- 1Amsterdam University Medical Centers (UMC), Tytgat Institute for Liver and Intestinal Research, University of Amsterdam, Research Institute Amsterdam Gastroenterology, Endocrinology and Metabolism (AG&M), Amsterdam, Netherlands
- 2Department of Pharmacology, Biological Sciences Sector, Federal University of Parana, Curitiba, Brazil
- 3Departments of Chemical Biology and Structural Biology, Leibniz-Forschungsinstitut für Molekulare Pharmakologie, Berlin, Germany
Introduction: The G-protein coupled receptor LPAR5 plays a prominent role in LPA-mediated pain and itch signaling. In this study we focus on the LPAR5-antagonist compound 3 (cpd3) and its ability to affect pain and itch signaling, both in vitro and in vivo.
Methods: Nociceptive behavior in wild type mice was induced by formalin, carrageenan or prostaglandin E2 (PGE2) injection in the hind paw, and the effect of oral cpd3 administration was measured. Scratch activity was measured after oral administration of cpd3, in mice overexpressing phospholipase A2 (), in wild type mice (WT) and in TRPA1-deficient mice (Trpa1 KO). In vitro effects of cpd3 were assessed by measuring intracellular calcium release in HMC-1 and HEK-TRPA1 cells.
Results: As expected, nociceptive behavior (induced by formalin, carrageenan or PGE2) was reduced after treatment with cpd3. Unexpectedly, cpd3 induced scratch activity in mice. In vitro addition of cpd3 to HEK-TRPA1 cells induced an intracellular calcium wave that could be inhibited by the TRPA1-antagonist A-967079. In Trpa1 KO mice, however, the increase in scratch activity after cpd3 administration was not reduced.
Conclusions: Cpd3 has in vivo antinociceptive effects but induces scratch activity in mice, probably by activation of multiple pruriceptors, including TRPA1. These results urge screening of antinociceptive candidate drugs for activity with pruriceptors.
Introduction
Lysophosphatidic acid (LPA) is a bioactive lipid involved in multiple functions like cell shape and migration, wound healing, platelet aggregation, lung fibrosis and hair growth, but it is also involved in pain and itch signaling (1–6). Intraplantar injection of LPA into the hind limb of mice showed dose-dependent nociceptive flexor responses (2). Intracellularly, LPA is the natural ligand of peroxisome proliferator-activated receptor gamma (PPARγ), whereas circulating LPA activates at least six different LPA receptors (LPAR1−6) (7–13). Neuropathic pain was shown to be mediated by LPAR1, as mice lacking Lpar1 did not develop signs of neuropathic pain after peripheral nerve injury (4). However, recent studies have shown a prominent role of LPAR5 in neuropathic and inflammatory pain as well as in itch signaling (14–17).
Multiple LPAR5 specific antagonists have been developed to modulate the LPA/LPAR5 signaling pathway (17–21). In this study we focus on the LPAR5-antagonist compound 3 (cpd3), both in vitro and in vivo, in relation to sensory activity. Cpd3 (C30H26Cl2F3N3O4) is a non-lipid diphenylpyrazole derivative, which can prevent LPA-mediated activation in human mast cells (HMC-1) (IC50 0.141 μM) and mouse microglia cells (BV-2) (IC50 730 nM) (20). The compound is metabolically stable in vivo, with good absorption properties and is bioavailable after oral administration in mice.
Our research question was whether inhibition of LPAR5 by cpd3 would affect pain and itch perception in mice. Upon oral administration, cpd3 did have a reducing effect on inflammatory nociceptive behavior, induced by hindpaw formalin injection. In addition, mechanical hyperalgesia induced by carrageenan or PGE2 could be reduced with cpd3 administration. Strikingly however, we found that both in and WT mice, cpd3 induced a strong increase in scratch activity, which was dose-dependent. A possible explanation for this phenomenon was the activation of the itch-channel TRPA1 by cpd3, although administration of cpd3 to TRPA1-deficient mice still induced an increase in scratch activity upon cpd3 administration. These results suggest that an LPAR5-antagonist like cpd3 can have opposite effects on pain and itch perception, most likely by having opposite effects on noci- and pruriceptors.
Materials and methods
In vitro experiments
Chemicals
LPA 18:1 was purchased from Avanti; Cpd3 was a kind gift from the group of Marc Nazaré; DMSO from Merck, A-967079 from Sanbio; Glycofurol/tetraglycol and Solutol/Kolliphor from Sigma; PEG400 from Affymetrix; formalin from Alphatec; morphine from Merck S.A; carrageenan and PGE2 from Sigma.
Cell culture
HEK cells were cultured in Dulbecco's Modified Eagle Medium (DMEM; Lonza) containing phenol red (pH: 4,5g/L glucose), which was supplemented with L-glutamine (1%; Lonza). HMC-1 cells were cultured in suspension in DMEM/F12 Glutamax (Thermofisher). Both media were supplemented with penicillin/streptomycin (1%; Lonza), and fetal bovine serum (10% vol/vol; Gibco). Cells were cultured at 37°C, 10% CO2 in T75 and T160 culture flasks.
Calcium assay
HMC-1 and HEK-TRPA1 cells were used for calcium measurements. Cells transduced with a doxycycline-inducible TRPA1 construct (22) were incubated with doxycycline (200ng/mL) 24 hours before start of the experiment. Attached cells were washed in HBSS without calcium, containing phenol red (Lonza) and trypsinized. They were resuspended in DMEM without serum and washed twice with HBSS containing calcium, without phenol red, buffered with 10mm HEPES. Cells were counted and diluted to a concentration of ± 1·106 cells/mL. After incubation with 10μM INDO-1 AM (Invitrogen) for 30 minutes at 37°C with repeated shaking, the cells were washed and resuspended in HEPES-buffered HBSS with calcium, and kept on ice until use. 100μL of cell suspension was prewarmed in a UV-STAR microtiter black plate (Greiner) at 37°C in the Clariostar Analyser (BMG Labtech, Ortenberg, Germany; excitation 350 nm, emission 395 nm, and 460 nm). In Figure 1, baseline fluorescence (±100–200 s) was measured, cpd3 was added (measured for 150–400 s) and then LPA was added. In Figure 6, when necessary, A-96 was added before measurement, baseline fluorescence (±250 sec) was measured, and cpd3 or DMSO was added. Data was calculated by dividing fluorescence emitted at 395 nm (calcium-sensitive) by the fluorescence emitted at 460 nm (calcium-insensitive) for each time point (further described as ratio). The mean baseline ratio was subtracted from the peak effect ratio for each condition (delta ratio).
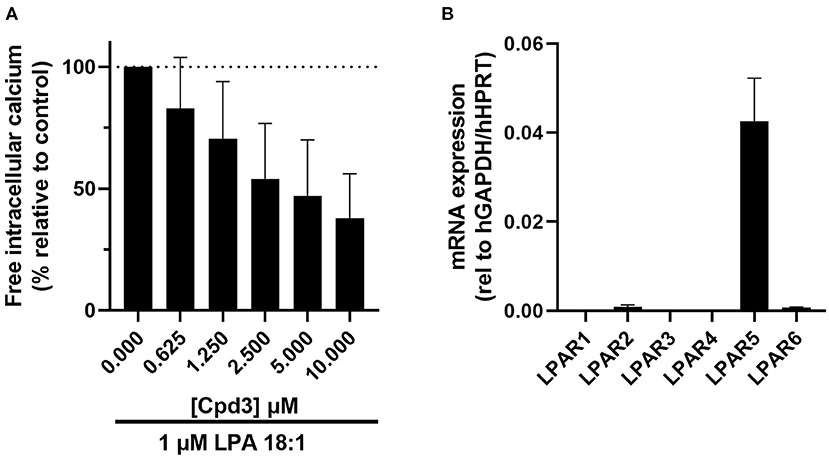
Figure 1. (A) Increase of free Ca2+ in HMC-1 cells, induced by 1μM LPA 18:1, was dose-dependently inhibited by cpd3 (maximal DMSO (vehicle) concentration 0.1%). Expressed in percentage of delta ratio relative to control (B) mRNA expression of LPA receptor 1-6 (LPAR1-6) in HMC-1 cells.
