- F.M. Kirby Neurobiology Center and Department of Neurology, Boston Children’s Hospital and Harvard Medical School, Boston, MA, United States
Melanopsin is a light-activated G protein coupled receptor that is expressed widely across phylogeny. In mammals, melanopsin is found in intrinsically photosensitive retinal ganglion cells (ipRGCs), which are especially important for “non-image” visual functions that include the regulation of circadian rhythms, sleep, and mood. Photochemical and electrophysiological experiments have provided evidence that melanopsin has at least two stable conformations and is thus multistable, unlike the monostable photopigments of the classic rod and cone photoreceptors. Estimates of melanopsin’s properties vary, challenging efforts to understand how the molecule influences vision. This article seeks to reconcile disparate views of melanopsin and offer a practical guide to melanopsin’s complexities.
Introduction
Organisms produce electrical responses to light in part by expressing photopigments, molecules that absorb photons, change conformation, and signal downstream. Over a thousand photopigments are known (1). Among them is melanopsin (2, 3). This molecule has features that are, presently, both unique and controversial. A unified understanding of these features is desirable because melanopsin exerts vital influences (4–7). For example, melanopsin helps synchronize the circadian clock to the solar day, thereby setting normal patterns of physiology and gene expression in practically all tissues of the body (8). Circadian dysregulation is implicated in disorders that range from mental illness to cancer (9–11). Melanopsin also plays roles in other species, such as fishes, frogs, lancelets, and reef corals (12). This review provides a practical synthesis of knowledge concerning the melanopsin molecules of mammals.
The spectral sensitivity of melanopsin
A cardinal feature of a photopigment is its spectral sensitivity. Examining the literature, one has difficulty settling on the spectral sensitivity of mammalian melanopsin. Most estimates indicate that melanopsin is most sensitive to a wavelength near 480 nm (13–19). This wavelength of maximum sensitivity is referred to as the λmax. A single λmax suggests that the molecule activates from a single state (or from multiple states that have the same spectral sensitivity). However, photochemical and electrophysiological measurements indicate that mouse melanopsin activates from two spectrally distinct states (Figures 1A, B) (20, 21). The ground state, melanopsin, abbreviated “R” for historical reasons, has a λmax of ~470 nm. The second state, extramelanopsin (E), has a λmax of ~450 nm. These values also apply to the melanopsin of macaque monkeys (22) (Figure 1B). Puzzlingly, none of these λmax values are near 480 nm. Consideration of two factors offers a potential reconciliation.
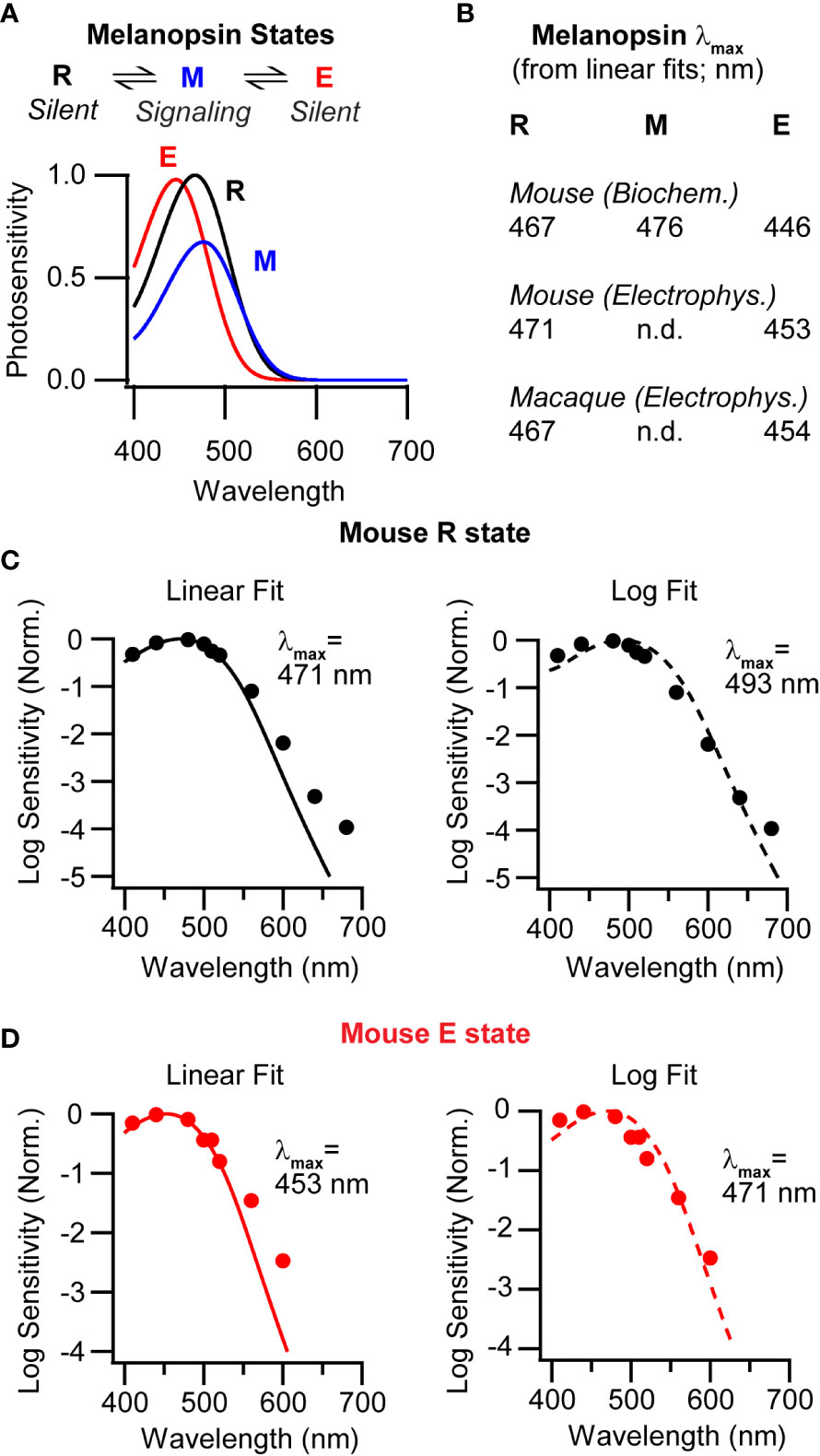
