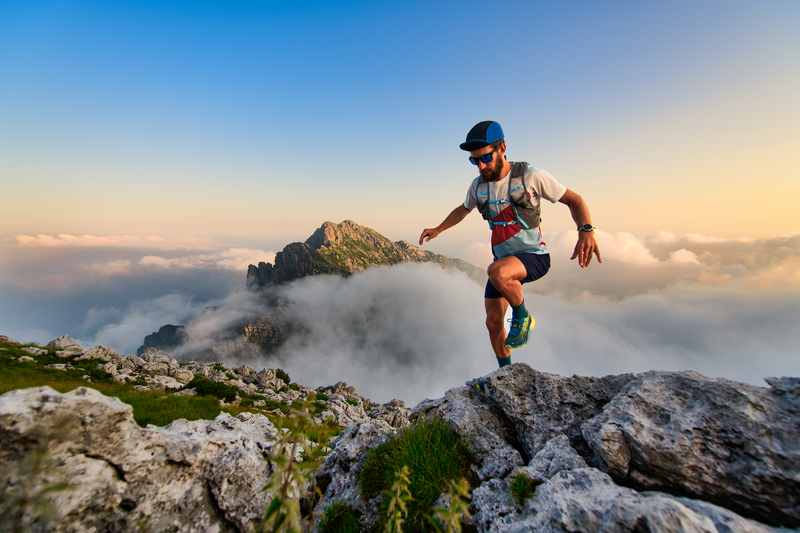
95% of researchers rate our articles as excellent or good
Learn more about the work of our research integrity team to safeguard the quality of each article we publish.
Find out more
REVIEW article
Front. Oncol. , 07 February 2024
Sec. Cancer Immunity and Immunotherapy
Volume 14 - 2024 | https://doi.org/10.3389/fonc.2024.1323070
This article is part of the Research Topic Community Series in Tumor Ablation and Immunity: Volume II View all 6 articles
Cryoablation, as a minimally invasive technology for the treatment of tumors, destroys target tumors with lethal low temperatures. It simultaneously releases a large number of tumor-specific antigens, pro-inflammatory cytokines, and nucleoproteins, known as “danger signals”, activating the body’s innate and adaptive immune responses. However, tumor cells can promote the inactivation of immune effector cells by reprogramming immune checkpoints, leading to the insufficiency of these antigens to induce an immune response capable of eradicating the tumor. Immune checkpoint blockers rejuvenate exhausted T cells by blocking immune checkpoints that induce programmed death of T cells, and are therefore considered a promising therapeutic strategy to enhance the immune effects of cryoablation. In this review, we provide a detailed explanation of the immunological mechanisms of cryoablation and articulate the theoretical basis and research progress of the treatment of cancer with cryoablation combined with immune checkpoint blockers. Preliminary data indicates that this combined treatment strategy exhibits good synergy and has been proven to be safe and effective.
In this century, cancer is set to become the leading cause of premature death worldwide (1). Despite some significant achievements in the field of cancer treatment, overall cancer-related mortality remains relatively stable (2). Over the past few decades, the five-year survival rate for lung cancer, liver cancer, and esophageal cancer has hovered around 15%, and the five-year survival rate for cancer patients with distant metastases is less than 10% (3). Hence, in the face of these challenges, it is imperative to explore new treatment techniques and optimize treatment plans.
Currently, ablation techniques, as a minimally invasive treatment approach, have been widely implemented in clinical practice. They offer a safe and effective alternative for elderly patients who are not suitable for surgery, patients with underlying health conditions, and those at a higher surgical risk. Compared to thermal ablation methods like radiofrequency ablation, cryoablation demonstrates advantages in ease of operation, reduced patient pain, clear delineation of the ablation zone, and adaptability to tumor shapes through multi-needle combination. Therefore, in certain cases, it is considered a viable treatment option (4–9). In the proposed cryoimmunotherapy mechanism, cryoablation induces coagulative necrosis in tumor tissues through ultra-low temperature physical methods (10–12), while simultaneously releasing tumor-specific antigens in situ (akin to autologous vaccine inoculation), promoting the body’s anti-tumor specific immune response (13–15). However, numerous studies show that the immune activation capability of cryoablation alone is limited. Therefore, it is important to seek immunotherapy that can assist cryoablation in generating stronger immune effects.
In 2017, the immune checkpoint blockade (ICB) pembrolizumab (anti-PD-1) was added to the first-line anti-tumor therapy, significantly improving the overall survival (OS) of patients (16, 17). ICBs enhance the body’s anti-tumor immune response by blocking immune checkpoints that cause programmed death of T cells, thus preventing T cell exhaustion (18–21). However, a drawback of immunotherapy is the low objective remission rate (22–24). In addition, certain genetic mutations can lead to primary resistance to ICBs, and some patients may develop acquired resistance or disease hyper-progression (25–31). Therefore, it is important to find combination therapies that can expand the ICB beneficiary population and enhance its immunotherapeutic effects.
Here, we elucidate the immunological mechanisms of cryoablation. Concurrently, we summarize the clinical and preclinical research on cryoablation combined with ICBs in tumors and detail the theoretical basis and potential advantages supporting this combination approach. Finally, we discuss the challenges faced by this combined approach and its future directions.
The cryoablation technique utilizes the Joule-Thomson effect by releasing compressed liquid gas into the target tumor tissue via specialized cryoablation probes. As the compressed liquid gas rapidly expands and transforms into a gaseous state, ultra-low temperatures are produced, causing the temperature of the target tissue to rapidly drop to around -140°C. Following cryoablation, the ablation zone can be divided into a central region and a peripheral region (Figure 1). In the central area, which is closer to the probe, intracellular fluid forms ice crystals, leading to physical cellular damage; the extracellular fluid freezes, resulting in osmotic cellular damage. Both types of injuries ultimately lead to coagulative necrosis of the tumor cells (32, 33). However, in the peripheral region where lethal temperatures are not reached, damaged cells that have not undergone necrosis mediate tumor cell apoptosis through the mitochondrial pathway (32).
Figure 1 lustration of tumor tissue after cryoablation. The cryoablation zone is divided into the central zone and the edge zone. In the central zone, tumor cells undergo coagulative necrosis and release antigens and damage-associated molecular patterns (DAMPs), which induce innate and adaptive immune responses against the tumor. In the sublethal temperature zone at the edge of the ablation, tumor cells undergo apoptosis, and this region is characterized by a Th2 cytokine environment, inducing immune tolerance.
In the central region, necrotic tumor cells release antigens (such as cell surface antigens, intracellular, and nuclear antigens) as well as damage-associated molecular patterns (DAMPs: endogenous molecules released from dying cells, such as high mobility group box 1 (HMGB1), DNA, calreticulin, or f-actin) (11, 15). Among these, DAMPs are believed to activate dendritic cells (DCs) through Toll-like receptors (TLRs) such as TLR4, promoting the generation of a specific immune response against tumor antigens (34, 35). Furthermore, some DAMPs, such as pro-inflammatory cytokines and nuclear proteins, can attract and activate neutrophils, macrophages, and natural killer (NK) cells, stimulating an innate immune response. Activated NK cells can directly lyse tumor cells (32, 36). Additionally, research on autoimmune diseases has shown that nuclear and organelle-derived antigens may be more effective stimulants for the host’s innate and adaptive immune systems (37). Under the influence of cytokines and chemokines in the central region of cryoablation, immature dendritic cells (IDC) reach the damaged tissue, and in the context of inflammation and abundant cytokines, take up the above tumor antigens (13). IDC enters the draining lymph nodes through the afferent lymphatic vessels, where they differentiate into mature DC, upregulating the expression of the Major Histocompatibility Complex (MHC) molecules, co-stimulatory molecules, and adhesion molecules. They then present these tumor-specific antigens through MHC class I and II molecules, activating CD8+T and CD4+T cells (13, 32). Moreover, within the activated CD4+ T lymphocytes, the helper T cell 1 (Th1) subset can release various Th1 cytokines, such as interleukin-2 (IL-2), interferon-γ (IFN-γ), and tumor necrosis factor-alpha (TNF-α). These cytokines promote the proliferation of CD8+ T cells and their differentiation into cytotoxic T lymphocytes (CTL), enhancing the anti-tumor immune response (32) (Figure 2A). These activated tumor-specific T cells possess the ability to recognize and destroy both local and distant tumor cells, thereby killing tumor cells and inhibiting the growth of untreated tumors at distant sites. This demonstrates the abscopal effect of cryoablation. However, unlike necrotic cells, apoptotic cells in the marginal area not only fail to stimulate an immune response but can also induce immune tolerance (32, 38). In the marginal region, after phagocytizing apoptotic cells, macrophages and dendritic cells do not upregulate the expression of co-stimulatory molecules. Additionally, they secrete anti-inflammatory cytokines such as transforming growth factor-β (TGF-β1) and interleukin-10 (IL-10), leading to the inability to activate T cells (35, 39) (Figure 1). Current research suggests that the ratio of immunogenic necrosis induced by cryoablation to immune tolerogenic cell apoptosis is a decisive factor leading to anti-tumor immune responses or immune tolerance. Therefore, we should continue to improve cryoablation techniques, increase the area of tumor cell necrosis, reduce the area of apoptosis, and find the best cryoablation conditions to induce anti-tumor immune responses.
