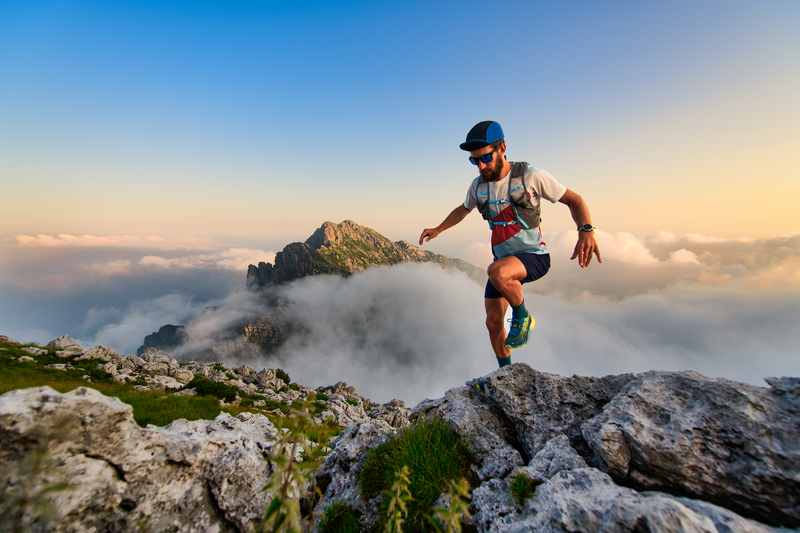
94% of researchers rate our articles as excellent or good
Learn more about the work of our research integrity team to safeguard the quality of each article we publish.
Find out more
REVIEW article
Front. Oncol. , 05 April 2024
Sec. Radiation Oncology
Volume 14 - 2024 | https://doi.org/10.3389/fonc.2024.1372780
Radiotherapy stands as a cornerstone in the treatment of numerous malignant tumors, including non-small cell lung cancer. However, the critical challenge of amplifying the tumoricidal effectiveness of radiotherapy while minimizing collateral damage to healthy tissues remains an area of significant research interest. Radiosensitizers, by methods such as amplifying DNA damage and fostering the creation of free radicals, play a pivotal role in enhancing the destructive impact of radiotherapy on tumors. Over recent decades, nano-dimensional radiosensitizers have emerged as a notable advancement. Their mechanisms include cell cycle arrest in the G2/M phase, combating tumor hypoxia, and others, thereby enhancing the efficacy of radiotherapy. This review delves into the evolving landscape of nanomaterials used for radiosensitization in non-small cell lung cancer. It provides insights into the current research progress and critically examines the challenges and future prospects within this burgeoning field.
Radiotherapy, a prevalent treatment for non-small cell lung cancer (NSCLC) and various other malignant tumors, is often used in conjunction with chemotherapy and other treatments (1–3). Its fundamental principle involves the interaction of ionizing radiation with tumor cell components, either directly or indirectly. Direct interaction leads to the damage of critical biological molecules like DNA and proteins, hindering cell division and proliferation, ultimately causing cell death. Indirectly, radiation induces the production of reactive oxygen species (ROS) and free radicals, disrupting these biological molecules (4).
However, the use of radiotherapy encounters many challenges. Factors such as tumor stem cells, tumor heterogeneity, and angiogenesis can limit its effectiveness. Moreover, complications may arise, making it difficult for patients to tolerate prolonged radiotherapy (5). A strategic approach to surmount these challenges involves the use of radiosensitizers, which are designed to enhance radiotherapy’s efficacy while mitigating side effects on normal tissue (6).
In recent years, nanoparticles, known for their excellent biocompatibility, high drug loading capacity, and robust tumor permeability and retention (7, 8), have become a focal point in the realm of tumor radiosensitization. When delivered to tumors, these nanoparticles not only exert their therapeutic effects but also sensitize tumor cells to radiotherapy through various mechanisms. This review delves into the advancements in nano-radiosensitizers, particularly focusing on their underlying mechanisms and contributions to enhancing radiotherapy outcomes.
Metal materials have been used in radiotherapy research for decades. In a pioneering study by Regulla in 1998, it was observed that mouse embryonic fibroblasts irradiated with X-rays on a gold surface exhibited increased biological effects compared to those in a tissue-like environment (9). Further research by Herold et al. revealed enhanced radiation effects in cancer cells with gold particles (10). By 2010, T. Marques et al. demonstrated that gold nanoparticles (GNPs) in tissues could selectively increase radiation doses to target areas, illustrating the potential of metals in refining radiotherapy (11).
Cuihong Wang et al. were the first to use thio-glucose-bound gold nanoparticles (Glu-GNPs) on NSCLC cells. They found that glucose enhances the uptake of Glu-GNPs by A549 cells, leading to their accumulation in vesicular endosomes or lysosomes. Upon X-ray exposure, Glu-GNPs triggered cell apoptosis through the modulation of Bcl-2 family proteins and activation of the mitochondrial apoptotic pathway (12). Tao Li’s team also worked with Glu-GNPs in A549 cells, achieving sensitization enhancement ratios (SER) of 1.41 and 1.15 for 160 kV and 6 MV X-rays, respectively (13). Shokouhozaman’s research indicated increased inhibitory effects of Glu-GNPs on QU-DB lung cancer cells by 64.4% and 32.4% under 100 kV and 6 MV X-rays (14). These findings proposed that Glu-GNPs may possess superior efficacy in combating NSCLC cells under conditions of low-energy radiation. However, the substantiation of these claims is somewhat feeble, primarily due to the omission of in vivo experiments by the researchers.
Additionally, GNPs have demonstrated potential in impeding the migratory capabilities of A549 cells post-radiation therapy, perhaps as a result of alterations in the cytoskeleton affecting overall cellular adhesion (15). Nevertheless, it would behoove the research to consider testing a broader range of dosages, extending beyond the used levels of 2Gy and 5Gy. Moreover, albumin-bound GNPs, known for their favorable biosafety profile, exhibited radiosensitization and anti-tumor activity both in vivo and in vitro experiments, boasting a SER of 1.432 (16). The study could be strengthened by incorporating more tumor models beyond A549 for validation. Zhongli Cai’s team found that the dose enhancement ratio of GNPs was lower in 3D culture models compared to single-monolayer (1.3 vs 1.6) (17). Sherif et al. argued that the common algorithm for calculating the dose enhancement ratio is overly simplistic and fails to consider specific profiles, leading to a decrease in the dose enhancement ratio due to potential rupture and detachment of GNPs’ surface coating (18). Therefore, optimizing the surface coating of GNPs was crucial, although it would certainly be better if the models were more closely aligned with the actual lung environment.
Fatma et al. modified GNPs with Schiff bases derived from galactose, resulting in larger particles that showed greater radiosensitization in A549 cells compared to unmodified GNPs (19). Arvind’s study compared 3.9 and 37.4 nm GNPs in Lewis lung cancer cells, finding significant radiosensitizing effects post-X-ray irradiation with both sizes, but no significant difference between them (20). Both studies might benefit from the inclusion of animal experiments and a broader spectrum of radiotherapy dose configurations.
Table 1 summarizes researches on GNP combined with radiotherapy in NSCLC. These researches are extensive, indicating that particle size, coating, and surface modifiers significantly influence their radiosensitizing effect. Future studies are needed to optimize these factors for better clinical application.
In the realm of NSCLC radiosensitization, silver nanoparticles (AgNPs) and gadolinium nanoparticles (AGuIX) have also shown promise. Gowda et al. discovered that AgNPs, when modified with gallic acid, effectively inhibited the expression of epithelial-mesenchymal transition markers induced by X-ray in A549 cells like Vimentin and N-cadherin, simultaneously promoting E-cadherin upregulation. This modification thereby reduced tumor cell radioresistance (21). Reetta’s team, in their study of AgNPs across multiple NSCLC cell lines, observed that these nanoparticles led to cell cycle arrest in different phases (A549 and Calu-1 cells in G2 phase; BEAS-2B cells in S phase) and increased ROS production and protein oxidation in cell mitochondria, thus elevating the cells’ sensitivity to radiotherapy. It’s noteworthy, however, that these nanoparticles did not modify the mitochondrial redox profiles altered by radiation therapy (22). Adding GNPs as a horizontal comparison would be more meaningful when studying AgNPs, as gold and silver are both precious metals.
AGuIX, a polysiloxane nanoparticle containing gadolinium ions (Gd3+), was first applied in H1299 and A549 cell. Applied initially to H1299 and A549 cells, Upon X-ray exposure, AGuIX produced photoelectrons, reactive oxygen species, and free radicals, leading to G2/M phase arrest and enhancing both radiosensitization and apoptosis in NSCLC cells (23). Wu Liu’s team innovated further by attaching gadolinium nanoparticles to a pH-low insertion peptide, boosting cellular Gd uptake dramatically and prolonging its tumor residence, significantly improving radiosensitivity in A549 cells (24). The design and validation of these two studies are fairly comprehensive, and we anticipate more profound research in the future.
Chaebin et al. developed gadolinium-embedded carbon dots (Gd@C-dots) via hydrothermal reaction, which compared to AGuIX, showed reduced toxicity due to lower Gd leakage in vivo and enhanced radiosensitivity in H1299 cells due to the catalytic properties of carbon (25). Another research compared CA or amino (pPD)-modified Gd@C-dots, with the pPD-modified Gd@C-dots demonstrating better uptake and retention in H1299 cells, indicating a higher potential for clinical application (26). Both studies are considerably thorough, however, there is room for further refinement particularly in the realms of in-situ tumor models or dose escalation studies.
