- 1Department of Medical Oncology and Hematology, University of Zurich and University Hospital Zurich, Zurich, Switzerland
- 2Institute of Molecular Cancer Research, University of Zurich, Zurich, Switzerland
- 3Pathology, Institute of Medical Genetics and Pathology, University Hospital Basel, University of Basel, Basel, Switzerland
Introduction: KMT2A-rearrangements define a subclass of acute leukemias characterized by a distinct gene expression signature linked to the dysfunctional oncogenic fusion proteins arising from various chromosomal translocations involving the KMT2A (also known as MLL1) gene. Research on the disease pathomechanism in KMT2A-rearranged acute leukemias has mainly focused on the upregulation of the stemness-related genes of the HOX-family and their co-factor MEIS1.
Results: Here we report the KMT2A::AFF1 and KMT2A::MLLT3 fusion gene-dependent downregulation of SMAD1, a TGF-β signaling axis transcription factor. SMAD1 expression is lost in the majority of AML patient samples and cell lines containing the two fusion genes KMT2A::AFF1 and KMT2A::MLLT3 compared to non-rearranged controls. Loss of SMAD1 expression is inducible by introducing the respective two KMT2A fusion genes into hematopoietic stem and progenitor cells. The loss of SMAD1 correlated with a markedly reduced amount of H3K4me3 levels at the SMAD1 promoter in tested cells with KMT2A::AFF1 and KMT2A::MLLT3. The expression of SMAD1 in cells with KMT2A::AFF1 fusion genes impacted the growth of cells in vitro and influenced engraftment of the KMT2A::AFF1 cell line MV4-11 in vivo. In MV4-11 cells SMAD1 expression caused a downregulation of HOXA9 and MEIS1, which was reinforced by TGF-β stimulation. Moreover, in MV4-11 cells SMAD1 presence sensitized cells for TGF-β mediated G1-arrest.
Conclusion: Overall, our data contributes to the understanding of the role of TGF-β signaling in acute myeloid leukemia with KMT2A::AFF1 by showing that SMAD1 loss can influence the growth dynamics and contribute to the pathogenic expression of disease driving factors.
Introduction
Acute leukemias with rearrangement of the Lysine Methyltransferase 2A (KMT2A, also known as MLL1) gene belong to the adverse risk group and are predominantly found in pediatric cases but also in adult acute lymphoblastic and myeloid leukemias (AML) (1, 2). More than a hundred fusion partners are described for translocations involving KMT2A (3, 4). Specific fusion genes have been linked to myeloid or lymphoid lineage disease (3).
KMT2A fusion genes and their respective fusion proteins are strong oncogenic drivers, which are sufficient to induce leukemic transformation in hematopoietic stem and progenitor cells (HSPCs) by eminently altering gene expression patterns (5–8). The two most important aberrantly expressed genes in KMT2A-rearranged leukemias are HOXA9 and MEIS1 since their upregulation was shown to be crucial for leukemogenesis (7, 9–11).
KMT2A is part of a multi-protein complex involved in chromatin modification and gene expression regulation. It is a histone-lysine-methyltransferase with a SET domain, which confers H3K4 mono-, di- and trimethylation activity to histone H3 (12–14). H3K4 trimethylation (H3K4me3) is associated with active transcription at promoter regions of genes (15, 16), and KMT2A chromatin occupancy correlates with H3K4me3 presence (15, 17). Other studies showed that H3K4me3 binding by plant homeodomain 3 (PHD3) finger of KMT2A is critical for transcriptional activity at HOXA9 and MEIS1 promoters (17). This implicates a role of H3K4me3 in KMT2A-dependent gene regulation. Upregulation of stemness-related and self-renewal-related genes, including HOXA9, other HOX family members and their co-factor MEIS1, constitute a key component of the pathomechanism of KMT2A-rearranged leukemogenesis (7, 9, 10, 18, 19).
SMAD1 is part of the transforming growth factor-β (TGF-β) and bone morphogenetic protein (BMP) signaling axes. The TGF-β pathway is crucial in the regulation of cellular processes such as cell growth, differentiation, homeostasis and regeneration throughout tissues. Intriguingly, TGF-β signaling can have opposing effects depending on the cell type and contexts it is active in (20). In hematopoietic cells, it contributes to the regulation of proliferation, differentiation, growth arrest and apoptosis depending on factors such as cell type, differentiation stage and environmental conditions (21, 22). It is widely acknowledged to be a potent negative regulator of hematopoiesis by maintaining hematopoietic stem cell (HSC) quiescence (23–25) and inhibiting the growth of early hematopoietic progenitors (26–28). One of the direct effects of TGF-β is G1 cell cycle arrest mediated by the downregulation of cyclin-dependent kinases (CDKs) and cyclins (29–31) or upregulation of CDK inhibitors such as p15INK4B, p21CIP1, p27KIP1 and p57KIP2 (32–39). During normal myeloid differentiation SMAD1 is highly expressed in HSCs, megakaryocyte-erythrocyte progenitors (MEPs) and granulocyte-monocyte progenitors (GMPs) (40). Thereafter, SMAD1 expression is gradually reduced during myeloid differentiation and consequently low in terminally differentiated cells (41). Interestingly, in the literature SMAD1 has not yet been mentioned in the context of KMT2A-rearranged leukemia. However, in diffuse large B-cell lymphoma (DLBCL) and Hodgkin lymphoma aberrant loss of SMAD1 expression has been involved in the pathogenesis of the disease (42, 43).
Transcriptomic changes in KMT2A-rearranged cells involve both upregulation and downregulation of KMT2A targets (12, 44). Here, we describe the loss of the transcription factor SMAD1 in KMT2A-rearranged AML with KMT2A::AFF1 and KMT2A::MLLT3 fusion genes by analyzing a panel of patient samples and selected cell lines. Moreover, we provide evidence for the potential of SMAD1 to influence leukemic propagation. The purpose of this study was to shed light on the role of TGF-β pathway components, such as SMAD1, in the disease mechanism of acute leukemias.
Materials and methods
CRISPR/Cas9 gene editing of KMT2A and SMAD1
We used a lentiviral delivery system for CRISPR/Cas9-mediated KMT2A-N and SMAD1 gene disruption in MV4-11 and MM6 cells. Lentiviral particles were generated in HEK293T cells, which were cultured in DMEM (Gibco, +10% FBS, +1% penicillin/streptomycin) and polyethylenimine (PEI, Polysciences, MW 25000) was added for plasmid transfection. HEK293T cells were plated in a 10 cm dish and 4 μg of the respective plasmid of interest, 2 μg of packaging plasmid psPAX2 (Addgene plasmid #12260), and 1 μg of envelope plasmid pCMV-VSV-G (Addgene plasmid #8454) were combined. At 48 hours post-transfection, MV4-11 and MM6 cells were infected with virus particles in the presence of polybrene. Antibiotic selection (puromycin and neomycin (G418)) was performed and selected bulk cultures were used for experiments. To generate the KMT2A N-terminal knockout (KO), the lentiCRISPRv2 neo (Addgene plasmid #98292) vector was used. KMT2A genomic target sequences (exon 2): sgRNA1: TTGTAGGATGAGCAATTCTT, sgRNA2: TCAGAGTGCGAAGTCCCACA. Sequence of the non-targeting control (NTC) sgRNA: GAACAGTCGCGTTTGCGACT. For the SMAD1 KO, the pKLV2-U6gRNA-PGK-puro-2A-BFP (Addgene plasmid #67974) vector was used with the sgRNA: TTAGCTCAGTTCCGTAACTT.
ChIP-qPCR
Four million (Mio) cells were crosslinked (10 minutes (min)) in 1% formaldehyde. Subsequently, cell lysis was performed which was followed by MNase digestion for 15 min and sonication for 3 cycles on ice for 30 seconds (s) each (1/10 per s pulse, 29-31% power) with a 30 s break between cycles. For sonication the Bandelin Sonopuls HD2070 machine was used. Input samples were collected after this step. DNA fragments were incubated with 2.5 ug H3K4me3 antibody (Cat #C15410030, Diagenode) or IgG control (ctrl) and afterward reverse crosslinked and purified. We used the Pierce™ Magnetic ChIP Kit (Thermo Fisher Scientific) according to the manufacturer’s instructions. Primers for the quantitative PCR (qPCR) were generated using Primer-BLAST in the Genome Data Viewer and designed to cover the H3K4me3 peak area. To locate the H3K4me3 peaks at the SMAD1 promoter in human hematopoietic cells (KMT2A WT), we used the tracks available at the NCBI Genome Data Viewer (45). Results were analyzed using the % Input method. Adjusted CT Input value (adj. CT IN): adj. CT IN = CT IN – (log2(Input dilution factor)). % Input: 100*(2^(adj. CT IN – CT IP)). Primers are listed in Supplementary Table 2 and antibodies are listed in Supplementary Table 3.
Cell cycle assay
Cells were seeded at a density of 0.5 Mio cells/ml in a 12-well plate with three technical replicates for each condition. TGF-β was used at a concentration of 2 ng/ml. The cell cycle phase was measured after 48 hours (h). Cells were stained in CytoPhase™ Violet (BioLegend Cat #425701) at a 1:250 ratio in medium and incubated for 1.5 h at 37°C in the incubator. Samples were analyzed using a BD LSRFortessa™ flow cytometer and data was analyzed with the FlowJo software package (TreeStar).
In vivo xenotransplantation and tissue processing
For the orthotopic xenotransplantation model, female NOD.Cg-PrkdcscidIl2rgtm1Wjl/SzJ (NSG) mice at 6-8 weeks of age were intravenously injected into the tail vein with 1x107 cells SMAD1 overexpressing green fluorescent protein (GFP) positive (SMAD1 OE) or empty vector control red fluorescent protein (RFP) positive (RFP ctrl) MV4-11 or MM6 cells in 100 µl PBS. When the first animals showed disease symptoms (rough fur, low activity, paleness), the study was terminated (30-31 days). Spleen cells and bone marrow isolated from the tibia and femur were harvested. Cells were treated with ACK red blood cell lysis buffer pH 7.2–7.4 (150 mM NH4Cl, 10 mM KHCO3, 0.1 mM Na2EDTA) and strained with a 40 μM cell strainer. Engraftment of MV4-11 cells in the spleen and the bone marrow was analyzed by flow cytometry. All animal studies were reviewed and approved by the Zurich Cantonal Veterinary Office (license 132/2019 and their amendments to A.M., license 035/2023 to A.T.).