Figure 2 Mechanism of action of cryoablation combined with immune checkpoint blockers. Following cryoablation, coagulative necrotic tumor tissue releases a large number of antigens, prompting APCs to uptake these antigens and increase the expression of MHC molecules and co-stimulatory ligands CD80/CD86. T lymphocyte activation requires two signals: Firstly, the TCR recognizes antigen peptides bound to MHC molecules on APCs, providing an antigen recognition signal; secondly, the co-stimulatory receptor CD28 on T cells binds with CD80/CD86 on APCs, transmitting a co-stimulatory signal. These two signals together promote the activation and proliferation of T cells. Activated tumor-specific T cells can recognize and destroy both local and distant tumor cells, realizing the immunological effect of cryoablation. (A) However, in the abscopal tumor tissue, the binding of PD-1 on some T cell surfaces to PD-L1 on tumor cells inhibits co-stimulatory signals, leading to impaired proliferation and reduced cytotoxicity of T cells, transforming them into “exhausted” T cells, and thus triggering tumor immune escape. (B) The combined use of PD-1 inhibitors can block the PD-1/PD-L1 axis, restoring the signaling pathway and thus reviving the proliferative and effector capabilities of these “exhausted” T cells to achieve the objective of killing tumor cells.
A vast body of literature indicates that cryoablation has immunological effects. Cryoablation not only directly destroys tumor cells at the local treatment site but also activates the body’s immune system, impacting metastatic tumors at distant sites that have not been treated – a phenomenon known as the abscopal effect. A study by Sabel et al. (40, 41) found that in mice with cryoablated or surgically removed breast cancer, the recurrence rate in the cryoablation group was significantly lower than in the surgical group (16% vs 86%). After cryoablation, levels of interleukin-12 (IL-12) and IFN-γ in the mice’s serum significantly increased, and T-cell toxicity in tumor-draining lymph nodes (TDLNs) was significantly enhanced. Transferring cryoablated TDLNs to untreated tumor-bearing mice resulted in fewer lung metastases and a higher proportion of tumor-specific T cells, compared to the group receiving TDLNs from surgically removed tumors. These results suggest that cryoablation can trigger a tumor-specific T cell response in TDLNs, inhibiting the growth of secondary tumors. A study by Den Brok et al. (42) found that when necrotic tumor tissue was left in situ after cryoablation, 50% of B16-OVA tumor-bearing mice could resist re-challenge experiments with B16-OVA cells. In contrast, when necrotic tumor tissue was removed immediately after cryoablation, the survival rate of the mice subjected to subsequent challenge experiments dropped to 0%. This suggests that the anti-tumor immune response induced by cryoablation heavily relies on the necrotic tumor tissue produced by the cryoablation process. It further suggests that cryoablation of solid tumors can release tumor antigens in situ, thereby creating an antigen reservoir essential for inducing in situ an anti-tumor immune response. Takahashi Y et al. (43) investigated the impact of one, two, and three cycles of cryoablation on the immune modulation of abscopal tumors by inoculating Lewis lung carcinoma and B16 melanoma cells in bilateral flanks of mice. The results showed that mice undergoing two freeze/thaw cycles on the left-sided tumor had the longest survival, the slowest growth rate of the right-sided tumor, and a higher proportion of CD4+ and CD8+ tumor-infiltrating lymphocytes(TILs). Additionally, there was a significant increase in the levels of IL-1β, IL-2, IL-6, IL-12β, IFN-γ, and TNF-α in the lavage fluid surrounding the cryoablated tumor. This suggests that two cycles of cryoablation effectively activate pro-inflammatory cytokines, enhancing the activity of immune cells in abscopal tumors, thereby producing the strongest abscopal effect. In a study conducted on a mouse model of prostate cancer (44), compared with the surgical and control groups, mice treated with cryoablation exhibited suppressed growth of distant untreated tumors, reduced rates of lung metastasis, extended survival, and an increase in the proportion and activity of CD4+ and CD8+ T cells as well as NK cells in peripheral blood. This indicates that the abscopal effect generated by cryoablation is mediated by an anti-tumor immune response. In a study on a breast cancer tumor-bearing mouse model, Rakhshanda L. Rahman et al. (45) found that compared to the baseline group, mice treated with cryoablation showed a significant increase in TILs in both the stroma and margins of distant tumors (2.8% increase in stroma, p = 0.015; 50% increase at the margin, p = 0.02), as well as a significant rise in CD8+ T cells and granzyme B (GzmB) (increases of 15.7, p = 0.02, and 4.8, p = 0.048, respectively). The cryoablation group of mice exhibited no recurrence or metastasis, whereas 40% of the mice in the surgical group showed recurrence and lung metastasis. Proportional tests further revealed a significant correlation between the increase in the percentage of TILs in distant tumors and the prevention of tumor recurrence (p = 0.02). These results suggest that cryoablation, compared to traditional surgical removal, more effectively stimulates TIL responses and prevents cancer recurrence and metastasis through the abscopal effect. In another study on a breast cancer tumor-bearing mouse model, Wenjun Fan et al. (46) observed that mice treated with cryoablation had prolonged survival and an increase in the number of CD8+T and CD4+T cells in distant tumors compared to the untreated control group. Further multi-omics analysis revealed that cryoablation activates the lysosomal pathway in tumor tissues, leading to overexpression of key proteins such as SNAP23 (Synaptosome Associated Protein 23) and STXBP2 (Syntaxin Binding Protein 2). This process not only promotes the activation of immune effector cells but also suppresses immunosuppressive cells like regulatory T cells (Treg) and M2 macrophages. The study confirms that cryoablation enhances anti-tumor immune responses and induces the abscopal effect, effectively prolonging the survival of mice. Clinical trials have also obtained similar results. Osada et al. (47) found that among patients with liver metastatic tumors who underwent cryoablation treatment, those who exhibited an “abscopal effect” showed a significantly higher Th1/Th2 ratio in their peripheral blood compared to those who did not experience this effect. This observation suggests that the enhanced systemic anti-tumor immune response following cryoablation can mediate the abscopal effect in patients. In another study on cryoablation in 22 renal cancer patients (15), the authors, through T cell receptor β (TCRβ) sequencing and TCR diversity analysis of tissue and peripheral blood samples before and after cryoablation, found an increase in anti-tumor-specific T cells in both local tumor tissues and peripheral blood of renal cancer patients who underwent cryoablation. Concurrently, the study also observed an increase in the number of tumor-infiltrating DCs. This experiment indicates that cryoablation induced both local and systemic anti-tumor immune responses in renal cancer patients. In summary, the aforementioned data provides substantive immunological evidence for the cryoablation therapy, demonstrating that cryoablation can stimulate a tumor-specific immune response and effectively promote the occurrence of the abscopal effect.
Despite these findings, some experiments have shown that cryoablation does not inhibit tumor growth (48), and some results even suggest that cryoablation may increase the rate of metastasis and mortality in Tumor transplant mouse model (such as the fibrosarcoma KMT-17 transplant rat model) (49). Current research suggests that the conditions of cryoablation are closely related to the intensity of the anti-tumor immune response, and these inconsistent experimental results may be related to factors such as the temperature of cryoablation, freezing time, freeze-thaw cycle number, and ablation area.
Furthermore, unlike the denatured antigens released by thermotherapy-based ablation (such as radiofrequency ablation, microwave ablation, high-intensity focused ultrasound, etc.), cryoablation releases natural tumor antigens that retain their full immunogenicity by destroying tumor tissue through ultra-low temperatures. This feature allows cryoablation to induce a stronger immune response than thermal ablation (50). In the study by Shao et al. (50), they utilized the B16-F10 cell line (B16), which is a mouse melanoma cell line, to assess the ability of cryoablation and thermal therapy to release proteins, antigens, and activate tumor antigen-specific CD8+T cells. The results indicated that after cryoablation treatment of B16 tumors, the amount of protein released and the known antigen, Tyrosinase-related protein 2 (TRP-2), were both significantly higher than that with thermal therapy. Moreover, unlike thermal therapy, which released a substantial amount of denatured proteins (72.9 ± 18.1%), the proteins released by cryoablation were almost all in their native form, with denatured proteins accounting for only (7.35 ± 28.2%). This study elucidated, at the cellular level, the mechanism by which cryoablation is superior to thermal therapy in promoting T cell activation and proliferation. Den Brok et al. (13) also found that in TDLNs, the proportions of antigen-bearing DCs and mature DCs in the cryoablation group were higher than in the radiofrequency ablation group. Also, when combined with ICB treatment, the cryoablation combination group was stronger than the radiofrequency ablation combination group in inducing the quantity and function of tumor-specific T cells. These results suggest that cryoablation, compared to other thermal ablation techniques, may be more suitable for combination with immunotherapy to enhance the body’s anti-tumor immune response.
In the past decade, ICB has made significant progress in the treatment of advanced malignancies. It employs monoclonal antibodies to inhibit immune checkpoint molecules, activating T lymphocytes, including tumor-specific T cells, thereby enhancing the immune system’s ability to attack tumor cells. However, due to the excessive activation of T cells, the body’s self-tolerance is lost, leading to immune-related adverse events in some patients, such as skin inflammation, gastrointestinal issues, and liver function abnormalities (18, 51).
The immune checkpoints currently targeted by immunotherapy mainly include programmed cell death protein 1 (PD-1, CD279), programmed cell death ligand 1 (PD-L1), and cytotoxic T lymphocyte-associated antigen-4 (CTLA-4).
CTLA-4 is an inhibitory receptor specifically expressed on the surface of T cells. Its expression is upregulated upon T cell activation, thereby preventing sustained T cell activation in the early stages of an immune response (52–54). Once the TCR signal is activated, CTLA-4 repositions from within the cell to the cell surface and competitively binds to the CD80/CD86 molecules on the surface of Antigen-Presenting Cells (APCs), in competition with the T cell’s CD28. It’s important to note that CTLA-4 has 20 times the affinity for CD80/CD86 than CD28 does, which results in the suppression of co-stimulatory signal transmission, thus exerting an immune inhibitory effect (55). CTLA-4 blockers can lift the restrictions CTLA-4 places on T cell signaling, allowing antitumor lymphocytes to continue their effector responses against tumor cells (56).
