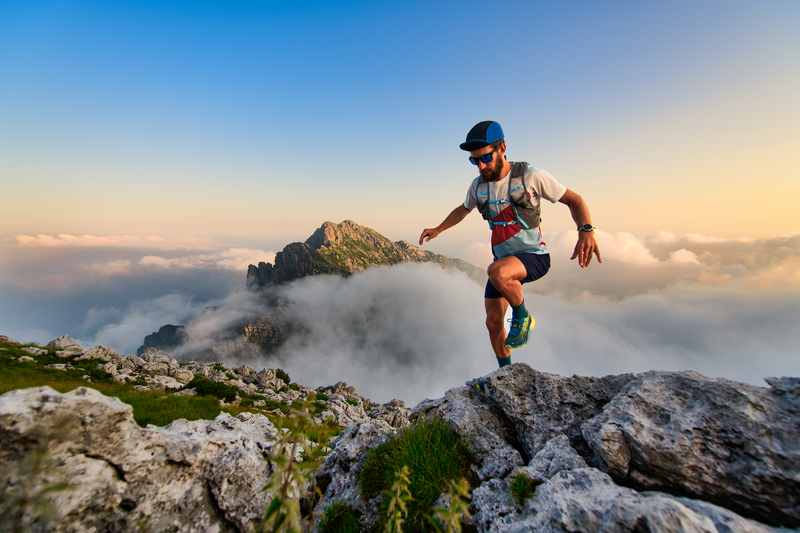
95% of researchers rate our articles as excellent or good
Learn more about the work of our research integrity team to safeguard the quality of each article we publish.
Find out more
SYSTEMATIC REVIEW article
Front. Oncol. , 22 February 2024
Sec. Head and Neck Cancer
Volume 14 - 2024 | https://doi.org/10.3389/fonc.2024.1358854
This scoping review identifies the mechanistic pathways of metformin when used to treat head and neck cancer cells, in the pre-clinical setting. Understanding the underlying mechanisms will inform future experimental designs exploring metformin as a potential adjuvant for head and neck cancer. This scoping review was conducted according to the Joanna-Briggs Institute framework. A structured search identified 1288 studies, of which 52 studies fulfilled the eligibility screen. The studies are presented in themes addressing hallmarks of cancer. Most of the studies demonstrated encouraging anti-proliferative effects in vitro and reduced tumor weight and volume in animal models. However, a few studies have cautioned the use of metformin which supported cancer cell growth under certain conditions.
Head and neck squamous cell carcinoma (HNSCC) is the 6th most commonly diagnosed cancer worldwide (1), with an overall five year survival of only 72% (2). The key risk factors for developing HNSCC are consumption of carcinogens (tobacco, betel quid, alcohol) and the oncogenic human papillomavirus (HPV) (3). The management of HNSCC involves a multidisciplinary approach comprising surgical resection, radiotherapy and chemotherapy (3). During the last few decades, there has been little advancement in the chemotherapeutic options available (4).
HNSCC and its treatment(s) have significant consequences on patients’ daily functions and quality of life. Despite recent advances in modern medicine, there remains a high risk of local recurrence and distant metastases (5). Treatment options for local recurrences are generally limited to salvage surgery with minimal improvement in overall survival rates (6, 7). There is an urgent need for novel solutions to improve treatment opportunities for patients with head and neck cancer.
Drug repurposing is the use of currently approved drugs for an alternative indication (8), often reducing costs associated with drug development and the time to clinical translation (9). Metformin is an oral biguanide derived from the French lilac, Galega officinalis, and is widely used as the first-line treatment for type 2 diabetes mellitus. Other indications for metformin include polycystic ovary disease, obesity and gestational diabetes, thereby demonstrating its safety profile in a wide range of patients (10). The concept of metformin having anti-tumor properties was first introduced by Dilman in the early 1970s, using a derivative of metformin, phenformin, to reduce tumor formation (11, 12). This concept was revisited in 2005 following an epidemiological study that suggested patients with type 2 diabetes taking metformin have a reduced risk of cancer (13). This has since stimulated significant interest in the potential of using metformin as a treatment for cancer.
Metformin has been described to indirectly activate AMP-activated protein kinase (AMPK) in the liver to reduce blood glucose levels (14). AMPK is also described as a metabolic tumor suppressor in cancer development (15). It has therefore been hypothesized that metformin also acts directly on cancer cells by activating the AMPK pathway (16, 17). However, there is increasing evidence of alternative mechanistic pathways which are both dependent and independent of AMPK. A systematic review on this topic was conducted in 2015 including 11 studies (18). Since then, there have been an additional 41 studies published on the action of metformin in HNSCC, requiring an updated review. Scoping reviews have been designed to systematically map the available evidence and therefore suitable to address the aim of this study (19). This scoping review aims to collate the reported mechanisms of action of metformin in HNSCC in the pre-clinical setting. Mapping the identified cellular pathways will help inform study designs when using metformin, either alone or as a combination therapy, for the treatment of head and neck cancer.
This scoping review followed the methodology published by the Joanna Briggs Institute (20).
This search aims to identify published literature. To identify keywords for the search strategy, an initial search of MEDLINE and CINAHL using keywords (Head and neck cancer) AND (Metformin) was conducted to screen for articles and develop full search terms through a screen of the title, abstract and indexed terms in consultation with a Flinders University librarian in March 2021. An updated search was conducted in May 2023. Details on the search terms are provided in Supplementary Table 1. The databases subsequently searched include MEDLINE, CINAHL, Embase, SCOPUS, and Cochrane. Reference lists of included studies were reviewed to identify additional studies.
This review systematically maps the available evidence from studies that used cell culture and animal models in the pre-clinical laboratory setting, specifically in HNSCC of mucosal origin: oral cavity, oropharynx, hypopharynx, and larynx. The studies are limited to English, with no limitation to the timeframe.
The exclusion criteria are HNSCC of other subsites (salivary gland, sinonasal cavity, nasopharynx and cutaneous). Studies using results derived from reported contaminated cell lines (Hep-2, KB, Ca9-22) (21–24) were also excluded. One study using a syngeneic mouse model was also excluded as it utilized murine cancer cell lines (25). Clinical studies involving patients are beyond the scope of this review.
Following the search, all identified studies were uploaded into Covidence systematic review software (Veritas Health Innovation, Melbourne, Australia) and duplicates were removed. Two independent reviewers (LH and ND) screened studies based on title and abstract assessment. Disagreements were resolved with a full-text review and a discussion amongst the two reviewers. Full-text review was then assessed against the inclusion and exclusion criteria. The reasons for exclusion following full-text review are listed in Figure 1 (PRISMA 2020) (26).
Studies were discussed according to the hallmarks and enabling characteristics of cancer (27, 28). A few studies discussed properties of metformin that do not fall within the described hallmarks and will be discussed separately.
The described molecular pathways are compiled and illustrated using BioRender (BioRender.com).
A total of 1288 studies were identified with 829 remaining after removal of duplicates, amongst which 52 studies met the inclusion criteria (Figure 1). The year, country and experimental designs of the identified studies are provided in Supplementary Data 1 and Supplementary Table 2. There has been an increase in the number of studies published with a peak of 10 studies published in 2019. Geographically, China has published the greatest number of studies (n=26) since 2011. The experimental designs of the preclinical studies are listed in Supplementary Table 3. A summary table of the cell lines, their original anatomical subsites and the reported mutations are provided in Supplementary Table 4. 29 HNSCC cell lines were used, with the oral tongue being the most common subsite. 4 cell lines were reported to be HPV-positive. The studies of metformin as a single agent in vitro (n=44) are summarized in Supplementary Table 5, metformin in combination with another therapy in vitro (n=24) are summarized in Supplementary Table 6, and metformin use in vivo (n=25) are summarized in Supplementary Table 7. Metformin doses vary significantly from 10 μM (29) to 100 mM (30).
In pre-clinical studies of mucosal HNSCC, metformin is reported to modulate several hallmarks of cancer (31), resulting in reduced cell growth in vitro and in vivo. Transcriptomic profiling identified differentially expressed genes following metformin treatment in HNSCC cell lines affecting all aspects of cancer development (32). Numerous mechanisms have been proposed and are summarized in this discussion.
Due to metformin’s hydrophilic properties, cellular uptake is dependent on active organic cation transporters (OCTs) (33). OCT3 is highly expressed in histological samples of dysplastic oral lesions and well-to-moderately differentiated HNSCC tumors but is significantly reduced in poorly differentiated tumors (34, 35). The viability of HN4 cell lines, which lack OCT3 expression, was not affected by metformin treatment (34). Further support was provided by the knockdown of OCT3 xenograft mouse models, which also demonstrated reduced activation of AMPK and increased mammalian target of rapamycin (mTOR) activity, negating the inhibitory effects of metformin (34, 36). These studies demonstrate that OCT3 is necessary for metformin to affect HNSCC cells. Therefore, cancer cells in poorly differentiated HNSCC, with low or no OCT3 expression, may not be affected by metformin. Future studies could investigate how HNSCC differentiation status and OCT3 expression may affect the response of metformin clinically.
The Warburg Effect, first discussed by Otto Warburg in 1956, is a metabolic shift in cancer cells that leads to the production of ATP via aerobic glycolysis, rather than oxidative phosphorylation, despite the presence of abundant oxygen (37). Many mechanisms contribute to this metabolic shift; with one being the oncogenic phosphoinositide-3-kinase (PI3K)/AKT/mTOR pathway, increasing glucose uptake and glycolysis (38, 39). Metformin targets the Warburg effect by activating AMPK to downregulate the mTOR pathway which in turn reduces HIF-1α, increases pyruvate dehydrogenase (PDH), and increases the conversion of pyruvate to acetyl-CoA rather than lactate (Figure 2). The chemotherapeutic agent, 5-fluorouracil (5-FU) has also been reported to inhibit AKT and mTOR, thereby reducing the downstream Warburg effect. The combination of metformin and 5-FU demonstrated a greater reduction in cell or tumor proliferation and an increase in apoptosis compared to single agents alone in vitro and in vivo, both acting on this mechanistic pathway (40).