Statistical analysis
We performed all statistical analyses on GraphPad Prism 9 (Version 9.5.1). For normally distributed data and comparisons between two groups, we used an unpaired Student’s t-test. For not normally distributed data and comparisons between two groups, we used Mann-Whitney test, also if data in only one of the groups was not normally distributed. For normally distributed data and comparisons between more than two groups we used a one-way ANOVA with Tuckey’s multiple comparisons test. For not normally distributed data and comparisons between more than two groups, we used the Kruskall-Wallis test together with Dunn’s multiple comparisons test, also if data in only one of the groups was not normally distributed. To test for correlation of two variables, we used the Spearman rank order correlation test. P-value summary: ns not significant p>0.05, *p < 0.05, **p < 0.005, ***p < 0.0005, ****p < 0.0001. Error bars represent mean ± standard deviation.
Results
SMAD1 expression is downregulated in AML with KMT2A rearrangement
In silico analysis of various hematopoietic malignancies showed that, SMAD1 expression is significantly heterogeneous in the peripheral blood and bone marrow, with particularly low levels observed in chronic lymphocytic leukemia (CLL) and acute leukemia with KMT2A rearrangements (Figure 1A) (41).
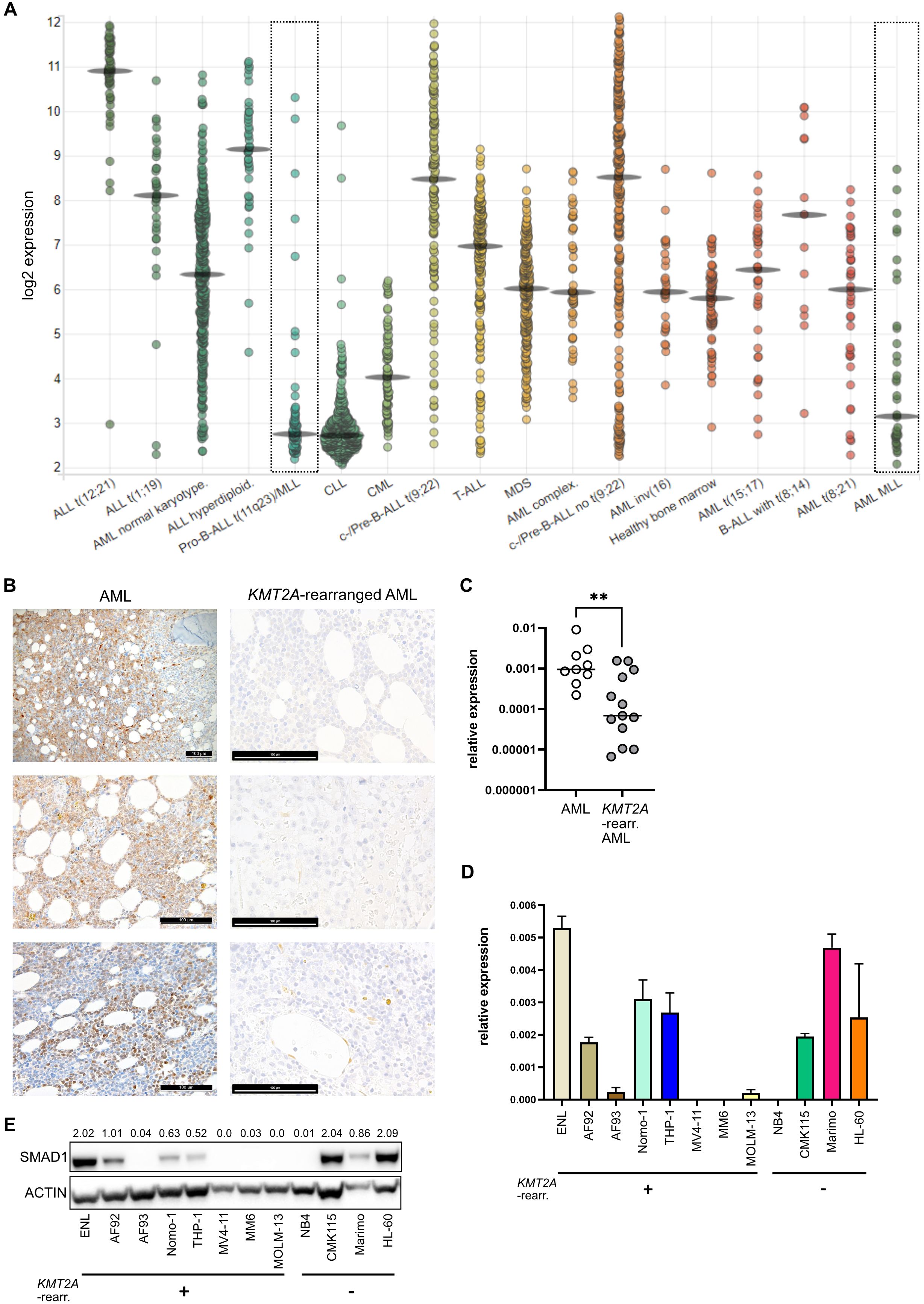
Figure 1. SMAD1 expression is decreased in KMT2A::MLLT3 and KMT2A::AFF1 containing AML patient samples and cell lines. (A) SMAD1 expression in hematologic cancers and healthy bone marrow, as determined by microarray data available on BloodSpot (41). (B) Immunohistochemical staining of SMAD1 in bone marrow sections of three representative AML patients without KMT2A rearrangement and three representative KMT2A-rearranged AML patients (all with KMT2A::MLLT3), scale bar represents 100 µM. (C) qPCR relative expression of SMAD1 in AML with and without KMT2A rearrangement (eleven KMT2A::MLLT3, one KMT2A::AFF1, one KMT2A::AFDN) patients (each dot represents one patient sample, each 3 technical replicates). (D) RT-qPCR relative expression of SMAD1 in AML cell lines with and without KMT2A rearrangement, 2-5 biological replicates per cell line, each 3 technical replicates. (E) Western blot of SMAD1 in AML cell lines with and without KMT2A rearrangement. Numbers display densitometry (adjusted lane volume normalized to the respective loading control). Statistics: in (C) Mann-Whitney-U test, **p<0.005.
To assess the baseline expression and expressional distribution of SMAD1 in normal bone marrow, we analyzed bone marrow biopsies from four healthy hematopoietic cell donors. Single erythroid precursors within erythrons and HSCs displayed positive SMAD1 staining, while almost all other bone marrow cells remained negative (Supplementary Figure 1A). Next, we analyzed bone marrow specimens from AML patients, each six with and without KMT2A rearrangement. Four of the six KMT2A-rearranged samples contained the KMT2A::MLLT3 fusion gene, one the SEPTIN9 fusion gene and one an unknown fusion partner. SMAD1 expression was absent in all four samples with KMT2A::MLLT3 in comparison to other AML subtypes (Figure 1B), confirming the transcriptional data retrieved from the public database BloodSpot. SMAD1 expression in the other two KMT2A-rearranged samples was dim but detectable (Supplementary Figure 3B). Significantly lower SMAD1 expression was confirmed by reverse transcriptase quantitative PCR (RT-qPCR) analysis of a different cohort including bone marrow samples from AML patients with and without KMT2A rearrangement (Figure 1C). We next aimed to identify AML cell lines with KMT2A rearrangement and low SMAD1 expression for mechanistic in vitro studies. Additionally, we assessed SMAD1 expression in human cord blood (CB)-derived cell lines genetically modified to carry KMT2A fusion genes KMT2A::MLLT1 (ELN) and KMT2A::MLLT3 (AF92 and AF93) (46). Both, RT-qPCR and Western blot analyses revealed that the three KMT2A-rearranged cell lines MV4-11 (KMT2A::AFF1), MM6 (KMT2A::MLLT3) and MOLM-13 (KMT2A::MLLT3) and the CB-derived cell line AF93 (KMT2A::MLLT3), recapitulate the low expression levels of SMAD1 observed in AML patients with KMT2A rearrangement. Of note, the KMT2A::MLLT3 containing cell lines Nomo-1 and THP-1 showed an intermediate SMAD1 expression, not a complete loss. In contrast, the CB-derived cell line with KMT2A::MLLT1 demonstrated comparably high SMAD1 levels, consistent with findings in primary T-ALL patient cells with KMT2A::MLLT1 (47) (Figures 1D, E). Overall, these findings demonstrate an evident correlation between KMT2A::AFF1 and KMT2A::MLLT3 fusion genes and low SMAD1 levels.
Loss of SMAD1 is linked to the presence of KMT2A fusion proteins
To investigate whether SMAD1 expression correlates with the presence of the KMT2A fusion proteins KMT2A::AFF1 and KMT2A::MLLT3, we next employed CRISPR/Cas9 technology to genetically reduce KMT2A fusion gene expression in MM6 and MV4-11 cells. We used a single-guide RNA (sgRNA) designed to target the N-terminal part of the KMT2A gene, which is conserved in most KMT2A fusion genes (Figure 2A; Supplementary Figure 2A). Notably, by targeting the N-terminal region of the KMT2A gene the wild-type KMT2A allele may also be affected. The CRISPR/Cas9 editing led to a 50% reduction of the KMT2A-N-terminal mRNA, while the KMT2A-C-terminal gene expression remained unaffected, which confirmed the on-target editing effect (Figure 2B). We observed a 3-fold increase in SMAD1 mRNA in MM6 and a 2-fold increase in MV4-11 cells by RT-qPCR analysis (Figure 2C). Moreover, the densitometry of the western blots showed that also protein levels of SMAD1 were increased in KMT2A-N KD MV4-11 (173%) and MM6 (122%) cells compared to the respective NTC controls (Figure 2D). To confirm that SMAD1 expression levels are directly affected by KMT2A fusion proteins, we examined CB-derived CD34+ cells containing common KMT2A fusion genes with different breakpoints that had been engineered by CRISPR/Cas9-mediated gene editing (48). In line with the data generated in cell lines, cells derived from various CB donors showed a strong reduction of SMAD1 mRNA levels upon introduction of the fusion genes KMT2A::AFF1 and KMT2A::MLLT3 (Figure 2E). Specifically, KMT2A::MLLT3 with intron 9 breakpoint caused a reduction to 11%, while KMT2A::MLLT3 and KMT2A::AFF1 with an intron 11 breakpoint to 0.01% of SMAD1 mRNA levels of CD34+ CB cells cultured for 21 days. Similar effects were observed at the protein level, where the densitometry showed that KMT2A::AFF1 and KMT2A::MLLT3 containing cells have 2-3 fold lower SMAD1 protein levels compared to CD34+ CB control cells (Figure 2F). These results infer a direct role of KMT2A fusion genes and presumably KMT2A fusion proteins, in the loss of SMAD1 expression.
