- 1Department of Histology and Embryology, College of Basic Medical Sciences, Jilin University, Changchun, Jilin, China
- 2Department of Cell Biology, College of Basic Medical Sciences, Jilin University, Changchun, Jilin, China
- 3First Hospital of Jilin University, Changchun, Jilin, China
Triple-negative breast cancer (TNBC) is the most refractory subtype of breast cancer, and effective treatments are urgently needed owing to its poor prognosis. Surgery, radiotherapy, and chemotherapy, alone or in combination, are the leading choices for TNBC therapy. Although promising approaches and procedures have emerged, several challenges, such as off-target effects, drug resistance, and severe side effects, remain to be addressed. Recently, transient receptor potential channel mucolipin 1 (TRPML1) has attracted the attention of researchers because its expression has been implicated in numerous diseases, including cancer. TRPML1 regulates biological events and signaling pathways, including autophagic flux, exocytosis, ionic homeostasis, and lysosomal biogenesis, all contributing to tumorigenesis and cancer progression. TRPML1 also functions as a building block for cancer cell growth, mitogenic signaling, priming tissues for metastasis, and activation of transcriptional programs, processes involved in several malignant tumors. This review provides an overview of breast cancer epidemiology and diagnostic techniques and then discusses the existing therapeutics. Additionally, we elaborate on the development of, and associated challenges to, TNBC diagnostics and treatment and the feasibility of TRPML1 as a therapeutic target for TNBC.
1 Introduction
Breast cancer is a global health challenge as it is highly aggressive and commonly affects women. The latest data from 2020 show that breast cancer has surpassed lung cancer to become the most common cancer among female patients, with new cases comprising 11.7% of all cancer cases and a mortality rate of 6.9%, ranking fifth among all cancer-related deaths (1) (Figure 1). Although considerable progress has been made in the diagnosis and treatment of breast cancer in recent years, the issues of drug resistance, recurrence, low overall survival (OS) rate, and poor prognosis remain unresolved (2, 3).
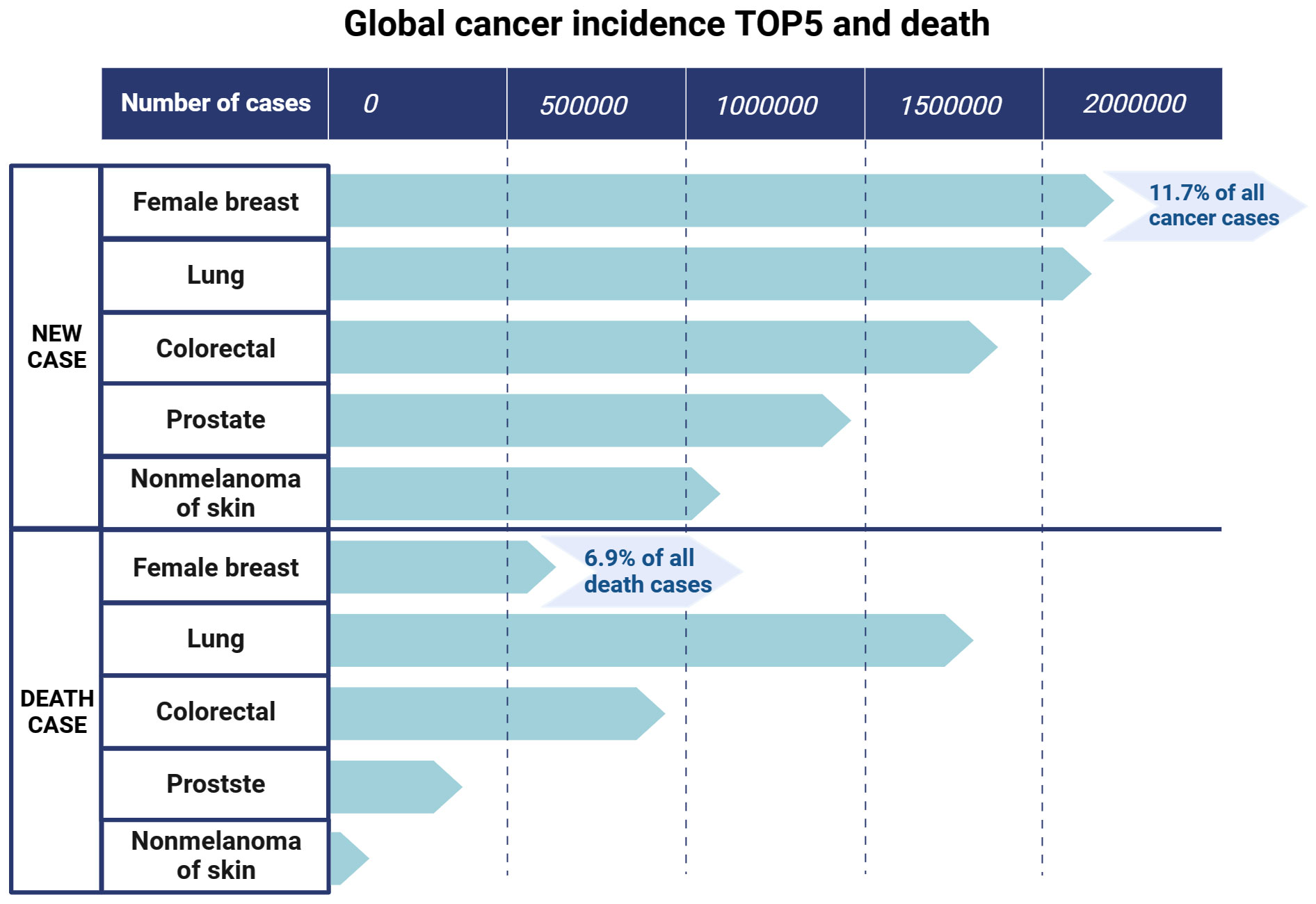
Figure 1 The top five new cancer cases and deaths in the world. Data (2020) are from the Global Cancer Observatory of the World Health Organization. Created with BioRender.com.
Clinically, breast cancer is a heterogeneous disease with multiple subtypes (4, 5). Based on the expression of hormone receptors (HRs), namely estrogen receptor (ER), progesterone receptor (PR), and human epidermal growth factor receptor 2 (HER2). The molecular classification of breast cancer can be divided into four types: luminal A (HR+ [ER+ and/or PR+], HER2-), luminal B (HR+ [ER+ and/or PR+], HER2+/-), HER2-positive (HR- [ER-, PR-], HER2+), and triple-negative breast cancer (TNBC; HR- [ER-, PR-], HER2-). The molecular and biological characteristics of each subtype are presented in Figure 2. Among the four subtypes, TNBC is the most aggressive, with a high rate of metastasis and poor prognosis. Approximately 46% of patients with TNBC develop distant metastasis and the median survival time after metastasis is substantially shortened to only 13.3 months (6–8), with the highest death rate within five years of diagnosis (9). TNBC is also heterogeneous. Lehmann et al. (2011, 2014) divided TNBC into six subtypes, according to gene expression profiles, which include basal-like 1 and basal-like 2, immunomodulatory (IM), mesenchymal (M), mesenchymal stem-like (MSL), and luminal androgen receptor (LAR) (10, 11), and found an association between each subtype and multiple gene mutations (12). Since TNBC is the most metastatic cancer and no effective targeted therapy currently exists, early diagnosis and treatment are essential to control its development (13).