RNA isolation and RT-qPCR
HMC-1, HEK and HEK-LPAR5-TRPA1 cells were used to study LPAR1-6 mRNA expression levels. The day before start of the incubation, ± 5·105 cells per well were seeded in six-well plates. Total RNA was isolated after cell lysis with TRIreagent® (Sigma), chloroform extraction and isopropanol precipitation. After DNase treatment (Promega), cDNA was synthesized by oligo(dT) primers (Invitrogen) and random hexamers (Promega) followed by reverse transcriptase (Fermentas). qPCR analysis was performed using a LightCycler®480 II (Roche) detection system using the SensiFAST™ Sybr No-Rox mix (Bioline). All graphs depict LPAR1-6 mRNA expression levels normalized to the following reference genes that were proven to be stably expressed throughout experimental conditions: geometric mean of GAPDH and HPRT or 36B4 and HPRT.
Sequences of primers used are LPAR1: GGGCCTCATTGACACCAGC (forward) and GGAAAACCGTAATGTGCCTCTC (reverse); LPAR2: CCTGGTCAAGACTGTTGTCATC (forward) and GACTCACAGCCTAAACCATCC (reverse); LPAR3: GCTGCCGATTTCTTCGCTG (forward) and AGCAGTCAAGCTACTGTCCAG (reverse); LPAR4: TCCTTACCAACATCTATGGGAGC (forward) and ACGTTTGGAGAAGCCTTCAAAG (reverse); LPAR5: CACTTGGTGGTCTACAGCTTG (forward) and GCGTAGTAGGAGAGACGAACG (reverse); LPAR6: GGACAATGTACCCAATCACTCTC (forward) and ACTTCTCCTGACAGACCAGTTT (reverse); 36B4: TCATCAACGGTACAAACGA (forward) and GCCTTGACCTTTTCAGCAAG (reverse); GAPDH: CAAGATCATCAGCAATGCCT (forward) and CAGGGATGATGTTCTGGAGAG (reverse); HPRT: TGACCTTGATTTATTTTGCATACC (forward) and CGAGCAAGACGTTCAGTCCT (reverse).
In vivo experiments
Experimental animals
Experiments were performed with female C57Bl/6 wild type mice (Janvier), mice (23), Trpa1 KO mice [Jackson; (24)] and male Swiss wild type mice (Federal University of Parana colony). The mouse model contains a transgene for secreted phospholipase A2. The background strain is C57Bl/6. The Trpa1 KO mouse background strain is a mix of C57Bl/6J with FVB 129P2/OlaHsd. All mice were aged 8 weeks to 8 months. The difference in age is considered to have no influence on the nociception and itch responses. Mice were housed conventionally with ad libitum water and food, consisting of regular chow (Teklad 2916, Envigo). Experiments were conducted according to the institutional Animal Care and Use Committee regulations. All methods were carried out in accordance with relevant guidelines and regulations. The study was also carried out in compliance with the ARRIVE guidelines.
Scratch activity assay
Scratch activity of the animals (, Trpa1 KO and WT mice) was measured using an in-house developed system reported in (25, 26) which allows long-term measurements of scratch activity. Teflon coated 5 x 2 mm magnets (VWR European) were implanted subcutaneously under general anesthesia in both hind paws (below the knee) a week before the experiment. Accompanied by a littermate of the same gender without magnets, mice were permanently placed in a cage surrounded by a magnetic coil. An oscillograph attached to a computer registered the electric currents induced by movements of the implanted magnets. Chronic scratch activity was assessed at night (7 pm−7 am) for the duration as described with each experiment. Custom made software was used to quantify scratch and total movements exactly as described in (26). Scratch activity was expressed as the average total duration (in seconds) of scratch movements per 12 h measurement.
Cpd3 was dissolved in vehicle (15% glycofurol, 5% solutol and 40% PEG400) and administered by oral gavage (100 μl) at 17h. Measurements where started at 19h.
Formalin test
Male Swiss mice (25 ± 3g) were used and pre-treated 24 h and/or 2 h before assessment of nociceptive responses by oral gavage of vehicle or cpd3. Mice that received morphine (s.c.) were treated 30 min before the test. The treatments were performed in a blinded manner. After the treatments, the mice were habituated in the acrylic chambers during 10 min, then received formalin (5%/10 μL) or vehicle (saline, 10 μL) injection into the right hindpaw, and were returned immediately to the chambers for behavioral analyses. The time animals spent licking the injected paw was registered for 50 min, which was counted in 5-min intervals. Phase I of the formalin response was considered the interval from 0–5 min, while phase II was the interval from 15 to 30 min.
Paw withdrawal test
Male Swiss mice (25 ± 3g) were treated 24 h beforehand and again on the subsequent day, after the baseline measurement with oral gavage of cpd3. The treatments were performed in a blinded manner. Mice were habituated for about 2 h in acrylic chambers and the baseline paw withdrawal threshold (in gram) was assessed by an electronic version of von Frey filaments. A handheld force transducer (electronic anesthesiometer; Insight, Ribeirão Preto, SP, Brazil) adapted with a 0.5 mm2 polypropylene tip was used to evoke hind paw nociceptive withdrawal response. The intensity of the pressure (in gram) at the moment of paw withdrawal was automatically recorded. Carrageenan, PGE2, or saline was injected into the right hindpaw and the paw withdrawal threshold was assessed hourly up to 6 hours.
Statistical analyses
GraphPad Prism (Version 8.3.0 for Mac OS X, GraphPad Software, La Jolla, California USA) was used for statistical analyses. For nociceptive behavior, time course results were analyzed by two-way ANOVA followed by Bonferroni post hoc test. Cumulative time results were analyzed by one-way ANOVA followed by Bonferroni post hoc test. Both were expressed as the F-ratio with degrees of freedom for the numerator and denominator, followed by the p-value. For analysis of chronic scratch activity, differences between two groups under the same condition were tested by unpaired t-test, differences within the same group under different consecutive conditions by paired t-test. Results were considered statistically significant when p < 0.05.
Results
Cpd3 inhibits LPAR5 in HMC-1 cells
Cpd3 has been developed as an LPAR5-antagonist by Kozian and colleagues (20). To verify this function of cpd3, we cultured HMC-1 cells, which express high levels of LPAR5 (Figure 1B) (27), and triggered a calcium response with the agonist LPA 18:1 (1 μM). Addition of LPA in the absence of cpd3, led to an induction of free intracellular calcium. As expected, the release of calcium was diminished with increasing amounts of the LPAR5-antagonist cpd3 (Figure 1A; Supplementary Figure 1).