CuPRiX, created by partially dehydrogenating Gd from DOTAGA(Gd) within AGuIX in an acidic environment, resulted in the unchelated Gd chelating free Cu in A549 cells. This led to the inhibition of the copper oxide-dependent enzyme (LOX) and reduced cell migration, thereby improving tumor radiosensitization over AGuIX (27). Research on copper nanoparticles is sparse, marking a novel aspect of this study. However, it is apparent that this research could benefit from a deeper exploration of the underlying mechanisms. Currently, AGuIX is undergoing phase I clinical trials for brain metastases and gliomas (28, 29), with research on its application in NSCLC still in the early stages. Below is Table 2 concluding metal nanoparticles above.
In the field of NSCLC radiosensitization, semiconductor nanoparticles have gained prominence. TiO2 nanoparticles, when excited by high Cerenkov radiation from X-rays, form electron-hole pairs that trigger the production of ROS, leading to DNA damage. Utilizing this principle, Zi Ouyang’s team designed TiO2 nanoparticles that significantly heightened the radiosensitivity of A549 cells (30). However, the lack of in vivo experiments was a shortcoming of this study.
Similarly, semiconductor zinc oxide (ZnO) exhibits comparable properties. Masoumeh wt al. developed ZnO nanoparticles, doping them with gadolinium to create Gd-ZnO-NPs. At concentrations of 10 and 20 μg/mL in SKLC-6 cells, these nanoparticles showed SER of 1.47 and 1.61, respectively, demonstrating a concentration-dependent increase in radiosensitivity. Flow cytometry analysis revealed that Gd-ZnO-NPs elevated apoptosis in NSCLC cells and caused more cells to arrest in G1 phase. Combined with X-rays, these nanoparticles downregulated the mRNA levels of DNA damage repair genes such as XRCC2 and XRCC4, hindering DNA repair and leading to increased cell death (31). Additionally, Gd-ZnO NP enhanced the contrast of cancer cell CT and MR images, further increasing its potential for clinical translation.
Jingfang Xiao et al. experimented with bismuth selenide nanoparticles (Bi2Se3s), combining them with adipose-derived mesenchymal stromal cells (adipose-derived MSCs) to create adipose-derived MSCs/Bi2Se3s. These nanoparticles were found to be more effectively enriched in lung tumors in tumor-bearing mice compared to bare Bi2Se3s, thereby amplifying the radiosensitivity of A549 cells and extending the survival time of mice (32). This study used an in-situ tumor model, with rigorous design and thorough verification, making it persuasive. Table 3 describes semiconductor nanoparticles in the previous section.
Beyond metal and semiconductor nano-radiosensitizers, alloys, oxides, and various other nano-radiosensitizing agents have been explored. Yingming Sun et al. enhanced the solubility and stability of platinum-iron alloy nanoparticles by integrating cysteine to form FePt-Cys NPs. These nanoparticles, when combined with radiotherapy in A549 and H1975 cells, notably reduced VEGF and MMP2 expression, potentially contributing to their radiosensitizing effects (33). Similarly, Shijing Ma’s team utilized FePt NPs anchored on graphene oxide, successfully inhibiting the proliferation of A549, H460, and H1975 cells. This approach prompted autophagy, escalated ROS generation, and consequently increased NSCLC cell radiosensitivity (34). Both researches were well-conducted, but the former lacked a systemic toxicity examination while the latter lacked a dose escalation study.
MnO2, a widely used oxidant, demonstrated the capability to diminish reduced glutathione levels in both PC9 and TKI-resistant PC9GR cells. This activity ameliorated the tumor hypoxic environment and augmented radiotherapy effectiveness (35). The absence of animal experimentation was a flaw in it. In another study, MnO2 NPs, in combination with radiotherapy, enhanced ROS synthesis and activated the cGAS/STING pathway in A549 and H520 cells, triggering anti-tumor immune responses in mice (36). Feifei Li’s team synthesized gadolinium oxide nanoparticles that, post X-ray irradiation, spurred hydroxyl radical and ROS production in various cell lines, fostering cellular oxidative stress, autophagy, and enhancing radiotherapy efficacy (37). These two studies have explored the fields of anti-tumor immunity and autophagy respectively, which standed out as their highlights. Yingbo Li et al. developed pH-sensitive superparamagnetic iron oxide nanoclusters, which disintegrated in the tumor’s acidic milieu and, under X-ray exposure, intensified ROS production, lipid peroxidation, DNA damage, apoptosis, and iron death response, thereby improving H460 cell radiosensitivity (38). This research was also very solid, and it would be better if the long-term toxicity of the drug could be detected.
Selenium nanoparticles (SeNPs), a well-known inorganic nanomaterial, effectively augmented caspase-3 expression in A549 cells when used with radiotherapy, initiating apoptotic pathways leading to cell death (39, 40). Jingxia Tian and his colleagues discovered that SeNPs, in synergy with radiotherapy, significantly inhibited proliferation-associated proteins (CCND1, c-Myc) and invasion-related proteins (MMP2, MMP9) in A549 and H23 cells. This synergy also promoted apoptosis-related proteins, thereby curbing NSCLC cell migration and invasion and inducing apoptosis (41). Shiqing Nie’s team evaluated the effects of various selenium compounds in SPC-A1 cells, concluding that selenadiazole SeD exhibited the most pronounced radiosensitizing impact in vitro (42). All of these studies have investigated the mechanisms involved, but they lacked in vivo experiments that could be improved.
Thangirala et al. synthesized nano-diaminotetraacetic acid from tetraiodothyroacetic acid, a ligand of thyroid integrin αvβ3. Applied to a thymus-less H1299 xenograft tumor model in mice, this compound, upon external irradiation, achieved more significant tumor regression than radiotherapy alone (43). Min Hua Chen’s team designed hafnium-doped hydroxyapatite (Hf: HAp) nanoparticles that, in conjunction with radiotherapy, led to a substantial ROS accumulation in A549 cells, enhancing cellular damage (44). Matthias and colleagues developed lutetium phosphate nanoparticles doped with praseodymium cations, which emitted photons upon X-ray irradiation, causing DNA damage, cell cycle blockage, and thus amplifying radiosensitization in hypoxic A549 cells (45). Thao et al.’s design of lutetium phosphate nanoparticles doped with praseodymium and neodymium cations showed similar outcomes (46). The first two studies were relatively comprehensive, and further exploration in situ tumor irradiation and dose escalation was the direction for follow-up expansion. The last two studies attempted to simulate the in vivo tumor environment in vitro. However, if in vivo modeling could be conducted, it would be more convincing.
Table 4 is a summary of the nanoparticles mentioned above.
Chemotherapy remains a cornerstone in the treatment of NSCLC and various other cancers. Agents such as platinum, paclitaxel, and pemetrexed have shown to augment radiotherapy’s effectiveness through diverse mechanisms. For instance, cisplatin disrupts the ATM pathway crucial for repairing cellular damage caused by irradiation (47). Paclitaxel (PTX) orchestrates NSCLC cells to pause at the radiosensitive G2/M phase (48), while pemetrexed impedes nucleotide precursor synthesis, impacting DNA repair (49). Nanotechnology’s advent has pioneered the encapsulation and delivery of these radiosensitizing chemotherapeutics directly into lung tumors, forging a novel and synergistic approach in chemotherapy-radiotherapy treatments.
PTX, known for its poor water solubility, is traditionally dissolved in polyoxyethylated castor oil, a substance linked to allergic reactions and neurotoxicity (50). In 2006, T Negishi and team leveraged NK105, a micellar nanoparticle formulation of PTX, in mice inoculated with Lewis cells. Administering this formulation followed by X-ray irradiation led to enhanced efficacy compared to conventional PTX, notably inducing a higher rate of tumor cell arrest in the G2/M phase (51). The experimental group setup in this study was quite reasonable. However, in the absence of in-vitro experiments to determine the IC50 of NK105, directly using a dose of 45 mg kg−1 in vivo might not be quite suitable. Genexol-PM, another micellar formulation of paclitaxel free from polyoxyethylated castor oil, mirrored NK105’s antitumor effects and radiosensitization properties (52). The study would be improved by incorporating a group that was solely treated with the drugs. Exploring further, Wheemoon et al. developed LOXab NPs by fusing LOX antibodies with PTX, yielding a highly targeted approach against A549 cells, resulting in increased apoptosis and radiosensitization (53). Despite the unirradiated tumor sites not presenting a remarkable abscopal effect, the study which utilized a model of tumors implanted on both sides of mice was well-designed. Additionally, the FDA has approved an albumin-bound form of paclitaxel for NSCLC’s frontline treatment, which will be described in detail in later sections.
In the realm of platinum-based treatments, platinum nanoparticles, particularly those of cisplatin-NPs and carboplatin-NPs, are garnering significant attention. Y Hao and his colleagues observed that cisplatin-NPs and carboplatin-NPs, when administered via inhalation, concentrate more effectively in lung tumors, enhancing radiotherapy’s synergistic effects while minimizing normal tissue toxicity (54). Utilizing liposomes, nanoscale drug carriers, coupled with EGFR antibodies and cisplatin, showed promising results in targeting mouse A549 xenograft tumors and boosting radiosensitivity (55). Joseph et al. crafted cisplatin precursor nanoparticles through alkali-catalyzed sol-gel polymerization and modified them with polyethylene glycol (PEG) to evade mononuclear phagocytic system uptake, resulting in superior performance in vivo and in vitro (56). Maofan Zhang et al. synthesized PEG-PLGA NPs encapsulating etoposide and cisplatin, achieving significant SERs (1.6 and 1.65) in 344SQ and H460 cells without added toxicity (57). Ling-Yu Chen and his team developed albumin-based cisplatin-gold nanoparticles (Au-cisplatin NPs), demonstrating remarkable superiority in tumor control and anti-tumor immunity when combined with radiotherapy (58). The aforementioned five studies each possessed their unique attributes, including features such as inhalation drug delivery and liposome encapsulation. Apart from the first study which did not undertake a safety evaluation, the remaining investigations enhanced efficacy without amplifying toxicity, representing particular value in terms of clinical application utility.
