- 1Unit of Experimental Biology, Department of Biomedicine, Faculty of Medicine, University of Porto, Porto, Portugal
- 2Institute of Molecular and Cell Biology, University of Porto, Porto, Portugal
- 3Institute of Investigation and Innovation in Health, University of Porto, Porto, Portugal
- 4Faculty of Nutrition and Food Science, University of Porto, Porto, Portugal
The treatment of neuropathic pain remains a clinical challenge. Analgesic drugs and antidepressants are frequently ineffective, and opioids may induce side effects, including hyperalgesia. Recent results on brainstem pain modulatory circuits may explain those clinical challenges. The dual action of noradrenergic (NA) modulation was demonstrated in animal models of neuropathic pain. Besides the well-established antinociception due to spinal effects, the NA system may induce pronociception by directly acting on brainstem pain modulatory circuits, namely, at the locus coeruleus (LC) and medullary dorsal reticular nucleus (DRt). The serotoninergic system also has a dual action depending on the targeted spinal receptor, with an exacerbated activity of the excitatory 5-hydroxytryptamine 3 (5-HT3) receptors in neuropathic pain models. Opioids are involved in the modulation of descending modulatory circuits. During neuropathic pain, the opioidergic modulation of brainstem pain control areas is altered, with the release of enhanced local opioids along with reduced expression and desensitization of μ-opioid receptors (MOR). In the DRt, the installation of neuropathic pain increases the levels of enkephalins (ENKs) and induces desensitization of MOR, which may enhance descending facilitation (DF) from the DRt and impact the efficacy of exogenous opioids. On the whole, the data discussed in this review indicate the high plasticity of brainstem pain control circuits involving monoaminergic and opioidergic control. The data from studies of these neurochemical systems in neuropathic models indicate the importance of designing drugs that target multiple neurochemical systems, namely, maximizing the antinociceptive effects of antidepressants that inhibit the reuptake of serotonin and noradrenaline and preventing desensitization and tolerance of MOR at the brainstem.
Descending Pain Modulation: General View
The existence of top-down modulation of nociceptive transmission was already postulated by the gate control theory [1]. The periaqueductal gray (PAG) matter, the first brainstem structure with a demonstrated involvement in top-down pain modulation, has reciprocal projections with cortical areas, amygdala [2], and the rostral ventromedial medulla (RVM). The PAG matter does not project directly to the spinal cord (SC) and relays the descending input through the RVM [3], the main serotoninergic spinally projecting neuronal population [4, 5]. Top-down modulation is also mediated by the release of noradrenaline at the dorsal horn of the SC. Descending noradrenergic (NA) projections to the SC arise from three brainstem NA neuronal populations: the A5, A6 (comprising the nucleus subcoeruleus and the LC), and A7 NA cell groups [6–9]. Noradrenaline inhibits nociceptive transmission at the SC through the activation of α2-adrenergic receptors (α2-AR) located at peripheral nociceptors or spinal neurons [10]. Top-down modulation includes bidirectional control, i.e., inhibitory and facilitatory. Pronociceptive actions are well represented by the medullary dorsal reticular nucleus (DRt), which is reciprocally connected with the dorsal horn of the SC in a reverberating circuit that amplifies nociceptive transmission [11, 12]. Descending facilitation (DF) from the DRt is enhanced in sustained pain models [13, 14] and may account for spinal sensitization during neuropathic pain [15]. The RVM comprises two classes of non-serotoninergic neurons classified by their role in nociceptive modulation: OFF- and ON-cells [16]. The activity of OFF-cells decreases during nociceptive-like behaviors, while the opposite occurs with ON-cells, and OFF-cells were proposed to play antinociceptive effects, whereas ON-cells are likely to exert pronociceptive actions in descending pain modulation [17].
The components of the descending pain modulatory system show neuroplastic changes during neuropathic pain. In the RVM, the imbalance in the activity of ON- and OFF-cells toward the increased activity of the former during traumatic and diabetic neuropathy may facilitate nociceptive spinal transmission [18–20]. The LC also plays bidirectional control of pain modulation. Besides the well-established inhibitory actions through its descending projections to the SC, the LC exerts pain-facilitatory actions through projections to several areas of the pain control modulatory system, such as the DRt [14, 21]. Recent studies described alterations of descending serotoninergic and NA systems and local opioidergic modulation in several neuropathic pain models. These data will be critically analyzed throughout this review to discuss perspectives of designing analgesic drugs that tackle the challenges of neuropathic pain management.
Descending Monoaminergic Pain Modulation During Neuropathic Pain
The descending serotoninergic and NA systems are altered during neuropathic pain (Figure 1). Regarding the descending serotoninergic modulatory system, an imbalance toward facilitation was detected, which may account for the persistence of pain [23, 24]. In neuropathic pain, descending serotoninergic modulation from the RVM is involved in the maintenance, rather than in the installation, of chronic pain [23, 25]. The depletion of serotoninergic RVM neurons or of serotoninergic pathways reduces nociceptive behaviors after, but not before, nerve injury [26] and prevents nociceptive hypersensitivity in traumatic neuropathic pain (TNP) models [27, 28]. In traumatic and diabetic neuropathic pain (DNP) models, an increase in the serotoninergic input to the SC from hyperactive serotoninergic RVM neurons was proposed to represent an adaptation of descending pain modulation to the increased barrage of nociceptive input [29, 30]. The increased serotonin (5-HT)-mediated input to the SC is likely to be pronociceptive due to the higher activity of facilitatory 5-hydroxytryptamine 3 (5-HT3) receptors at the SC (see below). Further, accounting for pronociceptive actions of the RVM during neuropathic pain, an increase in spontaneous activity of ON-cells was reported in traumatic and DNP models [17]. In a chemotherapy-induced neuropathic pain (CINP) model, similar results were obtained, with higher activation of serotoninergic RVM neurons and increased serotoninergic output to the SC [31]. This indicates that the RVM serotoninergic system is similarly affected at least in some neuropathic pain models. Increases in descending serotoninergic pain recruitment may yield facilitation or inhibition, depending on the targeted subtype of the spinal serotoninergic receptor [32]. The activation of 5-HT1A/B and 5-HT7 spinal receptors inhibits nociception, while the activation of 5-HT3 and 5-HT2A receptors has the opposite effect [32–34]. The administration of 5-HT1A agonists produces strong antinociceptive effects and attenuates depression-like behaviors related to TNP [35], whereas 5-HT1A antagonist reduces or abolishes antinociceptive responses [33, 36, 37]. Spinal 5-HT1A receptors mediate the analgesic effects of cannabidiol during diabetic neuropathy [38]. The systemic administration of 5-HT1A antagonists reduces the antidepressant-like effect of venlafaxine [39]. However, the antidepressant and antinociceptive effects seem to use different groups of 5-HT1A receptors [40]. The administration of the 5-HT7 agonist induces analgesia [41], while the antagonist increases neuropathic pain-like behaviors [42–44]. In contrast, the activation of the 5-HT2A receptors increases pain-like behaviors in models of traumatic and diabetic neuropathy [45, 46]. The 5-HT3R, the only 5-HT ionotropic receptor with excitatory functions, plays a crucial role in pronociception during neuropathic pain [47]. In TNP models, namely, animals with SC injury, intrathecal administration of 5-HT3R antagonist induces antinociception whereas the agonist intensifies allodynia [48, 49]. Moreover, pharmacological spinal blockade of the overexpressed 5-HT3R reverted the neuropathic pain-like behaviors during CINP [31] and attenuated neuronal hyperactivity in diabetic neuropathy [47]. As a whole, the studies indicate that 5-HT3Rs account for central sensitization in neuropathic pain [50]. More studies are necessary to better understand the net balance between the increased serotoninergic input to the SC and the role of spinal receptors, namely, in long-term neuropathic pain models, and also considering the intensity of the noxious stimulus inasmuch that biphasic modulation from the RVM was reported [51].
