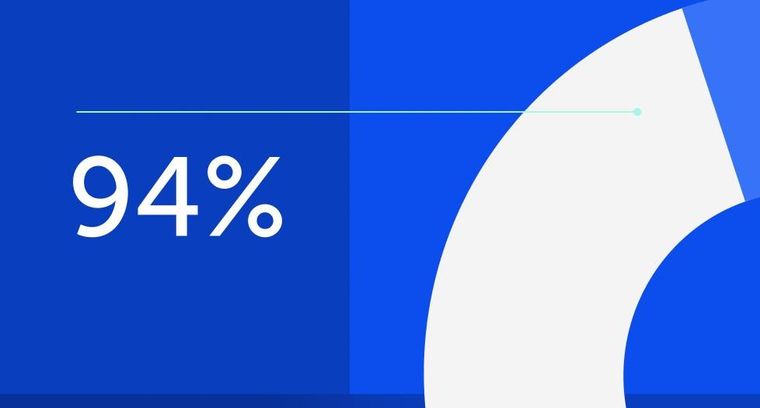
94% of researchers rate our articles as excellent or good
Learn more about the work of our research integrity team to safeguard the quality of each article we publish.
Find out more
REVIEW article
Front. Oncol., 20 September 2023
Sec. Molecular and Cellular Oncology
Volume 13 - 2023 | https://doi.org/10.3389/fonc.2023.1265347
This article is part of the Research TopicRole of nanocarriers and non-coding RNAs in solid malignanciesView all 4 articles
Alzheimer’s disease (AD) and brain tumors are debilitating neurological conditions that pose significant challenges in current medical practices. Existing treatment options for AD primarily focus on symptom management, and brain tumors often require aggressive therapeutic approaches. Novel disease-modifying strategies and therapeutic agents are urgently needed to address the underlying causes of AD pathogenesis and improve brain tumor management. In recent years, nanoparticles (NPs) have shown promise as valuable tools in diagnosing and managing various brain disorders, including AD. Among these, carbon nanotubes (CNTs) have garnered attention for their unique properties and biomedical potential. Their ability to cross the blood-brain barrier (BBB) with ease opens up new possibilities for targeted drug delivery and neuroprotection. This literature review aims to explore the versatile nature of CNTs, which can be functionalized with various biomolecules or substances due to their sp2 hybridization. This adaptability enables them to specifically target cells and deliver medications under specific environmental conditions. Moreover, CNTs possess an exceptional capacity to penetrate cell membranes, making them valuable tools in the treatment of AD and brain tumors. By delving into the role of CNTs in biomedicine, this review sheds light on their potential in managing AD, offering a glimpse of hope for effective disease-modifying options. Understanding the mechanisms of CNTs’ action and their capabilities in targeting and delivering medication to affected cells will pave the way for innovative therapeutic strategies that can improve the lives of those afflicted with these devastating neurological conditions. The exploration of CNTs as a dual therapeutic arsenal for both brain tumors and Alzheimer’s disease holds great promise and may usher in a new era of effective treatment strategies for these challenging conditions.
Alzheimer’s disease (AD) and brain tumors represent two of the most complex and devastating neurological conditions, affecting millions worldwide (1, 2). Despite significant research efforts, effective disease-modifying treatments for both AD and brain tumors remain elusive (3). Current approaches often focus on managing symptoms rather than addressing the underlying causes, burdening patients and their families with the progressive deterioration of cognitive function or the challenges of aggressive tumor growth (4).
In recent years, nanotechnology has emerged as a promising frontier in biomedical research, offering potential solutions to various brain disorders (5). Among these nanomaterials, carbon nanotubes (CNTs) have garnered substantial attention for their exceptional properties and biomedical versatility (6). With their unique ability to easily traverse the blood-brain barrier (BBB), CNTs open new avenues for targeted drug delivery and neuroprotection in the intricate environment of the brain (7).
CNTs possess remarkable properties stemming from their sp2 hybridization, allowing them to be functionalized with various biomolecules and substances (8). This adaptability enables CNTs to specifically target cells and deliver therapeutic agents precisely where needed, even under specific environmental conditions. Additionally, CNTs’ exceptional capacity to penetrate cell membranes makes them potential game-changers in treating both AD and brain tumors (9).
CNTs have several advantages as compared to other nanosystems, such as unique physicochemical properties, biological interaction with brain cancerous tissues, ability to penetrate BBB, and bio-corona effect (10).
In this literature review, we aim to explore the transformative potential of CNTs as a dual therapeutic arsenal for both brain tumors and Alzheimer’s disease. By comprehending the intricacies of CNTs’ applications in biomedicine, we seek to shed light on their role in neuroprotection and targeted medication delivery. Understanding the mechanisms of CNTs’ action and their capabilities in targeting affected cells holds promise for devising innovative and effective disease-modifying strategies.
As the search for more sustainable and potent treatments for AD and brain tumors intensifies, exploring CNTs as a therapeutic tool provides hope for groundbreaking advancements in neurology and oncology. Our quest to unveil the true potential of carbon nanotubes brings us closer to addressing the challenges posed by these devastating neurological conditions, offering a glimmer of hope to those affected and their loved ones.
The types of nanoparticles (NPs) can be transported are shown in (Figure 1).
Figure 1 Representation of Blood Brain Barrier and Transport of Nanoparticles. The transport of NPs across BBB is represented by four prominent mechanisms commonly enrooted for the transport of solute molecules. A: Transport Proteins - these channel proteins facilitate the passage of glucose, amino acids, and ketones across the BBB and hence, can be used to transport NPs. B: Diffusion - passage of molecules from higher concentration to lower such as small lipophilic molecules, O2, and AuNPs. C: Adsorption-mediated Transcytosis - several cationic molecules, liposomes, and cationic NPs are endocytosed across BBB. D: Receptor-mediated transcytosis is facilitated by receptor-ligand interaction as seen in the transport of iron mediated by transferrin (receptor). NPs can be transported along this mechanism via the transferrin NPs conjugates.
Carbon nanotubes (CNTs), one of the types of NPs, are a potent tool for enhancing biomedical methods in treating and managing many diseases, including AD and Brain malignancies (11, 12). CNTs can adsorb different molecules of drugs on their surface (13). CNTs have good electronic properties, significant capability to cross the cell membrane and BBB, thermal properties, high drug loading capacity, and can be easily modified with other molecules (9). In this literature review, we discuss the role of CNTs as a tool for delivering drugs or chemical compounds to the brain.
Nanoparticles have revolutionized medicine, offering new and promising possibilities for managing challenging conditions like Alzheimer’s disease and brain tumors. Their unique properties, particularly their small size, make them excellent candidates for targeted drug delivery and therapeutic interventions, allowing precise treatment targeting specific areas, such as the brain (14). In this context, Table 1 presents a comprehensive overview of diverse nanoparticle types that have been extensively investigated in the pursuit of effective Alzheimer’s disease management and Brain tumor.
Table 1 Comparative analysis of nanoparticle-based approaches for alzheimer’s disease and brain tumor.
CNTs discovered in 1991 (11), are a new allotrope of carbon that warrants special attention because of their inborn characteristics like their surface, form, and physical properties, which make them particularly ideal for therapeutic applications (30). A graphene sheet is folded into a cylindrical shape to create CNTs, which are tubular objects (31). Single-walled carbon nanotubes (SWCNTs), composed of one graphene sheet with a diameter between 0.4 and 40 nm, and multiwalled carbon nanotubes (MWCNTs), consisting of many layers forming cylinders with a distance of 0.35 nm between the concentric layers as shown in Figure 2. Typically, half-fullerene molecules shut nanotubes’ ends, while pentagonal defects serve as the tips. Furthermore, CNTs can be divided into three groups on how the sheets are revolved: zig-zag, chiral nanotubes, or armchair. Each carbon atom in CNTs is associated with an sp2 hybridization, giving them exceptional mechanical, optical, thermal, and electrical capabilities. One electron in the s orbital is promoted to one of the 2p atomic orbitals as part of the sp2 hybridization, a combination of one s and two p atomic orbitals. These two atomic orbitals combine to form three new, equally energetic hybrid orbitals. The energy of the hybrid orbitals is higher than the energy of the s orbital, lower than that of the p orbitals, but closer to the energy of the p orbitals. Trigonal structures are produced by the newly created hybrid orbitals, giving rise to a 120-degree molecular geometry. Therefore, sp2 hybridization makes stronger bonds. They are among the most reliable nanomaterials for potential uses in nanomedicine and nanoelectronics (11). Moreover, because of their capacity to cross cell membranes, these substances have been studied for their ability to generate ion transport channels, i.e., transporters for various drugs, biomolecules, and Deoxyribonucleic acid (DNA), and Ribonucleic acid (RNA). Additionally, because of their smaller internal size and unique electrical properties brought on by the curvature of their walls, CNTs have also been employed as nanoreactors (32). However, despite having a huge range of biomedical uses, carbon nanotubes possess two flaws that need to be addressed: their limited solubility in water and inherent toxicity induced by the metal catalyst residue left over from their production process.