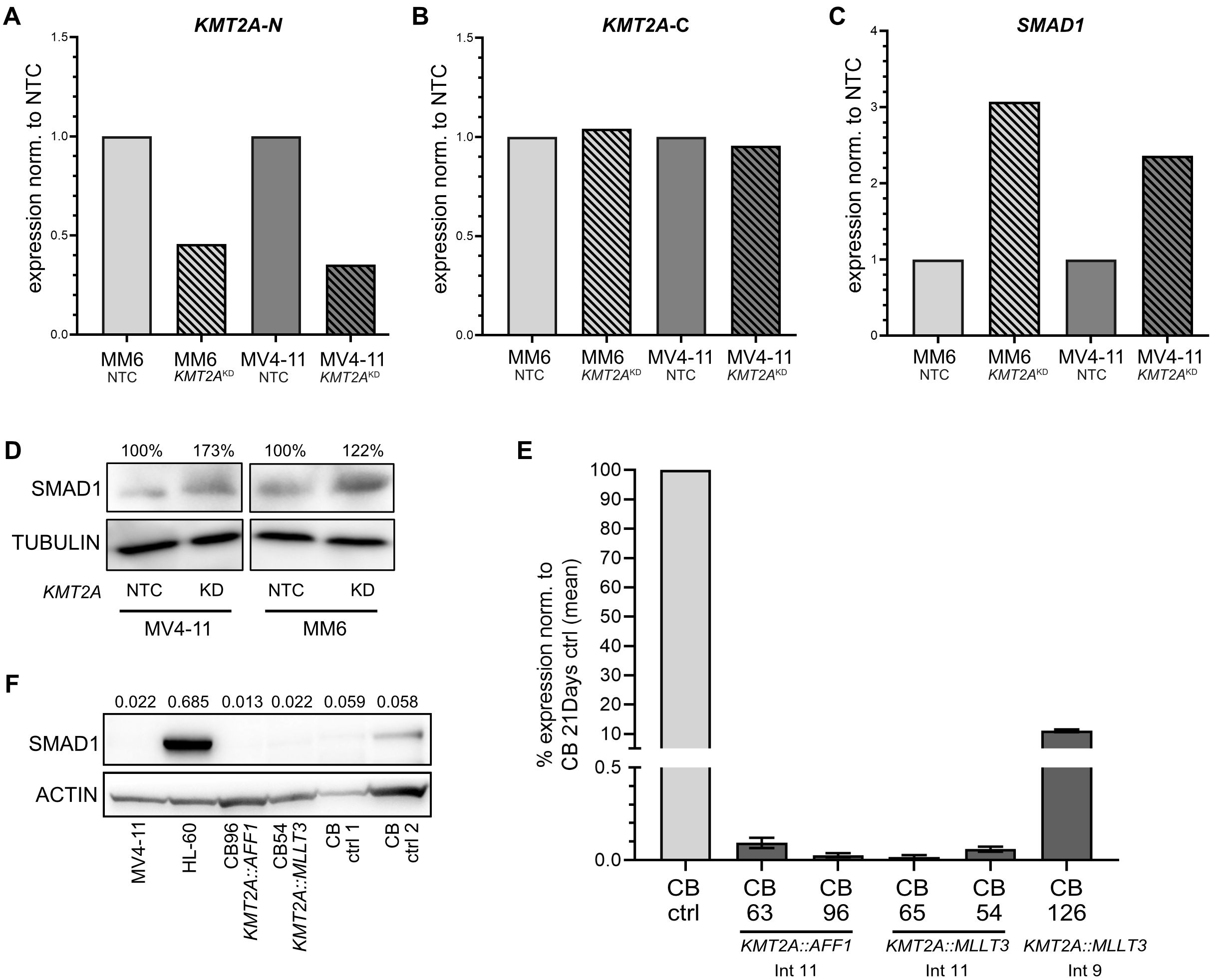
Figure 2. SMAD1 expression is linked to KMT2A::MLLT3 and KMT2A::AFF1 containing AML cell lines and cord blood cells. (A) CRISPR/Cas9-mediated KMT2A-N KD in MM6 (KMT2A::MLLT3) and MV4-11 (KMT2A::AFF1) cells. RT-qPCR expression of KMT2A-N normalized to NTC (B), expression of KMT2A-C normalized to NTC (C) and expression of SMAD1 normalized to NTC. For (A-C) (3 technical replicates) (D) Western blot of SMAD1 in MV4-11 and MM6 with KMT2A-N KD and NTC (long exposure time), percent values are based on densitometry (adjusted lane volume normalized to respective loading control, KD normalized to NTC). (E) RT-qPCR expression of SMAD1 in CB-derived cell lines with KMT2A::AFF1 and KMT2A::MLLT3 with different intron breakpoints normalized to cultured CD34+ CB-derived control cells (mean expression of CB ctrl 1 and CB ctrl 2), 3 technical replicates. (F) Western blot of SMAD1 in KMT2A-rearranged cell lines, CB-derived cell lines with KMT2A::AFF1 and KMT2A::MLLT3 and cultured CD34+ CB-derived control cells. Numbers display densitometry (adjusted lane volume normalized to the respective loading control).
H3K4me3 levels at the SMAD1 promoter are reduced in AML with KMT2A::AFF1 and KMT2A::MLLT3
To determine the mechanism of SMAD1 downregulation in the presence of KMT2A fusion genes, we sought to investigate the role of epigenetic alterations. In diffuse large B-cell lymphoma (DLBCL), DNA hypermethylation at the SMAD1 promoter leads to SMAD1 and subsequent surface receptor sphingosine 1 phosphate receptor 2 (S1PR2) loss, a process that is reversible by treatment with hypomethylating agents (43, 49). However, available Cancer Cell Line Encyclopedia (CCLE) datasets (50) indicate the absence of hypermethylation of the SMAD1 promoter and reveal no correlation between its methylation and KMT2A rearrangement status (Supplementary Figures 3A, 3B). Since H3K4 trimethylation belongs to the enzymatic functions of KMT2A and the enzymatic domain is lost in KMT2A fusion proteins we analyzed the H3K4me3 status of the SMAD1 promoter in KMT2A WT and KMT2A-rearranged cells using chromatin immunoprecipitation coupled with qPCR (ChIP-qPCR) in AML cell lines. H3K4me3 levels at the SMAD1 promoter in KMT2A WT and SMAD1 positive HL-60 cells were significantly higher than in SMAD1 negative MV4-11, MM6 and MOLM-13 cells (12.4% input versus 1.1%, 0.7% and 3.7% input, respectively, Figure 3A). CMK115 cells, which are KMT2A WT and SMAD1 positive also showed a similar yet not significant trend of higher H3K4me3 levels at the SMAD1 promoter compared to the three tested KMT2A-rearranged cell lines. Next, we performed the same analysis for the two CRISPR-engineered CB-derived cell lines, CB96 KMT2A::AFF1 intron 11 (4.0% input) and CB54 KMT2A::MLLT3 intron 11 (1.6% input), and compared it to control CD34+ CB-derived cells (CB ctrl 2, 12.6% input). Hence, KMT2A::AFF1 and KMT2A::MLLT3 containing CB-derived cell lines also showed significantly lower H3K4me3 levels at the SMAD1 promoter compared to CB-derived CD34+ cells (Figure 3B). Considering the role of H3K4 trimethylation in the regulation of gene transcription, these results suggest that reduced H3K4me3 levels at the SMAD1 promoter in KMT2A-rearranged cells could contribute to the loss of SMAD1 expression.