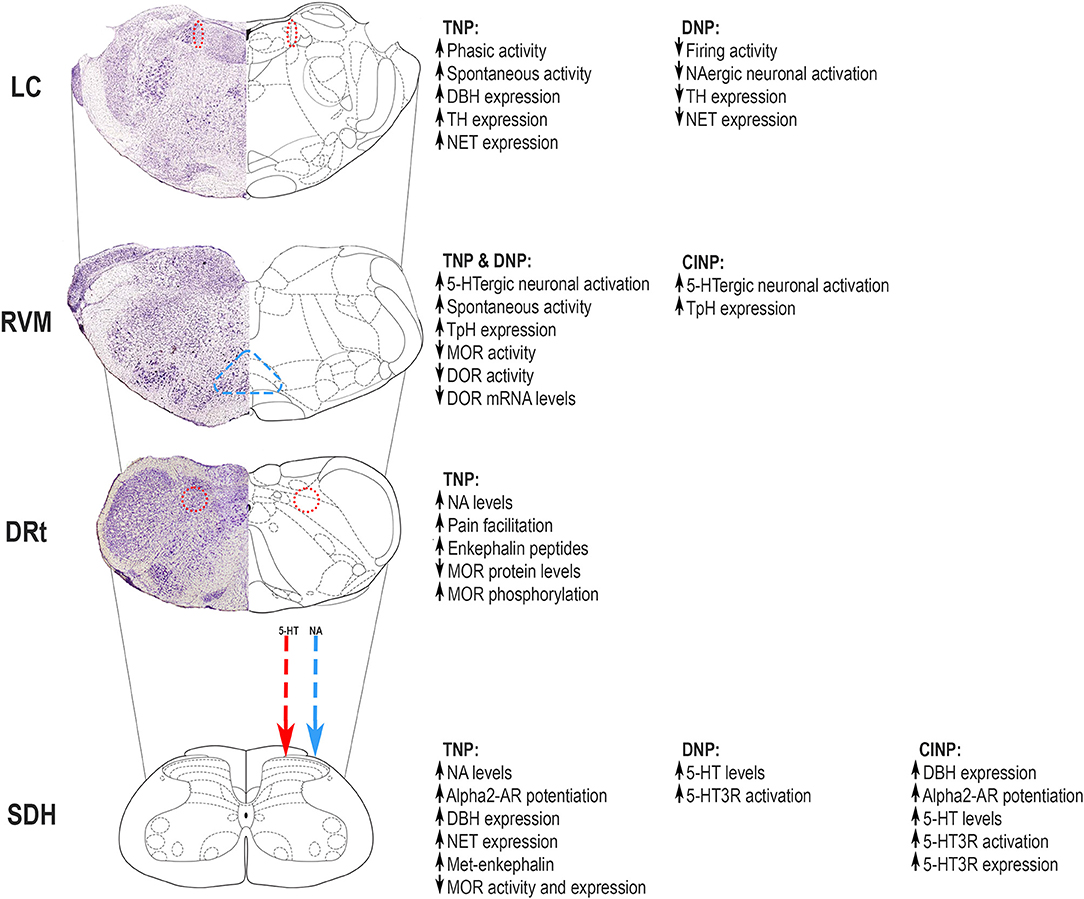
Figure 1. Neuropathic pain induces adaptations in the descending modulatory pain systems involved in serotoninergic and noradrenergic (NA) neurochemical control, some of which are mediated by local opioidergic control. Major changes occur at the locus coeruleus (LC), rostral ventromedial medulla (RVM), and medullary dorsal reticular nucleus (DRt) and affect top-down modulation of nociceptive transmission at the superficial dorsal horn (SDH). The figure summarizes the alterations occurring in different pain models, namely, traumatic neuropathic pain (TNP), diabetic neuropathic pain (DNP), and chemotherapy-induced neuropathic pain (CINP). The mechanisms underlying top-down modulation in different neuropathic pain models are discussed in this review. Adapted from Paxinos and Watson [22]. 5-HT, serotonin; 5-HT3R, 5-HT3 receptor; α2-AR - α2 adrenoreceptor; DBH, dopamine beta hydroxylase; DOR, delta opioid receptor; DRt, dorsal reticular nucleus; LC, locus coeruleus; MOR, μ-opioid receptor; NA, noradrenergic; NET, noradrenaline transporter; RVM, rostral ventromedial medulla; SDH, superficial dorsal horn; TH, tyrosine hydroxylase; TpH, tryptophan hydroxylase.
Neuropathic pain also induces neuroplastic changes in NA pain modulatory areas that may account for pain persistence [52, 53]. Several studies showed that LC neurons present higher electrophysiological responses evoked by noxious stimulation at early stages of nerve injury, but spontaneous activity does not change at that early stages [53–55]. It was shown that, during chronic pain, the balance of LC function may shift from pain inhibition to pain facilitation, which may account for chronic pain installation [56]. A recent study showed higher spontaneous activity and enhanced response of LC neurons after noxious stimulation in long-term traumatic neuropathy [57]. Some reports have also shown that the LC has increased expression of noradrenaline-synthetizing enzymes and noradrenaline transporter (NET) at late stages in long-term traumatic neuropathic pain models [58, 59]. The other NA brainstem cell groups also contribute to the maintenance of neuropathic pain. In the models of diabetic neuropathy, an increase in neuronal activation at the A5 NA cell group was reported at 4 weeks after the induction of diabetes [29], and in traumatic neuropathic models, the administration of α2-AR agonist into the A7 NA cell group reduces neuropathic hypersensitivity [60]. The alterations in the NA pain modulatory centers affect nociceptive transmission at the dorsal horn of the SC with a clear involvement of α2-AR. In TNP models, NA spinal upregulation occurs with increased spinal noradrenaline levels and enhanced efficacy of G protein-coupled α2-AR [61–63]. Furthermore, nerve injury increases the density of NA fibers in the SC, which is associated with increased brain-derived nerve growth factor (BDNF), a neurotrophin involved in neuronal differentiation and neuroplastic pain-related mechanisms [64]. Moreover, upregulation of the spinal NET was reported in TNP models [65]. With the progression of traumatic neuropathy, a gradual loss of descending NA inhibition occurs [66]. In the CINP animal model, increased expression of NA biosynthetic enzymes at the dorsal horn and the potentiation of the α2-AR-mediated antinociception at the SC were recently described [67]. Although the studies of NA descending modulation have been mostly directed to α2-AR, recent demonstrations that noxious stimulation activates astrocytes at the superficial dorsal horn (SDH) through α1A-AR [68] will open new avenues for pain research in the future.
Besides the direct effects in the direct input to the SC, the alterations of the serotoninergic and NA systems also affect the brainstem pain modulatory system. The LC and A5 NA cell groups project to the DRt [12]. The increased activation of NA LC and A5 neurons in traumatic neuropathic models leads to increased release of noradrenaline into DRt, which was proposed to enhance DF of nociceptive transmission from that medullary area [14]. The studies of the endogenous pain modulatory system should, therefore, be performed considering the connectivity between areas, which is a clinically relevant issue. Patients with neuropathic pain have higher complaints of pain when the PAG-RVM connectivity is stronger [69]. The enhanced recruitment of descending NA inhibition during CINP likely aims to compensate for the increased 5-HT3-mediated descending serotoninergic facilitation from the RVM [67]. Preclinical studies would benefit from shifting from studying a single pain modulatory system in the brain to studying about approaching the connectivity issues, namely, in what concerns the interplay between the serotoninergic and NA systems.
Opioidergic Modulation of Brainstem Pain Control Circuits
Endogenous opioids are involved in the control of the descending pain modulatory system through the activation of mu (MOR), delta (DOR), kappa (KOR), and nociceptin opioid peptide (NOP) receptors [70]. The endogenous opioids, such as enkephalins (ENKs), β-endorphins, and dynorphins, bind, by order of preference, to DOR, MOR, and KOR, respectively. Regarding nociceptin, it binds to NOP and its role as an independent neural “anti-opioid” system has been proposed [71].
The PAG and RVM constitute major sites of supraspinal MOR analgesia [72, 73]. Genetic approaches confirmed that MOR activates the PAG-RVM descending pathway via suppression of the inhibitory influence of local GABAergic interneurons (Figure 2) [74, 75]. The administration of opioids in the RVM produces antinociception through direct inhibition of pronociceptive MOR-expressing ON-cells and indirect activation (i.e., disinhibition) of antinociceptive OFF-cells [17]. The neurochemical nature and synaptic mechanisms of the PAG-RVM circuitry were recently addressed using genetic approaches [76]. Neurons co-expressing gamma aminobutyric acid (GABA) and preproenkephalin functionally correspond to OFF-cells and directly project onto nociceptor terminals in the dorsal horn to inhibit nociceptive transmission (Figure 2) [77]. Other GABAergic RVM neurons express MOR and project to preproenkephalin dorsal horn interneurons, facilitating the transmission of nociceptive information [76]. The activation of DOR and KOR also modulates the PAG-RVM circuit. The mechanisms and consequences of DOR activation in the PAG-RVM circuit are similar to MOR (Figure 2) [78, 79]. DOR agonists typically show lower adverse effects than MOR agonists, but their efficacy is also lower, probably due to intracellular trafficking [80]. The administration of KOR agonists in the RVM inhibits OFF-cells and blocks the antinociceptive actions of MOR activation [81, 82]. The NOP receptor is abundant in the PAG and RVM [83]. The role of the nociceptin/orphanin FQ peptide (N/OFQ)–NOP receptor system is better studied in the RVM, and NOP is expressed in OFF-cells and co-expressed with MOR in ON-cells (Figure 2) [84, 85]. Supraspinal N/OFQ induces pronociceptive effects along with an anti-opioid analgesic action [86]. In contrast, N/OFQ attenuates opioid withdrawal-induced hyperalgesia by inhibiting ON-cells [84]. Given the role of activation of ON-cells in the maintenance of neuropathic pain [87], the inhibition of ON-cells likely contributes to the antihyperalgesic and antiallodynic effects of systemic and supraspinal NOP ligands in neuropathic pain models [88, 89].
