- Academic Ophthalmology, School of Medicine, University of Nottingham, Nottingham, United Kingdom
There is growing evidence that the pathogenesis of retinal diseases such as diabetic retinopathy (DR) and age-related macular degeneration (AMD) have a significant chronic inflammatory component. A vital part of the inflammatory cascade is through the activation of pattern recognition receptors (PRR) such as toll-like receptors (TLR). Here, we reviewed the past and current literature to ascertain the cumulative knowledge regarding the effect of TLRs on the development and progression of retinal diseases. There is burgeoning research demonstrating the relationship between TLRs and risk of developing retinal diseases, utilising a range of relevant disease models and a few large clinical investigations. The literature confirms that TLRs are involved in the development and progression of retinal diseases such as DR, AMD, and ischaemic retinopathy. Genetic polymorphisms in TLRs appear to contribute to the risk of developing AMD and DR. However, there are some inconsistencies in the published reports which require further elucidation. The evidence regarding TLR associations in retinal dystrophies including retinitis pigmentosa is limited. Based on the current evidence relating to the role of TLRs, combining anti-VEGF therapies with TLR inhibition may provide a longer-lasting treatment in some retinal vascular diseases.
1 Introduction
The retina is an important neuro-sensory organ that transduces photons of light into electrical signals and conveys visual information about our environment to the brain. Normal functioning of the retina is essential for good vision and quality of life. Retinal diseases such as diabetic retinopathy (DR), including diabetic macular oedema (DMO) and proliferative diabetic retinopathy (PDR), and age-related macular degeneration (AMD), are the leading causes of irreversible blindness worldwide (1–4). These diseases have common pathogenetic themes of inflammation, and ischaemia (5–7).
The retina is the most metabolically active tissue in the body. It is susceptible to ischaemia in situations where there is a mismatch between retinal blood flow/perfusion and retinal metabolic requirements (8), and leads to the activation of harmful compensatory mechanisms. Aberrant activation of specific innate immune responses has been implicated in the progression of retinal diseases (9), with inherited variations in genes related to immune function significantly contributing to the increased risk of disease development. The pathways and mechanisms by which immune activation and inflammation contribute to retinal diseases are not fully characterised. Microbial infections usually initiate acute inflammatory responses; however, in many retinal conditions, chronic inflammatory damage occurs due to sterile inflammation. Specifically, activation of the innate immune system is triggered when damage-associated molecular patterns (DAMPs) are released by distressed cells, which bind to pattern recognition receptors (PRR) such as toll-like receptors (TLRs) (6, 10–13).
TLRs are expressed in several ocular tissues (14–16), and cells including the retinal pigment epithelium, and vascular endothelial cells of the retina and choroid (Figure 1) (17–19). Knowledge on TLR expression and pathways in the choroido-retinal complex have continued to expand. In addition, there has been a significantly improved understanding of the pathophysiology of different retinal diseases, including contributions from chronic inflammation, and hypoxia-activated signalling pathways. This review aims to provide a detailed landscape of TLRs regulation/interaction in the causation of retinal diseases.
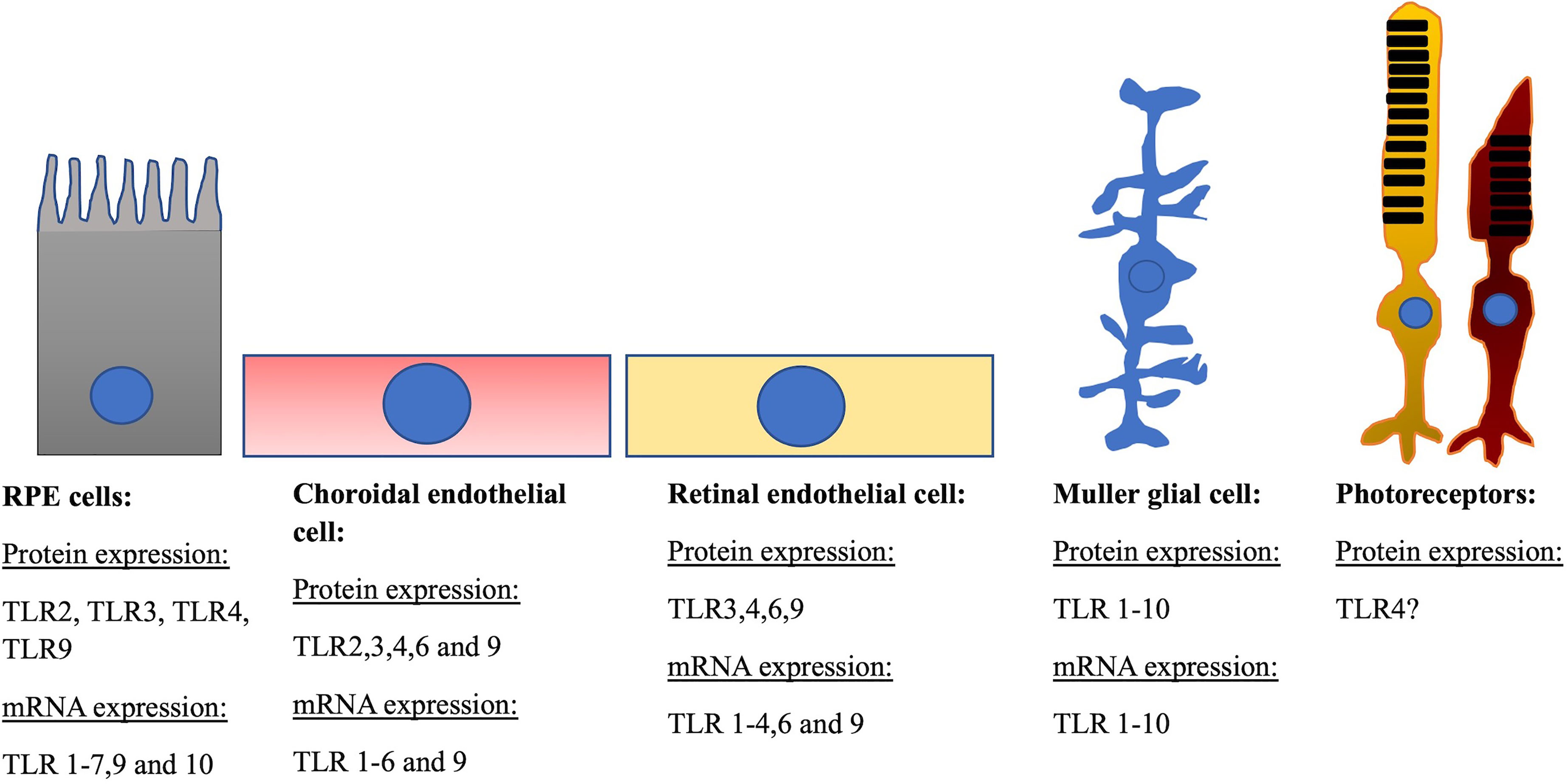
Figure 1 Location of TLRs on retinal cells. RPE cells: Human RPE cells express mRNA for TLRs 1-10 excluding TLR8. TLRs 1 and 3 being the most highly expressed at mRNA level. Protein of TLR 2-4, and -9 have also been identified. Choroidal endothelial cell: Human choroidal endothelial cells express mRNA for TLRs 1-6 and 9 and protein for TLRs 2-6 and 9. Retinal endothelial cell: Human retinal endothelial cells express mRNA for TLRs 1-4, -6 and -9 and protein for TLRs 3, 4, 6 and 9 (17). Muller glial cell: Human muller cells express mRNA and protein for TLRs 1-10. Photoreceptors: Photoreceptors from the 661W murine cell line express TLR4 protein.
1.1 Toll-Like Receptors
Nüsslein-Volhard and Wieschaus first discovered the Toll gene in 1980 as a mutated gene that led to changes during the embryonic development of the drosophila fly (20). Later, studies into the mechanisms used by the Drosophila fly to fight against and recognize infections contributed to the discovery of Toll protein and subsequently, TLRs function in the innate immunity of other species (21, 22).
TLRs are a family of type-1 transmembrane receptors (23) that contribute to the activation of innate and adaptive immunity through inflammatory mediators produced in response to molecular patterns associated with micro-organisms (Table 1 and Figure 2) known as pathogen-associated molecular patterns (PAMPs). In addition, TLRs can also respond to self-derived molecules, also known as damage-associated molecular patterns (DAMPs), released from cells as a result of aging or injury. DAMPs include self-derived molecules such as HMGB-4, nucleic acids, uric acid crystals, hyaluronan, surfactant protein A and other extracellular matrix products (35).
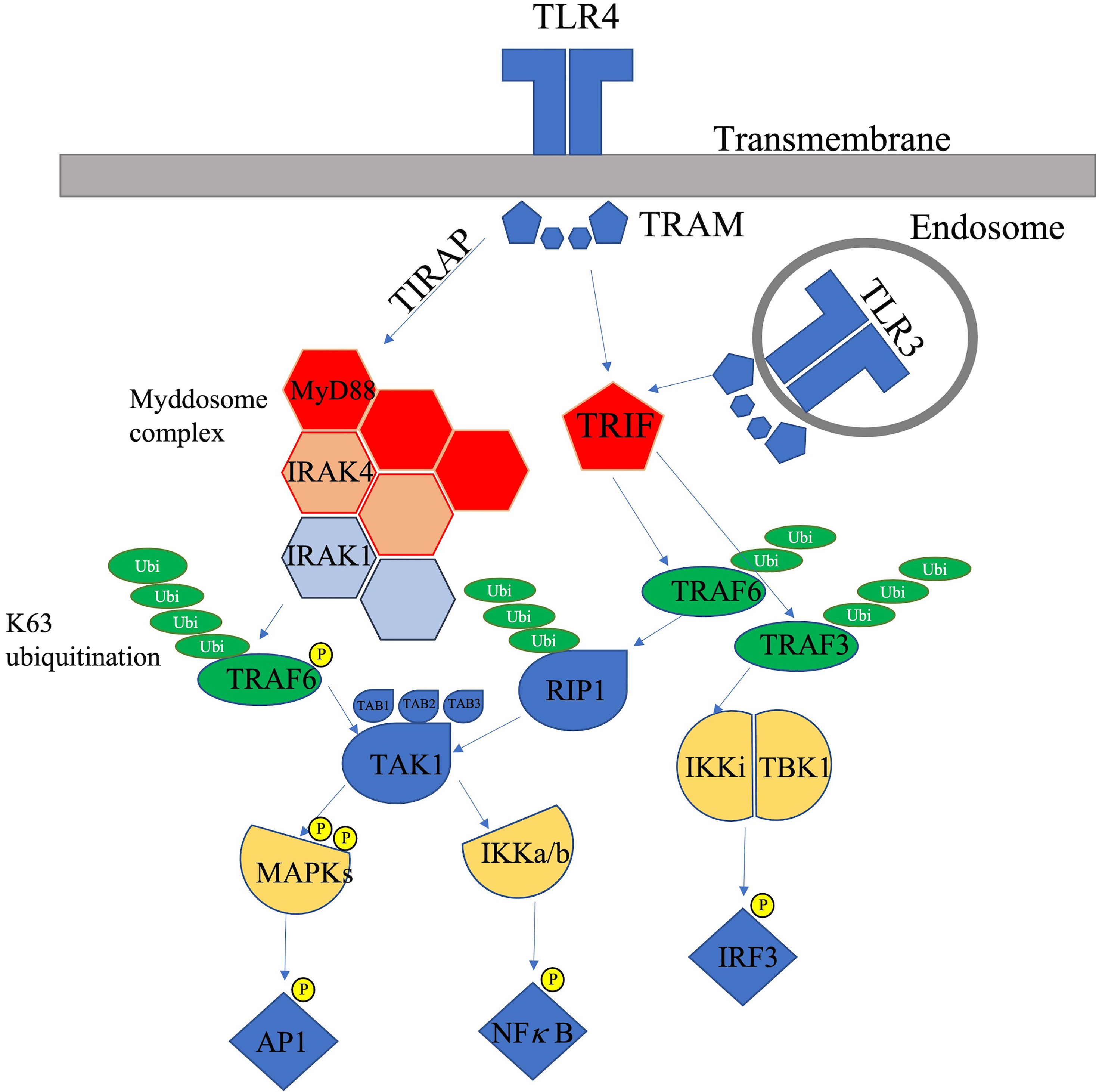
Figure 2 TLR-3 and TLR-4 signalling pathways [adapted from (34)]. TLR3 receptors are located intracellularly in endosomal compartments whereas TLR4 is located on the cell surface. Activation of the TLRs initiate signalling pathways downstream of two adaptor proteins, TRIF and MyD88. Activation of the TIRAP pathway leads to formation of the myddosome complex which comprises MyD88, IRAK 4 and IRAK1. Activated IRAK1 induces TRAF6 after k63 polyubiquitination, this leads to activation of TAK1 which subsequently activates the IKK complex-NF-κB and MAPK. Activation of the TRAM pathway via the TRIF adaptor leads to the activation of TRAF6 and TRAF3, TRAF6 recruits the RIP1 kinase which activates the TAK1 complex which subsequently triggers the MAPK and NF-κB signalling pathways. The IKK related kinases, IKKI and TBK1, are recruited by TRAF3 and this leads to phosphorylation of IRF3 leading to Type 1 interferon transcription.
The TLR family consists of 10 members in humans (TLR1-10) (Table 1) and 12 members in mice (TLR1-LTR9, TLR11-TLR13) (36). The location and distribution of TLRs vary in different cell types. TLR1-2, TLR4-6 and TLR10 are found predominantly on the extracellular surface, whereas TLR3, TLR7-9, TLR11-13 (which are only found in mice) are found intracellularly (Figure 2).
TLRs consist of a horseshoe-shaped ectodomain containing leucine-rich repeats (LRRs), which function to recognize PAMPs, a transdomain component and an intracellular cytoplasmic Toll/IL1 (TIR) domain that triggers signalling of downstream molecules (34). The ectodomain portion of TLRs form either a homo or heterodimer. Upon binding of a PAMP or DAMP to the ectodomain of a TLR, specific TIR domain-containing adaptor proteins such as TIR domain-containing adapter inducing interferon-β (TRIF) or Myeloid differentiation factor 88 (MyD88) are recruited. These trigger the initiation of signalling pathways that lead to the activation of molecules such as nuclear factor kappa-B (NF-κB), type-1 interferons, or IRFs (34). The signalling pathways mediated by TLRs is divided into two groups: TRIF dependent or MyD88 dependent.
TLR2 forms a heterodimer with TLR1 leading to recognition of tri-acyl lipopeptides (26), whereas the heterodimer formed between TLR2 and TLR6 recognizes di-acyl lipopeptides (26). TLR10, the most recently discovered, is the only TLR with anti-inflammatory properties (33), and can detect the influenza A virus (37) and works with TLR2 to detect Listeria spp. (38).
1.1.1 MyD88 Pathway
Activation of MyD88 associated TLRs leads to activation of Toll/Interleukin-1 receptor domain-containing adapter protein (TIRAP), which conducts signal from the TLR2 and 4 to MyD88 (Figure 2). Recruitment of MyD88 after ligand binding to corresponding TLRs leads to MyD88 forming a ‘myddosome’ complex with interleukin-1 receptor-associated kinase (IRAK) family members (39). MyD88 recruits IRAK4, leading to the formation of the MyD88-IRAK4 complex which subsequently recruits the substrates of IRAK4, IRA2 or IRAK1. This leads to the formation of a myddosome complex bringing the IRAKs kinase domains into proximity for phosphorylation and activation (39).
IRAK4/1 recruits tumour necrosis factor (TNF)-receptor-associated factor (TRAF)6, which along with ubiquitin-conjugating enzyme 13 (UBC13), leads to the k63 ubiquitination of TRAF6 (40). The ubiquitination of TRAF6 leads to the recruitment of TAK-1 binding proteins (TAB1,2,3), which form a TAK-1 protein kinase complex that activates the IκB kinase (IKK)–NF-κB complex and MAPK kinases through phosphorylation (41). The binding of TAK1 to the IKK complex leads to activation of the IKK complex and subsequent activation of NF-κB mediated genes (42).
1.1.2 TRIF Pathway
The binding of ligands to TRIF-associated TLRs leads to the interaction of the receptor adaptor, TRIF, with TRAF-3 and -6 (43). TRAF-6 subsequently recruits the RIP-1 kinase, which activates the TAK1 complex, ultimately triggering the activation of NF-κB and MAPK pathways (44) and induces the production of inflammatory cytokines. TRAF3 activates and recruits the IKKi, TBK1, and NEMO, which phosphorylates IRF3 and induces the expression of type 1 interferon (IFN) associated genes.
