- Department of Urology, The Third Affiliated Hospital of Gansu University of Traditional Chinese Medicine, Baiyin, China
Prostate cancer is a major contributor to male cancer-related mortality globally. It has a particular affinity for the skeletal system with metastasis to bones seriously impacting prognosis. The identification of prostate cancer biomarkers can significantly enhance diagnosis and patient monitoring. Research has found that cancer and metastases exhibit abnormal expression of numerous non-coding RNA. Some of these RNA facilitate prostate cancer bone metastasis by activating downstream signaling pathways, while others inhibit this process. Elucidating the functional processes of non-coding RNA in prostate cancer bone metastasis will likely lead to innovative treatment strategies for this malignant condition. In this review, the mechanistic role of the various RNA in prostate cancer is examined. Our goal is to provide a new avenue of approach to the diagnosis and treatment of bone metastasis in this cancer.
Introduction
Among the leading causes of cancer deaths, prostate cancer (PCa) comes in fifth globally, and is the second most common cancer in men (1). The spread of cancer is the primary reason for mortality in PCa. PCa has a strong affinity for bone, with 80% of metastases involving various bones such as the hip, spine and pelvis (2, 3). About half of men with androgen-responsive advanced PCa are likely to experience bone metastasis within two years. Patients without bone metastasis have a 5-year survival rate of 56%, whereas those with have a significantly lower rate of 3% (4). Thus, patients with bone metastasis have a poor prognosis, and are associated with significant mortality. PCa bone metastasis presents a thorny problem in patient management. Reported studies showed that bone tissue-related protein molecules - WISP-1, BHLHE22, KLF5, CXCL12/CXCR4, GDF15 - regulate the adhesion and colonization of PCa cells in bone tissue (5–9). In addition, molecular signaling interactions between cancer cells and the marrow environment lead to preferential establishment of metastatic PCa cells in bone (10–13). The effect of these molecules on PCa bone metastasis provides a basis for the development of targeted therapies (14). In recent years, researchers have also tried to explore the mechanism of non-coding RNA (ncRNA) in the process of prostate cancer bone metastasis.
Recent research has shown that ncRNA have a major impact on tumor progression, spread, and drug resistance (15, 16). Numerous investigations have been undertaken to study the mechanism by which ncRNA control these processes. The ncRNA can be divided into categories based on their length, shape and localization. In cancer, three main categories are known: micro RNA (miRNA), long non-coding RNA (lncRNA), and circular RNA (circRNA), with each playing distinct roles (17). miRNA, which are short RNA molecules of about 20 nucleotides, bind to matching sequences in target mRNA to initiate degradation through the RNA-induced silencing complex (18). lncRNA are more than 200 nucleotides in length, and circRNA are of hundreds to thousands of nucleotides long. They can originate from exons, introns, intergenic regions, or the 5’ and 3’ untranslated regions. These molecules can adopt intricate secondary structures enabling their interaction with DNA, RNA, and proteins (19). Various mechanisms are utilized by lncRNA and circRNA to modulate gene expression. By functioning as lure for miRNA, they can short circuit the degradation of targeted mRNA, and tweak the interaction between transcription factors and promoters (20). They can serve as scaffolds to affect interactions between proteins and downstream signaling pathways. Recent studies have also shown that lncRNA and circRNA are involved in epigenetic modification of chromatin to regulate gene expression. In addition, circRNA can function to encode proteins (21). A substantial amount of evidence has shown the influence of ncRNA on multiple tumors. These molecules can behave as oncogenes or tumor suppressor genes in the initiation and progression of cancer (22).
In PCa metastasis, ncRNA not only participate in proliferation and spread but also regulate progression of tumor cells in bone (23–25) (Figure 1). More than half of miRNA are localized in genomic regions that affect the expression levels of pro-transfer genes by targeting their transcribed mRNA (26, 27). miRNA expression found in PCa is highly abnormal, and miRNA promote or inhibit bone metastasis by regulating invasion and migration (28–30). Secondly, the biological function of miRNA in the tumor microenvironment requires the diffusion of exosomes. Exosomes are tiny vesicles containing multiple miRNA (31, 32). They function as intercellular messengers facilitating the transfer of miRNA from one cell to another. Studies have reported that exosomic miRNA could have an impact on the microenvironment of bone metastasis through their action in signaling (33–36). Some lncRNA promote tumor progression in PCa. For example, lncRNA AC245100.4 was shown to promote progression via the miRNA-145-5p/RBBP5 axis (37), and lncRNA TUG1 via miRNA-128-3p/YES1 (38). Dysregulated lncRNAs expression has been shown to exert a role in the disease course of many types of malignancies. More recent research emphasized the significance of lncRNA as critical mediators of signal transduction in cancer (39). The disruption of certain lncRNA targets was linked to the stage and prognosis of cancer (40–42). Mutant expression of lncRNA in PCa was reported regarding its prognostic effect and specific expression patterns in cancer subtypes (43). Lastly, circRNAs are circular molecules formed by transsplicing, which are highly stable and can be detected in body fluids. They are involved in many important molecular regulatory mechanisms (44), and may have unique advantages in diagnosis, treatment, monitoring, and prognosis. Studies have found a role of circRNA in the onset and progression of PCa. By snaring miRNA-646 and enhancing TGF1 production, circRNA-51217 increases TGF1/P-SMAD signaling in PCa cell invasion (45). Through upregulating XIAP, the hsa-circ-0005276/FUS axis promotes PCa cell proliferation, migration, epithelial-to-mesenchymal transition (EMT) (46), and therapeutic resistance (47). In sum, ncRNA may serve as therapeutic targets, especially for patients with bone metastases. (NB: transition between the first two paragraphs is problematic, please correct the reference numbering. I made it so that the flow is smoother: miRNA first, then lncRNA, and last circRNA).
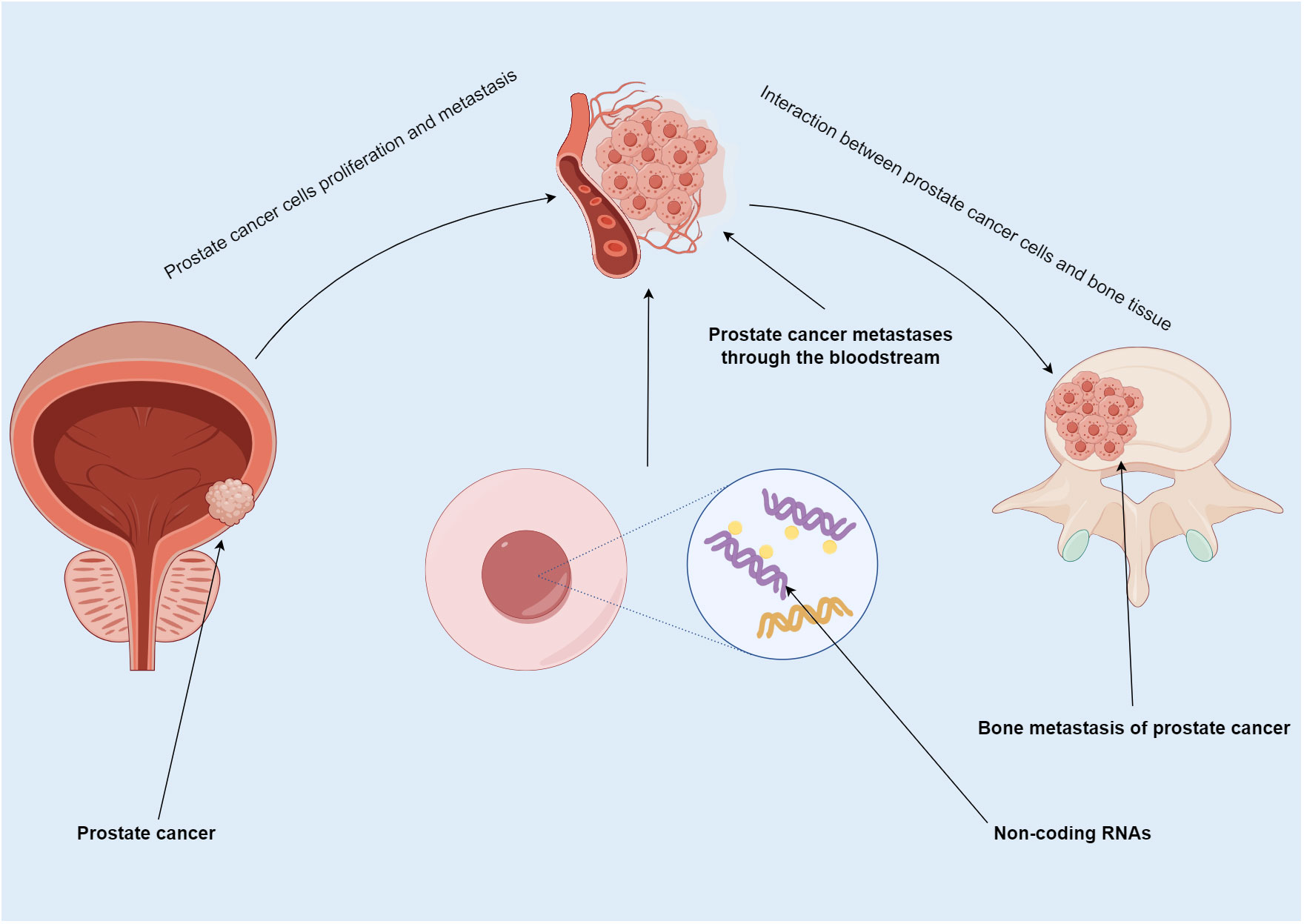
Figure 1 Summary map of non-coding RNA involvement in PCa bone metastasis. PCa usually spreads to bone. In this process, ncRNA not only participate in the proliferation and metastasis of PCa, but also regulate the progression of tumor cells in bone.
We searched on PUBMED relevant studies of ncRNA in PCa bone metastasis from June 2013 to June 2023, summarized their mechanism of action and biological function, and discussed their diagnostic and therapeutic value (Figure 2). The studies retrieved have confirmed their importance in PCa bone metastasis. Parenthetically, telomerase RNA is also found highly expressed in PCa, and is involved in tumor progression (48–50). This review summarizes the unique mechanism of ncRNA in the development of bone metastasis, suggests their utility despite current limitations.