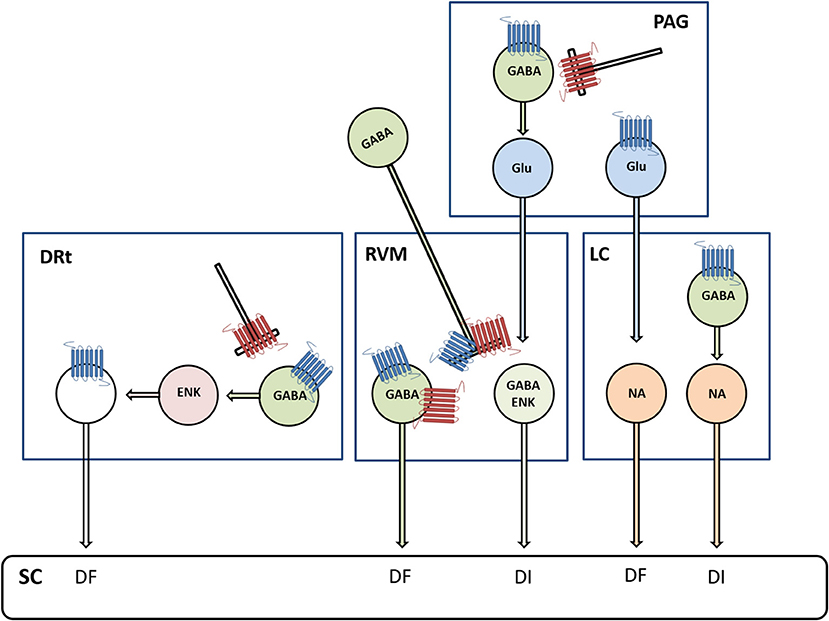
Figure 2. Diagram depicting the involvement of μ-opioid receptors (MOR, marked in blue) and δ-opioid receptors (DOR, marked in red) in descending modulation from the periaqueductal gray (PAG) matter, RVM, LC, and the DRt. Opioids are involved in the mediation of descending inhibition (DI) and descending facilitation (DF) from PAG circuits relayed in the RVM and LC, respectively. In the PAG-RVM circuit, MOR- and DOR-mediated inhibition of gamma aminobutyric acid (GABA)ergic neurons disinhibits glutamatergic (Glu) neurons projecting to the RVM. In the PAG-LC circuit, MOR inhibits a subtype of Glu neurons, which express the phospholipase C β4, projecting to NA LC neurons. This newly discovered circuit is thought to counterbalance the adverse excitatory effects of stress on the LC-NA system. Opioids are also involved in the mediation of DI from the LC through MOR-mediated inhibition of GABAergic neurons that disinhibit NA neurons projecting to the spinal cord (SC). Opioids in the RVM produce antinociception via direct inhibition of pronociceptive cells expressing MOR and DOR, which are GABAergic and functionally correspond to ON-cells, and indirect activation (i.e., disinhibition) of antinociceptive cells, which co-express GABA and enkephalins (ENKs) and functionally correspond to OFF-cells. Opioids in the DRt act through direct inhibition of DRt spinally projecting neurons, which express MOR, and indirectly through activation (i.e., disinhibition) of local ENK neurons.
The effects of opioids have also been extensively studied in the LC, where MOR is highly expressed [90]. Opioid receptors in the LC are implicated in pain modulation, stress responses, and opioid drug effects [91, 92]. Early studies indicate that opioids produce antinociception by enhancing the descending NA inhibition [93]. In the extreme, opioids inhibit LC neurons, and following chronic morphine infusion, LC neurons undergo desensitization, accounting for tolerance to opioids [94]. Opioidergic modulation of the LC is complex as opioids can also suppress descending inhibition (DI) through the PAG-LC pathway (Figure 2) [74]. In this respect, the opioidergic system has two different roles at the PAG: one enhances DI, through the PAG-RVM pathway, and the other suppresses DI, through the PAG-LC pathway. Notwithstanding, the final output to spinal nociceptive information will likely further involve the opioidergic modulation of upstream brain structures, such as the amygdala, with which the PAG is connected [95].
The LC plays a complex role in pain modulation with facilitatory and inhibitory modes of modulation of nociception. It exerts pain-facilitatory actions through its ascending projections to several supraspinal areas [13, 14, 21] and inhibitory actions through its descending projections to the SC. Opioids produce antinociception partly by enhancing the descending NA inhibition [96–99]. LC neurons have also been implicated in tolerance to opioids. The LC has a high density of MORs [90, 100]. In the extreme, opioids inhibit LC neurons; however, following chronic treatment with morphine, LC neurons undergo desensitization, which contributes to the development of tolerance to opioid effects (reviewed by 91). The desensitization of LC neurons was inhibited in mice expressing a mutant MOR that renders the receptor increasingly unable to interact with β-arrestins, and these exhibit enhanced opioid-induced analgesia [101].
At the DRt, opioids are a key local modulatory system that can directly and indirectly modulate the spinal-DRt-spinal reverberative pathway. Opioids act through direct inhibition of DRt spinally projecting neurons that express MOR and through disinhibition of enkephalinergic interneurons that receive input from GABAergic interneurons that express MOR (Figure 2) [102, 103]. These GABAergic interneurons are also presynaptically inhibited by DOR-expressing fibers [102]. Local overexpression of opioid peptides, namely ENK, was shown to inhibit DRt pain facilitation [104]. The activation of MOR at the DRt plays a fundamental inhibitory role at the DRt and was recently shown to account for the analgesic effects of systemic opioids [105].
The supraspinal opioidergic modulation may account for neuropathic pain. During neuropathic pain, the increased DF is not compensated by enhanced DI [50, 87]. The engagement of DI mediated by the RVM-OFF cells, through which opioids produce descending pain inhibition, protects against the development of neuropathic pain [106]. Evidence of a dysfunctional descending opioidergic inhibition in neuropathic pain is further provided by the decrease of diffuse noxious inhibitory control (DNIC) in animal models [107–111]. DNIC is mainly a neurophysiological phenomenon, and many authors consider [102–104] that its psychophysical paradigm in humans is represented by conditioned pain modulation (CPM). DNIC is a unique form of endogenous analgesia that requires descending inhibitory pathways [73] and is partly mediated by opioids [107, 112]. Tapentadol, a MOR agonist and noradrenaline reuptake inhibitor [113], can restore DNIC/CPM [108, 114]. The effects of tapentadol are mostly attributed to a synergistic effect of MOR activation and the inhibition of noradrenaline reuptake at the SC [115]. In the SC, MOR serves as an interface for ascending inhibition and descending opioidergic inhibition triggered from PAG-RVM [70, 116].
During neuropathic pain, the endogenous opioid peptides and opioid receptors are altered at the supraspinal pain control system. Local release of endogenous opioids was shown in cortical and subcortical brain areas of patients with persistent neuropathic pain [117–120]. In animal models of neuropathic pain, increased ENK peptide levels were detected in several components of the supraspinal pain control system, including the PAG, RVM, and DRt [121, 122]. The role of endogenous opioid peptides has been studied using knockout mice deficient in opioid-encoding genes revealing deficits in supraspinal modulation [123]. Dynorphin knockouts showed the involvement of dynorphins in the affective component of pain. Increased release of dynorphin together with increased KOR signaling was recently detected in the mesolimbic circuit and amygdala, and the upregulation of this system was responsible for mediating the aversiveness/unpleasantness of neuropathic pain [124, 125]. The role of dynorphin in the amygdalo-parabrachial pathway and its involvement in emotional and pain control were recently proposed [126]. The studies performed in β-endorphin knockout mice suggest that the continuous release of β-endorphin induces activation of MOR and subsequent phosphorylation and desensitization [127–129]. Mice lacking proenkephalin and/or β-endorphin showed that these peptides modulate the activity and the levels of MOR, DOR, and KOR in descending pain control areas of the brainstem [130]. Neuropathic pain is associated with a reduction in MOR function in the brainstem, with decreased activation of G proteins likely due to increased phosphorylation of the receptor, leading to its desensitization [131]. Reduced MOR-mediated G-protein activity was shown in the PAG [132] and RVM [133] of neuropathic pain models. At the DRt, we recently showed that neuropathic pain leads to increased release of ENK peptides and desensitization of MOR [105]. Additionally, we showed a reduction in protein levels of MOR and an increase in phosphorylation of MOR [105]. The reduction in MOR protein was likely associated with increased phosphorylation, leading to desensitization and subsequent degradation of the receptor, since no alteration in mRNA levels of MOR was detected [105]. These molecular adaptations of MOR impair the analgesic function of MOR at the DRt [105]. Neuropathic pain is associated with altered expression of the opioid receptors [105, 133] at several supraspinal pain modulatory areas. Downregulation of MOR in the brain seems to be common during neuropathic pain [134, 135]. In patients with neuropathic pain, reduced MOR availability was observed in cortical brain areas involved in pain modulation and in the PAG [119, 120, 136]. DOR was also found to be downregulated in the RVM after nerve injury [133]. Recent characterization of DOR confirmed its relevance in the development of neuropathic pain. DOR knockouts developed increased thermal and mechanical sensitivities in neuropathic pain models [137], suggesting a protective role of DOR. At the RVM, the downregulation of DOR together with the desensitization of MOR [133] likely contributes to a decrease in descending opioidergic inhibition. The downregulation of MOR at SC and descending pain modulatory areas likely contributes to the reduced potency of morphine in the neuropathic pain state [105, 138, 139].
