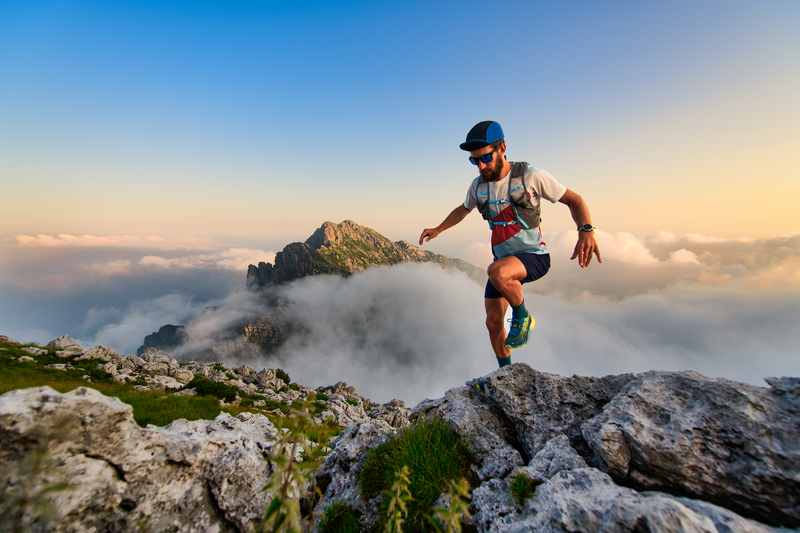
94% of researchers rate our articles as excellent or good
Learn more about the work of our research integrity team to safeguard the quality of each article we publish.
Find out more
REVIEW article
Front. Oncol. , 10 April 2024
Sec. Cancer Immunity and Immunotherapy
Volume 14 - 2024 | https://doi.org/10.3389/fonc.2024.1363695
This article is part of the Research Topic Regulators of the Immune-Tumor Microenvironment: A New Frontier for Cancer Immunotherapy View all 18 articles
Hepatocellular carcinoma (HCC) is a prevalent malignant cancer worldwide, characterized by high morbidity and mortality rates. Alpha-fetoprotein (AFP) is a glycoprotein synthesized by the liver and yolk sac during fetal development. However, the serum levels of AFP exhibit a significant correlation with the onset and progression of HCC in adults. Extensive research has demonstrated that the tumor microenvironment (TME) plays a crucial role in the malignant transformation of HCC, and AFP is a key factor in the TME, promoting HCC development. The objective of this review was to analyze the existing knowledge regarding the role of AFP in the TME. Specifically, this review focused on the effect of AFP on various cells in the TME, tumor immune evasion, and clinical application of AFP in the diagnosis and treatment of HCC. These findings offer valuable insights into the clinical treatment of HCC.
Hepatocellular carcinoma (HCC) is a prevalent malignant neoplasm worldwide, with the third highest fatality rate and increasing incidence and mortality rates (1–3). Despite substantial advancements in contemporary medical knowledge regarding the diagnosis and treatment of HCC, its incidence and mortality rates remain high. Given the high heterogeneity of HCC, early diagnosis and treatment play a crucial role in improving patients’ survival (4, 5). Alpha-fetoprotein (AFP) is an important tumor marker for diagnosing and treating liver cancer. It is also an important indicator for clinical diagnosis of liver cancer metastasis (6, 7). AFP, a glycoprotein primarily synthesized by the yolk sac and fetal liver during embryogenesis, is critically involved in sustaining embryonic development and placental functionality (8). However, the expression of AFP is extremely low in normal adults (9, 10). Nevertheless, when hepatocytes undergo malignant transformation, AFP expression exhibits a substantial increase (11, 12). Consequently, elevated AFP levels serve as a reliable indicator for the development and progression of HCC. Generally, high levels of AFP indicate the presence of HCC, and dynamic changes in AFP level can be used to predict the prognosis and response to treatment in HCC (7). However, there are also some patients with liver cancer who have normal AFP levels or only mildly elevated AFP levels, which may be related to differences in the type, location, and size of the tumor and the regulatory mechanism of AFP secretion (11).
The tumor microenvironment (TME) refers to the local tissue surrounding the tumor, including tumor cells, blood vessels, immune cells, stromal cells, and matrix molecules (13). The interaction between the TME and tumor cells plays an important role in tumorigenesis (14–16). HCCs caused by different etiologies (hepatitis B virus (HBV), hepatitis C virus (HCV), and chronic alcohol drinking) exhibit notable differences in their TME. Alcohol intake has inhibitory effects on immune cells in the TME, decreasing immune surveillance and enabling tumor cells to evade the immune response (17, 18). The expression level of AFP significantly increases during the progression of alcoholic liver disease to HCC (19). However, the molecular mechanisms underlying this process are still poorly understood. Chronic infections with HBV and HCV can lead to immune tolerance, allowing the viral infection to persist and cause hepatocyte damage, finally leading to carcinogenesis (20, 21). Studies have found that in the TME, the X protein of HBV can stimulate the expression of reprogramming-related proteins by increasing AFP expression, thus inducing the proliferation of liver cancer stem cells (LCSCs) (22). HCV infection, on the other hand, upregulates AFP expression, which decreases the activity of NK cells and reduces CD4+ cells in the TME (23, 24). The immune dysregulation in the TME of HCV-related HCC patients is closely related to the elevated levels of AFP. Although there are significant differences in the TME, the function of AFP in the TME remains consistent. AFP promotes the formation of an immune-tolerant microenvironment and facilitates immune evasion of tumor cells.
As a marker of HCC, AFP plays an important regulatory role in the TME. However, the current role of AFP in the TME of HCC is not fully understood. Therefore, a deeper insight into the role of AFP in the TME is of great significance for the early diagnosis and treatment of HCC. This article summarizes the role of AFP in the TME of HCC, especially discussing its effect on liver cancer stem cells, tumor-associated macrophages, cancer-associated fibroblasts (CAFs), endothelial cells, mesenchymal stem cells, tumor immune escape, etc., providing a reference for the clinical diagnosis and treatment of HCC.
AFP is a fetal-specific alpha-globulin synthesized during fetal development and present in fetal blood and tissues. It can also be detected in adult liver and some malignant tumors (25). Human AFP is similar to albumin, composed of a single polypeptide chain with a molecular weight of nearly 69 kDa (25, 26). The single-chain polypeptide of AFP consists of 609 amino acid residues. These amino acid residues are arranged in a specific order to form three structural domains, namely the N-terminal domain (1-210 amino acid residues; domain I), the central domain (211-402 amino acid residues; domain II), and the C-terminal domain (403-609; domain III) (27) (Figure 1A). These three domains of AFP are linked by disulfide bonds to form a V-shaped structure (Figure 1B). AFP possesses three distinct structural domains, each exhibiting diverse biological activities. The N-terminal domain can interact with other molecules, thereby affecting the function of AFP. For example, previous studies have found that AFP domain I can bind to the phosphatase domain of PTEN, thereby affecting PTEN activity (25). The central domain (domain II) consists of approximately 192 amino acid residues, possesses a high degree of flexibility, and is easily digested by proteases. The C-terminal domain (domain III) is the last domain of AFP, which is the most conserved domain, consisting of about 207 amino acid residues. This domain possesses a continuous sequence of several hydrophobic amino acids forming a leucine zipper-like structure. Domain III is responsible for binding to signaling proteins and receptors, thereby regulating their biological activity (26–28). Studies also found that AFP domain III can bind to the C2 domain of PTEN, forming the AFP-PTEN complex, inhibiting PTEN, activating the PI3K/AKT signaling pathway, and promoting HCC progression (25). Domain III can also bind to mucin, scavenger receptors, chemokines, etc. After binding to its receptor, AFP is endocytosed and packaged with the receptor and transported to the cell organelles via the Golgi complex, where it is degraded or activates/blocks cell signaling pathways (26–28).
Figure 1 The basic structure of AFP. (A) The schematic diagram of the human AFP protein sequence with three domains. (B) The structure of AFP and the binding sites of AFP with fatty acids and N-glycosylation. The structure of AFP displays a V-shaped configuration, consisting of domain I (N-terminal) on the left side of the V, domain III (C-terminal) on the right side of the V, and domain II (middle region) at the base of the V.
