- 1Department of Anesthesiology, Perioperative and Pain Medicine, Stanford University, Stanford, CA, United States
- 2Department of Neurological Surgery and Behavioral Neuroscience, Oregon Health & Science University, Portland, OR, United States
The sensory experience of pain depends not only on the transmission of noxious information (nociception), but on the state of the body in a biological, psychological, and social milieu. A brainstem pain-modulating system with its output node in the rostral ventromedial medulla (RVM) can regulate the threshold and gain for nociceptive transmission. This review considers the current understanding of how RVM pain-modulating neurons, namely ON-cells and OFF-cells, are engaged by “top-down” cognitive and emotional factors, as well as by “bottom-up” sensory inputs, to enhance or suppress pain.
Introduction
Pain is an unpleasant sensory and affective experience associated with actual or potential tissue damage, serving as a survival mechanism that triggers escape from injurious or potentially injurious events, promotes recuperative behaviors, and motivates learning that leads to avoidance of such stimuli in the future. The neural system encoding and processing noxious or potentially harmful stimuli is termed “nociception.” However, pain, a sensory experience, is not a direct readout of nociceptive inputs. Indeed, pain associated with a given stimulus varies quite strongly with context and behavioral state.
A dramatic example of the gap between nociception and pain is “stress-induced analgesia,” which allows an organism to escape from an immediate threat, e.g., a predator, even in the face of injuries that would normally evoke significant pain (1–3). Stress-induced analgesia prevents pain behaviors, such as tending to an injured extremity, that could interfere with an escape from the threat (4). At the opposite end of the spectrum, pain can be enhanced by stress that is less intense or by anxiety. In a state of “stress-induced hyperalgesia,” innocuous inputs may be perceived as painful (5, 6). Both stress-induced analgesia and stress-induced hyperalgesia have been demonstrated in humans and can be seen outside of the artificial environment of the laboratory, for example after severe trauma (3, 7–10). More broadly, there is abundant evidence that pain is influenced by cognitive and emotional factors, and that it can vary, subtly or dramatically, with context and behavioral state (11–13).
This flexibility in the experience of pain arises from plasticity and modulation of multiple neural circuits. This can include changes in sensitivity of primary sensory neurons, alterations in the function of nociceptive neurons at spinal and supraspinal levels, and engagement of specific pain-modulating systems. Here we focus on the latter, and specifically on the rostral ventromedial medulla (RVM), the output node of a complex pain-modulating system. The RVM projects to the dorsal horn where it controls the processing and transmission of nociceptive information, which in turn modifies the ascending signal reaching the brain (14, 15). The present review will emphasize recent findings revealing how this pain-modulating system, and specifically the RVM, can be brought into play by both “top-down” (cognitive, emotional) and “bottom-up” (sensory) inputs to enhance or suppress pain.
Rostral Ventromedial Medulla (RVM)
The RVM is defined functionally, as an area over which low-intensity ( ≤ 10 μA) electrical stimulation produces profound antinociception (18). Anatomically, the RVM is not only centered on the nucleus raphe magnus but also includes adjacent ventromedial reticular formation. The positioning of the RVM as the output node of a complex, brain-spanning network is schematized in Figure 1. The RVM receives top-down input from higher structures such as the amygdala, hypothalamus, infralimbic and prelimbic cortex, and insula, both directly and indirectly via the midbrain periaqueductal gray (PAG). The RVM also receives bottom-up input, including nociceptive information, which allows ongoing or recent noxious stimuli to influence the response to a new insult (17, 19, 20). The primary output of the RVM is to the spinal and trigeminal dorsal horns, where it regulates nociceptive processing. Importantly, this modulation is not limited to spinally mediated manifestations of nociception such as withdrawal reflexes; because it controls ascending transmission, it influences both affective and sensory dimensions of pain (21–23).
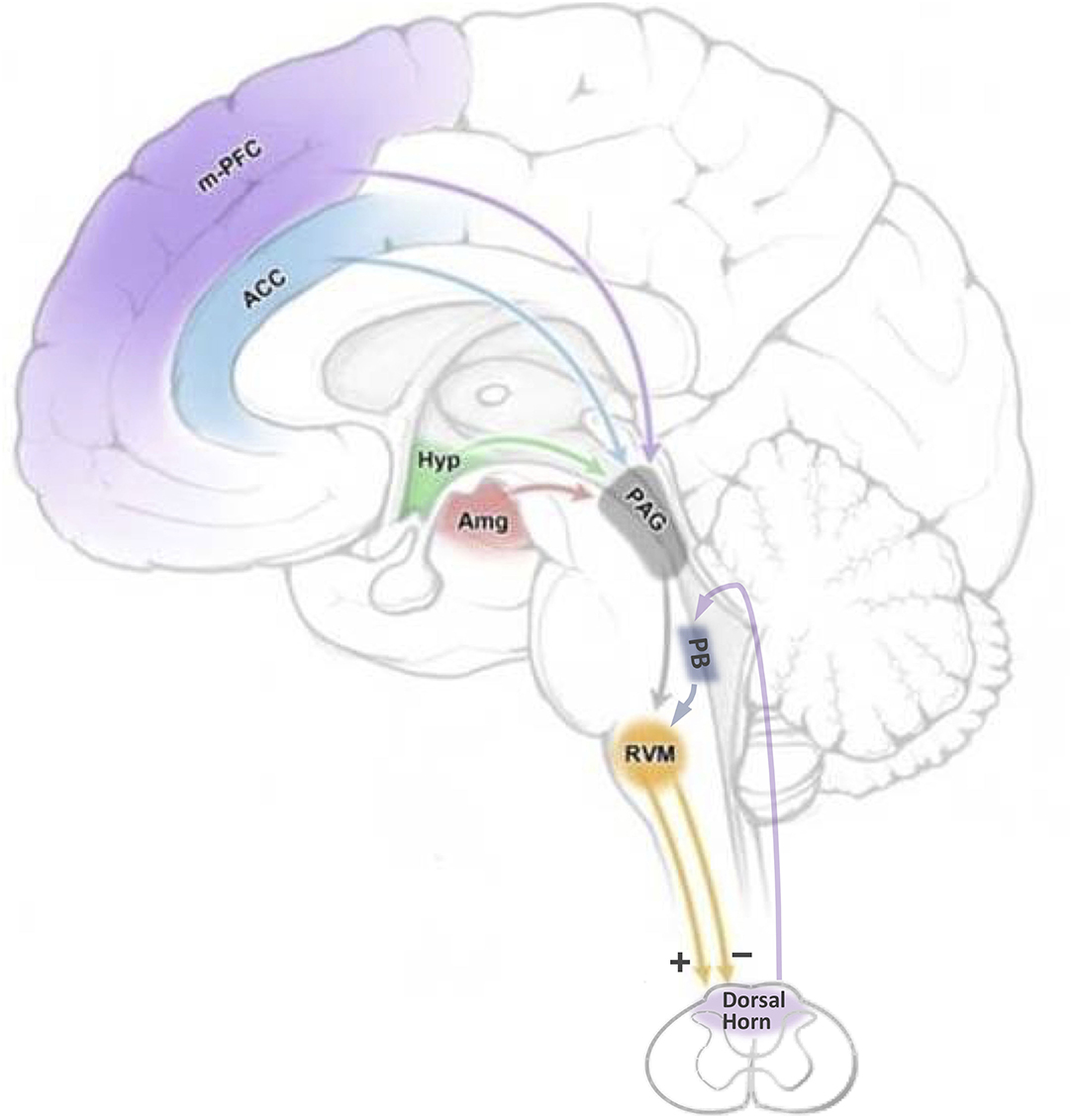
Figure 1. Top-down and bottom-up inputs through which cognitive and emotional factors could influence nociceptive transmission by modulating the firing of RVM pain-modulating neurons. Cognitive and emotional processes mediated by higher structures have the potential to influence the RVM through the PAG, and in some cases, directly. Bottom-up, nociceptive, inputs are relayed from the spinal cord to the RVM via the parabrachial complex. ACC: anterior cingulate cortex; Amg: amygdala; Hyp: hypothalamus; m-PFC: medial prefrontal cortex; PAG: periaqueductal gray; PB: parabrachial complex; RVM: rostral ventromedial medulla. Adapted from Cleary, D. and Heinricher, M.M. Neuroanatomy and neurophysiology of pain. In Winn, H.R. (Ed)., Youmans and Winn Neurological Surgery, 8th Ed., Elsevier Saunders, Philadelphia, 2022.
