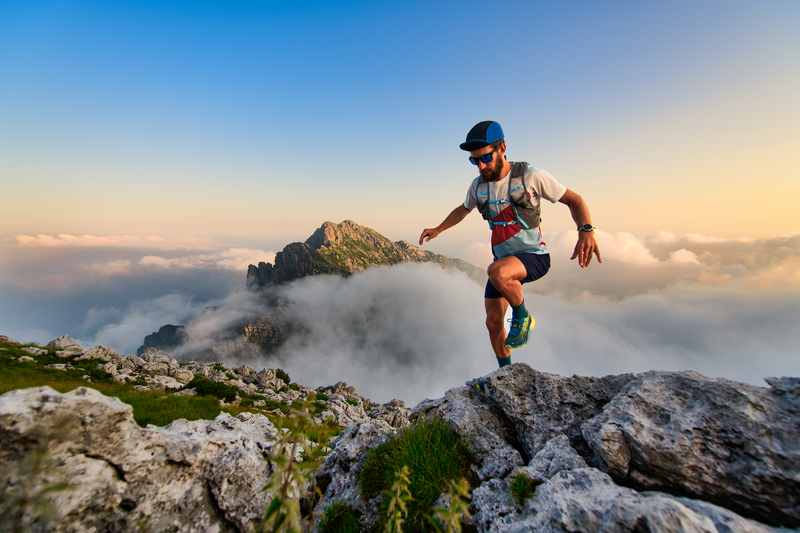
95% of researchers rate our articles as excellent or good
Learn more about the work of our research integrity team to safeguard the quality of each article we publish.
Find out more
ORIGINAL RESEARCH article
Front. Oncol. , 20 March 2025
Sec. Skin Cancer
Volume 15 - 2025 | https://doi.org/10.3389/fonc.2025.1554059
This article is part of the Research Topic Aging, Cancer, and Neurodegenerative Diseases View all articles
Introduction: Strong evidence suggests links between Parkinson’s Disease (PD) and melanoma, as studies have found that people with PD are at an increased risk of developing melanoma and those with melanoma are at increased risk of developing PD. Although these clinical associations are well-established, the cellular and molecular pathways linking these diseases are poorly understood. Recent studies have found a previously unrecognized role for the neurodegeneration-associated protein alpha-synuclein (αSyn) in melanoma; the overexpression of αSyn promotes melanoma cell proliferation and metastasis. However, to our knowledge, no studies have investigated the role of αSyn in in vivo melanoma models outside of a xenograft paradigm.
Methods: Our study created and characterized Snca knockout in the spontaneously developing melanoma TG3 mouse line, TG3+/+Snca-/-.
Results: We show that αSyn loss-of-function significantly delays melanoma onset and slows tumor growth in vivo in males. Furthermore, decreased tumor volume is correlated with a decreased DNA damage signature and increased apoptotic markers, indicating a role for αSyn in modulating the DNA damage response (DDR) pathway.
Discussion: Overall, our study may suggest that targeting αSyn and its role in modulating the DDR and melanomagenesis could serve as a promising new therapeutic target.
The association between Parkinson’s Disease (PD) and melanoma has been well established. Many epidemiological studies have found a significant increase in the risk of melanoma among individuals with PD compared to healthy individuals, ranging from 1.4-20-fold (1–17). Likewise, there is also an increased risk for PD in melanoma patients, ranging from 1.7-4.2 fold (9, 12, 18–20). Altogether, it is clear that common environmental, genetic, and/or molecular mechanisms are at play to influence this clinical association, yet the underlying mechanism is still poorly understood.
One potentially promising avenue of investigation is the biological function of the neurodegeneration-associated protein, alpha-synuclein (αSyn). Misfolded and aggregated forms of αSyn are found in cytoplasmic inclusions called Lewy bodies, which are neuropathological hallmarks in PD and other Lewy body disorders (21–23). Lewy bodies are found primarily in the central nervous system, where their presence in dopaminergic neurons in the midbrain is associated with the degeneration of these cells in PD (24). αSyn is not only found in the central nervous system, but can also be found in the periphery, including in melanocytes (25, 26) and therefore could be a key molecular link between these disease pathologies. In primary and metastatic melanoma, ~85% of biopsies show high expression of αSyn1 (2, 27–29). Since this initial characterization, there have been several studies investigating the role of αSyn in melanoma growth and metastasis; the majority of these being in vitro studies. Overall, these studies using human and mouse melanoma cell lines have found that αSyn expression is important in cell proliferation1 (30–32), motility (33), and protects against cell death (32, 34), through multiple potential mechanisms, such as altering the inflammatory response (35–37), autophagy pathways (32, 34), and DNA damage repair1.
Fewer studies have investigated the role of αSyn in in vivo melanoma mouse models and all this previous in vivo work, to our knowledge, has used a xenograft paradigm. In general, these xenograft studies corroborate previous in vitro work and find that αSyn is important in melanoma tumor growth and metastasis. Specifically, αSyn knockout (KO) human/mouse melanoma cells implanted as xenografts in mice exhibited slower growth and increased apoptosis (30), and reduced tumor-induced mechanical allodynia (38). Furthermore, WT melanoma cells in αSyn overexpressing mice show increased metastasis (31). Lastly, human melanoma xenografts implanted in mice and treated with an αSyn aggregation inhibitor (anle138b) led to increased cell death (32) and upregulation of anti-melanoma immune responses (35). Despite this substantial data linking αSyn to melanoma tumor growth in vivo, whether αSyn expression within melanocytes influences tumorigenesis is still not understood. In our current study, we aimed to create and characterize a new TG3 Snca-/- mouse line to better understand the function of αSyn in melanomagenesis, tumor growth, and metastasis in a spontaneous melanoma-forming mouse line. TG3 mice display melanin-pigmented lesions after a short latency and with complete penetrance (39–42). This model is driven by multiple tandem insertions of a transgene into intron 3 of Grm1 (metabotropic glutamate receptor 1) with concomitant deletion of an intronic sequence that increases expression of Grm1. Homozygous TG3 mice form primary melanoma tumors on pinna and perianal regions, in addition to metastatic tumors in lymph nodes, lung, and liver (39–42). The TG3 line also has the advantage of being mono-allelic, therefore making breeding to other genetically modified mice practical.
Our previous work has shown αSyn’s role in modulating nuclear DNA damage response (DDR) pathways in human melanoma cells1 and other cell types (43, 44). Specifically, we found a novel function of αSyn in DNA double-strand break (DSB) repair, where αSyn colocalizes with DSB repair components and its knockout leads to increased DSBs and their slowed repair1 (43). In this study, we aimed to investigate whether similar mechanisms are important for melanomagenesis and growth using the TG3+/+Snca -/- mouse model to test whether αSyn loss-of-function dysregulated DNA damage pathways and led to downstream cell death phenotypes.
The transgenic TG3 mice (39–42), were established at the Department of Chemical Biology, Rutgers University, Piscataway, USA and provided by Dr. Suzie Chen. αSyn KO mice (C57BL/6N-Sncatm1Mjff/J) were obtained from Jackson Laboratories (strain #016123, RRID: IMSR_JAX:016123). Homozygous αSyn KO mice were crossed with TG3 heterozygous mice and double heterozygote F1 mice were crossed to each other to generate F2 mice for analysis. Genotyping of mice was carried out by Transnetyx Inc. and primer sequences and protocols are available upon request. For all analyses, homozygous transgenic TG3 Snca+/+ and TG3 Snca-/- animals (litter mates) were used. Mice were housed in OHSU’s Department of Comparative Medicine (DCM) facilities in a light-dark cycle vivarium. Animals were maintained under ad libitum food and water diet. All animal protocols were approved by OHSU IACUC, and all experiments were performed with every effort to reduce animal lives and animal suffering, according to the US National Research Council’s Guide for the Care and Use of Laboratory Animals, the US Public Health Service’s Policy on Humane Care and Use of Laboratory Animals, and Guide for the Care and Use of Laboratory Animals.