Ipilimumab and Tremelimumab are drugs that can block the CTLA-4 checkpoint. Both of these drugs have been proven to enhance the body’s anti-tumor immune response. In 2011, The U.S. Food and Drug Administration (FDA) has approved ipilimumab for the treatment of patients with advanced melanoma. In 2020, FDA has approved the combination of Ipilimumab and Nivolumab, along with two cycles of platinum-based dual chemotherapy, as a first-line treatment for patients with metastatic or recurrent non-small cell lung cancer (NSCLC) that do not have epidermal growth factor receptor (EGFR) or anaplastic lymphoma kinase (ALK) genomic tumor aberrations (57). Currently, research on Tremelimumab is ongoing in phase III clinical trials for urothelial carcinoma, non-small cell lung cancer, small cell lung cancer, and head and neck squamous cell carcinoma, as well as in phase I/II clinical trials for hepatocellular carcinoma (58–64).
PD-1 is also a negative co-stimulatory molecule, expressed on the surface of T lymphocytes, B cells, macrophages, and other cells, and is a member of the B7-CD28 family. PD-1 has two ligands, namely PD-L1 (also known as B7-H1 or CD274) and PD-L2 (B7-DC, CD273). In tissues, the expression of PD-L1 and PD-L2 can inhibit local immune responses, control tissue damage, and maintain immune tolerance in tissues (65). However, tumor cells use PD-L1 as a molecular “shield”. When PD-L1 binds to PD-1, the T cells’ ability to proliferate decreases and their cytotoxic ability diminishes, transforming into “exhausted” T cells, leading to immune evasion of the tumor (66–74) (as shown in Figure 2A). However, PD-1/PD-L1 blockers can block the PD-1/PD-L1 axis, thereby reactivating these exhausted T cells, restoring their proliferation and effector capabilities, and thereby killing tumor cells (74, 75) (as shown in Figure 2B). Based on this mechanism, PD-1/PD-L1 blockers have become a valuable method of enhancing the killing power of T lymphocytes in anti-tumor immunotherapy.
Currently, the FDA has approved five anti-PD-1 or anti-PD-L1 antibodies (pembrolizumab, nivolumab, atezolizumab, durvalumab, and avelumab) for the treatment of 11 types of cancer, including refractory melanoma, advanced non-small cell lung cancer, Merkel cell carcinoma, Hodgkin’s lymphoma, highly microsatellite instability (MSI-h) tumors, head and neck cancer, bladder cancer, urothelial carcinoma, gastric esophageal cancer, hepatocellular carcinoma, and renal cell carcinoma (69).
However, it is worth noting that not all types of cancer respond to ICB. In fact, only about 20-30% of patients in cancer types that have shown response to ICB can benefit from this treatment. Additionally, some patients may experience acquired resistance or disease progression (76–80). Therefore, it is crucial to search for combination therapies that can broaden the population of beneficiaries from ICB immunotherapy and enhance immune efficacy.
Due to the heterogeneity of malignant tumors and the complexity of immune regulatory mechanisms, monotherapy often has limitations, and therefore, combination therapy is becoming a major strategy for clinical cancer treatment (81). When considering rational combination therapies, the primary focus is on how to combine drugs to alleviate tumor burden and enhance anti-tumor immune responses, thus improving patient prognosis (81). Numerous studies have indicated that cryoablation not only reduces tumor burden in the local treatment area through physical means but also activates the immune system to recognize and attack distant tumor cells by releasing tumor antigens, thereby inducing the abscopal effect (11, 13–15). The addition of ICBs further enhances this systemic immune response by blocking inhibitory signals, thus strengthening the ability of T cells to attack tumor cells. Furthermore, it is recognized that the quantity of pre-existing T cells in the tumor microenvironment is intimately correlated with the therapeutic efficacy of ICB (82–84). Therefore, combining ICB with cryoablation could significantly enhance the efficacy of each treatment modality when used alone (85).
It is well-known that the activation of naïve T lymphocytes requires dual signals: The first signal involves the recognition of antigenic peptides bound to the MHC molecules on the surface of APCs through the TCR, thereby transmitting an antigen recognition signal. The second signal comes from the binding of the co-stimulatory receptor CD28 on the T cell surface to the co-stimulatory ligands CD80/CD86 on the APC surface, thereby transmitting a co-stimulatory signal. With the combined stimulation of the first and second signals, T cell activation and proliferation are induced (38, 86) (Figure 2A). Cryoablation can release of tumor antigens, inducing APCs to uptake antigens and upregulate the expression of MHC molecules and CD80/CD86, thereby promoting T lymphocyte activation (13, 32, 83). However, in tumor tissues, PD-1 and PD-L1 are often overexpressed. The PD-1/PD-L1 axis can block the transmission of the second signal, also known as the co-stimulatory signal, resulting in T lymphocytes becoming unresponsive (87) (Figure 2A). Similarly, CTLA-4 binding to CD80/CD86 on APCs also prevents co-stimulatory signal transmission, exerting an immune inhibitory effect (55, 88). Tumors exploit these immune checkpoints as escape mechanisms, blocking further activation and proliferation of T cells. As a result, tumor antigens released by cryoablation are insufficient to induce a significant anti-tumor immune response (38, 89). However, PD-1/PD-L1 blockers can block the binding of PD-1 to PD-L1, while CTLA-4 blockers can disrupt the binding of CTLA-4 to CD80/CD86. This releases the “braking” mechanism of the co-stimulatory signal pathway, restores signal transmission, and consequently revives the activation and proliferation of T cells (56, 74, 75, 90). (Figure 2B). These tumor-specific T cells, synergistically activated by cryoablation and ICB treatment, possess the capability to recognize and destroy both local residual tumors and distant macroscopic and microscopic metastases. The aforementioned mechanism provides a theoretical foundation for the combined application of cryoablation and immunotherapy.
Here, we summarize the research progress on the combination of cryoablation and ICB therapy in treating specific types of tumors. These studies include preclinical mouse model research and preliminary results from clinical trials (Tables 1, 2). Our analysis indicates that this combined therapy exhibits a notable synergistic effect in enhancing anti-tumor immune responses. Furthermore, a series of related clinical trials are currently underway (Table 3), and the progression of these trials will provide further evidence for assessing the effectiveness of this combination therapy in clinical applications.
In this section, we will delve into the current basic research findings for several types of cancers, including prostate cancer, melanoma, renal cell carcinoma, and breast cancer. These studies offer preliminary insights into the potential of the combined therapy in cancer treatment.
In their research on prostate cancer TRAMP C2 bilateral tumor-bearing mice, Waitz R et al. (91) found that the combination of cryoablation with CTLA-4 inhibitors was more effective in inhibiting secondary tumor growth compared to monotherapy, and significantly increased the number of CD4+T and CD8+T cells in the tumor, as well as the ratio of effector T cells to Treg. These results suggest that the combined therapy can enhance anti-tumor immune responses and resist tumor metastasis. Further supporting these findings, Benzon B et al. (92) confirmed the advantages of this combined treatment in suppressing distant prostate cancer tumor growth and reducing mortality rates. Their research underscored the importance of T cells in the combined treatment, noting that the advantages of the combination therapy would be lost if T cells were depleted prior to treatment.
In their study of B16-OVA tumor-bearing mice, den Brok MH et al. (13) discovered that CTLA-4 inhibitors could enhance the weak anti-tumor immune response produced by cryoablation alone. The combined therapy not only protected the mice from secondary tumor attacks but also increased the number of tumor-specific T cells in the body and enhanced the secretion of IFN-γ.
Research on renal cell carcinoma mouse models (93) also supports these findings, showing that combined therapy (cryoablation and anti-PD-1 treatment) significantly inhibited the growth of distant tumors in mice. Immunological analysis revealed that in the tumors of mice receiving combined therapy, there was a significant increase in the infiltration of CD8+T lymphocytes and levels of IFN-γ and GzmB mRNA, with a significant decrease in IL-10 mRNA levels. This suggests that cryoablation combined with anti-PD-1 treatment is more effective in enhancing the body’s anti-tumor immune response compared to using either therapy alone.
In a recent study, Jin Y et al. (94), found in a breast cancer mouse model that cryoablation caused transient growth inhibition and an anti-tumor immune response in distant tumors, accompanied by an increase in PD-1/PD-L1 expression levels. When cryoablation was combined with an anti-PD-1 antibody, compared to monotherapy, there was a significant extension in the survival of mice, marked inhibition of distant tumor growth, and a significant increase in TILs. Additionally, the expression levels of anti-tumor immune cytokines such as TNF-α, IL-12α, T-bet, and GzmB mRNA were also elevated. These findings reveal that cryoablation combined with a PD-1 antibody can improve the immunosuppressive state of tumors and enhance the immune response induced by cryoablation, synergistically producing a more potent abscopal effect.
In summary, cryoablation combined with ICB therapy has shown significant anti-tumor potential in several types of cancers, including prostate cancer, melanoma, renal cell carcinoma, and breast cancer. Current research primarily focuses on describing changes in immune phenotypes, yet there remains a gap in deeply understanding the underlying mechanisms. This highlights the direction for future basic research.
