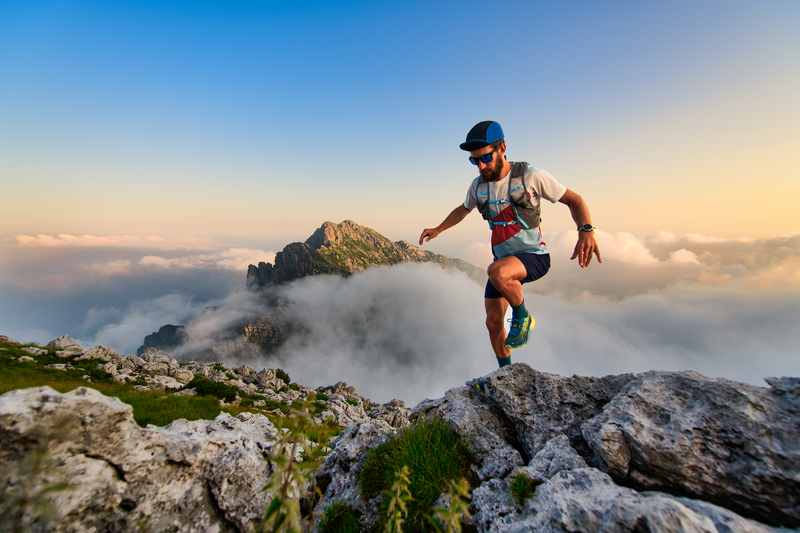
94% of researchers rate our articles as excellent or good
Learn more about the work of our research integrity team to safeguard the quality of each article we publish.
Find out more
REVIEW article
Front. Oncol. , 29 February 2024
Sec. Head and Neck Cancer
Volume 14 - 2024 | https://doi.org/10.3389/fonc.2024.1346413
Nasopharyngeal carcinoma (NPC) is a malignant tumor originating from the nasopharyngeal epithelial cells. Common treatment methods for NPC include radiotherapy, chemotherapy, and surgical intervention. Despite these approaches, the prognosis for NPC remains poor due to treatment resistance and recurrence. Hence, there is a crucial need for more comprehensive research into the mechanisms underlying treatment resistance in NPC. Long non coding RNAs (LncRNAs) are elongated RNA molecules that do not encode proteins. They paly significant roles in various biological processes within tumors, such as chemotherapy resistance, radiation resistance, and tumor recurrence. Recent studies have increasingly unveiled the mechanisms through which LncRNAs contribute to treatment resistance in NPC. Consequently, LncRNAs hold promise as potential biomarkers and therapeutic targets for diagnosing NPC. This review provides an overview of the role of LncRNAs in NPC treatment resistance and explores their potential as therapeutic targets for managing NPC.
Nasopharyngeal carcinoma (NPC) is a malignant tumor originating in the epithelial cells of the nasopharynx (1). Although NPC has been linked to various factors, such as viral infections, environmental influences, and genetics, its precise pathogenesis remains unclear. At present, the latest research suggests that NPC is not caused by a single factor, but a unified disease of ecology and evolution, and cancer cells and tumor microenvironment (TME) constitute a complex pathological ecosystem (2). The global incidence of NPC varies significantly, with higher rates observed in high-risk regions. In areas such as South China and Hong Kong, the incidence can reach 25-50 cases per 100,000 people, while in the Western world, NPC relatively rarely occurs, with an annual incidence of less than one in 100,000 people (3, 4). In low-risk groups, NPC incidence is positively correlated with age. However, in medium and high-risk groups, the peak incidence of NPC occurs among the youth and those aged 45-54 years, with a decline in NPC cases among older individuals (3). Moreover, men are 2-3 times more likely than women to develop NPC (1). Early-stage NPC often presents nonspecific symptoms, leading to potential misdiagnosis. Many NPCs were not detected until the intermediate and late stages. Because of its hidden anatomical location and high sensitivity to radiotherapy, surgical treatment for NPC is not the primary choice; instead, radiotherapy remains the mainstay. Although most patients with NPC undergo chemoradiotherapy, resistance to this treatment is common, leading to very high recurrence and metastasis rates and low overall survival (OS). Approximately 10% of patients with NPC experience recurrence, while 10%-20% develop distant metastasis (5). Globally, the OS rate for patients with NPC is less than 80%, with a 5-year OS rate of 51.5% for stage III NPC and as low as 32.4% for stage IVA NPC (6). Consequently, due to the late detection, high recurrence rates, and unsatisfactory treatment outcomes, there is an urgent need for extensive research into NPC pathogenesis to improve diagnosis and treatment.
Long noncoding RNAs (LncRNAs) are transcripts exceeding 200 nucleotides that cannot encode proteins (7). They play a very important role in physiological and pathological regulation (8) (Figure 1). Because LncRNAs lack protein-coding ability, it was believed that they die not have a role in the physiological and pathological processes of the body, thus receiving less attention from researchers (9). Historically overlooked, recent years have seen a shift in understanding their significance in various physiological and pathological processes, notably in tumor initiation and progression. LncRNAs have shown substantial involvement in chemoradiotherapy resistance in NPC. For instance, LHFPL3 is implicated in regulating NPC radiotherapy resistance by modulating the expression of the target gene HOXA6 through sponge miR-143-5p, thereby regulating NPC sensitivity to radiotherapy; this suggests that LHFPL3 may serve as a potential target in NPC treatment (10). NHG16 is highly expressed in NPC cells, and sponge miR-31-5p is believed to enhance the expression of the target gene SFN, thereby enhancing the radioresistance of NPC (11). However, the exact function of NHG16 is not fully understood. KCNQ1OT1, another LncRNA, significantly influences NPC drug resistance. KCNQ10T1 knockout promotes chemotherapy sensitivity of NPC cells through the miR-454/USP47 axis, offering a potential solution to chemotherapy resistance in patients with NPC (12). While the precise mechanism of LncRNA in mediating NPC chemoradiotherapy resistance remains unclear, current studies highlight its potential as a therapeutic target for NPC chemoradiotherapy resistance. This review introduces the role of LncRNAs in NPC chemoradiotherapy resistance and summarizes LncRNAs associated with NPC resistance (Figure 2) (Table 1).