Starting at P30, mice underwent isoflurane anesthesia every 10 days to assess weight and tumor growth. Researchers were blinded to condition. To quantitate the severity of melanoma progression, detailed observation and photodocumentation was used to assign numerical scores of 0 to 5 to the thickness of pinna tumors (see Supplementary Figure S2 for detailed description) or quantitative measurements for thickness of perianal tumors. For pinna tumors, 0=tumor not palpable or visible; 1=individual small, clearly recognizable nodes or elevations in skin; 2=small, numerous recognizable nodes or elevations; 3=significantly thickened ears, clearly nodular tumors; 4=severely thickened ears or coarse tumors; 5=extreme tumor growth with risk of ulceration. Tumor onset was designated as time when tumor changed from 0 to 1. For perianal tumors, a ruler was used to manually measure the length of the perianal tumor in centimeters.
For immunofluorescence staining of perianal tumors, 5μm sections of formalin-fixed and paraffin-embedded tissue blocks were deparaffinized and bleached in a H2O2 solution for 1 hour at room temperature (1% dipotassium phosphate, 0.5% potassium hydroxide, 3% hydrogen peroxide). Tissue underwent antigen retrieval overnight at 56C (10 mM Tris base, 1mM EDTA solution, 0.05% Tween 20, pH 9.0). Samples were permeabilized in 0.25% Triton X-100 in PBS for 10 minutes and blocked in 2% FBS/1% BSA in PBS for 2 hours and then placed in the primary antibody overnight at 4C. The next morning, samples were washed in 1x PBS and placed in secondary antibody for 1 hour at 37C. Samples were washed 4 times in 1x PBS. The third wash contained DAPI (2.5µg/ml) for 20min. Coverslips were mounted using CFM2 antifade reagent and sealed with BioGrip. All immunofluorescence images were taken on a Zeiss Laser-Scanning Confocal Microscope 980 with Airyscan and analyzed in Arivis Software. Mean intensity was measured after imposing DAPI masks over each nucleus. All cells within a 63x image were analyzed and numbers of n are provided in each figure legend. Statistical significance was assigned using T-test.
Antibody specifics were as follows: LB509 (Abcam #27766, RRID: AB_727020, 1:500), RPA32 (Bethyl #A300-245A, RRID: AB_210547, 1:1000), γH2AX (Cell Signaling #9718, RRID: AB_2118009, 1:500), 53BP1 (BD Biosciences #612522, RRID: AB_2206766, 1:1000).
Pinna tumors, perianal tumors, and lymph nodes were homogenized in RNeasy mini kit buffer (Qiagen) using a hand-held tissue homogenizer followed by Qiashredder centrifugation (Qiagen). Total RNA was isolated using the RNeasy mini kit (Qiagen) according to the manufacturer’s instructions. RNA concentration was measured with a NanoDrop spectrophotometer and cDNA was synthesized from 500ng RNA with M-MLV reverse transcriptase (Promega). Analysis of mRNA expression was performed using quantitative Real-Time PCR on the QuantStudio 3 (Applied Biosystems). A volume of 1 μl cDNA template, 1 μl of forward and reverse primers (each 10 μM) and 10 μl of SYBR Green I (Roche) were combined to a total volume of 20 μl. Primers used are described in Supplementary Table S1. Each sample was analyzed in duplicates. The target cDNA was normalized to β-Actin levels. Statistical significance was assigned using T-test.
Beyond individualized analysis within each assay methodology, all data was processed using GraphPad Prism version 9.0 (RRID: SCR_002798). Data was analyzed using T-test, unless stated otherwise, and considered statistically significant if p < 0.05. All data was presented as a mean +/- standard error of the mean (SEM).
To study the role of αSyn in melanoma tumorigenesis in vivo, TG3 mice (39) were crossed with Snca-knockout mice. The generated TG3+/+Snca+/+ (“wildtype”) and TG3+/+Snca-/- (“homozygous KO”) mice were then analyzed for tumor growth from P30 to P100, at which point the mice were sacrificed and dissected for tissue processing (Figure 1A). There was no significant difference in weight of the mice between wildtype and homozygous KO genotypes (Supplementary Figure S1). Melanoma tumor onset was evaluated, and homozygous KO mice developed melanoma significantly later compared to the wildtype control group (Figure 1B). Wildtype mice on average exhibited tumors at P43, whereas melanoma onset was observed on average at P50 for homozygous KO mice. This difference is driven primarily by male mice, since when stratified by sex there was no significant difference between genotypes in female mice but there was in male mice (Figure 1B). Further, the progression of melanoma growth on pinna and perianal regions were followed for ~70 days. Here, a graded scoring system from minimal (0) to extreme tumor growth (5) was used to quantify melanoma progression on pinna as previously described (45) (Supplementary Figure S2) and quantitative size measurement was used to quantify melanoma progression in perianal regions. This analysis revealed no significant differences in tumor progression of the pinna between wildtype and homozygous KO genotypes, even when stratified by sex (Figure 1B). However, homozygous KO mice display decreased perianal tumor growth compared to wildtype mice, which becomes significant at later time points (Figure 1C). Again, this difference is driven primarily by male mice when stratified by sex (Figure 1C).
Figure 1. Alpha-synuclein knockout significantly delays tumor onset and slows tumor progression. (A) Schematic representing experimental timeline. (B, C) Pinna melanoma onset in TG3+/+Snca+/+ (n=15) and TG3+/+Snca-/- (n=14). Pinna and perianal tumor progression of the TG3+/+Snca-/- compared to the TG3+/+Snca+/+ after tumor onset. The grading system to evaluate the progression of tumor growth at the pinna region until endpoint at P110 is further described in Supplementary Figure S2. Analysis was further stratified by sex with TG3+/+Snca+/+ male (n=10), TG3+/+Snca+/+ female (n=5), TG3+/+Snca-/- male (n=7), and TG3+/+Snca-/- female (n=7). Error bars represent Standard Error of the Mean (SEM). *p<0.05 by unpaired T-test for tumor onset or Two-way ANOVA for tumor progression.
We next wanted to confirm the presence of melanoma-like cells in the primary tumors of these mice through histopathological analysis. Hematoxylin and eosin (H+E) staining revealed significant levels of pigmented cell growth in the primary pinna and perianal tumors of both wildtype and homozygous KO mice compared to control C57BL/6 wildtype mice without tumors (Figure 2A). qRT-PCR analyses revealed comparable Grm1 mRNA expression levels between the wildtype and homozygous KO pinna and perianal primary tumors. This suggests that mice express the Grm1 transgene at similar levels regardless of Snca expression (Figure 2B). These levels were compared to positive control cerebellum tissue where Grm1 mRNA expression is known to be high.
