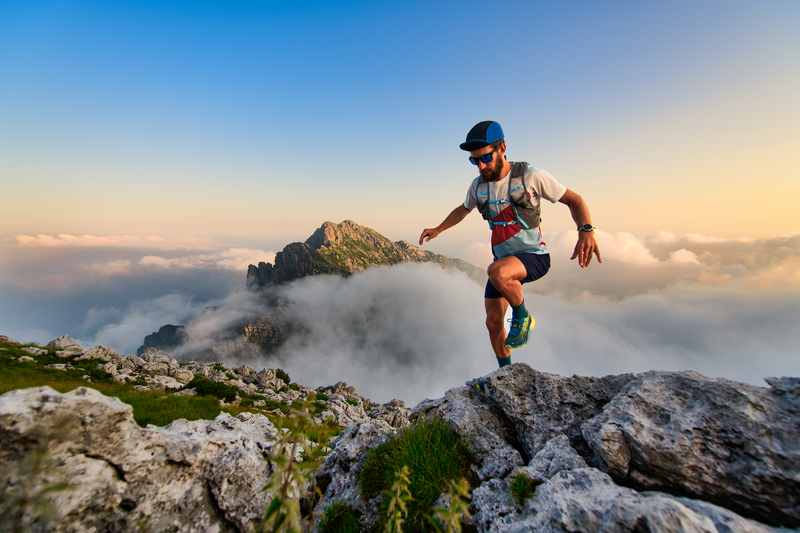
95% of researchers rate our articles as excellent or good
Learn more about the work of our research integrity team to safeguard the quality of each article we publish.
Find out more
REVIEW article
Front. Pain Res. , 25 August 2021
Sec. Musculoskeletal Pain
Volume 2 - 2021 | https://doi.org/10.3389/fpain.2021.698157
This article is part of the Research Topic Highlights in Musculoskeletal Pain 2021/22 View all 13 articles
Intractable neuropathic pain is a frequent consequence of nerve injury or disease. When peripheral nerves are injured, damaged axons undergo Wallerian degeneration. Schwann cells, mast cells, fibroblasts, keratinocytes and epithelial cells are activated leading to the generation of an “inflammatory soup” containing cytokines, chemokines and growth factors. These primary mediators sensitize sensory nerve endings, attract macrophages, neutrophils and lymphocytes, alter gene expression, promote post-translational modification of proteins, and alter ion channel function in primary afferent neurons. This leads to increased excitability and spontaneous activity and the generation of secondary mediators including colony stimulating factor 1 (CSF-1), chemokine C-C motif ligand 21 (CCL-21), Wnt3a, and Wnt5a. Release of these mediators from primary afferent neurons alters the properties of spinal microglial cells causing them to release tertiary mediators, in many situations via ATP-dependent mechanisms. Tertiary mediators such as BDNF, tumor necrosis factor α (TNF-α), interleukin 1β (IL-1β), and other Wnt ligands facilitate the generation and transmission of nociceptive information by increasing excitatory glutamatergic transmission and attenuating inhibitory GABA and glycinergic transmission in the spinal dorsal horn. This review focusses on activation of microglia by secondary mediators, release of tertiary mediators from microglia and a description of their actions in the spinal dorsal horn. Attention is drawn to the substantial differences in the precise roles of various mediators in males compared to females. At least 25 different mediators have been identified but the similarity of their actions at sensory nerve endings, in the dorsal root ganglia and in the spinal cord means there is considerable redundancy in the available mechanisms. Despite this, behavioral studies show that interruption of the actions of any single mediator can relieve signs of pain in experimental animals. We draw attention this paradox. It is difficult to explain how inactivation of one mediator can relieve pain when so many parallel pathways are available.
This review outlines aspects of the etiology of neuropathic pain at both the spinal and peripheral level. A variety of chemical mediators effect communication between the various cell types involved in the generation of pathological pain. We focus on mediators that affect spinal microglia, mediators released from microglia and their actions on their target cell types.
Peripheral nerve trauma, post herpetic neuralgia, spinal cord injury, traumatic brain injury, stroke and neuropathies associated with chemotherapy, diabetes or HIV infection can give rise to intractable neuropathic pain (1–13). Neuropathic components also contribute to pain associated with COVID-19, multiple sclerosis, fibromyalgia, migraine, osteoarthritis, rheumatoid arthritis, autoimmune disease, and complex regional pain syndromes (14–23). Although the signs and symptoms of neuropathic pain are similar in males and females, it is now well-established that the underlying cellular mechanisms are very different (24–33). Unlike nociceptive pain, which signals and protects an individual from tissue injury, neuropathic pain persists long after tissue healing and recovery has taken place (2). It is therefore maladaptive and serves no obvious biological purpose (5, 34, 35).
Many of the investigations into the etiology of neuropathic pain involve controlled, traumatic perturbations leading to defined and reproducible injuries to the spinal cord or peripheral nerves. Surgical, chemical or genetically-induced lesions to rodent peripheral neurons are followed by in vivo or ex vivo investigations of the properties of primary afferent, spinal or supra-spinal neurons. These are correlated with behavioral studies that seek to assess pain intensity by indices such as thermal or mechanical allodynia and hyperalgesia (36–40). Improvements in behavioral approaches within the last 15 years have focused on assessing pain in terms of its accepted definition as “An unpleasant sensory and emotional experience associated with, or resembling that associated with, actual or potential tissue damage,” (41). Thus, contemporary operant models seek to provide quantification of pain per se as opposed to nociception (39). For example, rodents may be required to make a conscious choice between being in a pain-inducing environment and an otherwise undesirable environment such as a brightly illuminated space (4, 42–44). The time spent in the undesirable environment gives an index of the pain the animal is experiencing. A complementary approach to pain quantification involves assessment of behaviors such as social interaction, nest-building, ultrasonic vocalization, burrowing behavior and “facial grimace score” (45–47).
Regardless of the methodology used to assess the behavioral consequences of peripheral nerve injury, it is generally accepted that;
1. Peripheral nerve injury promotes Wallerian degeneration of severed axons, macrophage, neutrophil and T-lymphocyte invasion, Schwann cell, fibroblast, mast cell, and epithelial cell activation and the generation of an “inflammatory soup” containing primary mediators such as chemokines, cytokines, Wnt ligands, neuropeptides, and growth factors (see Table 1 and Figure 1).
2. Primary mediators sensitize sensory nerve endings, attract additional macrophages and lymphocytes, alter gene expression, promote post-translational modification of proteins, and alter ion channel function in primary afferent neurons. This leads to increased excitability, spontaneous activity and the generation of secondary mediators (see Table 2 and Figure 1).
3. Secondary mediators such as colony stimulating factor 1 (CSF-1), chemokine (C-C motif) ligand 21 (CCL21), and wingless-type mammary tumor virus integration site family, member 5A (Wnt5a) are released from primary afferent terminals in the spinal dorsal horn. They affect the properties of spinal microglial cells causing them to release tertiary mediators. In this way, spinal microglia can detect and respond to peripheral nerve injury.
4. Microglial-derived tertiary mediators such as BDNF, TNF-α, and IL-1β (Brain derived neurotrophic factor, tumor necrosis factor alpha, and interleukin-1β) increase excitatory transmission and attenuate inhibitory synaptic transmission in the superficial dorsal horn (see Table 3 and Figure 1).
5. This and other aspects of synaptic plasticity facilitate the transfer of nociceptive information and promote misprocessing of sensory information leading to central sensitization at both the spinal and supra-spinal level.
6. Although it was once believed that altered microglial function was transient and confined to the onset phase of neuropathic pain, newer data implicates sustained alteration of microglial function in its long term maintenance. This is associated with long-term changes in astrocyte function.
7. Cell type involvement is sex dependent. Whereas, microglia play a predominant role in central sensitization in males, this is effected by macrophages and T-lymphocytes in females.
8. In addition to release of mediators, recent evidence suggests that cell to cell communication may be affected by the transfer of materials such as microRNA's in secreted extracellular vesicles or exosomes.
Figure 1. Sites of action of primary, secondary, and tertiary mediators in signaling of neuropathic pain. Sources of primary mediators include Schwann cells (s), epithelial cells (e), mast cells (m-c), t-lymphocytes (t-l), macrophages (m), fibroblasts (f), and neutrophils (n).
Each of these steps will be discussed below with special emphasis on the actions of secondary mediators on microglial activity and the release and actions of tertiary mediators in the spinal dorsal horn (Figure 1). Cytokine/chemokine/growth factor/glial cell interactions are also involved in modulation of sensory information in supraspinal structures following peripheral nerve injury. This includes the mesolimbic system (185) thalamus, sensory cortex, and amygdala (186–188). Interestingly, microglial activation appears on the contralateral side following nerve injury thus reflecting the projections of ascending tracts. Activation is not seen in areas which are not involved in pain processing such as the motor cortex (186). This implies that microglial activation in higher centers is not simply the result of diffusion of messengers via the cerebrospinal fluid (CSF). The present review will however focus on microglia activity within the spinal dorsal horn.
Wallerian degeneration of injured peripheral nerves is associated with neutrophil, macrophage and T-lymphocyte infiltration, mast cell, endothelial cell, keratinocyte and fibroblast activation and alteration of Schwann cell properties (2, 54, 68, 80, 98, 189–196). All of these cell types produce and release a variety of inflammatory mediators and a few anti-inflammatory agents at the site of injury (2, 190, 197) and Table 1. These primary mediators include pro-inflammatory agents such as interleukin 1β (IL-1β) (48–50, 55, 57–59, 141), leukemia inhibitory factor (LIF) (74–76, 79, 198), interleukin 15 (IL-15) (66), interleukin 17 (IL-17) (21, 68, 70), interleukin 18 (IL-18) (199) tumor necrosis factor (TNF-α) (48, 51, 80, 83, 85–88, 200–203), monocyte chemoattractant protein 1 (MCP-1/CCL2) (49, 113–115, 118), chemokine (C-X-C motif) ligand 1 (CXCL1) (120–124) and CXCL4 (125), histamine (127–130), and the secreted glycoproteins Wnt3a (wingless-type mammary tumor virus integration site family, member 3A) and Wnt5a (133, 135). For a more complete list see Moalem and Tracey (54).
As discussed below, most of these mediators excite peripheral nerve endings as well as the cell bodies of primary afferent fibers in the dorsal root ganglion (DRG) (53). Release of pro-inflammatory primary mediators both at the site of injury and within the DRG provokes changes in the cell bodies, axons and peripheral endings of both injured and uninjured primary afferent axons (141, 204–206).
Satellite glial cells that surround the cell bodies of dorsal root ganglia (DRG) neurons represent an additional source of primary inflammatory mediators (2, 78, 142, 207–209). IL-1β may also be derived from macrophages that invade DRG after injury (141) as well as from sensory neuron resident macrophages (210). Peripheral nerve injury causes extensive satellite glial cell activation (as defined by glial fibrillary acidic protein [GFAP] immunoreactivity). This is prevented by local perfusion of TTX or bupivacaine. Na+ channel block also reduces levels of NGF at a time when activated glia (Schwann cells) are an important source of NGF. This implicates injury-induced increased spontaneous activity in primary afferents in the activation of satellite glial cells (211). This aligns with the general concept of “neurogenic neuroinflammation” whereby intense neuronal activity can orchestrate immune cell activation (212).
In addition to the interactions of inflammatory mediators with neurons, many of them promote plasma extravasation and exhibit chemoattractant properties, both of which enable the recruitment of immunocompetent leucocytes and lymphocytes to the site of injury (54, 66, 68, 194). As already mentioned, these myeloid and lymphoid cells themselves release a host of cytokines and chemokines thereby instigating a positive feedback process in the initiation of neuroinflammation.
Although inflammation is a primary response to tissue injury, it should be noted that some of the primary mediators associated with neuropathic pain also serve to initiate neuronal recovery and repair (213). Thus, production of NGF at the site of nerve injury (99, 100, 102, 103, 214) may be viewed as both an initiator of inflammation and an activator of neuronal regeneration and repair. Moreover, functional recovery after peripheral nerve injury may depend on the pro-inflammatory cytokines IL-1β and TNF (48).
The situation with GDNF family ligands such as artemin is complex, whilst some reports describe its pro-inflammatory action and possible involvement in neuropathic pain, others suggest that artemin may be anti-inflammatory and activation of its receptors provide pain relief (215–219).
Interleukin 4 (IL-4) produced by peripheral nerve injury has exclusive anti-inflammatory and anti-nociceptive actions (220). These findings relate to the generalization that both inflammatory and anti-inflammatory mediators are released by nerve injury and it is disturbance of the balance between these two processes that can lead to pain (197).
Although receptors for individual cytokines are selective for their respective ligands, the downstream transduction pathways often converge, resulting in translocation of transcription factors to the nucleus and transcription of additional downstream mediators. Common signaling pathways activated following cytokine receptor activation include (1) nuclear factor-κB (NF-κB), (2) the mitogen-activated protein kinases (MAPKs), (3) the janus kinase (JAK) and signal transducer and activator of transcription (STAT), and (4) the Smad family signaling pathways (50, 187).
By contrast, chemokines, histamine and neuropeptides such as substance P signal via heptahelical G-protein coupled receptors (221, 222).
At least some of the actions of inflammatory cytokines involve activation of cyclo-oxygenase 2 (105, 223, 224) and products such as prostaglandins (93, 94, 167, 180, 225, 226) and prostacyclin (227).
Wnt ligands (Wnt; wingless-type mammary tumor virus integration site family) are a family of 19 secreted glycoproteins that are important and versatile mediators of cell–cell communication, cell morphology and development. Ligands signal by the canonical Wnt pathway, the non-canonical planar cell polarity pathway, and the non-canonical Wnt/calcium pathway (133, 228). Wnt3a acts through the canonical pathway which involves β catenin. Wnt5a acts through the non-canonical β catenin independent planar cell polarity pathway and the Ryk (134).
The downstream mediators of BDNF activation of TrkB and NGF activation of TrkA are well-characterized and include the phosphatidyl inositol-3 (PI3)-kinase (also known as Akt or protein kinase B), phospholipase C-γ1 and the ras-MAPK pathway, also known as the extracellular receptor kinase (ERK) pathway (229). Since ras-MAPK is a mediator of both neurotrophin and cytokine receptor activation, there is considerable interest in its potential as a drug target (230–232).
Gene array analysis of perturbations in primary afferent neurons following nerve injury have identified marked changes in genes coding for neuropeptides, cytokines, chemokines, receptors, ion channels, signal transduction molecules and synaptic vesicle proteins (146, 233) as well as changes in expression of long non-coding RNA's (234) and microRNA's (235–238). The latter post-transcriptionally regulate the protein expression of hundreds of genes in a sequence-specific manner (239). For example the microRNA (miRNA-let-7b) can be released from DRG neurons by neuronal activation. It acts in a paracrine function to induce rapid inward currents and action potentials in other DRG neurons by inducing toll like receptor 7 (TLR7)/TRPA1-dependent single-channel activities. Intraplantar injection of miRNA-let-7b elicits rapid spontaneous pain via TLR7 and TRPA1 (240). These observations again align with the concept of neurogenic neuroinflammation (212).
In addition, miR-21-5p which is released in the exosomal fraction of cultured DRG neurons, may be involved in neuron-macrophage communication after nerve injury (238, 241). The concept of cell-to-cell transport of material via exosomes or extracellular vesicles represents an exciting new direction for pain research (238, 241–245). A recent review focussed on release of extracellular vesicles from microglia (246).
Recordings from rodent DRG neurons both ex vivo and in vitro confirmed that peripheral nerve injury increases their excitability and may provoke spontaneous discharge of action potentials (247–253). This peripheral sensitization and ongoing, aberrant spontaneous activity is a well-established harbinger of central sensitization and chronic pain (5, 7, 9, 251, 252, 254–260). Spontaneous activity is also known to promote activation of spinal microglia and astrocytes (211, 212). Suppression of this activity in vivo by either pharmacological (257, 261) or optogenetic methodologies (262) leads to abatement of injury-induced allodynia and attenuation of hyperalgesia.
Increased DRG excitability is driven by increased expression and/or function of voltage-gated Na+, Ca2+ and hyperpolarization activated cyclic nucleotide gated channels (HCN channels) (263–265) as well as decreased expression and/or function of K+ channels (260) and altered expression, modulation and function of acid sensing ion channels (ASIC channels) and transient receptor potential (TRP) channels including TRPV1, TRPA1, and TRPM8 (215, 266–268).
Acute and/or long term exposure of DRG neurons to pro-inflammatory primary mediators such as IL-1β (interleukin 1β), IL-17 (interleukin 17), TNF (tumor necrosis factor), MCP-1/CCL-2 (monocyte chemoattractant protein-1/chemokine ligand 2), stromal cell-derived factor 1 (CXCL12), Wnt3a or prostaglandin E2 increases their excitability (21, 61, 64, 65, 88, 91, 116, 118, 119, 123, 125, 131, 133, 269, 270).
In general, the effects of primary mediators on cation channel function parallel the changes provoked by peripheral nerve injury (62, 63, 92, 125, 184, 271, 271–274) and it is now well-established that these excitatory actions play an indispensable role in the development and/or persistence of neuropathic pain. For example, administration of antibodies to interleukin I-receptor (IL-lR) or its genetic deletion or overexpression of interleukin receptor antagonist (IL-RA) reduce pain behavior in mice with experimental neuropathy thereby implicating IL-1β in the onset of neuropathic pain (2, 54, 57, 58, 202). Although IL-1β is involved at several points in the sensory system following nerve injury (176, 179, 187, 275, 276), its peripheral actions are underlined by the observation that local microinjection of recombinant IL-1β at the site of sciatic nerve injury in IL-1β-knock-out mice lowers mechanical pain thresholds to levels observed in injured wild-type animals (48).
The role of IL-17 has been studied in the paclitaxel model of chemotherapy induced pain. In addition to increasing DRG excitability, both IL-17 and paclitaxel facilitate sEPSC activity and attenuate sIPSC activity in the lamina II outer of the mouse dorsal horn. Selective knockdown of IL-17R in certain dorsal horn cells reduces paxlitaxel-induced hypersensitivity. Taken together these findings provide strong support for a role for IL-17 in this type of chronic pain (21).
Actions and involvement of TNF-α as a primary mediator very much parallel those of IL-1β Levels of TNF-α are elevated in sciatic nerve after injury (82, 85) and Nadeau et al. (48) showed that microinjection of TNF-α into TNF-knock-out mice lowered mechanical pain threshold in a similar fashion to IL-1β TNF-α also upregulates Nav1.7 in DRG (89) and inhibition of TNF-α signaling results in attenuation or accelerated recovery from injury induced neuropathic pain (52, 84, 277). TNF-α receptors are also upregulated (84). Unlike IL-1β, TNF-α does not appear to participate in macrophage to DRG neuron signaling (141) but like IL-1β actions of TNF-α are not confined to the peripheral nervous system (180, 187, 277).
Although IL-6 is markedly upregulated in the peripheral and central nervous systems following nerve injury (50–52, 278, 279) and is released by macrophages at the site of nerve injury (51, 279), it fails to affect DRG excitability (53) yet has been reported to attenuate peripheral nociceptive transmission (280). This contradicts the finding that sciatic chronic constriction injury (CCI) failed to induce hypersensitivity to cutaneous heat and pressure in mice with a null mutation of the IL-6 gene (281). Its potential role as a primary mediator thus remains to be resolved. One possibility is that IL-6 serves as an “off signal” to ensure the transient nature of injury-induced neuroinflammation. It may fulfill this function in the spinal cord where it promotes a desensitized phenotype of microglia (282). Some lines of evidence implicate IL-15, IL-17, and IL-18 as primary mediators in the generation of neuropathic pain (Table 1).
Wnt3a also increases sensory neuron excitability via upregulation of P2X3 and TRPA1 receptor channels and stimulates production of inflammatory cytokines such as TNF-α and IL-18. Intraplantar injection promotes mechanical hypersensitivity and thermal hyperalgesia. These effects are prevented by inhibition of disheveled; one of the downstream effectors of Wnt3a action (133). Nerve injury also provokes the release of Wnt5a from Schwann cells and since its cognate receptors are upregulated in DRG neurons (135), it, like Wnt3a, may serve as a primary mediator in the onset of neuropathic pain.
Appearance of ectopic excitatory α-adrenoceptors and sprouting of perivascular sympathetic axons both within DRG and on nerve terminals at the site of injury is yet another means by which primary afferent excitability is increased (283–287), leading to signs of neuropathic pain in animal models (288). Sympathetic-sensory interaction is a characteristic feature of complex regional pain syndromes in humans (289). This may reflect a neurotrophic action of LIF or NGF on noradrenergic perivascular axons (76–78) and/or may be a consequence of spontaneous afferent activity (290).
Nerve injury alters expression of neuropeptides and their cognate receptors in DRG cell bodies (291–293). Studies have focussed on galanin, NPY, calcitonin gene related peptide (CGRP) and substance P. Since there is evidence for a role of a diffusible substance in soma—soma interactions (294), neuropeptides may play a role in controlling DRG excitability (295). For example, substance P is released in a Ca2+ dependent manner from DRG cell bodies (296) and its expression is increased after nerve injury (106, 297). Because large DRG neurons start to express excitatory substance P receptors after nerve injury, it may well play a role in pain etiology (298). This is because alterations in the properties of large DRG neurons and their associated low threshold Aβ fiber axons play major role in neuropathic pain (249, 299–303).
CGRP is also released in DRG where it may fulfill an excitatory autocrine and/or paracrine function in a similar fashion to substance P (122, 295, 304, 305).
Nerve injury upregulates mRNA and/or protein for a variety of secreted proteins, including chemokines, Wnt ligands, and cytokines and/or their receptors in primary afferent neurons. This includes IL-6 and its receptor (209, 278), MCP-1/CCL2 and CC chemokine receptor 2 (CCR2) (270, 306–308), TNF-α (309), IL-1β and IL-10 (306, 310), CCL-21 (146), and Wnt5a (134). As will be discussed below, several of these substances are released from primary afferent nerve terminals and serve as secondary mediators in the dorsal horn; conveying altered peripheral activity to microglia and/or to dorsal horn neurons. The weight of the evidence supports a secondary mediator role for CSF-1 and for the chemokine CCL-21 (Table 2).
Following nerve injury, several substances generated in and released from primary afferents serve as secondary mediators that influence the properties of spinal microglia (238). In this way microglia can detect and mount a response to peripheral nerve injury.
Injury-induced release of inflammatory mediators such as interleukin 1β from satellite glial cells and invading macrophages in DRG induces Csf1 in the cell bodies of primary afferent neurons (136, 137, 141, 142). mRNA for colony stimulating factor (CSF-1) is also upregulated by nerve injury as is mRNA for the CSF-1 receptor in spinal microglia (138). Intrathecal injection of recombinant CSF-1 induces microglial proliferation and renewal as well as mechanical allodynia in naïve animals (138–140). When Csf1 gene expression is selectively depleted from sensory neurons, nerve injury-induced CSF-1 expression and the development of mechanical hypersensitivity are prevented as is the injury-induced microglial activation and proliferation (141).
Release of CSF-1 from primary afferent terminals transforms the phenotype of resting microglia such that they expresses the ionotropic ATP receptor, P2X4R (138, 139, 143). The membrane adaptor protein DAP12 is required for nerve injury-induced upregulation of P2X4R but not for microglial proliferation. Taken together, with the observation that long term exposure of dorsal horn neurons to CSF-1 increases their excitability (143), these data support its role as a secondary mediator signaling between injured primary afferents and microglia which then release tertiary mediators such as BDNF and IL-1β (150, 157, 311).
ATP derived from dorsal horn neurons activates P2X4 receptors on microglia, promoting Ca2+ influx and BDNF release (151, 312–318). As will be discussed below, this mechanism is crucial to glial signaling and the development of central sensitization in males (313, 319) but not in females (27, 320).