Cpd3 reduces inflammatory nociceptive behavior induced by formalin
We tested the effects of the LPAR5 inhibitor cpd3 on formalin-induced nociception. Male Swiss mice were treated with a single dose of vehicle, cpd3 (11 mg/kg p.o., 2 h before measurement), or morphine (5 mg/kg, s.c., 30 min before measurement). At starting point, the mice received formalin (5%/10 μL) or vehicle (saline, 10 μL) injection into the right hindpaw. The amount of licking time was measured for 50 min.
Injection of formalin into the hindpaw evoked an increase in licking behavior compared to saline-injected mice. A single dose of cpd3 (11 mg/kg) did not reduce licking behavior, but did induce a small delay in the onset of the formalin effect (Supplementary Figure 2). In order to reach a higher concentration of cpd3 in the circulation, we subsequently treated male Swiss mice twice with vehicle or cpd3 (11 mg/kg, p.o., i.e. 24 h and 2 h before formalin injection). Mice received formalin (5%/10 μL) or vehicle (saline, 10 μL) injection into the right hindpaw.
Injection of formalin into the hindpaw evoked an increase in licking behavior in both phase I [F (3, 34) = 30.68; p < 0.0001] and phase II [F (3, 34) = 8.945; p = 0.0004], which was significantly different from control mice (vehicle + saline; Figure 2). Pre-treatment with cpd3 did not change the licking response evoked by formalin in phase I [F (3, 34) = 32.99; p > 0.9999]. However, in phase II, pre-treatment with a double dose of cpd3 did reduce the licking response compared to vehicle treated mice [F (3, 34) = 8.945; p = 0.0079] (Figures 2B,C). These data indicate that cpd3 has anti-nociceptive effects affecting phase II, which may be related to modulation of inflammatory pain. Similar to pre-treatment with a single dose of cpd3, a double dose of cpd3 caused a delay in the onset of the formalin effect (Figure 2A).
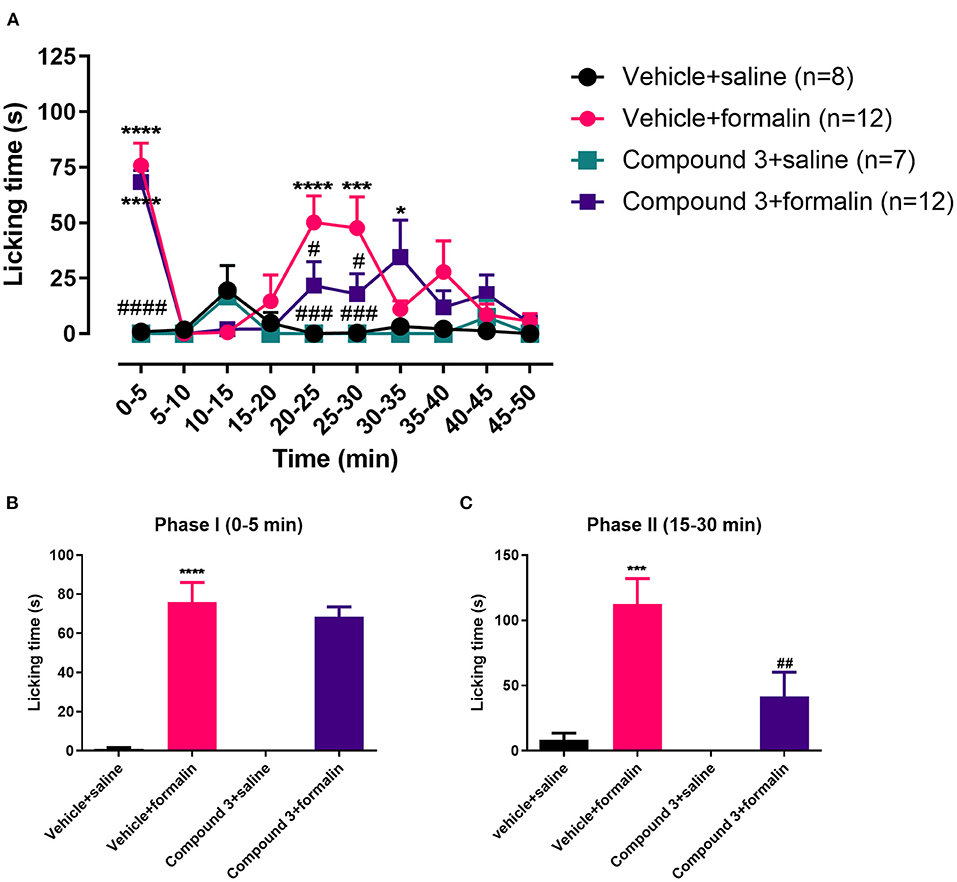
Figure 2. Time course (A) and cumulative licking time (B,C) induced by formalin injected in the hindpaw, after pre-treatment with a double dose of vehicle or cpd3 (11 mg/kg). (A) Two-way ANOVA followed by Bonferroni post hoc test. (B,C) One-way ANOVA followed by Bonferroni post hoc test. *p < 0.05; ***p < 0.001; ****p < 0.0001 compared to vehicle + saline group. #p < 0.05; ###p < 0.001; ####p < 0.0001 compared to vehicle + formalin group.
High single dosage of cpd3 reduces inflammatory nociceptive behavior in both phase I and II
In the results above, a single dose of 11 mg/kg cpd3 had no effect on licking time induced by formalin injection, whereas a double dose of 11 mg/kg cpd3 reduced the licking time in phase II. Therefore, we wanted to measure the effect of a single, higher dose of cpd3 (110 mg/kg, p.o.), 2 h before measurement. Increased licking behavior in phase I and phase II, induced by injection of formalin, was significantly reduced in both phases by a single high dose of cpd3 compared to vehicle-treated rats [phase I, t (29) = 2.238; p = 0.0331; phase II, t (29) = 2.342; p = 0.0263] (Figure 3).
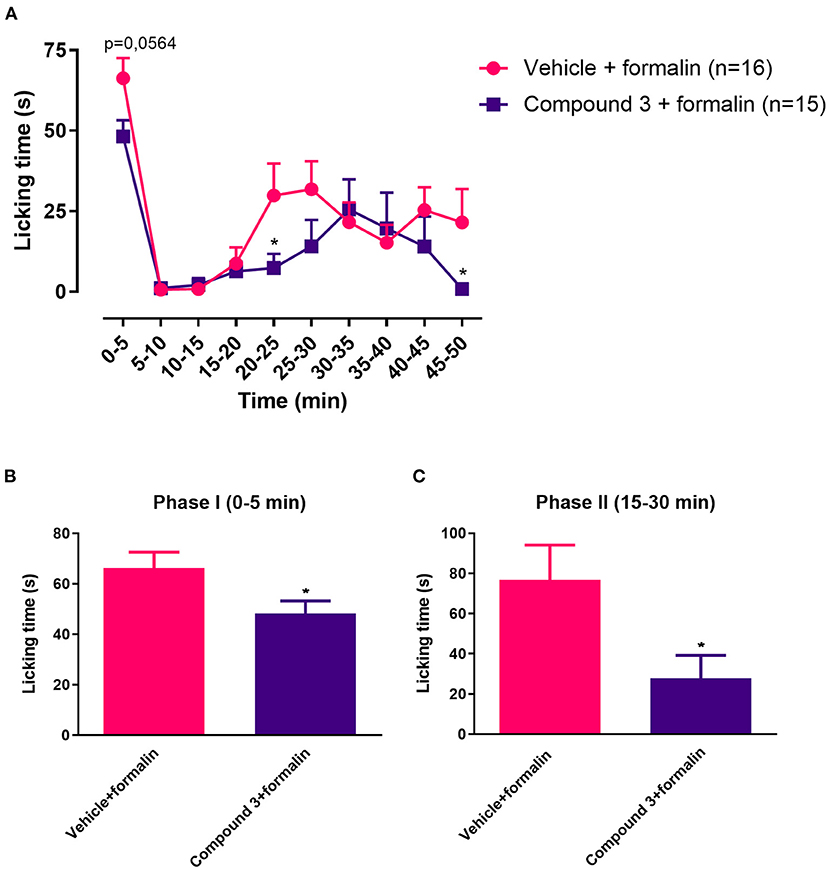
Figure 3. Time course (A) and cumulative licking time (B,C) induced by formalin injected in the hindpaw, after pre-treatment with a single dose of vehicle or cpd3 (110 mg/kg). (A) Multiple unpaired T-test of Two-way ANOVA. (B,C) T-test, *p < 0.05 compared to vehicle + formalin group.