Platinum drugs are cytotoxic drugs used as first-line chemotherapeutic agents for many cancers. Their mode of action involves binding to DNA after hydrolyzing one or two chloride ions in the body, thereby impeding replication and transcription and, consequently, the proliferation of cancer cells (35, 36). However, prolonged usage of platinum-based drugs may lead to the development of resistance, which can compromise treatment efficacy. The mechanisms underlying drug resistance to platinum drugs in malignant tumors include several facets: First, overexpression of drug efflux transporters diminishes intracellular drug accumulation. Second, increased DNA repair diminishes sensitivity to chemotherapy. Third, intracellular drug inactivation also contributes to this resistance (37, 38).Recent studies have emphasized the significant role of LncRNA in tumor chemotherapy resistance (13). For instance, in NPC tissues, upregulated expression of LncRNA DLEU1 enhances the expression of BIRC6 through the sponge miR-381-3p, thereby promoting cisplatin resistance in NPC cells (13). Competitive endogenous RNA (ceRNA) is a novel mechanism for regulating gene expression. LncRNAs with the same miRNA response elements competitively bind to the same miRNA, thus diminishing its inhibition of target gene mRNA and modulating NPC development (39). HOTAIR, highly expressed in cisplatin-resistant cells, regulates target gene expression through ceRNA. Knockdown of HOTAIR can reduce cell viability, expression, migration and invasion of chemical resistance related proteins, increase cell apoptosis, reduces the expression of target gene SOX4 through miR-106a-5p, heightening the chemosensitivity of NPC cells to cisplatin. This suggests that HOTAIR is a potential therapeutic target in NPC therapy (14). LINC00346, located on chromosome 13q34, has shown abnormal expression in various diseases including NPC. Its aberrant expression significantly correlates with tumor proliferation, invasion, and drug resistance, establishing it as a potential biomarker for disease diagnosis and treatment (40). There is no doubt that chemotherapy sensitivity of malignant tumor cells is closely related to cell proliferation and apoptosis (41). XIST, an important mammalian LncRNA derived from the XIST gene, upregulated in NPC cisplatin cells, decreases the expression of programmed cell death 4 (PDCD4) and Fas ligand (Fas-L), thereby increasing drug resistance and reducing chemosensitivity in NPC cells (15, 16). ROR, an oncogenic LncRNA located on chromosome 18, is overexpressed in NPC. Studies have shown that the enrichment of ROR is related to the chemotherapy resistance of NPC. P53 is one of the important tumor suppressors in the body. ROR inhibits the P53 signaling pathway, which may elucidate the mechanism of NPC resistance to chemotherapy, indicating the pivotal role of ROR in NPC progression (17–19). It could serve as a potential therapeutic target to mitigate chemotherapy resistance in NPC. NEAT1 up-regulates the expression of Rsf-1 through let-7a-5p, thereby enhancing the resistance of NPC cells to cisplatin. In addition, NEAT1 can also regulate the resistance of nasopharyngeal carcinoma to cisplatin through the regulation of Ras-MAPK signaling pathway (20). Epithelial-mesenchymal transition (EMT) is a complex process that can regulate the changes of cell morphology and function during embryogenesis and tissue development, and tumor cells can obtain drug resistance through EMT (42, 43). MAGI2-AS3 can regulate the expression of target genes GDPD5 and SEC61A1 through sponge miR-2185p, thereby regulating the proliferation, migration and EMT of NPC cells, as well as the cisplatin resistance of NPC (22). HOXA11-AS is pivotal in various cancers such as lung adenocarcinoma (44), oral squamous cell carcinoma (45), gastric cancer (46), prostate cancer (47), and breast cancer (48) and is closely related to NPC. HOXA11-AS is increasingly expressed in cisplatin-resistant NPC cells and knocking down HOXA11-AS could increase the expression of miR-454-3p and inhibit the expression of target gene c-Met, thereby increasing the sensitivity of the tumor cells to cisplatin (23). Knocking out HOXA11-AS can also decrease the expression of the target gene PBX3 through sponge miR-98, further increasing the sensitivity of NPC cells to cisplatin. These findings highlight a potential target for treating drug-resistant NPC (24).
Paclitaxel, derived from the Pacific redwood, is the first approved herb-derived chemotherapeutic drug (49, 50), and is widely used in the treatment of many types of cancer. Paclitaxel binds to microtubules, disrupting microtubule protein depolymerization. This interference inhibits mitosis, ultimately leading to the death of cancer cells (51, 52). Although paclitaxel is effective in treating malignant tumors, resistance to paclitaxel remains a significant impediment to its effectiveness. The exact mechanisms underpinning paclitaxel resistance are presently unclear. However, certain studies propose its association with altered microdynamics, changes in micro-protein expression, and modifications in tumor- suppressing signaling pathways (51). H19, known as the imprinted maternally expressed transcript, represents one of the earliest identified LncRNAs. It is located in the 11p15.5 chromosomal region and is encoded by the H19 gene (53, 54). Accumulating evidence underscores the pivotal role of H19 in the progression and drug resistance of malignant tumors, rendering it a focal point of research. Studies have identified its resistance to various anticancer drugs such as paclitaxel, erlotinib, methotrexate, and 5-fluorouracil (55–58). Current research extensively demonstrates the substantial involvement of H19 in chemotherapy resistance in malignant tumors. It could potentially serve as a target for treating NPC chemotherapy resistance. For instance, increased H19 expression in paclitaxel-resistant cells, along with H19 silencing combined with paclitaxel, has shown promise in inhibiting NPC progression and enhancing chemotherapy sensitivity (54, 59). Moreover, a sequencing- based construction of LncRNA differential expression profiles associated with NPC paclitaxel resistance was conducted. LncRNAs exhibiting similar expression trends as predicted were further assessed using qRT-PCR. Among NPC cells, n375709 exhibited the highest expression level; its suppression was found to augment the sensitivity of NPC cells to paclitaxel (60). CCAT1, or colon cancer-associated transcript 1, also known as cancer-associated region LncRNA-5 or CCAT1-S, localizes to chromosome 8q24.2 and plays a critical role in biological processes such as invasion and drug resistance (61). In paclitaxel-resistant NPC tissues, CCAT1 is notably overexpressed, indicating its involvement in paclitaxel resistance. Investigations revealed that silencing CCAT1 can inhibit the target gene CPEB2 through sponge miR-181a, thereby enhancing the sensitivity of NPC cells to paclitaxel. This suggests that CCAT1 holds potential as a therapeutic target for NPC (25). At present, there have been many studies on the mechanism of IncRNA in drug resistance of malignant tumors, which is not only related to apoptosis, autophagy, control of carcinogenic signaling pathway, promotion of EMT, but also related to the regulation of cancer stem cells (CSCs) (62). CSCs play an important role in the progression and drug resistance of malignant tumors, and are a small subgroup of tumor cells. It has the ability of self-renewal, increasing DNA repair, body maintenance, drug efflux and redox (63). It has been confirmed that LncRNA is related to CSCs, and chemoradiotherapy can promote the unique self-renewal ability of CSCs through the production of cytokines and DNA repair mechanisms. For example, H19 has been found to participate in the regulation of CSCs, thereby regulating the sensitivity to chemotherapy (64, 65).