Figure 1 Melanopsin multistability in mice and macaques. (A) Top, Melanopsin states in the mouse. Melanopsin (R, the ground state, electrically silent) photoconverts with metamelanopsin (M, the signaling state), which photoconverts with extramelanopsin (E, electrically silent). Bottom, Photosensitivities of the three mouse melanopsin states (product of the extinction coefficient and quantum efficiency of isomerization) as a function of wavelength (20), normalized to that of the R state. (B) Wavelengths of peak sensitivity (λmax) for mouse melanopsin estimated by biochemistry and electrophysiology, and for macaque melanopsin estimated by electrophysiology (20–22). λmax values were obtained by fitting nomograms on linear ordinates. (C) Left, Average of action spectra measured from mouse ipRGCs in darkness (markers) Data were previously published (21). The line is a single-state nomogram fit using a least-squares algorithm on a linear scale. Fit λmax = 471 nm. Right, As on the left but the fit was made on a semi-log scale. Fit λmax = 493 nm. (D) Left, Average of action spectra measured from ipRGCs during background illumination with 600-nm light to enrich for the E state (markers). The line is a single-state nomogram fit using a least-squares algorithm on a linear scale. Fit λmax = 453 nm. Right, As on the left, but the fit was made on a semi-log scale. Fit λmax = 471 nm.
First, the λmax of a photopigment is often determined by fitting a discrete data set with a continuous function and taking the peak of that fit (23–25). These functions, often referred to as nomograms, are empirical and remarkably accurate (25). Given the λmax of a pigment as the only free parameter, a nomogram describes the spectral sensitivity well. However, ambiguity arises in how the fit is performed. If sensitivity is plotted on a linear scale, the fit tends to weigh the peak sensitivity more heavily. If the response is plotted on a log scale, the fit tends to weigh the long-wavelength decline of sensitivity more heavily. The aforementioned photochemical and electrophysiological estimates of melanopsin’s spectral sensitivity (λmax values of ~470 and ~450 nm) were made with fits on a linear scale. If one fits the electrophysiological data on a log scale, the λmax values are red-shifted to ~490 nm for the R state and ~470 nm for the E state (Figures 1C, D). This ~20-nm disparity between linear and log fits is substantial. Which λmax values to choose?
One might select according to context. A log fit might be undesirable because the long-wavelength decline of spectral sensitivity is labile. When wavelengths are longer than a certain value (λcritical, where λmax = 0.84 λcritical), sensitivity has a more positive slope at higher temperature (26). On a log scale, fitting the R state’s action spectrum (21) using all data points or only those below λcritical yields λmax values of 493 and 476 nm, respectively. For the E state, these values are 471 and 463 nm. Repeating the exercise on a linear scale yields no difference for either state. On the other hand, a log fit might be desirable because the long-wavelength decline encompasses a broad range of tested sensitivities, unlike the peak. This trade-off likely explains why measurements vary across studies that have different emphases. Practical advice is to use the λmax of the linear fit when short wavelengths are more relevant, and that of the log fit when long wavelengths are. Fits may also be made to portions of the data according to need (25).