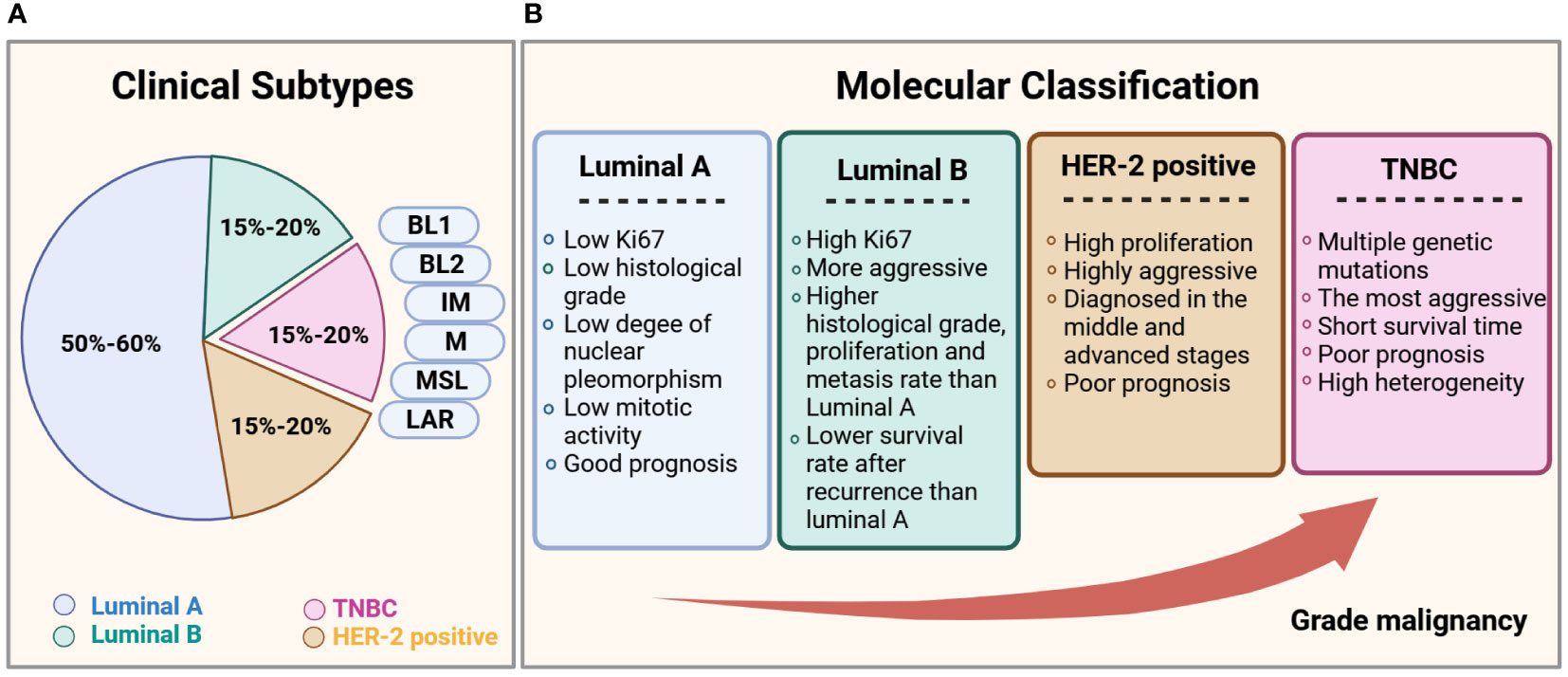
Figure 2 Subtypes of breast cancer. (A) Statistical chart of the proportion of each clinical subtype of breast cancer. (B) Comparison of the clinical subtypes of breast cancer, based primarily on histological features and immunohistochemical expression of ER, PR, HER2, and the proliferation marker Ki67.
Mammography is the most important screening method for breast cancer as it facilitates early diagnosis, substantially reducing the overall patient mortality (14). In high-risk and susceptible groups, mammography is usually combined with ultrasonography or magnetic resonance imaging (MRI) for diagnosis (15), with MRI-assisted mammography being the more sensitive approach (16). For detecting distant metastases, the sensitivity and specificity of positron emission tomography (PET)/CT scans are considerably higher than those of conventional imaging examinations and have a higher diagnostic value for prognostic risk stratification (17), with 18-fluoro-deoxyglucose PET/CT explicitly used to detect axillary lymph node metastases (97%) (18).
2 Current therapies for breast cancer
Advances in imaging, treatment, and posttreatment care over the past decade have provided a range of treatment options for breast cancer. For non-metastatic breast cancer, surgical intervention is the mainstay treatment. Notably, no considerable difference in OS has been observed between breast-conserving surgery plus radiotherapy and total mastectomy (19, 20). The mainstay treatment for hormone-responsive (HR+, HER2-) breast cancer is endocrine therapy, which blocks the action of estrogen at the receptor level or inhibits estrogen production using selective ER modulation agents, aromatase inhibitors, luteinizing hormone-releasing agonists, and cyclin-dependent kinases 4 and 6 inhibitor for resistant patients (21, 22). HER2 inhibitors are used primarily to treat the HER2 subtype of breast cancer, along with additional small-molecule tyrosine kinase inhibitors, such as afatinib and neratinib (23). These drugs can cross the blood-brain barrier and reduce the rate of brain metastasis in patients with HER2+ breast cancer (24). Interestingly, combined antibody–drug conjugates (ADC) in high-risk patients achieves a pathological complete response (pCR), suggesting that a proportion of HER2-positive tumors can be eradicated without chemotherapy (25).
As TNBC is not sensitive to estrogen-sensitive endocrine therapy or HER2-targeting drugs, the chemotherapy-based systemic therapy is the primary systemic treatment option; however, its efficacy is limited, and the associated prognosis is poor (26). Neoadjuvant systemic therapy (NAST) is becoming the standard treatment for TNBC in the early stages of the disease (12, 19, 27). However, no standard chemotherapeutic regimen for relapsed/refractory patients with TNBC is currently available. Response rates of TNBC increase with chemotherapy drug combinations compared to the use of single agents; however, this increase was at the expense of increased cytotoxicity (28, 29). Currently, prolonging the survival of patients with TNBC is not universally or effectively possible.
3 Molecularly targeted therapies for TNBC
Considering chemotherapy’s nonspecificity and high toxicity, developing targeted therapeutic approaches and regimen combinations is crucial for improving TNBC prognosis. With the continued elucidation and characterization of the biological nature of TNBC, molecularly targeted strategies have been identified, including DNA repair pathway inhibitors, immunotherapy, anti-angiogenic agents, ADCs, and phosphatidylinositol 3-kinase (PI3K)/protein kinase B (AKT)/mammalian target of rapamycin (mTOR) inhibitors. We listed clinical medicines and their advantages and disadvantages in Table 1.