Hypoxia-inducible factor (HIF)-1ɑ is activated in the hypoxic tumor environment, which in turn stimulates pyruvate dehydrogenase kinase (PDK) to inactivate pyruvate dehydrogenase (PDH) (41). This prevents the conversion of pyruvate to acetyl-CoA, instead promoting pyruvate conversion to lactate. As a result, lower PDH and higher HIF-1ɑ are present in patients with oral SCC compared to those with oral premalignant lesions (42). Metformin treatment reduced HIF-1ɑ, upregulated PDH mRNA and reduced heat shock protein 90 (HSP90), resulting in reduced cell proliferation and migration, increased apoptosis, and DNA fragmentation (42). Furthermore, metformin is reported to work synergistically with dichloroacetate (an inhibitor of PDK) in reducing cell viability (43) (Figure 2). While mechanisms were not explored, dichloroacetate is expected to prevent the inactivation of PDH and allow pyruvate to progress into the tricarboxylic acid cycle (Figure 2) (44).
Malic enzyme 2 catalyzes the conversion of malate to pyruvate by reducing NADH to NADPH within the mitochondria and plays a key role in redox balance and energy production (45). It modulates AMPK/AKT pathways, p53 function, cellular differentiation, glutamine oxidation and reactive oxygen species (ROS) production to promote the survival of cancer cells (45). Analysis of The Cancer Genome Atlas (TCGA) data demonstrates that overexpression of malic enzyme 2 in HNSCC is linked with lower overall survival (46). Metformin reduced malic enzyme 2 in both wild-type p53 (HN30) and mutant p53 (HN31) cell lines, which activate both p21 and ROS to induce senescence (46) (Figure 2).
TP53 is a gene that encodes for the p53 protein and is frequently mutated in HNSCC. HNSCC cells are highly dependent on glucose for survival with mutated (mut) TP53 preferencing glycolysis over mitochondrial respiration (47, 48). 2-deoxy-D-glucose (2-DG) is a glucose analogue that competitively inhibits glucose uptake and prevents further glycolysis (49). Therefore, targeting glucose metabolism with 2-DG, in combination with metformin in the isogenic cell lines HN30 and HN31, reduced overall cell numbers (47) (Figure 3). A follow-up study performed by the same investigators analyzed the differing responses to 2-DG and radiation in cells with mutTP53 compared to wtTP53 (48) to find mutTP53 cell lines more radioresistant than wtTP53. Cell lines with mutTP53 were found to have less mitochondrial reserve, with a greater dependence on glycolysis, thus rendering them more susceptible to glycolysis inhibitor 2-DG which increased their radiosensitivity. In contrast, cells with preserved wtTP53 prefer mitochondrial oxidative phosphorylation resulting in relative insensitivity to glycolytic inhibition by 2-DG. Metformin can inhibit mitochondrial respiration in both mutTP53 and wtTP53, reducing oxygen consumption rates, leading to a compensational increase in glycolytic activity (48). As a result, the combination of metformin and 2-DG significantly impacts on the glycolytic activity in wtTP53 cells that are less sensitive to 2-DG treatment. Depending on the TP53 mutational status, radio sensitization could occur using 2-DG with or without metformin (48) (Figure 3). This appears paradoxical, as the study describes using combination drugs (2-DG and metformin) in wtTP53 cells which are more radiosensitive but only using one drug (2-DG) for mutTP53 cell lines. However, it is an interesting concept to use 2-DG and metformin in combination to target the two metabolic pathways.
Glutamine is another important fuel for the proliferation and survival of HNSCC cells (50). Glutaminase 1 (GLS1) is a key enzyme converting glutamine to glutamate which is subsequently transformed to ɑ-ketoglutarate for the Krebs cycle (Figure 2). The TCGA database reports GLS1 to be highly expressed in HNSCC cell lines (51), stimulating an interest in using the selective glutaminase inhibitor bis-2-(5-phenylacetamido-1,3,4-thiadiazol-2-yl)ethyl sulfide (BPTES) in cancer treatment. The increase in GLS1 expression suggests altered glutamine metabolism in HNSCC cells allows for glutamine-dependent growth. The combination of BPTES and metformin reduced cell growth, cell viability and increased apoptosis by targeting apoptosis and cell cycle pathways (51). To induce cell cycle arrest, BPTES increased p21 while metformin reduced Cyclin E2 and cyclin B1/CDK1 complexes. Both BPTES and metformin were able to trigger the Caspase 3/PARP cascade to induce apoptosis (51) (Figure 4).
AMPK is a protein kinase that is essential in the restoration of energy homeostasis in response to metabolic stress with mTOR being one of its downstream targets (52). Metformin alone was demonstrated to reduce viability, proliferation, and colony formation in vitro (36, 53–56) and reduce tumor volume and weight in vivo (36, 55, 56). Treatment of HNSCC cell lines with metformin resulted in G0/G1 cell cycle arrest, activation of AMPK, and reduction in mTOR, p-S6K, p4EBP and p70S6K (36, 55–57) (Figure 2).
Dasatinib, a kinase inhibitor used for the treatment of chronic myeloid leukemia, activates AMPK by reducing ATP through the inactivation of ERK. The activation of AMPK by metformin goes on to induce endoplasmic reticulum stress which degrades EGFR through the c-Cbl lysosome pathway, resulting in apoptosis (58) (Figure 2). With both dasatinib and metformin activating AMPK, synergistically they reduce cell viability, increase apoptosis in vitro and reduce tumor volume in a HNSCC xenograft model (58).
Cell cycle dysregulation in cancer cells can result in uncontrolled division (59). The TCGA data showed overexpression of cell cycle regulators cyclin D1, cyclin-dependent kinase (CDK) 4 and CDK6 in HNSCC, which are associated with poor survival (60). Normal cell cycle progression from G1 to S-phase requires Cyclin D1, CDK4, and CDK6 to phosphorylate retinoblastoma protein (pRb) and release transcription factor elongation factor (EF) 2 (61). Downstream targets of mTOR, cyclin D1 and associated CDK4, and CDK6, are downregulated due to metformin treatment (55, 62, 63) (Figure 5). The level of CDK inhibitors (p21 and p27) increased in some studies (63, 64) but not in others (55). Metformin also activates p38, which inhibits JNK/STAT 3/AKT with subsequent reduction in cyclin D1 mRNA and protein levels (62) (Figure 5). Additionally, Sikka et al. proposed that metformin inhibits protein translation via inhibition of translation initiation protein 4E-BP1 and EF2 through upstream regulator AMPK (64), subsequently reducing expression of cyclin D1, cyclin E, CDK2, and CDK4, resulting in cell cycle arrest (64) (Figure 5).
Figure 5 The mechanisms of action of metformin affecting the cell cycle in HNSCC. The regulation of proteins (marked as up or downward arrows) in response to metformin is referenced accordingly.
Chen et al. described metformin inhibiting Aurora A through Late SV40 Factor (LSF) (65) (Figure 5). Aurora-A plays an important role in the progression of G2/M transition and over-expression has been found to correlate with advanced TNM staging and poorer prognosis (66). Interestingly, Chen reported no change in cell cycle distribution despite a reduction in cell viability, colony formation and the ability to migrate or invade. This is in contrast to other studies where both metformin (55, 60, 62–64) and Aurora-A inhibitors (67) resulted in cell cycle arrest.
Skinner et al. demonstrated using gene sequencing of 74 HNSCC samples that TP53 mutations are predictive of increasing risk of locoregional recurrence, poorer overall survival, and the likelihood of radio resistance. “Disruptive” TP53 mutations have a worse outcome than “wild-type” or “non-disruptive” mutations (68). Radiation was able to activate p21 and ROS in cell lines with wild-type and non-disruptive TP53 mutations to induce senescence, but not those with disruptive mutations (Figure 6). However, the combination of metformin and radiotherapy increased radiosensitivity in cells with disruptive TP53 mutations by increasing ROS (Figure 6). This was further supported by a reduction in clonogenic survival in vitro and reduced tumor growth in vivo when metformin and radiation were used in combination (68).
Figure 6 Metformin combined with radiotherapy targets disruptive TP53 mutations to cause cell senescence.
Metformin was also shown to reduce the development of premalignant lesions in a 4-nitroquinoline 1-oxide (4NQO) rodent model. 4NQO is a water-soluble carcinogen that simulates the step-wise progression of human tobacco-related HNSCC in the oral cavity of the rodent (69). In mice treated with metformin (via intra-peritoneal injection), there was a reduction in the basal proliferation of the hyperplastic regions on the tongue and the number, size, and progression of the oral lesions to malignancy (39). This study supports the use of metformin as a chemoprevention measure for the development of premalignant lesions, which may be beneficial in high-risk patients with leukoplakia.
Cell senescence is an outcome for many CDK inhibitors, aiming at cell cycle arrest, rendering them viable but unable to proliferate (60). Senescence-associated secretory phenotype (SASP) can act to either inhibit or promote cancer by secreting inflammatory cytokines. Metformin has been shown to act as a senostatic drug by reducing the release of tumor-promoting cytokines (interleukin (IL)6, IL8, monocyte chemoattractant protein, GRO-family chemokines) into the medium after treatment with CDK4/6 inhibitor LY2835219 (Ademaciclib) in oral squamous cell carcinoma (OSCC) cell lines, without changing senescence-inducing cytokines (IL1α, IL1β, transforming growth factor (TGF)-β, chemokine ligand 5) via the mTOR/STAT3 and IL6/STAT3 pathways (60) (Figure 5). The addition of metformin to LY2835219 did not change the proportion of senescent cells but changed the cytokines secreted by these cells. This study demonstrated the mechanisms that allow metformin and CDK inhibitors to work together to promote cell senescence.
