- 1Department of Oncology, Affiliated Hospital of Nanjing University of Chinese Medicine, Jiangsu Province Hospital of Chinese Medicine, Nanjing, Jiangsu, China
- 2No. 1 Clinical Medical College, Nanjing University of Chinese Medicine, Nanjing, Jiangsu, China
Immunotherapy for non-small cell lung cancer (NSCLC) has advanced considerably over the past two decades. In particular, immune checkpoint inhibitors are widely used for treating NSCLC. However, the overall cure and survival rates of patients with NSCLC remain low. Therefore, continuous investigation into complementary treatments is necessary to expand the clinical advantages of immunotherapy to a larger cohort of patients with NSCLC. Recently, the distinctive role of the gut microbiota (GM) in the initiation, progression, and dissemination of cancer has attracted increasing attention. Emerging evidence indicates a close relationship between the gut and lungs, known as the gut–lung axis (GLA). In this review, we aim to provide a comprehensive summary of the current knowledge regarding the connection between the GM and the outcomes of immunotherapy in NSCLC, with particular focus on the recent understanding of GLA. Overall, promising GM-based therapeutic strategies have been observed to improve the effectiveness or reduce the toxicity of immunotherapy in patients with NSCLC, thus advancing the utilization of microbiota precision medicine.
1 Introduction
Lung cancer is the leading cause of cancer-related deaths worldwide. In 2022, lung cancer was predicted to cause approximately 350 deaths per day in the United States (1). Non-small cell lung cancer (NSCLC) accounts for approximately 85% of lung cancers (2). Lung adenocarcinoma, squamous cell carcinoma, and large-cell carcinoma are considered the three main NSCLC subtypes (3). In the past two decades, there have been significant advancements in the therapeutic strategies targeting of NSCLC (4, 5).
Immunotherapies currently available for lung cancer include nonspecific immunotherapies, adoptive T-cell immunotherapy, and the use of monoclonal antibodies, oncolytic viruses, or cancer vaccines (3). Immune checkpoint inhibitors (ICIs) are monoclonal antibodies that have been demonstrated to prolong the life of patients with NSCLC; these ICIs have since become a routine treatment for this disease, causing a paradigm shift in the therapeutic management of NSCLC (6–9). Programmed cell death-1 (PD-1), programmed cell death ligand-1 (PD-L1), and monoclonal antibodies against cytotoxic T lymphocyte-associated antigen-4 (CTLA-4) are the most common ICIs currently used for NSCLC; these ICIs restrict T cell effector activity within tissues or downregulate the amplitude of T cell activation (10). ICIs can confer long-term therapeutic effects in some patients with lung cancer. However, only 17–21% of patients with advanced-stage NSCLC respond to ICIs, which is a significantly lower response rate than that seen for melanoma (11). Primary ICI resistance affects 25–44% of patients with NSCLC (12). Furthermore, immune-related adverse events (irAEs) hamper the increased overall survival (OS) of patients treated with ICIs (13). Therefore, studies aimed at enhancing ICI response rates and reducing irAEs are crucial for NSCLC.
To date, numerous combination treatments have been developed to delay or prevent ICI resistance, including modulating the tumor microenvironment (TME), blocking immunological co-inhibitory signals, targeting T-cell priming, and activating T-cells with co-stimulatory roles (14). The gut microbiota (GM) is drawing increasing attention with regard to its ability to shape systemic immune responses and influence ICI efficacy (15). The human body contains up to 4×1013 microbial cells, which predominantly consist of bacteria; these bacteria predominantly reside in the gut, accounting for >95% of the total microbial population (16). Additionally, this microbiome contain at least 100 times more genes than our own genome (17). The GM consists of four major phyla (Bacteroidetes, Firmicutes, Proteobacteria, and Actinobacteria) and two minor phyla (Verrucomicrobia and Fusobacteria) (18). Specifically, the GM is considered to be a potent “organ” capable of influencing metabolic, nutritional, physiological, and immunological processes and potentially modulating immunotherapy sensitivity (19).
Nonetheless, the effect of the GM and its metabolites on the efficacy of ICIs in some cancer types such as NSCLC remains poorly understood. In this review, we evaluate the latest information regarding how the GM influences immunotherapy in NSCLC, with an emphasis on the gut–lung axis (GLA). In addition, we summarize the continuing efforts of GM-based manipulation strategies in patients with NSCLC that are receiving immunotherapy. Through this, we aim to provide an effective resource to improve knowledge surrounding the importance of the GM in immunotherapy and NSCLC and provide a foundation for future research.
2 Gut microbiota modulates the effectiveness and toxicity of NSCLC immunotherapy
Microorganisms within the gut influence systemic immune responses and affect the efficacy of ICIs through several hallmark mechanisms (12, 15). Multifactorial clinical responses to ICIs can be divided into two categories: tumor-intrinsic factors (mutational status and oncogenic signaling) and tumor-extrinsic factors (microbiota, TME, and metabolic factors) (20). Insufficient production of antitumor T cells, limited function of tumor-specific T cells, and defective establishment of memory T-cells are the three key explanations for ICI therapy failure (21). Mounting evidence has linked the GM with the corresponding patient response to ICI treatment in preclinical and clinical studies (22). The GM augments the intermediate effects of innate immune cells (dendritic cells, macrophages, and natural killer cells), and enhances the antitumor effects of adaptive immune cells (CD8+ T cells, CD4+ T cells). Additionally, the GM alters TME immunity and host ICI responses (23). The overall effects of GM on the efficacy and toxicity of NSCLC immunotherapy are summarized herein.