Based on early studies using electrical stimulation to produce analgesia, the RVM was originally viewed as part of a descending pain-inhibitory circuit or “analgesia system” (24, 25). This point of view was reinforced by evidence that the analgesic effects of mu-opioid agonists are at least partly mediated by direct actions in the RVM (26–28). However, it subsequently became clear that the RVM can also enhance nociception, and that it functions to facilitate, as well as inhibit, pain. The facilitating output from the RVM has been implicated in hypersensitivity and spontaneous pain in a wide range of chronic pain models (22, 23, 29, 30).
The Antinociceptive and Pronociceptive Outputs From the RVM Are Mediated by Distinct, Physiologically Definable, Classes of Neurons
The pain-inhibiting and pain-facilitating outputs of the RVM are respectively mediated by two, physiologically defined, classes of neurons, referred to as “OFF-cells” and “ON-cells” [Figure 2, for historical review, see Fields and Heinricher (18)]. Pain-inhibiting OFF-cells exhibit a GABA-mediated “pause” in any ongoing firing beginning a few hundred milliseconds prior to nocifensive withdrawal behaviors, and the block of that pause produces antinociception. ON-cells enter an active state (“burst”) beginning just after the OFF-cells stop firing and immediately prior to a nocifensive withdrawal. ON-cell activity enhances nociception, and selective activation of ON-cells (e.g., pharmacologically) is sufficient to produce measurable behavioral hyperalgesia (31). Conversely, selective block of ON-cell activity can reverse hyperalgesia, including stress-induced hyperalgesia (32–37).
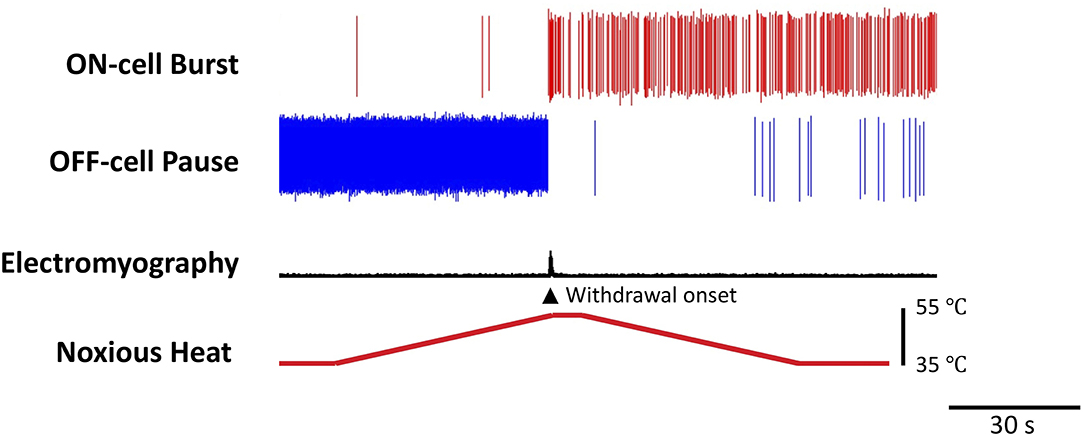
Figure 2. Reciprocal firing patterns of pain-modulating RVM ON- and OFF-cells. Examples of ON- and OFF-cell firing changes associated with nocifensive withdrawal evoked by a noxious heat stimulus. The OFF-cell pauses and ON-cell bursts immediately prior to the behavior. The threshold at which the OFF-cells pause correlates with the threshold for nocifensor withdrawals and periods of OFF-cell inactivity are associated with a lower threshold (16). In contrast, the magnitude of the ON-cell burst is positively correlated with the magnitude of behavioral responses (17). Heat (bottom trace) was applied to the plantar surface of the hind paw using a Peltier device. Withdrawal reflex was monitored using an electromyogram (EMG). The OFF-cell “pauses” and ON-cell “bursts” immediately prior to the withdrawal. The duration of the pause and burst can range from a few seconds, as here, to many minutes.
Not all RVM neurons are OFF-cells or ON-cells. However, the remaining cells, called “NEUTRAL-cells,” show no nociceptive responses and exhibit pharmacology distinct from that of ON- and OFF-cells. Although all three classes are output neurons, and project to the dorsal horn (38, 39), whether NEUTRAL-cells have a role in pain-modulation remains an open question.
ON-cells can be identified by expression of the mu-opioid receptor (40, 41), and express the neurokinin1 receptor (34). A molecular marker has not been identified as specific for either OFF-cells or NEUTRAL-cells, and it has become clear that none of the three classes maps to a particular neurotransmitter. For example, a majority of all three classes are GABAergic (42), and consistent with this, both pain-facilitating and pain-inhibiting outputs from the RVM have been shown to include GABAergic neurons in functional studies (43, 44). Serotonin is found in both ON-cells and NEUTRAL-cells (42, 45).
