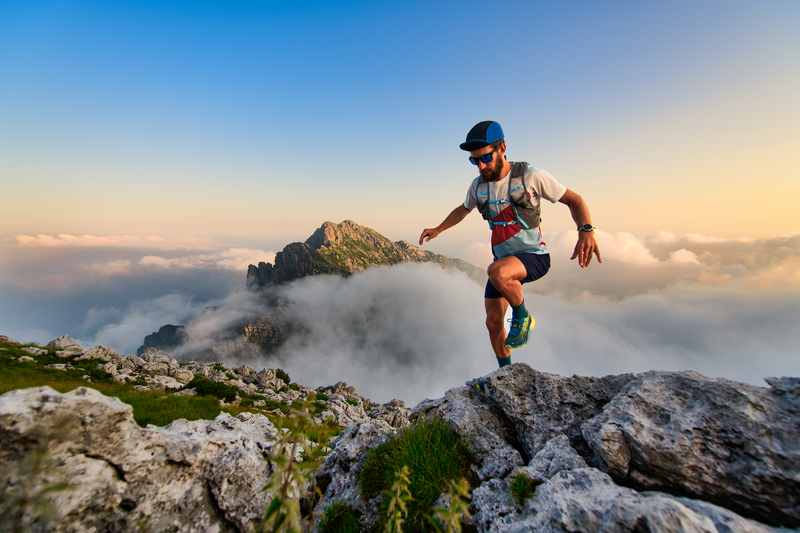
94% of researchers rate our articles as excellent or good
Learn more about the work of our research integrity team to safeguard the quality of each article we publish.
Find out more
REVIEW article
Front. Oncol. , 13 March 2024
Sec. Cancer Immunity and Immunotherapy
Volume 14 - 2024 | https://doi.org/10.3389/fonc.2024.1330254
This article is part of the Research Topic Translational Research in Surgical Applications and Spinal Tumors View all 9 articles
Animal models have been commonly used in immunotherapy research to study the cell response to external agents and to assess the effectiveness and safety of new therapies. Over the past few decades, immunocompromised (also called immunodeficient) mice allowed researchers to grow human tumor cells without the impact of the host’s immune system. However, while this model is very valuable to understand the tumor biology and to understand the underlying mechanism of immunotherapy, the results may not always directly translate to humans. The tumor microenvironment has significant implications for tumor engraftment, growth, invasion, etc., and the immune system plays a critical role in shaping the tumor microenvironment. Human immunocompetent mice, also named humanized mice, are engineered mice that possess functional human immune cells. This in vivo model can be used to effectively study the effect of the human immune system to a human implanted tumor. Moreover, this can effectively mimic the response to treatment. This section is an overview of the current understanding of the different humanized mice that could be utilized to mimic the tumor microenvironment in chordoma.
Graphical Abstract Created with BioRender.com.
For decades, animals have served as invaluable tools for studying human biology in research. Among these, mice, in particular, have gained widespread use owing to their small size, ease of handling and maintenance, cost-effectiveness, rapid reproductive cycles, and their shared genomic, anatomical, and physiological characteristics with humans. As a result, they have made an indelible mark on biomedical research and have emerged as the primary model for investigating human diseases and conducting preclinical trials, thanks to the discipline of comparative medicine (1). With the expansion of our understanding of human diseases, there is an escalating demand for increasingly sophisticated mouse models. These models are not only essential for comprehending the biology of the diseases but also for evaluating potential therapeutic interventions. In this review, we will provide a concise overview of the animal models employed in cancer research, with a particular focus on chordoma. Additionally, we will examine the importance of utilizing a specific mouse model, humanized mice, in chordoma studies.
A literature review was performed using PubMed. This review did not receive funding, a full review protocol is available by request. Search terms included [(chordoma) AND ((mouse) OR (microenvironment))] on all literature published before August 16, 2023. Articles were independently screened by (C.S, B.C, M.M.M, O.L, J.A), full text of remaining studies were screened by (C.S, M.M.M).
Inclusion criteria included all English language papers describing novel immunocompromised mouse models in chordoma. Fifty papers were excluded, 32 did not include mice, 1 was a duplicate study, 3 were not in English, 4 mouse studies contained non-immunocompromised mice, 8 contained non-novel models, and 3 did not use chordoma as the model. The initial search yielded 92 unique articles with 42 papers remaining after inclusion criteria was applied.
Chordomas are primary tumors of the axial skeleton and skull base, believed to be derived from primitive notochordal cells. The incidence of chordoma is approximately 0.08/100,000 (2). The progression of the tumor is slow and insidious, most often presenting clinically at the median age of 59 (3). However, the tumor can present in pediatric patients, most often presenting at age 10 (4). Since chordomas may present in the clivus, mobile spine, and sacrococcygeal region, symptoms can vary greatly. Clival chordomas typically manifest as cranial nerve palsy and headaches (5). While a mobile spine lesion typically presents with back pain in addition to neurological symptoms associated with the affected nerve roots (6). Sacrococcygeal lesions present with similar symptoms to mobile spine lesions with the addition of bowel and bladder complications (7). Current treatment for chordoma recommends radical en bloc resection with adjuvant radiotherapy. En bloc resection is recommended since the complete resection of the tumor with a wide margin and without tumor capsule invasion prevents the seeding of cancer cells, thus preventing recurrence (8). In addition to en bloc resection, some patients will receive adjuvant ration as it has been shown to improve disease-free survival, however, this is still debated (9). Currently, there are no medical treatments approved for chordoma (10). Creating a medical treatment could have utility in shrinking tumors preoperatively to reduce surgical margins and improve quality of life, as well as applied postoperatively to prevent recurrence.
The use of animals, particularly mice, in cancer research serves as an invaluable tool for advancing our understanding of various malignancies, including chordoma. The organism physiology and the tumor microenvironment is essential to study cancer and for conducting therapy testing: animals, with their biological complexity, provide a comprehensive perspective that cannot be replicated in in vitro models. This mini-review conducts a broad examination of the general use of mice in cancer research, with a subsequent in-depth exploration of its use in chordoma research, focusing on the use of humanized mice.
Animal models have become indispensable tools in cancer and immunotherapy research, providing insights into tumorigenesis, the safety and efficacy of developing therapeutics, and the tumor microenvironment in which their interactions occur. Pre-clinical in vivo studies using these models have been key in the advancement from simple mass surgical resection to more specific target-based treatments in modern medicine (11). The use of murine models, in particular, has been well-established in the existing literature. Compared to other available models, mice are not only low in cost and easy to maintain but also have a short lifespan and reproductive cycle, exhibit high tumor growth rates, and are susceptible to genetic manipulation (12). Numerous murine cancer models have been developed since the previous century, each with its own advantages and limitations, and have contributed to the discovery of novel cancer control and prevention strategies. Immunocompromised mouse models are among the most frequently utilized approaches to evaluating tumor biology. Also referred to as immunodeficient mice, given their suppression or lack of a functional immune system, these specialized models have enabled researchers to elucidate the underlying mechanisms driving tumor cell growth without the confounding effects of immune response or rejection. Since their initial discovery in the 1960s, multiple strains of immunocompromised mice with varying degrees of genetic defects have been developed and commonly employed in chemotherapeutic/cytotoxicity studies and patient-derived xenograft (PDX) models (13).
Athymic nude mice were the first known immunocompromised model, discovered in 1962 by Grist (Ruchill Hospital, Glasgow, UK) and was first published by Flanagan in 1966 (14). Widely recognized for their lack of body fur (therefore the nude nickname), they also possess severe T-cell dysfunction due to abnormal thymus development; however, their innate immune system and B cells remain intact. Alternatively, severe combined immunodeficiency (SCID) mice are deprived of DNA-dependent protein kinase encoded by the deleted PRKDC gene, resulting in a shortage of both functional B and T lymphocytes. These were later the first immunodeficient mice to receive human hematopoietic stem cell (HSCs) and peripheral blood mononuclear cell (PBMCs) transplantation to generate the first humanized mice, as we explain below (15). To improve the engraftment efficiency of human tumors in these models, SCID/Beige mice were later established by crossbreeding SCID and Beige mice (16). While improvements were observed, it still left much to be desired.
Non-obese diabetic (NOD) mice were then identified in 1980, and their crossbreeding with SCID mice produced the NOD/SCID model. NOD/SCID mice lose functional NK cells in addition to T and B lymphocytes, though residual NK cell activity remains prevalent, limiting engraftment efficiency (17). To address this, several NOD/SCID-based immunocompromised derivatives with even further reduced or complete loss of NK cells were also established, including NSB, which lack β2 microglobulin, and NOJ, which are Jak-3 deficient (17–19).
In the early 2000s, Ito et al. (20) and Shultz et al. (21) developed two more NOD/SCID-related models: NOG and NSG, respectively. These are frequently utilized given their lack of mature lymphocytes and NK cells, leading to high engraftment of human cells and tissues (22). These features arise from the deletion of a functional common interleukin-2 γ-chain (NOD/SCID IL- 2rγnull), which is vital for the high affinity binding and signaling of IL-2, IL-4, IL-7, IL-9, IL-15, and IL-21. Without this molecule, NK development is halted. Their ability to survive beyond 16 months is therefore of particular interest in light of their severe impairments in innate immunity. Although both may also be referred to as NOD/SCID IL- 2rγnull, NOG possesses a partial deficiency of IL2rγ while NSG has a complete deficiency (17). These numerous NOD/SCID-related strains emphasize the range of immunodeficiencies available to researchers depending on their particular targets of interest, lifespan requirements, or reported engraftment rates. Since the generation of humanized mice bearing the interleukin 2 receptor gamma chain mutation (IL-2rγnull)2 in the change of the millennia, investigators have been able to successfully engraft human tissues, including HSCs, as we will delve into in more detail below. A summary of the immunocompromised mice models is graphed in Figure 1.