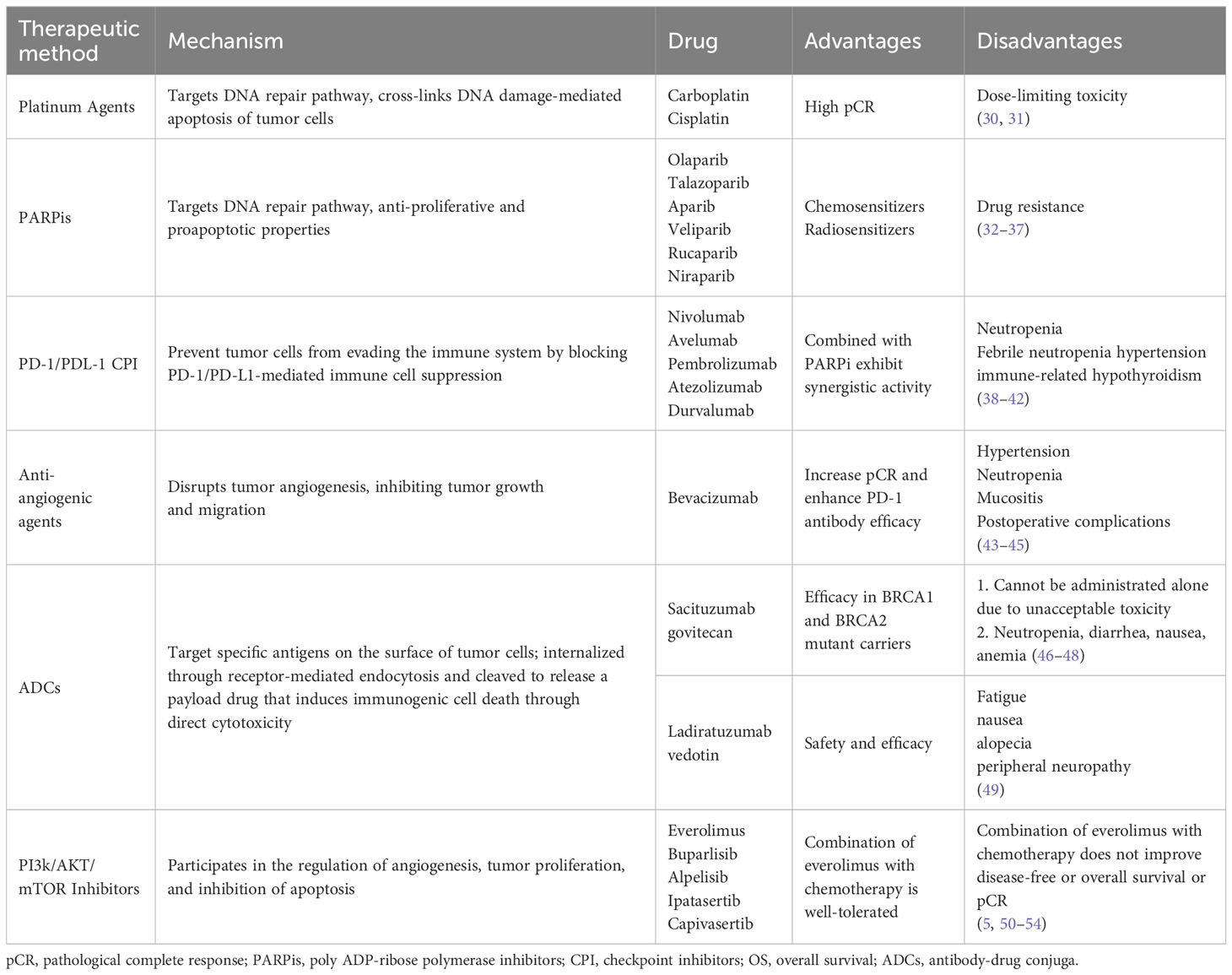
Table 1 Mechanism and characteristics of molecular targeted therapy for triple-negative breast cancer.
Generally, TNBC associated with BRCA1 or BRCA2 mutant is currently the only type sensitive to targeted therapy, approximately 5% of patients with breast cancer carry germline mutations in BRCA1 or BRCA2 (55, 56). However, the efficacy and safety of DNA repair pathway inhibitors differ concerning their potency and cytotoxicity regarding their ability to prevent poly ADP-ribose polymerase from directing DNA repair (33).
Compared to other types of breast cancer, TNBC has a higher mutation load, stronger immunogenicity, and more immune cell infiltration, implying that immunotherapy may be a viable treatment option for TNBC (57). Indeed, adding the programmed cell death protein 1 (PD-1)/programmed death-ligand (PD-L1) pathway blockers prevent tumor cells from evading the immune system by blocking PD-1/PD-L1-mediated immune cell suppression (38). Adding a PD1/PD-L1 blockade to NACT considerably increases the pCR rate in patients with TNBC with a good safety response, especially in patients with a high risk of recurrence (39). Therefore, immunotherapy may be an ideal direction for future TNBC treatment, particularly in combination with chemotherapy.
ADCs target specific antigens on the surface of tumor cells. They are internalized and cleaved to release a payload drug that drives antitumor activity and induces immunogenic cell death through direct cytotoxicity. The main ADCs currently used in TNBC are sacituzumab govitecan and ladiratuzumab vedotin. Other ADC drugs are also under development, including an anti-intracellular adhesion molecule 1 ADC; however, drug resistance continues to occur due to antigen-associated drug resistance, internalization failure, and impaired lysosomal function (30, 58).
Mutations in components of the PI3K/AKT/mTOR signaling pathway can result in its persistent activation, which is often observed in different malignancies (59, 60). The mutation frequency of PIK3CA (a PI3K subunit) in TNBC is approximately 10%, and activation of related pathways is more common in LAR and M subtypes (61). In clinical trials, drugs such as the pan-PI3K inhibitors, AKT inhibitors, and mTOR inhibitors have achieved satisfactory results (5, 50). However, in patients with early TNBC, adding the PI3K/AKT pathway inhibitor ipatasertib to paclitaxel-based neoadjuvant regimens does not improve pCR, suggesting that more precise guidance is needed for the use of this type of drug (51).
Although these targeted therapies prolong the development time of TNBC to varying degrees or temporarily prolong the survival period, there is still no effective solution to prevent disease recurrence and drug resistance. Currently, methods that can effectively prolong the survival of patients with TNBC, improve prognosis, and control its progression with mild adverse reactions are lacking. Furthering our understanding of the microscopic nature of TNBC, identifying novel therapeutic targets, and precisely defining a patient population suitable for existing targeted drug therapies are necessary to develop effective TNBC treatments.
4 TRPML1 as a potential therapeutic target for TNBC
A potential target for treating cancer has emerged in recent years—transient receptor potential channel mucolipin 1 (TRPML1). As a lysosomal channel protein, TRPML1 can permeate various cations, including calcium ions, maintain cell homeostasis, regulate lysosomal adaptation, exocytosis, autophagy, and other functions, and mediate cancer cell growth (62–64). The mechanisms through which TRPML1 contributes to disease pathogenesis are summarized in Figure 3. In particular, TRPML1 plays a critical role in TNBC (65). Xu et al. reported that TRPML1 is specifically up-regulated in TNBC and controls cancer cell survival by promoting mTORC1 activity. Meanwhile, decreased cell proliferation was observed in TRPML1-knockdown (KD) MDA-MB-231 cells. Moreover, TRPML1-KD MDA-MB-231 cells exhibit attenuated extracellular ATP content and invasive capacity, indicating that TRPML1 facilitates TNBC metastasis by enhancing extracellular ATP release. Similar results were reported by Shekoufeh et al. (66), who showed that TRPML1 promotes breast cancer cell line survival by supporting mitochondrial function and cellular metabolism. Specifically, TRPML1 KD inhibits TNBC mitochondrial respiration, glycolysis, and ATP production, leading to reduced proliferation, promotion of cell cycle arrest, and apoptosis with enhanced global and mitochondrial ROS in MDA-MB231 and HS578PT cells. Furthermore, TRPML1 downregulation enhances the sensitivity of TNBC cells to chemotherapy drugs. These studies strongly suggest that TRPML1 is a therapeutic target in TNBC. Additionally, TRPML1 is reportedly involved in various tumor-associated processes.