Considering the specific anatomy of NPC and its sensitivity to ionizing radiation, the primary treatment for NPC is radiotherapy (33). Resistance to radiation stands as a significant contributor to the failure of NPC treatment (66). The core of radiotherapy lies in causing DNA damage, potentially inducing cell apoptosis through radiation exposure (67). However, radiotherapy might trigger mechanisms that repair DNA damage, ultimately bolstering resistance to this form of treatment (68). Recent evidence increasingly supports the pivotal role of LncRNA in NPC radioresistance, suggesting LncRNA as a potential target for enhancing radiotherapy sensitivity and improving the 5-year survival rate of patients with NPC. The mechanism of LncRNA in radiotherapy resistance is similar to that in chemotherapy resistance. For instance, PVT1, encoded by the human PVT1 gene on chromosome 8q24.21, exhibits high expression in NPC tissues. PVT1 is reported to be correlated with radiation resistance and prognosis. Knocking out PVT1 can reduce PIK3CA expression by sequestering miR-515-5p, inhibit the proliferation of nasopharyngeal carcinoma cells, resist radiation, and promote cell apoptosis, so as to improve the radiotherapy sensitivity and prognosis of patients with NPC (26, 27). Additionally, decreased LncRNA levels post-radiotherapy activate cysteinyl aspartate–specific proteinases (caspase), initiating a cascade that triggers caspase 9, caspase 7, and PARP. The binding of caspase 7 and PARP induces apoptosis (69). PVT1, however, inhibits caspase 9, caspase 7, and PARP, impeding apoptosis, thus diminishing NPC sensitivity to radiotherapy and fortifying its resistance (26).Moreover, the downregulation of LINC01140 in NPC cells is closely related to NPC radiation resistance, cancer cell proliferation and apoptosis. It regulates ZNF621 expression by competitively binding miR-452-5p, thereby influencing NPC cell radiosensitivity. Hence, LINC01140 is a potential therapeutic target for NPC (28). Linc00312 exhibits dysregulation and reduced expression in NPC, linked to its role in NPC progression, drug resistance, short-term efficacy, and OS (70). Upregulating Linc00312 enhances NPC sensitivity to radiotherapy by inhibiting radiation-induced signal transduction pathways and DNA damage repair–related protein expression (29, 30). Aberrant expression of PTPRG-AS1, observed in various malignant tumors, including NPC, demonstrates its effect on radiosensitivity. For example, PTPRG-AS1, as a ceRNA of miR-124-3p, regulates the LHX2 target gene, affects the proliferation and apoptosis of NPC cells, and regulates the radiotherapy sensitivity of NPC cells (31). LncRNA can also affect the viability of malignant tumor cells. Furthermore, PTPRG-AS1 binds specifically to miR-194-3p, thereby affecting the target gene PRC1, regulating the activity and apoptosis of NPC cells, and regulating the sensitivity of nasopharyngeal carcinoma cells to radiotherapy (32). CASC19, another noteworthy LncRNA encoded by chromosome 8q24.21, shows high expression in various malignant tumors, including NPC. It participates in proliferation, radioresistance, invasion, and other processes, signifying its potential as a therapeutic target and biomarker for NPC (71). CASC19 expression is closely related to the resistance of NPC cells to radiotherapy. It regulates NPC sensitivity to radiotherapy by modulating not only AMPK-mTOR and blocking autophagy (33) but also FKBP5 expression, acting as a molecular sponge for miR-340-3p, thereby influencing radiation resistance (34). Because of its inherent resistance to radiation, NPC tends to relapse and metastasize distantly, resulting in a poor prognosis for patients (72). Thus, delving deeper into the molecular mechanisms and actively seeking potential targets for NPC treatment remains an urgent need.
NPC is one of the most prevalent human tumors, particularly among head and neck tumors. Despite its prevalence, the exact causes and mechanisms triggering NPC remain unclear. Radiotherapy combined with chemotherapy is the primary treatment for NPC. However, while this approach exhibits some efficacy against NPC, the 5-year OS rate for patients with NPC remains notably low due to drug resistance. Additionally, the recurrence and metastasis rates remain considerably high (68). To improve the survival rates and tackle drug resistance and recurrence in NPC, there is an urgent need for further exploration into NPC treatment methods. The role of exosomes in NPC has been a focus of ongoing research. Exosomes, which are tiny membrane vesicles ranging from 40 to 100 nm in diameter, are released when various cells fuse with the plasma membrane. Their contents comprise lipids, nucleic acids, and proteins (73, 74). These exosomes are extensively present in bodily fluids such as blood, saliva, cerebrospinal fluid, and urine, showcasing high biological stability, compatibility, and availability. They play crucial roles in signal transduction, immune regulation, and targeted delivery, among other significant functions (75–80). Exosomes derived from gamma-delta-T cells can counteract the radioresistance observed in NPC stem-like cells. Furthermore, when radiotherapy coincides with exosomes derived from gamma-delta-T cells, it exhibits control over NPC (81). Moreover, the upregulated expression of DDX53 in paclitaxel resistant NPC cells shows a correlation with NPC sensitivity to paclitaxel. DDX53 can be transferred through exosomes, thereby regulating the expression of MDR1 and subsequently influencing NPC sensitivity to paclitaxel (82). In summary, this study offers potential insights into NPC treatment and identifies possible therapeutic targets. Exosomes play a pivotal role in NPC by transmitting crucial information to target cells. Additionally, they affect the tumor microenvironment, contributing to NPC resistance against radiotherapy and chemotherapy. This suggests that exosomes might serve as potential biomarkers in NPC.
Immunotherapy is a vital approach for treating malignant tumors, as it fortifies the immune system against them (83). In the context of NPC, immunotherapy includes immune checkpoint inhibitors (ICIs), adoptive cellular immunotherapy (ACT), and tumor vaccines (84). In 2021, China approved the use of programmed death-1/programmed death-ligand 1 (PD-1/PD-L1) blocking ICIs for NPC treatment (85). When the T-cell receptor (TCR) signaling is activated, PD-1 is induced on the T cell, impairing their function. PD-1 then binds to the PD-L1 ligand on cell membranes, facilitating immune evasion and tumor development (83). PD-1 inhibitors block the PD-L1 and PD-1 binding on T-cell surfaces, thereby inhibiting NPC progression. PD-L1 carried by NPC cell–derived exosomes is an immune-related protein that binds to PD-1 on the surface of CD8+T cells, diminishing immune cell activity, fostering NPC immune escape, and advancing NPC occurrence and progression (86). Hyperthermia’s ability to regulate immune responses, bolster radiation resistance, eliminate tumors through heat, impede NPC metastasis and recurrence, and in conjunction with ICIs, elevate 5-year survival rates in patients with NPC (87). ACT employs a patient’s T cells and other structures to combat malignant tumors (88). This encompasses tumor-infiltrating lymphocytes, engineered TCRs, chimeric antigen receptor T cells, and natural killer cell therapies (89, 90). Biologically active immune effector cells are isolated from the patient, multiplied significantly, enhanced in cytotoxic function, or transformed into antiviral cells. Subsequently, these cells are reintroduced into the patient to exert antitumor effects (89). A retrospective analysis involving 38 patients with NPC in a Phase II trial showcased a higher overall response rate when chemotherapy with gemcitabine and carboplatin was combined with the adoptive transfer of six autoamplified Epstein-Barr virus–specific T cells than chemotherapy alone for NPC (91). Moreover, NPC vaccines substantially decreased NPC incidence (92). Glycoproteins on the surface of Epstein-Barr virus cells, such as gp350, gH/gL, and gp42, among others, can be used as vaccine targets. Notably, gp350 is distributed abundantly and holds promise as the most potential vaccine immunogen (93). gp350 has long been acknowledged as an Epstein-Barr virus vaccine candidate for both nonhuman primates and humans (94). A prospective cohort analysis underlines the role of Epstein-Barr virus–neutralizing antibodies, glycoprotein antibodies, and anti-EBNA1 IgA in reducing NPC risk. High levels of anti-GP350 antibodies and B- cell–neutralizing antibodies inhibit infection, suggesting their potential in vaccine development to curtail NPC prevalence (95). Epstein-Barr virus–encoded latent membrane protein 2 (LMP2) antigen can be used as a therapeutic target for NPC. The development of lipid-based LMP2-mRNA (mLMP2) vaccines efficiently expresses LMP2 for NPC immunotherapy (96).