Both ON- and OFF-cells exhibit fluctuating spontaneous activity, with alternating silent and active periods. Activity within each class is in phase, and activity of the two classes is antiphase-synchronized under unstimulated conditions, as well as when an OFF-cell pause and ON-cell burst occur in association with a nocifensive withdrawal (46, 47). Thus, OFF-cells are silent when ON-cells are active and vice-versa. The complementary outputs from the two classes, therefore, modulate dorsal horn nociceptive transmission in parallel. Under basal conditions (i.e., in the absence of persistent injury or inflammation), the nociceptive threshold is measurably lower if a noxious stimulus is delivered during a period when the ON-cell population is active compared to when the OFF-cells are active (16), and inactivation of RVM can produce hyperalgesia due to loss of OFF-cell output (48). Subtle shifts in nociceptive sensitivity mediated by these normal fluctuations in ON- and OFF-cell firing likely contribute to the prioritization of pain behaviors relative to other behavioral priorities, such as feeding (49, 50). However, factors that eliminate the OFF-cell pause, such as mu-opioid agonist administration, can entirely suppress nociceptive behavior (51). Conversely, treatments that lead to sustained activation of ON-cells (and suppression of OFF-cell activity) produce hyperalgesia (31–33, 52–54). Interestingly, the net behavioral effect of experimental co-activation of OFF- and ON-cells (e.g., electrical stimulation or local administration of a high dose of a GABAA receptor antagonist) is antinociception, implying that the antinociceptive effect of OFF-cell activity is sufficient to override the pro-nociceptive effect of ON-cell activity. However, these two classes are not generally simultaneously active in the absence of experimental manipulation.
Interactions within the RVM itself are almost entirely unexplored. One thing is known, however, which is that ON-cells are not inhibitory interneurons mediating the OFF-cell pause. This was originally seen as a possibility since the ON-cells are most active at the time of the OFF-cell pause (18). However, the inactivation of ON-cells has no effect on the OFF-cell pause (55), and the onset of the ON-cell burst almost invariably lags the start of the OFF-cell pause (56). These two lines of evidence argue strongly that the ON-cell burst and OFF-cell pause represent parallel processes, and that both are triggered by input from outside the RVM.
In sum, following the description of ON- and OFF-cells almost 40 years ago (47), the focus has been on how these neurons function as the output of a midline brainstem pain-modulating system. Experimental approaches using direct and selective activation or inactivation of each RVM cell class, as well as correlative analyses have demonstrated that OFF-cells exert a net antinociceptive effect and ON-cells a net pro-nociceptive effect. Moreover, a significant body of evidence points to a shift in the balance between OFF- and ON-cell activity such that ON-cells predominate as a factor in chronic pain (30, 57). This foundational work is now the basis for the next critical question: when and how is this system recruited to modulate pain? In the next sections, we consider “top-down” and “bottom-up” recruitment of the RVM to suppress or facilitate pain.
“Top-Down” Engagement of Descending Control
Pain Can Be Modulated by “Top-Down” Cognitive and Emotional Factors
The ability of “top-down” influences to modulate the experience of pain has been recognized for centuries. Stress-induced analgesia was an important stimulus to research in the latter half of the twentieth century: excitement was in part because delineation of the circuitry mediating stress-induced analgesia demonstrated the existence of an intrinsic capacity to modulate pain, and also because this same circuitry was discovered to mediate the analgesic effects of opioid analgesic drugs (11). However, modulation of pain by cognitive and emotional factors, including attention, placebo/nocebo, mood, social cues, and other motivational states is well documented, if generally less dramatic than stress-induced analgesia (12). Circuits mediating these effects are now a focus of research across the field, and cortico-cortical/cortico-limbic interactions almost certainly play some role. However, relevant cortical structures also have connections with the descending pain-modulation system (Figure 1). Anterior cingulate, prefrontal areas, and insula all project to the PAG and/or RVM, as do the amygdala and a number of hypothalamic nuclei (58–62). Moreover, there is evidence from imaging studies that the RVM is engaged in humans by a range of top-down factors, including attention and placebo (63–65). However, imaging studies, based on blood oxygenation, cannot distinguish recruitment of pain-facilitating ON-cells from activation of pain-suppressing OFF-cells, as these populations are not segregated anatomically. However, sophisticated studies using juxtacellular recording demonstrate that both ON-cells and OFF-cells receive inputs from the PAG, with the majority being GABAergic in both cases (NEUTRAL-cells also receive a substantial GABAergic projection from the PAG) (66). Below we consider some critical studies that tease out the recruitment of these defined pain-modulating populations by top-down influences.
Fear and Stress Engage the RVM to Suppress or Enhance Pain
Intense fear or stress triggers analgesia, mediated by engagement of RVM OFF-cells via the basolateral amygdala (67, 68). By contrast, mild stress, such as air-puff to the face in rodents, can produce hyperalgesia. Inactivation of the dorsomedial hypothalamus (DMH) has been shown to interfere with a variety of stress-related responses, such as increased sympathetic drive and behavioral hyperactivity (69–71). DMH projects to the RVM, and stimulation of the DMH induces hyperalgesia mediated by RVM ON-cells, as well as physiological and behavioral changes associated with mild stress (32, 37, 72–74).
Balance of RVM ON- and OFF-Cell Activity Is Modulated by Other Motivational States
The effect of behavioral state on pain is not limited to contexts with negative emotional valence. Feeding has been shown in several species to be accompanied by measurably reduced responses to noxious stimuli (49, 50, 75). This hypoalgesia is associated with reduced ON-cell activity and increased OFF-cell activity, and is eliminated by blockade of the RVM (75). However, the circuitry through which feeding-related input gains access to the RVM has not been determined.
Interestingly, there was a recent report that consumption of a sweet drink did not lead to hypoalgesia in adult humans, although sucrose is reported to ease pain in infants (76). These authors suggest that the lack of effect in adults may be because of the relative ease of access to sweets in modern society, reducing the hedonic impact of the manipulation. In any case, the idea that events with a positive hedonic valence can engage the RVM to modulate pain raises the question of how this happens, and tracing the relevant circuits is an interesting direction for future research. It should also be mentioned here that hunger, like feeding, has been reported to interfere with nociception (77). However, circuitry mediating this effect has been considered from a sensory perspective, and whether pain-modulating circuitry is engaged has not been investigated.