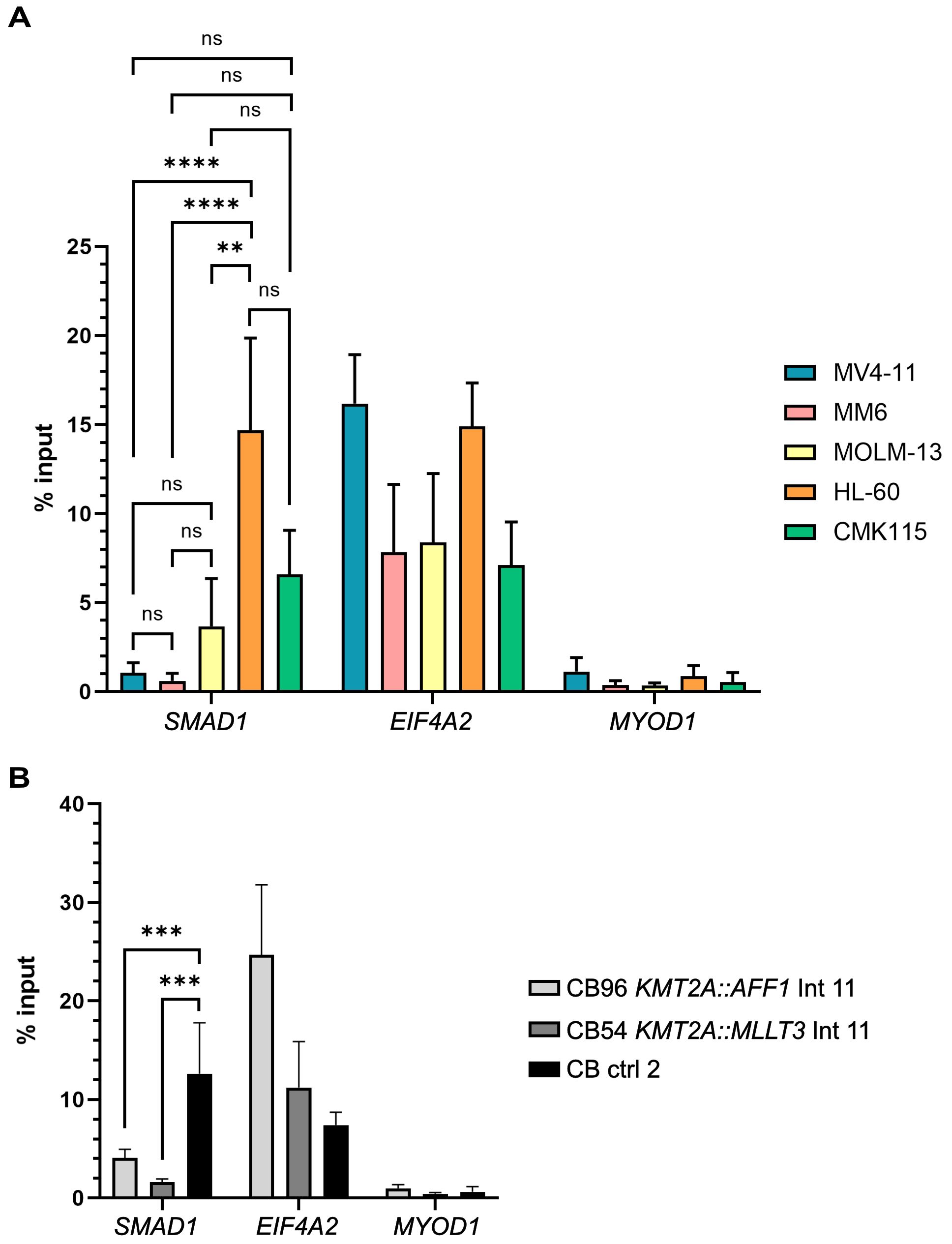
Figure 3. H3K4 trimethylation is reduced at the SMAD1 locus in KMT2A::MLLT3 and KMT2A::AFF1 containing AML cell lines and cord blood cells. (A) ChIP-qPCR of H3K4me3 at the SMAD1, EIF4A2 (positive control), MYOD1 (negative control) promoters in AML cell lines; three biological replicates for all cell lines except CMK115 (two biol. repl.), two biological replicates contain two technical replicates and one biological replicate contains three technical replicates. (B) ChIP-qPCR of H3K4me3 at SMAD1, EIF4A2 (positive control), MYOD1 (negative control) promoters in CB-derived cell lines with KMT2A::AFF1 and KMT2A::MLLT3 and cultured CD34+ CB-derived control cells (CB ctrl 2), two biological replicates with once two and once three technical replicates. Statistics: in (A, B) one-way ANOVA with Tukey’s multiple comparisons test, ns p>0.05, **p<0.005, ***p<0.0005, ****p<0.0001.
SMAD1 re-expression causes cell cycle arrest and downregulation of HOXA9 and MEIS1 expression in MV4-11 cells
Next, we aimed to investigate the role of SMAD1 in cellular processes influenced by TGF-β such as cell cycle and proliferation in cells with KMT2A::AFF1 and KMT2A::MLLT3 fusion genes. To study a potential growth-reducing effect of the SMAD1 rescue (shown in Figures 2C, D), we generated a SMAD1 KO in MV4-11 and MM6 cells with KMT2A fusion gene KD. KMT2A fusion gene KD cells combined with a non-targeting control are capable of re-expressing SMAD1, while KMT2A fusion gene KD cells with an additional SMAD1 KO are not. This allows to attribute differences in functional assays to the re-expression of SMAD1. Co-culture of MV4-11 cells containing the two different combinations revealed an outgrowth of KMT2A::AFF1 KD/SMAD1 KO cells, the cells that lack SMAD1 re-expression capacity (Figure 4A). However, in MM6 cells no clear outgrowth of cells with KMT2A::MLLT3 KD/SMAD1 KO was detected (Figure 4A). These results show that re-expression of SMAD1 upon KD of different KMT2A fusion genes in two cell lines can have diverging effects.
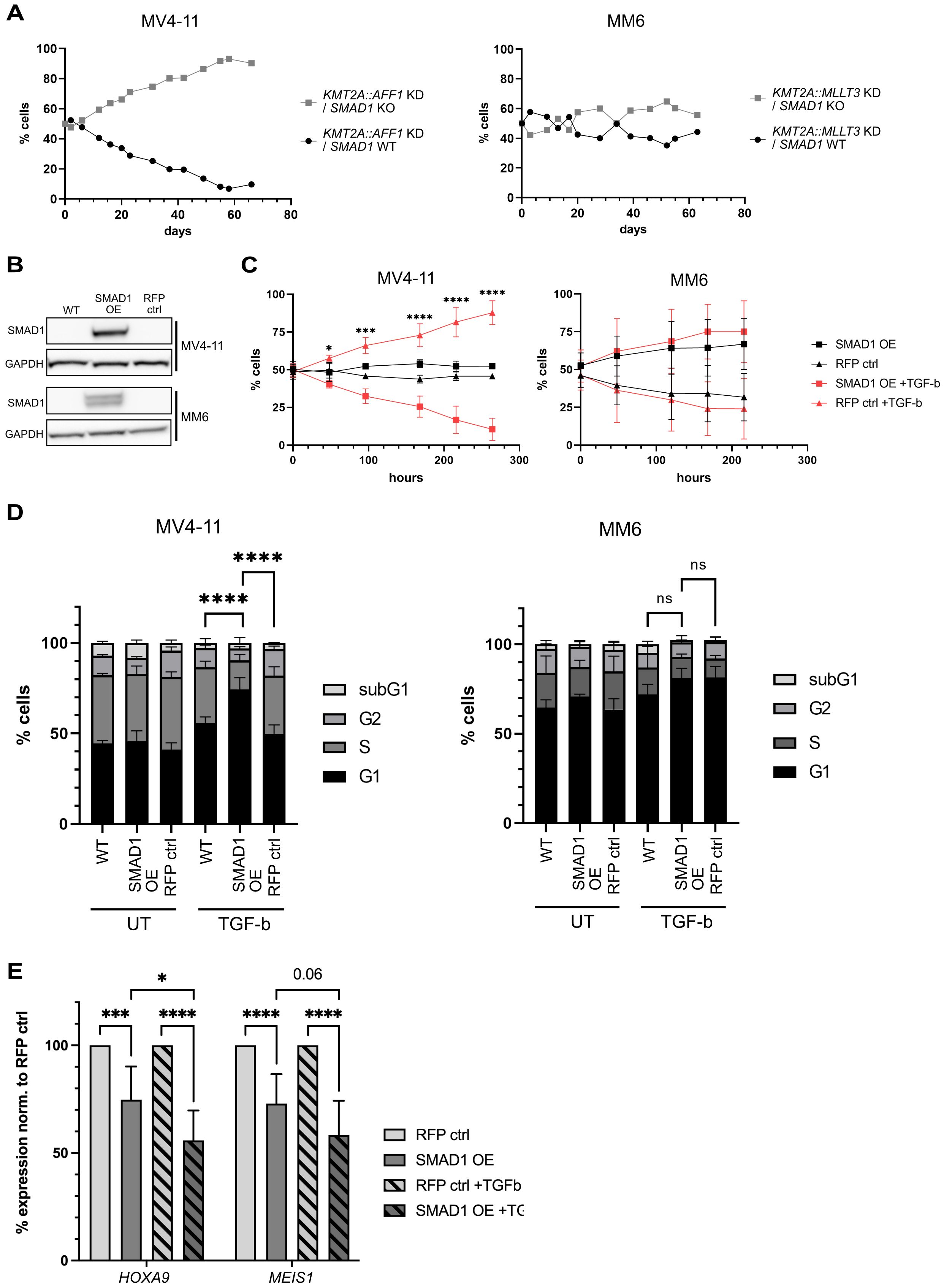
Figure 4. SMAD1 overexpression in KMT2A::MLLT3 and KMT2A::AFF1 containing AML cell lines has diverging effects on growth, cell cycle and expression of HOXA9 and MEIS1. (A) Growth competition assay with KMT2A KD vs KMT2A KD/SMAD1 KO in MV4-11 and MM6 cells, t=0 ratio 1:1. (B) Western blot SMAD1 levels upon SMAD1 overexpression (OE) in MV4-11 and MM6 cells. (C) Growth competition assays of MV4-11 and MM6 cells SMAD1 OE vs RFP control (ctrl) (statistics for MV4-11: comparison between SMAD1 OE +TGF-β and RFP ctrl +TGF-β), two biological replicates. (D) Cell cycle assay with MV4-11 and MM6 cells treated as indicated; % of cells in indicated cell cycle phases, 48h treatment, TGF-β 2 ng/ml (three biological replicates for MV4-11 and two biological replicates for MM6 with each three technical replicates of each condition, comparison of G1 phase). (E) HOXA9 and MEIS1 expression in MV4-11 cells with and without SMAD1 expression, three biological replicates with three technical replicates each. Statistics: in (C-E) unpaired Student’s t-test, ns p>0.05, *p<0.05, ***p<0.0005, ****p<0.0001.