Mice lacking the CCR2 receptor for the chemokine MCP-1/CCL-2 fail to develop signs of neuropathic pain following nerve injury (118, 321), a MCP-1/CCL2 antagonist blocks paclitaxel-induced neuropathic pain (52) and over expression of CCR2 enhances nociceptive responses (322). MCP-1/CCL2 is not found in undamaged peripheral nerves but is strongly upregulated following injury (221, 323). This may be a consequence of the action of TNF-α and spontaneous neural activity (118, 324). MCP-1/CCL2 is expressed in vesicles in DRG soma (117, 270) and is released from DRG cell bodies in a Ca2+ dependent manner (270). This evoked release is increased under neuropathic conditions (115, 325). Injury has also been reported to increase immunoreactivity for CCR2 in dorsal horn microglia (326) and spinal administration of CCL2 promotes microglial activation (325, 327). Although these findings might be expected if MCP-1/CCL2 serves as a secondary mediator between primary afferents and spinal microglia, recent work casts doubt on this conclusion. For example, Jung et al. (117) did not detect MCP-1/CCL2 in primary afferent terminals and other studies of microglia in vivo failed to confirm the presence of CCR2 either before or after nerve injury (117, 146, 328). Now that more specific biomarkers for cell types are available, one possible explanation for this discrepancy is that CCR2 may be expressed on infiltrating monocytes or on astrocytes rather than on microglia (221, 329, 330).
Rather than functioning as a secondary mediator between primary afferents and spinal microglia, MCP-1/CCL2 may fulfill an autocrine or paracrine function within DRG (118, 270). This possibility is supported by the aforementioned observation that MCP-1/CCL2 is released from DRG cell bodies in a Ca2+ dependent manner (270). It has also been shown to excite injured DRG neurons by transactivation of TRPA1 and TRPV1 channels (115, 118). MCP-1/CCL2 may thus stimulate first order neurons in the pain cascade and/or carry out its classical chemokine function to attract CCR2-expressing peripheral monocytes/macrophages to the spinal cord (117, 146). MCP-1/CCL2 may also promote the release of the excitatory neuropeptide CGRP within DRG (122).
Intrathecal administration of chemokine (C-C motif) ligand 21 (CCL21) rapidly induces pain-like behavior in naive mice whereas CCL21 neutralizing antibodies or blockade of its cognate CXCR3 receptors with (+/–)-NBI-74330 diminishes pain-related behavior in nerve injured animals (147). The failure of CCL21 deficient mice to display tactile allodynia following nerve injury (148) has been ascribed to the failure of microglia to upregulate the P2X4 receptor for ATP (144, 146). CCL21 is upregulated in DRG following nerve injury, vesicles containing CCL21 are preferentially transported into axons (145), CCL21 affects microglial function (148) and it can be released from terminals of injured or “endangered” neurons (149, 331). Taken together, these findings suggest that CCL21 is more likely than MCP-1/CCL2 to function as a secondary mediator between primary afferents and microglia following injury (146, 221). CCL21 has also been reported to signal to astrocytes (332).
Stromal cell-derived factor-l alpha (SDF-lα) also known as C-X-C motif chemokine 12 (CXCL12), and its cognate receptor CXCR4, are constitutively present in DRG neurons and satellite glia, spinal astrocytes and microglia (333, 334). Peripheral nerve injury upregulates both CXCL12 and CXCR4 in DRG and/or spinal cord (123, 221, 333, 335, 336) as a possible consequence of the action of TNF-α (336). The functional significance of these changes is demonstrated by the observation that CXCL12-induced Ca2+ response in DRG neurons is enhanced in nerve injured animals (123). Intrathecal administration of CXCL12 induces hypersensitivity in naive rats in a CXCR4 dependent manner (333, 333). In addition intrathecal injection of CXCL12 neutralizing antibody or the CXCL12 antagonist, AMD3100 transiently reverses allodynia after peripheral nerve injury (123, 336).
CXCL12 has been implicated in pain signaling following spinal cord injury (337) and may be involved in hyperalgesic priming (338). In view of this and the findings presented above, it is clear that the CXCL12–CXCR4 system has an important role in modulation of neuropathic pain. It may be particularly involved in astrocyte signaling and long term pain maintenance (333). Despite this, we could find no reports that CXCL12 is released from injured primary afferents to affect microglia. It thus remains to be determined whether CXCL12 functions as a bona fide secondary mediator.
Fractalkine (CX3CL1) is produced constitutively by spinal cord neurons (339, 340) and its receptors (CX3CR1) are primarily expressed by dorsal horn microglia (340, 341). These are upregulated after nerve injury via an IL-6 dependent mechanism (342). Intrathecal injection of fractalkine produces mechanical allodynia and thermal hyperalgesia whereas injection of a neutralizing antibody raised against CX3CR1 delays the onset of mechanical allodynia and/or thermal hyperalgesia in two different neuropathic pain models (341). This is consistent with the observation that mice lacking CX3CR1 do not display allodynia following peripheral nerve injury (343).
Fractalkine exists in both a membrane tethered form and as a soluble protein (344). Nerve injury increases the level of soluble fractalkine in cerebrospinal fluid (345) and this release by cathepsin S appears obligatory for the expression of neuropathic pain (221, 346). Soluble fractalkine promotes microglia activation and the generation of tertiary mediators including IL-1β and TNF (167, 341).
Cathepsin S is itself released from microglia by an ATP-P2X7 dependent mechanism (347). Since fractalkine immunoreactivity does not localize with CGRP, IB4 or NF200 in the dorsal horn, it has been suggested that under neuropathic conditions, stimulation of primary afferent fibers induces release of cathepsin S from microglia, which liberates soluble fractalkine from dorsal horn neurons, thereby contributing to the amplification and maintenance of chronic pain (345). Since production of soluble fractalkine requires prior release of cathepsin S from already activated microglia, it cannot be regarded as a straightforward secondary mediator, signaling between neurons and microglia in the same way as CCL21 or CSF-1.
Because antibodies raised against CX3CR1 reduce nociceptive responses when administered 5–7 days after CCI, the prolonged release of fractalkine may contribute to the maintenance as opposed to the onset of neuropathic pain. This may relate to the observation that nerve injury provokes de novo expression of CX3CL1 in dorsal horn astrocytes (340).
Fractalkine signaling has also been implicated in synaptic degeneration seen in HIV patients who experience painful neuropathy (8). This can be modeled in mice by intrathecal injection of the viral coat protein gp120. This upregulates fractalkine and knockout of its cognate receptor CX3CR1 protects synapses from gp120-induced toxicity. Inhibition of the Wnt/β-catenin signaling blocks both gp120-induced fractalkine upregulation and synaptic degeneration. Injection of gp120 stimulates Wnt/beta-catenin-regulated fractalkine expression via NMDA receptors and the NMDA antagonist APV, Wnt/beta-catenin signaling suppressor DKK1, or knockout of CX3CR1 alleviate gp120-induced mechanical allodynia. Taken together the results suggest that HIV-1 gp120 provokes synaptic degeneration in dorsal horn by activating microglia via Wnt3a/beta-catenin-regulated fractalkine expression.
Several lines of evidence implicate interferon gamma (IFN-γ) in the etiology of neuropathic pain. Spinal microglia in naive animals express the appropriate receptor (IFN-γR) and stimulation with IFN-γ induces both tactile allodynia and altered microglia function. Genetic ablation of IFN-γR impairs nerve injury-evoked activation of ipsilateral microglia and tactile allodynia (348). The purinergic P2X4 receptor is upregulated in IFN-γ stimulated—microglia and, as will be discussed below, the appearance of such receptors plays a crucial role in the onset of neuropathic pain in males (151, 312, 314, 316, 317). IFN-γ has also been shown to increase dorsal horn excitability (349, 350) and to facilitate synaptic transmission between C-fibers and Lamina 1 neurons via a microglial dependent mechanism (351). Although the level of IFN-γ is increased in spinal cord following peripheral nerve injury (352), this may originate from invading T-lymphocytes. This implies that IFN-γ does not have a major role as a secondary mediator to effect communication between injured primary afferents and microglia.
Apart from the role of glutamate and its involvement in long term potentiation (353), there are several situations where secondary messengers generated in, and released from primary afferents exert direct long term effects on dorsal horn neurons. For example, the primary mediator role of the secreted glycoprotein Wnt3a has already been alluded to Simonetti et al. (133). Recent evidence suggests that Wnt3a promotes the release of another ligand, Wnt5a from primary afferents which in turn promotes dendritic retraction of dorsal horn neurons (134). This occurs without the intervention of microglial signaling.
The secondary mediator CSF-1 decreases excitatory drive to inhibitory neurons in dorsal horn via a BDNF independent process (143). Since the presence of CSF-1 receptors on neurons has been questioned (354), it remains to be determined whether this reflects a direct effect of CSF-1 on neurons or whether other microglial derived tertiary mediators are recruited.
Initial studies on the release and actions of BDNF were predominantly done on male rodents in an attempt to avoid possible complications imposed by the female oestrous cycle. More recent data strongly suggest major differences in the mechanism of central sensation in females compared to males; microglial derived BDNF is probably not involved in females (24, 26, 27, 313, 320, 355). In males however, numerous lines of behavioral and cellular data strongly implicate the release of BDNF from spinal microglia in the etiology of neuropathic pain (4, 143, 150, 152, 154, 156, 157, 161, 164, 315, 356–359).
As already mentioned, the secondary mediator CSF-1 is released from injured primary afferents and interacts with its receptors on microglial cells (137). This leads to the up regulation of several genes that are implicated in the development of neuropathic pain. This includes Itgam (encoding CD11b), Cx3cr1 (encoding the fractalkine/CX3CL1 receptor, CX3CR1), Bndf (encoding BDNF), and Ctss (encoding cathepsin S) (139). BDNF which acts by increasing dorsal horn excitability, is a major tertiary mediator in the development of central sensitization (4, 143, 150, 151, 156, 157, 163, 314, 315).
Long-term exposure of dorsal horn neurons to CSF-1 also increases their excitability and this effect is abrogated by the BDNF binding protein TrkB-fc (143). These findings underline the importance of a sensory neuron—CSF-1—microglia—BDNF signaling process in the onset of neuropathic pain (4, 9, 139, 238, 360).
Although stimulation of primary afferents releases ATP and generates P2X mediated EPSC's in a subpopulation of lamina II neurons (361), primary afferent neurons do not appear to be the main source of ATP following peripheral nerve injury. It may rather derive from neurons in the superficial dorsal horn itself (362). BDNF release from microglia is brought about by ATP activation of upregulated P2X4R (151, 168, 312, 314, 316–318). This release is biphasic. An early phase occurs within 5 min, whereas a late phase peaks 60 min after ATP stimulation. The late phase of release is associated with an increased level of BDNF within the microglia. Both phases of BDNF release are dependent on extracellular Ca2+ but the late phase of release and accumulation is dependent on transcription and translation. This suggests that activation of P2X4R initially releases a pre-existing pool of BDNF and subsequently promotes de novo synthesis of BDNF. This vesicular release of BDNF is abolished by inhibiting SNARE (soluble N-ethylmaleimide-sensitive factor attachment protein receptor)-mediated exocytosis and the P2X4R-evoked release and synthesis of BDNF are dependent on activation of p38-mitogen-activated protein kinase (MAPK) (312, 314–317).
Activation of P2X4 on microglia and release of BDNF are involved in the onset of neuropathic pain in males, but as already mentioned, not in females. This is congruent with the observation that spinal microglia from female rodents do not express P2XR (26).
There is also evidence for a role for metabotropic P2Y receptors in microglial activation and the onset of neuropathic pain (363–365). This involves P2Y6, 11, 12, 13, and 14 (366–369). Whilst P2Y6 signals through Gq/11 and P2Y12, 13, and 14 signal through Gs, P2Y11 signals through both Gq and Gs (222).
P2Y12 mRNA and protein are increased in microglia after peripheral nerve injury and intrathecal injection of a P2Y12 antagonist or antisense knockdown of P2Y12 expression suppresses the development of injury-induced pain behaviors and the phosphorylation of microglial p38 MAPK. By contrast, intrathecal infusion of a P2Y12 agonist into naive rats mimics the nerve injury-induced activation of microglial p38 and increases pain behaviors (366). Since phosphorylation of p38MAPK by P2X4 agonists has been implicated in BDNF release (314) this may also be affected by P2Y12 activation.
Spared nerve injury also induces a p38MAPK-dependent increase in P2Y6, 13, and 14 mRNA in microglia. This is thought to depend on activation of ROCK Rho-associated coiled-coil-containing protein kinase (370). Since intrathecal injection of the specific P2Y6 antagonist MRS2578, the specific P2Y13 antagonist MRS2211 or P2Y14 antisense, attenuate mechanical pain hypersensitivity, these three receptors may function as downstream effectors that mediate some of the actions of ATP in microglia (367, 371).
Wnt signaling can also promote BDNF release (359, 372). This phenomenon has been examined in models of HIV pain which involve exposure of sensory neurons to viral coat proteins such as gp120 (12, 372). Intrathecal injection of gp120 produces mechanical allodynia and increases expression of Wnt3a, β catenin and microglial BDNF in the murine spinal cord. Blockade of Wnt or BDNF signaling alleviates mechanical allodynia as does inhibition of microglial activation with minocycline (12). Zhang et al. (359) have suggested a mechanism whereby Wnt signaling provides an important link between increased neuronal activity and BDNF expression. Increased glutamatergic neuronal activity activates NMDA receptors and increases the level of intraneuronal Ca2+ This promotes Wnt protein synthesis and release via MAPK/CREB signaling (373, 374). Activation of frizzed receptors on microglia promotes Wnt signaling via β catenin leading to increased BDNF expression and release. This is a further illustration of the concept of “neurogenic neuroinflammation” whereby intense neuronal activity promotes immune cell activation (212).
Inflammatory pain as induced by formalin or carrageenan exposure is attenuated using the Cre-loxP system to selectively delete BDNF from nociceptive sensory neurons. Despite this, these animals display normal signs of neuropathic pain following nerve injury (375). Whilst BDNF thus appears to be involved in both inflammatory and neuropathic pain (376), in the first case it is derived from peripheral nociceptors whereas in the second case it is derived from ATP-activated microglia.
Whereas, early studies of microglia activation in response to peripheral nerve injury focussed on short term changes (312), more recent work has shown that microglial activation in rodent dorsal horn persists for more than 3 months after injury (377). Activation even persists beyond the known involvement of pro-inflammatory cytokine signaling. Thus, selective depletion of spinal microglia with the targeted immunotoxin Mac1-saporin or sequestration of BDNF with the selective binding agent TrkBFc almost completely reversed thermal and mechanical alloynia in both the acute (2 week) and chronic (3 month) phase after injury. By contrast, neutralizing cytokine signaling using intrathecal injection of a cocktail of antibodies against IL-β, TNF-α, and IL-6 significantly attenuated tactile and cold allodynia at 2 weeks but not at 3 months after injury. These findings may have therapeutic relevance as they suggest different mediators should be targeted in the management of acute vs. chronic neuropathic pain (377).
It has recently been reported that some antidepressants bind to TrkB and augment BDNF signaling (378). Since the many lines of evidence outlined above implicate BDNF in central sensitization, augmentation of TrkB signaling by antidepressants would be expected to exacerbate pain. Despite this, tricyclic antidepressants and serotonin-noradrenaline reuptake inhibitors are first line treatments in neuropathic pain management (379). The relationship between these disparate observations remains to be studied and resolved.
IL-1β plays a modulatory or effector role in nociception in the periphery, dorsal root ganglia, spinal cord and higher centers. These effects assume particular importance in the etiology of neuropathic pain. Corroborative evidence for a role of IL-1β neuropathic pain comes from the observation that inhibition of matrix metalloproteases responsible for IL-1β processing leads to attenuation of pain in a rodent model (170).
Whilst the CSF-1, P2X4-microglia-BDNF pathway is well-characterized, less is known about the release of IL-1β. In the spinal cord, it is produced and released from macrophages, astrocytes and microglia (2, 380, 381). Release from microglia is a consequence of activation of P2X7 receptors (166, 168, 311, 319) and may be provoked by the action of fractalkine (167). In agreement with this, it has recently been reported that the Cav1 channel blocker, cilnidipine blocks microglial P2X7 receptors, impairs IL-1β release and reverses nerve injury-induced mechanical hypersensitivity (173). It has also been suggested that P2X4R interact intracellularly with P2X7R to augment P2X7R-mediated IL-1β release (168).
Release of IL-1β is unlikely to reflect a SNARE dependent process as has been suggested for BDNF (314). IL-1β is known to be processed intracellularly from its inactive pro-form by caspase-1 into its mature bioactive form (382). Release from macrophages and dendritic cells and partially from neutrophils, may be brought about via the formation of gasdermin D pores in the cell membrane (382–384). One recent report implicates gasdermin D in IL-1β release from microglia in Toxoplasma gondii (parasitic protozoan) infections (385) but it remains to be determined whether a similar mode of release is engaged in neuropathic pain. In this situation, IL-1β release may involve its excocytosis via panexin channels (166).
The role of TNF-α as a peripheral primary mediator has already been alluded to and several studies have shown that signs of neuropathic pain may be alleviated by perturbation of TNF-α signaling (84, 203, 277, 386). Several lines of evidence also support a role of TNF-α as a tertiary mediator responsible for signaling between microglia and dorsal horn neurons.
Nerve injury increases levels of TNF-α mRNA in spinal microglia and microglia derived cytokine induces COX2 and PGI2 synthase expression in endothelial cells suggesting that a TNF-α mediated glia-endothelial cell interaction is involved in the generation of neuropathic pain (180).
Wnt proteins are upregulated in the spinal cord of various pain models (3, 11, 134, 199). In a very consistent manner as seen in the pathogenesis of HIV-associated pain, Wnt ligands (e.g., Wnt5a) are specifically upregulated in the SDH of “pain-positive” HIV patients (11). By regulating the pathogenesis of gp 120—induced pain, Wnt5a sensitizes pain-processing SDH neurons through the JNK/TNF-α signaling pathway.
In male rats, intrathecal administration the BDNF binding protein TrkB-Fc prevents the development of mechanical allodynia after spared nerve injury (387). Several cellular mechanisms have been implicated in the excitatory actions of microglial-derived BDNF that lead to central sensitization.
In rat spinal organotypic cultures, 5–6 d exposure to BDNF increases excitatory synaptic drive to excitatory lamina II neurons whilst decreasing excitatory drive to inhibitory neurons (157, 356). In mice, effects of BDNF are dominated by increased excitatory drive to excitatory neurons. Whereas, presynaptic TrkB and p75 neurotrophin receptors are involved, postsynaptic effects are mediated exclusively by TrkB (143). Whilst the passive and active properties of lamina II neurons such as rheobase, resting potential, input resistance and excitability are little affected (143, 157, 356), the altered synaptic activity is capable of increasing spontaneous action potential discharge in excitatory neurons whilst reducing it in inhibitory neurons (356). Three observations show that these actions of BDNF are relevant to injury-CSF-1-microglia evoked central sensitization. Firstly BDNF—induced changes in synaptic transmission and its lack of effect on the intrinsic excitability of lamina II neurons very much parallel those invoked by peripheral nerve injury (157, 388, 389). Secondly, Ca2+ responses evoked by neuronal depolarization are enhanced by BDNF and also by conditioned medium from lipopolysaccharide-activated microglia. The effect of this conditioned medium is attenuated by sequestering BDNF with TrkBd5 (157). Thirdly, the putative microglial modulator CSF-1 increases synaptic excitation of excitatory lamina II neurons in mice and this effect is abrogated by sequestering BDNF with TrkBfc (143) whereas, as already mentioned, CSF-1 reduces excitation of putative inhibitory neurons in a BDNF-independent mechanism, suggesting that injured primary afferents can also signal directly to dorsal horn neurons without the involvement of microglia (143).
The BDNF effects alluded to above relate primarily to AMPA receptor mediated transmission as neurons were studied at a holding potential of −70 mV (143, 157, 356, 388, 389). There is however a considerable body of evidence to support a role for altered NMDA receptor function in the etiology of pathological pain. This is supported by the occasional success realized with NMDA blockers such as ketamine in the clinic (390, 391). The link between NMDA receptor function and BDNF was established over 20 years ago by the observation that it enhances excitatory responses to NMDA in rat spinal cord in vitro (392). BDNF phosphorylates GluN1 via ERK and PKC (393). It also acts through TrkB to phosphorylate the GluN2B subunit by the Src-family kinase Fyn and thereby potentiates excitatory NMDA receptor-mediated currents (165). Interestingly, this potentiation appears to require the coincident BDNF mediated Cl− disinhibition. The exact molecular mechanism of this interaction remains to be elucidated as it does not appear to reflect increased NMDA receptor availability as a result of GABA-induced depolarization (165).
BDNF also acts via TrkB and a Src-family kinase to potentiate the function of presynaptic NMDA receptors on primary afferent terminals (394). It has been reported that presynaptic NMDA receptors only potentiate glutamate release from primary afferents after nerve injury (395). This further underlines the presynaptic BDNF effect in the development of central sensitization.
Peripheral nerve injury reduces expression of the potassium-chloride exporter (KCC2) in spinal lamina 1 neurons (396, 397). The resulting accumulation of intracellular Cl− causes normally outward, inhibitory GABAergic synaptic currents mediated by Cl− influx to become inward excitatory currents mediated by Cl− efflux (396–398). Since the knockdown of spinal KCC2 in non-injured rats reduces pain thresholds and induces neuropathic pain behaviors, these changes contribute to the pathophysiology of central sensitization (150, 396).
In male rats, BDNF mediates this downregulation of KCC2 (164). Thus, administration of ATP activated microglia, but not control microglia, reproduces the shift in anion gradient seen after nerve injury as does application of BDNF. Also, blocking TrkB or using interfering RNA against BDNF reverses both injury induced pain behaviors and the shift in anion gradient (150). Further analysis of this phenomenon reveals that changes in KCC2 expression in deep dorsal horn neurons are confined to nociceptive neurons that project via the spinothalamic tract whereas wide dynamic range (WDR) neurons that are activated by a variety of sensory modalities are unaffected (399). It has also been shown that neurons in lamina I are more susceptible to changes in Cl− gradient than those in lamina II (397) and biophysical and modeling analysis shows this loss is especially effective in promoting increased neuronal firing (400). These are important observations as lamina I and deep dorsal horn nociceptive neurons are the most important sites for relay of nociceptive information to the brain (303, 401, 402). Since loss of GABAergic inhibition enables non-noxious Aβ fiber-mediated excitatory transmission to acess the superficial spinal dorsal horn, this process plays a major role in the establishment of allodynia (300, 403, 404).
Reversal of the Cl− gradient may rationalize the observation that BDNF increases GABA release in the dorsal horn (159, 161, 405). Under these conditions GABA produces inward currents (396) which would be enhanced and therefore strongly excitatory.
Long term potentiation (LTP) of synaptic transmission contributes to central sensitization in the dorsal horn (353, 406–408). LTP of C-fiber responses can also be augmented by BDNF (387) and LTP induced by high frequency nerve stimulation is occluded by BDNF treatment (409). This reflects functional upregulation GluN2B subunits of NMDA receptors by activation of the tyrosine phosphatase SHP2 (409) or Fyn kinase-mediated phosphorylation of GluN2B subunit at tyrosine 1472 (387). These authors also showed intrathecal administration of BDNF scavenger TrkB-Fc prior to surgery could prevent the nerve injury-induced increase of both phosphorylated Fyn and phosphorylated GluN2B expression and as mentioned above it also prevented the development of mechanical allodynia after spared nerve injury. The importance of these effects was recently underlined by the observation that spinal LTP induced by high frequency stimulation as well as microglial activation and upregulation of BDNF are inhibited by antibodies to CSF-1. This strongly implicates CSF-1/nerve injury driven microglial derived BDNF in the generation of spinal LTP (408).
Manipulations that increase neuronal excitability can induce synchronous waves in the level of cytosolic Ca2+ that propagate across the whole dorsal horn (410–412). Similarly, K+-induced depolarization invokes oscillatory activity as monitored by spontaneous field potentials (413). It has also recently been shown that action potential discharge encodes cytosolic Ca2+ levels in lamina 1 neurons and even a single action potential can provoke a measurable Ca2+ response (414). This implies that spontaneous bursting activity and oscillations of cytosolic Ca2+ level may be closely related. Although long term application of BDNF does not change the resting membrane potential, input resistance of rat dorsal horn neurons in organotypic culture (158) it promotes oscillations in the level of intracellular Ca2+ in some neurons whilst depressing it in others (163). There appear to be several mechanisms whereby oscillations may be set up, for example those observed by Alles et al. (163) and Chapman et al. (411) were prevented following blockade of AMPA glutamate receptors whereas those by Asghar et al. (413) were merely attenuated. The oscillations recorded by all three groups were however blocked by TTX, again underlining the tight assocaition between action potential activity and Ca2+ signalling which in turn may enable Ca2+-dependent gene expression. Whilst the oscillations appeared to be primarily originating from neurons the possible contribution of signal from astrocytes cannot completely be ruled out. Although any direct relationship between these oscillations and neuropathic pain mechanisms remains to be established, sciatic nerve injury has been reported to induce spontaneous bursting activity in a subgroup of dorsal horn neurons in vivo (415). MRI studies have also revealed oscillatory activity in the spinal cord of neuropathic pain patients (416). It may be posited therefore that oscillations in Ca2+ level and spontaneous bursting activity contribute to the bouts of spontaneous “electric shock like” pain experienced by those afflicted with painful neuropathies (163).