Three methods are frequently utilized to create carbon nanotubes:
1. Chemical Vapor Deposition (CVD) method
2. Arc Discharge method
3. Laser Ablation method
These techniques are based on forming single or multiple carbon atoms that can merge to form carbon nanotubes. To synthesize carbon nanotubes, all processes require a source of energy and carbon (Figure 3). Carbon sources can be gases or carbon electrodes, and energy sources can include arc discharge, heat, or laser beams (33). Additionally, it is proposed that the CNT diameter is influenced by the dimensions of the metal catalyst’s particle; finer metal catalyst particle sizes are likely to produce SWCNTs with a narrower diameter, whilst bigger metal catalyst particle sizes seem to make MWCNTs with a broader diameter (34).
The most popular technique for producing carbon nanotubes on a substantial scale is chemical vapor deposition. It uses hydrocarbons such as carbon monoxide, CH4, or acetylene, and elevated temperature gives the hydrocarbons enough energy to break down and generate carbon nanotubes. Commonly, SWCNTs can be created by chemical vapor deposition of acetylene utilizing a catalyst (iron or cobalt) supported by silica or zeolite; this leads to the production of large volumes of carbon nanotubes (34).
The arc discharge method is the most popular and simple method for manufacturing CNTs, which has been used for many years to create fullerenes. Carbon electrodes are being employed as the source, and with the help of current (roughly within the range of 50 A to 100 A) and potential difference (nearly 20V), an electric discharge is generated, which acts as an energy source. It is considered that an electric discharge inside an inert environment at reduced pressure and with a catalyst such as nickel, iron, or cobalt creates enough high temperature to cause one of the carbon electrode surfaces to evaporate and create a tiny rod-shaped deposition of nanotubes on another electrode (35). This technique produces nanotubes with a diameter ranging between 0.6 nm - 1.2 nm (34).
This approach uses carbon electrodes as the carbon and energy sources through laser pulses. The surface is uniformly vaporized by consecutive laser pulses, which also minimizes the quantity of carbon that has settled down as soot (36). The second pulse is believed to break up the bigger particles and the first laser pulse ablates smaller particles, subsequently developing into structures called nanotubes. Typically, transition metal catalysts are employed in this procedure, and the nanotubes produced with it are often achieved as rope-like structures with consistent diameters of around 10 nm to 20 nm, and the length of tubes is around 100 µm (37).
Since their discovery in the 1990s, the application of carbon nanotubes has been explored in several areas, including nanotechnology, industrial production, construction, electronics, wastewater management, and others (38–42). CNTs demonstrate a variety of distinctive attributes, including electrical and optical properties, a substantial surface area, and defined physicochemical characteristics. Solubility and toxicity limit the application of CNTs in biomedical sciences, but they can be reduced to a great extent with functionalization. The use of nano drug delivery in treating chronic neurological disorders results in neuroprotection. Nanomedicine encompasses various nanotechnology therapeutic applications (43). According to recent studies, significantly less nanomedicine has been used for treating neurodegenerative diseases compared to diseases like cancer and some infectious diseases (44). Although several studies have demonstrated that CNTs can efficiently permeate the BBB (45), the respective impermeability of the BBB is caused by tight connections created by endothelial cells of the brain’s capillaries. This causes some tiny and huge therapeutic molecules to not pass the BBB (46). The CNTs can penetrate the BBB through receptor-mediated pathways. CNTs have a massive surface area that allows loading and distributing high medication dosages to the therapeutic site. They also have inherent optical and thermal capabilities that could be used for photo-thermal and multimodal real-time tracking applications (45). The intrinsic barrier-crossing capacity of CNTs makes them ideal for use as nanocarriers for brain delivery, but because of unspecific bioaccumulation, this capacity is limited. As a result, choosing a particular brain target is crucial to enhance brain inflammation and minimizing side effects (45).
A study by Lohan et al. demonstrated that berberine (BRB) loaded MWCNTs with phospholipid and polysorbate coating effectively manage AD (47). In the SH-SY5Y cell lines, there was potential uptake of BRB-loaded MWCNT (47). Moreover, in rats, the enhanced performance was seen using the Morris Maze test. Also, the coating of phospholipid and polysorbate on MWCNT showed significant recovery in memory (47). The preservation of the usual biochemical amount in brain tissue established the ability of these coated MWCNTs to lessen β-amyloid-induced AD. The results show that polysorbate/phospholipid-coated MWCNTs of BRB have substantial potential for treating AD. A study by Mirali et al. showed that CNTs loaded with tacrine (Alzheimer’s drug) are safe for the specific delivery of drugs (36). The arrangement of Aβ (16-22) octamers was studied both in the presence and absence of SWCNT (48). It was observed that SWCNT could inhibit the Aβ (16-22) octamers and Aβ fibrillation (48). Furthermore, by correcting aberrant mTOR signaling activity and deficiencies in lysosomal proteolysis, SWNT restored healthy autophagy and facilitated the removal of autophagic substrates. These results suggest SWNT as a potential neuroprotective strategy for treating AD (49).
A holistic approach to managing brain tumors involves the innovative utilization of Carbon Nanotubes (CNTs) across various facets of diagnosis and treatment. A comprehensive strategy emerges by leveraging CNTs’ unique properties, such as their exceptional surface area and ability to traverse the blood-brain barrier. Functionalized CNTs can be tailored to target and image brain tumor cells, enabling early and precise detection through advanced imaging techniques. Figure 4 vividly illustrates the stark comparative distinction between conventional drugs and the remarkable efficacy of nanoparticles, carbon nanotubes (CNTs), and liposomes in traversing the formidable blood-brain barrier. While traditional drugs struggle to breach this barrier, the visual depiction showcases the extraordinary capability of nanoparticles, CNTs, and liposomes to overcome this challenge, opening new avenues for enhanced drug delivery to the brain.
Figure 4 Bridging the Barrier – Nanoparticles, CNTs, and Liposomes Showcase Effective Blood-Brain Barrier Penetration.
Various studies have highlighted the significant role of CNTs in managing brain tumors. Glioma cells that cause brain tumor produce immunosuppressive compounds such as transforming growth factor-β, prostaglandins E, and interleukin (IL)-10, which could make them capable of staying away from the host’s immune system. Because of this, it is difficult to treat these tumors with conventional chemotherapy properly. To address this problem, Van Handel and Colleagues proposed an innovative immunotherapy strategy utilizing the multiwalled carbon nanotubes (MWCNTs), leveraging that macrophages preferentially absorb CNTs over glioma cells. The administration of MWCNTs led to heightened macrophage infiltration into glioma cells, resulting in a time- and dose-dependent increase in tumor cytokine levels, particularly IL-10. Notably, this treatment did not induce notable toxicity in either healthy mice or mice with tumors. These findings indicate that employing CNTs for immune modulation could be a promising approach for brain tumor therapy.
Indeed, the results imply that harnessing the immune-modulating characteristics of CNTs holds great promise as a strategy for addressing brain tumors. By bolstering the immune response against glioma cells, this approach offers a potential avenue for a more effective therapy for brain tumors.
Fan et al. (50) conducted a study investigating the systemic antitumor response when CpG was delivered intracerebrally with CNTs. They confirmed that this intracranial CNT–CpG therapy not only inhibited the growth of brain tumors but also subcutaneous melanomas in a melanoma mouse model. Based on their results, they suggested that intracerebral CNT–CpG therapy could be a viable treatment for gliomas and metastatic brain tumors.