Figure 1 Flowchart of the different immunocompromised mice models used for cancer research. Created with BioRender.com.
It has been demonstrated that such immunocompromised mice models have served a pivotal role in understanding various cancers. For instance, both SCID and NOD/SCID mice were deemed a vital step forward in the study of acute myeloid leukemia (AML) (23). Relative to athymic nude mice, the SCID model led to improved engraftment rates of primary AML when injected intraperitoneally or implanted under the kidney capsules, and was found to be a reproducible system for the expansion of blastic human myeloid leukemias (24). NOD/SCID mice, with reduced NK cell and macrophage activity, produced superior engraftment rates and seemed to mostly preserve the morphologic and phenotypic characteristics of AML (25). Agliano et al. (18) have also systematically compared the transplantation of primary AML cells into NOD/SCID, NSB, and NSG strains and highlighted the faster engraftment observed in the latter. Other models have been similarly helpful in exploring head and neck squamous cell carcinoma, pancreatic cancer, non-small cell lung cancer, and prostate cancer, to name a few (26–29).
At present, the primary concern surrounding the use of immunocompromised mice is their limited replicability from preclinical to human trials. These models may have exceeded their capabilities as they are unable to faithfully represent the complex tumor microenvironment that is necessary for an accurate evaluation of immunotherapeutic approaches in humans (30). Nevertheless, they have been and continue to be instrumental in discerning disease pathology and serve as a bridge from bench to bedside therapies.
Mouse models currently exist for chordoma research utilizing immunocompromised mice. Chordoma mouse models began with three different cell lines, each capable of forming a tumor after being implanted in immunocompromised mice (31–33). However, the first primary chordoma patient derived xenograft mouse model transplanted directly from a human patient was established in 2012 (34). Eventually this model of xenograft was modified to enable serial transplantation of primary chordoma tumors in mice (35). Athymic nude mice are the basis of many tumor grafting experiments due to the decreased likelihood of tumor rejection. This makes them a common model for chordoma xenografting as well through subcutaneous injection to the flank of cells combined with Matrigel (36).
A common specific mouse model used for cellular derived xenograft (CDX) and patient derived xenograft (PDX) in chordoma are BALB/C nude mice. These are athymic mice which are used as xenograft recipients through a subcutaneous injection of cells combined with Matrigel into either the hindleg, thigh, forearm, flank, and inguinal fold, all with success (37–46).
Another popular method for PDX and CDX in chordoma are SCID variants of mice, differing from the most rudimentary model of SCID to the most modern NOD-SCID interleukin 2 receptor gamma (NSG) null mice (33, 47–57). These models follow suit with other studies, injecting the cells into various locations subcutaneously. The exception to this only study that implanted the cells into the subscapular fat pad of the mice (58).
Other models of PDX chordoma in SCID mice have also been done to try to emulate the growth of clival chordomas. For example, one model implants the cells subcutaneously in the epicranial space, creating an anatomical environment closer to the tumor in humans (59). Other models exist that use similar models but are less commonly seen in chordoma studies. Such as the use of athymic CD-1 nu/nu mice (60). Nearly every model uses subcutaneous injections of cells into mice. However, one student implanted chordoma cells into scraped areas of the sacrum in addition to doing subcutaneous flank injections (61). This method yielded a statistically significant 23% increase in engraftment success in the scraped sacrum compared to the commonly used flank.
While these models are excellent for studying chordoma in vivo, they lack the capacity to study the tumor microenvironment created in an immunocompetent human host. The tumor microenvironment has been shown to be critical in understanding the progression of chordoma and outcomes in patients (62, 63).
In recent years, there has been a significant advancement in our comprehension of the interactions between oncogenesis and the host’s immune system. Concurrently, there has been remarkable progress in the development of therapeutics aimed at augmenting the immune system’s anti-tumoral responses. Notably, immune checkpoint blockade therapy, exemplified by Keytruda® (pembrolizumab), an FDA-approved humanized monoclonal antibody that binds to the PD-1 receptor on T-cells, preventing tumor cells from suppressing the immune response (64, 65) has emerged as one of the most successful treatments available to the public. As a result, there is a growing demand for a model that can faithfully replicate the human immune system to evaluate new therapies. One such model is the humanized mice.
In broad terms, humanized mice refer to mice that have been generated or bioengineered by incorporating human cells or tissues into the mouse model. This concept extends beyond models of immunity and encompasses various types of cells, tissues, or molecular components from the human system. For instance, mice that are immunocompetent and express human genes through transgenesis are categorized as humanized mice. These mice can carry multiple transgenes, whether or not they are associated with the immune system. Over the past three decades, numerous scientific studies have utilized these models to introduce a variety of human genes, both within and outside the realm of immunology (66, 67), models that are very useful because they can inherit and express human genes (68). To clarify, the term “humanized/immunocompetent” as discussed refers to the use of immunocompromised mice that have been engrafted with functional human cells or tissues, resulting in the development of a functional human immune system within the mouse model. These mice have become increasingly important over the past decades as preclinical models of disease (69).
Immunocompetent or humanized have been used since 1988 when SCID mice were transplanted with human hematolymphoid organs: fetal bone, liver, thymus, spleen, intestine, skin, and lymph node (70). The breakthrough that allowed the study of HIV pathogenesis not only paved the way for research on infectious diseases but also unlocked various applications for humanized mice in other fields, including cancer (71). During the past 3 decades, diverse protocols have been presented to generate humanized mice (72). The mice that are most commonly used to create humanized mice are the SCID model-based (SCID, NOD, NSD or NSG) that, as explained above, its genetic disorder is characterized by the complete disability of the adaptive immune system to generate and sustain an immune response, due to the lack of B and T cells (13, 72–75). These mice can be stably engrafted since they lack this response.
In this context, humanized mice can be classified into two categories depending on the type of human immune or hematopoietic cells that are engrafted. Mice can be engrafted with human CD34+ HSCs, hCD34+, or they can be engrafted with human PBMCs, hPBMC.
The hu-SRC mice, which stand for human SCID repopulating cell mice, are the type of immunocompromised mice that serve as recipients for human CD34+ HSCs. These mice produce T, B, and myeloid cells, especially Natural Killer (NK) cells, derived from the engrafted CD34+ HSCs. The tissue sources of CD34+ cells in this engraftment process are human bone marrow, cord blood, or mobilized peripheral blood. However, the percentage of CD34+ varies depending on the tissue source or the mobilization agent (76, 77). Importantly, these cells mature within the mouse host, leading to the development of tolerance to the host’s antigens. This unique characteristic allows researchers to utilize this model for studying not only the development of the hematopoietic lineage but also the mechanisms involved in immune system development and the generation of primary immune responses, particularly with naïve T cells (77). Moreover, the lifespan of the newly acquired human immune cells results in a 12-week window before exhaustion. This timeframe allows for the study of immune cell memory response and long-term antitumor effects (78). Furthermore, this model facilitates the study of drug immunogenicity as it possesses a complete human immune system (79).
The humanized hu-PBL mice, also known as human peripheral blood leukocyte mice (hPBMC), are NSG immunocompromised mice engrafted with a pool of human PBMCs, resulting in the creation of mouse chimeras (80). These mice are often considered transiently humanized mice since the mature T cells can last between a few weeks and, much less often, a few months (81). This is caused by the Graft-versus-host disease (GvHD), which is usually manifested at week 4 after engraftment. Since the engrafted cells are already matured, they end up rejecting the host tissues given the differences in the major histocompatibility complex (MHC) (82). The advantage lies in the cost-effectiveness of this model, albeit with a limitation on experiment duration. Experiments conducted within a concise timeframe, as seen in cancer research, specifically focus on T and NK cells, including investigations into immune checkpoints (83).
In both PBMC and HSC-engrafted mice models, human immune cells naturally develop, migrate, and infiltrate the tumor microenvironment, mirroring the conditions observed in primary patient tumors. This model is extremely useful for cancer research since it provides a model to study tumor-immune system interactions (13, 30, 69, 72, 74, 75, 84). An overview of these two types of humanized mice is summarized in Table 1.
Table 1 A comparison between the humanized mice models hPBMC and hCD34+. Created with BioRender.com
Both immunological models of mice humanization can be performed on different immunocompromised strains, being the NSG™ (from Jackson Laboratories) and the NOG (from Tactonic) the most commonly used to create the humanized models.
As mentioned earlier, NSG mice, which are immunodeficient (NOD/SCID) mice with a specific mutation in the IL-2 receptor common gamma chain (IL2rγ null), exhibit significantly enhanced engraftment capabilities. These mice are extensively employed in research related to immunity, infectious diseases, cancer biology, regenerative medicine, and various other fields (72, 74). The generation of commercially available cohorts of stem cell humanized mice starts by the treatment of the NSG/NOG mice with busulfan to clear the stem cell niches, alternative to the irradiation, first described at Tisdale’s laboratory (85). This approach enhances the engraftment of CD34+ human hematopoietic stem cells derived from cord blood, resulting in the development of chimeric human CD45+ (hCD45+) fully matured B and T lymphocytes within 4 to 5 months, all while minimizing associated toxicity (85–90). These engrafted cells differentiate into various immune cell lineages, resulting in a chimeric mouse possessing a human immune system. This system is characterized by hematopoietic stem cells reconstituting into CD4+ T helper cells and cytotoxic CD8+ T cells, along with reduced levels of B cells, macrophages, NK cells, and dendritic cells (89–91). Moreover, new transgenic strains that express human hematopoietic growth factors have conferred these mice with a more robust immune system and with the expression of human HLA molecules (92).
The benefit of utilizing commercially available humanized mice is that the companies typically assess chimerism and engraftment levels in the animals before they are shipped. However, a drawback of this approach is that it may limit the experimental window, potentially reducing the time available for conducting experiments, as humanized mice suffer a shortened survival time and may have HSC exhaustion (93).