Figure 2. Alpha-synuclein knockout does not interfere with Grm1 expression. (A) Formalin-fixed paraffin-embedded pinna and perianal primary tumors and lymph nodes were stained for hematoxylin and eosin in TG3-/-Snca+/+, TG3+/+Snca+/+, or TG3+/+Snca-/- mice. Stained samples were imaged on the Zeiss ApoTome2 microscope. Scale bar=100µm, except for TG3-/-Snca+/+ perianal scale bar=200µm and lymph node scale bar=50µm. (B) Total RNA was isolated from pinna and perianal primary tumors and lymph nodes from TG3+/+Snca+/+ or TG3+/+Snca-/- mice. Using primers against the Grm1 gene, qRT-PCR amplification was determined when normalized to beta-actin. For pinna and perianal analysis, TG3+/+Snca+/+ (n=5) and TG3+/+Snca-/- (n=6). For lymph node analysis, TG3+/+Snca+/+ (n=12) and TG3+/+Snca-/- (n=9). Cerebellum samples (n=3). Each sample was run with 2 technical replicates. Statistical analysis via unpaired T-test.
Additionally, H+E staining confirmed the presence of pigmented melanoma cells in the lymph nodes of both wildtype and homozygous KO mice, indicating lymph node metastasis had occurred in both genotypes (Figure 2A). The Grm1 mRNA expression in lymph node tissues of wildtype and homozygous KO mice was analyzed as a marker for melanoma cell dissemination. There was no significant difference in Grm1 expression in lymph nodes between the homozygous KO mice compared to wildtype mice (Figure 2B), although larger cohorts would be needed to detect differences in metastasis given inter-animal variability.
αSyn has been previously linked to DNA DSB repair, since knocking out αSyn significantly increases DNA damage levels in SK-Mel28 cells1, Hap1 cells (43, 44), and mouse brain (43) due to less efficient DNA DSB repair. We wanted to investigate whether there were differences in DNA damage burden and repair mechanisms between TG3+/+Snca +/+ (“wildtype”) and Snca -/- (“homozygous KO”) mice. Formalin-fixed paraffin-embedded perianal primary tumor samples from wildtype and homozygous KO mice underwent immunofluorescence (IF) staining. Genotypes were first validated via IF when stained using an αSyn antibody, LB509, where homozygous KO tissue showed significantly reduced levels of staining compared to wildtype mice (Figure 3A). In addition, when analyzing the localization of αSyn labelling in the wildtype samples, discrete nuclear foci were seen in the melanoma tumor cells, similar to our previous studies where these foci are implicated in DNA damage repair processes1 (43, 44). Given these findings and previous data, these samples were next stained for DNA damage and damage repair markers: γH2AX, RPA32, and 53BP1.
Figure 3. Alpha-synuclein loss-of-function leads to lower DNA damage signature in P110 perianal tumors. (A–D) Formalin-fixed paraffin-embedded perianal primary tumors from TG3+/+Snca+/+ (n=5) and TG3+/+Snca-/- (n=5) were stained for LB509, γH2AX, RPA32, 53BP1, or DAPI. Stained samples were imaged on the Zeiss 980 confocal microscope with Airyscan processing. Mean intensity, number of foci, and density of foci within DAPI masks were analyzed using Arivis. *p<0.05, **p<0.01, *** p<0.001, ****p<0.0001 by unpaired T-test. Error bars denote SEM. Scale bar=5µm (A) or 2µm (B–D). Quantification from 5 biological replicates (separate animals) per group were performed (n=163-249 nuclei analyzed per condition).
γH2AX, a phosphorylated form of histone H2AX, is involved in the early stages of DNA DSB detection and is a sensitive marker for DNA damage burden. IF staining for γH2AX revealed a significant decrease in mean intensity of γH2AX signal, number of nuclear γH2AX foci, and density of nuclear γH2AX foci in the homozygous KO group compared to the wildtype group (Figure 3B). These trends remained similar when stratified by sex. RPA32, replication protein A2, binds and stabilizes single-stranded DNA intermediates that form during DNA repair and is important in homologous recombination (HR) DSB repair. IF staining for RPA32 revealed no significant differences in the mean nuclear intensity, number of nuclear RPA32 foci and their density in the homozygous KO group compared to the wildtype group (Figure 3C). Interestingly when stratified by sex, there were significant, but opposite, differences in mean nuclear intensity of RPA32 between the wildtype and homozygous KO group, despite no significant differences when combined. Male homozygous KO mice exhibited a significant increase in mean nuclear RPA32 intensity compared to wildtype mice, whereas female homozygous KO mice exhibited a significant decrease in mean nuclear RPA32 intensity compared to wildtype mice (Figure 3C). Lastly, 53BP1, p53-binding protein 1, is an important regulator of DNA DSB repair and promotes non-homologous end-joining (NHEJ) DSB repair. IF staining for 53BP1 revealed a significant increase in mean nuclear 53BP1 intensity in homozygous KO mice compared to wildtype mice, driven by both male and female mice (Figure 3D). Homozygous KO female mice showed a significant increase in number and density of 53BP1 foci compared to wildtype mice, but male mice showed no genotype differences.
There is evidence from previous publications that αSyn KO upregulates in vivo melanoma xenograft apoptosis at the protein level, via the TUNEL assay (30) and autophagy-related protein, p62 (38). To further understand the downstream cellular consequences of altered DNA damage repair mechanisms in αSyn homozygous KO mice, we assayed various cell death markers via qRT-PCR. These included markers for apoptosis (Caspase-3, Caspase-9), necroptosis (RIP3), autophagic cell death (LC3B), and senescence (Cdkn2a-p16). We found that perianal tumors of homozygous KO mice exhibited significantly higher gene expression levels of Caspase-9, LC3B, and p16 compared to wildtype tumors (Figure 4A). There were no significant differences when stratified by sex, therefore data in Figure 4 represent a combination of both male and female mice. Furthermore, to directly correlate the DNA damage signatures seen with immunofluorescence (Figure 3) with Caspase-9, LC3B, and p16, we ran linear regression analyses. Average nuclear mean intensity, foci number, and foci density of γH2AX and 53BP1 showed no significant associations with these cell death markers (data not shown). However, RPA32 nuclear mean intensity and foci density significantly correlated with the levels of p16, with mean foci number close to significance, but did not correlate with Caspase-9 or LC3B levels (Figure 4B). This indicates that mice with higher RPA32 levels potentially show higher p16 mRNA levels and could suggest that αSyn loss-of-function and the subsequent dysregulation of the DDR this causes leads to a senescence-like phenotype, potentially driving the impaired tumor growth we measured in vivo.
Figure 4. Alpha-synuclein knockout increases apoptosis, autophagy, and senescence marker expression. (A) Total RNA was isolated from perianal primary tumors from TG3+/+Snca+/+ or TG3+/+Snca-/- mice. Using primers against the various genes described in Supplementary Table S1, qRT-PCR amplification was determined when normalized to beta-actin internal control. For analysis, TG3+/+Snca+/+ (n=5) and TG3+/+Snca-/- (n=6). Each sample was run with 2 technical replicates. *p<0.05 by unpaired Mann-Whitney T-test. (B) Simple linear regression analysis of mean nuclear intensity, number of foci, and density of foci of RPA32 immunofluorescence (Figure 3) compared to gene expression of Caspase-9, LC3B, and p16. Each point represents a single animal, with TG3+/+Snca+/+ (n=5, same animals from Figure 3) and TG3+/+Snca-/- (n=5, same animals from Figure 3). *p<0.05 by simple linear regression with 95% confidence intervals.