In this section, we will summarize key clinical trials to further evaluate the efficacy and potential value of cryoablation combined with ICB in patient treatment. In the clinical setting, this combined therapeutic approach has already begun to demonstrate its therapeutic potential. The following part will provide a comprehensive analysis of the results from several pivotal clinical studies:
In a preliminary clinical study (95), 19 female patients with breast cancer underwent preoperative tumor cryoablation, monotherapy with Ipilimumab, or a combination of both treatments, followed by breast surgery. The results showed that compared to the groups receiving cryoablation alone or Ipilimumab monotherapy, the combination therapy group had sustained elevation of peripheral blood levels of IL-2, IL-12, and IFN-γ. Activation and proliferation of CD4+ T and CD8+ T cells in peripheral blood and within the tumor also significantly increased. Among them, only one patient who underwent the combined treatment experienced a Grade 3 maculopapular rash, which is speculated to be related to the administration of the antiseptic chlorhexidine and/or cefamandole. This study suggests that the combination of cryoablation and Ipilimumab as a neoadjuvant therapy is safe, well-tolerated, and can synergistically induce local and systemic anti-tumor immune responses.
In another preliminary study on metastatic renal cell carcinoma (96), 18 patients with clear cell carcinoma and 11 patients with non-clear cell carcinoma underwent treatment with anti-CTLA-4 (Tremelimumab) monotherapy (n=14) or a combination of cryoablation and anti-CTLA-4 (n=15). The results showed a significant increase in tumor-infiltrating T lymphocytes in patients with clear cell carcinoma in the combination therapy group compared to anti-CTLA-4 monotherapy, while no similar phenomenon was observed in patients with non-clear cell carcinoma. These findings suggest that the combination of anti-CTLA-4 and cryoablation is feasible and can modulate the immune microenvironment in patients with metastatic clear cell renal carcinoma.
In a preliminary study (97) involving 16 metastatic melanoma patients who received a combination treatment of cryoablation and ICB (ipilimumab n=8 or pembrolizumab n=4) (97), the results showed a 6-month progression-free survival rate of 57%, a local disease control rate (DCR) of 83%, a distant DCR of 60%, and an overall DCR of 75%. This study demonstrates that the combination of cryoablation and ICB is safe, well-tolerated, and may be an effective strategy to enhance anti-tumor immune responses.
In another prospective cohort study (98) involving 15 patients with hepatic metastases from melanoma, it was shown that after a single cycle of cryoablation combined with a PD-1 blocker (pembrolizumab), there was a significant increase in NK cells in the patients’ peripheral blood and a decrease in Tregs. Furthermore, among these 15 patients, no grade 3-4 adverse events or major complications were observed. Of them, one case (7.3%) achieved complete remission, and three cases (20%) achieved partial remission. Numerous studies have reported that an increase in NK cells (100, 101) and a decrease in Tregs (102, 103) are associated with favorable immune responses in melanoma immunotherapy. These results indicate that the combination treatment is safe and effective, and can enhance anti-tumor immune responses in patients with melanoma liver metastases.
In a retrospective analysis of 64 patients with advanced NSCLC (99), patients who received cryoablation in combination with anti-PD-1(nivolumab) therapy (n=32) showed significant improvement in immune function and short-term efficacy (P < 0.05). Additionally, this group of patients had significantly lower levels of circulating tumor cells and tumor markers CYFRA21-1 and NSE compared to patients who received cryoablation alone (P < 0.05, n=32). Additionally, all adverse reactions were manageable. This study suggests that the combination of cryoablation and anti-PD-1 therapy has good tolerability, safety, and superior clinical efficacy compared to cryoablation alone in improving outcomes for patients with advanced NSCLC.
In a case report (104), a patient with metastatic renal cell carcinoma underwent CT-guided percutaneous cryoablation combined with local administration of anti-PD-1 (nivolumab). A follow-up PET scan one month after the procedure showed reduced uptake in two smaller metastatic bone lesions, with the smallest lesion completely eliminated. The largest bone metastasis showed slight shrinkage and increased uptake. The patient reported a significant reduction in hip pain and regained the ability to walk independently without assistance. This case report suggests that cryoablation combined with local administration of PD-1 blockers can enhance systemic tumor-specific immune responses.
In summary, these study results collectively support the potential of cryoablation combined with ICB therapy in the treatment of various cancers. This combined modality has not only demonstrated good tolerability and safety but also shown significant efficacy in enhancing anti-tumor immune responses. These findings provide important scientific evidence for the further clinical application of cryoablation and ICB therapy. However, more clinical research and long-term follow-up are needed to thoroughly understand the mechanisms of this combined treatment strategy, and to assess its long-term efficacy and applicability.
Firstly, ICB has been approved by the FDA for cancer treatment, and cryoablation has also been widely accepted in cancer therapy (105–115). Therefore, this combination treatment approach holds practical clinical value.
Secondly, cryoablation may enhance the sensitivity of tumor cells to ICB. The expression level of PD-L1 is often considered as an indicator for predicting the tumor’s response to ICB (116). Studies have shown that cryoablation may increase the expression levels of PD-L1 in tumor tissues and PD-1 on T cell surfaces, thereby enhancing the sensitivity of tumor cells to ICB. For example, Ock et al. found an upregulation of PD-L1 and PD-1 expression in head and neck squamous cell carcinoma (HNSCC) patients within one week after cryoablation treatment. Zhu C et al. (93) demonstrated in a renal cell carcinoma mouse model study that cryoablation can increase the expression of PD-L1 in tumor cells and PD-1 in CD8+ T cells.
Thirdly, tumor neoantigens released by cryoablation may help alleviate the problem of acquired resistance to ICBs (117, 118). During the use of ICB for tumor treatment, cancer cells may lose the most immunogenic mutations through intense immune selection pressure via cancer immune editing processes (119–121), or reduce mutations or expressions of genes associated with antigen presentation pathways, thereby reducing T cell recognition. This may result in primary or acquired resistance of tumors to PD-1 blockers (122, 123). Recent research has found that tumor tissues of NSCLC patients with acquired resistance to immune checkpoint blockade may have 7-18 presumed mutation-associated neoantigens missing (124, 125). Therefore, increasing the exposure of neoantigens may be a way to alleviate acquired resistance to ICB. Previous studies have shown that in situ tumor ablation techniques, such as radiofrequency and cryoablation, can lead to extensive tumor cell lysis, and the fragmented tumor cells may release more neoantigens, increasing the exposure of tumor neoantigens and reinducing endogenous immune responses (126, 127). The combination of ICB with localized cryoablation therapy may provide a valuable approach to enhance immune cell recognition of tumor neoantigens (117, 118). Therefore, in the face of the increasingly common occurrence of acquired resistance to immune checkpoint blockade, cryoablation may become a new combination therapy for ICB-resistant patients by increasing the release of neoantigens. In a case report of a 75-year-old female patient with advanced NSCLC (117), cryoablation successfully prevented the recurrence of lymph node metastasis in the aortic region, in a patient who had acquired resistance to ICB. This case suggests that cryoablation can provide a successful, safe, and feasible strategy to enhance the anti-tumor effect of ICB and may help overcome acquired resistance to ICB.
In summary, the combination of cryoablation and ICB therapy has two important considerations. On one hand, ICB can enhance the weak anti-tumor immune response induced by cryoablation. When ICB blocks the immune checkpoints that cause T cell exhaustion, the circulating cryoablation-induced antigens recognized by the immune system can bypass the tumor’s immune checkpoint escape mechanism, resulting in a potent anti-tumor T cell response. On the other hand, cryoablation may enhance the expression of PD-L1 on tumor tissues and PD-1 on CD8+ T cells, thereby increasing the sensitivity of tumor cells to ICB. Additionally, the release of a large number of tumor neoantigens by cryoablation may help alleviate the issue of acquired resistance to ICB.
The combination of cryoablation and ICB therapy is an effective strategy that outperforms monotherapy. This combination therapy has shown significant effects in enhancing the patient’s anti-tumor immune response and eliminating tumors, providing strong theoretical support for the clinical integration of cryoablation and ICB, bringing new hope for patients with advanced metastatic tumors.
Currently, research on the combined treatment of cancer using cryoablation technology and ICB remains relatively limited. We need to develop various solid tumor models and employ techniques such as RNA sequencing, single-cell sequencing, flow cytometry, and multiplex immunohistochemistry. These methods will allow us to thoroughly analyze the intensity of the anti-tumor immune response elicited by the combined treatment, as well as changes in the tumor microenvironment. This will help us to more comprehensively and precisely assess the response of different types of tumors to the combination therapy and clearly define its potential mechanisms of action.
Furthermore, to validate the tolerability, safety, and efficacy of this combination therapy, extensive clinical studies are also necessary. These studies should cover key issues such as the optimal parameter settings for cryoablation (such as duration, number of freeze-thaw cycles, volume of the ablated tumor), the ideal dosage and administration method for ICB, as well as the sequence of cryoablation and ICB treatment, and the appropriate duration of combination therapy for different types of tumors. Given that the efficacy of the combination treatment may be influenced by factors such as tumor tissue type, individual patient differences, and variations between organs, even the same treatment protocol may yield different immunological effects in different patients. Therefore, in clinical application, it is crucial to optimize treatment parameters personalized to the specific situation of each tumor patient, in order to develop the most effective combination therapy plan.
As we explore the possibilities of future combination therapies, we might consider the depletion of Tregs as a potential approach, which could help enhance the efficacy of cryo-immunomodulation multimodal therapies. Tregs play a key role in suppressing the effector functions of CD8+ T cells and have long been considered a factor influencing the effectiveness of ICB therapies, as well as a potential target in treatment strategies (128). Interventions targeting Tregs can alter the immunosuppressive state within the tumor microenvironment, potentially eliciting a more effective anti-tumor immune response. This theoretically may enhance the efficacy of combined cryoablation and ICB therapy. For instance, cyclophosphamide has been proven to effectively reduce the number of Tregs in the tumor microenvironment and increase the presence of tumor-infiltrating T cells producing IFN-γ, thereby strengthening the anti-tumor immune response (129–131). Consequently, strategies to deplete Tregs might serve as an effective supplement to cryoablation combined with ICB therapy in the future. Future research could explore how to optimize the immunomodulatory effects of cryoablation and ICB combination therapy through pharmacological intervention in Treg cells, potentially offering a new perspective in cancer treatment. Although these forward-looking views provide new ideas for future combination therapies, their clinical application and long-term effects still need further exploration and validation in future scientific research.