As shown in these examples, top-down inputs can fine-tune the activity of RVM pain-modulating neurons, allowing an organism to adjust sensitivity to potentially painful stimuli depending on other behavioral and physiological priorities.
“Bottom-Up” Inputs
The burst and pause that define ON- and OFF-cells are responses to “bottom-up” sensory inputs. This rapid “switch” in RVM activity is closely linked to the execution of nocifensive withdrawals that limit or prevent serious injury but has further value as a short-term (seconds to minutes) positive feedback loop reducing the threshold for responding to subsequent stimuli in a potentially dangerous environment (17, 19). Further, sensitization of ON- and OFF-cells, so that the burst and pause are evoked during innocuous stimulation, contributes to allodynia and hyperalgesia in persistent inflammation and following nerve injury (29, 30, 33, 54, 78–80).
A relay through the parabrachial complex (PB) to RVM contributes to both the ON-cell pause and OFF-cell burst. PB is the primary supraspinal target of nociceptive transmission neurons in the superficial dorsal horn (81–83) and projects to the RVM as well as to the PAG and amygdala (84–87). Both cell classes respond to optogenetic stimulation of local parabrachial terminals at short latency, indicating that both receive direct input from PB (88). Optogenetic inhibition of PB terminals in the RVM attenuates ON- and OFF-cell responses to acute noxious stimuli (88). PB also conveys information from inflamed tissue to the RVM, contributing to sensitization of ON- and OFF-cells and hyperalgesia (89). Interestingly, while information related to an acute noxious stimulus or inflammation is relayed through the PB contralateral to the inflamed site, information about chronic inflammation is relayed through the PB ipsilateral to the inflammation (89).
Other potential sources of nociceptive input to the RVM include direct spinoreticular projections, although these have been considered sparse (90, 91). Relays through higher structures such as the amygdala or insula are also likely, although these higher structures are not required under basal conditions (60, 92, 93).
Adaptation and Latent Sensitization
As reviewed above, acute injury triggers positive feedback mediated by the recruitment of ON-cells and suppression of OFF-cell firing. This circuit helps establish behavioral hyperalgesia and can be considered protective against further tissue damage. However, a lowered threshold for triggering the ON-cell burst and OFF-cell pause cannot be the whole story. The net influence of the RVM may be time-dependent and reflect a combination of the lowered threshold for evoked responses (i.e., sensitization) and the balance of ongoing activity in the two classes. The latter may serve to limit hyperalgesia (23, 54). For example, in the Complete Freund's adjuvant (CFA) model of inflammatory pain, CFA administration triggers a shift to a prolonged (hours) period of ON-cell firing and OFF-cell suppression. And as would be expected, blocking the RVM within this period early in developing inflammation reverses hyperalgesia in the inflamed paw. However, over subsequent days, this ongoing firing returns to a more normal pattern of alternation, with periods of ON-cell and OFF-cell activity, although the neurons remain sensitized to stimulation of the inflamed paw. Silencing the RVM in fully developed inflammation does not block hyperalgesia and in fact, can enhance hyperalgesia (54). Similar apparently contradictory effects have also been reported after nerve injury. Comparing animals that did and did not develop allodynia after spinal nerve ligation, De Felice and colleagues (23) reported that inactivating the RVM reversed hyperalgesia in the subset of animals that displayed allodynia, but precipitated allodynia and conditioned place aversion in those animals that had not developed behavioral allodynia. This implies that an aberrant nociceptive transmission system can be masked by descending control, preventing the emergence of a pathological pain state. These findings also suggest that understanding the modulatory influence of the RVM requires consideration of ongoing “tone” as well as evoked responses.
The idea that descending control can simultaneously promote and suppress hypersensitivity is particularly compelling in the context of “latent sensitization” (94). This refers to the fact that pain behaviors frequently resolve following an injury, yet animals demonstrate greater susceptibility to a pain response upon subsequent injury or stress (95). Administration of opioid antagonists or blocking of descending inhibition reveals hyperalgesia in animals that have apparently recovered revealing latent sensitization (96, 97). This suggests that the restored balance between ON- and OFF-cell output described above (54) masks the fact that nociceptive transmission remains sensitized. When this compensatory inhibitory system fails, pathological pain results.
Discussion
The sensation of pain is subjective, and unique to a given individual in a specific context. This is because the sensory experience of pain depends not only on the input of noxious information (nociception), but on the state of the body in a biological, psychological, and social milieu. The brainstem pain-modulating system provides a dedicated circuit through which “bottom-up” sensory inputs and “top-down” cognitive and emotional factors can adjust the threshold and gain for nociceptive transmission.
The output of the best-studied modulatory system is through the RVM. The bidirectional modulatory effects exerted by two RVM cell populations, “ON-cells” and “OFF-cells” are now well documented. However, important open questions remain. One is whether there is a molecular “marker” that defines each cell class. The use of optogenetic manipulation of molecularly defined populations is increasingly popular but should be interpreted with appropriate caution. The fact that activation of a molecularly defined population evokes a particular behavior, or that suppression of such a population interferes with this behavior, is frequently interpreted to mean that this population as a whole is responsible for the behavior. However, without evidence that a molecularly defined population is functionally coherent, this is an overinterpretation. Without additional evidence, a more correct conclusion would be that at least some subset of the population contributes to the behavior. A second key open question is exactly how the output from the RVM interfaces with the complex nociceptive circuitry within the dorsal horn. This is certainly an important opportunity for further understanding, and will likely advance in parallel with increasingly sophisticated analyses of dorsal horn circuits.
Finally, efforts to understand top-down inputs to the RVM are starting to bear fruit. The analysis of how intense and mild stress respectively recruit OFF-cells and ON-cells to produce analgesia and hyperalgesia provides a model for teasing out the influence of other cognitive and emotional factors.
In sum, both bottom-up and top-down inputs can influence the output from the RVM, modulating nociceptive transmission, and hence pain. Understanding the interaction between these inputs and defined RVM cell classes will continue to elucidate central mechanisms of pain modulation. This may ultimately make it possible to use these inputs to engage this system, treating clinically relevant pathological pain with fewer side effects.
Author Contributions
QC and MH wrote the paper. Both authors contributed to the article and approved the submitted version.
Funding
MH is supported by grants from the NIH (NS098660 and NS120486).
Conflict of Interest
The authors declare that the research was conducted in the absence of any commercial or financial relationships that could be construed as a potential conflict of interest.
Publisher's Note
All claims expressed in this article are solely those of the authors and do not necessarily represent those of their affiliated organizations, or those of the publisher, the editors and the reviewers. Any product that may be evaluated in this article, or claim that may be made by its manufacturer, is not guaranteed or endorsed by the publisher.