AFP secretion and expression are not consistent with HCC development. One reason is that AFP has two basic forms: native AFP (nAFP) and tumor-derived AFP (tAFP). nAFP is mainly produced by the fetal yolk sac and fetal liver and secreted into fetal circulation. It is a normal plasma protein and does not promote HCC development. Sometimes, adult individuals express nAFP, which is mainly observed during physiological cell regeneration and hematopoiesis. However, tAFP expressed in TME mainly originates from HCC and can support tumor growth and metastasis. Interestingly, tAFP is not a mutated form of nAFP and differs only slightly in terms of glycosylation and lipid-binding characteristics (29, 30).
AFP described in this article specifically refers to tAFP. AFP exhibits numerous variability, thereby presenting considerable challenges in comprehending its biological activity with HCC (31). AFP variability relies on the types of species, tissues, isoforms, binding ligands, binding partners, and post-translational modifications such as N-glycosylation (Figure 1B). Different variants of AFP possess different biological functions (29); for example, it was found that the serum levels of fucosylated AFP variant increased in patients with HCC but were undetectable in the serum of normal patients (32). Polyunsaturated fatty acids binding AFP variants play an important role in the TME and affect dendritic cells (DCs) and natural killer cells (NK cells) activation (33). Despite extensive studies on the structure of AFP, its biological functions remain unclear. Further studies are imperative to unveil its precise association with various physiological and pathological processes.
The interplay among cells within the TME is a crucial factor in tumor progression, metastasis, and treatment (34, 35). AFP, as a tumor biomarker, directly modulates tumor cell behavior and the TME by interacting with tumor-associated cells and molecules.
Liver cancer stem cells (LCSCs), also known as liver cancer-initiating cells, refer to a subpopulation of cells with self-renewal capacity and stem cell characteristics in the HCC microenvironment. They can produce heterogeneous tumors, are highly invasive and tumorigenic, and can drive tumor growth, metastasis, and recurrence (12, 36). Targeted killing of LCSCs can ameliorate tumor drug resistance and recurrence while preserving normal tissue activity with high specificity. Due to the presence of various subpopulations of tumor cells expressing different markers in HCC, some scholars believe that LCSCs are the key to the development and heterogeneity of HCC (37, 38).
Previous studies indicated that AFP can enhance the expansion of LCSCs by activating the PI3K/Akt signaling pathway (39, 40). To investigate the impact of AFP on LCSCs, researchers measured the AFP expression in HCC cells firstly, then demonstrated that AFP not only facilitates the expression of proteins associated with cell reprogramming but also promotes the expression of LCSCs markers, including CD44, CD133, and EpCAM. Further analyses revealed that AFP can induce the malignant transformation of liver cells by activating the PI3K/AKT signaling pathway, thereby stimulating the expression of reprogramming-related proteins and oncogenes and inducing the generation of stem cells (39) (Figure 2). Simultaneously, several studies have demonstrated that AFP can accelerate liver cancer progression by upregulating LCSC markers K19 and CXCR4 (Figure 2) (41–44). It has also been suggested that AFP-induced tumor stem cells can secrete AFP (43). LCSCs-derived AFP can facilitate immune evasion by regulating the behavior of crucial immune cells in liver cancer cells. Consequently, it has been suggested that AFP can serve as a biomarker for LCSCs (44). AFP primarily facilitates stem cell expansion and preserves stemness. These effects enhance the survival and metastatic potential of LCSCs, thereby fostering the initiation and progression of HCC. Subsequent investigations should elucidate the precise mechanism underlying the interaction between AFP and LCSCs and explore intervention approaches targeting AFP to enhance the treatment effect of liver cancer.
Figure 2 AFP can promote the expansion of LCSCs. AFP upregulates the expression of CD133/CD44 and EPCAM by activating the PI3K/AKT signaling pathway, thereby promoting the expansion of LCSCs. In addition, AFP can directly upregulate the expression of LCSCs marker proteins K19 and CXCR4 to accelerate liver cancer progression.
A significant number of macrophages infiltrate the tumor stroma, referred to as tumor-associated macrophages (TAMs) (45). Experimental evidence indicated that TAMs possess immune regulatory functions and are closely associated with tumor growth and progression (46–48). Tumor cell-derived chemokines, such as CCL2-8 and VEGF, can attract macrophages into the TME and facilitate their differentiation into the M2 phenotype, thereby promoting tumor progression (49). TAMs play a crucial role in the malignant progression of HCC (50–52).
Previous studies have demonstrated that purified human recombinant AFP protein can prevent the phagocytosis of chicken red blood cells by macrophages. Downregulation of AFP dampens this inhibitory effect. Subsequent studies have elucidated that AFP can impede the phagocytic activity of macrophages toward hepatoma cells and other tumor cells through its interaction with macrophage receptors (53–55). A recent study demonstrated that AFP can affect the function of macrophages in phagocytizing liver cancer cells by inducing the polarization of TAMs (55). This study employed human monocytic leukemia cells (THP-1) and monocytes from healthy donors to measure the effect of AFP on macrophage phenotype and phagocytosis. Their findings revealed that AFP can facilitate the polarization of macrophages toward the M2 phenotype and undermine the phagocytic ability of M1 macrophages toward liver cancer cells. Further analyses revealed that this process is related to the activation of the PI3K/Akt signaling pathway. Therefore, AFP is a key cytokine that inhibits liver cancer cell phagocytosis by macrophages (54).
The effect of AFP on TAMs is multifaceted. It is involved in the initiation and progression of HCC, thus holding significant implications for the design of immunotherapeutic approaches for HCC. Nevertheless, research into the influence of AFP on TAMs remains nascent. Further elucidation of the mechanisms involved in the interaction between AFP and TAMs and potential intervention strategies can undoubtedly enhance the clinical efficacy of immunotherapy for liver cancer.
Immune surveillance serves as the principal protective mechanism of the human body against external intrusion or internal genetic alteration, enabling the identification and eradication of tumor cells. Nevertheless, mutant cells can evade immune surveillance through diverse mechanisms, accelerating their proliferation and metastasis, a phenomenon commonly referred to as tumor immune escape. The mechanisms underlying immune evasion of tumor cells encompass a typical expression of antigens on the tumor cell surface, modification of the molecular structure of the tumor cell surface, and secretion of immunosuppressive factors (56).
Based on recent findings, AFP can inhibit the activity of various immune cells, such as DCs, NK cells, and T cells (56, 57). Specifically, AFP can reduce the antigen presentation ability of DCs, thereby preventing DC-mediated activation of T cells (58, 59). It was found that plasmacytoid DCs (pDCs, immunosuppressive DCs) are abundant and localized in type 1 Tregs and promote the production of IL-10 in the TME of HCC. AFP levels correlated with the high numbers of pDCs, tumor metastasis, and increased tumor infiltration of Tregs (2).Studies have confirmed that AFP isolated from human umbilical cord blood acts on monocytes in patients with liver cancer, inhibiting the conversion of monocytes into mature DCs. Since AFP can inhibit the maturation of DCs, it can promote tumor immune escape (29, 60).
Simultaneously, AFP can attenuate its cytotoxicity toward liver cancer cells by regulating signaling pathways in NK cells (54). AFP typically does not directly impair the function of NK cells; instead, it indirectly hampers their function by impeding the maturation of DCs and decreasing IL-12 secretion by DCs (54, 56). Furthermore, AFP also suppresses the proliferation and cytotoxicity of T cells, thereby attenuating their response to liver cancer cells. AFP can hinder the immune response by inducing lymphocyte apoptosis and modulating T lymphocyte differentiation into CD4+ T cells and CD8+ T cells. AFP promotes DC-mediated differentiation of regulatory T cells (Tregs) and dampens the function of CD8+T cells and NK cells, thus inducing an immunosuppressive environment and tumor progression (Figure 3). Despite the limited immunogenicity of AFP, it can provoke immune evasion by suppressing the function of DCs, NK cells, and T lymphocytes, forming an immunosuppressive microenvironment, thereby promoting tumor progression. Myeloid-derived suppressor cells (MDSCs) are a subset of immature myeloid cells originating from myeloid hematopoietic stem cells. These cells express CD11b, CD14, CD15, and CD33 antigens but do not differentiate into macrophages, DCs, or granulocytes. MDSCs possess AFP receptors on their cell membranes, which interact with AFP and inhibit the function of NK cells and T lymphocytes. Consequently, this interaction establishes a microenvironment conducive to immune evasion in cancer (30) (Figure 3).