QL: Conceptualization, Writing – original draft. CZ: Writing – review & editing. XC: Writing – review & editing. ZH: Funding acquisition, Resources, Supervision, Writing – review & editing.
The author(s) declare financial support was received for the research, authorship, and/or publication of this article. This work was supported by the General Project of Military Logistics Scientific Research 674 Program (NO. CLB20J019, NO. B20201A020033 and NO.LB20211A010007) and the Natural Science Foundation of Beijing Municipal (Grant No. 7232169).
The authors declare that the research was conducted in the absence of any commercial or financial relationships that could be construed as a potential conflict of interest.
The handling editor YX declared a shared parent affiliation with the authors at the time of review.
All claims expressed in this article are solely those of the authors and do not necessarily represent those of their affiliated organizations, or those of the publisher, the editors and the reviewers. Any product that may be evaluated in this article, or claim that may be made by its manufacturer, is not guaranteed or endorsed by the publisher.
1. Soerjomataram I, Bray F. Planning for tomorrow: global cancer incidence and the role of prevention 2020-2070. Nat Rev Clin Oncol (2021) 18(10):663–72. doi: 10.1038/s41571-021-00514-z
2. Shen YW, Zhou YD, Chen HZ, Luan X, Zhang WD. Targeting CTGF in cancer: an emerging therapeutic opportunity. Trends Cancer (2021) 7(6):511–24. doi: 10.1016/j.trecan.2020.12.001
3. Ganesh K, Massague J. Targeting metastatic cancer. Nat Med (2021) 27(1):34–44. doi: 10.1038/s41591-020-01195-4
4. Littrup PJ, Jallad B, Chandiwala-Mody P, D'Agostini M, Adam BA, Bouwman D. Cryotherapy for breast cancer: a feasibility study without excision. J Vasc Interv Radiol (2009) 20(10):1329–41. doi: 10.1016/j.jvir.2009.06.029
5. Vikingstad EM, de Ridder GG, Glisson RR, Cardona DM, DiPalma D, Eward WC, et al. Comparison of acute histologic and biomechanical effects of radiofrequency ablation and cryoablation on periarticular structures in a swine model. J Vasc Interv Radiol (2015) 26(8):1221–8 e1. doi: 10.1016/j.jvir.2015.04.013
6. Soule E, Bagherpour A, Matteo J. Freezing fort knox: mesenteric carcinoid cryoablation. Gastrointest Tumors (2017) 4(1-2):53–60. doi: 10.1159/000479794
7. Derstine L, Soule E, Shabandi N, Arutyunova Z, Lall C, Scuderi C, et al. Rare treatment for a rare tumor: cryoablation of a granular cell tumor. Gastrointest Tumors (2020) 7(1-2):41–9. doi: 10.1159/000504134
8. Hegarty NJ, Gill IS, Desai MM, Remer EM, O'Malley CM, Kaouk JH. Probe-ablative nephron-sparing surgery: cryoablation versus radiofrequency ablation. Urology (2006) 68(1 Suppl):7–13. doi: 10.1016/j.urology.2005.12.049
9. Palussiere J, Cazayus M, Cousin S, Cabart M, Chomy F, Catena V, et al. Is there a role for percutaneous ablation for early stage lung cancer? What is the evidence? Curr Oncol Rep (2021) 23(7):81. doi: 10.1007/s11912-021-01072-4
10. Kwak K, Yu B, Lewandowski RJ, Kim DH. Recent progress in cryoablation cancer therapy and nanoparticles mediated cryoablation. Theranostics (2022) 12(5):2175–204. doi: 10.7150/thno.67530
11. Baust JG, Snyder KK, Santucci KL, Robilotto AT, Van Buskirk RG, Baust JM. Cryoablation: physical and molecular basis with putative immunological consequences. Int J Hyperthermia (2019) 36(sup1):10–6. doi: 10.1080/02656736.2019.1647355
12. Erinjeri JP, Clark TW. Cryoablation: mechanism of action and devices. J Vasc Interv Radiol (2010) 21(8 Suppl):S187–91. doi: 10.1016/j.jvir.2009.12.403
13. den Brok MH, Sutmuller RP, Nierkens S, Bennink EJ, Frielink C, Toonen LW, et al. Efficient loading of dendritic cells following cryo and radiofrequency ablation in combination with immune modulation induces anti-tumour immunity. Br J Cancer (2006) 95(7):896–905. doi: 10.1038/sj.bjc.6603341
14. Veenstra JJ, Gibson HM, Freytag S, Littrup PJ, Wei WZ. In situ immunization via non-surgical ablation to prevent local and distant tumor recurrence. Oncoimmunology (2015) 4(3):e989762. doi: 10.4161/2162402X.2014.989762
15. Kato T, Iwasaki T, Uemura M, Nagahara A, Higashihara H, Osuga K, et al. Characterization of the cryoablation-induced immune response in kidney cancer patients. Oncoimmunology (2017) 6(7):e1326441. doi: 10.1080/2162402X.2017.1326441
16. Gadgeel S, Rodriguez-Abreu D, Speranza G, Esteban E, Felip E, Domine M, et al. Updated analysis from KEYNOTE-189: pembrolizumab or placebo plus pemetrexed and platinum for previously untreated metastatic nonsquamous non-small-cell lung cancer. J Clin Oncol (2020) 38(14):1505–17. doi: 10.1200/JCO.19.03136
17. Paz-Ares L, Luft A, Vicente D, Tafreshi A, Gumus M, Mazieres J, et al. Pembrolizumab plus chemotherapy for squamous non-small-cell lung cancer. N Engl J Med (2018) 379(21):2040–51. doi: 10.1056/NEJMoa1810865
18. Morad G, Helmink BA, Sharma P, Wargo JA. Hallmarks of response, resistance, and toxicity to immune checkpoint blockade. Cell (2021) 184(21):5309–37. doi: 10.1016/j.cell.2021.09.020
19. Kirchhammer N, Trefny MP, Auf der Maur P, Laubli H, Zippelius A. Combination cancer immunotherapies: Emerging treatment strategies adapted to the tumor microenvironment. Sci Transl Med (2022) 14(670):eabo3605. doi: 10.1126/scitranslmed.abo3605
20. Petitprez F, Meylan M, de Reynies A, Sautes-Fridman C, Fridman WH. The tumor microenvironment in the response to immune checkpoint blockade therapies. Front Immunol (2020) 11:784. doi: 10.3389/fimmu.2020.00784
21. Kaiser M, Semeraro MD, Herrmann M, Absenger G, Gerger A, Renner W. Immune aging and immunotherapy in cancer. Int J Mol Sci (2021) 22(13):7016. doi: 10.3390/ijms22137016
22. Ruan H, Bu L, Hu Q, Cheng H, Lu W, Gu Z. Strategies of combination drug delivery for immune checkpoint blockades. Adv Healthc Mater (2019) 8(4):e1801099. doi: 10.1002/adhm.201801099
23. Sesma A, Pardo J, Cruellas M, Galvez EM, Gascon M, Isla D, et al. From tumor mutational burden to blood T cell receptor: looking for the best predictive biomarker in lung cancer treated with immunotherapy. Cancers (Basel) (2020) 12(10):2974. doi: 10.3390/cancers12102974
24. Tang S, Qin C, Hu H, Liu T, He Y, Guo H, et al. Immune checkpoint inhibitors in non-small cell lung cancer: progress, challenges, and prospects. Cells (2022) 11(3):320. doi: 10.3390/cells11030320
25. Yi M, Zheng X, Niu M, Zhu S, Ge H, Wu K. Combination strategies with PD-1/PD-L1 blockade: current advances and future directions. Mol Cancer (2022) 21(1):28. doi: 10.1186/s12943-021-01489-2
26. Lopez-Soto A, Gonzalez S, Folgueras AR. IFN signaling and ICB resistance: time is on tumor's side. Trends Cancer (2017) 3(3):161–3. doi: 10.1016/j.trecan.2017.01.004
27. Yu R, Zhu B, Chen D. Type I interferon-mediated tumor immunity and its role in immunotherapy. Cell Mol Life Sci (2022) 79(3):191. doi: 10.1007/s00018-022-04219-z
28. Gomez S, Tabernacki T, Kobyra J, Roberts P, Chiappinelli KB. Combining epigenetic and immune therapy to overcome cancer resistance. Semin Cancer Biol (2020) 65:99–113. doi: 10.1016/j.semcancer.2019.12.019
29. Shi H, Lan J, Yang J. Mechanisms of resistance to checkpoint blockade therapy. Adv Exp Med Biol (2020) 1248:83–117. doi: 10.1007/978-981-15-3266-5_5
30. Aspeslagh S, Chabanon RM, Champiat S, Postel-Vinay S. Understanding genetic determinants of resistance to immune checkpoint blockers. Semin Cancer Biol (2020) 65:123–39. doi: 10.1016/j.semcancer.2019.12.020
31. Xiong J, Wang QQ. Mechanisms and strategies to overcome immunotherapy resistance in hepatobiliary Malignancies. Hepatobiliary Pancreat Dis Int (2022) 21(5):430–9. doi: 10.1016/j.hbpd.2022.07.006
32. Sabel MS. Cryo-immunology: a review of the literature and proposed mechanisms for stimulatory versus suppressive immune responses. Cryobiology (2009) 58(1):1–11. doi: 10.1016/j.cryobiol.2008.10.126
