- 1Research and Development Institute, Transilvania University of Braşov, Braşov, Romania
- 2Ophthalmology Clinic, Cluj County Emergency Hospital, Cluj-Napoca, Romania
- 3National Center for Brain Research, Institutul de Cercetări pentru Inteligență Artificială, Romanian Academy, Bucharest, Romania
Sending an axon out of the eye and into the target brain nuclei is the defining feature of retinal ganglion cells (RGCs). The literature on RGC axon pathfinding is vast, but it focuses mostly on decision making events such as midline crossing at the optic chiasm or retinotopic mapping at the target nuclei. In comparison, the exit of RGC axons out of the eye is much less explored. The first checkpoint on the RGC axons’ path is the optic cup - optic stalk junction (OC-OS). OC-OS development and the exit of the RGC pioneer axons out of the eye are coordinated spatially and temporally. By the time the optic nerve head domain is specified, the optic fissure margins are in contact and the fusion process is ongoing, the first RGCs are born in its proximity and send pioneer axons in the optic stalk. RGC differentiation continues in centrifugal waves. Later born RGC axons fasciculate with the more mature axons. Growth cones at the end of the axons respond to guidance cues to adopt a centripetal direction, maintain nerve fiber layer restriction and to leave the optic cup. Although there is extensive information on OC-OS development, we still have important unanswered questions regarding its contribution to the exit of the RGC axons out of the eye. We are still to distinguish the morphogens of the OC-OS from the axon guidance molecules which are expressed in the same place at the same time. The early RGC transcription programs responsible for axon emergence and pathfinding are also unknown. This review summarizes the molecular mechanisms for early RGC axon guidance by contextualizing mouse knock-out studies on OC-OS development with the recent transcriptomic studies on developing RGCs in an attempt to contribute to the understanding of human optic nerve developmental anomalies. The published data summarized here suggests that the developing optic nerve head provides a physical channel (the closing optic fissure) as well as molecular guidance cues for the pioneer RGC axons to exit the eye.
1 Introduction
The mammalian retina comprises five classes of neuronal cells (1): the photoreceptors transduce light into an electrical signal and transmit it in the outer plexiform layer to bipolar cells. At this level lateral interactions are provided by horizontal cells. In the inner plexiform layer (IPL), the bipolar cells connect to the retinal ganglion cells (RGCs), which send the visual information in the form of nerve spikes to the retinorecipient nuclei in the brain. At IPL level, amacrine cells assist in retinal computation by a variety of inhibitory and excitatory lateral connections with bipolar cells and RGCs. Until now, more than 40 RGC types have been identified, which receive various combinations of signals from the approximately 70 types of interneurons so that they extract distinct visualqualities (2–10). RGCs of the same type form anatomical and functional mosaics within the retina, namely the dendritic arbors of a given RGC type tile the retina uniformly and their receptive fields sample the visual scene and extract specific visual features (11–13). As a result, every point in the visual field is reported to the brain through multiple parallel channels (14) dedicated to different visual modalities such as contrast, color, or motion (1, 2, 15–18) and the brain receives a number of parallel images of the world (3). The anatomical basis of this connection is the optic nerve, a fascicle of RGC axons linking the retina to the brain. In the last decades, the field of developmental neuroscience has predominantly focused on the study of cell type specification, especially encouraged by the advent of single-cell RNA sequencing tools (19–21). Sending an axon towards the optic disk and through the optic nerve is a defining feature of all RGCs, regardless of the cell type. It is one of the earliest developmental events, occurring right after RGCs differentiate (22–25), at a time when RGC types are not yet specified (26). Although numerous papers have reviewed RGC axon guidance mechanisms (27–36) – most of them are focused on population - level axon steering events such as chiasm crossing and retinotopic mapping at the targets while the determinants of RGC axon emergence and optic nerve formation are far less explored.
The need to more comprehensively approach this subject is enforced by the increase in frequency of optic nerve development anomalies in humans. A significant cause of congenital blindness, human optic nerve developmental anomalies are a heterogeneous group of diseases ranging from optic pits, segmental or global optic nerve hypoplasia to optic nerve aplasia, optic disc conformation anomalies and syndromes associating microphthalmia, colobomas, aniridia or brain anomalies (37–43). Single-case reports or small case series have identified a variety of genetic mutations linked to these anomalies and recent whole-genome-sequencing studies extend these lists considerably (44, 45). These findings can only be valued if mechanistic roles of these genes in the development of the optic nerve is demonstrated in animal models.
Similar to other white matter tracts in the brain, the optic nerve develops based on a few pioneer axons which use their growth cones to follow various guidance cues on their way to the targets (30). They are joined by the axons of the later-born RGCs by fasciculation (46). The first intermediate target for RGC axons is the optic nerve head (ONH), a region located at the junction between the future retina (the optic cup) and optic nerve (the optic stalk), resulting from complex morphogenetic movements of the optic vesicle – extensively reviewed (39, 40, 47–54). The necessity for the ONH in RGC axon development is demonstrated by the cases of retinal organoid cultures. In the absence of an optic stalk, retinal organoids are still differentiating and developing many anatomical and functional aspect of in vivo retinas, but are unable to grow RGC axons, as RGC survival is compromised (55, 56). This limitation was recently partly overcome by assembling retinal and thalamic organoids (57) or by culturing optic vesicle bearing brain organoids, that maintain the continuity between the optic vesicle and the brain (58). Identifying the ONH signals dedicated to RGC axon pathfinding is complicated by the coincident timing of morphogenesis of the optic cup and optic stalk, optic fissure closure and the escape of the first RGC axons out of the optic cup (59–61). An added challenge is to discriminate between primary RGC axon guidance defects and axon misrouting secondary to optic cup/stalk developmental anomalies such as coloboma or patterning defects (62, 63). The aim of this review is to survey the experimental results of the past decades on optic cup/optic stalk morphogenesis and early RGC axon guidance in conjunction with the recent RNA sequencing studies on developing retinas/RGCs in order to characterize the interplay between extracellular signaling molecules and intrinsic transcriptional pathways involved in the initiation of RGC axons pathfinding, which could be targeted in future retina/optic nerve regeneration strategies. The information presented in this review mostly comes from experiments done on mouse models. In case findings are coming from other species, the experimental models are mentioned in the text. We propose that the key to early RGC axon guidance is the spatial and chronological correlation between optic fissure closure and RGC differentiation initiation allowing the closing optic fissure to serve as a permissive channel for the pioneer axons, which are followed by the next axons by fasciculation.
2 Retina morphogenesis
The first target of the RGC axons in their way to the retinorecipient nuclei in the brain is the optic disc. The position of the optic disc precursor region changes during the successive morphological rearrangements that take place during the morphogenesis of the eye (Figure 1A).
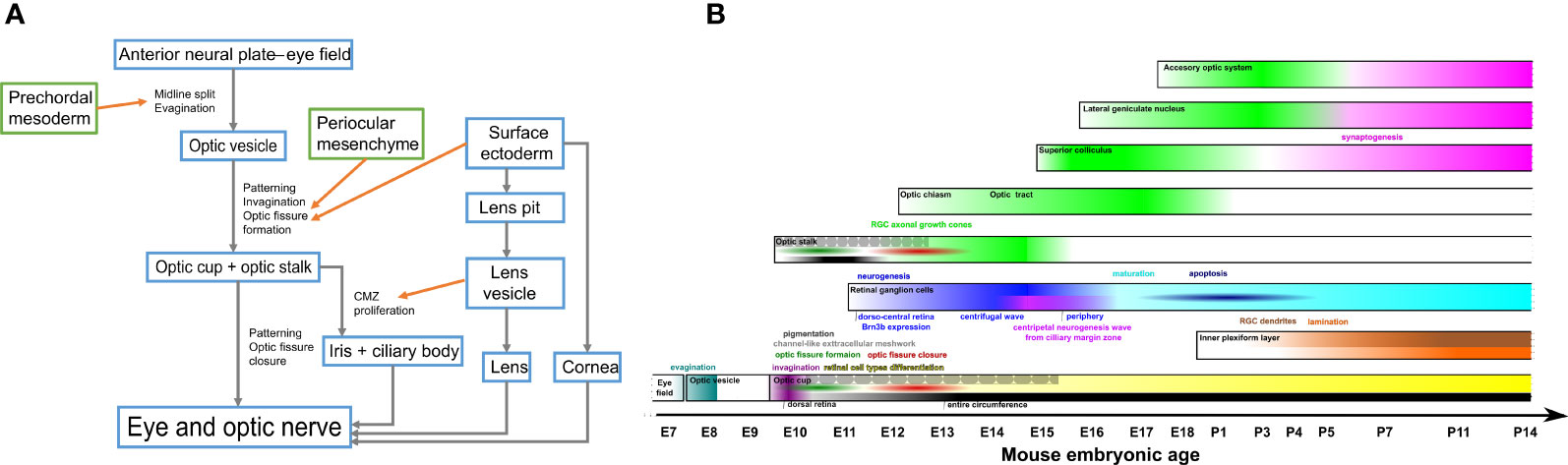
Figure 1 (A) Schematic illustration of eye morphogenesis. (B) Schematic developmental timeline of main events in mouse retinal ganglion cells development. grey arrow, developmental transformation; orange arrow, influence; RGC, retinal ganglion cells.
2.1 Eye field differentiation
The origin of the nervous system is the ectoderm, where a neuroectoderm is specified by BMP4 inhibition via follistatin, chordin and noggin (64). The anterior neuroectoderm is further induced by Wnt downregulation (64). As demonstrated in xenopus, within this region an eye field will be induced by signals coming from the adjacent mesenchymal tissues (65). Signals from the prechordal mesoderm including cyclops (Cyc), sonic hedgehog (Shh) and SIX3 split the eyefield in two (47, 49, 66). It further develops into two laterally placed optic vesicles under the influence of Eph/Ephrin signaling at an intersection between the Wnt and FGF pathways (40, 67).
2.2 Optic vesicle evagination
Lateral evagination of the forebrain precursor region leads for the formation of the optic pit that evolves to an optic vesicle (47, 68). The lateral expansion of the optic vesicles brings them in the vicinity of the lens-competent surface ectoderm. Close contact between the two structures is ensured by the displacement of the interposed mesenchyme and by a meshwork of collagen and cellular processes (47). The cavity of the optic vesicle is in direct communication with the ventricular cavity of the brain (69).
Optic vesicle formation occurs under the control of Rax, Pax6 and Tll (48). Activated by Sox2 and Otx from the anterior neuroectoderm, Rax represses NLCAM and induces CXCR4 acting on cell shape and movement with the important contribution of laminin (48, 70, 71).
2.3 Optic vesicle patterning
A recent single-cell RNA sequencing study (72) highlights the cellular heterogeneity of the optic vesicle comprising seven distinct neuroepithelial cell populations, four of which are stage-dependent presumptive retinal precursors. The optic vesicle becomes regionalized under the influence of eye field specific transcription factors including Lhx2, Pax6 and Six3, upregulated by Rax (48, 73). Anatomical orientation of optic vesicle patterning also relies on optic neuroepithelium cilia required for Hedgehog signaling, expressed in a proximal-high to distal-low gradient, as well as for PCP, Wnt, TGF-β, PDGFα, RTK, mTOR and Notch signal transduction (74).
The proximal domain of the optic vesicle will form the optic nerve whereas its distal domain will become the neural retina (NR) and retinal pigment epithelium (RPE) (75). These domains are initially delineated on a dorso-ventral axis so that the dorso-distal optic vesicle will form the future NR and RPE, and the proximo-ventral optic vesicle will become the ventral optic stalk (vOS) (47). The presumptive RPE and the ventral optic stalk each are continuous with the presumptive forebrain, but are separated from one another by the ventricular space (69). At this stage, RPE-NR and NR-vOS boundaries are fluid. Proximo-ventral fate is specified by hedgehog (Hh) through activation of Pax2, Vax1 and Vax2. In zebrafish, Hh also represses Pax6, the dorso-distal specifier, by expression of Mid1, a regulator of Pax6 ubiquitination (76). The NRE-vOS boundary is gradually sharpened also by Pax2-Pax6 mutual repression and Hes1 activity in the vOS (47, 77). Future ONH cells are tripotential and need Pax2 to shut off NRE and RPE fates, to adopt glial fate and to activate Hes1 (63). NR/RPE fates are specified by Vsx2 (or Chx10)/Mitf expression respectively, regulated by Lhx2 and lens-derived FGF signaling (48, 51). FGF soaked beads have the ability to convert RPE to neural retina in chicken (78, 79). In mice, the surface ectoderm provides FGF1 and 2 which activate VSX2, that in turn represses Mitf (80, 81). Among the multiple FGF ligands, FGF8 coming from the telencephalic vesicles has the main role in optic vesicle patterning, while the others are able to compensate in its absence, as shown in zebrafish (82). RPE differentiation requires the Wnt/beta-catenin pathway, including Porcn function (83).
2.4 Optic cup and optic stalk invagination – optic fissure
The optic vesicle undergoes a process of invagination between becoming a bi-layered optic cup (inner NR and outer RPE) and optic stalk in coordination with the invagination of the lens placode to a lens vesicle (69). The surface ectoderm covering the lens will become the cornea thus defining the mature appearance of the eye (47). The edge where the inner and outer layers meet plays a role in the invagination process and evolves into the ciliary body and the iris (40, 47, 84). Interactions between the optic vesicle and the surface ectoderm are essential for the invagination process (85) and are based on Pax6 regulated fibronectin 1 expression along with the retinoic acid (RA) signaling pathway (86, 87). RA local concentration is controlled by synthesizing (RALDH1/3) and catabolizing (CYP26A1/C1) enzymes and in chick retina it is localized complementary to FGF8 expression (88). Lhx2 is a regulator of both optic cup and lens formation (89). Optic cup derived RA also control the expression of periocular mesenchyme markers such as Pitx2 and FoxC1 (51).
Optic vesicle invagination is asymmetric (35, 90), more accelerated on the ventral side leading to the formation of a grove, the optic fissure (91) and to a reflection of the proximo-ventral/dorso-distal axis as the RPE enwraps the NR (47). The region where the proximal and distal portions of the optic fissure join will develop in to the optic nerve head (92). Formation of the optic fissure allows the mesenchymal cells to invade the optic cup and to form the hyaloid vasculature (93). While the vOS invaginates and forms the tissue through which the RGC axons will travel, the dorsal optic stalk will transform in non-neural tissue sheathing the optic nerve (47). The optic fissure domain is characterized by Netrin 1, Pax2, Vax1, Vax2 and Raldh3 expression (50, 93). Lower levels of Raldh3, Vax2 and Tbx5 and expansion of the Pax2 domain associated with increased apoptosis in the ventral retina was seen in Fz5 (a Wnt receptor) conditional knock-out mice (94). Optic fissure formation is induced by lens-independent signals including Pax2, Vax1, Vax2, Bmp7 (from the periocular mesenchyme), Shh and FGF (50, 91). Optic fissure formation is disturbed by experimental manipulations of these morphogens. Bmp7 knock-out mice have no optic fissure, Pax2 knock-out mice bear proximal optic fissure defects and RA induces optic fissure invagination in zebrafish (50, 91, 95).
As the optic cup grows, the optic fissure margins get closer to each other, displace the intertwining periocular mesenchyme and come in contact (51, 91). Optic fissure closure begins at midway and progresses both distally and proximally based on two distinct processes: fusion (basement membrane elimination) and intercalation (filling of the optic fissure space with newly differentiated astrocytes and incoming axons), which is more characteristic for the proximal part of the optic fissure (47, 51, 91). The hyaloid artery remains separated from the axons in the OS by a laminin cap contact (91). Optic fissure closure requires sharp delineation between the NR/RPE domains based on mutual restricting Mitf and Pax2 expression, regulated by Zfp503 (96) and FGF signaling via FGF receptors associated with Frs2α-Shp2 complex, ERK/Ras signaling (62, 97, 98) and Wnt-Fz5 signaling (94). The actual fusion process is promoted by TGFbeta (99). Netrin1 is directly involved in the fusion process in chicken (100).