The second consideration is that melanopsin equilibrates among its three known states during illumination (20, 21). Under common lighting conditions, the population of melanopsin molecules activates about equally from the R and E states (21). Taking the λmax values of these states as 450 and 470 nm (from fits on a linear ordinate), one obtains an effective λmax of ~460 nm (Figure 2A). Using log ordinate fits instead, where λmax values are 470 and 490 nm, the effective λmax is ~480 nm. Most studies of melanopsin’s spectral sensitivity use stimuli that are sufficiently long and intense that they are likely to produce an equilibrium of states. Thus, the commonly observed λmax of 480 nm can be explained by melanopsin’s multistable nature and the popularity of fitting on a log ordinate.
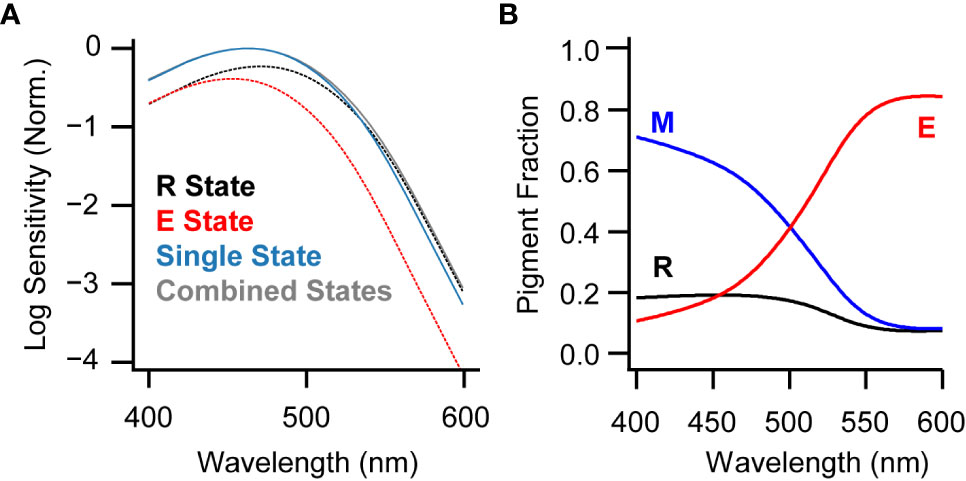
Figure 2 Melanopsin states under broadband and narrowband illumination. (A) Fits made to the action spectrum measured from ipRGCs on a background of broadband white light (21) using either a single-state nomogram (blue; λmax = 463 nm when fit on linear scale) or the combination of the R and E spectra (gray; λmax = 463 nm). Black and red dotted lines represent the R and E state nomograms, respectively, scaled to their contributions to the combined fit and using λmax values measured electrophysiologically (471 and 453 nm, respectively). The λmax of the combined nomogram, when fit on a log scale, is 480 nm. (B) Melanopsin states at photoequilibrium for monochromatic illumination with wavelengths spanning the visible range, predicted by a model based on biochemical parameters of mouse melanopsin (21).
Melanopsin’s activation from two spectrally distinct states causes the population of molecules to have a broader spectral sensitivity than a single state (21). This broadening may be considered ample, as the difference in λmax values between the R and E states is roughly comparable to that between the long- and medium-wavelength sensitive cone pigments that give rise to the red-green color axis in humans (27). Indeed, a broadened spectral sensitivity is consistent with the role of melanopsin in sensing the overall light intensity and not necessarily specific wavelengths (but see 28). On the other hand, this broadening may be considered slight. Consider the predicted activation of melanopsin in sunlight (CIE spectrum G174), comparing the realistic case of a combined-state spectral sensitivity and the hypothetical case of a single-state spectral sensitivity, each having a λmax value of 480 nm. The combined-state, broader spectrum absorbs ~3% more than the single-state, narrower spectrum. It would appear that, at least under sunlight, the spectral broadening caused by melanopsin multistability is subtle. Nevertheless, in cases that require precision, it is not much trouble to use the combined-state spectrum rather than its single-state approximation.
To conclude this section, it may be sufficient in most contexts to approximate melanopsin’s spectral sensitivity with a standard Govardovskii nomogram that has a λmax of 480 nm (on a log scale) or 460 nm (on a linear scale). Accommodating additional complexity increases accuracy.
