- 1Division of Clinical Neuroscience, School of Medicine, University of Nottingham, Nottingham, United Kingdom
- 2Sir Peter Mansfield Imaging Centre, School of Medicine, University of Nottingham, Nottingham, United Kingdom
- 3National Institute for Health Research (NIHR), Nottingham Biomedical Research Centre, Queens Medical Center, Nottingham, United Kingdom
- 4Versus Arthritis Pain Centre, University of Nottingham, Nottingham, United Kingdom
- 5School of Psychology, University of Nottingham, Nottingham, United Kingdom
The ability of non-invasive brain stimulation to induce neuroplasticity and cause long-lasting functional changes is of considerable interest for the reversal of chronic pain and disability. Stimulation of the primary motor cortex (M1) has provided some of the most encouraging after-effects for therapeutic purposes, but little is known about its underlying mechanisms. In this study we combined transcranial Direct Current Stimulation (tDCS) and fMRI to measure changes in task-specific activity and interregional functional connectivity between M1 and the whole brain. Using a randomized counterbalanced sham-controlled design, we applied anodal and cathodal tDCS stimulation over the left M1. In agreement with previous studies, we demonstrate that tDCS applied to the target region induces task-specific facilitation of local brain activity after anodal tDCS, with the stimulation effects having a negative relationship to the resting motor threshold. Beyond the local effects, tDCS also induced changes in multiple downstream regions distinct from the motor system that may be important for therapeutic efficacy, including the operculo-insular and cingulate cortex. These results offer opportunities to improve outcomes of tDCS for the individual patient based on the degree of presumed neuroplasticity. Further research is still warranted to address the optimal stimulation targets and parameters for those with disease-specific symptoms of chronic pain.
Introduction
In recent years there has been a move towards utilizing non-invasive brain stimulation (NIBS) and neuromodulation in the management of disability and chronic neurological diseases (1, 2). Of these, transcranial Direct Current Stimulation (tDCS) is a promising technique as it can change brain activity in a safe and reversable manner (3, 4). tDCS can be used independently or concomitantly with other treatments, thus offering more flexible therapeutic control to patients and physicians (5).
Evidence-based guidelines on the therapeutic use of tDCS in certain types of pain disorders have been characterized by stimulation of the primary motor cortex (M1) (6, 7). Additionally, M1-tDCS has shown promise for motor rehabilitation therapy (8). Alongside these clinical studies, detailed experimental work has been performed to assess the safety and mechanisms underlying the observed effects of M1 stimulation under a wide range of conditions (9, 10). Current available evidence suggests that the acute effects of tDCS are driven by a shift in resting neuronal membrane potential, which depends on the polarity of the electric current relative to the orientation of cortical columns, while the after-effects involve synaptic plasticity (11–13). Studies exploring the polarity-specific effects of tDCS suggest that anodal stimulation can increase cortical excitability, while cathodal stimulation leads to a decrease in excitability within the cortex (14, 15).
Despite efforts to characterize tDCS-induced effects at the neuronal level, the underlying mechanisms driving the induction and expression of cortical plasticity in humans remains largely unknown. Experimental work suggests that tDCS can interact with various neurotransmitters in the brain (16–21), and may trigger changes in brain-derived neurotrophic factor (BDNF) (22). Beyond the local effects, tDCS can alter neuronal network physiology with significant effects on brain activity in remote interconnected cortical and subcortical areas (23–26). It has been proposed that such modifications in connectivity and synchronization are the result of changes in the fidelity of signal transmission by neurons (27). However, it is still not clear to what extent M1 stimulation modulates brain activity beyond the motor system.
In order to investigate the effects on neural networks, brain stimulation has been combined with neuroimaging techniques (28, 29). Using fMRI, widespread changes in functional activity and connectivity have been reported as a result of M1-tDCS. This includes evidence of modified coupling within motor networks due to cathodal and anodal stimulation (21, 30, 31), and reduced long-range connectivity due to anodal stimulation (25, 32, 33). Further studies combining MRS and fMRI approaches have found an inverse relationship between network strength and local inhibitory tone within M1 (21, 34, 35). These results clearly demonstrate that local application of tDCS can have wide-ranging neurobiological effects. However, currently the use of tDCS to modulate pain is limited by an incomplete understanding of the exact mechanisms by which tDCS affects brain function.
In the present study, we aim to establish the feasibility and rationale for use of tDCS to modulate clinically relevant brain systems beyond the motor network suitable for targeting pain. We combined tDCS and fMRI to measure changes in task-specific activity and interregional functional connectivity between the primary motor cortex (M1) and the whole brain. In a randomized counterbalanced sham-controlled design, we applied anodal tDCS (atDCS) and cathodal tDCS (ctDCS) stimulation over the left M1. We chose a well-established electrode montage, which has been reliability shown to modulate plasticity in both experimental and clinical settings (36). The purpose was to functionally characterize the after-effects of tDCS and determine the spatial extent of changes in neural activity following motor cortex stimulation.
Materials and methods
Participants
Twenty-seven healthy volunteers participated in this study (mean age, 23.3 ± 2.5 years; age range: 19–30 years, 10 males). Subject were randomized according to three stimulus types: anodal (N = 9, 5 males), cathodal (N = 9, 2 males), and sham (N = 9, 3 males). All participants were right handed assessed by the Edinburgh Handedness Inventory (37). We obtained written informed consent from all participants prior to the experiment. The work was approved by local research ethics committees at the University of Nottingham.
Experimental design and procedures
Subjects were assigned to the stimulus type of tDCS in a randomized, counterbalanced design. A between subject design was used to ensure all subjects were naïve to the tDCS stimulation. fMRI and resting motor thresholds (RMTs) were assessed before and after tDCS stimulation (pre/post-stimulation) (Figure 1A). The fMRI session consisted of resting-state and hand motor task. In the task condition, participants performed a simple hand clenching task. The hand clenching task required participant to clench and unclench continuously with their right hand when they saw the word “Fist” on the screen. We used an fMRI block design (total 10mins) consisting of a fist condition (30s) and a fixation (15s) (Figure 1B).