2.1 Evidence in preclinical mouse models
Preclinical mouse models are important tools for cancer research. The effect of GM on NSCLC immunotherapy was initially illustrated using a mouse model (Table 1; Figure 1). Individual mice were observed to possess varied responses to NSCLC immunotherapy when fed different GM. Additionally, Newsome et al. transplanted the feces of 65 patients with NSCLC undergoing ICI treatment into germ-free wild-type (GF-WT) mice; this transplantation reduced Lewis lung carcinoma (LLC) growth in these mice. Overall, Bacteroides was enriched in the mouse cohort that received transplantation from ICI responders, whereas Ruminococcus was considerably enriched in the non-responding mouse group (24). In the same mouse model, Routy et al. demonstrated that the GM is vital for the response to PD-1 blockade. Specifically, they established that mice receiving fecal microbiota transplantation (FMT) from responders exhibited a greater response to anti-PD-1 treatment than mice receiving FMT from non-responsive individuals. In addition, in mice recolonized with fecal microbiota from non-responsive patients with NSCLC, gavage therapy with Akkermansia muciniphila alone or in combination with Enterococcus hirae alleviated anti-PD1 unresponsiveness (26). Interestingly, they also discovered that the adjuvant impact of A. muciniphila on the anti-PD1 response resulted in a corresponding increase in CD4+ T cells expressing the chemokine receptor 9(CCR9), which was initiated via the IL-12-dependent signaling pathway (26). Although both studies employed identical LLC mouse models and anti-PD-1 ICIs, differences in the detected bacteria may be attributed to the different experimental conditions.
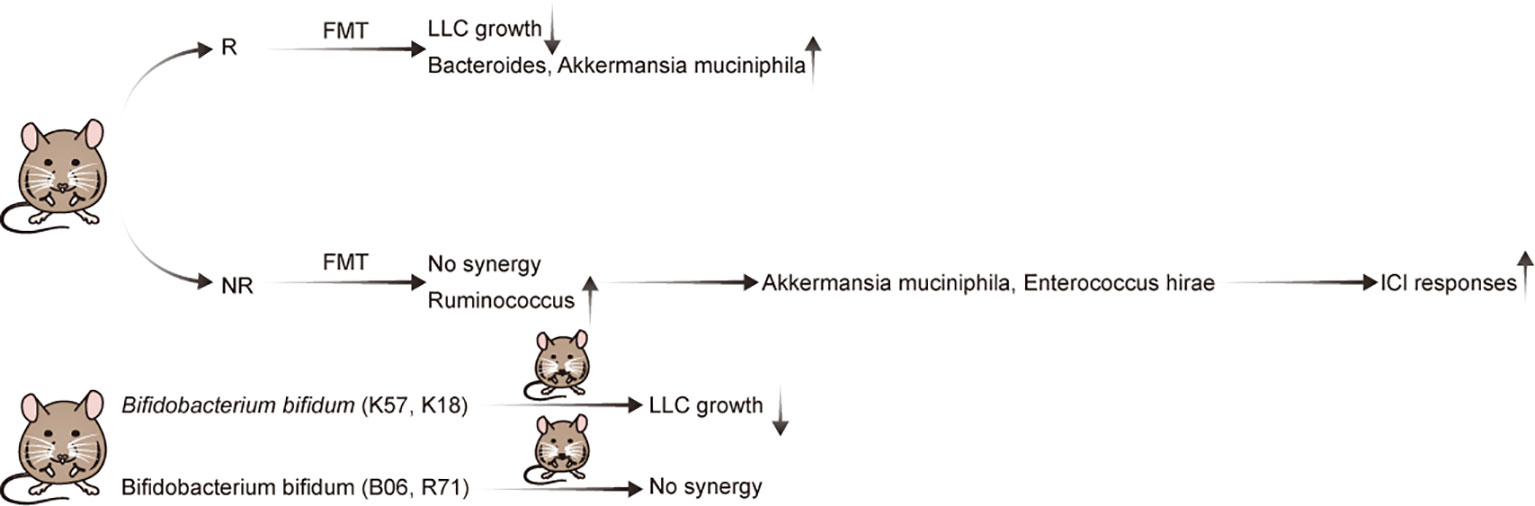
Figure 1 Evidence of the effect of the gut microbiota in preclinical mouse models of NSCLC with immunotherapy.
Strain-level differences in the GM can also affect the expression and synthesis of relevant immunomodulatory components in NSCLC and can, thereby, modulate immunotherapy efficacy (25). A key study established that Bifidobacterium bifidum is enriched in patients with NSCLC who are responsive to ICI treatment (25). Subsequently, Lee et al. supplemented the GM in syngeneic mouse models with four strains (K57, K18, B06, or R71) of B. bifidum. Although all B. bifidum strains inhibited LLC growth, only two (K57 and K18) exhibited beneficial synergistic effects with anti-PD-1 therapy (25). Therefore, as not all strains exhibit identical effects, elucidating the underlying mechanisms is crucial for creating live biotherapeutic products (27). Importantly, it was determined that certain strains of B. bifidum produce large quantities of peptidoglycan, which activate T cells in NSCLC via peptidoglycan-mediated interferon (IFN)signaling. In mouse models of LLC, this ultimately leads to an enhanced immunotherapy response (25).