Increasing evidence highlights the dysregulation of LncRNAs in NPC, closely linking them to tumor progression (97). This study summarizes key LncRNAs significant in NPC (Table 1). Understanding the mechanisms and biology of LncRNAs in NPC suggests their potential as diagnostic and therapeutic targets. For instance, SNHG14, highly expressed in NPC cells, interacts with miR-5590-3p and elevates the expression of ZEB1, leading to increased programmed cell death receptor-1 (PD-L1) expression and promoting NPC’s epithelial-mesenchymal transition (EMT). Yet, research indicates that nano-coated si-SNHG14 downregulates PD-L1 expression, diminishes EMT, and impedes NPC progression, offering a new potential target for NPC immunotherapy (98). To enhance the efficacy of chemoradiotherapy for NPC, RGD-targeted platinum-based nanoparticles (RGD-PtNPs, termed RPNs) have been developed. RPNs, through RGD, are absorbed by NPC cells, augmenting cisplatin’s effect. Additionally, RGD nanoparticles bind to RGD receptors in cancer cells, effectively targeting NPC (99, 100). Targeted ligands, such as pH and redox dual stimulation-responsive folate-targeted folic acid–β-cyclodextrin–hyperbranched poly (amido amine) (FA-DS-PAAs) nanocarriers, are currently used in precision-targeted therapy for NPC by co-delivering docetaxel and tissue factor pathway inhibitor 2 (TFPI-2) (101). Drug resistance significantly affects the low 5-year survival rate of patients with NPC. Ongoing studies elucidating the intricate relationship between LncRNAs and drug resistance underscore the potential of LncRNAs as treatment targets for NPC. Further clinical investigations are warranted to enhance the 5-year survival rate of patients with NPC.
YY: Writing – original draft. QY: Writing – review & editing. WT: Writing – review & editing. YM: Writing – review & editing. JD: Writing – review & editing. GY: Conceptualization, Writing – review & editing. YF: Conceptualization, Writing – review & editing.
The author(s) declare that no financial support was received for the research, authorship, and/or publication of this article.
The authors declare that the research was conducted in the absence of any commercial or financial relationships that could be construed as a potential conflict of interest.
All claims expressed in this article are solely those of the authors and do not necessarily represent those of their affiliated organizations, or those of the publisher, the editors and the reviewers. Any product that may be evaluated in this article, or claim that may be made by its manufacturer, is not guaranteed or endorsed by the publisher.
1. Linton R, Daker M, Khoo A, Choo D, Viljoen M, Neilsen P. Nasopharyngeal carcinoma among the Bidayuh of Sarawak, Malaysia: History and risk factors. Oncol Lett (2021) 22(1):514. doi: 10.3892/ol.2021.12775
2. Luo W. Nasopharyngeal carcinoma ecology theory: cancer as multidimensional spatiotemporal "unity of ecology and evolution" pathological ecosystem. Theranostics (2023) 13(5):1607–31. doi: 10.7150/thno.82690
3. Renaud S, Lefebvre A, Mordon S, Moralès O, Delhem N. Novel therapies boosting T cell immunity in Epstein Barr virus-associated nasopharyngeal carcinoma. Int J Mol Sci (2020) 21(12). doi: 10.3390/ijms21124292
4. Jain A, Chia WK, Toh HC. Immunotherapy for nasopharyngeal cancer-a review. Chin Clin Oncol (2016) 5(2):22. doi: 10.21037/cco.2016.03.08
5. Liu Z, Chen Y, Su Y, Hu X, Peng X. Nasopharyngeal carcinoma: clinical achievements and considerations among treatment options. Front Oncol (2021) 11:635737. doi: 10.3389/fonc.2021.635737
6. Dwijayanti F, Prabawa A, Besral, Herawati C. The five-year survival rate of patients with nasopharyngeal carcinoma based on tumor response after receiving neoadjuvant chemotherapy, followed by chemoradiation, in Indonesia: A retrospective study. Oncology (2020) 98(3):154–60. doi: 10.1159/000504449
7. Zakutansky PM, Feng Y. The long non-coding RNA GOMAFU in Schizophrenia:Function, disease risk, and beyond. cells (2022) 11(12). doi: 10.3390/cells11121949
8. Soghli N, Yousefi T, Abolghasemi M, Qujeq D. NORAD, a critical long non-coding RNA in human cancers. Life Sci (2021) 264:118665. doi: 10.1016/j.lfs.2020.118665
9. Liu X, Hao J, Xie T, Pant O, Lu C, Lu C, et al. The BRAF activated non-coding RNA: A pivotal long non-coding RNA in human Malignancies. Cell Prolif (2018) 51(4):e12449. doi: 10.1111/cpr.12449
10. Wang W, Zhang Z, Li Y, Gu A, Wang Y, Cai Y, et al. Down-regulated long non-coding RNA LHFPL3 antisense RNA 1 inhibits the radiotherapy resistance of nasopharyngeal carcinoma via modulating microRNA-143-5p/homeobox A6 axis. Bioengineered (2022) 13(3):5421–33. doi: 10.1080/21655979.2021.2024386
11. Zhang W, Zhou X, Tang Z, Fu L, Zou S, Tang S, et al. Knockdown of lncRNA SNHG16 Attenuates the Proliferation and Radioresistance of Nasopharyngeal Carcinoma Cells by Mediating miR-31-5p/SFN Axis. Radiat Res (2023) 199(2):124–31. doi: 10.1667/rade-22-00163.1
12. Yuan F, Lou Z, Zhou Z, Yan X. Long non−coding RNA KCNQ1OT1 promotes nasopharyngeal carcinoma cell cisplatin resistance via the miR−454/USP47 axis. Int J Mol Med (2021) 47(4). doi: 10.3892/ijmm.2021.4887
13. Li H, Huang J, Yu S, Lou Z. Long non-coding RNA DLEU1 up-regulates BIRC6 expression by competitively sponging miR-381-3p to promote cisplatin resistance in nasopharyngeal carcinoma. OncoTargets Ther (2020) 13:2037–45. doi: 10.2147/ott.S237456