33. Yakkala C, Chiang CL, Kandalaft L, Denys A, Duran R. Cryoablation and immunotherapy: an enthralling synergy to confront the tumors. Front Immunol (2019) 10:2283. doi: 10.3389/fimmu.2019.02283
34. Germain RN, Stefanova I. The dynamics of T cell receptor signaling: complex orchestration and the key roles of tempo and cooperation. Annu Rev Immunol (1999) 17:467–522. doi: 10.1146/annurev.immunol.17.1.467
35. Mahoney JA, Rosen A. Apoptosis and autoimmunity. Curr Opin Immunol (2005) 17(6):583–8. doi: 10.1016/j.coi.2005.09.018
36. Katzman D, Wu S, Sterman DH. Immunological aspects of cryoablation of non-small cell lung cancer: A comprehensive review. J Thorac Oncol (2018) 13(5):624–35. doi: 10.1016/j.jtho.2018.01.017
37. Blum JS, Wearsch PA, Cresswell P. Pathways of antigen processing. Annu Rev Immunol (2013) 31:443–73. doi: 10.1146/annurev-immunol-032712-095910
38. Chen J, Qian W, Mu F, Niu L, Du D, Xu K. The future of cryoablation: An abscopal effect. Cryobiology (2020) 97:1–4. doi: 10.1016/j.cryobiol.2020.02.010
39. Gallucci S, Lolkema M, Matzinger P. Natural adjuvants: endogenous activators of dendritic cells. Nat Med (1999) 5(11):1249–55. doi: 10.1038/15200
40. Sabel MS, Nehs MA, Su G, Lowler KP, Ferrara JL, Chang AE. Immunologic response to cryoablation of breast cancer. Breast Cancer Res Treat (2005) 90(1):97–104. doi: 10.1007/s10549-004-3289-1
41. Sabel MS, Arora A, Su G, Chang AE. Adoptive immunotherapy of breast cancer with lymph node cells primed by cryoablation of the primary tumor. Cryobiology (2006) 53(3):360–6. doi: 10.1016/j.cryobiol.2006.07.004
42. den Brok MH, Sutmuller RP, Nierkens S, Bennink EJ, Toonen LW, Figdor CG, et al. Synergy between in situ cryoablation and TLR9 stimulation results in a highly effective in vivo dendritic cell vaccine. Cancer Res (2006) 66(14):7285–92. doi: 10.1158/0008-5472.CAN-06-0206
43. Takahashi Y, Izumi Y, Matsutani N, Dejima H, Nakayama T, Okamura R, et al. Optimized magnitude of cryosurgery facilitating anti-tumor immunoreaction in a mouse model of Lewis lung cancer. Cancer Immunol Immunother (2016) 65(8):973–82. doi: 10.1007/s00262-016-1858-x
44. Yang X, Guo Y, Guo Z, Si T, Xing W, Yu W, et al. Cryoablation inhibition of distant untreated tumors (abscopal effect) is immune mediated. Oncotarget (2019) 10(41):4180–91. doi: 10.18632/oncotarget.24105
45. Khan SY, Melkus MW, Rasha F, Castro M, Chu V, Brandi L, et al. Tumor-infiltrating lymphocytes (TILs) as a biomarker of abscopal effect of cryoablation in breast cancer: A pilot study. Ann Surg Oncol (2022) 29(5):2914–25. doi: 10.1245/s10434-021-11157-w
46. Wu Y, Cao F, Zhou D, Chen S, Qi H, Huang T, et al. Cryoablation reshapes the immune microenvironment in the distal tumor and enhances the anti-tumor immunity. Front Immunol (2022) 13:930461. doi: 10.3389/fimmu.2022.930461
47. Osada S, Imai H, Tomita H, Tokuyama Y, Okumura N, Matsuhashi N, et al. Serum cytokine levels in response to hepatic cryoablation. J Surg Oncol (2007) 95(6):491–8. doi: 10.1002/jso.20712
48. Hoffmann NE, Coad JE, Huot CS, Swanlund DJ, Bischof JC. Investigation of the mechanism and the effect of cryoimmunology in the Copenhagen rat. Cryobiology (2001) 42(1):59–68. doi: 10.1006/cryo.2001.2305
49. Yamashita T, Hayakawa K, Hosokawa M, Kodama T, Inoue N, Tomita K, et al. Enhanced tumor metastases in rats following cryosurgery of primary tumor. Gan (1982) 73(2):222–8.
50. Shao Q, O'Flanagan S, Lam T, Roy P, Pelaez F, Burbach BJ, et al. Engineering T cell response to cancer antigens by choice of focal therapeutic conditions. Int J Hyperthermia (2019) 36(1):130–8. doi: 10.1080/02656736.2018.1539253
51. Dong Y, Wong JSL, Sugimura R, Lam KO, Li B, Kwok GGW, et al. Recent advances and future prospects in immune checkpoint (ICI)-based combination therapy for advanced HCC. Cancers (Basel) (2021) 13(8):1949. doi: 10.3390/cancers13081949
52. Valk E, Rudd CE, Schneider H. CTLA-4 trafficking and surface expression. Trends Immunol (2008) 29(6):272–9. doi: 10.1016/j.it.2008.02.011
53. Walunas TL, Lenschow DJ, Bakker CY, Linsley PS, Freeman GJ, Green JM, et al. CTLA-4 can function as a negative regulator of T cell activation. Immunity (1994) 1(5):405–13. doi: 10.1016/1074-7613(94)90071-X
54. Krummel MF, Allison JP. CD28 and CTLA-4 have opposing effects on the response of T cells to stimulation. J Exp Med (1995) 182(2):459–65. doi: 10.1084/jem.182.2.459
55. Valk E, Leung R, Kang H, Kaneko K, Rudd CE, Schneider H. T cell receptor-interacting molecule acts as a chaperone to modulate surface expression of the CTLA-4 coreceptor. Immunity (2006) 25(5):807–21. doi: 10.1016/j.immuni.2006.08.024
56. Kubli SP, Berger T, Araujo DV, Siu LL, Mak TW. Beyond immune checkpoint blockade: emerging immunological strategies. Nat Rev Drug Discov (2021) 20(12):899–919. doi: 10.1038/s41573-021-00155-y
57. Vellanki PJ, Mulkey F, Jaigirdar AA, Rodriguez L, Wang Y, Xu Y, et al. FDA approval summary: nivolumab with ipilimumab and chemotherapy for metastatic non-small cell lung cancer, A collaborative project orbis review. Clin Cancer Res (2021) 27(13):3522–7. doi: 10.1158/1078-0432.CCR-20-4338
58. Kelley RK, Sangro B, Harris W, Ikeda M, Okusaka T, Kang YK, et al. Safety, efficacy, and pharmacodynamics of tremelimumab plus durvalumab for patients with unresectable hepatocellular carcinoma: randomized expansion of a phase I/II study. J Clin Oncol (2021) 39(27):2991–3001. doi: 10.1200/JCO.20.03555
59. Goldman JW, Dvorkin M, Chen Y, Reinmuth N, Hotta K, Trukhin D, et al. Durvalumab, with or without tremelimumab, plus platinum-etoposide versus platinum-etoposide alone in first-line treatment of extensive-stage small-cell lung cancer (CASPIAN): updated results from a randomised, controlled, open-label, phase 3 trial. Lancet Oncol (2021) 22(1):51–65. doi: 10.1016/S1470-2045(20)30539-8
60. Kappauf H, Gallmeier WM, Wunsch PH, Mittelmeier HO, Birkmann J, Buschel G, et al. Complete spontaneous remission in a patient with metastatic non-small-cell lung cancer. Case report, review of the literature, and discussion of possible biological pathways involved. Ann Oncol (1997) 8(10):1031–9. doi: 10.1023/A:1008209618128
61. Rizvi NA, Cho BC, Reinmuth N, Lee KH, Luft A, Ahn MJ, et al. Durvalumab with or without tremelimumab vs standard chemotherapy in first-line treatment of metastatic non-small cell lung cancer: the MYSTIC phase 3 randomized clinical trial. JAMA Oncol (2020) 6(5):661–74. doi: 10.1001/jamaoncol.2020.0237
62. Schoenfeld JD, Giobbie-Hurder A, Ranasinghe S, Kao KZ, Lako A, Tsuji J, et al. Durvalumab plus tremelimumab alone or in combination with low-dose or hypofractionated radiotherapy in metastatic non-small-cell lung cancer refractory to previous PD(L)-1 therapy: an open-label, multicentre, randomised, phase 2 trial. Lancet Oncol (2022) 23(2):279–91. doi: 10.1016/S1470-2045(21)00658-6
63. Powles T, van der Heijden MS, Castellano D, Galsky MD, Loriot Y, Petrylak DP, et al. Durvalumab alone and durvalumab plus tremelimumab versus chemotherapy in previously untreated patients with unresectable, locally advanced or metastatic urothelial carcinoma (DANUBE): a randomised, open-label, multicentre, phase 3 trial. Lancet Oncol (2020) 21(12):1574–88. doi: 10.1016/S1470-2045(20)30541-6
64. Ferris RL, Haddad R, Even C, Tahara M, Dvorkin M, Ciuleanu TE, et al. Durvalumab with or without tremelimumab in patients with recurrent or metastatic head and neck squamous cell carcinoma: EAGLE, a randomized, open-label phase III study. Ann Oncol (2020) 31(7):942–50. doi: 10.1016/j.annonc.2020.04.001
65. Schildberg FA, Klein SR, Freeman GJ, Sharpe AH. Coinhibitory pathways in the B7-CD28 ligand-receptor family. Immunity (2016) 44(5):955–72. doi: 10.1016/j.immuni.2016.05.002