In a similar study, Zhao et al. (51) examined using CNTs to deliver CpG in brain tumor models. The researchers observed that CNTs enhanced the uptake of CpG by tumor-associated phagocytic cells, leading to their activation both in vitro and in vivo. When a low dose of CNT–CpG complexes was administered via a single injection, it eradicated intracranial GL261 gliomas in half of the tumor-bearing mice by activating NK and CD8 cells. These findings demonstrated that CNTs facilitated improved CpG uptake into tumor-associated inflammatory cells without causing toxicity and resulted in a robust antitumor response.
The key challenges with present drug administration methods include lack of selectivity among normal and diseased tissues, metabolic elimination, failure to pass the cellular and blood-brain barrier (BBB), and drug degradation before reaching the target organs, which can drastically limit the overall therapeutic effects. As the outcome, ongoing research is focusing more on developing a mechanism for delivering drugs that is highly effective, intending to create tailored medicines using a carrier or vehicle. Targeted drug delivery can be accomplished using several approaches and routes, Table 2. For instance, several vectors, including nanocomposites, quantum dots, emulsions, dendrimers, and polymers, are used. These approaches, however, have a few drawbacks. For example, since the polymers in a polymer hydrogel formulation containing erythropoietin (EPO) are not biodegradable, surgical removal is necessary once the medicine has been delivered. CNTs can be used to solve these problems instead of polymers. Additionally, since CNTs are biodegradable, there is no need for surgical removal following drug administration. Due to their excellent drug-loading capacity, extensive surface area, remarkable mechanical strength, and adequate chemical stability, CNTs can be ideal nanocarriers for drug delivery (57).
Numerous anti-cancerous CNT-based drug delivery schemes work by incorporating a particular drug or gene into the tips and walls of CNTs that find cancer-specific cell surface receptors. This allows CNTs to pass through the membrane of a mammalian cell through main endocytosis, carrying therapeutic drugs/genes more effectively and reliably into cells that were not previously accessible. Functionalized CNTs have been evaluated as a vehicle for drug delivery for a range of antineoplastic drugs, including topoisomerase inhibitors (irinotecan, topotecan, doxorubicin (DOX), and epirubicin), platinum-based compounds (cisplatin, 5-fluorouracil, carboplatin), and anti-microtubule drugs (paclitaxel, docetaxel). Interestingly, Maleki et al. also evaluated N-isopropyl acrylamide carbon nanotube as a drug delivery structure through molecular dynamics simulation study using DOX loading (58). The interactions between the medicines and the carrier, hydrogen bonds, the gyration radius, and the radial distribution function are key notable elements for examining this carrier. Furthermore, by analyzing the DOX simulation studies, N-isopropyl acrylamide and carbon nanotubes can be used for drug delivery.
CNTs are also being researched as potential carriers for other diseases, including HIV, diabetes, and brain disorders (9, 59–61). Additionally, Yoosefian et al. conducted a molecular analysis study of the drug delivery system for an anti-Perkinson drug, Droxidopa, loaded with functionalized SWCNTs. The result of this simulation study showed improved bioavailability with reduced systemic toxicity (55).
Carbon nanotubes can function as an efficient vector system for the production and delivery of the therapeutic gene in the target tissues because nucleic acids and carbon nanotubes interact electrostatically to generate a variety of chemical complexes. This makes a variety of procedures involving gene therapy, vaccinations, and immuno-stimulating activities possible. (62). The delivery capability of CNTs is demonstrated by Cationic Polymer Brush-Modified Carbon Nanotubes for effective siRNA suppression of PD-L1 (Programmed death-ligand 1), enhanced biocompatibility, and cellular uptake in cancer immunotherapy (63). SWNTs demonstrate improved biocompatibility and stability as a delivery system. Chen et al. created SWNTs with amylose derivatives containing poly(L-lysine) dendrons and TNFα (ADP@SWNT/TNFα). Invitro and in-vivo studies were done on the complex’s aqueous dissipation stability, cytotoxicity, gene transfection potency, and photothermal effect. The findings suggested that ADP@SWNT/TNFα had a high potential for use in tumor therapy by being able to inhibit tumor growth and metastasis in both studies (64).
The carbon nanotube’s adjuvant property might be able to resolve the main obstacles associated with vaccine delivery. The fundamental idea behind using CNTs in vaccine delivery is to attach the antigen to them while maintaining its conformation, which will cause an antibody response with the appropriate specificity (65). The CNTs can activate several immune response-related genes, including NF- κB, ILs, and TNFα. This activation can lead to both acute and chronic immune responses. Acute inflammation occurs rapidly after exposure, while chronic activation can result from prolonged or repeated exposure. Chronic immune activation can contribute to sustained inflammation, oxidative stress, and neurodegenerative processes. Reactive oxygen species (ROS) and oxidative stress may be produced due to CNT-induced immune activation. The study by Mostovenkoet al. reveals that exposure to carbon nanomaterials can cause neurological changes reflected in the peptidomics profile of the cerebral spinal fluid (CSF). These changes resemble early-stage neurodegenerative disease, emphasizing the need to monitor CSF peptidomics markers for assessing neurotoxicity and identifying potential risks associated with carbon nanomaterial exposure. The duration, dose, and frequency of exposure determine the extent of immune activation and its long-term effects on the brain (66, 67). Further research is needed to understand the underlying mechanisms associated with this fully.
This inspired clinical research on CNT-based delivery systems to improve the body’s immune response to challenging diseases like cancer (68). Luna Labs recently created the “CNTVac” delivery platform, which uses short carbon nanotubes. Carbon nanotube size is carefully regulated to have an HIV-1 particle-like morphology and be effective at intranasal delivery of a wide variety of antigens. It has increased local IgA and systemic antibody IgG responses in mice and rabbits. In addition to acting as an effective delivery system, CNTVac lowers the number of lipids necessary for vaccine dosing, thereby removing potential side effects. The information points to a possible platform technology for the delivery of vaccines (69).
The utilization of carbon nanotubes in tissue engineering is yet another significant application. The primary goal of tissue engineering is to switch out unhealthy or eroded tissue with biological alternatives that can be repaired and maintain normal function. Whereas the study of self-healing, in which the body employs its systems occasionally with extra assistance from external biological material to regenerate cells and repair tissues and organs, is a component of regenerative medicine, which also encompasses tissue engineering (31). The 3D scaffold is applied in tissue engineering to keep the tissues’ mechanical integrity, flexibility, and cell-specific biochemical environment. CNT-based 3D scaffolds are better compared for use in composite scaffolds due to their superior mechanical attributes, which include high tensile strength and elastic moduli. In neurology, osteology, and cardiology fields, using CNTs as scaffolds for neural system rejuvenation is a promising advancement.
Researchers have demonstrated that MWCNTs can oxidize and aggregate enzymes like rhBMP-2 (Recombinant bone morphogenetic protein 2), promoting the stimulation of alkaline phosphatase and the genomes Cbfa1(Core-binding factor subunit alpha-1) and COLIA1 (Collagen type 1), which supports osteogenic discrepancy in cultured mesenchymal stem cells distinct from human adipose. MWCNTs can also control subsequent gene therapy reactions without adding exogenous signaling molecules or any other particular ligands, as evidenced by the frequent stimulation of in-vivo ectopic bone repair in mouse dorsal muscles. Therefore, the cultivation of renewable bone tissue is also made possible by this CNT material (70). Nanofiber scaffolds based on chitosan, polyvinyl alcohol, and carbon nanotube seem to be a favorable approach in cardiovascular tissue engineering (71).
A recent study by Li et al. raises the possibility of a new method for repairing transected peripheral nerves that combines electrical stimulation with an electric conductive CNT/sericin conduit. This CNT/sericin conduit has advantageous qualities like bio-compatibility, bio-degradability, porous microarchitecture, and appropriate swelling properties (72). According to a study by Xia et al., multivalent polyanion-dispersed CNTs can be used to create nano-structured fibrous scaffolds that can control the future of induced pluripotent stem cells (IPS). These hPGS (hyperbranched polyglycerol sulfate) CNTs have a distinct 1D morphology, are stable in both physiological medium and water, and have good biocompatibility compared to commercially available dispersed SDS (sodium dodecyl sulfonate) and PSS (poly sodium-styrene sulfonate). It was discovered that the suggested technique could act as a biocompatible program for encouraging IPS cells’ adhesion and growth. Additionally, it opens up a new method for creating composites with functionalized carbon nanomaterials to regenerate musculoskeletal and cardiovascular tissue (73).