14. Cao W, Sun Y, Liu L, Yu J, Ji J, Wang Y, et al. HOTAIR mediates cisplatin resistance in nasopharyngeal carcinoma by regulating miR-106a-5p/SOX4 axis. Bioengineered (2022) 13(3):6567–78. doi: 10.1080/21655979.2022.2038429
15. Yang J, Qi M, Fei X, Wang X, Wang K. Long non-coding RNA XIST: a novel oncogene in multiple cancers. Mol Med (Cambridge Mass.) (2021) 27(1):159. doi: 10.1186/s10020-021-00421-0
16. Wang H, Li W, Tan G. in vitro[Long non-coding RNA XIST modulates cisplatin resistance by altering PDCD4 and Fas-Lexpressions in human nasopharyngeal carcinoma HNE1 cells ]. Nan fang yi ke da xue xue bao = J South Med Univ (2019) 39(3):357–63. doi: 10.12122/j.issn.1673-4254.2019.03.15
17. Chen W YJ, Fang H, Li L, Sun J. Relevance function of linc-ROR in the pathogenesis of cancer. Front Cell Dev Biol (2020). doi: 10.3389/fcell.2020.00696
18. Huang J. Current developments of targeting the p53 signaling pathway for cancer treatment. Pharmacol Ther (2021). doi: 10.1016/j.pharmthera.2020.107720
19. Li L GM, You B, Shi S, Shan Y, Bao L, You Y. Long non-coding RNA ROR promotes proliferation, migration and chemoresistance of nasopharyngeal carcinoma. Cancer Sci (2016) 107(9):1215–22. doi: 10.1111/cas.12989
20. Liu F, Tai Y, Ma J. LncRNA NEAT1/let-7a-5p axis regulates the cisplatin resistance in nasopharyngeal carcinoma by targeting Rsf-1 and modulating the Ras-MAPK pathway. Cancer Biol Ther (2018) 19(6):534–42. doi: 10.1080/15384047.2018.1450119
21. Xue F, Cheng Y, Xu L, Tian C, Jiao H, Wang R, et al. LncRNA NEAT1/miR-129/Bcl-2 signaling axis contributes to HDAC inhibitor tolerance in nasopharyngeal cancer. Aging (2020) 12(14):14174–88. doi: 10.18632/aging.103427
22. Cao C, Zhou S, Hu J. Long noncoding RNA MAGI2-AS3/miR-218-5p/GDPD5/SEC61A1 axis drives cellular proliferation and migration and confers cisplatin resistance in nasopharyngeal carcinoma. Int Forum Allergy Rhinol (2020) 10(8):1012–23. doi: 10.1002/alr.22562
23. Lin F, Lin X, Xu L, Zhu S. Long Noncoding RNA HOXA11-AS Modulates the Resistance of Nasopharyngeal Carcinoma Cells to Cisplatin via miR-454-3p/c-Met. Molecules Cells (2020) 43(10):856–69. doi: 10.14348/molcells.2020.0133
24. Li H, Huang J, Yu S, Li H, Zhou Y, Wu Q. HOXA11-AS induces cisplatin resistance by modulating the microRNA-98/PBX3 axis in nasopharyngeal carcinoma. Oncol Lett (2021) 21(6):493. doi: 10.3892/ol.2021.12754
25. Wang Q, Zhang W, Hao S. LncRNA CCAT1 modulates the sensitivity of paclitaxel in nasopharynx cancers cells via miR-181a/CPEB2 axis. Cell Cycle (Georgetown Tex.) (2017) 16(8):795–801. doi: 10.1080/15384101.2017.1301334
26. He Y, Jing Y, Wei F, Tang Y, Yang L, Luo J, et al. Long non-coding RNA PVT1 predicts poor prognosis and induces radioresistance by regulating DNA repair and cell apoptosis in nasopharyngeal carcinoma. Cell Death Dis (2018) 9(2):235. doi: 10.1038/s41419-018-0265-y
27. Han Y, Li F, Xie J, Wang Y, Zhang H. PVT1 mediates cell proliferation, apoptosis and radioresistance in nasopharyngeal carcinoma through regulating miR-515-5p/PIK3CA axis. Cancer Manage Res (2020) 12:10077–90. doi: 10.2147/cmar.S257583
28. Li J, Li Y, Liu L, Wu D, Yang T, Fan Y. LINC01140 regulates radiosensitivity of nasopharyngeal carcinoma cells through the ceRNA network. Cell Mol Biol (Noisy-le-Grand France) (2022) 68(4):75–85. doi: 10.14715/cmb/2022.68.4.10
29. Guo Z, Wang Y, He B, Zhou J. Linc00312 single nucleotide polymorphism as biomarker for chemoradiotherapy induced hematotoxicity in nasopharyngeal carcinoma patients. Dis Markers (2022) 2022:6707821. doi: 10.1155/2022/6707821
30. Guo Z, Wang Y, Xu H, Yuan C, Zhou H, Huang W, et al. LncRNA linc00312 suppresses radiotherapy resistance by targeting DNA-PKcs and impairing DNA damage repair in nasopharyngeal carcinoma. Cell Death Dis (2021) 12(1):69. doi: 10.1038/s41419-020-03302-2
31. Shen Z, Wu Y, He G. Long non-coding RNA PTPRG-AS1/microRNA-124-3p regulates radiosensitivity of nasopharyngeal carcinoma via the LIM Homeobox 2-dependent Notch pathway through competitive endogenous RNA mechanism. Bioengineered (2022) 13(4):8208–25. doi: 10.1080/21655979.2022.2037364
32. Yi L, Ouyang L, Wang S, Li S, Yang X. Long noncoding RNA PTPRG-AS1 acts as a microRNA-194-3p sponge to regulate radiosensitivity and metastasis of nasopharyngeal carcinoma cells via PRC1. J Cell Physiol (2019) 234(10):19088–102. doi: 10.1002/jcp.28547
33. Liu H, Zheng W, Chen Q, Zhou Y, Pan Y, Zhang J, et al. lncRNA CASC19 contributes to radioresistance of nasopharyngeal carcinoma by promoting autophagy via AMPK-mTOR pathway. Int J Mol Sci (2021) 22(3). doi: 10.3390/ijms22031407
34. Liu H, Chen Q, Zheng W, Zhou Y, Bai Y, Pan Y, et al. LncRNA CASC19 enhances the radioresistance of nasopharyngeal carcinoma by regulating the miR-340-3p/FKBP5 axis. Int J Mol Sci (2023) 24(3). doi: 10.3390/ijms24033047
35. Mondal P, Meeran S. Emerging role of non-coding RNAs in resistance to platinum-based anti-cancer agents in lung cancer. Front Pharmacol (2023) 14:1105484. doi: 10.3389/fphar.2023.1105484
36. Kritsch D, Hoffmann F, Steinbach D, Jansen L, Photini Mary S, Gajda M, et al. Tribbles 2 mediates cisplatin sensitivity and DNA damage response in epithelial ovarian cancer. Int J Cancer (2017) 141(8):1600–14. doi: 10.1002/ijc.30860
37. Mondal P, Meeran S. microRNAs in cancer chemoresistance: The sword and the shield. Non-coding RNA Res (2021) 6(4):200–10. doi: 10.1016/j.ncrna.2021.12.001
38. Liu J, Liu Y, Yang C, Liu J, Hao J. Comprehensive analysis for the immune related biomarkers of platinum-based chemotherapy in ovarian cancer. Trans Oncol (2023) 37:101762. doi: 10.1016/j.tranon.2023.101762