Finally, comparable findings have been reported in other preclinical solid tumor models, suggesting that the synergistic effect of GM and ICI therapy may be applicable to ICI-resistant cancer. In preclinical mouse models of melanoma, two studies published in Science revealed that PD-1 and CTLA-4 inhibition only suppressed tumor development in mice carrying Bifidobacterium and Bacteroides fragilis species, respectively (28, 29). Further, Griffin et al. determined that Enterococcus spp. with unique NlpC/p60 peptidoglycan hydrolase activity may produce NOD2-active muropeptides; these muropeptides could then alter the effectiveness of checkpoint blockade immunotherapy in vivo (30). In mouse models of colorectal cancer, Mager et al. revealed that bacterial inosine is associated with the GM-dependent response to ICIs (31). FMT from responsive and non-responsive patients into mice with human epidermal growth factor (HER2)-positive breast cancer mimicked the responses to ICIs observed in animal models of breast cancer (32). In renal cell carcinoma (RCC) tumor-bearing mice, oral gavage with feces from ICI-responding patients with RCC reversed the initial resistance of these mice to ICI treatment (33). In NSCLC, the relevant research is still blank. However, in melanoma, gut dysbiosis attenuated the anti-tumour efficacy of Tim-3 blockade in mice (34). In colon adenocarcinoma tumor-bearing mice, the immunotoxicity of anti-CD137 immunotherapies is dependent on GM (35).
These preclinical studies have successfully demonstrated that GM composition has a clear impact on checkpoint blockade effectiveness, and alteration of the GM can enhance response to ICI treatment. Nevertheless, most preclinical investigations that demonstrated the influence of GM on ICI response have been limited to melanoma and colorectal cancer. Therefore, NSCLC should receive more attention in future pre-clinical experiments into the effect of the GM on ICI response.
2.2 Evidence in patients with NSCLC
The human GM does not necessarily colonize mice in the same manner as it colonizes humans. Furthermore, the mucosal and immunological responses of mice to introduced commensals are not comparable to those in humans (36). Consequently, various research groups have sought to define the function of the GM in NSCLC immunotherapy outcomes to better understand the difference in responders (Rs) and non-responders (NRs) (Tables 2, 3). Overall, 16S rDNA sequencing and metagenomic shotgun sequencing (MSS) were used to analyze the composition of GM in these studies.
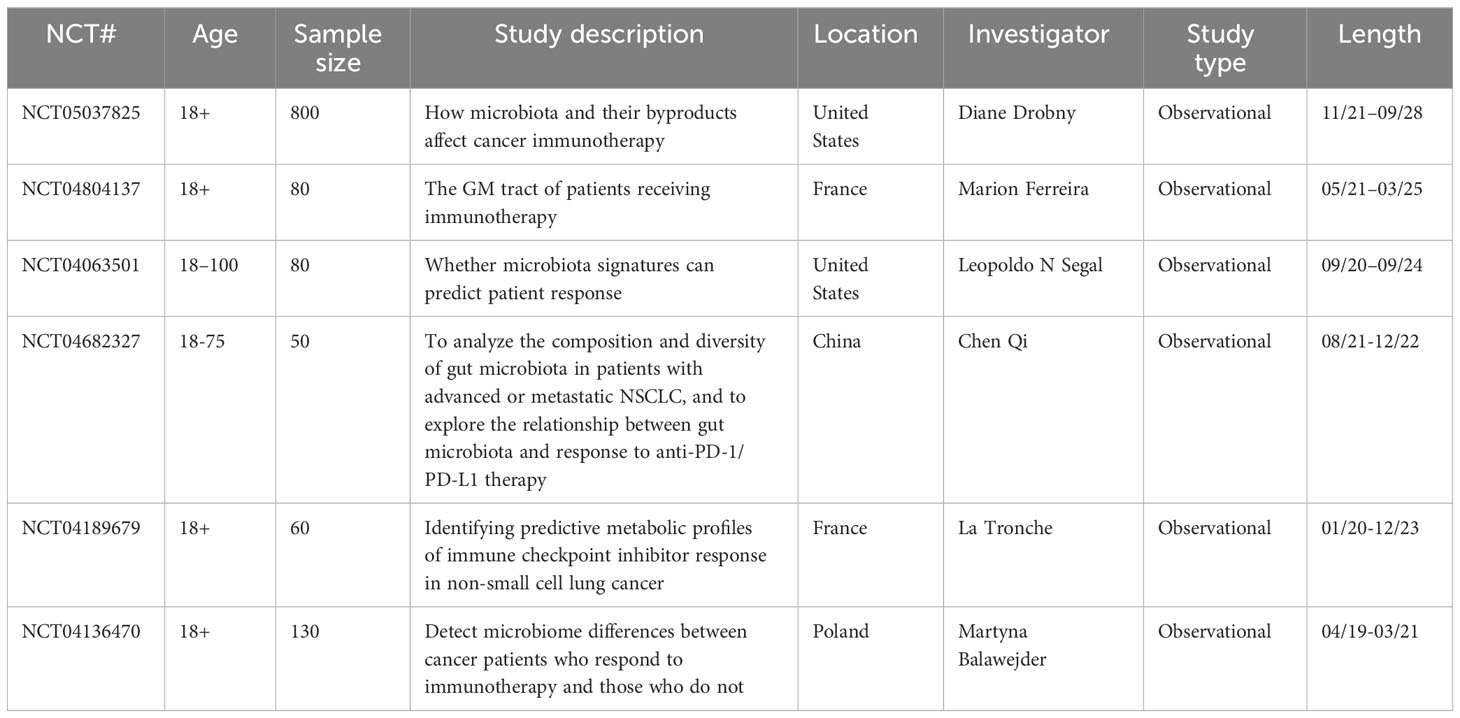
Table 3 Ongoing clinical trials on the association between the intestinal microbiome and immunotherapy in NSCLC (www.clinicaltrials.gov).