Figure 3 DCs and MDSCs involved in AFP-induced immune escape. In the absence of AFP, DCs enhance the function of CD4+ T cells, CD8+ T cells, and NK lymphocytes, thereby impeding tumor growth. MDSCs activate T and NK lymphocytes, inhibiting tumorigenesis. In the presence of AFP, DCs promote Treg differentiation but suppress the function of CD8+ T cells and NK cells, consequently fostering an immunosuppressive milieu and facilitating tumor growth. Additionally, in the presence of AFP, MDSCs hinder the function of T cells and NK cells, thereby promoting tumorigenesis.
AFP diversely affects the immune evasion of HCC; for example, it inhibits immune cell activity, disrupts antigen presentation, and induces immune tolerance. These effects collectively enable liver cancer cells to evade immune surveillance, thereby augmenting their immune evasion. Therefore, investigating the mechanisms governing the interaction between AFP and immune evasion of liver cancer holds promise for the discovery of novel approaches for immunotherapy in HCC.
Cancer-associated fibroblasts (CAFs) are the most important cell population in the TME. They promote HCC growth and drug resistance. They mediate HCC progression by activating signaling pathways, such as the JAK/STAT, MAPK, and Wnt/β-catenin pathways (61). AFP also activates these signaling pathways to promote the malignant transformation of cancer cells. The interaction of AFP with CAFs is unclear, but AFP can promote HCC development in patients with liver fibrosis. The blood levels of secreted AFP can be used to accurately stratify patients with advanced liver fibrosis for their HCC risk and guide HCC screening (62). Eliminating serum lectin-reactive alpha-fetoprotein can suppress post-treatment recurrence of HCC in cirrhotic patients (63).
The liver is formed by hexagonally shaped anatomical units named ‘liver lobules’. Blood flows through sinusoidal channels of the liver lobules and drained into central veins. Sinusoidal endothelial cells form the wall of liver sinusoids which play critical roles in liver homeostasis (64). Liver Sinusoidal endothelial cells have multiple fenestrate that facilitate transferring substrates between blood and the extravascular compartment (65).
Sinusoidal endothelial cells play important roles in cancer development. In TME, sinusoidal endothelial cells become capillarized or defenestrated after exposure to inflammatory factors, such as IL-6 and AFP, which promote liver fibrosis, cirrhosis, and liver cancer. AFP is expressed around fibrotic bridges in chronic liver damage and at the margins of necrotic tissues. It promotes the recruitment and proliferation of endothelial cells, hepatic stellate cells (HSCs), and macrophages. This may imply AFP reprograms live cells and remodels the extracellular matrix to promote endothelial-to-mesenchymal transition and tumor angiogenesis (66, 67). It was found that ramucirumab, a monoclonal antibody specific for VEGFR2, combined with tyrosine kinase inhibitors regorafenib and cabozantinib, present specific benefits for advanced HCC patients with high serum concentrations of AFP (68).
Mesenchymal stem cells (MSCs) are multipotent cells initially discovered from bone marrow. MSCs can be differentiated into chondrocytes, osteocytes, adipocytes, myocytes, astrocytes, and other cells in vitro. MSCs can be recruited into the TME and promote or inhibit the development of HCC. The contradictory effects of MSCs mainly come from TME of HCC, and whether these cells are endogenous or exogenous. In TME, endogenous MSCs can promote hepatic fibrosis by regulating the inflammatory microenvironment. However, in clinical trials, exogenous MSCs were used to treat hepatic fibrosis (69, 70).
It was found that exogenous MSCs can suppress tumor growth in animal experiments. For example, co-cultured umbilical cord-derived MSCs with hepatocarcinoma cell line HepG2 downregulated the protein expression of AFP, Bcl-2, and survivin and accelerated cancer cell apoptosis, which was related to the apoptosis signal pathway. Thus, exogenous umbilical cord-derived MSCs can inhibit growth and promote the apoptosis of cancer cells by downregulating AFP, Bcl-2, and survivin (71).
Exogenous MSCs are a potential source of stem cells for cell therapy in treating liver cirrhosis due to their many advantages. MSCs are easy to obtain and easy to culture without losing their characteristics. Exogenous MSCs can be recruited into the injury sites and TME and have strong immunoregulatory abilities. In addition, they have multiple differentiation potential and capacity to repair injuries and regenerate (70). Several clinical trials have investigated the potential of MSCs in treating liver cirrhosis, particularly alcoholic liver cirrhosis (NCT02705742, NCT03626090, NCT05080465, NCT05227846, and NCT05155657). Satisfactory outcomes have been observed in clinical trials of exogenous MSCs. New strategies are needed to improve cell sources for recipients. In the future, synergistic downregulation of AFP with exogenous MSCs may improve their therapeutic efficacy in liver cirrhosis and HCC (70).
AFP is as a serological biomarker for HCC screening, diagnosis, treatment response monitoring, and prognosis. The sensitivity and specificity of AFP for diagnosing HCC are 41–65% and 80–94%, respectively. However, nearly 50% of patients with HCC are AFP-negative and AFP may be elevated in benign liver diseases, such as hepatitis and cirrhosis (72, 73).
AFP-negative and AFP-positive patients display differential proteomic profiles and metabolomic profiles. The symptoms of AFP-negative patients are generally mild and they benefit more from treatment compared with AFP-positive patients. AFP level has strong relationships with malignant features of HCC (72, 73). The analysis of tumor-infiltrating lymphocytes in AFP-positive TME reveals an enrichment of Tregs and exhausted CD8+ T cells. Accordingly, the number of cytotoxic T-lymphocyte associated protein 4 (CTLA4) and programmed death 1 (PD-1) T cells is higher in AFP-positive patients than in AFP-negative patients. Also, high abundances of regulatory T cells were found in AFP-positive patients, which drive cytotoxic T cell dysfunction in the TME (74).
TAMs, DCs, and monocytes were more abundant in the adjacent normal tissues of AFP-positive patients. AFP upregulates IL-6, transforming growth factor beta 1 (TGF-β1), CXCR4 and NF-κB, to recruit TAMs into TME. TAMs promote the expression of angiogenesis-associated genes and downregulate phagocytosis and lymphocyte response, which may recruit fibroblasts and induce extracellular matrix remodeling to promote tumorigenesis (75).
Elevated serum levels of AFP typically signify the existence or recurrence of HCC, thus rendering AFP a valuable tool for diagnosis and treatment (76).Some patients with HCC are AFP-negative preoperatively; however, AFP may increase after surgery. AFP can acutely elevate for about 5 days after partial hepatectomy (77). Rising concentrations of AFP are closely associated with hepatocyte regeneration. AFP can be synthesized and released by hepatocytes undergoing proliferation and mitosis after operation (78). AFP expression was also seen in the regenerative phase after liver injury, which was associated with hepatocyte proliferation. During proliferation, AFP is produced by hepatocytes and released into the microenvironment and circulation. It was also found that AFP is not only related to cell proliferation but also related to cellular death and apoptosis (52). After surgery, AFP level will decline with healing. However, in postoperative recurrence, AFP is reproduced by HCC cells and cancer stem cells. AFP production during postoperative recurrence is related to fast-growing, poorly differentiated, and malignant HCC cells (44). HCC is an extraordinarily heterogeneous malignant liver cancer. Preoperative and postoperative recurrence of HCC are different in molecular characteristics, signal transduction, and genomic instability. They are characterized by great heterogeneity. AFP can arise from cancer stem cells, which are silent in patients before surgery. These cells are more active in the TME during hepatocyte necrosis, myeloid cell migration, immune evasion, and matrix remodeling after postoperative recurrence (44, 79, 80). Thus, AFP not only functions as a marker but also aids in the interpretation of imaging in liver cancer. The value of AFP can be associated with the dimensions, progression, and prognosis of HCC. Elevated levels of AFP may serve as an indicator of a larger or more aggressive tumor and an unfavorable prognosis (81). Moreover, post-treatment monitoring of AFP levels in patients with liver cancer can be employed to assess treatment efficacy and disease recurrence. A substantial decrease in AFP level following treatment generally signifies successful intervention, whereas an increase in AFP level may suggest tumor relapse or progression (60). Thus, AFP plays a crucial role in the diagnosis and management of HCC. As a marker, it can be used for early screening, diagnosis, and follow-up of patients with liver cancer. In addition, AFP levels can also be used to evaluate the therapeutic effect and predict the prognosis.