Specific features of melanopsin multistability
At this point, it appears that melanopsin can be considered in relatively simple terms. However, there are cases where specific features of melanopsin multistability are especially salient. This section will highlight five.
Persistent activity
Melanopsin’s signaling state, metamelanopsin (M), is subject to termination mechanisms that include phosphorylation, arrestin binding, bleaching, and internalization (29–39). Nevertheless, some melanopsin signaling endures and drives persistent cellular activation (21, 22, 40, 41). This activity is consistent with the melanopsin system’s encoding of environmental irradiance. Prolonged activation tends to blur spatial and temporal details in the scene, emphasizing the overall light intensity (21, 22). The overall light intensity is information that is used, for instance, by the circadian clock for synchronization to the day/night cycle (42). Persistent melanopsin activity is also thought to drive the post-illumination pupil response (PIPR), which has been used to diagnose the melanopsin system in contexts ranging from seasonal affective disorder to Alzheimer’s disease (41).
Photoswitching
As mentioned above, photon absorptions interconvert melanopsin among its states. Sunlight and most common sources of artificial white light produce a photoequilibrium in which roughly half the melanopsin molecules are in M state and a quarter each are in the R and E states. Photoswitching maintains a pool of melanopsin molecules for activation, as those that are driven from the M state are available for reactivation (21, 22, 43).
The photoequilibrium fractions of melanopsin can be manipulated using narrow-band light (Figure 2B) (20, 21). The M state, having the longest λmax, dominates under short wavelengths and is minimal under long wavelengths. The E state, having the shortest λmax, follows the opposite pattern (while the R state has an intermediate λmax and only a small fraction is present after exposure to any wavelength). This trade between M and E states is reflected in the magnitude of persistent activity in ipRGCs. Short wavelengths produce the largest persistent activity and long wavelengths the smallest (21, 22). Thus, ipRGC activation can be switched high and low with acute illumination with short and long wavelengths. Photoswitching of persistent activity has been demonstrated for mouse and macaque ipRGCs (21, 22), as well as for cell lines expressing human melanopsin (40).
Practically speaking, deactivation of ipRGCs requires intense and prolonged illumination because all melanopsin states absorb long wavelengths poorly. The optimal wavelength for deactivation is near 560 nm and reflects a balance between being long enough for preferential absorption by the M state but not so long that it is scarcely absorbed at all (21). At 560 nm, deactivation can be produced by delivering ~109 photons µm-2 sec-1 for 30 s or more (21, 22). This kind of light is probably not found in nature, though artificial sources are available.
Bleaching and regeneration
Bleaching is the process by which an activated photopigment dissociates into opsin and chromophore (44). Neither opsin nor chromophore absorb well in the visible spectrum so this process causes the appearance of an actual bleach to the human eye. For example, a solution of rhodopsin—a molecule once called visual purple—loses its color in light. Bleaching and multistability are not mutually exclusive. Thus, though active melanopsin is stable and can be photoconverted to a silent state, it can also release its chromophore (36, 37, 39, 45). Indeed, applying exogenous chromophore to ipRGCs increases their sensitivity, though it is unclear if this effect is due to natural levels of bare opsin or bleaching during the course of the experiment (36, 45, 46).
The only chromophore found in dark-adapted ipRGCs is 11-cis retinal (47), which defines melanopsin’s R state (20). Curiously, there appears to be no kind of illumination that produces only the R state in ipRGCs. As mentioned above, this state seems sparse during illumination with any spectrum (21). Therefore, a light-independent pathway should recover melanopsin to the R state. Evidence exists for chromophore supply from the retinal pigment epithelium to ipRGCs via Müller glia cells (48) even though questions remain (49). Perhaps the process of dark regeneration involves melanopsin bleaching and 11-cis retinal resupply.
Adaptation
Melanopsin activity drives adaptive processes that tamp down on melanopsin activity (29–39, 50–52). Consequently, melanopsin deactivation may reverse adaptation to produce sensitization. Sensitization of this kind has been suggested by in vivo experiments (53). Anecdotal evidence can be found in ex vivo experiments as well (21). Melanopsin phototransduction also drives light adaptation at the level of the ipRGC population (54). The interplay of activation and adaptation in the melanopsin system merits further study.
Species variation
At least two stable, silent states of melanopsin have been observed in mice and macaques (21, 22). In humans and the lancelet, amphioxus, only one silent state has been reported (55, 56). Thus, the evolutionary conservation of melanopsin multistability may be incomplete. Also, across species, melanopsins vary in their bleaching rates (37). This variation may influence the lifetimes of persistent responses across species. Indeed, mouse melanopsin (reluctant to bleach) and human melanopsin (willing to bleach) have relatively long and brief persistent responses, respectively (37, 40). Another layer of intricacy is that persistent response lifetime may be modulated, melanopsin can be alternatively spliced, melanopsins are functionally diverse, and melanopsins show sufficient molecular distinctions to be grouped into two gene families (Opn4m and Opn4x) (57–63). Further study may reveal additional diversity in melanopsins across species.