39. Tay Y, Rinn J, Pandolfi P. The multilayered complexity of ceRNA crosstalk and competition. Nature (2014) 505(7483):344–52. doi: 10.1038/nature12986
40. Lu J, Xiao Z, Xu M, Li L. New Insights into LINC00346 and its Role in Disease. Front Cell Dev Biol (2021) 9:819785. doi: 10.3389/fcell.2021.819785
41. Giussani P, Tringali C, Riboni L, Viani P, Venerando B. Sphingolipids: key regulators of apoptosis and pivotal players in cancer drug resistance. Int J Mol Sci (2014) 15(3):4356–92. doi: 10.3390/ijms15034356
42. Smith B, Bhowmick N. Role of EMT in metastasis and therapy resistance. J Clin Med (2016) 5(2). doi: 10.3390/jcm5020017
43. Dong B, Li S, Zhu S, Yi M, Luo S, Wu K. MiRNA-mediated EMT and CSCs in cancer chemoresistance. Exp Hematol Oncol (2021) 10(1):12. doi: 10.1186/s40164-021-00206-5
44. Chen W, Li X, Du B, Cui Y, Ma Y, Li Y. The long noncoding RNA HOXA11-AS promotes lung adenocarcinoma proliferation and glycolysis via the microRNA-148b-3p/PKM2 axis. Cancer Med (2023) 12(4):4421–33. doi: 10.1002/cam4.5103
45. Li B, Lv Y, Zhang C, Xiang C. lncRNA HOXA11-AS maintains the stemness of oral squamous cell carcinoma stem cells and reduces the radiosensitivity by targeting miR-518a-3p/PDK1. J Oral Pathol Med (2023) 52(3):216–25. doi: 10.1111/jop.13405
46. You L, Wu Q, Xin Z, Zhong H, Zhou J, Jiao L, et al. The long non-coding RNA HOXA11-AS activates ITGB3 expression to promote the migration and invasion of gastric cancer by sponging miR-124-3p. Cancer Cell Int (2021) 21(1):576. doi: 10.1186/s12935-021-02255-6
47. Xing Z, Li S, Liu Z, Zhang C, Bai Z. CTCF-induced upregulation of HOXA11-AS facilitates cell proliferation and migration by targeting miR-518b/ACTN4 axis in prostate cancer. Prostate (2020) 80(5):388–98. doi: 10.1002/pros.23953
48. Xu Y, Ren Z, Wang X, Ren M. The lncRNA HOXA11-AS acts as a tumor promoter in breast cancer through regulation of the miR-125a-5p/TMPRSS4 axis. J Gene Med (2022) 24(5):e3413. doi: 10.1002/jgm.3413
49. Zhao S, Tang Y, Wang R, Najafi M. Mechanisms of cancer cell death induction by paclitaxel: an updated review. Apoptosis an Int J programmed Cell Death (2022) 27:647–67. doi: 10.1007/s10495-022-01750-z
50. Doğan Şiğva Z, Balci Okcanoğlu T, Biray Avci Ç., Süslüer Yilmaz S, Kayabaşi Ç, Turna B, et al. Investigation of the synergistic effects of paclitaxel and herbal substances and endemic plant extracts on cell cycle and apoptosis signal pathways in prostate cancer cell lines. Gene (2019) 687:261–71. doi: 10.1016/j.gene.2018.11.049
51. Gallego-Jara J, Lozano-Terol G, Sola-Martínez R, Cánovas-Díaz M, Puente Diego T. A compressive review about taxol: history and future challenges. Molecules (Basel Switzerland) (2020) 25(24). doi: 10.3390/molecules25245986
52. Mahabady M, Mirzaei S, Saebfar H, Gholami M, Zabolian A, Hushmandi K, et al. Noncoding RNAs and their therapeutics in paclitaxel chemotherapy: Mechanisms of initiation, progression, and drug sensitivity. J Cell Physiol (2022) 237(5):2309–44. doi: 10.1002/jcp.30751
53. Smits G MA, Griffiths-Jones S, Smith P, Beury D, Matthews L, Rogers J, et al. Conservation of the H19 noncoding RNA and H19-IGF2 imprinting mechanism in therians. Nat Genet (2008) 40. doi: 10.1038/ng.168
54. Ghafouri-Fard S SH, Bahroudi Z, Abak A, Taheri M. The role of H19 lncRNA in conferring chemoresistance in cancer cells. BioMed Pharmacother (2021) 138):111447. doi: 10.1016/j.biopha.2021.111447
55. Gao H HG, Sun Y, Li L, Wang Y. Long noncoding RNA H19 mediated the chemosensitivity of breast cancer cells via Wnt pathway and EMT process. Onco Targets Ther (2018) 11:8001–12. doi: 10.2147/OTT.S172379
56. Wang M HD, Yuan Z, Hu H, Zhao Z, Yang R, Jin Y, et al. Long non-coding RNA H19 confers 5-Fu resistance in colorectal cancer by promoting SIRT1-mediated autophagy. Cell Death Dis (2018) 9(12):1149. doi: 10.1038/s41419-018-1187-4
57. Chen C LW, Zhang B, Zhang LM, Li CG, Liu C, Zhang H, et al. LncRNA H19 downregulation confers erlotinib resistance through upregulation of PKM2 and phosphorylation of AKT in EGFR-mutant lung cancers. Cancer Lett (2020) 486:58–70. doi: 10.1016/j.canlet.2020.05.009
58. Wu KF LW, Feng L, Pang JX, Waye MM, Zhang JF, Fu WM. H19 mediates methotrexate resistance in colorectal cancer through activating Wnt/β-catenin pathway. Exp Cell Res (2017) 350(2):312–7. doi: 10.1016/j.yexcr.2016.12.003
59. Zhu H. Silencing long non-coding RNA H19 combined with paclitaxel inhibits nasopharyngeal carcinoma progression. Int J Pediatr otorhinolaryngology (2020) 138:110249. doi: 10.1016/j.ijporl.2020.110249
60. Ren S, Li G, Liu C, Cai T, Su Z, Wei M, et al. Next generation deep sequencing identified a novel lncRNA n375709 associated with paclitaxel resistance in nasopharyngeal carcinoma. Oncol Rep (2016) 36(4):1861–7. doi: 10.3892/or.2016.4981
61. Liu Z CQ, Hann SS. The functions and oncogenic roles of CCAT1 in human cancer. BioMed Pharmacother (2019) 115:108943. doi: 10.1016/j.biopha.2019.108943
62. Liu Y, Ao X, Wang Y, Li X, Wang J. Long non-coding RNA in gastric cancer: mechanisms and clinical implications for drug resistance. Front Oncol (2022) 12:841411. doi: 10.3389/fonc.2022.841411
63. Shen C, Yang C, Xia B, You M. Long non-coding RNAs: Emerging regulators for chemo/immunotherapy resistance in cancer stem cells. Cancer Lett (2021) 500:244–52. doi: 10.1016/j.canlet.2020.11.010