The evasion of cell death is a hallmark of cancer (70). In some instances, metformin induced apoptosis in HNSCC cells (54, 55). Anti-apoptotic proteins Bcl-2 and Bcl-xL were down-regulated (62) while pro-apoptotic protein Bax was up-regulated (55, 63). The combination of metformin and histone deacetylase inhibitor 4SC-202 increased Bax, p53 and intrinsic apoptosis (cleaved caspase-9, cleaved caspase-3, cleaved- PARP), but not extrinsic apoptosis marker caspase-8 (71). This pro-apoptotic characteristic has been validated in vivo with increased apoptotic tumor cells detected with TUNEL staining (55, 71).
Autophagy is a physiological process of cellular degradation and recycling in response to stress and damage (72). Autophagy is complex and can function both as a tumor suppressor by progressive cellular consumption or as a tumor promoter by sustaining survival through catabolic degradation (72). In other cancer types, metformin has been reported to both stimulate and inhibit autophagy (73). In this review of HNSCC, studies demonstrate that metformin can both decrease (74) and increase (62, 75), autophagy.
Metformin increased the markers of vesicle nucleation and elongation, LC3B and Beclin-1, which represented autophagy (62) (75). This involved activating p27 via both AMPK/mTOR and MEK/ERK/RSK pathways (62) (Figure 7). Even though metformin alone reduced cell viability and increased apoptosis and autophagy, the anti-tumor effects were further enhanced when metformin was combined with the autophagy inhibitor hydroxychloroquine in vitro and in vivo (75).
In contrast, primary OSCC cells co-cultured with normal oral fibroblasts (NOF) in transwell chambers, produced an environment with high concentrations of ROS and intercellular ATP, significantly increasing the growth of OSCC (74). The presence of NOFs promoted autophagy and mitophagy proteins, LC3B and BNIP3, and autophagosome-lysosome fusion to sustain proliferation. In this instance, metformin reduced the tumor-promoting autophagy by inhibiting LC3B and BNIP3 (74) (Figure 7). However, in this co-cultured OSCC model, NOFs inhibit AMPK by stabilizing the mitochondrial membrane potential and reduce metformin-induced apoptosis; thereby preventing a reduction in cell proliferation (74).
These studies present interesting and opposing effects of metformin on autophagy. The activation of autophagy by metformin in FaDu cells resulted in a dose-dependent reduction in cell viability (62). While metformin was reported to inhibit tumor-promoting autophagy initiated by NOFs in the co-culture model, it did not result in a reduction in OSCC cell proliferation (74). The NOFs represent the tumor microenvironment, better reflecting the in vivo interactions of cancer cells with their surrounding supporting cells. The protective effect of NOFs and other environmental factors may be overlooked in monocultures and warrants consideration in future research.
The Hippo pathway plays a critical role in modulating cell proliferation and cellular phenotypes (76). Yes-associated protein (YAP) is an oncogene within the Hippo pathway that is frequently overexpressed in the HNSCC invasive front and associated with tumor aggressiveness, nodal metastasis, epithelial-mesenchymal transition (EMT) progression and drug resistance (76). Metformin is reported to activate the Hippo pathway, which reduces the expression of YAP and subsequent mTOR and c-Myc expression (63) (Figure 8). As a result, the combination of metformin and verteporfin, a YAP inhibitor, has an additional inhibitory effect on cell cycle and proliferation than either agent alone (63).
EMT plays a key role in cancer progression, invasion, and metastasis. It is a biological process of polarized epithelial cells transitioning to mesenchymal phenotypes and is frequently initiated and regulated by hypoxia and the STAT3-TWIST pathway (77, 78). In the hypoxic environment, metformin reduced proliferation, migration, invasion and EMT by decreasing mTOR, HIF-1a, PKM2, STAT3 and EMT markers (78) (Figure 9).
Upregulation of TWIST1 promotes EMT and cancer invasiveness which is associated with poorer prognosis (79). Metformin and histone deacetylase inhibitor, 4SC-202, reduced EMT via the STAT3/TWIST1 axis (80). The drug combination is proposed to target STAT 3 via two different mechanisms: metformin suppresses STAT3 through activation of the AMPK/mTOR pathway and 4SC-202 suppresses histone deacetylase 3 enzymatic activity, preventing it from forming a complex to activate STAT3 (80) (Figure 9).
Epigenetic regulation alters gene expression, without altering the DNA sequence, by DNA methylation, histone modification or through the effects of non-coding RNA (81).
Tet methylcytosine dioxygenase 2 (TET2) is considered a tumor suppressor protein which converts 5-methylcytosine (5mC) to 5-hydroxymethylcytosine (5hmC) (82). Expression of TET2, and subsequently 5hmC, are reduced in HNSCC resulting in an alteration of gene expression to develop more aggressive features (83). Metformin, along with other treatments such as 5-azacytidine and Vitamin C are reported to increase the expression of TET2 and 5hmC (Figure 10), resulting in reduced proliferation and migration, but increased apoptosis (83).
Small nucleolar RNA host gene 7 (SNHG7) is a long non-coding RNA with oncogenic potential. It affects several transcription factors and signaling pathways by targeting microRNAs (miRNA) (84). High expression of SNHG7 correlates with increased tumor size, differentiation, advanced TNM staging, metastases, taxol chemotherapy resistance and radio resistance (85). Metformin was reported to inhibit HNSCC cell growth and increase apoptosis by silencing SNHG7 through the activation of S-adenosylhomocysteine (SAH) which upregulates DNA methyltransferase 1 (DNMT1), leading to increased methylation of the SNHG7 promoter (85) (Figure 10). Metformin’s action is likely a result of activating the S-adenosylhomocysteine hydrolase (SAHH), an enzyme that hydrates SAH into adenosine and homocysteine (86, 87). By targeting SNHG7, metformin appears to increase chemosensitivity to taxol and increase radiosensitivity (85).
MicroRNA-21-5p (miR-21-5p), a known oncogenic miRNA (88), is often over-expressed in HNSCC. It reduces the expression of the tumor suppressor gene Programmed Cell Death 4 (PDCD4) (89). Metformin, when used at supraphysiological doses of 50-100 mM, is reported to reduce miR-21-5p and increase the expression of PDCD4 (30, 90) (Figure 9). However, a metformin dose of 50-100 mM is unlikely to be achievable in vivo and therefore difficult to translate clinically.
LIN28 is a major developmental regulator and, therefore, an important target in cancer research. It can transform cancer cells into cancer stem cells (91) by inhibiting the maturation process of tumor suppressor miRNA let-7 (92). When metformin was combined with LIN28 inhibitor, C1632, it demonstrated a reduction in proliferation, migration, invasion in vitro, and a reduction in tumor weight and size in vivo (91). In addition, metformin induces Dicer expression via AMPK (93), which is key to the maturation of pre-let7 to miRNA let7 (91). HMGA2 is a non-histone chromosomal protein that regulates chromatin structure which promotes cell cycle entry and inhibition of apoptosis in human malignancies (94). It is a downstream target of tumor suppressor let-7 and was synergistically reduced when OSCC cell lines were treated with the C1632 and metformin combination. The proposed mechanism of this synergistic outcome of metformin and C1632 is two-pronged - C1632 inhibits LIN28, allowing the primary mRNA (pri-let7) to be converted to let7; metformin activates AMPK which activates Dicer, converting pre-let7 to let7 (91) (Figure 10).
Metformin was also found to target circular RNAs (circRNA) which regulate miRNAs (95). Circ_0003214 was noted to be highly expressed while tumor suppressor miR-489-3p was under-expressed in HNSCC (95). Increased level of ADAM10 in OSCC is reported to contribute to migration and invasion (96). Metformin reduces circ_0003214 which subsequently increases miR-489-3p and reduces its target ADAM10 (95) (Figure 10). This was reflected in the reduction of cell viability and colony formation in vitro and reduced tumor weight and volume in vivo (95).
Aberrant splicing events can result in cancer (97). Metformin is reported to be involved in 1521 alternative splicing events involved in processes including: centrosome function, DNA damage, ATPase activity, RIG-I-like receptor signaling pathway and Wnt signaling (98). Among them, exon 3 skipping of nucleotide binding protein 2 (NUBP2), an ATP-binding protein, was increased following metformin treatment (98). Metformin induces a splicing switch to the canonical long isoform, which in turn reduces intracellular ATP to inhibit cancer cell proliferation and reduce colony formation (98).
Metformin treatment of HNSCC cell lines induced acetylation of the lysine residue of histone H3 protein (H3K27ac) (32). H3K27ac is an enhancer of transcription. Transcriptomic profiling revealed metformin affects transcripts involved in the cell cycle, ribosome, oncogenes, tumor suppressors, metabolism and cytokines via H3K27ac (32).
Cancer stem cells (CSCs) are a small subpopulation of the cells within a tumor that have self-renewal and differentiating abilities. These cells are easily cultured and highly tumorigenic with distinct surface markers such as CD44, CD133 and ALDH (99).
Metformin reduced HNSCC CSC markers (NOTCH1, STAT3, CD44, JAGGED) and stemness-related transcription factors (OCT4, SOX2, KLF4, c-Myc, NANOG), suggesting it has an impact on CSCs (29, 56, 100) (Figure 11). When combined with a high dose of curcumin (100 μM), it reduced proliferation and increased apoptosis in cancer cell lines (53). This activity was supported in a 4NQO rodent model when metformin was combined with low-dose curcumin, demonstrating decreased carcinogenesis progression with reduced expression of NF-kB, pS6 and the CSC profile (100).
In contrast, Kuo et al. showed that low-dose metformin increased cell proliferation in laryngeal cancer stem cells and ALDH+ CSC, but reduced proliferation in ALDH- non-CSC (101). Metformin increased stemness markers and protected CSCs against cisplatin. However, this protective effect was not observed in non-stem cells, resulting in a reduction in proliferation (101). Metformin is known to inhibit mitochondrial complex I. However, in vitro and in silico data suggest that metformin binds strongly and inhibits mitochondrial complex III activity, which reduces ROS production (101). Reduced ROS is essential to maintain and promote CSC, whereas it has the opposite effect on non-CSC (101) (Figure 11). Therefore, Kuo et al. proposed that metformin reduces proliferation by targeting non-CSC, but protected CSCs which then causes treatment resistance. This study is important to highlight the heterogeneity of a cancer cell population and how the different cell types within the tumor may respond differently to metformin.