References
1. Butler RK, Finn DP. Stress-induced analgesia. Prog Neurobiol. (2009) 88:184–202. doi: 10.1016/j.pneurobio.2009.04.003
2. Amit Z, Galina ZH. Stress-induced analgesia: adaptive pain suppression. Physiol Rev. (1986) 66:1091–120. doi: 10.1152/physrev.1986.66.4.1091
3. Wall PD. On the relation of injury to pain. Pain. (1979) 6:253–64. doi: 10.1016/0304-3959(79)90047-2
4. Bolles RC, Fanselow MS. A perceptual-defensive-recuperative model of fear and pain. Behav Brain Sci. (1980) 3:291–301. doi: 10.1017/S0140525X0000491X
5. Rivat C, Laboureyras E, Laulin JP, Le Roy C, Richebe P, Simonnet G. Non-nociceptive environmental stress induces hyperalgesia, not analgesia, in pain and opioid-experienced rats. Neuropsychopharmacology. (2007) 32:2217–28. doi: 10.1038/sj.npp.1301340
6. Imbe H, Iwai-Liao Y, Senba E. Stress-induced hyperalgesia: animal models and putative mechanisms. Front Biosci. (2006) 11:2179–92. doi: 10.2741/1960
7. Elman I, Borsook D. Threat response system: parallel brain processes in pain Vis-À-Vis fear and anxiety. Front Psychiatry. (2018) 9:29. doi: 10.3389/fpsyt.2018.00029
8. Rhudy JL, Meagher MW. Fear and anxiety: divergent effects on human pain thresholds. Pain. (2000) 84:65–75. doi: 10.1016/S0304-3959(99)00183-9
9. Gracely RH, McGrath P, Dubner R. Validity and sensitivity of ratio scales of sensory and affective verbal pain descriptors: manipulation of affect by diazepam. Pain. (1978) 5:19–29. doi: 10.1016/0304-3959(78)90021-0
10. Willer JC, Dehen H, Cambier J. Stress-induced analgesia in humans: endogenous opioids and naloxone-reversible depression of pain reflexes. Science. (1981) 212:689–91. doi: 10.1126/science.6261330
11. Fields H. State-dependent opioid control of pain. Nat Rev Neurosci. (2004) 5:565–75. doi: 10.1038/nrn1431
12. Bushnell MC, Ceko M, Low LA. Cognitive and emotional control of pain and its disruption in chronic pain. Nat Rev Neurosci. (2013) 14:502–11. doi: 10.1038/nrn3516
13. Wiech K, Ploner M, Tracey I. Neurocognitive aspects of pain perception. Trends Cogn Sci. (2008) 12:306–13. doi: 10.1016/j.tics.2008.05.005
14. Heinricher MM, Fields HL. Central Nervous System Mechanisms of Pain Modulation. In: McMahon S, Koltzenburg M, Tracey I, Turk DC, editors. Wall and Melzack's Textbook of Pain, 6th Ed. London: Elsevier. (2013). p. 129–42.
15. Heinricher MM, Ingram SL. The brainstem and nociceptive modulation. In: Bushnell MC, Basbaum AI, editors. The Science of Pain. San Diego: Academic Press (2008). p. 593–626.
16. Heinricher MM, Barbaro NM, Fields HL. Putative nociceptive modulating neurons in the rostral ventromedial medulla of the rat: firing of On- and Off-cells is related to nociceptive responsiveness. Somatosens Mot Res. (1989) 6:427–39. doi: 10.3109/08990228909144685
17. Jinks SL, Carstens EE, Antognini JF. Glutamate receptor blockade in the rostral ventromedial medulla reduces the force of multisegmental motor responses to supramaximal noxious stimuli. Neurosci Lett. (2007) 426:175–80. doi: 10.1016/j.neulet.2007.08.060
18. Fields HL, Heinricher MM. Anatomy and physiology of a nociceptive modulatory system. Philos Trans of the R Soc Lond B Biol Sci. (1985) 308:361–74. doi: 10.1098/rstb.1985.0037
19. Hernandez N, Dmitrieva N, Vanegas H. Medullary on-cell activity during tail-flick inhibition produced by heterotopic noxious stimulation. Pain. (1994) 58:393–401. doi: 10.1016/0304-3959(94)90134-1
20. Martenson ME, Halawa OI, Tonsfeldt KJ, Maxwell CA, Hammack N, Mist SD, et al. Possible neural mechanism for photosensitivity in chronic pain. Pain. (2016) 157:868–78. doi: 10.1097/j.pain.0000000000000450
21. Gomtsian L, Bannister K, Eyde N, Robles D, Dickenson AH, Porreca F, et al. Morphine effects within the rodent anterior cingulate cortex and rostral ventromedial medulla reveal separable modulation of affective and sensory qualities of acute or chronic pain. Pain. (2018) 159:2512–21. doi: 10.1097/j.pain.0000000000001355
22. King T, Vera-Portocarrero L, Gutierrez T, Vanderah TW, Dussor G, Lai J, et al. Unmasking the tonic-aversive state in neuropathic pain. Nat Neurosci. (2009) 12:1364–6. doi: 10.1038/nn.2407
23. De Felice M, Sanoja R, Wang R, Vera-Portocarrero L, Oyarzo J, King T, et al. Engagement of descending inhibition from the rostral ventromedial medulla protects against chronic neuropathic pain. Pain. (2011) 152:2701–9. doi: 10.1016/j.pain.2011.06.008
24. Mayer DJ, Wolfle TL, Akil H, Carder B, Liebeskind JC. Analgesia from electrical stimulation in the brainstem of the rat. Science. (1971) 174:1351–4. doi: 10.1126/science.174.4016.1351
25. Oliveras JL, Besson JM. Stimulation-produced analgesia in animals: behavioural investigations. Prog Brain Res. (1988) 77:141–57. doi: 10.1016/S0079-6123(08)62781-8
26. Dickenson AH, Oliveras JL, Besson JM. Role of the nucleus raphe magnus in opiate analgesia as studied by the microinjection technique in the rat. Brain Res. (1979) 170:95–111. doi: 10.1016/0006-8993(79)90943-0
27. Proudfit HK. Reversible inactivation of raphe magnus neurons: effects on nociceptive threshold and morphine-induced analgesia. Brain Res. (1980) 201:459–64. doi: 10.1016/0006-8993(80)91053-7
28. Jensen TS, Yaksh TLIII. Comparison of the antinociceptive action of mu and delta opioid receptor ligands in the periaqueductal gray matter, medial and paramedial ventral medulla in the rat as studied by the microinjection technique. Brain Res. (1986) 372:301–12. doi: 10.1016/0006-8993(86)91138-8
29. Porreca F, Ossipov MH, Gebhart GF. Chronic pain and medullary descending facilitation. Trends Neurosci. (2002) 25:319–25. doi: 10.1016/S0166-2236(02)02157-4
30. Heinricher MM, Tavares I, Leith JL, Lumb BM. Descending control of nociception: specificity, recruitment and plasticity. Brain Res Rev. (2009) 60:214–25. doi: 10.1016/j.brainresrev.2008.12.009
31. Neubert MJ, Kincaid W, Heinricher MM. Nociceptive facilitating neurons in the rostral ventromedial medulla. Pain. (2004) 110:158–65. doi: 10.1016/j.pain.2004.03.017