Gemcitabine, a first-line therapy for advanced NSCLC, saw innovation through Ji Liu et al.’s work, who attached RGDc peptides to lipid GNPs loaded with gemcitabine. Activated by near-infrared light, this combination hindered tumor growth and bolstered radiosensitivity by facilitating ROS production in NSCLC cells (59). For advanced NSCLC, doxorubicin (DOX) serves as a second-line treatment. Jing Wang et al. prepared epidermal growth factor-modified adriamycin nanoparticles (EGF@DOX-NPs), targeting cells overexpressing EGFR, significantly heightening A549 cells’ radiosensitivity both in vitro and in vivo (60). Recognizing the high expression of Glucose-regulated protein 78 on NSCLC surfaces, Abhay et al. employed Glucose-regulated protein 78 targeting peptides with DOX liposomes, enhancing drug delivery efficiency and markedly improving radiotherapy efficacy in both A549 and H460 cells (61). These three studies also boasted robust designs. Their future exploration lies in the field of in-situ tumor studies.
There is Table 5 summarizing nano-radiosensitizers loaded with FDA approved chemotherapy drugs in NSCLC.
Table 5 Types of nano-radiosensitizers loading FDA approved NSCLC chemotherapy drugs combined with IR in NSCLC.
Olaparib, an FDA-approved poly (ADP-ribose) polymerase inhibitor, plays a pivotal role in inhibiting poly (ADP-ribose) polymerase, essential for repairing radiation-induced DNA damage. It’s widely recognized for its efficacy in treating ovarian, breast, pancreatic cancer and various other cancers (62–64). Min Wu and colleagues innovatively synthesized Olaparib-NPs, which demonstrated a significantly higher SER of 3.81 in A549 cells compared to free Ola’s 1.66, without introducing additional toxicity (65). Similarily, if in-situ tumor research was conducted, it could make their studies more intriguing.
Curcumin (Cum), known for its antitumor properties in lung cancer, faces challenges in clinical use due to low solubility and bioavailability. Overcoming this, Cum-NPs, made by encapsulating Cum with polyvinylpyrrolidone-polycaprolactone, significantly enhanced apoptosis in A549 cells compared to free Cum, thereby enhancing the efficacy of radiotherapy (with a SER at 10% cell survival of 1.55 versus 1.13) (66).
Cannabinoids, active compounds in cannabis, are able to inhibit tumor growth. Wilfred and his team attached cannabinoids to ‘nanoparticle drones’ using gold nanoparticles, targeting lung tumors in a transgenic mouse NSCLC model. Administered by inhalation, these drones improved radiosensitivity while minimizing side effects (67).
Maytansinoid DM1, an alkaloid with cancer-fighting properties linked to maytansine, has been further optimized by Shi Gao’s group. They nitrosylated DM1 to produce DM1-NO and then loaded it onto PLGA-βPEG nanoparticles, creating DM1-NO PLGA-NPs that targeted NSCLC effectively. X-ray irradiation breaks the drug’s S-N bond, releasing DM1 and nitric oxide (NO), which interacts with ROS to form free radicals and block cells in the G2/M phase, enhancing radiosensitizing effect on H1299 cells (68).
Baicalein, an active anticancer agent derived from Scutellaria baicalensis, suffers from low bioavailability. This challenge was addressed by formulating it into solid lipid NPs. Applied to A549 cells, solid lipid NPs increased ROS and apoptosis, sensitizing them to radiotherapy, while also providing radioprotection in normal cells (69).
Absolutely, natural anti-tumor substances extracted from plants in nature have advantages such as being inexpensive and readily available. Figuring out how to better deliver them to tumors, enhance anti-tumor effects, and reduce toxic side effects presents a significant area of research. The studies above have provided good examples.
Presented below is Table 6, summarizing nano-radiosensitizers that encapsulate FDA-approved chemotherapy drugs for various tumor treatments and natural anti-tumor compounds.
Table 6 Types of nano-radiosensitizers loading FDA approved chemotherapy drugs for other tumors or natural anti-tumor compounds in combination with IR in NSCLC.
Jinghui Zhang’s team discovered that H1299 cells, which survived repeated X-ray irradiation, exhibit high expression of ALDH1 and CD133 proteins. Notably, in the ALDH1+ CD133+ NSCLC cell subset, miR-21 and miR-95 levels were significantly elevated compared to the ALDH1- CD133- group. Addressing this, the researchers used calcium carbonate nanoparticles to deliver anti-miR21 and anti-miR95 to NSCLC cells. This approach markedly inhibited tumor growth and enhanced radiosensitivity in H1299 cells, potentially by upregulating PTEN, SNX1, and SGPP1, while concurrently suppressing the PI3K-Akt pathway (70).
The overexpression of the MUC1-C subunit, commonly found in NSCLC tumors, led Alexandre et al. to develop MUC1-C antibody-conjugated Gd-based nanoparticles. These nanoparticles achieved a SER of 1.86 in H460 cells and showed prolonged retention in tumor models, boosting the effectiveness of fractionated radiotherapy (71).
Specificity protein 1 (SP1), often overexpressed in NSCLC, was targeted by GNPs-siSP1, comprising siSP1 and gold nanoparticles. GNPs-siSP1, easily internalized by A549 cells, reduced SP1 expression, upregulated granzyme B, and arrested cells in the G2/M phase, thereby enhancing radiosensitivity with SERs of 2.09 and 2.13 at 10 nM and 20 nM concentrations, respectively (72).
KRAS is a member of the human Ras gene family, with KRAS mutations present in 20% -25% of NSCLCs. Linlin Yang et al. engineered EGFRapt-3WJ-siKRASG12C nanoparticles targeting KRAS mutations, effectively reducing KRASG12C expression in H2122, H2030, and H1299 cells. This innovation inhibited the downstream MAPK pathway and amplified the tumor-suppressive impact of radiotherapy (73).
HPNAS-4 has been recognized as a pro-apoptotic gene. When plasmids carrying the HPNAS-4 gene were delivered to NSCLC cells using liposomes, there was a notable overexpression of the hPNAS-4 protein. This led to increased apoptosis in A549 and Lewis cells, significantly enhancing the efficacy of radiotherapy (74).
In another study, Chang’s team developed plasmids that combined radiation-responsive Egr1 promoters with hypoxia-responsive enhancers. These plasmids, when introduced into A549 cells via liposomes, triggered the overexpression of the pro-apoptotic protein Smac. This intervention promoted apoptosis and caused G2/M phase arrest in the cells, ultimately improving the radiosensitivity of A549 cells under hypoxic conditions (75).
Nowadays, people can initially select the research targets by screening the genes that are differentially expressed in normal tissues and tumor tissues from the public database. How to further screen the preliminary data to pinpoint the specific molecules and design rigorous experiments around them is a topic worthy of deep investigation.
Table 7 presents an overview of nano-radiosensitizers encapsulating drugs specifically aimed at targeting highly expressed biomarkers or genes in NSCLC.
Table 7 Types of nano-radiosensitizers loading drugs targeting high expression biomarkers or genes in NSCLC in combination with IR.
The exceptional targeting and loading capacity of nanoparticles allow for the efficient delivery of increased drug quantities to tumors. Jyothi et al. developed multifunctional dual drug loaded nanoparticles, encapsulating the DNA-PK inhibitor NU7441 and gemcitabine in superparamagnetic iron oxide nanoparticles, and augmented them with folate for targeting folate receptors, which are overexpressed in various cancers, including NSCLC. These folate-coupled MDNPs demonstrated prolonged retention in NSCLC compared to their uncoupled counterparts. Upon reaching tumors, multifunctional dual drug loaded nanoparticles underwent vesicle-mediated endocytosis, releasing their contents in the acidic environment of endosomes or lysosomes, thereby exerting cytotoxic effects and enhancing the radiosensitivity of A549 and H460 cells (76). Roshni and his colleagues co-loaded NU7441 and cisplatin into nanoparticles, attaching them to antibodies targeting the Ephrin receptor A2, prevalent in NSCLC. These Ephrin-coupled NPs significantly increased A549 cells’ radiosensitivity (77). Kin et al. crafted diblock copolymer nanoparticles for the sequential release of warfarin and docetaxel into H460 cells, which, when combined with radiotherapy, outperformed other treatment modalities (78).
Moataz’s team developed C-siPLK1-NPs carrying cetuximab, an EGFR-targeting monoclonal antibody, and siPLK1, an siRNA targeting the mitotic regulator PLK1. These nanoparticles effectively targeted A549 and H460 cells, reduced PLK1 expression, induced G2/M blockade, and acted as radiosensitizers (79). Shuzhen Chen et al. utilized Fe3O4 magnetic NPs to carry SiBIRC5 and BIRC5 antisense oligodeoxynucleotides, addressing the upregulated anti-apoptotic protein BIRC5 in NSCLC. These magnetic NPs enhanced drug uptake in A549 and H460 cells, diminished BIRC5 expression, and increased death receptor 5 expression, thereby improving radiotherapy’s therapeutic effect. Moreover, Magnetic field guidance further amplified drug enrichment in tumors (80). Jinghua Han’s team engineered nanoparticles loaded with DOX and 5-aminolevulinic acid, a radiosensitizer, coupled with a neurotensin receptor 1 ligand to target neurotensin receptor 1-high-expressing H1299 cells. The acidic tumor environment triggered DOX release for cytotoxic impact, while 5-aminolevulinic acid targeted mitochondria, enhancing radiation-induced oxidative stress and the overall efficacy of radiation therapy in treating H1299 cells (81).
Packaging two types of drugs into nanoparticles, one being an FDA-approved chemotherapy drug and the other a radiosensitizer, has become the choice of many researchers. On the basis of guaranteeing precision in tumor targeting and releasing medications in the optimal order, this approach is capable of amalgamating traditional drugs, consequently contributing to a more efficacious anti-cancer impact.