As already mentioned, peripheral nerve injury produces neuron type specific effects on synaptic transmission in the dorsal horn; excitation of excitatory neurons is increased whereas excitation of inhibitory neurons is decreased (143, 156–159, 356, 388, 389). In addition to altered neurotransmitter release and alterations in postsynaptic sensitivity, connectivity is lost at some synapses (8, 417, 418) but new connections and/or reorganization of dendritic spines occurs at others (408, 419).
Microglia are clearly capable of releasing mediators which promote neuronal loss in an animal model of multiple sclerosis (140) and synaptic degeneration in a model of HIV pain (8). This process of microgliosis is also seen following peripheral nerve injury (420, 421). As discussed below, these processes are likely to reflect the action of microglia-derived BDNF and in the case of HIV pain may reflect phagocytosis of damaged synapses by activated microglia (8).
Peripheral nerve injury promotes transient loss of glutamatergic excitatory terminals from non-peptidergic IB-4 positive nociceptive fibers in the substantia gelatinosa (418, 422). These fibers form the synaptic terminals of the “type 1” synaptic glomeruli (423) which contact GABAergic neurons (402, 424). Morphological changes may therefore contribute to injury-induced reductions in the amplitude and frequency of spontaneous and miniature EPSCs in tonic firing, putative inhibitory neurons (388). This attenuation of excitatory drive to inhibitory neurons would be expected to contribute to an overall increase in dorsal horn excitability (158). Since BDNF also reduces mEPSC amplitude and frequency in putative inhibitory neurons in rat dorsal horn (356) it is possible that BDNF accounts for loss of primary afferent terminals (418, 422). This possibility requires further investigation as BDNF stimulates overall axon growth and regeneration in the spinal cord (425, 426).
This differs from the situation in mice where BDNF does not affect excitatory drive to inhibitory neurons (143). It remains to be determined whether this simply reflects a species difference or whether it is a consequence of the more rigorous criteria to define inhibitory neurons in mice (143, 412) compared to rats (157, 356).
BDNF is not involved in injury-induced loss of GABA terminals. Nerve injury also promotes loss of GABAergic inhibitory terminals in laminae I and II of the dorsal horn (422, 427). Because BDNF enhances GABAergic transmission at various synaptic loci in the dorsal horn (158, 159, 161), the nerve injury-induced loss of inhibitory terminals is unlikely to involve BDNF.
In rats, both nerve injury and BDNF increase excitatory synaptic drive to putative excitatory neurons (157, 356, 388, 389, 428) and a similar effect of BDNF is seen in mice. CSF-1 also increases synaptic drive in a BDNF dependent fashion (143). These observations parallel the observation that both BDNF and CSF-1 increase CGRP containing terminals in response to nocigenic high frequency stimulation (408) as these terminals primarily innervate excitatory neurons (402).
In addition to its actions on neurons as described above, BDNF also activates astrocytes such that they release additional mediators that participate in the establishment of central sensitization (429).
IL-1β levels are increased in the cerebrospinal fluid (CSF) of patients with complex regional pain syndrome (275) and in spinal cords obtained post-mortem from patients with painful HIV related neuropathy (3). Although there are several reports of the effectiveness of IL-1β antagonists and genetic impairment of cytokine function in animal models of neuropathic pain (57–59, 171) studies of the effectiveness of the modified human interleukin 1 receptor antagonist protein (anakinra) in the clinic have been limited by the pharmacokinetic issues imposed by the blood brain barrier (171).
As mentioned above, release of IL-1β from microglia is primarily affected by activation of P2X7 receptors (166, 173, 311, 319) and/or by the action of fractalkine (167). In a similar fashion to BDNF, IL-1β increases overall dorsal horn excitability, glutamate release from primary afferents and excitatory synaptic transmission between primary afferent C-fibers and lamina 1 neurons (167, 176, 430).
Like BDNF, IL-1β does not affect the membrane potential or rheobase of lamina II neurons, suggesting that most of its effect on dorsal horn excitability can be ascribed to changes in synaptic transmission (175, 176). We found that exposing organotypic cultures of rat spinal cord to 100 pM IL-1β for 6–8 d increased the amplitude of spontaneous EPSC's (sEPSC) in putative excitatory “delay” neurons, and decreased the frequency of spontaneous IPSC's (sIPSC). These are somewhat similar to those seen with peripheral nerve injury (388, 389). IL-1β would therefore be expected to increase dorsal horn excitability and to facilitate the transfer of nociceptive information. This was confirmed by the observation that Ca2+ responses evoked by exposure of neurons to 20 mM K+ were augmented by IL-1β exposure (176). However, other actions of IL-1β included disinhibition of putative inhibitory “tonic” neurons and although the frequency of sIPSC's in putative excitatory “delay” neurons was decreased, their amplitude was increased. The latter observations may be rationalized if GABA assumes an excitatory role in the injury situation due to perturbation of Cl− gradients by BDNF (150).
We used long-term application of IL-1β to parallel the time course of injury-induced changes in spinal cytokine levels (48, 176). Our findings are paralleled by the observations that acute application of IL-1β increases the amplitude of AMPA and NMDA currents in the spinal dorsal horn (178) and increases glutamate release via an interaction with presynaptic NMDA receptors (430). Acute cytokine application also enhances both the frequency and amplitude of sEPSCs in unidentified lamina II neurons (175). These authors reported a reduction in the frequency and amplitude of sIPSCs. The differences between this work and ours may not only represent the different time course of cytokine activation as Kawasaki et al. used 600 pM IL-1β in their work whereas we used a somewhat lower concentration of 100pM and observed differential actions on excitatory vs. inhibitory neurons.
Further analysis of fractalkine—microglia—IL-1β signaling led Clark et al. (167) to propose the following sequence of events. Soluble fractalkine activates CX3CR1 on microglial cells leading to the rapid release of IL-1β. IL-1β activates IL-1r on lamina 1 neurons and modulates function of postsynaptic NMDA receptors such that Ca2+ influx is increased when they are activated by glutamate. Elevated levels of intracellular Ca2+ in lamina I neurons activates phospholipase A2 leading to the liberation of arachidonic acid and the generation of prostaglandins. Within a few minutes of fractalkine application, prostaglandins increase transmitter release from primary afferents both directly and indirectly via iNOS activation and release of NO from microglia.
Presynaptic NMDA receptors have also been implicated in spinal actions of IL-1β where signaling between IL-1r and NMDA may be affected by the sphingomyelinase/ceramide signaling pathway to enhance glutamate release from the primary afferents in neuropathic rats (395, 430). IL-1β enhances endocytosis of glial glutamate transporters in the dorsal horn astrocytes through activating protein kinase C (431), the resultant augmentation of glutamate responses represents a complementary mechanism where cytokine enhances excitatory synaptic transmission.
Acute activation of TNF receptor 1 by TNF-α inhibits the excitability of a subset of spinal GABAergic neurons. This effect involves p38 mitogen-activated protein kinase dependent suppression hyperpolarization-activated cation current (Ih) (182). These effects have been reported to diminish with time suggesting TNF-α may be primarily involved with the induction rather than the persistence of neuropathic pain (40).
Although fractalkine action on microglia and potentiation of synaptic transmission in the dorsal horn involves IL-1β but not TNF-α (167), it does appear to facilitate long term potentaition (183). This has led to the suggestion that the differential contributions of TNF-α and IL-1β to fractalkine-induced enhancement of synaptic transmission may reflect the well-characterized phenotypic diversity of microglia (432). Thus, activation of microglia by different secondary mediators may result in release of specific mixtures of tertiary mediators which in turn promote diverse effects on synaptic transmission (183).
Astrocytes become rapidly and persistently activated after peripheral nerve injury, suggesting they play a role in both the onset and maintenance of central sensitization (3, 433–435). As mentioned above, recent evidence also implicates microglial function in the long-term maintenance of neuropathic pain in animal models (377) but this may not be the case in all types of neuropathic pain in the clinic (3).
It is well-established that IL-1β from microglia stimulates astrocytic production of TNF–α and IL-6 as well as IL-1β itself (381, 434) thereby amplifying the initial IL-1β signal. Microglial derived IL-1β reduces the capacity of astrocytes to take up glutamate (179, 430) as a result of internalization of the astrocytic glutamate transporter (EAAT2) (179). Loss of EAAT2 function induces hyperalgesia, augmentation of glutamatergic synaptic responses and increased sensitivity of dorsal horn neurons to primary afferent stimulation (436, 437). Activated astrocytes have also been reported to release the NMDA receptor co-agonist D-serine (438) thereby augmenting overall dorsal horn excitability. Evidence for astrocyte involvement in the clinic has been obtained by post-mortem studies of HIV-patients with painful neuropathy (3). These authors showed that expression levels of the microglial markers CD11b and Iba1 were not elevated whereas the astrocytic markers GFAP and S100 beta were clearly increased. This was accompanied by increased levels of TNF-α and IL-1β, as well as components of MAPK signaling pathway, including pERK, pCREB, and c-Fos.
Since astrocytes are not the primary focus of this review, readers are directed to the recent review by Ji et al. (435) which underlines the role of astrocytic gap junctions and astrocyte derived chemokines in pathological pain. Several other comprehensive reviews have appeared (439–441) and recent work has underlined the role of astrocyte derived IL-17 in paxlitaxel induced pain (21).
We have used the term primary mediator to cover substances released from the site of nerve injury, secondary mediator to describe substances released from primary afferent terminals and tertiary mediators to define substances released from microglia (Figure 1). Whereas, BDNF selectively released from microglia can be described as a tertiary mediator, production and effect of cytokines and chemokines is far more widespread. For example, IL-1β which is a classical macrophage derived signal, can be released from Schwann cells, microglia, astrocytes, neutrophils, granulocytes, mast cells and endothelial cells (2, 190, 381, 434, 442, 443) it would thus be classified both as a primary and tertiary messenger. In general it can be said that cytokines such as IL-1β can be released from more or less any cell type in response to an appropriate stimulus. IL-17 appears to be a primary mediator which is also released from spinal astrocytes in a model of chemotherapy pain (21).
Opening of the blood brain barrier is a well-known correlate of nerve injury induced allodynia (50, 444) and this may be initiated by aberrant afferent nerve activity (445). This enables lymphocyte and macrophage invasion of neural tissue. In addition, mediators generated in damaged nerves, microglia, Schwann cells or astrocytes might be expected to enter the circulation and exert actions throughout the body. This is supported by the observation that plasma levels of IL-1β are elevated in rodents subjected to spared nerve injury (446) or exposure to paclitaxel which models chemotherapy pain (52).
Mediators generated in the spinal cord would also be expected to have access to other brain regions via the CSF. IL-1β levels are increased in the CSF of patients with complex regional pain syndrome (275) and with thoracic disc herniation (447). Inflammatory mediators may also be elevated in the CSF of osteoarthritis patients (15).
Taken together these finding suggest that the diffusion of spinally and DRG generated mediators may gain access to other brain regions via both the CSF and systemic circulation. This may lead to mirror image pain following unilateral nerve injury (448) and/or mediator actions in higher brain regions that contribute to the analysis of nociceptive phenomena, the affective components of pain, sickness syndrome and formation of memory traces (446, 449). For example, microglia activation and BDNF release in the mesolimbic reward circuitry may contribute to the negative affect associated with chronic pain (185). With the possible exception of BDNF, all of the mediators described (cytokines, chemokines and Wnt ligands) can be released from multiple cell types and as such may play a role in the initiation or maintenance of neuropathic pain throughout the nervous system. Discussion of the actions of mediators in higher brain centers is outside the scope of this review.
Neuropathic pain is seen more frequently in women than in men (29) and it is now recognized that understanding of divergent pain mechanisms in males vs. females is crucial to the development of appropriate therapeutic approaches (28, 33, 450, 451).
Investigations over the last 15 years or so have started to unravel cellular and molecular mechanisms that may contribute to this difference (29, 31–33, 452, 453). For example, microglia are not required for mechanical sensitivity to pain in female mice which require activation of adaptive immune cells such as T-lymphocytes (27, 320). The difference may result from a lack of P2X4 receptors in the microglia of females (26, 313). Despite this, behavioral responses to nerve injury in female rats are similar to those seen in males and both involve downregulation of KCC2 and perturbation of Cl- gradients (25). Because BDNF is not necessary for the development of allodynia in female rodents (27), the mediator released from adaptive immune cells remains to be determined. Similar findings have been found in the Freund's adjuvant in vivo model of inflammatory pain in rodents and confirmed in human neurons (33). These authors also showed that ex vivo BDNF enhanced synaptic NMDA receptor responses in lamina I neurons from males but not from females and that ovariectomy eliminated these differences. Importantly, the findings illustrate how sexual convergence onto shared cellular and behavioral endpoints, such as allodynia, pain sensitivity or KCC2 downregulation, may mask sex differences in underlying molecular and cellular mechanisms (28). Other recent work has shown that macrophage invasion of DRG is predominant in males and not in females although both show similar amounts of allodynia following peripheral nerve injury (454).
The realization that different mechanism are engaged to generate neuropathic pain in males vs. females has obvious therapeutic implications. For example, blockade of Nav1.8 channels in the peripheral nervous system with A-803467 is more effective in females than in males in a rodent model of joint neuropathic pain (455). Might blockers of Nav1.8 be more effective in women than in men? On the other hand, restoration of KCC2 function (456) may be effective in both males and females?
It is well-known that different types of nerve injury provoke different types or behavioral or physiological response. Thus, while mechanical allodynia produced by spared nerve injury persists for many weeks, that produced by chronic constriction injury is short-lived and recovery is seen in about 4 weeks (38, 142). Similarly, changes in synaptic transmission in the superficial dorsal horn are more robust after sciatic CCI than after complete sciatic nerve section (axotomy) (389). These findings may be consistent with an earlier observation that CCI promotes stronger and more long lasting upregulation of the primary mediators TNF-α, IL-1β, IL-10, MCP-1/CCL-2 in nerve stumps than nerve crush (306). Whilst neuropathic pain associated with multiple sclerosis is characterized by loss of spinal neurons (140), this effect is not produced with CCI (457, 458).
Recent work has shown how the nature of peripheral injury dictates the precise spinal circuitry involved in the generation of mechanical allodynia (459). Thus, neuropathic injuries generate allodynia by activation of excitatory protein kinase C gamma positive (PKCγ) neurons at the lamina II/III interface (460) whereas mechanical allodynia induced by inflammation involves excitatory calretinin positive neurons in inner lamina II (461). Cholecystokinin (CCK) positive neurons in laminae III-IV are important in both situations. Peirs et al. (459) also distinguished punctate allodynia (as produced by Von Frey filaments) from dynamic allodynia (produced by brushing a cotton swab across the hindpaw skin). This allowed them to identify a subset of CCK neurons which expressed the musculoaponeurotic fibrosarcoma oncogene homolog (Maf) and the transient vesicular glutamate transporter 3 (tVGLUT3), which are primarily involved in conveying dynamic rather than punctate allodynia.
Other work using knockout mice has shown that deficiency of CCL19/21 attenuates nerve injury evoked pain but not the hyperalgesia evoked by the autoimmune encephalomyelitis model of multiple sclerosis (149).
The above findings point to the possibility that different types of injury provoke the generation of different sets of mediators (276). This may be due to differential damage to various subsets of primary afferent fibers.
The above sections outline the actions of many of the proposed primary, secondary and tertiary mediators involved in the development and persistence of neuropathic pain. There are several pathways by which a peripheral nerve injury can lead to pain but as shown in Tables 1–3, interruption of the actions of any single mediator seems to be capable of alleviating pain. For example, ATP activation of P2X7 receptors on microglia promotes release of IL-1β and activation of P2X receptors promotes release of BDNF. This would imply that it would be necessary to prevent the action of both IL-1β and BDNF to prevent the development of allodynia but it is known that inhibition of the actions of either individual mediator is effective. In other words if BDNF is inhibited why can't pain be initiated by IL-1β If IL-1β is inhibited why can't pain be initiated by BDNF? Also as mentioned above the actions of inflammatory mediators are mediated by a limited number of downstream signaling processes: ERK-MAPK signaling seems particularly important in this regard. If one signaling cytokine is blocked or knocked out why aren't its downstream effector mechanisms activated by other cytokines?
A better understanding of the interactions between mediators and their receptors and downstream effectors is clearly required for a more complete understanding of mechanisms underlying neuropathic pain in animal models that will lead to a better understanding of pain etiology in individual patients. This in turn may enable the application of personalized medicine approaches to pain management (459, 462).
All authors were involved in the writing and/or review of the manuscript.
PS was supported by Canadian Institutes of Health Grant MOP 81089 and research supplements from the Faculty of Medicine and Dentistry, University of Alberta S-JT was supported by NIH Grants R01NS079166, R01DA036165, R01NS095747, and 1R01DA050530.
The authors declare that the research was conducted in the absence of any commercial or financial relationships that could be construed as a potential conflict of interest.
All claims expressed in this article are solely those of the authors and do not necessarily represent those of their affiliated organizations, or those of the publisher, the editors and the reviewers. Any product that may be evaluated in this article, or claim that may be made by its manufacturer, is not guaranteed or endorsed by the publisher.
1. Acharjee S, Noorbakhsh F, Stemkowski PL, Olechowski C, Cohen EA, Ballanyi K, et al. HIV-1 viral protein R causes peripheral nervous system injury associated with in vivo neuropathic pain. FASEB J. (2010) 24:4343–53. doi: 10.1096/fj.10-162313
2. Scholz J, Woolf CJ. The neuropathic pain triad: neurons, immune cells and glia. Nat Neurosci. (2007) 10:1361–8. doi: 10.1038/nn1992
3. Shi Y, Gelman BB, Lisinicchia JG, Tang SJ. Chronic-pain-associated astrocytic reaction in the spinal cord dorsal horn of human immunodeficiency virus-infected patients. J Neurosci. (2012) 32:10833–40. doi: 10.1523/JNEUROSCI.5628-11.2012
4. Alles SRA, Smith PA. The etiology and pharmacology of neuropathic pain. Pharmacol Rev. (2018) 70:315–47. doi: 10.1124/pr.117.014399
5. Costigan M, Scholz J, Woolf CJ. Neuropathic pain: a maladaptive response of the nervous system to damage. Annu Rev Neurosci. (2009) 32:1–32. doi: 10.1146/annurev.neuro.051508.135531
6. von Hehn CA, Baron R, Woolf CJ. Deconstructing the neuropathic pain phenotype to reveal neural mechanisms. Neuron. (2012) 73:638–52. doi: 10.1016/j.neuron.2012.02.008
7. Gold MS, Gebhart GF. Nociceptor sensitization in pain pathogenesis. Nat Med. (2010) 16:1248–57. doi: 10.1038/nm.2235
8. Ru W, Liu X, Bae C, Shi Y, Walikonis R, Mo CJ, et al. Microglia mediate HIV-1 gp120-induced synaptic degeneration in spinal pain neural circuits. J Neurosci. (2019) 39:8408–21. doi: 10.1523/JNEUROSCI.2851-18.2019
9. Finnerup NB, Kuner R, Jensen TS. Neuropathic pain: from mechanisms to treatment. Physiol Rev. (2021) 101:259–301. doi: 10.1152/physrev.00045.2019
10. Grace PM, Tawfik VL, Svensson CI, Burton MD, Loggia ML, Hutchinson MR. The neuroimmunology of chronic pain: from rodents to humans. J Neurosci. (2021) 41:855. doi: 10.1523/JNEUROSCI.1650-20.2020
11. Shi Y, Shu J, Gelman BB, Lisinicchia JG, Tang SJ. Wnt signaling in the pathogenesis of human HIV-associated pain syndromes. J Neuroimmune Pharmacol. (2013) 8:956–64. doi: 10.1007/s11481-013-9474-4
12. Zhou X, Tao L, Zhao M, Wu S, Obeng E, Wang D, et al. Wnt/beta-catenin signaling regulates brain-derived neurotrophic factor release from spinal microglia to mediate HIV1 gp120-induced neuropathic pain. Mol Pain. (2020) 16:1744806920922100. doi: 10.1177/1744806920922100
13. Gradwell MA, Callister RJ, Graham BA. Reviewing the case for compromised spinal inhibition in neuropathic pain. J Neural Trans. (2020) 127:481–503. doi: 10.1007/s00702-019-02090-0
14. Olechowski CJ, Truong JJ, Kerr BJ. Neuropathic pain behaviours in a chronic-relapsing model of experimental autoimmune encephalomyelitis (EAE). Pain. (2009) 141:156–64. doi: 10.1016/j.pain.2008.11.002
15. Lu VB, Smith PA, Rashiq S. The excitability of dorsal horn neurons is affected by cerebrospinal fluid from humans with osteoarthritis. Can J Physiol Pharmacol. (2012) 90:783–90. doi: 10.1139/y2012-014
16. Rifbjerg-Madsen S, Christensen AW, Christensen R, Hetland ML, Bliddal H, Kristensen LE, et al. Pain and pain mechanisms in patients with inflammatory arthritis: a Danish nationwide cross-sectional DANBIO registry survey. PLoS ONE. (2017) 12:e0180014. doi: 10.1371/journal.pone.0180014
17. Sumpton JE, Moulin DE. Fibromyalgia. Handb Clin Neurol. (2014) 119:513–27. doi: 10.1016/B978-0-7020-4086-3.00033-3
18. Mifflin KA, Kerr BJ. Pain in autoimmune disorders. J Neurosci Res. (2017) 95:1282–94. doi: 10.1002/jnr.23844
19. Yuan S, Shi Y, Tang SJ. Wnt signaling in the pathogenesis of multiple sclerosis-associated chronic pain. J Neuroimmune Pharmacol. (2012) 7:904–13. doi: 10.1007/s11481-012-9370-3
20. Descoeur J, Pereira V, Pizzoccaro A, Francois A, Ling B, Maffre V, et al. Oxaliplatin-induced cold hypersensitivity is due to remodelling of ion channel expression in nociceptors. EMBO Mol Med. (2011) 3:266–78. doi: 10.1002/emmm.201100134
21. Luo H, Liu HZ, Zhang WW, Matsuda M, Lv N, Chen G, et al. Interleukin-17 regulates neuron-glial communications, synaptic transmission, and neuropathic pain after chemotherapy. Cell Rep. (2019) 29:2384–97. doi: 10.1016/j.celrep.2019.10.085
22. Rosen JM, Yaggie RE, Woida PJ, Miller RJ, Schaeffer AJ, Klumpp DJ. TRPV1 and the MCP-1/CCR2 axis modulate post-UTI chronic pain. Sci Rep. (2018) 8:7188. doi: 10.1038/s41598-018-24056-0
23. Attal N, Martinez VR, Bouhassira D. Potential for increased prevalence of neuropathic pain after the COVID-19 pandemic. PAIN Rep. 6:e884. doi: 10.1097/PR9.0000000000000884
24. Halievski K, Ghazisaeidi S, Salter MW. Sex-dependent mechanisms of chronic pain: a focus on microglia and P2X4R. J Pharmacol Exp Ther. (2020) 375:202–9. doi: 10.1124/jpet.120.265017
25. Mapplebeck JCS, Lorenzo LE, Lee KY, Gauthier C, Muley MM, De KY, et al. Chloride dysregulation through downregulation of KCC2 mediates neuropathic pain in both sexes. Cell Rep. (2019) 28:590–6. doi: 10.1016/j.celrep.2019.06.059
26. Mapplebeck JCS, Dalgarno R, Tu Y, Moriarty O, Beggs S, Kwok CHT, et al. Microglial P2X4R-evoked pain hypersensitivity is sexually dimorphic in rats. Pain. (2018) 159:1752–63. doi: 10.1097/j.pain.0000000000001265
27. Sorge RE, Mapplebeck JC, Rosen S, Beggs S, Taves S, Alexander JK, et al. Different immune cells mediate mechanical pain hypersensitivity in male and female mice. Nat Neurosci. (2015) 18:1081–3. doi: 10.1038/nn.4053
28. Mogil JS. Qualitative sex differences in pain processing: emerging evidence of a biased literature. Nat Rev Neurosci. (2020) 21:353–65. doi: 10.1038/s41583-020-0310-6
29. Mogil JS. Sex differences in pain and pain inhibition: multiple explanations of a controversial phenomenon. Nat Rev Neurosci. (2012) 13:859–66. doi: 10.1038/nrn3360
30. Mifflin KA, Yousuf MS, Thorburn KC, Huang J, Perez-Munoz ME, Tenorio G, et al. Voluntary wheel running reveals sex specific nociceptive factors in murine experimental autoimmune encephalomyelitis. Pain. (2018) 160:870–81. doi: 10.1097/j.pain.0000000000001465
31. Mifflin KA, Benson C, Thorburn KC, Baker GB, Kerr BJ. Manipulation of neurotransmitter levels has differential effects on formalin-evoked nociceptive behavior in male and female mice. J Pain. (2016) 17:483–98. doi: 10.1016/j.jpain.2015.12.013
32. Mifflin KA, Kerr BJ. Sex-related differences in acute and chronic pain: a bench to bedside perspective. Can J Anaesth. (2013) 60:221–6. doi: 10.1007/s12630-012-9881-7
33. Dedek A, Xu J, Lorenzo LE, Godin AG, Kandegedara CM, et al. Sexual dimorphism in a neuronal mechanism of spinal hyperexcitability across rodent and human models of pathological pain. BioRxiv [Preprint]. (2021). doi: 10.1101/2021.06.15.447407
34. Iadarola MJ, Caudle RM. Good pain, bad pain. Science. (1997) 278:239–40. doi: 10.1126/science.278.5336.239
35. Li XY, Wan Y, Tang SJ, Guan Y, Wei F, Ma D. Maladaptive plasticity and neuropathic pain. Neural Plast. (2016) 2016:4842159. doi: 10.1155/2016/4842159
36. Stemkowski PL, Smith PA. An overview of animal models of neuropathic pain. In: Toth C. Moulin DE, editors. Neuropathic Pain, Causes, Management and Understanding. Cambridge: Cambridge University Press (2013). p. 33–50.