Patient-derived cancer organoids are generated from pluripotent stem cells and differentiate into a three-dimensional complex structure of various cell types (102). This model, consisting of CSCs, allows a more accurate representation of human cancer and may affect metformin’s anti-proliferative activity.
Tumors are surrounded by fibroblasts, immune cells, inflammatory cells, epithelial, endothelial, mesenchymal cells, and the extracellular matrix, Together, they make up the tumor microenvironment (103). The tumor microenvironment plays an active role in the promotion of tumor survival and progression. This section discusses how metformin can affect various factors in the tumor microenvironment.
Cancer-associated fibroblasts (CAFs) surround the tumor and secrete various factors to support and promote cancer cell growth (104). Co-culturing primary OSCC with normal oral fibroblasts (NOF) increases proliferation and autophagy (74) as discussed earlier (Section 5.7.1).
Metabolic coupling, also termed “reverse Warburg effect”, occurs when the cancer stroma metabolically supports the cancer cells by catabolite transfer. Monocarboxylate transporters (MCTs) are essential for the cancer cells to undergo metabolic coupling. Caveolin-1(CAV1) is a membrane protein frequently downregulated in CAFs and associated with tumor aggressiveness (105). Xenografts with CAV1 knockdown fibroblasts did not show a significant impact on tumor growth when treated with metformin, suggesting CAV1 is essential for metformin’s action (105). In a co-injection rodent model with fibroblasts, treatment with metformin decreased metabolic coupling by reducing cancer cell MCT1 and restoring fibroblast CAV1 expression (105) (Figure 12).
Integrin beta 2 (ITGB2) is a subunit of integrin and a cell surface glycoprotein that regulates cell survival, proliferation, and movement. It is involved in the control of metabolic pathways by coordinating intracellular and extracellular signaling (106). ITGB2 is upregulated on OSCC CAFs membrane and cytoplasm. It is associated with higher TNM staging, greater depth of invasion, shorter survival, and early recurrence (106). The presence of ITGB2, whether on CAFs or overexpressed on NOFs, was able to promote tumor cell proliferation by activating the PI3K/AKT/mTOR pathway resulting in increased glycolysis, generating pyruvate which is then converted to lactate. Lactate exits CAFs through MCT4, enters cancer cells through MCT1, and is used by mitochondrial complex I to generate NADH (106) (Figure 12). Metformin targets the electron transport chain, disrupting the availability of NAD+ that is required for the oxidation of lactate for cancer cells. Zhang et al. concluded that even though the presence of ITGB2 on the CAFs in the tumor microenvironment promotes OSCC proliferation, it also makes them more sensitive to the effect of metformin.
Cellular communication network factor 1 (CCN1/Cyr16) is a regulatory protein of the tumor microenvironment and is over-expressed in many cancers including HNSCC (107). Metformin reduces Cyr16 and its downstream target p-AKT resulting in a reduction in cell viability in cell line SCC25 (108) (Figure 12).
ΔNp63 is the predominant p63 isoform and is frequently overexpressed in HNSCC (109). It is essential for regulating adhesion molecules and can influence the tumor microenvironment (110). The combination of metformin with histone deacetylase inhibitor, 4SC-202, synergistically reduced cell proliferation and colony formation in vitro, and reduced tumor weight and volume in vivo (71). The combination treatment destabilized oncogene ΔNp63 in a post-translational manner by increasing protein ubiquitination and proteasome-mediated degradation through ubiquitin E3 ligase, WWP1(Figure 13) (111), which led to reduced fibronectin resulting in reduced cell matrix adhesion (111) and increased apoptosis (71). Metformin in a glucose-deprived environment, or used in combination with 2-DG, further enhanced the effects of reduced ΔNp63 (Figure 13) (111).
Figure 13 The effect of metformin in combination with other treatments (4SC-202 and 2-DG) on ΔNp63a.
Nerve growth factor receptor (NGFR) is a transmembrane protein that promotes cell survival, and its overexpression in OSCC is linked with poorer prognosis (112). Proteolytic processing withɑ-secretase and 𝛾-secretase cleaves NGFR into two components: NGFR-N and the intracellular domain (ICD). NGFR-N degrades p53 (113), while ICD activates the NF-𝜅B pathway (114), both leading to tumor progression. Metformin was shown to inhibit NGFR proteolysis by reducing the expression of ɑ-secretase and 𝛾-secretase genes, thereby reducing cell proliferation and growth (Figure 14).
The rapid proliferation of cells and lack of vasculature within a solid tumor can result in tumor hypoxia, which contributes to chemo- and radio-resistance (115). Higher cisplatin concentrations were required when HNSCC cells were grown in a hypoxic (1% O2) compared to a normoxic (20% O2) environment (116). Metformin was shown to inhibit hypoxia-induced NF-𝜅B and thereby downregulate HIF-1a expression and downstream proteins GLUT-1 and Bcl-2 in vitro and in vivo, potentially through the activation of AMPK (116) (Figure 14). Qi et al. then showed the IC50 of cisplatin was significantly less when used in combination with metformin during hypoxia, suggesting metformin sensitizes HNSCC cells to cisplatin and reduces chemoresistance despite hypoxia (116). However, it is important to also note that metformin has been reported to protect esophageal SCC from cisplatin by reducing the formation of cisplatin-DNA adduct complexes (117). These two studies (116, 117) differ in the concentration of oxygen and may suggest metformin is more effective against cells in a more hypoxic environment.
Gefitinib is an effective epidermal growth factor receptor tyrosine kinase inhibitor in lung cancer but has not been approved by the FDA for use in HNSCC (118). Hypoxia has also been shown to induce gefitinib resistance by inducing EMT (118). In HNSCC in vitro and in vivo models the combination of metformin and gefitinib reduced HIF-1ɑ, cyclin D1 and EMT to overcome hypoxia-induced gefitinib resistance (118) (Figure 14). Clinical trials combining metformin and gefitinib are underway in lung cancer (NCT01864681, NCT03071705; www.ClinicalTrials.gov), however, such trials have not been considered for HNSCC. These two studies (116, 118) suggest that the addition of metformin to cisplatin and gefitinib may reduce the development of hypoxia-induced chemoresistance in HNSCC and may be worth further investigation.
Tumor hypoxia contributes to radio resistance, and metformin was reported to increase tumor oxygen saturation and hemoglobin concentration using non-invasive photoacoustic imaging in an orthotopic xenograft rodent model (119). This study suggests that metformin may target tumor tissue and supports its use as a radiosensitizer (119). It is the first study that demonstrated metformin modulates tumor oxygenation in HNSCC without increasing the vessel count. This suggests metformin increased tumor oxygen saturation by reducing oxygen consumption (119). However, the benefit of metformin is temporary, with oxygen saturation returning to baseline 48 hours after cessation.
The immune microenvironment plays a role in protecting tumors from chemotherapy by producing pro-tumor mediators (120). Tumor-associated macrophages (TAM) contribute to chemotherapy resistance (121). Hypoxia in the tumor microenvironment polarizes macrophages to M2-macrophages (M2-TAM) via HIF-2ɑ, which secretes C-C motif chemokine ligand 15 (CCL15). CCL15 then reacts with the C-C motif chemokine receptor (CCR1) on the tumor cell surface to activate NF-𝜅B, promoting gefitinib resistance. Metformin was found to inhibit CCL15 secretion by M2-TAM and reduce CCR1 expression in HNSCC cells (Figure 14); therefore, reducing the development of drug resistance when used in combination with gefitinib (122).
Metformin has also been reported to impact both the peripheral blood and tumor infiltrating natural killer (NK) cells (123). Metformin-treated NK cells increased NK cell cytotoxicity compared to vehicle control. This is likely a result of metformin increasing pSTAT1, which stimulates an increase in perforin secretion. Perforin plays an important role in forming transmembrane pores and subsequent apoptosis in tumor cells (124). Metformin also inhibits mTOR and pSTAT3, with subsequent impact on the tumor promoting chemokine C-X-C motif ligand 1 (CXCL1). This prevents interaction with CXC receptor 2, which then reduces the proliferation and progression of cancer cells (125). A comprehensive diagram has been included in Crist et al’s paper and therefore not replicated here (123).
The tumorigenesis of HPV-positive HNSCC relies on the inhibition of p53 by HPV viral proteins E6 and E7. Hoppe-Seyler demonstrated that metformin was able to reduce E6 and E7 expression in HPV-positive HNSCC and cervical cancer cells in a dose- and time-dependent manner, but this was reversible after changing to metformin-free media (126). Exploration of the mechanistic pathways has only been performed in cervical cancer cell lines HeLa and SiHa cells and not in HNSCC, but the results suggested that PI3K is key in the suppression of E6 and E7 proteins. Metformin induced only a reversible reduction in the oncoproteins, suggesting senescence was not achieved. Furthermore, metformin also counteracted the pro-senescent effects when combined with chemotherapeutic agents Etoposide and Doxorubicin in this study (126).These findings suggest that metformin could potentially interfere with senescence in the presence of oncoproteins E6 and E7 and should be used with caution when combined with other chemotherapy drugs. This should also be further investigated before extrapolating the results to HPV-positive HNSCC cell lines.
Several limitations need to be acknowledged when translating these pre-clinical findings into clinical treatment. The doses of metformin used for in vitro studies are generally much higher in concentration than the reported physiological concentrations in the systemic circulation, with one in vitro study using up to 100 mM metformin (30). Therapeutic doses of metformin in human systemic circulation, approximately 10-40 μM, may not be sufficient to affect tumor growth (127), therefore alternative modes of delivering metformin to the tumor cells may need to be explored. Song et al. designed a metformin-cisplatin nanoparticle that is activated by laser and targets ligand low-density lipoprotein receptor which is specific to the hypoxic centers of HNSCC (128). Metformin sensitized the effect of cisplatin in cell culture and in vivo. This novel method of drug delivery was able to target the xenograft tumor with high doses of cisplatin (10 mg/kg) combined with metformin (1 mg/kg) to overcome the low systemic concentration of metformin and reduce potential toxicities. Drug delivery methods incorporating metformin in micro- and nanoparticles are currently being explored to overcome low oral bioavailability and to target dose delivery for effective cancer treatment (129).