First, Routy et al. established that A. muciniphila is overrepresented in the stool samples of patients with NSCLC who benefit from PD-1 treatment. Specifically, they reported an enrichment of A. muciniphila in R vs. NR, as well as a relative underrepresentation of Bifidobacterium adolescentis and Parabacteroides distasonis in those that responded to PD-1 treatment. Antibiotic (ATB) use has also been observed to have a deleterious influence on the immune checkpoint blockade response (26). Four years later, the same research group used microbiome profiling to prospectively evaluate the benefits of fecal A. muciniphila in a large cohort of patients with advanced NSCLC (n = 338) who were treated with first- or second-line ICIs. In this study, ATB usage and the Clostridium genus were linked to ICI resistance (39). Additionally, Martini et al. provided clinical evidence that two butyrate-producing GM (Agathobacter M104/1 and Blautia SR1/5) may improve immune responses in patients with metastatic and chemorefractory NSCLC (44). Jin et al. also reported that memory T cell and natural killer cell signatures in peripheral blood were higher in patients with advanced NSCLC with high gut bacterial diversity (43). Therefore, patients with NSCLC with high microbiome diversity were determined to exhibit a longer progression-free survival (PFS) than those with a low-diversity microbiome (43). Five other studies reported similar results; the various GM compositions associated with improved ICI responses in these studies are listed in Table 2. Overall, there are substantial variations between the diversity and composition of GM in the R and NR groups in patients with NSCLC. GM profiling in patients with NSCLC revealed that a higher gut bacterial diversity and an increased abundance of specific bacteria, such as A. muciniphila, Bacteroides vulgatus, P. distasonis, bacterium LF-3, Sutterella wadsworthensis HGA0223, Agathobacter, Blautia, Alistipes, Anaerostipes, Desulfovibrio, Faecalibacterium, Bifidobacterium, Escherichia-Shigella, Olsenella, Ruminococcaceae UCG 13, Methanobrevibacter, Alistipes putredinis, and Prevotella copri, were associated with a stronger T-cell dependent antitumor response, resulting in favorable ICI clinical outcomes in multiple independent trials. Li et al. analyzed the gut microbiota to explore potential causal associations with various subtypes of lung cancer using mendelian randomization. They identified 10 groups associated with lung adenocarcinoma and 9 groups associated with squamous cell lung cancer. Notably, a significant causal relationship between Peptococcaceae and lung adenocarcinoma was observed, as reported in the literature (45). However, the gastrointestinal microbiome does not always play a beneficial role in NSCLC immunotherapy. According to a retrospective investigation of two independent cohorts of patients with NSCLC, Helicobacter pylori has been associated with an evident decrease in the PFS of patients with NSCLC undergoing anti-PD-1 therapy (46) (Figure 2). Studies have shown that gut microbiota plays an important role in the response of NSCLC patients to anti-PD-1 therapy, and that gut microbiota diversity is significantly increased in patients who respond to anti-PD-1 therapy (47). A prospective study of 63 patients with advanced NSCLC taking anti-PD-1 therapy found that β diversity in gut microbiota at baseline was significantly increased in patients with progression-free survival (PFS)≥ 6 months compared with patients with PFS<6 months; The LEfSe analysis suggested that the most significantly associated groups in patients with PFS≥6 months were Paracterium (LDA score=3.8) and Methanobrevibacterium (LDA score=3.4), whereas the PFS<6 months group was rich in Veillonella, Seleniomonas, and Negativicutes. Further analysis showed that Paracobacterium and Methanobrevibacterium species in NSCLC patients enhance their responsiveness to Immune checkpoint inhibitors (42). The results reported in a study showed that in NSCLC receiving first-or second-line immunotherapy to detect its pre-treatment gut microbiota, Akkermansia muciniphila (AKK+) patients were found to have a higher immunotherapy response rate than AKK-patients (28% VS 18%), among which AKK+ patients, the response rate of first-line immunotherapy was as high as 41%. For survival, overall survival(OS) was longer in the AKK+ group than in the AKK-group (18.8 months vs 15.4 months) (39).