To better understand the growth-regulating effects of SMAD1, we overexpressed SMAD1 (SMAD1 OE) in SMAD1 negative KMT2A-rearranged cell lines MV4-11 and MM6, and performed in vitro growth competition assays with empty vector control cells (RFP ctrl) (Figure 4B). Additionally, we assessed the importance of pathway activation for SMAD1-dependent effects, by adding TGF-β to the co-cultures. In MV4-11 cells, SMAD1 expression without TGF-β did not affect cell growth. However, in the presence of TGF-β, there was a prominent growth disadvantage of SMAD1-expressing cells compared to control cells (Figure 4C). In contrast, neither SMAD1 alone nor the addition of TGF-β significantly affected cell growth in MM6 cells (Figure 4C). SMAD1 enabled growth reduction in MV4-11 cells, while MM6 cells were insensitive to both SMAD1 and additional TGF-β pathway activation. To further reveal underlying mechanistic reasons for this discrepancy, we performed cell cycle analysis, given the well-known role of TGF-β signaling in cell cycle regulation, especially in hematopoietic cells (26, 51, 52). SMAD1-expressing MV4-11 cells displayed a significant G1-arrest of 30% increase upon treatment with TGF-β compared to wild-type (WT) and RFP ctrl cells, which showed a minor response to TGF-β treatment (Figure 4D). On the contrary, in MM6 cells, SMAD1 expression combined with TGF-β treatment did not significantly change the cell cycle phase distribution compared to WT and RFP ctrl cells (Figure 4D), consistent with the results of the growth competition assay (Figure 4C).
We tested the effect of SMAD1 on HOXA9 and MEIS1 expression levels with and without TGF-β. Indeed, SMAD1 significantly decreased HOXA9 mRNA levels by 25%, which was further decreased by the addition of TGF-β to 44% (Figure 4E). MEIS1 mRNA levels were decreased by 27% upon SMAD1 expression and TGF-β addition caused a 41% reduction. The expression of both genes was normalized to their expression in RFP control cells with and without TGF-β. Overall, these findings suggest that SMAD1 can play a role in growth regulation by cell cycle control and downregulation of the critical oncogenes HOXA9 and MEIS1 in KMT2A::AFF1 containing MV4-11 cells.
SMAD1 reduces engraftment of AML cells with KMT2A::AFF1 in vivo
To validate the growth-reducing effects of SMAD1 in vivo, we tested SMAD1 OE and RFP ctrl MV4-11 cells in a xenotransplantation model, where 10 Mio cells were injected intravenously into the tail vein of NSG (NOD.Cg-Prkdcscid Il2rgtm1Wjl/SzJ) mice. Four weeks post transplantation, mice were euthanized and the engraftment of human CD45+ cells in the bone marrow and the spleen was determined. We detected a significant reduction in bone marrow and spleen engraftment in mice transplanted with SMAD1 OE cells compared to mice transplanted with RFP ctrl cells (Figures 5B, C). This was also reflected by a significantly lower spleen weight in mice transplanted with SMAD1 OE cells (Figure 5A).
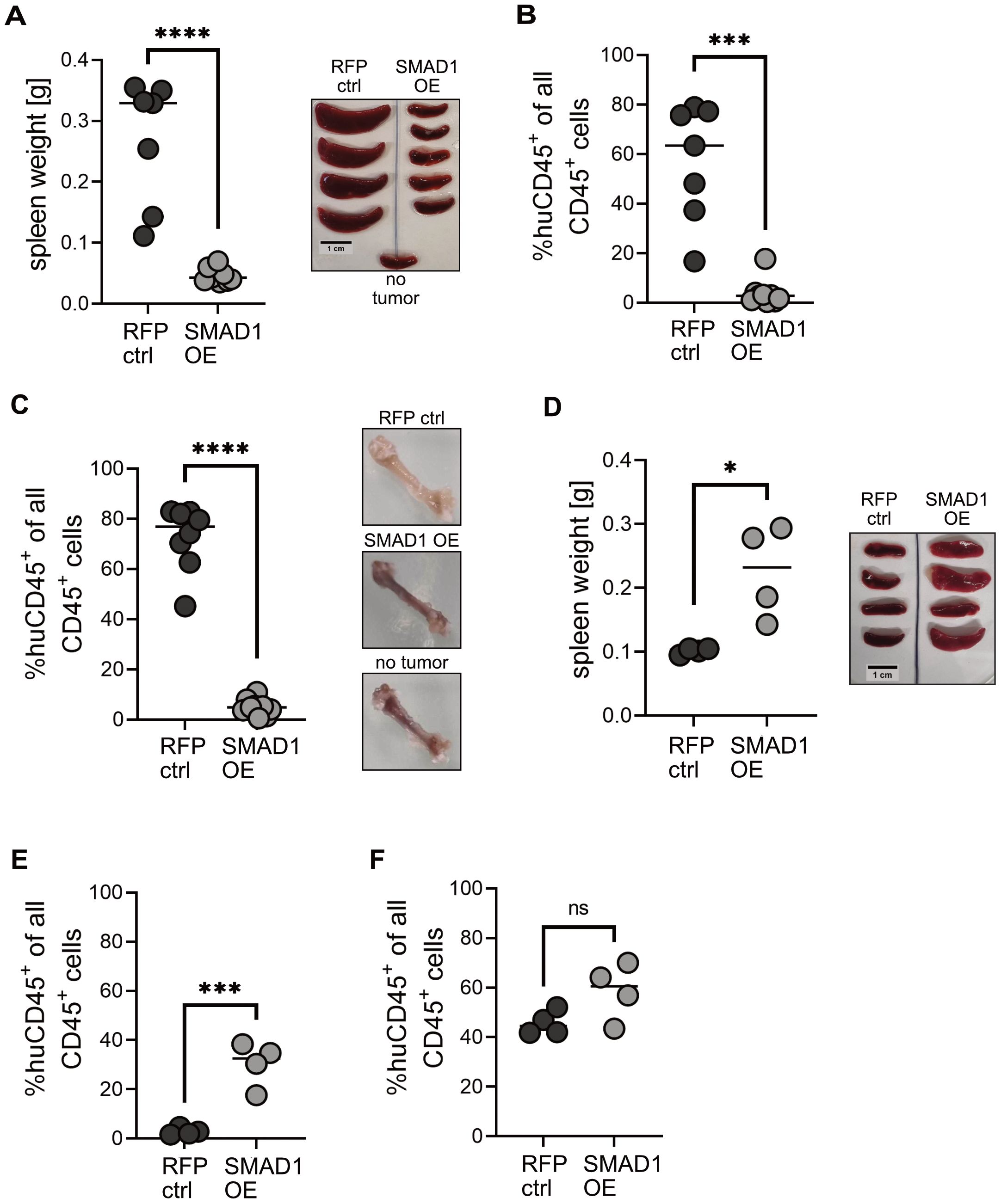
Figure 5. SMAD1 overexpression in a KMT2A::AFF1 cell line-derived xenograft model reduces engraftment in the bone marrow and spleen. (A) Spleen weight and representative spleen images of NSG mice transplanted with indicated MV4-11 cells. (B) Comparison of % human CD45+ cell engraftment in the spleen and (C) bone marrow of NSG mice 31 days after orthotopic transplantation (10 Mio cells, i.v. tail vein, mice 6-8 weeks old) of MV4-11 cells with SMAD1 overexpression (SMAD1 OE) or RFP control (RFP ctrl), with representative femur images. (D) Spleen weight and representative spleen images of NSG mice transplanted with indicated MM6 cells. (E) Comparison of % human CD45+ cell engraftment in the spleen and (F) bone marrow of NSG mice 31 days after orthotopic transplantation (10 Mio cells, i.v. tail vein, mice 6-8 weeks old) of MM6 cells with SMAD1 overexpression (SMAD1 OE) or RFP control (RFP ctrl). Each dot represents one mouse, in (A), (C-F) unpaired Student’s t-test, in (B) Mann-Whitney-U test. ns p>0.05, *p>0.05, ***p<0.0005, ****p<0.0001.
Conversely, we observed inconsistent engraftment differences in the comparative xenotransplantation with SMAD1 OE and RFP ctrl MM6 cells. SMAD1 OE MM6 cells caused higher spleen weight (Figure 5D) and reached higher engraftment levels in the spleen (Figure 5E), contrasting with the findings in MV4-11 cells. The engraftment in the bone marrow did not significantly differ between SMAD1 OE and RFP ctrl MM6 cells (Figure 5F).
These results support the in vitro data. Interestingly, we observed higher overall engraftment of MV4-11 cells in female compared to male NSG mice (Supplementary Figure 4B), which was also observed in earlier studies investigating the engraftment of human HSCs (53).
Discussion
We here provide evidence that SMAD1 expression is lost or reduced in the majority of AML patient samples and seven AML cell lines, harboring KMT2A::MLLT3 and KMT2A::AFF1 fusion genes (three cell lines with reduced and four with absent SMAD1 expression). Notably, one out of four non-rearranged cell lines also showed lack of SMAD1 expression. We demonstrate that SMAD1 repression is dependent on the presence of KMT2A::MLLT3 and KMT2A::AFF1 fusion genes and that attenuation of the expression of these two fusion genes leads to the re-expression of SMAD1 in MV4-11 and MM6 cells. Moreover, we confirmed the impact of KMT2A::MLLT3 and KMT2A::AFF1 fusion genes on SMAD1 expression in CB-derived cells. These results confirm previous studies that have shown that SMAD1 expression can be disturbed by KMT2A deficiency (12). In our study this pathobiology was observed in KMT2A::MLLT3 and KMT2A::AFF1 containing models and not in other models containing different KMT2A fusion genes.
Interestingly, SMAD1 repression seems to be a recurrent but not a coercive phenomenon in KMT2A-rearranged malignancies (41). One limitation of this study is that the assessment of the relationship between SMAD1 expression and KMT2A fusion genes was only performed for the two selected fusion genes KMT2A::MLLT3 and KMT2A::AFF1. Theoretically, there are multiple potential mechanisms for the diverging effects of KMT2A fusion genes on SMAD1 expression such as different interaction partners recruited into the KMT2A fusion protein complex or variable activity of counteractive SMAD1 regulating pathways.