XIAP, an inhibitor of apoptotic protease-3, plays a crucial role in cellular apoptosis. By using liposomes to transport siXIAP into both p53 wild-type and mutant H1299 cells, researchers significantly enhanced radiosensitivity, particularly in p53-mutant cells (82). This study also lacked in vivo experiments.
Survivin, known as a radioresistance factor, can be counteracted by mS-T34A, a plasmid that prevents survivin from binding to activated caspase-9. Qing-Zhong’s team used liposomes to create Lip-mS, effectively increasing cell apoptosis, inhibiting tumor angiogenesis, and enhancing radiosensitivity in Lewis cells (83).
The DNA double-strand repair inhibitor KU55933, known for inhibiting DNA double-strand break repair in H460 cells, saw improved radiosensitizing effects when loaded onto nano-lipid polymers by Xi Tian and his colleagues (84).
Radiotherapy of primary tumors in concert with immunoadjuvants can lead to regression of tumors out of the radiation field, which is called as abscopal effect (85). Yao Hao et al. used biodegradable nanopolymers encapsulated with anti-CD40 antibody to significantly enhance radiotherapy’s effect in Lewis cells, slowing tumor growth both within and outside irradiated fields and improving mice survival (86).
All three studies mentioned above were deficient in drug safety evaluations. There was room for enhancement in this area.
Lonidamine, an oxidative phosphorylation inhibitor, was innovatively combined with mitochondria-targeted triphenylphosphine cation and encapsulated in liposomes by Saijun Wang’s team to form TPP- Lonidamine@Lip, which activated AMP-dependent protein kinase through oxidative phosphorylation inhibition, reduced PD-L1 expression, and bolstered anti-tumor immunity. Additionally, it reversed tumor hypoxia, making A549 cells more radiosensitive (87). While this research was quite comprehensive, it would be more beneficial if an in-situ tumor model was utilized.
Presented below, Table 8 enumerates various nano-radiosensitizers designed to carry other types of drugs or multiple drugs.
Wensha Yang and his colleagues utilized polyethylene glycol-coated, amine-functionalized semiconductor nanocrystals (QDs), which, under X-ray irradiation, excited photons to activate photosensitizers. Applied to H460 cells, these QD-photosensitizer conjugates, in conjunction with radiotherapy, were more effective in cell destruction compared to other treatments (88).
Jun Ma’s team developed Ce6/PTX 2-NP/G@NHs, polymer nanoparticles composed of the paclitaxel prodrug and photosensitizer Ce6. These nanoparticles were readily uptaken by NSCLC cells, with Ce6 promoting ROS production and creating a hypoxic environment under laser irradiation. This induced PTX release, directly killing cancer cells and inactivating the PI3K/AKT pathway. As a result, the nanoparticles increased apoptosis in A549 cells, especially when combined with radiotherapy (89).
Cypate (Cyp), an indocyanine green derivative, generates heat under near-infrared light irradiation. Cyp-polymethylmethacrylic acid-Fe@MSCs, comprising polymethylmethacrylic acid nanoparticles loaded with iron and Cyp, encapsulated in mesenchymal stem cell membranes, were more effective in targeting Lewis cells, leading to significant tumor shrinkage under laser and X-ray irradiation (90).
Magnetic nanoparticle clusters (MNCs), wrapped with polyacrylic acid for biocompatibility, generated heat in an alternating magnetic field and were used for cancer thermotherapy. Jia Ma et al. found that MNCs-treated H460 cells showed increased expression of Hsp70 and caspase-3 under alternating magnetic field and radiotherapy, significantly suppressing tumors more than other treatments (91).
Mn-Zn ferrite magnetic nanoparticles, similar to MNCs, were integrated into PEG-β-PCL block copolymer micelles and modified with hyaluronic acid targeting A549 cells. In tumors, Mn-Zn ferrite magnetic NPs not only generated heat, but also raised oxygenation levels under alternating magnetic field, thereby enhancing A549 cells’ radiosensitivity (92).
Tsl-MTX, comprising 1-methylxanthine and temperature-sensitive liposomes, released its contents upon local heating of tumors, achieving pronounced tumor regression, particularly when combined with radiotherapy (93).
Yun Hu’s team explored the potential of hafnium oxide nanoparticles NBTXR3 in an anti-PD1 resistant lung cancer model 344SQR. Only the group receiving NBTXR3 with high and low-dose irradiation (12Gy*3F and 1Gy*2F) plus immunotherapy (anti-PD1 and anti-CTLA-4) exhibited significant CD8+ T cell/Treg cell ratio improvement and tumor regression, highlighting NBTXR3’s synergy with radiation and immunotherapy (94). In further studies, combining NBTXR3 with radiotherapy and inhibitors of TIGIT and LAG3, the team demonstrated a significantly enhanced treatment effect, supporting the clinical translation of NBTXR3 (95).
Ying Wang et al. found that cisplatin-loaded nanoparticles induced CXCL10 secretion in tumors. Post-irradiation, there was increased CD8+ T cell infiltration in both irradiated and unirradiated tumors. Combining this with anti-PD1 therapy resulted in significantly greater tumor regression, illustrating cisplatin-loaded NPs’ role in boosting anti-tumor immunity post-radiotherapy and achieving an abscopal effect (96).
Leveraging nanotechnology to enhance radiation sensitization, and pairing it with phototherapy or thermotherapy could significantly improve the tumor-targeting ability and anti-cancer efficacy of drugs. Moreover, immunotherapy has emerged as one of the most promising research fields in oncology in recent years, suggesting that boosting anti-cancer immunity holds substantial potential for exploration. By integrating the use of nanomedicine, it allows us to combine differing methods of cancer treatment, thus offering profound clinical implications.
Presented next is Table 9, showcasing nano-radiosensitizers that are employed in combination with radiotherapy plus phototherapy, thermotherapy, or immunotherapy.
Table 9 Types of nano-radiosensitizers in combination with IR plus phototherapy, thermotherapy, or immunotherapy.
The combination of paclitaxel and platinum represents a primary treatment option for advanced NSCLC (97). Paclitaxel’s poor water solubility often necessitates its dissolution in lox, which is linked to allergic reactions and neurotoxicity (50). In a significant development, Neil Desai et al., in 2006, synthesized 130nm albumin-paclitaxel particles (nab-P), which demonstrated enhanced anti-tumor effects and reduced toxicity compared to traditional paclitaxel (98). The FDA approved nab-P for first-line NSCLC treatment in 2012.
The inaugural phase I clinical study combining nab-P and radiotherapy in NSCLC was conducted by V. L. Keedy’s team in 2010. Administering a 66Gy/33F + nab-P+ carboplatin regimen to 11 pts with locally advanced NSCLC, they observed 9 partial responses (PR), 1 stable disease (SD), and 1 withdrawal post-consent. The most severe adverse event was grade 3, indicating that a 40mg/m2 weekly nab-P regimen is safer in combination with carboplatin and radiotherapy (99).
In 2017, Kan Wu et al. reported a phase II clinical trial of radiotherapy combined with carboplatin + 60mg/m2 nab-P in locally advanced squamous cell lung cancer. Of 8 pts, 5 showed PR, 2 had SD, and 1 experienced progressed disease (PD). The median progression-free survival (mPFS) and overall survival (mOS) were 12.1 and 15.2 months, respectively (100).
These two studies represented the earliest phase I or II clinical trials involving the use of nab-P l during radiotherapy for NSCLC pts, with the disadvantage of having few participants. In subsequent clinical trials, the number of participants was relatively increased, compensating for this drawback.
Ryo Shimoyama et al. initiated a phase 3 clinical trial in 2020, investigating synchronized carboplatin with or without nab-P during radiotherapy for stage III NSCLC. Results of this ongoing study are highly anticipated (101).
Up to now, clinical studies on nab-P plus carboplatin during radiotherapy are limited. Although the combination is a class I recommendation for NSCLC patients, conventional paclitaxel plus carboplatin remains the recommended synchronous chemotherapy regimen during radiotherapy. Further research is needed to validate nab-P plus carboplatin as synchronized chemotherapy in NSCLC.
In parallel, C. Shen et al. conducted a multicenter, open-label phase I study on NBTXR3, involving patients with various cancers, including lung cancer. Early results from this ongoing trial, which is still recruiting patients, have shown overall tumor regression in 8 out of 9 patients, including 4 with lung cancer. These promising findings highlight the potential of combining SBRT with NBTXR3 and anti PD-1 therapy in solid tumors, and emphasize the necessity for further research involving more patients with inoperable NSCLC and other malignancies (102).
More clinical trials are described in Table 10 (99–108).
The burgeoning advancement of novel nanomaterials in biomedicine offers an array of possibilities for augmenting the efficacy of radiotherapy in treating tumors clinically. This article delves into various nano-radiosensitizers, which either intrinsically heighten the radiosensitivity of NSCLC cells or act as carriers for radiosensitive drugs, thereby localizing their delivery to tumors. This synchronization of radiotherapy and targeted drug action amplifies the radiosensitivity of NSCLC cells through diverse mechanisms.
Nonetheless, the clinical adoption of these nano-radiosensitizers is not without hurdles. Unlike traditional drugs, nanodrugs, due to their smaller sizes, may not biodegrade, potentially leading to long-term accumulation in the body and resultant unknown toxic side effects, which limits their utility in radiotherapy. As such, the future direction in this field lies in bolstering biocompatibility, enhancing tumor-targeting capabilities, optimizing drug loading capacity, and cutting costs without compromising biosafety. Moreover, the multifunctionality of nanoparticles should be fully harnessed to facilitate tumor imaging and to extend the amalgamation of various treatments such as radiotherapy, chemotherapy, thermotherapy, phototherapy, and immunotherapy, thereby transcending the restrictions of singular treatment modes. Current clinical trials predominantly involve small sample sizes and have follow-up periods generally ranging from one to two years. Hence, there is a pressing need for more comprehensive studies with extended follow-up periods to evaluate the long-term efficacy of nano-radiosensitizers.