Recently, AFP has increasingly gained interest in HCC immunotherapy. AFP can be applied in immunization. Particularly, it can be a marker for immunotherapy and predict the efficacy of immunotherapy. AFP is highly expressed in nearly 65% of patients with HCC, and serum AFP is as a biomarker for diagnosing and treating HCC because it is inversely correlated with prognosis (2, 82). HCC patients cannot tolerate to high serum levels of AFP which induce significant tumor burdens. AFP is a tumor-associated antigen, and cytotoxic T lymphocyte (CTL) epitopes for AFP were identified during tumorigenesis (83, 84). Patients with high circulating levels of AFP have strong T cell responses. Both murine and human T cells can recognize the self-antigen AFP, indicating that it can serve as a tumor rejection antigen. AFP-based CAR-T cells and AFP-based vaccines have shown an antitumor immune response in vivo and in clinical trials (85, 86).
AFP is produced intracellularly and secreted by HCC, making it “untargetable” for chimeric antigen receptor T (CAR-T) cell immunotherapy. However, peptides derived from AFP are processed and presented by class I MHC on the surface of HCC cells. Therefore, antibodies against AFP-derived peptides/MHC complexes can be designed and engineered into CAR-T cells. CAR-T cell therapy was successful in liver cancer xenograft models in vivo (85). Also, CD8+ T cells were found in transgenic mice that recognized AFP-derived peptide epitope on human HCC cells. Adoptive transfer of AFP-derived peptide-specific CD8+ T cells eradicated HepG2 tumor xenografts in mice (87).
Preclinical studies found that AFP-derived peptide-specific TCR-engineered T cells are unlikely to cause severe off-target toxicity (88). AFP peptide-based CAR-T therapy in advanced HCC is being tested in a phase I clinical trial (NCT03132792) to find the safety of adoptive transfer of engineered T cells, which target AFP-positive liver cancer (89).
Dysfunction of DCs is also the main mechanism of tumor immune escape. Vaccination can promote the activity of DCs and stimulate antitumor responses in HCC. The beneficial immune responses of CD8+ and CD4+ T cells can be generated if AFP is presented by more AFP-engineered DCs. It was found that AFP-pulsed DCs can shift specific cytotoxic T lymphocytes toward AFP-producing HCC cells (90, 91).
Using AFP-coding adenoviruses to transduce DCs can induce the antitumor immune response, delay tumor growth, and improve long-term survival in vivo subcutaneous HCCs. The combination of the AFP-DCs vaccine with CD40 ligand-DCs vaccine has shown synergistic results. It changes the TME, enhances the tumor infiltration of cytotoxic T lymphocytes and DCs, and upregulates Th1-derived cytokines, leading to tumor apoptosis and regression (92, 93). A phase I/II trial measuring immunization in HCC patients found that AFP-DCs vaccine can produce AFP-specific T cell responses with increasing IFN-γ in the environment of high oncofetal antigen (86). Nevertheless, more comprehensive studies are imperative to ascertain the efficacy and safety of these approaches and vaccines before their implementation in clinical settings.
TME is a complex ecosystem comprising tumor cells, blood vessels, immune cells, stromal cells, and matrix molecules. AFP and its downstream pathways play a key role in this system, making them targets for novel tumor treatment. The expression level of AFP is associated with tumorigenesis and tumor prognosis (94, 95). Hence, therapeutic strategies that target AFP or its downstream pathways may be beneficial for treating these tumors. One potential therapeutic approach is to inhibit the expression or activity of AFP. For instance, using small interfering RNA (siRNA) or antisense oligonucleotides (ASO) technology to reduce AFP expression. These technologies can specifically inhibit the synthesis of AFP or prevent its interaction with target cells, thereby suppressing tumor growth and diffusion (96, 97). Secondly, the use of recombinant AFP, combined with anticancer drugs, has been widely explored. Besides, recombinant AFP fragment derived from AFP domain-3 was found to suppress tumor cell growth. Various anticancer drugs exhibited therapeutic prospects when combined with AFP or AFP fragments, such as carminomycin, doxorubicin, paclitaxel, sorafenib, and maytansinoid (98–103). Moreover, AFP can activate downstream signaling pathways, such as PI3K/Akt in tumor cells, promoting proliferation, survival, and migration (22, 104). Targeting these pathways with small-molecule inhibitors or antibodies can inhibit tumor growth. The complexity of TME makes a single therapy ineffective. Combining multiple strategies, like targeting AFP and its downstream pathways with immunotherapy and radiotherapy, may more effectively control tumor growth and invasion.
In the TME, AFP-targeted therapies still face challenges and limitations. Firstly, the expression level of AFP has limited sensitivity and specificity for HCC patients, it is not a marker for all HCC, and about one-third of the patients with advanced HCC have no AFP expression (105, 106). Moreover, patients with acute and chronic liver without evidence of HCC may have high AFP elevation. Therefore, therapeutic strategies targeting AFP may not be applicable to all HCC patients. Secondly, the heterogeneity of the tumor microenvironment is another challenge. AFP expression varies among different types and stages of HCC (7, 105), and the accuracy value with advanced HCC varies according to patient characteristics (7). Due to the significant differences in cell types, gene expression, and signal pathway activation among different regions of a tumor (106). Drug resistance is another concern. Tumor cells may adapt to treatment pressure by activating other signaling pathways or mutations, leading to treatment failure (107). Overall, there are challenges in AFP-targeted therapies, and future studies are needed to understand the mechanism by which AFP is involved in tumorigenesis and assess the efficacy and safety of AFP-based treatment strategies.
AFP utility is limited and challenges, however, targeting the AFP-TME interaction in HCC treatment has significant benefits to AFP positive patients (75). Blocking the function of AFP or inhibiting its interaction with TME can disrupt the connection between liver cancer cells and TME, thereby inhibiting tumor growth and diffusion. This strategy may provide a new approach for treating HCC. Secondly, AFP is also involved in the interaction between liver cancer cells and the immune microenvironment (29). AFP overexpression can promote the immune evasion of HCC cells, protecting them from the immune response. Targeting AFP may help attenuate this immune evasion, enhancing the immune recognition and response, thereby improving the efficacy of immunotherapy. In summary, targeting the AFP-TME interaction in HCC can disrupt the link between liver cancer cells and the TME, inhibiting tumor growth, invasion, and immune evasion.
In conclusion, AFP holds significant value as an HCC biomarker, and its high expression is intricately linked to the development and progression of HCC. AFP can stimulate the expansion of LCSCs in the TME by activating the PI3K/Akt signaling pathway. Additionally, AFP can facilitate HCC progression by upregulating the expression of genes associated with LCSCs. Furthermore, AFP can undermine macrophage-mediated phagocytosis of tumor cells by interacting with macrophage receptors and inducing tumor immune escape. Simultaneously, AFP may also suppress the function of NK cells, macrophages, DCs, CAFs, endothelial cells, and mesenchymal stem cells, and impair the ability of cytotoxic T lymphocytes to eliminate tumor cells, thus facilitating tumor immune evasion (Figure 4). Recent findings have demonstrated that AFP inhibition can effectively inhibit the malignant behaviors of HCC cells, suppressing their proliferation, invasion, and metastasis and inducing cancer cell apoptosis. Consequently, AFP plays a crucial role in facilitating the malignant progression of HCC. Nevertheless, AFP is a double-edged sword in the development, diagnosis, and treatment of HCC (Figure 5). For clinical practice, measuring AFP levels holds immense significance for the early detection, prognosis, and treatment of HCC. By monitoring the fluctuations in AFP levels, medical professionals can monitor disease progression and treatment response, guiding clinical decision-making. Furthermore, clinicians can use AFP vaccines to generate AFP-specific CD8+ T cells and kill cancer cells. In addition, AFP combined with immunotherapy, can improve the therapeutic efficacy.