The loss or disruption of TGF-β pathway components is a pattern observed in other malignancies (54). For example SMAD1 levels are particularly low in DLBCL subtypes (43), but also in Hodgkin lymphoma (42). In T-ALL patient cells, absence of SMAD3 has been reported, correlating with insensitivity to growth-inhibitory effects of TGF-β. While SMAD3 heterozygous or homozygous KO was insufficient to induce T-cell leukemogenesis, it showed growth-promoting effects when combined with homozygous KO of the tumor suppressor p27Kip1 (55). Interestingly, despite its oncogenic potential, inactivating mutations involving TGF-β pathway components are surprisingly rare in hematopoietic malignancies with only a few instances reported, such as TGFβRII mutations in T-ALL (56, 57). Although SMAD4 has been described as a tumor suppressor protecting from the leukemic transformation of HSPCs (58), only very few cases of SMAD4 mutations in leukemia have been reported (21, 56, 59).
We showed reduced H3K4me3 levels at the SMAD1 promoter in KMT2A-rearranged cell lines, representing a first hint on a potential mechanism for the loss of SMAD1. Yet, at this stage this finding is just an observation without a proof for a causal relationship, which represents an evident limitation of this study. The interaction of KMT2A with H3K4me3 marks was shown to be important for transcription of KMT2A target genes (60, 61). In general H3K4me3 is found at active gene promoters (16), and low H3K4me3 levels are linked to reduced transcriptional output (62). Conversely, although the expression of KMT2A target genes was altered in KMT2A-deficient hematopoietic cells, the levels of H3K4 methylation remained stable, indicating that target gene expression and KMT2A fusion protein-driven leukemogenesis are independent of the histone methyltransferase activity of KMT2A (63). This infers that potentially the reduction of H3K4me3 levels at the SMAD1 promoter is not caused by a lack of KMT2A fusion protein H3K4 trimethylation activity but might stem from the altered activity of other histone methyltransferases. Another study highlighted the importance of H3K4me3, generated by KMT2B (also known as MLL2), for the expression of certain KMT2A::MLLT3 fusion protein target genes and reduced cell viability of KMT2A-rearranged cells (64). This suggests that KMT2B is a potential source for H3K4me3 and is crucial for KMT2A WT and KMT2A fusion protein-dependent gene expression. Understanding whether the H3K4 trimethylation activity of KMT2B is disturbed in KMT2A-rearranged leukemia, and particularly in context with KMT2A::AFF1 and KMT2A::MLLT3 fusion genes, represents an intriguing next chapter in illuminating the pathobiology of KMT2A-rearranged leukemia.
TGF-β signaling is a negative regulator in HSPCs (23–28, 55). This study contributes to a deeper understanding of TGF-β signaling and the role of SMAD1, particularly concerning its potential effects on tumor growth in KMT2A-rearranged leukemia. In vitro, the growth-reducing effect of SMAD1 in MV4-11 cells was only unleashed by the addition of TGF-β. In our xenotransplantation model, we refrained from administering TGF-β to the mice during the study due to the high homology between human and murine TGF-β (65) and since TGF-β is expressed in sufficient amounts in the bone marrow of mice (65, 66). The diminished growth of SMAD1 expressing MV4-11 cells was confirmed in vivo. Loss of TGF-β signaling has been implicated in leukemic progression, due to increased cell cycle induction in HSCs (21). Our findings suggest that the loss of SMAD1 in KMT2A-rearranged leukemia with KMT2A::AFF1 promotes tumor growth. The growth-reducing effects were characterized by TGF-β-dependent G1-arrest. However, in the scope of this study we only show this in the KMT2A::AFF1 AML (MV4-11) model, which restricts the impact of our findings and does not allow for extrapolation to other KMT2A:.AFF1 harboring leukemias.
Further, our data showed a dampening effect of SMAD1 presence combined with TGF-β stimulation on the expression of the two KMT2A-rearranged leukemia-driving genes HOXA9 and MEIS1, which has not been described in literature yet. A potential mechanism for this effect, at least for the part of HOXA9, is provided by the findings of Wang et al., which revealed that SMAD1, alongside SMAD4, can bind to HOXA9 upon TGF-β activation, thereby preventing HOXA9-induced gene expression and consequently impeding leukemic transformation (67). Notably, HOXA9 self-regulates its expression via a positive feedback loop and scavenging of HOXA9 by SMAD1 was shown to inhibit this effect (67). If the demonstrated moderate impact on the expression of these two important leukemic drivers in KMT2A-rearranged leukemia has a disease modifying effect remains to be confirmed, which will be part of future investigations.
The opposing results of SMAD1 expression in MM6 cells demonstrate that TGF-β signaling can have diverse outcomes in KMT2A-rearranged leukemia, compatible with the versatile nature of TGF-β signaling (20, 68). The effects of the TGF-β signaling pathway and its components are highly context-dependent and hence exhibit a duality in the context of carcinogenesis as well (20, 54, 69). The divergent effects of SMAD1 expression and TGF-β pathway activation between MV4-11 and MM6 cells may be influenced by factors impacting signaling outcomes, such as post-translational modifications of SMAD1. In mammalian cells, SMAD1 signaling is affected by Mitogen-activated protein kinase (MAPK) and Wingless-related integration site (Wnt) signaling, which phosphorylates its linker region, resulting in reduced nuclear translocation and degradation of SMAD1 respectively (70, 71). Western blot analysis of SMAD1 in MM6 cells showed a second, slightly heavier band than the main band of SMAD1 (Figure 4B). This second band could represent a post-translationally modified version of SMAD1, potentially influencing the signal transduction activity of SMAD1. In addition, other factors, such as interfering transcription factors, may influence the outcome of TGF-β pathway activation. In neuroepithelial and glioblastoma cells, the growth inhibitory effects mediated by the interaction of forkhead box (FoxO) transcription factors and SMADs were impeded by FoxG1 and caused reduced expression of the CDK inhibitor p21Cip1 (72). Although the interaction of FoxOs was shown for SMAD3 and SMAD4, SMAD1 signaling is likely to be affected as well, due to nuclear translocation in complex with SMAD4. Therefore, assessing the expression of factors such as FoxOs and FoxG1 in MV4-11 and MM6 cells could provide more information the diverging effects of SMAD1 in the two cell lines.
In conclusion, we showed that the loss of SMAD1 expression, in AML patient samples and cell lines with KMT2A::AFF1 and KMT2A::MLLT3, might contribute to leukemogenesis by influencing the response to TGF-β and the individual downstream effects of it.
Future studies could evaluate whether the pharmacological induction of SMAD1 expression may represent a therapeutic strategy in KMT2A-rearranged AML with KMT2A::AFF1 and KMT2A::MLLT3 fusion genes.
Data availability statement
The original contributions presented in the study are included in the article/Supplementary Material. Further inquiries can be directed to the corresponding author.
Ethics statement
The studies involving humans were approved by Kantonale Ethikkommission, Stampfenbachstrasse 121, 8090 Zürich. The studies were conducted in accordance with the local legislation and institutional requirements. The participants provided their written informed consent to participate in this study. The animal study was approved by Veterinäramt Kanton Zürich, Waltersbachstrasse 5, 8090 Zürich. The study was conducted in accordance with the local legislation and institutional requirements.
Author contributions
LD: Conceptualization, Data curation, Formal analysis, Investigation, Methodology, Project administration, Visualization, Writing – original draft, Writing – review & editing. KS: Conceptualization, Data curation, Formal Analysis, Investigation, Methodology, Project administration, Visualization, Writing – review & editing. VL: Investigation, Methodology, Writing – review & editing. CS: Writing – review & editing, Resources. AT: Investigation, Writing – review & editing. AM: Conceptualization, Funding acquisition, Project administration, Resources, Supervision, Validation, Writing – review & editing. APAT: Conceptualization, Funding acquisition, Project administration, Resources, Supervision, Validation, Writing – review & editing, Writing – original draft.
Funding
The author(s) declare financial support was received for the research, authorship, and/or publication of this article. APAT and AM were supported by the University of Zurich Clinical Research Priority Program “Next Generation Drug Response Profiling for Personalized Cancer Care”. AM and AT were supported by the Swiss Cancer League KFS-5228-02-2021.
Conflict of interest
The authors declare that the research was conducted in the absence of any commercial or financial relationships that could be construed as a potential conflict of interest.
Publisher’s note
All claims expressed in this article are solely those of the authors and do not necessarily represent those of their affiliated organizations, or those of the publisher, the editors and the reviewers. Any product that may be evaluated in this article, or claim that may be made by its manufacturer, is not guaranteed or endorsed by the publisher.