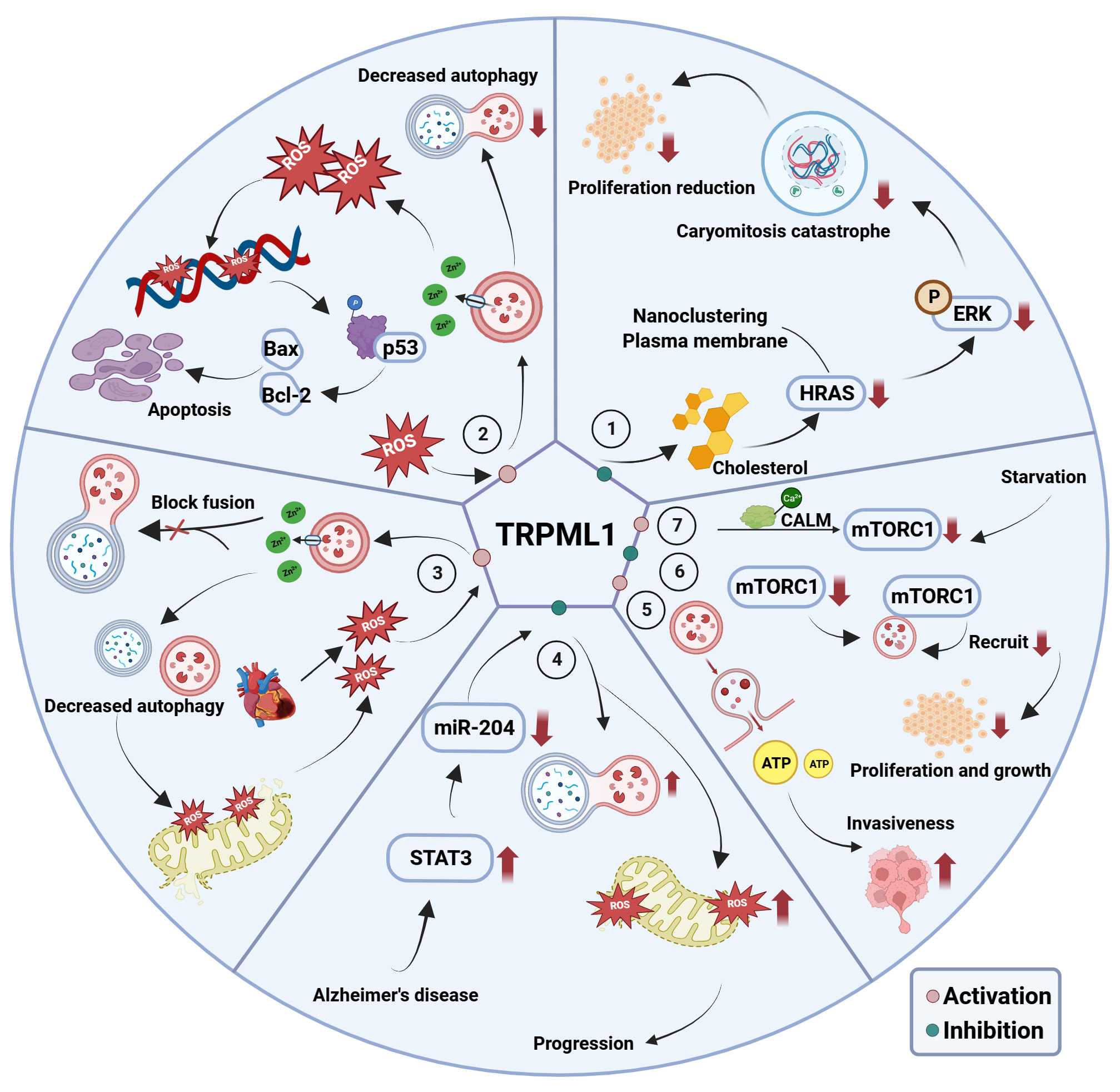
Figure 3 The mechanism of TRPML1’s involvement in associated diseases. ① TRPML1 inhibition disrupts cholesterol distribution and abundance, attenuating HRAS nanoclustering and plasma membrane abundance. This evokes unrestricted ERK phosphorylation, inhibitory mitogenic pathways and decreased cell proliferation; ② In melanoma and glioblastoma cells, activation of TRPML1 releases lysosomal Zn2+ to disrupt the fusion between autophagosomes and lysosomes, thereby triggering ‘autophagy inhibition, disrupted mitochondria turnover, ROS elevation, DNA damage, p53 activity, apoptosis’ axis, and ultimately triggering mitochondrial-mediated apoptosis; ③ TRPML1 is activated secondary to ROS elevation following ischemia/reperfusion, which in turn induces the release of lysosomal Zn2+ into the cytosol. This disrupts the fusion between autophagosomes and lysosomes, as well as mitochondria turnover, and further excessive ROS release; ④ In AD, high expression of miR-204 targets TRPML1, thus promoting ROS production and inhibiting mitochondrial autophagy, thereby promoting AD progression; ⑤ Elevated TRPML1 in MDA-MB-231 cell promotes cell invasion through enhanced lysosomal ATP release via lysosomal exocytosis; ⑥ Inhibition of TRPML1 reduction in mTORC1 activity as well as attenuation of lysosomal mTORC1 recruitment and decreased cell proliferation; ⑦ Activity of mTORC1 decreases during nutrients starvation, and then causing TRPML1 to be activated to release Ca2+ which binds to CALM, leading to mTORC1 recruitment onto lysosomes.
4.1 TRPML1 is a biomarker of various tumors
Tumor cells driven by HRas proto-oncogene GTPas (HRAS), a small GTPase member of the Ras superfamily, are more sensitive to TRPML1 inhibition, resulting in reduced growth, invasion, and proliferation of cancer cells (67). High TRPML1 expression is markedly associated with highly malignant cancers (68). The expression of TRPML1 in metastatic melanoma is higher than that in benign melanoma and normal skin tissue (69). Patients with pancreatic ductal adenocarcinoma with high expression of TRPML1 also have a poorer postoperative prognosis (70). Additionally, TRPML1 plays a role in breast cancer, especially in TNBC with high metastasis, high recurrence, and poor prognosis, as high TRPML1 expression is closely associated with the occurrence, development, and prognosis of TNBC (65, 66). Therefore, TRPML1 is an notable candidate for research on targeted therapy of TNBC.
4.2 TRPML1 regulates mTOR
mTOR regulates cell growth, proliferation, and metabolism in response to multiple substances, including growth factors, hormones, and energy (ATP). mTOR is a major signaling pathway for cancer cell growth and survival (71). Patients with phospho-mTOR expression showed substantially worse OS and recurrence-free survival rates. The association between mTOR activity and TNBC has attracted the attention of many researchers because mTOR overactivation is common in many cancers, including breast cancer, especially TNBC, as more activated mTOR is observed in TNBC than in other breast cancer subtypes (72).
As mentioned earlier, the mTOR inhibitor everolimus has entered the clinical trial stage for efficacy evaluation. However, since mTOR is widely involved in various biological processes in most cells, mTOR inhibitors do not provide substantial benefits to patients with cancer. Lysosomal calcium signaling plays a vital role in regulating the mTOR-dependent autophagy pathway, and TRPML1 provides negative feedback regulation of mTOR through calmodulin (73). Therefore, based on the regulatory effect of TRPML1 on mTOR, the development of regulatory drugs targeting TRPML1 activity to achieve tumor suppression may provide greater specificity and better therapeutic effects than mTOR inhibitors.