In this study, we extend our knowledge of the molecular connection between PD and melanoma, by uncovering roles for the neurodegeneration-associated protein, αSyn, in melanoma formation and growth. We developed a model to investigate αSyn deficiency on melanomagenesis and metastasis in vivo in TG3 mice (39–42). Our data suggest that αSyn loss-of-function significantly delayed melanoma tumor onset in primary pinna tumors and growth of primary perianal tumors. Furthermore, there was a non-significant, but trending, decrease in the metastasis to lymph nodes as measured by Grm1 mRNA expression. Immunofluorescence staining of the primary perianal tumors revealed a significantly decreased DNA damage signature in Snca KO mice, as measured by quantifying nuclear γH2AX. Interestingly, there were sex-dependent differences in nuclear 53BP1 and RPA32 levels in homozygous KO mice compared to wildtype. Lastly, cell death marker analysis revealed that homozygous KO perianal tumors exhibited significantly higher levels of the apoptosis marker Caspase-9, autophagic marker LC3B, and senescence marker p16. In homozygous KO tumors, RPA32 immunofluorescence significantly correlated with p16 mRNA levels, suggesting a potential senescence-like phenotype partly controlled by dysregulated RPA32-dependent DDR.
These results fit into a larger landscape of links between cancer growth, genomic instability, and DDR. Due to their highly proliferative nature, cancers are especially vulnerable to replication-induced DNA damage and genome instability. Inherently, this leads to the high DNA damage signatures seen in many cancer types (46) and melanoma cells upregulate DSB repair pathway proteins (47, 48) to increase metastatic potential (49). Our findings suggest that when αSyn is present (“wildtype” mice), DSB repair pathways remain intact, allowing for cell survival and tumor growth. However, DNA damage from hyperproliferation creates large DNA damage signatures in late-stage tumors (Figure 5). In contrast, when αSyn is not present (“homozygous KO” mice), there is impaired DSB repair due to a decrease in DSB repair machinery1. Accumulation of unrepaired DSBs ultimately leads to cell death and senescence phenotypes, with data suggesting that RPA32 levels synergize with senescence marker p16 upregulation. This could result in the impaired tumor growth and a decreased DNA damage signature (γH2AX) we detect, since the cells with a high DNA damage signature die and are removed from late-stage tumors (Figure 5). As a consequence of this unrepaired DNA damage and subsequent cell death, remaining cells may upregulate DDR pathways components 53BP1 and RPA32 and this may be sex dependent. Overall, our findings suggest that αSyn upregulation in melanoma may be part of a mechanism to improve DSB repair, allowing cells to evade the programmed cell death that would normally be triggered by high DSB levels, similar to what is seen with the upregulation of other DSB repair pathway proteins (47, 48).
Loss of αSyn resulted in upregulation of various cell death and senescence markers, likely downstream of dysregulated DDR and resulting in the decreased tumor growth in vivo. Caspase-9 is an initiator caspase in the intrinsic apoptosis pathway that is activated when cytochrome c is released from mitochondria in response to death signals (50). LC3B, microtubule-associated protein 1 light chain-3B, is an autophagic protein that plays a role in cell death and autophagy. Autophagic cell death, also known as type 2 cell death, is characterized by large-scale autophagic vacuolization of the cytoplasm (51, 52). In general, autophagy can protect cells from stresses like nutrient depletion or starvation, but excessive autophagy can lead to cell death. Furthermore, LC3B can also promote apoptosis through interactions with the extrinsic apoptotic factor Fas (53). Lastly, p16(INK4a) is a cyclin-dependent kinase inhibitor that is often expressed in senescent cells, which have stopped growing due to stress (54). This tumor suppressor gene is commonly mutated in human tumors, allowing precancerous lesions to bypass senescence (55). These processes have all been associated with DNA damage accumulation and implicated in melanoma, where suppression of Caspase-9 and p16 and over-stimulation of LCB3 have been linked to melanomagenesis, contributing to disease progression and resistance to therapy (56–65). Targeted therapy of some of these modulators is currently being explored as potential therapeutic strategies for melanoma (59, 66). Interestingly, our data could suggest a significant synergistic effect of RPA32 protein levels and p16 expression coincides with previous reports of “RPA exhaustion”. This is a phenomenon by which persistent DNA damage can lead to replication catastrophe and cells then acquire senescent traits and is associated with various age-related pathologies (67, 68). It is plausible that αSyn loss-of-function can induce such a pattern, however further investigation is necessary to elucidate mechanistic insight.
The mechanism of how αSyn regulates DNA DSB repair is still an area for investigation. Our previous work uncovered a novel role for αSyn in the recruitment of 53BP1 to ribosomal DNA DSBs, downstream of γH2AX signaling and upstream of MDC1 activity, in the SK-Mel28 melanoma cell line1. Furthermore, αSyn has been implicated in regulating DSB repair through a DNA-PK-dependent manner in Hap1 cells (44). These data suggest that αSyn may modulate the NHEJ repair pathway, where both 53BP1 and DNA-PK are important. However, the choice between NHEJ and HR is particularly interesting and at the intersection of neurodegeneration and cancer. NHEJ is thought to be the primary DSB repair pathway in post-mitotic cells, like neurons, since it does not require a sister chromatid to act as a template, yet is more error prone (69). In contrast, there is growing evidence that different cancers rely primarily on the error-free HR to counteract the genomic instability associated with replicative stress (70). Studies have shown that a high frequency of melanoma patients harbor mutations in HR-associated genes (71–73), making these tumors vulnerable to immunotherapies and treatments that target HR (71, 72, 74). Yet, the choice between HR and NHEJ is a growing topic in the field (75). In our data, αSyn loss-of-function resulted in decreased γH2AX intensity and foci, sex-dependent differences in RPA32 intensity (increased in males, decreased in females), and an increase in nuclear 53BP1 (more robust response in females). This potentially suggests that αSyn upregulation in the TG3 melanoma mouse line is important for functional DDR in a sex dependent way and that when αSyn is no longer present and there is a buildup of unrepaired DNA breaks, male mice can better upregulate HR machinery (RPA32) in the surviving cells, while female mice can better upregulate NHEJ machinery (53BP1) in the surviving cells to try to compensate. Further investigation is necessary to uncover the specific mechanism of how αSyn is influencing the DDR as a function of melanomagenesis and sex in vivo.
The sex differences we detect (in tumor onset, growth, and DDR components) are interesting since male sex is a recognized risk factor to the prevalence and outcome of both PD and melanoma. In PD, the prevalence is twice as high in males compared to females and is frequently associated with earlier disease onset (76, 77); men may develop a postural instability-dominant phenotype, which includes freezing of gait and falling (77, 78); and men experience more sleep and cognitive issues associated with the disease, such as REM sleep behavior disorder (78) and mild cognitive impairment with a more rapid progression to dementias (79, 80). In melanoma, men have a higher risk of developing melanoma across all ages and ethnicities (81); men exhibit a higher risk of melanoma progression and metastasis than females (81, 82), with a greater risk of mortality (82–84); and pathologically, thicker and more ulcerated tumors were observed in men (85). In both diseases, there have been many hypotheses as to what is driving these sex differences, including the involvement of sex hormones, the immune system response, and potential environmental exposures. However, in the context of our study, it is interesting to note previously reported sex disparities in DDR pathways. Others have found a greater accumulation of somatic mutations in male cells compared to female cells (86), suggesting decreased DNA damage repair. Females have an increased capacity to repair DNA damage by base excision repair (BER) compared to males in mice (87). Additionally, analysis of molecular difference in 13 cancers from The Cancer Genome Atlas database revealed that DNA repair genes are expressed at higher levels in female patients (88). Furthermore, steroid hormones can regulate DSB repair, both NHEJ and HR (89). Specifically, androgen receptors stimulate the activity and expression of DNA-PK in the NHEJ pathway (90), estrogens positively regulate the expression of NBS1 (91), and steroid hormones can regulate HR (92, 93). Our tumor growth and immunofluorescence data suggest that αSyn plays a role in modulating DSB repair pathways in a sex-dependent manner. Females may be better at upregulating compensatory mechanisms to counteract the unrepaired DNA damage (53BP1 upregulation), and therefore are more resistant to negative effects of αSyn loss-of-function in tumor onset and growth phenotypes. Males may be more vulnerable to DNA damage dysregulation as a consequence of αSyn loss-of function and serve as a more appropriate candidate for therapeutics that target αSyn in melanoma treatment regimens. For example, our data showed a direct relationship between RPA32 increase and p16 mRNA senescence marker upregulation in male mice and our in vitro data, in the male human melanoma cell line, SK-Mel28, αSyn KO significantly impaired growth phenotypes1.