64. Maugeri-Saccà M, Bartucci M, De Maria R. DNA damage repair pathways in cancer stem cells. Mol Cancer Ther (2012) 11(8):1627–36. doi: 10.1158/1535-7163.Mct-11-1040
65. Lecerf C, Peperstraete E, Le Bourhis X, Adriaenssens E. H19Propagation and maintenance of cancer stem cells: A major influence of the long non-coding RNA. Cells (2020) 9(12). doi: 10.3390/cells9122613
66. Lin P, Xing W, Ren Q, Wang Q, Yan J, Mao G, et al. LncRNAs as theragnostic biomarkers for predicting radioresistance in cancer: A systematic review and meta-analysis. Front Oncol (2022) 12:767750. doi: 10.3389/fonc.2022.767750
67. Wang Y, Guo Z, An L, Zhou Y, Xu H, Xiong J, et al. LINC-PINT impedes DNA repair and enhances radiotherapeutic response by targeting DNA-PKcs in nasopharyngeal cancer. Cell Death Dis (2021) 12(5):454. doi: 10.1038/s41419-021-03728-2
68. Lei F, Lei T, Huang Y, Yang M, Liao M, Huang W, et al. Radio-susceptibility of nasopharyngeal carcinoma: focus on Epstein- Barr virus, microRNAs, long non-coding RNAs and circular RNAs. Curr Mol Pharmacol (2020) 13(3):192–205. doi: 10.2174/1874467213666191227104646
69. Yao W LS, Liu R, Jiang M, Gao L, Lu Y, Liang X, et al. Long non-coding RNA PVT1: A promising chemotherapy and radiotherapy sensitizer. Front Oncol (2022) 12:959208. doi: 10.3389/fonc.2022.959208
70. Guo Z BM, Fan YX, Zhang Y, Liu HY, Zhou XL, Wu B, et al. Genetic polymorphisms of long non-coding RNA linc00312 are associated with susceptibility and predict poor survival of nasopharyngeal carcinoma. Front Cell Dev Biol (2021) 9:698558. doi: 10.3389/fcell.2021.698558
71. Wang S QC, Fang R, Yang S, Zhao G, Liu S, Li P. LncRNA CASC19: a novel oncogene involved in human cancer. Clin Transl Oncol (2023) 25(10):2841–51. doi: 10.1007/s12094-023-03165-x
72. Lee A, Ng W, Chan J, Corry J, Mäkitie A, Mendenhall W, et al. Management of locally recurrent nasopharyngeal carcinoma. Cancer Treat Rev (2019) 79:101890. doi: 10.1016/j.ctrv.2019.101890
73. Luo H, Yi B. The role of Exosomes in the Pathogenesis of Nasopharyngeal Carcinoma and the involved Clinical Application. Int J Biol Sci (2021) 17(9):2147–56. doi: 10.7150/ijbs.59688
74. Zhou Y, Xia L, Lin J, Wang H, Oyang L, Tan S, et al. Exosomes in nasopharyngeal carcinoma. J Cancer (2018) 9(5):767–77. doi: 10.7150/jca.22505
75. Natasha G, Gundogan B, Tan A, Farhatnia Y, Wu W, Rajadas J, et al. Exosomes as immunotheranostic nanoparticles. Clin Ther (2014) 36(6):820–9. doi: 10.1016/j.clinthera.2014.04.019
76. Syn N, Wang L, Chow E, Lim C, Goh B. Exosomes in cancer nanomedicine and immunotherapy: prospects and challenges. Trends Biotechnol (2017) 35(7):665–76. doi: 10.1016/j.tibtech.2017.03.004
77. Elsharkawi F, Elsabah M, Shabayek M, Khaled H. Urine and serum exosomes as novel biomarkers in detection of bladder cancer. Asian Pacific J Cancer Prev APJCP (2019) 20(7):2219–24. doi: 10.31557/apjcp.2019.20.7.2219
78. Tamkovich S, Tutanov O, Efimenko A, Grigor'eva A, Ryabchikova E, Kirushina N, et al. Blood circulating exosomes contain distinguishable fractions of free and cell-surface-associated vesicles. Curr Mol Med (2019) 19(4):273–85. doi: 10.2174/1566524019666190314120532
79. Nair S, Tang K, Kenny L, Punyadeera C. Salivary exosomes as potential biomarkers in cancer. Oral Oncol (2018) 84:31–40. doi: 10.1016/j.oraloncology.2018.07.001
80. Li Z, Chen H, Xia H, Xu X, Gu J, Jin T, et al. Altered microRNAs in cerebrospinal fluid exosomes in paraneoplastic and autoimmune encephalitis: A possible feedback in cancer development. Life Sci (2021), 119339. doi: 10.1016/j.lfs.2021.119339
81. Wang X, Zhang Y, Mu X, Tu C, Chung Y, Tsao S, et al. Exosomes derived from γδ-T cells synergize with radiotherapy and preserve antitumor activities against nasopharyngeal carcinoma in immunosuppressive microenvironment. J Immunother Cancer (2022) 10(2). doi: 10.1136/jitc-2021-003832
82. Yuan F, Zhou Z. Exosomes derived from Taxol-resistant nasopharyngeal carcinoma (NPC) cells transferred DDX53 to NPC cells and promoted cancer resistance to Taxol. Eur Rev Med Pharmacol Sci (2021) 25(1):127–38. doi: 10.26355/eurrev_202101_24375
83. Xu L, Zou C, Zhang S, Chu T, Zhang Y, Chen W, et al. Reshaping the systemic tumor immune environment (STIE) and tumor immune microenvironment (TIME) to enhance immunotherapy efficacy in solid tumors. J Hematol Oncol (2022) 15(1):87. doi: 10.1186/s13045-022-01307-2
84. Chow J, Ngan R, Cheung K, Chov W, Chov W. Immunotherapeutic approaches in nasopharyngeal carcinoma. Expert Opin Biol Ther (2019) 19(11):1165–72. doi: 10.1080/14712598.2019.1650910
85. Xu J, Wei X, Wang Y, Wang F. Current status and advances of immunotherapy in nasopharyngeal carcinoma. Ther Adv Med Oncol (2022) 14:17588359221096214. doi: 10.1177/17588359221096214
86. Yang J, Chen J, Liang H, Yu Y. Nasopharyngeal cancer cell-derived exosomal PD-L1 inhibits CD8+ T-cell activity and promotes immune escape. Cancer Sci (2022) 113(9):3044–54. doi: 10.1111/cas.15433
87. Yang X, Gao M, Xu R, Tao Y, Luo W, Wang B, et al. Hyperthermia combined with immune checkpoint inhibitor therapy in the treatment of primary and metastatic tumors. Front Immunol (2022) 13:969447. doi: 10.3389/fimmu.2022.969447
88. Chhabra N, Kennedy J. A review of cancer immunotherapy toxicity II: adoptive cellular therapies, kinase inhibitors, monoclonal antibodies, and oncolytic viruses. J Med Toxicol (2022) 18(1):43–55. doi: 10.1007/s13181-021-00835-6
89. Chen P, Liu B, Xia X, Huang P, Zhao J. Current progress in immunotherapy of nasopharyngeal carcinoma. Am J Cancer Res (2023) 13(4):1140–7.