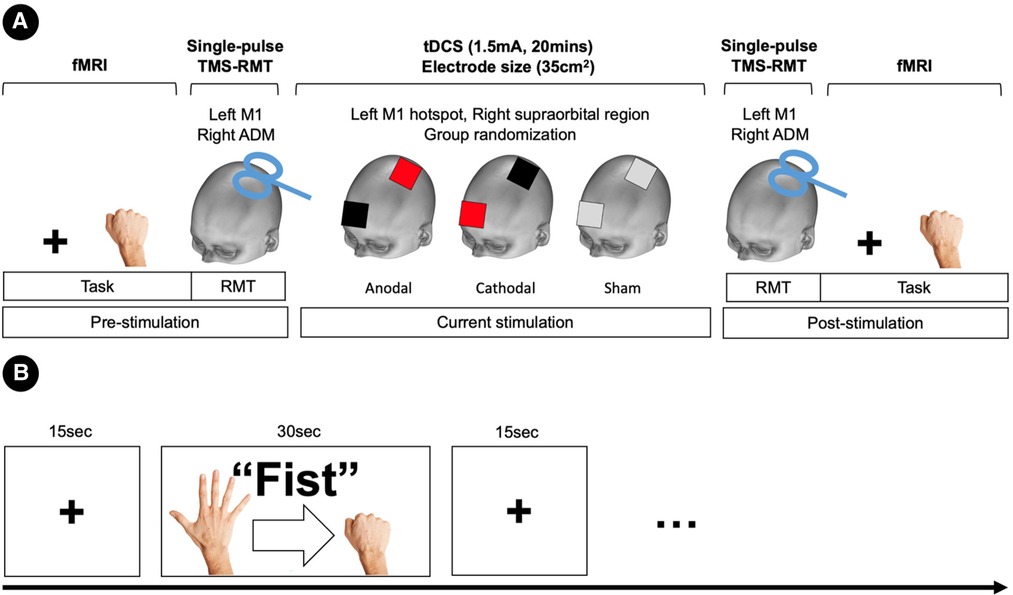
Figure 1. Experimental deign and procedures. (A) Task-fMRI and resting motor thresholds (RMTs) were assessed before and after tDCS stimulation (pre/post-stimulation). RMTs were elicited in the left primary motor cortex (M1) with single-pulse TMS of the abductor digiti minimi (ADM) muscle of the right hand. Subjects were assigned to a stimulus type in a randomized, counterbalanced design. Anodal stimulation is represented in red, cathodal stimulation in black, and sham in grey. (B) Block fMRI design for the hand motor task.
Magnetic resonance imaging
Functional MR images were acquired at the Sir Peter Mansfield Imaging Centre, University of Nottingham, using a Philips Achieva 3.0-Tesla scanner equipped with an eight-channel SENSE head coil. Structural images were acquired using 3D MPRAGE sequence (1 mm3 isotropic resolution, repetition time (TR)/echo time (TE) = 8.278/2.3 ms, flip angle = 8°, 160 slices, FOV = 256 mm × 256 mm). For functional images, echo-planar imaging (EPI) sequence (TR/TE = 2,000/35 ms, flip angle 90°, 32 slices, FOV = 240 mm × 240 mm, voxel size = 3 mm × 3 mm × 3 mm) was used for the task and resting conditions.
Transcranial direct current stimulation
Transcranial direct current stimulation (tDCS) was delivered through a pair of sponge electrodes (7 cm × 5 cm, 35 cm2). The electrodes were connected to a battery-driven constant-current stimulator (NeuroConn, Germany). In the experiment, the target electrode (anodal and cathodal) was placed over the hand motor hotspot within the left primary motor cortex (the right hand area) as identified by transcranial magnetic stimulation (TMS), and the other electrode was positioned over the right supraorbital region as a reference electrode. Current strength was 1.5 mA and the stimulation was delivered for 20 min (10 s for ramp up and down). For sham stimulation, the current (1.5 mA) was ramped up over 10 s then immediately turned off. tDCS was delivered the outside of the scanner, and there was a 10 min period between the tDCS and fMRI sessions (pre- and post-stimulation).
Resting motor thresholds
To assess the tDCS-induced changes of an individual's RMT, we used TMS before and after the current stimulation. The TMS machine (Magstim Rapid2 stimulator, UK) was delivered using a figure-of-eight coil (70 mm outer wing diameter) and applied over the hand motor hotspot in the left M1. The hand motor hotspot was determined as the site elicited a muscle twitch of the right hand. RMTs were elicited in the left primary motor cortex (M1) with single-pulse TMS of the abductor digiti minimi (ADM) muscle of the right hand. The TMS coil was oriented perpendicular to the central sulcus at a 45° angle from the mid-sagittal line approximately. RMT was defined as the minimum stimulator output that induces an observable muscle twitch at the right hand for five of ten TMS pulses. We repeated the RMT three times and used the average value as a measure of corticospinal excitability.
fMRI data analysis
Functional images were preprocessed and analysed with the Statistical Parametric Mapping software package (SPM12, Wellcome Department of Imaging Neuroscience, UK, http://www.fil.ion.ucl.ac.uk/spm/software/spm12/). Preprocessing included realignment, coregistration, normalization to the Montreal Neurological Institute (MNI) space, and spatial smoothing using a Gaussian kernel 8 mm full width half maximum (FWHM). For the task fMRI, a general linear model (GLM) was used to compute individual contrasts and a design matrix was comprised of the task condition and fMRI sessions (pre and post) at an individual level. Head movement parameters were included as regressors. For each participant, two contrasts were estimated (pre: fist > fixation and post: fist > fixation). In the random effect analysis, two-factorial ANOVA with the stimulation (anodal, cathodal, and sham) and session (pre and post) was performed. Statistical significance was set at p < 0.005 at a voxel level and p < 0.05, ks > 30 at a cluster level with false-discovery rate (FDR) correction.
Functional connectivity analysis
We used CONN toolbox (CONN 20.b, http://web.mit.edu/swg/software.html) to estimate functional connectivity (FC) from a seed area to the whole brain. Prepocessed images were entered into the toolbox. Denoising using the component-based noise correction method (CompCor) implemented in the CONN toolbox (38) was conducted to remove white matter, cerebrospinal fluid, and physiological noise as well as head motion related artifacts. The data was also detrended, despiked, and filtered with a band pass (0.001 < f <0.008 Hz) to remove slow fluctuating signal such as scanner drift. Head movements were taken into account and motion parameters and their first-order temporal derivatives were regressed out. The onset and duration of the experimental condition (fist and fixation) were entered to the toolbox to generate FC for each condition in the fMRI task.