The use of humanized mice to study immunotherapies has served to the advancement of medical research, to reduce animal testing and to accelerate drug testing and development (69, 94, 95). For example, the immune checkpoint inhibitor that we started this section talking about, keytruda®, has been extensively used in humanized mice since its commercialization in 2013 in a wide array of cancers and in combination therapies (96–106).
However, similar to any model, humanized mice have their practical limitations. For example, they may not completely replicate the entire immune system, potentially resulting in suboptimal functionality of components like B and NK cells. Nevertheless, researchers have the opportunity to explore specific protocol adjustments to promote the maturation of specific cell populations in these mice (107–109). Another drawback pertains to the potentially high cost for researchers, especially if they opt to purchase commercially available humanized animals. Additionally, experiments may be constrained by the shipping and engraftment schedules of the companies, and the humanization status will not be retained if these animals reproduce. Moreover, when attempting in-house humanization, graft-rejection rates can be quite high, as the innate murine immune system, albeit diminished, remains present (110). In some robust transgenic models and in the transiently humanized mice, acquired innate immune response from the HSC may reject some PDX models if there is a Major histocompatibility complex (MHC)-mismatch between the engrafted HSC and the PDX. This is precisely why these models are particularly valuable for studying graft-versus-host disease in the transplantation (75, 92, 110–116). However, as previously described, transiently humanized mice may allow the use of specific HSCs that are histocompatible with the PDX models, enabling the incorporation of patient-derived cells or tissues, facilitating personalized medicine (79, 114). On the other hand, models of humanized mouse models lack the ability to process and present antigens efficiently, primarily due to deficient B cells in responding to foreign antigens. This is crucial for mounting an effective defense against infections (75, 117, 118).
Tumor cells are not isolated entities; instead, they coexist within a complex environment made up of various cell types, extracellular matrix, and other molecular components. A tumor isn’t solely a cluster of cancer cells; it represents a heterogeneous collection of many different types of resident and infiltrating cells, secreted factors and the ECM. This complex entity is commonly referred as the tumor microenvironment (TME) (119–121). It has been suggested that the TME is not just a silent bystander, but plays a crucial role in cancer progression, either promoting or inhibiting cancer growth and metastasis depending on its composition (121).
Immune cells are a major component of the TME and its presence and activity can greatly influence cancer progression. Tumor-infiltrating lymphocytes (TILs), such as cytotoxic T cells, and Natural Killer (NK) cells can recognize and attack the cancer cells. However, tumor cells have different mechanisms to evade the immune system, such as immune checkpoints, that allows cancer to thrive (122). Understanding the complexity and the mechanisms for which the tumor immune microenvironment (TIME) affects the tumor may unveil immunotherapies (123–125). The TIME refers to the different subpopulations of the immune system that may infiltrate the tumor at a certain time and that may have different effects in tumor initiation, progression and response to therapies (124, 126, 127).
While immunocompromised mice have been valuable options for in vivo cancer research, emerging case studies, cellular data, and clinical trial findings related to the immunological alterations observed in chordoma, as well as the potential chordoma therapies that leverage the immune system, underscore the significance of possessing a representative model of the human immune system. Such a model is essential for comprehensively characterizing the underlying mechanisms involved in chordoma and its potential therapeutic strategies (128–135). As previously discussed, TME plays a crucial role in tumor progression, and this is particularly true for the TIME, which can significantly influence the malignancy of tumor cells, including chordoma. For instance, tumor-associated macrophages, which are among the most prevalent cell types within the TIME and infiltrate the chordoma tumor parenchyma, are highly versatile macrophages capable of transitioning between M1 and M2 phenotypes depending on the specific requirements of the tumor (136). Therefore, it has been suggested that one of the ways to target cancer is to enhance immune regulation (137, 138). For example, the programmed death-1 (PD-1)/programmed death ligand-1 (PD-L1) pathway has been widely studied by Zou and colleagues (35, 62, 128, 139, 140) as TILs and macrophages express PD-1 receptor (138). Besides the PD1/PDL1 pathway, TGF-beta-related genes, HHLA2, and cytotoxic T-lymphocyte antigen-4 (CTLA-4) are among some of the immune-associated molecules analyzed so far (128, 129, 131). Other examples of interactions between chordoma and the immune system are the above mentioned plastic macrophages that, via the ccl5/ccr5 axis, are recruited and polarized by chordoma to enhance the proliferation, invasion and migration capabilities of chordoma (136); galectin-9 that interacts with TIM3-positive TILs and that promotes cell apoptosis (141) and the expression of CTLA-4, a promising immune checkpoint inhibitor target that it is expressed by the TILs that are infiltrating chordoma (129). We can learn from these examples that chordoma can evade the immune system by evading the recognition via antigen restriction by the TIL and marrow-infiltrating lymphocytes (MIL), or by directly interacting with the immune system and inhibiting it or by causing T cell exhaustion, as happens in many other cancers (142). Moreover, not only the TIME can regulate immune escape, it has been suggested in other cancers that can also promote distant metastasis and the acquired resistance to chemotherapy and radiotherapy (143–145). CAR-modified T cells that could inhibit the molecule B7-H3 are also being explored in chordoma (146). Various types of humanized mice have been used to assess therapies for other cancers and could potentially be applied to chordoma as well (147).
As we discussed above, most of the immunocompromised mice lack one or more cells from the immune system so the host doesn’t reject the human cell/graft. For example, NU/J athymic nude mice lack T cells and that partially affects B cells maturation. However, understanding the complex interactions between the immune system, the TME, the TIME and the tumor itself is crucial for, not only the development of new immunotherapies, but also takes into account the effect of these interactions in cell transformation and resistance to therapies. Humanized mice are then valuable tools for studying cancer and the interactions between the human immune system and tumor cells. These mice are engineered to generate functional human immune cells, enabling the research of the complex dynamics of the human immune response within a living organism, providing a bridge between in vitro studies and clinical trials. Also, they provide a system that is ideal for targeting immunotherapies that involve T and B cells responses. Also, it is a system that is ideal for the PDXs as it more closely recapitulates the tumor heterogeneity and the TIME interactions, being the most predictive preclinical oncological model.
Even though immunocompromised mice have served to obtain an immense amount of knowledge on basic biology, there are limitations on the use to study human biology and disease. The first of all is that the host innate immune system of a mouse is different from the immune system of a human. That’s why many drugs and pathogens are species-specific (148). Immunocompromised mice are leading to the implementation of personalized medicine: engraftment of mice with patient-derived tumors allows researchers to find and evaluate specific therapies that can ultimately help the patient. Humanized mice not only allow the study of immunotherapies that depend on the engrafted immune system, it also gives the researcher context on the response of the tumor cells and the TIME against any other chemotherapeutic drug and even combination of drugs (149). Moreover, they are a good model on investigating mechanisms of tumor metastasis and to study the immune checkpoint mechanisms (69, 149). It has also been demonstrated that the effect of the TME and the TIME may lead to intra-tumoral heterogeneity and differential chordoma classification that may lead to differential prognosis and response to treatment (150–155).
Previously, altered expression of some genes was found to be associated with chordoma, and molecular-based treatments may mitigate the downstream changes instigated by this genetic variability (34). In vivo cancer models, as compared to in vitro studies, may more accurately reflect dynamic molecular changes, including genetic expression, of tumors (156), and these models are therefore a crucial component to the research process. Prior attempts to grow chordoma in immunocompromised mice produced tumors that were largely histologically and genetically akin to the original patient-derived cells. However, with researchers exploring immunotherapy as an additional route of chordoma treatment, an equally effective in vivo model preserving immune function is needed to determine efficacy.
Lim et al. (2015) noted this limitation. They identified PD-1 expression in chordoma immune cells in vitro, potentially implicating this molecule’s interaction with PD-L1 receptors on chordoma cells in cancer cell survival (157). A partnership with Jackson Laboratories led to novel development of a humanized mouse for chordoma in 2015 (156). A team of researchers, which included those involved in the creation of this mouse, initiated a study calling for the need to investigate chordomas through such mice, wherein the immune system would be compatible with the human chordoma cells (158). They then utilized a humanized mouse to evaluate the efficacy of potential chordoma treatments. Interestingly, the study identified that treatments against PD-1 adjuvant to radiation resulted in relatively few cytotoxic T cells expressing PD-1 and high levels of several other types of human immune cells alongside a reduced tumor size (159). Since immunodeficient mice do not possess these other immune cells, these cells’ involvement in tumor suppression following immunotherapy—such as in this context of attenuated PD-1 and PD-L1 binding—may be insufficiently studied without a humanized mouse model. As we already mentioned, Xu et al. (136) found that ccl5 and M2 macrophages drive chordoma growth, and while they acquired this data through use of an immunodeficient mouse, the lack of a humanized mouse limited the team’s ability to assess the underlying molecular characteristics. Another research team from Northwestern University has also proposed a project to create a humanized mouse for the study of chordoma therapies (160). Other research has further elucidated potential immunotherapies for chordoma, and a humanized mouse model may enable more extensive in vivo studies of these therapy candidates. Gounder et al. (2019) showed through clinical trial data that impeding EZH2 activity in a chordoma patient extended the expected survival while changing the immune composition of the tumor (161). Hoke et al. (2019) and Fujii et al. (2020) enhanced natural killer cell effects in vitro by targeting the epidermal growth factor receptor and other ligands and cytokines to activate antibody-dependent cell-mediated cytotoxicity (ADCC) (162, 163).
Chordoma possesses diverse genetic, epigenetic, and immunotherapeutic targets that may be a target for combinatorial therapies (164), which will need the appropriate models, from in vitro to the different animal models to test those therapies. In summary, immunocompromised mice are useful for studying tumor growth and responses to treatment independently of the immune system interference, while humanized mice provide a platform to study the interactions between human tumors and the human tumor immune microenvironment (Summarized in Table 2). The choice between the two models, and the different array of models within each one, will depend on the research questions being asked.