In a recent article, functionalized single-walled carbon nanotubes (f-SWCNTs) were used to reinforce high molecular weight polyethylene (UHMWPE) material used to make unicompartmental knee implants. The surface biocompatibility of the prepared nanocomposite samples tested with human osteoblast cells leads to an improvement in cell viability with great cell differentiation and growth and affirms the effectiveness of the produced nanocomposite matter in the formation of artificial hip and knee inserts (74). The dispersibility of the CNTs was enhanced by using functionalized MWCNT (f-MWCNT). In the presence of human osteoblast cells, the manufacturing of silver-exchanged hydroxyapatite/functionalized multiwall carbon nanotube (Ag-HA/f-MWCNT) implants significantly inhibits proliferation and induces significant apoptosis (75).
Neuroprotection is the protection of neurons’ structure and functionality. This method is frequently selected for patients with CNS disorders such as stroke, brain injuries, and neurodegenerative diseases, including AD, to end or slow the loss of neurons. Despite varied signs and symptoms, the mechanisms causing neurodegeneration are the same (76). Using neuroprotective medications can shield neurons from neurodegeneration by diminishing neuro-inflammation and apoptotic pathways (77).
Nanotechnology has several benefits in managing CNS disorders; in the future, patients and doctors may have access to more therapeutic alternatives. It is crucial to connect the development of synthetic and characterization approaches in chemistry and materials science with the advancement of nano-engineered applications in the nervous system (78). Although there are many published studies and proven conclusions, there are few treatments for neurodegenerative diseases, and more research is required to understand the mechanisms underlying neurological disorders. Regrowth of axons and rearrangement of the neural circuitry are the two critical strategies for encouraging the self-repair of broken axonal connections in neurons. Thus, for successful regenerative engineering (neuroplasticity) as a therapeutic approach for managing AD, the preservation of neurons (neurorestorative), promotion of an environment favorable for the regrowth of the degenerated neurons, also known as neurogenesis, and reconnection of neuronal circuits, followed by advancement of the plasticity of neuronal tissue, are required (79). According to Lovat et al., using a pure MWNT substrate improved hippocampus neuron cell adhesion and dendritic elongation. The signal processing of neurons is better when the dendritic extension is increased (80). Similarly, Mazzatenta et al. demonstrated that pure SWNTs encourage the development of neural circuits and the growth of hippocampus neurons. The neural circuit expands noticeably as electrical signal transmission within the neuronal network increases ((81) Jan et al. evaluated the compatibility of CNT substrates with neural stem cells based on cell survival and the development of neuronal processes equivalent to those seen with the frequently used growth substrate poly-L-ornithine (NSCs) (82). The successful transport of NSCs to CNS injury sites and their differentiation into neurons served as evidence of the effectiveness of CNTs (82).
Numerous groups of researchers have investigated CNTs as sensing elements for biosensors because of their robust electrical or optical properties, which are highly sensitive to be changed by exposure to biomolecules. The increased surface/volume ratio of CNTs allows the ultra-rapid detection of biological components even at lower concentrations. CNT-based biosensors possess better sensitivity, faster response times, higher stability, greater lifespans, and improved shelf life compared to most metal oxide or silicon-based commercially available sensors. Hence, CNT-based biosensors are acknowledged as a next-gen aspect of ultrasensitive biosensing systems (83).
Biosensors for glucose are essential. They monitor glucose levels, making them an excellent product for people with diabetes. Protein sensors, immuno-sensors, nucleic-acid sensors, and infection sensors are critical attributes of nanotube biosensors (84–87). Azimiet al. proposed a new technique for modifying straight lined-up carbon nanotube arrays (VACNTs) for targeted glucose detection. The constructed electrode worked well as a point-of-care (POC) biosensor to identify glucose in human blood plasma with a detection limit of 1.1 μM (88). Amidated MWCNTs coupled with gold nanocage decorated SPCE (screen-printed carbon electrode) were capable of ultrasensitive detection of MALAT1 (Metastasis Associated Lung Adenocarcinoma Transcript 1) biomarkers in non-small cell lung cancer. The formed biosensor depicted a broad linear range and low detection limit (42.8 fM) with sufficient selectivity and stability (89). A paper-based peptide-encapsulated SWCNTs dipstick optical biosensor has been advanced for protease observation. The feasibility of the sensor was demonstrated by detecting trypsin activity in a urine sample of a pancreatitis patient (90).
An electrochemical biosensor was created on a carbon nanotube field-effect transistor (CNT-FET) to detect SARS-CoV-2 S1 in saliva samples. The biosensor was generated using CNT printing on a Si/SiO2 surface and immobilized anti-SARS-CoV-2 S1. The presented biosensor achieves a diagnosis range of 0.1 fg mL-1 - 5.0 pg mL-1 and a detection limit of 4.12 fg mL-1 in 2-3 minutes. And hence, it can serve as an example for detecting SARS-CoV-2 S1 antigen (91).
Utilizing CNTs allows for the enhancement of imaging features and functionality. Carbon nanotubes are very desirable in the area of biomedical imaging due to their optical, mechanical, physiochemical, and versatile photophysical properties. In nuclear imaging, Raman scattering, magnetic resonance, photoacoustic, optical detection, and fluorescent video imaging, CNTs can be exploited as imaging contrast agents (92). Semiconductive SWCNTs make remarkable fluorescent bioimaging sensors due to their near-infrared (NIR) fluorescence emission. A Fluorescence near-infrared (NIR) nanosensor based on SWCNTs was developed to visualize the release of the neurotransmitter serotonin from blood platelets in real-time. Such nano-sensors have great potential for defining how cells modulate chemical signals in space and time to convey information (93). Another real-time imaging strategy for tumor imaging has been proposed in which bioengineered small mussel adhesive proteins (MAPs) CNTs were used as tumor-targeted carriers. Brief CNT hybrid near-infrared fluorophores (NIRF) were deposited in the tumor cells with excellent targeting and clearance (94). Stronger NIR emission of SWCNTs in contrast with E11 release by radical polymer grafting on the SWCNT surface can be achieved by radical polymerization utilizing surfactant-dispersed SWCNTs (95).
Saghatchi et al. reported that the multiwalled carbon nanotubes equipped with magnetic Fe3O4 and gold NPs (mf-MWCNT/AuNPs) are favorable in comparison with factors in imaging by ultrasounds, CT scans, and MRI due to the beneficial qualities in radio-, thermo-, and imaging therapies. Moreover, mf-MWCNT/AuNPs is a strong candidate for use as a multi-modal instrument in cancer therapy (96).
Due to their carcinogenicity and other harmful effects, nano-biomaterials may have adverse effects if they break down in the body. Nanoparticles that are not biodegradable can build up in tissues and be detrimental to people’s health. Studies on toxicity have been carried out to learn more about how to regulate the effects of CNT-based drugs on degradation (97). The degree of aggregation has also been shown to be one of the major factors affecting SWNT cytotoxicity. It has been demonstrated that human mesothelial cells are less damaging to CNT bundles than to CNT agglomerates. When dispersing nanotubes, cytotoxicity can be decreased by adding biocompatible surfactants such as poly-oxyethylene sorbitan mono-oleate 80 (PS80) (98).
(CNTs interact with different organs and bodily fluids. According to several experimental findings, CNTs are harmful to varying degrees in different tissues. Cells have been observed to experience oxidative stress when exposed to CNTs. Numerous studies have shown that the spleen, kidneys, and lungs are among the organs that are most susceptible to the oxidative stress brought on by the generation of free radicals. ROS are often produced as by-products of the metabolism of oxygen. Because CNTs induce oxidative stress within the cell, the quantity of ROS also rises. However, research into how they affect various organs is required before using them for medicinal purposes (99) Figure 5.
Figure 5 The figure represents the cytotoxic effects of CNTs on different body organs and immune systems. It depicts that penetration of carbon nanotubes leads to oxidative stress, increasing ROS (reactive oxygen species) levels in organs. The organs, such as the spleen, kidneys, and lungs, are most susceptible to the oxidative stress brought on by the generation of free radicals. Moreover, an inflammatory reaction is also observed towards the CNTs due to their immunogenicity, as these are considered foreign material, thus, eliciting a foreign body response (FBR) (99).