In summary, the newly generated mouse model, TG3+/+Snca-/-, allows for the investigation of the function of αSyn in malignant melanoma. It is possible that individuals with upregulated expression of αSyn may predispose them to Lewy body aggregation in neurons (43, 94), but also melanocytic transformation and melanoma progression. The resulting loss-of-function due to αSyn aggregation (in PD) or gain-of-function of αSyn by increased expression without aggregation (in melanoma) would have differential effects on DNA damage repair pathways, potentially contributing to either neuronal cell death or melanoma cell growth, respectively. Our findings demonstrate the impact of αSyn on melanoma onset, progression, and metastasis in a sex-dependent manner and provide novel therapeutic targets focused on reducing αSyn-mediated DNA repair in melanoma.
The raw data supporting the conclusions of this article will be made available by the authors, without undue reservation.
The animal study was approved by Oregon Health and Science University IACUC. The study was conducted in accordance with the local legislation and institutional requirements.
MA: Conceptualization, Data curation, Formal analysis, Funding acquisition, Investigation, Methodology, Project administration, Resources, Software, Supervision, Validation, Visualization, Writing – original draft, Writing – review & editing. SC: Resources, Writing – review & editing. VU: Conceptualization, Data curation, Formal analysis, Funding acquisition, Methodology, Project administration, Resources, Software, Supervision, Validation, Writing – original draft, Writing – review & editing.
The author(s) declare that financial support was received for the research and/or publication of this article. The work was supported by: National Institute on Aging of the National Institutes of Health F30AG082406, National Institute of Health R01NS102227, National Institute of Health P30NS061800, National Institute of Health P30CA065823, Melanoma Research Alliance, Michael J Fox Foundation, Kuni Foundation, Melanoma Research Foundation Medical Student Research Award, Oregon Partnership for Alzheimer’s Research.
All immunofluorescence imaging could not have been possible without help from Stefanie Kaech Petrie, Felice Kelly, and Hannah Bronstein in the OHSU Advanced Light Microscopy Core (RRID: SCR_009961). We thank Dr. Pamela Cassidy (OHSU) for the invaluable discussions. Thank you to Dr. Suzie Chen (Rutgers University) for providing the TG3 mouse line and help with genotyping protocols. We thank Dr. Sebastian Staebler and Dr. Anja Bosserhoff (Friedrich-Alexander University of Erlangen-Nurnberg) for the invaluable advice and help with TG3 mouse line management, qRT-PCR protocols, and IF protocols. Lastly, many thanks to Unni Lab members who helped with the revision process: Dr. Carlos Soto Faguas, Dr. Elizabeth Rose, Anna Bowman, Elias Wisdom, Jessica Keating, and Samuel Kim.
The authors declare that the research was conducted in the absence of any commercial or financial relationships that could be construed as a potential conflict of interest.
The author(s) declare that no Generative AI was used in the creation of this manuscript.
All claims expressed in this article are solely those of the authors and do not necessarily represent those of their affiliated organizations, or those of the publisher, the editors and the reviewers. Any product that may be evaluated in this article, or claim that may be made by its manufacturer, is not guaranteed or endorsed by the publisher.
The Supplementary Material for this article can be found online at: https://www.frontiersin.org/articles/10.3389/fonc.2025.1554059/full#supplementary-material
1. Bajaj A, Driver JA, Schernhammer ES. Parkinson’s disease and cancer risk: A systematic review and meta-analysis. Cancer Causes Control. (2010) 21:697–707. doi: 10.1007/s10552-009-9497-6
2. Rugbjerg K, Friis S, Lassen CF, Ritz B, Olsen JH. Malignant melanoma, breast cancer and other cancers in patients with Parkinson’s disease. Int J Cancer. (2012) 131:1904–11. doi: 10.1002/ijc.27443
3. Olsen JH, Friis S, Frederiksen K. Malignant melanoma and other types of cancer preceding Parkinson disease. Epidemiology. (2006) 17:582–7. doi: 10.1097/01.ede.0000229445.90471.5e
4. Olsen JH, Friis S, Frederiksen K, McLaughlin JK, Mellemkjaer L, Moller H. Atypical cancer pattern in patients with Parkinson’s disease. Br J Cancer. (2005) 92:201–5. doi: 10.1038/sj.bjc.6602279
5. Olsen JH, Tangerud K, Wermuth L, Frederiksen K, Friis S. Treatment with levodopa and risk for Malignant melanoma. Mov Disord. (2007) 22:1252–7. doi: 10.1002/mds.21397
6. Elbaz A, Peterson BJ, Yang P, Van Gerpen JA, Bower JH, Maraganore DM, et al. Nonfatal cancer preceding Parkinson’s disease: A case-control study. Epidemiology. (2002) 13:157–64. doi: 10.1097/00001648-200203000-00010
7. Lo RY, Tanner CM, Van Den Eeden SK, Albers KB, Leimpeter AD, Nelson LM. Comorbid cancer in Parkinson’s disease. Mov Disord. (2010) 25:1809–17. doi: 10.1002/mds.23246
8. Ryu HJ, Park JH, Choi M, Jung JH, Han K, Kwon DY, et al. Parkinson’s disease and skin cancer risk: A nationwide population-based cohort study in Korea. J Eur Acad Dermatol Venereol. (2020) 34:2775–80. doi: 10.1111/jdv.16462
9. Kareus SA, Figueroa KP, Cannon-Albright LA, Pulst SM. Shared predispositions of parkinsonism and cancer: A population-based pedigree-linked study. Arch Neurol. (2012) 69:1572–7. doi: 10.1001/archneurol.2012.2261
10. Becker C, Brobert GP, Johansson S, Jick SS, Meier CR. Cancer risk in association with Parkinson disease: A population-based study. Parkinsonism Relat Disord. (2010) 16:186–90. doi: 10.1016/j.parkreldis.2009.11.005
11. Constantinescu R, Elm J, Auinger P, Sharma S, Augustine EF, Khadim L, et al. Malignant melanoma in early-treated Parkinson’s disease: the net-pd trial. Mov Disord. (2014) 29:263–5. doi: 10.1002/mds.25734
12. Dalvin LA, Damento GM, Yawn BP, Abbott BA, Hodge DO, Pulido JS. Parkinson disease and melanoma: confirming and reexamining an association. Mayo Clin Proc. (2017) 92:1070–9. doi: 10.1016/j.mayocp.2017.03.014
13. Driver JA, Logroscino G, Buring JE, Gaziano JM, Kurth T. A prospective cohort study of cancer incidence following the diagnosis of Parkinson’s disease. Cancer Epidemiol Biomarkers Prev. (2007) 16:1260–5. doi: 10.1158/1055-9965.EPI-07-0038
14. Bertoni JM, Arlette JP, Fernandez HH, Fitzer-Attas C, Frei K, Hassan MN, et al. Increased melanoma risk in Parkinson disease: A prospective clinicopathological study. Arch Neurol. (2010) 67:347–52. doi: 10.1001/archneurol.2010.1
15. Schwid SR, Bausch J, Oakes D, Schuchter L, Tanner C, Forrest M, et al. Cancer incidence in a trial of an antiapoptotic agent for Parkinson’s disease. Mov Disord. (2010) 25:1801–8. doi: 10.1002/mds.23006
16. Moller H, Mellemkjaer L, McLaughlin JK, Olsen JH. Occurrence of different cancers in patients with Parkinson’s disease. BMJ. (1995) 310:1500–1. doi: 10.1136/bmj.310.6993.1500
17. Zhang X, Guarin D, Mohammadzadehhonarvar N, Chen X, Gao X. Parkinson’s disease and cancer: A systematic review and meta-analysis of over 17 million participants. BMJ Open. (2021) 11:e046329. doi: 10.1136/bmjopen-2020-046329
18. Freedman DM, Travis LB, Gridley G, Kuncl RW. Amyotrophic lateral sclerosis mortality in 1.9 million us cancer survivors. Neuroepidemiology. (2005) 25:176–80. doi: 10.1159/000087447
19. Gao X, Simon KC, Han J, Schwarzschild MA, Ascherio A. Family history of melanoma and Parkinson disease risk. Neurology. (2009) 73:1286–91. doi: 10.1212/WNL.0b013e3181bd13a1
20. Baade PD, Fritschi L, Freedman DM. Mortality due to amyotrophic lateral sclerosis and Parkinson’s disease among melanoma patients. Neuroepidemiology. (2007) 28:16–20. doi: 10.1159/000097851
21. Spillantini MG, Crowther RA, Jakes R, Hasegawa M, Goedert M. Alpha-synuclein in filamentous inclusions of lewy bodies from Parkinson’s disease and dementia with lewy bodies. Proc Natl Acad Sci U.S.A. (1998) 95:6469–73. doi: 10.1073/pnas.95.11.6469
22. Baba M, Nakajo S, Tu PH, Tomita T, Nakaya K, Lee VM, et al. Aggregation of alpha-synuclein in lewy bodies of sporadic Parkinson’s disease and dementia with lewy bodies. Am J Pathol. (1998) 152:879–84.