4.3 TRPML1 regulates autophagy
TRPML1 is critical for autophagy regulation. For example, upregulation of TRPML1 in Alzheimer’s disease (AD) inhibits reactive oxygen species (ROS) generation and mitophagy in AD through the signal transducer and activator of transcription 3 (STAT3) pathway, which is implicated in many aspects of autophagy (74). Pharmacological or genetic inhibition of TRPML1 restores impaired cardiomyocyte autophagy following myocardial ischemia/reperfusion injury (75). Concerning tumor cell survival in a more complex environment, TRPML1 activation inhibits autophagy in various cancer cell lines by disrupting the fusion between autophagosomes and lysosomes. More importantly, TRPML1-mediated inhibition of autophagy inhibits cancer cell growth by triggering apoptosis (62). The investigation aimed at elucidating how autophagy regulates apoptosis has revealed that TRPML1-induced autophagy inhibition triggers mitochondrial turnover disruption, leading to ROS elevation, which causes severe DNA damage in cancer cells (76). As mentioned previously, platinum drugs and PARPi are effective in treating TNBC by inhibiting DNA repair, however, drug resistance and side effects exist. Thus, TRPML1 may represent a new target for TNBC intervention via DNA repair pathway strategies.
In contrast, TRPML1 expression also has a protective survival effect in patients with glioblastoma (77); loss of TRPML1 impairs melanoma growth, reduces cell proliferation, and attenuates cell viability (78). Therefore, the conflicting roles of TRPML1 in regulating autophagy suggest that its role in tumors deserves further exploration. Regardless, TRPML1 plays an undeniably important role in mediating the occurrence and development of tumors through autophagy. Since TRPML1 is highly expressed in TNBC and is tightly linked to mTOR-dependent autophagy, we speculate that TRPML1 may affect TNBC by regulating autophagy.
4.4 Application of TRPML1 agonists in various treatments
Many studies have targeted TRPML1 to seek novel therapeutic methods for various diseases. In Parkinson’s disease, Artemisia annua leaf extract rescues neurons and elicits a neuroprotective effect by stimulating TRPML1 activity, promoting autophagy/mitochondrial autophagy, and upregulating survival pathways (79). In AD, endosomal–autophagic–lysosomal system defects in AD neurons are rescued by the reactivation of TRPML1 using the TRPML agonist ML-SA1 (80). Furthermore, treatment with a specific activator of TRPML1, ML1-SA1, decreases mitochondrial membrane potential through excessive activation of TRPML1 in liver cancer cells, resulting in mitochondrial damage and dysfunction (81). The same phenomenon was observed when TRPML1 was knocked out using the CRISPR/Cas9 gene editing technique. Studies on liver cancer have shown that TRPML1-mediated exocytosis promotes metastasis (81, 82). In malignant melanoma, TRPML1-induced inhibition of autophagy inhibits cancer metastasis by stimulating the ROS-mediated TP53/p53 pathway (83). TRPML1 agonist, ML-SA5, induces substantial cell death in malignant melanoma cell lines but fully preserves normal melanocytes in vitro. However, it fully preserves normal melanocytes (69). Another in vivo study showed that ML-SA5 improves muscle atrophy in Duchenne muscular dystrophy mice in vivo by promoting myosin repair (84). These studies demonstrate that clinical drugs targeting TRPML1 are possible. Further experiments should be conducted to identify drugs or techniques that change the function or expression of TRPML1 to clarify the specific mechanism of TRPML1 regulation in TNBC and other tumors and diseases for further development of novel treatments.
5 Discussion
TNBC accounts for only 15–20% of all breast cancer cases. However, it remains a challenging disease due to its poor cellular differentiation, high heterogeneity, and rapid metastasis (85). Clinically, in the early stages of TNBC, complete surgical excision with NAST is the standard treatment. Anthracycline/taxane-based combination chemotherapy is the first-line treatment for patients with metastatic or advanced TNBC. Although developing precise targets and inhibitors for TNBC has gradually attracted attention, their clinical effectiveness and safety require further verification. Finding new therapeutic targets and treatment methods remains an essential topic in TNBC research.
TRPML1 is an important biomarker of various tumors, and treatment with small-molecule activators that target TRPMLI has therapeutic potential in neurodegenerative diseases and various tumors. High expression of TRPML1 is closely associated with the occurrence, development, and prognosis of TNBC, and TRPML1 regulates multiple biological events and molecular targets associated with TNBC pathology. Therefore, TRPML1 could be a potential target for TNBC-targeted therapy. With the deepening of our understanding of the molecular characteristics and tumor microenvironment structure of TNBC and the development of new detection methods, more accurate and effective targeted treatment methods will emerge, resulting in improved curative effects in patients with TNBC.
Author contributions
YP: Writing – original draft. QZ: Writing – original draft. HH: Funding acquisition, Writing – review & editing. YQ: Writing – review & editing. YB: Writing – review & editing. JZ: Conceptualization, Writing – review & editing. YY: Conceptualization, Funding acquisition, Supervision, Writing – review & editing.
Funding
The author(s) declare financial support was received for the research, authorship, and/or publication of this article. This work was supported by the National Natural Science Foundation of China (Grant No. 82203696), “Medicine + X” Cross-Innovation team of Bethune Medical Department of Jilin University (Grant No. 2022JBGS10), the Health Science and Technology Capacity Improvement Project of Jilin Province (Grant No. 2022JC079).
Acknowledgments
We appreciate the encouragement and helpful comments from other members of the Department of Histology and Embryology, and the Department of Cell Biology, College of Basic Medical Sciences, Jilin University.
Conflict of interest
The authors declare that the research was conducted in the absence of any commercial or financial relationships that could be construed as a potential conflict of interest.
Publisher’s note
All claims expressed in this article are solely those of the authors and do not necessarily represent those of their affiliated organizations, or those of the publisher, the editors and the reviewers. Any product that may be evaluated in this article, or claim that may be made by its manufacturer, is not guaranteed or endorsed by the publisher.