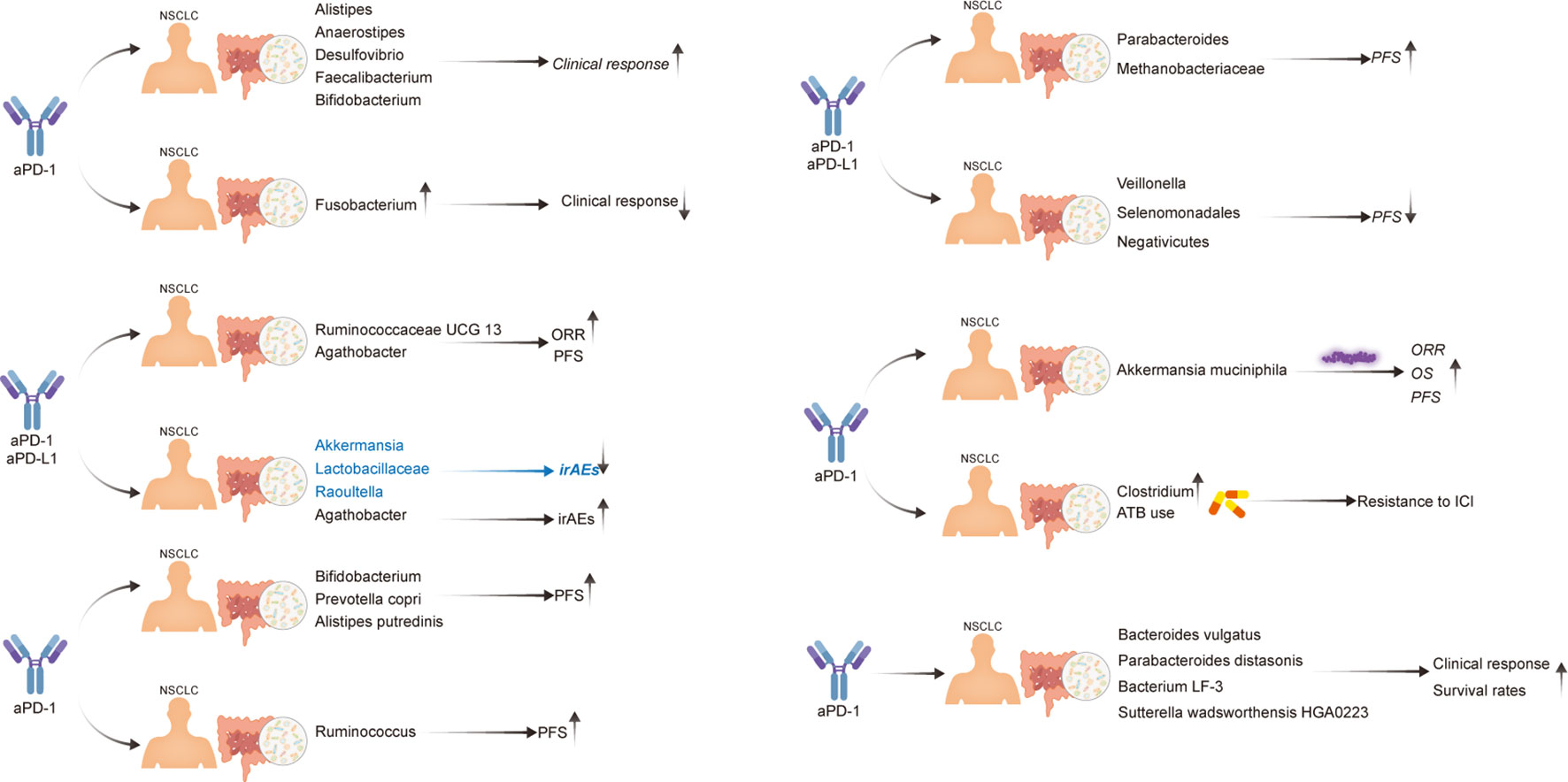
Figure 2 Evidence of the effect of the gut microbiota in patients with NSCLC undergoing immunotherapy.
In summary, experimental and clinical evidence has demonstrated that GM plays a key role in modifying the effectiveness of immunotherapy in NSCLC. However, these studies have several limitations that should be addressed. For example, most clinical trials have been undertaken on a relatively small number of patients with NSCLC; additionally, current research seems to lack consensus on what GM compositions are advantageous or antagonistic to ICI treatment of NSCLC. Accordingly, several clinical studies are being conducted to address these issues (Table 3). Further, the cause-and-effect relationship between the GM and immunotherapy in NSCLC remains unclear. Therefore, further research is required to determine whether the GM modulates immunotherapy in patients with NSCLC or whether immunotherapy alters the GM of patients with NSCLC. Furthermore, the correlations between B cell responses and the GM are yet to be thoroughly investigated in the context of NSCLC immunotherapy.
2.3 Microbiota-derived metabolites and immune-related adverse events
Although ICIs are the first-line therapy for NSCLC, several irAEs have been reported. According to a multicenter cohort study, 623 patients with NSCLC treated with PD-(L)1 monotherapy developed multisystem irAEs, the most prevalent of which were pneumonitis thyroiditis, hepatitis thyroiditis, dermatitis thyroiditis, and dermatitis pneumonitis (48). Anti-PD-(L)1-induced checkpoint inhibitor pneumonitis is more common in patients with NSCLC than in those with other malignancies. Disordered T-cell subsets, elevated preexisting and emerging autoantibodies, and imbalanced inflammatory cytokines are three pathways that may explain this PD-(L)1 inhibitor–mediated pulmonary damage (49). Additionally, according to Tang et al., the most frequently observed irAE is checkpoint inhibitor colitis (50).
However, the treatment of irAEs remains a key source of concern in NSCLC immunotherapy. In most cases of irAE, immunotherapy may be withheld or stopped completely, depending on the severity of toxicity (51). Several studies considering the putative link between GM and irAEs in NSCLC have been conducted. Hakozaki et al. obtained pre-ICI fecal samples from 70 Japanese patients with advanced NSCLC who were then treated with ICIs. Ultimately, they determined that Akkermansia, Lactobacillaceae, and Raoultella were associated with milder irAEs, whereas Agathobacter was associated with more severe irAEs (15). Another study in which 37 patients were enrolled indicated that the GM of patients who did not experience irAEs was relatively enriched in Bifidobacterium and Desulfovibrio spp (52). Bifidobacterium, Faecalibacterium, and Agathobacter were also determined to be less abundant in patients with irAEs (53).