Cancer cell culture provides an invaluable model for investigating mechanistic pathways, but several factors may limit the relevance to responses in human disease. Cell culture growth medium provides a protected environment favoring cell growth, containing high concentrations of glucose, essential nutrients, growth factors, various hormones, and antibiotics, which are non-physiological. As a result, cells growing in this favorable environment may require higher doses of metformin. The in vitro studies discussed largely use cancer cells grown in a monolayer. Again, this is not reflective of the in vivo environment where solid tumors are 3D structures, consisting of differentiated cell types and CSC, constantly influenced by their microenvironment. This review discussed the different impact metformin has on CSC (101), hypoxic regions (116) and surrounding cells (105). 3D models such as spheroids or organoids can potentially address some of these issues.
The in vivo animal studies discussed used varying doses of metformin, with diverse methods of administration (oral intake, intraperitoneal injection or xenografts pre-treated with metformin), for a variable duration of treatment. Furthermore, xenograft models largely employ immunodeficient animals, which do not fully reflect how the human immune system interacts with cancer development. None of the studies measured metformin concentration in the animal systemic circulation, nor correlated the doses to the human systemic circulation, which is worth exploring.
In most of these pre-clinical studies, HNSCC cells have been classified as a single entity, whereas clinically HNSCC vary significantly depending on the subsite origin, differentiation status, and HPV status (currently only clinically relevant to oropharyngeal SCC). These factors impact pathophysiology, disease development and treatment response. Moving forward, a better description of cell line characteristics (including subsites, HPV status, and mutations) will be important for accurate interpretation of metformin response, characterization of the molecular pathways involved, and clinical translation.
This scoping review provides a comprehensive presentation of the biological actions of metformin in HNSCC in the pre-clinical setting. A vast array of pathways has been presented and discussed, with many impacting the hallmarks of cancer. While the AMPK/mTOR pathway is the most well-described mechanistic action of metformin in HNSCC, there is a growing focus on metformin’s role in epigenetic regulation and the surrounding tumor microenvironment. It is important to be aware that even though each study focuses on a select pathway, metformin acts through many pathways simultaneously.
A recently updated meta-analysis, including 14694 patients in 11 cohort studies, concluded that adjuvant metformin use benefits overall survival, disease-free survival and disease-specific survival in HNSCC patients (130). This review raises the concept that HNSCC disease stratification, such as OCT3 expression, TP53 mutation, and HPV status, may improve the clinical outcomes when using metformin. The response to metformin may also be affected by the surrounding tumor microenvironment, hypoxia and the heterogeneity within each tumor.
These studies are encouraging and support ongoing research into the use of metformin as an adjuvant treatment for HNSCC. However, epidemiological studies suggest that metformin is unlikely to be used as a monotherapy to effectively treat HNSCC. Combining metformin with existing or novel treatments provides an opportunity to exploit the benefit of metformin at lower doses. This review highlights additive or synergistic responses when metformin is combined with other therapies, ranging from compounds such as curcumin to radiation treatment. Validation in 3D models using co-cultured spheroids or organoids could provide valuable and novel information on metformin’s impact on surrounding cells, hypoxia and cancer stem cells.
Metformin is a widely used medication with a proven safety profile that demonstrates anti-tumor properties in HNSCC. This comprehensive scoping review of the pre-clinical literature summarizes the proposed mechanisms of action of metformin on HNSCC cancer cells, the surrounding tumor microenvironment, and animal models. Further validation of metformin combinations in 3D cancer models will provide interesting and valuable information. It is important to consider the limitations of in vitro and in vivo animal models when designing clinical trials to further explore the benefit of metformin. Metformin is at the forefront of drug repurposing and presents an exciting and promising agent for use as an adjuvant therapy in the treatment of HNSCC.
The original contributions presented in the study are included in the article/Supplementary Material. Further inquiries can be directed to the corresponding author.
LH: Conceptualization, Data curation, Funding acquisition, Methodology, Writing – original draft, Writing – review & editing. CW: Supervision, Validation, Writing – review & editing. ND: Data curation, Methodology, Writing – review & editing. MM: Supervision, Writing – review & editing. EO: Supervision, Writing – review & editing.
The author(s) declare financial support was received for the research, authorship, and/or publication of this article. The primary author is supported by the Passe and Williams Foundation Academic Surgeon-Scientist Research scholarship.
We thank Flinders University librarian Shannon Brown for her assistance and expertise with the scoping review search. This work was supported with funding from the Garnett Passe and Rodney Williams Memorial Foundation.
The authors declare that the research was conducted in the absence of any commercial or financial relationships that could be construed as a potential conflict of interest.
All claims expressed in this article are solely those of the authors and do not necessarily represent those of their affiliated organizations, or those of the publisher, the editors and the reviewers. Any product that may be evaluated in this article, or claim that may be made by its manufacturer, is not guaranteed or endorsed by the publisher.
The Supplementary Material for this article can be found online at: https://www.frontiersin.org/articles/10.3389/fonc.2024.1358854/full#supplementary-material
1. Vigneswaran N, Williams MD. Epidemiologic trends in head and neck cancer and aids in diagnosis. Oral Maxillofac Surg Clin North Am (2014) 26:123–41. doi: 10.1016/j.coms.2014.01.001
2. Australia AGC. Head and Neck Cancer in Australia Statistics (2022). Available online at: https://www.cancerAustralia.gov.au/cancer-types/head-and-neck-cancer/statistics.
3. Johnson DE, Burtness B, Leemans CR, Lui VWY, Bauman JE, Grandis JR. Head and neck squamous cell carcinoma. Nat Rev Dis Primers (2020) 6:92. doi: 10.1038/s41572-020-00224-3
4. Sindhu SK, Bauman JE. Current concepts in chemotherapy for head and neck cancer. Oral Maxillofac Surg Clin North Am (2019) 31:145–54. doi: 10.1016/j.coms.2018.09.003
5. Savvides PP. The role of chemotherapy in the management of patients with head and neck cancer. Semin Plast Surg (2010) 24:137–47. doi: 10.1055/s-0030-1255331
6. Kao SS, Ooi EH. Survival outcomes following salvage surgery for oropharyngeal squamous cell carcinoma: systematic review. J Laryngol Otol (2018) 132:299–313. doi: 10.1017/s0022215117000998
7. Arnold DJ, Goodwin WJ, Weed DT, Civantos FJ. Treatment of recurrent and advanced stage squamous cell carcinoma of the head and neck. Semin Radiat Oncol (2004) 14:190–5. doi: 10.1053/j.semradonc.2004.03.001
8. Ashburn TT, Thor KB. Drug repositioning: identifying and developing new uses for existing drugs. Nat Rev Drug Discov (2004) 3:673–83. doi: 10.1038/nrd1468
9. Pushpakom S, Iorio F, Eyers PA, Escott KJ, Hopper S, Wells A, et al. Drug repurposing: progress, challenges and recommendations. Nat Rev Drug Discov (2019) 18:41–58. doi: 10.1038/nrd.2018.168
10. Aljofan M, Riethmacher D. Anticancer activity of metformin: A systematic review of the literature. Future Sci OA (2019) 5:Fso410. doi: 10.2144/fsoa-2019-0053
11. Dilman VM. Age-associated elevation of hypothalamic threshold to feedback control, and its role in development, ageing, and disease. Lancet (1971) 297:1211–9. doi: 10.1016/S0140-6736(71)91721-1
12. Dilman VM, Anisimov VN. Effect of treatment with phenformin, diphenylhydantoin or L-dopa on life span and tumor incidence in C3h/Sn mice. Gerontology (1980) 26:241–6. doi: 10.1159/000212423
13. Evans JM, Donnelly LA, Emslie-Smith AM, Alessi DR, Morris AD. Metformin and reduced risk of cancer in diabetic patients. BMJ (2005) 330:1304–5. doi: 10.1136/bmj.38415.708634.F7
14. Rena G, Hardie DG, Pearson ER. The mechanisms of action of metformin. Diabetologia (2017) 60:1577–85. doi: 10.1007/s00125-017-4342-z
15. Li W, Saud SM, Young MR, Chen G, Hua B. Targeting Ampk for cancer prevention and treatment. Oncotarget (2015) 6:7365–78. doi: 10.18632/oncotarget.3629
16. Pernicova I, Korbonits M. Metformin–mode of action and clinical implications for diabetes and cancer. Nat Rev Endocrinol (2014) 10:143–56. doi: 10.1038/nrendo.2013.256
17. Zhou G, Myers R, Li Y, Chen Y, Shen X, Fenyk-Melody J, et al. Role of amp-activated protein kinase in mechanism of metformin action. J Clin Invest (2001) 108:1167–74. doi: 10.1172/jci13505
18. Rego DF, Elias ST, Amato AA, Canto GL, Guerra EN. Anti-tumor effects of metformin on head and neck carcinoma cell lines: a systematic review. Oncol Lett (2017) 13:554–66. doi: 10.3892/ol.2016.5526
19. Munn Z, Peters MDJ, Stern C, Tufanaru C, McArthur A, Aromataris E. Systematic review or scoping review? Guidance for authors when choosing between a systematic or scoping review approach. BMC Med Res Method (2018) 18:143. doi: 10.1186/s12874-018-0611-x
20. Peters MDJ, Marnie C, Tricco AC, Pollock D, Munn Z, Alexander L, et al. Updated methodological guidance for the conduct of scoping reviews. JBI Evidence Synthesis (2020) 18:2119–26. doi: 10.11124/JBIES-20-00167
21. Toolan HW. Transplantable human neoplasms maintained in cortisonetreated laboratory animals: H.S. #1; H.Ep. #1; H.Ep. #2; H.Ep. #3; and H.Emb.Rh. 1. Cancer Res (1954) 14:660–6.