Table 2 A comparison of the applications of immunocompromised and humanized mouse models in cancer and tumor microenvironment studies. Created with BioRender.com.
Humanized mice models provide a platform with which to investigate different cancers in the context of the tumor microenvironment and the field of immuno-oncology (75, 115, 165). These models are generally generated by the engraftment of HSCs or PMBCs into immunocompromised mice to mimic the human immune system and are very useful for the implantation of human cancer CDXs and PDXs. Even though some humanized mice approaches have been made in relation to chordoma, there is a wide array of possibilities that can be applied to chordoma research to find therapies. In conclusion, there is rationale for the use of humanized mice to study chordoma in the context of the TME.
BC: Conceptualization, Investigation, Writing – original draft, Writing – review & editing. CS: Conceptualization, Investigation, Writing – original draft, Writing – review & editing. ES: Conceptualization, Investigation, Writing – original draft, Writing – review & editing. JA: Investigation, Writing – original draft, Writing – review & editing. OL: Investigation, Writing – original draft, Writing – review & editing. ZG: Writing – review & editing, Supervision. PS: Supervision, Writing – review & editing, Conceptualization. MM-M: Conceptualization, Supervision, Writing – review & editing, Formal analysis, Investigation, Writing – original draft.
The author(s) declare that no financial support was received for the research, authorship, and/or publication of this article.
The authors declare that the research was conducted in the absence of any commercial or financial relationships that could be construed as a potential conflict of interest.
The author(s) declared that they were an editorial board member of Frontiers, at the time of submission. This had no impact on the peer review process and the final decision.
All claims expressed in this article are solely those of the authors and do not necessarily represent those of their affiliated organizations, or those of the publisher, the editors and the reviewers. Any product that may be evaluated in this article, or claim that may be made by its manufacturer, is not guaranteed or endorsed by the publisher.
1. Bryda EC. The mighty mouse: the impact of rodents on advances in biomedical research. Mo Med. (2013) 110:207–11.
2. Frezza AM, Botta L, Trama A, Dei Tos AP, Stacchiotti S. Chordoma: update on disease, epidemiology, biology and medical therapies. Curr Opin Oncol. (2019) 31:114–20. doi: 10.1097/CCO.0000000000000502
3. McMaster ML, Goldstein AM, Bromley CM, Ishibe N, Parry DM. Chordoma: incidence and survival patterns in the United States, 1973-1995. Cancer Causes Control. (2001) 12:1–11. doi: 10.1023/a:1008947301735
4. Beccaria K, Sainte-Rose C, Zerah M, Puget S. Paediatric chordomas. Orphanet J Rare Dis. (2015) 10:116. doi: 10.1186/s13023-015-0340-8
5. Harbour JW, Lawton MT, Criscuolo GR, Holliday MJ, Mattox DE, Long DM. Clivus chordoma: a report of 12 recent cases and review of the literature. Skull Base Surg. (1991) 1:200–6. doi: 10.1055/s-2008-1057099
6. Boriani S, Bandiera S, Biagini R, Bacchini P, Boriani L, Cappuccio M, et al. Chordoma of the mobile spine: fifty years of experience. Spine (Phila Pa 1976). (2006) 31:493–503. doi: 10.1097/01.brs.0000200038.30869.27
7. Jeys L, Gibbins R, Evans G, Grimer R. Sacral chordoma: a diagnosis not to be sat on? Int Orthop. (2008) 32:269–72. doi: 10.1007/s00264-006-0296-3
8. Kaiser TE, Pritchard DJ, Unni KK. Clinicopathologic study of sacrococcygeal chordoma. Cancer. (1984) 53:2574–8. doi: 10.1002/1097-0142(19840601)53:11<2574::aid-cncr2820531136>3.0.co;2-5
9. Moojen WA, Vleggeert-Lankamp CLA, Krol ADG, Dijkstra SPD. Long-term results: adjuvant radiotherapy in en bloc resection of sacrococcygeal chordoma is advisable. Spine (Phila Pa 1976). (2011) 36:E656–661. doi: 10.1097/BRS.0b013e3181f8d1f3
10. Stacchiotti S, Sommer J. Building a global consensus approach to chordoma: a position paper from the medical and patient community. Lancet Oncol. (2015) 16:e71–83. doi: 10.1016/S1470-2045(14)71190-8
11. Yang Q-E. Human cancer xenografts in immunocompromised mice provide an advanced genuine tumor model for research and drug development-A revisit of murine models for human cancers. Biochim Biophys Acta Gen Subj. (2021) 1865:129929. doi: 10.1016/j.bbagen.2021.129929
12. Li E, Lin L, Chen C-W, Ou D-L. Mouse models for immunotherapy in hepatocellular carcinoma. Cancers (Basel). (2019) 11:1800. doi: 10.3390/cancers11111800
13. Olson B, Li Y, Lin Y, Liu ET, Patnaik A. Mouse models for cancer immunotherapy research. Cancer Discovery. (2018) 8:1358–65. doi: 10.1158/2159-8290.CD-18-0044
14. Flanagan SP. “Nude”, a new hairless gene with pleiotropic effects in the mouse. Genet Res. (1966) 8:295–309. doi: 10.1017/s0016672300010168
15. Mosier DE, Gulizia RJ, Baird SM, Wilson DB. Transfer of a functional human immune system to mice with severe combined immunodeficiency. Nature. (1988) 335:256–9. doi: 10.1038/335256a0
16. Roder J, Duwe A. The beige mutation in the mouse selectively impairs natural killer cell function. Nature. (1979) 278:451–3. doi: 10.1038/278451a0
17. Okada S, Vaeteewoottacharn K, Kariya R. Application of highly immunocompromised mice for the establishment of patient-derived xenograft (PDX) models. Cells. (2019) 8:889. doi: 10.3390/cells8080889
18. Agliano A, Martin-Padura I, Mancuso P, Marighetti P, Rabascio C, Pruneri G, et al. Human acute leukemia cells injected in NOD/LtSz-scid/IL-2Rgamma null mice generate a faster and more efficient disease compared to other NOD/scid-related strains. Int J Cancer. (2008) 123:2222–7. doi: 10.1002/ijc.23772
19. Okada S, Harada H, Ito T, Saito T, Suzu S. Early development of human hematopoietic and acquired immune systems in new born NOD/Scid/Jak3null mice intrahepatic engrafted with cord blood-derived CD34 + cells. Int J Hematol. (2008) 88:476–82. doi: 10.1007/s12185-008-0215-z
20. Ito M, Hiramatsu H, Kobayashi K, Suzue K, Kawahata M, Hioki K, et al. NOD/SCID/gamma(c)(null) mouse: an excellent recipient mouse model for engraftment of human cells. Blood. (2002) 100:3175–82. doi: 10.1182/blood-2001-12-0207
21. Shultz LD, Lyons BL, Burzenski LM, Gott B, Chen X, Chaleff S, et al. Human lymphoid and myeloid cell development in NOD/LtSz-scid IL2R gamma null mice engrafted with mobilized human hemopoietic stem cells. J Immunol. (2005) 174:6477–89. doi: 10.4049/jimmunol.174.10.6477
22. Zhou Q, Facciponte J, Jin M, Shen Q, Lin Q. Humanized NOD-SCID IL2rg–/– mice as a preclinical model for cancer research and its potential use for individualized cancer therapies. Cancer Lett. (2014) 344:13–9. doi: 10.1016/j.canlet.2013.10.015
23. Almosailleakh M, Schwaller J. Murine models of acute myeloid leukaemia. Int J Mol Sci. (2019) 20:453. doi: 10.3390/ijms20020453
24. Sawyers CL, Gishizky ML, Quan S, Golde DW, Witte ON. Propagation of human blastic myeloid leukemias in the SCID mouse. Blood. (1992) 79:2089–98. doi: 10.1182/blood.V79.8.2089.2089
25. Bonnet D, Dick JE. Human acute myeloid leukemia is organized as a hierarchy that originates from a primitive hematopoietic cell. Nat Med. (1997) 3:730–7. doi: 10.1038/nm0797-730
26. Lei Z-G, Ren X-H, Wang S-S, Liang X-H, Tang Y-L. Immunocompromised and immunocompetent mouse models for head and neck squamous cell carcinoma. Onco Targets Ther. (2016) 9:545–55. doi: 10.2147/OTT.S95633
27. Mallya K, Gautam SK, Aithal A, Batra SK, Jain M. Modeling pancreatic cancer in mice for experimental therapeutics. Biochim Biophys Acta Rev Cancer. (2021) 1876:188554. doi: 10.1016/j.bbcan.2021.188554
28. Owonikoko TK, Zhang G, Kim HS, Stinson RM, Bechara R, Zhang C, et al. Patient-derived xenografts faithfully replicated clinical outcome in a phase II co-clinical trial of arsenic trioxide in relapsed small cell lung cancer. J Transl Med. (2016) 14:111. doi: 10.1186/s12967-016-0861-5
29. Wetterauer C, Vlajnic T, Schüler J, Gsponer JR, Thalmann GN, Cecchini M, et al. Early development of human lymphomas in a prostate cancer xenograft program using triple knock-out immunocompromised mice. Prostate. (2015) 75:585–92. doi: 10.1002/pros.22939
30. Chulpanova DS, Kitaeva KV, Rutland CS, Rizvanov AA, Solovyeva VV. Mouse tumor models for advanced cancer immunotherapy. Int J Mol Sci. (2020) 21:4118. doi: 10.3390/ijms21114118
31. Rinner B, Froehlich EV, Buerger K, Knausz H, Lohberger B, Scheipl S, et al. Establishment and detailed functional and molecular genetic characterisation of a novel sacral chordoma cell line, MUG-Chor1. Int J Oncol. (2012) 40:443–51. doi: 10.3892/ijo.2011.1235
32. Hsu W, Mohyeldin A, Shah SR, ap Rhys CM, Johnson LF, Sedora-Roman NI, et al. Generation of chordoma cell line JHC7 and the identification of Brachyury as a novel molecular target. J Neurosurg. (2011) 115:760–9. doi: 10.3171/2011.5.JNS11185