Closing remarks
Melanopsin’s discovery indicated that the mammalian retina is not duplex—relying on rods and cones to sense light—but multiplex. The multiplicity of melanopsin states adds richness to this picture. This review intends to provide a concise summary of melanopsin’s complexities and a practical guide on how to navigate them.
Author contributions
Writing and figures: MTHD. Data, analyses, figures, and critiques: AJE. All authors contributed to the article and approved the submitted version.
Funding
National Institutes of Health (R01EY023648, R01EY034089, R01EY025555, and R21EY032731 to MTHD; P50HD105351 to Boston Children’s Hospital IDDRC; P30EY012196 to Harvard Medical School). The National Science Foundation (GRFP to AJE). The Harvard Brain Science Initiative Bipolar Seed Disorder Grant Program, Broderick Phytocannabinoid Research Initiative Grant Program, and The Molloy Family Innovation in Research grant (to MTHD).
Acknowledgments
Annette Allen and Richard McDowell provided thoughtful discussions. This piece is dedicated to Stephen Massey’s contributions of knowledge, technique, beauty, and spirit to vision research.
Conflict of interest
The authors declare that the research was conducted in the absence of any commercial or financial relationships that could be construed as a potential conflict of interest.
Publisher’s note
All claims expressed in this article are solely those of the authors and do not necessarily represent those of their affiliated organizations, or those of the publisher, the editors and the reviewers. Any product that may be evaluated in this article, or claim that may be made by its manufacturer, is not guaranteed or endorsed by the publisher.
References
1. Shichida Y, Matsuyama T. Evolution of opsins and phototransduction. Philos Trans R Soc Lond B Biol Sci (2009) 364:2881–95. doi: 10.1098/rstb.2009.0051
2. Provencio I, Jiang G, De Grip WJ, Hayes WP, Rollag MD. Melanopsin: an opsin in melanophores, brain, and eye. Proc Natl Acad Sci (1998) 95:340–5. doi: 10.1073/pnas.95.1.340
3. Provencio I, Rodriguez IR, Jiang G, Hayes WP, Moreira EF, Rollag MD. A novel human opsin in the inner retina. J Neurosci (2000) 20:600–5. doi: 10.1523/JNEUROSCI.20-02-00600.2000
4. Do MTH. Melanopsin and the intrinsically photosensitive retinal ganglion cells: biophysics to behavior. Neuron (2019) 104:205–26. doi: 10.1016/j.neuron.2019.07.016
5. Lazzerini Ospri L, Prusky G, Hattar S. Mood, the circadian system, and melanopsin retinal ganglion cells. Annu Rev Neurosci (2017) 40:539–56. doi: 10.1146/annurev-neuro-072116-031324
6. Lucas RJ, Peirson SN, Berson DM, Brown TM, Cooper HM, Czeisler CA, et al. Measuring and using light in the melanopsin age. Trends Neurosci (2014) 37:1–9. doi: 10.1016/j.tins.2013.10.004
7. Aranda ML, Schmidt TM. Diversity of intrinsically photosensitive retinal ganglion cells: circuits and functions. Cell Mol Life Sci (2021) 78:889–907. doi: 10.1007/s00018-020-03641-5
8. Mohawk JA, Green CB, Takahashi JS. Central and peripheral circadian clocks in mammals. Annu Rev Neurosci (2012) 35:445–62. doi: 10.1146/annurev-neuro-060909-153128
9. Rijo-Ferreira F, Takahashi JS. Genomics of circadian rhythms in health and disease. Genome Med (2019) 11:82. doi: 10.1186/s13073-019-0704-0
10. Green CB, Takahashi JS, Bass J. The meter of metabolism. Cell (2008) 134:728–42. doi: 10.1016/j.cell.2008.08.022
11. Sahar S, Sassone-Corsi P. Metabolism and cancer: the circadian clock connection. Nat Rev Cancer (2009) 9:886–96. doi: 10.1038/nrc2747
12. Do MTH, Yau K-W. Intrinsically photosensitive retinal ganglion cells. Physiol Rev (2010) 90:1547–81. doi: 10.1152/physrev.00013.2010