As any morphogenetic movement based on proliferation and sculpting, optic cup invagination and optic fissure closure are accompanied by significant cell death. In mice, there is a sequential wave of cell death starting from the ventral optic cup, continuing along the fusing edges of the optic fissure and proceeding into the optic stalk followed by an invasion of macrophages from the surrounding mesenchyme that phagocytize the cell debris and are in close contact with the emerging RGC axons (101).
2.5 Optic cup and optic stalk patterning
Patterning in the optic cup and stalk follows the general domains established at the optic vesicle stage (102) and further compartmentalizes the structure along three axes (dorso-ventral, naso-temporal and proximo-distal) under the control of Hh signaling, as demonstrated in xenopus (103). The dorso-ventral patterning is achieved by dorsal Tbx5, Xbr1, COUPTFI/II and ventral Pax2, Vax2 expression (47, 69) as a result of Hh versus Bmp signaling, according to studies done in chick and frog embryos (65, 104). In zebrafish, nasal Foxg1 and temporal Foxd1 restriction is regulated by interaction between FGF and Hh signaling (105). EphA receptors and EfnA proteins are expressed in complementary nasal to temporal gradients, while EphBs/EfnBs have opposing dorso-ventral gradient expression in the NR (27, 106). Dorso-ventral patterning of the RPE is influenced by Zfp503 (96). Patterning along the third axis, the proximo-distal one, entails centro-peripheric regionalization in the optic cup, ONH delineation and OS-OC boundary delineation. The ONH domain expresses markers of the optic stalk (Pax2 and Vax1) and ventral neural retina (Netrin1, Vax2 and Raldh3) under the control of Bmp7 and Shh (93). The periphery of the OC is represented by the ciliary margin zone expressing Msx1 and Otx1 (107). The ciliary margin zone has a distal Bmp4 domain and a proximal CyclinD1/Msx domain containing multipotential retinal precursor cells (108). Optic cup periphery specification requires Wnt and Shh signaling, transduced via Cdon, Boc, Gas1 and Lrp2 (40). The outer and inner layers of the ciliary margins generate the outer and inner layers of the iris and cilliary body respectively, under Pax6 signaling (109). Sub-patterning of this region is based on FGF gradients interacting with Wnt signaling (98, 110). The NR/RPE boundary from the ciliary margin zone continues on the optic fissure margins (51).
The optic stalk also has two layers. In analogy to the RPE completely surrounding the neural retina as a result of invagination and optic fissure closure, the non-neuronal tissue derived from the dorsal OS is completely encasing the vOS derived Vax1 positive epithelium (111). The ventricular cavity of the brain is still continuous with the future subretinal space (112) as a narrow space separating the two layers in the optic stalk. As a directly visible mark of the ongoing patterning process at the optic cup-optic stalk boundary, melanin observable in the RPE as well as in the wall of the distal optic stalk, which are continuous, and is gradually eliminated from the optic stalk and restricted to the RPE (113). The transient optic stalk melanization is concomitant with the exit of the first RGC axons in the optic stalk, but pigmented or previously pigmented Pax2 negative optic stalk regions are avoided by nerve fibers (93, 113).The inner optic stalk Pax2 positive astrocyte precursor cells extend in the retina as a cuff that enwraps the exiting RGC axons, separating them from the subretinal space (68). There is a mutual influence between the RGC axons and these cells: on one hand, the Pax2 ONH cells provide axon guidance cues including Netrin1, NCAM or, laminin but on the other hand once ONH fate is induced by Bmp7 from the periocular mesenchyme, Shh secreted by the RGC axons is needed to maintain the ONH Pax2/Netrin1 cell population, which express Gli1 and Ptch Shh receptors (68, 93). In the absence of RGC secreted Shh, melanin, Pax6 and Mitf appear in the optic stalk (114). Transdifferentiation of optic stalk tissue to RPE was also seen in FGFr1/2 or heparin sulfate deficient mice (62, 115).
The developmental sequence of optic vesicle – optic cup and stalk morphogenesis and patterning ensures the anatomical continuity between the neural retina, the residence of RGC cell bodies, and the optic nerve precursor so that the RGC axons travel a natural course to the future optic chiasm region. As Table 1 illustrates, any disruption in this sequence can disturb the early developmental steps of the RGCs.
3 Retinal ganglion cells development
3.1 RGC differentiation
Retinal precursor cells (RPCs) are able to generate all retinal neural cell classes and Müller glia, while astrocytes, macrophages and microglia later migrate into the retina (120). They commit to a specific fate as they transition from proliferative to terminal division states (121–123). The retinal cell types are produced in a stereotypic sequence, with RGCs, cones, horizontal and amacrine cells in a first wave and a second wave for bipolar, glial and a part of the amacrine cells, while rods differentiate throughout the retinal development time frame (120). RGCs differentiate in a central-to peripheral wave starting from the dorso-central retina, adjacent to the ONH (124, 125)
Uncommitted and lineage-restricted RPCs are located in the neuroblast layer, at the apical side of the NR, similar to the ventricular zone in the developing brain (126). Apolar RGC precursors become postmitotic in the neuroblast layer and become bipolar as their cell body translocates to the basal surface of the retina, where the ganglion cell layer will be located (122, 127). As they differentiate to RGCs, the apical process detaches and they become multipolar, growing an axon and dendrites (24). RGC precursors failing to differentiate undergo apoptosis in the ganglion cell layer (122). A subset of non-apoptotic new-born RGCs are eliminated 24h after birth by microglia based on complement signaling through phagoptosis (128).
A second source of retinal cells is the Msx1 precursor cell located at the ciliary margin zone (129). RGCs from the ciliary margin zone differentiate later than the central ones (31). Instead of translocating from the ventricular layer, they migrate laterally from the CyclinD1 zone directly in the ganglion cell layer (31, 108)
Still multipotential, RGC precursors are already committed to a specific type. The cell-type specification is continued in late embryonic and postnatal life through intrinsic transcriptional programs to reach the 40 types of mouse RGCs (26). For example, Ret-Brn3a interactions in postmitotic neurons can switch cell type/morphology (130).
3.2 RGC axon pathfinding
The vitreal process of the bipolar RGC precursors transforms into an axonal growth cone (24). RGC axons emerge very early during differentiation, even before the cell body has translocated to the ganglion cell layer (114) and start to express Gap43 and Tuj1 (131). RGC axons are already seen in the optic stalk coming from bipolar precursor cells with cell bodies at different hights in the retinal epithelium (132).
The most distal expansion of the axon is known as the growth cone (132), a sensory-motor structure capable of extending retracting processes called filopodia (thin) and lamellipodia (flat) in response to external signals (29, 133). Lamellipodia have a branched network of F-actin maintained by branching proteins such as Arp2/3 whereas in filopodia F-actin bundling proteins like alpha-actinin and fascin keep F-actin in parallel bundles (134, 135).
Growth cone steering (chemotropic turning) or growth cone collapse under the influence of axon guidance cues implies rapid changes in local protein levels achieved by local translation and protein ubiquitination (136, 137). Axon pathfinding is based on growth cone cytoskeletal reorganization, a sequence of F-actin addition on the plus-end of microtubules, retrograde F-actin flow and microtubule–F-actin coupling influenced by the strength of the adhesion on the substratum, as shown in aplysia ex vivo studies (138). Growth cones have a spread form and move fast on adhesive substrates and adopt contracted forms and stall on less adhesive substrates (132). Axon growth is an intermittent process, characterized by advances and pauses (139).
Once generated, RGC axons grow centripetally (Figure 2), within the optic nerve fiber layer and exit the eye through the ONH, enter the optic stalk within the neuro-epithelial lining of the optic fissure and travel along the optic stalk to the midline (24, 29, 140). Dye implant studies in rats and ferrets and mouse electron microscopy studies have shown that axon fibers do not preferentially occupy certain depths within optic nerve fiber layer or the optic stalk, newly added fibers being intermingled arbitrarly with the already present ones (112, 141, 142). In human fetuses, maturing and newly born axons are intermingled and the only ordering is at the entrance in the optic disc, where retina quadrant provenience is respected (143). This order of the axons at the ONH is lost within the optic nerve, so that axon guidance cues at the following checkpoints on their path to the targets are needed in order to ensure final retinotopic mapping (144). The next intermediate target is the optic chiasm, where the ipsi/contra-lateral projection decision is made. The axons continue their path in the optic tract and defasciculate at their final targets where they assume retinotopic positions according to their cell type and retinal eccentricities. These processes have been extensively studied and reviewed and are beyond the scope of this paper (29–31, 145).
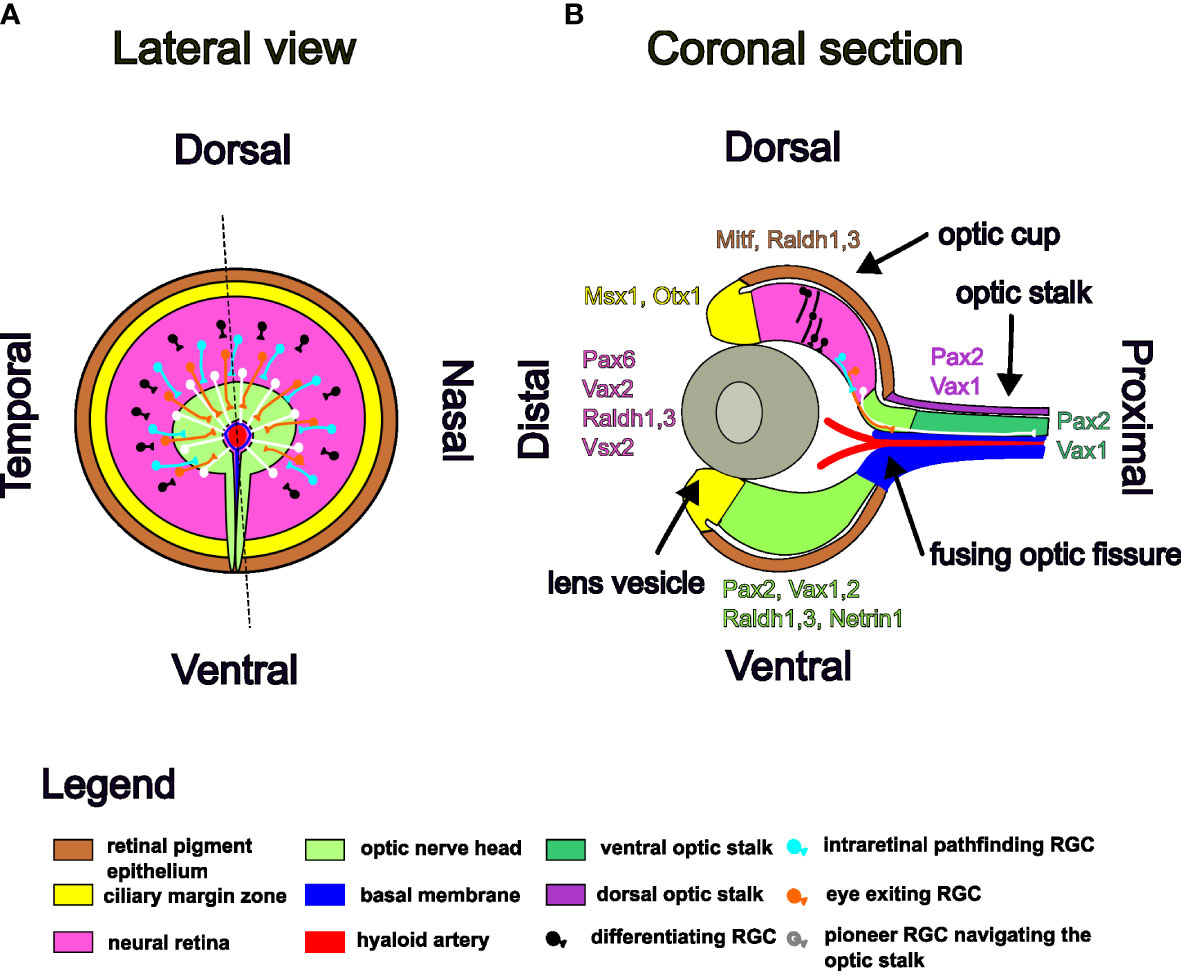
Figure 2 Schematic illustration of RGC axons pathfinding in the E12.5 mouse optic cup and stalk. Markers for each domain of the optic cup and stalk are listed in color code. (A) Lateral view. (B) Coronal section.
3.3 Developmental timeline of mouse RGCs
The main events in the developmental timeline of mouse RGCs are summarized in Table 2 and Figure 1B.
4 Transcriptional profiles of early mouse RGCs
The behavior of RGC axons in Atoh7−/−and Atoh7−/−;Bax−/− mice, growing in the nerve fiber layer but failing to exit an apparently normal ONH, suggests that intrinsic RGC transcription programs are required for eye exit in addition to ONH guidance (173). Brn3b and Isl1 ectopic expression from the Atoh7 locus in Atoh7 knock-out mouse retinas rescues the axon guidance phenotype (174) showing that Atoh7 is indirectly involved in axon guidance by inducing Brn3b and Isl1. There is very little knowledge on transcription factors and downstream genes involved in RGC axon guidance, and identified phenotypes involve events that occur later than eye exit. Delayed axon growth and abnormal axon de-fasciculation from the optic tracts was seen in Brn3b knock-out mice (23, 169) and ipsi-/contra-lateral projection phenotypes were observed in Zic2, Isl2 and Sox4,11,12 mutants (117, 175–181).
RGCs are the first differentiated cells in the neural retina and axon emergence and pathfinding is the major developmental process they are involved in. In this context, RNA sequencing studies of newly born RGCs have the potential to identify the transcriptional pathways involved in pioneer axon pathfinding. However, due to the technical challenges such as the small size of the retina and the small cell number there are only a few published papers more or less directly focused on newly born RGCs (21, 26, 168, 173, 182–186). According to Shekhar et al. (26), there is a good overlap between their developing retina single cell RNA sequencing data and the other two studies using the same methodology, namely Clark et al., (184) and Giudice et al., (182).
Table 3 presents a selection of genes resulted from three RNA sequencing studies using different approaches: the first study (168) used immunomagnetic sorting of dissociated E15 retinas to sequence RGC RNA against retina supernatant, the second study (182) performed single-cell RNA sequencing on E15 retinas and identified newly born RGCs by unbiased clustering, and a third study (183) performed bulk RNA sequencing on E11 to P28 retinas. The genes were also looked up in microarray studies in embryonic retinas of Atoh7 knock-out mice (185, 186), RNA sequencing in isolated embryonic RGC growth cones (137) and public in situ hybridization databases (Allen Brain Institute and Eurexpress). The selection resulted from the logical intersections between lists of genes identified in relevant categories of samples in the three studies (for complete lists and intersection strategies see Supplementary Table 1). Cellular localization of the genes according to https://www.ncbi.nlm.nih.gov/gene/ is presented in Supplementary Table 2. Our selection included some proteins belonging to the Netrin1-Dcc signal transduction pathway, namely App (Stmn2, Kif1b), Cdc42, Trim67, Tubb3 (187–191). Manipulations of some of the identified genes/proteins produce RGC axon guidance errors: Cntn2 deficiency is linked with axon fasciculation and contralateral projection defects (192); Dcc knock-out results in failure of RGC axons to exit the eye (151); Gap43 null RGC axons have chiasm crossing defects (193); Igf1 and Igfbpl1 contribute to RGC axon growth by intracellular Calcium level modulation and mTOR pathway activation (194); antibodies against Nfasc induce de-fasciculation in chick RGC cultures (195); Nrcam is required for chiasm crossing (196); Nrp1 conditional knock-out causes chiasm crossing and optic tract fasciculation defects (197) and Tenm3 deficient RGC axons fail to project ipsi-laterally (198, 199). Others genes in the list - App, Cdc42, Celsr3, Chl1, Elavl4, Evl, Islr2, Kif1b, Kit, Kitl, Mmp24, Stmn2, Tubb3 - are associated with axon growth or guidance defects in other regions of the nervous system or in cultured neurons (191, 200–213).