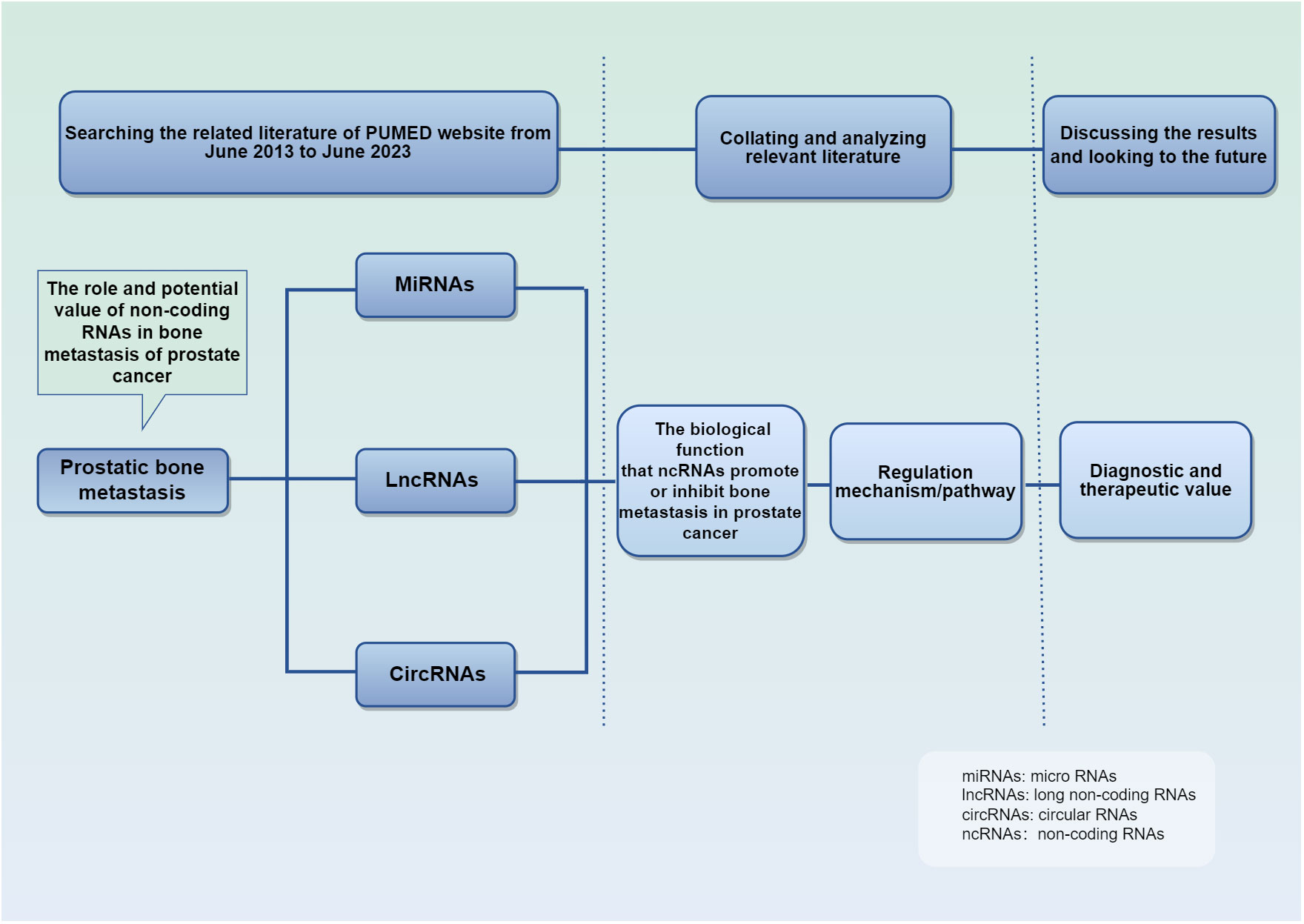
Figure 2 Schematic conceptual framework for the research questions and methods. It details the purpose and methods of the studies.
Association of miRNA with PCa bone metastasis
The role of miRNAs in tumor metastasis has been documented in various cancer types, in particular, PCa bone metastasis (51–53). Some miRNA promote bone metastasis, while some inhibit (Table 1). The controlling role either stimulates or impedes signaling by nuclear transcription factor-κB (NF-κB), Wnt, transforming growth factor β (TGF-β), PI3K/AKT, and others (Figure 3). miRNA promoting bone metastasis include miR-210-3p, miR-375, miR-18a-5p, miR-210-3p, miR-1-3p/143-3p/145-5p, miR-409-3p/-5p, and miR-378a-3p. miRNA inhibiting bone metastasis include miR-532-3p, miR-141-3p, miR-204-5p, miR-34a, miR-133b and miR-19a - 3p, miR-33a-5p, miR-582-3p/5p, miRNA-145-5p, miR-1-1, miR-133a-3p, miR-466 (Table 1).
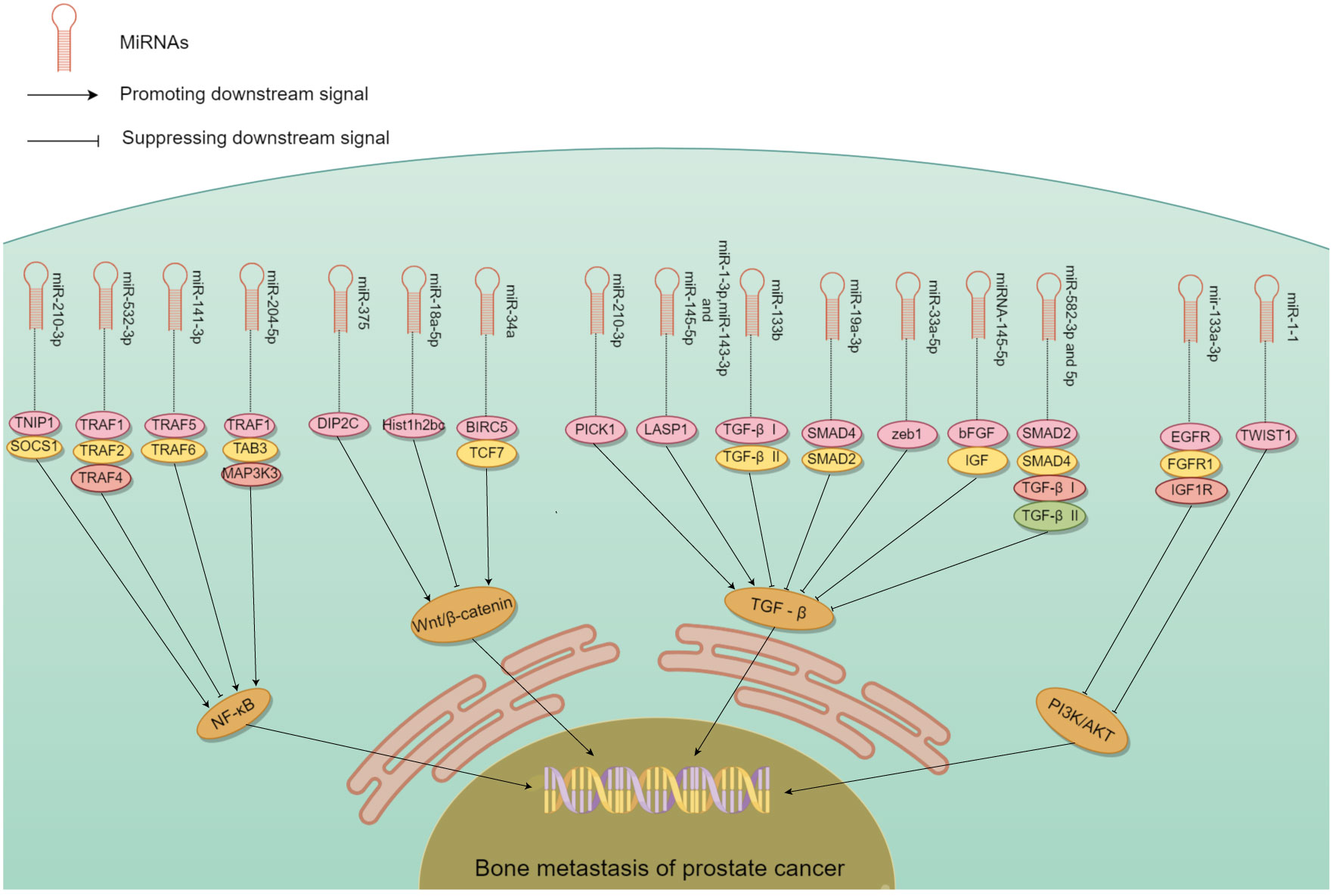
Figure 3 The mechanism of different miRNA involved in PCa bone metastasis. Many miRNA act on downstream molecules to regulate PCa bone metastasis through NF-κB, Wnt, TGF-β, PI3K/AKT and other signaling pathways. Some miRNA play a role in promoting PCa bone metastasis, while others play an inhibitory role.
NF-κB signaling pathway
In PCa patients, NF-κB regulates the expression of multiple genes in bone metastasis (73–75). It interacts with ncRNA synergistically in this process relating to tumor progression (76). miR-210-3p was linked to the formation, growth, and spread of tumors. Ren et al. (60) used clinical samples to detect miR-210-3p in PCa bone metastasis and non-bone metastasis, and study its mechanism of action. miR-210-3p maintains sustained NF-κB signaling by targeting its negative regulators TNIP1 (TNF-α-induced protein-3 interaction protein 1) and SOCS1 (cytokine signal transduction inhibitor 1) and leading to cancer cell migration and establishment of bone metastasis. This miRNAs can be a candidate target in the treatment. Whether its action on PCa bone metastasis is specific needs to be confirmed.
miRNA that have an inhibitory role in PCa bone metastasis was found by Wa et al. (67). The level of miR-532-3p was reduced in PCa that had spread to bone. Decreased expression of it showed a strong association with Gleason grade and serum level of prostate-specific antigen. Upregulation of miR-532-3p inhibited bone metastasis of PCa cells in vivo. Its overexpression suppressed activation of the NF-κB pathway by specifically targeting TRAF1 (tumor necrosis factor receptor-associated factor 1), TRAF2, and TRAF4 to impede migration and metastasis. Huang et al. (59) similarly found that miR-141-3p suppressed activation of the NF-κB pathway by targeting TRAF5 and TRAF6. Wa et al. (66) reported that miR-204-5p inactivated the NF-κB pathway by simultaneously binding TRAF1, TAB3, and MAP3K3 mRNA. The dual role of miRNA in PCa bone metastasis highlights the potential use of these miRNA as serum biomarkers.