References
1. Sung H, Ferlay J, Siegel RL, Laversanne M, Soerjomataram I, Jemal A, et al. Global cancer statistics 2020: GLOBOCAN estimates of incidence and mortality worldwide for 36 cancers in 185 countries. CA Cancer J Clin (2021) 71:209–49. doi: 10.3322/caac.21660
2. Ovcaricek T, Frkovic SG, Matos E, Mozina B, Borstnar S. Triple negative breast cancer - prognostic factors and survival. Radiol Onco (2011) 45:46–52. doi: 10.2478/v10019-010-0054-4
3. Cummings MC, Simpson PT, Reid LE, Jayanthan J, Skerman J, Song S, et al. Metastatic progression of breast cancer: insights from 50 years of autopsies. J Pathol (2014) 232:23–31. doi: 10.1002/path.4288
4. Yersal O, Barutca S. Biological subtypes of breast cancer: Prognostic and therapeutic implications. World J Clin Oncol (2014) 5:412–24. doi: 10.5306/wjco.v5.i3.412
5. Vagia E, Mahalingam D, Cristofanilli M. The landscape of targeted therapies in TNBC. Cancers (Basel) (2020) 12:916. doi: 10.3390/cancers12040916
6. Dent R, Trudeau M, Pritchard KI, Hanna WM, Kahn HK, Sawka CA, et al. Triple-negative breast cancer: clinical features and patterns of recurrence. Clin Cancer Res (2007) 13:4429–34. doi: 10.1158/1078-0432.CCR-06-3045
7. Lin NU, Claus E, Sohl J, Razzak AR, Arnaout A, Winer EP. Sites of distant recurrence and clinical outcomes in patients with metastatic triple-negative breast cancer: high incidence of central nervous system metastases. Cancer (2008) 113:2638–45. doi: 10.1002/cncr.23930
8. Gluz O, Liedtke C, Gottschalk N, Pusztai L, Nitz U, Harbeck N. Triple-negative breast cancer—current status and future directions. Ann Oncol (2009) 20:1913–27. doi: 10.1093/annonc/mdp492
9. Taylor C, McGale P, Probert J, Broggio J, Charman J, Darby SC, et al. Breast cancer mortality in 500 000 women with early invasive breast cancer diagnosed in England, 1993-2015: population based observational cohort study. BMJ (2023) 381:e074684. doi: 10.1136/bmj-2022-074684
10. Lehmann BD, Bauer JA, Chen X, Sanders ME, Chakravarthy AB, Shyr Y, et al. Identification of human triple-negative breast cancer subtypes and preclinical models for selection of targeted therapies. J Clin Invest (2011) 121:2750–67. doi: 10.1172/JCI45014
11. Lehmann BD, Pietenpol JA. Identification and use of biomarkers in treatment strategies for triple-negative breast cancer subtypes. J Pathol (2014) 232:142–50. doi: 10.1002/path.4280
12. Yin L, Duan JJ, Bian XW, Yu SC. Triple-negative breast cancer molecular subtyping and treatment progress. Breast Cancer Res (2020) 22:61. doi: 10.1186/s13058-020-01296-5
13. Kwapisz D. Pembrolizumab and atezolizumab in triple-negative breast cancer. Cancer Immunol Immunother (2021) 70:607–17. doi: 10.1007/s00262-020-02736-z
14. Pace LE, Keating NL. A systematic assessment of benefits and risks to guide breast cancer screening decisions. JAMA (2014) 311:1327–35. doi: 10.1001/jama.2014.1398
15. Kuhl CK. Abbreviated magnetic resonance imaging (MRI) for breast cancer screening: rationale, concept, and transfer to clinical practice. Annu Rev Med (2019) 70:501–19. doi: 10.1146/annurev-med-121417-100403
16. Berg WA. Tailored supplemental screening for breast cancer: what now and what next? AJR Am J Roentgenol (2009) 192:390–9. doi: 10.2214/AJR.08.1706
17. Groheux D. FDG-PET/CT for primary staging and detection of recurrence of breast cancer. Semin Nucl Med (2022) 52:508–19. doi: 10.1053/j.semnuclmed.2022.05.001
18. Paydary K, Seraj SM, Zadeh MZ, Emamzadehfard S, Shamchi SP, Gholami S, et al. The evolving role of FDG-PET/CT in the diagnosis, staging, and treatment of breast cancer. Mol Imaging Biol (2019) 21:1–10. doi: 10.1007/s11307-018-1181-3
19. McDonald ES, Clark AS, Tchou J, Zhang P, Freedman GM. Clinical diagnosis and management of breast cancer. J Nucl Med (2016) 57 Suppl 1:9S–16S. doi: 10.2967/jnumed.115.157834
20. De la Cruz Ku G, Karamchandani M, Chambergo-Michilot D, Narvaez-Rojas AR, Jonczyk M, Príncipe-Meneses FS, et al. Does breast-conserving surgery with radiotherapy have a better survival than mastectomy? A meta-analysis of more than 1,500,000 patients. Ann Surg Oncol (2022) 29:6163–88. doi: 10.1245/s10434-022-12133-8
21. Harbeck N, Gnant M. Breast cancer. Lancet (2017) 389:1134–50. doi: 10.1016/S0140-6736(16)31891-8
22. McAndrew NP, Finn RS. Clinical review on the management of hormone receptor-positive metastatic breast cancer. JCO Oncol Pract (2022) 18:319–27. doi: 10.1200/OP.21.00384
23. Figueroa-Magalhães MC, Jelovac D, Connolly R, Wolff AC. Treatment of HER2-positive breast cancer. Breast (2014) 23:128–36. doi: 10.1016/j.breast.2013.11.011
24. Clayton AJ, Danson S, Jolly S, Ryder WD, Burt PA, Stewart AL, et al. Incidence of cerebral metastases in patients treated with trastuzumab for metastatic breast cancer. Br J Cancer (2004) 91:639–43. doi: 10.1038/sj.bjc.6601970
25. Gianni L, Pienkowski T, Im YH, Roman L, Tseng LM, Liu MC, et al. Efficacy and safety of neoadjuvant pertuzumab and trastuzumab in women with locally advanced, inflammatory, or early HER2-positive breast cancer (NeoSphere): a randomised multicentre, open-label, phase 2 trial. Lancet Oncol (2012) 13:25–32. doi: 10.1016/S1470-2045(11)70336-9
26. Carey LA, Dees EC, Sawyer L, Gatti L, Moore DT, Collichio F, et al. The triple negative paradox: primary tumor chemosensitivity of breast cancer subtypes. Clin Cancer Res (2007) 13:2329–34. doi: 10.1158/1078-0432.CCR-06-1109
27. Wahba HA, El-Hadaad HA. Current approaches in treatment of triple-negative breast cancer. Cancer Biol Med (2015) 12:106–16. doi: 10.7497/j.issn.2095-3941.2015.0030
28. Mittendorf EA, Zhang H, Barrios CH, Sajo S, Jung H, Hegg R, et al. Neoadjuvant atezolizumab in combination with sequential nab-paclitaxel and anthracycline-based chemotherapy versus placebo and chemotherapy in patients with early-stage triple-negative breast cancer (IMpassion031): a randomised, double-blind, phase 3 trial. Lancet (2020) 396:1090–100. doi: 10.1016/S0140-6736(20)31953-X
29. Li ZY, Zhang Z, Cao XZ, Feng Y, Ren SS. Platinum-based neoadjuvant chemotherapy for triple-negative breast cancer: a systematic review and meta-analysis. J Int Med Res (2020) 48:300060520964340. doi: 10.1177/0300060520964340
30. Guo P, Huang J, Zhu B, Huang AC, Jiang L, Fang J, et al. A rationally designed ICAM1 antibody drug conjugate eradicates late-stage and refractory triple-negative breast tumors in vivo. Sci Adv (2023) 9:eabq7866. doi: 10.1126/sciadv.abq7866
31. Sikov WM, Berry DA, Perou CM, Singh B, Cirrincione CT, Tolaney SM, et al. Impact of the addition of carboplatin and/or bevacizumab to neoadjuvant once-per-week paclitaxel followed by dose-dense doxorubicin and cyclophosphamide on pathologic complete response rates in stage II to III triple-negative breast cancer: CALGB 40603 (Alliance). J Clin Oncol (2015) 33:13–21. doi: 10.1200/JCO.2014.57.0572