1.1.3 TLRs Localization in Human Retina
TLRs are widely expressed on a variety of immune cells such as dendritic cells, natural killer cells, and B-cells. They are also found on non-immune cells such as fibroblasts, endothelial and epithelial cells (34).
In humans, RPE cells have been shown to express genes for TLRs 1-7, -9 and TLR10 (18, 26, 45) with TLR1 and -3 being shown to be highly expressed. Proteins of TLR2 (18, 46), TLR3 (18, 45), TLR4 (18, 47, 48) and TLR9 (45) have also been localized in human RPE cells.
Our group has previously demonstrated the mRNA expression of TLRs 1-6 and 9 in primary human choroidal endothelial cells (hCEC) (17). Utilizing western blotting, we quantitated the protein expression of TLRs -3, -4 and -9 in hCEC, whilst with flow cytometry, TLR -3, -4, -6 and -9 were shown to be localized on the surface of cells and intracellularly (17). Subsequently, Feng and co-workers confirmed the localization of TLR2 in hCECs (46). In primary human retinal endothelial cells (hREC), mRNA for TLRs 1-4, 6 and 9 were expressed (17). In addition, TLRs -3, -4, -6, and -9 were shown to be localized both on the surface and intracellularly in hREC (17). Expression of key TLRs were further validated in hCECs and hRECs using synthetic PAMPs. Overall, this study showed for the first time that TLRs are localized in retinal and choroidal endothelial cells and potentially may be involved in the inflammatory damage of retina during DR and AMD. Human Muller cells have been shown to express mRNA and protein for TLR 1-10, MyD88, TRIF, TRAM and TRAF6 along with MD2 and CD14 co-receptors (49). Photoreceptors from the 661W murine cell line have been shown to express TLR4 protein (50).
1.2 Literature Search: Characteristics of Selected Studies
We performed a literature search of the National Library of Medicine (PubMed) and Ovid MEDLINE(R) from July 1946 to August 2021 using the following search criteria. Only full-text articles published in English were included. ((retinal or inflammatory retinal or inflammatory retinopathy or diabetic retinopathy or hypertensive retinopathy or age-related macular degeneration, AMD or ARMD or birdshot chorioretinopathy or Vogt-Koyanagi-Harada or VKH or retinal vascular occlusion or central retinal vein occlusion or central retinal artery occlusion or branch retinal vein occlusion or branch retinal artery occlusion or retinitis pigmentosa or cystoid macular oedema) and (toll-like receptor or TLR or toll-like)).
Our initial search criteria yielded 779 studies (Figure 3). After removing duplicates, we obtained 401 studies. Screening of the title and abstract yielded 183 studies as unrelated studies, and editorial/commentaries were removed. Further screening by review of article contents yielded 113 studies as the main body of our literature review, between January 2004 and September 2021. Another 52 articles were acquired by direct database search.
2 Retinal Diseases and TLRs
2.1 Diabetic Retinopathy
2.1.1 Background
Diabetes mellitus (DM) can be divided into two broad categories: type 1 and type 2 diabetes. Type 1 DM occurs due to a complex interaction between genetic and environmental factors, resulting in autoimmune destruction of the beta cells in the islet of Langerhans. Type 2 DM also occurs due to a complex interaction between genetic and environmental factors, resulting in reduced insulin sensitivity (51) and eventual beta-cell depletion in the later stages of type 2 DM. Both types subsequently lead to a loss of homeostatic control of blood glucose levels.
DM is one of the modern world’s most significant epidemics, affecting both developing and developed countries, estimated to affect approximately 464 million adults worldwide (20-79 years of age) in 2019, with a projection to increase to 700 million by 2045 (52). Historically, DM was perceived as a disease that mainly affected developing countries. However, 79% of adult patients with DM are from low or middle-income countries (53).
DM is associated with various vascular complications varying from microvascular diseases affecting the retinal vasculature, renal vasculature, or microvasculature of the cerebral circulation. Vascular pathology in blood vessels supplying nerves can contribute to the development of diabetic neuropathy. It can also have deleterious effects on larger vessels, contributing to peripheral limb ischaemia, coronary artery disease, and carotid artery stenosis.
Diabetes causes a wide range of ocular diseases, the most severe and commonest (54) being DR, which is also a leading cause of blindness in the developed world (55, 56). The risk of developing DR is related to the duration of DM (57) but also influenced by diabetic control (58), high blood pressure (59), and high blood lipid levels (60).
2.1.2 Pathophysiology of Diabetic Retinopathy
The blood-retinal barrier (BRB) prevents the passage of harmful molecules from the systemic circulation into retinal tissue (61). The blood-retinal barrier consists of an inner and outer BRB. The inner BRB is formed by tight junctions between the continuous endothelium lining retinal vessels, and the outer BRB is formed by the tight junctions between RPE cells (61).
The RPE consists of a single layer of cuboidal epithelial cells that lie between the photoreceptors and choroid (47), they aid with removal of waste products and secretes cytokines/chemokines to facilitate cellular messaging. Aged or damaged photoreceptor outer segments are constantly shed and digested by RPE cells (47).
Various pathological mechanisms compromised the BRB in diabetes. Increased paracellular permeability due to disruption of tight junction proteins such as occludin (61), which is frequently reported as being associated with diabetes-induced BRB failure (62). Endothelial cell loss occurs due to leucostasis, oxidative stress, and endothelial progenitor dysfunction (61).
Pericyte loss contributes to the progression of diabetic retinopathy due to a loss of the regulation of blood flow through the contractile properties of pericytes and loss of endothelial cell maintenance (61). Loss of retinal endothelial cells can lead to vascular occlusions and create a hypoxic cellular environment, which subsequently enhances the secretion of angiogenic factors and the growth of abnormal retinal vessels (proliferative DR). Rupture of these abnormal vessels, lead to vitreous hemorrhage or result in tractional retinal detachment. Proliferative/late-stage DR is frequently managed with laser photocoagulation and anti-VEGF monoclonal antibody therapy (63).
Activation of TLR signalling pathways has been shown to contribute to the development of diabetes-related renal complications. TLR4/NFκB pathway mediated activation of peroxisome proliferator-activated receptor-y coactivator-1a (PGC-1a) has been shown to accelerate the development of diabetic kidney disease (64).
DR is associated with a chronic subclinical inflammatory state, characterised by elevated levels of inflammatory proteins such as heat shock protein (HSP) 60/70, fibrinogen, oxidized LDL, and fibronectin (55). It is thought that activation of PRR involved in the innate immune response, such as receptor for advanced glycation end-product (RAGE), may be associated with the development of diabetes or the manifestation of diabetic complications.
2.1.3 Toll-Like Receptors in Diabetic Retinopathy
2.1.3.1 Leucocyte Adhesion
Diabetes is associated with increased expression of TLR4 (63, 65–72), TLR2 (65, 68, 73) and TLR7 (74) in human and mouse retinal cells. Hyperglycaemia has been shown to increase MyD88 independent and dependent signalling (75) along with pro-inflammatory/angiogenic mediators downstream to MyD88 such as NFκB, VEGF, TNF-α and IL-1β (65, 67–69, 72, 75).
Deleting MyD88 or TLR2/4 from bone marrow-derived immune cells significantly reduces diabetes-induced pathological changes in the retina such as leucostasis, vascular permeability, superoxide generation and intercellular adhesion molecule 1 (ICAM-1) (76). Bone marrow-derived leukocytes from mice with diabetes have been shown to kill endothelial cells more than those from naïve mice (76). A subsequent further study confirmed these phenotypic differences of BM cells. TLR4 deficient BM cells from diabetic mice showed reduced features of DR compared with diabetic wild-type mice (77). TLR4 deficient mice showed lower levels of inflammatory cytokines and angiogenic factors. Notably, transplantation of TLR4 sufficient BM-derived cells from wild type mice into TLR4 deficient mice leads to restoration of pro-inflammatory cytokine (TNF-α, IL-1β) release and angiogenesis-related genes (VEGF, HIF-1α) (77).
There is a substantial amount of evidence showing increased expression of TLRs, in particular TLR4, in models of diabetes in both human and mouse retinal cells, along with increased expression of molecules downstream to TLR. Overall, hyperglycaemic conditions appear to increase the activity of leucocytes in the retinal vascular endothelium in a TLR4-dependent fashion (Figure 4). To summarize, hyperglycaemic conditions appear to increase the activity of leucocytes in the retinal vascular endothelium in a TLR-4 dependent fashion; however, other TLRs are seen to be increased in both mouse and human retinal cells.
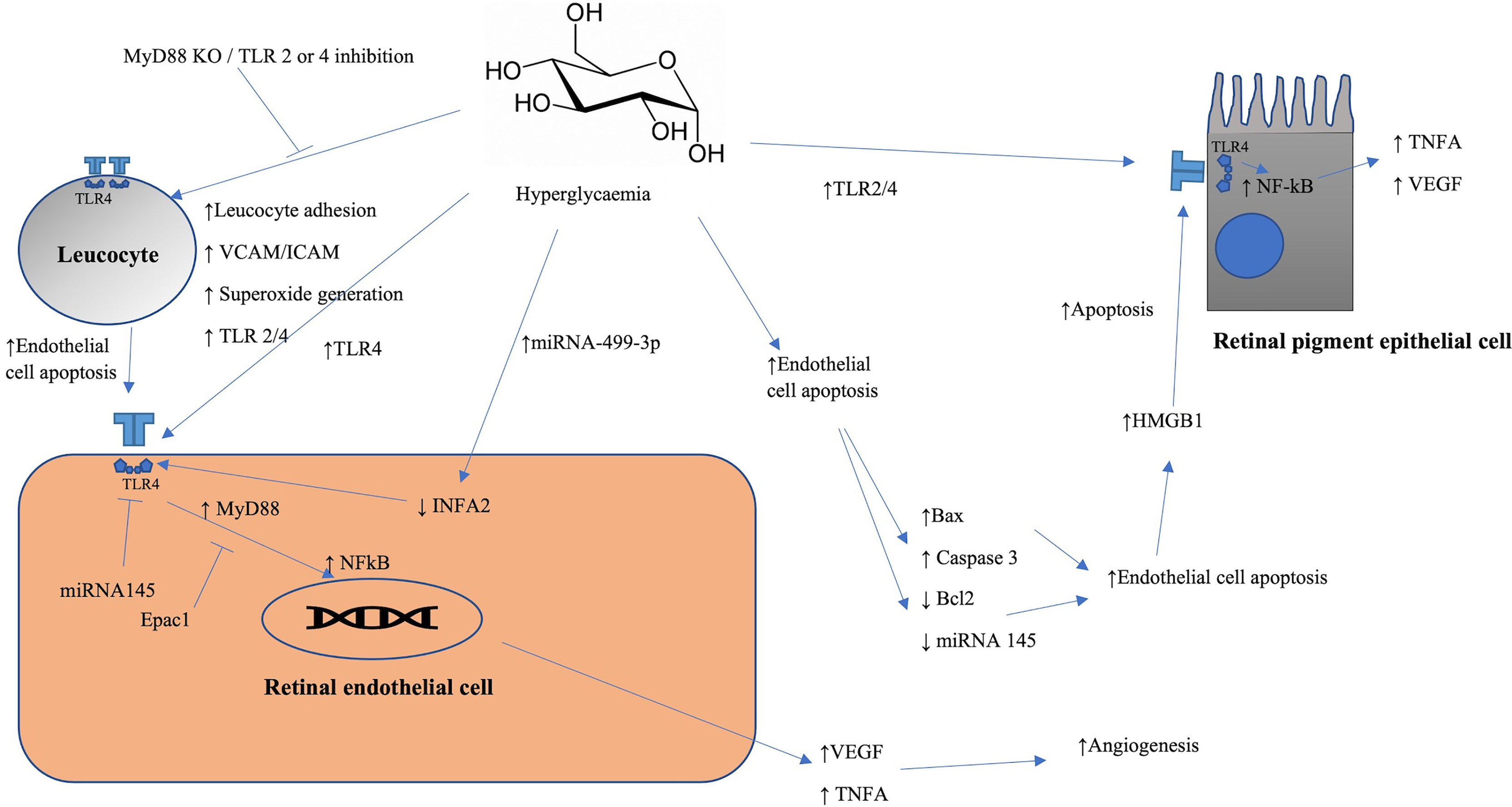
Figure 4 Schematic representation of TLRs involvement in diabetic retinopathy. Initiation of the pathological endothelial changes seen in diabetes are dependent on TLR-2 and -4. HMGB1 levels are raised in response to hyperglycaemia in rat endothelial cells and the source may be from bone marrow derived cells or from dying endothelial cells. HMGB1 binds to the TLR4 receptor initiating activation of the NF-κB pathway leading to subsequent increased levels of TNF-α and VEGF in RPE cells. Diabetes induced miRNA-499-3p triggers the levels of TLR4 via inhibition of INFA2 leading to reduced endothelial cell proliferation and increased apoptosis. Retinal endothelial cell apoptosis is associated with raised levels of markers of apoptosis such as bax and caspase-3 and reduction of anti-apoptotic markers such as BCL-2. Hyperglycaemic condition exacerbates the levels of TNF-α, NF-κB and VEGF production in ARPE19.
2.1.3.2 Endothelial Cell Apoptosis
High mobility group box -1 (HMGB1) is a nuclear protein present in the nucleus of most cells and is secreted by macrophages, natural killer cells and mature dendritic cells in response to cellular distress or injury (78). Levels are raised in response to hyperglycaemia in rat retinal endothelial cells and ARPE-19 cells (65). HMGB1 has been shown to decrease insulin receptor and Akt phosphorylation via signalling through TLR4 in human retinal endothelial cells (79).
Hyperglycaemia is also associated with hREC apoptosis (80, 81), reduced RPE viability (82) and raised levels of TLR4 and NF-κB (81) in HRECs. The effect of which is inhibited by the application of gastrodin via induced activation of the SIRT1/TLR4 pathway (81). Hyperglycaemia has been shown to increase TNF-α, NF-κB activity and VEGF production in ARPE19 cells (65). This is alleviated by the administration of antibodies against HMGB1 (65). Hyperglycaemia-induced increased levels of TLR4 and HMGB1 are inhibited by the application of the Box A portion of HMGB1 (Box A) and glycyrrhizin (83) in both cultured human REC and mouse retina.
Hyperglycaemia induced endothelial cell apoptosis is associated with raised levels of apoptotic markers such as Bax and cleaved Caspase-3, whilst anti-apoptotic markers such as BCL-2 are reduced (80). Application of Box A and glycyrrhizin to REC was associated with reduced insulin receptor substrate (IRS-1)Ser307 phosphorylation and reduced cleavage of caspase 3 (83). Microglial matrix metalloproteinase 9 (MMP-9) levels are raised in response to hyperglycaemia via HMGB1/TLR4 signalling (84) in BV2 murine glial cells.
TLR4 signalling in RECs is attenuated by exchange protein activated by cAMP 1 (Epac1) leading to inhibition of hyperglycaemia induced MyD88-mediated signalling, suggesting that Epac1 acts upstream of TLR4 dependent signalling (75). In addition, the loss of Epac1 has been shown to increase the levels of inflammatory mediators downstream to TLR4 (NFκB, TNF-α, IL-1β) (75).
Diabetic rat retinal cell have decreased IFNα2 expression associated with upregulated micro-RNA-499-3p (71). The miRNA-499-3p reduces IFNα2 levels by targeting 3’-UTR of IFNα2, which subsequently reduces the retinal cell proliferation and lead to increased apoptosis.
Inhibition of miRNA-27a has been shown to increase the caspase-3/9 activity and induces expression of IL-6, TNF-α, and IL-1β in human RPE-1 cells exposed to high glucose levels, which are associated with reduced cell viability (82). miRNA-27a transfection of RPE cells lead to TLR4 inhibition, reduced cell viability and levels of IL6, TNF-α, IL-1β, respectively (82).
TLR4 deletion in diabetic mice reduces VEGF and glial fibrillary acidic protein (GFAP) levels and retinal tissue thickness compared to the naïve mice (70, 77). This is further associated with the reduced retinal inflammation which is characterised by the reduced levels of IL6, TNF-α, and IL-1β (70). Hyperglycaemia induced apoptosis and oxidative stress in hRECs is reduced by the overexpression of miRNA-145 (80) which targets TLR4 (80).
TLR7 deficiency attenuates hyperglycaemia induced retinal damage in mice RPE cells (74) and has an anti-angiogenic effect associated with reduced vascular tufts, fluorescein leakage and retinal hemorrhages (74). In addition, TLR7 deficiency in DR mouse model also reduces the pro-inflammatory cytokines such as TNF-α, IL-1β, IL-6, IL-12 in retina (74).