Numerous investigations into the biocompatibility and carcinogenicity of CNTs have been made. The results have been quite inconsistent; while some have claimed that the substance has great biocompatibility, others have claimed that it is carcinogenic (100). While more research has revealed that CNTs are potentially dangerous substances that may have adverse acute and long-term effects on a variety of living systems, some preliminary studies have revealed that CNTs are physiologically benign to particular cells, tissues, and organs like kidneys, stomach, bones, lymph nodes and lungs. In bone, CNTs may interfere in bone repair and new bone formation (101, 102). Whereas it seems that the biological effects of CNTs are sample-dependent and must be assessed individually. Therefore, more study is required to determine the nanotoxicity of CNTs.
● Lack of solubility in the majority of biologically compatible (aqueous-based) solvents.
● The creation of batch CNTs with the same chemically and structurally repeatable properties.
● Maintaining high quality and minimizing contaminants is challenging (103).
CNTs have a relatively low specific surface area and density and are often challenging to manufacture into thick electrodes using standard electrode preparation procedures, in contrast to advanced activated carbons(Acs), Carbide-derived carbons(CDCs) and zero -templated carbons (ZTCs) (104).
The potential applications of carbon nanotubes (CNTs) in the field of medicine and biotechnology are vast and promising. CNTs have already proven to be effective gene delivery and drug carriers for a wide range of medical purposes, including cancer treatment and anti-inflammatory therapies. The ability to transport various anticancer agents and therapeutic substances such as docetaxel (DTX), doxorubicin (DOX), methotrexate (MTX), paclitaxel (PTX), gemcitabine (GEM), and osteogenic dexamethasone (DEX) steroids via CNTs showcases their versatility.
Moreover, CNTs possess unique optical properties that make them valuable in phototherapy applications. Their simple surface functionalization has enabled their use as gene delivery vectors for addressing various diseases, including cancer, by transferring genes like plasmid DNA (PDNA), micro-RNA (miRNA), and small interfering RNA (siRNA). These advancements hold great promise for personalized medicine and targeted therapies.
However, it's important to acknowledge that concerns regarding CNT nanotoxicology and environmental impact remain. Since CNTs are not biodegradable, their long-term effects on the environment and human health need thorough investigation. Furthermore, despite decades of research and numerous in vivo and in vitro studies, widespread use of CNTs in medical applications awaits regulatory approval from agencies like the FDA (105).
Carbon nanotubes (CNTs) hold immense promise as versatile tools in the fields of medicine and biotechnology. Their ability to efficiently deliver genes and therapeutic agents to a wide range of cell types, including cancer cells, has the potential to revolutionize drug delivery and personalized medicine. Additionally, CNTs' unique optical and physiochemical properties make them valuable assets in imaging techniques and biosensors, enhancing their diagnostic capabilities.
DE: Writing – original draft. GR: Supervision, Writing – original draft, Writing – review & editing. NK: Writing – original draft. PS: Writing – original draft. RJ: Writing – original draft. FK: Writing – original draft. BS: Writing – original draft. MK: Writing – review & editing. AB: Writing – review & editing. SF: Writing – review & editing.
The author(s) declare that no financial support was received for the research, authorship, and/or publication of this article.
The authors declare that the research was conducted in the absence of any commercial or financial relationships that could be construed as a potential conflict of interest.
All claims expressed in this article are solely those of the authors and do not necessarily represent those of their affiliated organizations, or those of the publisher, the editors and the reviewers. Any product that may be evaluated in this article, or claim that may be made by its manufacturer, is not guaranteed or endorsed by the publisher.
1. Holtzman DM, Morris JC, Goate AM. Alzheimer’s disease: the challenge of the second century. Sci Trans Med (2011) 3(77):77sr1–1.
3. Graham WV, Bonito-Oliva A, Sakmar TP. Update on alzheimer’s disease therapy and prevention strategies. Annu Rev Med (2017) 68:413–30. doi: 10.1146/ANNUREV-MED-042915-103753
4. Taillibert S, Laigle-Donadey F, Sanson M. Palliative care in patients with primary brain tumors. Curr Opin Oncol (2004) 16(6):587–92. doi: 10.1097/01.CCO.0000142075.75591.02
5. Picone P. Nanobiotechnology: A new frontier for brain disorders. Int J Mol Sci (2022) 23(17). doi: 10.3390/IJMS23179603
6. Sinha N, Yeow JTW. Carbon nanotubes for biomedical applications. IEEE Trans Nanobioscience (2005) 4(2):180–95. doi: 10.1109/TNB.2005.850478
7. Saraiva C, Praça C, Ferreira R, Santos T, Ferreira L, Bernardino L. Nanoparticle-mediated brain drug delivery: Overcoming blood-brain barrier to treat neurodegenerative diseases. J Controlled Release: Off J Controlled Release Soc (2016) 235:34–47. doi: 10.1016/J.JCONREL.2016.05.044
8. Katz E, Willner I. Biomolecule-functionalized carbon nanotubes: applications in nanobioelectronics. Chemphyschem: A Eur J Chem Phys Phys Chem (2004) 5(8):1084–104. doi: 10.1002/CPHC.200400193
9. Guo Q, Shen X, Li Y, Xu S. Carbon nanotubes-based drug delivery to cancer and brain. Curr Med Sci (2017) 37:635–41. doi: 10.1007/s11596-017-1783-z
10. Debnath SK, Srivastava R. Drug delivery with carbon-based nanomaterials as versatile nanocarriers: progress and prospects. Front Nanotechnol (2021) 3:644564. doi: 10.3389/fnano.2021.644564
11. Negri V, Pacheco-Torres J, Calle D, López-Larrubia P. Carbon nanotubes in biomedicine. Top Curr Chem (2020) 378:15. doi: 10.1007/s41061-019-0278-8
12. Son KH, Hong JH, Lee JW. Carbon nanotubes as cancer therapeutic carriers and mediators. Int J Nanomed (2020) 11:5163–85. doi: 10.2147/IJN.S112660
13. Ilbasmiş-Tamer S, Yilmaz Ş, Banoğlu E, Değim IT. Carbon nanotubes to deliver drug molecules. J BioMed Nanotechnol (2010) 6:20–7. doi: 10.1166/jbn.2010.1099
14. Jin GZ, Chakraborty A, Lee JH, Knowles JC, Kim HW. Targeting with nanoparticles for the therapeutic treatment of brain diseases. J. Tissue Eng (2020), 11. doi: 10.1177/2041731419897460
15. Wang C, Zhang M, Mao X, Yu Y, Wang CX, Yang YL. Nanomaterials for reducing amyloid cytotoxicity. Adv Mater (2013) 25(28):3780–801. doi: 10.1002/ADMA.201301210
16. Yingchoncharoen P, Kalinowski DS, Richardson DR. Lipid-based drug delivery systems in cancer therapy: What is available and what is yet to come. Pharmacol Rev (2016) 68(3):701–87. doi: 10.1124/PR.115.012070
17. Zielinska A, Carreiró F, Oliveira AM, Neves A, Pires B, Nagasamy Venkatesh D, et al. Polymeric nanoparticles: Production, characterization, toxicology and ecotoxicology. Molecules (2020) 25(16). doi: 10.3390/MOLECULES25163731