32. Khoury R, Saleh K, Khalife N, Saleh M, Chahine C, Ibrahim R, et al. Mechanisms of resistance to antibody-drug conjugates. Int J Mol Sci (2023) 24:1278. doi: 10.3390/ijms24119674
33. LaFargue CJ, Dal Molin GZ, Sood AK, Coleman RL. Exploring and comparing adverse events between PARP inhibitors. Lancet Oncol (2019) 20:e15–28. doi: 10.1016/S1470-2045(18)30786-1
34. Cortesi L, Rugo HS, Jackisch C. An overview of PARP inhibitors for the treatment of breast cancer. Target Oncol (2021) 16:255–82. doi: 10.1007/s11523-021-00796-4
35. Isakoff SJ, Puhalla S, Domchek SM, Friedlander M, Kaufman B, Robson M, et al. A randomized Phase II study of veliparib with temozolomide or carboplatin/paclitaxel versus placebo with carboplatin/paclitaxel in BRCA1/2 metastatic breast cancer: design and rationale. Future Oncol (2017) 13:307–20. doi: 10.2217/fon-2016-0412
36. Slade D. PARP and PARG inhibitors in cancer treatment. Future Oncol (2017) 13:307–20. doi: 10.2217/fon-2016-0412
37. Hahnen E, Lederer B, Hauke J, Loibl S, Kröber S, Schneeweiss A, et al. Germline mutation status, pathological complete response, and disease-free survival in triple-negative breast cancer: secondary analysis of the GeparSixto randomized clinical trial. JAMA Oncol (2017) 3:1378–85. doi: 10.1001/jamaoncol.2017.1007
38. Bergin ART, Loi S. Triple-negative breast cancer: recent treatment advances. F1000Res (2019) 8:F1000 Faculty Rev–1342. doi: 10.12688/f1000research.18888.1
39. Tarantino P, Gandini S, Trapani D, Criscitiello C, Curigliano G. Immunotherapy addition to neoadjuvant chemotherapy for early triple negative breast cancer: A systematic review and meta-analysis of randomized clinical trials. Crit Rev Oncol Hematol (2021) 159:103223. doi: 10.1016/j.critrevonc.2021.103223
40. Leon-Ferre RA, Goetz MP. Advances in systemic therapies for triple negative breast cancer. BMJ (2023) 381:e071674. doi: 10.1136/bmj-2022-071674
41. Latif F, Bint Abdul Jabbar H, Malik H, Sadaf H, Sarfraz A, Sarfraz Z, et al. Atezolizumab and pembrolizumab in triple-negative breast cancer: a meta-analysis. Expert Rev Anticancer Ther (2022) 22:229–35. doi: 10.1080/14737140.2022.2023011
42. Dirix LY, Takacs I, Jerusalem G, Nikolinakos P, Arkenau HT, Forero-Torres A, et al. Avelumab, an anti-PD-L1 antibody, in patients with locally advanced or metastatic breast cancer: a phase 1b JAVELIN Solid Tumor study. Breast Cancer Res Treat (2018) 167:671–86. doi: 10.1007/s10549-017-4537-5
43. Shih T, Lindley C. Bevacizumab: an angiogenesis inhibitor for the treatment of solid Malignancies. Clin Ther (2006) 28:1779–802. doi: 10.1016/j.clinthera.2006.11.015
44. Miller KD, O’Neill A, Perez EA, Seidman AD, Sledge GW. A phase II pilot trial incorporating bevacizumab into dose-dense doxorubicin and cyclophosphamide followed by paclitaxel in patients with lymph node positive breast cancer: a trial coordinated by the Eastern Cooperative Oncology Group. Ann Oncol (2012) 23:331–7. doi: 10.1093/annonc/mdr344
45. Miller K, Wang M, Gralow J, Dickler M, Cobleigh M, Perez EA, et al. Paclitaxel plus bevacizumab versus paclitaxel alone for metastatic breast cancer. N Engl J Med (2007) 357:2666–76. doi: 10.1056/NEJMoa072113
46. Son S, Shin S, Rao NV, Um W, Jeon J, Ko H, et al. Anti-Trop2 antibody-conjugated bioreducible nanoparticles for targeted triple negative breast cancer therapy. Int J Biol Macromol (2018) 110:406–15. doi: 10.1016/j.ijbiomac.2017.10.113
47. Bardia A, Hurvitz SA, Tolaney SM, Loirat D, Punie K, Oliveira M, et al. Sacituzumab govitecan in metastatic triple-negative breast cancer. N Engl J Med (2021) 384:1529–41. doi: 10.1056/NEJMoa2028485
48. Michaleas S, Moreno Oliver A, Mueller-Berghaus J, Sarac SB, van der Elst ME, Müller-Egert S, et al. The European Medicines Agency review of 13acituzumab govitecan for the treatment of triple-negative breast cancer. ESMO Open (2022) 7:100497. doi: 10.1016/j.esmoop.2022.100497
49. Rizzo A, Cusmai A, Acquafredda S, Rinaldi L, Palmiotti G. Ladiratuzumab vedotin for metastatic triple negative cancer: preliminary results, key challenges, and clinical potential. Expert Opin Investig Drugs (2022) 31:495–8. doi: 10.1080/13543784.2022.2042252
50. Hua H, Kong Q, Zhang H, Wang J, Luo T, Jiang Y. Targeting mTOR for cancer therapy. J Hematol Oncol (2019) 12:71. doi: 10.1186/s13045-019-0754-1
51. Oliveira M, Saura C, Nuciforo P, Calvo I, Andersen J, Passos-Coelho JL, et al. FAIRLANE, a double-blind placebo-controlled randomized phase II trial of neoadjuvant ipatasertib plus paclitaxel for early triple-negative breast cancer. Ann Oncol (2019) 30:1289–97. doi: 10.1093/annonc/mdz177
52. Ganesan P, Moulder S, Lee JJ, Janku F, Valero V, Zinner RG, et al. Triple-negative breast cancer patients treated at MD Anderson Cancer Center in phase I trials: improved outcomes with combination chemotherapy and targeted agents. Mol Cancer Ther (2014) 13:3175–84. doi: 10.1158/1535-7163.MCT-14-0358
53. Schmid P, Abraham J, Chan S, Wheatley D, Brunt AM, Nemsadze G, et al. Capivasertib plus paclitaxel versus placebo plus paclitaxel as first-line therapy for metastatic triple-negative breast cancer: The PAKT Trial. J Clin Oncol (2020) 38:423–33. doi: 10.1200/JCO.19.00368
54. Lee JS, Yost SE, Blanchard S, Schmolze D, Yin HH, Pillai R, et al. Phase I clinical trial of the combination of eribulin and everolimus in patients with metastatic triple-negative breast cancer. Breast Cancer Res (2019) 21:119. doi: 10.1186/s13058-019-1202-4
55. Cleator S, Heller W, Coombes RC. Triple-negative breast cancer: therapeutic options. Lancet Oncol (2007) 8:235–44. doi: 10.1016/S1470-2045(07)70074-8
56. Waks AG, Winer EP. Breast cancer treatment: A review. JAMA (2019) 321:288–300. doi: 10.1001/jama.2018.19323
57. Salgado R, Denkert C, Demaria S, Sirtaine N, Klauschen F, Pruneri G. The evaluation of tumor-infiltrating lymphocytes (TILs) in breast cancer: recommendations by an International TILs Working Group 2014. Ann Oncol (2015) 26:259–71. doi: 10.1093/annonc/mdu450
58. Bou Zerdan M, Ghorayeb T, Saliba F, Allam S, Bou Zerdan M, Yaghi M, et al. Triple negative breast cancer: updates on classification and treatment in 2021. Cancers (Basel) (2022) 14:1253. doi: 10.3390/cancers14051253
59. Fruman DA, Rommel C. PI3K and cancer: lessons, challenges and opportunities. Nat Rev Drug Discovery (2014) 13:140–56. doi: 10.1038/nrd4204
60. Paplomata E, O’Regan R. The PI3K/AKT/mTOR pathway in breast cancer: targets, trials and biomarkers. Ther Adv Med Oncol (2014) 6:154–66. doi: 10.1177/1758834014530023