33. Liu X, Nielsen GP, Rosenberg AE, Waterman PR, Yang W, Choy E, et al. Establishment and characterization of a novel chordoma cell line: CH22. J Orthop Res. (2012) 30:1666–73. doi: 10.1002/jor.22113
34. Siu I-M, Salmasi V, Orr BA, Zhao Q, Binder ZA, Tran C, et al. Establishment and characterization of a primary human chordoma xenograft model: Laboratory investigation. J Neurosurg. (2012) 116:801–9. doi: 10.3171/2011.12.JNS111123
35. Zou M-X, Lv G-H, Wang X-B, Huang W, Li J, Jiang Y, et al. Clinical impact of the immune microenvironment in spinal chordoma: immunoscore as an independent favorable prognostic factor. Neurosurgery. (2019) 84:E318–33. doi: 10.1093/neuros/nyy274
36. Kim J-Y, Lee J, Koh J-S, Park M-J, Chang U-K. Establishment and characterization of a chordoma cell line from the tissue of a patient with dedifferentiated-type chordoma. J Neurosurgery: Spine. (2016) 25:626–35. doi: 10.3171/2016.3.SPINE151077
37. Zhang K, Liu Z, Wang Z, Zhou Z, Shao X, Hua X, et al. Long non-coding RNA MDFIC-7 promotes chordoma progression through modulating the miR-525-5p/ARF6 axis. Front Oncol. (2021) 11:743718. doi: 10.3389/fonc.2021.743718
38. Li M, Shen Y, Xiong Y, Wang S, Li C, Bai J, et al. Loss of SMARCB1 promotes autophagy and facilitates tumour progression in chordoma by transcriptionally activating ATG5. Cell Prolif. (2021) 54:e13136. doi: 10.1111/cpr.13136
39. Wang L, Tang L, Xu R, Ma J, Tian K, Liu Y, et al. DEPDC1B regulates the progression of human chordoma through UBE2T-mediated ubiquitination of BIRC5. Cell Death Dis. (2021) 12:753. doi: 10.1038/s41419-021-04026-7
40. Yang J, Huang H, Xiao D, Duan Y, Zheng Y, Chen Z. Knockdown of TMED3 inhibits cell viability and migration and increases apoptosis in human chordoma cells. Int J Oncol. (2021) 58:15. doi: 10.3892/ijo.2021.5195
41. Gong F, Wang X, Sun Q, Su X, Hu X, Liu B. Long non-coding RNA LINC00525 interacts with miR-31-5p and miR-125a-5p to act as an oncogenic molecule in spinal chordoma. Biochem Biophys Res Commun. (2021) 536:80–7. doi: 10.1016/j.bbrc.2020.12.042
42. Chen L, Zuo Y, Pan R, Ye Z, Wei K, Xia S, et al. GSK-3β Regulates the expression of P21 to promote the progression of chordoma. Cancer Manag Res. (2021) 13:201–14. doi: 10.2147/CMAR.S289883
43. Liu L, Wang T, Yang X, Xu C, Liao Z, Wang X, et al. MTNR1B loss promotes chordoma recurrence by abrogating melatonin-mediated β-catenin signaling repression. J Pineal Res. (2019) 67:e12588. doi: 10.1111/jpi.12588
44. Zhang H, Yang K, Ren T, Huang Y, Tang X, Guo W. miR-16-5p inhibits chordoma cell proliferation, invasion and metastasis by targeting Smad3. Cell Death Dis. (2018) 9:680. doi: 10.1038/s41419-018-0738-z
45. Magnaghi P, Salom B, Cozzi L, Amboldi N, Ballinari D, Tamborini E, et al. Afatinib is a new therapeutic approach in chordoma with a unique ability to target EGFR and brachyury. Mol Cancer Ther. (2018) 17:603–13. doi: 10.1158/1535-7163.MCT-17-0324
46. Xu G, Liu J, He J, He H, Su X, Gui Q. LOC554202 contributes to chordoma progression by sponging miR-377-3p and up-regulating SMAD3. Anticancer Drugs. (2022) 34(1):15–28. doi: 10.1097/CAD.0000000000001327
47. Walker CJ, Chang H, Henegar L, Kashyap T, Shacham S, Sommer J, et al. Selinexor inhibits growth of patient derived chordomas in vivo as a single agent and in combination with abemaciclib through diverse mechanisms. Front Oncol. (2022) 12:808021. doi: 10.3389/fonc.2022.808021
48. Yuan W, Wei F, Ouyang H, Ren X, Hang J, Mo X, et al. CMTM3 suppresses chordoma progress through EGFR/STAT3 regulated EMT and TP53 signaling pathway. Cancer Cell Int. (2021) 21:510. doi: 10.1186/s12935-021-02159-5
49. Zhao T, Siu I-M, Williamson T, Zhang H, Ji C, Burger PC, et al. AZD8055 enhances in vivo efficacy of afatinib in chordomas. J Pathol. (2021) 255:72–83. doi: 10.1002/path.5739
50. Yang C, Yong L, Liang C, Li Y, Ma Y, Wei F, et al. Genetic landscape and ligand-dependent activation of sonic hedgehog-Gli1 signaling in chordomas: a novel therapeutic target. Oncogene. (2020) 39:4711–27. doi: 10.1038/s41388-020-1324-2
51. Michmerhuizen NL, Owen JH, Heft Neal ME, Mann JE, Leonard E, Wang J, et al. Rationale for the advancement of PI3K pathway inhibitors for personalized chordoma therapy. J Neurooncol. (2020) 147:25–35. doi: 10.1007/s11060-020-03418-7
52. Cao X, Lu Y, Liu Y, Zhou Y, Song H, Zhang W, et al. Combination of PARP inhibitor and temozolomide to suppress chordoma progression. J Mol Med (Berl). (2019) 97:1183–93. doi: 10.1007/s00109-019-01802-z
53. Owen JH, Komarck CM, Wang AC, Abuzeid WM, Keep RF, McKean EL, et al. UM-Chor1: establishment and characterization of the first validated clival chordoma cell line. J Neurosurg. (2018) 128:701–9. doi: 10.3171/2016.10.JNS16877
54. Ricci-Vitiani L, Runci D, D’Alessandris QG, Cenci T, Martini M, Bianchi F, et al. Chemotherapy of skull base chordoma tailored on responsiveness of patient-derived tumor cells to rapamycin. Neoplasia. (2013) 15:773–82. doi: 10.1593/neo.13150
55. Presneau N, Shalaby A, Ye H, Pillay N, Halai D, Idowu B, et al. Role of the transcription factor T (brachyury) in the pathogenesis of sporadic chordoma: a genetic and functional-based study. J Pathol. (2011) 223:327–35. doi: 10.1002/path.2816
56. Davies JM, Robinson AE, Cowdrey C, Mummaneni PV, Ducker GS, Shokat KM, et al. Generation of a patient-derived chordoma xenograft and characterization of the phosphoproteome in a recurrent chordoma. J Neurosurg. (2014) 120:331–6. doi: 10.3171/2013.10.JNS13598
57. Kesari S, Wang F, Juarez T, Ashili S, Patro CPK, Carrillo J, et al. Activity of pemetrexed in pre-clinical chordoma models and humans. Sci Rep. (2023) 13:7317. doi: 10.1038/s41598-023-34404-4
58. Passeri T, Dahmani A, Masliah-Planchon J, Naguez A, Michou M, El Botty R, et al. Dramatic in vivo efficacy of the EZH2-inhibitor tazemetostat in PBRM1-mutated human chordoma xenograft. Cancers. (2022) 14:1486. doi: 10.3390/cancers14061486
59. Diaz RJ, Luck A, Bondoc A, Golbourn B, Picard D, Remke M, et al. Characterization of a clival chordoma xenograft model reveals tumor genomic instability. Am J Pathol. (2018) 188:2902–11. doi: 10.1016/j.ajpath.2018.08.004
60. Bozzi F, Manenti G, Conca E, Stacchiotti S, Messina A, Dagrada G, et al. Development of transplantable human chordoma xenograft for preclinical assessment of novel therapeutic strategies. Neuro Oncol. (2014) 16:72–80. doi: 10.1093/neuonc/not238
61. Salle H, Pocard M, Lehmann-Che J, Bourthoumieu S, Labrousse F, Pimpie C, et al. Development of a novel orthotopic primary human chordoma xenograft model: A relevant support for future research on chordoma. J Neuropathol Exp Neurol. (2020) 79:314–24. doi: 10.1093/jnen/nlz121
62. Zou M-X, Zheng B-W, Liu F-S, Wang X-B, Hu J-R, Huang W, et al. The relationship between tumor-stroma ratio, the immune microenvironment, and survival in patients with spinal chordoma. Neurosurgery. (2019) 85:E1095–110. doi: 10.1093/neuros/nyz333
63. Patel P, Brooks C, Seneviratne A, Hess DA, Séguin CA. Investigating microenvironmental regulation of human chordoma cell behaviour. PloS One. (2014) 9:e115909. doi: 10.1371/journal.pone.0115909
64. Kardile V, Kulkarni A, Nadar B, Saldanha T. Monoclonal antibodies in oncology: A decade of novel options. Cell Biochem Biophys. (2023) 81:395–408. doi: 10.1007/s12013-023-01144-1
65. PD-1 inhibitor becomes “breakthrough therapy”. Cancer Discovery. (2013) 3:OF14. doi: 10.1158/2159-8290.CD-NB2013-074
66. Wang Y, Wang M, Bao R, Wang L, Du X, Qiu S, et al. A novel humanized tri-receptor transgenic mouse model of HAdV infection and pathogenesis. J Med Virol. (2023) 95:e29026. doi: 10.1002/jmv.29026
67. Murthy AK, Cong Y, Murphey C, Guentzel MN, Forsthuber TG, Zhong G, et al. Chlamydial protease-like activity factor induces protective immunity against genital chlamydial infection in transgenic mice that express the human HLA-DR4 allele. Infect Immun. (2006) 74:6722–9. doi: 10.1128/IAI.01119-06
68. Hickman-Davis JM, Davis IC. Transgenic mice. Paediatr Respir Rev. (2006) 7:49–53. doi: 10.1016/j.prrv.2005.09.005
69. Walsh N, Kenney L, Jangalwe S, Aryee K-E, Greiner DL, Brehm MA, et al. Humanized mouse models of clinical disease. Annu Rev Pathol. (2017) 12:187–215. doi: 10.1146/annurev-pathol-052016-100332
70. McCune JM, Namikawa R, Kaneshima H, Shultz LD, Lieberman M, Weissman IL. The SCID-hu mouse: murine model for the analysis of human hematolymphoid differentiation and function. Science. (1988) 241:1632–9. doi: 10.1126/science.241.4873.1632
71. McCune JM, Weissman IL. The ban on US government funding research using human fetal tissues: how does this fit with the NIH mission to advance medical science for the benefit of the citizenry? Stem Cell Rep. (2019) 13:777–86. doi: 10.1016/j.stemcr.2019.10.003
72. Pearson T, Greiner DL, Shultz LD. Creation of “Humanized” Mice to study human immunity. Curr Protoc Immunol. (2008) 81:15–21. doi: 10.1002/0471142735.im1521s81. CHAPTER:Unit-15.21.