18. Choi J, Rui Y, Kim J, Gorelick N, Wilson DR, Kozielski K, et al. Nonviral polymeric nanoparticles for gene therapy in pediatric CNS malignancies. Nanomedicine: Nanotechnology Biology Med (2020), 23. doi: 10.1016/J.NANO.2019.102115
19. Anderson D, Anderson T, Fahmi F. Advances in applications of metal oxide nanomaterials as imaging contrast agents. Physica Status Solidi (a) (2019) 216(16):1801008. doi: 10.1002/PSSA.201801008
20. Geng J, Li M, Wu L, Chen C, Qu X. Mesoporous silica nanoparticle-based H2O2 responsive controlled-release system used for alzheimer’s disease treatment. Advanced Healthcare Materials (2012) 1(3):332–6. doi: 10.1002/ADHM.201200067
21. Jiao Y, Shen S, Sun Y, Jiang X, Yang W. A functionalized hollow mesoporous silica nanoparticles-based controlled dual-drug delivery system for improved tumor cell cytotoxicity. Part. Part. Syst. Charact. 32(2):222–33. doi: 10.1002/PPSC.201400115
22. Gillies ER, Fréchet JMJ. Dendrimers and dendritic polymers in drug delivery. Drug Discovery Today (2005) 10(1):35–43. doi: 10.1016/S1359-6446(04)03276-3
23. Gorain B, Choudhury H, Pandey M, Amin MCIM, Singh B, Gupta U, et al. Dendrimers as effective carriers for the treatment of brain tumor. Nanotechnology-Based Targeted Drug Delivery Syst. Brain Tumors (2018), 267–305. doi: 10.1016/B978-0-12-812218-1.00010-5
24. Mishra J, Kumar B, Pandey M, Pottoo FH, Fayaz F, Khan FA, et al. Carbon nano tubes: Novel drug delivery system in amelioration of alzheimer’s disease. Combinatorial Chem. High Throughput Screening (2021) 24(10):1528–43. doi: 10.2174/1386207323999200918112538
25. Kesharwani P, Gajbhiye V, Jain NK. A review of nanocarriers for the delivery of small interfering RNA. Biomaterials (2012) 33(29):7138–50. doi: 10.1016/J.BIOMATERIALS.2012.06.068
26. Mohapatra S, Jain S, Shukla K, J Abdul AP, Yahya Elshimali JI. Formulation and evaluationation of anti-alzheimer drug MEM HCL nanogel. J. Pharm. Res. Int. (2021) 33(41A):323–29. doi: 10.9734/JPRI/2021/V33I41A32332
27. Wong KE, Ngai SC, Chan KG, Lee LH, Goh BH, Chuah LH. Curcumin nanoformulations for colorectal cancer: A review. Front. Pharmacol (2019), 10. doi: 10.3389/FPHAR.2019.00152
28. Zhang C, Zheng X, Wan X, Shao X, Liu Q, Zhang Z, et al. The potential use of H102 peptide-loaded dual-functional nanoparticles in the treatment of Alzheimer’s disease. J Controlled Release (2014) 192:317–24. doi: 10.1016/j.jconrel.2014.07.050
29. Alibolandi M, Charbgoo F, Taghdisi SM, Abnous K, Ramezani M. Active targeted nanoscale delivery systems for brain tumor therapeutics. Nanotechnology-Based Targeted Drug Delivery Syst. Brain Tumors (2018), 75–110. doi: 10.1016/B978-0-12-812218-1.00004-X
30. Simon J, Flahaut E, Golzio M. Overview of carbon nanotubes for biomedical applications. Materials (2019) 12:624. doi: 10.3390/ma12040624
31. Eatemadi A, Daraee H, Karimkhanloo H, Kouhi M, Zarghami N, Akbarzadeh A, et al. Carbon nanotubes: properties, synthesis, purification, and medical applications. Nanoscale Res Lett (2014) 9:393. doi: 10.1186/1556-276X-9-393
32. Pan X, Bao X. The effects of confinement inside carbon nanotubes on catalysis. Acc Chem Res (2011) 44:553–62. doi: 10.1021/ar100160t
33. Sinnott SB, Andrews R, Qian D, Rao AM, Mao Z, Dickey EC, et al. Model of carbon nanotube growth through chemical vapor deposition. Chem Phys Lett (1999) 315:25–30. doi: 10.1016/S0009-2614(99)01216-6
34. Sajid MI, Jamshaid U, Jamshaid T, Zafar N, Fessi H, Elaissari A. Carbon nanotubes from synthesis to in vivo biomedical applications. Int J Pharm (2016) 501:278–99. doi: 10.1016/j.ijpharm.2016.01.064
35. Shi Z, Lian Y, Zhou X, Gu Z, Zhang Y, Iijima S, et al. Mass-production of single-wall carbon nanotubes by arc discharge method11This work was supported by the National Natural Science Foundation of China, No. 29671030. Carbon N Y (1999) 37:1449–53. doi: 10.1016/S0008-6223(99)00007-X
36. Mirali M, Jafariazar Z, Mirzaei M. Loading tacrine alzheimer’s drug at the carbon nanotube: DFT approach. Lab-in-Silico (2021) 2:3–8. doi: 10.22034/labinsilico21021003
37. Thess A, Lee R, Nikolaev P, Dai H, Petit P, Robert J, et al. Crystalline ropes of metallic carbon nanotubes. Sci (1979) (1996) 273:483–7. doi: 10.1126/science.273.5274.483
38. Cao Q, Rogers JA. Ultrathin films of single-walled carbon nanotubes for electronics and sensors: A review of fundamental and applied aspects. Adv Mater (2009) 21:29–53. doi: 10.1002/adma.200801995
39. Moseenkov SI, Kuznetsov VL, Kolesov BA, Zavorin AV, Serkova AN, Zolotarev NA. (2021). Design of effective surface contacts on polymer composites modified with multiwalled carbon nanotubes. Express Polym Lett 15(9):826-38. doi: 10.3144/expresspolymlett.2021.66
40. Verma B, Balomajumder C. Surface modification of one-dimensional Carbon Nanotubes: A review for the management of heavy metals in wastewater. Environ TechnolInnov (2020) 17:100596. doi: 10.1016/j.eti.2019.100596
41. Shoukat R, Khan MI. Carbon nanotubes: a review on properties, synthesis methods and applications in micro and nanotechnology. Microsystem Technol (2021) 27:4183–92. doi: 10.1007/s00542-021-05211-6
42. Cui K, Chang J, Feo L, Chow CL, Lau D. Developments and applications of carbon nanotube reinforced cement-based composites as functional building materials. Front Mater (2022) 9:861646. doi: 10.3389/fmats.2022.861646
43. Sharma HS, Muresanu DF, Sharma A. Novel therapeutic strategies using nanodrug delivery, stem cells and combination therapy for CNS trauma and neurodegenerative disorders. Expert Rev Neurother (2013) 13:1085–8. doi: 10.1586/14737175.2013.836297
44. Kanwar JR, Sun X, Punj V, Sriramoju B, Mohan RR, Zhou S-F, et al. Nanoparticles in the treatment and diagnosis of neurological disorders: untamed dragon with fire power to heal. Nanomedicine (2012) 8:399–414. doi: 10.1016/j.nano.2011.08.006
45. Costa PM, Bourgognon M, Wang JT-W, Al-Jamal KT. Functionalised carbon nanotubes: From intracellular uptake and cell-related toxicity to systemic brain delivery. J Controlled Release (2016) 241:200–19. doi: 10.1016/j.jconrel.2016.09.033
46. Han H, Mann A, Ekstein D, Eyal S. Breaking bad: the structure and function of the blood-brain barrier in epilepsy. AAPS J (2017) 19:973–88. doi: 10.1208/s12248-017-0096-2
47. Lohan S, Raza K, Mehta SK, Bhatti GK, Saini S, Singh B. Anti-Alzheimer’s potential of berberine using surface decorated multiwalled carbon nanotubes: A preclinical evidence. Int J Pharm (2017) 530:263–78. doi: 10.1016/j.ijpharm.2017.07.080
48. Li H, Luo Y, Derreumaux P, Wei G. Carbon nanotube inhibits the formation of β-sheet-rich oligomers of the alzheimer’s amyloid-β(16-22) peptide. Biophys J (2011) 101:2267–76. doi: 10.1016/j.bpj.2011.09.046
49. Xue X, Wang L-R, Sato Y, Jiang Y, Berg M, Yang D-S, et al. Single-walled carbon nanotubes alleviate autophagic/lysosomal defects in primary glia from a mouse model of alzheimer’s disease. Nano Lett (2014) 14:5110–7. doi: 10.1021/nl501839q
50. Fan H, Zhang I, Chen X, Zhang L, Wang H, Da Fonseca A, et al. Intracerebral CpG immunotherapy with carbon nanotubes abrogates growth of subcutaneous melanomas in mice. Clin Cancer Res (2012) 18(20):5628–38. doi: 10.1158/1078-0432.CCR-12-1911
51. Zhao D, Alizadeh D, Zhang L, Liu W, Farrukh O, Manuel E, et al. Carbon nanotubes enhance CpG uptake and potentiate antiglioma immunity. Clin Cancer Res (2011) 17(4):771–82. doi: 10.1158/1078-0432.CCR-10-2444