5 Signaling – transcription interactions in early axon pathfinding in mouse RGCs
5.1 General principles
RGC axon pathfinding implies pioneer axon guidance and later born axons fasciculation (214). Pioneer axons navigate in the retina based on chemotaxis (attractive and repulsive cues forming gradients) and haptotaxis (physical interactions with permissive substrates) (30, 91, 215, 216). The next paragraphs survey the evidence on the regulation of RGC differentiation timing, haptotaxis and chemotaxis conditions for the pioneer RGC axon guidance and on RGC transcriptional programs involved in pioneer axon pathfinding and cofasciculation (Figure 3). The molecular determinats of retina development known from mouse studies and their corresponding human phenotypes are listed in Supplementary Table 3.
5.2 Regulation of RGC differentiation
Retinal cell type differentiation sequence is a result of RPC intrinsic programs and extrinsic cues (48). RPC proliferation and multipotential state is regulated by Vsx2, Pax6, Six3, Six6, and Sox2 (126). Notch and Shh signaling keep RPCs in the proliferative state (217). Notch-Delta signaling maintains the progenitor pool by lateral inhibition (49, 218) and contributes to the transition from naïve to competent RPCs (145). When Delta-Serrate-LAG2 ligands from adjacent cells bind to the Notch extracellular domain, the intracellular domain together with RBPJ and MAML1 translocates in the nucleus and activates Hes1 and Hes5 transcription. When ligands and receptors are expressed by the same cell, the Notch pathway is inhibited. Notch expression is activated by Sox2 and suppressed by cell type specific factors like Atoh7, Ascl1, Ptf1a, and Foxn4 (175, 219). Sfrp1/2 also deactivate Notch signaling via Adam10 (220). miRNAs maintain the RPC competence window for RGC differentiation (221, 222).
Experiments in zebrafish and chicken have shown that Shh signaling from the midline and FGF signaling from the OS trigger RGC differentiation (223, 224). OS derived FGF3 and FGF8 initiate neurogenesis in the central retina (78, 224). FGF8 is negatively regulated by retinoic acid (88). Retinoic acid catabolizing enzymes Cyp26a1 and Cyp26c1 are expressed in an equatorial streak (225) characterized by higher RGC density (88). Conditional FGFR1/2 double knock-out in mouse RPCs impairs RGC differentiation onset (97). The FGF–Frs2–Shp2 pathway controls RPC proliferation (62, 97, 115). Ikaros is involved in the production of early RGCs (121, 226).
Once started by FGF signaling, the RGC genesis wave progresses to the periphery based on Shh signaling, as observed in zebrafish (227). Brn3b regulated (168) secretion of Shh from RGCs modulates proliferation and differentiation of RPCs (68) and is required for the maintenance of the RPC pool (120). Neurogenin2 and Ascl1 are also responsible for the propagation of the RGC genesis wave (175). Neurogenin2 is expressed ahead of the RGC wave edge and regulates Atoh7 transcription in RPCs (228). Secondary RGC genesis from the ciliary margin zone is regulated by CyclinD2, a cell cycle facilitator (31, 108).
Pax6 activates transcription factors that commit RPC to different fates so that in absence of Pax6 only amacrine cells are produced (126). Downstream of Pax6, two proneural transcription factors are Neurog2 and Atoh7, which is also under the control of Gdf11 and follistatin (49). Atoh7 expression in RPCs determines competence acquisition, not RGC fate commitment and its absence nearly eliminates RGCs (229). Neurog2 and Atoh7 activate RGC specification transcription factors including Sox4, Sox11, Neurod1, Brn3b and Isl1 (230). The Atoh7-Brn3b pathway suppresses non-RGC transcriptional programs and accounts for 70% of RGC differentiation (123). Brn3b further activates Brn3a, Brn3c, Eomesodermin, Ebfs, Onecut1, and Onecut2. Isl1 is required for RGC specification having overlapping targets with Brn3b (168, 231). Ectopic expression of Brn3b and Isl1 from the Atoh7 locus in Atoh7 knock-out mouse retinas rescues RGC differentiation (174). Other than Brn3b and Isl1, NeuroD1 or SoxC can also partly compensate for the absence of Atoh7 (175, 232). Dlx1/2 are expressed at transition stages of RGC fate commitment, and are negatively regulated by Brn3b and Isl1, and the Bmp and Vegf pathways also contribute to RGC differentiation (175, 233).
5.3 Regulation of RGC axonogenesis
The axons grow directly from the basal aspect of the RGCs concomitant with apical process detachment (127). Polarized organization of cytoskeletal structures governed by instrinsic mechanisms was identified in various neuronal populations prior to axon emergence (27, 234). RGC axon sprouting is controlled by integrins and cadherins (22). FGFs stimulate axon generation and growth in xenopus RGC cultures (235). Experiments in mouse cortex have shown that apically oriented axon genesis is linked with the movement of the centrosome apical to the nucleus and that this polarization is regulated by TGF-beta – LKB1 –BDNF signaling (127, 236). The orientation is reversed in the retinal neuroepithelium, which has a basal lamina made of laminin, collagen IV, nidogen, agrin, condroitin sulfate proteoglycan (CSPG) and heparan sulfate proteoglycan (HSPG) (114). Laminin contact directly promotes axon sprouting by stimulating the accumulation of Kifc560, an early axonal marker, and the formation of growth cones (127). Glial polarity precedes neuronal polarity and studies in chicken retina explants have shown that glial endfeet promote axon formation while glial somata support dendritic growth (215). As the axon grows, the proximal segment loses the filopodia and takes a cylindrical shape and the ventricular process completely disappears (24). In zebrafish, apical retraction requires Slit1b-Robo3 signaling (237). Dominant negative N-cadherin expression leads to premature detachment in zebrafish (237) and blocks RGC axonogenesis in xenopus (18). Brn3b and Brn3c activate genes involved in axon formation and in their absence RGC neurites adopt dendrite-like features (152).
5.4 Regulation of growth cone dynamics
Growth cone steering and axon growth imply cytoskeleton reorganization which is mainly triggered by cell adhesion molecules (CAMs) (238, 239). Microtubule dynamic is modulated by several signal transduction pathways mostly based on kinases (240). Immunoglobulin superfamily CAMs involved (L1CAM, NCAM1, ALCAM, and CNTN2) activate Erk MAP kinase to promote axon growth in fasciculation because they are only expressed on axons, and not on the other substrates (241). RGC axons grow preferentially on L1CAM compared to extracellular matrix proteins such as laminin (242). Anti-L1 Fab and anti-NCAM Fab treatment had different effects on RGC axon growth cones in culture: direction change and lower growth speed versus increased elongation speed and premature growth stop respectively (243). FGF receptor mediated activation of the phospholipase C gamma cascade is needed for RGC axon growth in response to L1CAM in mice (242, 244). FGF signaling is also transduced by the Ras/MAPK and PI3K pathways (40, 115).
Cadherins are adhesion molecules expressed in the retina that promote axon growth by homophilic interactions. N-cadherin may play a dual role: it promotes neurite extension by sequestrating beta-catenin, and preventing the inhibition of adenomatous polyposis coli (APC) protein, a positive regulator of neurite growth. On the other hand, by binding to the cytoplasmic p120 catenin N-cadherin prevents GTPases Cdc42 and Rac1 from actin remodeling and thus has a growth inhibitory effect that prevents excessive axon growth at specific locations (245). In rats, transmembranar cadherins Celsr2 and Celsr3 have opposite effects on neuron-neuron contact triggered neurite extension based on homophilic interactions and downstream CAMKII (calcium/calmodulin-dependent protein kinase II) or calcineurin induction (246).
Studies in xenopus have revealed that in response to external cues such as a Netrin1 gradient, asymmetric cap-dependent translation of beta-actin is activated via phosphorylation of the translation initiation factor 4EBP, resulting in the pronounced extension of the filopodia located in the part of the growth cone exposed to the highest Netrin1 concentration (247).
5.5 Optic disc directionality
At the time of axon emergence, RGCs extend multiple transient minor processes to probe the environment for guidance cues and the ones oriented towards the attractive and away from the repellant cues will develop into the single axon, directed to the optic disc (243).
5.5.1 Attractive cues
The ONH domain exerts attraction on the RGC axons as illustrated by the misrouting of RGC axons towards the margins of the unclosed optic fissure, expressing ONH markers, in Fz5 conditional knock-out mice (94). Netrin-1 on the processes of optic nerve head glial precursor cells is acting as a chemotactic attractant for the axons expressing its canonical Dcc receptor (238, 248). DCC is preferentially expressed by the newly born RGCs that are sending their axons to the optic disc (182). A central-high/periphery-low gradient of Shh is also an attractive guidance cue acting on Ptc-Smoothened, Hedgehog interacting protein (HiP) and Boc receptors expressed by the RGCs (92, 249). RGCs themselves are a source of Shh having a dual role in axon guidance and glial cell development (68). Blocking the FGF receptor or the signal transduction pathway in rat retina explant cultures causes new RGC axons to lose the optic disc directionality and to grow towards the periphery (242).
5.5.2 Repulsive cues
The expression of the repulsive cues is complementary to that of the attractive cues, namely a periphery-high/central-low gradient (29). They are either secreted by the lens like Slit2 (250), or they are produced in the basal lamina in a wave preceeding the peripheral side of the newly born RGCs as it is the case for chondroitin sulphate proteoglycan (CSPG) (243, 251). Repulsive cues are regulated by transcription factor Zic3, with a periphery-high to central-low gradient of expression (114).
5.6 Optic nerve fiber layer restriction
5.6.1 Physical substrate
RGC axons grow in a narrow space delineated by RGC cell bodies and the vitreal basal lamina (the inner limiting membrane) (35). This space is occupied by the endfeet of glial precursor cells, similar to the radial glia in the brain, which are organized in a channel-like structures forming a network that orients the emerging axons (216). In chicken retina cryocultures, axons preferentially follow glial precursors endfeet compared to preexisting axons or laminin (215).
5.6.2 Attractive cues
Contact with glial precursors endfeet and the basal lamina is maintained on the basis of cell adhesion molecules such as NCAM and L1CAM as well as extracellular matrix proteins including Neurolin/DM-GRASP/BEN and NrCAM (29). In chicken retina, growth cones respond to a CRYPa1 receptor ligand expressed on the glial precursors endfeet by activation of rac and rho via the Trio protein resulting in axon growth and maintained contact between the RGC lamellipodia and the basal membrane (252). Basal membrane laminin binds to integrin receptors on growth cones and activate Rac and Cdc42 to promote axon extension (252).
5.6.3 Repulsive cues
RGC growth cones are prevented from entering the deeper layers of the retina by neuroepithelial precursor cells somas, which have a repulsive effect on RGC axons but are permissive for RGC dendrites in cryoculture experiments (35, 253). Slit1 and Slit2 from the RGC and inner nuclear layers also repel Robo2 expressing RGC axons and their absence causes RGC axon misrouting in the outer retinal layers (29, 254–256) . RGC axon fasciculation defects within the optic nerve fiber layer, together with invasion of the INL, ONL and subretinal space are also seen in mice missing both Sfrp1 and Sfrp2 (257) and repulsive signals from pigmented cells in the outer retina keep the RGC axons from entering the subretinal space (113, 118).
5.7 Intraretinal fasciculation
Pioneer RGC axons serve as guides for the newly born axons so that optic disc targeting and nerve fiber layer restriction are achieved by fasciculation. Transient minor processes of newly born RGCs contact axons of more mature RGCs (243) and form bundles mainly based on immunoglobulin superfamily CAMs trans-homophilic interactions (27, 46). In goldfish, such molecules include L1, NrCAM or neurolin (258). In addition to hemophilic interactions, L1CAM also has heterophilic interactions with integrin receptors (259). FGF receptor blocking causes de-fasciculation in rat embryonic retina explant cultures (242). Transcription factor Irx4 has been shown to play a role in RGC axon fasciculation by down-regulating Slit1 (260). Inhibitory EphB proteins contribute to fasciculation in the dorsal retina (261). Several receptor-ligand pairs have been found to be complementary expressed in newly born versus maturing RGC and assumed to contribute to fasciculation (182).
5.8 Entering optic stalk/exiting the optic cup
5.8.1 Physical substrate
After reaching the ONH region RGC axons pause, make a 90 degrees turn and exit the eye into the OS (262). The optic fissure margins are in contact and the fusion process is ongoing when the first RGC axons are exiting the eye (91). The presence of the optic fissure is essential for RGC axon exit, as demonstrated by the aberrant projection of axons in the vitreous or in the subretinal space leading to optic nerve aplasia in Bmp7 knock-out mice lacking an optic fissure and hyaloid artery (68, 93, 114, 263).
The path of the axons is not in the fissures’ lumen, which is occupied by the hyaloid artery, but within the neuroepithelial cells forming its walls (216). Axons are separated from the hyaloid artery by a laminin sheet (91). In continuity with their retinal homologues, optic stalk glial precursor cells have processes that form channel-like networks enclosing the axons (151). The timing of appearance and propagation of this meshwork of cellular processes is correlated and preceding the wave of RGC differentiation (216).
A potential physical substrate in the optic stalk is represented by the rare retinopetal fibers coming from the diencephalon (264). In the ferret, these fibers are transient and occupy the optic stalk before the entrance of the pioneer retinofugal axons (265).
5.8.2 Attractive cues
The formation of the channel-like extracellular spaces is accompanied by cell death (216). NGF secreting macrophages invade the central retina and optic stalk shortly before RGC axon emergence to clear cell debris resulted from the apoptosis related to optic cup morphogenesis (101). The NGF receptors TrkA and p75NTR are expressed by RGC axons at this developmental stage (101, 266).
For the molecules expressed in the ONH region it is difficult to distinguish their role as OC-OS morphogens from the role as axon guidance cues (114). ONH Pax2 positive cells form a cuff that guides the axons to the OS keeping them isolated from the RPE domain (153). They extend processes expressing Netrin1, an attractive cue acting on Dcc (93). Mutant mice deficient for Netrin1 or Dcc have optic nerve hypoplasia due to the inability of RGC axons to leave the eye in spite of having arrived at the ONH (248). R-cadherin is also an attractive molecule expressed by the ONH cells in chicken (267). ONH cells identity and function are under the control of Pax2, Vax1 and Vax2 (93). Shh secreted by early-born RGCs is also involved in the development of the ONH Pax2 positive cells, so that its conditional deletion from RGCs in ThyCre Shh null/floxed mice is associated with reduced number of axons exiting the eye and misrouting in the subretinal space (68). The fact that a good number of axons are still exiting the eye in these mice may indicate that to a certain extent pioneer RGC axons are able to exit the eye without attractive cues, only based on optic fissure vicinity (optic fissure formation is Bmp7 dependent, unaffected in these mice). As RGCs do not secrete Shh, the differentiation of the ONH cells is impaired and they do not provide attractive cues for the later born RGC axons, maybe counting for the misrouting observed. From this we can infer that pioneer RGC axons enter the optic stalk physically guided by the closing optic fissure and secrete Shh to make the ONH produce attractive cues for the later born RGCs.
5.8.3 Repulsive cues
The change in growth direction at the ONH requires reverse signaling from attraction to repulsion so that axons growing towards the optic disc do not pass over it attracted by the cues on its opposite edge, but stop and enter through its center in the optic stalk. Fasciculation on other L1CAM expressing RGC axons coming from the opposite side of the retina must also be avoided otherwise axons are misrouted from one half of the retina to the other (27). Expression of inhibitory molecules such as EphA4, EphBs, Bmpr1b and NrCAM counteracts excessive fasciculation or axon stray in the subretinal space (29, 268).
The response of RGC axons to Netrin1 can be reversed based on the concomitant signals that regulate the intracellular level of cAMP or on expression of different Netrin1 receptors (187, 269). Laminin1, abundant in the basal lamina of the retinal vitreal side and closing optic fissure margins (91), binds to beta1 integrin receptors and blocks the cAMP increase induced by Netrin1 in RGCs thus changing Netrin1 attraction to repulsion (32, 262). By this mechanism, axons are guided away from the vitreal cavity and the optic fissure lumen and towards the intercellular spaces of the optic stalk neuroepithelium where laminin is absent and Netrin1 maintains its attractive effect (Figure 3, neuron represented in orange).