Wnt/β-catenin signaling pathway
The Wnt/β-catenin pathway is well-studied in many cancers, and is modulated by gene mutations, extracellular inhibitors, and transcriptional cofactors (77). miRNA have a significant impact on PCa bone metastsis by their interaction with Wnt/β-catenin signaling. Liu et al. (69) showed that miR-375 exhibited high levels in PCa, and could be transported to human mesenchymal stem cells (hMSC) via exosomes. miR-375 specifically targeted DIP2C, and enhanced Wnt signaling to facilitate the transformation of hMSC into osteoblasts, which in turn stimulated the growth, infiltration, and motility of PCa cells in vitro, as well as tumor progression and osteogenic metastasis in vivo. Zeng et al. (68) found that miR-18a-5p was transported to osteoblasts by exosomes derived from PCa cells. It specifically targeted the Hist1h2bc gene, leading to an increase in Ctnnb1 expression in the Wnt/β-catenin pathway. In vivo studies have shown that anti-mir-18a-5p can significantly improve bone biomechanics of osteoblastic metastasis and reduce sclerosis. Blocking the exosome transport of miR-18a-5p also ameliorates osteoblastopathy caused by PCa.
Another crucial molecule in bone metastasis is miR-34a, which directly targeted TCF7, a gene linked to Wnt signaling (55). In PCa patient samples, miR-34a levels were negatively correlated with TCF7 expression. Chen et al. (55) demonstrated the role of miR-34a in tumor proliferation by targeting TCF7. miR-34a defects are necessary for the anti-apoptotic effects observed in Ras signaling-activated PCa cells. Furthermore, these defects enhanced tumor cell survival by the activation of Wnt and anti-apoptotic signaling pathways leading to the expression of TCF7 and BIRC5. In Ras-dependent xenotransplantation models, expression of miR-34a outside its normal site hindered the spread of cancer to bone, and reduced cancer cell growth. Thus, some miRNA could be converted into tumor suppressors due to their inhibitory function on bone metastasis.
TGF-β signaling pathway
Sustained activation of the TGF-β signaling pathway plays an essential role in the development of bone metastasis, which could be affected by miRNA (8, 78). Overexpression of PICK1 could impede invasion and migration of PCa cells in vitro, as well as bone metastasis in vivo. Dai et al. (58) found that overexpression of miR-210-3p in PCa bone metastases led to downregulation of PICK1 and, as a result, promotion of bone metastasis. Guo et al. (70) found that expression of miR-1-3p, miR-143-3p and miR-145-5p was correlated with bone metastasis. Functional experiments demonstrated that miR-1-3p/143-3p/145-5p promoted the proliferation and migration of PCa cells in vitro. LASP1 is the common target of these miRNA, which interacts with TGF-β. Shouldn’t this belong in the previous paragraph?
Decrease in other miRNA was linked to PCa spread to bone, while their overexpression suppressed bone metastasis. Huang et al. (61) found that increasing the expression of miR-133b hindered invasion and motility of PCa cells in vitro as well as frequency of bone metastasis in vivo. miR-133b hindered TGF-β signaling by targeting TGF-β receptors I and II to suppress bone metastasis. Dai et al. (64) found that increasing the expression of miR-33a-5p suppressed EMT, invasive behavior of PCa cells. Conversely, silencing of miR-33a-5p led to promotion of invasive behavior. Elevated zeb1 copy number by mir-33a-5p apparently induced a TGF-β signaling-dependent negative feedback loop. Huang et al. (65) reported that upregulation of miR-582-3p and miR-582-5p also had an inhibitory effect on PCa cells in vitro and bone metastasis in vivo. Both miR-582-3p and miR-582-5p suppressed the TGF-β pathway by targeting its multiple elements including SMAD2, SMAD4, TGF-β receptor I (TGFBRI), and TGFBRII. Luo et al. (72) showed that miRNA-145-5p had the ability to suppress growth, motility, and invasiveness of bone metastatic PCa cells. Additionally, miRNA-145-5p could suppress the expression of bFGF, IGF, and TGF-β in PC3 cells. miRNA-145-5p negatively regulated EMT, inhibited bone metastasis, and promoted apoptosis of bone metastatic cells. Thus, miRNA could both promote or inhibit cancer via the TGF-β pathway.
PI3K/AKT signaling pathway
Some miRNA appeared to inhibit bone metastasis by disrupting the PI3K/AKT signaling pathway (79, 80). Tang et al. (62) found that the levels of miR-133a-3p were lower in tumor tissues than nearby normal/benign tissues, particularly in PCa that had spread to the bone. Importantly, mir-133-a-3p overexpression could effectively hinder the development of bone metastasis in vivo. This miRNA effectively reduced the spread of PCa to bone by targeting multiple cytokine receptors such as EGFR, FGFR1, IGF1R, and MET. This inhibition of PI3K/AKT signaling plays a crucial role in preventing PCa bone metastasis. Likewise, miR-1 exhibited an inhibiting impact on PCa cells, and its expression was linked to a decrease in the likelihood of metastasis. Chang et al. (56) demonstrated that translocation of EGFR could regulate the transcription of miR-1-1, which then acted directly on TWIST1 expression. Nuclear EGFR functioned as a transcription inhibitor to restrict the tumor-suppressing impact of miR-1, and sustain the oncogenic stimulation of TWIST1 resulting ultimately in enhanced bone metastasis. These studies validated that miRNA could hinder the PI3K/AKT pathway in PCa bone metastasis.
Other pathways (?)
Additional miRNA in the spread of PCa to bone were also identified. Josson et al. (54) showed that embryonic stem cell miR-409-3p/-5p was expressed in elevated levels in PCa bone metastatic cell lines. miR-409-3p/-5p showed a significant impact on PCa by facilitating tumor growth, promoting EMT and bone metastasis. The role of extracellular vesicles (EV) in the creation of a pre-metastasis microenvironment is also significant. In PCa bone metastasis, the release of miR-378a-3p from cancer cells via EV was increased (71). This release maintained a low concentration of miR-378a-3p inside the cells, thereby promoting proliferation and EMT. Activation of the Dyrk1a/Nfatc1/Angptl2 axis by miR-378-a-3p promoted PCa bone metastasis. By using multimodal strategy analysis with xenograft models, miR-466 was shown to inhibit orthotopic tumor growth and spontaneous bone metastasis (57). This inhibition was mediated by miR-466 through targeting of the bone-related transcription factor RUNX2.
Association of lncRNA with PCa bone metastasis
lncRNA have the ability to bind DNA, RNA and protein, and play a role in cell proliferation, migration, differentiation, as well as angiogenesis. Abnormal expression of lncRNA is common in a variety of cancers (41). They have a significant impact on the onset and progression of various malignancies (81, 82). According to the findings in Table 2, a large quantity of lncRNA was shown to involve in PCa bone metastasis. Their biological functions utilized Wnt/β-catenin, TGF-β, MMP, and chemokine signaling pathways (Figure 4).
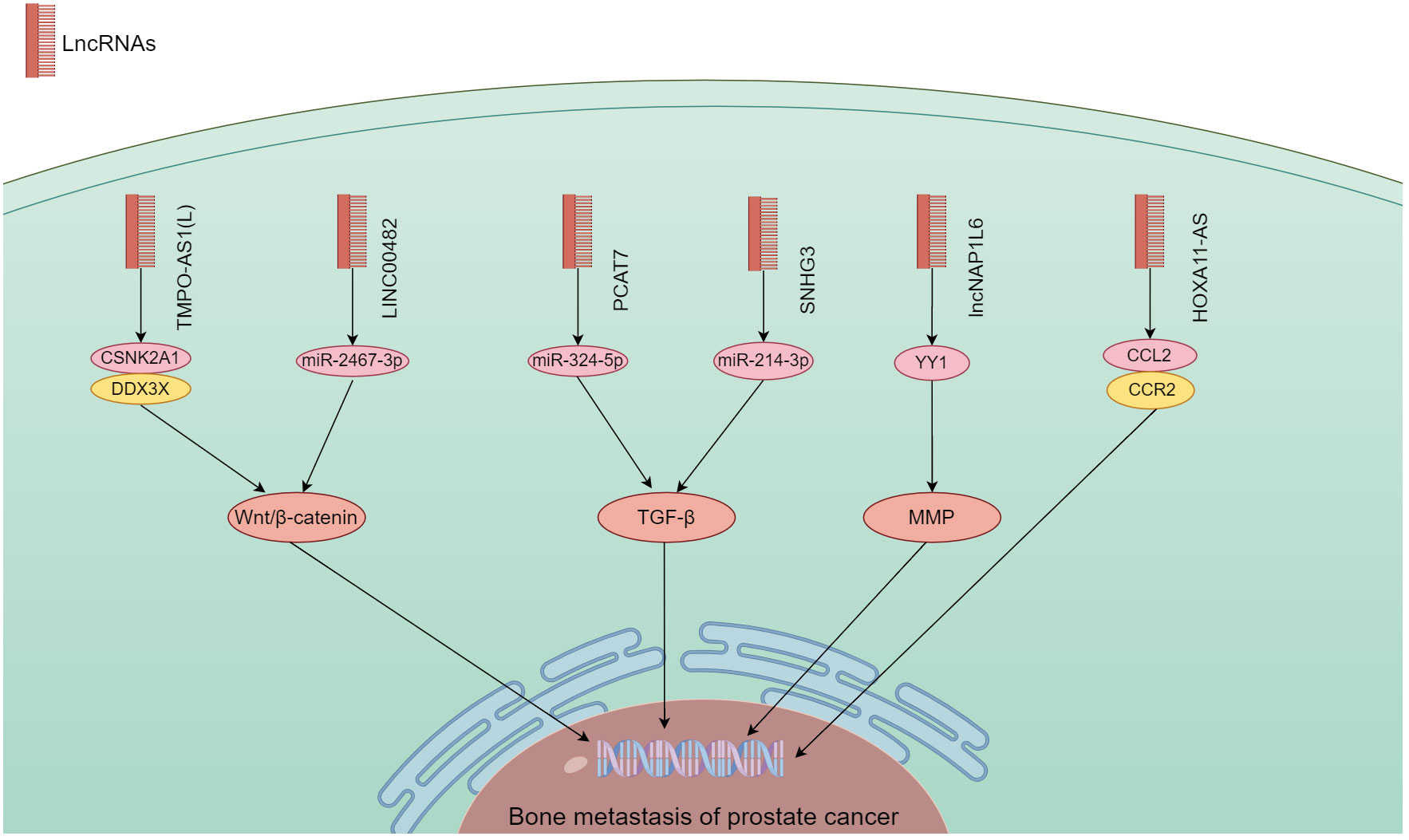
Figure 4 The mechanism of different lncRNA responsible in PCa bone metastasis. The lncRNA carry out their biological functions by utilizing the Wnt/β-catenin, TGF-β, MMP, and chemokine signaling pathways.