Excess calorie consumption and/or obesity contributes to the risk of developing type-2 diabetes and metabolic syndrome (85). Administration of a high-fat diet to TLR4 knockout and wild type mice revealed that the high-fat diet induces increased oxidative stress and DNA damage in the inner retina in a TLR4-dependent fashion (85).
Activation of β-adrenergic receptor (β-AR) by administering an agonist compound-49b (67) inhibits diabetes-activated TLR4 signalling in mice retina. In addition, compound-49b also reduces TLR4-signalling activation in human retinal endothelial, and Muller cells cultured in high glucose conditions (67).
Diabetic rats show higher levels of insulin like growth factor (IGF), VEGF, TNF-α, glutamate, and malondialdehyde (MDA), from retinal supernatants, which were shown to be reduced by the administration of metformin (63). The expression of mRNA for NFκB, TLR4, and TNF-α are elevated in diabetic rat retinas and implicated with the reduced thickness of the cell layers and ganglion cell vacuolization, both of which are reduced by the administration of metformin (63). The authors implicated that the effect of metformin is mediated via the attenuation of TLR4-induced oxidative stress.
TLR4 has been shown to regulate permeability in murine retinal endothelial cells (86, 87) and the loss of which reduces diabetes-induced thinning of murine retinal vasculature (86, 87) and retinal vascular damage, respectively (86).
Hyperglycaemia has been shown to be associated with increased production of DAMPs such as HMGB1. This subsequently leads to activation of TLR mediated apoptotic pathways and raised levels of markers of apoptosis such as Bax and caspase 3. Hyperglycaemia is also associated with raised levels of pro-inflammatory cytokines via a TLR dependent mechanism. This subsequently leads to loss of retinal endothelial cells, a crucial step in the pathophysiology of diabetic retinopathy.
2.1.3.3 Endothelial Cell Permeability
Diabetes has been shown to induce retinal endothelial permeability in Cdh5Cre-Epac1f/f mice, the effect of which is reduced up on administration of a TLR4 antagonist, TAK-242 (75). TLR4 has been shown to regulate the levels of occludin and ZO-1 (tight-junction proteins) in murine retinal endothelial cells (86).
Increased endothelial cell permeability allows large plasma proteins such as low-density lipoproteins (LDLs) to cross the blood-retinal barrier (BRB), which can subsequently get oxidized in the retina (72). The retina is especially susceptible to oxidative damage due to its high energy demand, high amounts of polyunsaturated fatty acids and exposure to UV radiation (63). This can lead to the production of reactive oxygen species (ROS) (88), which can generate oxidized lipids through lipid peroxidation.
Oxidized LDLs can trigger inflammation in murine retinal Muller cells by stimulating the release of pro-inflammatory cytokines such as IL-6, TNF-α, and IL-1β (72, 88). Oxidized LDL also triggers the production of DAMPs which can activate TLRs leading to retinal inflammation. Raised levels of oxidized LDL are associated with reduced Muller cell survival (72), which is reversed by inhibition of a TLR4 co-receptor complex protein, myeloid differentiation protein-2 (MD2) (72).
In murine Muller cells, TLR4 knockout in mice leads to increased phosphorylation of the insulin receptor (89, 90) along with decreased phosphorylation of Insulin receptor substrate 1 (IRS-1) and caspase 3 (90). Hyperglycaemia induced impairment of the insulin receptor and Akt phosphorylation in rMC-1-O (rat Muller) cells is reduced on the administration of TLR4 siRNA, implying high glucose induces dysfunctional insulin signalling via TLR4 in retinal Muller cells (90).
Changes in retinal endothelial permeability are a crucial part of the pathophysiology of diabetic retinopathy. TLR4 appears to play a role in regulation of endothelial permeability via levels of intercellular adhesion molecules such as occluding and ZO-1. This facilitates the passage of pro-inflammatory DAMPs such as oxidized lipids triggering further inflammation and cellular damage.
2.1.3.4 Platelet-Rich Plasma
Rat platelets exposed to high glucose levels are associated with increased levels of CD61 and Annexin-V positive platelet-rich plasma exosomes (PRP-exos) (91). PRP-exos are taken up by retinal endothelial cells and, diabetic PRP-exos increases the production of MDA and ROS whilst reducing the activity of superoxide dismutase (SOD) (91). Increased levels of ICAM-1 and VCAM-1 when compared to PRP-exos from non-diabetic rats (91) were also reported, indicating endothelial injury via the activation of TLR4.
PRP-exos were associated with increased levels of TLR4, MyD88, and NF-κB/p65, which were inhibited by a TLR4 receptor antagonist (TAK-242) (91). This also reverses the PRP-exo induced MDA, ROS, ICAM1, and VCAM1 levels, respectively. Moreover, TAK-242 also reduces PRP-exos triggered blood vessel leakage (91). PRP-exo stimulation of TLR4 was shown to be mediated via CXCL10 (91).
2.1.4 Toll-Like Receptor Genetic Polymorphisms in Diabetic Retinopathy
DM is a multifactorial condition and various factors contribute to the risk of development and progression of DM and its complications, including inherited risk factors. Although numerous laboratory-based studies demonstrated that the effect of TLRs activation in DR is quite complex, the genetic association studies demonstrating the relationship between TLRs and DR risk are far less and scarce. In humans, the TLR4-Asp299Gly polymorphism has specifically shown an association with the DR risk (Table 2).
2.1.4.1 Asp299Gly Polymorphism
A cohort of 864 patients with T2DM, 352 had DR, was compared to 420 healthy individuals. The carriage of the GG and AG genotypes (299Gly) in the rs4986790 polymorphism was found to be associated with a higher rate of diabetic retinopathy (p<0.001) as carriers of this genotype (63%) had a higher rate of DR compared to non-carriers (39%). The 299Gly genotype of the rs4986790 polymorphism was associated with a higher risk of early-onset DR compared to late-onset DR (OR = 5.0, 95% CI = 2.33–10.71) (55).
Another cohort of 1090 patients with T2DM, of which 342 had DR, and 748 did not have DR, was compared to 716 healthy individuals. There was a significant association between the Asp299Gly G allele and the risk of developing diabetic retinopathy in diabetic patients (p = 0.0002, OR = 2.12, 95% CI = 1.43-3.12) (92).
Another study included a cohort of 113 patients with type 2 diabetes, 10 of which had DR, were compared to 75 patients with T2DM without DR. There was a significant association between the Asp299Gly polymorphism and DR (p=0.018, OR =7.61, 95% CI = 1.41–41.08) (93).
A cohort of 198 patients with T2DM, including 120 with DR, was compared to 200 healthy controls. The Asp299Gly polymorphism appeared to have a protective effect against diabetic retinopathy, contrary to previous studies (94).
The Asp299Gly TLR4 polymorphism has been reported to show an increased TNF-a response to LPS stimulation (95). Furthermore, crystallography has revealed that TLR4 has two highly preserved regions involved in ligand binding (95). The Asp299Gly polymorphism is located close to the TLR4-MD2 binding area, it does not appear to have a direct effect on the binding location but is thought to increase the peptide bonds rotation freedom and a loss of negative charge at position 299 (95).
2.1.4.2 Others
A cohort of 128 patients with DR were compared to 320 healthy controls. The TLR4 polymorphism rs10759931 (p = 0.05, OR = 1.50, 95% CI 0.99–2.26) and rs1927914 (p = 0.05, OR = 1.48, 95% CI 1.0–2.24) were significantly associated with the development of DR when compared to the control group (51). No haplotypes were associated with either susceptibility or resistance to DR (51).
A cohort of 198 patients with T2DM, of which 120 had DR, was compared to 200 healthy controls. The Thr399Ile (P<0.001, OR=0.487, 95% CI=0.211-0.648) polymorphisms appeared to provide a protective status against DR when compared to the healthy controls (94).
2.2 Age-Related Macular Degeneration
2.2.1 Background Information
Age-related macular degeneration (AMD) is the commonest cause of vision loss in patients above the age of 50 (25, 48, 96), affecting almost 200 million people worldwide (1), which is projected to increase due to an ageing population in the developed world. AMD is usually divided into the dry form, the end stage of which is geographic atrophy (GA) or wet form (neovascular AMD) (3, 97, 98).
Although the exact etiology of AMD is not clear, the most significant risk factor is age with smoking being the biggest modifiable risk factor (88) amongst many others, such as hypertension (48), family history and body mass index.
Several genes are associated with AMD risk. The age-related maculopathy susceptibility 2 (ARMS2) and high-temperature requirement A serine peptidase (HTRA1) genes are found on the 10q26 (Ch10) locus (99), which has the greatest contribution to the genetic risk of developing AMD. Other genes such as complement factor H (CFH) and complement factor H-related genes (CFHR) 1 to 5 which are located on the 1q32 (Ch1) region (99), are associated with a risk of developing AMD. Polymorphisms in the TLRs have also been shown to be related to the risk of AMD development (100).
It is clinically divided into two categories; early (visual symptoms are not significant) and late (usually associated with significant vision loss). AMD can also be divided according to the pathological features (of neovascularisation and vascular leakage) as wet (exudative/neovascularization associated) or dry (non-exudative), with the majority of patients suffering from dry (90%) (101) compared to wet (10%) AMD.
The initial identifying feature of dry AMD is the occurrence of soft drusen; these are extracellular deposits located below the RPE consisting of protein- and lipid-rich cellular debris (5). The accumulation of extracellular materials results in the development of low-grade chronic inflammation (102) because of the activation of innate immune system. The natural history of dry AMD manifests as geographic atrophy of the RPE (25). It occurs due to confluent regions of photoreceptor and RPE cell death (103). In more than half of patients, it is bilateral i.e., affecting both eyes. The average rate at which geographic atrophy propagates is between 1.3 to 2.8 mm2 per year (96).
Neovascular or wet AMD is one of the leading causes of blindness in the elderly population in developed countries, affecting over 1 million patients in the United States (98). It is characterised by the development of abnormal choroidal neovascularisation (CNV) or retinal angiomatous proliferation (RAP), which invade the retina leading to fluid leakage (25, 104).
2.2.2 Toll-Like Receptors in AMD
2.2.2.1 Cell Viability
Stimulation of TLR3 and TLR4 using their respective ligands, poly I:C and lipopolysaccharide (LPS) on porcine RPE cells significantly decreases RPE cell viability and the levels of IL-6 and IL-8 (105). Poly I:C inhibits wound healing of a damaged RPE after prolonged treatment, whereas LPS enhance wound healing (105). TLR3 activation by poly I:C reduces porcine RPE cell viability, which is mediated via JNK activation (106).
Intra-vitreous administration of siRNA targeting Cdh16 (kidney-specific cadherin 16), and bone-specific osteocalcin (Bglap1) into wild type mice inhibits the choroidal neovascularization (CNV) (107, 108). siRNAs that target Vegfr1 (AGN211745) and VEGFA (bevasiranib) have been shown to inhibit CNV formation. Interestingly, this effect is lost in TLR-3 deficient mice (107) implying that specific siRNA inhibits CNV via cell surface TLR-3. The use of siRNA molecules for the treatment of AMD appears to be very appealing; however, intravitreal administration of siRNA molecules, regardless of target or sequence, have been shown to induce retinal degeneration characterised by disruption of the retinal pigmented epithelium. This is dependent on TLR-3 activation leading to caspase-3 mediated cell death (109), thereby limiting the potential use of siRNA molecules in the treatment of CNV.
Stimulation of TLR3 using poly I:C, on mouse photoreceptor cells causes significant structural damage and functional loss characterised by reduced a-wave and b-wave amplitudes on the electroretinograph (110).
CD36 is an obligate co-receptor for TLR2 (111). CD36 deficiency in Cx3cr1 deficient background mice reduces the age- or photo-stress induced accumulation of subretinal mononuclear phagocytes and subretinal inflammation (111).
ATP Binding Cassette Subfamily A Member 4 (Abca4)/Retinol Dehydrogenase 8 (Rdh8) deficient mice were shown to develop AMD-like phenotypes on prolonged photosensitisation (112). When compared to wild-type mice, light exposure in Abca4/Rdh8 deficient mice led to increased expression of TLR2 and 4 in association with elevated pro-inflammatory/chemotactic cytokines such as CCL2, CR2, CCCL12, Cx3cr1, IL-1b, TNF-α, CFH, and VEGFA genes (112). Deletion of TLR4 resulted in milder retinal degeneration in this model (112), which concluded that endogenous ligand release from photoreceptor injury/death triggers retinal inflammation via TLR4 signalling activation.
TLR2/4 deficiency has been associated with increased area of subretinal fibrosis compared to wild type mice (113). Similar phenotype was also observed with the application of anti-TLR2/4 antibodies (113). Notably, administration of recombinant HSP70 increased intraocular IL-10 levels and significantly reduced the subretinal fibrosis in TLR2/4 deficient mice (113). These studies have implicated the pathologic role of TLR-2, -4, and -3 signalling pathways in the progression of AMD disease phenotype in murine models.
The pathophysiology of AMD includes the accumulation of extracellular debris and subsequent cellular apoptosis. This cellular debris may contain DAMPs which triggers the immune responses via TLRs leading to reduced RPE and photoreceptor viability.
2.2.2.2 Oxidative Stress
Oxidative stress is a key step in the pathogenesis of AMD, due to the high metabolic requirements of the retina and direct exposure to light. With age, RPE cells lose their inherent abilities to digest photoreceptor outer segments which result in lipofuscin accumulation leading to oxidative stress (9, 46).
Inhibition of TLR2 limits photo-oxidative stress-induced retinal degeneration and outer nuclear layer thinning (88) in murine retina, and is associated with reduced complement C3 deposition in photoreceptors (88). Photo-oxidative stress was shown to activate TLR2 via endogenous DAMP production, which induces the production of C3 and further activation/deposition (88).
Previous studies showed that patients with AMD have 4.6 times higher levels of CEP-Eps (114). Exposure of human primary RPE cells in vitro to oxidized lipids, carboxyethyl pyrrole (CEP) and Pam2CSK4 (PAM) in combination increased IL6, MCP1, CXCL8 and TLR2 gene expression (46) compared to Pam2CSK4 alone. However, CEP alone treatment did not affect these genes (46). Furthermore, TLR2 blockage suppressed CNV initiation and progression in their mouse model and that blockage of TLR2 and VEGF resulted in additive effects on CNV suppression.
Endogenous lipid peroxidation may lead to production of DAMPs. The 7-ketocholesterol (7KCh) is formed by auto-oxidation of cholesterol and is associated with Alzheimer’s disease, atherosclerosis, and AMD, respectively (115). Treatment of ARPE19 cells with 7KCh induces pro-inflammatory molecules such as IL-1β, CAAT-enhancer-binding protein homologous protein (CHOP), IL-8 and VEGF, which is attenuated by administration of a TLR4 inhibitor, CLI-095. In addition, CLI-095 also inhibits 7KCh induced angiogenesis in vivo (115).
Sodium iodate (NaIO3), a potent oxidizing agent, was shown to activate the complement alternative pathway causing degradation of photoreceptors (88), RPE fragmentation, deposition of complement C3 fragments and induction of TLR4 mRNA in wild-type mice (116). In addition, TLR2 deficient mice following NaIO3 exposure reduced the RPE degeneration and preserved ONL layers (88). Overall, this suggests that TLR2 plays an important role in oxidative damage in the retina in response to NaIO3.
Activation of the TLR-3 receptor by poly I:C during paraquat-induced oxidative stress modifies murine photoreceptor (117) and murine RPE (118) cell viability in a STAT3-dependent manner (117, 118).
In a co-culture of murine photoreceptor cells and Muller cells exposed to H202 induced oxidative stress, the activation of TLR4 receptor with LPS has been shown to further increase the photoreceptor loss more than when compared to H202 alone treatment (119). On the other hand, the Wnt3a treatment showed protective effect against H202 induced photoreceptor loss. If Wnt3a is incubated along with LPS in an H202 induced oxidative stress model, the protective effect of Wnt3a appears to be lost. TLR4 activation is thought to regulate the Wnt3a via decreased phosphorylation of the Wnt receptor LRP6 (119).
With age, the ability to process extracellular debris is lost, accumulation of extracellular debris can contribute to increased photo-oxidative stress mediated via TLR dependent pathways.
2.2.2.3 Inflammatory Pathways
ARPE-19 cells exposed to poly I:C was shown to increase the activation of TLR3 signalling and RelA production. Notably, repeated exposure with poly I:C increases RelA levels above that induced by the initial exposure (120). It is thought that the initial poly I:C exposure primes ARPE-19 cells to produce higher amounts of RelA. Activation of TLR3 regulates RelA levels via TBK1/IKKϵ and JAK/STAT pathways (120). Poly I:C treatment also leads to increased expression of IL6, IL8, TNF-α, MCP1, ICAM-1 and VEGF in a dose-dependent manner (121). Activation of TLR3 on porcine RPE cells is also associated with a dose-dependent cellular apoptosis and VEGF release (106).