5.9 Traveling along the optic stalk
5.9.1 Physical substrate
In the optic stalk, RGC axons grow mostly in the ventral part, between non-neuronal cells with processes enwrapping the axon bundles (256). These cells are differentiated from vOS precursors or migrated from the diencephalon, as is the case for oligodendrocytes (118). Small separated bundles travelling between the neuroepithelial cells as well as independent growth cones are seen at the early stages, whereas later the optic stalk is occupied by compact axon fascicles with intermingled astrocyte precursor cells (112, 270).
5.9.2 Attractive cues
Later born axons fasciculate on the more mature ones in tight bundles (256) based on homophilic L1CAM interaction (243). Vax1 expression in the optic stalk and ventral diencephalon promotes growth cone progression from the ONH to the chiasm region (30). Transient retino-retinal projections were identified in multiple species including mice, projecting to the nasal retina (271). These misrouted axons probably come from the chiasm region and wrongly fasciculate with the fibers from the contralateral optic stalk.
5.9.3 Repulsive cues
While traveling through the optic stalk, RGC axons have to be prevented from straying away in the surrounding tissues. Netrin1 expression extends from the ONH cells to the OS neuroepithelium displaced peripherally by the incoming nerve fibers and has a repulsive effect thus keeping the axons in the center of the OS (248). Growth promoting Dcc receptor expression in newly born RGCs is switched to Unc5c and DsCAM on the maturing RGCs (whose axons are already in the optic stalk), receptors that respond to Netrin1 signals by inducing growth cone collapse (182, 269). Another barrier is a ring of Sema5a expression at the basal side of the optic stalk neuro-epithelial cells with axon growth inhibition and growth cone collapse effect (272, 273). Repulsive Slit2 expression is also detectable in the OS at the time it is invaded by RGC axons and is thought to contribute to their restriction to the ventral side of the OS (256, 273).
6 Discussion
This review harmonizes recent findings with classic studies on optic cup and stalk morphogenesis and early RGC axon guidance. We summarized the key molecular determinants of the two processes, as proven by genetic, immunological or pharmacological manipulations in animal models and we also extracted a list of genes expressed in RGCs during the developmental period of early axon path finding, whose functions in this process remains to be explored in future studies. The reviewed data orients our current understanding of this developmental event towards new directions which will be exposed here along with some unanswered questions that we propose for this research field.
As it was described in the first paragraphs of this review, morphogenetic movements bring the precursor tissue of the optic nerve head from the ventral diencephalic midline region to its final position, in the center of the neural retina. Such movements have been documented by software based cell tracking in zebrafish embryos (274). The RGC pioneer axons will follow almost the same path, but in the opposite sense, on the way to their next target, the optic chiasm. Anatomical continuity on this path is maintained throughout the morphogenesis of the optic cup and stalk by means of the optic fissure formation. By the time the pioneer RGC axons approach the optic stalk entrance, the fissure margins grow towards each-other, come in contact and begin to fuse. There is a narrow slit left at the OC-OS junction which guides the pioneer axons into the optic stalk. OS cells have apical – basal polarity, such that certain permissive cues are present on the lumen side, while repulsive cues are sequestered in the lateral walls of the epithelium, restricting penetration (273). Pioneer RGCs differentiate in the dorso-central retina next to the ONH precursor domain. The spatial and temporal correlation between ONH morphogenesis and pioneer RGCs differentiation appears to be essential for the correct pathfinding of the RGC axons.
Common trigger signals for the two events are yet to be identified. As a first evidence, FGF-RA signaling seems to play a key role in linking optic cup/optic stalk boundary delineation and optic fissure formation and closure to RGC differentiation (62, 78, 88, 97, 242). However, future work is needed in order to establish whether RGC axon misrouting caused by disruption of FGF-RA signaling is the result of ONH development anomalies or of intrinsic RGC developmental defects.
The interaction between the developing ONH and the emerging RGC axons is bidirectional. In one direction, the ONH guides RGC axons based on chemotaxis and haptotaxis. Early born RGCs are able to respond to the signals coming from the ONH by expressing cell surface receptors and by activating transcription programs that promote axon growth and axon steering events. Axons of RGCs from Atoh7 null mice, kept alive by Bax knock-out, are unable to target the ONH and to exit the eye in spite of receiving the correct signals from the target (173). Recently, adult mouse Mueller glia were reprogrammed to neurogenic state in vitro by virus mediated expression of Atoh7 (275) and in vivo conditional expression of Brn3b, Islet1, Ascl1 and Atoh1 resulted in RGC-like neurons with morphological and electrophysiological RGC properties, which did not send axons to the ONH in spite of expressing many axon growth and guidance promoting genes (276). A potential future direction of research would be to also reprogram optic nerve astrocytes to secrete axon guidance molecules for the new RGC axons or to engineer the new RGCs to penetrate the adult lamina cribrosa, by uncovering and neutralizing the inhibitory cues. Another direction could be to simulate the physical properties of the closing optic fissure in order to promote haptotaxis based axon guidance. Experiments with spheroids of human stem cell-derived motor neurons showed their capacity to spontaneously assemble into an unidirectional fascicle when cultured next to a narrow channel (277). Simulating developing ONH environment locally may be also a solution for promoting RGC axonogenesis and survival in retinal organoids. Later born axons find their way to the ONH based on fasciculation on pioneer axons. In zebrafish retina, late born RGC axons cannot target the OD in the absence of early RGCs although the OD cues are present (278). This observation has two possible explanations: the canonical one is that pioneer axons actively find the ONH region and later born RGCs are guided passively so that they are not able to actively respond to ONH attractive cues but an alternative possibility would be that both pioneer and late born axons are guided passively to the ONH and only the position of the first RGCs next to the closing optic fissure enables them to exit the eye. The approaching margins of the optic fissure bringing the first axons in contact so that they can fasciculate may be enough for their growth out of the eye. For the RGC axons arriving at the optic disc after the optic fissure closed there is no physical path out of the eye, so that chemotaxis and fasciculation are become essential for axon guidance. Live imaging studies capturing the behavior of these later born axons at the optic disc are needed to confirm this hypothesis. Further studies involving desynchronizing optic fissure fusion and initiation of RGC differentiation or de-localizing the initial RGC differentiation spot could verify this later hypothesis.
In the other direction, RGCs also influence the ONH development. The reviewed studies on Shh secretion by RGCs and its role in ONH cells development as well as retinal precursor cell modulation indicate that RGCs actively influence the development of their path to the brain as well as the surrounding retina. More work in this direction should be done in order to find all the secreted molecules involved in this processes and their potential application in RGC regeneration strategies.
In summary, RGC axons follow a centripetal course within the inner most layer of the retina towards the optic disc and enter the optic stalk. In spite of the appearance of the mature optic nerve, RGC axons are not piercing through the wall of the eye, but they are gliding on a continuous path that is created during the morphogenesis of the optic nerve. They are guided by chemotaxis and haptotaxis cues provided by the developing optic cup and stalk and by fasciculation with their more mature neighbors. A profound understanding of the developmental events described in this review should encourage the perception of the eye not as a peripheral sensory organ that later connects with the brain, but as a continuous extension of the subcortical brain. The developing ventral diencephalon projects to the surface of the head to capture light stimuli and attracts back the RGC axons to receive the processed visual information.
Author contributions
RP and TB framed the organization of the review and gathered literature. RP wrote the manuscript draft, generated tables and figures. RP and TB edited manuscript. All authors contributed to the article and approved the submitted version.
Funding
Research funded by UEFISCDI, through grant number PN-III-P4-PCE-2021-0333, to TB and RP.
Conflict of interest
The authors declare that the research was conducted in the absence of any commercial or financial relationships that could be construed as a potential conflict of interest.
Publisher’s note
All claims expressed in this article are solely those of the authors and do not necessarily represent those of their affiliated organizations, or those of the publisher, the editors and the reviewers. Any product that may be evaluated in this article, or claim that may be made by its manufacturer, is not guaranteed or endorsed by the publisher.
Supplementary material
The Supplementary Material for this article can be found online at: https://www.frontiersin.org/articles/10.3389/fopht.2023.1180142/full#supplementary-material
References
1. Wässle H. Parallel processing in the mammalian retina. Nat Rev Neurosci (2004) 5(10):747–57. doi: 10.1038/nrn1497
2. Rousso DL, Qiao M, Kagan RD, Yamagata M, Palmiter RD, Sanes JR. Two pairs of ON and OFF retinal ganglion cells are defined by intersectional patterns of transcription factor expression. Cell Rep (2016) 15(9):1930–44. doi: 10.1016/j.celrep.2016.04.069
3. Martersteck EM, Hirokawa KE, Evarts M, Bernard A, Duan X, Li Y, et al. Diverse central projection patterns of retinal ganglion cells. Cell Rep (2017) 18(8):2058–72. doi: 10.1016/j.celrep.2017.01.075
4. Briggman KL, Helmstaedter M, Denk W. Wiring specificity in the direction-selectivity circuit of the retina. Nature (2011) 471(7337):183–90. doi: 10.1038/nature09818
5. Masland RH. The neuronal organization of the retina. Neuron (2012) 76(2):266–80. doi: 10.1016/j.neuron.2012.10.002
6. Masland RH. The fundamental plan of the retina. Nat Neurosci (2001) 4(9):877–86. doi: 10.1038/nn0901-877
7. Bae JA, Mu S, Kim JS, Turner NL, Tartavull I, Kemnitz N, et al. Digital museum of retinal ganglion cells with dense anatomy and physiology. Cell (2018) 173(5):1293–1306.e19. doi: 10.1016/j.cell.2018.04.040
8. Badea TC, Nathans J. Quantitative analysis of neuronal morphologies in the mouse retina visualized by using a genetically directed reporter. J Comp Neurol (2004) 480(4):331–51. doi: 10.1002/cne.20304
9. Sun H, Rüttiger L, Lee BB. The spatiotemporal precision of ganglion cell signals: a comparison of physiological and psychophysical performance with moving gratings. Vision Res (2004) 44(1):19–33. doi: 10.1016/j.visres.2003.08.017
10. Coombs J, van der List D, Wang GY, Chalupa LM. Morphological properties of mouse retinal ganglion cells. Neuroscience (2006) 140(1):123–36. doi: 10.1016/j.neuroscience.2006.02.079
11. Rivlin-Etzion M, Zhou K, Wei W, Elstrott J, Nguyen PL, Barres BA, et al. Transgenic mice reveal unexpected diversity of on-off direction-selective retinal ganglion cell subtypes and brain structures involved in motion processing. J Neurosci (2011) 31(24):8760–9. doi: 10.1523/JNEUROSCI.0564-11.2011
12. Berens P, Euler T. Neuronal diversity in the retina. Neuroforum (2017) 23(2):431–6. doi: 10.1515/nf-2016-A055
13. Baden T, Berens P, Franke K, Román Rosón M, Bethge M, Euler T. The functional diversity of retinal ganglion cells in the mouse. Nature (2016) 529(7586):345. doi: 10.1038/nature16468
14. He S, Dong W, Deng Q, Weng S, Sun W. Seeing more clearly: recent advances in understanding retinal circuitry. Science (2003) 302(5644):408–11. doi: 10.1126/science.1085457
15. Gollisch T, Meister M. Eye smarter than scientists believed: neural computations in circuits of the retina. Neuron (2010) 65(2):150–64. doi: 10.1016/j.neuron.2009.12.009
16. Ölveczky BP, Baccus SA, Meister M. Segregation of object and background motion in the retina. Nature (2003) 423(6938):401–8. doi: 10.1038/nature01652
17. Meister M, Berry MJ. The neural code of the retina review a sample problem. Neuron (1999) 22:435–50. doi: 10.1016/S0896-6273(00)80700-X
18. Riehl R, Johnson K, Bradley R, Grunwald GB, Cornel E, Lilienbaum A, et al. Cadherin function is required for axon outgrowth in retinal ganglion cells in vivo. Neuron (1996) 17(5):837–48. doi: 10.1016/S0896-6273(00)80216-0
19. Goetz J, Jessen ZF, Jacobi A, Mani A, Cooler S, Greer D, et al. Unified classification of mouse retinal ganglion cells using function, morphology, and gene expression. Cell Rep (2022) 40(2):111040. doi: 10.1016/j.celrep.2022.111040
20. Tran NM, Shekhar K, Whitney IE, Jacobi A, Benhar I, Hong G, et al. Single-cell profiles of retinal ganglion cells differing in resilience to injury reveal neuroprotective genes. Neuron (2019) 104(6):1039–1055.e12. doi: 10.1016/j.neuron.2019.11.006
21. Rheaume BA, Jereen A, Bolisetty M, Sajid MS, Yang Y, Renna K, et al. Single cell transcriptome profiling of retinal ganglion cells identifies cellular subtypes. Nat Commun (2018) 9(1):2759. doi: 10.1038/s41467-018-05134-3
22. Lilienbaum A, Reszka AA, Horwitz AF, Holt CE. Chimeric integrins expressed in retinal ganglion cells impair process outgrowth in vivo. Mol Cell Neurosci (1995) 6(2):139–52. doi: 10.1006/mcne.1995.1013
23. Sajgo S, Ali S, Popescu O, Badea TC. Dynamic expression of transcription factor Brn3b during mouse cranial nerve development. J Comp Neurol (2016) 524(5):1033–61. doi: 10.1002/cne.23890
24. Hinds JW, Hinds PL. Early ganglion cell differentiation in the mouse retina: an electron microscopic analysis utilizing serial sections. Dev Biol (1974) 37(2):381–416. doi: 10.1016/0012-1606(74)90156-0
25. Silver J, Sidman RL. A mechanism for the guidance and topographic patterning of retinal ganglion cell axons. J Comp Neurol (1980) 189(1):101–11. doi: 10.1002/cne.901890106
26. Shekhar K, Whitney IE, Butrus S, Peng YR, Sanes JR. Diversification of multipotential postmitotic mouse retinal ganglion cell precursors into discrete types. Elife (2022) 11:e73809. doi: 10.7554/eLife.73809
27. Oster SF, Deiner M, Birgbauer E, Sretavan DW. Ganglion cell axon pathfinding in the retina and optic nerve. Semin Cell Dev Biol (2004) 15(1):125–36. doi: 10.1016/j.semcdb.2003.09.006
28. Herrera E, Erskine L, Morenilla-Palao C. Guidance of retinal axons in mammals. Semin Cell Dev Biol (2019) 85:48–59. doi: 10.1016/j.semcdb.2017.11.027
29. Erskine L, Herrera E. The retinal ganglion cell axon’s journey: insights into molecular mechanisms of axon guidance. Dev Biol (2007) 308(1):1–14. doi: 10.1016/j.ydbio.2007.05.013
30. Erskine L, Herreral E. Connecting the retina to the brain. ASN Neuro (2015) 6(6):1759091414562107. doi: 10.1177/1759091414562107
31. Mason C, Slavi N. Retinal ganglion cell axon wiring establishing the binocular circuit. Annu Rev Vision Science (2020) 6:215–36. doi: 10.1146/annurev-vision-091517-
32. Mann F, Harris WA, Holt CE. New views on retinal axon development: a navigation guide. Int J Dev Biol (2004) 48(8–9):957–64. doi: 10.1387/ijdb.041899fm
33. Sánchez-Camacho C, Bovolenta P. Emerging mechanisms in morphogen-mediated axon guidance. BioEssays (2009) 31:1013–25. doi: 10.1002/bies.200900063
34. Rebsam A, Petros TJ, Mason CA. Switching retinogeniculate axon laterality leads to normal targeting but abnormal eye-specific segregation that is activity dependent. J Neurosci (2009) 29(47):14855–63. doi: 10.1523/JNEUROSCI.3462-09.2009
35. Stuermer CAO, Bastmeyer M. The retinal axon’s pathfinding to the optic disk. Prog Neurobiology (2000) 62:197–214. doi: 10.1016/S0301-0082(00)00012-5
36. Petros TJ, Rebsam A, Mason CA. Retinal axon growth at the optic chiasm: to cross or not to cross. Annu Rev Neurosci (2008) 31:295–315. doi: 10.1146/annurev.neuro.31.060407.125609
37. Taylor D. Optic nerve axons: life and death before birth. Eye (2005) 19(5):499–527. doi: 10.1038/sj.eye.6701857
38. Borchert M. Reappraisal of the optic nerve hypoplasia syndrome. J Neuro-Ophthalmology (2012) 32:58–67. doi: 10.1097/WNO.0b013e31824442b8
39. Bovolenta P, Martinez-Morales JR. Genetics of congenital eye malformations: insights from chick experimental embryology. Hum Genet Springer Verlag; (2019) 138:1001–6. doi: 10.1007/s00439-018-1900-5
40. Cardozo MJ, Almuedo-Castillo M, Bovolenta P. Patterning the vertebrate retina with morphogenetic signaling pathways. Neuroscientist. SAGE Publications Inc. (2020) 26:185–96. doi: 10.1177/1073858419874016
41. Cavalheiro S, Yagmurlu K, da Costa MDS, Nicácio JM, Rodrigues TP, Chaddad-Neto F, et al. Surgical approaches for brainstem tumors in pediatric patients. Child’s Nerv Syst (2015) 31(10):1815–40. doi: 10.1007/s00381-015-2799-y
42. Hocking JC, Famulski JK, Yoon KH, Widen SA, Bernstein CS, Koch S, et al. Morphogenetic defects underlie superior coloboma, a newly identified closure disorder of the dorsal eye. Mol Biol (2018) 14(3):e1007246. doi: 10.1371/journal.pgen.1007246.g001
43. Marcos S, González-Lázaro M, Beccari L, Carramolino L, Martin-Bermejo MJ, Amarie O, et al. Meis1 coordinates a network of genes implicated in eye development and microphthalmia. Development (2015) 142(17):3009–20. doi: 10.1242/dev.122176
44. Dahl S, Pettersson M, Eisfeldt J, Schro AK. Whole genome sequencing unveils genetic heterogeneity in optic nerve hypoplasia. PLoS One (2020) 15(20):1–16. doi: 10.1371/journal.pone.0228622
45. Wall PB, Traboulsi EI. Congenital abnormalities of the optic Nerve: from gene mutation to clinical expression. Curr Neurol Neurosci Rep (2013) 13(7):363. doi: 10.1007/s11910-013-0363-2
46. Brittis PA, Lemmon V, Rutishauser U, Silver J. Unique changes of ganglion cell growth cone behavior following cell adhesion molecule perturbations: a time-lapse study of the living retina. Mol Cell Neurosci (1995) 6(5):433–49. doi: 10.1006/mcne.1995.1032
47. Chow RL, Lang RA. Early eye development in vertebrates. Annu Rev Cell Dev Biol (2001) 17:255–96. doi: 10.1146/annurev.cellbio.17.1.255
48. Heavner W, Pevny L. Eye development and retinogenesis. Cold Spring Harb Perspect Biol (2012) 4(12):a008391. doi: 10.1101/cshperspect.a008391
49. Miesfeld JB, Brown NL. Eye organogenesis: a hierarchical view of ocular development. In: Current topics in developmental biology. Cambridge, MA, United States: Academic Press Inc (2019). p. 351–93.