Supplementary material
The Supplementary Material for this article can be found online at: https://www.frontiersin.org/articles/10.3389/fonc.2024.1481713/full#supplementary-material
References
1. Balgobind BV, Raimondi SC, Harbott J, Zimmermann M, Alonzo TA, Auvrignon A, et al. Novel prognostic subgroups in childhood 11q23/MLL-rearranged acute myeloid leukemia: results of an international retrospective study. Blood. (2009) 114:2489–96. doi: 10.1182/blood-2009-04-215152
2. Estey EH. Acute myeloid leukemia: 2019 update on risk-stratification and management. Am J Hematol. (2018) 93:1267–91. doi: 10.1002/ajh.v93.10
3. Meyer C, Burmeister T, Gröger D, Tsaur G, Fechina L, Renneville A, et al. The MLL recombinome of acute leukemias in 2017. Leukemia. (2018) 32:273–84. doi: 10.1038/leu.2017.213
4. Collins EC, Rabbitts TH. The promiscuous MLL gene links chromosomal translocations to cellular differentiation and tumour tropism. Trends Mol Med. (2002) 8:436–42. doi: 10.1016/S1471-4914(02)02397-3
5. Krivtsov AV, Feng Z, Lemieux ME, Faber J, Vempati S, Sinha AU, et al. H3K79 methylation profiles define murine and human MLL-AF4 leukemias. Cancer Cell. (2008) 14:355–68. doi: 10.1016/j.ccr.2008.10.001
6. Forster A, Pannell R, Drynan LF, McCormack M, Collins EC, Daser A, et al. Engineering de novo reciprocal chromosomal translocations associated with Mll to replicate primary events of human cancer. Cancer Cell. (2003) 3:449–58. doi: 10.1016/S1535-6108(03)00106-5
7. Krivtsov AV, Twomey D, Feng Z, Stubbs MC, Wang Y, Faber J, et al. Transformation from committed progenitor to leukaemia stem cell initiated by MLL-AF9. Nature. (2006) 442:818–22. doi: 10.1038/nature04980
8. Schwaller J. Learning from mouse models of MLL fusion gene-driven acute leukemia. Biochim Biophys Acta Gene Regul Mech. (2020) 1863:194550. doi: 10.1016/j.bbagrm.2020.194550
9. Armstrong SA, Staunton JE, Silverman LB, Pieters R, den Boer ML, Minden MD, et al. MLL translocations specify a distinct gene expression profile that distinguishes a unique leukemia. Nat Genet. (2002) 30:41–7. doi: 10.1038/ng765
10. Ayton PM, Cleary ML. Transformation of myeloid progenitors by MLL oncoproteins is dependent on Hoxa7 and Hoxa9. Genes Dev. (2003) 17:2298–307. doi: 10.1101/gad.1111603
11. Thorsteinsdottir U, Kroon E, Jerome L, Blasi F, Sauvageau G. Defining roles for HOX and MEIS1 genes in induction of acute myeloid leukemia. Mol Cell Biol. (2001) 21:224–34. doi: 10.1128/MCB.21.1.224-234.2001
12. Artinger EL, Mishra BP, Zaffuto KM, Li BE, Chung EK, Moore AW, et al. An MLL-dependent network sustains hematopoiesis. Proc Natl Acad Sci U.S.A. (2013) 110:12000–5. doi: 10.1073/pnas.1301278110
13. Patel A, Dharmarajan V, Vought VE, Cosgrove MS. On the mechanism of multiple lysine methylation by the human mixed lineage leukemia protein-1 (MLL1) core complex. J Biol Chem. (2009) 284:24242–56. doi: 10.1074/jbc.M109.014498
14. Steward MM, Lee JS, O'Donovan A, Wyatt M, Bernstein BE, Shilatifard A. Molecular regulation of H3K4 trimethylation by ASH2L, a shared subunit of MLL complexes. Nat Struct Mol Biol. (2006) 13:852–4. doi: 10.1038/nsmb1131
15. Blobel GA, Kadauke S, Wang E, Lau AW, Zuber J, Chou MM, et al. A reconfigured pattern of MLL occupancy within mitotic chromatin promotes rapid transcriptional reactivation following mitotic exit. Mol Cell. (2009) 36:970–83. doi: 10.1016/j.molcel.2009.12.001
16. Santos-Rosa H, Schneider R, Bannister AJ, Sherriff J, Bernstein BE, Emre NC, et al. Active genes are tri-methylated at K4 of histone H3. Nature. (2002) 419:407–11. doi: 10.1038/nature01080
17. Chang PY, Hom RA, Musselman CA, Zhu L, Kuo A, Gozani O, et al. Binding of the MLL PHD3 finger to histone H3K4me3 is required for MLL-dependent gene transcription. J Mol Biol. (2010) 400:137–44. doi: 10.1016/j.jmb.2010.05.005
18. Kroon E, Krosl J, Thorsteinsdottir U, Baban S, Buchberg AM, Sauvageau G. Hoxa9 transforms primary bone marrow cells through specific collaboration with Meis1a but not Pbx1b. EMBO J. (1998) 17:3714–25. doi: 10.1093/emboj/17.13.3714
19. Rozovskaia T, Feinstein E, Mor O, Foa R, Blechman J, Nakamura T, et al. Upregulation of Meis1 and HoxA9 in acute lymphocytic leukemias with the t(4: 11) abnormality. Oncogene. (2001) 20:874–8. doi: 10.1038/sj.onc.1204174
20. Zhang Y, Alexander PB, Wang XF. TGF-β Family signaling in the control of cell proliferation and survival. Cold Spring Harb Perspect Biol. (2017) 9:a022145. doi: 10.1101/cshperspect.a022145
21. Blank U, Karlsson S. The role of Smad signaling in hematopoiesis and translational hematology. Leukemia. (2011) 25:1379–88. doi: 10.1038/leu.2011.95
22. Fortunel NO, Hatzfeld A, Hatzfeld JA. Transforming growth factor-beta: pleiotropic role in the regulation of hematopoiesis. Blood. (2000) 96:2022–36. doi: 10.1182/blood.V96.6.2022
23. Soma T, Yu JM, Dunbar CE. Maintenance of murine long-term repopulating stem cells in ex vivo culture is affected by modulation of transforming growth factor-beta but not macrophage inflammatory protein-1 alpha activities. Blood. (1996) 87:4561–7. doi: 10.1182/blood.V87.11.4561.bloodjournal87114561
24. Sitnicka E, Ruscetti FW, Priestley GV, Wolf NS, Bartelmez SH. Transforming growth factor beta 1 directly and reversibly inhibits the initial cell divisions of long-term repopulating hematopoietic stem cells. Blood. (1996) 88:82–8. doi: 10.1182/blood.V88.1.82.82
25. Hatzfeld J, Li ML, Brown EL, Sookdeo H, Levesque JP, O'Toole T, et al. Release of early human hematopoietic progenitors from quiescence by antisense transforming growth factor beta 1 or Rb oligonucleotides. J Exp Med. (1991) 174:925–9. doi: 10.1084/jem.174.4.925
26. Ottmann OG, Pelus LM. Differential proliferative effects of transforming growth factor-beta on human hematopoietic progenitor cells. J Immunol. (1988) 140:2661–5. doi: 10.4049/jimmunol.140.8.2661
27. Sing GK, Keller JR, Ellingsworth LR, Ruscetti FW. Transforming growth factor beta selectively inhibits normal and leukemic human bone marrow cell growth in vitro. Blood. (1988) 72:1504–11. doi: 10.1182/blood.V72.5.1504.1504
28. Keller JR, McNiece IK, Sill KT, Ellingsworth LR, Quesenberry PJ, Sing GK, et al. Transforming growth factor beta directly regulates primitive murine hematopoietic cell proliferation. Blood. (1990) 75:596–602. doi: 10.1182/blood.V75.3.596.596
29. Ewen ME, Sluss HK, Whitehouse LL, Livingston DM. TGF beta inhibition of Cdk4 synthesis is linked to cell cycle arrest. Cell. (1993) 74:1009–20. doi: 10.1016/0092-8674(93)90723-4
30. Geng Y, Weinberg RA. Transforming growth factor beta effects on expression of G1 cyclins and cyclin-dependent protein kinases. Proc Natl Acad Sci USA. (1993) 90:10315–9. doi: 10.1073/pnas.90.21.10315
31. Iavarone A, Massagué J. Repression of the CDK activator Cdc25A and cell-cycle arrest by cytokine TGF-beta in cells lacking the CDK inhibitor p15. Nature. (1997) 387:417–22. doi: 10.1038/387417a0
32. Warner BJ, Blain SW, Seoane J, Massagué J. Myc downregulation by transforming growth factor beta required for activation of the p15(Ink4b) G(1) arrest pathway. Mol Cell Biol. (1999) 19:5913–22. doi: 10.1128/MCB.19.9.5913
33. Hannon GJ, Beach D. p15INK4B is a potential effector of TGF-beta-induced cell cycle arrest. Nature. (1994) 371:257–61. doi: 10.1038/371257a0
34. Seoane J, Pouponnot C, Staller P, Schader M, Eilers M, Massagué J. TGFbeta influences Myc, Miz-1 and Smad to control the CDK inhibitor p15INK4b. Nat Cell Biol. (2001) 3:400–8. doi: 10.1038/35070086
35. Sandhu C, Garbe J, Bhattacharya N, Daksis J, Pan CH, Yaswen P, et al. Transforming growth factor beta stabilizes p15INK4B protein, increases p15INK4B-cdk4 complexes, and inhibits cyclin D1-cdk4 association in human mammary epithelial cells. Mol Cell Biol. (1997) 17:2458–67. doi: 10.1128/MCB.17.5.2458
36. Dao MA, Hwa J, Nolta JA. Molecular mechanism of transforming growth factor beta-mediated cell-cycle modulation in primary human CD34(+) progenitors. Blood. (2002) 99:499–506. doi: 10.1182/blood.V99.2.499
37. Datto MB, Li Y, Panus JF, Howe DJ, Xiong Y, Wang XF, et al. Transforming growth factor beta induces the cyclin-dependent kinase inhibitor p21 through a p53-independent mechanism. Proc Natl Acad Sci U.S.A. (1995) 92:5545–9. doi: 10.1073/pnas.92.12.5545
38. Scandura JM, Boccuni P, Massagué J, Nimer SD. Transforming growth factor beta-induced cell cycle arrest of human hematopoietic cells requires p57KIP2 up-regulation. Proc Natl Acad Sci U.S.A. (2004) 101:15231–6. doi: 10.1073/pnas.0406771101
39. Ducos K, Panterne B, Fortunel N, Hatzfeld A, Monier MN, Hatzfeld J. p21(cip1) mRNA is controlled by endogenous transforming growth factor-beta1 in quiescent human hematopoietic stem/progenitor cells. J Cell Physiol. (2000) 184:80–5. doi: 10.1002/(SICI)1097-4652(200007)184:1<80::AID-JCP8>3.0.CO;2-Q