37. Kim KJ, Yoon YW, Chung JM. Comparison of three rodent models of neuropathic pain. Exp Brain Res. (1997) 113:200–6. doi: 10.1007/BF02450318
38. Decosterd I, Woolf CJ. Spared nerve injury: an animal model of persistent peripheral neuropathic pain. Pain. (2000) 87:149–58. doi: 10.1016/S0304-3959(00)00276-1
39. Mogil JS. Animal models of pain: progress and challenges. Nat Rev Neurosci. (2009) 10:283–94. doi: 10.1038/nrn2606
40. Zhang H, Zhang H, Dougherty PM. Dynamic effects of TNF-+| on synaptic transmission in mice over time following sciatic nerve chronic constriction injury. J Neurophysiol. (2013) 110:1663–71. doi: 10.1152/jn.01088.2012
41. IASP Subcommittee on Taxonomy. Pain terms: a list with definitions and notes on usage. Recommended by the IASP Subcommittee on Taxonomy. Pain. (1979) 6:249.
42. Mauderli AP, Acosta-Rua A, Vierck CJ. An operant assay of thermal pain in conscious, unrestrained rats. J Neurosci Methods. (2000) 97:19–29. doi: 10.1016/S0165-0270(00)00160-6
43. Negus SS, Vanderah TW, Brandt MR, Bilsky EJ, Becerra L, Borsook D. Preclinical assessment of candidate analgesic drugs: recent advances and future challenges. J Pharmacol Exp Ther. (2006) 319:507–14. doi: 10.1124/jpet.106.106377
44. Harte SE, Meyers JB, Donahue RR, Taylor BK, Morrow TJ. Mechanical conflict system: a novel operant method for the assessment of nociceptive behavior. PLoS ONE. (2016) 11:e0150164. doi: 10.1371/journal.pone.0150164
45. Andrews N, Legg E, Lisak D, Issop Y, Richardson D, Harper S, et al. Spontaneous burrowing behaviour in the rat is reduced by peripheral nerve injury or inflammation associated pain. Eur J Pain. (2012) 16:485–95. doi: 10.1016/j.ejpain.2011.07.012
46. Turner PV, Pang DS, Lofgren JL. A review of pain assessment methods in laboratory rodents. Comp Med. (2019) 69:451–67. doi: 10.30802/AALAS-CM-19-000042
47. Sotocinal SG, Sorge RE, Zaloum A, Tuttle AH, Martin LJ, Wieskopf JS, et al. The Rat Grimace Scale: a partially automated method for quantifying pain in the laboratory rat via facial expressions. Mol Pain. (2011) 7:55. doi: 10.1186/1744-8069-7-55
48. Nadeau S, Filali M, Zhang J, Kerr BJ, Rivest S, Soulet D, et al. Functional recovery after peripheral nerve injury is dependent on the pro-inflammatory cytokines IL-1beta and TNF: implications for neuropathic pain. J Neurosci. (2011) 31:12533–42. doi: 10.1523/JNEUROSCI.2840-11.2011
49. Perrin FE, Lacroix S, Aviles-Trigueros M, David S. Involvement of monocyte chemoattractant protein-1, macrophage inflammatory protein-1alpha and interleukin-1beta in Wallerian degeneration. Brain. (2005) 128:854–66. doi: 10.1093/brain/awh407
50. Bastien D, Lacroix S. Cytokine pathways regulating glial and leukocyte function after spinal cord and peripheral nerve injury. Exp Neurol. (2014) 258:62–77. doi: 10.1016/j.expneurol.2014.04.006
51. Cui JG, Holmin S, Mathiesen T, Meyerson BA, Linderoth B. Possible role of inflammatory mediators in tactile hypersensitivity in rat models of mononeuropathy. Pain. (2000) 88:239–48. doi: 10.1016/S0304-3959(00)00331-6
52. Al-Mazidi S, Alotaibi M, Nedjadi T, Chaudhary A, Alzoghaibi M, Djouhri L. Blocking of cytokines signalling attenuates evoked and spontaneous neuropathic pain behaviours in the paclitaxel rat model of chemotherapy-induced neuropathy. Eur J Pain. (2018) 22:810–21. doi: 10.1002/ejp.1169
53. Ozaktay AC, Kallakuri S, Takebayashi T, Cavanaugh JM, Asik I, DeLeo JA, et al. Effects of interleukin-1 beta, interleukin-6, and tumor necrosis factor on sensitivity of dorsal root ganglion and peripheral receptive fields in rats. Eur Spine J. (2006) 15:1529–37. doi: 10.1007/s00586-005-0058-8
54. Moalem G, Tracey DJ. Immune and inflammatory mechanisms in neuropathic pain. Brain Res Rev. (2006) 51:240–64. doi: 10.1016/j.brainresrev.2005.11.004
55. Zelenka M, Schafers M, Sommer C. Intraneural injection of interleukin-1beta and tumor necrosis factor-alpha into rat sciatic nerve at physiological doses induces signs of neuropathic pain. Pain. (2005) 116:257–63. doi: 10.1016/j.pain.2005.04.018
56. Ferreira SH, Lorenzetti BB, Bristow AF, Poole S. Interleukin-1 beta as a potent hyperalgesic agent antagonized by a tripeptide analogue. Nature. (1988) 334:698–700. doi: 10.1038/334698a0
57. Sommer C, Petrausch S, Lindenlaub T, Toyka KV. Neutralizing antibodies to interleukin 1-receptor reduce pain associated behavior in mice with experimental neuropathy. Neurosci Lett. (1999) 270:25–8. doi: 10.1016/S0304-3940(99)00450-4
58. Wolf G, Gabay E, Tal M, Yirmiya R, Shavit Y. Genetic impairment of interleukin-1 signaling attenuates neuropathic pain, autotomy, and spontaneous ectopic neuronal activity, following nerve injury in mice. Pain. (2006) 120:315–24. doi: 10.1016/j.pain.2005.11.011
59. Gabay E, Wolf G, Shavit Y, Yirmiya R, Tal M. Chronic blockade of interleukin-1 (IL-1) prevents and attenuates neuropathic pain behavior and spontaneous ectopic neuronal activity following nerve injury. Eur J Pain. (2011) 15:242–8. doi: 10.1016/j.ejpain.2010.07.012
60. Honore P, Wade CL, Zhong C, Harris RR, Wu C, Ghayur T, et al. Interleukin-1alphabeta gene-deficient mice show reduced nociceptive sensitivity in models of inflammatory and neuropathic pain but not post-operative pain. Behav Brain Res. (2006) 167:355–64. doi: 10.1016/j.bbr.2005.09.024
61. Stemkowski PL, Smith PA. Long-term IL-1beta exposure causes subpopulation-dependent alterations in rat dorsal root ganglion neuron excitability. J Neurophysiol. (2012) 107:1586–97. doi: 10.1152/jn.00587.2011
62. Stemkowski PL, Noh MC, Chen Y, Smith PA. Increased excitability of medium-sized dorsal root ganglion neurons by prolonged interleukin-1beta exposure is K(+) channel dependent and reversible. J Physiol. (2015) 593:3739–55. doi: 10.1113/JP270905
63. Noh MC, Stemkowski PL, Smith PA. Long-term actions of interleukin-1beta on K(+), Na(+) and Ca(2+) channel currents in small, IB4-positive dorsal root ganglion neurons; possible relevance to the etiology of neuropathic pain. J Neuroimmunol. (2019) 332:198–211. doi: 10.1016/j.jneuroim.2019.05.002
64. Stemkowsi PL, Bukhanova-Schulz N, Baldwin T, Posse de Chaves E, Smith PA. Are Sensory neurons exquisitely sensitive to interleukin 1β. J Neuroimmunol. (2021) 354:577529. doi: 10.1016/j.jneuroim.2021.577529
65. Binshtok AM, Wang H, Zimmermann K, Amaya F, Vardeh D, Shi L, et al. Nociceptors are interleukin-1{beta} sensors. J Neurosci. (2008) 28:14062–73. doi: 10.1523/JNEUROSCI.3795-08.2008
66. Gomez-Nicola D, Valle-Argos B, Suardiaz M, Taylor JS, Nieto-Sampedro M. Role of IL-15 in spinal cord and sciatic nerve after chronic constriction injury: regulation of macrophage and T-cell infiltration. J Neurochem. (2008) 107:1741–52. doi: 10.1111/j.1471-4159.2008.05746.x
67. Warner SC, Nair A, Marpadga R, Chubinskaya S, Doherty M, Valdes AM, et al. IL-15 and IL15RA in osteoarthritis: association with symptoms and protease production, but not structural severity. Front Immunol. (2020) 11:1385. doi: 10.3389/fimmu.2020.01385
68. Kleinschnitz C, Hofstetter HH, Meuth SG, Braeuninger S, Sommer C, Stoll G. T cell infiltration after chronic constriction injury of mouse sciatic nerve is associated with interleukin-17 expression. Exp Neurol. (2006) 200:480–5. doi: 10.1016/j.expneurol.2006.03.014
69. Noma N, Khan J, Chen IF, Markman S, Benoliel R, Hadlaq E, et al. Interleukin-17 levels in rat models of nerve damage and neuropathic pain. Neurosci Lett. (2011) 493:86–91. doi: 10.1016/j.neulet.2011.01.079
70. Kim CF, Moalem-Taylor G. Interleukin-17 contributes to neuroinflammation and neuropathic pain following peripheral nerve injury in mice. J Pain. (2011) 12:370–83. doi: 10.1016/j.jpain.2010.08.003
71. Meng X, Zhang Y, Lao L, Saito R, Li A, Backman CM, et al. Spinal interleukin-17 promotes thermal hyperalgesia and NMDA NR1 phosphorylation in an inflammatory pain rat model. Pain. (2013) 154:294–305. doi: 10.1016/j.pain.2012.10.022
72. Day YJ, Liou JT, Lee CM, Lin YC, Mao CC, Chou AH, et al. Lack of interleukin-17 leads to a modulated micro-environment and amelioration of mechanical hypersensitivity after peripheral nerve injury in mice. Pain. (2014) 155:1293–302. doi: 10.1016/j.pain.2014.04.004
73. Wannamaker W, Davies R, Namchuk M, Pollard J, Ford P, Ku G, et al. (S)-1-((S)-2-{[1-(4-amino-3-chloro-phenyl)-methanoyl]-amino}-3,3-dimethyl-butanoy l)-pyrrolidine-2-carboxylic acid ((2R,3S)-2-ethoxy-5-oxo-tetrahydro-furan-3-yl)-amide (VX-765), an orally available selective interleukin (IL)-converting enzyme/caspase-1 inhibitor, exhibits potent anti-inflammatory activities by inhibiting the release of IL-1beta and IL-18. J Pharmacol Exp Ther. (2007) 321:509–16. doi: 10.1124/jpet.106.111344
74. Sun Y, Zigmond RE. Leukaemia inhibitory factor induced in the sciatic nerve after axotomy is involved in the induction of galanin in sensory neurons. Eur J Neurosci. (1996) 8:2213–20. doi: 10.1111/j.1460-9568.1996.tb00744.x
75. Spofford CM, Mohan S, Kang S, Jang JH, Brennan TJ. Evaluation of leukemia inhibitory factor (LIF) in a rat model of postoperative pain. J Pain. (2011) 12:819–32. doi: 10.1016/j.jpain.2011.02.351
76. Thompson SW, Majithia AA Leukemia inhibitory factor induces sympathetic sprouting in intact dorsal root ganglia in the adult rat in vivo. J Physiol. (1998) 506(Pt. 3):809–16. doi: 10.1111/j.1469-7793.1998.809bv.x
77. Cheng CF, Cheng JK, Chen CY, Rau RH, Chang YC, Tsaur ML. Nerve growth factor-induced synapse-like structures in contralateral sensory ganglia contribute to chronic mirror-image pain. Pain. (2015) 156:2295–309. doi: 10.1097/j.pain.0000000000000280
78. Smithson LJ, Krol KM, Kawaja MD. Neuronal degeneration associated with sympathosensory plexuses in the trigeminal ganglia of aged mice that overexpress nerve growth factor. Neurobiol Aging. (2014) 35:2812–21. doi: 10.1016/j.neurobiolaging.2014.06.014
79. Engert S, Wendland JR, Schwab A, Petersen M. Leukemia inhibitory factor differentially regulates capsaicin and heat sensitivity in cultured rat dorsal root ganglion neurons. Neuropeptides. (2008) 42:193–7. doi: 10.1016/j.npep.2007.12.005
80. Wagner R, Myers RR. Schwann cells produce tumor necrosis factor alpha: expression in injured and non-injured nerves. Neuroscience. (1996) 73:625–9. doi: 10.1016/0306-4522(96)00127-3
81. George A, Schmidt C, Weishaupt A, Toyka KV, Sommer C. Serial determination of tumor necrosis factor-alpha content in rat sciatic nerve after chronic constriction injury. Exp Neurol. (1999) 160:124–32. doi: 10.1006/exnr.1999.7193
82. Schafers M, Geis C, Svensson CI, Luo ZD, Sommer C. Selective increase of tumour necrosis factor-alpha in injured and spared myelinated primary afferents after chronic constrictive injury of rat sciatic nerve. Eur J Neurosci. (2003) 17:791–804. doi: 10.1046/j.1460-9568.2003.02504.x
83. Schafers M, Svensson CI, Sommer C, Sorkin LS. Tumor necrosis factor-alpha induces mechanical allodynia after spinal nerve ligation by activation of p38 MAPK in primary sensory neurons. J Neurosci. (2003) 23:2517–21. doi: 10.1523/JNEUROSCI.23-07-02517.2003
84. Andrade P, Hoogland G, Del Rosario JS, Steinbusch HW, Visser-Vandewalle V, Daemen MA. Tumor necrosis factor-alpha inhibitors alleviation of experimentally induced neuropathic pain is associated with modulation of TNF receptor expression. J Neurosci Res. (2014) 92:1490–8. doi: 10.1002/jnr.23432
85. George A, Marziniak M, Schafers M, Toyka KV, Sommer C. Thalidomide treatment in chronic constrictive neuropathy decreases endoneurial tumor necrosis factor-alpha, increases interleukin-10 and has long-term effects on spinal cord dorsal horn met-enkephalin. Pain. (2000) 88:267–75. doi: 10.1016/S0304-3959(00)00333-X
86. Sommer C, Schmidt C, George A, Toyka KV. A metalloprotease-inhibitor reduces pain associated behavior in mice with experimental neuropathy. Neurosci Lett. (1997) 237:45–8. doi: 10.1016/S0304-3940(97)00813-6
87. Sommer C, Schmidt C, George A. Hyperalgesia in experimental neuropathy is dependent on the TNF receptor 1. Exp Neurol. (1998) 151:138–42. doi: 10.1006/exnr.1998.6797
88. Sorkin LS, Xiao WH, Wagner R, Myers RR. Tumour necrosis factor-alpha induces ectopic activity in nociceptive primary afferent fibres. Neuroscience. (1997) 81:255–62. doi: 10.1016/S0306-4522(97)00147-4
89. de Macedo FHP, Aires RD, Fonseca EG, Ferreira RCM, Machado DPD, Chen L, et al. TNF-alpha mediated upregulation of NaV1.7 currents in rat dorsal root ganglion neurons is independent of CRMP2 SUMOylation. Mol Brain. (2019) 12:117. doi: 10.1186/s13041-019-0538-0
90. Zhang JM, Li H, Liu B, Brull SJ. Acute topical application of tumor necrosis factor alpha evokes protein kinase A-dependent responses in rat sensory neurons. J Neurophysiol. (2002) 88:1387–92. doi: 10.1152/jn.2002.88.3.1387
91. Gudes S, Barkai O, Caspi Y, Katz B, Lev S, Binshtok AM. The role of slow and persistent TTX-resistant sodium currents in acute tumor necrosis factor-alpha-mediated increase in nociceptors excitability. J Neurophysiol. (2015) 113:601–19. doi: 10.1152/jn.00652.2014
92. Chen X, Pang RP, Shen KF, Zimmermann M, Xin WJ, Li YY, et al. TNF-alpha enhances the currents of voltage gated sodium channels in uninjured dorsal root ganglion neurons following motor nerve injury. Exp Neurol. (2011) 227:279–86. doi: 10.1016/j.expneurol.2010.11.017
93. Ma W, Eisenach JC. Morphological and pharmacological evidence for the role of peripheral prostaglandins in the pathogenesis of neuropathic pain. Eur J Neurosci. (2002) 15:1037–47. doi: 10.1046/j.1460-9568.2002.01940.x
94. Ma W, Eisenach JC. Cyclooxygenase 2 in infiltrating inflammatory cells in injured nerve is universally up-regulated following various types of peripheral nerve injury. Neuroscience. (2003) 121:691–704. doi: 10.1016/S0306-4522(03)00495-0
95. Sisignano M, Park CK, Angioni C, Zhang DD, von HC, Cobos EJ, et al. 5,6-EET is released upon neuronal activity and induces mechanical pain hypersensitivity via TRPA1 on central afferent terminals. J Neurosci. (2012) 32:6364–72. doi: 10.1523/JNEUROSCI.5793-11.2012
96. Michaelis M, Vogel C, Blenk KH, Arnarson A, Janig W. Inflammatory mediators sensitize acutely axotomized nerve fibers to mechanical stimulation in the rat. J Neurosci. (1998) 18:7581–7. doi: 10.1523/JNEUROSCI.18-18-07581.1998
97. Gold MS, Reichling DB, Shuster MJ, Levine JD. Hyperalgesic agents increase a tetrodotoxin-resistant Na+ current in nociceptors. Proc Natl Acad Sci USA. (1996) 93:1108–12. doi: 10.1073/pnas.93.3.1108
98. Ma W, Quirion R. Targeting invading macrophage-derived PGE2, IL-6 and calcitonin gene-related peptide in injured nerve to treat neuropathic pain. Expert Opin Ther Targets. (2006) 10:533–46. doi: 10.1517/14728222.10.4.533
99. Herzberg U, Eliav E, Dorsey JM, Gracely RH, Kopin IJ. NGF involvement in pain induced by chronic constriction injury of the rat sciatic nerve. Neuroreport. (1997) 8:1613–8. doi: 10.1097/00001756-199705060-00012
100. Zhou XF, Deng YS, Xian CJ, Zhong JH. Neurotrophins from dorsal root ganglia trigger allodynia after spinal nerve injury in rats. Eur J Neurosci. (2000) 12:100–5. doi: 10.1046/j.1460-9568.2000.00884.x
101. Fjell J, Cummins TR, Davis BM, Albers KM, Fried K, Waxman SG, et al. Sodium channel expression in NGF-overexpressing transgenic mice. J Neurosci Res. (1999) 57:39–47.
102. Theodosiou M, Rush RA, Zhou XF, Hu D, Walker JS, Tracey DJ. Hyperalgesia due to nerve damage: role of nerve growth factor. Pain. (1999) 81:245–55. doi: 10.1016/S0304-3959(99)00018-4
103. Pezet S, McMahon SB. Neurotrophins: mediators and modulators of pain. Ann Rev Neurosci. (2006) 29:507–38. doi: 10.1146/annurev.neuro.29.051605.112929
104. Dib-Hajj SD, Black JA, Cummins TR, Kenney AM, Kocsis JD, Waxman SG. Rescue of alpha-SNS sodium channel expression in small dorsal root ganglion neurons after axotomy by nerve growth factor in vivo. J Neurophysiol. (1998) 79:2668–76. doi: 10.1152/jn.1998.79.5.2668
105. Inoue A, Ikoma K, Morioka N, Kumagai K, Hashimoto T, Hide I, et al. Interleukin-1beta induces substance P release from primary afferent neurons through the cyclooxygenase-2 system. J Neurochem. (1999) 73:2206–13. doi: 10.1046/j.1471-4159.1999.02206.x
106. Noguchi K, Kawai Y, Fukuoka T, Senba E, Miki K. Substance P induced by peripheral nerve injury in primary afferent sensory neurons and its effect on dorsal column nucleus neurons. J Neurosci. (1995) 15:7633–43. doi: 10.1523/JNEUROSCI.15-11-07633.1995
107. Chen W, Marvizon JC. Neurokinin 1 receptor activation in the rat spinal cord maintains latent sensitization, a model of inflammatory and neuropathic chronic pain. Neuropharmacology. (2020) 177:108253. doi: 10.1016/j.neuropharm.2020.108253
108. Cahill CM, Coderre TJ. Attenuation of hyperalgesia in a rat model of neuropathic pain after intrathecal pre- or post-treatment with a neurokinin-1 antagonist. Pain. (2002) 95:277–85. doi: 10.1016/S0304-3959(01)00410-9
109. Guo TZ, Wei T, Shi X, Li WW, Hou S, Wang L, et al. Neuropeptide deficient mice have attenuated nociceptive, vascular, and inflammatory changes in a tibia fracture model of complex regional pain syndrome. Mol Pain. (2012) 8:85. doi: 10.1186/1744-8069-8-85
110. Dray A, Pinnock RD. Effects of substance P on adult rat sensory ganglion neurones in vitro. Neurosci Lett. (1982) 33:61–6. doi: 10.1016/0304-3940(82)90130-6
111. Abdulla FA, Smith PA. Axotomy alters the response of dorsal root ganglion neurons to substance P. Soc Neurosci Abs. (1998) 24:2089.
112. Sculptoreanu A, Artim DE, de Groat WC. Neurokinins inhibit low threshold inactivating K+ currents in capsaicin responsive DRG neurons. Exp Neurol. (2009) 219:562–73. doi: 10.1016/j.expneurol.2009.07.016
113. Subang MC, Richardson PM. Influence of injury and cytokines on synthesis of monocyte chemoattractant protein-1 mRNA in peripheral nervous tissue. Eur J Neurosci. (2001) 13:521–8. doi: 10.1046/j.1460-9568.2001.01425.x
114. Toews AD, Barrett C, Morell P. Monocyte chemoattractant protein 1 is responsible for macrophage recruitment following injury to sciatic nerve. J Neurosci Res. (1998) 53:260–7.