50. Tao C, Zhang X. Development of astrocytes in the vertebrate eye. Dev Dynamics (2014) 243:1501–10. doi: 10.1002/dvdy
51. Patel A, Sowden JC. Genes and pathways in optic fissure closure. Semin Cell Dev Biol (2019) 91:55–65. doi: 10.1016/j.semcdb.2017.10.010
52. Van Cruchten S, Vrolyk V, Perron Lepage MF, Baudon M, Voute H, Schoofs S, et al. Pre- and postnatal development of the eye: a species comparison. Birth Defects Res (2017) 109:1540–67. doi: 10.1002/bdr2.1100
53. Martínez-Morales JR, Rodrigo I, Bovolenta P. Eye development: a view from the retina pigmented epithelium. BioEssays (2004) 26(7):766–77. doi: 10.1002/bies.20064
54. Sinn R, Wittbrodt J. An eye on eye development. Mech Dev (2013) 130(6–8):347–58. doi: 10.1016/j.mod.2013.05.001
55. Nakano T, Ando S, Takata N, Kawada M, Muguruma K, Sekiguchi K, et al. Self-formation of optic cups and storable stratified neural retina from human ESCs. Cell Stem Cell (2012) 10(6):771–85. doi: 10.1016/j.stem.2012.05.009
56. Hasegawa Y, Takata N, Okuda S, Kawada M, Eiraku M, Sasai Y. Emergence of dorsal-ventral polarity in ESC-derived retinal tissue. Development (2016) 143(21):3895–906. doi: 10.1242/dev.134601
57. Fligor CM, Lavekar SS, Harkin J, Shields PK, VanderWall KB, Huang KC, et al. Extension of retinofugal projections in an assembled model of human pluripotent stem cell-derived organoids. Stem Cell Rep (2021) 16(9):2228–41. doi: 10.1016/j.stemcr.2021.05.009
58. Gabriel E, Albanna W, Pasquini G, Ramani A, Josipovic N, Mariappan A, et al. Human brain organoids assemble functionally integrated bilateral optic vesicles. Cell Stem Cell (2021) 28(10):1740–1757.e8. doi: 10.1016/j.stem.2021.07.010
59. Esteve P, Bovolenta P. Secreted inducers in vertebrate eye development: more functions for old morphogens. Curr Opin Neurobiol (2006) 16(1):13–9. doi: 10.1016/j.conb.2006.01.001
60. McCabe KL, Gunther EC, Reh TA. The development of the pattern of retinal ganglion cells in the chick retina: mechanisms that control differentiation. Development (1999) 126(24):5713–24. doi: 10.1242/dev.126.24.5713
61. Steinfeld J, Steinfeld I, Bausch A, Coronato N, Hampel ML, Depner H, et al. BMP-induced reprogramming of the neural retina into retinal pigment epithelium requires wnt signalling. Biol Open (2017) 6(7):979–92. doi: 10.1242/bio.018739
62. Cai Z, Tao C, Li H, Ladher R, Gotoh N, Feng GS, et al. Deficient FGF signaling causes optic nerve dysgenesis and ocular coloboma. Development (2013) 140(13):2711–23. doi: 10.1242/dev.089987
63. Bosze B, Suarez-Navarro J, Soofi A, Lauderdale JD, Dressler GR, Brown NL. Multiple roles for Pax2 in the embryonic mouse eye. Dev Biol (2021) 472(January):18–29. doi: 10.1016/j.ydbio.2020.12.020
64. Bailey TJ, El-Hodiri H, Zhang L, Shah R, Mathers PH, Jamrich M. Regulation of development by rx genes. Int J Dev Biol (2004) 48(8–9):761–70. doi: 10.1387/ijdb.041878tb
65. Wang X, Lupo G, He R, Barsacchi G, Harris WA, Liu Y. Dorsoventral patterning of the xenopus eye involves differential temporal changes in the response of optic stalk and retinal progenitors to hh signalling. Neural Dev (2015) 10(1):1–11. doi: 10.1186/s13064-015-0035-9
66. Chiang C, Litingtung Y, Lee E, Youngt KE, Cordent JL, Westphal H, et al. Mice lacking sonic hedgehog gene function. Nature (1996) 383:407–13. doi: 10.1038/383407a0
67. Hyer J, Mima T, Mikawa T. FGF1 patterns the optic vesicle by directing the placement of the neural retina domain. Development (1998) 125(5):869–77. doi: 10.1242/dev.125.5.869
68. Dakubo GD, Wang YP, Mazerolle C, Campsall K, McMahon AP, Wallace VA. Retinal ganglion cell-derived sonic hedgehog signaling is required for optic disc and stalk neuroepithelial cell development. Development (2003) 130(13):2967–80. doi: 10.1242/dev.00515
69. Tang K, Xie X, Park JI, Jamrich M, Tsai S, Tsai MJ. COUP-TFs regulate eye development by controlling factors essential for optic vesicle morphogenesis. Development (2010) 137(5):725–34. doi: 10.1242/dev.040568
70. Grindley JC, Davidson DR, Hill RE. The role of pax-6 in eye and nasal development. Development (1995) 121(5):1433–42. doi: 10.1242/dev.121.5.1433
71. Ivanovitch K, Cavodeassi F, Wilson SW. Precocious acquisition of neuroepithelial character in the eye field underlies the onset of eye morphogenesis. Dev Cell (2013) 27(3):293–305. doi: 10.1016/j.devcel.2013.09.023
72. Yamada R, Oguri A, Fujiki K, Shirahige K, Takezoe H, Takahashi N, et al. Single-cell transcriptional analysis reveals developmental stage-dependent changes in retinal progenitors in the murine early optic vesicle. Biochem Biophys Res Commun (2021) 543:80–6. doi: 10.1016/j.bbrc.2021.01.043
73. Tétreault N, Champagne MP, Bernier G. The LIM homeobox transcription factor Lhx2 is required to specify the retina field and synergistically cooperates with Pax6 for Six6 trans-activation. Dev Biol (2009) 327(2):541–50. doi: 10.1016/j.ydbio.2008.12.022
74. Burnett JB, Lupu FI, Eggenschwiler JT. Proper ciliary assembly is critical for restricting hedgehog signaling during early eye development in mice. Dev Biol (2017) 430(1):32–40. doi: 10.1016/j.ydbio.2017.07.012
75. Take-uchi M, Clarke JDW, Wilson SW. Hedgehog signalling maintains the optic stalk-retinal interface through the regulation of Vax gene activity. Development (2003) 130(5):955–68. doi: 10.1242/dev.00305
76. Pfirrmann T, Jandt E, Ranft S, Lokapally A, Neuhaus H, Perron M, et al. Hedgehog-dependent E3-ligase Midline1 regulates ubiquitin-mediated proteasomal degradation of Pax6 during visual system development. Proc Natl Acad Sci USA (2016) 113(36):10103–8. doi: 10.1073/pnas.1600770113
77. Bosze B, Moon MS, Kageyama R, Brown NL. Simultaneous requirements for Hes1 in retinal neurogenesis and optic cup⇓stalk boundary maintenance. J Neurosci (2020) 40(7):1501–13. doi: 10.1523/JNEUROSCI.2327-19.2020
78. Martinez-Morales JR, Del Bene F, Nica G, Hammerschmidt M, Bovolenta P, Wittbrodt J. Differentiation of the vertebrate retina is coordinated by an FGF signaling center. Dev Cell (2005) 8(4):565–74. doi: 10.1016/j.devcel.2005.01.022
79. Vogel-Höpker A, Momose T, Rohrer H, Yasuda K, Ishihara L, Rapaport DH. Multiple functions of fibroblast growth factor-8 (FGF-8) in chick eye development. Mech Dev (2000) 94(1–2):25–36. doi: 10.1016/S0925-4773(00)00320-8
80. Horsford DJ, Nguyen MTT, Sellar GC, Kothary R, Arnheiter H, McInnes RR. Chx10 repression of mitf is required for the maintenance of mammalian neuroretinal identity. Development (2005) 132(1):177–87. doi: 10.1242/dev.01571
81. Nguyen MTT, Arnheiter H. Signaling and transcriptional regulation in early mammalian eye development: a link between FGF and MITF. Development (2000) 127(16):3581–91. doi: 10.1242/dev.127.16.3581
82. Picker A, Brand M. Fgf signals from a novel signaling center determine axial patterning of the prospective neural retina. Development (2005) 132(22):4951–62. doi: 10.1242/dev.02071
83. Fuhrmann S, Ramirez S, Abouda MM, Campbell CD. Porcn is essential for growth and invagination of the mammalian optic cup. Front Cell Dev Biol (2022) 10:1016182. doi: 10.3389/fcell.2022.1016182
84. Carpenter AC, Smith AN, Wagner H, Cohen-Taya Y, Rao S, Wallace V, et al. Wnt ligands from the embryonic surface ectoderm regulate “bimetallic strip” optic cup morphogenesis in mouse. Development (2015) 142(5):972–82. doi: 10.1242/dev.120022
85. Klimova L, Lachova J, Machon O, Sedlacek R, Kozmik Z. Generation of mRx-cre transgenic mouse line for efficient conditional gene deletion in early retinal progenitors. PloS One (2013) 8(5):e63029. doi: 10.1371/journal.pone.0063029
86. Zhang XM, Liu DTL, Chiang SWY, Choy KW, Pang CP, Lam DSC, et al. Immunopanning purification and long-term culture of human retinal ganglion cells. Mol Vis (2010) 16(December):2867–72.