32. Martenson ME, Cetas JS, Heinricher MM. A possible neural basis for stress-induced hyperalgesia. Pain. (2009) 142:236–44. doi: 10.1016/j.pain.2009.01.011
33. Pacharinsak C, Khasabov SG, Beitz AJ, Simone DA. NK-1 receptors in the rostral ventromedial medulla contribute to hyperalgesia produced by intraplantar injection of capsaicin. Pain. (2008) 139:34–46. doi: 10.1016/j.pain.2008.02.032
34. Brink TS, Pacharinsak C, Khasabov SG, Beitz AJ, Simone DA. Differential modulation of neurons in the rostral ventromedial medulla by neurokinin-1 receptors. J Neurophysiol. (2012) 107:1210–21. doi: 10.1152/jn.00678.2011
35. Porreca F, Burgess SE, Gardell LR, Vanderah TW, Malan TP Jr, Ossipov MH, et al. Inhibition of neuropathic pain by selective ablation of brainstem medullary cells expressing the μ-opioid receptor. J Neurosci. (2001) 21:5281–8. doi: 10.1523/JNEUROSCI.21-14-05281.2001
36. Burgess SE, Gardell LR, Ossipov MH, Malan TP Jr, Vanderah TW, Lai J, et al. Time-dependent descending facilitation from the rostral ventromedial medulla maintains, but does not initiate. Neuropathic Pain J Neurosci. (2002) 22:5129–36. doi: 10.1523/JNEUROSCI.22-12-05129.2002
37. Wagner KM, Roeder Z, Desrochers K, Buhler AV, Heinricher MM, Cleary DR. The dorsomedial hypothalamus mediates stress-induced hyperalgesia and is the source of the pronociceptive peptide cholecystokinin in the rostral ventromedial medulla. Neuroscience. (2013) 238:29–38. doi: 10.1016/j.neuroscience.2013.02.009
38. Fields HL, Malick A, Burstein R. Dorsal horn projection targets of ON and OFF cells in the rostral ventromedial medulla. J Neurophysiol. (1995) 74:1742–59. doi: 10.1152/jn.1995.74.4.1742
39. Vanegas H, Barbaro NM, Fields HL. Tail-flick related activity in medullospinal neurons. Brain Res. (1984) 321:135–41. doi: 10.1016/0006-8993(84)90689-9
40. Barbaro NM, Heinricher MM, Fields HL. Putative pain modulating neurons in the rostral ventral medulla: reflex-related activity predicts effects of morphine. Brain Res. (1986) 366:203–10. doi: 10.1016/0006-8993(86)91296-5
41. Heinricher MM, Morgan MM, Fields HL. Direct and indirect actions of morphine on medullary neurons that modulate nociception. Neuroscience. (1992) 48:533–43. doi: 10.1016/0306-4522(92)90400-V
42. Winkler CW, Hermes SM, Chavkin CI, Drake CT, Morrison SF, Aicher SA. Kappa opioid receptor (Kor) and GAD67 immunoreactivity are found in OFF and NEUTRAL cells in the rostral ventromedial medulla. J Neurophysiol. (2006) 96:3465–73. doi: 10.1152/jn.00676.2006
43. Francois A, Low SA, Sypek EI, Christensen AJ, Sotoudeh C, Beier KT, et al. A brainstem-spinal cord inhibitory circuit for mechanical pain modulation by GABA and enkephalins. Neuron (2017) 93:822–39.e6. doi: 10.1016/j.neuron.2017.01.008
44. Zhang Y, Zhao E, Takatoh S, Rodriguez J, Han BX, Zhou X, et al. Identifying local and descending inputs for primary sensory neurons. J Clin Invest. (2015) 125:3782–94. doi: 10.1172/JCI81156
45. Gau R, Sevoz-Couche C, Hamon M, Bernard JF. Noxious stimulation excites serotonergic neurons: a comparison between the lateral paragigantocellular reticular and the raphe magnus nuclei. Pain. (2012) 154:647–59. doi: 10.1016/j.pain.2012.09.012
46. Barbaro NM, Heinricher MM, Fields HL. Putative nociceptive modulatory neurons in the rostral ventromedial medulla of the rat display highly correlated firing patterns. Somatosens Mot Res. (1989) 6:413–25. doi: 10.3109/08990228909144684
47. Fields HL, Bry J, Hentall I, Zorman G. The activity of neurons in the rostral medulla of the rat during withdrawal from noxious heat. J Neurosci. (1983) 3:2545–52. doi: 10.1523/JNEUROSCI.03-12-02545.1983
48. Heinricher MM, Kaplan HJ. GABA-mediated inhibition in rostral ventromedial medulla: role in nociceptive modulation in the lightly anesthetized rat. Pain. (1991) 47:105–13. doi: 10.1016/0304-3959(91)90017-R
49. Casey KL, Morrow TJ. Supraspinal nocifensive responses of cats: spinal cord pathways, monoamines, and modulation. J Comp Neurol. (1988) 270:591–605. doi: 10.1002/cne.902700412
50. LaGraize SC, Borzan J, Rinker MM, Kopp JL, Fuchs PN. Behavioral evidence for competing motivational drives of nociception and hunger. Neurosci Lett. (2004) 372:30–4. doi: 10.1016/j.neulet.2004.09.008
51. Heinricher MM, Morgan MM, Tortorici V, Fields HL. Disinhibition of Off-cells and antinociception produced by an opioid action within the rostral ventromedial medulla. Neuroscience. (1994) 63:279–88. doi: 10.1016/0306-4522(94)90022-1
52. Heinricher MM, Neubert MJ, Martenson ME, Gonçalves L. Prostaglandin E2 in the medial preoptic area produces hyperalgesia and activates pain-modulating circuitry in the rostral ventromedial medulla. Neuroscience. (2004) 128:389–98. doi: 10.1016/j.neuroscience.2004.06.050
53. Khasabov SG, Malecha P, Noack J, Tabakov J, Giesler GJ Jr, Simone DA. Hyperalgesia and sensitization of dorsal horn neurons following activation of NK-1 receptors in the rostral ventromedial medulla. J Neurophysiol. (2017) 118:2727–44. doi: 10.1152/jn.00478.2017
54. Cleary DR, Heinricher MM. Adaptations in responsiveness of brainstem pain-modulating neurons in acute compared with chronic inflammation. Pain. (2013) 154:845–55. doi: 10.1016/j.pain.2013.02.019
55. Heinricher MM, McGaraughty S. Analysis of excitatory amino acid transmission within the rostral ventromedial medulla: implications for circuitry. Pain. (1998) 75:247–55. doi: 10.1016/S0304-3959(97)00226-1
56. Cleary DR, Neubert MJ, Heinricher MM. Are opioid-sensitive neurons in the rostral ventromedial medulla inhibitory interneurons? Neuroscience. (2008) 151:564–71. doi: 10.1016/j.neuroscience.2007.10.023
57. Heinricher MM. Pain modulation and the transition from acute to chronic pain. Adv Exp Med Biol. (2016) 904:105–15. doi: 10.1007/978-94-017-7537-3_8
58. An X, Bandler R, Ongur D, Price JL. Prefrontal cortical projections to longitudinal columns in the midbrain periaqueductal gray in macaque monkeys. J Comp Neurol. (1998) 401:455–79.