Generalized psychophysiological interaction analysis
In order to examine the interaction effects of tDCS from the left M1 to other brain regions, we used a generalised psychophysiological interaction (gPPI) analysis implemented in CONN toolbox (39). PPI analysis can be used to identify regions that differ in connectivity by context or condition in fMRI studies, which makes inferences of context-dependent functional integration between a seed and other regions (40). Compared to the standard PPI analysis, the gPPI reduces both false negatives and false positives, especially in experiments involving more than two conditions (39, 41).
To perform the gPPI, we extracted an averaged blood oxygenation level-dependent (BOLD) time-course from a seed region as the physiological regressor. We generated a PPI regressor for each condition as the product between the psychological (the vector of the condition) and physiological regressors. The physiological and PPI regressors were convolved with the hemodynamic response function. We compared voxel-wise how strongly the time-course of a seed/ROI is correlated with the PPI regressors of another. At a group-level, we performed a random-effects analysis to measure differences in FC between the active stimulation (anodal and cathodal) and sham stimulation after the stimulation. For the seed to whole brain connectivity, statistical significance was set at p < 0.01 at the voxel level with FDR-corrected cluster threshold of p < 0.05, ks > 30 (42).
Results
RMT results
A planned comparison of RMTs was conducted within each stimulation group. Cathodal stimulation showed a minor increase in RMT after stimulation (pre: 63.12% ± 3.06, post: 65.74 ± 3.72), however this effect was not significant. Anodal stimulation (pre: 61.07% ± 3.59, post: 60.00 ± 3.60) and sham (pre: 62.48% ± 2.91, post: 63.55 ± 2.76) also did not show any significant changes in the RMT. At the group-level, there was no significant effect of stimulus type (Z < −1.12, ps > 0.15). The results of the RMT analysis and individual values are displayed in Figure 2.
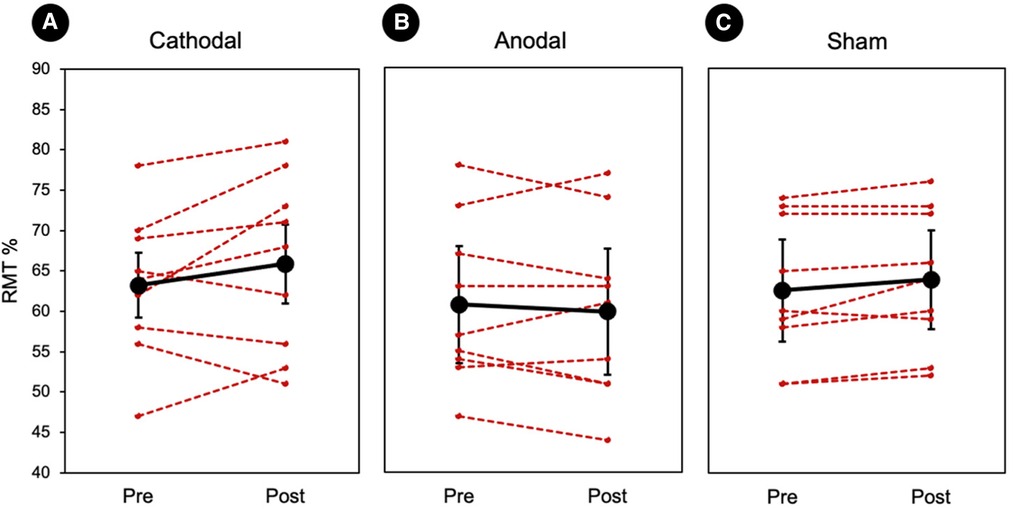
Figure 2. Individual resting motor thresholds. (A–C) Three randomized tDCS groups. Red represents each individual and the black represents the group average. There was no significant difference between the session (pre vs. post) or groups (cathodal vs. anodal vs. sham). Error bars represents standard errors.
Whole-brain GLM results
To examine the effect of task (fist > fixation) prior to the stimulation, we performed a conjunction analysis across the stimulation groups (anodal, cathodal, and sham). The hand motor task induced significant activations in the left M1 and supplementary motor cortex (Figure 3A). In addition, the main effect of stimulation revealed significant activations in brain areas, including the bilateral insula, operculum, middle cingulate cortex, thalamus, and precuneus (Figure 3B). To examine the polarity-specific effects, we compared the active tDCS groups with the sham group in the post-stimulation session. The anodal group showed increased activation in the insula, operculum, thalamus, and cuneus (Figure 3C). The cathodal group revealed a similar pattern of activity, but only the right insula showed a significant activation compared to sham (Figure 3D). In comparison of active stimulation groups, the anodal group showed increased activation in the visual cortex, whereas the cathodal group did not show any significant activation (Figure 3E). There were no significant effects in the comparisons between pre- and post-stimulation for all groups. In addition, there were no significant effects pre-stimulation between the groups. These results are summarized in Table 1.