Wnt/β-catenin signaling pathway
lncRNA have a regulatory function in the development, spread, and drug resistance of human malignancies (92, 93). By modulating different signaling pathways, lncRNA exert their influence on cancer cells (94). Wang et al. (89) found that an extended version of TPMP-AS1(L) exhibited increased expression in PCa tissues with bone metastasis. An association was noted between overexpression of TPMP-AS1(L) and clinicopathological characteristics plus patient survival. TPMO-AS1(L) could serve as a framework for the association between casein kinase 2α1 (CSNK2A1) and DEAD-box helicase 3 X-linked (DDX3X) leading to activation of the Wnt/β-catenin pathway, and fostering the myelodysplasia of PCa. Liao et al. (90) analyzed the TCGA database set of PCa for the expression of LINC00482, and used real-time quantitative PCR to verify its expression levels in PCa and PCa bone metastases. The biological function of LINC00482 in vitro was shown by CCK-8, clone formation. This molecule exhibited notable expression in PCa with bone metastasis, and a correlation with PCa progression. Its silencing inhibited the functioning of PCa cells. LINC00482 had the potential to compete against miR-2467-3p leading to the activation of Wnt/β-catenin.
TGFβ signaling pathway
There is growing evidence that lncRNAs are involved in TGF-β-driven cancer progression, highly disease-specific, and ideal targets for therapeutic development (95). lncRNA PCAT7 was found associated with bone metastasis through analysis of TCGA data. It showed elevated expression in PCa with bone metastasis (83). Increased expression of PCAT7 facilitated the in vivo spread of PCa to bone, as well as migration, invasion, and EMT of PCa cells in vitro. It up-regulated TGFBR1 expression by uptake of miR-324-5p to activate TGF-β/SMAD signaling. Disrupting the continuous activation loop of PCAT7 and TGF-β signaling could potentially provide a therapeutic approach in treating bone metastasis. Xi et al. (91) found that SNHG3 expression in PCa bone metastasis was considerably higher than non-metastatic PCa and para-cancerous tissues. PCa patients who exhibited high levels of SNHG3 were likely to have advanced clinicopathology and unfavorable prognosis. By targeting miR-214-3p, SNHG3 boosted the expression of TGFBR1 and triggered TGF-β signaling. It showcased a novel function for the SNHG3/miR-214-3p/TGF-β pathway in the proliferation of tumors and the spread of cancer to bone in PCa.
MMP and chemokine signaling pathways
lncRNA could also promote bone metastasis by regulating MMP and chemokine signaling. Zheng et al. (88) elucidated the effect of lncNAP1L6 on PCa progression regarding its possible regulatory mechanism. PCa cells exhibited an increase in lncNAP1L6 expression. Cancer cell functions were enhanced by its overexpression in functional testing. Mechanistic experiments demonstrated lncNAP1L6 to interact with YY1 promotion of MMP2 and MMP9 transcription, and activate their signaling. Misawa et al. (86) found that invasion and proliferation of PC3 cells were enhanced by overexpression of HOXA11-AS, which was similar to box A11 antisense RNA, in bone metastatic cell lines. HOXA11-AS appeared to regulate the bone metastasis-associated CCL2/CCR2 signaling pathway in PC3 cells and SaOS2 osteoblasts. In PCa, the HOXB13/HOXA11-AS axis also controlled the integrin subunits specific to bone metastasis.
Other lncRNA that could contribute to the spread of PCa to bone include a complex of KCNQ1OT1/miR-211-5p/CHI3L1 (84). In PCa, the expression of KCNQ1OT1 was increased. Down-regulation of its expression inhibited the functioning of PCa cells. KCNQ1OT1, functioning as a competing endogenous RNA, enhanced the expression of CHI3L1, and facilitated PCa progression by binding to miR-211-5p. Mo et al. (87) investigated the physiological mechanism by which NEAT1-encapsulated exosomes affected PCa progression. NEAT1, carried by exosomes derived from PCa, competitively attached to miR-205-5p via SFPQ/PTBP2 to increase RUNX2 expression in vitro and in vivo, thereby enhancing the osteogenic differentiation of hBMSC. This finding suggested a promising therapeutic target for promoting osteogenic differentiation in hBMSC affected by PCa. Our understanding of how lncRNA affect PCa bone metastasis will create prospects for better management of this disease.
Association of circRNA with PCa bone metastasis
circRNA are ncRNA formed by back-splicing. Due to its distinct composition and quality, it has great potential in diagnosis, management, and prognosis of cancerous growths. Differential expression of these molecules was conducted with circRNA microarrays in normal prostate epithelial cells and PCa cell lines. Clinical samples were used to confirm its expression. Investigations were then carried out in vitro and in vivo to determine the underlying mechanism of distinct circRNA on PCa metastasis. Advancements have been made in the contribution of circRNA to PCa progression as evidenced by the findings in Table 3. Recent research has focused on the regulation of circRNA expression in PCa, their potential value as biomarkers, their functioning in oncogenesis and treatment resistance (47).
Promotion of PCa bone metastasis
circRNA positively regulated PCa bone metastasis by inhibiting downstream miRNA through binding interaction (I don’t quite understand the precise meaning of spongification in this context, if you mean sopping up). Major circRNA/miRNA found to date include circMID1/miR-330-3p (100), circSCAF/miR-140-3p and miR-335 (102), circ_SLC19A1/miR-497 (104), hsa_circ_0062019/miR-195-5p (105), and circSMARCA5/MiR-432 (106). These circRNA were highly expressed in both PCa and bone metastasis, and functioned to promote bone metastasis through downstream signaling axes (Figure 5).
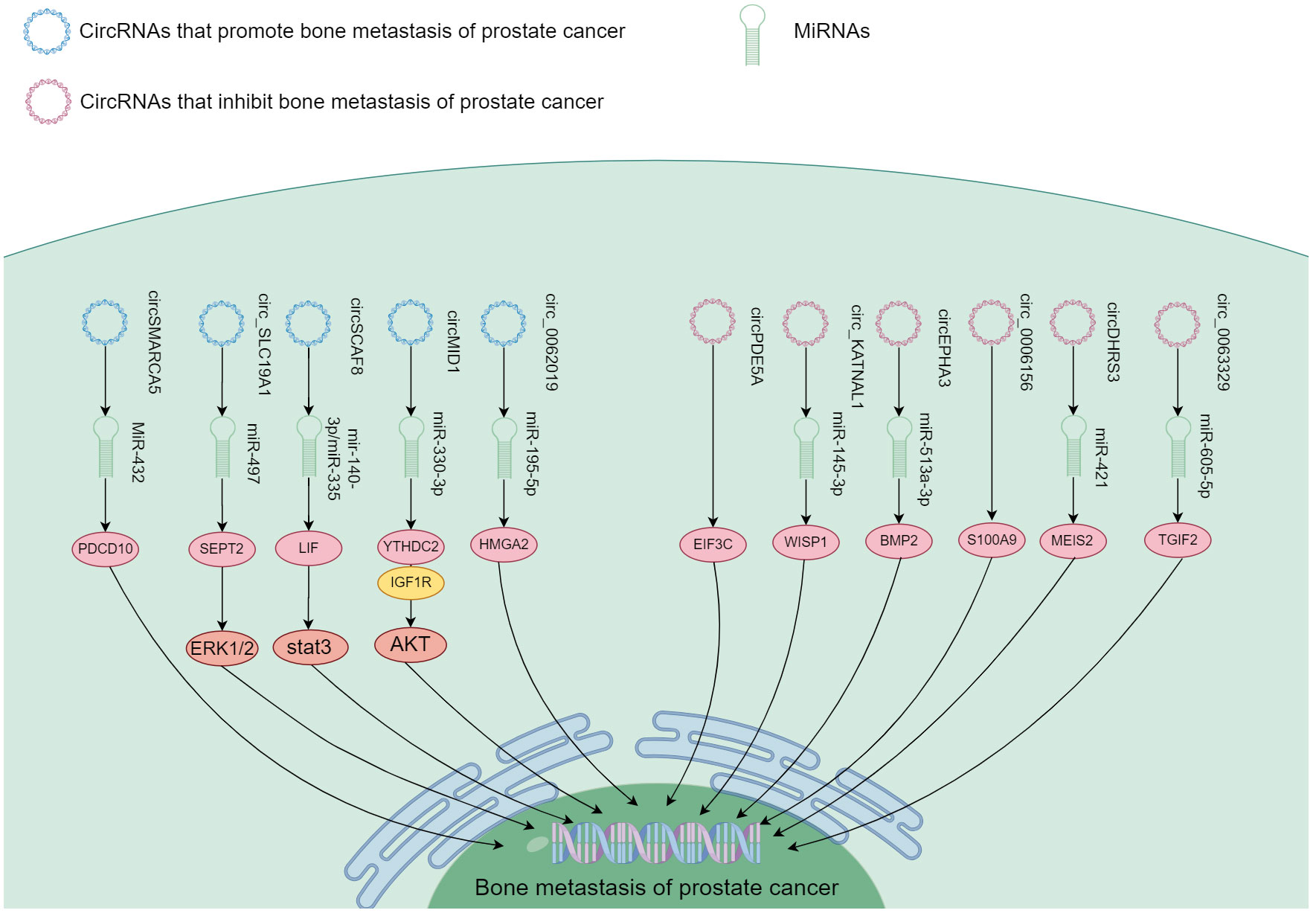
Figure 5 The mechanism of different circRNA responsible in PCa bone metastasis. These circRNA function in promoting and inhibiting PCa bone metastasis through the downstream signaling.