Clinical Implications of the Alterations in Descending Modulatory Systems During Neuropathic Pain
The treatment of neuropathic pain remains a challenge since many drugs show inadequate analgesia and considerable side effects. The studies of brainstem pain modulation in preclinical models of neuropathic pain unraveled some mechanisms that may account for the inadequate analgesia and indicate possible approaches to overpass the challenges of neuropathic pain management. Drugs with a primary function other than analgesia are used for neuropathic pain treatment, such as antidepressants that act upon the reuptake of serotonin and noradrenaline [140]. Antidepressants that act at the serotoninergic system have their net analgesic efficacy reduced by the increased activity of spinal 5-HT receptors involved in pain facilitation, namely, 5-HT3 [32, 44–46]. Regarding antidepressants that also inhibit noradrenaline reuptake, such as duloxetine [141], the analgesic effects due to inhibition of α2-AR at the SC may be attenuated by the pronociceptive effects of noradrenaline at supraspinal pain control centers [13, 14].
Weak and strong opioids are recommended as second- and third-line treatments, respectively, mainly because of lack of efficacy and safety concerns [reviewed by [140, 142, 143]]. The lack of effectiveness of MOR-acting drugs in neuropathic pain might be because both neuropathic pain and opioid treatment lead to desensitization of and tolerance to MOR [144]. Tolerance to opioids leads to increasing doses of opioids, which is critical as this can lead to opioid-induced hyperalgesia [145]. Chronic morphine treatment induces a shift of MOR signaling from inhibitory to excitatory at the DRt, enhancing DF from the DRt [146]. These findings indicate that the cumulative effects of neuropathic pain and opioid drugs on MOR are counterproductive. In the subsequent years, the study of the brainstem pain control system needs to have a more translational perspective. The effects of descending modulation should also consider interactions occurring at both local neurochemical modulation of supraspinal pain control circuits and between pain control centers of the brain. Imaging studies in human subjects have recently shown changes in the functional connectivity of pain control centers of the brain [147], and this “connectome perspective” should also be considered in the basic pain research studies. The involvement of opioids in the control of the connections and as a trigger of opioid-induced hyperalgesia should be considered. These perspectives may allow to overcome the current gaps in research studies deriving from the moderate translation of basic studies of brainstem pain modulatory circuits.
Author Contributions
IT and IM contributed to the initial conception of the paper. JC-P wrote the first draft of the 2 initial sections of the manuscript. IM and IT wrote the third and fourth sections, respectively. All authors contributed to manuscript revision and approved the submitted version.
Conflict of Interest
The authors declare that the research was conducted in the absence of any commercial or financial relationships that could be construed as a potential conflict of interest.
References
1. Melzack R, and Wall PD. Pain mechanisms: a new theory. Science. (1965) 150:971–9. doi: 10.1126/science.150.3699.971
2. Bandler R, and Keay KA. Columnar organization in the midbrain periaqueductal gray and the integration of emotional expression. Prog Brain Res. (1996) 107:285–300. doi: 10.1016/S0079-6123(08)61871-3
3. Behbehani MM, and Fields HL. Evidence that an excitatory connection between the periaqueductal gray and nucleus raphe magnus mediates stimulation produced analgesia. Brain Res. (1979) 170:85–93. doi: 10.1016/0006-8993(79)90942-9
4. Bowker RM, Steinbusch HW, and Coulter JD. Serotonergic and peptidergic projections to the spinal cord demonstrated by a combined retrograde HRP histochemical and immunocytochemical staining method. Brain Res. (1981) 211:412–7. doi: 10.1016/0006-8993(81)90965-3
5. Braz JM, and Basbaum AI. Genetically expressed transneuronal tracer reveals direct and indirect serotonergic descending control circuits. J Comp Neurol. (2008) 507:1990–2003. doi: 10.1002/cne.21665
6. Westlund KN, Bowker RM, Ziegler MG, and Coulter JD. Noradrenergic projections to the spinal cord of the rat. Brain Res. (1983) 263:15–31. doi: 10.1016/0006-8993(83)91196-4
7. Tavares I, Lima D, and Coimbra A. The ventrolateral medulla of the rat is connected with the spinal cord dorsal horn by an indirect descending pathway relayed in the A5 noradrenergic cell group. J Comp Neurol. (1996) 374:84–95. doi: 10.1002/(SICI)1096-9861(19961007)374:1<84::AID-CNE6>3.0.CO;2-J
8. Clark FM, and Proudfit HK. The projections of noradrenergic neurons in the A5 catecholamine cell group to the spinal cord in the rat: anatomical evidence that A5 neurons modulate nociception. Brain Res. (1993) 616:200–10. doi: 10.1016/0006-8993(93)90210-E
9. Kwiat GC, and Basbaum AI. The origin of brainstem noradrenergic and serotonergic projections to the spinal cord dorsal horn in the rat. Somatosens Mot Res. (1992) 9:157–73. doi: 10.3109/08990229209144768
10. Pertovaara A. Noradrenergic pain modulation. Prog Neurobiol. (2006) 80:53–83. doi: 10.1016/j.pneurobio.2006.08.001
11. Martins I, and Tavares I. Reticular formation and pain: the past and the future. Front Neuroanat. (2017) 11:51. doi: 10.3389/fnana.2017.00051
12. Lima D, and Almeida A. The medullary dorsal reticular nucleus as a pronociceptive centre of the pain control system. Prog Neurobiol. (2002) 66:81–108. doi: 10.1016/S0301-0082(01)00025-9
13. Martins I, Costa-Araujo S, Fadel J, Wilson SP, Lima D, and Tavares I. Reversal of neuropathic pain by HSV-1-mediated decrease of noradrenaline in a pain facilitatory area of the brain. Pain. (2010) 151:137–45. doi: 10.1016/j.pain.2010.06.027
14. Martins I, Carvalho P, de Vries MG, Teixeira-Pinto A, Wilson SP, Westerink BH, et al. Increased noradrenergic neurotransmission to a pain facilitatory area of the brain is implicated in facilitation of chronic pain. Anesthesiology. (2015) 123:642–53. doi: 10.1097/ALN.0000000000000749
15. Sotgiu ML, Valente M, Storchi R, Caramenti G, and Mario Biella GE. Contribution by DRt descending facilitatory pathways to maintenance of spinal neuron sensitization in rats. Brain Res. (2008) 1188:69–75. doi: 10.1016/j.brainres.2007.10.030
16. Fields HL, Bry J, Hentall I, and Zorman G. The activity of neurons in the rostral medulla of the rat during withdrawal from noxious heat. J Neurosci. (1983) 3:2545–52. doi: 10.1523/JNEUROSCI.03-12-02545.1983
17. Heinricher MM, Tavares I, Leith JL, and Lumb BM. Descending control of nociception: Specificity, recruitment and plasticity. Brain Res Rev. (2009) 60:214–25. doi: 10.1016/j.brainresrev.2008.12.009
18. Silva M, Amorim D, Almeida A, Tavares I, Pinto-Ribeiro F, and Morgado C. Pronociceptive changes in the activity of rostroventromedial medulla (RVM) pain modulatory cells in the streptozotocin-diabetic rat. Brain Res Bull. (2013) 96:39–44. doi: 10.1016/j.brainresbull.2013.04.008
19. Carlson JD, Maire JJ, Martenson ME, and Heinricher MM. Sensitization of pain-modulating neurons in the rostral ventromedial medulla after peripheral nerve injury. J Neurosci. (2007) 27:13222–31. doi: 10.1523/JNEUROSCI.3715-07.2007
20. Goncalves L, Almeida A, and Pertovaara A. Pronociceptive changes in response properties of rostroventromedial medullary neurons in a rat model of peripheral neuropathy. Eur J Neurosci. (2007) 26:2188–95. doi: 10.1111/j.1460-9568.2007.05832.x
21. Hirschberg S, Li Y, Randall A, Kremer EJ, and Pickering AE. Functional dichotomy in spinal- vs prefrontal-projecting locus coeruleus modules splits descending noradrenergic analgesia from ascending aversion and anxiety in rats. Elife. (2017) 6:e29808. doi: 10.7554/eLife.29808
22. Paxinos G, and Watson C. The Rat Brain in Stereotaxic Coordinates. 6th ed. Amsterdam Oxford: Elsevier Academic (2007). 420 p.