23. Emamzadeh FN, Surguchov A. Parkinson’s disease: biomarkers, treatment, and risk factors. Front Neurosci. (2018) 12:612. doi: 10.3389/fnins.2018.00612
24. Xu J, Kao SY, Lee FJ, Song W, Jin LW, Yankner BA. Dopamine-dependent neurotoxicity of alpha-synuclein: A mechanism for selective neurodegeneration in Parkinson disease. Nat Med. (2002) 8:600–6. doi: 10.1038/nm0602-600
25. Ma LY, Liu GL, Wang DX, Zhang MM, Kou WY, Feng T. Alpha-synuclein in peripheral tissues in Parkinson’s disease. ACS Chem Neurosci. (2019) 10:812–23. doi: 10.1021/acschemneuro.8b00383
26. Fayyad M, Salim S, Majbour N, Erskine D, Stoops E, Mollenhauer B, et al. Parkinson’s disease biomarkers based on alpha-synuclein. J Neurochem. (2019) 150:626–36. doi: 10.1111/jnc.14809
27. Rodriguez-Leyva I, Chi-Ahumada E, Mejia M, Castanedo-Cazares JP, Eng W, Saikaly SK, et al. The presence of alpha-synuclein in skin from melanoma and patients with Parkinson’s disease. Mov Disord Clin Pract. (2017) 4:724–32. doi: 10.1002/mdc3.12494
28. Matsuo Y, Kamitani T. Parkinson’s disease-related protein, alpha-synuclein, in Malignant melanoma. PloS One. (2010) 5:e10481. doi: 10.1371/journal.pone.0010481
29. Welinder C, Jonsson GB, Ingvar C, Lundgren L, Baldetorp B, Olsson H, et al. Analysis of alpha-synuclein in Malignant melanoma - development of a srm quantification assay. PloS One. (2014) 9:e110804. doi: 10.1371/journal.pone.0110804
30. Shekoohi S, Rajasekaran S, Patel D, Yang S, Liu W, Huang S, et al. Knocking out alpha-synuclein in melanoma cells dysregulates cellular iron metabolism and suppresses tumor growth. Sci Rep. (2021) 11:5267. doi: 10.1038/s41598-021-84443-y
31. Israeli E, Yakunin E, Zarbiv Y, Hacohen-Solovich A, Kisos H, Loeb V, et al. Alpha-synuclein expression selectively affects tumorigenesis in mice modeling parkinson’s Disease. PloS One. (2011) 6:e19622. doi: 10.1371/journal.pone.0019622
32. Turriani E, Lazaro DF, Ryazanov S, Leonov A, Giese A, Schon M, et al. Treatment with diphenyl-pyrazole compound anle138b/C reveals that alpha-synuclein protects melanoma cells from autophagic cell death. Proc Natl Acad Sci U.S.A. (2017) 114:E4971–E7. doi: 10.1073/pnas.1700200114
33. Gajendran N, Rajasekaran S, Witt SN. Knocking out alpha-synuclein in melanoma cells downregulates L1cam and decreases motility. Sci Rep. (2023) 13:9243. doi: 10.1038/s41598-023-36451-3
34. Nandakumar S, Vijayan B, Kishore A, Thekkuveettil A. Autophagy enhancement is rendered ineffective in presence of alpha-synuclein in melanoma cells. J Cell Commun Signal. (2017) 11:381–94. doi: 10.1007/s12079-017-0402-x
35. Fokken C, Silbern I, Shomroni O, Pan KT, Ryazanov S, Leonov A, et al. Interfering with aggregated alpha-synuclein in advanced melanoma leads to a major upregulation of mhc class ii proteins. Melanoma Res. (2024) 34:393–407. doi: 10.1097/CMR.0000000000000982
36. Aloy NM, Coughlan C, Graner MW, Witt SN. Possible regulation of the immune modulator tetraspanin cd81 by alpha-synuclein in melanoma. Biochem Biophys Res Commun. (2024) 734:150631. doi: 10.1016/j.bbrc.2024.150631
37. Rajasekaran S, Cheng S, Gajendran N, Shekoohi S, Chesnokova L, Yu X, et al. Transcriptomic analysis of melanoma cells reveals an association of alpha-synuclein with regulation of the inflammatory response. Sci Rep. (2024) 14:27140. doi: 10.1038/s41598-024-78777-6
38. Niederberger E, Moller M, Mungo E, Hass M, Wilken-Schmitz A, Manderscheid C, et al. Distinct molecular mechanisms contribute to the reduction of melanoma growth and tumor pain after systemic and local depletion of alpha-synuclein in mice. FASEB J. (2023) 37:e23287. doi: 10.1096/fj.202301489R
39. Pollock PM, Cohen-Solal K, Sood R, Namkoong J, Martino JJ, Koganti A, et al. Melanoma mouse model implicates metabotropic glutamate signaling in melanocytic neoplasia. Nat Genet. (2003) 34:108–12. doi: 10.1038/ng1148
40. Zhu H, Reuhl K, Botha R, Ryan K, Wei J, Chen S. Development of early melanocytic lesions in transgenic mice predisposed to melanoma. Pigment Cell Res. (2000) 13:158–64. doi: 10.1034/j.1600-0749.2000.130307.x
41. Zhu H, Reuhl K, Zhang X, Botha R, Ryan K, Wei J, et al. Development of heritable melanoma in transgenic mice. J Invest Dermatol. (1998) 110:247–52. doi: 10.1046/j.1523-1747.1998.00133.x
42. Chen S, Zhu H, Wetzel WJ, Philbert MA. Spontaneous melanocytosis in transgenic mice. J Invest Dermatol. (1996) 106:1145–51. doi: 10.1111/1523-1747.ep12340194
43. Schaser AJ, Osterberg VR, Dent SE, Stackhouse TL, Wakeham CM, Boutros SW, et al. Alpha-synuclein is a DNA binding protein that modulates DNA repair with implications for lewy body disorders. Sci Rep. (2019) 9:10919. doi: 10.1038/s41598-019-47227-z
44. Rose EP, Osterberg VR, Gorbunova V, Unni VK. Alpha-synuclein modulates the repair of genomic DNA double-strand breaks in a DNA-pk(Cs)-regulated manner. Neurobiol Dis. (2024) 201:106675. doi: 10.1016/j.nbd.2024.106675
45. Schiffner S, Chen S, Becker JC, Bosserhoff AK. Highly pigmented tg(Grm1) mouse melanoma develops non-pigmented melanoma cells in distant metastases. Exp Dermatol. (2012) 21:786–8. doi: 10.1111/j.1600-0625.2012.01560.x
46. Groelly FJ, Fawkes M, Dagg RA, Blackford AN, Tarsounas M. Targeting DNA damage response pathways in cancer. Nat Rev Cancer. (2023) 23:78–94. doi: 10.1038/s41568-022-00535-5
47. Song L, Robson T, Doig T, Brenn T, Mathers M, Brown ER, et al. DNA repair and replication proteins as prognostic markers in melanoma. Histopathology. (2013) 62:343–50. doi: 10.1111/j.1365-2559.2012.04362.x