Cpd3 reduces hyperalgesia induced by carrageenan or PGE2
Besides formalin-induced inflammatory nociception, we also studied the effect of cpd3 on inflammatory mechanical hyperalgesia. Activation of LPAR5 results in an intracellular increase of cAMP (8), which increases CREB phosphorylation and thereby induces hyperalgesia via central sensitization (15, 28, 29). Intraplantar injection of carrageenan induces mechanical hyperalgesia in mice by triggering a cytokine cascade initiated by TNF-α and CXCL1 production, which stimulate the release of IL−1β and subsequent PGE2 production (30). In turn, PGE2 sensitizes the nociceptor, by increasing intracellular cAMP content, which can be detected as mechanical hyperalgesia (31, 32).
In this experiment, inhibition of LPAR5 by cpd3 was analyzed during hyperalgesia induced with carrageenan or PGE2 after which the paw withdrawal threshold was measured. Male Swiss mice were treated twice with vehicle or cpd3 (11 mg/kg, p.o.) 24 h before as well as right before measuring (after baseline measurement). Carrageenan (200 μg/20μL), PGE2 (1 nmol/10 μL) or saline (10 μL) was injected into the right hindpaw and the paw withdrawal threshold was assessed hourly up to 6 hours. Both carrageenan and PGE2 treatment led to a strongly decreased paw withdrawal threshold compared to saline, indicating mechanical hyperalgesia, which was diminished by pre-treatment with cpd3 [Figure 4A; F (18, 222) = 2,548; p = 0.0008], [Figure 4B; F (18, 240) = 7,636; p < 0.0001]. Cpd3 pre-treatment with subsequent saline injection had no effect on the paw withdrawal threshold. These results indicate that cpd3 diminishes hyperalgesia induced by carrageenan or PGE2.
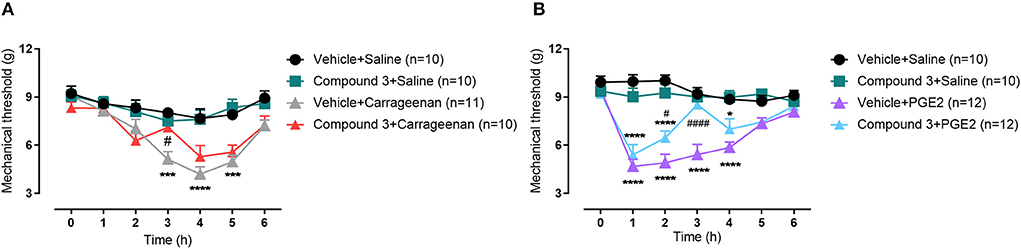
Figure 4. Time course of paw withdrawal threshold (g) induced by (A) carrageenan (200 μg/20μL) or (B) PGE2 (1 nmol/10 μL) injected in the hindpaw, after pre-treatment with a double dose of vehicle or cpd3 (11 mg/kg, p.o.). *p < 0.05; ***p < 0.001; ****p < 0.0001 compared to vehicle + saline group. #p < 0.05; ####p < 0.0001 compared to vehicle + carrageenan or vehicle + PGE2 group. Two-way ANOVA followed by Bonferroni post hoc test.
Cpd3 increases scratch activity in and WT mice
LPAR5 is not only involved in pain but also in itch perception (16). We hypothesized that LPAR5-inhibition by cpd3 would reduce scratch activity in mice. In previous studies on itch in mouse models, we have characterized phospholipase A2 transgenic mice () as a mouse model of chronic itch, which overexpress secreted PLA2 in, amongst other tissues, the skin. These mice have a skin phenotype due to this high sPLA2 activity and subsequent increased production of LPA could contribute to this phenotype (23). Hypothetically, high levels of LPA produced by sPLA2 could bind to LPAR5 and thereby induce itch signaling. Hence, inhibition of LPAR5 by cpd3 should reduce the scratch activity in mice to a level similar to WT mice.
In both and WT mice, basal scratch activity during four consecutive nights was measured without any intervention. The baseline scratch activity (mean of four nights) was significantly higher in mice (200 s Figure 5A baseline), compared to WT mice (150 s Figure 5B baseline) (unpaired t-test, p = 0.0125). This baseline difference might be due to the overexpression of secreted PLA2 in these mice, resulting in epidermal and adnexal hyperplasia, hyperkeratosis and almost total alopecia (23). Strikingly, in both and WT mice, oral administration of 11 mg/kg cpd3 showed a strong increase in scratch activity, instead of the expected decrease (Figures 5A,B). The mice showed a strong increase in scratch activity in the first night, which diminished during the following nights but remained higher than before administration (Supplementary Figure 3). We investigated the dose-dependent cpd3-induced scratch activity by treating five groups of naïve WT mice with a single dose of cpd3, containing increasing concentrations. Figure 5C shows that increasing concentrations of cpd3 from 1.375 up to 22 mg/kg, induced scratch activity up to 350% of baseline. These results suggest that cpd3 is a strong pruritogen rather than an antagonist of itch receptors.