73. Xia X, Li H, Satheesan S, Zhou J, Rossi JJ. Humanized NOD/SCID/IL2rγnull (hu-NSG) mouse model for HIV replication and latency studies. J Vis Exp. (2019) 143(JOVE). doi: 10.3791/58255. 10.3791/58255.
74. Pearson T, Greiner DL, Shultz LD. Humanized SCID mouse models for biomedical research. Curr Top Microbiol Immunol. (2008) 324:25–51. doi: 10.1007/978-3-540-75647-7_2
75. De La Rochere P, Guil-Luna S, Decaudin D, Azar G, Sidhu SS, Piaggio E. Humanized mice for the study of immuno-oncology. Trends Immunol. (2018) 39:748–63. doi: 10.1016/j.it.2018.07.001
76. Ivanovic Z. Hematopoietic stem cells in research and clinical applications: The “CD34 issue”. World J Stem Cells. (2010) 2:18–23. doi: 10.4252/wjsc.v2.i2.18
77. De Bruyn C, Delforge A, Lagneaux L, Bron D. Characterization of CD34+ subsets derived from bone marrow, umbilical cord blood and mobilized peripheral blood after stem cell factor and interleukin 3 stimulation. Bone Marrow Transplant. (2000) 25:377–83. doi: 10.1038/sj.bmt.1702145
78. Blümich S, Zdimerova H, Münz C, Kipar A, Pellegrini G. Human CD34+ Hematopoietic stem cell-engrafted NSG mice: morphological and immunophenotypic features. Vet Pathol. (2021) 58:161–80. doi: 10.1177/0300985820948822
79. Mehler VJ, Burns C, Moore ML. Concise review: exploring immunomodulatory features of mesenchymal stromal cells in humanized mouse models. Stem Cells. (2019) 37:298–305. doi: 10.1002/stem.2948
80. Tary-Lehmann M, Saxon A, Lehmann PV. The human immune system in hu-PBL-SCID mice. Immunol Today. (1995) 16:529–33. doi: 10.1016/0167-5699(95)80046-8
81. Barbeau J. When to Use Stable vs Transiently Humanized I/O Models - Crown Bioscience (2017). Available online at: https://blog.crownbio.com/stable-vs-transiently-humanized-immuno-oncology-model.
82. Haque M, Boardman DA, Lam AJ, MacDonald KN, Sanderink L, Huang Q, et al. Modelling graft-versus-host disease in mice using human peripheral blood mononuclear cells. Bio Protoc. (2022) 12:e4566. doi: 10.21769/BioProtoc.4566
83. Liu Y, Wu W, Cai C, Zhang H, Shen H, Han Y. Patient-derived xenograft models in cancer therapy: technologies and applications. Signal Transduct Target Ther. (2023) 8:160. doi: 10.1038/s41392-023-01419-2
84. Holzapfel BM, Wagner F, Thibaudeau L, Levesque J-P, Hutmacher DW. Concise review: humanized models of tumor immunology in the 21st century: convergence of cancer research and tissue engineering. Stem Cells. (2015) 33:1696–704. doi: 10.1002/stem.1978
85. Hayakawa J, Hsieh MM, Uchida N, Phang O, Tisdale JF. Busulfan produces efficient human cell engraftment in NOD/LtSz-scid IL2Rγ null mice. Stem Cells. (2009) 27:175–82. doi: 10.1634/stemcells.2008-0583
86. Kang YK, Ko Y, Choi A, Choi HJ, Seo J-H, Lee M, et al. Humanizing NOD/SCID/IL-2Rγnull (NSG) mice using busulfan and retro-orbital injection of umbilical cord blood-derived CD34+ cells. Blood Res. (2016) 51:31–6. doi: 10.5045/br.2016.51.1.31
87. Leonard A, Yapundich M, Nassehi T, Gamer J, Drysdale CM, Haro-Mora JJ, et al. Low-dose busulfan reduces human CD34+ Cell doses required for engraftment in c-kit mutant immunodeficient mice. Mol Ther - Methods Clin Dev. (2019) 15:430–7. doi: 10.1016/j.omtm.2019.10.017
88. Guo X, Yin X, Zhu W, Pan Y, Wang H, Liang Y, et al. The preconditioning of busulfan promotes efficiency of human CD133+ Cells engraftment in NOD shi-SCID IL2Rγcnull (NOG) mice via intra-bone marrow injection. Cell Transplant. (2019) 28:973–9. doi: 10.1177/0963689719842162
89. Chevaleyre J, Duchez P, Rodriguez L, Vlaski M, Villacreces A, Conrad-Lapostolle V, et al. Busulfan administration flexibility increases the applicability of scid repopulating cell assay in NSG mouse model. PloS One. (2013) 8:e74361. doi: 10.1371/journal.pone.0074361
90. Abraham DM, Lozano RJ, Guitart X, Liang JA, Mortlock RD, Espinoza DA, et al. Comparison of busulfan and total body irradiation conditioning on hematopoietic clonal dynamics following lentiviral gene transfer in rhesus macaques. Mol Ther - Methods Clin Dev. (2023) 28:62–75. doi: 10.1016/j.omtm.2022.12.001
91. Choi B, Chun E, Kim M, Kim S-T, Yoon K, Lee K-Y, et al. Human B cell development and antibody production in humanized NOD/SCID/IL-2Rγ(null) (NSG) mice conditioned by busulfan. J Clin Immunol. (2011) 31:253–64. doi: 10.1007/s10875-010-9478-2
92. Shultz LD, Brehm MA, Garcia JV, Greiner DL. Humanized mice for immune system investigation: progress, promise and challenges. Nat Rev Immunol. (2012) 12:786–98. doi: 10.1038/nri3311
93. Kumari R, Feuer G, Bourré L. Humanized mouse models for immuno-oncology drug discovery. Curr Protoc. (2023) 3:e852. doi: 10.1002/cpz1.852
94. Wang M, Yao L-C, Cheng M, Cai D, Martinek J, Pan C-X, et al. Humanized mice in studying efficacy and mechanisms of PD-1-targeted cancer immunotherapy. FASEB J. (2018) 32:1537–49. doi: 10.1096/fj.201700740R
95. Martinez-Lopez S, Angel-Gomis E, Sanchez-Ardid E, Pastor-Campos A, Picó J, Gomez-Hurtado I. The 3Rs in experimental liver disease. Animals. (2023) 13:2357. doi: 10.3390/ani13142357
96. Woods DM, Sodré AL, Villagra A, Sarnaik A, Sotomayor EM, Weber J. HDAC inhibition upregulates PD-1 ligands in melanoma and augments immunotherapy with PD-1 blockade. Cancer Immunol Res. (2015) 3:1375–85. doi: 10.1158/2326-6066.CIR-15-0077-T
97. Jespersen H, Lindberg MF, Donia M, Söderberg EMV, Andersen R, Keller U, et al. Clinical responses to adoptive T-cell transfer can be modeled in an autologous immune-humanized mouse model. Nat Commun. (2017) 8:707. doi: 10.1038/s41467-017-00786-z
98. Rosato RR, Dávila-González D, Choi DS, Qian W, Chen W, Kozielski AJ, et al. Evaluation of anti-PD-1-based therapy against triple-negative breast cancer patient-derived xenograft tumors engrafted in humanized mouse models. Breast Cancer Res. (2018) 20:108. doi: 10.1186/s13058-018-1037-4
99. Kuryk L, Møller A-SW, Jaderberg M. Combination of immunogenic oncolytic adenovirus ONCOS-102 with anti-PD-1 pembrolizumab exhibits synergistic antitumor effect in humanized A2058 melanoma huNOG mouse model. Oncoimmunology. (2019) 8:e1532763. doi: 10.1080/2162402X.2018.1532763
100. Meraz IM, Majidi M, Meng F, Shao R, Ha MJ, Neri S, et al. An improved patient-derived xenograft humanized mouse model for evaluation of lung cancer immune responses. Cancer Immunol Res. (2019) 7:1267–79. doi: 10.1158/2326-6066.CIR-18-0874
101. van der Veen EL, Giesen D, Pot-de Jong L, Jorritsma-Smit A, De Vries EGE, Lub-de Hooge MN. 89Zr-pembrolizumab biodistribution is influenced by PD-1-mediated uptake in lymphoid organs. J Immunother Cancer. (2020) 8:e000938. doi: 10.1136/jitc-2020-000938
102. Volk V, Theobald SJ, Danisch S, Khailaie S, Kalbarczyk M, Schneider A, et al. PD-1 blockade aggravates epstein-barr virus+ Post-transplant lymphoproliferative disorder in humanized mice resulting in central nervous system involvement and CD4+ T cell dysregulations. Front Oncol. (2020) 10:614876. doi: 10.3389/fonc.2020.614876
103. Lee YS, O’Brien LJ, Walpole CM, Pearson FE, Leal-Rojas IM, Masterman K-A, et al. Human CD141+ dendritic cells (cDC1) are impaired in patients with advanced melanoma but can be targeted to enhance anti-PD-1 in a humanized mouse model. J Immunother Cancer. (2021) 9:e001963. doi: 10.1136/jitc-2020-001963
104. Qiao T, Xiong Y, Feng Y, Guo W, Zhou Y, Zhao J, et al. Inhibition of LDH-A by oxamate enhances the efficacy of anti-PD-1 treatment in an NSCLC humanized mouse model. Front Oncol. (2021) 11:632364. doi: 10.3389/fonc.2021.632364
105. Wunderlich M, Manning N, Sexton C, O’Brien E, Byerly L, Stillwell C, et al. PD-1 inhibition enhances blinatumomab response in a UCB/PDX model of relapsed pediatric B-cell acute lymphoblastic leukemia. Front Oncol. (2021) 11:642466. doi: 10.3389/fonc.2021.642466