115. White FA, Sun J, Waters SM, Ma C, Ren D, Ripsch M, et al. Excitatory monocyte chemoattractant protein-1 signaling is up-regulated in sensory neurons after chronic compression of the dorsal root ganglion. Proc Natl Acad Sci USA. (2005) 102:14092–7. doi: 10.1073/pnas.0503496102
116. White FA, Wilson NM. Chemokines as pain mediators and modulators. Curr Opin Anaesthesiol. (2008) 21:580–5. doi: 10.1097/ACO.0b013e32830eb69d
117. Jung H, Bhangoo S, Banisadr G, Freitag C, Ren D, White FA, et al. Visualization of chemokine receptor activation in transgenic mice reveals peripheral activation of CCR2 receptors in states of neuropathic pain. J Neurosci. (2009) 29:8051–62. doi: 10.1523/JNEUROSCI.0485-09.2009
118. White FA, Jung H, Miller RJ. Chemokines and the pathophysiology of neuropathic pain. Proc Natl Acad Sci USA. (2007) 104:20151–8. doi: 10.1073/pnas.0709250104
119. Sun JH, Yang B, Donnelly DF, Ma C, LaMotte RH. MCP-1 enhances excitability of nociceptive neurons in chronically compressed dorsal root ganglia. J Neurophysiol. (2006) 96:2189–99. doi: 10.1152/jn.00222.2006
120. Silva RL, Lopes AH, Guimaraes RM, Cunha TM. CXCL1/CXCR2 signaling in pathological pain: Role in peripheral and central sensitization. Neurobiol Dis. (2017) 105:109–16. doi: 10.1016/j.nbd.2017.06.001
121. Zhang ZJ, Cao DL, Zhang X, Ji RR, Gao YJ. Chemokine contribution to neuropathic pain: respective induction of CXCL1 and CXCR2 in spinal cord astrocytes and neurons. Pain. (2013) 154:2185–97. doi: 10.1016/j.pain.2013.07.002
122. Qin X, Wan Y, Wang X. CCL2 and CXCL1 trigger calcitonin gene-related peptide release by exciting primary nociceptive neurons. J Neurosci Res. (2005) 82:51–62. doi: 10.1002/jnr.20612
123. Yu Y, Huang X, Di Y, Qu L, Fan N. Effect of CXCL12/CXCR4 signaling on neuropathic pain after chronic compression of dorsal root ganglion. Sci Rep. (2017) 7:5707. doi: 10.1038/s41598-017-05954-1
124. Wang JG, Strong JA, Xie W, Yang RH, Coyle DE, Wick DM, et al. The chemokine CXCL1/growth related oncogene increases sodium currents and neuronal excitability in small diameter sensory neurons. Mol Pain. (2008) 4:38. doi: 10.1186/1744-8069-4-38
125. Jayaraj ND, Bhattacharyya BJ, Belmadani AA, Ren D, Rathwell CA, Hackelberg S, et al. Reducing CXCR4-mediated nociceptor hyperexcitability reverses painful diabetic neuropathy. J Clin Invest. (2018) 128:2205–2225. doi: 10.1172/JCI92117
126. Luo X, Tai WL, Sun L, Qiu Q, Xia Z, Chung SK, et al. Central administration of C-X-C chemokine receptor type 4 antagonist alleviates the development and maintenance of peripheral neuropathic pain in mice. PLoS ONE. (2014) 9:e104860. doi: 10.1371/journal.pone.0104860
127. Kaur G, Singh N, Jaggi AS. Mast cells in neuropathic pain: an increasing spectrum of their involvement in pathophysiology. Rev Neurosci. (2017) 28:759–66. doi: 10.1515/revneuro-2017-0007
128. Obara I, Telezhkin V, Alrashdi I, Chazot PL. Histamine, histamine receptors, and neuropathic pain relief. Br J Pharmacol. (2020) 177:580–99. doi: 10.1111/bph.14696
129. Khalilzadeh E, Azarpey F, Hazrati R, Vafaei SG. Evaluation of different classes of histamine H1 and H2 receptor antagonist effects on neuropathic nociceptive behavior following tibial nerve transection in rats. Eur J Pharmacol. (2018) 834:221–9. doi: 10.1016/j.ejphar.2018.07.011
130. Yue JX, Wang RR, Yu J, Tang YY, Hou WW, Lou GD, et al. Histamine upregulates Nav1.8 expression in primary afferent neurons via H2 receptors: involvement in neuropathic pain. CNS Neurosci Ther. (2014) 20:883–92. doi: 10.1111/cns.12305
131. Liu S, Liu YP, Huang ZJ, Zhang YK, Song AA, Ma PC, et al. Wnt/Ryk signaling contributes to neuropathic pain by regulating sensory neuron excitability and spinal synaptic plasticity in rats. Pain. (2015) 156:2572–84. doi: 10.1097/j.pain.0000000000000366
132. Shi Y, Yuan S, Li B, Wang J, Carlton SM, Chung K, et al. Regulation of Wnt signaling by nociceptive input in animal models. Mol Pain. (2012) 8:47. doi: 10.1186/1744-8069-8-47
133. Simonetti M, Agarwal N, Stosser S, Bali KK, Karaulanov E, Kamble R, et al. Wnt-Fzd signaling sensitizes peripheral sensory neurons via distinct noncanonical pathways. Neuron. (2014) 83:104–21. doi: 10.1016/j.neuron.2014.05.037
134. Simonetti M, Kuner R. Spinal Wnt5a plays a key role in spinal dendritic spine remodeling in neuropathic and inflammatory pain models and in the proalgesic effects of peripheral Wnt3a. J Neurosci. (2020) 40:6664. doi: 10.1523/JNEUROSCI.2942-19.2020
135. van Vliet AC, Lee J, van der Poel M, Mason MRJ, Noordermeer JN, Fradkin LG, et al. Coordinated changes in the expression of Wnt pathway genes following human and rat peripheral nerve injury. PLoS ONE. (2021) 16:e0249748. doi: 10.1371/journal.pone.0249748
136. Lim H, Lee H, Noh K, Lee SJ. IKK/NF-kappaB-dependent satellite glia activation induces spinal cord microglia activation and neuropathic pain after nerve injury. Pain. (2017) 158:1666–77. doi: 10.1097/j.pain.0000000000000959
137. Yu X, Basbaum A, Guan Z. Contribution of colony-stimulating factor 1 to neuropathic pain. PAIN Rep. (2021) 6:e883. doi: 10.1097/PR9.0000000000000883
138. Okubo M, Yamanaka H, Kobayashi K, Dai Y, Kanda H, Yagi H, et al. Macrophage-colony stimulating factor derived from injured primary afferent induces proliferation of spinal microglia and neuropathic pain in rats. PLoS ONE. (2016) 11:e0153375. doi: 10.1371/journal.pone.0153375
139. Guan Z, Kuhn JA, Wang X, Colquitt B, Solorzano C, Vaman S, et al. Injured sensory neuron-derived CSF1 induces microglial proliferation and DAP12-dependent pain. Nat Neurosci. (2016) 19:94–101. doi: 10.1038/nn.4189
140. Gushchina S, Pryce G, Yip PK, Wu D, Pallier P, Giovannoni G, et al. Increased expression of colony-stimulating factor-1 in mouse spinal cord with experimental autoimmune encephalomyelitis correlates with microglial activation and neuronal loss. Glia. (2018) 66:2108–25. doi: 10.1002/glia.23464
141. Yu X, Liu H, Hamel KA, Morvan MG, Yu S, Leff J, et al. Dorsal root ganglion macrophages contribute to both the initiation and persistence of neuropathic pain. Nat Commun. (2020) 11:264. doi: 10.1038/s41467-019-13839-2
142. Noh MC, Mikler B, Joy T, Smith PA. Time course of inflammation in dorsal root ganglia correlates with differential reversibility of mechanical allodynia. Neuroscience. (2020) 428:199–216. doi: 10.1016/j.neuroscience.2019.12.040
143. Boakye PA, Rancic V, Whitlock KH, Simmons D, Longo FM, Ballanyi K, et al. Receptor dependence of BDNF actions in superficial dorsal horn: relation to central sensitization and actions of macrophage colony stimulating factor 1. J Neurophysiol. (2019) 121:2308–22. doi: 10.1152/jn.00839.2018
144. Biber K, Tsuda M, Tozaki-Saitoh H, Tsukamoto K, Toyomitsu E, Masuda T, et al. Neuronal CCL21 up-regulates microglia P2X4 expression and initiates neuropathic pain development. EMBO J. (2011) 30:1864–1873. doi: 10.1038/emboj.2011.89
145. de Jong EK, Vinet J, Stanulovic VS, Meijer M, Wesseling E, Sjollema K, et al. Expression, transport, and axonal sorting of neuronal CCL21 in large dense-core vesicles. FASEB J. (2008) 22:4136–45. doi: 10.1096/fj.07-101907
146. Biber K, Boddeke E. Neuronal CC chemokines: the distinct roles of CCL21 and CCL2 in neuropathic pain. Front Cell Neurosci. (2014) 8:210. doi: 10.3389/fncel.2014.00210
147. Piotrowska A, Rojewska E, Pawlik K, Kreiner G, Ciechanowska A, Makuch W, et al. Pharmacological blockade of CXCR3 by (+/-)-NBI-74330 reduces neuropathic pain and enhances opioid effectiveness - Evidence from in vivo and in vitro studies. Biochim Biophys Acta Mol Basis Dis. (2018) 1864:3418–37. doi: 10.1016/j.bbadis.2018.07.032
148. Honjoh K, Nakajima H, Hirai T, Watanabe S, Matsumine A. Relationship of inflammatory cytokines from M1-type microglia/macrophages at the injured site and lumbar enlargement with neuropathic pain after spinal cord injury in the CCL21 knockout (plt) mouse. Front Cell Neurosci. (2019) 13:525. doi: 10.3389/fncel.2019.00525
149. Schmitz K, Pickert G, Wijnvoord N, Haussler A, Tegeder I. Dichotomy of CCL21 and CXCR3 in nerve injury-evoked and autoimmunity-evoked hyperalgesia. Brain Behav Immun. (2013) 32:186–200. doi: 10.1016/j.bbi.2013.04.011
150. Coull JA, Beggs S, Boudreau D, Boivin D, Tsuda M, Inoue K, et al. BDNF from microglia causes the shift in neuronal anion gradient underlying neuropathic pain. Nature. (2005) 438:1017–21. doi: 10.1038/nature04223
151. Ulmann L, Hatcher JP, Hughes JP, Chaumont S, Green PJ, Conquet F, et al. Up-regulation of P2X4 receptors in spinal microglia after peripheral nerve injury mediates BDNF release and neuropathic pain. J Neurosci. (2008) 28:11263–8. doi: 10.1523/JNEUROSCI.2308-08.2008
152. Miletic G, Miletic V. Increases in the concentration of brain derived neurotrophic factor in the lumbar spinal dorsal horn are associated with pain behavior following chronic constriction injury in rats. Neurosci Lett. (2002) 319:137–40. doi: 10.1016/S0304-3940(01)02576-9
153. Yajima Y, Narita M, Usui A, Kaneko C, Miyatake M, Narita M, et al. Direct evidence for the involvement of brain-derived neurotrophic factor in the development of a neuropathic pain-like state in mice. J Neurochem. (2005) 93:584–94. doi: 10.1111/j.1471-4159.2005.03045.x
154. Smith PA. BDNF: no gain without pain? Neuroscience. (2014) 283:107–23. doi: 10.1016/j.neuroscience.2014.05.044
155. Yajima Y, Narita M, Narita M, Matsumoto N, Suzuki T. Involvement of a spinal brain-derived neurotrophic factor/full-length TrkB pathway in the development of nerve injury-induced thermal hyperalgesia in mice. Brain Res. (2002) 958:338–46. doi: 10.1016/S0006-8993(02)03666-1
156. Biggs JE, Lu VB, Stebbing MJ, Balasubramanyan S, Smith PA. Is BDNF sufficient for information transfer between microglia and dorsal horn neurons during the onset of central sensitization? Mol Pain. (2010) 6:44. doi: 10.1186/1744-8069-6-44
157. Lu VB, Biggs JE, Stebbing MJ, Balasubramanyan S, Todd KG, Lai AY, et al. BDNF drives the changes in excitatory synaptic transmission in the rat superficial dorsal horn that follow sciatic nerve injury. J Physiol. (2009) 587:1013–32. doi: 10.1113/jphysiol.2008.166306
158. Lu VB, Colmers WF, Smith PA. Long-term effects of brain-derived neurotrophic factor on the frequency of inhibitory synaptic events in the rat superficial dorsal horn. Neuroscience. (2009) 161:1135–43. doi: 10.1016/j.neuroscience.2009.04.030
159. Lu VB, Colmers WF, Smith PA. Long-term actions of BDNF on inhibitory synaptic transmission in identified neurons of the rat substantia gelatinosa. J Neurophysiol. (2012) 108:441–52. doi: 10.1152/jn.00457.2011
160. Garraway SM, Anderson AJ, Mendell LM. BDNF-induced facilitation of afferent-evoked responses in lamina II neurons is reduced after neonatal spinal cord contusion injury. J Neurophysiol. (2005) 94:1798–804. doi: 10.1152/jn.00179.2005
161. Bardoni R, Ghirri A, Salio C, Prandini M, Merighi A. BDNF-mediated modulation of GABA and glycine release in dorsal horn lamina II from postnatal rats. Dev Neurobiol. (2007) 67:960–75. doi: 10.1002/dneu.20401
162. Garraway SM, Mendell LM. Spinal cord transection enhances afferent-evoked inhibition in lamina II neurons and abolishes BDNF-induced facilitation of their sensory input. J Neurotrauma. (2007) 24:379–90. doi: 10.1089/neu.2006.0115
163. Alles SRA, Odem MA, Lu VB, Cassidy RM, Smith PA. Chronic BDNF simultaneously inhibits and unmasks superficial dorsal horn neuronal activity. Sci Rep. (2021) 11:2249. doi: 10.1038/s41598-021-81269-6
164. Ferrini F, De Koninck. Y. Microglia control neuronal network excitability via BDNF signalling. Neural Plast. (2013) 2013:429815. doi: 10.1155/2013/429815
165. Hildebrand ME, Xu J, Dedek A, Li Y, Sengar AS, Beggs S, et al. Potentiation of synaptic GluN2B NMDAR currents by Fyn kinase is gated through BDNF-mediated disinhibition in spinal pain processing. Cell Rep. (2016) 17:2753–65. doi: 10.1016/j.celrep.2016.11.024
166. Mousseau M, Burma NE, Lee KY, Leduc-Pessah H, Kwok CHT, Reid AR, et al. Microglial pannexin-1 channel activation is a spinal determinant of joint pain. Sci Adv. (2018) 4:eaas9846. doi: 10.1126/sciadv.aas9846
167. Clark AK, Gruber-Schoffnegger D, Drdla-Schutting R, Gerhold KJ, Malcangio M, Sandkuhler J. Selective activation of microglia facilitates synaptic strength. J Neurosci. (2015) 35:4552–70. doi: 10.1523/JNEUROSCI.2061-14.2015
168. Kohno K, Tsuda M. Role of microglia and P2X4 receptors in chronic pain. PAIN Rep. (2021) 6:e864. doi: 10.1097/PR9.0000000000000864
169. Ozaktay AC, Cavanaugh JM, Asik I, DeLeo JA, Weinstein JN. Dorsal root sensitivity to interleukin-1 beta, interleukin-6 and tumor necrosis factor in rats. Eur Spine J. (2002) 11:467–75. doi: 10.1007/s00586-002-0430-x
170. Kawasaki Y, Xu ZZ, Wang X, Park JY, Zhuang ZY, Tan PH, et al. Distinct roles of matrix metalloproteases in the early- and late-phase development of neuropathic pain. Nat Med. (2008) 14:331–6. doi: 10.1038/nm1723
171. Webster CI, Hatcher J, Burrell M, Thom G, Thornton P, Gurrell I, et al. Enhanced delivery of IL-1 receptor antagonist to the central nervous system as a novel anti-transferrin receptor-IL-1RA fusion reverses neuropathic mechanical hypersensitivity. Pain. (2017) 158:660–8. doi: 10.1097/j.pain.0000000000000810
172. Honore P, Wade CL, Zhong C, Harris RR, Wu C, Ghayur T, et al. Analgesic profile of il1- knockout mice in inflammatory and neuropathic pain models. In: 2004 Abstract Viewer/Itinerary Planner. Washington, DC: Society for Neuroscience, OnlineProgram No. 173.1 (2004).
173. Yamashita T, Kamikaseda S, Tanaka A, Tosaki-Saitoh H, Caaveiro J, Inoue K, et al. New inhibitory effects of cilnidipine on microglial P2X7 receptors and IL-1 beta release: an involvement in its alleviating effect on neuropathic pain. Cells. (2021) 10:434. doi: 10.3390/cells10020434
174. Mika J, Korostynski M, Kaminska D, Wawrzczak-Bargiela A, Osikowicz M, Makuch W, et al. Interleukin-1 alpha has antiallodynic and antihyperalgesic activities in a rat neuropathic pain model. Pain. (2008) 138:587–97. doi: 10.1016/j.pain.2008.02.015
175. Kawasaki Y, Zhang L, Cheng JK, Ji RR. Cytokine mechanisms of central sensitization: distinct and overlapping role of interleukin-1beta, interleukin-6, and tumor necrosis factor-alpha in regulating synaptic and neuronal activity in the superficial spinal cord. J Neurosci. (2008) 28:5189–94. doi: 10.1523/JNEUROSCI.3338-07.2008
176. Gustafson-Vickers SL, Lu VB, Lai AY, Todd KG, Ballanyi K, Smith PA. Long-term actions of interleukin-1beta on delay and tonic firing neurons in rat superficial dorsal horn and their relevance to central sensitization. Mol Pain. (2008) 4:63. doi: 10.1186/1744-8069-4-63
177. Kawasaki Y, Kohno T, Zhuang ZY, Brenner GJ, Wang H, Van Der Meer C, et al. Ionotropic and metabotropic receptors, protein kinase A, protein kinase C, and Src contribute to C-fiber-induced ERK activation and cAMP response element-binding protein phosphorylation in dorsal horn neurons, leading to central sensitization. J Neurosci. (2004) 24:8310–21. doi: 10.1523/JNEUROSCI.2396-04.2004
178. Liu T, Jiang CY, Fujita T, Luo SW, Kumamoto E. Enhancement by interleukin-1beta of AMPA and NMDA receptor-mediated currents in adult rat spinal superficial dorsal horn neurons. Mol Pain. (2013) 9:16. doi: 10.1186/1744-8069-9-16
179. Yan X, Li F, Maixner DW, Yadav R, Gao M, Ali MW, et al. Interleukin-1beta released by microglia initiates the enhanced glutamatergic activity in the spinal dorsal horn during paclitaxel-associated acute pain syndrome. Glia. (2019) 67:482–97. doi: 10.1002/glia.23557
180. Kanda H, Kobayashi K, Yamanaka H, Okubo M, Noguchi K. Microglial TNFalpha induces COX2 and PGI2 synthase expression in spinal endothelial cells during neuropathic pain. eNeuro. (2017) 4:ENEURO.0064-17.2017. doi: 10.1523/ENEURO.0064-17.2017
181. Cuellar JM, Montesano PX, Carstens E. Role of TNF-alpha in sensitization of nociceptive dorsal horn neurons induced by application of nucleus pulposus to L5 dorsal root ganglion in rats. Pain. (2004) 110:578–87. doi: 10.1016/j.pain.2004.03.029
182. Zhang H, Nei H, Dougherty PM. A p38 mitogen-activated protein kinase-dependent mechanism of disinhibition in spinal synaptic transmission induced by tumor necrosis factor-alpha. J Neurosci. (2010) 30:12844–55. doi: 10.1523/JNEUROSCI.2437-10.2010
183. Gruber-Schoffnegger D, Drdla-Schutting R, Honigsperger C, Wunderbaldinger G, Gassner M, Sandkuhler J. Induction of Thermal Hyperalgesia and Synaptic Long-Term Potentiation in the Spinal Cord Lamina I by TNF-alpha and IL-1beta is Mediated by Glial Cells. J Neurosci. (2013) 33:6540–51. doi: 10.1523/JNEUROSCI.5087-12.2013
184. Czeschik JC, Hagenacker T, Schafers M, Busselberg D. TNF-alpha differentially modulates ion channels of nociceptive neurons. Neurosci Lett. (2008) 434:293–8. doi: 10.1016/j.neulet.2008.01.070
185. Taylor AM, Castonguay A, Taylor AJ, Murphy NP, Ghogha A, Cook C, et al. Microglia disrupt mesolimbic reward circuitry in chronic pain. J Neurosci. (2015) 35:8442–50. doi: 10.1523/JNEUROSCI.4036-14.2015
186. Taylor AM, Mehrabani S, Liu S, Taylor AJ, Cahill CM. Topography of microglial activation in sensory- and affect-related brain regions in chronic pain. J Neurosci Res. (2016) 95:1330–5. doi: 10.1002/jnr.23883
187. Goncalves Dos SG, Delay L, Yaksh TL, Corr M. Neuraxial cytokines in pain states. Front Immunol. (2019) 10:3061. doi: 10.3389/fimmu.2019.03061
188. Tajerian M, Alvarado S, Millecamps M, Vachon P, Crosby C, Bushnell MC, et al. Peripheral nerve injury is associated with chronic, reversible changes in global DNA methylation in the mouse prefrontal cortex. PLoS ONE. (2013) 8:e55259. doi: 10.1371/journal.pone.0055259
189. Ramer MS, French GD, Bisby MA. Wallerian degeneration is required for both neuropathic pain and sympathetic sprouting into the DRG. Pain. (1997) 72:71–8. doi: 10.1016/S0304-3959(97)00019-5
190. Shamash S, Reichert F, Rotshenker S. The cytokine network of Wallerian degeneration: tumor necrosis factor-alpha, interleukin-1alpha, and interleukin-1beta. J Neurosci. (2002) 22:3052–60. doi: 10.1523/JNEUROSCI.22-08-03052.2002
191. Campana WM. Schwann cells: activated peripheral glia and their role in neuropathic pain. Brain Behav Immun. (2007) 21:522–7. doi: 10.1016/j.bbi.2006.12.008
192. Lee HK, Seo IA, Suh DJ, Hong JI, Yoo YH, Park HT. Interleukin-6 is required for the early induction of glial fibrillary acidic protein in Schwann cells during Wallerian degeneration. J Neurochem. (2009) 108:776–86. doi: 10.1111/j.1471-4159.2008.05826.x
193. McMahon SB, Cafferty WB, Marchand F. Immune and glial cell factors as pain mediators and modulators. Exp Neurol. (2005) 192:444–62. doi: 10.1016/j.expneurol.2004.11.001
