106-9
77. Levy JH, Dong WK. Vibration perception thresholds of human vital and nonvital maxillary incisors. Arch Oral Biol. (2022) 139:105426. doi: 10.1016/j.archoralbio.2022.105426
78. Greenwood F, Horiuchi H, Matthews B. Electrophysiological evidence on the types of nerve fibres excited by electrical stimulation of teeth with a pulp tester. Arch Oral Biol. (1972) 17:701–9. doi: 10.1016/0003-9969(72)90196-3
79. Närhi M, Virtanen A, Kuhta J, Huopaniemi T. Electrical stimulation of teeth with a pulp tester in the cat. Scand J Dent Res. (1979) 87:32–8. doi: 10.1111/j.1600-0722.1979.tb01937.x
80. Närhi MV. The characteristics of intradental sensory units and their responses to stimulation. J Dent Res. (1985) 64(Spec No):564–71. doi: 10.1177/002203458506400411
81. Ahn DK, Doutova EA, McNaughton K, Light AR, Närhi M, Maixner W. Functional properties of tooth pulp neurons responding to thermal stimulation. J Dent Res. (2012) 91:401–6. doi: 10.1177/0022034511435703
82. Byers MR, Närhi MVO, Mecifi KB. Acute and chronic reactions of dental sensory nerve fibers to cavities and desiccation in rat molars. Anat Rec. (1988) 221:872–83. doi: 10.1002/ar.1092210412
83. Jyväsjärvi E, Kniffki KD. Cold stimulation of teeth: a comparison between the responses of cat intradental A delta and C fibres and human sensation. J Physiol (Lond). (1987) 391:193–207. doi: 10.1113/jphysiol.1987.sp016733
84. Erdogan O, Malek M, Janal MN, Gibbs JL. Sensory testing associates with pain quality descriptors during acute dental pain. Eur J Pain. (2019) 23:1701–11. doi: 10.1002/ejp.1447
85. Bouhassira D, Attal N, Alchaar H, Boureau F, Brochet B, Bruxelle J, et al. Comparison of pain syndromes associated with nervous or somatic lesions and development of a new neuropathic pain diagnostic questionnaire (DN4). Pain. (2005) 114:29–36. doi: 10.1016/j.pain.2004.12.010
86. Ahlquist ML, Edwall LGA, Franzén OG, Haegerstam GAT. Perception of pulpal pain as a function of intradental nerve activity. Pain. (1984) 19:353–66. doi: 10.1016/0304-3959(84)90081-2
87. Zhan C, Huang M, Chen J, Lu Y, Yang X, Hou J. Sensory nerves, but not sympathetic nerves, promote reparative dentine formation after dentine injury via CGRP-mediated angiogenesis: an in vivo study. Int Endod J. (2024) 57(1):37–49. doi: 10.1111/iej.13989
88. Byers MR, Taylor PE. Effect of sensory denervation on the response of rat molar pulp to exposure injury. J Dent Res. (1993) 72:613–8. doi: 10.1177/00220345930720031001
89. Lundy FT, Linden GJ. Neuropeptides and neurogenic mechanisms in oral and periodontal inflammation. Crit Rev Oral Biol Med. (2004) 15:82–98. doi: 10.1177/154411130401500203
90. Basbaum AI, Bautista DM, Scherrer G, Julius D. Cellular and molecular mechanisms of pain. Cell. (2009) 139:267–84. doi: 10.1016/j.cell.2009.09.028
91. Brännström M, Aström A. The hydrodynamics of the dentine; its possible relationship to dentinal pain. Int Dent J. (1972) 22:219–27.