66. Wu X, Gu Z, Chen Y, Chen B, Chen W, Weng L, et al. Application of PD-1 blockade in cancer immunotherapy. Comput Struct Biotechnol J (2019) 17:661–74. doi: 10.1016/j.csbj.2019.03.006
67. Wherry EJ, Kurachi M. Molecular and cellular insights into T cell exhaustion. Nat Rev Immunol (2015) 15(8):486–99. doi: 10.1038/nri3862
68. Berger KN, Pu JJ. PD-1 pathway and its clinical application: A 20year journey after discovery of the complete human PD-1 gene. Gene (2018) 638:20–5. doi: 10.1016/j.gene.2017.09.050
69. Ribas A, Wolchok JD. Cancer immunotherapy using checkpoint blockade. Science (2018) 359(6382):1350–5. doi: 10.1126/science.aar4060
70. Khan M, Arooj S, Wang H. Soluble B7-CD28 family inhibitory immune checkpoint proteins and anti-cancer immunotherapy. Front Immunol (2021) 12:651634. doi: 10.3389/fimmu.2021.651634
71. Dolina JS, Van Braeckel-Budimir N, Thomas GD, Salek-Ardakani S. CD8(+) T cell exhaustion in cancer. Front Immunol (2021) 12:715234. doi: 10.3389/fimmu.2021.715234
72. Budimir N, Thomas GD, Dolina JS, Salek-Ardakani S. Reversing T-cell exhaustion in cancer: lessons learned from PD-1/PD-L1 immune checkpoint blockade. Cancer Immunol Res (2022) 10(2):146–53. doi: 10.1158/2326-6066.CIR-21-0515
73. Blank CU, Haining WN, Held W, Hogan PG, Kallies A, Lugli E, et al. Defining 'T cell exhaustion'. Nat Rev Immunol (2019) 19(11):665–74. doi: 10.1038/s41577-019-0221-9
74. Hashimoto M, Kamphorst AO, Im SJ, Kissick HT, Pillai RN, Ramalingam SS, et al. CD8 T cell exhaustion in chronic infection and cancer: opportunities for interventions. Annu Rev Med (2018) 69:301–18. doi: 10.1146/annurev-med-012017-043208
75. Farhood B, Najafi M, Mortezaee K. CD8(+) cytotoxic T lymphocytes in cancer immunotherapy: A review. J Cell Physiol (2019) 234(6):8509–21. doi: 10.1002/jcp.27782
76. Wang X, Wang F, Zhong M, Yarden Y, Fu L. The biomarkers of hyperprogressive disease in PD-1/PD-L1 blockage therapy. Mol Cancer (2020) 19(1):81. doi: 10.1186/s12943-020-01200-x
77. Tokaz MC, Baik CS, Houghton AM, Tseng D. New immuno-oncology targets and resistance mechanisms. Curr Treat Options Oncol (2022) 23(9):1201–18. doi: 10.1007/s11864-022-01005-8
78. Wang Z, Wang Y, Gao P, Ding J. Immune checkpoint inhibitor resistance in hepatocellular carcinoma. Cancer Lett (2023) 555:216038. doi: 10.1016/j.canlet.2022.216038
79. Bagchi S, Yuan R, Engleman EG. Immune checkpoint inhibitors for the treatment of cancer: clinical impact and mechanisms of response and resistance. Annu Rev Pathol (2021) 16:223–49. doi: 10.1146/annurev-pathol-042020-042741
80. Schoenfeld AJ, Hellmann MD. Acquired resistance to immune checkpoint inhibitors. Cancer Cell (2020) 37(4):443–55. doi: 10.1016/j.ccell.2020.03.017
81. Sharpe AH, Pauken KE. The diverse functions of the PD1 inhibitory pathway. Nat Rev Immunol (2018) 18(3):153–67. doi: 10.1038/nri.2017.108
82. Park YJ, Kuen DS, Chung Y. Future prospects of immune checkpoint blockade in cancer: from response prediction to overcoming resistance. Exp Mol Med (2018) 50(8):1–13. doi: 10.1038/s12276-018-0130-1
83. Tumeh PC, Harview CL, Yearley JH, Shintaku IP, Taylor EJ, Robert L, et al. PD-1 blockade induces responses by inhibiting adaptive immune resistance. Nature (2014) 515(7528):568–71. doi: 10.1038/nature13954
84. Chen PL, Roh W, Reuben A, Cooper ZA, Spencer CN, Prieto PA, et al. Analysis of immune signatures in longitudinal tumor samples yields insight into biomarkers of response and mechanisms of resistance to immune checkpoint blockade. Cancer Discov (2016) 6(8):827–37. doi: 10.1158/2159-8290.CD-15-1545
85. Liu YT, Sun ZJ. Turning cold tumors into hot tumors by improving T-cell infiltration. Theranostics (2021) 11(11):5365–86. doi: 10.7150/thno.58390
86. Abdo J, Cornell DL, Mittal SK, Agrawal DK. Immunotherapy plus cryotherapy: potential augmented abscopal effect for advanced cancers. Front Oncol (2018) 8:85. doi: 10.3389/fonc.2018.00085
87. Cha JH, Chan LC, Li CW, Hsu JL, Hung MC. Mechanisms controlling PD-L1 expression in cancer. Mol Cell (2019) 76(3):359–70. doi: 10.1016/j.molcel.2019.09.030
88. Linsley PS, Brady W, Urnes M, Grosmaire LS, Damle NK, Ledbetter JA. CTLA-4 is a second receptor for the B cell activation antigen B7. J Exp Med (1991) 174(3):561–9. doi: 10.1084/jem.174.3.561
89. Aarts BM, Klompenhouwer EG, Rice SL, Imani F, Baetens T, Bex A, et al. Cryoablation and immunotherapy: an overview of evidence on its synergy. Insights Imaging (2019) 10(1):53. doi: 10.1186/s13244-019-0727-5
90. Xiong W, Zhao Y, Du H, Guo X. Current status of immune checkpoint inhibitor immunotherapy for lung cancer. Front Oncol (2021) 11:704336. doi: 10.3389/fonc.2021.704336
91. Waitz R, Solomon SB, Petre EN, Trumble AE, Fasso M, Norton L, et al. Potent induction of tumor immunity by combining tumor cryoablation with anti-CTLA-4 therapy. Cancer Res (2012) 72(2):430–9. doi: 10.1158/0008-5472.CAN-11-1782
92. Benzon B, Glavaris SA, Simons BW, Hughes RM, Ghabili K, Mullane P, et al. Combining immune check-point blockade and cryoablation in an immunocompetent hormone sensitive murine model of prostate cancer. Prostate Cancer Prostatic Dis (2018) 21(1):126–36. doi: 10.1038/s41391-018-0035-z
93. Zhu C, Lin S, Liang J, Zhu Y. PD-1 blockade enhances the anti-tumor immune response induced by cryoablation in a murine model of renal cell carcinoma. Cryobiology (2019) 87:86–90. doi: 10.1016/j.cryobiol.2019.01.015
94. Yu ZP, Sun XW, He YP, Gu J, Jin Y. PD-1 monoclonal antibodies enhance the cryoablation-induced antitumor immune response: a breast cancer murine model research. Int J Hyperthermia (2023) 40(1):2164625. doi: 10.1080/02656736.2022.2164625
95. McArthur HL, Diab A, Page DB, Yuan J, Solomon SB, Sacchini V, et al. A pilot study of preoperative single-dose ipilimumab and/or cryoablation in women with early-stage breast cancer with comprehensive immune profiling. Clin Cancer Res (2016) 22(23):5729–37. doi: 10.1158/1078-0432.CCR-16-0190
96. Campbell MT, Matin SF, Tam AL, Sheth RA, Ahrar K, Tidwell RS, et al. Pilot study of Tremelimumab with and without cryoablation in patients with metastatic renal cell carcinoma. Nat Commun (2021) 12(1):6375. doi: 10.1038/s41467-021-26415-4
97. Kim DW, Haymaker C, McQuail N, Sirmans E, Spencer C, Glitza I, et al. Pilot study of intratumoral (IT) cryoablation (cryo) in combination with systemic checkpoint blockade in patients with metastatic melanoma (MM). J Immunother Cancer (2015) 3(S2):P137. doi: 10.1186/2051-1426-3-S2-P137
98. Shen L, Qi H, Chen S, Cao F, Xie L, Wu Y, et al. Cryoablation combined with transarterial infusion of pembrolizumab (CATAP) for liver metastases of melanoma: an ambispective, proof-of-concept cohort study. Cancer Immunol Immunother (2020) 69(9):1713–24. doi: 10.1007/s00262-020-02566-z
99. Feng J, Guiyu D, Xiongwen W. The clinical efficacy of argon-helium knife cryoablation combined with nivolumab in the treatment of advanced non-small cell lung cancer. Cryobiology (2021) 102:92–6. doi: 10.1016/j.cryobiol.2021.07.007
100. Lee H, Quek C, Silva I, Tasker A, Batten M, Rizos H, et al. Integrated molecular and immunophenotypic analysis of NK cells in anti-PD-1 treated metastatic melanoma patients. Oncoimmunology (2019) 8(2):e1537581. doi: 10.1080/2162402X.2018.1537581
101. Barry KC, Hsu J, Broz ML, Cueto FJ, Binnewies M, Combes AJ, et al. A natural killer-dendritic cell axis defines checkpoint therapy-responsive tumor microenvironments. Nat Med (2018) 24(8):1178–91. doi: 10.1038/s41591-018-0085-8
102. Gambichler T, Schroter U, Hoxtermann S, Susok L, Stockfleth E, Becker JC. Decline of programmed death-1-positive circulating T regulatory cells predicts more favourable clinical outcome of patients with melanoma under immune checkpoint blockade. Br J Dermatol (2020) 182(5):1214–20. doi: 10.1111/bjd.18379