An amyloid protein, Aβ-42, has been detected in AMD drusen (122). Treatment of ARPE19 cells with Aβ-42 peptides increases IL-6, IL-8, IL-33 and VEGF expression (122) via the activation of TLR4/MyD88/NFκB signalling cascade, the effect of which is reduced by TLR4 inhibition (122).
Nuclear factor erythroid 2-related factor 2 and peroxisome proliferator-activated receptor-gamma coactivator 1-alpha (NFE2L2/PGC1α) are usually involved in the regulation of antioxidant production. Deficiency of this complex was shown to increase the accumulation of drusen-like extracellular material in sub-RPE region (102) with a significant increase noted in TLR3/TLR9 levels in mice retina (102). This was also associated with the increased levels of complement component C5a (102).
2.2.2.4 Choroidal Neovascularization
The laser-induced CNV area in mice retina is enlarged by the application of TLR2 agonists, Pam2CSK4 (123, 124) and Chlamydia pneumoniae antigen or zymosan (123). Administration of anti-VEGF antibodies reduced laser-induced CNV area whilst CCL2 deletion increases the lesion area (124). Chlamydia pneumoniae antigen has also been shown to induce IL6 and VEGF via activation of TLR4/MyD88 axis (123).
TLR2 is involved in the recruitment of murine leucocytes to CNV (124) and increased levels of pro-inflammatory cytokines (46). In addition, increased levels of TLR3 are also detected on RPE cells within human CNV membranes (125). Systemic administration of the TLR-2 agonist Pam2CSK4 (PAM) in CNV mouse model leads to enlargement of CNV size compared to mice treated with saline control (124). Inhibition of TLR2 signalling by intravitreal injection of anti-TLR2 antibodies in murine eyes has been shown to suppress CNV formation, this suppressive effect is enhanced when anti-VEGFR2 is co-administered with anti-TLR2 antibodies (46).
The expression of TLR2/3 mRNA and protein in serum mononuclear leucocytes cells of patients with neovascular AMD is higher compared to the healthy controls. When the serum mononuclear leucocytes from patients with neovascular AMD are exposed to the TLR-2 ligand, PGN, they produce higher levels of IL6 and IL8. In addition, IL6 is also elevated in leucocytes from nAMD patients when exposed to the TLR-3 ligand poly IC compared to leucocytes from healthy controls (126).
Stromal cell-derived factor (SDF-1), also known as CXCL12, participates in CNV via CXCR4 and CXCR7 (127). LPS induces CXCR4 and CXCR7 levels in a choroid-retinal endothelial cell line (Rf/6A), derived from rhesus monkeys, via the activation of TLR4 (127). The transcription of CXCR4 and CXCR7 was regulated by the phosphorylation of ERK 1/2 and NFκB (127).
CNV is a defining feature of wet age-related macular degeneration. It has been shown to be influenced by activation of TLR-2 signalling pathway.
2.2.3 Toll-Like Receptor Genetic Polymorphisms in AMD
There are several studies into the association of TLR genetic polymorphisms with AMD (Table 3). There is a degree of inconsistency in the literature, with different studies reporting different results. This may be accounted for by the variation in ethnic origin of the patients involved in the studies.
2.2.3.1 TLR4
TLR4 is a prominent mediator of inflammatory pathways and regulator of cholesterol efflux through its stimulation by LPS which is regulated by APOE and ATP-binding cassette transport-1 (ABCA1) (48). Furthermore, altered binding of DAMP or changes in TLR related signal transduction pathways could lead to a loss of the immune privilege of the choroid/outer retina leading to subsequent accumulation of extracellularly deposited materials.
A cohort of 667 patients with AMD and 439 healthy controls were investigated. The frequency of the G allele in the TLR4-D299G polymorphism was higher in patients with AMD compared to the control (P = 0.001, age/sex-adjusted OR = 2.42, 95% CI = 1.43-4.08) (48). The frequency of the TLR4-T399I polymorphism was higher in patients with AMD compared to the control (P = 0.003, age/sex-adjusted OR = 2.37, 95% CI 1.33-4.22) (48). However, when both polymorphisms were included in a multiple logistic regression model, only the TLR4—D299G G allele was associated with a significant risk of AMD (P = 0.025, OR = 2.65, 95% CI = 1.13-6.25) (48).
A cohort of 120 patients with AMD was compared to 120 healthy controls. The TLR4-D299G and TLR4-T399I polymorphisms were not found to be associated with a significant risk of AMD (128).
A cohort of 368 patients with AMD and 368 healthy controls in the Netherlands were investigated, a significant association between D299G, T399I and K354K TLR4 polymorphisms and the risk of AMD was not found (129). The same article also investigated a cohort of 357 patients with AMD against 173 healthy controls from the United States, a significant association between AMD risk and the D299G, T399I and K354K TLR4 polymorphisms were not found (129).
A combined study based on three cohorts totalling 687 patients with AMD and 936 healthy controls was performed. No association was found between TLR4-rs4986790 in the three independent cohorts individually or combined (132).
The study by Guven et al. (136) included a cohort of 183 patients with AMD and were compared to 200 healthy control subjects. A significant association was not found between the risk of AMD and the TLR4-Asp299Gly and TLR4-Thr399Ile genotypes (136).
Ling and Xiong (25) investigated TLR associations in 138 patients with AMD and 146 healthy control subjects. No significant difference between the frequency of TLR4-rs1927914 in patients with AMD compared to the healthy control subjects (25) were found. There was a significant difference in the CC genotype of TLR4-rs1927914 between AMD patients and healthy controls with the CC genotype and allele appearing to be protective AMD (P = 0.010 OR= 0.358, 95% CI=0.162–0.791), (P = 0.039, OR = 0.698, CI = 0.497-0.983) (25).
A meta-analysis of the association between TLR4 polymorphisms and AMD risk was performed. It revealed an association between the TLR4-rs4986790 polymorphism and AMD in a heterozygote and dominant model (AG vs. AA, OR = 1.400, 95%CI = 1.049–1.867, P = .022), (GG+AG vs. AA, OR = 1.365, 95%CI = 1.028–1.813, P = .032) respectively (100). No association was found between the TLR4- rs4986791 polymorphism and AMD risk (100).
2.2.3.2 TLR3
A multistage study was performed involving three cohorts of patients. The first cohort, based in Dallas, USA, consisted of 396 patients suffering from AMD against 181 controls; 10 polymorphisms across TLRs 1,3-7,10 were identified, however, only the TLR3 polymorphism (rs3775291 L412F, P = 0.01) and TLR7 polymorphism (rs12663316 Xp22.3, P = 0.02) were associated with AMD (130), these were found to not be significant after correction for multiple testing was applied (130). The TLR3-L412F and TLR7- Xp22 polymorphisms were investigated in two other cohorts no significant association was found (130, 131).
TLR3 polymorphisms were studied in a cohort of 825 patients with AMD and compared to 359 healthy control subjects (131). The TLR3 polymorphisms rs5743303 and rs3775291 were investigated, and the risk of GA, CNV and soft drusen development was analysed. No significant association between TLR3- rs5743303 and AMD was found. A significant association between the ‘T’ allele of rs3775291 and a lower risk of geographic atrophy was identified (p=0.005, additive allele-dosage model, Orhet=0.712, 95% CI 0.503 -1.00; Orhom=0.437, 95% CI 0.227-0.839 (131). These results were replicated in a cohort of 450 patients with AMD, and 421 controls, where a significant association between rs3775291 and geographic atrophy (p=0.000543) (131) was identified. A second replication on a cohort of patients from AREDS consisting of 184 patients with AMD and 134 healthy controls further confirmed the relationship between rs3775291 and GA (p=0.002). A combined analysis of the three cohorts yielded an association (p= 0.000000124, FDR adjusted) between rs3775291 and GA, which was highly significant (131).
A combined study based on three cohorts totalling 687 patients with AMD and 936 healthy controls was performed. No association was found between TLR3-rs3775291 in the three independent cohorts individually (132).
In a cohort of 114 AMD patients and 448 healthy control subjects, no significant association between the risk of AMD and the TLR4- rs3775291 polymorphism was found (genotypic OR = 1.55, CI = 0.77-1.67, genotypic P = 0.149, allelic OR = 1.22, CI 0.896-1.67, allelic P = 0.233) (96).
Similarly, a cohort of 126 patients with CNV and 120 patients with polypoidal choroidal vasculopathy (PCV) was compared to 274 healthy controls, and no significant association between the TLR3-rs3775291 polymorphism and CNV in AMD was found genotypic p = 0.698, allelic = 0.5847) (133).
Cheng et al. (134) included 96 patients with AMD and 96 healthy control subjects in a study where the TLR3 gene was sequenced in its entirety, revealing 6 polymorphisms: rs5743303, rs5743305, rs5743312, rs3775291, rs3775290, and rs6830345. No significant difference was found between the distribution of TLR3 polymorphisms in the AMD cases versus the control group (134).
Sharma et al. (135) compared TLR3 polymorphisms in a cohort of 115 patients with AMD were compared to 61 healthy controls. No significant difference was found between the distribution of TLR3-rs3775291 GG or AG genotypes and AMD (OR value = 0.093, p = 0.112, CI = 0.005–0.1.73, OR = 0.163 and p = 0.222, CI = 0.009–2.99, respectively) (135).
A meta-analysis of the association between TLR3-rs3775291 polymorphism and AMD risk showed that the T allele of the TLR3-rs3775291 polymorphism was associated with a reduced risk of GA (p = 0.04, OR = 0.78, 95% CI: 0.62-0.98) (137). TLR3-rs3775291 was also associated with neovascular AMD (p = 0.01, OR = 0.78, 95% CI: 0.64–0.94) (137), and overall associated with AMD in a recessive model (p = 0.03, OR = 0.88, 95% CI: 0.79–0.99).
The TLR3-rs3775291 polymorphism does not appear to affect the mRNA, surface expression or protein of TLR3 (103), but the binding capacity of the polymorphism is 51.12 ± 3.96% (P<0.001) (103) compared to the wild type; subsequently reduced expression of TLR3 mediated NF-kB may explain the reduced risk of developing GA with this polymorphism.
2.2.3.3 Other TLRs
A cohort of 183 patients with AMD was compared to 200 healthy control subjects. The TLR2-Arg753Gln genotype was associated with a significant risk of developing AMD (OR= 3.88; 95% CI: 1.76–8.75, p = 0.001) (136).
2.3 Ischaemic Retinopathy
2.3.1 Background Information
Ischaemia is caused by the reduced blood supply to a localized area due to the obstruction of blood vessels, which subsequently leads to cellular hypoxia and eventual cell death (138). In the retina, ischaemia leads to neuronal cell death, activation of glial cells, and release of cytotoxic intercellular mediators (138).
Retinal ischaemia occurs in various conditions such as diabetic retinopathy, retinal vein/artery occlusion, and ocular ischaemic syndrome, which can lead to subsequent pathological blood vessel formation, otherwise known as neovascularization. Neovascularization is linked to the initiation of inflammatory pathways by the production and release of proangiogenic cellular mediators by TLRs through induction of angiogenic factors such as VEGF (139). Following a period of retinal ischaemia, restoration of blood supply may be associated with reperfusion injury, due to exhaustion of intracellular energy stores and subsequent mitochondrial dysfunction causing the production of large amounts of ROS. Reperfusion of ischaemic tissue can lead to increased levels of these factors resulting in retinal damage (140).
The second most common retinal vascular disease (after diabetic retinopathy) is retinal vein occlusion, which may be either central (CRVO) or branched (BRVO) depending on the site of the occlusion.
2.3.2 The Role of TLRs in Retinal Ischaemia and Neovascularization
Several animal models have been used to simulate retinal ischaemia. These include timed elevation of intraocular pressure, followed by reperfusion (138). Other models of retinal ischaemia include clipping retinal vessels for 30 minutes and then subsequently removing the clip (13).
2.3.2.1 Inflammatory Pathways
Raised levels of heat shock protein 70 (Hsp70) have been detected in the vitreous humour of mice exposed to retinal ischaemia-reperfusion injury (141). Extracellular Hsp70 was associated with RGC death in vitro, which was reduced on the administration of a protein kinase C (PKC) inhibitor in a glial-RGC co-culture (141). This indicates that the high levels of extracellular HSP70 induce a pro-inflammatory response when PKC is simultaneously activated. This was mediated via the TLR4-MyD88 pathway leading to TNF release (141).
HMGB1 is a nuclear protein present in the nucleus of most cells (142). It is secreted by macrophages, natural killer cells, and mature dendritic cells in response to cellular distress or injury (79, 142). HMGB1 levels are raised following the ischaemic-reperfusion (IR) injury (142) and accumulate in the vitreous humour (143).
HMGB1 inhibition (143) in murine RGCs or gene deletion (142) reduces IR induced retinal damage whilst the direct administration of recombinant HMGB1 increases the loss of RGCs in retina (143). HMGB1 initiates inflammatory pathways mainly via TLR4 activation (142, 143).
Microglial cell recruitment and subsequent inflammatory cytokine release is initiated by activation of TLRs. TLR-1 to -3 are elevated (138, 139, 144) in the mouse retina and enhances TLR3-dependent microglial cells recruitment following IR injury. Besides, TLR3 activation also increases the inflammatory cytokines such as IL-6, IL-1b, TNFa, TGFB in mouse retina following IR injury (138). Ischaemia increases caspase-3 reactivity in the ganglion cell layer (GCL) of mouse retina (138), which was mainly induced via TLR4-mediated proinflammatory cytokines (138).
Models of oxygen-induced retinopathy (OIR) in rats have been shown to increase the levels of TLR3 (145) and NF-κB. OIR induced TLR3 expression was significantly upregulated up on the administration of poly I:C (145). In response to OIR, increased levels of TLR2 and VEGF were observed in wild-type mice, whilst in mice deficient in TLR2, the levels of TGF-b, b-FGF and IL-6 were significantly reduced (139).
TLR4 levels are raised in response to retinal IR injury (13, 140, 142, 146–149) and hypoxia (7, 150). In particular, TLR4 levels are highly expressed in the INL (13, 146) and GCL (13, 142, 146) following I/R injury. TLR4 deficiency is associated with increased cell survival in the GCL and thickness of the INL following I/R injury (146, 151). I/R injury induces increased levels of tyrosine-protein kinase, Syk (SYK) and phosphorylated-Syk (146), in mice retina. This effect is lost in TLR4 KO mice treated with an SYK inhibitor, piceatannol, reducing I/R induced retinal damage (146).
TLR4 deficient mice develop reduced neovascularization (142) with preservation of normal vessel architecture in response to retinal I/R injury. This is associated with the increased secretion of TLR4-dependent inflammatory factors such as VEGF, NF-kB, b-FGF, TGF- β1, IL6 and IL-1β (142).
NLRP1 and NLRP3 play an essential role in the damage associated with retinal I/R injury. They are released in response to ischaemia and induce the release of IL-1β and IL18 (13). Ischaemia-induced NLRP3 is reduced by the administration of a TLR4 monoclonal antibody (clone HTA125) (13) and puerarin (149), which partially halts the development of retinal I/R injury.
Treatment of in vitro human retinal microvascular endothelial cells (HRMEC) with a TLR4 antagonist, TAK-242, reduces MAPK4-mediated inflammatory responses (148). Administration of TAK-242 in an OIR model has been shown to reduce the area of OIR avascularity, attenuate neovascularization, and increases vascular density (148). Furthermore, TAK-242 and anti-VEGF treatment have been shown to improve the vascular density and reduce aberrant angiogenesis in OIR mice (148).
I/R injury is characterised by the thickening of retinal layers due to oedema and disordered cell arrangement. Inhibition of TLR4 using N-acetyl serotonin (NAS) or TAK-242 (140) reduces the retinal thickening and pro-inflammatory cytokines, such as IL-1β in I/R (140).
Both CRVO and BRVO are associated with the elevated systemic levels of pro-inflammatory molecule, heparinase (152). Serum heparinase level and activity have been correlated with serum TLR4 and TLR2 levels (152).
MD2, a TLR4 co-adaptor molecule, plays a crucial role in the response to retinal I/R injury by forming an NADPH oxidase 4 (NOX4)-MD2-TLR4 complex. I/R stimulated release of HMGB1 activates downstream pathways which increases the intracellular ROS levels leading to cellular apoptosis (153). Inhibition of MD2 by L2H17 (154) reduces I/R injury by reducing apoptosis and suppression of oxidative stress-induced RPE cell death (154). In addition, MD2 inhibition reduces inflammatory injury during I/R through suppression of TBHP induced activation of the TLR4 pathways (154).