Figure 5 A double-edged sword of AFP. AFP is a double-edged sword due to its role as both an oncofetal antigen and a diagnostic marker for HCC. Its participation in various aspects of tumor biology, including proliferation, invasion, metastasis, apoptosis, and TME regulation, underscores its potential detrimental effects. Conversely, measuring AFP levels can help early diagnosis, prognostic evaluation, monitoring of treatment efficacy, and advancement of therapeutic interventions in HCC. Additionally, AFP can serve as a valuable target for developing drugs and vaccines (by Figdraw 2.0).
In summary, AFP plays a significant role in the TME of HCC. It modulates the function of tumor-associated macrophages, increases the population of tumor stem cells, and facilitates the immune evasion of liver cancer cells. Further studies are needed to gain a comprehensive understanding of the underlying mechanisms through which AFP is involved in the TME of HCC and provide new approaches for treatment and prognosis prediction in HCC.
YL: Data curation, Resources, Supervision, Writing – original draft, Writing – review & editing. BL: Supervision, Writing – original draft, Writing – review & editing. ML: Funding acquisition, Investigation, Supervision, Writing – review & editing.
The author(s) declare financial support was received for the research, authorship, and/or publication of this article. This work was supported by The Natural Science Foundation of Hainan Province (No. 822QN311), Hainan Provincial Science and Technology Special Fund (No.ZDYF2021SHFZ222 and No.ZDYF2024SHFZ118), Hainan Medical College Talent Launch Fund (No. XRC220017), the National Natural Science Foundation of China (Nos. 82060514 and 81960519), and the Research Project of Take off the Proclamation and Leadership of the Hainan Medical College Natural Science Foundation (No. JBGS202106).
The authors declare that the research was conducted in the absence of any commercial or financial relationships that could be construed as a potential conflict of interest.
All claims expressed in this article are solely those of the authors and do not necessarily represent those of their affiliated organizations, or those of the publisher, the editors and the reviewers. Any product that may be evaluated in this article, or claim that may be made by its manufacturer, is not guaranteed or endorsed by the publisher.
1. Rumgay H, Arnold M, Ferlay J, Lesi O, Cabasag CJ, Vignat J, et al. Global burden of primary liver cancer in 2020 and predictions to 2040. J Hepatol. (2022) 77:1598–606. doi: 10.1016/j.jhep.2022.08.021
2. Donne R, Lujambio A. The liver cancer immune microenvironment: Therapeutic implications for hepatocellular carcinoma. Hepatology. (2023) 77:1773–96. doi: 10.1002/hep.32740
3. Yin Z, Li X. Immunotherapy for hepatocellular carcinoma. Cancer Lett. (2020) 470:8–17. doi: 10.1016/j.canlet.2019.12.002
4. Vogel A, Meyer T, Sapisochin G, Salem R, Saborowski A. Hepatocellular carcinoma. Lancet. (2022) 400:1345–62. doi: 10.1016/S0140-6736(22)01200-4
5. Sidali S, Trépo E, Sutter O, Nault JC. New concepts in the treatment of hepatocellular carcinoma. United Eur Gastroenterol J. (2022) 10:765–74. doi: 10.1002/ueg2.12286
6. Johnson P, Zhou Q, Dao DY, Lo YMD. Circulating biomarkers in the diagnosis and management of hepatocellular carcinoma. Nat Rev Gastroenterol Hepatol. (2022) 19:670–81. doi: 10.1038/s41575-022-00620-y
7. Hanif H, Ali MJ, Susheela AT, Khan IW, Luna-Cuadros MA, Khan MM, et al. Update on the applications and limitations of alpha-fetoprotein for hepatocellular carcinoma. World J Gastroenterol. (2022) 28:216–29. doi: 10.3748/wjg.v28.i2.216
8. Mizejewski G. The phylogeny of alpha-fetoprotein in vertebrates: Survey of biochemical and physiological data. Crit Rev Eukaryot Gene Expr. (1995) 5:281–316. doi: 10.1615/CritRevEukarGeneExpr.v5.i3-4
9. Li W, Liu K, Chen Y, Zhu M, Li M. Role of alpha-fetoprotein in hepatocellular carcinoma drug resistance. Curr Med Chem. (2021) 28:1126–42. doi: 10.2174/0929867327999200729151247
10. Hu X, Chen R, Wei Q, Xu X. The landscape of alpha fetoprotein in hepatocellular carcinoma: where are we? Int J Biol Sci. (2022) 18:536–51. doi: 10.7150/ijbs.64537
11. Zheng Y, Zhu M, Li M. Effects of alpha-fetoprotein on the occurrence and progression of hepatocellular carcinoma. J Cancer Res Clin Oncol. (2020) 146:2439–46. doi: 10.1007/s00432-020-03331-6
12. Wang YQ, Wang AJ, Zhang TT, Chen SH. Association of alpha-fetoprotein and metastasis for small hepatocellular carcinoma: A propensity-matched analysis. Sci Rep. (2022) 12:15676. doi: 10.1038/s41598-022-19531-8
13. Xiao Y, Yu D. Tumor microenvironment as a therapeutic target in cancer. Pharmacol Ther. (2021) 221:107753. doi: 10.1016/j.pharmthera.2020.107753
14. Bader J, Voss K, Rathmell J. Targeting metabolism to improve the tumor microenvironment for cancer immunotherapy. Mol Cell. (2020) 78:1019–33. doi: 10.1016/j.molcel.2020.05.034
15. Jin M, Jin W. The updated landscape of tumor microenvironment and drug repurposing. Signal Transduct Target Ther. (2020) 5:166. doi: 10.1038/s41392-020-00280-x
16. Visser K, Joyce J. The evolving tumor microenvironment: From cancer initiation to metastatic outgrowth. Cancer Cell. (2023) 41:374–403. doi: 10.1016/j.ccell.2023.02.016