194. DeLeo JA, Sorkin LS, Watkins LR. Immune and Glial Regulation of Pain. Seattle, WA: IASP Press (2007).
195. Manjavachi MN, Costa R, Quintao NL, Calixto JB. The role of keratinocyte-derived chemokine (KC) on hyperalgesia caused by peripheral nerve injury in mice. Neuropharmacology. (2014) 79:17–27. doi: 10.1016/j.neuropharm.2013.10.026
196. Radtke C, Vogt PM, Devor M, Kocsis JD. Keratinocytes acting on injured afferents induce extreme neuronal hyperexcitability and chronic pain. Pain. (2010) 148:94–102. doi: 10.1016/j.pain.2009.10.014
197. Austin PJ, Moalem-Taylor G. The neuro-immune balance in neuropathic pain: involvement of inflammatory immune cells, immune-like glial cells and cytokines. J Neuroimmunology. (2010) 229:26–50. doi: 10.1016/j.jneuroim.2010.08.013
198. Thompson SW, Dray A, Urban L. Leukemia inhibitory factor induces mechanical allodynia but not thermal hyperalgesia in the juvenile rat. Neuroscience. (1996) 71:1091–4. doi: 10.1016/0306-4522(95)00537-4
199. Zhang YK, Huang ZJ, Liu S, Liu YP, Song AA, Song XJ. WNT signaling underlies the pathogenesis of neuropathic pain in rodents. J Clin Invest. (2013) 123:2268–86. doi: 10.1172/JCI65364
200. Wagner R, Myers RR. Endoneurial injection of TNF-alpha produces neuropathic pain behaviors. Neuroreport. (1996) 7:2897–901. doi: 10.1097/00001756-199611250-00018
201. George A, Buehl A, Sommer C. Wallerian degeneration after crush injury of rat sciatic nerve increases endo- and epineurial tumor necrosis factor-alpha protein. Neurosci Lett. (2004) 372:215–9. doi: 10.1016/j.neulet.2004.09.075
202. Sommer C, Kress M. Recent findings on how proinflammatory cytokines cause pain: peripheral mechanisms in inflammatory and neuropathic hyperalgesia. Neurosci Lett. (2004) 361:184–7. doi: 10.1016/j.neulet.2003.12.007
203. Leung L, Cahill CM. TNF-alpha and neuropathic pain–a review. J Neuroinflamm. (2010) 7:27. doi: 10.1186/1742-2094-7-27
204. Ma C, Shu Y, Zheng Z, Chen Y, Yao H, Greenquist KW, et al. Similar electrophysiological changes in axotomized and neighboring intact dorsal root ganglion neurons. J Neurophysiol. (2003) 89:1588–602. doi: 10.1152/jn.00855.2002
205. Obata K, Yamanaka H, Fukuoka T, Yi D, Tokunaga A, Hashimoto N, et al. Contribution of injured and uninjured dorsal root ganglion neurons to pain behavior and the changes in gene expression following chronic constriction injury of the sciatic nerve in rats. Pain. (2003) 101:65–77. doi: 10.1016/S0304-3959(02)00296-8
206. Schafers M, Lee DH, Brors D, Yaksh TL, Sorkin LS. Increased sensitivity of injured and adjacent uninjured rat primary sensory neurons to exogenous tumor necrosis factor-alpha after spinal nerve ligation. J Neurosci. (2003) 23:3028–38. doi: 10.1523/JNEUROSCI.23-07-03028.2003
207. Hanani M, Spray DC. Emerging importance of satellite glia in nervous system function and dysfunction. Nat Rev Neurosci. (2020) 21:485–98. doi: 10.1038/s41583-020-0333-z
208. Yuan Q, Liu X, Xian YF, Yao M, Zhang X, Huang P, et al. Satellite glia activation in dorsal root ganglion contributes to mechanical allodynia after selective motor fiber injury in adult rats. Biomed Pharmacother. (2020) 127:110187. doi: 10.1016/j.biopha.2020.110187
209. Dubovy P, Klusakova I, Svizenska I, Brazda V. Satellite glial cells express IL-6 and corresponding signal-transducing receptors in the dorsal root ganglia of rat neuropathic pain model. Neuron Glia Biol. (2010) 6:73–83. doi: 10.1017/S1740925X10000074
210. Silva CEA, Guimarães RMB, Cunha TMC. Sensory neuronΓçôassociated macrophages as novel modulators of neuropathic pain. PAIN Rep. (2021) 6:e873. doi: 10.1097/PR9.0000000000000873
211. Xie W, Strong JA, Zhang JM. Early blockade of injured primary sensory afferents reduces glial cell activation in two rat neuropathic pain models. Neuroscience. (2009) 160:847–57. doi: 10.1016/j.neuroscience.2009.03.016
212. Xanthos DN, Sandkuhler J. Neurogenic neuroinflammation: inflammatory CNS reactions in response to neuronal activity. Nat Rev Neurosci. (2014) 15:43–53. doi: 10.1038/nrn3617
213. Bernal L, Cisneros E, Roza C. Activation of the regeneration-associated gene STAT3 and functional changes in intact nociceptors after peripheral nerve damage in mice. Eur J Pain. (2021) 25:886–901. doi: 10.1002/ejp.1718
214. Webber CA, Xu Y, Vanneste KJ, Martinez JA, Verge VM, Zochodne DW. Guiding adult Mammalian sensory axons during regeneration. J Neuropathol Exp Neurol. (2008) 67:212–22. doi: 10.1097/NEN.0b013e3181654972
215. Ikeda-Miyagawa Y, Kobayashi K, Yamanaka H, Okubo M, Wang S, Dai Y, et al. Peripherally increased artemin is a key regulator of TRPA1/V1 expression in primary afferent neurons. Mol Pain. (2015) 11:8. doi: 10.1186/s12990-015-0004-7
216. Lippoldt EK, Ongun S, Kusaka GK, McKemy DD. Inflammatory and neuropathic cold allodynia are selectively mediated by the neurotrophic factor receptor GFRalpha3. Proc Natl Acad Sci USA. (2016) 113:4506–11. doi: 10.1073/pnas.1603294113
217. Merighi A. Targeting the glial-derived neurotrophic factor and related molecules for controlling normal and pathologic pain. Expert Opin Ther Targets. (2016) 20:193–208. doi: 10.1517/14728222.2016.1085972
218. Ossipov MH. Growth factors and neuropathic pain. Curr Pain Headache Rep. (2011) 15:185–92. doi: 10.1007/s11916-011-0183-5
219. Gardell LR, Wang R, Ehrenfels C, Ossipov MH, Rossomando AJ, Miller S, et al. Multiple actions of systemic artemin in experimental neuropathy. Nat Med. (2003) 9:1383–9. doi: 10.1038/nm944
220. Kiguchi N, Kobayashi Y, Saika F, Sakaguchi H, Maeda T, Kishioka S. Peripheral interleukin-4 ameliorates inflammatory macrophage-dependent neuropathic pain. Pain. (2015) 156:684–93. doi: 10.1097/j.pain.0000000000000097
221. Old EA, Malcangio M. Chemokine mediated neuron-glia communication and aberrant signalling in neuropathic pain states. Curr Opin Pharmacol. (2012) 12:67–73. doi: 10.1016/j.coph.2011.10.015
222. Alexander SPH, Christopoulos A, Davenport AP, Kelly E, Mathie A, Peters JA, et al. The Concise Guide to Pharmacology 2019/20: G protein-coupled receptors. Br J Pharmacol. (2019) 176(Suppl. 1):S21–141. doi: 10.1111/bph.14748
223. Igwe OJ, Murray JN, Moolwaney AS. Interleukin 1-induced cyclooxygenase and nitric oxide synthase gene expression in the rat dorsal root ganglia is modulated by antioxidants. Neuroscience. (2001) 105:971–85. doi: 10.1016/S0306-4522(01)00253-6
224. Fehrenbacher JC, Burkey TH, Nicol GD, Vasko MR. Tumor necrosis factor alpha and interleukin-1beta stimulate the expression of cyclooxygenase II but do not alter prostaglandin E2 receptor mRNA levels in cultured dorsal root ganglia cells. Pain. (2005) 113:113–22. doi: 10.1016/j.pain.2004.09.031
225. Syriatowicz JP, Hu D, Walker JS, Tracey DJ. Hyperalgesia due to nerve injury: role of prostaglandins. Neuroscience. (1999) 94:587–94. doi: 10.1016/S0306-4522(99)00365-6
226. Neeb L, Hellen P, Boehnke C, Hoffmann J, Schuh-Hofer S, Dirnagl U, et al. IL-1beta stimulates COX-2 dependent PGE(2) synthesis and CGRP release in rat trigeminal ganglia cells. PLoS ONE. (2011) 6:e17360. doi: 10.1371/journal.pone.0017360
227. Schuh CD, Pierre S, Weigert A, Weichand B, Altenrath K, Schreiber Y, et al. Prostacyclin mediates neuropathic pain through interleukin 1beta-expressing resident macrophages. Pain. (2014) 155:545–55. doi: 10.1016/j.pain.2013.12.006
228. Niehrs C. The complex world of WNT receptor signalling. Nat Rev Mol Cell Biol. (2012) 13:767–79. doi: 10.1038/nrm3470
229. Reichardt LF. Neurotrophin-regulated signalling pathways. Philos Trans R Soc Lond B Biol Sci. (2006) 361:1545–64. doi: 10.1098/rstb.2006.1894
230. Ciruela A, Dixon AK, Bramwell S, Gonzalez MI, Pinnock RD, Lee K. Identification of MEK1 as a novel target for the treatment of neuropathic pain. Br J Pharmacol. (2003) 138:751–6. doi: 10.1038/sj.bjp.0705103
231. Ji RR. The role of ERK/MAPK in spinal glia for neuropathic pain:signal transduction in spinal microglia and astrocytes after nerve injury. In: DeLeo JA, Sorkin LS, Watkins LR, editors. Immune and Glial Regulation of Pain. Seattle, WA: IASP Press (2007). p. 269–82.
232. Yang KY, Bae WS, Kim MJ, Bae YC, Kim YJ, Kim HJ, et al. Participation of the central p38 and ERK1/2 pathways in IL-1beta-induced sensitization of nociception in rats. Prog Neuropsychopharmacol Biol Psychiatry. (2013) 46:98–104. doi: 10.1016/j.pnpbp.2013.07.004
233. Zhang X, Xiao HS. Gene array analysis to determine the components of neuropathic pain signaling. Curr Opin Mol Ther. (2005) 7:532–7.
234. Baskozos G, Dawes JM, Austin JS, Antunes-Martins A, McDermott L, Clark AJ, et al. Comprehensive analysis of long noncoding RNA expression in dorsal root ganglion reveals cell-type specificity and dysregulation after nerve injury. Pain. (2019) 160:463–85. doi: 10.1097/j.pain.0000000000001416
235. Sakai A, Saitow F, Miyake N, Miyake K, Shimada T, Suzuki H. miR-7a alleviates the maintenance of neuropathic pain through regulation of neuronal excitability. Brain. (2013) 136:2738–50. doi: 10.1093/brain/awt191
236. Sakai A, Suzuki H. Emerging roles of microRNAs in chronic pain. Neurochem Int. (2014) 77:58–67. doi: 10.1016/j.neuint.2014.05.010
237. Sakai A, Saitow F, Maruyama M, Miyake N, Miyake K, Shimada T, et al. MicroRNA cluster miR-17-92 regulates multiple functionally related voltage-gated potassium channels in chronic neuropathic pain. Nat Commun. (2017) 8:16079. doi: 10.1038/ncomms16079
238. Malcangio M. Role of the immune system in neuropathic pain. Scand J Pain. (2020) 20:33–7. doi: 10.1515/sjpain-2019-0138
239. Qiu S, Liu B, Mo Y, Wang X, Zhong L, Han X, et al. MiR-101 promotes pain hypersensitivity in rats with chronic constriction injury via the MKP-1 mediated MAPK pathway. J Cell Mol Med. (2020) 24:8986–97. doi: 10.1111/jcmm.15532
240. Park CK, Xu ZZ, Berta T, Han Q, Chen G, Liu XJ, et al. Extracellular microRNAs activate nociceptor neurons to elicit pain via TLR7 and TRPA1. Neuron. (2014) 82:47–54. doi: 10.1016/j.neuron.2014.02.011
241. Simeoli R, Montague K, Jones HR, Castaldi L, Chambers D, Kelleher JH, et al. Exosomal cargo including microRNA regulates sensory neuron to macrophage communication after nerve trauma. Nat Commun. (2017) 8:1778. doi: 10.1038/s41467-017-01841-5
242. D'Agnelli S, Gerra MC, Bignami E, Arendt-Nielsen L. Exosomes as a new pain biomarker opportunity. Mol Pain. (2020) 16:1744806920957800. doi: 10.1177/1744806920957800
243. Hori N, Narita M, Yamashita A, Horiuchi H, Hamada Y, Kondo T, et al. Changes in the expression of IL-6-mediated microRNAs in the dorsal root ganglion under neuropathic pain in mice. Synapse. (2016) 70:317–324. doi: 10.1002/syn.21902
244. McDonald MK, Tian Y, Qureshi RA, Gormley M, Ertel A, Gao R, et al. Functional significance of macrophage-derived exosomes in inflammation and pain. Pain. (2014) 155:1527–39. doi: 10.1016/j.pain.2014.04.029
245. Yu X, Abdul M, Fan BQ, Zhang L, Lin X, Wu Y, et al. The release of exosomes in the medial prefrontal cortex and nucleus accumbens brain regions of chronic constriction injury (CCI) model mice could elevate the pain sensation. Neurosci Lett. (2020) 723:134774. doi: 10.1016/j.neulet.2020.134774
246. Paolicelli RC, Bergamini G, Rajendran L. Cell-to-cell communication by extracellular vesicles: focus on microglia. Neuroscience. (2019) 405:148–57. doi: 10.1016/j.neuroscience.2018.04.003
247. Study RE, Kral MG. Spontaneous action potential activity in isolated dorsal root ganglion neurons from rats with a painful neuropathy. Pain. (1996) 65:235–42. doi: 10.1016/0304-3959(95)00216-2
248. Djouhri L, Smith T, Ahmeda A, Alotaibi M, Weng X. Hyperpolarization-activated cyclic nucleotide-gated channels contribute to spontaneous activity in L4 C-fiber nociceptors, but not Abeta-non-nociceptors, after axotomy of L5-spinal nerve in the rat in vivo. Pain. (2018) 159:1392–402. doi: 10.1097/j.pain.0000000000001224
249. Abdulla FA, Smith PA. Axotomy and autotomy-induced changes in the excitability of rat dorsal root ganglion neurons. J Neurophysiol. (2001) 85:630–43. doi: 10.1152/jn.2001.85.2.630
250. Liu CN, Raber P, Ziv-Sefer S, Devor M. Hyperexcitability in sensory neurons of rats selected for high versus low neuropathic pain phenotype. Neuroscience. (2001) 105:265–75. doi: 10.1016/S0306-4522(01)00161-0
251. Govrin-Lippmann R, Devor M. Ongoing activity in severed nerves: source and variation with time. Brain Res. (1978) 159:406–10. doi: 10.1016/0006-8993(78)90548-6
252. Wall PD, Devor M, Inbal R, Scadding JW, Schonfeld D, Seltzer Z, et al. Autotomy following peripheral nerve lesions: experimental anaesthesia dolorosa. Pain. (1979) 7:103–13. doi: 10.1016/0304-3959(79)90002-2
253. Devor M. Ectopic discharge in Abeta afferents as a source of neuropathic pain. Exp Brain Res. (2009) 196:115–28. doi: 10.1007/s00221-009-1724-6
254. Waxman SG. Peripheral afferents and the pain experience. Pain. (2019) 160:1487–8. doi: 10.1097/j.pain.0000000000001527
255. Sexton JE, Cox JJ, Zhao J, Wood JN. The genetics of pain: implications for therapeutics. Annu Rev Pharmacol Toxicol. (2017) 58:123–42. doi: 10.1146/annurev-pharmtox-010617-052554
256. Koplovitch P, Devor M. Dilute lidocaine suppresses ectopic neuropathic discharge in dorsal root ganglia without blocking axonal propagation: a new approach to selective pain control. Pain. (2018) 159:1244–56. doi: 10.1097/j.pain.0000000000001205
257. Yatziv SL, Devor M. Suppression of neuropathic pain by selective silencing of dorsal root ganglion ectopia using nonblocking concentrations of lidocaine. Pain. (2019) 160:2105–14. doi: 10.1097/j.pain.0000000000001602
258. Yousuf MS, Noh MC, Friedman TN, Zubkow K, Johnson JC, Tenorio G, et al. Sensory neurons of the dorsal root ganglia become hyperexcitable in a T-cell-mediated MOG-EAE model of multiple sclerosis. eNeuro. (2019) 6:ENEURO.0024-19.2019. doi: 10.1523/ENEURO.0024-19.2019
259. Bedi SS, Yang Q, Crook RJ, Du J, Wu Z, Fishman HM, et al. chronic spontaneous activity generated in the somata of primary nociceptors is associated with pain-related behavior after spinal cord injury. J Neurosci. (2010) 30:14870. doi: 10.1523/JNEUROSCI.2428-10.2010
260. Smith PA. K+ channels in primary afferents and their role in pain produced by peripheral nerev injury. Front Cell Neurosci. (2020) 14:294. doi: 10.3389/fncel.2020.566418
261. Pitcher GM, Henry JL. Governing role of primary afferent drive in increased excitation of spinal nociceptive neurons in a model of sciatic neuropathy. Exp Neurol. (2008) 214:219–28. doi: 10.1016/j.expneurol.2008.08.003
262. Daou I, Beaudry H, Ase AR, Wieskopf JS, Ribeiro-da-Silva A, Mogil JS, et al. Optogenetic silencing of Nav1.8-positive afferents alleviates inflammatory and neuropathic pain. eNeuro. (2016) 3:ENEURO.0140-15.2016. doi: 10.1523/ENEURO.0140-15.2016
263. Waxman SG, Zamponi GW. Regulating excitability of peripheral afferents: emerging ion channel targets. Nat Neurosci. (2014) 17:153–63. doi: 10.1038/nn.3602
264. Tsantoulas C, Mooney ER, McNaughton PA. HCN2 ion channels: basic science opens up possibilities for therapeutic intervention in neuropathic pain. Biochem J. (2016) 473:2717–36. doi: 10.1042/BCJ20160287
265. Bennett DL, Clark AJ, Huang J, Waxman SG, Dib-Hajj SD. The role of voltage-gated sodium channels in pain signaling. Physiol Rev. (2019) 99:1079–151. doi: 10.1152/physrev.00052.2017
266. Staaf S, Oerther S, Lucas G, Mattsson JP, Ernfors P. Differential regulation of TRP channels in a rat model of neuropathic pain. Pain. (2009) 144:187–99. doi: 10.1016/j.pain.2009.04.013
267. Basso L, Altier C. Transient receptor potential channels in neuropathic pain. Curr Opin Pharmacol. (2017) 32:9–15. doi: 10.1016/j.coph.2016.10.002
268. Wei S, Qiu CY, Jin Y, Liu TT, Hu WP. TNF-alpha acutely enhances acid-sensing ion channel currents in rat dorsal root ganglion neurons via a p38 MAPK pathway. J Neuroinflammation. (2021) 18:92. doi: 10.1186/s12974-021-02151-w
269. Gold MS, Shuster MJ, Levine JD. Role of a Ca(2+)-dependent slow afterhyperpolarization in prostaglandin E2-induced sensitization of cultured rat sensory neurons. Neurosci Lett. (1996) 205:161–4. doi: 10.1016/0304-3940(96)12401-0
270. Jung H, Toth PT, White FA, Miller RJ. Monocyte chemoattractant protein-1 functions as a neuromodulator in dorsal root ganglia neurons. J Neurochem. (2008) 104:254–63. doi: 10.1111/j.1471-4159.2007.04969.x
271. Stemkowski PL, Garcia-Caballero A, Gadotti VM, MΓÇÖDahoma S, Chen L, Souza IA, et al. Identification of interleukin-1 beta as a key mediator in the upregulation of Cav3.2ΓÇôUSP5 interactions in the pain pathway. Mol Pain. (2017) 13:1744806917724698. doi: 10.1177/1744806917724698
272. Alvarez P, Bogen O, Levine JD. Interleukin 6 decreases nociceptor expression of the potassium channel KV1.4 in a rat model of hand-arm vibration syndrome. Pain. (2019) 160:1876–82. doi: 10.1097/j.pain.0000000000001570
273. Takeda M, Kitagawa J, Takahashi M, Matsumoto S. Activation of interleukin-1beta receptor suppresses the voltage-gated potassium currents in the small-diameter trigeminal ganglion neurons following peripheral inflammation. Pain. (2008) 139:594–602. doi: 10.1016/j.pain.2008.06.015
274. Gold MS, Levine JD. DAMGO inhibits prostaglandin E2-induced potentiation of a TTX-resistant Na+ current in rat sensory neurons in vitro. Neurosci Lett. (1996) 212:83–6. doi: 10.1016/0304-3940(96)12791-9
275. Alexander GM, van Rijn MA, van Hilten JJ, Perreault MJ, Schwartzman RJ. Changes in cerebrospinal fluid levels of pro-inflammatory cytokines in CRPS. Pain. (2005) 116:213–9. doi: 10.1016/j.pain.2005.04.013
276. DeLeo JA, Colburn RW, Rickman AJ. Cytokine and growth factor immunohistochemical spinal profiles in two animal models of mononeuropathy. Brain Res. (1997) 759:50–7. doi: 10.1016/S0006-8993(97)00209-6
277. del Rivero T, Fischer R, Yang F, Swanson KA, Bethea JR. Tumor necrosis factor receptor 1 inhibition is therapeutic for neuropathic pain in males but not in females. Pain. (2019) 160:889–93. doi: 10.1097/j.pain.0000000000001470
278. Murphy PG, Grondin J, Altares M, Richardson PM. Induction of interleukin-6 in axotomized sensory neurons. J Neurosci. (1995) 15:5130–8. doi: 10.1523/JNEUROSCI.15-07-05130.1995
279. Ma W, Quirion R. Up-regulation of interleukin-6 induced by prostaglandin E from invading macrophages following nerve injury: an in vivo and in vitro study. J Neurochem. (2005) 93:664–73. doi: 10.1111/j.1471-4159.2005.03050.x
280. Flatters SJ, Fox AJ, Dickenson AH. Nerve injury alters the effects of interleukin-6 on nociceptive transmission in peripheral afferents. Eur J Pharmacol. (2004) 484:183–91. doi: 10.1016/j.ejphar.2003.11.013
281. Murphy PG, Ramer MS, Borthwick L, Gauldie J, Richardson PM, Bisby MA. Endogenous interleukin-6 contributes to hypersensitivity to cutaneous stimuli and changes in neuropeptides associated with chronic nerve constriction in mice. Eur J Neurosci. (1999) 11:2243–53. doi: 10.1046/j.1460-9568.1999.00641.x
282. Recasens M, Almolda B, Perez-Clausell J, Campbell IL, Gonzalez B, Castellano B. Chronic exposure to IL-6 induces a desensitized phenotype of the microglia. J Neuroinflammation. (2021) 18:31. doi: 10.1186/s12974-020-02063-1
283. McLachlan EM, Janig W, Michalis M. Peripheral nerve injury triggers noradrenergic sprouting within dorsal root ganglia. Nature. (1993) 363:543–6. doi: 10.1038/363543a0
284. Abdulla FA, Smith PA. Ectopic α2-adrenoceptors couple to N-type Ca2+ channels in axotomized rat sensory neurons. J Neurosci. (1997) 17:1633–41. doi: 10.1523/JNEUROSCI.17-05-01633.1997
285. Yen LD, Bennett GJ, Ribeiro-da-Silva A. Sympathetic sprouting and changes in nociceptive sensory innervation in the glabrous skin of the rat hind paw following partial peripheral nerve injury. J Comp Neurol. (2006) 495:679–90. doi: 10.1002/cne.20899
286. Ramer MS, Bisby MA. Sympathetic axons surround neuropeptide-negative axotomized sensory neurons. Neuroreport. (1998) 9:3109–13. doi: 10.1097/00001756-199809140-00035
287. Devor M, Janig W, Michaelis M. Modulation of activity in dorsal root ganglion neurons by sympathetic activation in nerve-injured rats. J Neurophysiol. (1994) 71:38–47. doi: 10.1152/jn.1994.71.1.38
288. Pertin M, Allchorne AJ, Beggah AT, Woolf CJ, Decosterd I. Delayed sympathetic dependence in the spared nerve injury (SNI) model of neuropathic pain. Mol Pain. (2007) 3:21. doi: 10.1186/1744-8069-3-21
289. Rho RH, Brewer RP, Lamer TJ, Wilson PR. Complex regional pain syndrome. Mayo Clin Proc. (2002) 77:174–80. doi: 10.1016/S0025-6196(11)62332-X
290. Xie W, Strong JA, Li H, Zhang JM. Sympathetic sprouting near sensory neurons after nerve injury occurs preferentially on spontaneously active cells and is reduced by early nerve block. J Neurophysiol. (2007) 97:492–502. doi: 10.1152/jn.00899.2006
291. Landry M, Holmberg K, Zhang X, Hokfelt T. Effect of axotomy on expression of NPY. galanin, and NPY Y1 and Y2 receptors in dorsal root ganglia and the superior cervical ganglion studied with double-labeling in situ hybridization and immunohistochemistry. Exp Neurol. (2000) 162:361–84. doi: 10.1006/exnr.1999.7329
292. Wakisaka S, Kajander KC, Bennett GJ. Increased neuropeptide Y (NPY)-like immunoreactivity in rat sensory neurons following peripheral axotomy. Neurosci Lett. (1991) 124:200–3. doi: 10.1016/0304-3940(91)90093-9
293. Noguchi K, De Leon M, Nahin RL, Senba E, Ruda MA. Quantification of axotomy-induced alteration of neuropeptide mRNAs in dorsal root ganglion neurons with special reference to neuropeptide Y mRNA and the effects of neonatal capsaicin treatment. J Neurosci Res. (1993) 35:54–66. doi: 10.1002/jnr.490350108
294. Amir R, Devor M. Chemically mediated cross-excitation in rat dorsal root ganglia. J Neurosci. (1996) 16:4733–41. doi: 10.1523/JNEUROSCI.16-15-04733.1996
295. Matsuka Y, Afroz S, Dalanon JC, Iwasa T, Waskitho A, Oshima M. The role of chemical transmitters in neuron-glia interaction and pain in sensory ganglion. Neurosci Biobehav Rev. (2020) 108:393–9. doi: 10.1016/j.neubiorev.2019.11.019
296. Huang LY, Neher E. Ca(2+)-dependent exocytosis in the somata of dorsal root ganglion neurons. Neuron. (1996) 17:135–45. doi: 10.1016/S0896-6273(00)80287-1
297. Noguchi K, Dubner R, De LM, Senba E, Ruda MA. Axotomy induces preprotachykinin gene expression in a subpopulation of dorsal root ganglion neurons. J Neurosci Res. (1994) 37:596–603. doi: 10.1002/jnr.490370506
298. Abdulla FA, Stebbing MJ, Smith PA. Effects of substance P on excitability and ionic currents of normal and axotomized rat dorsal root ganglion neurons. Eur J Neurosci. (2001) 13:545–52. doi: 10.1046/j.0953-816x.2000.01429.x
299. Campbell JN, Raja SN, Meyer RA, Mackinnon SE. Myelinated afferents signal the hyperalgesia associated with nerve injury. Pain. (1988) 32:89–94. doi: 10.1016/0304-3959(88)90027-9
300. Baba H, Ji RR, Kohno T, Moore KA, Ataka T, Wakai A, et al. Removal of GABAergic inhibition facilitates polysynaptic A fiber-mediated excitatory transmission to the superficial spinal dorsal horn. Mol Cell Neurosci. (2003) 24:818–30. doi: 10.1016/S1044-7431(03)00236-7
301. Torsney C, MacDermott AB. Disinhibition opens the gate to pathological pain signaling in superficial neurokinin 1 receptor-expressing neurons in rat spinal cord. J Neurosci. (2006) 26:1833–43. doi: 10.1523/JNEUROSCI.4584-05.2006
302. Peirs C, Williams SP, Zhao X, Walsh CE, Gedeon JY, Cagle NE, et al. Dorsal horn circuits for persistent mechanical pain. Neuron. (2015) 87:797–812. doi: 10.1016/j.neuron.2015.07.029
303. Peirs C, Dallel R, Todd AJ. Recent advances in our understanding of the organization of dorsal horn neuron populations and their contribution to cutaneous mechanical allodynia. J Neural Transm. (2020) 127:505–25. doi: 10.1007/s00702-020-02159-1
304. Sanada M, Yasuda H, Omatsu-Kanbe M, Sango K, Isono T, Matsuura H, et al. Increase in intracellular Ca(2+) and calcitonin gene-related peptide release through metabotropic P2Y receptors in rat dorsal root ganglion neurons. Neuroscience. (2002) 111:413–22. doi: 10.1016/S0306-4522(02)00005-2
305. Eberhardt M, Hoffmann T, Sauer SK, Messlinger K, Reeh PW, Fischer MJ. Calcitonin gene-related peptide release from intact isolated dorsal root and trigeminal ganglia. Neuropeptides. (2008) 42:311–7. doi: 10.1016/j.npep.2008.01.002
306. Kleinschnitz C, Brinkhoff J, Zelenka M, Sommer C, Stoll G. The extent of cytokine induction in peripheral nerve lesions depends on the mode of injury and NMDA receptor signaling. J Neuroimmunol. (2004) 149:77–83. doi: 10.1016/j.jneuroim.2003.12.013
307. Chun S, Kwon YB. The CCL2 elevation in primary afferent fibers produces zymosan-induced hyperalgesia through microglia-mediated neuronal activation in the spinal dorsal horn. Brain Res Bull. (2019) 149:53–9. doi: 10.1016/j.brainresbull.2019.04.014
308. Hamed EA, Mohamed Farghaly HS, Abdel Mola AF, Fahmi MK, Makhlouf MM, Balfas MA. Role of monocyte chemoattractant protein-1, stromal derived factor-1 and retinoic acid in pathophysiology of neuropathic pain in rats. J Basic Clin Physiol Pharmacol. (2016) 27:411–24. doi: 10.1515/jbcpp-2015-0105
309. Sacerdote P, Franchi S, Trovato AE, Valsecchi AE, Panerai AE, Colleoni M. Transient early expression of TNF-alpha in sciatic nerve and dorsal root ganglia in a mouse model of painful peripheral neuropathy. Neurosci Lett. (2008) 436:210–3. doi: 10.1016/j.neulet.2008.03.023
310. Lee HL, Lee KM, Son SJ, Hwang SH, Cho HJ. Temporal expression of cytokines and their receptors mRNAs in a neuropathic pain model. Neuroreport. (2004) 15:2807–11.