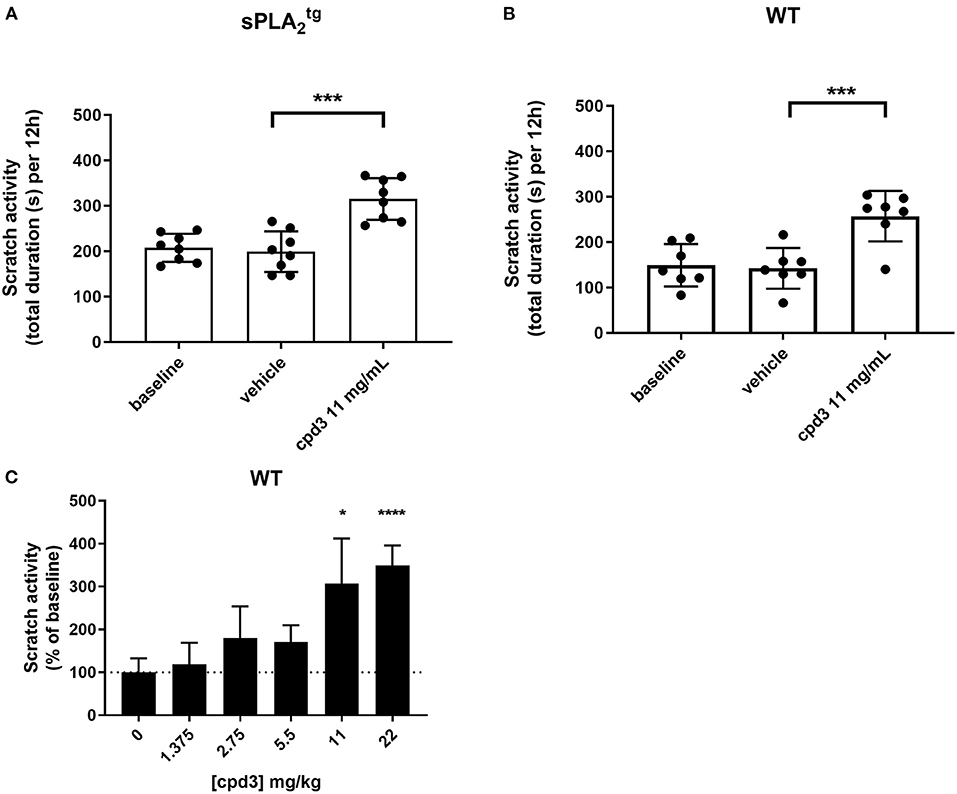
Figure 5. Scratch activity (total duration in seconds per 12 h, mean of four consecutive nights) in (A) mice (n = 8), (B) wild type (n = 7), receiving oral gavage of vehicle and subsequently cpd3 (11 mg/kg) and (C) wild type (n = 4 per group), during 12 hours after a single oral gavage of different doses of cpd3. Statistics: (A,B) paired t-test; ***p < 0.001, (C) paired t-test compared to baseline; *p < 0.05; ****p < 0.0001.
Cpd3 activates TRPA1
Based on the in vivo experiments, cpd3 appears to be an inducer of itch. One of the most prominent itch channels is the transient receptor potential ankyrin 1 (TRPA1) channel, located on sensory nerve endings (33, 34). Here, we used HEK cells with inducible TRPA1 expression and measured the free intracellular calcium induced by cpd3 administration, in the presence and absence of the TRPA1-antagonist A-967079 (A-96). Figure 6 shows a strong calcium releasing effect of cpd3 on HEK-TRPA1 cells. The competitive TRPA1-antagonist A-967079 (1 μM) was able to inhibit the effects of cpd3 completely at concentrations below 2.5 μM. The vehicle DMSO did not affect intracellular free calcium. Cpd3 also did not give a calcium response in non-transfected cells nor in cells transfected with TRPV1 (not shown).
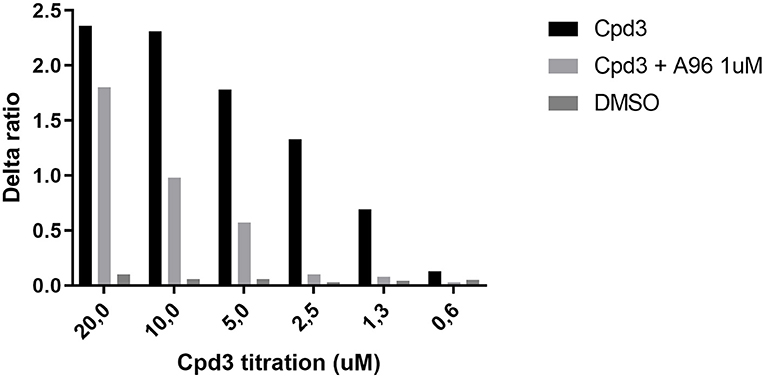
Figure 6. Increase of free Ca2+ in HEK-TRPA1 cells, measuring the effect of different concentrations of Cpd3, Cpd3 + TRPA1-antagonist A-967079, and DMSO control (max. final concentration 0.2%). Delta ratio indicates the ratio of emitted Indo-1 fluorescence (395/460 nm).
Cpd3 also induces scratch activity in Trpa1 KO mice
To establish the itch-signaling pathway of cpd3 on TRPA1 in vivo, we orally administered cpd3 at a concentration of 22 mg/kg to WT mice and to Trpa1 knockout mice (Trpa1 KO) and measured the duration of scratch activity for 12 h during the night. Figure 7A shows the increase of scratch activity in WT mice after oral gavage of 22 mg/kg cpd3. Surprisingly, in Trpa1 KO mice, cpd3 also induced a significant increase in scratch activity (Figure 7B). This suggests that the scratch-inducing effects of cpd3 are conducted by more factors than just TRPA1.
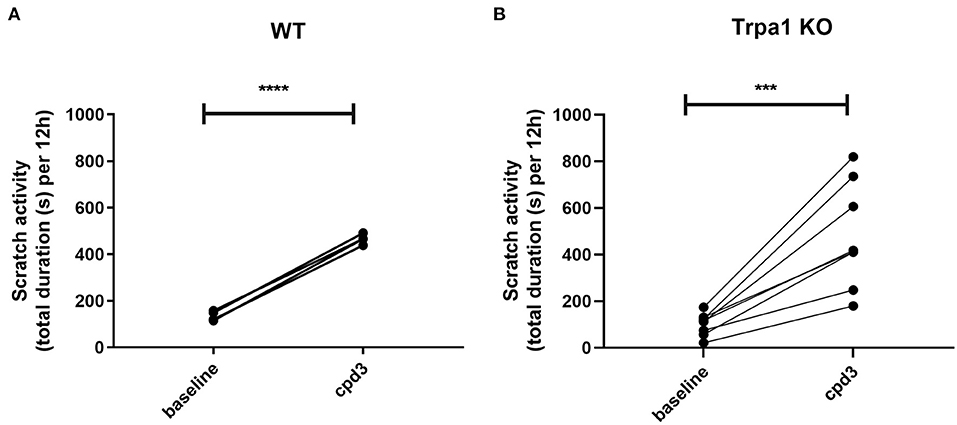
Figure 7. Scratch activity (total duration in seconds per 12 h) in (A) wild type (n = 4) and (B) Trpa1 KO mice (n = 7) receiving a single dose of 22 mg/kg cpd3 by oral gavage. Statistics: paired t-test; ***p < 0.001; ****p < 0.0001.
Discussion
Cpd3 has been developed as an LPAR5-antagonist to inhibit LPA signaling in mast cells and microglia, which play a critical role in innate immunity and inflammation (20). In inflammatory research, cpd3 can prevent LPAR5-mediated suppression of signaling in mouse CD8 T cells (35). Recently, it was also shown that cpd3 could be a versatile pharmacological tool to regulate inflammatory signaling in BV-2 microglia cells (36).
Next to inflammation, LPAR5-signaling also plays a prominent role in sensory activation (17, 37, 38). In the study of Lin et al., Lpar5-deficient mice were protected from neuropathic pain induced by partial sciatic nerve ligation (15). In a second, independent study, these mice also showed decreased pain sensitivity in tail withdrawal tests and faster recovery after inflammatory pain induced by complete Freund's adjuvant (14). Lpar5 gene expression is found in dorsal root ganglion neurons. It is both a Gα12/13- and Gq-coupled receptor, thereby able to increase cAMP production (8, 15).