Zeng et al. observed notable alterations in butyrate-producing bacteria within a cohort of patients experiencing secondary resistance (SR) to ICIs. There was a downward trend in these bacteria levels upon the onset of secondary resistance. Notably, Bacteroides played a significant role in the variance between the baseline and the occurrence of irAEs. The abundance of Bacteroides declined after the onset of irAEs and subsequently returned to levels comparable to the baseline after remission (54).
Furthermore, a multicenter non-interventional trial (NCT04107168), involving up to 40 UK sites, is currently underway. To examine mechanisms underlying irAEs, longitudinal stool samples were collected prior to NSCLC immunotherapy and following 6 weeks, 3 months, 6 months, and 12 months of treatment. A clear association between GM composition and ICI toxicity was one of the initial results established in this clinical trial (55).
Additionally, the GM has been reported to be associated with irAEs in other cancers. For example, Andrews et al. established that Bacteroides intestinalis mediates immune-related intestinal damage via IL-1β in melanoma (56). The link between irAEs and several Lachnospiraceae species in melanoma has also been verified by McCulloch et al. (57). Further, Mao et al. determined that a higher GM diversity and relative abundance of the Firmicutes phylum may be protective factors against irAEs in patients with hepatobiliary cancer (58). Finally, compared with the post-treatment microbiota of immune-related acute pancreatitis, a low Bacteroidetes/Firmicutes ratio, a low relative abundance of Alistipes and Bacteroides, and a high Lachnospiraceae abundance were observed in the baseline microbiota of individuals with pancreatic cancer (59).
3 Gut–lung axis
Gut microbiota not only regulates the immune response of gastrointestinal tract, but also influences the health and disease of distal organs such as respiratory system through intestinal microecology. The relationship and interaction between the gut and the lung is called Gut-lung axis (60).
Although the gut and the lungs are physically separate and possess substantially different functions, they share several similarities. First, they share a common embryonic origin and structural similarities (61, 62). Specifically, both organs are derived from the endoderm and consist of columnar epithelial cells with projections of microvilli (gut) or cilia (lungs). Both organs also secrete mucus through goblet cells (63). Additionally, both the gut and the lungs possess an enormous surface area exposed to the external environment; hence, they both serve as the first line of defense against invading foreign pathogens and play important roles in innate and adaptive immunity (64). The GLA hypothesis arises from the understanding that intestinal and respiratory disorders share pathological abnormalities; further, alterations in the gut microenvironment may influence the pathology of various lung disorders (65, 66).
Considerable evidence has indicated that dynamic crosstalk between the gut and the lungs establishes the GLA. Additionally, bronchi content aspiration can deliver up to 1011 live bacteria to the intestines each day. Further, gut-derived harmful substances can enter the lung circulation via intestinal lymphatics (67). Huang et al. discovered a unique cell type (group 2 innate lymphoid cells) that resides in the gut but can migrate to the lungs via sphingosine-1-phosphate receptors and can, ultimately, contribute to host defense (68). Moreover, Ruane et al. established that lung dendritic cells, such as CD103(+) mesenteric lymph node (MLN) dendritic cells, stimulate protective T lymphocyte migration to the gastrointestinal tract (69). Further, microbiota administered into the nasal cavity of mice can be observed in the gut following a short incubation period (70).
However, the mechanisms mediating the communication between the gut and lungs remain unclear. An idea, named the common mucosal immune system, was proposed in 1978 (71). This system operates according to the following scheme: Peyer’s patches (PP) capture inhaled antigens and transport them to antigen-presenting cells (APCs); naïve T and B cells are induced in the PPs; after sensitization, T and B lymphocytes travel to the MLNs and the thoracic duct to reach the bloodstream; subsequently, these sensitized T and B cells are distributed to several effector sites, including the gut-associated lymphoid tissue (GALT) and bronchus-associated lymphoid tissue (BALT) (67). The key immunological roles of GALT and BALT include IgA synthesis and secretion at mucosal surfaces, alongside establishing helper and cytotoxic T cell responses (72, 73). Local immune responses to GALT and BALT can affect systemic immunological responses; nonetheless, these immune responses can also operate independent of the systemic site (65). Thus, according to the common mucosal immune system, the circulation of lymphatic fluid and blood contributes to the interactions observed between the gut and the lungs (Figure 3).