23. Millan MJ. Descending control of pain. Prog Neurobiol. (2002) 66:355–474. doi: 10.1016/S0301-0082(02)00009-6
24. Tracey I, and Mantyh PW. The cerebral signature for pain perception and its modulation. Neuron. (2007) 55:377–91. doi: 10.1016/j.neuron.2007.07.012
25. Okubo M, Castro A, Guo W, Zou S, Ren K, Wei F, et al. Transition to persistent orofacial pain after nerve injury involves supraspinal serotonin mechanisms. J Neurosci. (2013) 33:5152–61. doi: 10.1523/JNEUROSCI.3390-12.2013
26. Wei F, Dubner R, Zou S, Ren K, Bai G, Wei D, et al. Molecular depletion of descending serotonin unmasks its novel facilitatory role in the development of persistent pain. J Neurosci. (2010) 30:8624–36. doi: 10.1523/JNEUROSCI.5389-09.2010
27. Suzuki R, Rahman W, Hunt SP, and Dickenson AH. Descending facilitatory control of mechanically evoked responses is enhanced in deep dorsal horn neurones following peripheral nerve injury. Brain Res. (2004) 1019:68–76. doi: 10.1016/j.brainres.2004.05.108
28. Rahman W, Suzuki R, Webber M, Hunt SP, and Dickenson AH. Depletion of endogenous spinal 5-HT attenuates the behavioural hypersensitivity to mechanical and cooling stimuli induced by spinal nerve ligation. Pain. (2006) 123:264–74. doi: 10.1016/j.pain.2006.02.033
29. Morgado C, Silva L, Pereira-Terra P, and Tavares I. Changes in serotoninergic and noradrenergic descending pain pathways during painful diabetic neuropathy: the preventive action of IGF1. Neurobiol Dis. (2011) 43:275–84. doi: 10.1016/j.nbd.2011.04.001
30. Satoh O, and Omote K. Roles of monoaminergic, glycinergic and GABAergic inhibitory systems in the spinal cord in rats with peripheral mononeuropathy. Brain Res. (1996) 728:27–36. doi: 10.1016/0006-8993(96)00371-X
31. Costa-Pereira JT, Serrao P, Martins I, and Tavares I. Serotoninergic pain modulation from the rostral ventromedial medulla (RVM) in chemotherapy-induced neuropathy: the role of spinal 5-HT3 receptors. Eur J Neurosci. (2020) 51:1756–69. doi: 10.1111/ejn.14614
32. Dogrul A, Ossipov MH, and Porreca F. Differential mediation of descending pain facilitation and inhibition by spinal 5HT-3 and 5HT-7 receptors. Brain Res. (2009) 1280:52–9. doi: 10.1016/j.brainres.2009.05.001
33. Viisanen H, and Pertovaara A. Roles of the rostroventromedial medulla and the spinal 5-HT(1A) receptor in descending antinociception induced by motor cortex stimulation in the neuropathic rat. Neurosci Lett. (2010) 476:133–7. doi: 10.1016/j.neulet.2010.04.014
34. Rahman W, Bannister K, Bee LA, and Dickenson AH. A pronociceptive role for the 5-HT2 receptor on spinal nociceptive transmission: an in vivo electrophysiological study in the rat. Brain Res. (2011) 1382:29–36. doi: 10.1016/j.brainres.2011.01.057
35. Hu B, Doods H, Treede RD, and Ceci A. Duloxetine and 8-OH-DPAT, but not fluoxetine, reduce depression-like behaviour in an animal model of chronic neuropathic pain. Neurosci Lett. (2016) 619:162–7. doi: 10.1016/j.neulet.2016.03.019
36. Di Cesare Mannelli L, Ghelardini C, Micheli L, Del Bello F, Giannella M, Piergentili A, et al. Synergic stimulation of serotonin 5-HT1A receptor and alpha2-adrenoceptors for neuropathic pain relief: preclinical effects of 2-substituted imidazoline derivatives. Eur J Pharmacol. (2017) 810:128–33. doi: 10.1016/j.ejphar.2017.06.023
37. Nadeson R, and Goodchild CS. Antinociceptive role of 5-HT1A receptors in rat spinal cord. Br J Anaesth. (2002) 88:679–84. doi: 10.1093/bja/88.5.679
38. Jesus CHA, Redivo DDB, Gasparin AT, Sotomaior BB, de Carvalho MC, Genaro K, et al. Cannabidiol attenuates mechanical allodynia in streptozotocin-induced diabetic rats via serotonergic system activation through 5-HT1A receptors. Brain Res. (2019) 1715:156–64. doi: 10.1016/j.brainres.2019.03.014
39. Berrocoso E, and Mico JA. Role of serotonin 5-HT1A receptors in the antidepressant-like effect and the antinociceptive effect of venlafaxine in mice. Int J Neuropsychopharmacol. (2009) 12:61–71. doi: 10.1017/S1461145708008766
40. Newman-Tancredi A, Bardin L, Auclair A, Colpaert F, Depoortere R, and Varney MA. NLX-112, a highly selective 5-HT1A receptor agonist, mediates analgesia and antidepressant-like activity in rats via spinal cord and prefrontal cortex 5-HT1A receptors, respectively. Brain Res. (2018) 1688:1–7. doi: 10.1016/j.brainres.2018.03.016
41. Santello M, Bisco A, Nevian NE, Lacivita E, Leopoldo M, and Nevian T. The brain-penetrant 5-HT7 receptor agonist LP-211 reduces the sensory and affective components of neuropathic pain. Neurobiol Dis. (2017) 106:214–21. doi: 10.1016/j.nbd.2017.07.005
42. Zhao X, Wang C, Cui WG, Ma Q, and Zhou WH. Fisetin exerts antihyperalgesic effect in a mouse model of neuropathic pain: engagement of spinal serotonergic system. Sci Rep. (2015) 5:9043. doi: 10.1038/srep09043
43. Lee HG, Song JA, Han DS, Woo KW, and Yoon MH. Antiallodynic Effects of Intrathecal Areca Nut for Spinal Nerve-Ligated and Chemotherapy-Induced Neuropathic Pain in Rats. Pharmacology. (2018) 102:332–8. doi: 10.1159/000492394
44. Dam LJ, Hai L, and Ha YM. Role of the 5-HT(7) receptor in the effects of intrathecal nefopam in neuropathic pain in rats. Neurosci Lett. (2014) 566:50–4. doi: 10.1016/j.neulet.2014.02.021
45. Thibault K, Van Steenwinckel J, Brisorgueil MJ, Fischer J, Hamon M, Calvino B, et al. Serotonin 5-HT2A receptor involvement and Fos expression at the spinal level in vincristine-induced neuropathy in the rat. Pain. (2008) 140:305–22. doi: 10.1016/j.pain.2008.09.006
46. Aira Z, Buesa I, Salgueiro M, Bilbao J, Aguilera L, Zimmermann M, et al. Subtype-specific changes in 5-HT receptor-mediated modulation of C fibre-evoked spinal field potentials are triggered by peripheral nerve injury. Neuroscience. (2010) 168:831–41. doi: 10.1016/j.neuroscience.2010.04.032
47. Silva M, Costa-Pereira JT, Martins D, and Tavares I. Pain modulation from the brain during diabetic neuropathy: uncovering the role of the rostroventromedial medulla. Neurobiol Dis. (2016) 96:346–56. doi: 10.1016/j.nbd.2016.10.002
48. Chen Y, Oatway MA, and Weaver LC. Blockade of the 5-HT3 receptor for days causes sustained relief from mechanical allodynia following spinal cord injury. J Neurosci Res. (2009) 87:418–24. doi: 10.1002/jnr.21860
49. Guo W, Miyoshi K, Dubner R, Gu M, Li M, Liu J, et al. Spinal 5-HT3 receptors mediate descending facilitation and contribute to behavioral hypersensitivity via a reciprocal neuron-glial signaling cascade. Mol Pain. (2014) 10:35. doi: 10.1186/1744-8069-10-35
50. Bannister K, and Dickenson AH. The plasticity of descending controls in pain: translational probing. J Physiol. (2017) 595:4159–66. doi: 10.1113/JP274165
51. Zhuo M, Sengupta JN, and Gebhart GF. Biphasic modulation of spinal visceral nociceptive transmission from the rostroventral medial medulla in the rat. J Neurophysiol. (2002) 87:2225–36. doi: 10.1152/jn.2002.87.5.2225
52. Wei H, and Pertovaara A. Spinal and pontine alpha2-adrenoceptors have opposite effects on pain-related behavior in the neuropathic rat. Eur J Pharmacol. (2006) 551:41–9. doi: 10.1016/j.ejphar.2006.08.064
53. Viisanen H, and Pertovaara A. Influence of peripheral nerve injury on response properties of locus coeruleus neurons and coeruleospinal antinociception in the rat. Neuroscience. (2007) 146:1785–94. doi: 10.1016/j.neuroscience.2007.03.016
54. Alba-Delgado C, Mico JA, Sanchez-Blazquez P, and Berrocoso E. Analgesic antidepressants promote the responsiveness of locus coeruleus neurons to noxious stimulation: implications for neuropathic pain. Pain. (2012) 153:1438–49. doi: 10.1016/j.pain.2012.03.034
55. Alba-Delgado C, Borges G, Sanchez-Blazquez P, Ortega JE, Horrillo I, Mico JA, et al. The function of alpha-2-adrenoceptors in the rat locus coeruleus is preserved in the chronic constriction injury model of neuropathic pain. Psychopharmacology (Berl). (2012) 221:53–65. doi: 10.1007/s00213-011-2542-7
56. Taylor BK, and Westlund KN. The noradrenergic locus coeruleus as a chronic pain generator. J Neurosci Res. (2017) 95:1336–46. doi: 10.1002/jnr.23956
57. Alba-Delgado C, Mico JA, and Berrocoso E. Neuropathic pain increases spontaneous and noxious-evoked activity of locus coeruleus neurons. Prog Neuropsychopharmacol Biol Psychiatry. (2021) 105:110121. doi: 10.1016/j.pnpbp.2020.110121
58. Alba-Delgado C, Llorca-Torralba M, Horrillo I, Ortega JE, Mico JA, Sanchez-Blazquez P, et al. Chronic pain leads to concomitant noradrenergic impairment and mood disorders. Biol Psychiatry. (2013) 73:54–62. doi: 10.1016/j.biopsych.2012.06.033