59. Neafsey EJ, Hurley-Gius KM, Arvanitis D. The topographical organization of neurons in the rat medial frontal, insular and olfactory cortex projecting to the solitary nucleus, olfactory bulb, periaqueductal gray and superior colliculus. Brain Res. (1986) 377:261–70. doi: 10.1016/0006-8993(86)90867-X
60. Jasmin L, Burkey AR, Granato A, Ohara PT. Rostral agranular insular cortex and pain areas of the central nervous system: a tract-tracing study in the rat. J Comp Neurol. (2004) 468:425–40. doi: 10.1002/cne.10978
61. Bandler R, Price JL, Keay KA. Brain mediation of active and passive emotional coping. Prog Brain Res. (2000) 122:333–49. doi: 10.1016/S0079-6123(08)62149-4
62. Fontes MAP, Xavier CH, de Menezes RCA, DiMicco JA. The dorsomedial hypothalamus and the central pathways involved in the cardiovascular response to emotional stress. Neuroscience. (2011) 184:64–74. doi: 10.1016/j.neuroscience.2011.03.018
63. Eippert F, Bingel U, Schoell ED, Yacubian J, Klinger R, Lorenz J, et al. Activation of the opioidergic descending pain control system underlies placebo analgesia. Neuron. (2009) 63:533–43. doi: 10.1016/j.neuron.2009.07.014
64. Crawford LS, Mills EP, Hanson T, Macey PM, Glarin R, Macefield VG, et al. Brainstem mechanisms of pain modulation: a within-subjects 7t fMRI study of placebo analgesic and nocebo hyperalgesic responses. J Neurosci. (2021) 41:9794–806. doi: 10.1523/JNEUROSCI.0806-21.2021
65. Oliva V, Hartley-Davies R, Moran R, Pickering AE, Brooks JC. Simultaneous brain, brainstem, and spinal cord pharmacological-fMRI reveals involvement of an endogenous opioid network in attentional analgesia. Elife. (2022) 11:e71877. doi: 10.7554/eLife.71877
66. Morgan MM, Whittier KL, Hegarty DM, Aicher SA. Periaqueductal gray neurons project to spinally projecting GABAergic neurons in the rostral ventromedial medulla. Pain. (2008) 140:376–86. doi: 10.1016/j.pain.2008.09.009
67. Helmstetter FJ, Tershner SA, Poore LH, Bellgowan PS. Antinociception following opioid stimulation of the basolateral amygdala is expressed through the periaqueductal gray and rostral ventromedial medulla. Brain Res. (1998) 779:104–18. doi: 10.1016/S0006-8993(97)01104-9
68. McGaraughty S, Heinricher MM. Microinjection of morphine into various amygdaloid nuclei differentially affects nociceptive responsiveness and RVM neuronal activity. Pain. (2002) 96:153–62. doi: 10.1016/S0304-3959(01)00440-7
69. DiMicco JA, Samuels BC, Zaretskaia MV, Zaretsky DV. The Dorsomedial hypothalamus and the response to stress: part renaissance, part revolution. Pharmacol Biochem Behav. (2002) 71:469–80. doi: 10.1016/S0091-3057(01)00689-X
70. Furlong T, Carrive P. Neurotoxic lesions centered on the perifornical hypothalamus abolish the cardiovascular and behavioral responses of conditioned fear to context but not of restraint. Brain Res. (2007) 1128:107–19. doi: 10.1016/j.brainres.2006.10.058
71. McDougall SJ, Widdop RE, Lawrence AJ. Medial prefrontal cortical integration of psychological stress in rats. Eur J Neurosci. (2004) 20:2430–40. doi: 10.1111/j.1460-9568.2004.03707.x
72. Samuels BC, Zaretsky DV, DiMicco JA. Tachycardia evoked by disinhibition of the dorsomedial hypothalamus in rats is mediated through medullary raphe. J Physiol. (2002) 538:941–6. doi: 10.1113/jphysiol.2001.013302
73. Johnson PL, Truitt WA, Fitz SD, Lowry CA, Shekhar A. Neural pathways underlying lactate-induced panic. Neuropsychopharmacology. (2008) 33:2093–107. doi: 10.1038/sj.npp.1301621
74. Sarkar S, Zaretskaia MV, Zaretsky DV, Moreno M, DiMicco JA. Stress- and lipopolysaccharide-induced c-fos expression and nnos in hypothalamic neurons projecting to medullary raphe in rats: a triple immunofluorescent labeling study. Eur J Neurosci. (2007) 26:2228–38. doi: 10.1111/j.1460-9568.2007.05843.x
75. Foo H, Mason P. Sensory suppression during feeding. Proc Natl Acad Sci USA. (2005) 102:16865–9. doi: 10.1073/pnas.0506226102
76. Mooney ER, Davies AJ, Pickering AE. Sweet taste does not modulate pain perception in adult humans. Wellcome Open Res. (2020) 5:43. doi: 10.12688/wellcomeopenres.15726.1
77. Alhadeff AL, Su Z, Hernandez E, Klima ML, Phillips SZ, Holland RA, et al. A neural circuit for the suppression of pain by a competing need state. Cell. (2018) 173:140–52. doi: 10.1016/j.cell.2018.02.057
78. Carlson JD, Maire JJ, Martenson ME, Heinricher MM. Sensitization of pain-modulating neurons in the rostral ventromedial medulla after peripheral nerve injury. J Neurosci. (2007) 27:13222–31. doi: 10.1523/JNEUROSCI.3715-07.2007
79. Hamity MV, White SR, Hammond DL. Effects of neurokinin-1 receptor agonism and antagonism in the rostral ventromedial medulla of rats with acute or persistent inflammatory nociception. Neuroscience. (2010) 165:902–13. doi: 10.1016/j.neuroscience.2009.10.064
80. Khasabov SG, Wang JC, Simone DA, Strichartz GR. A role for neurokinin-1 receptor neurons in the rostral ventromedial medulla in the development of chronic postthoracotomy pain. Pain. (2017) 158:1332–41. doi: 10.1097/j.pain.0000000000000919