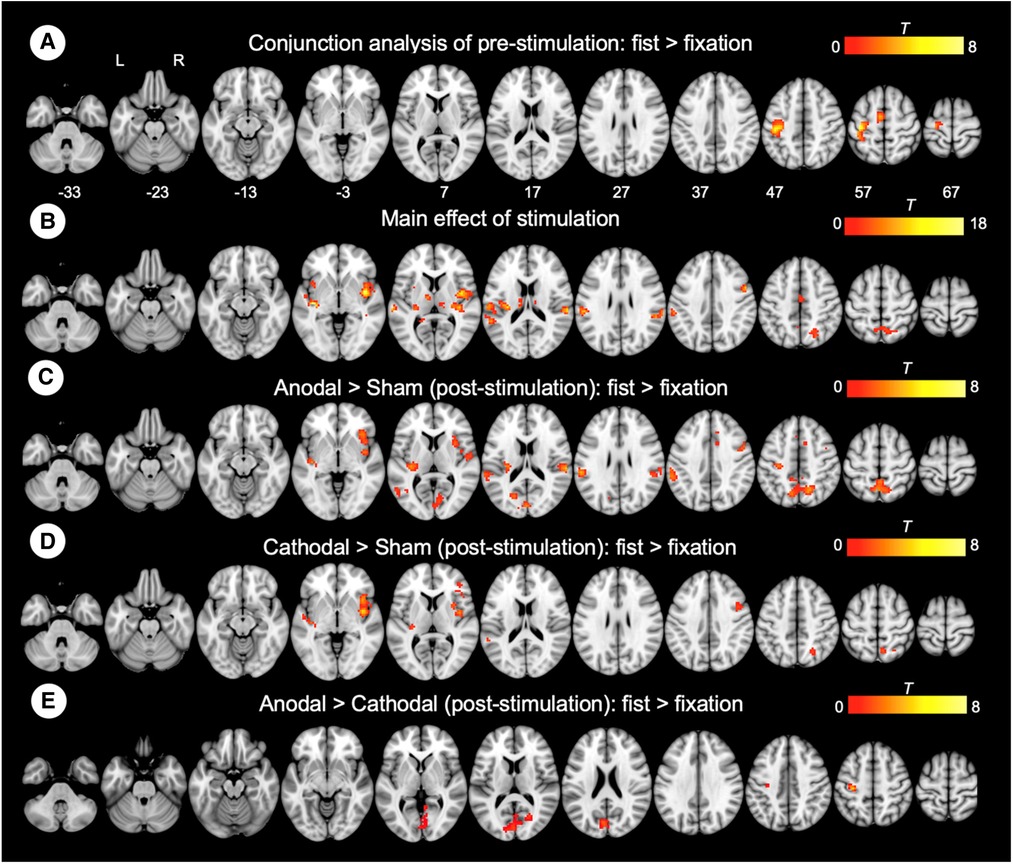
Figure 3. Whole-brain GLM results. (A) Conjunction analysis for the pre-stimulation task response across the groups. (B) Main effect of stimulation. (C) Comparison between anodal and sham group. (D) Comparison between cathodal and sham group. (E) Comparison between anodal and cathodal group. R (right) and L (left) indicate image orientations for slices in axial planes. Reference positions are in MNI coordinates. All images are displayed with a statistical significance of p < 0.005 at the voxel level and p < 0.05 (ks > 30) at a cluster level with false-discovery rate (FDR) correction.
ROI results
To assess the functional coupling to the site where non-invasive brain stimulation was effective at inducing neuroplasticity, a seed region was defined in the left M1 (target region, MNI: −39, −24, 54) based on the GLM task results (Figure 4A). Subsequent analyses of the regional activity changes (post—pre) were conducted to examine the effect of stimulation (Figure 4B). We found that anodal stimulation significantly increased regional activity in the left M1 (one-sample Wilcoxon Signed Rank Test, p = 0.025) whereas cathodal stimulation decreased activity (p = 0.07). We also assessed the stimulation effect on RMT by performing a correlation analysis between RMT changes (post—pre) and M1 activity changes (post—pre) (Figure 4C). We found a significant negative correlation (r = −0.40, p = 0.038). Individuals with increased RMT after stimulation showed decreased regional activity in the left M1 (target region).
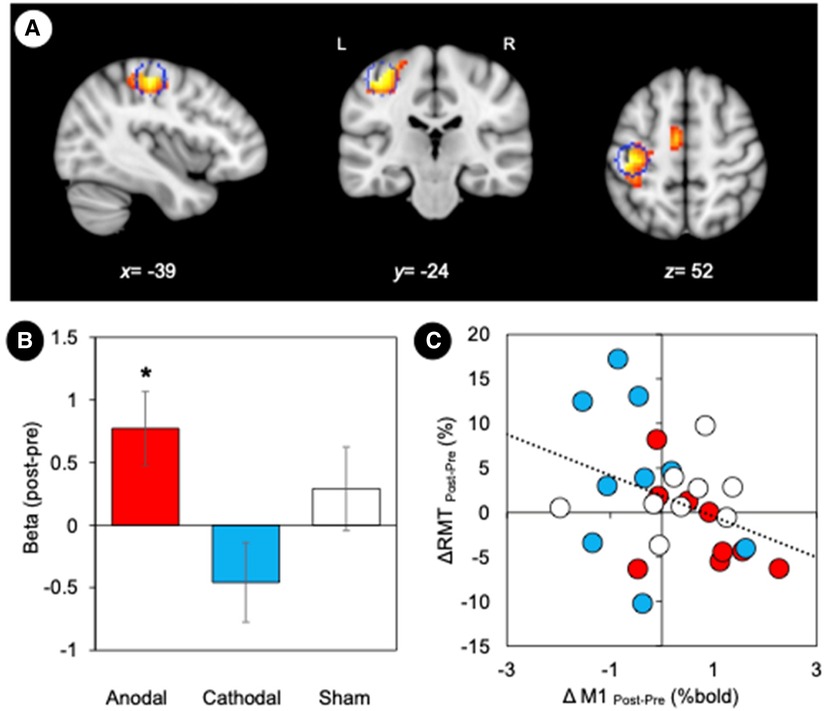
Figure 4. Motor cortex plasticity. (A) M1 location defined by the maximally activated voxel in the contralateral (left) hemisphere. Statistical maps based on the conjunction map of the responses elicited by the hand motor task (pre-stimulation). The coordinates of the group maxima are reported on the structural images from the MNI template, and a sphere of 6 mm radius marked for reference. Coordinates of the group maxima of activation are reported in Table 1. All images are displayed with a statistical significance of p < 0.005 at the voxel level and p < 0.05 (ks > 30) at a cluster level with false-discovery rate (FDR) correction. (B) GLM parameter estimates (weighted beta values) representing the magnitude of M1 responses to tDCS stimulation. Error bars represent standard error. *p < 0.05. (C) Relationship between changes in RMT and M1 activity. We found a significant negative correlation between RMT changes (post—pre) and M1 activity changes (post—pre) (r = −0.40, p = 0.038).