311. Clark AK, Staniland AA, Marchand F, Kaan TKY, McMahon SB, Malcangio M. P2X7-dependent release of interleukin-1{beta} and nociception in the spinal cord following lipopolysaccharide. J Neurosci. (2010) 30:573–82. doi: 10.1523/JNEUROSCI.3295-09.2010
312. Beggs S, Trang T, Salter MW. P2X4R+ microglia drive neuropathic pain. Nat Neurosci. (2012) 15:1068–73. doi: 10.1038/nn.3155
313. Tam TH, Salter MW. Purinergic signalling in spinal pain processing. Purinergic Signal. (2020) 17:49–54. doi: 10.1007/s11302-020-09748-5
314. Trang T, Beggs S, Wan X, Salter MW. P2X4-receptor-mediated synthesis and release of brain-derived neurotrophic factor in microglia is dependent on calcium and p38-mitogen-activated protein kinase activation. J Neurosci. (2009) 29:3518–28. doi: 10.1523/JNEUROSCI.5714-08.2009
315. Trang T, Beggs S, Salter MW. Brain-derived neurotrophic factor from microglia: a molecular substrate for neuropathic pain. Neuron Glia Biol. (2011) 7:99–108. doi: 10.1017/S1740925X12000087
316. Trang T, Beggs S, Salter MW. ATP receptors gate microglia signaling in neuropathic pain. Exp Neurol. (2012) 234:354–61. doi: 10.1016/j.expneurol.2011.11.012
317. Trang T, Salter MW. P2X4 purinoceptor signaling in chronic pain. Purinergic Signal. (2012) 8:621–8. doi: 10.1007/s11302-012-9306-7
318. Tsuda M, Shigemoto-Mogami Y, Koizumi S, Mizokoshi A, Kohsaka S, Salter MW, et al. P2X(4) receptors induced in spinal microglia gate tactile allodynia after nerve injury. Nature. (2003) 424:778–83. doi: 10.1038/nature01786
319. Malcangio M. Spinal mechanisms of neuropathic pain: is there a P2X4-BDNF controversy? Neurobiol Pain. (2017) 1:1–5. doi: 10.1016/j.ynpai.2017.04.001
320. Sorge RE, Totsch SK. Sex differences in pain. J Neurosci Res. (2016) 95:1271–81. doi: 10.1002/jnr.23841
321. Abbadie C, Bhangoo S, De KY, Malcangio M, Melik-Parsadaniantz S, White FA. Chemokines and pain mechanisms. Brain Res Rev. (2009) 60:125–34. doi: 10.1016/j.brainresrev.2008.12.002
322. Menetski J, Mistry S, Lu M, Mudgett JS, Ransohoff RM, DeMartino JA, et al. Mice overexpressing chemokine ligand 2 (CCL2) in astrocytes display enhanced nociceptive responses. Neuroscience. (2007) 149:706–14. doi: 10.1016/j.neuroscience.2007.08.014
323. Zhang J, de Koninck. Y. Spatial and temporal relationship between monocyte chemoattractant protein-1 expression and spinal glial activation following peripheral nerve injury. J Neurochem. (2006) 97:772–83. doi: 10.1111/j.1471-4159.2006.03746.x
324. Jung H, Miller RJ. Activation of the nuclear factor of activated T-cells (NFAT) mediates upregulation of CCR2 chemokine receptors in dorsal root ganglion (DRG) neurons: a possible mechanism for activity-dependent transcription in DRG neurons in association with neuropathic pain. Mol Cell Neurosci. (2008) 37:170–7. doi: 10.1016/j.mcn.2007.09.004
325. Thacker MA, Clark AK, Marchand F, McMahon SB. Pathophysiology of peripheral neuropathic pain: immune cells and molecules. Anesth Analg. (2007) 105:838–47. doi: 10.1213/01.ane.0000275190.42912.37
326. Abbadie C, Lindia JA, Cumiskey AM, Peterson LB, Mudgett JS, Bayne EK, et al. Impaired neuropathic pain responses in mice lacking the chemokine receptor CCR2. Proc Natl Acad Sci USA. (2003) 100:7947–52. doi: 10.1073/pnas.1331358100
327. Zhang J, Shi XQ, Echeverry S, Mogil JS, De KY, Rivest S. Expression of CCR2 in both resident and bone marrow-derived microglia plays a critical role in neuropathic pain. J Neurosci. (2007) 27:12396–406. doi: 10.1523/JNEUROSCI.3016-07.2007
328. Hickman SE, Kingery ND, Ohsumi TK, Borowsky ML, Wang LC, Means TK, et al. The microglial sensome revealed by direct RNA sequencing. Nat Neurosci. (2013) 16:1896–905. doi: 10.1038/nn.3554
329. Knerlich-Lukoschus F, Juraschek M, Blomer U, Lucius R, Mehdorn HM, Held-Feindt J. Force-dependent development of neuropathic central pain and time-related CCL2/CCR2 expression after graded spinal cord contusion injuries of the rat. J Neurotrauma. (2008) 25:427–48. doi: 10.1089/neu.2007.0431
330. Saederup N, Cardona AE, Croft K, Mizutani M, Cotleur AC, Tsou CL, et al. Selective chemokine receptor usage by central nervous system myeloid cells in CCR2-red fluorescent protein knock-in mice. PLoS ONE. (2010) 5:e13693. doi: 10.1371/journal.pone.0013693
331. de Jong EK, Dijkstra IM, Hensens M, Brouwer N, van AM, Liem RS, et al. Vesicle-mediated transport and release of CCL21 in endangered neurons: a possible explanation for microglia activation remote from a primary lesion. J Neurosci. (2005) 25:7548–57. doi: 10.1523/JNEUROSCI.1019-05.2005
332. van Weering HR, de Jong AP, de Haas AH, Biber KP, Boddeke HW. CCL21-induced calcium transients and proliferation in primary mouse astrocytes: CXCR3-dependent and independent responses. Brain Behav Immun. (2010) 24:768–75. doi: 10.1016/j.bbi.2009.04.007
333. Luo X, Tai WL, Sun L, Pan Z, Xia Z, Chung SK, et al. Crosstalk between astrocytic CXCL12 and microglial CXCR4 contributes to the development of neuropathic pain. Mol Pain. (2016) 12:1–15. doi: 10.1177/1744806916636385
334. Dubovy P, Klusakova I, Svizenska I, Brazda V. Spatio-temporal changes of SDF1 and its CXCR4 receptor in the dorsal root ganglia following unilateral sciatic nerve injury as a model of neuropathic pain. Histochem Cell Biol. (2010) 133:323–37. doi: 10.1007/s00418-010-0675-0
335. Liu ZY, Song ZW, Guo SW, He JS, Wang SY, Zhu JG, et al. CXCL12/CXCR4 signaling contributes to neuropathic pain via central sensitization mechanisms in a rat spinal nerve ligation model. CNS Neurosci Ther. (2019) 25:922–36. doi: 10.1111/cns.13128
336. Bai L, Wang X, Li Z, Kong C, Zhao Y, Qian JL, et al. Upregulation of chemokine CXCL12 in the dorsal root ganglia and spinal cord contributes to the development and maintenance of neuropathic pain following spared nerve injury in rats. Neurosci Bull. (2016) 32:27–40. doi: 10.1007/s12264-015-0007-4
337. Knerlich-Lukoschus F, von der Ropp-Brenner B, Lucius R, Mehdorn HM, Held-Feindt J. Spatiotemporal CCR1, CCL3(MIP-1alpha), CXCR4, CXCL12(SDF-1alpha) expression patterns in a rat spinal cord injury model of posttraumatic neuropathic pain. J Neurosurg Spine. (2011) 14:583–97. doi: 10.3171/2010.12.SPINE10480
338. Yang F, Sun W, Luo WJ, Yang Y, Yang F, Wang XL, et al. SDF1-CXCR4 signaling contributes to the transition from acute to chronic pain state. Mol Neurobiol. (2017) 54:2763–75. doi: 10.1007/s12035-016-9875-5
339. Verge GM, Milligan ED, Maier SF, Watkins LR, Naeve GS, Foster AC. Fractalkine (CX3CL1) and fractalkine receptor (CX3CR1) distribution in spinal cord and dorsal root ganglia under basal and neuropathic pain conditions. Eur J Neurosci. (2004) 20:1150–60. doi: 10.1111/j.1460-9568.2004.03593.x
340. Lindia JA, McGowan E, Jochnowitz N, Abbadie C. Induction of CX3CL1 expression in astrocytes and CX3CR1 in microglia in the spinal cord of a rat model of neuropathic pain. J Pain. (2005) 6:434–8. doi: 10.1016/j.jpain.2005.02.001
341. Milligan ED, Zapata V, Chacur M, Schoeniger D, Biedenkapp J, O'connor KA, et al. Evidence that exogenous and endogenous fractalkine can induce spinal nociceptive facilitation in rats. Eur J Neurosci. (2004) 20:2294–302. doi: 10.1111/j.1460-9568.2004.03709.x
342. Lee KM, Jeon SM, Cho HJ. Interleukin-6 induces microglial CX3CR1 expression in the spinal cord after peripheral nerve injury through the activation of p38 MAPK. Eur J Pain. (2010) 14:682–12. doi: 10.1016/j.ejpain.2009.10.017
343. Staniland AA, Clark AK, Wodarski R, Sasso O, Maione F, D'Acquisto F, et al. Reduced inflammatory and neuropathic pain and decreased spinal microglial response in fractalkine receptor (CX3CR1) knockout mice. J Neurochem. (2010) 114:1143–57. doi: 10.1111/j.1471-4159.2010.06837.x
344. Bazan JF, Bacon KB, Hardiman G, Wang W, Soo K, Rossi D, et al. A new class of membrane-bound chemokine with a CX3C motif. Nature. (1997) 385:640–4. doi: 10.1038/385640a0
345. Clark AK, Yip PK, Malcangio M. The liberation of fractalkine in the dorsal horn requires microglial cathepsin S. J Neurosci. (2009) 29:6945–54. doi: 10.1523/JNEUROSCI.0828-09.2009
346. Clark AK, Yip PK, Grist J, Gentry C, Staniland AA, Marchand F, et al. Inhibition of spinal microglial cathepsin S for the reversal of neuropathic pain. Proc Natl Acad Sci USA. (2007) 104:10655–60. doi: 10.1073/pnas.0610811104
347. Clark AK, Wodarski R, Guida F, Sasso O, Malcangio M. Cathepsin S release from primary cultured microglia is regulated by the P2X7 receptor. Glia. (2010) 58:1710–26. doi: 10.1002/glia.21042
348. Tsuda M, Masuda T, Kitano J, Shimoyama H, Tozaki-Saitoh H, Inoue K. IFN-gamma receptor signaling mediates spinal microglia activation driving neuropathic pain. Proc Natl Acad Sci USA. (2009) 106:8032–7. doi: 10.1073/pnas.0810420106
349. Vikman KS, Siddall PJ, Duggan AW. Increased responsiveness of rat dorsal horn neurons in vivo following prolonged intrathecal exposure to interferon-[gamma]. Neuroscience. (2005) 135:969–77. doi: 10.1016/j.neuroscience.2005.06.059
350. Vikman KS, Hill RH, Backstrom E, Robertson B, Kristensson K. Interferon-gamma induces characteristics of central sensitization in spinal dorsal horn neurons in vitro. Pain. (2003) 106:241–51. doi: 10.1016/S0304-3959(03)00262-8
351. Reischer G, Heinke B, Sandkühler J. Interferon-γ facilitates the synaptic transmission between primary afferent C-fibres and lamina I neurons in the rat spinal dorsal horn via microglia activation. Mol Pain. (2020) 16:1744806920917249. doi: 10.1177/1744806920917249
352. Costigan M, Moss A, Latremoliere A, Johnston C, Verma-Gandhu M, Herbert TA, et al. T-cell infiltration and signaling in the adult dorsal spinal cord is a major contributor to neuropathic pain-like hypersensitivity. J Neurosci. (2009) 29:14415–22. doi: 10.1523/JNEUROSCI.4569-09.2009
353. Ruscheweyh R, Wilder-Smith O, Drdla R, Liu XG, Sandkuhler J. Long-term potentiation in spinal nociceptive pathways as a novel target for pain therapy. Mol Pain. (2011) 7:20. doi: 10.1186/1744-8069-7-20
354. Chitu V, Gokhan S, Nandi S, Mehler MF, Stanley ER. Emerging roles for CSF-1 receptor and its ligands in the nervous system. Trends Neurosci. (2016) 39:378–393. doi: 10.1016/j.tins.2016.03.005
355. Mapplebeck JC, Beggs S, Salter MW. Molecules in pain and sex: a developing story. Mol Brain. (2017) 10:9. doi: 10.1186/s13041-017-0289-8
356. Lu VB, Ballanyi K, Colmers WF, Smith PA. Neuron type-specific effects of brain-derived neurotrophic factor in rat superficial dorsal horn and their relevance to 'central sensitization'. J Physiol. (2007) 584:543–63. doi: 10.1113/jphysiol.2007.141267
357. Ha SO, Kim JK, Hong HS, Kim DS, Cho HJ. Expression of brain-derived neurotrophic factor in rat dorsal root ganglia, spinal cord and gracile nuclei in experimental models of neuropathic pain. Neuroscience. (2001) 107:301–9. doi: 10.1016/S0306-4522(01)00353-0
358. Garraway SM, Petruska JC, Mendell LM. BDNF sensitizes the response of lamina II neurons to high threshold primary afferent inputs. Eur J Neurosci. (2003) 18:2467–76. doi: 10.1046/j.1460-9568.2003.02982.x
359. Zhang W, Shi Y, Peng Y, Zhong L, Zhu S, Zhang W, et al. Neuron activity-induced Wnt signaling up-regulates expression of brain-derived neurotrophic factor in the pain neural circuit. J Biol Chem. (2018) 293:15641–15651. doi: 10.1074/jbc.RA118.002840
360. Biggs JE, Boakye PA, Ganesan N, Stemkowski PL, Lantero A, Ballanyi K, et al. Analysis of the long-term actions of gabapentin and pregabalin in dorsal root ganglia and substantia gelatinosa. J Neurophysiol. (2014) 112:2398–412. doi: 10.1152/jn.00168.2014
361. Bardoni R, Goldstein PA, Lee CJ, Gu JG, MacDermott AB. ATP P2X receptors mediate fast synaptic transmission in the dorsal horn of the rat spinal cord. J Neurosci. (1997) 17:5297–304. doi: 10.1523/JNEUROSCI.17-14-05297.1997
362. Masuda T, Ozono Y, Mikuriya S, Kohro Y, Tozaki-Saitoh H, Iwatsuki K, et al. Dorsal horn neurons release extracellular ATP in a VNUT-dependent manner that underlies neuropathic pain. Nat Commun. (2016) 7:12529. doi: 10.1038/ncomms12529
363. Zhang X, Li G. P2Y receptors in neuropathic pain. Pharmacol Biochem Behav. (2019) 186:172788. doi: 10.1016/j.pbb.2019.172788
364. Inoue K, Koizumi S, Tsuda M. The role of nucleotides in the neuron–glia communication responsible for the brain functions. J Neurochem. (2007) 102:1447–458. doi: 10.1111/j.1471-4159.2007.04824.x
365. Barragan-Iglesias P, Pineda-Farias JB, Cervantes-Duran C, Bravo-Hernandez M, Rocha-Gonzalez HI, Murbartian J, et al. Role of spinal P2Y6 and P2Y11 receptors in neuropathic pain in rats: possible involvement of glial cells. Mol Pain. (2014) 10:29. doi: 10.1186/1744-8069-10-29
366. Kobayashi K, Yamanaka H, Fukuoka T, Dai Y, Obata K, Noguchi K. P2Y12 receptor upregulation in activated microglia is a gateway of p38 signaling and neuropathic pain. J Neurosci. (2008) 28:2892–02. doi: 10.1523/JNEUROSCI.5589-07.2008
367. Kobayashi K, Yamanaka H, Yanamoto F, Okubo M, Noguchi K. Multiple P2Y subtypes in spinal microglia are involved in neuropathic pain after peripheral nerve injury. Glia. (2012) 60:1529–39. doi: 10.1002/glia.22373
368. Inoue K. Neuropharmacological study of ATP receptors, especially in the relationship between glia and pain. Yakugaku Zasshi. (2017) 137:563–9. doi: 10.1248/yakushi.16-00262
369. Huang D, Yang J, Liu X, He L, Luo X, Tian H, et al. P2Y6 receptor activation is involved in the development of neuropathic pain induced by chronic constriction injury of the sciatic nerve in rats. J Clin Neurosci. (2018) 56:156–62. doi: 10.1016/j.jocn.2018.07.013
370. Tatsumi E, Yamanaka H, Kobayashi K, Yagi H, Sakagami M, Noguchi K. RhoA/ROCK pathway mediates p38 MAPK activation and morphological changes downstream of P2Y12/13 receptors in spinal microglia in neuropathic pain. Glia. (2015) 63:216–28. doi: 10.1002/glia.22745
371. Kobayashi K, Yamanaka H, Noguchi K. Expression of ATP receptors in the rat dorsal root ganglion and spinal cord. Anat Sci Int. (2013) 88:10–16. doi: 10.1007/s12565-012-0163-9
372. Huang J, Bloe CB, Zhou X, Wu S, Zhang W. The role of the spinal Wnt signaling pathway in HIV-related neuropathic pain. Cell Mol Neurobiol. (2020) 40:1075–85. doi: 10.1007/s10571-020-00805-6
373. Li Y, Li B, Wan X, Zhang W, Zhong L, Tang SJ. NMDA receptor activation stimulates transcription-independent rapid wnt5a protein synthesis via the MAPK signaling pathway. Mol Brain. (2012) 5:1. doi: 10.1186/1756-6606-5-1
374. Chen J, Park CS, Tang SJ. Activity-dependent synaptic Wnt release regulates hippocampal long term potentiation. J Biol Chem. (2006) 281:11910–16. doi: 10.1074/jbc.M511920200
375. Zhao J, Seereeram A, Nassar MA, Levato A, Pezet S, Hathaway G, et al. Nociceptor-derived brain-derived neurotrophic factor regulates acute and inflammatory but not neuropathic pain. Mol Cell Neurosci. (2006) 31:539–48. doi: 10.1016/j.mcn.2005.11.008
376. Matayoshi S, Jiang N, Katafuchi T, Koga K, Furue H, Yasaka T, et al. Actions of brain-derived neurotrophic factor on spinal nociceptive transmission during inflammation in the rat. J Physiol. (2005) 569:685–95. doi: 10.1113/jphysiol.2005.095331
377. Echeverry S, Shi XQ, Yang M, Huang H, Wu Y, Lorenzo LE, et al. Spinal microglia are required for long-term maintenance of neuropathic pain. Pain. (2017) 158:1792–801. doi: 10.1097/j.pain.0000000000000982
378. Casarotto PC, Girych M, Fred SM, Kovaleva V, Moliner R, Enkavi G, et al. Antidepressant drugs act by directly binding to TRKB neurotrophin receptors. Cell. (2021) 184:1–15. doi: 10.1016/j.cell.2021.01.034
379. Finnerup NB, Attal N, Haroutounian S, McNicol E, Baron R, Dworkin RH, et al. Pharmacotherapy for neuropathic pain in adults: a systematic review and meta-analysis. Lancet Neurol. (2015) 14:162–73. doi: 10.1016/S1474-4422(14)70251-0
380. Clark AK, D'Aquisto F, Gentry C, Marchand F, McMahon SB, Malcangio M. Rapid co-release of interleukin 1beta and caspase 1 in spinal cord inflammation. J Neurochem. (2006) 99:868–80. doi: 10.1111/j.1471-4159.2006.04126.x
381. Gajtko A, Bakk E, Hegedus K, Ducza L, Hollo K. IL-1beta induced cytokine expression by spinal astrocytes can play a role in the maintenance of chronic inflammatory pain. Front Physiol. (2020) 11:543331. doi: 10.3389/fphys.2020.543331
382. Heilig R, Dick MS, Sborgi L, Meunier E, Hiller S, Broz P. The gasdermin-D pore acts as a conduit for IL-1beta secretion in mice. Eur J Immunol. (2018) 48:584–92. doi: 10.1002/eji.201747404
383. Evavold CL, Ruan J, Tan Y, Xia S, Wu H, Kagan JC. The pore-forming protein gasdermin D regulates interleukin-1 secretion from living macrophages. Immunity. (2018) 48:35–44. doi: 10.1016/j.immuni.2017.11.013
384. Orning P, Lien E, Fitzgerald KA. Gasdermins and their role in immunity and inflammation. J Exp Med. (2019) 216:2453–65. doi: 10.1084/jem.20190545
385. Batista SJ, Still KM, Johanson D, Thompson JA, O'Brien CA, Lukens JR, et al. Gasdermin-D-dependent IL-1alpha release from microglia promotes protective immunity during chronic Toxoplasma gondii infection. Nat Commun. (2020) 11:3687. doi: 10.1038/s41467-020-17491-z
386. LaMacchia ZM, Spengler RN, Jaffari M, Abidi AH, Ahmed T, Singh N, et al. Perispinal injection of a TNF blocker directed to the brain of rats alleviates the sensory and affective components of chronic constriction injury-induced neuropathic pain. Brain Behav Immun. (2019) 82:93–105. doi: 10.1016/j.bbi.2019.07.036
387. Li S, Cai J, Feng ZB, Jin ZR, Liu BH, Zhao HY, et al. BDNF contributes to spinal long-term potentiation and mechanical hypersensitivity via Fyn-mediated phosphorylation of NMDA receptor GluN2B subunit at tyrosine 1472 in rats following spinal nerve ligation. Neurochem Res. (2017) 42:2712–29. doi: 10.1007/s11064-017-2274-0
388. Balasubramanyan S, Stemkowski PL, Stebbing MJ, Smith PA. Sciatic chronic constriction injury produces cell-type specific changes in the electrophysiological properties of rat substantia gelatinosa neurons. J Neurophysiol. (2006) 96:579–90. doi: 10.1152/jn.00087.2006