In tandem with this, the merging of radiosensitizers with proton therapy is viewed as a promising avenue of future development. Proton therapy, in contrast to traditional radiotherapy, offers specific dosimetric advantages and fewer off-target effects. Therefore, coupling proton therapy with nano-radiosensitizers corresponds more closely with the objectives of precision medicine. Nonetheless, up to this point, scant researches have been conducted on the combination of radiosensitizers with proton therapy in the treatment of NSCLC.
Researchers such as Bronk treated lung cancer cells by nanoscaffold, discovering it amplified the effectiveness of radiotherapy (109). In addition, Yun’s team inoculated one side of a mouse limb with PD-1 inhibitor-resistant 344SQR cells and treated them with NBTXR3, PD-1 inhibitors, or proton therapy. After 76 days, they inoculated 344SQR cells on the other side again. As a result, they found that mice subjected to the triple combination therapy displayed increased infiltration and activation of cytotoxic immune cells, underscoring remarkable anti-tumor treatment effects (110). While neither study compared proton therapy with X-ray therapy, the outstanding efficacy of proton therapy should not be sidelined. The future holds the promise of extensive research exploring the amalgamation of nano-radiosensitizers with proton therapy.
Despite these hurdles, the field of nano-radiosensitizers teems with immense potential. As studies advance, it is entirely conceivable that nano-radiosensitizers could emerge as a favored option in the treatment arsenal for NSCLC, thereby ushering in novel dimensions in cancer therapy.
XH: Conceptualization, Methodology, Project administration, Visualization, Writing – original draft, Writing – review & editing. JH: Data curation, Formal Analysis, Methodology, Writing – review & editing. YP: Formal Analysis, Project administration, Software, Writing – review & editing. MW: Investigation, Software, Writing – review & editing. WZ: Supervision, Validation, Writing – review & editing. XX: Supervision, Validation, Writing – review & editing. CZ: Funding acquisition, Resources, Visualization, Writing – review & editing. XW: Project administration, Resources, Visualization, Writing – review & editing. XS: Conceptualization, Writing – review & editing.
The authors declare financial support was received for the research, authorship, and/or publication of this article. This work was supported by National OutstandingYouth Science Fund Project of National Natural Science Foundation of China (82202929).
The authors declare that the research was conducted in the absence of any commercial or financial relationships that could be construed as a potential conflict of interest.
All claims expressed in this article are solely those of the authors and do not necessarily represent those of their affiliated organizations, or those of the publisher, the editors and the reviewers. Any product that may be evaluated in this article, or claim that may be made by its manufacturer, is not guaranteed or endorsed by the publisher.
NSCLC, non-small cell lung cancer; ROS, reactive oxygen species; GNPs, gold nanoparticles; Glu-GNPs, thio-glucose-bound gold nanoparticles; SER, sensitization enhancement ratio; AgNP, silver nanoparticle; AGuIX, gadolinium nanoparticle; LOX, the copper oxide-dependent enzyme; Bi2Se3, bismuth selenide nanoparticle; MSC, mesenchymal stromal cells; SeNP, Selenium nanoparticle; PTX, paclitaxel; PEG, polyethylene glycol; Cum, curcumin; NO, nitric oxide; SP1, specificity protein 1; QD, semiconductor nanocrystals; Cyp, cypate; MNC, magnetic nanoparticle clusters; DOX, doxorubicin; PR, partial response; SD, stable disease; PD, progressed disease; mPFS, median progression-free survival; mOS, median overall survival; nab-P, albumin-paclitaxel.
1. Nam J, Son S, Park K, Zou W, Shea LD, Moon JJ. Cancer nanomedicine for combination cancer immunotherapy. Nat Rev Mater. (2019) 4:398–414. doi: 10.1038/s41578-019-0108-1
2. Cramer JD, Burtness B, Le QT, Ferris RL. The changing therapeutic landscape of head and neck cancer. Nat Rev Clin Oncol. (2019) 16:669–83. doi: 10.1038/s41571-019-0227-z
3. Chaft JE, Rimner A, Weder W, Azzoli CG, Kris MG, Cascone T. Evolution of systemic therapy for stages I-III non-metastatic non-small-cell lung cancer. Nat Rev Clin Oncol. (2021) 18:547–57. doi: 10.1038/s41571-021-00501-4
4. Buckley AM, Lynam-Lennon N, O’Neill H, O’Sullivan J. Targeting hallmarks of cancer to enhance radiosensitivity in gastrointestinal cancers. Nat Rev Gastroenterol Hepatol. (2020) 17:298–313. doi: 10.1038/s41575-019-0247-2
5. Mechthild K, Anna D, Annett L, Michael B. Cancer stem cells: radioresistance. Adv Drug Deliv Rev. (2016) 109:63–73. doi: 10.1016/j.addr
6. Gill MR, Vallis KA. Transition metal compounds as cancer radiosensitizers. Chem Soc Rev. (2019) 48:540–57. doi: 10.1039/c8cs00641e
7. Lim EK, Kim T, Paik S, Haam S, Huh YM, Lee K. Nanomaterials for theranostics: recent advances and future challenges. Chem Rev. (2015) 115:327–94. doi: 10.1021/cr300213b
8. Acharya S, Sahoo SK. PLGA nanoparticles containing various anticancer agents and tumour delivery by EPR effect. Adv Drug Delivery Rev. (2011) 63:170–83. doi: 10.1016/j.addr.2010.10.008
9. Regulla DF, Hieber LB, Seidenbusch M. Physical and biological interface dose effects in tissue due to X-ray-induced release of secondary radiation from metallic gold surfaces. Radiat Res. (1998) 150:92–100.
10. Herold DM, Das IJ, Stobbe CC, Iyer RV, Chapman JD. Gold microspheres: a selective technique for producing biologically effective dose enhancement. Int J Radiat Biol. (2000) 76:1357–64. doi: 10.1080/09553000050151637
11. Marques T, Schwarcke M, Garrido C, Baffa O, Nicolucci P. Benefits of radiotherapy added nanoparticle assessed by a quantitative analysis of dose-gradient: an evaluation in soft and lung tissues by monte carlo. Med Phys. (2010) . 37:3174–4. doi: 10.1118/1.3468350
12. Wang C, Li X, Wang Y, Liu Z, Fu L, Hu L. Enhancement of radiation effect and increase of apoptosis in lung cancer cells by thio-glucose-bound gold nanoparticles at megavoltage radiation energies. J Nanopart Res. (2013) 15:1642. doi: 10.1007/s11051-013-1642-1
13. Tao L, Guo JZ. Enhancement of radiation effect on lung cancer A549 cells by gold nanoparticles. J Clin Oncol. (2018) 36:15. doi: 10.1200/jco.2018.36.15_suppl.e20505
14. Soleymanifard S, Rostami A, Aledavood SA, Matin MM, Sazgarnia A. Increased radiotoxicity in two cancerous cell lines irradiated by low and high energy photons in the presence of thio-glucose bound gold nanoparticles. Int J Radiat Biol. (2017) 93:407–15. doi: 10.1080/09553002.2017.1268282
15. Shahhoseini E, Feltis BN, Nakayama M, Piva TJ, Pouniotis D, Alghamdi SS, et al. Combined effects of gold nanoparticles and ionizing radiation on human prostate and lung cancer cell migration. Int J Mol Sci. (2019) 20:4488. doi: 10.3390/ijms20184488
16. Chen Y, Liu S, Liao Y, Yang H, Chen Z, Hu Y, et al. Albumin-modified gold nanoparticles as novel radiosensitizers for enhancing lung cancer radiotherapy. Int J Nanomed. (2023) 18:1949–64. doi: 10.2147/IJN.S398254
17. Oumano M, Ngwa W, Celli J, Arnoldussen M, Hanlon J, Hempstead J, et al. TU-H-CAMPUS-teP3-01: gold nanoparticle-enhanced radiation therapy in in vitro A549 lung carcinoma: studies in both traditional monolayer and three-dimensional cell culture models. Med Phys. (2016) 43:3787–7. doi: 10.1118/1.4957704
18. Gadoue SM, Toomeh D. Radio-sensitization efficacy of gold nanoparticles in inhalational nanomedicine and the adverse effect of nano-detachment due to coating inactivation. Phys Med. (2019) 60:7–13. doi: 10.1016/j.ejmp.2019.02.013
19. Telli FC, Demir B, Barlas FB, Guler E, Timur S, Salman Y. Novel glyconanoconjugates: synthesis, characterization and bioapplications. RSC Adv. (2016) 6:105806–13. doi: 10.1039/c6ra21976d