22. O’Neill ID. Continued misrepresentation of Kb cells as being of oral cancer phenotype requires action. Oral Oncol (2009) 45:e117–e8. doi: 10.1016/j.oraloncology.2009.02.005
23. Gorphe P. A comprehensive review of Hep-2 cell line in translational research for laryngeal cancer. Am J Cancer Res (2019) 9:644–9.
24. Zhao M, Sano D, Pickering CR, Jasser SA, Henderson YC, Clayman GL, et al. Assembly and initial characterization of a panel of 85 genomically validated cell lines from diverse head and neck tumor sites. Clin Cancer Res (2011) 17:7248. doi: 10.1158/1078-0432.CCR-11-0690
25. Veeramachaneni R, Yu W, Newton JM, Kemnade JO, Skinner HD, Sikora AG, et al. Metformin generates profound alterations in systemic and tumor immunity with associated antitumor effects. J Immunother Cancer (2021) 9:1–11. doi: 10.1136/jitc-2021-002773
26. Page MJ, McKenzie JE, Bossuyt PM, Boutron I, Hoffmann TC, Mulrow CD, et al. The Prisma 2020 statement: an updated guideline for reporting systematic reviews. BMJ (2021) 372:n71. doi: 10.1136/bmj.n71
27. Hanahan D, Weinberg Robert A. Hallmarks of cancer: the next generation. Cell (2011) 144:646–74. doi: 10.1016/j.cell.2011.02.013
28. Hanahan D. Hallmarks of cancer: new dimensions. Cancer Discov (2022) 12:31–46. doi: 10.1158/2159-8290.Cd-21-1059
29. Patil S. Metformin treatment decreases the expression of cancer stem cell marker Cd44 and stemness related gene expression in primary oral cancer cells. Arch Oral Biol (2020) 113:104710. doi: 10.1016/j.archoralbio.2020.104710
30. Sun R, Ma X, Cai X, Pan X, Liu D. The effect and mechanism of action of metformin on in vitro Fadu cell proliferation. J Int Med Res (2016) 44:1049–54. doi: 10.1177/0300060516642645
31. Morale MG, Tamura RE, Rubio IGS. Metformin and cancer hallmarks: molecular mechanisms in thyroid, prostate and head and neck cancer models. Biomolecules (2022) 12:357. doi: 10.3390/biom12030357
32. Liu S, Shi C, Hou X, Tian X, Li C, Ma X, et al. Transcriptional and H3k27ac related genome profiles in oral squamous cell carcinoma cells treated with metformin. J Cancer (2022) 13:1859–70. doi: 10.7150/jca.63234
33. Graham GG, Punt J, Arora M, Day RO, Doogue MP, Duong JK, et al. Clinical pharmacokinetics of metformin. Clin Pharmacokinet (2011) 50:81–98. doi: 10.2165/11534750-000000000-00000
34. Patel H, Younis RH, Ord RA, Basile JR, Schneider A. Differential expression of organic cation transporter Oct-3 in oral premalignant and Malignant lesions: potential implications in the antineoplastic effects of metformin. J Oral Pathol Med (2013) 42:250–6. doi: 10.1111/j.1600-0714.2012.01196.x
35. Hu Y, Qian Y, Lin L, Chen W, Yang L, Hu X, et al. Differential expression of organic Cation transporter 3 in oral submucous fibrosis–associated buccal squamous cell carcinoma. Oral Surgery Oral Medicine Oral Pathol Oral Radiol (2018) 126:48–53. doi: 10.1016/j.oooo.2018.01.009
36. Madera D, Vitale-Cross L, Martin D, Schneider A, Molinolo AA, Gangane N, et al. Prevention of tumor growth driven by Pik3ca and Hpv oncogenes by targeting Mtor signaling with metformin in oral squamous carcinomas expressing Oct3. Cancer Prev Res (2015) 8:197–207. doi: 10.1158/1940-6207.CAPR-14-0348
37. Warburg O. On the origin of cancer cells. Science (1956) 123:309–14. doi: 10.1126/science.123.3191.309
38. Chen Z, Lu W, Garcia-Prieto C, Huang P. The Warburg effect and its cancer therapeutic implications. J Bioenergetics Biomembranes (2007) 39:267. doi: 10.1007/s10863-007-9086-x
39. Vitale-Cross L, Molinolo AA, Martin D, Younis RH, Maruyama T, Patel V, et al. Metformin prevents the development of oral squamous cell carcinomas from carcinogen-induced premalignant lesions. Cancer Prev Res (2012) 5:562–73. doi: 10.1158/1940-6207.CAPR-11-0502
40. Harada K, Ferdous T, Harada T, Ueyama Y. Metformin in combination with 5-fluorouracil suppresses tumor growth by inhibiting the Warburg effect in human oral squamous cell carcinoma. Int J Oncol (2016) 49:276–84. doi: 10.3892/ijo.2016.3523
41. Kim JW, Tchernyshyov I, Semenza GL, Dang CV. Hif-1-mediated expression of pyruvate dehydrogenase kinase: A metabolic switch required for cellular adaptation to hypoxia. Cell Metab (2006) 3:177–85. doi: 10.1016/j.cmet.2006.02.002
42. Guimaraes TA, Farias LC, Santos ES, Fraga CAC, Orsini LA, Teles LF, et al. Metformin increases Pdh and suppresses Hif-1a under hypoxic conditions and induces cell death in oral squamous cell carcinoma. Oncotarget (2016) 7:55057–68. doi: 10.18632/oncotarget.10842
43. Inanc S, Keles D, Sipahi M, Baskin Y, Oktay G. Effects of metformin and dichloroacetate on mitochondrial energy metabolism in oral cavity cancer cells. ENT Updates (2018) 9:68–73. doi: 10.1002/2211-5463.12453
44. Inanc S, Keles D, Eskiizmir G, Baskin Y, Oktay G. Metformin and dichloroacetate combination exert a synergistic effect on cell viability of oral squamous cell carcinoma. ENT Updates (2019) 9:68–73. doi: 10.32448/entupdates.569464
45. Sarfraz I, Rasul A, Hussain G, Hussain SM, Ahmad M, Nageen B, et al. Malic enzyme 2 as a potential therapeutic drug target for cancer. IUBMB Life (2018) 70:1076–83. doi: 10.1002/iub.1930
46. Woo SH, Yang LP, Chuang HC, Fitzgerald A, Lee HY, Pickering C, et al. Down-regulation of Malic enzyme 1 and 2: sensitizing head and neck squamous cell carcinoma cells to therapy-induced senescence. Head Neck (2016) 38 Suppl 1:E934–40. doi: 10.1002/hed.24129
47. Sandulache VC, Ow TJ, Pickering CR, Frederick MJ, Zhou G, Fokt I, et al. Glucose, not glutamine, is the dominant energy source required for proliferation and survival of head and neck squamous carcinoma cells. Cancer (2011) 117:2926–38. doi: 10.1002/cncr.25868
48. Sandulache VC, Skinner HD, Ow TJ, Zhang A, Xia X, Luchak JM, et al. Individualizing antimetabolic treatment strategies for head and neck squamous cell carcinoma based on Tp53 mutational status. Cancer (2012) 118:711–21. doi: 10.1002/cncr.26321
49. Zhang D, Li J, Wang F, Hu J, Wang S, Sun Y. 2-deoxy-D-glucose targeting of glucose metabolism in cancer cells as a potential therapy. Cancer Lett (2014) 355:176–83. doi: 10.1016/j.canlet.2014.09.003
50. Jiang J, Srivastava S, Zhang J. Starve cancer cells of glutamine: break the spell or make a hungry monster? Cancers (2019) 11:804. doi: 10.3390/cancers11060804
51. Yang J, Guo Y, Seo W, Zhang R, Lu C, Wang Y, et al. Targeting cellular metabolism to reduce head and neck cancer growth. Sci Rep (2019) 9:4995. doi: 10.1038/s41598-019-41523-4
52. Chomanicova N, Gazova A, Adamickova A, Valaskova S, Kyselovic J. The role of Ampk/Mtor signaling pathway in anticancer activity of metformin. Physiol Res (2021) 70:501–8. doi: 10.33549/physiolres.934618
53. Lindsay C, Kostiuk M, Conrad D, O'Connell DA, Harris J, Seikaly H, et al. Antitumor effects of metformin and curcumin in human papillomavirus positive and negative head and neck cancer cells. Mol Carcinogenesis (2019) 58:1946–59. doi: 10.1002/mc.23087
54. Zhang J. Effect of Adriamycin combined with metformin on biological function of human tongue cancer ssc-15 cells. Oncol Lett (2019) 17:5674–80. doi: 10.3892/ol
55. Luo Q, Hu D, Hu S, Yan M, Sun Z, Chen F. In vitro and in vivo anti-tumor effect of metformin as a novel therapeutic agent in human oral squamous cell carcinoma. BMC Cancer (2012) 12:517. doi: 10.1186/1471-2407-12-517
56. Wu X, Yeerna H, Goto Y, Ando T, Wu VH, Zhang X, et al. Metformin inhibits progression of head and neck squamous cell carcinoma by acting directly on carcinoma-initiating cells. Cancer Res (2019) 79:4360–70. doi: 10.1158/0008-5472.CAN-18-3525
57. Chen Y, Liao L, Qiu J, Zheng Y, Tang S, Li R, et al. Research on the inhibitory effect of metformin on human oral squamous cell carcinoma Scc-4 and Cal-27 cells and the relevant molecular mechanism. Biomed Res (India) (2017) 28:6350–4.