92. Absi EG, Addy M, Adams D. Dentine hypersensitivity. A study of the patency of dentinal tubules in sensitive and non-sensitive cervical dentine. J Clin Periodontol. (1987) 14:280–4. doi: 10.1111/j.1600-051X.1987.tb01533.x
93. Närhi MV, Hirvonen TJ, Hakumäki MO. Activation of intradental nerves in the dog to some stimuli applied to the dentine. Arch Oral Biol. (1982) 27:1053–8. doi: 10.1016/0003-9969(82)90011-5
94. Ahlquist M, Franzén O, Coffey J, Pashley D. Dental pain evoked by hydrostatic pressures applied to exposed dentin in man: a test of the hydrodynamic theory of dentin sensitivity. J Endod. (1994) 20:130–4. doi: 10.1016/S0099-2399(06)80058-0
95. Hirvonen TJ, Narhi MV, Hakumaki MO. The excitability of dog pulp nerves in relation to the condition of dentine surface. J Endod. (1984) 10:294–8. doi: 10.1016/S0099-2399(84)80182-X
96. Brännström M, Johnson G. Movements of the dentine and pulp liquids on application of thermal stimuli. An in vitro study. Acta Odontol Scand. (1970) 28:59–70. doi: 10.3109/00016357009033132
97. Paphangkorakit J, Osborn JW. The effect of normal occlusal forces on fluid movement through human dentine in vitro. Arch Oral Biol. (2000) 45:1033–41. doi: 10.1016/S0003-9969(00)00090-X
98. Anderson DJ, Matthews B, Gorretta C. Fluid flow through human dentine. Arch Oral Biol. (1967) 12:209-IN13. doi: 10.1016/0003-9969(67)90040-4
99. Brannstrom M, Linden L-A, Johnson G. Movement of dentinal and pulpal fluid caused by clinical procedures. J Dent Res. (1968) 47:679–82. doi: 10.1177/00220345680470050201
100. Linsuwanont P, Palamara JEA, Messer HH. An investigation of thermal stimulation in intact teeth. Arch Oral Biol. (2007) 52:218–27. doi: 10.1016/j.archoralbio.2006.10.009
101. Lin M, Liu S, Niu L, Xu F, Lu TJ. Analysis of thermal-induced dentinal fluid flow and its implications in dental thermal pain. Arch Oral Biol. (2011) 56:846–54. doi: 10.1016/j.archoralbio.2011.02.011
102. Pashley DH, Matthews WG, Zhang Y, Johnson M. Fluid shifts across human dentine in vitro in response to hydrodynamic stimuli. Arch Oral Biol. (1996) 41:1065–72. doi: 10.1016/S0003-9969(96)00059-3
103. Lin M, Luo ZY, Bai BF, Xu F, Lu TJ. Fluid mechanics in dentinal microtubules provides mechanistic insights into the difference between hot and cold dental pain. PLoS One. (2011) 6:e18068. doi: 10.1371/journal.pone.0018068
104. Matthews B, Vongsavan N. Interactions between neural and hydrodynamic mechanisms in dentine and pulp. Arch Oral Biol. (1994) 39(Suppl):87S–95S. doi: 10.1016/0003-9969(94)90193-7
105. Andrew D, Matthews B. Displacement of the contents of dentinal tubules and sensory transduction in intradental nerves of the cat. J Physiol. (2000) 529(Pt 3):791–802. doi: 10.1111/j.1469-7793.2000.00791.x
106. Vongsavan N, Matthews B. The relationship between the discharge of intradental nerves and the rate of fluid flow through dentine in the cat. Arch Oral Biol. (2007) 52:640–7. doi: 10.1016/j.archoralbio.2006.12.019
107. Charoenlarp P, Wanachantararak S, Vongsavan N, Matthews B. Pain and the rate of dentinal fluid flow produced by hydrostatic pressure stimulation of exposed dentine in man. Arch Oral Biol. (2007) 52:625–31. doi: 10.1016/j.archoralbio.2006.12.014
108. Bautista DM, Siemens J, Glazer JM, Tsuruda PR, Basbaum AI, Stucky CL, et al. The menthol receptor TRPM8 is the principal detector of environmental cold. Nature. (2007) 448:204–8. doi: 10.1038/nature05910
109. Caterina MJ, Rosen TA, Tominaga M, Brake AJ, Julius D. A capsaicin-receptor homologue with a high threshold for noxious heat. Nature. (1999) 398:436–41. doi: 10.1038/18906
110. Ren H, Wen Q, Zhao Q, Wang N, Zhao Y. Atlas of human dental pulp cells at multiple spatial and temporal levels based on single-cell sequencing analysis. Front Physiol. (2022) 13. doi: 10.3389/fphys.2022.993478
111. Egbuniwe O, Grover S, Duggal AK, Mavroudis A, Yazdi M, Renton T, et al. TRPA1 and TRPV4 activation in human odontoblasts stimulates ATP release. J Dent Res. (2014) 93:911–7. doi: 10.1177/0022034514544507
112. Solé-Magdalena A, Martínez-Alonso M, Coronado CA, Junquera LM, Cobo J, Vega JA. Molecular basis of dental sensitivity: the odontoblasts are multisensory cells and express multifunctional ion channels. Ann Anat. (2018) 215:20–9. doi: 10.1016/j.aanat.2017.09.006