59. Tazawa T, Kamiya Y, Kobayashi A, Saeki K, Takiguchi M, Nakahashi Y, et al. Spinal cord stimulation modulates supraspinal centers of the descending antinociceptive system in rats with unilateral spinal nerve injury. Mol Pain. (2015) 11:36. doi: 10.1186/s12990-015-0039-9
60. Wei H, and Pertovaara A. Regulation of neuropathic hypersensitivity by alpha(2) -adrenoceptors in the pontine A7 cell group. Basic Clin Pharmacol Toxicol. (2013) 112:90–5. doi: 10.1111/j.1742-7843.2012.00930.x
61. Ma W, and Eisenach JC. Chronic constriction injury of sciatic nerve induces the up-regulation of descending inhibitory noradrenergic innervation to the lumbar dorsal horn of mice. Brain Res. (2003) 970:110–8. doi: 10.1016/S0006-8993(03)02293-5
62. Bantel C, Eisenach JC, Duflo F, Tobin JR, and Childers SR. Spinal nerve ligation increases alpha2-adrenergic receptor G-protein coupling in the spinal cord. Brain Res. (2005) 1038:76–82. doi: 10.1016/j.brainres.2005.01.016
63. Chen SR, Pan HM, Richardson TE, and Pan HL. Potentiation of spinal alpha(2)-adrenoceptor analgesia in rats deficient in TRPV1-expressing afferent neurons. Neuropharmacology. (2007) 52:1624–30. doi: 10.1016/j.neuropharm.2007.03.009
64. Hayashida K, Clayton BA, Johnson JE, and Eisenach JC. Brain derived nerve growth factor induces spinal noradrenergic fiber sprouting and enhances clonidine analgesia following nerve injury in rats. Pain. (2008) 136:348–55. doi: 10.1016/j.pain.2007.07.014
65. Rojo ML, Rodriguez-Gaztelumendi A, Pazos A, and Diaz A. Differential adaptive changes on serotonin and noradrenaline transporters in a rat model of peripheral neuropathic pain. Neurosci Lett. (2012) 515:181–6. doi: 10.1016/j.neulet.2012.03.050
66. Hughes SW, Hickey L, Hulse RP, Lumb BM, and Pickering AE. Endogenous analgesic action of the pontospinal noradrenergic system spatially restricts and temporally delays the progression of neuropathic pain following tibial nerve injury. Pain. (2013) 154:1680–90. doi: 10.1016/j.pain.2013.05.010
67. Costa-Pereira JT, Ribeiro J, Martins I, and Tavares I. Role of spinal cord alpha2-adrenoreceptors in noradrenergic inhibition of nociceptive transmission during chemotherapy-induced peripheral neuropathy. Front Neurosci. (2019) 13:1413. doi: 10.3389/fnins.2019.01413
68. Kohro Y, Matsuda T, Yoshihara K, Kohno K, Koga K, Katsuragi R, et al. Spinal astrocytes in superficial laminae gate brainstem descending control of mechanosensory hypersensitivity. Nat Neurosci. (2020) 23:1376–87. doi: 10.1038/s41593-020-00713-4
69. Mills EP, Alshelh Z, Kosanovic D, Di Pietro F, Vickers ER, Macey PM, et al. Altered brainstem pain-modulation circuitry connectivity during spontaneous pain intensity fluctuations. J Pain Res. (2020) 13:2223–35. doi: 10.2147/JPR.S252594
70. Corder G, Castro DC, Bruchas MR, and Scherrer G. Endogenous and exogenous opioids in pain. Annu Rev Neurosci. (2018) 41:453–73. doi: 10.1146/annurev-neuro-080317-061522
71. Gibula-Tarlowska E, and Kotlinska JH. Crosstalk between opioid and anti-opioid systems: an overview and its possible therapeutic significance. Biomolecules. (2020) 10:1376. doi: 10.3390/biom10101376
72. Bagley EE, and Ingram SL. Endogenous opioid peptides in the descending pain modulatory circuit. Neuropharmacology. (2020) 173:108131. doi: 10.1016/j.neuropharm.2020.108131
73. Bannister K, and Dickenson AH. Central nervous system targets: supraspinal mechanisms of analgesia. Neurotherapeutics. (2020) 17:839–45. doi: 10.1007/s13311-020-00887-6
74. Kim JH, Gangadharan G, Byun J, Choi EJ, Lee CJ, and Shin HS. Yin-and-yang bifurcation of opioidergic circuits for descending analgesia at the midbrain of the mouse. Proc Natl Acad Sci USA. (2018) 115:11078–83. doi: 10.1073/pnas.1806082115
75. Samineni VK, Grajales-Reyes JG, Copits BA, O'Brien DE, Trigg SL, Gomez AM, et al. Divergent Modulation of Nociception by Glutamatergic and GABAergic Neuronal Subpopulations in the Periaqueductal Gray. eNeuro. (2017) 4:ENEURO.0129-16.2017. doi: 10.1523/ENEURO.0129-16.2017
76. Francois A, Low SA, Sypek EI, Christensen AJ, Sotoudeh C, Beier KT, et al. A brainstem-spinal cord inhibitory circuit for mechanical pain modulation by GABA and enkephalins. Neuron. (2017) 93:822–39 e6. doi: 10.1016/j.neuron.2017.01.008
77. Zhang Y, Zhao S, Rodriguez E, Takatoh J, Han BX, Zhou X, et al. Identifying local and descending inputs for primary sensory neurons. J Clin Invest. (2015) 125:3782–94. doi: 10.1172/JCI81156
78. Commons KG, Beck SG, Rudoy C, and Van Bockstaele EJ. Anatomical evidence for presynaptic modulation by the delta opioid receptor in the ventrolateral periaqueductal gray of the rat. J Comp Neurol. (2001) 430:200–8. doi: 10.1002/1096-9861(20010205)430:2<200::AID-CNE1025>3.0.CO;2-B
79. Harasawa I, Fields HL, and Meng ID. Delta opioid receptor mediated actions in the rostral ventromedial medulla on tail flick latency and nociceptive modulatory neurons. Pain. (2000) 85:255–62. doi: 10.1016/S0304-3959(99)00280-8
80. Quirion B, Bergeron F, Blais V, and Gendron L. The delta-opioid receptor a target for the treatment of pain. Front Mol Neurosci. (2020) 13:52. doi: 10.3389/fnmol.2020.00052
81. Bie B, and Pan ZZ. Presynaptic mechanism for anti-analgesic and anti-hyperalgesic actions of kappa-opioid receptors. J Neurosci. (2003) 23:7262–8. doi: 10.1523/JNEUROSCI.23-19-07262.2003
82. Meng ID, Johansen JP, Harasawa I, and Fields HL. Kappa opioids inhibit physiologically identified medullary pain modulating neurons and reduce morphine antinociception. J Neurophysiol. (2005) 93:1138–44. doi: 10.1152/jn.00320.2004
83. Schroder W, Lambert DG, Ko MC, and Koch T. Functional plasticity of the N/OFQ-NOP receptor system determines analgesic properties of NOP receptor agonists. Br J Pharmacol. (2014) 171:3777–800. doi: 10.1111/bph.12744
84. Pan Z, Hirakawa N, and Fields HL. A cellular mechanism for the bidirectional pain-modulating actions of orphanin FQ/nociceptin. Neuron. (2000) 26:515–22. doi: 10.1016/S0896-6273(00)81183-6
85. Vaughan CW, Connor M, Jennings EA, Marinelli S, Allen RG, and Christie MJ. Actions of nociceptin/orphanin FQ and other prepronociceptin products on rat rostral ventromedial medulla neurons in vitro. J Physiol. (2001) 534(Pt 3):849–59. doi: 10.1111/j.1469-7793.2001.00849.x
86. Toll L, Bruchas MR, Calo G, Cox BM, and Zaveri NT. Nociceptin/Orphanin FQ receptor structure, signaling, ligands, functions, and interactions with opioid systems. Pharmacol Rev. (2016) 68:419–57. doi: 10.1124/pr.114.009209
87. Ossipov MH, Morimura K, and Porreca F. Descending pain modulation and chronification of pain. Curr Opin Support Palliat Care. (2014) 8:143–51. doi: 10.1097/SPC.0000000000000055
88. Kiguchi N, Ding H, and Ko MC. Central N/OFQ-NOP Receptor System in Pain Modulation. Adv Pharmacol. (2016) 75:217–43. doi: 10.1016/bs.apha.2015.10.001
89. Kiguchi N, Ding H, and Ko MC. Therapeutic potentials of NOP and MOP receptor coactivation for the treatment of pain and opioid abuse. J Neurosci Res. (2020) 1–12. doi: 10.1002/jnr.24624
90. Ding YQ, Kaneko T, Nomura S, and Mizuno N. Immunohistochemical localization of mu-opioid receptors in the central nervous system of the rat. J Comp Neurol. (1996) 367:375–402. doi: 10.1002/(SICI)1096-9861(19960408)367:3<375::AID-CNE5>3.0.CO;2-2
91. Williams JT, Ingram SL, Henderson G, Chavkin C, von Zastrow M, Schulz S, et al. Regulation of mu-opioid receptors: desensitization, phosphorylation, internalization, and tolerance. Pharmacol Rev. (2013) 65:223–54. doi: 10.1124/pr.112.005942
92. Nestler EJ, Alreja M, and Aghajanian GK. Molecular control of locus coeruleus neurotransmission. Biol Psychiatry. (1999) 46:1131–9. doi: 10.1016/S0006-3223(99)00158-4
93. Pertovaara A. The noradrenergic pain regulation system: a potential target for pain therapy. Eur J Pharmacol. (2013) 716:2–7. doi: 10.1016/j.ejphar.2013.01.067
94. Lemos Duarte M, and Devi LA. Post-translational modifications of opioid receptors. Trends Neurosci. (2020) 43:417–32. doi: 10.1016/j.tins.2020.03.011
95. Winters BL, Gregoriou GC, Kissiwaa SA, Wells OA, Medagoda DI, Hermes SM, et al. Endogenous opioids regulate moment-to-moment neuronal communication and excitability. Nat Commun. (2017) 8:14611. doi: 10.1038/ncomms14611
96. Morales L, Perez-Garcia C, and Alguacil LF. Effects of yohimbine on the antinociceptive and place conditioning effects of opioid agonists in rodents. Br J Pharmacol. (2001) 133:172–8. doi: 10.1038/sj.bjp.0704057
97. Ossipov MH, Harris S, Lloyd P, and Messineo E. An isobolographic analysis of the antinociceptive effect of systemically and intrathecally administered combinations of clonidine and opiates. J Pharmacol Exp Ther. (1990) 255:1107–16.