61. Jiang YZ, Ma D, Suo C, Shi J, Xue M, Hu X, et al. Genomic and transcriptomic landscape of triple-negative breast cancers: subtypes and treatment strategies. Cancer Cell (2019) 35:428–440.e5. doi: 10.1016/j.ccell.2019.02.001
62. Qi J, Xing Y, Liu Y, Wang MM, Wei X, Sui Z, et al. MCOLN1/TRPML1 finely controls oncogenic autophagy in cancer by mediating zinc influx. Autophagy (2021) 17:4401–22. doi: 10.1080/15548627.2021.1917132
63. Zhong D, Wang R, Zhang H, Wang M, Zhang X, Chen H. Induction of lysosomal exocytosis and biogenesis via TRPML1 activation for the treatment of uranium-induced nephrotoxicity. Nat Commun (2023) 14:3997. doi: 10.1038/s41467-023-39716-7
64. Wang W, Gao Q, Yang M, Zhang X, Yu L, Lawas M, et al. Up-regulation of lysosomal TRPML1 channels is essential for lysosomal adaptation to nutrient starvation. Proc Natl Acad Sci U.S.A. (2015) 112:E1373–81. doi: 10.1073/pnas.1419669112
65. Xu M, Almasi S, Yang Y, Yan C, Sterea AM, Rizvi Syeda AK, et al. The lysosomal TRPML1 channel regulates triple negative breast cancer development by promoting mTORC1 and purinergic signaling pathways. Cell Calcium (2019) 79:80–8. doi: 10.1016/j.ceca.2019.02.010
66. Shekoufeh A, Kennedy BE, Yoast RE, Emrich M, Trebak M, Hiani YE. The lysosomal TRPML1 channel promotes breast cancer survival by supporting mitochondrial function and cellular metabolism. bioRxiv (2020). doi: 10.1101/2020.09.04.283242
67. Jung J, Cho KJ, Naji AK, Clemons KN, Wong CO, Villanueva M, et al. HRAS-driven cancer cells are vulnerable to TRPML1 inhibition. EMBO Rep (2019) 20:e46685. doi: 10.15252/embr.201846685
68. Yang Y, Zhai X, El Hiani Y. TRPML1-emerging roles in cancer. Cells (2020) 9:2682. doi: 10.3390/cells9122682
69. Du W, Gu M, Hu M, Pinchi P, Chen W, Ryan M, et al. Lysosomal Zn2+ release triggers rapid, mitochondria-mediated, non-apoptotic cell death in metastatic melanoma. Cell Rep (2021) 37:109848. doi: 10.1016/j.celrep.2021.109848
70. Hu ZD, Jun Y, Cao KY, Yin ZQ, Xin WW, Zhang MF. MCOLN1 promotes proliferation and predicts poor survival of patients with pancreatic ductal adenocarcinoma. Dis Markers (2019) 2019:9436047. doi: 10.1155/2019/9436047
71. Wander SA, Zhao D, Besser AH, Hong F, Wei J, Ince TA, et al. PI3K/mTOR inhibition can impair tumor invasion and metastasis in vivo despite a lack of antiproliferative action in vitro: implications for targeted therapy. Breast Cancer Res Treat (2013) 138:369–81. doi: 10.1007/s10549-012-2389-6
72. Ueng SH, Chen SC, Chang YS, Hsueh S, Lin YC, Chien HP, et al. Phosphorylated mTOR expression correlates with poor outcome in early-stage triple negative breast carcinomas. Int J Clin Exp Pathol (2012) 5:806–13.
73. Sun X, Yang Y, Zhong XZ, Cao Q, Zhu XH, Zhu X, et al. A negative feedback regulation of MTORC1 activity by the lysosomal Ca(2+) channel MCOLN1 (mucolipin 1) using a CALM (calmodulin)-dependent mechanism. Autophagy (2018) 14:38–52. doi: 10.1080/15548627.2017.1389822
74. Zhang L, Fang Y, Zhao X, Zheng Y, Ma Y, Li S, et al. miR-204 silencing reduces mitochondrial autophagy and ROS production in a murine AD model via the TRPML1-activated STAT3 pathway. Mol Ther Nucleic Acids (2021) 24:822–31. doi: 10.1016/j.omtn.2021.02.010
75. Xing Y, Sui Z, Liu Y, Wang MM, Wei X, Lu Q, et al. Blunting TRPML1 channels protects myocardial ischemia/reperfusion injury by restoring impaired cardiomyocyte autophagy. Basic Res Cardiol (2022) 117:20. doi: 10.1007/s00395-022-00930-x
76. Liu Y, Wang X, Zhu W, Sui Z, Wei X, Zhang Y, et al. TRPML1-induced autophagy inhibition triggers mitochondrial mediated apoptosis. Cancer Lett (2022) 541:215752. doi: 10.1016/j.canlet.2022.215752
77. Morelli MB, Amantini C, Tomassoni D, Nabissi M, Arcella A, Santoni G. Transient receptor potential mucolipin-1 channels in glioblastoma: role in patient’s survival. Cancers (Basel) (2019) 11:525. doi: 10.3390/cancers11040525
78. Abrahamian C, Grimm C. Endolysosomal cation channels and MITF in melanocytes and melanoma. Biomolecules (2021) 11:1021. doi: 10.3390/biom11071021
79. Wu LK, Agarwal S, Kuo CH, Kung YL, Day CH, Lin PY, et al. Artemisia Leaf Extract protects against neuron toxicity by TRPML1 activation and promoting autophagy/mitophagy clearance in both in vitro and in vivo models of MPP+/MPTP-induced Parkinson’s disease. Phytomedicine (2022) 104:154250. doi: 10.1016/j.phymed.2022.154250
80. Somogyi A, Kirkham ED, Lloyd-Evans E, Winston J, Allen ND, Mackrill JJ, et al. The synthetic TRPML1 agonist ML-SA1 rescues Alzheimer-related alterations of the endosomal-autophagic-lysosomal system. J Cell Sci (2023) 136:jcs259875. doi: 10.1242/jcs.259875
81. Siow WX, Kabiri Y, Tang R, Chao YK, Plesch E, Eberhagen C, et al. Lysosomal TRPML1 regulates mitochondrial function in hepatocellular carcinoma cells. J Cell Sci (2022) 135:jcs259455. doi: 10.1242/jcs.259455
82. Lyu L, Jin X, Li Z, Liu S, Li Y, Su R, et al. TBBPA regulates calcium-mediated lysosomal exocytosis and thereby promotes invasion and migration in hepatocellular carcinoma. Ecotoxicol Environ Saf (2020) 192:110255. doi: 10.1016/j.ecoenv.2020.110255
83. Xing Y, Wei X, Liu Y, Wang MM, Sui Z, Wang X, et al. Autophagy inhibition mediated by MCOLN1/TRPML1 suppresses cancer metastasis via regulating a ROS-driven TP53/p53 pathway. Autophagy (2022) 18:1932–54. doi: 10.1080/15548627.2021.2008752
84. Yu L, Zhang X, Yang Y, Li D, Tang K, Zhao Z, et al. Small-molecule activation of lysosomal TRP channels ameliorates Duchenne muscular dystrophy in mouse models. Sci Adv (2020) 6:eaaz2736. doi: 10.1126/sciadv.aaz2736
Keywords: triple-negative breast cancer, TRPML1, therapeutic target, cancer treatment, autophagy
Citation: Pan Y, Zhao Q, He H, Qi Y, Bai Y, Zhao J and Yang Y (2023) TRPML1 as a potential therapeutic target for triple-negative breast cancer: a review. Front. Oncol. 13:1326023. doi: 10.3389/fonc.2023.1326023
Received: 22 October 2023; Accepted: 01 December 2023;
Published: 13 December 2023.
Edited by:
Armando Pérez Torres, National Autonomous University of Mexico, MexicoReviewed by:
Wuyang Wang, Xuzhou Medical University, ChinaLuis E Arias-Romero, National Autonomous University of Mexico, Mexico
Copyright © 2023 Pan, Zhao, He, Qi, Bai, Zhao and Yang. This is an open-access article distributed under the terms of the Creative Commons Attribution License (CC BY). The use, distribution or reproduction in other forums is permitted, provided the original author(s) and the copyright owner(s) are credited and that the original publication in this journal is cited, in accordance with accepted academic practice. No use, distribution or reproduction is permitted which does not comply with these terms.
*Correspondence: Yiming Yang, eW1feWFuZ0BqbHUuZWR1LmNu; Jia Zhao, emhhb2ozMTBAamx1LmVkdS5jbg==