Whole-brain gPPI connectivity results
Connectivity changes during the hand motor task were estimated with gPPI. This allowed us to directly contrast functional connectivity during fist clenching with functional connectivity in the fixation (rest) condition. The gPPI analysis with the left M1 as a seed region revealed that anodal stimulation enhanced connectivity with middle/posterior cingulate cortex relative to sham stimulation (Figure 5A), whereas cathodal stimulation strengthened connectivity with insula and operculum (Figure 5B). The anodal stimulation significantly increased connectivity between the M1 and anterior and middle cingulate cortex compared to the cathodal stimulation (Figure 5C). These results are summarized in Table 2.
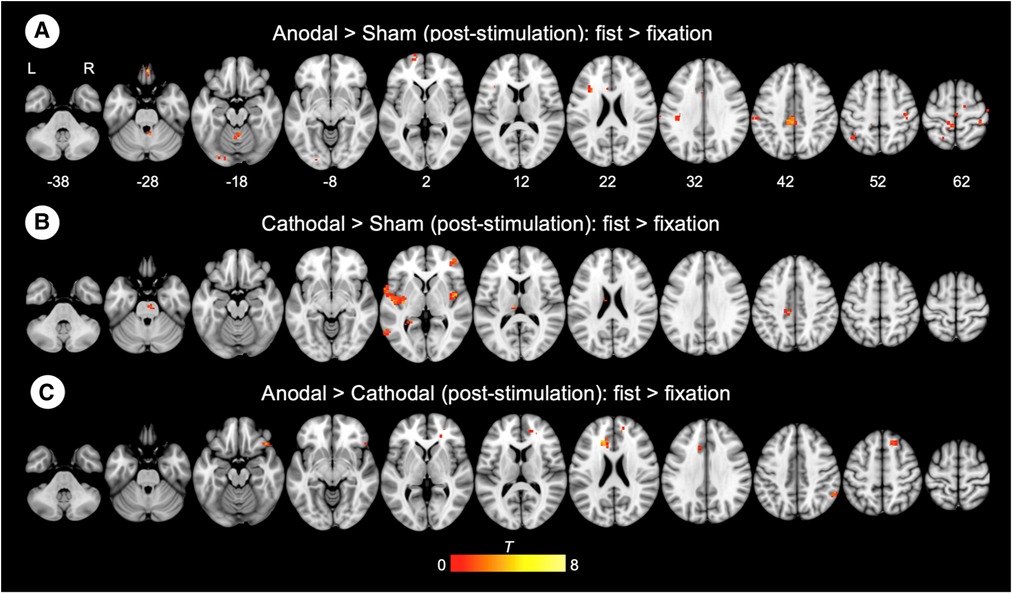
Figure 5. Whole-brain gPPI results for the left M1 during hand motor task. (A, B, C) Generalized psychophysiological interaction (gPPI) analysis: interaction of stimulation type x task condition (fist > fixation) on M1 connectivity. R (right) and L (left) indicate image orientations for slices in axial planes. Reference positions are in MNI coordinates. All images are displayed with a statistical significance of p < 0.005 at the voxel level and p < 0.05 (ks > 30) at a cluster level with false-discovery rate (FDR) correction.
Discussion
Neuromodulation with tDCS is an emerging tool for the treatment of chronic pain. However, issues relating to poor outcomes and replicability have compromised this method (43). We considered the concept that a target regions connectivity profile is fundamental to the therapeutic effects of modulation (44). We combined tDCS and fMRI to measure changes in task-specific activity and interregional functional connectivity between the primary motor cortex (M1) and the whole brain. In the following discussion, we consider the potential mechanisms underlying the observed neuroplastic after-effects on excitability induced by tDCS, and its potential relevance for the therapeutic effects of modulating disease-specific symptoms in chronic pain.
Modulation of task-dependent activity
tDCS has been shown to induce long-lasting and polarity-specific changes in motor cortex excitability (13, 45, 46) that relates to modifications in synaptic efficacy and LTP/LDP-like plasticity (47, 48). We demonstrated that tDCS applied to M1 (the target region) induces task-specific facilitation of local brain activity after anodal tDCS, with the stimulation effects having a negative relationship to the RMT. Previous studies have shown that functional changes in movement-related activity are modulated by tDCS (31, 49, 50). For example, Stagg et al. (31) reported a significant increase of M1 activation after anodal tDCS, which is in agreement with the findings of this study. However, in our study, the increases in brain activity were more widespread and extended to include several regions that potentially belong to the same network, such as the insular and cingulate cortex. Although widespread cortical changes have been reported as a result of tDCS (31, 49, 50), it is still not clear to what extent M1 stimulation modulates task-related activity outside of the motor system. This is in part due to the experimental differences between studies, including the tDCS protocols, fMRI acquisition parameters, and the specifics of the task. However, to our knowledge this is the first study demonstrating the modulating effects of M1-tDCS on a functionally distinct set of brain regions that may contribute to a variety of complex functions relevant for targeting pain (51, 52).
Modulation of task-dependent functional connectivity
The versatility of fMRI affords the additional opportunity to understand how brain regions interact in a task-dependent manner. Context-dependent connectivity, or the connectivity during the hand motor task conditions (i.e., fist/fixation), was examined using the left M1 as a seed region and revealed that anodal stimulation enhanced connectivity with middle/posterior cingulate cortex relative to sham stimulation, whereas cathodal stimulation strengthened connectivity with the insula and operculum. A further comparison of the two active tDCS groups showed that the anterior and midcingulate cortex is most susceptible to the polarity-dependent effects of M1 stimulation. Previous studies have exclusively focused on the effects of tDCS-induced functional connectivity alterations independent of task-related activity and performance (25, 26, 32, 33). However, to date, there have been no studies examining the psychophysiological interactions of motor cortex tDCS on such networks. The remote impact of tDCS is consistent with previous work that used TMS to modulate effective connectivity between M1 and other remote brain areas relevant for pain processing and modulation (53). Based on these data, we speculate that both tDCS and TMS can extensively modulate distributed functional systems in the human brain (51, 52), and that the spreading effects are not confined to a single network of interest. It may be the case that functional activity and connectivity of other areas are related to the plasticity-inducing effects of M1 stimulation, albeit to a weaker degree than the significant findings in the present study.