81. Todd AJ, McGill MM, Shehab SA. Neurokinin 1 receptor expression by neurons in laminae i, iii and iv of the rat spinal dorsal horn that project to the brainstem. Eur J Neurosci. (2000) 12:689–700. doi: 10.1046/j.1460-9568.2000.00950.x
82. Craig AD. Distribution of brainstem projections from spinal lamina I neurons in the cat and the monkey. J Comp Neurol. (1995) 361:225–48. doi: 10.1002/cne.903610204
83. Feil K, Herbert H. Topographic organization of spinal and trigeminal somatosensory pathways to the rat parabrachial and kölliker-fuse nuclei. J Comp Neurol. (1995) 353:506–28. doi: 10.1002/cne.903530404
84. Chiang MC, Nguyen EK, Canto-Bustos M, Papale AE, Oswald AM, Ross SE. Divergent neural pathways emanating from the lateral parabrachial nucleus mediate distinct components of the pain response. Neuron (2020) 106:927–39.e5. doi: 10.1016/j.neuron.2020.03.014
85. Gauriau C, Bernard JF. Pain pathways and parabrachial circuits in the rat. Exp Physiol. (2002) 87:251–8. doi: 10.1113/eph8702357
86. Lapirot O, Chebbi R, Monconduit L, Artola A, Dallel R, Luccarini P. NK1 Receptor-expressing spinoparabrachial neurons trigger diffuse noxious inhibitory controls through lateral parabrachial activation in the male rat. Pain. (2009) 142:245–54. doi: 10.1016/j.pain.2009.01.015
87. Roeder Z, Chen Q, Davis S, Carlson JD, Tupone D, Heinricher MM. The Parabrachial complex links pain transmission to descending pain modulation. Pain. (2016) 157:2697–708. doi: 10.1097/j.pain.0000000000000688
88. Chen Q, Roeder Z, Li MH, Zhang Y, Ingram SL, Heinricher MM. Optogenetic evidence for a direct circuit linking nociceptive transmission through the parabrachial complex with pain-modulating neurons of the rostral ventromedial medulla (RVM). eNeuro (2017) 4:1–16. doi: 10.1523/ENEURO.0202-17.2017
89. Chen Q, Heinricher MM. Plasticity in the link between pain-transmitting and pain-modulating systems in acute and persistent inflammation. J Neurosci. (2019) 39:2065–79. doi: 10.1523/JNEUROSCI.2552-18.2019
90. Chaouch A, Menetrey D, Binder D, Besson JM. Neurons at the origin of the medial component of the bulbopontine spinoreticular tract in the rat: an anatomical study using horseradish peroxidase retrograde transport. J Comp Neurol. (1983) 214:309–20. doi: 10.1002/cne.902140308
91. Sugiyo S, Takemura M, Dubner R, Ren K. Trigeminal transition zone/rostral ventromedial medulla connections and facilitation of orofacial hyperalgesia after masseter inflammation in rats. J Comp Neurol. (2005) 493:510–23. doi: 10.1002/cne.20797
92. Bernard JF, Besson JM. The Spino(Trigemino)pontoamygdaloid pathway: electrophysiological evidence for an involvement in pain processes. J Neurophysiol. (1990) 63:473–90. doi: 10.1152/jn.1990.63.3.473
93. Clarke RW, Morgan MM, Heinricher MM. Identification of nocifensor reflex-related neurons in the rostroventromedial medulla of decerebrated rats. Brain Res. (1994) 636:169–74. doi: 10.1016/0006-8993(94)90195-3
94. Simonnet G, Laboureyras E. Hyperalgesia modulation and chronic pain after surgery. In: Wilder-Smith O, Arendt-Nielsen L, Yarnitsky D, Vissers KCP, editors. Post-Operative Pain: Science and Clinical Practice. Philadelphia, USA: IASP Press (2015). p. 175–90.
95. Marvizon JC, Walwyn W, Minasyan A, Chen W, Taylor BK. Latent sensitization: a model for stress-sensitive chronic pain. Curr Protoc Neurosci (2015) 71:9.50.1–14. doi: 10.1002/0471142301.ns0950s71
96. Chen W, Tache Y, Marvizon JC. Corticotropin-releasing factor in the brain and blocking spinal descending signals induce hyperalgesia in the latent sensitization model of chronic pain. Neuroscience. (2018) 381:149–58. doi: 10.1016/j.neuroscience.2018.03.024
Keywords: pain-modulation, descending control, brainstem, analgesia, hyperalgesia, rostral ventromedial medulla, RVM
Citation: Chen Q and Heinricher MM (2022) Shifting the Balance: How Top-Down and Bottom-Up Input Modulate Pain via the Rostral Ventromedial Medulla. Front. Pain Res. 3:932476. doi: 10.3389/fpain.2022.932476
Received: 29 April 2022; Accepted: 23 May 2022;
Published: 28 June 2022.
Edited by:
Kenichiro Hayashida, Shin Nippon Biomedical Laboratories Ltd., JapanReviewed by:
Norikazu Kiguchi, Wakayama Medical University, JapanAntti Pertovaara, University of Helsinki, Finland
Copyright © 2022 Chen and Heinricher. This is an open-access article distributed under the terms of the Creative Commons Attribution License (CC BY). The use, distribution or reproduction in other forums is permitted, provided the original author(s) and the copyright owner(s) are credited and that the original publication in this journal is cited, in accordance with accepted academic practice. No use, distribution or reproduction is permitted which does not comply with these terms.
*Correspondence: Mary M. Heinricher, aGVpbnJpY20mI3gwMDA0MDtvaHN1LmVkdQ==
†ORCID: Qiliang Chen orcid.org/0000-0002-9588-1423