20. Pandey A, Vighetto V, Di Marzio N, Ferraro F, Hirsch M, Ferrante N, et al. Gold nanoparticles radio-sensitize and reduce cell survival in lewis lung carcinoma. Nanomater (Basel). (2020) 10:1717. doi: 10.3390/nano10091717
21. Sunil GSN, Rajasowmiya S, Vadivel V, Banu DS, Celestin JA, Marimuthu S, et al. Gallic acid-coated sliver nanoparticle alters the expression of radiation-induced epithelial-mesenchymal transition in non-small lung cancer cells. Toxicol In Vitro. (2018) 52:170–7. doi: 10.1016/j.tiv.2018.06.015
22. Holmila RJ, Vance SA, King SB, Tsang AW, Singh R, Furdui CM. Silver nanoparticles induce mitochondrial protein oxidation in lung cells impacting cell cycle and proliferation. Antioxid (Basel). (2019) 8:552. doi: 10.3390/antiox8110552
23. Du Y, Sun H, Lux F, Xie Y, Du L, Xu C, et al. Radiosensitization effect of AGuIX, a gadolinium-based nanoparticle, in non-small cell lung cancer. ACS Appl Mater Interfaces. (2020) 12:56874–85. doi: 10.1021/acsami.0c16548
24. Liu W, Deacon J, Yan H, Sun B, Liu Y, Hegan D, et al. Tumor-targeted pH-low insertion peptide delivery of theranostic gadolinium nanoparticles for image-guided nanoparticle-enhanced radiation therapy. Transl Oncol. (2020) 13:100839. doi: 10.1016/j.tranon
25. Lee C, Liu X, Zhang W, Duncan MA, Jiang F, Kim C, et al. Ultrasmall Gd@Cdots as a radiosensitizing agent for non-small cell lung cancer. Nanoscale. (2021) 13:9252–63. doi: 10.1039/d0nr08166c
26. Ma X, Lee C, Zhang T, Cai J, Wang H, Jiang F, et al. Image-guided selection of Gd@C-dots as sensitizers to improve radiotherapy of non-small cell lung cancer. J Nanobiotechnol. (2021) 19:284. doi: 10.1186/s12951-021-01018-9
27. Rocchi P, Brichart VD, Lux F, Morfin I, David L, Rodriguez LC, et al. A new generation of ultrasmall nanoparticles inducing sensitization to irradiation and copper depletion to overcome radioresistant and invasive cancers. Pharmaceutics. (2022) 14:814. doi: 10.3390/pharmaceutics14040814
28. Kotb S, Detappe A, Lux F, Appaix F, Barbier EL, Tran VL, et al. Gadolinium-based nanoparticles and radiation therapy for multiple brain melanoma metastases: proof of concept before phase I trial. Theranostics. (2016) 6:418–27. doi: 10.7150/thno.14018
29. Thivat E, Casile M, Moreau J, Molnar I, Dufort S, Seddik K, et al. Phase I/II study testing the combination of AGuIX nanoparticles with radiochemotherapy and concomitant temozolomide in patients with newly diagnosed glioblastoma (NANO-GBM trial protocol). BMC Cancer. (2023) 23:344. doi: 10.1186/s12885-023-10829-y
30. Ouyang Z, Liu B, Yasmin-Karim S, Sajo E, Ngwa W. Nanoparticle-aided external beam radiotherapy leveraging the Čerenkov effect. Phys Med. (2016) 32:944–7. doi: 10.1016/j.ejmp.2016.06.015
31. Zangeneh M, Nedaei HA, Mozdarani H, Mahmoudzadeh A, Salimi M. Enhanced cytotoxic and genotoxic effects of gadolinium-doped ZnO nanoparticles on irradiated lung cancer cells at megavoltage radiation energies. Mater Sci Eng C Mater Biol Appl. (2019) 103:109739. doi: 10.1016/j.msec.2019.109739
32. Xiao J, Zeng L, Ding S, Chen Y, Zhang X, Bian XW, et al. Tumor-tropic adipose-derived mesenchymal stromal cell mediated bi2 se3 nano-radiosensitizers delivery for targeted radiotherapy of non-small cell lung cancer. Adv Healthc Mater. (2022) 11:e2200143. doi: 10.1002/adhm.202200143
33. Sun Y, Miao H, Ma S, Zhang L, You C, Tang F, et al. FePt-Cys nanoparticles induce ROS-dependent cell toxicity, and enhance chemo-radiation sensitivity of NSCLC cells in vivo and in vitro. Cancer Lett. (2018) 418:27–40. doi: 10.1016/j.canlet.2018.01.024
34. Ma S, Miao H, Luo Y, Sun Y, Tian X, Wang F, et al. FePt/GO nanosheets suppress proliferation, enhance radiosensitization and induce autophagy of human non-small cell lung cancer cells. Int J Biol Sci. (2019) 15:999–1009. doi: 10.7150/ijbs.29805
35. Cho MH, Choi ES, Kim S, Goh SH, Choi Y. Redox-responsive manganese dioxide nanoparticles for enhanced MR imaging and radiotherapy of lung cancer. Front Chem. (2017) 5:109. doi: 10.3389/fchem
36. Liu X, Kifle MT, Xie H, Xu L, Luo M, Li Y, et al. Biomineralized manganese oxide nanoparticles synergistically relieve tumor hypoxia and activate immune response with radiotherapy in non-small cell lung cancer. Nanomater (Basel). (2022) 12:3138. doi: 10.3390/nano12183138
37. Li F, Li Z, Jin X, Liu Y, Zhang P, Li P, et al. Ultra-small gadolinium oxide nanocrystal sensitization of non-small-cell lung cancer cells toward X-ray irradiation by promoting cytostatic autophagy. Int J Nanomed. (2019) 14:2415–31. doi: 10.2147/IJN.S193676
38. Li Y, Yang J, Gu G, Guo X, He C, Sun J, et al. Pulmonary delivery of theranostic nanoclusters for lung cancer ferroptosis with enhanced chemodynamic/radiation synergistic therapy. Nano Lett. (2022) 22:963–72. doi: 10.1021/acs.nanolett.1c03786
39. Cruz LY, Wang D, Liu J. Biosynthesis of selenium nanoparticles, characterization and X-ray induced radiotherapy for the treatment of lung cancer with interstitial lung disease. J Photochem Photobiol B. (2019) 191:123–7. doi: 10.1016/j.jphotobiol.2018.12.008
40. Zhang H, Sun Q, Tong L, Hao Y, Yu T. Synergistic combination of PEGylated selenium nanoparticles and X-ray-induced radiotherapy for enhanced anticancer effect in human lung carcinoma. BioMed Pharmacother. (2018) 107:1135–41. doi: 10.1016/j.biopha.2018.08.074
41. Tian J, Wei X, Zhang W, Xu A. Effects of selenium nanoparticles combined with radiotherapy on lung cancer cells. Front Bioeng Biotechnol. (2020) 8:598997. doi: 10.3389/fbioe.2020.598997
42. Nie S, He X, Sun Z, Zhang Y, Liu T, Chen T, et al. Selenium speciation-dependent cancer radiosensitization by induction of G2/M cell cycle arrest and apoptosis. Front Bioeng Biotechnol. (2023) 11:1168827. doi: 10.3389/fbioe.2023.1168827
43. Thangirala S, Mahboob UR, Noureldien HED, Melis DC, Jahangir AS, Paul JD, et al. Nano-targeting of thyrointegrin αvβ3 receptor in solid tumors and impact on radiosensitization. Radiat Res. (2021) 196:375–85. doi: 10.1667/RADE-21-00031.1
44. Chen MH, Hanagata N, Ikoma T, Huang JY, Li KY, Lin CP, et al. Hafnium-doped hydroxyapatite nanoparticles with ionizing radiation for lung cancer treatment. Acta Biomater. (2016) 37:165–73. doi: 10.1016/j.actbio.2016.04.004
45. Müller M, Espinoza S, Jüstel T, Held KD, Anderson RR, Purschke M. UVC-emitting luPO4:Pr3+ Nanoparticles decrease radiation resistance of hypoxic cancer cells. Radiat Res. (2020) 193:82–7. doi: 10.1667/RR15491.1
46. Tran TA, Kappelhoff J, Jüstel T, Anderson RR, Purschke M. UV emitting nanoparticles enhance the effect of ionizing radiation in 3D lung cancer spheroids. Int J Radiat Biol. (2022) 98:1484–94. doi: 10.1080/09553002.2022.2027541
47. Toulany M, Mihatsch J, Holler M, Chaachouay H, Rodemann HP. Cisplatin-mediated radiosensitization of non-small cell lung cancer cells is stimulated by ATM inhibition. Radiother Oncol. (2014) 111:228–36. doi: 10.1016/j.radonc.2014.04.001
48. Loprevite M, Favoni RE, de Cupis A, Pirani P, Pietra G, Bruno S, et al. Interaction between novel anticancer agents and radiation in non-small cell lung cancer cell lines. Lung Cancer. (2001) 33:27–39. doi: 10.1016/s0169-5002(00)00247-6
49. Dorn P, Tièche CC, Peng RW, Froment L, Schmid RA, Marti TM. Schedule-dependent increased efficiency of pemetrexed-ionizing radiation combination therapy elicits a differential DNA damage response in lung cancer cells. Cancer Cell Int. (2016) 16:66. doi: 10.1186/s12935-016-0346-x
50. Gelderblom H, Verweij J, Nooter K, Sparreboom A. Cremophor EL: the drawbacks and advantages of vehicle selection for drug formulation. Eur J Cancer. (2001) 37:1590–8. doi: 10.1016/s0959-8049(01)00171-x
51. Negishi T, Koizumi F, Uchino H, Kuroda J, Kawaguchi T, Naito S, et al. NK105, a paclitaxel-incorporating micellar nanoparticle, is a more potent radiosensitising agent compared to free paclitaxel. Br J Cancer. (2006) 95:601–6. doi: 10.1038/sj.bjc.6603311
52. Werner ME, Cummings ND, Sethi M, Wang EC, Sukumar R, Moore DT, et al. Preclinical evaluation of Genexol-PM, a nanoparticle formulation of paclitaxel, as a novel radiosensitizer for the treatment of non-small cell lung cancer. Int J Radiat Oncol Biol Phys. (2013) 86:463–8. doi: 10.1016/j.ijrobp.2013.02.009