Representative mechanism of action was found for circMID1, which could bind miR-330-3p, and miR-330-3p could associate with IGF1R and YTHDC2. Functional experiments showed that circMID1/miR-330-3p regulated PCa progression through the YTHDC2/IGF1R/AKT axis (100). circ_SLC19A1 was found increased in PCa cells as well as the EV released by them. EVs with circ_SLC19A1 could be taken up by other PCa cells to promote cell proliferation and invasion. The expression of its target gene septin 2 (SEPT2) was significantly up-regulated. Hence, the expression of SEPT2 in cancer cells could be controlled through absorption of mir-497 by circ_SLC19A1 affecting the activation of the downstream ERK1/2 pathway, and ultimately growth and invasiveness of PCa cells (104). Another species, hsa_circ_0062019 enhanced HMGA2 expression by absorbing miR-195-5p. As a result, it stimulated PCa cells (105). Thus, a significant number of circRNA could function in the spread of PCa to bone.
Inhibition of PCa bone metastasis
Some circRNA appeared to contribute to suppression of PCa bone metastasis. These include circDHRS3/miR-421 (98), circ_KATNAL1/miR-145-3p (99), hsa_circ_0063329/miR-605-5p (101), and circEPHA3/miR-513a-3p (103). These circRNA exhibited reduced expression in both PCa and bone metastasis. They suppressed PCa metastasis to the bone through downstream signaling pathways shown in Figure 5.
circDHRS3 regulated the expression of MEIS2 through circDHRS3/miR-421/MEIS2. In vivo experiments confirmed that overexpression of circDHRS3 could inhibit lung and bone metastases of PCa cells. circDHRS3 inhibited the proliferation and metastasis of PCa cells through circDHRS3/miR-421/MEIS2 (98);circ_KATNAL1 could bind to miR-145-3p, which could target WISP1 highly expressed in many tumor types. Circ_KATNAL1 and miR-145-3p promoted their own expression and down-regulated the expression of WISP1 (99). Thus, circ_KATNAL1 exerted a cancer-fighting function in PCa via miR-145-3p/WISP1. Functional experiments conducted in vitro and in vivo demonstrated that upregulation of hsa_circ_0063329 impeded the progression of PCa cells, whereas its suppression had the opposite effect (101). In high-grade PCa and cell lines, the expression of circEPHA3 was reduced. Its role as a tumor suppressor was demonstrated through its inhibition of PCa progression and metastasis. This was achieved by direct interaction of circEPHA3 with miR-513-a-3p/BMP2 to regulate downstream genes (103). Zhang et al. (97) found that circ_0006156 impeded PCa progression by binding to S100A9. In vivo studies demonstrated that it suppressed the motility and infiltration of PCa cells by enhancing expression of S100A9. Ding et al. (96) found that circPDE5A expression was down-regulated in PCa. This circRNA blocked WTAP-dependent N6-methyladenine(m6A) methylation of eukaryotic translation initiation factor 3c (EIF3C) by forming a circpDE5A-WTAP complex, which interfered translation to inactivate the MAPK pathway, thereby inhibiting PCa progression. Although these ncRNA showed low expression in cancer, circRNA might also play an inhibitory role in bone metastasis.
Discussion
Considering the prevalence of PCa in men and the ongoing rise in incidence annually, it becomes more critical to devise novel screening techniques and therapies to supplement current ones. ncRNA are involved in gene expression, and are important in many signaling pathways. They regulate PCa bone metastasis, and can both promote and inhibit this process, which has been investigated in numerous studies. The findings indicate potential therapeutic and diagnostic development for PCa and bone metastasis. Their role still needs to be more thoroughly researched, and tested in clinical trials.
Abnormal miRNA expression plays a role in the onset of numerous cancer types (107). miRNA-associated signaling pathways could offer innovative approaches for treatment development. Takeshita et al. (108) demonstrated that miR-16 could be efficiently administered to PCa cells through tail vein injection in a mouse model of bone metastasis. A method for specific delivery of miRNA to target organs has, however, not yet been tested in the clinics. Importantly, miRNA could target numerous molecules, which poses a challenge in mitigating off-target effects. The same miRNA could have opposite effects in different tissues. Therefore, the total effect of miRNA-based treatments must be considered. The initial clinical testing involving liposome mimics of miR-34a for miRNA replacement therapy was ended early in two Phase I trials due to severe immune-related adverse events (109, 110). When the dosage of miR-16 mimics was lowered by approximately a thousand fold compared to that of miR-34a, no significant cytotoxicity was observed (110). Although this study particular showed acceptable safety and activity, additional trials are a must.
lncRNA are involved in gene expression as well, and important in the regulation of various signaling pathways. Abnormal lncRNA expression has been observed in different cancer types, including PCa (24). These observations suggested that they are a contributory factor in all stages of oncogenesis, PCa screening, diagnosis, prognosis and treatment. Currently, PCa suffers from overdiagnosis (111), and lncRNAs could become novel biomarkers in overcoming this problem.
circRNA are characterized by abundant, highly stable, evolutionarily conserved and tissue-specific expression. They play a part in oncogenesis, tumor spread, and treatment resistance (46). To date, tumor diagnosis and prognostic monitoring have largely relied on imaging and pathology. Currently, circRNA is employed as a biomarker in the identification and monitoring of urinary system tumors for diagnosis and prognosis. For example, circEGLN3 can distinguish renal clear cell carcinoma from normal tissue with 97 percent accuracy (112). Hence, circRNA could possess possibilities in the detection and surveillance of PCa bone metastasis. Furthermore, chimeric RNA could be another important type of ncRNA (113), and could influence the migration/invasion of PCa (114) although relevant studies are lacking.
Our current research suggests importance of ncRNA in PCa spread to bone. Their molecular mechanisms in cancer progression involves signaling pathways such as those of NF-κB, Wnt, TGF-β, PI3K/AKT, MMP and chemokines. Dysregulation of ncRNA may, however, occur due to other diseases or comorbidities. Further research is needed to determine the specific ones unique to PCa, if they exist. ncRNA are known to have multiple regulatory functions, and many are certainly not limited to PCa. Their action on normal human physiology needs to be fully explored to identify potential adverse side effects. At present, research on ncRNA employs mostly cell lines and animal models. Only when their functioning is thoroughly worked out can they be evaluated in clinical trials.
This review has some limitations. It only systematically summarized relevant studies in a 10 year time span. Due to the rather small number of confirmatory studies, quality could not be strictly evaluated.
Conclusions
In summary, ncRNA could promote as well as inhibit PCa bone metastasis through multiple signaling pathways. Therefore, ncRNA are expected to be used as therapeutic targets for PCa. Their specific mechanism of action in bone metastasis still needs to be explored. Research on their clinical application is minimal. With further research, ncRNA will gain importance in treatment and patient monitoring.
Author contributions
HS: Writing – original draft, Writing – review & editing. LL: Writing – original draft, Writing – review & editing. QZ: Writing – review & editing. YL: Writing – review & editing. JH: Writing – review & editing. PN: Writing – review & editing. ZH: Writing – original draft, Writing – review & editing. KC: Writing – original draft, Writing – review & editing.
Funding
The author(s) declare financial support was received for the research, authorship, and/or publication of this article. This work was supported by Baiyin Science and Technology Plan Project (Clinical Application research on early screening of Prostate cancer in Baiyin Area: 2022-3-6Y) and Baiyin First People’s Hospital Science and Technology Plan Project (2019YK-08).
Acknowledgments
We would like to thank all the authors who participated in the writing of this review, and also thank the Third Affiliated Hospital of Gansu University of Traditional Chinese Medicine for facilitating the publication of this review, and thank the FIGDRAW online graphics website for the convenience of making the images in this article.
Conflict of interest
The authors declare that the research was conducted in the absence of any commercial or financial relationships that could be construed as a potential conflict of interest.
Publisher’s note
All claims expressed in this article are solely those of the authors and do not necessarily represent those of their affiliated organizations, or those of the publisher, the editors and the reviewers. Any product that may be evaluated in this article, or claim that may be made by its manufacturer, is not guaranteed or endorsed by the publisher.
References
1. Sung H, Ferlay J, Siegel RL, Laversanne M, Soerjomataram I, Jemal A, et al. Global cancer statistics 2020: GLOBOCAN estimates of incidence and mortality worldwide for 36 cancers in 185 countries. CA Cancer J Clin. (2021) 71:209–49. doi: 10.3322/caac.21660
2. van der Toom EE, Axelrod HD, de la Rosette JJ, de Reijke TM, Pienta KJ, Valkenburg KC. Prostate-specific markers to identify rare prostate cancer cells in liquid biopsies. Nat Rev Urol. (2019) 16:7–22. doi: 10.1038/s41585-018-0119-5
3. Yin JJ, Pollock CB, Kelly K. Mechanisms of cancer metastasis to the bone. Cell Res. (2005) 15:57–62. doi: 10.1038/sj.cr.7290266
4. Smith MR, Cook R, Lee KA, Nelson JB. Disease and host characteristics as predictors of time to first bone metastasis and death in men with progressive castration-resistant nonmetastatic prostate cancer. Cancer. (2011) 117:2077–85. doi: 10.1002/cncr.25762
5. Tai HC, Chang AC, Yu HJ, Huang CY, Tsai YC, Lai YW, et al. Osteoblast-derived WNT-induced secreted protein 1 increases VCAM-1 expression and enhances prostate cancer metastasis by down-regulating miR-126. Oncotarget. (2014) 5:7589–98. doi: 10.18632/oncotarget.2280
6. Conley-LaComb MK, Semaan L, Singareddy R, Li Y, Heath EI, Kim S, et al. Pharmacological targeting of CXCL12/CXCR4 signaling in prostate cancer bone metastasis. Mol Cancer. (2016) 15:68. doi: 10.1186/s12943-016-0552-0
7. Yin C, Wang M, Wang Y, Lin Q, Lin K, Du H, et al. BHLHE22 drives the immunosuppressive bone tumor microenvironment and associated bone metastasis in prostate cancer. J Immunother Cancer. (2023) 11:e005532. doi: 10.1136/jitc-2022-005532
8. Zhang B, Li Y, Wu Q, Xie L, Barwick B, Fu C, et al. Acetylation of KLF5 maintains EMT and tumorigenicity to cause chemoresistant bone metastasis in prostate cancer. Nat Commun. (2021) 12:1714. doi: 10.1038/s41467-021-21976-w
9. Siddiqui JA, Seshacharyulu P, Muniyan S, Pothuraju R, Khan P, Vengoji R, et al. GDF15 promotes prostate cancer bone metastasis and colonization through osteoblastic CCL2 and RANKL activation. Bone Res. (2022) 10:6. doi: 10.1038/s41413-021-00178-6
10. Kolonin MG, Sergeeva A, Staquicini DI, Smith TL, Tarleton CA, Molldrem JJ, et al. Interaction between tumor cell surface receptor RAGE and proteinase 3 mediates prostate cancer metastasis to bone. Cancer Res. (2017) 77:3144–50. doi: 10.1158/0008-5472.Can-16-0708
11. Borel M, Lollo G, Magne D, Buchet R, Brizuela L, Mebarek S. Prostate cancer-derived exosomes promote osteoblast differentiation and activity through phospholipase D2. Biochim Biophys Acta Mol Basis Dis. (2020) 1866:165919. doi: 10.1016/j.bbadis.2020.165919
12. Zhao Z, Li E, Luo L, Zhao S, Liu L, Wang J, et al. A PSCA/PGRN-NF-κB-Integrin-α4 axis promotes prostate cancer cell adhesion to bone marrow endothelium and enhances metastatic potential. Mol Cancer Res. (2020) 18:501–13. doi: 10.1158/1541-7786.Mcr-19-0278
13. Yu G, Shen P, Lee YC, Pan J, Song JH, Pan T, et al. Multiple pathways coordinating reprogramming of endothelial cells into osteoblasts by BMP4. iScience. (2021) 24:102388. doi: 10.1016/j.isci.2021.102388
14. Zhang J, Sun J, Bakht S, Hassan W. recent development and future prospects of molecular targeted therapy in prostate cancer. Curr Mol Pharmacol. (2022) 15:159–69. doi: 10.2174/1874467214666210608141102
15. Yan H, Bu P. Non-coding RNA in cancer. Essays Biochem. (2021) 65:625–39. doi: 10.1042/ebc20200032
16. Slack FJ, Chinnaiyan AM. The role of non-coding RNAs in oncology. Cell. (2019) 179:1033–55. doi: 10.1016/j.cell.2019.10.017
17. Toden S, Zumwalt TJ, Goel A. Non-coding RNAs and potential therapeutic targeting in cancer. Biochim Biophys Acta Rev Cancer. (2021) 1875:188491. doi: 10.1016/j.bbcan.2020.188491
18. Vos PD, Leedman PJ, Filipovska A, Rackham O. Modulation of miRNA function by natural and synthetic RNA-binding proteins in cancer. Cell Mol Life Sci. (2019) 76:3745–52. doi: 10.1007/s00018-019-03163-9
19. Wang N, Yu Y, Xu B, Zhang M, Li Q, Miao L. Pivotal prognostic and diagnostic role of the long non−coding RNA colon cancer−associated transcript 1 expression in human cancer (Review). Mol Med Rep. (2019) 19:771–82. doi: 10.3892/mmr.2018.9721
20. Zhao W, An Y, Liang Y, Xie XW. Role of HOTAIR long noncoding RNA in metastatic progression of lung cancer. Eur Rev Med Pharmacol Sci. (2014) 18:1930–6.