40. Rapin N, Bagger FO, Jendholm J, Mora-Jensen H, Krogh A, Kohlmann A, et al. Comparing cancer vs normal gene expression profiles identifies new disease entities and common transcriptional programs in AML patients. Blood. (2014) 123:894–904. doi: 10.1182/blood-2013-02-485771
41. Gíslason MH, Demircan GS, Prachar M, Furtwängler B, Schwaller J, Schoof EM, et al. BloodSpot 3.0: a database of gene and protein expression data in normal and malignant haematopoiesis. Nucleic Acids Res. 52(D1):D1138–42. doi: 10.1093/nar/gkad
42. Gerlach MM, Stelling-Germani A, Wu Ting C, Newrzela S, Döring C, Vela V, et al. SMAD1 promoter hypermethylation and lack of SMAD1 expression in Hodgkin lymphoma: a potential target for hypomethylating drug therapy. Haematologica. (2021) 106:619–21. doi: 10.3324/haematol.2020.249276
43. Stelling A, Hashwah H, Bertram K, Manz MG, Tzankov A, Müller A. The tumor suppressive TGF-β/SMAD1/S1PR2 signaling axis is recurrently inactivated in diffuse large B-cell lymphoma. Blood. (2018) 131:2235–46. doi: 10.1182/blood-2017-10-810630
44. Wang P, Lin C, Smith ER, Guo H, Sanderson BW, Wu M, et al. Global analysis of H3K4 methylation defines MLL family member targets and points to a role for MLL1-mediated H3K4 methylation in the regulation of transcriptional initiation by RNA polymerase II. Mol Cell Biol. (2009) 29:6074–85. doi: 10.1128/MCB.00924-09
45. Rangwala SH, Kuznetsov A, Ananiev V, Asztalos A, Borodin E, Evgeniev V, et al. Accessing NCBI data using the NCBI Sequence Viewer and Genome Data Viewer (GDV). Genome Res. (2021) 31:159–69. doi: 10.1101/gr.266932.120
46. Barabé F, Kennedy JA, Hope KJ, Dick JE. Modeling the initiation and progression of human acute leukemia in mice. Science. (2007) 316:600–4. doi: 10.1126/science.1139851
47. Ferrando AA, Armstrong SA, Neuberg DS, Sallan SE, Silverman LB, Korsmeyer SJ, et al. Gene expression signatures in MLL-rearranged T-lineage and B-precursor acute leukemias: dominance of HOX dysregulation. Blood. (2003) 102:262–8. doi: 10.1182/blood-2002-10-3221
48. Secker KA, Keppeler H, Duerr-Stoerzer S, Schmid H, Schneidawind D, Hentrich T, et al. Inhibition of DOT1L and PRMT5 promote synergistic anti-tumor activity in a human MLL leukemia model induced by CRISPR/Cas9. Oncogene. (2019) 38:7181–95. doi: 10.1038/s41388-019-0937-9
49. Stelling A, Wu CT, Bertram K, Hashwah H, Theocharides APA, Manz MG, et al. Pharmacological DNA demethylation restores SMAD1 expression and tumor suppressive signaling in diffuse large B-cell lymphoma. Blood Adv. (2019) 3:3020–32. doi: 10.1182/bloodadvances.2019000210
50. Ghandi M, Huang FW, Jané-Valbuena J, Kryukov GV, Lo CC, McDonald ER 3rd, et al. Next-generation characterization of the cancer cell line encyclopedia. Nature. (2019) 569:503–8. doi: 10.1038/s41586-019-1186-3
51. Keller JR, Mantel C, Sing GK, Ellingsworth LR, Ruscetti SK, Ruscetti FW. Transforming growth factor beta 1 selectively regulates early murine hematopoietic progenitors and inhibits the growth of IL-3-dependent myeloid leukemia cell lines. J Exp Med. (1988) 168:737–50. doi: 10.1084/jem.168.2.737
52. Ohta M, Greenberger JS, Anklesaria P, Bassols A, Massagué J. Two forms of transforming growth factor-beta distinguished by multipotential haematopoietic progenitor cells. Nature. (1987) 329:539–41. doi: 10.1038/329539a0
53. Notta F, Doulatov S, Dick JE. Engraftment of human hematopoietic stem cells is more efficient in female NOD/SCID/IL-2Rgc-null recipients. Blood. (2010) 115:3704–7. doi: 10.1182/blood-2009-10-249326
54. Massagué J, Sheppard D. TGF-β signaling in health and disease. Cell. (2023) 186:4007–37. doi: 10.1016/j.cell.2023.07.036
55. Wolfraim LA, Fernandez TM, Mamura M, Fuller WL, Kumar R, Cole DE, et al. Loss of Smad3 in acute T-cell lymphoblastic leukemia. N Engl J Med. (2004) 351:552–9. doi: 10.1056/NEJMoa031197
56. Kim SJ, Letterio J. Transforming growth factor-β signaling in normal and Malignant hematopoiesis. Leukemia. (2003) 17:1731–7. doi: 10.1038/sj.leu.2403069
57. Scott S, Kimura T, Ichinohasama R, Bergen S, Magliocco A, Reimer C, et al. Microsatellite mutations of transforming growth factor-beta receptor type II and caspase-5 occur in human precursor T-cell lymphoblastic lymphomas/leukemias in vivo but are not associated with hMSH2 or hMLH1 promoter methylation. Leuk Res. (2003) 27:23–34. doi: 10.1016/S0145-2126(02)00078-4
58. Quéré R, Karlsson G, Hertwig F, Rissler M, Lindqvist B, Fioretos T, et al. Smad4 binds Hoxa9 in the cytoplasm and protects primitive hematopoietic cells against nuclear activation by Hoxa9 and leukemia transformation. Blood. (2011) 117:5918–30. doi: 10.1182/blood-2010-08-301879
59. Imai Y, Kurokawa M, Izutsu K, Hangaishi A, Maki K, Ogawa S, et al. Mutations of the Smad4 gene in acute myelogeneous leukemia and their functional implications in leukemogenesis. Oncogene. (2001) 20:88–96. doi: 10.1038/sj.onc.1204057
60. Guenther MG, Jenner RG, Chevalier B, Nakamura T, Croce CM, Canaani E, et al. Global and Hox-specific roles for the MLL1 methyltransferase. Proc Natl Acad Sci U.S.A. (2005) 102:8603–8. doi: 10.1073/pnas.0503072102
61. Wang Z, Song J, Milne TA, Wang GG, Li H, Allis CD, et al. Pro isomerization in MLL1 PHD3-bromo cassette connects H3K4me readout to CyP33 and HDAC-mediated repression. Cell. (2010) 141:1183–94. doi: 10.1016/j.cell.2010.05.016
62. Wang H, Fan Z, Shliaha PV, Miele M, Hendrickson RC, Jiang X, et al. H3K4me3 regulates RNA polymerase II promoter-proximal pause-release. Nature. (2023) 615:339–48. doi: 10.1038/s41586-023-05780-8
63. Mishra BP, Zaffuto KM, Artinger EL, Org T, Mikkola HK, Cheng C, et al. The histone methyltransferase activity of MLL1 is dispensable for hematopoiesis and leukemogenesis. Cell Rep. (2014) 7:1239–47. doi: 10.1016/j.celrep.2014.04.015
64. Chen Y, Anastassiadis K, Kranz A, Stewart AF, Arndt K, Waskow C, et al. MLL2, not MLL1, plays a major role in sustaining MLL-rearranged acute myeloid leukemia. Cancer Cell. (2017) 31:755–770.e6. doi: 10.1016/j.ccell.2017.05.002
65. Rongvaux A, Takizawa H, Strowig T, Willinger T, Eynon EE, Flavell RA, et al. Human hemato-lymphoid system mice: current use and future potential for medicine. Annu Rev Immunol. (2013) 31:635–74. doi: 10.1146/annurev-immunol-032712-095921
66. Javier J, Hinge A, Bartram J, Xu J, Filippi MD. Transforming growth factor-β signaling modifies the hematopoietic acute inflammatory response to drive bone marrow failure. Haematologica. (2022) 107:1323–34. doi: 10.3324/haematol.2020.273292
67. Wang N, Kim HG, Cotta CV, Wan M, Tang Y, Klug CA, et al. TGFbeta/BMP inhibits the bone marrow transformation capability of Hoxa9 by repressing its DNA-binding ability. EMBO J. (2006) 25:1469–80. doi: 10.1038/sj.emboj.7601037
68. Baba AB, Rah B, Bhat GR, Mushtaq I, Parveen S, Hassan R, et al. Transforming growth factor-beta (TGF-β) signaling in cancer-A betrayal within. Front Pharmacol. (2022) 13:791272. doi: 10.3389/fphar.2022.791272
69. Chen B, Mu C, Zhang Z, He X, Liu X. The love-hate relationship between TGF-β Signaling and the immune system during development and tumorigenesis. Front Immunol. (2022) 13:891268. doi: 10.3389/fimmu.2022.891268
70. Kretzschmar M, Doody J, Massagué J. Opposing BMP and EGF signalling pathways converge on the TGF-beta family mediator Smad1. Nature. (1997) 389:618–22. doi: 10.1038/39348
71. Fuentealba LC, Eivers E, Ikeda A, Hurtado C, Kuroda H, Pera EM, et al. Integrating patterning signals: Wnt/GSK3 regulates the duration of the BMP/Smad1 signal. Cell. (2007) 131:980–93. doi: 10.1016/j.cell.2007.09.027
Keywords: KMT2A rearrangement, acute myeloid leukaemia, SMAD1, TGF-beta signaling, KMT2A::AFF1, KMT2A::MLLT3
Citation: Dietsche L, Stirm K, Lysenko V, Schneidawind C, Tzankov A, Müller A and Theocharides APA (2025) Loss of SMAD1 in acute myeloid leukemia with KMT2A::AFF1 and KMT2A::MLLT3 fusion genes. Front. Oncol. 14:1481713. doi: 10.3389/fonc.2024.1481713
Received: 16 August 2024; Accepted: 10 December 2024;
Published: 06 January 2025.
Edited by:
Michele Redell, Baylor College of Medicine, United StatesReviewed by:
Jessica Heath, University of Vermont Children’s Hospital, United StatesPaulina Gil-Kulik, Medical University of Lublin, Poland
Copyright © 2025 Dietsche, Stirm, Lysenko, Schneidawind, Tzankov, Müller and Theocharides. This is an open-access article distributed under the terms of the Creative Commons Attribution License (CC BY). The use, distribution or reproduction in other forums is permitted, provided the original author(s) and the copyright owner(s) are credited and that the original publication in this journal is cited, in accordance with accepted academic practice. No use, distribution or reproduction is permitted which does not comply with these terms.
*Correspondence: Alexandre P. A. Theocharides, YWxleGFuZHJlLnRoZW9jaGFyaWRlc0B1c3ouY2g=
†These authors share first authorship
‡These authors share last authorship