87. Cvekl A, Wang WL. Retinoic acid signaling in mammalian eye development. Exp Eye Res (2009) 89(3):280–91. doi: 10.1016/j.exer.2009.04.012
88. da Silva S, Cepko CL. Fgf8 expression and degradation of retinoic acid are required for patterning a high-acuity area in the retina. Dev Cell (2017) 42(1):68–81.e6. doi: 10.1016/j.devcel.2017.05.024
89. Yun S, Saijoh Y, Hirokawa KE, Kopinke D, Murtaugh LC, Monukï ES, et al. Lhx2 links the intrinsic and extrinsic factors that control optic cup formation. Development (2009) 136(23):3895–906. doi: 10.1242/dev.041202
90. Macdonald R, Barth KA, Xu Q, Holder N, Mikkola I, Wilson SW. Midline signalling is required for pax gene regulation and patterning of the eyes. Development (1995) 121(10):3267–78. doi: 10.1242/dev.121.10.3267
91. Bernstein CS, Anderson MT, Gohel C, Slater K, Gross JM, Agarwala S. The cellular bases of choroid fissure formation and closure. Dev Biol (2018) 440(2):137–51. doi: 10.1016/j.ydbio.2018.05.010
92. Kolpak A, Zhang J, Bao ZZ. Sonic hedgehog has a dual effect on the growth of retinal ganglion axons depending on its concentration. J Neurosci (2005) 25(13):3432–41. doi: 10.1523/JNEUROSCI.4938-04.2005
93. Morcillo J, Martínez-Morales JR, Trousse F, Fermin Y, Sowden JC, Bovolenta P. Proper patterning of the optic fissure requires the sequential activity of BMP7 and SHH. Development (2006) 133(16):3179–90. doi: 10.1242/dev.02493
94. Liu C, Nathans J. An essential role for frizzled 5 in mammalian ocular development. Development (2008) 135(21):3567–76. doi: 10.1242/dev.028076
95. Hyatt GA, Schmitt EA, Marsh-Armstrong N, McCaffery P, Dräger UC, Dowling JE. Retinoic acid establishes ventral retinal characteristics. Development (1996) 122(1):195–204. doi: 10.1242/dev.122.1.195
96. Boobalan E, Thompson AH, Alur RP, McGaughey DM, Dong L, Shih G, et al. Zfp503/Nlz2 is required for RPE differentiation and optic fissure closure. Invest Ophthalmol Vis Sci (2022) 63(12):5–5. doi: 10.1167/iovs.63.12.5
97. Chen S, Li H, Gaudenz K, Paulson A, Guo F, Trimble R, et al. Defective FGF signaling causes coloboma formation and disrupts retinal neurogenesis. Cell Res (2013) 23(2):254–73. doi: 10.1038/cr.2012.150
98. Makrides N, Wang Q, Tao C, Schwartz S, Zhang X. Jack of all trades, master of each: the diversity of fibroblast growth factor signalling in eye development. Open Biol (2022) 12(1):210265. doi: 10.1098/rsob.210265
99. Knickmeyer MD, Mateo JL, Eckert P, Roussa E, Rahhal B, Zuniga A, et al. TGFβ-facilitated optic fissure fusion and the role of bone morphogenetic protein antagonism. Open Biol (2018) 8(3). doi: 10.1098/rsob.170134
100. Hardy H, Prendergast JG, Patel A, Dutta S, Trejo-Reveles V, Kroeger H, et al. Detailed analysis of chick optic fissure closure reveals netrin-1 as an essential mediator of epithelial fusion. Elife (2019) 8:1–23. doi: 10.7554/eLife.43877
101. Rodríguez-Gallardo L, Lineros-Domínguez MDC, Francisco-Morcillo J, Martín-Partido G. Macrophages during retina and optic nerve development in the mouse embryo: relationship to cell death and optic fibres. Anat Embryol (Berl) (2005) 210(4):303–16. doi: 10.1007/s00429-005-0051-3
102. Bäumer N, Marquardt T, Stoykova A, Ashery-Padan R, Chowdhury K, Gruss P. Pax6 is required for establishing naso-temporal and dorsal characteristics of the optic vesicle. Development (2002) 129(19):4535–45. doi: 10.1242/dev.129.19.4535
103. Lupo G, Liu Y, Qiu R, Chandraratna RAS, Barsacchi G, He RQ, et al. Dorsoventral patterning of the xenopus eye: a collaboration of retinoid, hedgehog and FGF receptor signaling. Development (2005) 132(7):1737–48. doi: 10.1242/dev.01726
104. Zhang XM, Yang XJ. Temporal and spatial effects of sonic hedgehog signaling in chick eye morphogenesis. Dev Biol (2001) 233(2):271–90. doi: 10.1006/dbio.2000.0195
105. Hernández-Bejarano M, Gestri G, Spawls L, Nieto-López F, Picker A, Tada M, et al. Opposing shh and fgf signals initiate nasotemporal patterning of the zebrafish retina. Development (2015) 142(22):3933–42. doi: 10.1242/dev.125120
106. Marcus RC, Gale NW, Morrison ME, Mason CA, Yancopoulos GD. Eph family receptors and their ligands distribute in opposing gradients in the developing mouse retina. Dev Biol (1996) 180(2):786–9. doi: 10.1006/dbio.1996.0347
107. Martinez-Morales JR, Signore M, Acampora D, Simeone A, Bovolenta P. Otx genes are required for tissue specification in the developing eye. Development (2001) 128(11):2019–30. doi: 10.1242/dev.128.11.2019
108. Marcucci F, Murcia-Belmonte V, Wang Q, Coca Y, Ferreiro-Galve S, Kuwajima T, et al. The ciliary margin zone of the mammalian retina generates retinal ganglion cells. Cell Rep (2016) 17(12):3153–64. doi: 10.1016/j.celrep.2016.11.016
109. M.hanson I, Seawright A, Hardman K, Hodgson S, Zaletayev D, Fekete G, et al. PAX6 mutations in aniridia. Hum Mol Genet (1993) 2(7):915–20. doi: 10.1093/hmg/2.7.915
110. Liu J, Sanes JR. Cellular and molecular analysis of dendritic morphogenesis in a retinal cell type that senses color contrast and ventral motion. J Neurosci (2017) 37(50):12247–62. doi: 10.1523/JNEUROSCI.2098-17.2017
111. Mui SH, Kim JW, Lemke G, Bertuzzi S. Vax Genes ventralize the embryonic eye. Genes Dev (2005) 19(10):1249–59. doi: 10.1101/gad.1276605
112. Colello RJ, Guillery RW. Observations on the early development of the optic nerve and tract of the mouse. J Comp Neurol (1992) 317(4):357–78. doi: 10.1002/cne.903170404
113. Silver J, Sapiro J. Axonal guidance during development of the optic nerve: the role of pigmented epithelia and other extrinsic factors. J Comp Neurol (1981) 202:521–38. doi: 10.1002/cne.902020406
114. Bao ZZ. Intraretinal projection of retinal ganglion cell axons as a model system for studying axon navigation. Brain Res (2008) 1192:165–77. doi: 10.1016/j.brainres.2007.01.116
115. Cai Z, Grobe K, Zhang X. Role of heparan sulfate proteoglycans in optic disc and stalk morphogenesis. Dev Dyn (2014) 243(10):1310–6. doi: 10.1002/dvdy.24142
116. Huang J, Liu Y, Oltean A, Beebe DC. Bmp4 from the optic vesicle specifies murine retina formation. Dev Biol (2015) 402(1):119–26. doi: 10.1016/j.ydbio.2015.03.006
117. Chang KC, Hertz J, Zhang X, Jin XL, Shaw P, Derosa BA, et al. Novel regulatory mechanisms for the SoxC transcriptional network required for visual pathway development. J Neurosci (2017) 37(19):4967–81. doi: 10.1523/JNEUROSCI.3430-13.2017
118. Torres M, Gómez-Pardo E, Gruss P. Pax2 contributes to inner ear patterning and optic nerve trajectory. Development (1996) 122(11):3381–91. doi: 10.1242/dev.122.11.3381
119. Bertuzzi S, Hindges R, Mui SH, O’Leary DM, Lemke G. The homeodomain protein Vax1 is required for axon guidance and major tract formation in the developing forebrain. Genes Dev (1999) 13(23):3092–105. doi: 10.1101/gad.13.23.3092
120. Wang Y, Dakubo GD, Thurig S, Mazerolle CJ, Wallace VA. Retinal ganglion cell-derived sonic hedgehog locally controls proliferation and the timing of RGC development in the embryonic mouse retina. Development (2005) 132(22):5103–13. doi: 10.1242/dev.02096
121. Elliott J, Jolicoeur C, Ramamurthy V, Cayouette M. Ikaros confers early temporal competence to mouse retinal progenitor cells. Neuron (2008) 60(1):26–39. doi: 10.1016/j.neuron.2008.08.008
122. Xiang M. Requirement for brn-3b in early differentiation of postmitotic retinal ganglion cell precursors. Dev Biol (1998) 197:155–69. doi: 10.1006/dbio.1998.8868
123. Qiu F, Jiang H, Xiang M. A comprehensive negative regulatory program controlled by Brn3b to ensure ganglion cell specification from multipotential retinal precursors. J Neurosci (2008) 28(13):3392–403. doi: 10.1523/JNEUROSCI.0043-08.2008
124. Colello RJ, Guillery RW. The early development of retinal ganglion cells with uncrossed axons in the mouse: retinal position and axonal course. Development (1990) 108(3):515–23. doi: 10.1242/dev.108.3.515
125. Pan L, Yang Z, Feng L, Gan L. Functional equivalence of Brn3 POU-domain transcription factors in mouse retinal neurogenesis. Development (2005) 132(4):703–12. doi: 10.1242/dev.01646
126. Marquardt T, Ashery-Padan R, Andrejewski N, Scardigli R, Guillemot F, Gruss P. Pax6 is required for the multipotent state of retinal progenitor cells. Cell (2001) 105(1):43–55. doi: 10.1016/S0092-8674(01)00295-1
127. Yi JJ, Barnes AP, Hand R, Polleux F, Ehlers MD. TGF-β signaling specifies axons during brain development. Cell (2010) 142(1):144–57. doi: 10.1016/j.cell.2010.06.010
128. Anderson SR, Zhang J, Steele MR, Romero CO, Kautzman AG, Schafer DP, et al. Complement targets newborn retinal ganglion cells for phagocytic elimination by microglia. J Neurosci (2019) 39(11):2025–40. doi: 10.1523/JNEUROSCI.1854-18.2018
129. Bélanger MC, Robert B, Cayouette M. Msx1-positive progenitors in the retinal ciliary margin give rise to both neural and non-neural progenies in mammals. Dev Cell (2017) 40(2):137–50. doi: 10.1016/j.devcel.2016.11.020
130. Muzyka VV, Badea TC. Genetic interplay between transcription factor Pou4f1/Brn3a and neurotrophin receptor ret in retinal ganglion cell type specification. Neural Dev (2021) 16(1):1–26. doi: 10.1186/s13064-021-00155-z
131. Kim N, Min KWoo., Kang K, Lee EJ, Kim HT, Moon K, et al. Regulation of retinal axon growth by secreted Vax1 homeodomain protein. Elife (2014) 3:e02671. doi: 10.7554/eLife.02671
132. Bovolenta P, Mason C. Growth cone morphology varies with position in the developing mouse visual pathway from retina to first targets. J Neurosci (1987) 7(5):1447–60. doi: 10.1523/JNEUROSCI.07-05-01447.1987
133. Shewan D, Dwivedy A, Anderson R, Holt CE. Age-related changes underlie switch in netrin-1 responsiveness as growth cones advance along visual pathway. Nat Neurosci (2002) 5(10):955–62. doi: 10.1038/nn919
134. Geraldo S, Gordon-Weeks PR. Cytoskeletal dynamics in growth-cone steering. J Cell Sci (2009) 122(20):3595–604. doi: 10.1242/jcs.042309
135. Sobue K, Kanda K. Alpha-actinins, calspectin (brain spectrin or fodrin), and actin participate in adhesion and movement of growth cones. Neuron (1989) 3(3):311–9. doi: 10.1016/0896-6273(89)90255-9
136. Campbell DS, Holt CE. Chemotropic responses of retinal growth cones mediated by rapid local protein synthesis and degradation. Neuron (2001) 32(6):1013–26. doi: 10.1016/S0896-6273(01)00551-7
137. Zivraj KH, Tung YCL, Piper M, Gumy L, Fawcett JW, Yeo GSH, et al. Subcellular profiling reveals distinct and developmentally regulated repertoire of growth cone mRNAs. J Neurosci (2010) 30(46):15464–78. doi: 10.1523/JNEUROSCI.1800-10.2010
138. Aih CL, Suter DM. Quantitative analysis of microtubule dynamics during adhesion-mediated growth cone guidance. Dev Neurobiol (2008) 68(12):1363–77. doi: 10.1002/dneu.20662
139. Mason CA, Wang LC. Growth cone form is behavior-specific and, consequently, position- specific along the retinal axon pathway. J Neurosci (1997) 17(3):1086–100. doi: 10.1523/JNEUROSCI.17-03-01086.1997
140. Godement P, Salaün J, Imbert M. Prenatal and postnatal development of retinogeniculate and retinocollicular projections in the mouse. J Comp Neurol (1984) 230(4):552–75. doi: 10.1002/cne.902300406
141. Erkman L, Yates PA, McLaughlin T, McEvilly RJ, Whisenhunt T, O’Connell SM, et al. A POU domain transcription factor-dependent program regulates axon pathfinding in the vertebrate visual system. Neuron (2000) 28(3):779–92. doi: 10.1016/S0896-6273(00)00153-7
142. Fitzgibbon T, Reese BE. Organization of retinal ganglion cell axons in the optic fiber layer and nerve of fetal ferrets. Vis Neurosci (1996) 13(5):847–61. doi: 10.1017/S095252380000910X
143. Fitzgibbon T. The human fetal retinal nerve fiber layer and optic nerve head: a DiI and DiA tracing study. Vis Neurosci (1997) 14(3):433–47. doi: 10.1017/S0952523800012116
144. Plas DT, Lopez JE, Crair MC. Pretarget sorting of retinocollicular axons in the mouse. J Comp Neurol (2005) 491(4):305–19. doi: 10.1002/cne.20694
145. Murcia-Belmonte V, Coca Y, Vegar C, Negueruela S, de Juan Romero C, Valiño AJ, et al. A retino-retinal projection guided by Unc5c emerged in species with retinal waves. Curr Biol (2019) 29(7):1149–1160.e4. doi: 10.1016/j.cub.2019.02.052
146. Lu F, Kar D, Gruenig N, Zhang ZW, Cousins N, Rodgers HM, et al. Rax is a selector gene for mediobasal hypothalamic cell types. J Neurosci (2013) 33(1):259–72. doi: 10.1523/JNEUROSCI.0913-12.2013
147. Muranishi Y, Terada K, Furukawa T. An essential role for rax in retina and neuroendocrine system development. Dev Growth Differentiation (2012) 54:341–8. doi: 10.1111/j.1440-169X.2012.01337.x
148. Schulte D, Furukawa T, Peters MA, Kozak CA, Cepko CL. Misexpression of the emx-related homeobox genes cVax and mVax2 ventralizes the retina and perturbs the retinotectal map. Neuron (1999) 24(3):541–53. doi: 10.1016/S0896-6273(00)81111-3