21. Patop IL, Wüst S, Kadener S. Past, present, and future of circRNAs. EMBO J. (2019) 38:e100836. doi: 10.15252/embj.2018100836
22. Zhang Y, Yang M, Yang S, Hong F. Role of noncoding RNAs and untranslated regions in cancer: A review. Med (Baltimore). (2022) 101:e30045. doi: 10.1097/md.0000000000030045
23. Mugoni V, Ciani Y, Nardella C, Demichelis F. Circulating RNAs in prostate cancer patients. Cancer Lett. (2022) 524:57–69. doi: 10.1016/j.canlet.2021.10.011
24. An C, Wang I, Li X, Xia R, Deng F. Long non-coding RNA in prostate cancer. Am J Clin Exp Urol. (2022) 10:170–9.
25. Weidle UH, Epp A, Birzele F, Brinkmann U. The functional role of prostate cancer metastasis-related micro-RNAs. Cancer Genomics Proteomics. (2019) 16:1–19. doi: 10.21873/cgp.20108
26. Calin GA, Sevignani C, Dumitru CD, Hyslop T, Noch E, Yendamuri S, et al. Human microRNA genes are frequently located at fragile sites and genomic regions involved in cancers. Proc Natl Acad Sci U.S.A. (2004) 101:2999–3004. doi: 10.1073/pnas.0307323101
27. Lopez-Camarillo C, Marchat LA, Arechaga-Ocampo E, Perez-Plasencia C, Moral-Hernandez OD, Castaneda-Ortiz EJ, et al. MetastamiRs: non-coding microRNAs driving cancer invasion and metastasis. Int J Mol Sci. (2012) 13:1347–79. doi: 10.3390/ijms13021347
28. Bhagirath D, Yang TL, Dahiya R, Saini S. MicroRNAs as regulators of prostate cancer metastasis. Adv Exp Med Biol. (2018) 1095:83–100. doi: 10.1007/978-3-319-95693-0_5
29. Bonci D, Coppola V, Patrizii M, Addario A, Cannistraci A, Francescangeli F, et al. A microRNA code for prostate cancer metastasis. Oncogene. (2016) 35:1180–92. doi: 10.1038/onc.2015.176
30. Aghdam SG, Ebrazeh M, Hemmatzadeh M, Seyfizadeh N, Shabgah AG, Azizi G, et al. The role of microRNAs in prostate cancer migration, invasion, and metastasis. J Cell Physiol. (2019) 234:9927–42. doi: 10.1002/jcp.27948
31. Ruivo CF, Adem B, Silva M, Melo SA. The biology of cancer exosomes: insights and new perspectives. Cancer Res. (2017) 77:6480–8. doi: 10.1158/0008-5472.Can-17-0994
32. Liu Y, Wen J, Huang W. Exosomes in nasopharyngeal carcinoma. Clin Chim Acta. (2021) 523:355–64. doi: 10.1016/j.cca.2021.10.013
33. Vlaeminck-Guillem V. Exosomes and prostate cancer management. Semin Cancer Biol. (2022) 86:101–11. doi: 10.1016/j.semcancer.2021.08.004
34. Cui X, Fu Q, Wang X, Xia P, Cui X, Bai X, et al. Molecular mechanisms and clinical applications of exosomes in prostate cancer. biomark Res. (2022) 10:56. doi: 10.1186/s40364-022-00398-w
35. Hashimoto K, Ochi H, Sunamura S, Kosaka N, Mabuchi Y, Fukuda T, et al. Cancer-secreted hsa-miR-940 induces an osteoblastic phenotype in the bone metastatic microenvironment via targeting ARHGAP1 and FAM134A. Proc Natl Acad Sci U.S.A. (2018) 115:2204–9. doi: 10.1073/pnas.1717363115
36. Ye Y, Li SL, Ma YY, Diao YJ, Yang L, Su MQ, et al. Exosomal miR-141-3p regulates osteoblast activity to promote the osteoblastic metastasis of prostate cancer. Oncotarget. (2017) 8:94834–49. doi: 10.18632/oncotarget.22014
37. Xie H, Zhao J, Wan J, Zhao J, Wang Q, Yang X, et al. Long non−coding RNA AC245100. 4 promotes prostate cancer tumorigenesis via the microRNA−145−5p/RBBP5 axis. Oncol Rep. (2021) 45:619–29. doi: 10.3892/or.2020.7894
38. Hao SD, Ma JX, Liu Y, Liu PJ, Qin Y. Long non-coding TUG1 accelerates prostate cancer progression through regulating miR-128-3p/YES1 axis. Eur Rev Med Pharmacol Sci. (2020) 24:619–32. doi: 10.26355/eurrev_202001_20038
39. Lin C, Yang L. Long noncoding RNA in cancer: wiring signaling circuitry. Trends Cell Biol. (2018) 28:287–301. doi: 10.1016/j.tcb.2017.11.008
40. Chi Y, Wang D, Wang J, Yu W, Yang J. Long non-coding RNA in the pathogenesis of cancers. Cells. (2019) 8:1015. doi: 10.3390/cells8091015
41. Ashrafizadeh M, Rabiee N, Kumar AP, Sethi G, Zarrabi A, Wang Y. Long noncoding RNAs (lncRNAs) in pancreatic cancer progression. Drug Discovery Today. (2022) 27:2181–98. doi: 10.1016/j.drudis.2022.05.012
42. He Y, Du X, Chen M, Han L, Sun J. Novel insight into the functions of N(6)−methyladenosine modified lncRNAs in cancers (Review). Int J Oncol. (2022) 61:152. doi: 10.3892/ijo.2022.5442
43. Kumar S, Prajapati KS, Singh AK, Kushwaha PP, Shuaib M, Gupta S. Long non-coding RNA regulating androgen receptor signaling in breast and prostate cancer. Cancer Lett. (2021) 504:15–22. doi: 10.1016/j.canlet.2020.11.039
44. Zhang C, Yang Q, Li W, Kang Y, Zhou F, Chang D. Roles of circRNAs in prostate cancer: expression, mechanism, application and potential. Int J Biochem Cell Biol. (2021) 134:105968. doi: 10.1016/j.biocel.2021.105968
45. Xu H, Sun Y, You B, Huang CP, Ye D, Chang C. Androgen receptor reverses the oncometabolite R-2-hydroxyglutarate-induced prostate cancer cell invasion via suppressing the circRNA-51217/miRNA-646/TGFβ1/p-Smad2/3 signaling. Cancer Lett. (2020) 472:151–64. doi: 10.1016/j.canlet.2019.12.014
46. Feng Y, Yang Y, Zhao X, Fan Y, Zhou L, Rong J, et al. Circular RNA circ0005276 promotes the proliferation and migration of prostate cancer cells by interacting with FUS to transcriptionally activate XIAP. Cell Death Dis. (2019) 10:792. doi: 10.1038/s41419-019-2028-9
47. Guarnerio J, Bezzi M, Jeong JC, Paffenholz SV, Berry K, Naldini MM, et al. Oncogenic role of fusion-circRNAs derived from cancer-associated chromosomal translocations. Cell. (2016) 165:289–302. doi: 10.1016/j.cell.2016.03.020
48. Baena-Del Valle JA, Zheng Q, Esopi DM, Rubenstein M, Hubbard GK, Moncaliano MC, et al. MYC drives overexpression of telomerase RNA (hTR/TERC) in prostate cancer. J Pathol. (2018) 244:11–24. doi: 10.1002/path.4980
49. Sieron P, Hader C, Hatina J, Engers R, Wlazlinski A, Müller M, et al. DKC1 overexpression associated with prostate cancer progression. Br J Cancer. (2009) 101:1410–6. doi: 10.1038/sj.bjc.6605299
50. Folini M, Brambilla C, Villa R, Gandellini P, Vignati S, Paduano F, et al. Antisense oligonucleotide-mediated inhibition of hTERT, but not hTERC, induces rapid cell growth decline and apoptosis in the absence of telomere shortening in human prostate cancer cells. Eur J Cancer. (2005) 41:624–34. doi: 10.1016/j.ejca.2004.12.002
51. Shah V, Shah J. Recent trends in targeting miRNAs for cancer therapy. J Pharm Pharmacol. (2020) 72:1732–49. doi: 10.1111/jphp.13351
52. Petri BJ, Klinge CM. Regulation of breast cancer metastasis signaling by miRNAs. Cancer Metastasis Rev. (2020) 39:837–86. doi: 10.1007/s10555-020-09905-7
53. Wang JY, Chen LJ. The role of miRNAs in the invasion and metastasis of cervical cancer. Biosci Rep. (2019) 39:BSR20181377. doi: 10.1042/bsr20181377
54. Josson S, Gururajan M, Hu P, Shao C, Chu GY, Zhau HE, et al. miR-409-3p/-5p promotes tumorigenesis, epithelial-to-mesenchymal transition, and bone metastasis of human prostate cancer. Clin Cancer Res. (2014) 20:4636–46. doi: 10.1158/1078-0432.Ccr-14-0305
55. Chen WY, Liu SY, Chang YS, Yin JJ, Yeh HL, Mouhieddine TH, et al. MicroRNA-34a regulates WNT/TCF7 signaling and inhibits bone metastasis in Ras-activated prostate cancer. Oncotarget. (2015) 6:441–57. doi: 10.18632/oncotarget.2690
56. Chang YS, Chen WY, Yin JJ, Sheppard-Tillman H, Huang J, Liu YN. EGF Receptor promotes prostate cancer bone metastasis by downregulating miR-1 and activating TWIST1. Cancer Res. (2015) 75:3077–86. doi: 10.1158/0008-5472.Can-14-3380
57. Colden M, Dar AA, Saini S, Dahiya PV, Shahryari V, Yamamura S, et al. MicroRNA-466 inhibits tumor growth and bone metastasis in prostate cancer by direct regulation of osteogenic transcription factor RUNX2. Cell Death Dis. (2017) 8:e2572. doi: 10.1038/cddis.2017.15