53. Cho W, Kim MS, Lee KH, Park SJ, Shin HJ, Lee YJ, et al. Ionizing radiation attracts tumor targeting and apoptosis by radiotropic lysyl oxidase traceable nanoparticles. Nanomedicine. (2020) 24:102141. doi: 10.1016/j.nano.2019.102141
54. Hao Y, Altundal Y, Sajo E, Detappe A, Makrigiorgos G, Berbeco R, et al. WE-G-BRE-06: new potential for enhancing external beam radiotherapy for lung cancer using FDA-approved concentrations of cisplatin or carboplatin nanoparticles administered via inhalation. Med Phys. (2014) 41:518–8. doi: 10.1118/1.4889481
55. Jung J, Jeong SY, Park SS, Shin SH, Ju EJ, Choi J, et al. A cisplatin−incorporated liposome that targets the epidermal growth factor receptor enhances radiotherapeutic efficacy without nephrotoxicity. Int J Oncol. (2015) 46:1268–74. doi: 10.3892/ijo.2014.2806
56. Rocca JD, Werner ME, Kramer SA, Huxford PRC, Sukumar R, Cummings ND, et al. Polysilsesquioxane nanoparticles for triggered release of cisplatin and effective cancer chemoradiotherapy. Nanomedicine. (2015) 11:31–8. doi: 10.1016/j.nano.2014.07.004
57. Zhang M, Hagan CT4, Foley H, Tian X, Yang F, Au KM, et al. Co-delivery of etoposide and cisplatin in dual-drug loaded nanoparticles synergistically improves chemoradiotherapy in non-small cell lung cancer models. Acta Biomater. (2021) 124:327–35. doi: 10.1016/j.actbio.2021.02.001
58. Chen JL, Yang SJ, Pan CK, Lin LC, Tsai CY, Wang CH, et al. Cisplatin and albumin-based gold-cisplatin nanoparticles enhance ablative radiation therapy-induced antitumor immunity in local and distant tumor microenvironment. Int J Radiat Oncol Biol Phys. (2023) 116:1135–49. doi: 10.1016/j.ijrobp.2023.02.014
59. Ji L, Dong SH, Tian JH, Yu MH, Yan Z, Zhen L, et al. Gold mineralized “hybrid nanozyme bomb” for NIR-II triggered tumor effective permeation and cocktail therapy. Chin Chem Lett. (2023), 109296. doi: 10.1016/j.cclet.2023.109296
60. Wang J, Zhang Y, Zhang G, Xiang L, Pang H, Xiong K, et al. Radiotherapy-induced enrichment of EGF-modified doxorubicin nanoparticles enhances the therapeutic outcome of lung cancer. Drug Delivery. (2022) 29:588–99. doi: 10.1080/10717544.2022.2036871
61. Abhay KS, Chandresh S, Calvin L, Wendy Z, Sapna D, Vaishali K, et al. Abstract 1446: GRP78 targeting peptides deliver liposomal doxorubicin specifically to cancer and enhance the efficacy of radiation therapy. Cancer Res. (2021) 81:1446. doi: 10.1158/1538-7445.AM2021-1446
62. Cilento MA, Poplawski NK, Paramasivam S, Thomas DM, Kichenadasse G. Germline PALB2 variants and PARP inhibitors in endometrial cancer. J Natl Compr Canc Netw. (2021) 19:1212–7. doi: 10.6004/jnccn.2021.7067
63. Melinda LT, William JG. Updates in HER2-positive and triple-negative breast cancers. J Natl Compr Canc Netw. (2021) 19:605–9. doi: 10.6004/jnccn.2021.5005
64. Tempero MA. NCCN guidelines updates: pancreatic cancer. J Natl Compr Canc Netw. (2019) 17:603–5. doi: 10.6004/jnccn.2019.5007
65. Wu M, Liu J, Hu C, Li D, Yang J, Wu Z, et al. Olaparib nanoparticles potentiated radiosensitization effects on lung cancer. Int J Nanomed. (2018) 13:8461–72. doi: 10.2147/IJN.S181546
66. Wen C, Zhou Y, Zhou C, Zhang Y, Hu X, Li J, et al. Enhanced radiosensitization effect of curcumin delivered by PVP-PCL nanoparticle in lung cancer. J Nanomater. (2017). doi: 10.1155/2017/9625909
67. Ngwa W, Kumar R, Moreau M, Dabney R, Herman A. Nanoparticle drones to target lung cancer with radiosensitizers and cannabinoids. Front Oncol. (2017) 7:208. doi: 10.3389/fonc.2017.00208
68. Gao S, Zhang W, Wang R, Hopkins SP, Spagnoli JC, Racin M, et al. Nanoparticles encapsulating nitrosylated maytansine to enhance radiation therapy. ACS Nano. (2020) 14:1468–81. doi: 10.1021/acsnano.9b05976
69. Joshi HA, Patwardhan RS, Sharma D, Sandur SK, Devarajan PV. Pre-clinical evaluation of an innovative oral nano-formulation of baicalein for modulation of radiation responses. Int J Pharm. (2021) 595:120181. doi: 10.1016/j.ijpharm.2020.120181
70. Zhang J, Zhang C, Hu L, He Y, Shi Z, Tang S, et al. Abnormal Expression of miR-21 and miR-95 in Cancer Stem-Like Cells is Associated with Radioresistance of Lung Cancer. Cancer Invest. (2015) 33:165–71. doi: 10.3109/07357907.2015.1019676
71. Detappe A, Mathieu C, Jin C, Agius MP, Diringer MC, Tran VL, et al. Anti-MUC1-C antibody-conjugated nanoparticles potentiate the efficacy of fractionated radiation therapy. Int J Radiat Oncol Biol Phys. (2020) 108:1380–9. doi: 10.1016/j.ijrobp.2020.06.069
72. Zhuang M, Jiang S, Gu A, Chen X, Mingyan E. Radiosensitizing effect of gold nanoparticle loaded with small interfering RNA-SP1 on lung cancer: AuNPs-si-SP1 regulates GZMB for radiosensitivity. Transl Oncol. (2021) 14:101210. doi: 10.1016/j.tranon.2021.101210
73. Yang L, Li Z, Binzel DW, Guo P, Williams TM. Targeting oncogenic KRAS in non-small cell lung cancer with EGFR aptamer-conjugated multifunctional RNA nanoparticles. Mol Ther Nucleic Acids. (2023) 33:559–71. doi: 10.1016/j.omtn.2023.07.027
74. Zeng H, Yuan Z, Zhu H, Li L, Shi H, Wang Z, et al. Expression of hPNAS-4 radiosensitizes Lewis lung cancer. Int J Radiat Oncol Biol Phys. (2012) 84:e533–40. doi: 10.1016/j.ijrobp.2012.06.028
75. Li CF, Chen LB, Li DD, Yang L, Zhang BG, Jin JP, et al. Dual−sensitive HRE/Egr1 promoter regulates Smac overexpression and enhances radiation−induced A549 human lung adenocarcinoma cell death under hypoxia. Mol Med Rep. (2014) 10:1108–16. doi: 10.3892/mmr.2014.2233
76. Menon JU, Kuriakose A, Iyer R, Hernandez E, Gandee L, Zhang S, et al. Dual-drug containing core-shell nanoparticles for lung cancer therapy. Sci Rep. (2017) 7:13249. doi: 10.1038/s41598-017-13320-4
77. Iyer R, Ramachandramoorthy H, Nguyen T, Xu C, Fu H, Kotadia T, et al. Lung cancer targeted chemoradiotherapy via dual-stimuli responsive biodegradable core-shell nanoparticles. Pharmaceutics. (2022) 14:1525. doi: 10.3390/pharmaceutics14081525
78. Au KM, Min Y, Tian X, Zhang L, Perello V, Caster JM, et al. Improving cancer chemoradiotherapy treatment by dual controlled release of wortmannin and docetaxel in polymeric nanoparticles. ACS Nano. (2015) 9:8976–96. doi: 10.1021/acsnano.5b02913
79. Reda M, Ngamcherdtrakul W, Gu S, Bejan DS, Siriwon N, Gray JW, et al. PLK1 and EGFR targeted nanoparticle as a radiation sensitizer for non-small cell lung cancer. Cancer Lett. (2019) 467:9–18. doi: 10.1016/j.canlet.2019.09.014
80. Chen S, Han F, Huang D, Meng J, Chu J, Wang M, et al. Fe3O4 magnetic nanoparticle-enhanced radiotherapy for lung adenocarcinoma via delivery of siBIRC5 and AS-ODN. J Transl Med. (2021) 19:337. doi: 10.1186/s12967-021-02971-7
81. Han J, Yang W, Li Y, Li J, Jiang F, Xie J, et al. Combining doxorubicin-conjugated polymeric nanoparticles and 5-aminolevulinic acid for enhancing radiotherapy against lung cancer. Bioconjug Chem. (2022) 33:654–65. doi: 10.1021/acs.bioconjchem.2c00066
82. Ohnishi K, Nagata Y, Takahashi A, Taniguchi S, Ohnishi T. Effective enhancement of X-ray-induced apoptosis in human cancer cells with mutated p53 by siRNA targeting XIAP. Oncol Rep. (2008) 20:57–61. doi: 10.3892/or.20.1.57
83. Yuan QZ, Wang CT, Mao YQ, Zhang P, Shi HS, Li ZY, et al. Enhanced tumor radiosensitivity by a survivin dominant-negative mutant. Oncol Rep. (2010) 23:97–103. doi: 10.3892/or_00000610
84. Tian X, Lara H, Wagner KT, Saripalli S, Hyder SN, Foote M, et al. Improving DNA double-strand repair inhibitor KU55933 therapeutic index in cancer radiotherapy using nanoparticle drug delivery. Nanoscale. (2015) 7:20211–9. doi: 10.1039/c5nr05869d
85. Ashrafizadeh M, Farhood B, Eleojo MA, Taeb S, Rezaeyan A, Najafi M. Abscopal effect in radioimmunotherapy. Int Immunopharmacol. (2020) 85:106663. doi: 10.1016/j.intimp.2020.106663
86. Hao Y, Yasmin KS, Moreau M, Sinha N, Sajo E, Ngwa W. Enhancing radiotherapy for lung cancer using immunoadjuvants delivered in situ from new design radiotherapy biomaterials: a preclinical study. Phys Med Biol. (2016) 61:N697–707. doi: 10.1088/1361-6560/61/24/N697