17. French SW. Epigenetic events in liver cancer resulting from alcoholic liver disease. Alcohol Res. (2013) 35:57–67.
18. Grewal P, Viswanathen VA. Liver cancer and alcohol. Clin Liver Dis. (2012) 16:839–50. doi: 10.1016/j.cld.2012.08.011
19. Duggan AE, Duggan JM. Alcoholic liver disease-assessment and management. Aust Fam Physician. (2011) 40:590–3.
20. Yang P, Markowitz GJ, Wang XF. The hepatitis B virus-associated tumor microenvironment in hepatocellular carcinoma. Natl Sci Rev. (2014) 1:396–412. doi: 10.1093/nsr/nwu038
21. Lim CJ, Lee YH, Pan L, Lai L, Chua C, Wasser M, et al. Multidimensional analyses reveal distinct immune microenvironment in hepatitis B virus-related hepatocellular carcinoma. Gut. (2019) 68:916–27. doi: 10.1136/gutjnl-2018-316510
22. Zhu M, Li W, Lu Y, Dong X, Lin B, Chen Y, et al. HBx drives alpha fetoprotein expression to promote initiation of liver cancer stem cells through activating PI3K/AKT signal pathway. Int J Cancer. (2017) 140:1346–55. doi: 10.1002/ijc.30553
23. Xuan SY, Xin YN, Chen H, Shi GJ, Guan HS, Li Y. Significance of hepatitis B virus surface antigen, hepatitis C virus expression in hepatocellular carcinoma and pericarcinomatous tissues. World J Gastroenterol. (2007) 13:1870–4. doi: 10.3748/wjg.v13.i12.1870
24. Khatun M, Ray R, Ray RB. Hepatitis C virus associated hepatocellular carcinoma. Adv Cancer Res. (2021) 149:103–42. doi: 10.1016/bs.acr.2020.10.003
25. Zhu M, Lin B, Zhou P, Li M. Molecular analysis of AFP and HSA interactions with PTEN protein. BioMed Res Int. (2015), 256916. doi: 10.1155/2015/256916
26. Tomasi TB. Structure and function of alpha-fetoprotein. Annu Rev Med. (1977) 28:453–65. doi: 10.1146/annurev.me.28.020177.002321
27. Mizejewski GJ. Alpha-fetoprotein structure and function: relevance to isoforms, epitopes, and conformational variants. Exp Biol Med. (2001) 226:377–408. doi: 10.1177/153537020122600503
28. Wang SS, Li H, Li G. Molecular structure and biological function of alpha-fetoprotein. World J Chin Digestion. (2014) 22:1487–94. doi: 10.11569/wcjd.v22.i11.1487
29. Munson PV, Adamik J, Butterfield LH. Immunomodulatory impact of α-fetoprotein. Trends Immunol. (2022) 43:438–48. doi: 10.1016/j.it.2022.04.001
30. Głowska-Ciemny J, Szymański M, Kuszerska A, Malewski Z, von Kaisenberg C, Kocyłowski R. The role of alpha-fetoprotein (AFP) in contemporary oncology: the path from a diagnostic biomarker to an anticancer drug. Int J Mol Sci. (2023) 24:2539. doi: 10.3390/ijms24032539
31. Lin B, Liu K, Wang W, Li W, Dong X, Chen Y, et al. Expression and bioactivity of human α-fetoprotein in a Bac-to-Bac system. Biosci Rep. (2017) 37:BSR20160161. doi: 10.1042/BSR20160161
32. Egashira Y, Suganuma M, Kataoka Y, Higa Y, Ide N, Morishita K, et al. Establishment and characterization of a fucosylated α-fetoprotein-specific monoclonal antibody: a potential application for clinical research. Sci Rep. (2019) 9:12359. doi: 10.1038/s41598-019-48821-x
33. Santos PM, Menk AV, Shi J, Tsung A, Delgoffe GM, Butterfield LH. Tumor-derived α-fetoprotein suppresses fattyAcid metabolism and oxidative phosphorylation in dendritic cells. Cancer Immunol Res. (2019) 7:1001–12. doi: 10.1158/2326-6066.CIR-18-0513
34. Hinshaw DC, Shevde LA. The tumor microenvironment innately modulates cancer progression. Cancer Res. (2019) 79:4557–66. doi: 10.1158/0008-5472.CAN-18-3962
35. Wu T, Dai Y. Tumor microenvironment and therapeutic response. Cancer Lett. (2017) 387:61–8. doi: 10.1016/j.canlet.2016.01.043
36. Henkin RI. Clinical and therapeutic implications of cancer stem cells. N Engl J Med. (2019) 381:e19. doi: 10.1056/NEJMc1908886
37. Sun JH, Luo Q, Liu LL, Song GB. Liver cancer stem cell markers: progression and therapeutic implications. World J Gastroenterol. (2016) 22:3547–57. doi: 10.3748/wjg.v22.i13.3547
38. Cheng Z, Li X, Ding J. Characteristics of liver cancer stem cells and clinical correlations. Cancer Lett. (2016) 379:230–8. doi: 10.1016/j.canlet.2015.07.041
39. Xu Y, Guo Q, Wei L. The emerging influences of alpha-fetoprotein in the tumorigenesis and progression of hepatocellular carcinoma. Cancers (Basel). (2021) 13:5096. doi: 10.3390/cancers13205096
40. Jeng KS, Chang CF, Sheen IS, Jeng CJ, Wang CH. Cellular and molecular biology of cancer stem cells of hepatocellular carcinoma. Int J Mol Sci. (2023) 24:1417. doi: 10.3390/ijms24021417
41. Lu Y, Zhu M, Li W, Lin B, Dong X, Chen Y, et al. Alpha fetoprotein plays a critical role in promoting metastasis of hepatocellular carcinoma cells. J Cell Mol Med. (2016) 20:549–58. doi: 10.1111/jcmm.12745
42. Mansour AH, Elkhodary TR, Anwar RS, Habeeb MR, Mohammed MA. Regulation of cancer stem cell marker (CD133) by transforming growth factor beta in hepatocellular carcinoma. Int J Cancer Res. (2014) 10:65–73. doi: 10.1002/hep.23544
43. Ashokachakkaravarthy K, Pottakkat B. Mitotic quiescence in hepatic cancer stem cells: An incognito mode. Oncol Rev. (2020) 14:452. doi: 10.4081/oncol.2020.452
44. Sell S. Alpha-fetoprotein, stem cells and cancer: how study of the production of alpha-fetoprotein during chemical hepatocarcinogenesis led to reaffirmation of the stem cell theory of cancer. Tumour Biol. (2008) 29:161–80. doi: 10.1159/000143402
45. Boutilier AJ, Elsawa SF. Macrophage polarization states in the tumor microenvironment. Int J Mol Sci. (2021) 22:6995. doi: 10.3390/ijms22136995
46. Cassetta L, Pollard JW. A timeline of tumour-associated macrophage biology. Nat Rev Cancer. (2023) 23:238–57. doi: 10.1038/s41568-022-00547-1
47. Locati M, Mantovani A, Sica A. Macrophage activation and polarization as an adaptive component of innate immunity. Adv Immunol. (2013) 120:163–84. doi: 10.1016/B978-0-12-417028-5.00006-5
48. Murray PJ, Wynn TA. Protective and pathogenic functions of macrophage subsets. Nat Rev Immunol. (2011) 11:723–37. doi: 10.1038/nri3073
49. Ruffell B, Affara NI, Coussens LM. Differential macrophage programming in the tumor microenvironment. Trends Immunol. (2012) 33:119–26. doi: 10.1016/j.it.2011.12.001
50. Goswami KK, Ghosh T, Ghosh S, Sarkar M, Bose A, Baral R. Tumor promoting role of anti-tumor macrophages in tumor microenvironment. Cell Immunol. (2017) 316:1–10. doi: 10.1016/j.cellimm.2017.04.005
51. Singh S, Mehta N, Lilan J, Budhthoki MB, Chao F, Yong L. Initiative action of tumor-associated macrophage during tumor metastasis. Biochim Open. (2017) 4:8–18. doi: 10.1016/j.biopen.2016.11.002
52. Doak GR, Schwertfeger KL, Wood DK. Distant relations: macrophage functions in the metastatic niche. Trends Cancer. (2018) 4:445–59. doi: 10.1016/j.trecan.2018.03.011
53. Zhang X, Wei Z, Yong T, Li S, Bie N, Li J, et al. Cell microparticles loaded with tumor antigen and resiquimod reprogram tumor-associated macrophages and promote stem-like CD8+ T cells to boost anti-PD-1 therapy. Nat Commun. (2023) 14(1):5653. doi: 10.1038/s41467-023-41438-9
54. Lu CY, Changelian PS, Unanue ER. Alpha-fetoprotien inhibits macrophage expression of ia antigens. J Immunol. (1984) 132:1722–7. doi: 10.4049/jimmunol.132.4.1722
55. Zhang M, Liu K, Zhang Q, Xu J, Liu J, Lin H, et al. Alpha fetoprotein promotes polarization of macrophages towards M2-like phenotype and inhibits macrophages to phagocytize hepatoma cells. Front Immunol. (2023) 14:1081572. doi: 10.3389/fimmu.2023.1081572
56. Murgita RA, Goidl EA, Kontiainen S, WigzellI H. Alpha-Fetoprotein induces suppressor T cells in vitro. Nature. (1977) 267:257–9. doi: 10.1038/267257a0
57. Li MS, Li PF, Chen Q, Du GG, Li G. Alphafetoprotein stimulated the expression of some oncogenes in human hepatocellular carcinoma Bel 7402 cells. World J Gastroenterol. (2004) 10:819–24. doi: 10.3748/wjg.v10.i6.819
58. Wang X, Wang Q. Alpha-fetoprotein and hepatocellular carcinoma immunity. Can J Gastroenterol Hepatol. (2018) 2018:9049252. doi: 10.1155/2018/9049252
59. Um SH, Mulhall C, Alisa A, Ives AR, Karani J, Williams R, et al. Alpha-fetoprotein impairs APC function and induces their apoptosis. J Immunol. (2004) 173:1772–8. doi: 10.4049/jimmunol.173.3.1772
60. Kim HI, Lim J, Shim JH. Role of the alpha-fetoprotein response in immune checkpoint inhibitor-based treatment of patients with hepatocellular carcinoma. J Cancer Res Clin Oncol. (2022) 148:2069–77. doi: 10.1007/s00432-021-03727-y
61. Akkız H. Emerging role of cancer-associated fibroblasts in progression and treatment of hepatocellular carcinoma. Int J Mol Sci. (2023) 24:3941. doi: 10.3390/ijms24043941
62. Fujiwara N, Kobayashi M, Fobar AJ, Hoshida A, Marquez CA, Koneru B, et al. A blood-based prognostic liver secretome signature and long-term hepatocellular carcinoma risk in advanced liver fibrosis. Med. (2021) 2:836–850.e10. doi: 10.1016/j.medj.2021.03.017
63. Okuno M, Kojima S, Akita K, Matsushima-Nishiwaki R, Adachi S, Sano T, et al. Retinoids in liver fibrosis and cancer. Front Biosci. (2002) 7:d204–18. doi: 10.2741/A775
64. Yang M, Zhang C. The role of liver sinusoidal endothelial cells in cancer liver metastasis. Am J Cancer Res. (2021) 11:1845–60.