58. Dai Y, Ren D, Yang Q, Cui Y, Guo W, Lai Y, et al. The TGF-β signalling negative regulator PICK1 represses prostate cancer metastasis to bone. Br J Cancer. (2017) 117:685–94. doi: 10.1038/bjc.2017.212
59. Huang S, Wa Q, Pan J, Peng X, Ren D, Huang Y, et al. Downregulation of miR-141-3p promotes bone metastasis via activating NF-κB signaling in prostate cancer. J Exp Clin Cancer Res. (2017) 36:173. doi: 10.1186/s13046-017-0645-7
60. Ren D, Yang Q, Dai Y, Guo W, Du H, Song L, et al. Oncogenic miR-210-3p promotes prostate cancer cell EMT and bone metastasis via NF-κB signaling pathway. Mol Cancer. (2017) 16:117. doi: 10.1186/s12943-017-0688-6
61. Huang S, Wa Q, Pan J, Peng X, Ren D, Li Q, et al. Transcriptional downregulation of miR-133b by REST promotes prostate cancer metastasis to bone via activating TGF-β signaling. Cell Death Dis. (2018) 9:779. doi: 10.1038/s41419-018-0807-3
62. Tang Y, Pan J, Huang S, Peng X, Zou X, Luo Y, et al. Downregulation of miR-133a-3p promotes prostate cancer bone metastasis via activating PI3K/AKT signaling. J Exp Clin Cancer Res. (2018) 37:160. doi: 10.1186/s13046-018-0813-4
63. Wa Q, Li L, Lin H, Peng X, Ren D, Huang Y, et al. Downregulation of miR−19a−3p promotes invasion, migration and bone metastasis via activating TGF−β signaling in prostate cancer. Oncol Rep. (2018) 39:81–90. doi: 10.3892/or.2017.6096
64. Dai Y, Wu Z, Lang C, Zhang X, He S, Yang Q, et al. Copy number gain of ZEB1 mediates a double-negative feedback loop with miR-33a-5p that regulates EMT and bone metastasis of prostate cancer dependent on TGF-β signaling. Theranostics. (2019) 9:6063–79. doi: 10.7150/thno.36735
65. Huang S, Zou C, Tang Y, Wa Q, Peng X, Chen X, et al. miR-582-3p and miR-582-5p suppress prostate cancer metastasis to bone by repressing TGF-β signaling. Mol Ther Nucleic Acids. (2019) 16:91–104. doi: 10.1016/j.omtn.2019.01.004
66. Wa Q, Huang S, Pan J, Tang Y, He S, Fu X, et al. miR-204-5p represses bone metastasis via inactivating NF-κB signaling in prostate cancer. Mol Ther Nucleic Acids. (2019) 18:567–79. doi: 10.1016/j.omtn.2019.09.008
67. Wa Q, Zou C, Lin Z, Huang S, Peng X, Yang C, et al. Ectopic expression of miR-532-3p suppresses bone metastasis of prostate cancer cells via inactivating NF-κB signaling. Mol Ther Oncolytics. (2020) 17:267–77. doi: 10.1016/j.omto.2020.03.024
68. Zeng F, Zhao C, Wang R, Ren L, Qiu H, Zou Z, et al. Antagonizing exosomal miR-18a-5p derived from prostate cancer cells ameliorates metastasis-induced osteoblastic lesions by targeting Hist1h2bc and activating Wnt/β-catenin pathway. Genes Dis. (2023) 10:1626–40. doi: 10.1016/j.gendis.2022.06.007
69. Liu Y, Yang C, Chen S, Liu W, Liang J, He S, et al. Cancer-derived exosomal miR-375 targets DIP2C and promotes osteoblastic metastasis and prostate cancer progression by regulating the Wnt signaling pathway. Cancer Gene Ther. (2023) 30:437–49. doi: 10.1038/s41417-022-00563-1
70. Guo H, Zhao J, Li X, Sun F, Qin Y, Yang X, et al. Identification of miR-1-3p, miR-143-3p and miR-145-5p association with bone metastasis of Gleason 3 + 4 prostate cancer and involvement of LASP1 regulation. Mol Cell Probes. (2023) 68:10190. doi: 10.1016/j.mcp.2023.101901
71. Wang J, Du X, Wang X, Xiao H, Jing N, Xue W, et al. Tumor-derived miR-378a-3p-containing extracellular vesicles promote osteolysis by activating the Dyrk1a/Nfatc1/Angptl2 axis for bone metastasis. Cancer Lett. (2022) 526:76–90. doi: 10.1016/j.canlet.2021.11.017
72. Luo B, Yuan Y, Zhu Y, Liang S, Dong R, Hou J, et al. microRNA-145-5p inhibits prostate cancer bone metastatic by modulating the epithelial-mesenchymal transition. Front Oncol. (2022) 12:988794. doi: 10.3389/fonc.2022.988794
73. Thomas-Jardin SE, Dahl H, Nawas AF, Bautista M, Delk NA. NF-κB signaling promotes castration-resistant prostate cancer initiation and progression. Pharmacol Ther. (2020) 211:107538. doi: 10.1016/j.pharmthera.2020.107538
74. Lv Z, Li W, Wei X. S100A9 promotes prostate cancer cell invasion by activating TLR4/NF-κB/integrin β1/FAK signaling. Onco Targets Ther. (2020) 13:6443–52. doi: 10.2147/ott.S192250
75. Lian Z, Chang T, Ma S, Li J, Zhang H, Wang X, et al. MiR-96-5p induced NDRG1 deficiency promotes prostate cancer migration and invasion through regulating the NF-κB signaling pathway. Cancer biomark. (2022) 35:83–98. doi: 10.3233/cbm-210072
76. Ghafouri-Fard S, Abak A, Fattahi F, Hussen BM, Bahroudi Z, Shoorei H, et al. The interaction between miRNAs/lncRNAs and nuclear factor-κB (NF-κB) in human disorders. BioMed Pharmacother. (2021) 138:111519. doi: 10.1016/j.biopha.2021.111519
77. Wang C, Chen Q, Xu H. Wnt/β-catenin signal transduction pathway in prostate cancer and associated drug resistance. Discovery Oncol. (2021) 12:40. doi: 10.1007/s12672-021-00433-6
78. Esposito M, Fang C, Cook KC, Park N, Wei Y, Spadazzi C, et al. TGF-β-induced DACT1 biomolecular condensates repress Wnt signalling to promote bone metastasis. Nat Cell Biol. (2021) 23:257–67. doi: 10.1038/s41556-021-00641-w
79. Chen H, Zhou L, Wu X, Li R, Wen J, Sha J, et al. The PI3K/AKT pathway in the pathogenesis of prostate cancer. Front Biosci (Landmark Ed). (2016) 21:1084–91. doi: 10.2741/4443
80. Zhu S, Jiao W, Xu Y, Hou L, Li H, Shao J, et al. Palmitic acid inhibits prostate cancer cell proliferation and metastasis by suppressing the PI3K/Akt pathway. Life Sci. (2021) 286:120046. doi: 10.1016/j.lfs.2021.120046
81. Ebrahimi N, Parkhideh S, Samizade S, Esfahani AN, Samsami S, Yazdani E, et al. Crosstalk between lncRNAs in the apoptotic pathway and therapeutic targets in cancer. Cytokine Growth Factor Rev. (2022) 65:61–74. doi: 10.1016/j.cytogfr.2022.04.003
82. Rathinasamy B, Velmurugan BK. Role of lncRNAs in the cancer development and progression and their regulation by various phytochemicals. BioMed Pharmacother. (2018) 102:242–8. doi: 10.1016/j.biopha.2018.03.077
83. Lang C, Dai Y, Wu Z, Yang Q, He S, Zhang X, et al. SMAD3/SP1 complex-mediated constitutive active loop between lncRNA PCAT7 and TGF-β signaling promotes prostate cancer bone metastasis. Mol Oncol. (2020) 14:808–28. doi: 10.1002/1878-0261.12634
84. Hao H, Chen H, Xie L, Liu H, Wang D. LncRNA KCNQ1OT1 promotes proliferation, invasion and metastasis of prostate cancer by regulating miR-211-5p/CHI3L1 pathway. Onco Targets Ther. (2021) 14:1659–71. doi: 10.2147/ott.S288785
85. Hu CY, Chen J, Qin XH, You P, Ma J, Zhang J, et al. Long non-coding RNA NORAD promotes the prostate cancer cell extracellular vesicle release via microRNA-541-3p-regulated PKM2 to induce bone metastasis of prostate cancer. J Exp Clin Cancer Res. (2021) 40:98. doi: 10.1186/s13046-021-01891-0
86. Misawa A, Kondo Y, Takei H, Takizawa T. Long noncoding RNA HOXA11-AS and transcription factor HOXB13 modulate the expression of bone metastasis-related genes in prostate cancer. Genes (Basel). (2021) 12:182. doi: 10.3390/genes12020182
87. Mo C, Huang B, Zhuang J, Jiang S, Guo S, Mao X. LncRNA nuclear-enriched abundant transcript 1 shuttled by prostate cancer cells-secreted exosomes initiates osteoblastic phenotypes in the bone metastatic microenvironment via miR-205-5p/runt-related transcription factor 2/splicing factor proline- and glutamine-rich/polypyrimidine tract-binding protein 2 axis. Clin Transl Med. (2021) 11:e493. doi: 10.1002/ctm2.493