113. Bernal L, Sotelo-Hitschfeld P, König C, Sinica V, Wyatt A, Winter Z, et al. Odontoblast TRPC5 channels signal cold pain in teeth. Sci Adv. (2021) 7:eabf5567. doi: 10.1126/sciadv.abf5567
114. Allard B, Couble ML, Magloire H, Bleicher F. Characterization and gene expression of high conductance calcium-activated potassium channels displaying mechanosensitivity in human odontoblasts. J Biol Chem. (2000) 275:25556–61. doi: 10.1074/jbc.M002327200
115. Allard B, Magloire H, Couble ML, Maurin JC, Bleicher F. Voltage-gated sodium channels confer excitability to human odontoblasts: possible role in tooth pain transmission. J Biol Chem. (2006) 281:29002–10. doi: 10.1074/jbc.M601020200
116. Son AR, Yang YM, Hong JH, Lee SI, Shibukawa Y, Shin DM. Odontoblast TRP channels and thermo/mechanical transmission. J Dent Res. (2009) 88:1014–9. doi: 10.1177/0022034509343413
117. Magloire H, Lesage F, Couble ML, Lazdunski M, Bleicher F. Expression and localization of TREK-1 K+ channels in human odontoblasts. J Dent Res. (2003) 82:542–5. doi: 10.1177/154405910308200711
118. Solé-Magdalena A, Revuelta EG, Menénez-Díaz I, Calavia MG, Cobo T, García-Suárez O, et al. Human odontoblasts express transient receptor protein and acid-sensing ion channel mechanosensor proteins. Microsc Res Tech. (2011) 74:457–63. doi: 10.1002/jemt.20931
119. Shibukawa Y, Sato M, Kimura M, Sobhan U, Shimada M, Nishiyama A, et al. Odontoblasts as sensory receptors: transient receptor potential channels, pannexin-1, and ionotropic ATP receptors mediate intercellular odontoblast-neuron signal transduction. Pflugers Arch. (2015) 467:843–63. doi: 10.1007/s00424-014-1551-x
120. Sato M, Ogura K, Kimura M, Nishi K, Ando M, Tazaki M, et al. Activation of mechanosensitive transient receptor potential/piezo channels in odontoblasts generates action potentials in cocultured isolectin B4–negative medium-sized trigeminal ganglion neurons. J Endod. (2018) 44:984–991.2. doi: 10.1016/j.joen.2018.02.020
121. Sato M, Sobhan U, Tsumura M, Kuroda H, Soya M, Masamura A, et al. Hypotonic-induced stretching of plasma membrane activates transient receptor potential vanilloid channels and sodium-calcium exchangers in mouse odontoblasts. J Endod. (2013) 39:779–87. doi: 10.1016/j.joen.2013.01.012
122. Matsunaga M, Kimura M, Ouchi T, Nakamura T, Ohyama S, Ando M, et al. Mechanical stimulation-induced calcium signaling by Piezo1 channel activation in human odontoblast reduces dentin mineralization. Front Physiol. (2021) 12:704518. doi: 10.3389/fphys.2021.704518
123. Alavi AM, Dubyak GR, Burnstock G. Immunohistochemical evidence for ATP receptors in human dental pulp. J Dent Res. (2001) 80:476–83. doi: 10.1177/00220345010800021501
124. Moehring F, Cowie AM, Menzel AD, Weyer AD, Grzybowski M, Arzua T, et al. Keratinocytes mediate innocuous and noxious touch via ATP-P2X4 signaling. eLife. (2018) 7:e31684. doi: 10.7554/eLife.31684
125. Sadler KE, Moehring F, Stucky CL. Keratinocytes contribute to normal cold and heat sensation. eLife. (2020) 9:e58625. doi: 10.7554/eLife.58625
Keywords: dental innervation, sensory nerve endings, trigeminal somatosensation, tooth pain, teeth, dental pulp
Citation: Ronan EA, Nagel M and Emrick JJ (2024) The anatomy, neurophysiology, and cellular mechanisms of intradental sensation. Front. Pain Res. 5:1376564. doi: 10.3389/fpain.2024.1376564
Received: 25 January 2024; Accepted: 11 March 2024;
Published: 25 March 2024.
Edited by:
Armen N. Akopian, The University of Texas Health Science Center at San Antonio, United StatesReviewed by:
Anibal Diogenes, The University of Texas Health Science Center at San Antonio, United States© 2024 Ronan, Nagel and Emrick. This is an open-access article distributed under the terms of the Creative Commons Attribution License (CC BY). The use, distribution or reproduction in other forums is permitted, provided the original author(s) and the copyright owner(s) are credited and that the original publication in this journal is cited, in accordance with accepted academic practice. No use, distribution or reproduction is permitted which does not comply with these terms.
*Correspondence: Joshua J. Emrick amplbXJpY2tAdW1pY2guZWR1