58. Lin YCL, Wu MH, Wei TT, Lin YC, Chen CC. (2014). Ampk activation mediates dasatinib-induced Egfr degradation and apoptosis in head and neck cancer, in: Cancer Research Conference: AACR Special Conference on Cellular Heterogeneity in the Tumor Microenvironment, , Vol. 75. doi: 10.1158/1538-7445.CHTME14-A73
59. Matthews HK, Bertoli C, de Bruin RAM. Cell cycle control in cancer. Nat Rev Mol Cell Biol (2022) 23:74–88. doi: 10.1038/s41580-021-00404-3
60. Hu Q, Peng J, Jiang L, Li W, Su Q, Zhang J, et al. Metformin as a senostatic drug enhances the anticancer efficacy of Cdk4/6 inhibitor in head and neck squamous cell carcinoma. Cell Death Dis (2020) 11:925. doi: 10.1038/s41419-020-03126-0
61. Diehl JA. Cycling to cancer with cyclin D1. Cancer Biol Ther (2002) 1:226–31. doi: 10.4161/cbt.72
62. Tsou YA, Chang WC, Lin CD, Chang RL, Tsai MH, Shih LC, et al. Metformin increases survival in hypopharyngeal cancer patients with diabetes mellitus: retrospective cohort study and cell-based analysis. Pharmaceuticals (2021) 14:1–16. doi: 10.3390/ph14030191
63. Wang Y, Zhang Y, Feng X, Tian H, Fu X, Gu W, et al. Metformin inhibits Mtor and C-Myc by decreasing yap protein expression in Oscc cells. Oncol Rep (2021) 45:1249–60. doi: 10.3892/or
64. Sikka A, Kaur M, Agarwal C, Deep G, Agarwal R. Metformin suppresses growth of human head and neck squamous cell carcinoma via global inhibition of protein translation. Cell Cycle (2012) 11:1374–82. doi: 10.4161/cc.19798
65. Chen CH, Tsai HT, Chuang HC, Shiu LY, Su LJ, Chiu TJ, et al. Metformin disrupts Malignant behavior of oral squamous cell carcinoma via a novel signaling involving late Sv40 factor/Aurora-A. Sci Rep (2017) 7:1358. doi: 10.1038/s41598-017-01353-8
66. Chien C-Y, Tsai H-T, Su L-J, Chuang H-C, Shiu L-Y, Huang C-C, et al. Aurora-a signaling is activated in advanced stage of squamous cell carcinoma of head and neck cancer and requires osteopontin to stimulate invasive behavior. Oncotarget (2014) 5:2243–62. doi: 10.18632/oncotarget.1896
67. Bavetsias V, Linardopoulos S. Aurora kinase inhibitors: current status and outlook. Front Oncol (2015) 5:278. doi: 10.3389/fonc.2015.00278
68. Skinner HD, Sandulache VC, Ow TJ, Meyn RE, Yordy JS, Beadle BM, et al. Tp53 disruptive mutations lead to head and neck cancer treatment failure through inhibition of radiation-induced senescence. Clin Cancer Res (2012) 18:290–300. doi: 10.1158/1078-0432.CCR-11-2260
69. Wang Z, Wu VH, Allevato MM, Gilardi M, He Y, Luis Callejas-Valera J, et al. Syngeneic animal models of tobacco-associated oral cancer reveal the activity of in situ anti-Ctla-4. Nat Commun (2019) 10:5546. doi: 10.1038/s41467-019-13471-0
70. Wong RSY. Apoptosis in cancer: from pathogenesis to treatment. J Exp Clin Cancer Res (2011) 30:87. doi: 10.1186/1756-9966-30-87
71. He Y, Tai S, Deng M, Fan Z, Ping F, He L, et al. Metformin and 4sc-202 synergistically promote intrinsic cell apoptosis by accelerating Deltanp63 ubiquitination and degradation in oral squamous cell carcinoma. Cancer Med (2019) 8:3479–90. doi: 10.1002/cam4.2206
72. Yun CW, Lee SH. The roles of autophagy in cancer. Int J Mol Sci (2018) 19. doi: 10.3390/ijms19113466
73. Lu G, Wu Z, Shang J, Xie Z, Chen C, Zhang C. The Effects of Metformin on Autophagy. Biomedicine Pharmacotherapy (2021) 137:111286. doi: 10.1016/j.biopha.2021.111286
74. Zhang Z, Liang X, Fan Y, Gao Z, Bindoff LA, Costea DE, et al. Fibroblasts rescue oral squamous cancer cell from metformin-induced apoptosis via alleviating metabolic disbalance and inhibiting Ampk pathway. Cell Cycle (2019) 18:949–62. doi: 10.1080/15384101.2019.1598727
75. Zhao W, Chen C, Zhou J, Cai K, Shen M, Chen X, et al. Inhibition of autophagy promotes the anti-tumor effect of metformin in oral squamous cell carcinoma. Cancers (2022) 14. doi: 10.3390/cancers14174185
76. Shin E, Kim J. The potential role of Yap in head and neck squamous cell carcinoma. Exp Mol Med (2020) 52:1264–74. doi: 10.1038/s12276-020-00492-9
77. Wendt MK, Balanis N, Carlin CR, Schiemann WP. Stat3 and epithelial-mesenchymal transitions in carcinomas. Jakstat (2014) 3:e28975. doi: 10.4161/jkst.28975
78. Yin W, Liu Y, Liu X, Ma X, Sun B, Yu Z. Metformin inhibits epithelial-mesenchymal transition of oral squamous cell carcinoma via the mtor/Hif-1alpha/Pkm2/Stat3 pathway. Oncol Lett (2021) 21:31. doi: 10.3892/ol.2020.12292
79. Qin Q, Xu Y, He T, Qin C, Xu J. Normal and disease-related biological functions of twist1 and underlying molecular mechanisms. Cell Res (2012) 22:90–106. doi: 10.1038/cr.2011.144
80. He Y, Fan Z, He L, Zhang C, Ping F, Deng M, et al. Metformin combined with 4sc-202 inhibited the migration and invasion of Oscc via Stat3/Twist1. OncoTargets Ther (2020) 13:11019–29. doi: 10.2147/OTT.S268851
81. Sharma S, Kelly TK, Jones PA. Epigenetics in cancer. Carcinogenesis (2009) 31:27–36. doi: 10.1093/carcin/bgp220
82. Jäwert F, Hasséus B, Kjeller G, Magnusson B, Sand L, Larsson L. Loss of 5-hydroxymethylcytosine and tet2 in oral squamous cell carcinoma. Anticancer Res (2013) 33:4325–8.
83. Huang R, Wang Y, Ge H, Wang D, Wang Y, Zhang W, et al. Restoration of tet2 deficiency inhibits tumor growth in head neck squamous cell carcinoma. Ann Trans Med (2020) 8:329. doi: 10.21037/atm
84. Najafi S, Ghafouri-Fard S, Hussen BM, Jamal HH, Taheri M, Hallajnejad M. Oncogenic roles of small nucleolar rna host gene 7 (Snhg7) long noncoding Rna in human cancers and potentials. Front Cell Dev Biol (2022) 9:809345. doi: 10.3389/fcell.2021.809345
85. Wu P, Tang Y, Fang X, Xie C, Zeng J, Wang W, et al. Metformin suppresses hypopharyngeal cancer growth by epigenetically silencing long non-coding Rna Snhg7 in Fadu cells. Front Pharmacol (2019) 10:143. doi: 10.3389/fphar.2019.00143
86. Cuyàs E, Fernández-Arroyo S, Verdura S, García R, Stursa J, Werner L, et al. Metformin regulates global DNA methylation via mitochondrial one-carbon metabolism. Oncogene (2018) 37:963–70. doi: 10.1038/onc.2017.367
87. Zhong T, Men Y, Lu L, Geng T, Zhou J, Mitsuhashi A, et al. Metformin alters DNA methylation genome-wide via the H19/Sahh axis. Oncogene (2017) 36:2345–54. doi: 10.1038/onc.2016.391
88. Medina PP, Nolde M, Slack FJ. Oncomir addiction in an in vivo model of microrna-21-induced pre-B-cell lymphoma. Nature (2010) 467:86–90. doi: 10.1038/nature09284
89. Zhang X, Gee H, Rose B, Lee CS, Clark J, Elliott M, et al. Regulation of the tumor suppressor pdcd4 by Mir-499 and Mir-21 in oropharyngeal cancers. BMC Cancer (2016) 16:86. doi: 10.1186/s12885-016-2109-4
90. Wang S, Wang J, Wang L. Metformin inhibits proliferation of hypopharyngeal carcinoma cells by regulating Mir-21-5p and Pdcd4 expression. Acta Med Mediterr (2020) 36:1465–9. doi: 10.19193/0393-6384_2020_3_228
91. Chen H, Sa G, Li L, He S, Wu T. In vitro and in vivo synergistic anti-tumor effect of Lin28 inhibitor and metformin in oral squamous cell carcinoma. Eur J Pharmacol (2021) 891:173757. doi: 10.1016/j.ejphar.2020.173757
92. Balzeau J, Menezes MR, Cao S, Hagan JP. The lin28/let-7 pathway in cancer. Front Genet (2017) 8:31. doi: 10.3389/fgene.2017.00031
93. Blandino G, Valerio M, Cioce M, Mori F, Casadei L, Pulito C, et al. Metformin elicits anticancer effects through the sequential modulation of dicer and C-Myc. Nat Commun (2012) 3:865. doi: 10.1038/ncomms1859
94. Mansoori B, Mohammadi A, Ditzel HJ, Duijf PHG, Khaze V, Gjerstorff MF, et al. Hmga2 as a critical regulator in cancer development. Genes (Basel) (2021) 12. doi: 10.3390/genes12020269
95. Chen X, Li C, Chen W, Lin S, Yi X, Lin Q, et al. Metformin inhibits the development of hypopharyngeal squamous cell carcinoma through circ_0003214-mediated mir-489-3p-adam10 pathway. J Oncol (2021) 2021:2265475. doi: 10.1155/2021/2265475
96. Wetzel S, Seipold L, Saftig P. The metalloproteinase adam10: A useful therapeutic target? Biochim Biophys Acta (BBA) - Mol Cell Res (2017) 1864:2071–81. doi: 10.1016/j.bbamcr.2017.06.005
97. Sveen A, Kilpinen S, Ruusulehto A, Lothe RA, Skotheim RI. Aberrant Rna splicing in cancer; expression changes and driver mutations of splicing factor genes. Oncogene (2016) 35:2413–27. doi: 10.1038/onc.2015.318
98. Ji M, Lv Y, Chen C, Xing D, Zhou C, Zhao J, et al. Metformin inhibits oral squamous cell carcinoma progression through regulating Rna alternative splicing. Life Sci (2023) 315:121274. doi: 10.1016/j.lfs.2022.121274