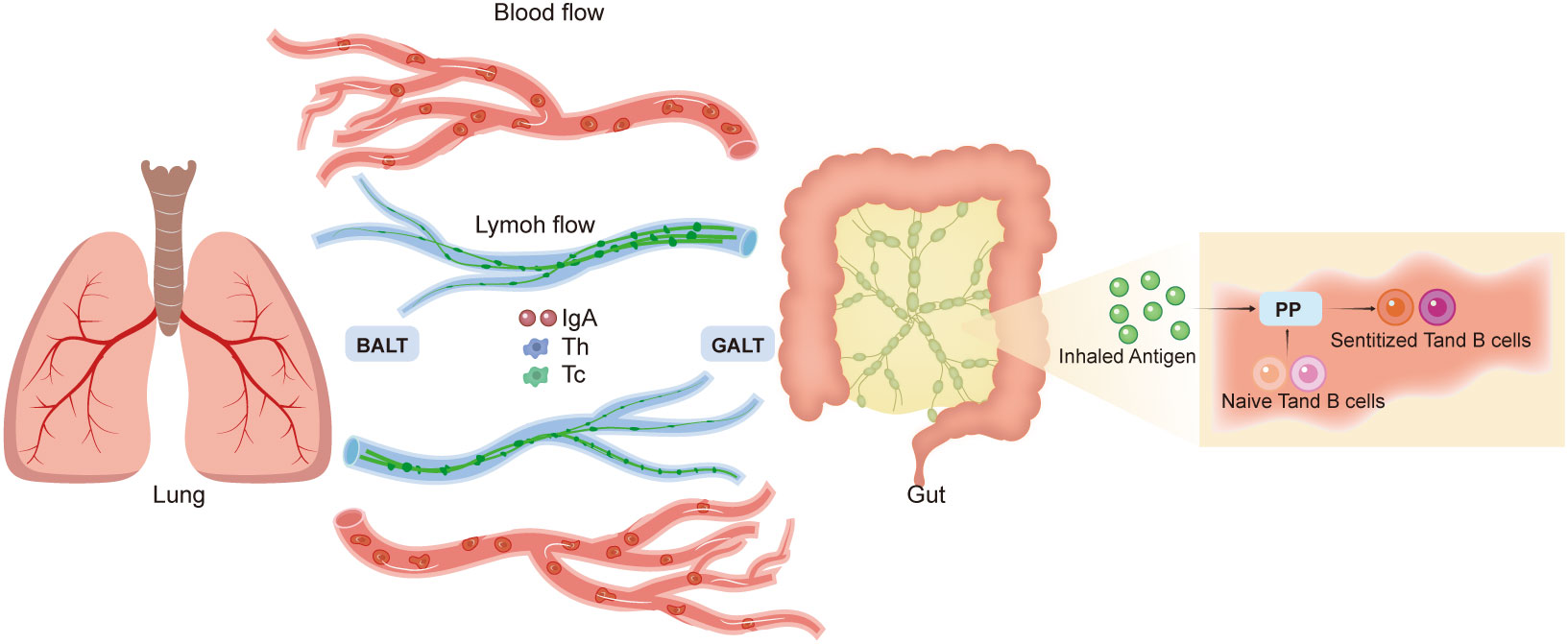
Figure 3 Gut–lung axis. The mechanisms of gut–lung communication are unclear. The common mucosal immune system theory suggests that Peyer’s patches capture inhaled antigens and induce naive T and B cells. These sensitized T and B lymphocytes travel to the mesenteric lymph nodes and bloodstream, which distribute these lymphocytes to effector sites, such as the gut-associated lymphoid tissue (GALT) and bronchus-associated lymphoid tissue (BALT). GALT and BALT play immunological roles and can impact systemic responses. According to this system, circulation of the lymphatic fluid and blood can contribute to gut–lung interactions.
The bidirectional relationship between the gut and the lungs is the foundation for understanding the role of the GM in NSCLC immunotherapy. Different GM can act on the epithelial barrier to activate innate and cognate immune responses against NSCLC antigens; this activation occurs through a range of mechanisms, including endoplasmic reticulum stress, intestinal crypt apoptosis, inflammasome activation, Toll-like receptor signaling, nucleotide-binding oligomerization domain activation, and chemokine receptor signaling (74). In addition to the mucosal immune system, three other mechanisms have been postulated to explain the effects of the GM on lung immunity, which may be associated with NSCLC immunotherapy response rate. First, cytokines and growth factors generated by the gut mucosa in response to the GM may enter the systemic circulation and affect other mucosal tissues. Second, microbial-associated molecular patterns can be absorbed and transported to the lungs, where they can regulate immune responses. Third, metabolites ingested by the GM have been linked to a phenomenon known as “metabolic reprogramming,” which involves the manipulation of mucosal immunity (75); for example, the concentration of circulating short-chain fatty acids (SCFAs) in the intestine affects IL-6 and IL-8 levels at the NSCLC tumor site (76).
Through the down-regulation of mucosal addressin cell adhesion molecule 1 (MAdCAM-1) in the ileum, post-antibiotic (ABX) gut recolonization by Enterocloster species facilitated the migration of enterotropic α4β7CD4 regulatory T 17 cells towards the tumor. Conversely, fecal microbiota transplantation (FMT) or neutralization of interleukin-17A prevented the ABX-induced immunosuppression. Notably, in distinct cohorts of lung cancer patients, reduced serum levels of soluble MAdCAM-1 were associated with an adverse prognosis. This underscores the significance of the MAdCAM-1-α4β7 axis as a modifiable gut immune checkpoint in the context of cancer immunosurveillance (77, 78).
4 Strategies to manipulate the GM for NSCLC immunotherapy
There are a number of potential approaches to target regulation of the gut microbiota to enhance NSCLC immunotherapy response rates, including FMT, ATBs, prebiotics, probiotics, postbiotics, synbiotics, bacteriophages, and diet. These approaches can alter the composition and function of the gut microbiota and can be used to promote gut microbiota balance and function in NSCLC immunotherapy.