Conclusion and future perspectives
tDCS of the primary motor cortex is promising treatment for several brain disorders including chronic, but its mechanism of action remains unclear (Gan et al., 2021). The findings presented here support the hypothesis that a target regions connectivity profile is relevant to the therapeutic effects of modulation (44). The downstream regions susceptible to tDCS manipulation include the operculo-insular and cingulate cortex, which have reciprocal interconnections relevant for supporting complex behavioral functions, such as pain, valence, and arousal. No measures of corticospinal excitability (i.e., resting motor threshold, RMT) could independently explain the observed changes in brain function. However, as with any exploratory investigation, there several limitations we must consider. The main issue of this study pertains to the small cohort of subjects used within the stimulation groups (54). The next step will be to repeat this type of investigation with a much larger cohort of subjects and select patient groups, especially those with pain conditions relevant for tDCS therapy. The lack of an explicit nociceptive stimulus or task means that caution must be applied when interpreting the selected brain areas as pain specific. Nevertheless, these results offer opportunities to improve outcomes of tDCS for the individual patient based on the degree of presumed neuroplasticity. Further research is still warranted to address the optimal stimulation target and parameters for those with disease-specific symptoms of chronic pain.
Data availability statement
The raw data supporting the conclusions of this article will be available on request to the corresponding author.
Ethics statement
The studies involving human participants were reviewed and approved by University of Nottingham REC. The patients/participants provided their written informed consent to participate in this study.
Author contributions
DH, SJ, JJ contributed to conception and design of the study. JJ organized the data. JJ performed the statistical analysis. DH and JJ wrote the first draft of the manuscript. All authors contributed to manuscript revision, read, and approved the submitted version.
Funding
This work was supported by the Medical Research Council, and NIHR Nottingham Biomedical Research Centre.
Acknowledgments
We would like to thank Richard Bowtell for assistance with the MRI protocol.
Author disclaimer
The views expressed are those of the authors and not necessarily those of the NHS, the NIHR, or the Department of Health.
Conflict of interest
The authors declare that the research was conducted in the absence of any commercial or financial relationships that could be construed as a potential conflict of interest.
Publisher's note
All claims expressed in this article are solely those of the authors and do not necessarily represent those of their affiliated organizations, or those of the publisher, the editors and the reviewers. Any product that may be evaluated in this article, or claim that may be made by its manufacturer, is not guaranteed or endorsed by the publisher.
References
1. Knotkova H, Hamani C, Sivanesan E, Le Beuffe MFE, Moon JY, Cohen SP, et al. Neuromodulation for chronic pain. Lancet. (2021) 397(10289):2111–24. doi: 10.1016/S0140-6736(21)00794-7
2. James ND, McMahon SB, Field-Fote EC, Bradbury EJ. Neuromodulation in the restoration of function after spinal cord injury. Lancet Neurol. (2018) 17(10):905–17. doi: 10.1016/S1474-4422(18)30287-4
3. Stagg CJ, Nitsche MA. Physiological basis of transcranial direct current stimulation. Neuroscientist. (2011) 17(1):37–53. doi: 10.1177/1073858410386614
4. Woods AJ, Antal A, Bikson M, Boggio PS, Brunoni AR, Celnik P, et al. A technical guide to tDCS, and related non-invasive brain stimulation tools. Clin Neurophysiol. (2016) 127(2):1031–48. doi: 10.1016/j.clinph.2015.11.012
5. Polania R, Nitsche MA, Ruff CC. Studying and modifying brain function with non-invasive brain stimulation. Nat Neurosci. (2018) 21(2):174–87. doi: 10.1038/s41593-017-0054-4
6. Cruccu G, Aziz TZ, Garcia-Larrea L, Hansson P, Jensen TS, Lefaucheur JP, et al. EFNS guidelines on neurostimulation therapy for neuropathic pain. Eur J Neurol. (2007) 14(9):952–70. doi: 10.1111/j.1468-1331.2007.01916.x
7. Cruccu G, Garcia-Larrea L, Hansson P, Keindl M, Lefaucheur JP, Paulus W, et al. EAN guidelines on central neurostimulation therapy in chronic pain conditions. Eur J Neurol. (2016) 23(10):1489–99. doi: 10.1111/ene.13103
8. Lefaucheur JP, Antal A, Ayache SS, Benninger DH, Brunelin J, Cogiamanian F, et al. Evidence-based guidelines on the therapeutic use of transcranial direct current stimulation (tDCS). Clin Neurophysiol. (2017) 128(1):56–92. doi: 10.1016/j.clinph.2016.10.087
9. Nitsche MA, Cohen LG, Wassermann EM, Priori A, Lang N, Antal A, et al. Transcranial direct current stimulation: state of the art 2008. Brain Stimul. (2008) 1(3):206–23. doi: 10.1016/j.brs.2008.06.004
10. Ziemann U, Paulus W, Nitsche MA, Pascual-Leone A, Byblow WD, Berardelli A, et al. Consensus: motor cortex plasticity protocols. Brain Stimul. (2008) 1(3):164–82. doi: 10.1016/j.brs.2008.06.006
11. Nitsche MA, Fricke K, Henschke U, Schlitterlau A, Liebetanz D, Lang N, et al. Pharmacological modulation of cortical excitability shifts induced by transcranial direct current stimulation in humans. J Physiol. (2003) 553(Pt 1):293–301. doi: 10.1113/jphysiol.2003.049916