103. Gambichler T, Schroter U, Hoxtermann S, Susok L, Stockfleth E, Becker JC. A brief communication on circulating PD-1-positive T-regulatory lymphocytes in melanoma patients undergoing adjuvant immunotherapy with nivolumab. J Immunother (2019) 42(7):265–8. doi: 10.1097/CJI.0000000000000277
104. Soule E, Bandyk M, Matteo J. Percutaneous ablative cryoimmunotherapy for micrometastaic abscopal effect: No complications. Cryobiology (2018) 82:22–6. doi: 10.1016/j.cryobiol.2018.04.013
105. Vora BMK, Munk PL, Somasundaram N, Ouellette HA, Mallinson PI, Sheikh A, et al. Cryotherapy in extra-abdominal desmoid tumors: A systematic review and meta-analysis. PloS One (2021) 16(12):e0261657. doi: 10.1371/journal.pone.0261657
106. Moore W, Talati R, Bhattacharji P, Bilfinger T. Five-year survival after cryoablation of stage I non-small cell lung cancer in medically inoperable patients. J Vasc Interv Radiol (2015) 26(3):312–9. doi: 10.1016/j.jvir.2014.12.006
107. Sanda MG, Cadeddu JA, Kirkby E, Chen RC, Crispino T, Fontanarosa J, et al. Clinically localized prostate cancer: AUA/ASTRO/SUO guideline. Part I: risk stratification, shared decision making, and care options. J Urol (2018) 199(3):683–90. doi: 10.1016/j.juro.2017.11.095
108. Mercader C, Musquera M, Franco A, Alcaraz A, Ribal MJ. Primary cryotherapy for localized prostate cancer treatment. Aging Male (2020) 23(5):1460–6. doi: 10.1080/13685538.2020.1796960
109. Luerken L, Haimerl M, Doppler M, Uller W, Beyer LP, Stroszczynski C, et al. Update on percutaneous local ablative procedures for the treatment of hepatocellular carcinoma. Rofo (2022) 194(10):1075–86. doi: 10.1055/a-1768-0954
110. Olagunju A, Forsman T, Ward RC. An update on the use of cryoablation and immunotherapy for breast cancer. Front Immunol (2022) 13:1026475. doi: 10.3389/fimmu.2022.1026475
111. Scandiffio R, Bozzi E, Ezeldin M, Capanna R, Ceccoli M, Colangeli S, et al. Image-guided cryotherapy for musculoskeletal tumors. Curr Med Imaging (2021) 17(2):166–78. doi: 10.2174/1573405616666200825162712
112. Subiela JD, Mercade A, Balana J, Rubio Galisteo JM, Gallioli A, Breda A, et al. Ultrasound-guided cryotherapy of small renal masses: Systematic review. Arch Esp Urol (2019) 72(8):750–8.
113. Sundet A, McConnell J, Walker K, Lindeque B. Intraoperative cryotherapy in the treatment of metastatic renal cell carcinoma of the bone. Orthopedics (2021) 44(5):e645–e52. doi: 10.3928/01477447-20210817-04
114. Steinfort DP, Herth FJF. Bronchoscopic treatments for early-stage peripheral lung cancer: Are we ready for prime time? Respirology (2020) 25(9):944–52. doi: 10.1111/resp.13903
115. Holmes D, Iyengar G. Breast cancer cryoablation in the multidisciplinary setting: practical guidelines for patients and physicians. Life (Basel) (2023) 13(8):1756. doi: 10.3390/life13081756
116. Chan TA, Yarchoan M, Jaffee E, Swanton C, Quezada SA, Stenzinger A, et al. Development of tumor mutation burden as an immunotherapy biomarker: utility for the oncology clinic. Ann Oncol (2019) 30(1):44–56. doi: 10.1093/annonc/mdy495
117. Adam LC, Raja J, Ludwig JM, Adeniran A, Gettinger SN, Kim HS. Cryotherapy for nodal metastasis in NSCLC with acquired resistance to immunotherapy. J Immunother Cancer (2018) 6(1):147. doi: 10.1186/s40425-018-0468-x
118. Regen-Tuero HC, Ward RC, Sikov WM, Littrup PJ. Cryoablation and immunotherapy for breast cancer: overview and rationale for combined therapy. Radiol Imaging Cancer (2021) 3(2):e200134. doi: 10.1148/rycan.2021200134
119. Luksza M, Riaz N, Makarov V, Balachandran VP, Hellmann MD, Solovyov A, et al. A neoantigen fitness model predicts tumour response to checkpoint blockade immunotherapy. Nature (2017) 551(7681):517–20. doi: 10.1038/nature24473
120. Schreiber RD, Old LJ, Smyth MJ. Cancer immunoediting: integrating immunity's roles in cancer suppression and promotion. Science (2011) 331(6024):1565–70. doi: 10.1126/science.1203486
121. Rooney MS, Shukla SA, Wu CJ, Getz G, Hacohen N. Molecular and genetic properties of tumors associated with local immune cytolytic activity. Cell (2015) 160(1-2):48–61. doi: 10.1016/j.cell.2014.12.033
122. Sade-Feldman M, Jiao YJ, Chen JH, Rooney MS, Barzily-Rokni M, Eliane JP, et al. Resistance to checkpoint blockade therapy through inactivation of antigen presentation. Nat Commun (2017) 8(1):1136. doi: 10.1038/s41467-017-01062-w
123. Gettinger S, Choi J, Hastings K, Truini A, Datar I, Sowell R, et al. Impaired HLA class I antigen processing and presentation as a mechanism of acquired resistance to immune checkpoint inhibitors in lung cancer. Cancer Discov (2017) 7(12):1420–35. doi: 10.1158/2159-8290.CD-17-0593
124. Jiang T, Shi T, Zhang H, Hu J, Song Y, Wei J, et al. Tumor neoantigens: from basic research to clinical applications. J Hematol Oncol (2019) 12(1):93. doi: 10.1186/s13045-019-0787-5
125. Anagnostou V, Smith KN, Forde PM, Niknafs N, Bhattacharya R, White J, et al. Evolution of neoantigen landscape during immune checkpoint blockade in non-small cell lung cancer. Cancer Discov (2017) 7(3):264–76. doi: 10.1158/2159-8290.CD-16-0828
126. den Brok MH, Sutmuller RP, van der Voort R, Bennink EJ, Figdor CG, Ruers TJ, et al. In situ tumor ablation creates an antigen source for the generation of antitumor immunity. Cancer Res (2004) 64(11):4024–9. doi: 10.1158/0008-5472.CAN-03-3949
127. Raaijmakers TK, van den Bijgaart RJE, den Brok MH, Wassink M, de Graaf A, Wagenaars JA, et al. Tumor ablation plus co-administration of CpG and saponin adjuvants affects IL-1 production and multifunctional T cell numbers in tumor draining lymph nodes. J Immunother Cancer (2020) 8(1):e000649. doi: 10.1136/jitc-2020-000649
128. Principe DR, Chiec L, Mohindra NA, Munshi HG. Regulatory T-cells as an emerging barrier to immune checkpoint inhibition in lung cancer. Front Oncol (2021) 11:684098. doi: 10.3389/fonc.2021.684098
129. Hughes E, Scurr M, Campbell E, Jones E, Godkin A, Gallimore A. T-cell modulation by cyclophosphamide for tumour therapy. Immunology (2018) 154(1):62–8. doi: 10.1111/imm.12913
130. Scurr M, Pembroke T, Bloom A, Roberts D, Thomson A, Smart K, et al. Low-dose cyclophosphamide induces antitumor T-cell responses, which associate with survival in metastatic colorectal cancer. Clin Cancer Res (2017) 23(22):6771–80. doi: 10.1158/1078-0432.CCR-17-0895
Keywords: cryoablation, immune checkpoint blockade, immunotherapy, combined therapy, cancer immunology
Citation: Liu Q, Zhang C, Chen X and Han Z (2024) Modern cancer therapy: cryoablation meets immune checkpoint blockade. Front. Oncol. 14:1323070. doi: 10.3389/fonc.2024.1323070
Received: 17 October 2023; Accepted: 16 January 2024;
Published: 07 February 2024.
Edited by:
Yueyong Xiao, Chinese People’s Liberation Army General Hospital, ChinaReviewed by:
Michael W. Melkus, Texas Tech University Health Sciences Center, United StatesCopyright © 2024 Liu, Zhang, Chen and Han. This is an open-access article distributed under the terms of the Creative Commons Attribution License (CC BY). The use, distribution or reproduction in other forums is permitted, provided the original author(s) and the copyright owner(s) are credited and that the original publication in this journal is cited, in accordance with accepted academic practice. No use, distribution or reproduction is permitted which does not comply with these terms.
*Correspondence: Zhihai Han, aGFuemhpaGFpQDMwMWhvc3BpdGFsLmNvbS5jbg==
†These authors have contributed equally to this work
Disclaimer: All claims expressed in this article are solely those of the authors and do not necessarily represent those of their affiliated organizations, or those of the publisher, the editors and the reviewers. Any product that may be evaluated in this article or claim that may be made by its manufacturer is not guaranteed or endorsed by the publisher.
Research integrity at Frontiers
Learn more about the work of our research integrity team to safeguard the quality of each article we publish.