In our previous work, we have shown that LPA concentrations were increased in the sera of women with intrahepatic cholestasis of pregnancy (ICP, which is characterized by chronic pruritus), and intradermal injection of LPA in mice induced an increased scratch response (25). Kittaka et al. (16) showed that of all six LPA receptors, LPAR5 is the most prominent receptor involved in itch signaling. LPAR5 stimulation causes intracellular activation of phospholipase A2 and phospholipase D, leading to intracellular LPA release, activating TRPA1 and TRPV1 channels and thereby eliciting itch sensation. LPA-induced itch-related behaviors are decreased in TRPA1 and TRPV1 deficient mice (16).
So far, cpd3 has not been studied in terms of sensory signaling. Therefore, we tested the effect of cpd3 on established pain models. The formalin test has been used for chemical pain assessment for many years (39). Formalin produces a distinct biphasic excitatory response in dorsal horn neurons. It shows an immediate acute or phasic peak of neuronal firing 0–10 min post injection, followed by a quiescent period (5 min) and a more prolonged tonic excitatory response over a period of 20–60 min after formalin injection (40). The acute phase is suggested to be due to direct activation of peripheral nociceptors and is susceptible to inhibition by local anesthetic agents and opioids. The late phase would be a response to inflammatory pain that can be inhibited by anti-inflammatory drugs and opioids (41).
In this study, we show that inflammatory nociception, induced by formalin injection, can be reduced by cpd3, when administered twice daily, or with a single high dose (Figures 2, 3). This finding corroborates previous evidence that LPAR5-antagonists may reduce inflammatory pain (17). In the presence of cpd3, a delay can be noted in the onset of the formalin effect. This might be due to the anti-inflammatory effect of cpd3 which reduces peripheral sensitization and consequently, delays the start of central sensitization. Secondly, we show that cpd3 reduces mechanical hyperalgesia, induced by carrageenan or PGE2 (Figure 4). Hyperalgesia is described as an abnormally increased sensitivity to pain, which may be caused by sensitization of nociceptors or damage to peripheral nerves and can cause hypersensitivity to a stimulus (42–44). Intraplantar injection of carrageenan is extensively used as a model of inflammatory hyperalgesia. It induces mechanical hyperalgesia in mice by triggering a cytokine cascade that culminates in stimulation of COX-2 expression and PGE2 production (30, 45). Cpd3 administration restored the paw withdrawal threshold in carrageenan treated-mice, indicating a possible effect in this pathway. In addition, cpd3 caused a significant and sustained reversal of PGE2-induced hyperalgesia, suggesting that it causes analgesia by interfering with the hyperalgesic action of the final mediator (i.e. PGE2), but not in an upstream step of the cascade. However, it has been reported that some LPAR5-antagonists are capable to reduce the expression of pro-inflammatory markers, including COX-2 (36). Peripherally, one mechanism of PGE2 induced hyperalgesia is activation of EP2 and EP4 receptors, which are coupled to GS protein and, when activated, stimulate the cAMP/PKA pathway (32). Moreover, intrathecal PGE2 causes hyperalgesia by facilitating glutamate release from presynaptic terminals in the spinal dorsal horn via EP1 receptors (46, 47). In the study of Murai et al., it has been shown that the LPAR5-antagonist AS2717638 significantly reduced mechanical allodynia induced by intrathecal PGE2 in mice (17). Altogether, these results indicate that LPAR5-antagonists may interfere with PGE2 induced hyperalgesia in the periphery as well as in the spinal cord. Our results are consistent with the study of Murai et al., in which AS2717638 could ameliorate both static mechanical allodynia and thermal hyperalgesia in a rat model of chronic constriction injury (CCI)-induced neuropathic pain (17). Together, they show the efficacy of LPAR5-antagonists in the treatment against inflammatory and neuropathic pain.
Notably, in this study we also show that cpd3 induces scratch activity in mice. We hypothesized that cpd3 would inhibit scratch activity similar to pain perception. In a mouse model for chronic itch ( mice), administration of cpd3 was expected to reduce scratch activity to the level of WT mice. However, cpd3 administration showed a surprising difference in itch perception compared to pain perception. Scratch activity was increased rather than reduced in both and WT mice after administration of cpd3. This increase happened in a dose-dependent manner (Figure 5). The results also showed that the induction of scratch activity by cpd3 is in part a transient phenomenon (Supplementary Figure 3). Administration of cpd3 caused the strongest increase in scratch activity at the first day, after which the activity decreased on subsequent days with administration of the same concentration. This suggests that involved receptors may get desensitized. However, this desensitization is only partly, since the scratch activity flattens at a level that is still higher compared to baseline, indicating a sustained increase in scratch activity after cpd3 administration. Together, these results suggest that, independent of the inhibition on LPAR5, cpd3 has a second, scratch inducing way of action.
One of the sensory channels involved in itch signaling is TRPA1. Opening of this channel leads to an influx of cations, including calcium and when located on nerve endings, this might contribute to eliciting an action potential, leading to itch sensation. Cpd3 appears to be a very strong TRPA1 agonist (Figure 6). Therefore, we administered cpd3 to Trpa1 KO mice, and hypothesized that the scratch activity would remain unchanged. However, Figure 7 shows that cpd3 administration induces scratch activity in Trpa1 KO mice as well. This suggests that TRPA1 is not the only sensory channel that can be activated by cpd3 and induce scratch activity. The pruriceptor TRPV1 is not likely to be involved since we did not see in vitro activation of TRPV1-expressing cells upon cpd3 addition. Other pruriceptors such as those from the MRGPR family could be involved.
This study showed that LPAR5 antagonism can reduce nociception. Hence, LPAR5-antagonists like cpd3 could be mediators in the reduction of inflammatory pain and hyperalgesia, but more selective compounds need to be designed to avoid off-target pruritogenic effects.
Data availability statement
The raw data supporting the conclusions of this article will be made available by the authors, without undue reservation.
Ethics statement
The animal study was reviewed and approved by Institutional Animal Care and Use Committee of the University of Amsterdam (Amsterdam, Netherlands) and the Federal University of Parana (Brasil) Ethics Committee.
Author contributions
JL wrote the manuscript with intellectual guidance by MN, HB, JS, JC, and RO. Experiments were designed by JL, JC, and RO. Experiments were performed and data was analyzed by JL, EA, AB, DT, JC, and RO. All authors contributed to the article and approved the submitted version.
Funding
This research was funded by a TOP ZonMW Grant (#40-00812-98-10054) and a grant from the Dioraphte Foundation (#1115) to RO.
Acknowledgments
We thank CAPES for providing the scholarship for EA and AB. JC is recipient of CNPq research productivity fellowship.
Conflict of interest
The authors declare that the research was conducted in the absence of any commercial or financial relationships that could be construed as a potential conflict of interest.
Publisher's note
All claims expressed in this article are solely those of the authors and do not necessarily represent those of their affiliated organizations, or those of the publisher, the editors and the reviewers. Any product that may be evaluated in this article, or claim that may be made by its manufacturer, is not guaranteed or endorsed by the publisher.
Supplementary material
The Supplementary Material for this article can be found online at: https://www.frontiersin.org/articles/10.3389/fpain.2022.963174/full#supplementary-material
Abbreviations
BV-2 cell, microglia cell; cAMP, cyclic adenosine monophosphate; Cpd3, compound 3; CREB, cAMP response element-binding protein; DMSO, dimethyl sulfoxide; HEK cell, human embryonic kidney cell; HMC-1, human mast cell; LPA, lysophosphatidic acid; LPAR5, lysophosphatidic acid receptor 5; PGE2, prostaglandin E2; PKA, protein kinase A; PKC, protein kinase C; sPLA2, secreted phospholipase A2; TRPA1, transient receptor potential ankyrin 1; TRPV1, transient receptor potential vanilloid 1.