52. Tan JM, Foo JB, Fakurazi S, Hussein MZ. Release behaviour and toxicity evaluation of levodopa from carboxylated single-walled carbon nanotubes. Beilstein J Nanotechnol (2015) 6:243–53. doi: 10.3762/bjnano.6.23
53. Zhu S, Huang A-G, Luo F, Li J, Li J, Zhu L, et al. Application of virus targeting nanocarrier drug delivery system in virus-induced central nervous system disease treatment. ACS Appl Materials Interfaces (2019) 11(21):19006–16. doi: 10.1021/acsami.9b06365
54. Yoosefian M, Rahmanifar E, Etminan N. Nanocarrier for levodopa Parkinson therapeutic drug; comprehensive benserazide analysis. Artif Cells Nanomedicine Biotechnol (2018) 46(sup1):434–46. doi: 10.1080/21691401.2018.1430583
55. Yoosefian M, Jahani M. A molecular study on drug delivery system based on carbon nanotube for the novel norepinephrine prodrug, Droxidopa. J Mol Liq (2019) 284:258–64. doi: 10.1016/j.molliq.2019.04.016
56. Ren J, Shen S, Wang D, Xi Z, Guo L, Pang Z, et al. The targeted delivery of anticancer drugs to brain glioma by PEGylated oxidized multiwalled carbon nanotubes modified with angiopep-2. Biomaterials (2012) 33(11):3324–33. doi: 10.1016/j.biomaterials.2012.01.025
57. Murjani BO, Kadu PS, Bansod M, Vaidya SS, Yadav MD. Carbon nanotubes in biomedical applications: current status, promises, and challenges. Carbon Lett (2022) 32:1207–26. doi: 10.1007/s42823-022-00364-4
58. Maleki R, Afrouzi HH, Hosseini M, Toghraie D, Rostami S. Molecular dynamics simulation of Doxorubicin loading with N-isopropyl acrylamide carbon nanotube in a drug delivery system. Comput Methods Programs BioMed (2020) 184:105303. doi: 10.1016/j.cmpb.2019.105303
59. Bilal M, Barani M, Sabir F, Rahdar A, Kyzas GZ. Nanomaterials for the treatment and diagnosis of Alzheimer’s disease: An overview. NanoImpact (2020) 20:100251. doi: 10.1016/j.impact.2020.100251
60. Khalid A, Madni A, Raza B, Islam M, Hassan A, Ahmad F, et al. Multiwalled carbon nanotubes functionalized bacterial cellulose as an efficient healing material for diabetic wounds. Int J BiolMacromol (2022) 203:256–67. doi: 10.1016/j.ijbiomac.2022.01.146
61. Panda M, Purohit P, Wang Y, Meher BR. Functionalized carbon nanotubes as an alternative to traditional anti-HIV-1 protease inhibitors: An understanding towards Nano-medicine development through MD simulations. J Mol Graph Model (2022) 117:108280. doi: 10.1016/j.jmgm.2022.108280
62. Singh R, Pantarotto D, McCarthy D, Chaloin O, Hoebeke J, Partidos CD, et al. Binding and condensation of plasmid DNA onto functionalized carbon nanotubes: toward the construction of nanotube-based gene delivery vectors. J Am Chem Soc (2005) 127:4388–96. doi: 10.1021/ja0441561
63. Li D, Ahmed M, Khan A, Xu L, Walters AA, Ballesteros B, et al. Tailoring the architecture of cationic polymer brush-modified carbon nanotubes for efficient siRNA delivery in cancer immunotherapy. ACS Appl Mater Interfaces (2021) 13:30284–94. doi: 10.1021/acsami.1c02627
64. Chen Z, Zhuang J, Pang J, Liu Z, Zhang P, Deng H, et al. Application of a cationic amylose derivative loaded with single-walled carbon nanotubes for gene delivery therapy and photothermal therapy of colorectal cancer. J BioMed Mater Res A (2022) 110:1052–61. doi: 10.1002/jbm.a.37351
65. Raphey VR, Henna TK, Nivitha KP, Mufeedha P, Sabu C, Pramod K. Advanced biomedical applications of carbon nanotube. Materials Sci Engineering: C (2019) 100:616–30. doi: 10.1016/j.msec.2019.03.043
66. Dong J. Signaling pathways implicated in carbon nanotube-induced lung inflammation. Front Immunol (2020) 11:552613. doi: 10.3389/fimmu.2020.552613
67. Mostovenko E, Saunders S, Muldoon PP, Bishop L, Campen MJ, Erdely A, et al. Carbon nanotube exposure triggers a cerebral peptidomic response: barrier compromise, neuroinflammation, and a hyperexcited state. Toxicological Sci (2021) 182:107–19. doi: 10.1093/toxsci/kfab042
68. Hassan HAFM, Diebold SS, Smyth LA, Walters AA, Lombardi G, Al-Jamal KT. Application of carbon nanotubes in cancer vaccines: Achievements, challenges and chances. J Controlled Release (2019) 297:79–90. doi: 10.1016/j.jconrel.2019.01.017
69. Xu Y, Jiang X, Zhou Z, Ferguson T, Obliosca J, Luo CC, et al. Mucosal delivery of HIV-1 glycoprotein vaccine candidate enabled by short carbon nanotubes. Particle Particle Syst Characterization (2022) 39:2200011. doi: 10.1002/ppsc.202200011
70. Li X, Liu H, Niu X, Yu B, Fan Y, Feng Q, et al. The use of carbon nanotubes to induce osteogenic differentiation of human adipose-derived MSCs in vitro and ectopic bone formation in vivo. Biomaterials (2012) 33:4818–27. doi: 10.1016/j.biomaterials.2012.03.045
71. Mombini S, Mohammadnejad J, Bakhshandeh B, Narmani A, Nourmohammadi J, Vahdat S, et al. Chitosan-PVA-CNT nanofibers as electrically conductive scaffolds for cardiovascular tissue engineering. Int J BiolMacromol (2019) 140:278–87. doi: 10.1016/j.ijbiomac.2019.08.046
72. Li X, Yang W, Xie H, Wang J, Zhang L, Wang Z, et al. CNT/sericin conductive nerve guidance conduit promotes functional recovery of transected peripheral nerve injury in a rat model. ACS Appl Mater Interfaces (2020) 12:36860–72. doi: 10.1021/acsami.0c08457
73. Xia Y, Li S, Nie C, Zhang J, Zhou S, Yang H, et al. A multivalent polyanion-dispersed carbon nanotube toward highly bioactive nanostructured fibrous stem cell scaffolds. Appl Mater Today (2019) 16:518–28. doi: 10.1016/j.apmt.2019.07.006
74. Diabb Zavala JM, Leija Gutiérrez HM, Segura-Cárdenas E, Mamidi N, Morales-Avalos R, Villela-Castrejón J, et al. Manufacture and mechanical properties of knee implants using SWCNTs/UHMWPE composites. J Mech Behav BioMed Mater (2021) 120:104554. doi: 10.1016/j.jmbbm.2021.104554
75. Sivaraj D, Vijayalakshmi K. Enhanced antibacterial and corrosion resistance properties of Ag substituted hydroxyapatite/functionalized multiwall carbon nanotube nanocomposite coating on 316L stainless steel for biomedical application. UltrasonSonochem (2019) 59:104730. doi: 10.1016/j.ultsonch.2019.104730
76. Seidl SE, Potashkin JA. The promise of neuroprotective agents in parkinson?s disease. Front Neurol (2011) 2:68. doi: 10.3389/fneur.2011.00068
77. Hajialyani M, HoseinFarzaei M, Echeverría J, Nabavi S, Uriarte E, Sobarzo-Sánchez E. Hesperidin as a neuroprotective agent: A review of animal and clinical evidence. Molecules (2019) 24:648. doi: 10.3390/molecules24030648
78. Lockman PR, Mumper RJ, Khan MA, Allen DD. Nanoparticle technology for drug delivery across the blood-brain barrier. Drug Dev Ind Pharm (2002) 28:1–13. doi: 10.1081/DDC-120001481
79. Ellis-Behnke R. Nano neurology and the four P’s of central nervous system regeneration: preserve, permit, promote, plasticity. Med Clinics North America (2007) 91:937–62. doi: 10.1016/j.mcna.2007.04.005
80. Lovat V, Pantarotto D, Lagostena L, Cacciari B, Grandolfo M, Righi M, et al. Carbon nanotube substrates boost neuronal electrical signaling. Nano Lett (2005) 5:1107–10. doi: 10.1021/nl050637m
81. Mazzatenta A, Giugliano M, Campidelli S, Gambazzi L, Businaro L, Markram H, et al. Interfacing neurons with carbon nanotubes: electrical signal transfer and synaptic stimulation in cultured brain circuits. J Neurosci (2007) 27:6931–6. doi: 10.1523/JNEUROSCI.1051-07.2007