88. Zheng Y, Qi F, Li L, Yu B, Cheng Y, Ge M, et al. LncNAP1L6 activates MMP pathway by stabilizing the m6A-modified NAP1L2 to promote Malignant progression in prostate cancer. Cancer Gene Ther. (2023) 30:209–18. doi: 10.1038/s41417-022-00537-3
89. Wang M, Yin C, Wu Z, Wang X, Lin Q, Jiang X, et al. The long transcript of lncRNA TMPO-AS1 promotes bone metastases of prostate cancer by regulating the CSNK2A1/DDX3X complex in Wnt/β-catenin signaling. Cell Death Discovery. (2023) 9:287. doi: 10.1038/s41420-023-01585-w
90. Liao S, Fang X, Zhou K, Zhao T, Ji L, Zhang W, et al. LINC00482 sponged miR-2467-3p to promote bone metastasis of prostate cancer through activating Wnt/β-catenin signaling pathway. J Bone Oncol. (2023) 41:100494. doi: 10.1016/j.jbo.2023.100494
91. Xi X, Hu Z, Wu Q, Hu K, Cao Z, Zhou J, et al. High expression of small nucleolar RNA host gene 3 predicts poor prognosis and promotes bone metastasis in prostate cancer by activating transforming growth factor-beta signaling. Bioengineered. (2022) 13:1895–907. doi: 10.1080/21655979.2021.2020393
92. Qiu X, Chen J. LSINCT5: A novel lncRNA in cancers. Curr Med Chem. (2023) 30:4409–20. doi: 10.2174/0929867330666230123144602
93. Farzaneh M, Nasrolahi A, Ghaedrahmati F, Masoodi T, Najafi S, Sheykhi-Sabzehpoush M, et al. Potential roles of lncRNA-XIST/miRNAs/mRNAs in human cancer cells. Clin Transl Oncol. (2023) 25:2015–42. doi: 10.1007/s12094-023-03110-y
94. Rahmani F, Safavi P, Fathollahpour A, Tanhaye Kalate Sabz F, Tajzadeh P, Arefnezhad M, et al. The interplay between non-coding RNAs and Wnt/ß-catenin signaling pathway in urinary tract cancers: from tumorigenesis to metastasis. Excli J. (2022) 21:1273–84. doi: 10.17179/excli2022-5348
95. Tang PC, Zhang YY, Li JS, Chan MK, Chen J, Tang Y, et al. LncRNA-dependent mechanisms of transforming growth factor-β: from tissue fibrosis to cancer progression. Noncoding RNA. (2022) 8:36. doi: 10.3390/ncrna8030036
96. Ding L, Wang R, Zheng Q, Shen D, Wang H, Lu Z, et al. circPDE5A regulates prostate cancer metastasis via controlling WTAP-dependent N6-methyladenisine methylation of EIF3C mRNA. J Exp Clin Cancer Res. (2022) 41:187. doi: 10.1186/s13046-022-02391-5
97. Zhang Y, Liu F, Feng Y, Xu X, Wang Y, Zhu S, et al. CircRNA circ_0006156 inhibits the metastasis of prostate cancer by blocking the ubiquitination of S100A9. Cancer Gene Ther. (2022) 29:1731–41. doi: 10.1038/s41417-022-00492-z
98. Dai X, Chen X, Chen W, Ou Y, Chen Y, Wu S, et al. CircDHRS3 inhibits prostate cancer cell proliferation and metastasis through the circDHRS3/miR-421/MEIS2 axis. Epigenetics. (2023) 18:2178802. doi: 10.1080/15592294.2023.2178802
99. Zheng Y, Chen CJ, Lin ZY, Li JX, Liu J, Lin FJ, et al. Circ_KATNAL1 regulates prostate cancer cell growth and invasiveness through the miR-145-3p/WISP1 pathway. Biochem Cell Biol. (2020) 98:396–404. doi: 10.1139/bcb-2019-0211
100. Ding Y, Wang M, Yang J. Circular RNA midline-1 (circMID1) promotes proliferation, migration, invasion and glycolysis in prostate cancer. Bioengineered. (2022) 13:6293–308. doi: 10.1080/21655979.2022.2037367
101. Lv D, Cen S, Yang S, Zou Z, Zhong J, Pan Z, et al. Hsa_circ_0063329 inhibits prostate cancer growth and metastasis by modulating the miR-605-5p/tgif2 axis. Cell Cycle. (2023) 22:1101–15. doi: 10.1080/15384101.2023.2174658
102. He T, Tao W, Zhang LL, Wang BY, Li K, Lu HM, et al. CircSCAF8 promotes growth and metastasis of prostate cancer through the circSCAF8-miR-140-3p/miR-335-LIF pathway. Cell Death Dis. (2022) 13:517. doi: 10.1038/s41419-022-04913-7
103. Feng H, Deng Z, Peng W, Wei X, Liu J, Wang T. Circular RNA EPHA3 suppresses progression and metastasis in prostate cancer through the miR-513a-3p/BMP2 axis. J Transl Med. (2023) 21:288. doi: 10.1186/s12967-023-04132-4
104. Zheng Y, Li JX, Chen CJ, Lin ZY, Liu JX, Lin FJ. Extracellular vesicle-derived circ_SLC19A1 promotes prostate cancer cell growth and invasion through the miR-497/septin 2 pathway. Cell Biol Int. (2020) 44:1037–45. doi: 10.1002/cbin.11303
105. Wang P, Zhang L, Yin S, Xu Y, Tai S, Zhang LI, et al. hsa_circ_0062019 promotes the proliferation, migration, and invasion of prostate cancer cells via the miR-195-5p/HMGA2 axis. Acta Biochim Biophys Sin (Shanghai). (2021) 53:815–22. doi: 10.1093/abbs/gmab058
106. Dong C, Fan B, Ren Z, Liu B, Wang Y. CircSMARCA5 facilitates the progression of prostate cancer through miR-432/PDCD10 axis. Cancer Biother Radiopharm. (2021) 36:70–83. doi: 10.1089/cbr.2019.3490
107. Pencheva N, Tavazoie SF. Control of metastatic progression by microRNA regulatory networks. Nat Cell Biol. (2013) 15:546–54. doi: 10.1038/ncb2769
108. Takeshita F, Patrawala L, Osaki M, Takahashi RU, Yamamoto Y, Kosaka N, et al. Systemic delivery of synthetic microRNA-16 inhibits the growth of metastatic prostate tumors via downregulation of multiple cell-cycle genes. Mol Ther. (2010) 18:181–7. doi: 10.1038/mt.2009.207
109. Hong DS, Kang YK, Borad M, Sachdev J, Ejadi S, Lim HY, et al. Phase 1 study of MRX34, a liposomal miR-34a mimic, in patients with advanced solid tumours. Br J Cancer. (2020) 122:1630–7. doi: 10.1038/s41416-020-0802-1
110. van Zandwijk N, Pavlakis N, Kao SC, Linton A, Boyer MJ, Clarke S, et al. Safety and activity of microRNA-loaded minicells in patients with recurrent Malignant pleural mesothelioma: a first-in-man, phase 1, open-label, dose-escalation study. Lancet Oncol. (2017) 18:1386–96. doi: 10.1016/s1470-2045(17)30621-6
111. Xu YH, Deng JL, Wang G, Zhu YS. Long non-coding RNAs in prostate cancer: Functional roles and clinical implications. Cancer Lett. (2019) 464:37–55. doi: 10.1016/j.canlet.2019.08.010
112. Franz A, Ralla B, Weickmann S, Jung M, Rochow H, Stephan C, et al. Circular RNAs in clear cell renal cell carcinoma: their microarray-based identification, analytical validation, and potential use in a clinico-genomic model to improve prognostic accuracy. Cancers (Basel). (2019) 11:1473. doi: 10.3390/cancers11101473
113. Li H, Wang Q. Chimeric RNAs and their implication in prostate cancer. Cancer Pathogenesis Ther. (2023) 1:216–9. doi: 10.1016/j.cpt.2023.04.003
Keywords: prostate cancer, bone metastasis, non-coding RNA, micro RNA, long non-coding RNA, circular RNA, signaling pathway, targeted therapy
Citation: Sang H, Li L, Zhao Q, Liu Y, Hu J, Niu P, Hao Z and Chai K (2024) The regulatory process and practical significance of non-coding RNA in the dissemination of prostate cancer to the skeletal system. Front. Oncol. 14:1358422. doi: 10.3389/fonc.2024.1358422
Received: 19 December 2023; Accepted: 12 March 2024;
Published: 21 March 2024.
Edited by:
Alvin Liu, University of Washington, United StatesReviewed by:
Qiong Wang, Southern Medical University, ChinaXiaolong Wang, Temple University, United States
Copyright © 2024 Sang, Li, Zhao, Liu, Hu, Niu, Hao and Chai. This is an open-access article distributed under the terms of the Creative Commons Attribution License (CC BY). The use, distribution or reproduction in other forums is permitted, provided the original author(s) and the copyright owner(s) are credited and that the original publication in this journal is cited, in accordance with accepted academic practice. No use, distribution or reproduction is permitted which does not comply with these terms.
*Correspondence: Keqiang Chai, MzEyMzA2Mzc5QHFxLmNvbQ==; Zhenming Hao, NTA0NjM2NDRAcXEuY29t
†These authors have contributed equally to this work