Retinal ischaemia triggers the release of various DAMPs such as HSP70 and HMGB1, these are known to trigger cellular apoptosis via TLR mediated pathways (Figure 5). Retinal ischaemia has also been shown to induce increased levels of TLRs and there is a substantial amount of evidence showing an association between TLR4 levels and retinal ischemia. Retinal neovascularization following ischaemic injury is also influenced by TLR mediated pathways.
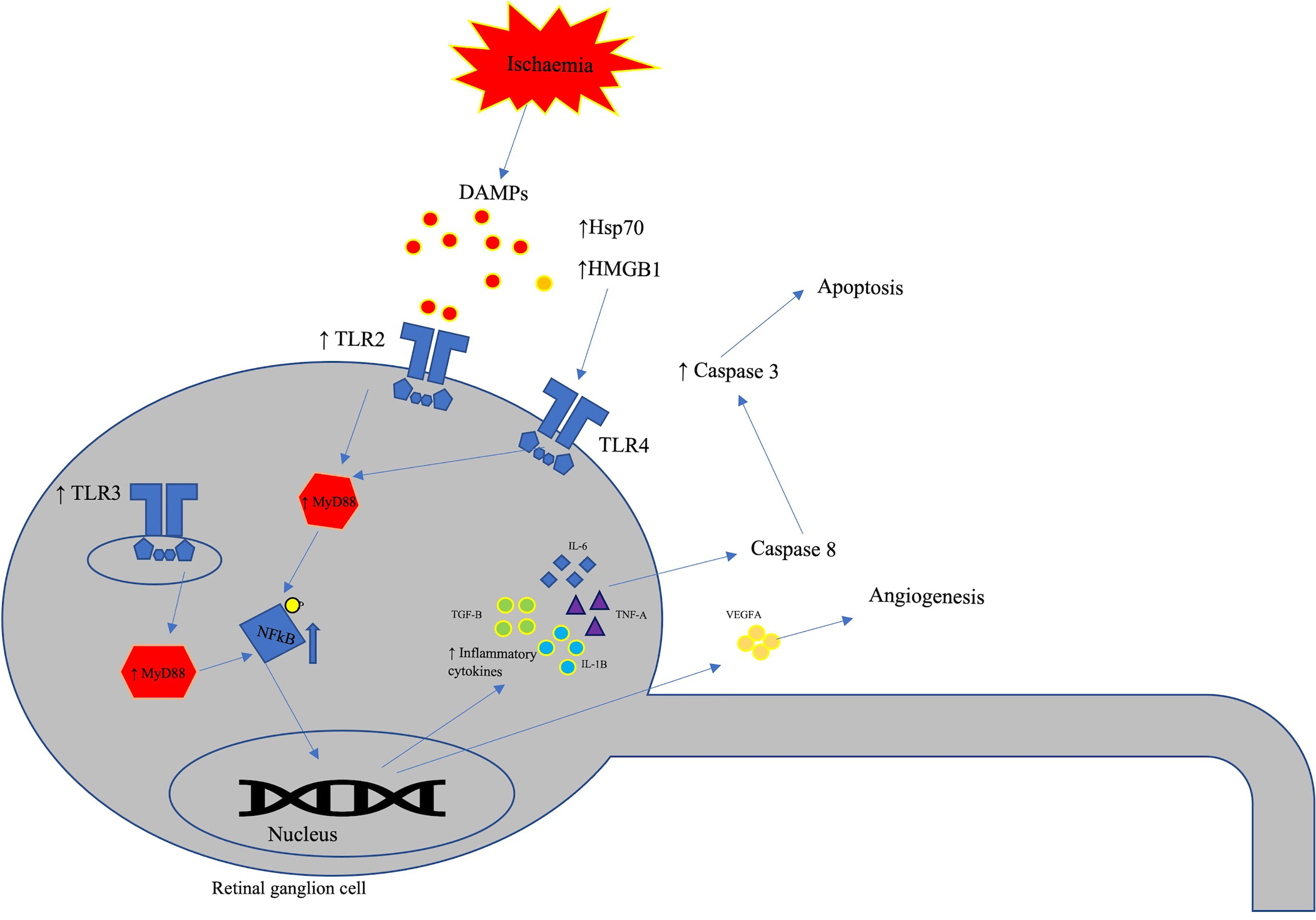
Figure 5 Schematic representation of TLRs involvement in retinal ischaemia. In response to retinal ischaemia, various damage associated molecular patterns (DAMPs) such as HSP70 and HMGB1 induces inflammatory response via the TLR4-MyD88 pathway. HMGB1 binds to TLR4 receptors leading to retinal ganglion cell loss and activation of inflammatory pathways. Retinal ischaemia also elevates the levels of TLR-1 to -3 in retinal ganglion cells leading to pro-inflammatory tissue damage.
2.4 Retinal Dystrophies
2.4.1 Retinitis Pigmentosa
Retinitis pigmentosa (RP) represents a group of retinal dystrophies with genetic inheritance. They are a frequent cause of hereditary blindness, affecting approximately 2 million people worldwide (155). RP consists of a wide range of heterogeneous conditions resulting from >3000 mutations affecting more than 60 individual genes (155). The different RP mutations have similar pathological characteristics, primarily a physiological dysfunction of photoreceptors, leading to their death. The continued loss of photoreceptor cells leads to continued stimulation of microglial proliferation, sterile inflammation, and further disease progression. Unfortunately, there is no identified treatment for RP, although gene therapy has been proposed as a potential management option.
As TLRs are a crucial component of the innate immune response and initiation of inflammatory pathways, their relevance in the sterile inflammatory pathways of late RP is important.
2.4.1.1 TLRs in Photoreceptor Survival
In an RP model based on Pde6b (rd10) and RhoP23H/+ (P23H/+) background showed increased production of TLR2/MyD88 mediated IL-1β compared to wild type mice (155). Furthermore, RP mice with heterozygous TLR2 mutation were unable to improve photoreceptor survival. While those with TLR2 deficiency showed improved visual function and photoreceptor survival (155).
In a mouse model of inherited retinal degeneration, inhibition of MyD88 has been shown to reduce the photoreceptor apoptosis and functional loss (156). MyD88 inhibition also reduces microglia/macrophage infiltration in the neurosensory retina (156). Retinol dehydrogenase 8 (RDH8) and ATP-binding cassette transporter 4 (ABCA4) deficient mice that develop cone-rod dystrophy (CORD) showed increased expression of TLR signalling elements (157). ABCA4 and RDH8 deficiency have been associated with the development of features of CORD and worsened by light exposure. Additionally, TLR3 deficient mice failed to exhibit the signs of retinal damage (157).
2.5 Uveitis
2.5.1 Background Information
Inflammation of the uvea, known as uveitis, can be triggered by infectious or non-infectious mechanisms. Uveitis can manifest in various conditions such as Behcet disease, ankylosing spondylitis, Vogt-Koyanagi-Harada, amongst others.
A short overview of TLR associations of uveitis is included in this review because of the common association between posterior uveitis and retinal inflammation which is broadly manifested as chorioretinitis. Our initial search criteria did not include uveitis and TLRs in general per se, but we investigated the relationship between the conditions that include posterior uveitis as part of ‘chorioretinitis’.
2.5.2 TLRs in Uveitis
2.5.2.1 Inflammatory Pathways
Patients with retinal vasculitis secondary to Behcet disease and idiopathic uveitis showed elevated levels of type 1 IFN (IFN A/B) than healthy individuals (158). Furthermore, levels of adhesion molecules such as soluble intracellular adhesion molecule-1 (sICAM-1) and soluble E-selectin were higher in patients with retinal vasculitis compared to healthy individuals (158). An in vitro investigation showed that retinal endothelial cells produced sE-selectin, sICAM-1 and IFN-β in response to TLR3 activation by poly I:C (158), implying that TLR3 activation may play a role in raised inflammatory markers in Behcet disease and idiopathic uveitis.
2.5.2.2 Inherited Genetic Risk
A study was performed on the relationship between copy-number variants in the TLRs and various uveitis-related conditions, which included 400 patients with Vogt-Koyanagi-Harada syndrome, 400 Bechet disease patients, 400 patients with acute anterior uveitis and 600 healthy patients (159). The frequency of copy number variations in TLR1-3, TLR5-7 and TLR9-10 were compared between the patient groups, where only TLR7 was shown to have variation (159). One copy of TLR7 had a significantly increased frequency in male patients (p = 0.021) with Behcet disease, and two copies in female patients (p = 0.048). This result was confirmed in a second study with 587 Behcet’s disease patients compared to 1000 healthy control subjects (159) as a single copy in males (p = 1.14 x 10-6) and females (p = 9.12 x 10-5) (159). No association was found between TLR7 copy number variants and VKH or AAU.
Another study reported by (160) included 400 patients with Behcet’s, 400 patients with VKH, 400 patients with acute anterior uveitis, 400 patients with paediatric uveitis and 600 healthy controls, investigated the relationship between TLR2, TLR4, TLR8 and TLR9 (160). Behcet disease was associated with a higher frequency of the TLR2-rs2289318 A and C allele (p=0.048, p = 0.008) and TLR2-rs3804099 CT genotype (p = 0.005). This association was confirmed in a second state study consisting of 438 Behcet disease patients and 1000 healthy subjects; TLR2-rs2289318 A and C allele (p=0.001, p = 6.89E-06) and TLR2-rs3804099 CT genotype (p = 2.426E-06).
The relationship between TLR9 polymorphisms rs352140, rs352139, and rs187084 and VKH was investigated in a population of 94 patients and 125 healthy controls (161) and reported no statistically significant association.
The existing literature suggests that TLR polymorphisms do not appear to play a role in VKH or acute anterior uveitis. However, TLR2-rs3804099 and TLR2-rs2289318 polymorphisms appear to contribute to the risk of developing Behcet disease in a cohort of Han Chinese patients. Furthermore, copy number variants in TLR7 also appear to be associated with an increased risk of Behcet’s disease, with at least one contributing to risk in males and two contributing to risk in females.
2.6 Potential Ligands
2.6.1 Tetramethylpyrazine
Chuanxiong, otherwise known as Ligusticum wallichii Franchat, is a Chinese herbal medicine containing the bioactive component 2,3,5,6 tetramethylpyrazine (TMP). It is used in traditional Chinese medicine to treat various ocular diseases such as glaucoma, DR, and AMD, acting through an unknown mechanism.
TMP pre-treatment has been shown to inhibit CD68 upregulation induced by LPS (162). TMP has been shown to inhibit endotoxin (lipopolysaccharide)-induced retinal inflammation (TNF-α, IL-6 and IL-1β) in rat retinal microglial cells via inhibition of the TLR4/NF-kB pathway (162).
2.6.2 Puerarin
Puerarin, an isoflavone glycoside, is the bioactive component of the traditional Chinese herb known as Pueraria. Puerarin has been shown to have a protective role in DR and is thought to do so by attenuation of the NLRP3 inflammasome stimulation through inhibition of AB1-40 (149). In I/R injury, the administration of puerarin reduced the degeneration of rat RGC and attenuated the oxidative stress in vivo. This was thought to be mediated through the suppression of the TLR4/NLRP3 crosstalk mechanisms (149).
2.6.3 Progranulin
Progranulin is a growth factor that regulates inflammatory responses, axonal growth and nerve cell survival (150). Cobalt chloride-induced retinal hypoxia in mice retina is associated with raised levels of HIF-α, VEGF, TLR4, and NOX4 (150). Ischaemia induced inflammation was attenuated by progranulin administration through a reduction of leukocyte adhesion and down-regulation of the TLR4-NOX4 signalling pathway (150).
2.6.4 Apocynin
Apocynin is a methoxy-substituted catechol derived from Picrorhiza kurroa, which primarily inhibits NADPH oxidase dependent oxidative stress (163). Apocynin reduces hyperglycaemia induced retinal apoptosis, restores retinal morphology, and reduces oxidative stress through what was presumed to be via TLR4-NF-kB signalling axis (163).
2.6.5 Gastrodin
Gastrodin, also known as 4-Hydroxybenzyl alcohol 4-O-beta-D-glucopyranoside, is the active component of the Chinese herb Gastrodia elata Blume (81), which has been previously shown to have anti-apoptotic and anti-inflammatory effects. Gastrodin inhibits hyperglycaemia induced HREC apoptosis by regulation of SIRT-1 induced inhibition of TLR4 signalling mechanisms (81).
2.6.6 Paeoniflorin
Paeoniflorin is a monoterpene glucoside derived from the root of Paeonia lactiflora plant. It has been shown to exhibit immunomodulatory effects on microglial cells (84). High glucose has been shown to induce MMP-9 via HMGB1/TLR4 signalling in BV2 microglial cells (84). Hyperglycaemia induced MMP-9 is inhibited by the administration of paeoniflorin via induction of SOCS3 (negative regulator of TLR signalling cascade), leading to inhibition of TLR4 signalling (84).
2.6.7 Wogonin
Wogonin, also known as 7−dihydroxy−8−methoxyflavone, is the active ingredient isolated from the roots of the Scutellaria baicalensis Georgi plant, commonly referred to as Huang-Qin (164). Administration of Wogonin to ARPE-19 cells has been shown to reduce LPS/TLR4 induced inflammatory mediators such as IL-1β, IL-8, IL-6, COX-2, iNOS and TNF-α (164).
3 Conclusions
Over the past two decades, there has been a great deal of progress in the understanding of TLRs in general, and their role in the eye, especially retinal diseases.
TLRs contribute to inflammatory pathways that occur in ischaemic retinal diseases, DR, and AMD. There appears to be a relationship between genetic polymorphisms and the risk of developing DR and AMD. In particular, the TLR3- Asp299Gly with DR, and TLR4-rs4986790/TLR3-rs3775291 with AMD associations have been documented. However, the full extent of these relationships remains unclear and require further research that includes larger and different population groups. There is only limited research on TLR associations in inherited retinal degenerations, although inhibition of the TLR/MyD88 axis has been shown to improve the cell survival. Furthermore, it is important to consider that cell-lines and in particular non-human cell lines have limitations when extrapolated to other situations.
Most retinal diseases with described TLR association are non-infectious. It is, therefore, likely that the DAMP release from tissue damage due to drusen and associated molecular changes in AMD, or hyperglycaemic and associate conditions need to be closely evaluated for further clues.
Several studies have suggested that different substances may potentially reduce inflammation/apoptosis mediated via the TLRs. A greater understanding of TLR mechanisms in retinal diseases will allow us to identify potential new molecular targets for the treatment and diagnosis of these conditions. Identification of TLR modifiers or biomarkers (e.g., HMGB1 or DAMPs) in the vitreous and/or retinal tissue of patients with retinal disease will provide essential cues to develop selective or targeted inhibitors against them. Combining anti-VEGF therapies with TLR inhibition may provide a longer-lasting treatment in retinal vascular disease (such as neovascular AMD and DR) compared to anti-VEGF blockage alone.
Author Contributions
All authors have made significant contributions to the manuscript. Conception and design: WA and IM; acquisition, analysis and interpretation of data: OT-L, IM, and WA; drafting and finalisation of manuscript: OT-L, IM, WA; final approval:OT-L, IM, WA.
Author Disclaimer
The views expressed in this article are of the author and do not necessarily reflect the position or policy of the institutions they represent.
Conflict of Interest
The authors declare that the research was conducted in the absence of any commercial or financial relationships that could be construed as a potential conflict of interest.
Publisher’s Note
All claims expressed in this article are solely those of the authors and do not necessarily represent those of their affiliated organizations, or those of the publisher, the editors and the reviewers. Any product that may be evaluated in this article, or claim that may be made by its manufacturer, is not guaranteed or endorsed by the publisher.