References
1. Hashimoto T, Ohata H, Momose K. Itch-scratch responses induced by lysophosphatidic acid in mice. Pharmacology. (2004) 72:51–6. doi: 10.1159/000078632
2. Renback K, Inoue M, Ueda H. Lysophosphatidic acid-induced, pertussis toxin-sensitive nociception through a substance P release from peripheral nerve endings in mice. Neurosci Lett. (1999) 270:59–61. doi: 10.1016/S0304-3940(99)00464-4
3. Moolenaar WH. Lysophosphatidic acid, a multifunctional phospholipid messenger. J Biol Chem. (1995) 270:12949–52. doi: 10.1074/jbc.270.22.12949
4. Inoue M, Rashid MH, Fujita R, Contos JJ, Chun J, Ueda H. Initiation of neuropathic pain requires lysophosphatidic acid receptor signaling. Nat Med. (2004) 10:712–8. doi: 10.1038/nm1060
5. Oude Elferink RP, Bolier R, Beuers UH. Lysophosphatidic acid and signaling in sensory neurons. Biochim Biophys Acta. (2015) 1851:61–5. doi: 10.1016/j.bbalip.2014.09.004
6. Wilson SR, Gerhold KA, Bifolck-Fisher A, Liu Q, Patel KN, Dong X, et al. TRPA1 is required for histamine-independent, Mas-related G protein-coupled receptor-mediated itch. Nat Neurosci. (2011) 14:595–602. doi: 10.1038/nn.2789
7. Choi JW, Herr DR, Noguchi K, Yung YC, Lee CW, Mutoh T, et al. LPA receptors: subtypes and biological actions. Annu Rev Pharmacol Toxicol. (2010) 50:157–86. doi: 10.1146/annurev.pharmtox.010909.105753
8. Lee CW, Rivera R, Gardell S, Dubin AE, Chun J. GPR92 as a new G12/13- and Gq-coupled lysophosphatidic acid receptor that increases cAMP, LPA5. J Biol Chem. (2006) 281:23589–97. doi: 10.1074/jbc.M603670200
9. McIntyre TM, Pontsler AV, Silva AR, St Hilaire A, Xu Y, Hinshaw JC, et al. Identification of an intracellular receptor for lysophosphatidic acid (LPA): LPA is a transcellular PPARgamma agonist. Proc Natl Acad Sci U S A. (2003) 100:131–6. doi: 10.1073/pnas.0135855100
10. Pasternack SM, von Kugelgen I, Al Aboud K, Lee YA, Ruschendorf F, Voss K, et al. G protein-coupled receptor P2Y5 and its ligand LPA are involved in maintenance of human hair growth. Nat Genet. (2008) 40:329–34. doi: 10.1038/ng.84
11. Yung YC, Stoddard NC, Chun J. LPA receptor signaling: pharmacology, physiology, and pathophysiology. J Lipid Res. (2014) 55:1192–214. doi: 10.1194/jlr.R046458
12. Kotarsky K, Boketoft A, Bristulf J, Nilsson NE, Norberg A, Hansson S, et al. Lysophosphatidic acid binds to and activates GPR92, a G protein-coupled receptor highly expressed in gastrointestinal lymphocytes. J Pharmacol Exp Ther. (2006) 318:619–28. doi: 10.1124/jpet.105.098848
13. Ohuchi H, Hamada A, Matsuda H, Takagi A, Tanaka M, Aoki J, et al. Expression patterns of the lysophospholipid receptor genes during mouse early development. Dev Dyn. (2008) 237:3280–94. doi: 10.1002/dvdy.21736
14. Callaerts-Vegh Z, Leo S, Vermaercke B, Meert T, D'Hooge R. LPA5 receptor plays a role in pain sensitivity, emotional exploration and reversal learning. Genes Brain Behav. (2012) 11:1009–19. doi: 10.1111/j.1601-183X.2012.00840.x
15. Lin ME, Rivera RR, Chun J. Targeted deletion of LPA5 identifies novel roles for lysophosphatidic acid signaling in development of neuropathic pain. J Biol Chem. (2012) 287:17608–17. doi: 10.1074/jbc.M111.330183
16. Kittaka H, Uchida K, Fukuta N, Tominaga M. Lysophosphatidic acid-induced itch is mediated by signalling of LPA5 receptor, phospholipase D and TRPA1/TRPV1. J Physiol. (2017) 595:2681–98. doi: 10.1113/JP273961
17. Murai N, Hiyama H, Kiso T, Sekizawa T, Watabiki T, Oka H, et al. Analgesic effects of novel lysophosphatidic acid receptor 5 antagonist AS2717638 in rodents. Neuropharmacology. (2017) 126:97–107. doi: 10.1016/j.neuropharm.2017.08.032
18. Williams JR, Khandoga AL, Goyal P, Fells JI, Perygin DH, Siess W, et al. Unique ligand selectivity of the GPR92/LPA5 lysophosphatidate receptor indicates role in human platelet activation. J Biol Chem. (2009) 284:17304–19. doi: 10.1074/jbc.M109.003194
19. Kozian DH, Evers A, Florian P, Wonerow P, Joho S, Nazare M. Selective non-lipid modulator of LPA5 activity in human platelets. Bioorg Med Chem Lett. (2012) 22:5239–43. doi: 10.1016/j.bmcl.2012.06.057
20. Kozian DH, von Haeften E, Joho S, Czechtizky W, Anumala UR, Roux P, et al. Modulation of Hexadecyl-LPA-mediated activation of mast cells and microglia by a chemical probe for LPA5. Chembiochem. (2016) 17:861–5. doi: 10.1002/cbic.201500559
21. Kawamoto Y, Seo R, Murai N, Hiyama H, Oka H. Identification of potent lysophosphatidic acid receptor 5 (LPA5) antagonists as potential analgesic agents. Bioorg Med Chem. (2018) 26:257–65. doi: 10.1016/j.bmc.2017.11.038
22. Lieu T, Jayaweera G, Zhao P, Poole DP, Jensen D, Grace M, et al. The bile acid receptor TGR5 activates the TRPA1 channel to induce itch in mice. Gastroenterology. (2014) 147:1417–28. doi: 10.1053/j.gastro.2014.08.042
23. Grass DS, Felkner RH, Chiang MY, Wallace RE, Nevalainen TJ, Bennett CF, et al. Expression of human group II PLA2 in transgenic mice results in epidermal hyperplasia in the absence of inflammatory infiltrate. J Clin Invest. (1996) 97:2233–41. doi: 10.1172/JCI118664
24. Kwan KY, Allchorne AJ, Vollrath MA, Christensen AP, Zhang DS, Woolf CJ, et al. TRPA1 contributes to cold, mechanical, and chemical nociception but is not essential for hair-cell transduction. Neuron. (2006) 50:277–89. doi: 10.1016/j.neuron.2006.03.042
25. Kremer AE, Martens JJ, Kulik W, Rueff F, Kuiper EM, van Buuren HR, et al. Lysophosphatidic acid is a potential mediator of cholestatic pruritus. Gastroenterology. (2010) 139:1008–18, 18 e1. doi: 10.1053/j.gastro.2010.05.009
26. Langedijk J, Bolier R, Tolenaars D, Ten Bloemendaal L, Duijst S, de Waart D, et al. Reduced spontaneous itch in mouse models of cholestasis. Sci Rep. (2021) 11:6127. doi: 10.1038/s41598-021-85660-1
27. Lundequist A, Boyce JA. LPA5 is abundantly expressed by human mast cells and important for lysophosphatidic acid induced MIP-1β release. PLoS ONE. (2011) 6:e18192. doi: 10.1371/journal.pone.0018192