12. Nitsche MA, Paulus W. Excitability changes induced in the human motor cortex by weak transcranial direct current stimulation. J Physiol. (2000) 527(Pt 3):633–9. doi: 10.1111/j.1469-7793.2000.t01-1-00633.x
13. Nitsche MA, Paulus W. Sustained excitability elevations induced by transcranial DC motor cortex stimulation in humans. Neurology. (2001) 57(10):1899–901. doi: 10.1212/WNL.57.10.1899
14. Batsikadze G, Moliadze V, Paulus W, Kuo MF, Nitsche MA. Partially non-linear stimulation intensity-dependent effects of direct current stimulation on motor cortex excitability in humans. J Physiol. (2013) 591(7):1987–2000. doi: 10.1113/jphysiol.2012.249730
15. Monte-Silva K, Kuo MF, Hessenthaler S, Fresnoza S, Liebetanz D, Paulus W, et al. Induction of late LTP-like plasticity in the human motor cortex by repeated non-invasive brain stimulation. Brain Stimul. (2013) 6(3):424–32. doi: 10.1016/j.brs.2012.04.011
16. Nitsche MA, Grundey J, Liebetanz D, Lang N, Tergau F, Paulus W. Catecholaminergic consolidation of motor cortical neuroplasticity in humans. Cereb Cortex. (2004) 14(11):1240–5. doi: 10.1093/cercor/bhh085
17. Nitsche MA, Jaussi W, Liebetanz D, Lang N, Tergau F, Paulus W. Consolidation of human motor cortical neuroplasticity by D-cycloserine. Neuropsychopharmacology. (2004) 29(8):1573–8. doi: 10.1038/sj.npp.1300517
18. Nitsche MA, Kuo MF, Karrasch R, Wachter B, Liebetanz D, Paulus W. Serotonin affects transcranial direct current-induced neuroplasticity in humans. Biol Psychiatry. (2009) 66(5):503–8. doi: 10.1016/j.biopsych.2009.03.022
19. Nitsche MA, Lampe C, Antal A, Liebetanz D, Lang N, Tergau F, et al. Dopaminergic modulation of long-lasting direct current-induced cortical excitability changes in the human motor cortex. Eur J Neurosci. (2006) 23(6):1651–7. doi: 10.1111/j.1460-9568.2006.04676.x
20. Nitsche MA, Liebetanz D, Schlitterlau A, Henschke U, Fricke K, Frommann K, et al. GABAergic modulation of DC stimulation-induced motor cortex excitability shifts in humans. Eur J Neurosci. (2004) 19(10):2720–6. doi: 10.1111/j.0953-816X.2004.03398.x
21. Bachtiar V, Near J, Johansen-Berg H, Stagg CJ. Modulation of GABA and resting state functional connectivity by transcranial direct current stimulation. Elife. (2015) 4:e08789. doi: 10.7554/eLife.08789
22. Fritsch B, Reis J, Martinowich K, Schambra HM, Ji Y, Cohen LG, et al. Direct current stimulation promotes BDNF-dependent synaptic plasticity: potential implications for motor learning. Neuron. (2010) 66(2):198–204. doi: 10.1016/j.neuron.2010.03.035
23. Polania R, Paulus W, Nitsche MA. Modulating cortico-striatal and thalamo-cortical functional connectivity with transcranial direct current stimulation. Hum Brain Mapp. (2012) 33(10):2499–508. doi: 10.1002/hbm.21380
24. Stagg CJ, Lin RL, Mezue M, Segerdahl A, Kong Y, Xie J, et al. Widespread modulation of cerebral perfusion induced during and after transcranial direct current stimulation applied to the left dorsolateral prefrontal cortex. J Neurosci. (2013) 33(28):11425–31. doi: 10.1523/JNEUROSCI.3887-12.2013
25. Polania R, Paulus W, Antal A, Nitsche MA. Introducing graph theory to track for neuroplastic alterations in the resting human brain: a transcranial direct current stimulation study. Neuroimage. (2011) 54(3):2287–96. doi: 10.1016/j.neuroimage.2010.09.085
26. Sehm B, Schafer A, Kipping J, Margulies D, Conde V, Taubert M, et al. Dynamic modulation of intrinsic functional connectivity by transcranial direct current stimulation. J Neurophysiol. (2012) 108(12):3253–63. doi: 10.1152/jn.00606.2012
27. Polania R, Paulus W, Nitsche MA. Reorganizing the intrinsic functional architecture of the human primary motor cortex during rest with non-invasive cortical stimulation. PLoS One. (2012) 7(1):e30971. doi: 10.1371/journal.pone.0030971
28. Venkatakrishnan A, Sandrini M. Combining transcranial direct current stimulation and neuroimaging: novel insights in understanding neuroplasticity. J Neurophysiol. (2012) 107(1):1–4. doi: 10.1152/jn.00557.2011
29. Siebner HR, Bergmann TO, Bestmann S, Massimini M, Johansen-Berg H, Mochizuki H, et al. Consensus paper: combining transcranial stimulation with neuroimaging. Brain Stimul. (2009) 2(2):58–80. doi: 10.1016/j.brs.2008.11.002
30. Amadi U, Ilie A, Johansen-Berg H, Stagg CJ. Polarity-specific effects of motor transcranial direct current stimulation on fMRI resting state networks. Neuroimage. (2014) 88:155–61. doi: 10.1016/j.neuroimage.2013.11.037
31. Stagg CJ, O'Shea J, Kincses ZT, Woolrich M, Matthews PM, Johansen-Berg H. Modulation of movement-associated cortical activation by transcranial direct current stimulation. Eur J Neurosci. (2009) 30(7):1412–23. doi: 10.1111/j.1460-9568.2009.06937.x
32. Alon G, Roys SR, Gullapalli RP, Greenspan JD. Non-invasive electrical stimulation of the brain (ESB) modifies the resting-state network connectivity of the primary motor cortex: a proof of concept fMRI study. Brain Res. (2011) 1403:37–44. doi: 10.1016/j.brainres.2011.06.013
33. Sehm B, Kipping J, Schafer A, Villringer A, Ragert P. A comparison between uni- and bilateral tDCS effects on functional connectivity of the human motor Cortex. Front Hum Neurosci. (2013) 7:183. doi: 10.3389/fnhum.2013.00183
34. Antonenko D, Schubert F, Bohm F, Ittermann B, Aydin S, Hayek D, et al. tDCS-induced modulation of GABA levels and resting-state functional connectivity in older adults. J Neurosci. (2017) 37(15):4065–73. doi: 10.1523/JNEUROSCI.0079-17.2017
35. Stagg CJ, Bachtiar V, Amadi U, Gudberg CA, Ilie AS, Sampaio-Baptista C, et al. Local GABA concentration is related to network-level resting functional connectivity. Elife. (2014) 3:e01465. doi: 10.7554/eLife.01465