87. Wang S, Zhou Z, Hu R, Dong M, Zhou X, Ren S, et al. Metabolic intervention liposome boosted lung cancer radio-immunotherapy via hypoxia amelioration and PD-L1 restraint. Adv Sci (Weinh). (2023) 10:e2207608. doi: 10.1002/advs.202207608
88. Yang W, Read PW, Mi J, Baisden JM, Reardon KA, Larner JM, et al. Semiconductor nanoparticles as energy mediators for photosensitizer-enhanced radiotherapy. Int J Radiat Oncol Biol Phys. (2008) 72:633–5. doi: 10.1016/j.ijrobp.2008.06.1916
89. Ma J, Wen C, Chen M, Zhang W, Wang L, Yin H. Ce6/PTX2-NP/G@NHs confer radiosensitivity in non-small cell lung cancer via promotion of apoptotic body-mediated neighboring effects. ACS Biomater Sci Eng. (2023) 9:2793–805. doi: 10.1021/acsbiomaterials.2c01549
90. Yin Y, Li Y, Wang S, Dong Z, Liang C, Sun J, et al. MSCs-engineered biomimetic PMAA nanomedicines for multiple bioimaging-guided and photothermal-enhanced radiotherapy of NSCLC. J Nanobiotechnol. (2021) 19:80. doi: 10.1186/s12951-021-00823-6
91. Ma J, Zhang Z, Zhang Z, Huang J, Qin Y, Li X, et al. Magnetic nanoparticle clusters radiosensitise human nasopharyngeal and lung cancer cells after alternating magnetic field treatment. Int J Hyperthermia. (2015) 31:800–12. doi: 10.3109/02656736.2015.1063168
92. Wang Y, Zou L, Qiang Z, Jiang J, Zhu Z, Ren J. Enhancing targeted cancer treatment by combining hyperthermia and radiotherapy using mn-zn ferrite magnetic nanoparticles. ACS Biomater Sci Eng. (2020) 6:3550–62. doi: 10.1021/acsbiomaterials.0c00287
93. Jeong SY, Yi SL, Lim SK, Park SJ, Jung J, Woo HN, et al. Enhancement of radiotherapeutic effectiveness by temperature-sensitive liposomal 1-methylxanthine. Int J Pharm. (2009) 372:132–9. doi: 10.1016/j.ijpharm.2008.12.040
94. Hu Y, Welsh J, Paris S, Barsoumian H, Abana C, Gandhi S, et al. 200 NBTXR3 nanoparticle with immunoradiation improves survival and generates long-term anti-tumor memory in an anti-PD1 resistant murine lung cancer model. J Immunother Cancer. (2020) 8. doi: 10.1136/jitc-2020-SITC2020.0200
95. Hu Y, Paris S, Bertolet G, Barsoumian HB, He K, Sezen D, et al. Combining a nanoparticle-mediated immunoradiotherapy with dual blockade of LAG3 and TIGIT improves the treatment efficacy in anti-PD1 resistant lung cancer. J Nanobiotechnol. (2022) 20:417. doi: 10.1186/s12951-022-01621-4
96. Wang Y, Shen N, Wang Y, Li M, Zhang W, Fan L, et al. Cisplatin nanoparticles boost abscopal effect of radiation plus anti-PD1 therapy. Biomater Sci. (2021) 9:3019–27. doi: 10.1039/d1bm00112d
97. Ettinger DS, Wood DE, Aisner DL, Akerley W, Bauman JR, Bharat A, et al. NCCN guidelines insights: non-small cell lung cancer, version 2. 2021 J Natl Compr Canc Netw. (2021) 19:254–66. doi: 10.6004/jnccn.2021.0013
98. Desai N, Trieu V, Yao Z, Louie L, Ci S, Yang A, et al. Increased antitumor activity, intratumor paclitaxel concentrations, and endothelial cell transport of cremophor-free, albumin-bound paclitaxel, ABI-007. compared with cremophor-based paclitaxel. Clin Cancer Res. (2006) 12:1317–24. doi: 10.1158/1078-0432.CCR-05-1634
99. Keedy VL, Lu B, Shyr Y, Horn L, Carbone DP, Sandler A, et al. A phase I study of nab-paclitaxel (nab-P) with carboplatin (C) and thoracic radiation (RT) in patients with locally advanced NSCLC. J Clin Oncol. (2010) 28:15. doi: 10.1200/jco.2010.28.15_suppl.e17504
100. Wu K, Zhu L, Wang J, Pan K, Wang B, Li X, et al. A phase II study of concurrent nab-paclitaxel/carboplatin combined with thoracic radiotherapy in locally advanced squamous cell lung cancer. J Thorac Dis. (2019) 11:4529–37. doi: 10.21037/jtd.2019.10.81
101. Shimoyama R, Omori S, Nomura S, Kenmotsu H, Takahashi T, Harada H, et al. Lung Cancer Study Group in the Japan Clinical Oncology Group. A multi-institutional randomized phase III study comparing weekly carboplatin plus nab-paclitaxel and daily low-dose carboplatin as regimens for concurrent chemoradiotherapy in elderly patients with unresectable locally advanced non-small cell lung cancer: Japan Clinical Oncology Group Study JCOG1914. Jpn J Clin Oncol. (2021) 51:836–41. doi: 10.1093/jjco/hyab025
102. Colette S, Jessica MF, Jia XN, Ari R, Jared W, Jimmy JC, et al. A phase I trial evaluating NBTXR3 activated by radiotherapy in combination with nivolumab or pembrolizumab in patients with advanced cancers. J Clin Oncol. (2021) 39:15. doi: 10.1200/jco.2021.39.15_suppl.2590
103. Tsuchiya KY, Sasaki T, Yamaguchi H, Hirano K, Horiike A, Satouchi M, et al. Updated survival data for a phase I/II study of carboplatin plus nab-paclitaxel and concurrent radiotherapy in patients with locally advanced non-small cell lung cancer. Oncologist. (2020) 25:475–e891. doi: 10.1634/theoncologist.2019-0746
104. Kaira K, Tomizawa Y, Imai H, Sakurai R, Matsuura M, Yoshii A, et al. Phase I study of nab-paclitaxel plus carboplatin and concurrent thoracic radiotherapy in patients with locally advanced non-small cell lung cancer. Cancer Chemother Pharmacol. (2017) 79:165–71. doi: 10.1007/s00280-016-3217-1
105. Omori S, Harada H, Mori K, Hisamatsu Y, Tsuboguchi Y, Yoshioka H, et al. Phase I study of weekly nab-paclitaxel plus carboplatin and concurrent thoracic radiotherapy in elderly patients with unresectable locally advanced non-small cell lung cancer. Invest New Drugs. (2022) 40:106–14. doi: 10.1007/s10637-021-01155-w
106. Tanaka H, Hasegawa Y, Makiguchi T, Okumura F, Tabe C, Shiratori T, et al. A phase I/II study of biweekly carboplatin and nab-paclitaxel with concurrent radiotherapy for patients with locally advanced unresectable stage III non-small-cell lung cancer. Clin Lung Cancer. (2021) 22:42–8. doi: 10.1016/j.cllc.2020.09.016
107. Yukihiro H, Dai M, Tomohiro O, Junichi Y. Phase I study of bi-weekly nab-paclitaxel and carboplatin with concurrent thoracic radiotherapy for locally advanced non-small cell lung cancer. J Clin Oncol. (2015) 33:15. doi: 10.1200/jco.2015.33.15_suppl.7529
108. Hasegawa T, Futamura Y, Horiba A, Yoshida T, Suzuki T, Kato T, et al. A phase II study of nab-paclitaxel plus carboplatin in combination with thoracic radiation in patients with locally advanced non-small-cell lung cancer. J Radiat Res. (2016) 57:50–4. doi: 10.1093/jrr/rrv062
109. Bronk LF, Polf JC, Driessen WHP, Gillin MT, Arap W, Pasqualini R. TU-A-108-11: nanoscaffold-enhanced proton therapy. Med Phys. (2013) 40:422–2. doi: 10.1118/1.4815334
Keywords: radiotherapy, nanomaterials, non-small cell lung cancer, radiosensitization, chemotherapy
Citation: Hu X, Hu J, Pang Y, Wang M, Zhou W, Xie X, Zhu C, Wang X and Sun X (2024) Application of nano-radiosensitizers in non-small cell lung cancer. Front. Oncol. 14:1372780. doi: 10.3389/fonc.2024.1372780
Received: 18 January 2024; Accepted: 07 March 2024;
Published: 05 April 2024.
Edited by:
François Chevalier, UMR6252 Centre de Recherche sur les Ions, les Matériaux et la Photonique (CIMAP), FranceReviewed by:
Mathieu Cesaire, Centre François Baclesse, FranceCopyright © 2024 Hu, Hu, Pang, Wang, Zhou, Xie, Zhu, Wang and Sun. This is an open-access article distributed under the terms of the Creative Commons Attribution License (CC BY). The use, distribution or reproduction in other forums is permitted, provided the original author(s) and the copyright owner(s) are credited and that the original publication in this journal is cited, in accordance with accepted academic practice. No use, distribution or reproduction is permitted which does not comply with these terms.
*Correspondence: Xiaonan Sun, c3VueGlhb25hbkB6anUuZWR1LmNu
Disclaimer: All claims expressed in this article are solely those of the authors and do not necessarily represent those of their affiliated organizations, or those of the publisher, the editors and the reviewers. Any product that may be evaluated in this article or claim that may be made by its manufacturer is not guaranteed or endorsed by the publisher.
Research integrity at Frontiers
Learn more about the work of our research integrity team to safeguard the quality of each article we publish.