98. Ossipov MH, Suarez LJ, and Spaulding TC. Antinociceptive interactions between alpha 2-adrenergic and opiate agonists at the spinal level in rodents. Anesth Analg. (1989) 68:194–200. doi: 10.1213/00000539-198903000-00002
99. Yaksh TL. Pharmacology of spinal adrenergic systems which modulate spinal nociceptive processing. Pharmacol Biochem Behav. (1985) 22:845–58. doi: 10.1016/0091-3057(85)90537-4
100. Mansour A, Fox CA, Burke S, Meng F, Thompson RC, Akil H, et al. Mu, delta, and kappa opioid receptor mRNA expression in the rat CNS: an in situ hybridization study. J Comp Neurol. (1994) 350:412–38. doi: 10.1002/cne.903500307
101. Kliewer A, Schmiedel F, Sianati S, Bailey A, Bateman JT, Levitt ES, et al. Phosphorylation-deficient G-protein-biased mu-opioid receptors improve analgesia and diminish tolerance but worsen opioid side effects. Nat Commun. (2019) 10:367. doi: 10.1038/s41467-018-08162-1
102. Pinto M, Castro AR, Tshudy F, Wilson SP, Lima D, and Tavares I. Opioids modulate pain facilitation from the dorsal reticular nucleus. Mol Cell Neurosci. (2008) 39:508–18. doi: 10.1016/j.mcn.2008.07.008
103. Pinto M, Sousa M, Lima D, and Tavares I. Participation of mu-opioid, GABA(B), and NK1 receptors of major pain control medullary areas in pathways targeting the rat spinal cord: implications for descending modulation of nociceptive transmission. J Comp Neurol. (2008) 510:175–87. doi: 10.1002/cne.21793
104. Martins I, Pinto M, Wilson SP, Lima D, and Tavares I. Dynamic of migration of HSV-1 from a medullary pronociceptive centre: antinociception by overexpression of the preproenkephalin transgene. Eur J Neurosci. (2008) 28:2075–83. doi: 10.1111/j.1460-9568.2008.06492.x
105. Costa AR, Carvalho P, Flik G, Wilson SP, Reguenga C, Martins I, et al. Neuropathic Pain Induced Alterations in the Opioidergic Modulation of a Descending Pain Facilitatory Area of the Brain. Front Cell Neurosci. (2019) 13:287. doi: 10.3389/fncel.2019.00287
106. De Felice M, Sanoja R, Wang R, Vera-Portocarrero L, Oyarzo J, King T, et al. Engagement of descending inhibition from the rostral ventromedial medulla protects against chronic neuropathic pain. Pain. (2011) 152:2701–9. doi: 10.1016/j.pain.2011.06.008
107. Phelps CE, Navratilova E, Dickenson AH, Porreca F, and Bannister K. Kappa opioid signaling in the right central amygdala causes hind paw specific loss of diffuse noxious inhibitory controls in experimental neuropathic pain. Pain. (2019) 160:1614–21. doi: 10.1097/j.pain.0000000000001553
108. Bannister K, Patel R, Goncalves L, Townson L, and Dickenson AH. Diffuse noxious inhibitory controls and nerve injury: restoring an imbalance between descending monoamine inhibitions and facilitations. Pain. (2015) 156:1803–11. doi: 10.1097/j.pain.0000000000000240
109. Gruener H, Zeilig G, Laufer Y, Blumen N, and Defrin R. Differential pain modulation properties in central neuropathic pain after spinal cord injury. Pain. (2016) 157:1415–24. doi: 10.1097/j.pain.0000000000000532
110. Suzan E, Treister R, Pud D, Haddad M, and Eisenberg E. The effect of hydromorphone therapy on psychophysical measurements of the descending inhibitory pain systems in patients with chronic radicular pain. Pain Med. (2015) 16:168–75. doi: 10.1111/pme.12565
111. Tuveson B, Leffler AS, and Hansson P. Heterotopic noxious conditioning stimulation (HNCS) reduced the intensity of spontaneous pain, but not of allodynia in painful peripheral neuropathy. Eur J Pain. (2007) 11:452–62. doi: 10.1016/j.ejpain.2006.06.007
112. de Resende MA, Silva LF, Sato K, Arendt-Nielsen L, and Sluka KA. Blockade of opioid receptors in the medullary reticularis nucleus dorsalis, but not the rostral ventromedial medulla, prevents analgesia produced by diffuse noxious inhibitory control in rats with muscle inflammation. J Pain. (2011) 12:687–97. doi: 10.1016/j.jpain.2010.12.009
113. Romualdi P, Grilli M, Canonico PL, Collino M, and Dickenson AH. Pharmacological rationale for tapentadol therapy: a review of new evidence. J Pain Res. (2019) 12:1513–20. doi: 10.2147/JPR.S190160
114. Niesters M, Proto PL, Aarts L, Sarton EY, Drewes AM, and Dahan A. Tapentadol potentiates descending pain inhibition in chronic pain patients with diabetic polyneuropathy. Br J Anaesth. (2014) 113:148–56. doi: 10.1093/bja/aeu056
115. Bee LA, Bannister K, Rahman W, and Dickenson AH. Mu-opioid and noradrenergic alpha(2)-adrenoceptor contributions to the effects of tapentadol on spinal electrophysiological measures of nociception in nerve-injured rats. Pain. (2011) 152:131–9. doi: 10.1016/j.pain.2010.10.004
116. Sun J, Chen SR, and Pan HL. mu-Opioid receptors in primary sensory neurons are involved in supraspinal opioid analgesia. Brain Res. (2020) 1729:146623. doi: 10.1016/j.brainres.2019.146623
117. Harris RE, Clauw DJ, Scott DJ, McLean SA, Gracely RH, and Zubieta JK. Decreased central mu-opioid receptor availability in fibromyalgia. J Neurosci. (2007) 27:10000–6. doi: 10.1523/JNEUROSCI.2849-07.2007
118. Jones AK, Kitchen ND, Watabe H, Cunningham VJ, Jones T, Luthra SK, et al. Measurement of changes in opioid receptor binding in vivo during trigeminal neuralgic pain using [11C] diprenorphine and positron emission tomography. J Cereb Blood Flow Metab. (1999) 19:803–8. doi: 10.1097/00004647-199907000-00011
119. Maarrawi J, Peyron R, Mertens P, Costes N, Magnin M, Sindou M, et al. Differential brain opioid receptor availability in central and peripheral neuropathic pain. Pain. (2007) 127:183–94. doi: 10.1016/j.pain.2006.10.013
120. Willoch F, Schindler F, Wester HJ, Empl M, Straube A, Schwaiger M, et al. Central poststroke pain and reduced opioid receptor binding within pain processing circuitries: a [11C]diprenorphine PET study. Pain. (2004) 108:213–20. doi: 10.1016/j.pain.2003.08.014
121. Hurley RW, and Hammond DL. Contribution of endogenous enkephalins to the enhanced analgesic effects of supraspinal mu opioid receptor agonists after inflammatory injury. J Neurosci. (2001) 21:2536–45. doi: 10.1523/JNEUROSCI.21-07-02536.2001
122. Williams FG, Mullet MA, and Beitz AJ. Basal release of Met-enkephalin and neurotensin in the ventrolateral periaqueductal gray matter of the rat: a microdialysis study of antinociceptive circuits. Brain Res. (1995) 690:207–16. doi: 10.1016/0006-8993(95)00554-4
123. Maldonado R, Banos JE, and Cabanero D. Usefulness of knockout mice to clarify the role of the opioid system in chronic pain. Br J Pharmacol. (2018) 175:2791–808. doi: 10.1111/bph.14088
124. Navratilova E, Ji G, Phelps C, Qu C, Hein M, Yakhnitsa V, et al. Kappa opioid signaling in the central nucleus of the amygdala promotes disinhibition and aversiveness of chronic neuropathic pain. Pain. (2019) 160:824–32. doi: 10.1097/j.pain.0000000000001458