References
1. Wong WL, Su X, Li X, Cheung CM, Klein R, Cheng CY, et al. Global Prevalence of Age-Related Macular Degeneration and Disease Burden Projection for 2020 and 2040: A Systematic Review and Meta-Analysis. Lancet Glob Health (2014) 2(2):e106–116. doi: 10.1016/S2214-109X(13)70145-1
2. Ting DS, Tan KA, Phua V, Tan GS, Wong CW, Wong TY. Biomarkers of Diabetic Retinopathy. Curr Diabetes Rep (2016) 16(12):125. doi: 10.1007/s11892-016-0812-9
3. Mitchell P, Liew G, Gopinath B, Wong TY. Age-Related Macular Degeneration. Lancet (2018) 392(10153):1147–59. doi: 10.1016/S0140-6736(18)31550-2
4. Yeo NJY, Chan EJJ, Cheung C. Choroidal Neovascularization: Mechanisms of Endothelial Dysfunction. Front Pharmacol (2019) 10:1363. doi: 10.3389/fphar.2019.01363
5. Hageman GS, Luthert PJ, Victor Chong NH, Johnson LV, Anderson DH, Mullins RF. An Integrated Hypothesis That Considers Drusen as Biomarkers of Immune-Mediated Processes at the RPE-Bruch’s Membrane Interface in Aging and Age-Related Macular Degeneration. Prog Retin Eye Res (2001) 20(6):705–32. doi: 10.1016/s1350-9462(01)00010-6
6. Blasiak J, Petrovski G, Vereb Z, Facsko A, Kaarniranta K. Oxidative Stress, Hypoxia, and Autophagy in the Neovascular Processes of Age-Related Macular Degeneration. BioMed Res Int (2014) 2014:768026. doi: 10.1155/2014/768026
7. Akhtar R, Tahir H, Stewart E, Wei R, Mohammed I, Amoaku WM. Toll-Like Receptor Signalling Pathways Regulate Hypoxic Stress Induced Fibroblast Growth Factor But Not Vascular Endothelial Growth Factor-a in Human Microvascular Endothelial Cells. Int J Trans Med (2021) 1(1):25–38. doi: 10.3390/ijtm1010003
8. Xu WQ, Wang YS. The Role of Toll-Like Receptors in Retinal Ischemic Diseases. Int J Ophthalmol (2016) 9(9):1343–51. doi: 10.18240/ijo.2016.09.19
9. Mulfaul K, Rhatigan M, Doyle S. Toll-Like Receptors and Age-Related Macular Degeneration. Adv Exp Med Biol (2018) 1074:19–28. doi: 10.1007/978-3-319-75402-4_3
10. O’Neill LA. When Signaling Pathways Collide: Positive and Negative Regulation of Toll-Like Receptor Signal Transduction. Immunity (2008) 29(1):12–20. doi: 10.1016/j.immuni.2008.06.004
11. Lin Q, Li M, Fang D, Fang J, Su SB. The Essential Roles of Toll-Like Receptor Signaling Pathways in Sterile Inflammatory Diseases. Int Immunopharmacol (2011) 11(10):1422–32. doi: 10.1016/j.intimp.2011.04.026
12. Tang D, Kang R, Coyne CB, Zeh HJ, Lotze MT. Pamps and Damps: Signal 0s That Spur Autophagy and Immunity. Immunol Rev (2012) 249(1):158–75. doi: 10.1111/j.1600-065X.2012.01146.x
13. Qi Y, Zhao M, Bai Y, Huang L, Yu W, Bian Z, et al. Retinal Ischemia/Reperfusion Injury Is Mediated by Toll-Like Receptor 4 Activation of NLRP3 Inflammasomes. Invest Ophthalmol Vis Sci (2014) 55(9):5466–75. doi: 10.1167/iovs.14-14380
14. Redfern RL, McDermott AM. Toll-Like Receptors in Ocular Surface Disease. Exp Eye Res (2010) 90(6):679–87. doi: 10.1016/j.exer.2010.03.012
15. Mohammed I, Abedin A, Tsintzas K, Abedin SA, Otri AM, Hopkinson A, et al. Increased Expression of Hepcidin and Toll-Like Receptors 8 and 10 in Viral Keratitis. Cornea (2011) 30(8):899–904. doi: 10.1097/ICO.0b013e31820126e5
16. Mohammed I, Said DG, Dua HS. Human Antimicrobial Peptides in Ocular Surface Defense. Prog Retin Eye Res (2017) 61:1–22. doi: 10.1016/j.preteyeres.2017.03.004
17. Stewart EA, Wei R, Branch MJ, Sidney LE, Amoaku WM. Expression of Toll-Like Receptors in Human Retinal and Choroidal Vascular Endothelial Cells. Exp Eye Res (2015) 138:114–23. doi: 10.1016/j.exer.2015.06.012
18. Kumar MV, Nagineni CN, Chin MS, Hooks JJ, Detrick B. Innate Immunity in the Retina: Toll-Like Receptor (TLR) Signaling in Human Retinal Pigment Epithelial Cells. J Neuroimmunol (2004) 153(1-2):7–15. doi: 10.1016/j.jneuroim.2004.04.018
19. Ambati J. Age-Related Macular Degeneration and the Other Double Helix. The Cogan Lecture. Invest Ophthalmol Vis Sci (2011) 52(5):2165–9. doi: 10.1167/iovs.11-7328
20. Nusslein-Volhard C, Wieschaus E. Mutations Affecting Segment Number and Polarity in Drosophila. Nature (1980) 287(5785):795–801. doi: 10.1038/287795a0
21. Lemaitre B, Nicolas E, Michaut L, Reichhart JM, Hoffmann JA. The Dorsoventral Regulatory Gene Cassette Spatzle/Toll/Cactus Controls the Potent Antifungal Response in Drosophila Adults. Cell (1996) 86(6):973–83. doi: 10.1016/s0092-8674(00)80172-5
22. O’Neill LA. The Interleukin-1 Receptor/Toll-Like Receptor Superfamily: 10 Years of Progress. Immunol Rev (2008) 226:10–8. doi: 10.1111/j.1600-065X.2008.00701.x
23. Medzhitov R, Janeway C Jr. The Toll Receptor Family and Microbial Recognition. Trends Microbiol (2000) 8(10):452–6. doi: 10.1016/s0966-842x(00)01845-x
24. Jin MS, Kim SE, Heo JY, Lee ME, Kim HM, Paik SG, et al. Crystal Structure of the TLR1-TLR2 Heterodimer Induced by Binding of a Tri-Acylated Lipopeptide. Cell (2007) 130(6):1071–82. doi: 10.1016/j.cell.2007.09.008
25. Ling Y, Xiong F. Associations of TLR4 Gene Polymorphisms With the Risk of Age-Related Macular Degeneration in a Chinese Han Population. Med (Baltimore) (2019) 98(19):e15583. doi: 10.1097/MD.0000000000015583
26. Chang JH, McCluskey PJ, Wakefield D. Toll-Like Receptors in Ocular Immunity and the Immunopathogenesis of Inflammatory Eye Disease. Br J Ophthalmol (2006) 90(1):103–8. doi: 10.1136/bjo.2005.072686
27. Lim HK, Seppänen M, Hautala T, Ciancanelli MJ, Itan Y, Lafaille FG, et al. TLR3 Deficiency in Herpes Simplex Encephalitis: High Allelic Heterogeneity and Recurrence Risk. Neurology (2014) 83(21):1888–97. doi: 10.1212/wnl.0000000000000999
28. Poltorak A, He X, Smirnova I, Liu MY, Van Huffel C, Du X, et al. Defective LPS Signaling in C3H/Hej and C57BL/10sccr Mice: Mutations in Tlr4 Gene. Science (1998) 282(5396):2085–8. doi: 10.1126/science.282.5396.2085
29. Gewirtz AT, Navas TA, Lyons S, Godowski PJ, Madara JL. Cutting Edge: Bacterial Flagellin Activates Basolaterally Expressed TLR5 to Induce Epithelial Proinflammatory Gene Expression. J Immunol (2001) 167(4):1882–5. doi: 10.4049/jimmunol.167.4.1882
30. Lund JM, Alexopoulou L, Sato A, Karow M, Adams NC, Gale NW, et al. Recognition of Single-Stranded RNA Viruses by Toll-Like Receptor 7. Proc Natl Acad Sci USA (2004) 101(15):5598–603. doi: 10.1073/pnas.0400937101
31. Gorden KB, Gorski KS, Gibson SJ, Kedl RM, Kieper WC, Qiu X, et al. Synthetic TLR Agonists Reveal Functional Differences Between Human TLR7 and TLR8. J Immunol (2005) 174(3):1259–68. doi: 10.4049/jimmunol.174.3.1259
32. Kezic JM, McMenamin PG. Systemic Exposure to Cpg-ODN Elicits Low-Grade Inflammation in the Retina. Exp Eye Res (2019) 186:107708. doi: 10.1016/j.exer.2019.107708
33. Fore F, Indriputri C, Mamutse J, Nugraha J. TLR10 and Its Unique Anti-Inflammatory Properties and Potential Use as a Target in Therapeutics. Immune Netw (2020) 20(3):e21. doi: 10.4110/in.2020.20.e21
34. Kawasaki T, Kawai T. Toll-Like Receptor Signaling Pathways. Front Immunol (2014) 5:461(461). doi: 10.3389/fimmu.2014.00461
35. Sato Y, Goto Y, Narita N, Hoon DS. Cancer Cells Expressing Toll-Like Receptors and the Tumor Microenvironment. Cancer Microenviron (2009) 2 Suppl 1(Suppl 1):205–14. doi: 10.1007/s12307-009-0022-y
36. Akira S, Uematsu S, Takeuchi O. Pathogen Recognition and Innate Immunity. Cell (2006) 124(4):783–801. doi: 10.1016/j.cell.2006.02.015
37. Lee SM, Kok KH, Jaume M, Cheung TK, Yip TF, Lai JC, et al. Toll-Like Receptor 10 Is Involved in Induction of Innate Immune Responses to Influenza Virus Infection. Proc Natl Acad Sci USA (2014) 111(10):3793–8. doi: 10.1073/pnas.1324266111
38. Regan T, Nally K, Carmody R, Houston A, Shanahan F, Macsharry J, et al. Identification of TLR10 as a Key Mediator of the Inflammatory Response to Listeria Monocytogenes in Intestinal Epithelial Cells and Macrophages. J Immunol (2013) 191(12):6084–92. doi: 10.4049/jimmunol.1203245
39. Lin S-C, Lo Y-C, Wu H. Helical Assembly in the Myd88–IRAK4–IRAK2 Complex in TLR/IL-1R Signalling. Nature (2010) 465(7300):885–90. doi: 10.1038/nature09121
40. Ajibade AA, Wang HY, Wang RF. Cell Type-Specific Function of TAK1 in Innate Immune Signaling. Trends Immunol (2013) 34(7):307–16. doi: 10.1016/j.it.2013.03.007
41. Ninomiya-Tsuji J, Kishimoto K, Hiyama A, Inoue J, Cao Z, Matsumoto K. The Kinase TAK1 can Activate the NIK-I Kappab as Well as the MAP Kinase Cascade in the IL-1 Signalling Pathway. Nature (1999) 398(6724):252–6. doi: 10.1038/18465
42. Sakurai H, Suzuki S, Kawasaki N, Nakano H, Okazaki T, Chino A, et al. Tumor Necrosis Factor-Alpha-Induced IKK Phosphorylation of NF-Kappab P65 on Serine 536 Is Mediated Through the TRAF2, TRAF5, and TAK1 Signaling Pathway. J Biol Chem (2003) 278(38):36916–23. doi: 10.1074/jbc.M301598200
43. Hoebe K, Beutler B. TRAF3: A New Component of the TLR-Signaling Apparatus. Trends Mol Med (2006) 12(5):187–9. doi: 10.1016/j.molmed.2006.03.008
44. Landström M. The TAK1–TRAF6 Signalling Pathway. Int J Biochem Cell Biol (2010) 42(5):585–9. doi: 10.1016/j.biocel.2009.12.023
45. Ebihara N, Chen L, Tokura T, Ushio H, Iwatsu M, Murakami A. Distinct Functions Between Toll-Like Receptors 3 and 9 in Retinal Pigment Epithelial Cells. Ophthalmic Res (2007) 39(3):155–63. doi: 10.1159/000103235
46. Feng L, Ju M, Lee KYV, Mackey A, Evangelista M, Iwata D, et al. A Proinflammatory Function of Toll-Like Receptor 2 in the Retinal Pigment Epithelium as a Novel Target for Reducing Choroidal Neovascularization in Age-Related Macular Degeneration. Am J Pathol (2017) 187(10):2208–21. doi: 10.1016/j.ajpath.2017.06.015
47. Kindzelskii AL, Elner VM, Elner SG, Yang D, Hughes BA, Petty HR. Toll-Like Receptor 4 (TLR4) of Retinal Pigment Epithelial Cells Participates in Transmembrane Signaling in Response to Photoreceptor Outer Segments. J Gen Physiol (2004) 124(2):139–49. doi: 10.1085/jgp.200409062
48. Zareparsi S, Buraczynska M, Branham KE, Shah S, Eng D, Li M, et al. Toll-Like Receptor 4 Variant D299G Is Associated With Susceptibility to Age-Related Macular Degeneration. Hum Mol Genet (2005) 14(11):1449–55. doi: 10.1093/hmg/ddi154
49. Kumar A, Shamsuddin N. Retinal Muller Glia Initiate Innate Response to Infectious Stimuli via Toll-Like Receptor Signaling. PloS One (2012) 7(1):e29830. doi: 10.1371/journal.pone.0029830
50. Tu Z, Portillo JA, Howell S, Bu H, Subauste CS, Al-Ubaidi MR, et al. Photoreceptor Cells Constitutively Express Functional TLR4. J Neuroimmunol (2011) 230(1-2):183–7. doi: 10.1016/j.jneuroim.2010.07.022
51. Singh K, Kant S, Singh VK, Agrawal NK, Gupta SK, Singh K. Toll-Like Receptor 4 Polymorphisms and Their Haplotypes Modulate the Risk of Developing Diabetic Retinopathy in Type 2 Diabetes Patients. Mol Vis (2014) 20:704–13.
52. International Diabetes Federation. IDF Diabetes Atlas. 9th ed. Brussels, Belgium (2019). Available at: https://www.diabetesatlas.org.