13. Lucas RJ, Douglas RH, Foster RG. Characterization of an ocular photopigment capable of driving pupillary constriction in mice. Nat Neurosci (2001) 4:621–6. doi: 10.1038/88443
14. Xue T, Do MTH, Riccio A, Jiang Z, Hsieh J, Wang HC, et al. Melanopsin signalling in mammalian iris and retina. Nature (2011) 479:67–73. doi: 10.1038/nature10567
15. Berson DM, Dunn FA, Takao M. Phototransduction by retinal ganglion cells that set the circadian clock. Science (2002) 295:1070–3. doi: 10.1126/science.1067262
16. Dacey DM, Liao HW, Peterson BB, Robinson FR, Smith VC, Pokorny J, et al. Melanopsin-expressing ganglion cells in primate retina signal colour and irradiance and project to the LGN. Nature (2005) 433:749–54. doi: 10.1038/nature03387
17. Hattar S, Lucas RJ, Mrosovsky N, Thompson S, Douglas RH, Hankins MW, et al. Melanopsin and rod-cone photoreceptive systems account for all major accessory visual functions in mice. Nature (2003) 424:76–81. doi: 10.1038/nature01761
18. Enezi J, Revell V, Brown T, Wynne J, Schlangen L, Lucas R. A “melanopic” spectral efficiency function predicts the sensitivity of melanopsin photoreceptors to polychromatic lights. J Biol Rhythms (2011) 26:314–23. doi: 10.1177/0748730411409719
19. Bailes HJ, Lucas RJ. Human melanopsin forms a pigment maximally sensitive to blue light (lambdamax approximately 479 nm) supporting activation of G(q/11) and g(i/o) signalling cascades. Proc Biol Sci (2013) 280:20122987. doi: 10.1098/rspb.2012.2987
20. Matsuyama T, Yamashita T, Imamoto Y, Shichida Y. Photochemical properties of mammalian melanopsin. Biochemistry (2012) 51:5454–62. doi: 10.1021/bi3004999
21. Emanuel AJ, Do MTH. Melanopsin tristability for sustained and broadband phototransduction. Neuron (2015) 85:1043–55. doi: 10.1016/j.neuron.2015.02.011
22. Liu A, Milner ES, Peng YR, Blume HA, Brown MC, Bryman GS, et al. Encoding of environmental illumination by primate melanopsin neurons. Science (2023) 379:376–81. doi: 10.1126/science.ade2024
23. Dartnall HJA. Photosensitivity. : H.J.A. Dartnall (Ed.) Photochem Vision Springer-Verlag New York (1972) pp:122–45. doi: 10.1007/978-3-642-65066-6_4
24. Lamb TD. Photoreceptor spectral sensitivities: common shape in the long-wavelength region. Vision Res (1995) 35:3083–91. doi: 10.1016/0042-6989(95)00114-F
25. Govardovskii VI, Fyhrquist N, Reuter T, Kuzmin DG, Donner K. In search of the visual pigment template. Visual Neurosci (2000) 17:509–28. doi: 10.1017/S0952523800174036
26. Luo DG, Yue WW, Ala-Laurila P, Yau KW. Activation of visual pigments by light and heat. Science (2011) 332:1307–12. doi: 10.1126/science.1200172
27. Merbs SL, Nathans J. Absorption spectra of human cone pigments. Nature (1992) 356:433–5. doi: 10.1038/356433a0
28. Brown TM. Using light to tell the time of day: sensory coding in the mammalian circadian visual network. J Exp Biol (2016) 219:1779–92. doi: 10.1242/jeb.132167
29. Somasundaram P, Wyrick GR, Fernandez DC, Ghahari A, Pinhal CM, Simmonds Richardson M, et al. C-terminal phosphorylation regulates the kinetics of a subset of melanopsin-mediated behaviors in mice. Proc Natl Acad Sci U.S.A. (2017) 114:2741–6. doi: 10.1073/pnas.1611893114
30. Blasic JR Jr., Lane Brown R, Robinson PR. Light-dependent phosphorylation of the carboxy tail of mouse melanopsin. Cell Mol Life Sci (2012) 69:1551–62. doi: 10.1007/s00018-011-0891-3
31. Blasic JR Jr., Matos-Cruz V, Ujla D, Cameron EG, Hattar S, Halpern ME, et al. Identification of critical phosphorylation sites on the carboxy tail of melanopsin. Biochemistry (2014) 53:2644–9. doi: 10.1021/bi401724r
32. Cameron EG, Robinson PR. Beta-arrestin-dependent deactivation of mouse melanopsin. PloS One (2014) 9:e113138. doi: 10.1371/journal.pone.0113138