125. Liu SS, Pickens S, Burma NE, Ibarra-Lecue I, Yang H, Xue L, et al. Kappa opioid receptors drive a tonic aversive component of chronic pain. J Neurosci. (2019) 39:4162–78. doi: 10.1523/JNEUROSCI.0274-19.2019
126. Raver C, Uddin O, Ji Y, Li Y, Cramer N, Jenne C, et al. An amygdalo-parabrachial pathway regulates pain perception and chronic pain. J Neurosci. (2020) 40:3424–42. doi: 10.1523/JNEUROSCI.0075-20.2020
127. Petraschka M, Li S, Gilbert TL, Westenbroek RE, Bruchas MR, Schreiber S, et al. The absence of endogenous beta-endorphin selectively blocks phosphorylation and desensitization of mu opioid receptors following partial sciatic nerve ligation. Neuroscience. (2007) 146:1795–807. doi: 10.1016/j.neuroscience.2007.03.029
128. Narita M, Imai S, Nakamura A, Ozeki A, Asato M, Rahmadi M, et al. Possible involvement of prolonging spinal micro-opioid receptor desensitization in the development of antihyperalgesic tolerance to micro-opioids under a neuropathic pain-like state. Addict Biol. (2013) 18:614–22. doi: 10.1111/j.1369-1600.2011.00354.x
129. Niikura K, Narita M, Narita M, Nakamura A, Okutsu D, Ozeki A, et al. Direct evidence for the involvement of endogenous beta-endorphin in the suppression of the morphine-induced rewarding effect under a neuropathic pain-like state. Neurosci Lett. (2008) 435:257–62. doi: 10.1016/j.neulet.2008.02.059
130. Gupta A, Gullapalli S, Pan H, Ramos-Ortolaza DL, Hayward MD, Low MJ, et al. Regulation of opioid receptors by their endogenous opioid peptides. Cell Mol Neurobiol. (2021) doi: 10.1007/s10571-020-01015-w
131. Ozaki S, Narita M, Narita M, Iino M, Miyoshi K, and Suzuki T. Suppression of the morphine-induced rewarding effect and G-protein activation in the lower midbrain following nerve injury in the mouse: involvement of G-protein-coupled receptor kinase 2. Neuroscience. (2003) 116:89–97. doi: 10.1016/S0306-4522(02)00699-1
132. Hoot MR, Sim-Selley LJ, Selley DE, Scoggins KL, and Dewey WL. Chronic neuropathic pain in mice reduces mu-opioid receptor-mediated G-protein activity in the thalamus. Brain Res. (2011) 1406:1–7. doi: 10.1016/j.brainres.2011.06.023
133. Llorca-Torralba M, Pilar-Cuellar F, da Silva Borges G, Mico JA, and Berrocoso E. Opioid receptors mRNAs expression and opioids agonist-dependent G-protein activation in the rat brain following neuropathy. Prog Neuropsychopharmacol Biol Psychiatry. (2020) 99:109857. doi: 10.1016/j.pnpbp.2019.109857
134. Thompson SJ, Pitcher MH, Stone LS, Tarum F, Niu G, Chen X, et al. Chronic neuropathic pain reduces opioid receptor availability with associated anhedonia in rat. Pain. (2018) 159:1856–66. doi: 10.1097/j.pain.0000000000001282
135. Wawrzczak-Bargiela A, Ziolkowska B, Piotrowska A, Starnowska-Sokol J, Rojewska E, Mika J, et al. Neuropathic pain dysregulates gene expression of the forebrain opioid and dopamine systems. Neurotox Res. (2020) 37:800–14. doi: 10.1007/s12640-020-00166-4
136. Jones AK, Watabe H, Cunningham VJ, and Jones T. Cerebral decreases in opioid receptor binding in patients with central neuropathic pain measured by [11C]diprenorphine binding and PET. Eur J Pain. (2004) 8:479–85. doi: 10.1016/j.ejpain.2003.11.017
137. Nadal X, Banos JE, Kieffer BL, and Maldonado R. Neuropathic pain is enhanced in delta-opioid receptor knockout mice. Eur J Neurosci. (2006) 23:830–4. doi: 10.1111/j.1460-9568.2006.04569.x
138. Niikura K, Narita M, Butelman ER, Kreek MJ, and Suzuki T. Neuropathic and chronic pain stimuli downregulate central mu-opioid and dopaminergic transmission. Trends Pharmacol Sci. (2010) 31:299–305. doi: 10.1016/j.tips.2010.04.003
139. Ossipov MH, Lai J, Malan TP Jr, and Porreca F. Spinal and supraspinal mechanisms of neuropathic pain. Ann N Y Acad Sci. (2000) 909:12–24. doi: 10.1111/j.1749-6632.2000.tb06673.x
140. Finnerup NB, Attal N, Haroutounian S, McNicol E, Baron R, Dworkin RH, et al. Pharmacotherapy for neuropathic pain in adults: a systematic review and meta-analysis. Lancet Neurol. (2015) 14:162–73. doi: 10.1016/S1474-4422(14)70251-0
141. Smith EM, Pang H, Cirrincione C, Fleishman S, Paskett ED, Ahles T, et al. Effect of duloxetine on pain, function, and quality of life among patients with chemotherapy-induced painful peripheral neuropathy: a randomized clinical trial. JAMA. (2013) 309:1359–67. doi: 10.1001/jama.2013.2813
142. Colloca L, Ludman T, Bouhassira D, Baron R, Dickenson AH, Yarnitsky D, et al. Neuropathic pain. Nat Rev Dis Primers. (2017) 3:17002. doi: 10.1038/nrdp.2017.2
143. Kalso E, Edwards JE, Moore AR, and McQuay HJ. Opioids in chronic non-cancer pain: systematic review of efficacy and safety. Pain. (2004) 112:372–80. doi: 10.1016/j.pain.2004.09.019
144. Martinez-Navarro M, Maldonado R, and Banos JE. Why mu-opioid agonists have less analgesic efficacy in neuropathic pain? Eur J Pain. (2019) 23:435–54. doi: 10.1002/ejp.1328
145. Rivat C, and Ballantyne J. The dark side of opioids in pain management: basic science explains clinical observation. Pain Rep. (2016) 1:e570. doi: 10.1097/PR9.0000000000000570
146. Costa AR, Sousa M, Wilson SP, Reguenga C, Teixeira-Pinto A, Tavares I, et al. Shift of micro-opioid receptor signaling in the dorsal reticular nucleus is implicated in morphine-induced Hyperalgesia in male rats. Anesthesiology. (2020) 133:628–44. doi: 10.1097/ALN.0000000000003412
Keywords: descending pain modulation, opioids, serotonin, noradrenaline, neuropathic pain, dorsal reticular nucleus
Citation: Tavares I, Costa-Pereira JT and Martins I (2021) Monoaminergic and Opioidergic Modulation of Brainstem Circuits: New Insights Into the Clinical Challenges of Pain Treatment? Front. Pain Res. 2:696515. doi: 10.3389/fpain.2021.696515
Received: 16 April 2021; Accepted: 08 June 2021;
Published: 05 July 2021.
Edited by:
Bridget Lumb, University of Bristol, United KingdomReviewed by:
Norberto Cysne Coimbra, University of São Paulo, BrazilArmando Almeida, University of Minho, Portugal
Copyright © 2021 Tavares, Costa-Pereira and Martins. This is an open-access article distributed under the terms of the Creative Commons Attribution License (CC BY). The use, distribution or reproduction in other forums is permitted, provided the original author(s) and the copyright owner(s) are credited and that the original publication in this journal is cited, in accordance with accepted academic practice. No use, distribution or reproduction is permitted which does not comply with these terms.
*Correspondence: Isaura Tavares, aXNhdGF2QG1lZC51cC5wdA==