53. Amoaku WM, Ghanchi F, Bailey C, Banerjee S, Banerjee S, Downey L, et al. Diabetic Retinopathy and Diabetic Macular Oedema Pathways and Management: UK Consensus Working Group. Eye (Lond) (2020) 34(Suppl 1):1–51. doi: 10.1038/s41433-020-0961-6
54. Yau JW, Rogers SL, Kawasaki R, Lamoureux EL, Kowalski JW, Bek T, et al. Global Prevalence and Major Risk Factors of Diabetic Retinopathy. Diabetes Care (2012) 35(3):556–64. doi: 10.2337/dc11-1909
55. Buraczynska M, Baranowicz-Gaszczyk I, Tarach J, Ksiazek A. Toll-Like Receptor 4 Gene Polymorphism and Early Onset of Diabetic Retinopathy in Patients With Type 2 Diabetes. Hum Immunol (2009) 70(2):121–4. doi: 10.1016/j.humimm.2008.12.003
56. Xu Y, Jiang Z, Huang J, Meng Q, Coh P, Tao L. The Association Between Toll-Like Receptor 4 Polymorphisms and Diabetic Retinopathy in Chinese Patients With Type 2 Diabetes. Br J Ophthalmol (2015) 99(9):1301–5. doi: 10.1136/bjophthalmol-2015-306677
57. Klein R, Klein BE, Moss SE, Davis MD, DeMets DL. The Wisconsin Epidemiologic Study of Diabetic Retinopathy. III. Prevalence and Risk of Diabetic Retinopathy When Age at Diagnosis Is 30 or More Years. Arch Ophthalmol (1984) 102(4):527–32. doi: 10.1001/archopht.1984.01040030405011
58. Stratton IM, Kohner EM, Aldington SJ, Turner RC, Holman RR, Manley SE, et al. UKPDS 50: Risk Factors for Incidence and Progression of Retinopathy in Type II Diabetes Over 6 Years From Diagnosis. Diabetologia (2001) 44(2):156–63. doi: 10.1007/s001250051594
59. UKPDS. Tight Blood Pressure Control and Risk of Macrovascular and Microvascular Complications in Type 2 Diabetes: UKPDS 38. UK Prospective Diabetes Study Group. BMJ (1998) 317(7160):703–13. doi: 10.1136/bmj.317.7160.703
60. Klein R, Marino EK, Kuller LH, Polak JF, Tracy RP, Gottdiener JS, et al. The Relation of Atherosclerotic Cardiovascular Disease to Retinopathy in People With Diabetes in the Cardiovascular Health Study. Br J Ophthalmol (2002) 86(1):84–90. doi: 10.1136/bjo.86.1.84
61. Klaassen I, Van Noorden CJ, Schlingemann RO. Molecular Basis of the Inner Blood-Retinal Barrier and Its Breakdown in Diabetic Macular Edema and Other Pathological Conditions. Prog Retin Eye Res (2013) 34:19–48. doi: 10.1016/j.preteyeres.2013.02.001
62. Antonetti DA, Barber AJ, Hollinger LA, Wolpert EB, Gardner TW. Vascular Endothelial Growth Factor Induces Rapid Phosphorylation of Tight Junction Proteins Occludin and Zonula Occluden 1. A Potential Mechanism for Vascular Permeability in Diabetic Retinopathy and Tumors. J Biol Chem (1999) 274(33):23463–7. doi: 10.1074/jbc.274.33.23463
63. Alomar SY, Barakat ,BM, Eldosoky M, Atef H, Mohamed AS, Elhawary R, et al. Protective Effect of Metformin on Rat Diabetic Retinopathy Involves Suppression of Toll-Like Receptor 4/Nuclear Factor-K B Expression and Glutamate Excitotoxicity. Int Immunopharmacol (2021) 90:107193. doi: 10.1016/j.intimp.2020.107193
64. Yuan S, Liu X, Zhu X, Qu Z, Gong Z, Li J, et al. The Role of TLR4 on PGC-1alpha-Mediated Oxidative Stress in Tubular Cell in Diabetic Kidney Disease. Oxid Med Cell Longev (2018) 2018:6296802. doi: 10.1155/2018/6296802
65. Chen XL, Zhang XD, Li YY, Chen XM, Tang DR, Ran RJ. Involvement of HMGB1 Mediated Signalling Pathway in Diabetic Retinopathy: Evidence From Type 2 Diabetic Rats and ARPE-19 Cells Under Diabetic Condition. Br J Ophthalmol (2013) 97(12):1598–603. doi: 10.1136/bjophthalmol-2013-303736
66. Zhao H, Zhang J, Yu J. HMGB-1 as a Potential Target for the Treatment of Diabetic Retinopathy. Med Sci Monit (2015) 21:3062–7. doi: 10.12659/MSM.894453
67. Berger EA, Carion TW, Jiang Y, Liu L, Chahine A, Walker RJ, et al. β-Adrenergic Receptor Agonist, Compound 49b, Inhibits TLR4 Signaling Pathway in Diabetic Retina. Immunol Cell Biol (2016) 94(7):656–61. doi: 10.1038/icb.2016.21
68. Zhao M, Li CH, Liu YL. Toll-Like Receptor (TLR)-2/4 Expression in Retinal Ganglion Cells in a High-Glucose Environment and Its Implications. Genet Mol Res (2016) 15(2):25. doi: 10.4238/gmr.15026998
69. Hu L, Yang H, Ai M, Jiang S. Inhibition of TLR4 Alleviates the Inflammation and Apoptosis of Retinal Ganglion Cells in High Glucose. Graefes Arch Clin Exp Ophthalmol (2017) 255(11):2199–210. doi: 10.1007/s00417-017-3772-0
70. Fu H, Liu H. Deletion of Toll-Like Receptor 4 Ameliorates Diabetic Retinopathy in Mice. Arch Physiol Biochem (2020) 1–7. doi: 10.1080/13813455.2020.1841795
71. Liu X, Zhang Y, Liang H, Zhang Y, Xu Y. Microrna-499-3p Inhibits Proliferation and Promotes Apoptosis of Retinal Cells in Diabetic Retinopathy Through Activation of the TLR4 Signaling Pathway by Targeting IFNA2. Gene (2020) 741:144539. doi: 10.1016/j.gene.2020.144539
72. Chen H, Yan T, Song Z, Ying S, Wu B, Ju X, et al. MD2 Blockade Prevents Modified LDL-Induced Retinal Injury in Diabetes by Suppressing NADPH Oxidase-4 Interaction With Toll-Like Receptor-4. Exp Mol Med (2021) 53(4):681–94. doi: 10.1038/s12276-021-00607-w
73. Madonna R, Balistreri CR, Geng YJ, De Caterina R. Diabetic Microangiopathy: Pathogenetic Insights and Novel Therapeutic Approaches. Vascul Pharmacol (2017) 90:1–7. doi: 10.1016/j.vph.2017.01.004
74. Liao YR, Li ZJ, Zeng P, Lan YQ. TLR7 Deficiency Contributes to Attenuated Diabetic Retinopathy via Inhibition of Inflammatory Response. Biochem Biophys Res Commun (2017) 493(2):1136–42. doi: 10.1016/j.bbrc.2017.08.085
75. Liu L, Jiang Y, Steinle J. Epac1 Regulates TLR4 Signaling in the Diabetic Retinal Vasculature. Cytokine (2021) 144:155576. doi: 10.1016/j.cyto.2021.155576
76. Tang J, Allen Lee C, Du Y, Sun Y, Pearlman E, Sheibani N, et al. Myd88-Dependent Pathways in Leukocytes Affect the Retina in Diabetes. PloS One (2013) 8(7):e68871. doi: 10.1371/journal.pone.0068871
77. Wang H, Shi H, Zhang J, Wang G, Zhang J, Jiang F, et al. Toll-Like Receptor 4 in Bone Marrow-Derived Cells Contributes to the Progression of Diabetic Retinopathy. Mediators Inflamm (2014) 2014:858763. doi: 10.1155/2014/858763
78. Lotze MT, Tracey KJ. High-Mobility Group Box 1 Protein (HMGB1): Nuclear Weapon in the Immune Arsenal. Nat Rev Immunol (2005) 5(4):331–42. doi: 10.1038/nri1594
79. Jiang Y, Steinle JJ. HMGB1 Inhibits Insulin Signalling Through TLR4 and RAGE in Human Retinal Endothelial Cells. Growth Factors (2018) 36(3-4):164–71. doi: 10.1080/08977194.2018.1539393
80. Hui Y, Yin Y. Microrna-145 Attenuates High Glucose-Induced Oxidative Stress and Inflammation in Retinal Endothelial Cells Through Regulating TLR4/NF-Kappab Signaling. Life Sci (2018) 207:212–8. doi: 10.1016/j.lfs.2018.06.005
81. Zhang TH, Huang CM, Gao X, Wang JW, Hao LL, Ji Q. Gastrodin Inhibits High Glucoseinduced Human Retinal Endothelial Cell Apoptosis by Regulating the SIRT1/TLR4/Nfkappabp65 Signaling Pathway. Mol Med Rep (2018) 17(6):7774–80. doi: 10.3892/mmr.2018.8841
82. Tang X, Dai Y, Wang X, Zeng J, Li G. Microrna-27a Protects Retinal Pigment Epithelial Cells Under High Glucose Conditions by Targeting TLR4. Exp Ther Med (2018) 16(1):452–8. doi: 10.3892/etm.2018.6150
83. Liu L, Jiang Y, Steinle JJ. Inhibition of HMGB1 Protects the Retina From Ischemia-Reperfusion, as Well as Reduces Insulin Resistance Proteins. PloS One (2017) 12(5):e0178236. doi: 10.1371/journal.pone.0178236
84. Zhu SH, Liu BQ, Hao MJ, Fan YX, Qian C, Teng P, et al. Paeoniflorin Suppressed High Glucose-Induced Retinal Microglia MMP-9 Expression and Inflammatory Response via Inhibition of TLR4/NF-Kappab Pathway Through Upregulation of SOCS3 in Diabetic Retinopathy. Inflammation (2017) 40(5):1475–86. doi: 10.1007/s10753-017-0571-z
85. Lee JJ, Wang PW, Yang IH, Huang HM, Chang CS, Wu CL, et al. High-Fat Diet Induces Toll-Like Receptor 4-Dependent Macrophage/Microglial Cell Activation and Retinal Impairment. Invest Ophthalmol Vis Sci (2015) 56(5):3041–50. doi: 10.1167/iovs.15-16504
86. Liu L, Jiang Y, Steinle JJ. Toll-Like Receptor 4 Reduces Occludin and Zonula Occludens 1 to Increase Retinal Permeability Both In Vitro and In Vivo. J Vasc Res (2017) 54(6):367–75. doi: 10.1159/000480455
87. Seidel A, Liu L, Jiang Y, Steinle JJ. Loss of TLR4 in Endothelial Cells But Not Muller Cells Protects the Diabetic Retina. Exp Eye Res (2021) 206:108557. doi: 10.1016/j.exer.2021.108557
88. Mulfaul K, Ozaki E, Fernando N, Brennan K, Chirco KR, Connolly E, et al. Toll-Like Receptor 2 Facilitates Oxidative Damage-Induced Retinal Degeneration. Cell Rep (2020) 30(7):2209–24.e2205. doi: 10.1016/j.celrep.2020.01.064
89. Liu L, Jiang Y, Curtiss E, Fukuchi KI, Steinle JJ. TLR4 Regulates Insulin-Resistant Proteins to Increase Apoptosis in the Mouse Retina. Inflammation Res (2017) 66(11):993–7. doi: 10.1007/s00011-017-1080-0
90. Liu L, Steinle JJ. Toll-Like Receptor 4 Regulates Insulin Signal Transduction in Retinal Muller Cells. Growth Factors (2017) 35(6):234–8. doi: 10.1080/08977194.2018.1442833
91. Zhang W, Dong X, Wang T, Kong Y. Exosomes Derived From Platelet-Rich Plasma Mediate Hyperglycemia-Induced Retinal Endothelial Injury via Targeting the TLR4 Signaling Pathway. Exp Eye Res (2019) 189:107813. doi: 10.1016/j.exer.2019.107813
92. Buraczynska M, Zukowski P, Ksiazek K, Wacinski P, Dragan M. The Effect of Toll-Like Receptor 4 Gene Polymorphism on Vascular Complications in Type 2 Diabetes Patients. Diabetes Res Clin Pract (2016) 116:7–13. doi: 10.1016/j.diabres.2016.04.002
93. Zaharieva ET, Kamenov ZA, Savov AS. TLR4 Polymorphisms Seem Not to be Associated With Prediabetes and Type 2 Diabetes But Predispose to Diabetic Retinopathy; TLR4 Polymorphisms in Glucose Continuum. Endocr Regul (2017) 51(3):137–44. doi: 10.1515/enr-2017-0014
94. Aioanei CS, Ilies RF, Bala C, Petrisor MF, Porojan MD, Popp RA, et al. The Role of Adiponectin and Toll-Like Receptor 4 Gene Polymorphisms on Non-Proliferative Retinopathy in Type 2 Diabetes Mellitus Patients. A Case-Control Study in Romanian Caucasians Patients. Acta Endocrinol (Buchar) (2019) -5(1):32–8. doi: 10.4183/aeb.2019.32
95. Ferwerda B, McCall MB, Verheijen K, Kullberg BJ, van der Ven AJ, van der Meer JW, et al. Functional Consequences of Toll-Like Receptor 4 Polymorphisms. Mol Med (2008) 14(5-6):346–52. doi: 10.2119/2007-00135.Ferwerda
96. Klein ML, Ferris FL 3rd, Francis PJ, Lindblad AS, Chew EY, Hamon SC, et al. Progression of Geographic Atrophy and Genotype in Age-Related Macular Degeneration. Ophthalmology (2010) 1171559(8):1554–9:e1551. doi: 10.1016/j.ophtha.2009.12.012
97. Fine SL, Berger JW, Maguire MG, Ho AC. Age-Related Macular Degeneration. N Engl J Med (2000) 342(7):483–92. doi: 10.1056/NEJM200002173420707
98. Friedman DS, O’Colmain BJ, Munoz B, Tomany SC, McCarty C, de Jong PT, et al. Prevalence of Age-Related Macular Degeneration in the United States. Arch Ophthalmol (2004) 122(4):564–72. doi: 10.1001/archopht.122.4.564
99. Williams BL, Seager NA, Gardiner JD, Pappas CM, Cronin MC, Amat di San Filippo C, et al. Chromosome 10q26-Driven Age-Related Macular Degeneration Is Associated With Reduced Levels of HTRA1 in Human Retinal Pigment Epithelium. Proc Natl Acad Sci USA (2021) 118(30):e2103617118. doi: 10.1073/pnas.2103617118
100. Zhou JY, Huang YQ, Zhang XY, Zheng PF, Li P, Chen Y, et al. Association Study of Toll-Like Receptors 4 Polymorphisms and the Risk of Age-Related Macular Degeneration: A Meta-Analysis. Ophthalmic Genet (2020) 41(6):579–84. doi: 10.1080/13816810.2020.1814348
101. Frederick PA, Kleinman ME. The Immune System and AMD. Curr Ophthalmol Rep (2014) 2(1):14–9. doi: 10.1007/s40135-013-0037-x
102. Sridevi Gurubaran I, Helotera H, Marry S, Koskela A, Hyttinen JMT, Paterno JJ, et al. Oxidative Stress and Mitochondrial Damage in Dry Age-Related Macular Degeneration Like NFE2L2/PGC-1alpha (-/-) Mouse Model Evoke Complement Component C5a Independent of C3. Biol (Basel) (2021) 10(7). doi: 10.3390/biology10070622
103. Zhou P, Fan L, Yu KD, Zhao MW, Li XX. Toll-Like Receptor 3 C1234T may Protect Against Geographic Atrophy Through Decreased Dsrna Binding Capacity. FASEB J (2011) 25(10):3489–95. doi: 10.1096/fj.11-189258
104. Ambati J, Atkinson JP, Gelfand BD. Immunology of Age-Related Macular Degeneration. Nat Rev Immunol (2013) 13(6):438–51. doi: 10.1038/nri3459
105. Klettner A, Brinkmann A, Winkelmann K, Kackenmeister T, Hildebrandt J, Roider J. Effect of Long-Term Inflammation on Viability and Function of RPE Cells. Exp Eye Res (2020) 200:108214. doi: 10.1016/j.exer.2020.108214
106. Klettner A, Koinzer S, Meyer T, Roider J. Toll-Like Receptor 3 Activation in Retinal Pigment Epithelium Cells - Mitogen-Activated Protein Kinase Pathways of Cell Death and Vascular Endothelial Growth Factor Secretion. Acta Ophthalmol (2013) 91(3):e211–218. doi: 10.1111/aos.12031
107. Kleinman ME, Yamada K, Takeda A, Chandrasekaran V, Nozaki M, Baffi JZ, et al. Sequence- and Target-Independent Angiogenesis Suppression by Sirna via TLR3. Nature (2008) 452(7187):591–7. doi: 10.1038/nature06765
108. Kleinman ME, Ambati J. A Window to Innate Neuroimmunity: Toll-Like Receptor-Mediated Cell Responses in the Retina. Adv Exp Med Biol (2012) 723:3–9. doi: 10.1007/978-1-4614-0631-0_1
109. Kleinman ME, Kaneko H, Cho WG, Dridi S, Fowler BJ, Blandford AD, et al. Short-Interfering Rnas Induce Retinal Degeneration via TLR3 and IRF3. Mol Ther (2012) 20(1):101–8. doi: 10.1038/mt.2011.212
110. Gao ML, Wu KC, Deng WL, Lei XL, Xiang L, Zhou GH, et al. Toll-Like Receptor 3 Activation Initiates Photoreceptor Cell Death In Vivo and In Vitro. Invest Ophthalmol Visual Sci (2017) 58(2):801–11. doi: 10.1167/iovs.16-20692
111. Lavalette S, Conart JB, Touhami S, Roubeix C, Houssier M, Augustin S, et al. CD36 Deficiency Inhibits Retinal Inflammation and Retinal Degeneration in Cx3cr1 Knockout Mice. Front Immunol (2019) 10:3032. doi: 10.3389/fimmu.2019.03032
112. Kohno H, Chen Y, Kevany BM, Pearlman E, Miyagi M, Maeda T, et al. Photoreceptor Proteins Initiate Microglial Activation via Toll-Like Receptor 4 in Retinal Degeneration Mediated by All-Trans-Retinal. J Biol Chem (2013) 288(21):15326–41. doi: 10.1074/jbc.M112.448712
113. Yang Y, Takeda A, Yoshimura T, Oshima Y, Sonoda KH, Ishibashi T. IL-10 Is Significantly Involved in HSP70-Regulation of Experimental Subretinal Fibrosis. PloS One (2013) 8(12):e80288. doi: 10.1371/journal.pone.0080288
114. Wang H, Guo J, West XZ, Bid HK, Lu L, Hong L, et al. Detection and Biological Activities of Carboxyethylpyrrole Ethanolamine Phospholipids (CEP-Eps). Chem Res Toxicol (2014) 27(12):2015–22. doi: 10.1021/tx500216a
115. Huang JD, Amaral J, Lee JW, Rodriguez IR. 7-Ketocholesterol-Induced Inflammation Signals Mostly Through the TLR4 Receptor Both In Vitro and In Vivo. PloS One (2014) 9(7):e100985. doi: 10.1371/journal.pone.0100985
116. Hwang N, Kwon MY, Woo JM, Chung SW. Oxidative Stress-Induced Pentraxin 3 Expression Human Retinal Pigment Epithelial Cells Is Involved in the Pathogenesis of Age-Related Macular Degeneration. Int J Mol Sci (2019) 20(23):29. doi: 10.3390/ijms20236028
117. Patel AK, Hackam AS. A Novel Protective Role for the Innate Immunity Toll-Like Receptor 3 (TLR3) in the Retina via Stat3. Mol Cell Neurosci (2014) 63:38–48. doi: 10.1016/j.mcn.2014.09.004
118. Patel AK, Hackam AS. Toll-Like Receptor 3 (TLR3) Protects Retinal Pigmented Epithelium (RPE) Cells From Oxidative Stress Through a STAT3-Dependent Mechanism. Mol Immunol (2013) 54(2):122–31. doi: 10.1016/j.molimm.2012.11.005
119. Yi H, Patel AK, Sodhi CP, Hackam DJ, Hackam AS. Novel Role for the Innate Immune Receptor Toll-Like Receptor 4 (TLR4) in the Regulation of the Wnt Signaling Pathway and Photoreceptor Apoptosis. PloS One (2012) 7(5):e36560. doi: 10.1371/journal.pone.0036560
120. Duncan RS, Rohowetz L, Vogt A, Koulen P. Repeat Exposure to Polyinosinic:Polycytidylic Acid Induces TLR3 Expression via JAK-STAT Signaling and Synergistically Potentiates Nfkappab-Rela Signaling in ARPE-19 Cells. Cell Signal (2020) 66:109494. doi: 10.1016/j.cellsig.2019.109494
121. Wornle M, Merkle M, Wolf A, Ribeiro A, Himmelein S, Kernt M, et al. Inhibition of TLR3-Mediated Proinflammatory Effects by Alkylphosphocholines in Human Retinal Pigment Epithelial Cells. Invest Ophthalmol Vis Sci (2011) 52(9):6536–44. doi: 10.1167/iovs.10-6993
122. Chen L, Bai Y, Zhao M, Jiang Y. TLR4 Inhibitor Attenuates Amyloid-Beta-Induced Angiogenic and Inflammatory Factors in ARPE-19 Cells: Implications for Age-Related Macular Degeneration. Mol Med Rep (2016) 13(4):3249–56. doi: 10.3892/mmr.2016.4890
123. Fujimoto T, Sonoda KH, Hijioka K, Sato K, Takeda A, Hasegawa E, et al. Choroidal Neovascularization Enhanced by Chlamydia Pneumoniae via Toll-Like Receptor 2 in the Retinal Pigment Epithelium. Invest Ophthalmol Visual Sci (2010) 51(9):4694–702. doi: 10.1167/iovs.09-4464
124. Will-Orrego A, Qiu Y, Fassbender ES, Shen S, Aranda J, Kotagiri N, et al. Amount of Mononuclear Phagocyte Infiltrate Does Not Predict Area of Experimental Choroidal Neovascularization (CNV). J Ocul Pharmacol Ther (2018) 34(7):489–99. doi: 10.1089/jop.2017.0131
125. Maloney SC, Antecka E, Orellana ME, Fernandes BF, Odashiro AN, Eghtedari M, et al. Choroidal Neovascular Membranes Express Toll-Like Receptor 3. Ophthalmic Res (2010) 44(4):237–41. doi: 10.1159/000313989
126. Zhu Y, Liang L, Qian D, Yu H, Yang P, Lei B, et al. Increase in Peripheral Blood Mononuclear Cell Toll-Like Receptor 2/3 Expression and Reactivity to Their Ligands in a Cohort of Patients With Wet Age-Related Macular Degeneration. Mol Vis (2013) 19:1826–33.