106. Li H, Liu Z, Liu L, Zhang H, Han C, Girard L, et al. AXL targeting restores PD-1 blockade sensitivity of STK11/LKB1 mutant NSCLC through expansion of TCF1+ CD8 T cells. Cell Rep Med. (2022) 3:100554. doi: 10.1016/j.xcrm.2022.100554
107. Brehm MA, Shultz LD, Luban J, Greiner DL. Overcoming current limitations in humanized mouse research. J Infect Dis. (2013) 208:S125–30. doi: 10.1093/infdis/jit319
108. Herndler-Brandstetter D, Shan L, Yao Y, Stecher C, Plajer V, Lietzenmayer M, et al. Humanized mouse model supports development, function, and tissue residency of human natural killer cells. Proc Natl Acad Sci. (2017) 114:E9626–34. doi: 10.1073/pnas.1705301114
109. Strowig T, Chijioke O, Carrega P, Arrey F, Meixlsperger S, Rämer PC, et al. Human NK cells of mice with reconstituted human immune system components require preactivation to acquire functional competence. Blood. (2010) 116:4158–67. doi: 10.1182/blood-2010-02-270678
110. Brehm MA, Shultz LD. Human allograft rejection in humanized mice: a historical perspective. Cell Mol Immunol. (2012) 9:225. doi: 10.1038/cmi.2011.64
111. Kenney LL, Shultz LD, Greiner DL, Brehm MA. Humanized mice and tissue transplantation. Am J Transplant. (2016) 16:389–97. doi: 10.1111/ajt.13520
112. Ajith A, Mulloy LL, Musa M, Bravo-Egana V, Horuzsko DD, Gani I, et al. Humanized mouse model as a novel approach in the assessment of human allogeneic responses in organ transplantation. Front Immunol. (2021) 12:687715. doi: 10.3389/fimmu.2021.687715
113. Kenney LL, Shultz LD, Greiner DL, Brehm MA. Humanized mouse models for transplant immunology. Am J Transplant. (2016) 16:389–97. doi: 10.1111/ajt.13520
114. Justiz Vaillant AA, Modi P, Mohammadi O. “Graft-versus-host disease.,” StatPearls. (2023). Treasure Island (FL: StatPearls Publishing. Available online at: http://www.ncbi.nlm.nih.gov/books/NBK538235/ (Accessed September 5, 2023).
115. Maurice Morillon Y, Sabzevari A, Schlom J, Greiner JW. The development of next-generation PBMC humanized mice for preclinical investigation of cancer immunotherapeutic agents. Anticancer Res. (2020) 40:5329–41. doi: 10.21873/anticanres.14540
116. Elhage A, Sligar C, Cuthbertson P, Watson D, Sluyter R. Insights into mechanisms of graft-versus-host disease through humanised mouse models. Biosci Rep. (2022) 42:BSR20211986. doi: 10.1042/BSR20211986
117. Haniffa M, Collin M, Ginhoux F. Chapter one - ontogeny and functional specialization of dendritic cells in human and mouse. In: Murphy KM, Merad M, editors. Advances in immunology. Development and function of myeloid subsets. Elsevier: Academic Press (2013). p. 1–49. doi: 10.1016/B978-0-12-417028-5.00001-6
118. Cruz-Tapias P, Castiblanco J, Anaya J-M. Major histocompatibility complex: Antigen processing and presentation, in: Autoimmunity: from bench to bedside [Internet]. (2013). El Rosario University Press. Available online at: https://www.ncbi.nlm.nih.gov/books/NBK459467/ (Accessed September 5, 2023).
119. Anderson NM, Simon MC. The tumor microenvironment. Curr Biol. (2020) 30:R921–5. doi: 10.1016/j.cub.2020.06.081
120. Baghban R, Roshangar L, Jahanban-Esfahlan R, Seidi K, Ebrahimi-Kalan A, Jaymand M, et al. Tumor microenvironment complexity and therapeutic implications at a glance. Cell Communication Signaling. (2020) 18:59. doi: 10.1186/s12964-020-0530-4
121. Truffi M, Sorrentino L, Corsi F. Fibroblasts in the tumor microenvironment. Adv Exp Med Biol. (2020) 1234:15–29. doi: 10.1007/978-3-030-37184-5_2
122. Pisibon C, Ouertani A, Bertolotto C, Ballotti R, Cheli Y. Immune checkpoints in cancers: from signaling to the clinic. Cancers (Basel). (2021) 13:4573. doi: 10.3390/cancers13184573
123. Binnewies M, Roberts EW, Kersten K, Chan V, Fearon DF, Merad M, et al. Understanding the tumor immune microenvironment (TIME) for effective therapy. Nat Med. (2018) 24:541–50. doi: 10.1038/s41591-018-0014-x
124. Surendran V, Rutledge D, Colmon R, Chandrasekaran A. A novel tumor-immune microenvironment (TIME)-on-Chip mimics three dimensional neutrophil-tumor dynamics and neutrophil extracellular traps (NETs)-mediated collective tumor invasion. Biofabrication. (2021) 13:035029. doi: 10.1088/1758-5090/abe1cf
125. Shinohara S, Takahashi Y, Komuro H, Matsui T, Sugita Y, Demachi-Okamura A, et al. New evaluation of the tumor immune microenvironment of non-small cell lung cancer and its association with prognosis. J Immunother Cancer. (2022) 10:e003765. doi: 10.1136/jitc-2021-003765
126. Xu L, Zou C, Zhang S, Chu TSM, Zhang Y, Chen W, et al. Reshaping the systemic tumor immune environment (STIE) and tumor immune microenvironment (TIME) to enhance immunotherapy efficacy in solid tumors. J Hematol Oncol. (2022) 15:87. doi: 10.1186/s13045-022-01307-2
127. Sun D, Liu J, Zhou H, Shi M, Sun J, Zhao S, et al. Classification of tumor immune microenvironment according to programmed death-ligand 1 expression and immune infiltration predicts response to immunotherapy plus chemotherapy in advanced patients with NSCLC. J Thorac Oncol. (2023) 18:869–81. doi: 10.1016/j.jtho.2023.03.012
128. Xia C, Huang W, Chen Y-L, Fu H-B, Tang M, Zhang T-L, et al. Coexpression of HHLA2 and PD-L1 on tumor cells independently predicts the survival of spinal chordoma patients. Front Immunol. (2021) 12:797407. doi: 10.3389/fimmu.2021.797407
129. He G, Liu X, Pan X, Ma Y, Liu X. Cytotoxic T lymphocyte antigen-4 (CTLA-4) expression in chordoma and tumor-infiltrating lymphocytes (TILs) predicts prognosis of spinal chordoma. Clin Transl Oncol. (2020) 22:2324–32. doi: 10.1007/s12094-020-02387-7
130. Migliorini D, Mach N, Aguiar D, Vernet R, Landis BN, Becker M, et al. First report of clinical responses to immunotherapy in 3 relapsing cases of chordoma after failure of standard therapies. OncoImmunology. (2017) 6:e1338235. doi: 10.1080/2162402X.2017.1338235
131. Freed DM, Sommer J, Punturi N. Emerging target discovery and drug repurposing opportunities in chordoma. Front Oncol. (2022) 12:1009193. doi: 10.3389/fonc.2022.1009193
132. Patel SS, Nota SP, Sabbatino F, Nielsen GP, Deshpande V, Wang X, et al. Defective HLA class I expression and patterns of lymphocyte infiltration in chordoma tumors. Clin Orthopaedics Related Research®. (2021) 479:1373. doi: 10.1097/CORR.0000000000001587
133. Lopez DC, Robbins YL, Kowalczyk JT, Lassoued W, Gulley JL, Miettinen MM, et al. Multi-spectral immunofluorescence evaluation of the myeloid, T cell, and natural killer cell tumor immune microenvironment in chordoma may guide immunotherapeutic strategies. Front Oncol. (2022) 12:1012058. doi: 10.3389/fonc.2022.1012058
134. Bishop AJ, Amini B, Lin H, Raza SM, Patel S, Grosshans DR, et al. Immune checkpoint inhibitors have clinical activity in patients with recurrent chordoma. J Immunotherapy. (2022) 45:374. doi: 10.1097/CJI.0000000000000431
135. Feng Y, Shen J, Gao Y, Liao Y, Cote G, Choy E, et al. Expression of programmed cell death ligand 1 (PD-L1) and prevalence of tumor-infiltrating lymphocytes (TILs) in chordoma. Oncotarget. (2015) 6:11139–49. doi: 10.18632/oncotarget.3576
136. Xu J, Shi Q, Lou J, Wang B, Wang W, Niu J, et al. Chordoma recruits and polarizes tumor-associated macrophages via secreting CCL5 to promote Malignant progression. J Immunother Cancer. (2023) 11:e006808. doi: 10.1136/jitc-2023-006808
137. Xie L, Bourne P. Developing multi-target therapeutics to fine-tune the evolutionary dynamics of the cancer ecosystem. Front Pharmacol. (2015) 6:209. doi: 10.3389/fphar.2015.00209
138. Traylor JI, Pernik MN, Plitt AR, Lim M, Garzon-Muvdi T. Immunotherapy for chordoma and chondrosarcoma: current evidence. Cancers. (2021) 13:2408. doi: 10.3390/cancers13102408
139. Zou M-X, Peng A-B, Lv G-H, Wang X-B, Li J, She X-L, et al. Expression of programmed death-1 ligand (PD-L1) in tumor-infiltrating lymphocytes is associated with favorable spinal chordoma prognosis. Am J Transl Res. (2016) 8:3274–87.