149. Bassett EA, Williams T, Zacharias AL, Gage PJ, Fuhrmann S, West-Mays JA. AP-2α knockout mice exhibit optic cup patterning defects and failure of optic stalk morphogenesis. Hum Mol Genet (2010) 19(9):1791–804. doi: 10.1093/hmg/ddq060
150. Mason CA, Sretavan DW. Glia, neurons, and axon pathfinding during optic chiasm development. Curr Opin Neurobiol (1997) 7(5):647–53. doi: 10.1016/S0959-4388(97)80084-0
151. Deiner MS, Sretavan DW. Altered midline axon pathways and ectopic neurons in the developing hypothalamus of netrin-1- and DCC-deficient mice. J Neurosci (1999) 19(22):9900–12. doi: 10.1523/JNEUROSCI.19-22-09900.1999
152. Wang SW, Mu X, Bowers WJ, Kim DS, Plas DJ, Crair MC, et al. Brn3b/Brn3c double knockout mice reveal an unsuspected role for Brn3c in retinal ganglion cell axon outgrowth. Development (2002) 129(2):467–77. doi: 10.1242/dev.129.2.467
153. Otteson DC, Shelden E, Jones JM, Kameoka J, Hitchcock PF. Pax2 expression and retinal morphogenesis in the normal and krd mouse. Dev Biol (1998) 193(2):209–24. doi: 10.1006/dbio.1997.8794
154. Nornes HO, Dressler GR, Knapik EW, Deutsch U, Gruss P. Spatially and temporally restricted expression of Pax2 during murine neurogenesis. Development (1990) 109(4):797–809. doi: 10.1242/dev.109.4.797
155. Mao CA, Kiyama T, Pan P, Furuta Y, Hadjantonakis AK, Klein WH. Eomesodermin, a target gene of Pou4f2, is required for retinal ganglion cell and optic nerve development in the mouse. Development (2008) 135(2):271–80. doi: 10.1242/dev.009688
156. Marcus RC, Mason CA. The first retinal axon growth in the mouse optic chiasm: axon patterning and the cellular environment. J Neurosci (1995) 15(10):6389–402. doi: 10.1523/JNEUROSCI.15-10-06389.1995
157. Ogata-Iwao M, Inatani M, Iwao K, Takihara Y, Nakaishi-Fukuchi Y, Irie F, et al. Heparan sulfate regulates intraretinal axon pathfinding by retinal ganglion cells. Investig Ophthalmol Vis Sci (2011) 52(9):6671–9. doi: 10.1167/iovs.11-7559
158. Barbieri AM, Broccoli V, Bovolenta P, Alfano G, Marchitiello A, Mocchetti C, et al. Vax2 inactivation in mouse determines alteration of the eye dorsal-ventral axis, misrouting of the optic fibres and eye coloboma. Development (2002) 129(3):805–13. doi: 10.1242/dev.129.3.805
159. Erskine L, Williams SE, Brose K, Kidd T, Rachel RA, Goodman CS, et al. Erratum: retinal ganglion cell axon guidance in the mouse optic chiasm: expression and function of robos and slits. J Neurosci (2000) 20:4975–82. doi: 10.1523/JNEUROSCI.20-13-04975.2000
160. Godement P, Wang LC, Mason CA. Retinal axon divergence in the optic chiasm: dynamics of growth cone behavior at the midline. J Neurosci (1994) 14(11 II):7024–39. doi: 10.1523/JNEUROSCI.14-11-07024.1994
161. Birgbauer E, Cowan CA, Sretavan DW, Henkemeyer M. Kinase independent function of EphB receptors in retinal axon pathfinding to the optic disc from dorsal but not ventral retina. Development (2000) 127(6):1231–41. doi: 10.1242/dev.127.6.1231
162. Mu X, Zhao S, Pershad R, Hsieh TF, Scarpa A, Wang SW, et al. Gene expression in the developing mouse retina by EST sequencing and microarray analysis. Nucleic Acids Res (2001) 29(24):4983–93. doi: 10.1093/nar/29.24.4983
163. Mu X, Beremand PD, Zhao S, Pershad R, Sun H, Scarpa A, et al. Discrete gene sets depend on POU domain transcription factor Brn3b/Brn-3.2/POU4f2 for their expression in the mouse embryonic retina. Development (2004) 131(6):1197–210. doi: 10.1242/dev.01010
164. Tiwari S, Dharmarajan S, Shivanna M, Otteson DC, Belecky-Adams TL. Histone deacetylase expression patterns in developing murine optic nerve. BMC Dev Biol (2014) 14(1):1–18. doi: 10.1186/1471-213X-14-30
165. Buhusi M, Demyanenko GP, Jannie KM, Dalal J, Darnell EPB, Weiner JA, et al. ALCAM regulates mediolateral retinotopic mapping in the superior colliculus. J Neurosci (2009) 29(50):15630–41. doi: 10.1523/JNEUROSCI.2215-09.2009
166. Reese BE, Baker GE. The re-establishment of the representation of the dorsoventral retinal axis in the chiasmatic region of the ferret. Vis Neurosci (1993) 10(5):957–68. doi: 10.1017/S0952523800006179
167. Chan SO, Guillery RW. Changes in fiber order in the optic nerve and tract of rat embryos. J Comp Neurol (1994) 344(1):20–32. doi: 10.1002/cne.903440103
168. Sajgo S, Ghinia MG, Brooks M, Kretschmer F, Chuang K, Hiriyanna S, et al. Molecular codes for cell type specification in Brn3 retinal ganglion cells. Proc Natl Acad Sci USA (2017) 114(20):E3974–83. doi: 10.1073/pnas.1618551114
169. Badea TC, Cahill H, Ecker J, Hattar S, Nathans J. Distinct roles of transcription factors Brn3a and Brn3b in controlling the development, morphology, and function of retinal ganglion cells. Neuron (2009) 61(6):852–64. doi: 10.1016/j.neuron.2009.01.020
170. Dhande OS, Hua EW, Guh E, Yeh J, Bhatt S, Zhang Y, et al. Development of single retinofugal axon arbors in normal and β2 knock-out mice. J Neurosci (2011) 31(9):3384–99. doi: 10.1523/JNEUROSCI.4899-10.2011
171. Badea TC, Hua ZL, Smallwood PM, Williams J, Rotolo T, Ye X, et al. New mouse lines for the analysis of neuronal morphology using CreER(T)/loxP-directed sparse labeling. PloS One (2009) 4(11):e7859. doi: 10.1371/journal.pone.0007859
172. Kim IJ, Zhang Y, Meister M, Sanes JR. Laminar restriction of retinal ganglion cell dendrites and axons: subtype-specific developmental patterns revealed with transgenic markers. J Neurosci (2010) 30(4):1452–62. doi: 10.1523/JNEUROSCI.4779-09.2010
173. Brodie-Kommit J, Clark BS, Shi Q, Shiau F, Kim DW, Langel J, et al. Atoh7-independent specification of retinal ganglion cell identity. Sci Adv (2021) 7(11):1–19. doi: 10.1126/sciadv.abe4983
174. Wu F, J.Kaczynski T, Sethuramanujam S, Li R, Jain V, Slaughter M, et al. Two transcription factors, Pou4f2 and Isl1, are sufficient to specify the retinal ganglion cell fate. Proc Natl Acad Sci USA (2015) 112(13):E1559–68. doi: 10.1073/pnas.1421535112
175. Lyu J, Mu X. Genetic control of retinal ganglion cell genesis. Cell Mol Life Sci (2021) 78:4417–33. doi: 10.1007/s00018-021-03814-w
176. García-Frigola C, Carreres MI, Vegar C, Mason C, Herrera E. Zic2 promotes axonal divergence at the optic chiasm midline by EphB1-dependent and -independent mechanisms. Development (2008) 135(10):1833–41. doi: 10.1242/dev.020693
177. Lee R, Petros TJ, Mason CA. Zic2 regulates retinal ganglion cell axon avoidance of ephrinB2 through inducing expression of the guidance receptor EphB1. J Neurosci (2008) 28(23):5910–9. doi: 10.1523/JNEUROSCI.0632-08.2008
178. Herrera E, Brown L, Aruga J, Rachel RA, Dolen GL, Mikoshiba K, et al. Zic2 patterns binocular vision by specifying the uncrossed retinal projection tory are located in the temporal retina, but in less binocu-lar species, ganglion cells that project ipsilaterally reside in the ventrotemporal (VT) retina. Cell (2003) 114:545–57. doi: 10.1016/s0092-8674(03)00684-6
179. Triplett JW, Wei W, Gonzalez C, Sweeney NT, Huberman AD, Feller MB, et al. Dendritic and axonal targeting patterns of a genetically-specified class of retinal ganglion cells that participate in image-forming circuits. Neural Dev (2014) 9(1):1–13. doi: 10.1186/1749-8104-9-2
180. Kuwajima T, Soares CA, Sitko AA, Lefebvre V, Mason C. SoxC transcription factors promote contralateral retinal ganglion cell differentiation and axon guidance in the mouse visual system. Neuron (2017) 93(5):1110–1125.e5. doi: 10.1016/j.neuron.2017.01.029
181. Pak W, Hindges R, Lim YS, Pfaff SL, O’Leary DDM. Magnitude of binocular vision controlled by islet-2 repression of a genetic program that specifies laterality of retinal axon pathfinding. Cell (2004) 119(4):567–78. doi: 10.1016/j.cell.2004.10.026
182. Giudice QL, Leleu M, Manno GL, Fabre PJ. Single-cell transcriptional logic of cell-fate specification and axon guidance in early-born retinal neurons. Development (2019) 146(17):dev178103. doi: 10.1101/497081
183. Brooks MJ, Chen HY, Kelley RA, Mondal AK, Nagashima K, De Val N, et al. Improved retinal organoid differentiation by modulating signaling pathways revealed by comparative transcriptome analyses with development in vivo. Stem Cell Rep (2019) 13(5):891–905. doi: 10.1016/j.stemcr.2019.09.009
184. Clark BS, Stein-O’Brien GL, Shiau F, Cannon GH, Davis-Marcisak E, Sherman T, et al. Single-cell RNA-seq analysis of retinal development identifies NFI factors as regulating mitotic exit and late-born cell specification. Neuron (2019) 102(6):1111–1126.e5. doi: 10.1016/j.neuron.2019.04.010
185. Gao Z, Mao CA, Pan P, Mu X, Klein WH. Transcriptome of Atoh7 retinal progenitor cells identifies new Atoh7-dependent regulatory genes for retinal ganglion cell formation. Dev Neurobiol (2014) 74(11):1123–40. doi: 10.1002/dneu.22188
186. Mu X, Fu X, Sun H, Beremand PD, Thomas TL, Klein WH. A gene network downstream of transcription factor Math5 regulates retinal progenitor cell competence and ganglion cell fate. Dev Biol (2005) 280(2):467–81. doi: 10.1016/j.ydbio.2005.01.028
187. Boyer NP, Gupton SL. Revisiting netrin-1: one who guides (Axons). Front Cell Neurosci (2018) 12(July):1–18. doi: 10.3389/fncel.2018.00221
188. Rama N, Goldschneider D, Corset V, Lambert J, Pays L, Mehlen P. Amyloid precursor protein regulates netrin-1-mediated commissural axon outgrowth. J Biol Chem (2012) 287(35):30014–23. doi: 10.1074/jbc.M111.324780
189. Huber AB, Kolodkin AL, Ginty DD, Cloutier JF. Signaling at the growth cone: ligand-receptor complexes and the control of axon growth and guidance. Annu Rev Neurosci (2003) 26:509–63. doi: 10.1146/annurev.neuro.26.010302.081139
190. Dharmarajan S, Gurel Z, Wang S, Sorenson CM, Sheibani N, Belecky-Adams TL. Bone morphogenetic protein 7 regulates reactive gliosis in retinal astrocytes and müller glia. Mol Vis (2014) 20(December 2013):1085–108.
191. Qu C, Dwyer T, Shao Q, Yang T, Huang H, Liu G. Direct binding of TUBB3 with DCC couples netrin-1 signaling to intracellular microtubule dynamics in axon outgrowth and guidance. J Cell Sci (2013) 126(14):3070–81. doi: 10.1242/jcs.122184
192. Chatzopoulou E, Miguez A, Savvaki M, Levasseur G, Muzerelle A, Muriel MP, et al. Structural requirement of TAG-1 for retinal ganglion cell axons and myelin in the mouse optic nerve. J Neurosci (2008) 28(30):7624–36. doi: 10.1523/JNEUROSCI.1103-08.2008
193. Kruger K, Tam AS, Lu C, Sretavan DW. Retinal ganglion cell axon progression from the optic chiasm to initiate optic tract development requires cell autonomous function of GAP- 43. J Neurosci (1998) 18(15):5692–705. doi: 10.1523/JNEUROSCI.18-15-05692.1998
194. Guo C, Cho KS, Li Y, Tchedre K, Antolik C, Ma J, et al. IGFBPL1 regulates axon growth through IGF-1-mediated signaling cascades. Sci Rep (2018) 8(1):1–13. doi: 10.1038/s41598-018-20463-5
195. Rathjen FG, Wolff JM, Chang S, Bonhoeffer F, Raper JA. Neurofascin: a novel chick cell-surface glycoprotein involved in neurite-neurite interactions. Cell (1987) 51(5):841–9. doi: 10.1016/0092-8674(87)90107-3
196. Williams SE, Grumet M, Colman DR, Henkemeyer M, Mason CA, Sakurai T. A role for nr-CAM in the patterning of binocular visual pathways. Neuron (2006) 50(4):535–47. doi: 10.1016/j.neuron.2006.03.037
197. Erskine L, François U, Denti L, Joyce A, Tillo M, Bruce F, et al. VEGF-a and neuropilin 1 (NRP1) shape axon projections in the developing CNS via dual roles in neurons and blood vessels. Development (2017) 144(13):2504–16. doi: 10.1242/dev.151621
198. Dharmaratne N, Glendining KA, Young TR, Tran H, Sawatari A, Leamey CA. Ten-m3 is required for the development of topography in the ipsilateral retinocollicular pathway. PloS One (2012) 7(9):e43083. doi: 10.1371/journal.pone.0043083
199. Glendining KA, Liu SC, Nguyen M, Dharmaratne N, Nagarajah R, Iglesias MA, et al. Downstream mediators of ten-m3 signalling in the developing visual pathway. BMC Neurosci (2017) 18(1):1–17. doi: 10.1186/s12868-017-0397-5
200. Wang B, Li H, Mutlu SA, Bowser DA, Moore MJ, Wang MC, et al. The amyloid precursor protein is a conserved receptor for slit to mediate axon guidance. eNeuro (2017) 4(3):1–18. doi: 10.1523/ENEURO.0185-17.2017
201. Koleske AJ. Molecular mechanisms of dendrite stability. Nat Rev Neurosci (2013) 14(8):536–50. doi: 10.1038/nrn3486
202. Guijarro P, Wang Y, Ying Y, Yao Y, Jieyi X, Yuan X. In vivo knockdown of cKit impairs neuronal migration and axonal extension in the cerebral cortex. Inc Dev Neurobiol (2013) 73:871–87. doi: 10.1002/dneu.22107
203. Hayashita-Kinoh H, Kinoh H, Okada A, Komori K, Itoh Y, Chiba T, et al. Membrane-type 5 matrix metalloproteinase is expressed in differentiated neurons and regulates axonal growth. Cell Growth Differ (2001) 12(11):573–80.