127. Feng YF, Guo H, Yuan F, Shen MQ. Lipopolysaccharide Promotes Choroidal Neovascularization by Up-Regulation of CXCR4 and CXCR7 Expression in Choroid Endothelial Cell. PloS One (2015) 10(8):e0136175. doi: 10.1371/journal.pone.0136175
128. Kaur I, Hussain A, Hussain N, Das T, Pathangay A, Mathai A, et al. Analysis of CFH, TLR4, and APOE Polymorphism in India Suggests the Tyr402His Variant of CFH to be a Global Marker for Age-Related Macular Degeneration. Invest Ophthalmol Vis Sci (2006) 47(9):3729–35. doi: 10.1167/iovs.05-1430
129. Despriet DD, Bergen AA, Merriam JE, Zernant J, Barile GR, Smith RT, et al. Comprehensive Analysis of the Candidate Genes CCL2, CCR2, and TLR4 in Age-Related Macular Degeneration. Invest Ophthalmol Vis Sci (2008) 49(1):364–71. doi: 10.1167/iovs.07-0656
130. Edwards AO, Chen D, Fridley BL, James KM, Wu Y, Abecasis G, et al. Toll-Like Receptor Polymorphisms and Age-Related Macular Degeneration. Invest Ophthalmol Vis Sci (2008) 49(4):1652–9. doi: 10.1167/iovs.07-1378
131. Yang Z, Stratton C, Francis PJ, Kleinman ME, Tan PL, Gibbs D, et al. Toll-Like Receptor 3 and Geographic Atrophy in Age-Related Macular Degeneration. N Engl J Med (2008) 359(14):1456–63. doi: 10.1056/NEJMoa0802437
132. Cho Y, Wang JJ, Chew EY, Ferris FL, Mitchell P, Chan CC, et al. Toll-Like Receptor Polymorphisms and Age-Related Macular Degeneration: Replication in Three Case-Control Samples. Invest Ophthalmol Vis Sci (2009) 50(12):5614–8. doi: 10.1167/iovs.09-3688
133. Sng CC, Cackett PD, Yeo IY, Thalamuthu A, Venkatraman A, Venkataraman D, et al. Toll-Like Receptor 3 Polymorphism Rs3775291 Is Not Associated With Choroidal Neovascularization or Polypoidal Choroidal Vasculopathy in Chinese Subjects. Ophthalmic Res (2011) 45(4):191–6. doi: 10.1159/000321387
134. Cheng Y, Li MW, Li HP, Zeng WT, Zhou P, Huang LZ, et al. Toll-Like Receptor 3 Polymorphism Is Not Associated With Neovascular Age-Related Macular Degeneration and Polypoidal Choroidal Vasculopathy in the Chinese. Genet Mol Res (2014) 13(1):302–9. doi: 10.4238/2014.January.17.15
135. Sharma NK, Sharma K, Gupta A, Prabhakar S, Singh R, Gupta PK, et al. Does Toll-Like Receptor-3 (TLR-3) Have Any Role in Indian AMD Phenotype? Mol Cell Biochem (2014) 393(1-2):1–8. doi: 10.1007/s11010-014-2040-4
136. Guven M, Batar B, Mutlu T, Bostanci M, Mete M, Aras C, et al. Toll-Like Receptors 2 and 4 Polymorphisms in Age-Related Macular Degeneration. Curr Eye Res (2016) 41(6):856–61. doi: 10.3109/02713683.2015.1067326
137. Ma L, Tang FY, Chu WK, Young AL, Brelen ME, Pang CP, et al. Association of Toll-Like Receptor 3 Polymorphism Rs3775291 With Age-Related Macular Degeneration: A Systematic Review and Meta-Analysis. Sci Rep (2016) 6:19718. doi: 10.1038/srep19718
138. Wagner N, Reinehr S, Palmhof M, Schuschel D, Tsai T, Sommer E, et al. Microglia Activation in Retinal Ischemia Triggers Cytokine and Toll-Like Receptor Response. J Mol Neurosci (2021) 71(3):527–44. doi: 10.1007/s12031-020-01674-w
139. Sun Y, Ni Y, Kong N, Huang C. TLR2 Signaling Contributes to the Angiogenesis of Oxygen-Induced Retinopathy. Exp Eye Res (2021) 210:108716. doi: 10.1016/j.exer.2021.108716
140. Liu J, Zhang N, Zhang M, Yin H, Zhang X, Wang X, et al. N-Acetylserotonin Alleviated the Expression of Interleukin-1beta in Retinal Ischemia-Reperfusion Rats via the TLR4/NF-Kappab/NLRP3 Pathway. Exp Eye Res (2021) 208:108595. doi: 10.1016/j.exer.2021.108595
141. Dvoriantchikova G, Santos AR, Saeed AM, Dvoriantchikova X, Ivanov D. Putative Role of Protein Kinase C in Neurotoxic Inflammation Mediated by Extracellular Heat Shock Protein 70 After Ischemia-Reperfusion. J Neuroinflamm (2014) 11:81. doi: 10.1186/1742-2094-11-81
142. He C, Sun Y, Ren X, Lin Q, Hu X, Huang X, et al. Angiogenesis Mediated by Toll-Like Receptor 4 in Ischemic Neural Tissue. Arterioscler Thromb Vasc Biol (2013) 33(2):330–8. doi: 10.1161/ATVBAHA.112.300679
143. Dvoriantchikova G, Hernandez E, Grant J, Santos AR, Yang H, Ivanov D. The High-Mobility Group Box-1 Nuclear Factor Mediates Retinal Injury After Ischemia Reperfusion. Invest Ophthalmol Vis Sci (2011) 52(10):7187–94. doi: 10.1167/iovs.11-7793
144. Dvoriantchikova G, Santos AR, Danek D, Dvoriantchikova X, Ivanov D. The TIR-Domain-Containing Adapter Inducing Interferon-Beta-Dependent Signaling Cascade Plays a Crucial Role in Ischemia-Reperfusion-Induced Retinal Injury, Whereas the Contribution of the Myeloid Differentiation Primary Response 88-Dependent Signaling Cascade Is Not as Pivotal. Eur J Neurosci (2014) 40(3):2502–12. doi: 10.1111/ejn.12603
145. Cai M, Zhang X, Li Y, Xu H. Toll-Like Receptor 3 Activation Drives the Inflammatory Response in Oxygen-Induced Retinopathy in Rats. Br J Ophthalmol (2015) 99(1):125–32. doi: 10.1136/bjophthalmol-2014-305690
146. Ishizuka F, Shimazawa M, Inoue Y, Nakano Y, Ogishima H, Nakamura S, et al. Toll-Like Receptor 4 Mediates Retinal Ischemia/Reperfusion Injury Through Nuclear Factor-Kappab and Spleen Tyrosine Kinase Activation. Invest Ophthalmol Vis Sci (2013) 54(8):5807–16. doi: 10.1167/iovs.13-11932
147. Chen Z, Qiu PY, Ma CG. Dexmedetomidine Preconditioning Protects Against Retinal Ischemia/Reperfusion Injury and Inhibits Inflammation Response via Toll-Like Receptor 4 (TLR4) Pathway. BioMed Pharmacother (2017) 93:1018–24. doi: 10.1016/j.biopha.2017.06.050
148. Chen W, Zhang J, Zhang P, Hu F, Jiang T, Gu J, et al. Role of TLR4-MAP4K4 Signaling Pathway in Models of Oxygen-Induced Retinopathy. FASEB J (2019) 33(3):3451–64. doi: 10.1096/fj.201801086RR
149. Guan L, Li C, Zhang Y, Gong J, Wang G, Tian P, et al. Puerarin Ameliorates Retinal Ganglion Cell Damage Induced by Retinal Ischemia/Reperfusion Through Inhibiting the Activation of TLR4/NLRP3 Inflammasome. Life Sci (2020) 256:117935. doi: 10.1016/j.lfs.2020.117935
150. You ZP, Yu MJ, Zhang YL, Shi K. Progranulin Protects the Mouse Retina Under Hypoxic Conditions via Inhibition of the Tolllike Receptor4nadph Oxidase 4 Signaling Pathway. Mol Med Rep (2019) 19(1):382–90. doi: 10.3892/mmr.2018.9634
151. Dvoriantchikova G, Barakat DJ, Hernandez E, Shestopalov VI, Ivanov D. Toll-Like Receptor 4 Contributes to Retinal Ischemia/Reperfusion Injury. Mol Vis (2010) 16:1907–12.
152. Hu Y, Yu Y, Bu Z, Cun B, Gong Y, Li D, et al. Increased Systemic Heparanase in Retinal Vein Occlusion Is Associated With Activation of Inflammation and Thrombophilia. Retina (2020) 40(2):345–9. doi: 10.1097/IAE.0000000000002374
153. Chen H, Song Z, Ying S, Yang X, Wu W, Tan Q, et al. Myeloid Differentiation Protein 2 Induced Retinal Ischemia Reperfusion Injury via Upregulation of ROS Through a TLR4-NOX4 Pathway. Toxicol Lett (2018) 282:109–20. doi: 10.1016/j.toxlet.2017.10.018
154. Ren L, Tao J, Chen H, Bian Y, Yang X, Chen G, et al. Myeloid Differentiation Protein 2-Dependent Mechanisms in Retinal Ischemia-Reperfusion Injury. Toxicol Appl Pharmacol (2017) 317:1–11. doi: 10.1016/j.taap.2017.01.001
155. Sanchez-Cruz A, Mendez AC, Lizasoain I, de la Villa P, de la Rosa EJ, Hernandez-Sanchez C. Tlr2 Gene Deletion Delays Retinal Degeneration in Two Genetically Distinct Mouse Models of Retinitis Pigmentosa. Int J Mol Sci (2021) 22(15):7815. doi: 10.3390/ijms22157815
156. Garces K, Carmy T, Illiano P, Brambilla R, Hackam AS. Increased Neuroprotective Microglia and Photoreceptor Survival in the Retina From a Peptide Inhibitor of Myeloid Differentiation Factor 88 (Myd88). J Mol Neurosci (2020) 70(6):968–80. doi: 10.1007/s12031-020-01503-0
157. Shiose S, Chen Y, Okano K, Roy S, Kohno H, Tang J, et al. Toll-Like Receptor 3 Is Required for Development of Retinopathy Caused by Impaired All-Trans-Retinal Clearance in Mice. J Biol Chem (2011) 286(17):15543–55. doi: 10.1074/jbc.M111.228551
158. Lee MT, Hooper LC, Kump L, Hayashi K, Nussenblatt R, Hooks JJ, et al. Interferon-Beta and Adhesion Molecules (E-Selectin and s-Intracellular Adhesion Molecule-1) Are Detected in Sera From Patients With Retinal Vasculitis and Are Induced in Retinal Vascular Endothelial Cells by Toll-Like Receptor 3 Signalling. Clin Exp Immunol (2007) 147(1):71–80. doi: 10.1111/j.1365-2249.2006.03253.x
159. Fang J, Chen L, Tang J, Hou S, Liao D, Ye Z, et al. Association Between Copy Number Variations of TLR7 and Ocular Behcet’s Disease in a Chinese Han Population. Invest Ophthalmol Vis Sci (2015) 56(3):1517–23. doi: 10.1167/iovs.14-15030
160. Fang J, Hu R, Hou S, Ye Z, Xiang Q, Qi J, et al. Association of TLR2 Gene Polymorphisms With Ocular Behcet’s Disease in a Chinese Han Population. Invest Ophthalmol Vis Sci (2013) 54(13):8384–92. doi: 10.1167/iovs.13-12878
161. Ito R, Ota M, Meguro A, Katsuyama Y, Uemoto R, Nomura E, et al. Investigation of Association Between TLR9 Gene Polymorphisms and VKH in Japanese Patients. Ocul Immunol Inflammation (2011) 19(3):202–5. doi: 10.3109/09273948.2011.553981
162. Han X, Chen X, Chen S, Luo Q, Liu X, He A, et al. Tetramethylpyrazine Attenuates Endotoxin-Induced Retinal Inflammation by Inhibiting Microglial Activation via the TLR4/NF-Kappab Signalling Pathway. BioMed Pharmacother (2020) 128:110273. doi: 10.1016/j.biopha.2020.110273
163. Wang Y, Tao J, Jiang M, Yao Y. Apocynin Ameliorates Diabetic Retinopathy in Rats: Involvement of TLR4/NF-κb Signaling Pathway. Int Immunopharmacol (2019) 73:49–56. doi: 10.1016/j.intimp.2019.04.062
Keywords: toll-like receptors, retinal diseases, age-related macular degeneration, diabetic retinopathy, ischaemic retinopathy, retinal dystrophies, inflammation, genetic polymorphisms
Citation: Titi-Lartey O, Mohammed I and Amoaku WM (2022) Toll-Like Receptor Signalling Pathways and the Pathogenesis of Retinal Diseases. Front. Ophthalmol. 2:850394. doi: 10.3389/fopht.2022.850394
Received: 07 January 2022; Accepted: 08 March 2022;
Published: 31 March 2022.
Edited by:
Matthew P. Simunovic, The University of Sydney, AustraliaReviewed by:
Graham Wallace, University of Birmingham, United KingdomRaymond “Scott” Duncan, University of Missouri–Kansas City, United States
Copyright © 2022 Titi-Lartey, Mohammed and Amoaku. This is an open-access article distributed under the terms of the Creative Commons Attribution License (CC BY). The use, distribution or reproduction in other forums is permitted, provided the original author(s) and the copyright owner(s) are credited and that the original publication in this journal is cited, in accordance with accepted academic practice. No use, distribution or reproduction is permitted which does not comply with these terms.
*Correspondence: Winfried M. Amoaku, V2luZnJpZWQuYW1vYWt1QG5vdHRpbmdoYW0uYWMudWs=