140. Zou M-X, Guo K-M, Lv G-H, Huang W, Li J, Wang X-B, et al. Clinicopathologic implications of CD8+/Foxp3+ ratio and miR-574-3p/PD-L1 axis in spinal chordoma patients. Cancer Immunol Immunother. (2018) 67:209–24. doi: 10.1007/s00262-017-2080-1
141. Zhou J, Jiang Y, Zhang H, Chen L, Luo P, Li L, et al. Clinicopathological implications of TIM3+ tumor-infiltrating lymphocytes and the miR-455-5p/Galectin-9 axis in skull base chordoma patients. Cancer Immunol Immunother. (2019) 68:1157–69. doi: 10.1007/s00262-019-02349-1
142. Kim SK, Cho SW. The evasion mechanisms of cancer immunity and drug intervention in the tumor microenvironment. Front Pharmacol. (2022) 13:868695. doi: 10.3389/fphar.2022.868695
143. Son B, Lee S, Youn H, Kim E, Kim W, Youn B. The role of tumor microenvironment in therapeutic resistance. Oncotarget. (2016) 8:3933–45. doi: 10.18632/oncotarget.13907
144. Wu P, Gao W, Su M, Nice EC, Zhang W, Lin J, et al. Adaptive mechanisms of tumor therapy resistance driven by tumor microenvironment. Front Cell Dev Biol. (2021) 9:641469. doi: 10.3389/fcell.2021.641469
145. Salemme V, Centonze G, Avalle L, Natalini D, Piccolantonio A, Arina P, et al. The role of tumor microenvironment in drug resistance: emerging technologies to unravel breast cancer heterogeneity. Front Oncol. (2023) 13:1170264. doi: 10.3389/fonc.2023.1170264
146. Long C, Li G, Zhang C, Jiang T, Li Y, Duan X, et al. B7-H3 as a target for CAR-T cell therapy in skull base chordoma. Front Oncol. (2021) 11:659662. doi: 10.3389/fonc.2021.659662
147. Yin L, Wang X-J, Chen D-X, Liu X-N, Wang X-J. Humanized mouse model: a review on preclinical applications for cancer immunotherapy. Am J Cancer Res. (2020) 10:4568–84.
148. Ai M, Curran MA. Immune checkpoint combinations from mouse to man. Cancer Immunol Immunother. (2015) 64:885–92. doi: 10.1007/s00262-014-1650-8
149. Yip H, Haupt C, Maresh G, Zhang X, Li L. Humanized mice for immune checkpoint blockade in human solid tumors. Am J Clin Exp Urol. (2019) 7:313–20.
150. Baluszek S, Kober P, Rusetska N, Wągrodzki M, Mandat T, Kunicki J, et al. DNA methylation, combined with RNA sequencing, provide novel insight into molecular classification of chordomas and their microenvironment. Acta Neuropathol Commun. (2023) 11:113. doi: 10.1186/s40478-023-01610-0
151. Duan W, Zhang B, Li X, Chen W, Jia S, Xin Z, et al. Single-cell transcriptome profiling reveals intra-tumoral heterogeneity in human chordomas. Cancer Immunol Immunother. (2022) 71(9):2185–95. doi: 10.1007/s00262-022-03152-1
152. Williamson LM, Rive CM, Di Francesco D, Titmuss E, Chun H-JE, Brown SD, et al. Clinical response to nivolumab in an INI1-deficient pediatric chordoma correlates with immunogenic recognition of brachyury. NPJ Precis Onc. (2021) 5:1–12. doi: 10.1038/s41698-021-00238-4
153. Meng T, Huang R, Jin J, Gao J, Liu F, Wei Z, et al. A comparative integrated multi-omics analysis identifies CA2 as a novel target for chordoma. Neuro Oncol. (2021) 23:1709–22. doi: 10.1093/neuonc/noab156
154. Dridi M, Krebs-Drouot L, Meyronet D, Dumollard JM, Vassal F, Jouanneau E, et al. The immune microenvironment of chordomas: an immunohistochemical analysis. Cancers (Basel). (2021) 13:3335. doi: 10.3390/cancers13133335
155. Karpathiou G, Dridi M, Krebs-Drouot L, Vassal F, Jouanneau E, Jacquesson T, et al. Autophagic markers in chordomas: immunohistochemical analysis and comparison with the immune microenvironment of chordoma tissues. Cancers (Basel). (2021) 13:2169. doi: 10.3390/cancers13092169
156. Chordoma Foundation. First humanized mouse model of chordoma enables. Available online at: https://www.chordomafoundation.org/latest-updates/first-humanized-mouse-model-of-chordoma-enables-testing-of-promising-immune-therapies/ (Accessed September 11, 2023).
157. Mathios D, Ruzevick J, Jackson CM, Xu H, Shah S, Taube JM, et al. PD-1, PD-L1, PD-L2 expression in the chordoma microenvironment. J Neurooncol. (2015) 121:251–9. doi: 10.1007/s11060-014-1637-5
158. Ishida W, McCormick K, Mahajan A, Feldstein E, Lim M, Bruce J, et al. TMOD-37. in vivo synergistic effect of checkpoint blockade and radiation therapy against chordomas in a humanized mouse model. Neuro Oncol. (2018) 20:vi276. doi: 10.1093/neuonc/noy148.1149
159. Ishida W, McCormick KL, Mahajan A, Feldstein E, Lim M, Bruce JN, et al. Abstract B165: Investigating in vivo synergistic effect of checkpoint blockade and radiation therapy against chordomas in a humanized mouse model. Cancer Immunol Res. (2019) 7:B165. doi: 10.1158/2326-6074.CRICIMTEATIAACR18-B165
160. The identification and rational targeting of immune suppression in Malignant chordoma. Northwestern Scholars. Available online at: https://www.scholars.northwestern.edu/en/projects/the-identification-and-rational-targeting-of-immune-suppression-i (Accessed September 11, 2023).
161. Gounder MM, Zhu G, Roshal L, Lis E, Daigle SR, Blakemore SJ, et al. Immunologic correlates of the abscopal effect in a SMARCB1/INI1-negative poorly differentiated chordoma after EZH2 inhibition and radiotherapy. Clin Cancer Res. (2019) 25:2064–71. doi: 10.1158/1078-0432.CCR-18-3133
162. Hoke ATK, Padget MR, Fabian KP, Nandal A, Gallia GL, Bilusic M, et al. Combinatorial natural killer cell-based immunotherapy approaches selectively target chordoma cancer stem cells. Cancer Res Commun. (2021) 1:127–39. doi: 10.1158/2767-9764.crc-21-0020
163. Fujii R, Schlom J, Hodge JW. A potential therapy for chordoma via antibody-dependent cell-mediated cytotoxicity (ADCC) employing NK or high affinity NK (haNK) cells in combination with cetuximab. J Neurosurg. (2018) 128:1419–27. doi: 10.3171/2017.1.JNS162610
164. Martinez Moreno M, Wang E, Schroeder C, Sullivan P, Gokaslan Z. Shedding light on emerging therapeutic targets for chordoma. Expert Opin Ther Targets. (2023) 27(8):705–13. doi: 10.1080/14728222.2023.2248382
Keywords: chordoma, humanized mice, immunocompromised mice, immunotherapy, tumor microenvironment
Citation: Campilan B, Schroeder C, Sagaityte E, Arditi J, Leary OP, Gokaslan ZL, Sullivan PLZ and Martinez-Moreno M (2024) Animal model considerations for chordoma research: reproducing the tumor microenvironment in vivo with humanized mice. Front. Oncol. 14:1330254. doi: 10.3389/fonc.2024.1330254
Received: 20 November 2023; Accepted: 20 February 2024;
Published: 13 March 2024.
Edited by:
Steven Fiering, Dartmouth College, United StatesReviewed by:
Nyall London, Johns Hopkins University, United StatesCopyright © 2024 Campilan, Schroeder, Sagaityte, Arditi, Leary, Gokaslan, Sullivan and Martinez-Moreno. This is an open-access article distributed under the terms of the Creative Commons Attribution License (CC BY). The use, distribution or reproduction in other forums is permitted, provided the original author(s) and the copyright owner(s) are credited and that the original publication in this journal is cited, in accordance with accepted academic practice. No use, distribution or reproduction is permitted which does not comply with these terms.
*Correspondence: Margot Martinez-Moreno, bWFyZ290X21hcnRpbmV6X21vcmVub0Bicm93bi5lZHU=
†These authors have contributed equally to this work
Disclaimer: All claims expressed in this article are solely those of the authors and do not necessarily represent those of their affiliated organizations, or those of the publisher, the editors and the reviewers. Any product that may be evaluated in this article or claim that may be made by its manufacturer is not guaranteed or endorsed by the publisher.
Research integrity at Frontiers
Learn more about the work of our research integrity team to safeguard the quality of each article we publish.