33. Mure LS, Hatori M, Ruda K, Benegiamo G, Demas J, Panda S. Sustained melanopsin photoresponse is supported by specific roles of beta-arrestin 1 and 2 in deactivation and regeneration of photopigment. Cell Rep (2018) 25:2497–2509 e4. doi: 10.1016/j.celrep.2018.11.008
34. Mure LS, Hatori M, Zhu Q, Demas J, Kim IM, Nayak SK, et al. Melanopsin-encoded response properties of intrinsically photosensitive retinal ganglion cells. Neuron (2016) 90:1016–27. doi: 10.1016/j.neuron.2016.04.016
35. Sheng Y, Chen L, Ren X, Jiang Z, Yau KW. Molecular determinants of response kinetics of mouse M1 intrinsically-photosensitive retinal ganglion cells. Sci Rep (2021) 11:23424. doi: 10.1038/s41598-021-02832-9
36. Do MTH, Kang SH, Xue T, Zhong H, Liao HW, Bergles DE, et al. Photon capture and signalling by melanopsin retinal ganglion cells. Nature (2009) 457:281–7. doi: 10.1038/nature07682
37. Tsukamoto H, Kubo Y, Farrens DL, Koyanagi M, Terakita A, Furutani Y. Retinal attachment instability is diversified among mammalian melanopsins. J Biol Chem (2015) 290:27176–87. doi: 10.1074/jbc.M115.666305
38. Valdez-Lopez JC, Gebreegziabher M, Bailey RJ, Flores J, Awotunde O, Burnett T, et al. Protein phosphatase 2A and clathrin-mediated endocytosis facilitate robust melanopsin light responses and resensitization. Invest Ophthalmol Vis Sci (2020) 61:10. doi: 10.1167/iovs.61.12.10
39. Sexton TJ, Golczak M, Palczewski K, Van Gelder RN. Melanopsin is highly resistant to light and chemical bleaching in vivo. J Biol Chem (2012) 287:20888–97. doi: 10.1074/jbc.M111.325969
40. Spoida K, Eickelbeck D, Karapinar R, Eckhardt T, Mark MD, Jancke D, et al. Melanopsin variants as intrinsic optogenetic on and off switches for transient versus sustained activation of G protein pathways. Curr Biol (2016) 26:1206–12. doi: 10.1016/j.cub.2016.03.007
41. Gamlin PD, McDougal DH, Pokorny J, Smith VC, Yau K-W, Dacey DM. Human and macaque pupil responses driven by melanopsin-containing retinal ganglion cells. Vision Res (2007) 47:946–54. doi: 10.1016/j.visres.2006.12.015
42. Mouland JW, Stinchcombe AR, Forger DB, Brown TM, Lucas RJ. Responses to spatial contrast in the mouse suprachiasmatic nuclei. Curr Biol (2017) 27:1633–1640 e3. doi: 10.1016/j.cub.2017.04.039
43. Hillman P, Hochstein S, Minke B. Transduction in invertebrate photoreceptors: role of pigment bistability. Physiol Rev (1983) 63:668–772. doi: 10.1152/physrev.1983.63.2.668
44. Wang JS, Kefalov VJ. The cone-specific visual cycle. Prog Retin Eye Res (2011) 30:115–28. doi: 10.1016/j.preteyeres.2010.11.001
45. Fu Y, Zhong H, Wang MH, Luo DG, Liao HW, Maeda H, et al. Intrinsically photosensitive retinal ganglion cells detect light with a vitamin a-based photopigment, melanopsin. Proc Natl Acad Sci (2005) 102:10339–44. doi: 10.1073/pnas.0501866102
46. Mure LS, Vinberg F, Hanneken A, Panda S. Functional diversity of human intrinsically photosensitive retinal ganglion cells. Science (2019) 366:1251–5. doi: 10.1126/science.aaz0898
47. Walker MT, Brown RL, Cronin TW, Robinson PR. Photochemistry of retinal chromophore in mouse melanopsin. Proc Natl Acad Sci (2008) 105:8861–5. doi: 10.1073/pnas.0711397105
48. Zhao X, Pack W, Khan NW, Wong KY. Prolonged inner retinal photoreception depends on the visual retinoid cycle. J Neurosci (2016) 36:4209–17. doi: 10.1523/JNEUROSCI.2629-14.2016
49. Tu DC, Owens LA, Anderson L, Golczak M, Doyle SE, McCall M, et al. Inner retinal photoreception independent of the visual retinoid cycle. Proc Natl Acad Sci U.S.A. (2006) 103:10426–31. doi: 10.1073/pnas.0600917103
50. Do MTH, Yau K-W. Adaptation to steady light by intrinsically photosensitive retinal ganglion cells. Proc Natl Acad Sci U.S.A. (2013) 110:7470–5. doi: 10.1073/pnas.1304039110