36. Buch ER, Santarnecchi E, Antal A, Born J, Celnik PA, Classen J, et al. Effects of tDCS on motor learning and memory formation: a consensus and critical position paper. Clin Neurophysiol. (2017) 128(4):589–603. doi: 10.1016/j.clinph.2017.01.004
37. Oldfield RC. The assessment and analysis of handedness: the Edinburgh inventory. Neuropsychologia. (1971) 9(1):97–113. doi: 10.1016/0028-3932(71)90067-4
38. Behzadi Y, Restom K, Liau J, Liu TT. A component based noise correction method (CompCor) for BOLD and perfusion based fMRI. Neuroimage. (2007) 37(1):90–101. doi: 10.1016/j.neuroimage.2007.04.042
39. McLaren DG, Ries ML, Xu G, Johnson SC. A generalized form of context-dependent psychophysiological interactions (gPPI): a comparison to standard approaches. Neuroimage. (2012) 61(4):1277–86. doi: 10.1016/j.neuroimage.2012.03.068
40. Friston KJ, Buechel C, Fink GR, Morris J, Rolls E, Dolan RJ. Psychophysiological and modulatory interactions in neuroimaging. Neuroimage. (1997) 6(3):218–29. doi: 10.1006/nimg.1997.0291
41. Masson H L, Pillet I, Boets B, de Beeck H O. Task-dependent changes in functional connectivity during the observation of social and non-social touch interaction. Cortex. (2020) 125:73–89. doi: 10.1016/j.cortex.2019.12.011
42. O’Reilly JX, Woolrich MW, Behrens TE, Smith SM, Johansen-Berg H. Tools of the trade: psychophysiological interactions and functional connectivity. Soc Cogn Affect Neurosci. (2012) 7(5):604–9. doi: 10.1093/scan/nss055
43. Wiethoff S, Hamada M, Rothwell JC. Variability in response to transcranial direct current stimulation of the motor cortex. Brain Stimul. (2014) 7(3):468–75. doi: 10.1016/j.brs.2014.02.003
44. Fox MD, Buckner RL, Liu H, Chakravarty MM, Lozano AM, Pascual-Leone A. Resting-state networks link invasive and noninvasive brain stimulation across diverse psychiatric and neurological diseases. Proc Natl Acad Sci U S A. (2014) 111(41):E4367–75. doi: 10.1073/pnas.1405003111
45. Lang N, Nitsche MA, Dileone M, Mazzone P, De Andres-Ares J, Diaz-Jara L, et al. Transcranial direct current stimulation effects on I-wave activity in humans. J Neurophysiol. (2011) 105(6):2802–10. doi: 10.1152/jn.00617.2010
46. Lang N, Nitsche MA, Paulus W, Rothwell JC, Lemon RN. Effects of transcranial direct current stimulation over the human motor cortex on corticospinal and transcallosal excitability. Exp Brain Res. (2004) 156(4):439–43. doi: 10.1007/s00221-003-1800-2
47. Islam N, Aftabuddin M, Moriwaki A, Hattori Y, Hori Y. Increase in the calcium level following anodal polarization in the rat brain. Brain Res. (1995) 684(2):206–8. doi: 10.1016/0006-8993(95)00434-R
48. Liebetanz D, Nitsche MA, Tergau F, Paulus W. Pharmacological approach to the mechanisms of transcranial DC-stimulation-induced after-effects of human motor cortex excitability. Brain. (2002) 125(Pt 10):2238–47. doi: 10.1093/brain/awf238
49. Baudewig J, Nitsche MA, Paulus W, Frahm J. Regional modulation of BOLD MRI responses to human sensorimotor activation by transcranial direct current stimulation. Magn Reson Med. (2001) 45(2):196–201. doi: 10.1002/1522-2594(200102)45:2%3C196::AID-MRM1026%3E3.0.CO;2-1
50. Lang N, Siebner HR, Ward NS, Lee L, Nitsche MA, Paulus W, et al. How does transcranial DC stimulation of the primary motor cortex alter regional neuronal activity in the human brain? Eur J Neurosci. (2005) 22(2):495–504. doi: 10.1111/j.1460-9568.2005.04233.x
51. Coghill RC. The distributed nociceptive system: a framework for understanding pain. Trends Neurosci. (2020) 43(10):780–94. doi: 10.1016/j.tins.2020.07.004
52. Mano H, Seymour B. Pain: a distributed brain information network? PLoS Biol. (2015) 13(1):e1002037. doi: 10.1371/journal.pbio.1002037
53. Hodkinson DJ, Bungert A, Bowtell R, Jackson SR, Jung J. Operculo-insular and anterior cingulate plasticity induced by transcranial magnetic stimulation in the human motor cortex: a dynamic casual modeling study. J Neurophysiol. (2021) 125(4):1180–90. doi: 10.1152/jn.00670.2020
Keywords: primary motor cortex, motor, tDCS, plasticity, connectivity, brain networks, fMRI
Citation: Hodkinson DJ, Jackson SR and Jung J (2022) Task-dependent plasticity in distributed neural circuits after transcranial direct current stimulation of the human motor cortex: A proof-of-concept study. Front. Pain Res. 3:1005634. doi: 10.3389/fpain.2022.1005634
Received: 28 July 2022; Accepted: 7 November 2022;
Published: 25 November 2022.
Edited by:
Marco L Loggia, Massachusetts General Hospital and Harvard Medical School, United StatesReviewed by:
Wanalee Klomjai, Mahidol University, ThailandDesmond Agboada, Munich University of the Federal Armed Forces, Germany
© 2022 Hodkinson, Jackson and Jung. This is an open-access article distributed under the terms of the Creative Commons Attribution License (CC BY). The use, distribution or reproduction in other forums is permitted, provided the original author(s) and the copyright owner(s) are credited and that the original publication in this journal is cited, in accordance with accepted academic practice. No use, distribution or reproduction is permitted which does not comply with these terms.
*Correspondence: Duncan J. Hodkinson ZHVuY2FuLmhvZGtpbnNvbkBub3R0aW5naGFtLmFjLnVr JeYoung Jung amV5b3VuZy5qdW5nQG5vdHRpbmdoYW0uYWMudWs=
Specialty Section: This article was submitted to Non-Pharmacological Treatment of Pain, a section of the journal Frontiers in Pain Research