48. Puts G, Jarrett S, Leonard M, Matsangos N, Snyder D, Wang Y, et al. Metastasis suppressor nme1 modulates choice of double-strand break repair pathways in melanoma cells by enhancing alternative nhej while inhibiting nhej and hr. Int J Mol Sci. (2020) 21. doi: 10.3390/ijms21165896
49. Kauffmann A, Rosselli F, Lazar V, Winnepenninckx V, Mansuet-Lupo A, Dessen P, et al. High expression of DNA repair pathways is associated with metastasis in melanoma patients. Oncogene. (2008) 27:565–73. doi: 10.1038/sj.onc.1210700
50. Sever AIM, Alderson TR, Rennella E, Aramini JM, Liu ZH, Harkness RW, et al. Activation of caspase-9 on the apoptosome as studied by methyl-trosy nmr. Proc Natl Acad Sci U.S.A. (2023) 120:e2310944120. doi: 10.1073/pnas.2310944120
51. Liu S, Yao S, Yang H, Liu S, Wang Y. Autophagy: regulator of cell death. Cell Death Dis. (2023) 14:648. doi: 10.1038/s41419-023-06154-8
52. Kroemer G, Levine B. Autophagic cell death: the story of a misnomer. Nat Rev Mol Cell Biol. (2008) 9:1004–10. doi: 10.1038/nrm2529
53. Chen ZH, Lam HC, Jin Y, Kim HP, Cao J, Lee SJ, et al. Autophagy protein microtubule-associated protein 1 light chain-3b (Lc3b) activates extrinsic apoptosis during cigarette smoke-induced emphysema. Proc Natl Acad Sci U.S.A. (2010) 107:18880–5. doi: 10.1073/pnas.1005574107
54. Coppe JP, Rodier F, Patil CK, Freund A, Desprez PY, Campisi J. Tumor suppressor and aging biomarker P16(Ink4a) induces cellular senescence without the associated inflammatory secretory phenotype. J Biol Chem. (2011) 286:36396–403. doi: 10.1074/jbc.M111.257071
55. LaPak KM, Burd CE. The molecular balancing act of P16(Ink4a) in cancer and aging. Mol Cancer Res. (2014) 12:167–83. doi: 10.1158/1541-7786.MCR-13-0350
56. Szmurlo A, Dopytalska K, Szczerba M, Szymanska E, Petniak A, Kocki M, et al. The role of caspases in melanoma pathogenesis. Curr Issues Mol Biol. (2024) 46:9480–92. doi: 10.3390/cimb46090562
57. Berthenet K, Castillo Ferrer C, Fanfone D, Popgeorgiev N, Neves D, Bertolino P, et al. Failed apoptosis enhances melanoma cancer cell aggressiveness. Cell Rep. (2020) 31:107731. doi: 10.1016/j.celrep.2020.107731
58. Hartman ML. Non-apoptotic cell death signaling pathways in melanoma. Int J Mol Sci. (2020) 21. doi: 10.3390/ijms21082980
59. Fratta E, Giurato G, Guerrieri R, Colizzi F, Dal Col J, Weisz A, et al. Autophagy in braf-mutant cutaneous melanoma: recent advances and therapeutic perspective. Cell Death Discovery. (2023) 9:202. doi: 10.1038/s41420-023-01496-w
60. Soengas MS, Capodieci P, Polsky D, Mora J, Esteller M, Opitz-Araya X, et al. Inactivation of the apoptosis effector apaf-1 in Malignant melanoma. Nature. (2001) 409:207–11. doi: 10.1038/35051606
61. Soengas MS, Lowe SW. Apoptosis and melanoma chemoresistance. Oncogene. (2003) 22:3138–51. doi: 10.1038/sj.onc.1206454
62. Tang DY, Ellis RA, Lovat PE. Prognostic impact of autophagy biomarkers for cutaneous melanoma. Front Oncol. (2016) 6:236. doi: 10.3389/fonc.2016.00236
63. Gray-Schopfer VC, Cheong SC, Chong H, Chow J, Moss T, Abdel-Malek ZA, et al. Cellular senescence in naevi and immortalisation in melanoma: A role for P16? Br J Cancer. (2006) 95:496–505. doi: 10.1038/sj.bjc.6603283
64. Haferkamp S, Becker TM, Scurr LL, Kefford RF, Rizos H. P16ink4a-induced senescence is disabled by melanoma-associated mutations. Aging Cell. (2008) 7:733–45. doi: 10.1111/j.1474-9726.2008.00422.x
65. Constantinou SM, Bennett DC. Cell senescence and the genetics of melanoma development. Genes Chromosomes Cancer. (2024) 63:e23273. doi: 10.1002/gcc.23273
66. Jaune E, Cavazza E, Ronco C, Grytsai O, Abbe P, Tekaya N, et al. Discovery of a new molecule inducing melanoma cell death: dual ampk/melk targeting for novel melanoma therapies. Cell Death Dis. (2021) 12:64. doi: 10.1038/s41419-020-03344-6
67. Ibler AEM, ElGhazaly M, Naylor KL, Bulgakova NA, Fe-K S, Humphreys D. Typhoid toxin exhausts the rpa response to DNA replication stress driving senescence and salmonella infection. Nat Commun. (2019) 10:4040. doi: 10.1038/s41467-019-12064-1
68. Feringa FM, Raaijmakers JA, Hadders MA, Vaarting C, Macurek L, Heitink L, et al. Persistent repair intermediates induce senescence. Nat Commun. (2018) 9:3923. doi: 10.1038/s41467-018-06308-9
69. Shrivastav M, De Haro LP, Nickoloff JA. Regulation of DNA double-strand break repair pathway choice. Cell Res. (2008) 18:134–47. doi: 10.1038/cr.2007.111
70. Helleday T. Homologous recombination in cancer development, treatment and development of drug resistance. Carcinogenesis. (2010) 31:955–60. doi: 10.1093/carcin/bgq064
71. Kim KB, Soroceanu L, de Semir D, Millis SZ, Ross J, Vosoughi E, et al. Prevalence of homologous recombination pathway gene mutations in melanoma: rationale for a new targeted therapeutic approach. J Invest Dermatol. (2021) 141:2028–36 e2. doi: 10.1016/j.jid.2021.01.024
72. You Z, Lv M, He X, Pan Y, Ge J, Hu X, et al. Homologous recombination repair gene mutations as a predictive biomarker for immunotherapy in patients with advanced melanoma. Front Immunol. (2022) 13:871756. doi: 10.3389/fimmu.2022.871756
73. Fang M, Xia F, Mahalingam M, Virbasius CM, Wajapeyee N, Green MR. Men1 is a melanoma tumor suppressor that preserves genomic integrity by stimulating transcription of genes that promote homologous recombination-directed DNA repair. Mol Cell Biol. (2013) 33:2635–47. doi: 10.1128/MCB.00167-13
74. Chan WY, Brown LJ, Reid L, Joshua AM. Parp inhibitors in melanoma-an expanding therapeutic option? Cancers (Basel). (2021) 13. doi: 10.3390/cancers13184520
75. Brandsma I, Gent DC. Pathway choice in DNA double strand break repair: observations of a balancing act. Genome Integr. (2012) 3:9. doi: 10.1186/2041-9414-3-9