389. Chen Y, Balasubramanyan S, Lai AY, Todd KG, Smith PA. Effects of sciatic nerve axotomy on excitatory synaptic transmission in rat substantia gelatinosa. J Neurophysiol. (2009) 102:3203–15. doi: 10.1152/jn.00296.2009
390. Felsby S, Nielsen J, Arendt-Nielsen L, Jensen TS. NMDA receptor blockade in chronic neuropathic pain: a comparison of ketamine and magnesium chloride. Pain. (1996) 64:283–91. doi: 10.1016/0304-3959(95)00113-1
391. Hewitt DJ. The use of NMDA-receptor antagonists in the treatment of chronic pain. Clin J Pain. (2000) 16:S73–S9. doi: 10.1097/00002508-200006001-00013
392. Kerr BJ, Bradbury EJ, Bennett DL, Trivedi PM, Dassan P, French J, et al. Brain-derived neurotrophic factor modulates nociceptive sensory inputs and NMDA-evoked responses in the rat spinal cord. J Neurosci. (1999) 19:5138–48. doi: 10.1523/JNEUROSCI.19-12-05138.1999
393. Slack SE, Pezet S, McMahon SB, Thompson SW, Malcangio M. Brain-derived neurotrophic factor induces NMDA receptor subunit one phosphorylation via ERK and PKC in the rat spinal cord. Eur J Neurosci. (2004) 20:1769–78. doi: 10.1111/j.1460-9568.2004.03656.x
394. Chen W, Walwyn W, Ennes HS, Kim H, McRoberts JA, Marvizon JC. BDNF released during neuropathic pain potentiates NMDA receptors in primary afferent terminals. Eur J Neurosci. (2014) 39:1439–54. doi: 10.1111/ejn.12516
395. Yan X, Jiang E, Gao M, Weng HR. Endogenous activation of presynaptic NMDA receptors enhances glutamate release from the primary afferents in the spinal dorsal horn in a rat model of neuropathic pain. J Physiol. (2013) 591:2001–19. doi: 10.1113/jphysiol.2012.250522
396. Coull JA, Boudreau D, Bachand K, Prescott SA, Nault F, Sik A, et al. Trans-synaptic shift in anion gradient in spinal lamina I neurons as a mechanism of neuropathic pain. Nature. (2003) 424:938–42. doi: 10.1038/nature01868
397. Ferrini F, Perez-Sanchez J, Ferland S, Lorenzo LE, Godin AG, Plasencia-Fernandez I, et al. Differential chloride homeostasis in the spinal dorsal horn locally shapes synaptic metaplasticity and modality-specific sensitization. Nat Commun. (2020) 11:3935. doi: 10.1038/s41467-020-17824-y
398. Prescott SA, Sejnowski TJ, de Koninck. Y. Reduction of anion reversal potential subverts the inhibitory control of firing rate in spinal lamina I neurons: towards a biophysical basis for neuropathic pain. Mol Pain. (2006) 2:32. doi: 10.1186/1744-8069-2-32
399. Lavertu G, Côté SL, De Koninck Y. Enhancing KΓÇôCl co-transport restores normal spinothalamic sensory coding in a neuropathic pain model. Brain. (2014) 137:724–738. doi: 10.1093/brain/awt334
400. Prescott SA, de Koninck Y, Sejnowski TJ. Biophysical basis for three distinct dynamical mechanisms of action potential initiation. PLoS Comput Biol. (2008) 4:e1000198. doi: 10.1371/journal.pcbi.1000198
401. Todd AJ. Neuronal circuitry for pain processing in the dorsal horn. Nat Rev Neurosci. (2010) 11:823–36. doi: 10.1038/nrn2947
402. Peirs C, Seal RP. Neural circuits for pain: Recent advances and current views. Science. (2016) 354:578–84. doi: 10.1126/science.aaf8933
403. Prescott SA, Ma Q, De KY. Normal and abnormal coding of somatosensory stimuli causing pain. Nat Neurosci. (2014) 17:183–91. doi: 10.1038/nn.3629
404. Price TJ, Prescott SA. Inhibitory regulation of the pain gate and how its failure causes pathological pain. Pain. (2015) 156:789–92. doi: 10.1097/j.pain.0000000000000139
405. Pezet S, Cunningham J, Patel J, Grist J, Gavazzi I, Lever IJ, et al. BDNF modulates sensory neuron synaptic activity by a facilitation of GABA transmission in the dorsal horn. Mol Cell Neurosci. (2002) 21:51–62. doi: 10.1006/mcne.2002.1166
406. Sandkuhler J. Models and mechanisms of hyperalgesia and allodynia. Physiol Rev. (2009) 89:707–58. doi: 10.1152/physrev.00025.2008
407. Sandkuhler J, Gruber-Schoffnegger D. Hyperalgesia by synaptic long-term potentiation (LTP): an update. Curr Opin Pharmacol. (2012) 12:18–27. doi: 10.1016/j.coph.2011.10.018
408. Zhou LJ, Peng J, Xu YN, Zeng WJ, Zhang J, Wei X, et al. Microglia are indispensable for synaptic plasticity in the spinal dorsal horn and chronic pain. Cell Rep. (2019) 27:3844–59. doi: 10.1016/j.celrep.2019.05.087
409. Ding X, Cai J, Li S, Liu XD, Wan Y, Xing GG. BDNF contributes to the development of neuropathic pain by induction of spinal long-term potentiation via SHP2 associated GluN2B-containing NMDA receptors activation in rats with spinal nerve ligation. Neurobiol Dis. (2015) 73:428–51. doi: 10.1016/j.nbd.2014.10.025
410. Ruscheweyh R, Sandkuhler J. Long-range oscillatory Ca2+ waves in rat spinal dorsal horn. Eur J Neurosci. (2005) 22:1967–76. doi: 10.1111/j.1460-9568.2005.04393.x
411. Chapman RJ, Cilia La Corte PF, Asghar AU, King AE. Network-based activity induced by 4-aminopyridine in rat dorsal horn in vitro is mediated by both chemical and electrical synapses. J Physiol. (2009) 587:2499–510. doi: 10.1113/jphysiol.2009.171777
412. Boakye PA, Schmidt EKA, Rancic V, Kerr B, Ballanyi K, Smith PA. Characterization of superficial dorsal horn neurons from “tamamaki” mice and stability of their GAD67-EGFP phenotype in defined-medium organotypic culture. Neuroscience. (2018) 372:126–40. doi: 10.1016/j.neuroscience.2017.12.047
413. Asghar AU, Cilia La Corte PF, LeBeau FE, Al DM, Reilly SC, Buhl EH, et al. Oscillatory activity within rat substantia gelatinosa in vitro: a role for chemical and electrical neurotransmission. J Physiol. (2005) 562:183–98. doi: 10.1113/jphysiol.2004.076398
414. Harding EK, Boivin B, Salter MW. Intracellular calcium responses encode action potential firing in spinal cord lamina I neurons. J Neurosci. (2020) 40:4439–56. doi: 10.1523/JNEUROSCI.0206-20.2020
415. Dalal A, Tata M, Allègre G, Gekiere F, Bons N, Albe-Fessard D. Spontaneous activity of rat dorsal horn cells in spinal segments of sciatic projection following transection of sciatic nerve or of corresponding dorsal roots. Neuroscience. (1999) 94:217–28. doi: 10.1016/S0306-4522(99)00328-0
416. Alshelh Z, Di PF, Youssef AM, Reeves JM, Macey PM, Vickers ER, et al. Chronic neuropathic pain: it's about the rhythm. J Neurosci. (2016) 36:1008–18. doi: 10.1523/JNEUROSCI.2768-15.2016
417. Zhang H, Li Y, Yang Q, Liu XG, Dougherty PM. Morphological and physiological plasticity of spinal lamina II GABA neurons is induced by sciatic nerve chronic constriction injury in mice. Front Cell Neurosci. (2018) 12:143. doi: 10.3389/fncel.2018.00143
418. Bailey AL, Ribeiro-da-Silva A. Transient loss of terminals from non-peptidergic nociceptive fibers in the substantia gelatinosa of spinal cord following chronic constriction injury of the sciatic nerve. Neuroscience. (2006) 138:675–90. doi: 10.1016/j.neuroscience.2005.11.051
419. Stratton HJ, Khanna R. Sculpting dendritic spines during initiation and maintenance of neuropathic pain. J Neurosci. (2020) 40:7578. doi: 10.1523/JNEUROSCI.1664-20.2020
420. Gu N, Peng J, Murugan M, Wang X, Eyo UB, Sun D, et al. Spinal microgliosis due to resident microglial proliferation is required for pain hypersensitivity after peripheral nerve injury. Cell Rep. (2016) 16:605–14. doi: 10.1016/j.celrep.2016.06.018
421. Calvo M, Bennett DL. The mechanisms of microgliosis and pain following peripheral nerve injury. Exp Neurol. (2012) 234:271–82. doi: 10.1016/j.expneurol.2011.08.018
422. Lorenzo LE, Godin AG, Ferrini F, Bachand K, Plasencia-Fernandez I, Labrecque S, et al. Enhancing neuronal chloride extrusion rescues alpha2/alpha3 GABAA-mediated analgesia in neuropathic pain. Nat Commun. (2020) 11:869. doi: 10.1038/s41467-019-14154-6
423. Ribeiro-da-Silva A, Coimbra A. Two types of synaptic glomeruli and their distribution in laminae I-III of the rat spinal cord. J Comp Neurol. (1982) 209:176–186. doi: 10.1002/cne.902090205
424. Todd AJ, Watt C, Spike RC, Sieghart W. Colocalization of GABA. Glycine, and their receptors at synapses in the rat spinal cord. J Neurosci. (1996) 16:974–82. doi: 10.1523/JNEUROSCI.16-03-00974.1996
425. Bouhy D, Malgrange B, Multon S, Poirrier AL, Scholtes F, Schoenen J, et al. Delayed GM-CSF treatment stimulates axonal regeneration and functional recovery in paraplegic rats via an increased BDNF expression by endogenous macrophages. FASEB J. (2006) 20:1239–41. doi: 10.1096/fj.05-4382fje
426. Lu P, Jones LL, Tuszynski MH. BDNF-expressing marrow stromal cells support extensive axonal growth at sites of spinal cord injury. Exp Neurol. (2005) 191:344–60. doi: 10.1016/j.expneurol.2004.09.018
427. Lorenzo LE, Magnussen C, Bailey AL, St LM, De KY, Ribeiro-da-Silva A. Spatial and temporal pattern of changes in the number of GAD65-immunoreactive inhibitory terminals in the rat superficial dorsal horn following peripheral nerve injury. Mol Pain. (2014) 10:57. doi: 10.1186/1744-8069-10-57
428. Abbadie C, McManus OB, Sun SY, Bugianesi RM, Dai G, Haedo RJ, et al. Analgesic effects of a substituted N-triazole oxindole (TROX-1), a state-dependent, voltage-gated calcium channel 2 blocker. J Pharmacol Exp Ther. (2010) 334:545–55. doi: 10.1124/jpet.110.166363
429. Zhang X, Wang J, Zhou Q, Xu Y, Pu S, Wu J, et al. Brain-derived neurotrophic factor-activated astrocytes produce mechanical allodynia in neuropathic pain. Neuroscience. (2011) 199:452–60. doi: 10.1016/j.neuroscience.2011.10.017
430. Yan X, Weng HR. Endogenous interleukin-1beta in neuropathic rats enhances glutamate release from the primary afferents in the spinal dorsal horn through coupling with presynaptic N-methyl-D-aspartic acid receptors. J Biol Chem. (2013) 288:30544–57. doi: 10.1074/jbc.M113.495465
431. Yan X, Yadav R, Gao M, Weng HR. Interleukin-1 beta enhances endocytosis of glial glutamate transporters in the spinal dorsal horn through activating protein kinase C. Glia. (2014) 62:1093–109. doi: 10.1002/glia.22665
432. Hanisch UK, Kettenmann H. Microglia: active sensor and versatile effector cells in the normal and pathologic brain. Nat Neurosci. (2007) 10:1387–94. doi: 10.1038/nn1997
433. Latremoliere A, Woolf CJ. Central sensitization: a generator of pain hypersensitivity by central neural plasticity. J Pain. (2009) 10:895–926. doi: 10.1016/j.jpain.2009.06.012
434. Pineau I, Lacroix S. Proinflammatory cytokine synthesis in the injured mouse spinal cord: Multiphasic expression pattern and identification of the cell types involved. J Comp Neurol. (2007) 500:267–85. doi: 10.1002/cne.21149
435. Ji RR, Donnelly CR, Nedergaard M. Astrocytes in chronic pain and itch. Nat Rev Neurosci. (2019) 20:667–85. doi: 10.1038/s41583-019-0218-1
436. Weng HR, Chen JH, Cata JP. Inhibition of glutamate uptake in the spinal cord induces hyperalgesia and increased responses of spinal dorsal horn neurons to peripheral afferent stimulation. Neuroscience. (2006) 138:1351–60. doi: 10.1016/j.neuroscience.2005.11.061
437. Weng HR, Chen JH, Pan ZZ, Nie H. Glial glutamate transporter 1 regulates the spatial and temporal coding of glutamatergic synaptic transmission in spinal lamina II neurons. Neuroscience. (2007) 149:898–907. doi: 10.1016/j.neuroscience.2007.07.063
438. Miraucourt LS, Peirs C, Dallel R, Voisin DL. Glycine inhibitory dysfunction turns touch into pain through astrocyte-derived D-serine. Pain. (2011) 152:1340–8. doi: 10.1016/j.pain.2011.02.021
439. Grace PM, Hutchinson MR, Maier SF, Watkins LR. Pathological pain and the neuroimmune interface. Nat Rev Immunol. (2014) 14:217–31. doi: 10.1038/nri3621
440. Milligan ED, Watkins LR. Pathological and protective roles of glia in chronic pain. Nat Rev Neurosci. (2009) 10:23–36. doi: 10.1038/nrn2533
441. Greenhalgh AD, David S, Bennett FC. Immune cell regulation of glia during CNS injury and disease. Nat Rev Neurosci. (2020) 21:139–52. doi: 10.1038/s41583-020-0263-9
442. Pinteaux E, Parker LC, Rothwell NJ, Luheshi GN. Expression of interleukin-1 receptors and their role in interleukin-1 actions in murine microglial cells. J Neurochem. (2002) 83:754–63. doi: 10.1046/j.1471-4159.2002.01184.x
443. Copray JC, Mantingh I, Brouwer N, Biber K, Kust BM, Liem RS, et al. Expression of interleukin-1 beta in rat dorsal root ganglia. J Neuroimmunol. (2001) 118:203–11. doi: 10.1016/S0165-5728(01)00324-1
444. Cahill LS, Laliberte CL, Liu XJ, Bishop J, Nieman BJ, Mogil JS, et al. Quantifying blood-spinal cord barrier permeability after peripheral nerve injury in the living mouse. Mol Pain. (2014) 10:60. doi: 10.1186/1744-8069-10-60
445. Beggs S, Liu XJ, Kwan C, Salter MW. Peripheral nerve injury and TRPV1-expressing primary afferent C-fibers cause opening of the blood-brain barrier. Mol Pain. (2010) 6:74. doi: 10.1186/1744-8069-6-74
446. Gui WS, Wei X, Mai CL, Murugan M, Wu LJ, Xin WJ, et al. Interleukin-1beta overproduction is a common cause for neuropathic pain, memory deficit, and depression following peripheral nerve injury in rodents. Mol Pain. (2016) 12:1–15. doi: 10.1177/1744806916646784
447. Andrade P, Cornips EMJ, Sommer C, Daemen MA, Visser-Vandewalle V, Hoogland G. Elevated inflammatory cytokine expression in CSF from patients with symptomatic thoracic disc herniation correlates with increased pain scores. Spine J. (2018) 18:2316–22. doi: 10.1016/j.spinee.2018.07.023
448. Milligan ED, Twining C, Chacur M, Biedenkapp J, O'Connor K, Poole S, et al. Spinal glia and proinflammatory cytokines mediate mirror-image neuropathic pain in rats. J Neurosci. (2003) 23:1026–40. doi: 10.1523/JNEUROSCI.23-03-01026.2003
449. Ren K, Torres R. Role of interleukin-1beta during pain and inflammation. Brain Res Rev. (2009) 60:57–64. doi: 10.1016/j.brainresrev.2008.12.020
450. Martin LJ, Acland EL, Cho C, Gandhi W, Chen D, Corley E, et al. Male-specific conditioned pain hypersensitivity in mice and humans. Curr Biol. (2019) 29:192–201. doi: 10.1016/j.cub.2018.11.030
451. Shansky RM, Murphy AZ. Considering sex as a biological variable will require a global shift in science culture. Nat Neurosci. (2021) 24:457–64. doi: 10.1038/s41593-021-00806-8
452. Dodds KN, Beckett EA, Evans SF, Grace PM, Watkins LR, Hutchinson MR. Glial contributions to visceral pain: implications for disease etiology and the female predominance of persistent pain. Transl Psychiatry. (2016) 6:e888. doi: 10.1038/tp.2016.168
453. Mifflin KA, Frieser E, Benson C, Baker G, Kerr BJ. Voluntary wheel running differentially affects disease outcomes in male and female mice with experimental autoimmune encephalomyelitis. J Neuroimmunol. (2017) 305:135–44. doi: 10.1016/j.jneuroim.2017.02.005
454. Szabo-Pardi TA, Syed UM, Castillo ZW, Burton MD. Use of integrated optical clearing and 2-photon imaging to investigate sex differences in neuroimmune interactions after peripheral nerve injury. Front Cell Dev Biol. (2021) 9:119. doi: 10.3389/fcell.2021.624201
455. O'Brien MS, Philpott HTA, McDougall JJ. Targeting the Nav1.8 ion channel engenders sex-specific responses in lysophosphatidic acid-induced joint neuropathy. Pain. (2019) 160:269–78. doi: 10.1097/j.pain.0000000000001399
456. Gagnon M, Bergeron MJ, Lavertu G, Castonguay A, Tripathy S, Bonin RP, et al. Chloride extrusion enhancers as novel therapeutics for neurological diseases. Nat Med. (2013) 19:1524–8. doi: 10.1038/nm.3356
457. Polgar E, Hughes DI, Riddell JS, Maxwell DJ, Puskar Z, Todd AJ. Selective loss of spinal GABAergic or glycinergic neurons is not necessary for development of thermal hyperalgesia in the chronic constriction injury model of neuropathic pain. Pain. (2003) 104:229–39. doi: 10.1016/S0304-3959(03)00011-3
458. Polgar E, Gray S, Riddell JS, Todd AJ. Lack of evidence for significant neuronal loss in laminae I-III of the spinal dorsal horn of the rat in the chronic constriction injury model. Pain. (2004) 111:144–50. doi: 10.1016/j.pain.2004.06.011
459. Peirs C, Williams SG, Zhao X, Arokiaraj CM, Ferreira DW, Noh MC, et al. Mechanical allodynia circuitry in the dorsal horn is defined by the nature of the injury. Neuron. (2021) 109:73–90. doi: 10.1016/j.neuron.2020.10.027
460. Polgar E, Fowler JH, McGill MM, Todd AJ. The types of neuron which contain protein kinase C gamma in rat spinal cord. Brain Res. (1999) 833:71–80. doi: 10.1016/S0006-8993(99)01500-0
461. Smith KM, Browne TJ, Davis OC, Coyle A, Boyle KA, Watanabe M, et al. Calretinin positive neurons form an excitatory amplifier network in the spinal cord dorsal horn. Elife. (2019) 8:e49190. doi: 10.7554/eLife.49190
Keywords: central sensitization, dorsal horn, nerve injury, neuropathy, cytokine, chemokine, growth factor, synaptic transmission
Citation: Boakye PA, Tang S-J and Smith PA (2021) Mediators of Neuropathic Pain; Focus on Spinal Microglia, CSF-1, BDNF, CCL21, TNF-α, Wnt Ligands, and Interleukin 1β. Front. Pain Res. 2:698157. doi: 10.3389/fpain.2021.698157
Received: 20 April 2021; Accepted: 14 July 2021;
Published: 25 August 2021.
Edited by:
Xisheng Yan, Wuhan Third Hospital, ChinaReviewed by:
Kazue Mizumura, Nihon University, JapanCopyright © 2021 Boakye, Tang and Smith. This is an open-access article distributed under the terms of the Creative Commons Attribution License (CC BY). The use, distribution or reproduction in other forums is permitted, provided the original author(s) and the copyright owner(s) are credited and that the original publication in this journal is cited, in accordance with accepted academic practice. No use, distribution or reproduction is permitted which does not comply with these terms.
*Correspondence: Peter A. Smith, cGFzM0B1YWxiZXJ0YS5jYQ==
Disclaimer: All claims expressed in this article are solely those of the authors and do not necessarily represent those of their affiliated organizations, or those of the publisher, the editors and the reviewers. Any product that may be evaluated in this article or claim that may be made by its manufacturer is not guaranteed or endorsed by the publisher.
Research integrity at Frontiers
Learn more about the work of our research integrity team to safeguard the quality of each article we publish.