99. Krishnamurthy S, Nör JE. Head and neck cancer stem cells. J Dent Res (2012) 91:334–40. doi: 10.1177/0022034511423393
100. Siddappa G, Kulsum S, Ravindra DR, Kumar VV, Raju N, Raghavan N, et al. Curcumin and metformin-mediated chemoprevention of oral cancer is associated with inhibition of cancer stem cells. Mol Carcinogenesis (2017) 56:2446–60. doi: 10.1002/mc.22692
101. Kuo SZ, Honda CO, Li WT, Honda TK, Kim E, Altuna X, et al. Metformin results in diametrically opposed effects by targeting non-stem cancer cells but protecting cancer stem cells in head and neck squamous cell carcinoma. Int J Mol Sci (2019) 20:07. doi: 10.3390/ijms20010193
102. Kim J, Koo B-K, Knoblich JA. Human organoids: model systems for human biology and medicine. Nat Rev Mol Cell Biol (2020) 21:571–84. doi: 10.1038/s41580-020-0259-3
103. Anderson NM, Simon MC. The tumor microenvironment. Curr Biol (2020) 30:R921–r5. doi: 10.1016/j.cub.2020.06.081
104. Ping Q, Yan R, Cheng X, Wang W, Zhong Y, Hou Z, et al. Cancer-associated fibroblasts: overview, progress, challenges, and directions. Cancer Gene Ther (2021) 28:984–99. doi: 10.1038/s41417-021-00318-4
105. Tassone P, Domingo-Vidal M, Whitaker-Menezes D, Lin Z, Roche M, Tuluc M, et al. Metformin effects on metabolic coupling and tumor growth in oral cavity squamous cell carcinoma Coinjection xenografts. Otolaryngol - Head Neck Surg (2018) 158:867–77. doi: 10.1177/0194599817746934
106. Zhang X, Dong Y, Zhao M, Ding L, Yang X, Jing Y, et al. Itgb2-mediated metabolic switch in Cafs promotes Oscc proliferation by oxidation of Nadh in mitochondrial oxidative phosphorylation system. Theranostics (2020) 10:12044–59. doi: 10.7150/thno.47901
107. Jia Q, Xu B, Zhang Y, Ali A, Liao X. Ccn family proteins in cancer: insight into their structures and coordination role in tumor microenvironment. Front Genet (2021) 12:649387. doi: 10.3389/fgene.2021.649387
108. Zhang L, Sun Q, Ou Y, Zhang Q, Hu J. Metformin induces cytotoxicity in oral squamous cell carcinoma cells by targeting Ccn1/Akt-axis. Int J Pharmacol (2022) 18:182–9. doi: 10.3923/ijp.2022.182.189
109. Gatti V, Fierro C, Annicchiarico-Petruzzelli M, Melino G, Peschiaroli A. Δnp63 in squamous cell carcinoma: defining the oncogenic routes affecting epigenetic landscape and tumor microenvironment. Mol Oncol (2019) 13:981–1001. doi: 10.1002/1878-0261.12473
110. Moses MA, George AL, Sakakibara N, Mahmood K, Ponnamperuma RM, King KE, et al. Molecular mechanisms of P63-mediated squamous cancer pathogenesis. Int J Mol Sci (2019) 20:3590. doi: 10.3390/ijms20143590
111. Yi Y, Chen D, Ao J, Sun S, Wu M, Li X, et al. Metformin promotes amp-activated protein Kinaseindependent suppression of deltanp63alpha protein expression and inhibits cancer cell viability. J Biol Chem (2017) 292:5253–61. doi: 10.1074/jbc.M116.769141
112. Søland TM, Brusevold IJ, Koppang HS, Schenck K, Bryne M. Nerve growth factor receptor (P75 Ntr) and pattern of invasion predict poor prognosis in oral squamous cell carcinoma. Histopathology (2008) 53:62–72. doi: 10.1111/j.1365-2559.2008.03063.x
113. Zhou X, Hao Q, Liao P, Luo S, Zhang M, Hu G, et al. Nerve growth factor receptor negates the tumor suppressor P53 as a feedback regulator. Elife (2016) 5. doi: 10.7554/eLife.15099
114. Bao X, Shi J, Xie F, Liu Z, Yu J, Chen W, et al. Proteolytic release of the P75(Ntr) intracellular domain by adam10 promotes metastasis and resistance to Anoikis. Cancer Res (2018) 78:2262–76. doi: 10.1158/0008-5472.Can-17-2789
115. Xia Y, Jiang L, Zhong T. The role of Hif-1α in chemo-/radioresistant tumors. Onco Targets Ther (2018) 11:3003–11. doi: 10.2147/ott.S158206
116. Qi X, Xu W, Xie J, Wang Y, Han S, Wei Z, et al. Metformin sensitizes the response of oral squamous cell carcinoma to cisplatin treatment through inhibition of Nf-Kappab/Hif-1alpha signal axis. Sci Rep (2016) 6:35788. doi: 10.1038/srep35788
117. Damelin LH, Jivan R, Veale RB, Rousseau AL, Mavri-Damelin D. Metformin Induces an Intracellular Reductive State That Protects Oesophageal Squamous Cell Carcinoma Cells against Cisplatin but Not Copper-Bis(Thiosemicarbazones). BMC Cancer (2014) 14:314. doi: 10.1186/1471-2407-14-314
118. Yin X, Wei Z, Song C, Tang C, Xu W, Wang Y, et al. Metformin sensitizes hypoxia-induced gefitinib treatment resistance of Hnscc via cell cycle regulation and Emt reversal. Cancer Manage Res (2018) 10:5785–98. doi: 10.2147/CMAR
119. Verma A, Rich LJ, Vincent-Chong VK, Seshadri M. Visualizing the effects of metformin on tumor growth, vascularity, and metabolism in head and neck cancer. J Oral Pathol Med (2018) 47:484–91. doi: 10.1111/jop.12705
120. Shiao SL, Ganesan AP, Rugo HS, Coussens LM. Immune microenvironments in solid tumors: new targets for therapy. Genes Dev (2011) 25:2559–72. doi: 10.1101/gad.169029.111
121. Mantovani A, Allavena P. The interaction of anticancer therapies with tumor-associated macrophages. J Exp Med (2015) 212:435–45. doi: 10.1084/jem.20150295
122. Yin X, Han S, Song C, Zou H, Wei Z, Xu W, et al. Metformin enhances gefitinib efficacy by interfering with interactions between tumor-associated macrophages and head and neck squamous cell carcinoma cells. Cell Oncol (2019) 42:459–75. doi: 10.1007/s13402-019-00446-y
123. Crist M, Yaniv B, Palackdharry S, Lehn MA, Medvedovic M, Stone T, et al. Metformin increases natural killer cell functions in head and neck squamous cell carcinoma through cxcl1 inhibition. J Immunother Cancer (2022) 10. doi: 10.1136/jitc-2022-005632
124. Sankar J, Arora S, Joshi G, Kumar R. Pore-forming proteins and their role in cancer and inflammation: mechanistic insights and plausible druggable targets. Chemico-Biological Interact (2022) 366:110127. doi: 10.1016/j.cbi.2022.110127
125. Yu S, Yi M, Xu L, Qin S, Li A, Wu K. Cxcl1 as an unfavorable prognosis factor negatively regulated by dach1 in non-small cell lung cancer. Front Oncol (2020) 9:1515. doi: 10.3389/fonc.2019.01515
126. Hoppe-Seyler K, Herrmann AL, Däschle A, Kuhn BJ, Strobel TD, Lohrey C, et al. Effects of metformin on the virus/host cell crosstalk in human papillomavirus-positive cancer cells. Int J Cancer (2021) 149:1137–49. doi: 10.1002/ijc.33594
127. He L, Wondisford Fredric E. Metformin action: concentrations matter. Cell Metab (2015) 21:159–62. doi: 10.1016/j.cmet.2015.01.003
128. Song C, Tang C, Xu W, Ran J, Wei Z, Wang Y, et al. Hypoxia-targeting multifunctional nanoparticles for sensitized chemotherapy and phototherapy in head and neck squamous cell carcinoma. Int J Nanomedicine (2020) 15:347–61. doi: 10.2147/IJN
129. Cetin M, Sahin S. Microparticulate and nanoparticulate drug delivery systems for metformin hydrochloride. Drug Deliv (2016) 23:2796–805. doi: 10.3109/10717544.2015.1089957
Keywords: metformin, head and neck cancer, squamous cell carcinoma, cell lines, xenograft
Citation: Huang L, Woods CM, Dharmawardana N, Michael MZ and Ooi EH (2024) The mechanisms of action of metformin on head and neck cancer in the pre-clinical setting: a scoping review. Front. Oncol. 14:1358854. doi: 10.3389/fonc.2024.1358854
Received: 20 December 2023; Accepted: 05 February 2024;
Published: 22 February 2024.
Edited by:
Jelena Milasin, University of Belgrade, SerbiaReviewed by:
Nadja Nikolic, University of Belgrade, SerbiaCopyright © 2024 Huang, Woods, Dharmawardana, Michael and Ooi. This is an open-access article distributed under the terms of the Creative Commons Attribution License (CC BY). The use, distribution or reproduction in other forums is permitted, provided the original author(s) and the copyright owner(s) are credited and that the original publication in this journal is cited, in accordance with accepted academic practice. No use, distribution or reproduction is permitted which does not comply with these terms.
*Correspondence: Lucy Huang, bHVjeS5odWFuZy50d0BnbWFpbC5jb20=
Disclaimer: All claims expressed in this article are solely those of the authors and do not necessarily represent those of their affiliated organizations, or those of the publisher, the editors and the reviewers. Any product that may be evaluated in this article or claim that may be made by its manufacturer is not guaranteed or endorsed by the publisher.
Research integrity at Frontiers
Learn more about the work of our research integrity team to safeguard the quality of each article we publish.