65. Cogger VC, McNerney GP, Nyunt T, DeLeve LD, McCourt P, Smedsrød B, et al. Three-dimensional structured illumination microscopy of liver sinusoidal endothelial cell fenestrations. J Struct Biol. (2010) 171:382–8. doi: 10.1016/j.jsb.2010.06.001
66. Velliou RI, Legaki AI, Nikolakopoulou P, Vlachogiannis NI, Chatzigeorgiou A. Liver endothelial cells in NAFLD and transition to NASH and HCC. Cell Mol Life Sci. (2023) 80:314. doi: 10.1007/s00018-023-04966-7
67. Iwai M, Morikawa T, Muramatsu A, Tanaka S, Mori T, Harada Y, et al. Biological significance of AFP expression in liver injury induced by CCL4. Acta Histochem Cytochem. (2001) 33:17–22. doi: 10.1267/ahc.33.17
68. Zhu AX, Kang YK, Yen CJ, Finn RS, Galle PR, Llovet JM, et al. Ramucirumab after sorafenib in patients with advanced hepatocellular carcinoma and increased alpha-fetoprotein concentrations (REACH-2): a randomised, double-blind, placebo-controlled, phase 3 trial. Lancet Oncol. (2019) 20:282–96. doi: 10.1016/S1470-2045(18)30937-9
69. Yang X, Li Q, Liu W, Zong C, Wei L, Shi Y, et al. Mesenchymal stromal cells in hepatic fibrosis/cirrhosis: from pathogenesis to treatment. Cell Mol Immunol. (2023) 20:583–99. doi: 10.1038/s41423-023-00983-5
70. Zhang X, Li N, Zhu Y, Wen W. The role of mesenchymal stem cells in the occurrence, development, and therapy of hepatocellular carcinoma. Cancer Med. (2022) 11:931–43. doi: 10.1002/cam4.4521
71. Tang YM, Bao WM, Yang JH, Ma LK, Yang J, Xu Y, et al. Umbilical cord-derived mesenchymal stem cells inhibit growth and promote apoptosis of HepG2 cells. Mol Med Rep. (2016) 14:2717–24. doi: 10.3892/mmr.2016.5537
72. Sun J, Zhao Y, Qin L, Li K, Zhao Y, Sun H, et al. Metabolomic profiles for HBV related hepatocellular carcinoma including alpha-fetoproteins positive and negative subtypes. Front Oncol. (2019) 9:1069. doi: 10.3389/fonc.2019.01069
73. Luo P, Wu S, Yu Y, Ming X, Li S, Zuo X, et al. Current status and perspective biomarkers in AFP negative HCC: towards screening for and diagnosing hepatocellular carcinoma at an earlier stage. Pathol Oncol Res. (2020) 26:599–603. doi: 10.1007/s12253-019-00585-5
74. Granito A, Muratori L, Lalanne C, Quarneti C, Guidi M, Lenzi M, et al. Hepatocellular carcinoma in viral and autoimmune liver diseases: Role of CD4+ CD25+ Foxp3+ regulatory T cells in the immune microenvironment. World J Gastroenterol. (2021) 27:2994–3009. doi: 10.3748/wjg.v27.i22.2994
75. Lurje I, Hammerich L. The suppressive TME of AFP-positive hepatocellular carcinoma and its therapeutic implications. Transl Gastroenterol Hepatol. (2023) 22:9:1. doi: 10.21037/tgh-23-81
76. Glowska-Ciemny J, Pankiewicz J, Malewski Z, von Kaisenberg C, Kocylowski R. Alpha-fetoprotein (AFP)-New aspects of a well-known marker in perinatology. Ginekol Pol. (2022) 93:70–5. doi: 10.5603/GP.a2021.0226
77. Sell S, Nichols M, Becker FF, Leffert JL. Hepatocyte proliferation and alpha1-fetoprotein in pregnant, neonatal and partially hepatectomized rets. Cancer Res. (1974) 34:865–71.
78. Sell S. Heterogeneity of Alpha-fetoprotein (AFP) and albumin containing cells in normal and pathological permissive states for AFP production: AFP containing cells induced in adult rats recapitulate the appearance of AFP containing hepatocytes in fetal rats. Oncodev Biol Med. (1980) 1:93–105.
79. Li L, Wang H. Heterogeneity of liver cancer and personalized therapy. Cancer Lett. (2016) 379:191–7. doi: 10.1016/j.canlet.2015.07.018
80. Lazarevich NL. Molecular mechanisms of alpha-fetoprotein gene expression. Biochem (Mosc). (2000) 65:117–33.
81. Ridder DA, Weinmann A, Schindeldecker M, Urbansky LL, Berndt K, Gerber TS, et al. Comprehensive clinicopathologic study of alpha fetoprotein-expression in a large cohort of patients with hepatocellular carcinoma. Int J Cancer. (2022) 150:1053–66. doi: 10.1002/ijc.33898
82. Johnson PJ. Role of alpha-fetoprotein in the diagnosis and management of hepatocellular carcinoma. J Gastroenterol Hepatol. (1999) Suppl:S32–6. doi: 10.1046/j.1440-1746.1999.01873.x
83. Butterfield LH, Koh A, Meng W, Vollmer CM, Ribas A, Dissette V, et al. Generation of human T-cell responses to an HLA-A2.1-restricted peptide epitope derived from alpha-fetoprotein. Cancer Res. (1999) 59:3134–42.