82. Jan E, Hendricks JL, Husaini V, Richardson-Burns SM, Sereno A, Martin DC, et al. Layered carbon nanotube-polyelectrolyte electrodes outperform traditional neural interface materials. Nano Lett (2009) 9:4012–8. doi: 10.1021/nl902187z
83. Dai B, Zhou R, Ping J, Ying Y, Xie L. Recent advances in carbon nanotube-based biosensors for biomolecular detection. TrAC Trends Analytical Chem (2022) 154:116658. doi: 10.1016/j.trac.2022.116658
84. Gomes-Filho SLR, Dias ACMS, Silva MMS, Silva BVM, Dutra RF. A carbon nanotube-based electrochemical immunosensor for cardiac troponin T. Microchemical J (2013) 109:10–5. doi: 10.1016/j.microc.2012.05.033
85. Silva MMS, Dias ACMS, Silva BVM, Gomes-Filho SLR, Kubota LT, Goulart MOF, et al. Electrochemical detection of dengue virus NS1 protein with a poly(allylamine)/carbon nanotube layered immunoelectrode. J Chem Technol Biotechnol (2015) 90:194–200. doi: 10.1002/jctb.4305
86. Comba FN, Romero MR, Garay FS, Baruzzi AM. Mucin and carbon nanotube-based biosensor for detection of glucose in human plasma. Anal Biochem (2018) 550:34–40. doi: 10.1016/j.ab.2018.04.006
87. Rostamabadi PF, Heydari-Bafrooei E. Impedimetric aptasensing of the breast cancer biomarker HER2 using a glassy carbon electrode modified with gold nanoparticles in a composite consisting of electrochemically reduced graphene oxide and single-walled carbon nanotubes. Microchimica Acta (2019) 186:495. doi: 10.1007/s00604-019-3619-y
88. Azimi S, Farahani A, Sereshti H. Plasma-functionalized highly aligned CNT-based biosensor for point of care determination of glucose in human blood plasma. Electroanalysis (2020) 32:394–403. doi: 10.1002/elan.201800895
89. Chen M, Wu D, Tu S, Yang C, Chen D, Xu Y. A novel biosensor for the ultrasensitive detection of the lncRNA biomarker MALAT1 in non-small cell lung cancer. Sci Rep (2021) 11:3666. doi: 10.1038/s41598-021-83244-7
90. Shumeiko V, Paltiel Y, Bisker G, Hayouka Z, Shoseyov O. A paper-based near-infrared optical biosensor for quantitative detection of protease activity using peptide-encapsulated SWCNTs. Sensors (2020) 20:5247. doi: 10.3390/s20185247
91. Zamzami MA, Rabbani G, Ahmad A, Basalah AA, Al-Sabban WH, Nate Ahn S, et al. Carbon nanotube field-effect transistor (CNT-FET)-based biosensor for rapid detection of SARS-CoV-2 (COVID-19) surface spike protein S1. Bioelectrochemistry (2022) 143:107982. doi: 10.1016/j.bioelechem.2021.107982
92. Sapna K, Sonia J, Kumara BN, Arun AB, Prasad KS. Carbon nanotubes for bio-imaging applications. In: Handbook of carbon nanotubes. Cham: Springer International Publishing (2021). p. 1–21. doi: 10.1007/978-3-319-70614-6_40-1
93. Dinarvand M, Neubert E, Meyer D, Selvaggio G, Mann FA, Erpenbeck L, et al. Near-infrared imaging of serotonin release from cells with fluorescent nanosensors. Nano Lett (2019) 19:6604–11. doi: 10.1021/acs.nanolett.9b02865
94. Park S, Kim T, Jo D, Jung JS, Jo G, Park Y, et al. Bioengineered short carbon nanotubes as tumor-targeted carriers for biomedical imaging. Macromol Res (2019) 27:833–8. doi: 10.1007/s13233-019-7141-1
95. Nagai Y, Nakamura K, Yudasaka M, Shiraki T, Fujigaya T. Radical polymer grafting on the surface of single-walled carbon nanotubes enhances photoluminescence in the near-infrared region: implications for bioimaging and biosensing. ACS Appl Nano Mater (2020) 3:8840–7. doi: 10.1021/acsanm.0c01561
96. Saghatchi F, Mohseni-Dargah M, Akbari-Birgani S, Saghatchi S, Kaboudin B. Cancer therapy and imaging through functionalized carbon nanotubes decorated with magnetite and gold nanoparticles as a multimodal tool. Appl Biochem Biotechnol (2020) 191:1280–93. doi: 10.1007/s12010-020-03280-3
97. Merum S, Veluru JB, Seeram R. Functionalized carbon nanotubes in bio-world: Applications, limitations and future directions. Materials Sci. Engineering: B (2017) 223:43–63.
98. Prakash S, Kulamarva A. Recent advances in drug delivery: potential and limitations of carbon nanotubes. Recent Pat Drug Deliv Formul (2007) 1:214–21. doi: 10.2174/187221107782331601
99. Mohanta D, Patnaik S, Sood S, Das N. Carbon nanotubes: Evaluation of toxicity at biointerfaces. J Pharm Anal (2019) 9:293–300. doi: 10.1016/j.jpha.2019.04.003
100. Aoki K, Saito N. Biocompatibility and carcinogenicity of carbon nanotubes as biomaterials. Nanomaterials (2020) 10:264. doi: 10.3390/nano10020264
101. Elgrabli D, Floriani M, Abella-Gallart S, Meunier L, Gamez C, Delalain P, et al. Biodistribution and clearance of instilled carbon nanotubes in rat lung. Particle Fibre Toxicol (2008) 5:20. doi: 10.1186/1743-8977-5-20
102. Usui Y, Aoki K, Narita N, Murakami N, Nakamura I, Nakamura K, et al. Carbon nanotubes with high bone-tissue compatibility and bone-formation acceleration effects. Small (2008) 4(2):240–6. doi: 10.1002/smll.200700670
103. Pitroda J, Jethwa B, Dave SK. A critical review on carbon nanotubes. Int J Constructive Res Civil Eng (2016) 2(5):36–42. doi: 10.20431/2454-8693.0205007
104. Gu W, Yushin G. Review of nanostructured carbon materials for electrochemical capacitor applications: advantages and limitations of activated carbon, carbide-derived carbon, zeolite-templated carbon, carbon aerogels, carbon nanotubes, onion-like carbon, and graphene. Wiley Interdiscip Rev Energy Environ (2014) 3:424–73. doi: 10.1002/wene.102
Keywords: nanotubes, nanoparticles, Alzheimer’s disease, carbon nanotubes, brain tumor
Citation: Elsori D, Rashid G, Khan NA, Sachdeva P, Jindal R, Kayenat F, Sachdeva B, Kamal MA, Babker AM and Fahmy SA (2023) Nanotube breakthroughs: unveiling the potential of carbon nanotubes as a dual therapeutic arsenal for Alzheimer’s disease and brain tumors. Front. Oncol. 13:1265347. doi: 10.3389/fonc.2023.1265347
Received: 22 July 2023; Accepted: 23 August 2023;
Published: 20 September 2023.
Edited by:
Mahmood Barani, Kerman University of Medical Sciences, IranReviewed by:
Mai Ragab, University of Hertfordshire, United KingdomCopyright © 2023 Elsori, Rashid, Khan, Sachdeva, Jindal, Kayenat, Sachdeva, Kamal, Babker and Fahmy. This is an open-access article distributed under the terms of the Creative Commons Attribution License (CC BY). The use, distribution or reproduction in other forums is permitted, provided the original author(s) and the copyright owner(s) are credited and that the original publication in this journal is cited, in accordance with accepted academic practice. No use, distribution or reproduction is permitted which does not comply with these terms.
*Correspondence: Gowhar Rashid, Z293aGFyOUBnbWFpbC5jb20=
†These authors have contributed equally to this work and share first authorship
‡These authors have contributed equally to this work
Disclaimer: All claims expressed in this article are solely those of the authors and do not necessarily represent those of their affiliated organizations, or those of the publisher, the editors and the reviewers. Any product that may be evaluated in this article or claim that may be made by its manufacturer is not guaranteed or endorsed by the publisher.
Research integrity at Frontiers
Learn more about the work of our research integrity team to safeguard the quality of each article we publish.