28. Hoeger-Bement MK, Sluka KA. Phosphorylation of CREB and mechanical hyperalgesia is reversed by blockade of the cAMP pathway in a time-dependent manner after repeated intramuscular acid injections. J Neurosci. (2003) 23:5437–45. doi: 10.1523/JNEUROSCI.23-13-05437.2003
29. Kawasaki Y, Kohno T, Zhuang ZY, Brenner GJ, Wang H, Van Der Meer C, et al. Ionotropic and metabotropic receptors, protein kinase A, protein kinase C, and Src contribute to C-fiber-induced ERK activation and cAMP response element-binding protein phosphorylation in dorsal horn neurons, leading to central sensitization. J Neurosci. (2004) 24:8310–21. doi: 10.1523/JNEUROSCI.2396-04.2004
30. Cunha TM, Verri WA Jr., Silva JS, Poole S, Cunha FQ, Ferreira SH. A cascade of cytokines mediates mechanical inflammatory hypernociception in mice. Proc Natl Acad Sci U S A. (2005) 102:1755–60. doi: 10.1073/pnas.0409225102
31. Sachs D, Villarreal C, Cunha F, Parada C, Ferreira S. The role of PKA and PKCepsilon pathways in prostaglandin E2-mediated hypernociception. Br J Pharmacol. (2009) 156:826–34. doi: 10.1111/j.1476-5381.2008.00093.x
32. Kawabata A. Prostaglandin E2 and pain–an update. Biol Pharm Bull. (2011) 34:1170–3. doi: 10.1248/bpb.34.1170
33. Biro T, Toth BI, Marincsak R, Dobrosi N, Geczy T, Paus R, et al. channels as novel players in the pathogenesis and therapy of itch. Biochim Biophys Acta. (2007) 1772:1004–21. doi: 10.1016/j.bbadis.2007.03.002
34. Kobayashi K, Fukuoka T, Obata K, Yamanaka H, Dai Y, Tokunaga A, et al. Distinct expression of TRPM8, TRPA1, and TRPV1 mRNAs in rat primary afferent neurons with adelta/c-fibers and colocalization with trk receptors. J Comp Neurol. (2005) 493:596–606. doi: 10.1002/cne.20794
35. Mathew D, Kremer KN, Strauch P, Tigyi G, Pelanda R, Torres RM. LPA5 Is an Inhibitory Receptor That Suppresses CD8 T-Cell Cytotoxic Function via Disruption of Early TCR Signaling. Front Immunol. (2019) 10:1159. doi: 10.3389/fimmu.2019.01159
36. Plastira I, Joshi L, Bernhart E, Schoene J, Specker E, Nazare M, et al. Small-Molecule Lysophosphatidic Acid Receptor 5 (LPAR5) Antagonists: Versatile Pharmacological Tools to Regulate Inflammatory Signaling in BV-2 Microglia Cells. Front Cell Neurosci. (2019) 13:531. doi: 10.3389/fncel.2019.00531
37. Tsukahara R, Yamamoto S, Yoshikawa K, Gotoh M, Tsukahara T, Neyama H, et al. LPA5 signaling is involved in multiple sclerosis-mediated neuropathic pain in the cuprizone mouse model. J Pharmacol Sci. (2018) 136:93–6. doi: 10.1016/j.jphs.2018.01.001
38. Ueda H. Lysophosphatidic acid signaling is the definitive mechanism underlying neuropathic pain. Pain. (2017) 158 Suppl 1:S55–65. doi: 10.1097/j.pain.0000000000000813
39. Dubuisson D, Dennis SG. The formalin test: a quantitative study of the analgesic effects of morphine, meperidine, and brain stem stimulation in rats and cats. Pain. (1977) 4:161–74. doi: 10.1016/0304-3959(77)90130-0
40. Dickenson AH, Sullivan AF. Subcutaneous formalin-induced activity of dorsal horn neurones in the rat: differential response to an intrathecal opiate administered pre or post formalin. Pain. (1987) 30:349–60. doi: 10.1016/0304-3959(87)90023-6
41. Hunskaar S, Hole K. The formalin test in mice: dissociation between inflammatory and non-inflammatory pain. Pain. (1987) 30:103–14. doi: 10.1016/0304-3959(87)90088-1
42. Jensen TS, Finnerup NB. Allodynia and hyperalgesia in neuropathic pain: clinical manifestations and mechanisms. Lancet Neurol. (2014) 13:924–35. doi: 10.1016/S1474-4422(14)70102-4
43. Sandkuhler J. Models and mechanisms of hyperalgesia and allodynia. Physiol Rev. (2009) 89:707–58. doi: 10.1152/physrev.00025.2008
44. van Amerongen G, de Boer MW, Groeneveld GJ, Hay JL, A. literature review on the pharmacological sensitivity of human evoked hyperalgesia pain models. Br J Clin Pharmacol. (2016) 82:903–22. doi: 10.1111/bcp.13018
45. Zarpelon AC, Cunha TM, Alves-Filho JC, Pinto LG, Ferreira SH, McInnes IB, et al. IL-33/ST2 signalling contributes to carrageenin-induced innate inflammation and inflammatory pain: role of cytokines, endothelin-1 and prostaglandin E2. Br J Pharmacol. (2013) 169:90–101. doi: 10.1111/bph.12110
46. Minami T, Nishihara I, Uda R, Ito S, Hyodo M, Hayaishi O. Characterization of EP-receptor subtypes involved in allodynia and hyperalgesia induced by intrathecal administration of prostaglandin E2 to mice. Br J Pharmacol. (1994) 112:735–40. doi: 10.1111/j.1476-5381.1994.tb13139.x
Keywords: compound 3, hyperalgesia, inflammatory pain, itch (pruritus), LPAR5, nociception, TRPA1 (transient receptor potential A1)
Citation: Langedijk J, Araya EI, Barroso AR, Tolenaars D, Nazaré M, Belabed H, Schoene J, Chichorro JG and Oude Elferink R (2022) An LPAR5-antagonist that reduces nociception and increases pruriception. Front. Pain Res. 3:963174. doi: 10.3389/fpain.2022.963174
Received: 07 June 2022; Accepted: 01 July 2022;
Published: 26 July 2022.
Edited by:
Liang Han, Georgia Institute of Technology, United StatesReviewed by:
Bruno Marinho, Universidade Federal Rural do Rio de Janeiro, BrazilChun Hu, South China Normal University, China
Copyright © 2022 Langedijk, Araya, Barroso, Tolenaars, Nazaré, Belabed, Schoene, Chichorro and Oude Elferink. This is an open-access article distributed under the terms of the Creative Commons Attribution License (CC BY). The use, distribution or reproduction in other forums is permitted, provided the original author(s) and the copyright owner(s) are credited and that the original publication in this journal is cited, in accordance with accepted academic practice. No use, distribution or reproduction is permitted which does not comply with these terms.
*Correspondence: Ronald Oude Elferink, r.p.oude-elferink@amsterdamumc.nl