204. Sharma TP, Liu Y, Wordinger RJ, Pang IH, Clark AF. Neuritin 1 promotes retinal ganglion cell survival and axonal regeneration following optic nerve crush. Cell Death Dis (2015) 6(2):e1661–13. doi: 10.1038/cddis.2015.22
205. Drerup CM, Lusk S, Nechiporuk A. Kif1B interacts with KBP to promote axon elongation by localizing a microtubule regulator to growth cones. J Neurosci (2016) 36(26):7014–26. doi: 10.1523/JNEUROSCI.0054-16.2016
206. Garvalov BK, Flynn KC, Neukirchen D, Meyn L, Teusch N, Wu X, et al. Cdc42 regulates cofilin during the establishment of neuronal polarity. J Neurosci (2007) 27(48):13117–29. doi: 10.1523/JNEUROSCI.3322-07.2007
207. Lindenmaier LB, Parmentier N, Guo C, Tissir F, Wright KM. Dystroglycan is a scaffold for extracellular axon guidance decisions. Elife (2019) 8:1–26. doi: 10.7554/eLife.42143
208. Zhou L, Bar I, Achouri Y, Campbell K, De Backer O, Hebert JM, et al. Early forebrain wiring: genetic dissection using conditional Celsr3 mutant mice. Sci (80-) (2008) 320(5878):946–9. doi: 10.1126/science.1155244
209. Katic J, Loers G, Kleene R, Karl N, Schmidt C, Buck F, et al. Interaction of the cell adhesion molecule CHL1 with vitronectin, integrins, and the plasminogen activator inhibitor-2 promotes CHL1-induced neurite outgrowth and neuronal migration. J Neurosci (2014) 34(44):14606–23. doi: 10.1523/JNEUROSCI.3280-13.2014
210. Perrone-Bizzozero NI, Tanner DC, Mounce J, Bolognani F. Increased expression of axogenesis-related genes and mossy fibre length in dentate granule cells from adult HuD overexpressor mice. ASN Neuro (2011) 3(5):259–70. doi: 10.1042/AN20110015
211. Kwiatkowski AV, Rubinson DA, Dent EW, Edward van Veen J, Leslie JD, Zhang J, et al. Ena/VASP is required for neuritogenesis in the developing cortex. Neuron (2007) 56(3):441–55. doi: 10.1016/j.neuron.2007.09.008
212. Mandai K, Reimert DV, Ginty DD. Linx mediates interaxonal interactions and formation of the internal capsule. Neuron (2014) 83(1):93–103. doi: 10.1016/j.neuron.2014.05.020
213. Hirst CS, Stamp LA, Bergner AJ, Hao MM, Tran MX, Morgan JM, et al. Kif1bp loss in mice leads to defects in the peripheral and central nervous system and perinatal death. Sci Rep (2017) 7(1):1–14. doi: 10.1038/s41598-017-16965-3
214. Peng J, Fabre PJ, Dolique T, Swikert SM, Kermasson L, Shimogori T, et al. Sonic hedgehog is a remotely produced cue that controls axon guidance trans-axonally at a midline choice point. Neuron (2018) 97(2):326–340.e4. doi: 10.1016/j.neuron.2017.12.028
215. Steinbach K, Schlosshauer B. Regulatory cell interactions between retinal ganglion cells and radial glia during axonal and dendritic outgrowth. Microsc Res Tech (2000) 48(1):12–24. doi: 10.1002/(SICI)1097-0029(20000101)48:1<12::AID-JEMT3>3.0.CO;2-O
216. Silver J, Robb RM. Studies on the development of the eye cup and optic nerve in normal mice and in mutants with congenital optic nerve aplasia. Dev Biol (1979) 68(1):175–90. doi: 10.1016/0012-1606(79)90252-5
217. Cwinn MA, Mazerolle C, McNeill B, Ringuette R, Thurig S, Hui CC, et al. Suppressor of fused is required to maintain the multipotency of neural progenitor cells in the retina. J Neurosci (2011) 31(13):5169–80. doi: 10.1523/JNEUROSCI.5495-10.2011
218. Fernández-Nogales M, López-Cascales MT, Murcia-Belmonte V, Escalante A, Fernández-Albert J, Muñoz-Viana R, et al. Multiomic analysis of neurons with divergent projection patterns identifies novel regulators of axon pathfinding. Adv Sci (2022) 2200615:e2200615. doi: 10.1002/advs.202200615
219. Nelson BR, Gumuscu B, Hartman BH, Reh TA. Notch activity is downregulated just prior to retinal ganglion cell differentiation. Dev Neurosci (2006) 28(1–2):128–41. doi: 10.1159/000090759
220. Esteve P, Sandonà SA, Cardozo M, Malapeira J, Ibañez C, Crespo I, et al. SFRPs act as negative modulators of ADAM10 to regulate retinal neurogenesis. Nat Neurosci (2011) 14(5):562–70. doi: 10.1038/nn.2794
221. Miltner AM, Torre AL. Retinal ganglion cell replacement: current status and challenges ahead. Dev Dyn (2019) 248:118–28. doi: 10.1002/dvdy
222. Georgi SA, Reh TA. Dicer is required for the transition from early to late progenitor state in the developing mouse retina. J Neurosci (2010) 30(11):4048–61. doi: 10.1523/JNEUROSCI.4982-09.2010
223. Carl M, Wittbrodt J. Graded interference with FGF signalling reveals its dorsoventral asymmetry at the mid-hindbrain boundary. Development (1999) 126(24):5659–67. doi: 10.1242/dev.126.24.5659
224. Masai I, Stemple DL, Okamoto H, Wilson SW. Midline signals regulate retinal neurogenesis in zebrafish. Neuron (2000) 27(2):251–63. doi: 10.1016/S0896-6273(00)00034-9
225. Luo T, Sakai Y, Wagner E, Dräger UC. Retinoids, eye development, and maturation of visual function. J Neurobiol (2006) 66(7):677–86. doi: 10.1002/neu.20239
226. Alsiö JM, Tarchini B, Cayouette M, Livesey FJ. Ikaros promotes early-born neuronal fates in the cerebral cortex. Proc Natl Acad Sci USA (2013) 110(8):E716–25. doi: 10.1073/pnas.1215707110
227. Neumann CJ, Nuesslein-Volhard C. Patterning of the zebrafish retina by a wave of sonic hedgehog activity. Science (2000) 289(5487):2137–9. doi: 10.1126/science.289.5487.2137
228. Hufnagel RB, Le TT, Riesenberg AL, Brown NL. Neurog2 controls the leading edge of neurogenesis in the mammalian retina. Dev Biol (2010) 340(2):490–503. doi: 10.1016/j.ydbio.2010.02.002
229. Brown NL, Patel S, Brzezinski J, Glaser T. Math5 is required for retinal ganglion cell and optic nerve formation. Development (2001) 128(13):2497–508. doi: 10.1242/dev.128.13.2497
230. Gan L, Wang SW, Huang Z, Klein WH. POU domain factor brn-3b is essential for retinal ganglion cell differentiation and survival but not for initial cell fate specification. Dev Biol (1999) 210(2):469–80. doi: 10.1006/dbio.1999.9280
231. Mu X, Fu X, Beremand PD, Thomas TL, Klein WH. Gene-regulation logic in retinal ganglion cell development: Isl1 defines a critical branch distinct from but overlapping with Pou4f2. Proc Natl Acad Sci USA (2008) 105(19):6942–7. doi: 10.1073/pnas.0802627105
232. Mao CA, Wang SW, Pan P, Klein WH. Rewiring the retinal ganglion cell gene regulatory network: Neurod1 promotes retinal ganglion cell fate in the absence of Math5. Development (2008) 135(20):3379–88. doi: 10.1242/dev.024612
233. de Melo J, Du G, Fonseca M, Gillespie LA, Turk WJ, Rubenstein JLR, et al. Dlx1 and Dlx2 function is necessary for terminal differentiation and survival of late-born retinal ganglion cells in the developing mouse retina. Development (2005) 132(2):311–22. doi: 10.1242/dev.01560
234. Lefcort F, Bentley D. Organization of cytoskeletal elements and organelles preceding growth cone emergence from an identified neuron in situ. J Cell Biol (1989) 108(5):1737–49. doi: 10.1083/jcb.108.5.1737
235. McFarlane S, Cornel E, Amaya E, Holt CE. Inhibition of FGF receptor activity in retinal ganglion cell axons causes errors in target recognition. Neuron (1996) 17(2):245–54. doi: 10.1016/S0896-6273(00)80156-7
236. Karali M, Persico M, Mutarelli M, Carissimo A, Pizzo M, Singh Marwah V, et al. High-resolution analysis of the human retina miRNome reveals isomiR variations and novel microRNAs. Nucleic Acids Res (2016) 44(4):1525–40. doi: 10.1093/nar/gkw039
237. Wong GKW, Baudet ML, Norden C, Leung L, Harris WA. Slit1b-Robo3 signaling and n-cadherin regulate apical process retraction in developing retinal ganglion cells. J Neurosci (2012) 32(1):223–8. doi: 10.1523/JNEUROSCI.2596-11.2012
238. Missaire M, Hindges R. The role of cell adhesion molecules in visual circuit formation: from neurite outgrowth to maps and synaptic specificity. Dev Neurobiol (2015) 75(6):569–83. doi: 10.1002/dneu.22267
239. Votin V, Nelson WJ, Barth AIM. Neurite outgrowth involves adenomatous polyposis coli protein and β-catenin. J Cell Sci (2005) 118(24):5699–708. doi: 10.1242/jcs.02679
240. Zhou FQ, Cohan CS. How actin filaments and microtubules steer growth cones to their targets. J Neurobiol (2004) 58(1):84–91. doi: 10.1002/neu.10278
241. Pollerberg GE, Thelen K, Theiss MO, Hochlehnert BC. The role of cell adhesion molecules for navigating axons: density matters. Mech Dev (2013) 130:359–72. doi: 10.1016/j.mod.2012.11.002
242. Brittis PA, Silver J, Walsh FS, Doherty P. Fibroblast growth factor receptor function is required for the orderly projection of ganglion cell axons in the developing mammalian retina. Mol Cell Neurosci (1996) 8(2–3):120–8. doi: 10.1006/mcne.1996.0051
243. Brittis PA, Silver J. Multiple factors govern intraretinal axon guidance: a time-lapse study. Mol Cell Neurosci (1995) 6(5):413–32. doi: 10.1006/mcne.1995.1031
244. Viollet C, Doherty P. CAMs and the FGF receptor: an interacting role in axonal growth. Cell Tissue Res (1997) 290(2):451–5. doi: 10.1007/s004410050952
245. Noren NK, Liu BP, Burridge K, Kreft B. p120 catenin regulates the actin cytoskeleton via rho family GTPases. J Cell Biol (2000) 150(3):567–79. doi: 10.1083/jcb.150.3.567
246. Shima Y, Kawaguchi SY, Kosaka K, Nakayama M, Hoshino M, Nabeshima Y, et al. Opposing roles in neurite growth control by two seven-pass transmembrane cadherins. Nat Neurosci (2007) 10(8):963–9. doi: 10.1038/nn1933
247. Leung K, Van HFPG, AC L, Allison R, Holt CE. Asymmetrical β -actin mRNA translation in growth cones mediates attractive turning to netrin-1. Nat Neurosci (2007) 9(10):1247–56. doi: 10.1038/nn1775
248. Deiner MS, Kennedy TE, Fazeli A, Serafini T, Tessier-Lavigne M, Sretavan DW. Netrin-1 and DCC mediate axon guidance locally at the optic disc: loss of function leads to optic nerve hypoplasia. Neuron (1997) 19(3):575–89. doi: 10.1016/S0896-6273(00)80373-6
249. Bourikas D, Pekarik V, Baeriswyl T, Grunditz Å, Sadhu R, Nardó M, et al. Sonic hedgehod guides commissural axons along the longitudinal axis of the spinal cord. Nat Neurosci (2005) 8(3):297–304. doi: 10.1038/nn1396
250. Thompson H, Barker D, Camand O, Erskine L. Slits contribute to the guidance of retinal ganglion cell axons in the mammalian optic tract. Dev Biol (2006) 296(2):476–84. doi: 10.1016/j.ydbio.2006.06.017
251. Snow DM, Watanabe M, Letourneau PC, Silver J. A chondroitin sulfate proteoglycan may influence the direction of retinal ganglion cell outgrowth. Development (1991) 113(4):1473–85. doi: 10.1242/dev.113.4.1473
252. Ledig MM, Haj F, Bixby JL, Stoker AW, Mueller BK. The receptor tyrosine phosphatase CRYPα promotes intraretinal axon growth. J Cell Biol (1999) 147(2):375–88. doi: 10.1083/jcb.147.2.375
253. Bauch H, Stier H, Schlosshauer B. Axonal versus dendritic outgrowth is differentially affected by radial glia in discrete layers of the retina. J Neurosci (1998) 18(5):1774–85. doi: 10.1523/JNEUROSCI.18-05-01774.1998
254. Nonet ML. AEXpulsing a retrograde signal. Neuron (2002) 33:155–6. doi: 10.1016/S0896-6273(02)00562-7
255. Ringstedt T, Braisted JE, Brose K, Kidd T, Goodman C, Tessier-Lavigne M, et al. Slit inhibition of retinal axon growth and its role in retinal axon pathfinding and innervation patterns in the diencephalon. J Neurosci (2000) 20(13):4983–91. doi: 10.1523/JNEUROSCI.20-13-04983.2000
256. Niclou SP, Jia L, Raper JA. Slit2 is a repellent for retinal ganglion cell axons. J Neurosci (2000) 20(13):4962–74. doi: 10.1523/JNEUROSCI.20-13-04962.2000
257. Marcos S, Nieto-Lopez F, Sandonìs A, Cardozo MJ, Di Marco F, Esteve P, et al. Secreted frizzled related proteins modulate pathfinding and fasciculation of mouse retina ganglion cell axons by direct and indirect mechanisms. J Neurosci (2015) 35(11):4729–40. doi: 10.1523/JNEUROSCI.3304-13.2015
258. Ott H, Bastmeyer M, Stuermer CAO. Neurolin, the goldfish homolog of DM-GRASP, is involved in retinal axon pathfinding to the optic disk. J Neurosci (1998) 18(9):3363–72. doi: 10.1523/JNEUROSCI.18-09-03363.1998
259. Thelen K, Kedar V, Panicker AK, Schmid RS, Midkiff BR, Maness PF. The neural cell adhesion molecule L1 potentiates integrin-dependent cell migration to extracellular matrix proteins. J Neurosci (2002) 22(12):4918–31. doi: 10.1523/JNEUROSCI.22-12-04918.2002
260. Jin Z, Zhang J, Klar A, Chédotal A, Rao Y, Cepko CL, et al. Irx4-mediated regulation of Slit1 expression contributes to the definition of early axonal paths inside the retina. Development (2003) 130(6):1037–48. doi: 10.1242/dev.00326
261. Birgbauer E, Oster SF, Severin CG, Sretavan DW. Retinal axon growth cones respond to EphB extracellular domains as inhibitory axon guidance cues. Development (2001) 128(15):3041–8. doi: 10.1242/dev.128.15.3041
262. Höpker VH, Shewan D, Tessier-Lavigne M, Poo MM, Holt C. Growth-cone attraction to netrin-1 is converted to repulsion by laminin-1. Nature (1999) 401(6748):69–73. doi: 10.1038/43441
263. Wyatt AW, Osborne RJ, Stewart H, Ragge NK. Bone morphogenetic protein 7 (BMP7) mutations are associated with variable ocular, brain, ear, palate, and skeletal anomalies. Hum Mutat (2010) 31(7):781–7. doi: 10.1002/humu.21280
264. Drager UC, Edwards DL, Barnstable CJ. Antibodies against filamentous components in discrete cell types of the mouse retina. J Neurosci (1984) 4(8):2025–42. doi: 10.1523/JNEUROSCI.04-08-02025.1984
265. Reese BE, Geller SF. Precocious invasion of the optic stalk by transient retinopetal axons. J Comp Neurol (1995) 353(4):572–84. doi: 10.1002/cne.903530408
266. Frade JM, Barde YA. Genetic evidence for cell death mediated by nerve growth factor and the neurotrophin receptor p75 in the developing mouse retina and spinal cord. Development (1999) 126(4):683–90. doi: 10.1242/dev.126.4.683
267. Wöhrn JC, Puelles L, Nakagawa S, Takeichi M, Redies C. Cadherin expression in the retina and retinofugal pathways of the chicken embryo. J Comp Neurol (1998) 396(1):20–38. doi: 10.1002/(SICI)1096-9861(19980622)396:1<20::AID-CNE3>3.0.CO;2-K
268. Petros TJ, Williams SE, Mason CA. Temporal regulation of EphA4 in astroglia during murine retinal and optic nerve development. Mol Cell Neurosci (2006) 32(1–2):49–66. doi: 10.1016/j.mcn.2006.02.002
269. Purohit AA, Li W, Qu C, Dwyer T, Shao Q, Guan KL, et al. Down syndrome cell adhesion molecule (DSCAM) associates with uncoordinated-5C (UNC5C) in netrin-1-mediated growth cone collapse. J Biol Chem (2012) 287(32):27126–38. doi: 10.1074/jbc.M112.340174
270. Reese BE, Maynard TM, Hocking DR. Glial domains and axonal reordering in the chiasmatic region of the developing ferret. J Comp Neurol (1994) 349(2):303–24. doi: 10.1002/cne.903490211
271. Nadal-Nicolás FM, Valiente-Soriano FJ, Salinas-Navarro M, Jiménez-López M, Vidal-Sanz M, Agudo-Barriuso M. Retino-retinal projection in juvenile and young adult rats and mice. Exp Eye Res (2015) 134:47–52. doi: 10.1016/j.exer.2015.03.015
272. Adams RH, Betz H, Püschel AW. A novel class of murine semaphorins with homology to thrombospondin is differentially expressed during early embryogenesis. Mech Dev (1996) 57(1):33–45. doi: 10.1016/0925-4773(96)00525-4
273. Oster SF, Bodeker MO, He F, Sretavan DW. Invariant Sema5A inhibition serves an ensheathing function during optic nerve development. Development (2003) 130:775–84. doi: 10.1242/dev.00299
274. Gordon HB, Lusk S, Carney KR, Wirick EO, Murray BF, Kwan KM. Hedgehog signaling regulates cell motility and optic fissure and stalk formation during vertebrate eye morphogenesis. Development (2018) 145(22):dev165068. doi: 10.1242/dev.165068
275. Todd L, Hooper MJ, Haugan AK, Finkbeiner C, Jorstad N, Radulovich N, et al. Efficient stimulation of retinal regeneration from müller glia in adult mice using combinations of proneural bHLH transcription factors. Cell Rep (2021) 37(3):109857. doi: 10.1016/j.celrep.2021.109857
276. Todd L, Jenkins W, Finkbeiner C, Hooper MJ, Donaldson PC, Pavlou M, et al. Reprogramming müller glia to regenerate ganglion-like cells in adult mouse retina with developmental transcription factors. Sci Adv (2022) 8(47):eabq7219. doi: 10.1126/sciadv.abq7219
277. Kawada J, Kaneda S, Kirihara T, Maroof A, Levi T, Eggan K, et al. Generation of a motor nerve organoid with human stem cell-derived neurons. Stem Cell Rep (2017) 9(5):1441–9. doi: 10.1016/j.stemcr.2017.09.021
Keywords: optic nerve, retinal ganglion cells, transcription, development, optic stalk
Citation: Paşcalău R and Badea TC (2023) Signaling – transcription interactions in mouse retinal ganglion cells early axon pathfinding –a literature review. Front. Ophthalmol. 3:1180142. doi: 10.3389/fopht.2023.1180142
Received: 05 March 2023; Accepted: 21 April 2023;
Published: 17 May 2023.
Edited by:
David W. Marshak, University of Texas Health Science Center, United StatesReviewed by:
Benjamin E. Reese, University of California, Santa Barbara, United StatesJoel Miesfeld, Medical College of Wisconsin, United States
Copyright © 2023 Paşcalău and Badea. This is an open-access article distributed under the terms of the Creative Commons Attribution License (CC BY). The use, distribution or reproduction in other forums is permitted, provided the original author(s) and the copyright owner(s) are credited and that the original publication in this journal is cited, in accordance with accepted academic practice. No use, distribution or reproduction is permitted which does not comply with these terms.
*Correspondence: Tudor Constantin Badea, YmFkZWF0Y0B1bml0YnYucm8=; Raluca Paşcalău, cmFsdWNhLnBhc2NhbGF1QHVuaXRidi5ybw==