76. Moisan F, Kab S, Mohamed F, Canonico M, Le Guern M, Quintin C, et al. Parkinson disease male-to-female ratios increase with age: French nationwide study and meta-analysis. J Neurol Neurosurg Psychiatry. (2016) 87:952–7. doi: 10.1136/jnnp-2015-312283
77. Elbaz A, Bower JH, Maraganore DM, McDonnell SK, Peterson BJ, Ahlskog JE, et al. Risk tables for parkinsonism and Parkinson’s disease. J Clin Epidemiol. (2002) 55:25–31. doi: 10.1016/s0895-4356(01)00425-5
78. Reekes TH, Higginson CI, Ledbetter CR, Sathivadivel N, Zweig RM, Disbrow EA. Sex specific cognitive differences in Parkinson disease. NPJ Parkinsons Dis. (2020) 6:7. doi: 10.1038/s41531-020-0109-1
79. Cholerton B, Johnson CO, Fish B, Quinn JF, Chung KA, Peterson-Hiller AL, et al. Sex differences in progression to mild cognitive impairment and dementia in Parkinson's disease. Parkinsonism Relat Disord. (2018) 50:29–36. doi: 10.1016/j.parkreldis.2018.02.007
80. Liu R, Umbach DM, Peddada SD, Xu Z, Troster AI, Huang X, et al. Potential sex differences in nonmotor symptoms in early drug-naive Parkinson disease. Neurology. (2015) 84:2107–15. doi: 10.1212/WNL.0000000000001609
81. Scoggins CR, Ross MI, Reintgen DS, Noyes RD, Goydos JS, Beitsch PD, et al. Gender-related differences in outcome for melanoma patients. Ann Surg. (2006) 243:693–8. doi: 10.1097/01.sla.0000216771.81362.6b
82. Joosse A, de Vries E, Eckel R, Nijsten T, Eggermont AM, Holzel D, et al. Gender differences in melanoma survival: female patients have a decreased risk of metastasis. J Invest Dermatol. (2011) 131:719–26. doi: 10.1038/jid.2010.354
83. Joosse A, Collette S, Suciu S, Nijsten T, Patel PM, Keilholz U, et al. Sex is an independent prognostic indicator for survival and relapse/progression-free survival in metastasized stage iii to iv melanoma: A pooled analysis of five European organisation for research and treatment of cancer randomized controlled trials. J Clin Oncol. (2013) 31:2337–46. doi: 10.1200/JCO.2012.44.5031
84. Smith AJ, Lambert PC, Rutherford MJ. Understanding the impact of sex and stage differences on melanoma cancer patient survival: A seer-based study. Br J Cancer. (2021) 124:671–7. doi: 10.1038/s41416-020-01144-5
85. Joosse A, Collette S, Suciu S, Nijsten T, Lejeune F, Kleeberg UR, et al. Superior outcome of women with stage I/ii cutaneous melanoma: pooled analysis of four European organisation for research and treatment of cancer phase iii trials. J Clin Oncol. (2012) 30:2240–7. doi: 10.1200/JCO.2011.38.0584
86. Podolskiy DI, Lobanov AV, Kryukov GV, Gladyshev VN. Analysis of cancer genomes reveals basic features of human aging and its role in cancer development. Nat Commun. (2016) 7:12157. doi: 10.1038/ncomms12157
87. Winkelbeiner N, Wandt VK, Ebert F, Lossow K, Bankoglu EE, Martin M, et al. A multi-endpoint approach to base excision repair incision activity augmented by parylation and DNA damage levels in mice: impact of sex and age. Int J Mol Sci. (2020) 21. doi: 10.3390/ijms21186600
88. Yuan Y, Liu L, Chen H, Wang Y, Xu Y, Mao H, et al. Comprehensive characterization of molecular differences in cancer between male and female patients. Cancer Cell. (2016) 29:711–22. doi: 10.1016/j.ccell.2016.04.001
89. Carusillo A, Mussolino C. DNA damage: from threat to treatment. Cells. (2020) 9. doi: 10.3390/cells9071665
90. Mohiuddin IS, Kang MH. DNA-pk as an emerging therapeutic target in cancer. Front Oncol. (2019) 9:635. doi: 10.3389/fonc.2019.00635
91. Komatsu K. Nbs1 and multiple regulations of DNA damage response. J Radiat Res. (2016) 57 Suppl 1:i11–i7. doi: 10.1093/jrr/rrw031
92. Bowen C, Zheng T, Gelmann EP. Nkx3.1 suppresses tmprss2-erg gene rearrangement and mediates repair of androgen receptor-induced DNA damage. Cancer Res. (2015) 75:2686–98. doi: 10.1158/0008-5472.CAN-14-3387
93. Wilk A, Waligorska A, Waligorski P, Ochoa A, Reiss K. Inhibition of erbeta induces resistance to cisplatin by enhancing rad51-mediated DNA repair in human medulloblastoma cell lines. PloS One. (2012) 7:e33867. doi: 10.1371/journal.pone.0033867
Keywords: alpha-synuclein, melanoma, Parkinson’s disease, DNA damage response, double strand break (DSB)
Citation: Arnold MR, Chen S and Unni VK (2025) Alpha-synuclein knockout impairs melanoma development and alters DNA damage repair in the TG3 mouse model in a sex-dependent manner. Front. Oncol. 15:1554059. doi: 10.3389/fonc.2025.1554059
Received: 31 December 2024; Accepted: 26 February 2025;
Published: 20 March 2025.
Edited by:
Daniele Vergara, University of Salento, ItalyReviewed by:
Ellen Niederberger, University Hospital Frankfurt, GermanyCopyright © 2025 Arnold, Chen and Unni. This is an open-access article distributed under the terms of the Creative Commons Attribution License (CC BY). The use, distribution or reproduction in other forums is permitted, provided the original author(s) and the copyright owner(s) are credited and that the original publication in this journal is cited, in accordance with accepted academic practice. No use, distribution or reproduction is permitted which does not comply with these terms.
*Correspondence: Vivek K. Unni, dW5uaUBvaHN1LmVkdQ==
Disclaimer: All claims expressed in this article are solely those of the authors and do not necessarily represent those of their affiliated organizations, or those of the publisher, the editors and the reviewers. Any product that may be evaluated in this article or claim that may be made by its manufacturer is not guaranteed or endorsed by the publisher.
Research integrity at Frontiers
Learn more about the work of our research integrity team to safeguard the quality of each article we publish.