84. Thimme R, Neagu M, Boettler T, Neumann-Haefelin C, Kersting N, Geissler M, et al. Comprehensive analysis of the alpha-fetoprotein-specific CD8+ T cell responses in patients with hepatocellular carcinoma. Hepatology. (2008) 48:1821–33. doi: 10.1002/hep.22535
85. Liu H, Xu Y, Xiang J, Long L, Green S, Yang Z, et al. Targeting alpha-fetoprotein (AFP)-MHC complex. with CAR T-cell therapy for liver cancer. Clin Cancer Res. (2017) 23:478–88. doi: 10.1158/1078-0432.CCR-16-1203
86. Butterfield LH, Ribas A, Dissette VB, Lee Y, Yang JQ, de la Rocha P, et al. A phase I/II trial testing immunization of hepatocellular carcinoma patients with dendritic cells pulsed with four alpha-fetoprotein peptides. Clin Cancer Res. (2006) 12:2817–25. doi: 10.1158/1078-0432.CCR-05-2856
87. Zhu W, Peng Y, Wang L, Hong Y, Jiang X, Li Q, et al. Identification of α-fetoprotein-specific T-cell receptors for hepatocellular carcinoma immunotherapy. Hepatology. (2018) 68:574–89. doi: 10.1002/hep.29844
88. Cai L, Caraballo Galva LD, Peng Y, Luo X, Zhu W, Yao Y, et al. Preclinical studies of the offTarget. Reactivity of AFP(158)-specific TCR engineered T cells. Front Immunol. (2020) 11:607. doi: 10.3389/fimmu.2020.00607
89. Docta RY, Ferronha T, Sanderson JP, Weissensteiner T, Pope GR, Bennett AD, et al. Tuning T-cell receptor affinity to optimize clinical risk-benefit when targeting alpha-fetoprotein-positive liver cancer. Hepatology. (2019) 69:2061–75. doi: 10.1002/hep.30477
90. He Y, Hong Y, Mizejewski GJ. Engineering alpha-fetoprotein-based gene vaccines to prevent and treat hepatocellular carcinoma: review and future prospects. Immunotherapy. (2014) 6:725–36. doi: 10.2217/imt.14.46
91. González-Carmona MA, Märten A, Hoffmann P, Schneider C, Sievers E, Schmidt-Wolf IG, et al. Patient-derived dendritic cells transduced with an a-fetoprotein-encoding adenovirus and co-cultured with autologous cytokine-induced lymphocytes induce a specific and strong immune response against hepatocellular carcinoma cells. Liver Int. (2006) 26:369–79. doi: 10.1111/j.1478-3231.2005.01235.x
92. Butterfield LH, Ribas A, Potter DM, Economou JS. Spontaneous and vaccine induced AFP-specific T cell phenotypes in subjects with AFP-positive hepatocellular cancer. Cancer Immunol Immunother. (2007) 56:1931–43. doi: 10.1007/s00262-007-0337-9
93. Vogt A, Sadeghlar F, Ayub TH, Schneider C, Möhring C, Zhou T, et al. Alpha-fetoprotein- and CD40Ligand-expressing dendritic cells for immunotherapy of hepatocellular carcinoma. Cancers (Basel). (2021) 13:3375. doi: 10.3390/cancers13133375
94. He R, Yang Q, Dong X, Wang Y, Zhang W, Shen L, et al. Clinicopathologic and prognostic characteristics of alpha-fetoprotein-producing gastric cancer. Oncotarget. (2017) 8:23817–30. doi: 10.18632/oncotarget.v8i14
95. Moncada V, Srivastava S. Biomarkers in oncology research and treatment: early detection research network: a collaborative approach. biomark Med. (2008) 2:181–95. doi: 10.2217/17520363.2.2.181
96. Punuch K, Wongwan C, Jantana S, Somboonyosdech C, Rodponthukwaji K, Kunwong N, et al. Study of siRNA Delivery via Polymeric Nanoparticles in Combination with Angiogenesis Inhibitor for The Treatment of AFP-Related Liver Cancer. Int J Mol Sci. (2022) 23:12666. doi: 10.3390/ijms232012666
97. Rodponthukwaji K, Pingrajai P, Jantana S, Taya S, Duangchan K, Nguyen KT, et al. Epigallocatechin gallate potentiates the anticancer effect of AFP-siRNA-loaded polymeric nanoparticles on hepatocellular carcinoma cells. Nanomaterials (Basel). (2023) 14:47. doi: 10.3390/nano14010047
98. Griffin P, Hill WA, Rossi F, Boohaker R, Stinson K, Sherman I. High anti-tumor activity of a novel alpha-fetoprotein-maytansinoid conjugate targeting alpha-fetoprotein receptors in colorectal cancer xenograft model. Cancer Cell Int. (2023) 23:60. doi: 10.1186/s12935-023-02910-0
99. Lutsenko SV, Feldman NB, Finakova GV, Gukasova NV, Petukhov SP, Posypanova GA, et al. Antitumor activity of alpha fetoprotein and epidermal growth factor conjugates in vitro and in vivo. Tumour Biol. (2000) 21:367–74. doi: 10.1159/000030142
100. Sotnichenko AI, Severin SE, Posypanova GA, Feldman NB, Grigor’ev MI, Severin ES, et al. Water-soluble 2,3,7,8-tetrachlorodibenzo-p-dioxin complex with human alpha-fetoprotein: Properties, toxicity in vivo and antitumor activity in vitro. FEBS Lett. (1999) 450:49–51. doi: 10.1016/S0014-5793(99)00440-8
101. Moskaleva E, Posypanova GA, Shmyrev II, Rodina AV, Muizhnek EL, Severin ES, et al. Alpha-fetoprotein-mediated targeting-a new strategy to overcome multidrug resistance of tumour cells in vitro. Cell Biol Int. (1997) 21:793–9. doi: 10.1006/cbir.1998.0201
102. Posypanova GA, Makarov VA, Savvateeva MV, Bereznikova AV, Severin ES. The receptor binding fragment of alpha-fetoprotein is a promising new vector for the selective delivery of antineoplastic agents. J Drug Targeting. (2013) 21:458–65. doi: 10.3109/1061186X.2013.765441
103. Wang Q, Li W, Zhang M, Zou Z, Dong X, Chen Y, et al. α-Fetoprotein fragment synergizes with sorafenib to inhibit hepatoma cell growth and migration and promote the apoptosis. J Cell Mol Med. (2022) 26:5426–38. doi: 10.1111/jcmm.17565
104. Gao R, Cai C, Gan J, Yang X, Shuang Z, Liu M, et al. miR-1236 down-regulates alpha-fetoprotein, thus causing PTEN accumulation, which inhibits the PI3K/Akt pathway and Malignant phenotype in hepatoma cells. Oncotarget. (2015) 6:6014–28. doi: 10.18632/oncotarget.v6i8
105. Chon YE, Choi GH, Lee MH, Kim SU, Kim DY, Ahn SH, et al. Combined measurement of preoperative α-fetoprotein and des-γ-carboxy prothrombin predicts recurrence after curative resection in patients with hepatitis-B-related hepatocellular carcinoma. Int J Cancer. (2012) 131:2332–41. doi: 10.1002/ijc.27507
106. Salazar J, Le A. The heterogeneity of liver cancer metabolism. Adv Exp Med Biol. (2021) 1311:127–36. doi: 10.1007/978-3-030-65768-0_9
Keywords: hepatocellular carcinoma, AFP, tumor microenvironment, immune escape, liver cancer stem cells, immunotherapy
Citation: Lu Y, Lin B and Li M (2024) The role of alpha-fetoprotein in the tumor microenvironment of hepatocellular carcinoma. Front. Oncol. 14:1363695. doi: 10.3389/fonc.2024.1363695
Received: 31 December 2023; Accepted: 28 March 2024;
Published: 10 April 2024.
Edited by:
Noha Mousaad Elemam, University of Sharjah, United Arab EmiratesReviewed by:
Reem Amr Assal, Heliopolis University for Sustainable Development, EgyptCopyright © 2024 Lu, Lin and Li. This is an open-access article distributed under the terms of the Creative Commons Attribution License (CC BY). The use, distribution or reproduction in other forums is permitted, provided the original author(s) and the copyright owner(s) are credited and that the original publication in this journal is cited, in accordance with accepted academic practice. No use, distribution or reproduction is permitted which does not comply with these terms.
*Correspondence: Mengsen Li, bWVuZ3NlbmxpQDE2My5jb20=; Bo Lin, bGluYm9fNzUyQDE2My5jb20=
Disclaimer: All claims expressed in this article are solely those of the authors and do not necessarily represent those of their affiliated organizations, or those of the publisher, the editors and the reviewers. Any product that may be evaluated in this article or claim that may be made by its manufacturer is not guaranteed or endorsed by the publisher.
Research integrity at Frontiers
Learn more about the work of our research integrity team to safeguard the quality of each article we publish.