51. Wong KY, Dunn FA, Berson DM. Photoreceptor adaptation in intrinsically photosensitive retinal ganglion cells. Neuron (2005) 48:1001–10. doi: 10.1016/j.neuron.2005.11.016
52. Emanuel AJ, Kapur K, Do MTH. Biophysical variation within the M1 type of ganglion cell photoreceptor. Cell Rep (2017) 21:14. doi: 10.1016/j.celrep.2017.09.095
53. Mure LS, Rieux C, Hattar S, Cooper HM. Melanopsin-dependent nonvisual responses: evidence for photopigment bistability in vivo. J Biol Rhythms (2007) 22:411–24. doi: 10.1177/0748730407306043
54. Milner ESM, Do MTH. A population representation of absolute light intensity in the mammalian retina. Cell (2017) 171:11. doi: 10.1016/j.cell.2017.09.005
55. Koyanagi M, Kubokawa K, Tsukamoto H, Shichida Y, Terakita A. Cephalochordate melanopsin: evolutionary linkage between invertebrate visual cells and vertebrate photosensitive retinal ganglion cells. Curr Biol (2005) 15:1065–9. doi: 10.1016/j.cub.2005.04.063
56. Mure LS, Cornut PL, Rieux C, Drouyer E, Denis P, Gronfier C, et al. Melanopsin bistability: a fly’s eye technology in the human retina. PloS One (2009) 4:e5991. doi: 10.1371/journal.pone.0005991
57. Cleymaet AM, Gallagher SK, Tooker RE, Lipin MY, Renna JM, Sodhi P, et al. Mu-opioid receptor activation directly modulates intrinsically photosensitive retinal ganglion cells. Neuroscience (2019) 408:400–17. doi: 10.1016/j.neuroscience.2019.04.005
58. Pires SS, Hughes S, Turton M, Melyan Z, Peirson SN, Zheng L, et al. Differential expression of two distinct functional isoforms of melanopsin (Opn4) in the mammalian retina. J Neurosci (2009) 29:12332–42. doi: 10.1523/JNEUROSCI.2036-09.2009
59. Bellingham J, Chaurasia SS, Melyan Z, Liu C, Cameron MA, Tarttelin EE, et al. Evolution of melanopsin photoreceptors: discovery and characterization of a new melanopsin in nonmammalian vertebrates. PloS Biol (2006) 4:e254. doi: 10.1371/journal.pbio.0040254
60. Davies WI, Zheng L, Hughes S, Tamai TK, Turton M, Halford S, et al. Functional diversity of melanopsins and their global expression in the teleost retina. Cell Mol Life Sci (2011) 68:4115–32. doi: 10.1007/s00018-011-0785-4
61. Melyan Z, Tarttelin EE, Bellingham J, Lucas RJ, Hankins MW. Addition of human melanopsin renders mammalian cells photoresponsive. Nature (2005) 433:741–5. doi: 10.1038/nature03344
62. Jagannath A, Hughes S, Abdelgany A, Pothecary CA, Di Pretoro S, Pires SS, et al. Isoforms of melanopsin mediate different behavioral responses to light. Curr Biol (2015) 25:2430–4. doi: 10.1016/j.cub.2015.07.071
Keywords: melanopsin (OPN4), opsin, pigment, bistable, tristable, bleaching, persistent activity, intrinsically photosensitive ganglion cell
Citation: Emanuel AJ and Do MTH (2023) The multistable melanopsins of mammals. Front. Ophthalmol. 3:1174255. doi: 10.3389/fopht.2023.1174255
Received: 26 February 2023; Accepted: 10 April 2023;
Published: 26 April 2023.
Edited by:
David W. Marshak, University of Texas Health Science Center, United StatesReviewed by:
Robert James Lucas, The University of Manchester, United KingdomCopyright © 2023 Emanuel and Do. This is an open-access article distributed under the terms of the Creative Commons Attribution License (CC BY). The use, distribution or reproduction in other forums is permitted, provided the original author(s) and the copyright owner(s) are credited and that the original publication in this journal is cited, in accordance with accepted academic practice. No use, distribution or reproduction is permitted which does not comply with these terms.
*Correspondence: Michael Tri H. Do, bWljaGFlbC5kb0BjaGlsZHJlbnMuaGFydmFyZC5lZHU=
†Present address: Alan J. Emanuel, Department of Cell Biology, Emory University School of Medicine, Atlanta, GA, United States