90. Etxebeste-Mitxeltorena M, Del Rincón-Loza I, Martín-Antonio B. Tumor secretome to adoptive cellular immunotherapy: reduce me before I make you my partner. Front Immunol (2021) 12:717850. doi: 10.3389/fimmu.2021.717850
91. Hopkins R, Xiang W, Marlier D, Au V, Ching Q, Wu L. Monocytic myeloid-derived suppressor cells underpin resistance to adoptive T cell therapy in nasopharyngeal carcinoma. Mol Ther (2021) 29(2):734–43. doi: 10.1016/j.ymthe.2020.09.040
92. Balfour H, Schmeling D, Grimm-Geris J. The promise of a prophylactic Epstein-Barr virus vaccine. Pediatr Res (2020) 87(2):345–52. doi: 10.1038/s41390-019-0591-5
93. Zhu Q, Zhao G, Li Y, Talakatta G, Mai H, Le Q, et al. Advances in pathogenesis and precision medicine for nasopharyngeal carcinoma. MedComm (2021) 2(2):175–206. doi: 10.1002/mco2.32
94. Cohen J. Epstein-barr virus vaccines. Clin Trans Immunol (2015) 4(1):e32. doi: 10.1038/cti.2014.27
95. Coghill A, Bu W, Nguyen H, Hsu W, Yu K, Lou P, et al. High levels of antibody that neutralize B-cell infection of Epstein-Barr virus and that bind EBV gp350 are associated with a lower risk of nasopharyngeal carcinoma. Clin Cancer Res (2016) 22(14):3451–7. doi: 10.1158/1078-0432.Ccr-15-2299
96. Guo M, Duan X, Peng X, Jin Z, Huang H, Xiao W, et al. A lipid-based LMP2-mRNA vaccine to treat nasopharyngeal carcinoma. Nano Res (2023) 16(4):5357–67. doi: 10.1007/s12274-022-5254-x
97. Wang H, Wang W, Fan S. Emerging roles of lncRNA in Nasopharyngeal Carcinoma and therapeutic opportunities. Int J Biol Sci (2022) 18(7):2714–28. doi: 10.7150/ijbs.70292
98. Yu H, Zhang C, Li W, Sun X, Liu Q, Wang D, et al. Nano-coated si-SNHG14 regulated PD-L1 expression and decreased epithelial-mesenchymal transition in nasopharyngeal carcinoma cells. J Biomed Nanotechnol (2021) 17(10):1993–2002. doi: 10.1166/jbn.2021.3162
99. Ding Y, Xiao X, Zeng L, Shang Q, Jiang W, Xiong S, et al. Platinum-crosslinking polymeric nanoparticle for synergetic chemoradiotherapy of nasopharyngeal carcinoma. Bioactive Mater (2021) 6(12):4707–16. doi: 10.1016/j.bioactmat.2021.05.010
100. Cheng T, Zhang Y, Liu J, Ding Y, Ou H, Huang F, et al. Ligand-switchable micellar nanocarriers for prolonging circulation time and enhancing targeting efficiency. ACS Appl Mater interfaces (2018) 10(6):5296–304. doi: 10.1021/acsami.7b18137
Keywords: nasopharyngeal carcinoma, long noncoding RNAs, drug resistance, radiation resistance, recurrence
Citation: Yang Y, Yuan Q, Tang W, Ma Y, Duan J, Yang G and Fang Y (2024) Role of long non-coding RNA in chemoradiotherapy resistance of nasopharyngeal carcinoma. Front. Oncol. 14:1346413. doi: 10.3389/fonc.2024.1346413
Received: 29 November 2023; Accepted: 29 January 2024;
Published: 29 February 2024.
Edited by:
Raffaele Addeo, ASLNAPOLI2NORD ONCOLOGIA, ItalyReviewed by:
Weiren Luo, The Second Affiliated hospital of Southern University of Science and Technology, ChinaCopyright © 2024 Yang, Yuan, Tang, Ma, Duan, Yang and Fang. This is an open-access article distributed under the terms of the Creative Commons Attribution License (CC BY). The use, distribution or reproduction in other forums is permitted, provided the original author(s) and the copyright owner(s) are credited and that the original publication in this journal is cited, in accordance with accepted academic practice. No use, distribution or reproduction is permitted which does not comply with these terms.
*Correspondence: GuoNing Yang, eWFuZ2duQDEyNi5jb20=; Yuan Fang, MjAyMTE3NTlAa21tdS5lZHUuY24=
†These authors share first authorship
Disclaimer: All claims expressed in this article are solely those of the authors and do not necessarily represent those of their affiliated organizations, or those of the publisher, the editors and the reviewers. Any product that may be evaluated in this article or claim that may be made by its manufacturer is not guaranteed or endorsed by the publisher.
Research integrity at Frontiers
Learn more about the work of our research integrity team to safeguard the quality of each article we publish.