4.1 Fecal microbiota transplantation
FMT involves the transfer of GM from a known donor to a recipient via the upper or lower gastrointestinal route to restore microbial diversity within the intestine (79). In 1958, Eiseman published the first report on the use of FMT for the treatment of severe pseudomembranous enterocolitis (80). Despite being a well-established therapy for recurrent Clostridium difficile infections, FMT is now being explored in the context of cancer treatment (81).
FMT is a potential therapy for various types of cancer and cancer treatment–associated complications. In preclinical mouse models, Shaikh et al. utilized FMTs from two NSCLC patients who had contrasting responses to ICIs (R and NR); overall, R-FMT mice exhibited superior antitumor responses when paired with ICI treatment compared to that of NR-FMT mice (11). Baruch et al. and Davar et al. reported the first-in-human clinical trials conducted to determine whether FMT can influence the response of patients with metastatic melanoma to anti–PD-1 immunotherapy. Both trials demonstrated signs of therapeutic benefit in some treated patients, including a higher abundance of taxa associated with an anti–PD-1 response and enhanced CD8+ T cell activation (82, 83). Several clinical trials of NSCLC are currently being conducted to evaluate whether combining FMT with immunotherapy can enhance the outcomes of patients with NSCLC (Table 4).
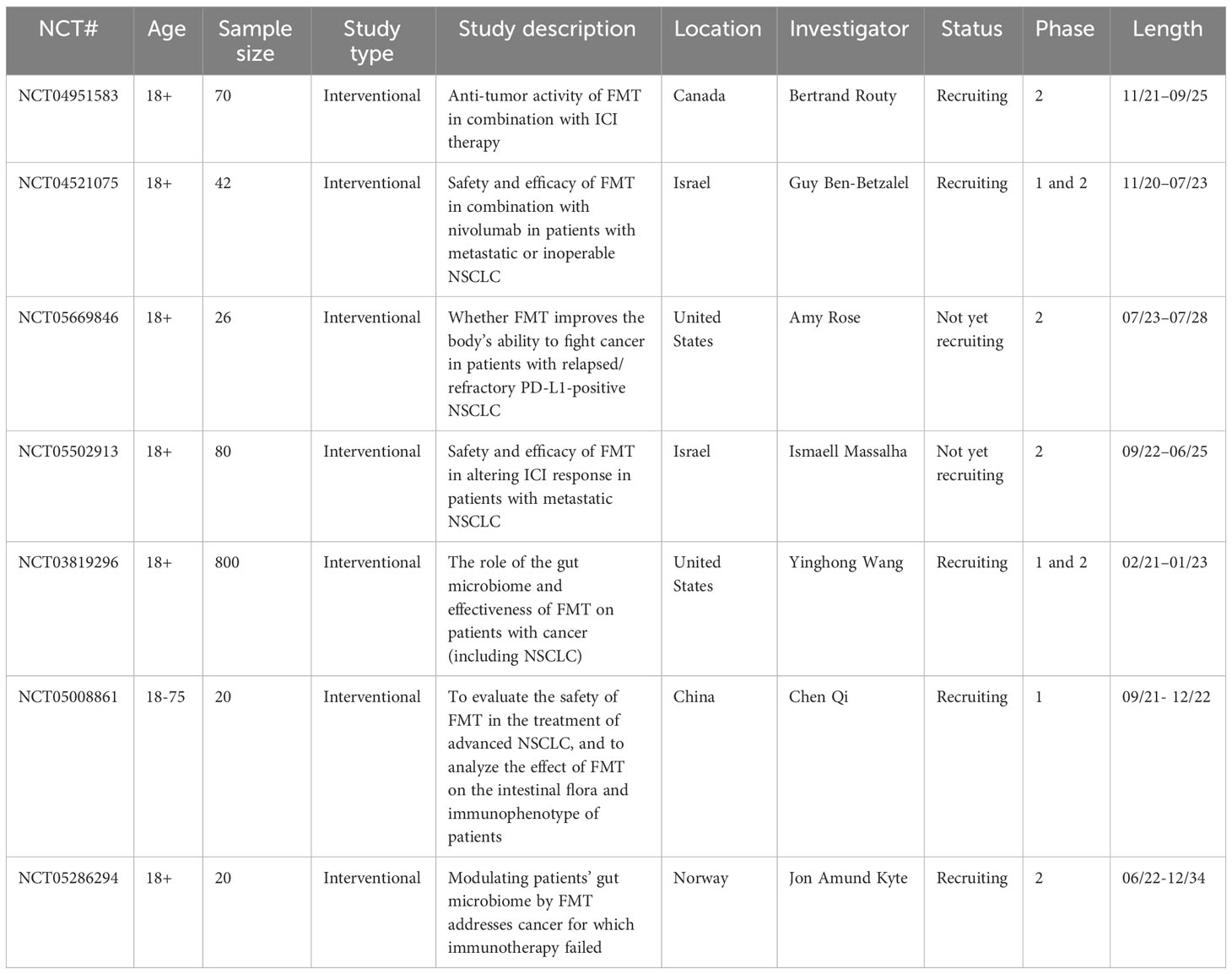
Table 4 Ongoing clinical trials on the effects of FMT on NSCLC outcomes (www.clinicaltrials.gov).
Despite the promising results of FMT in patients treated with ICIs, concerns remain regarding its long-term safety. According to a study published in Lancet, 32 (78%) of the 41 individuals receiving FMT treatment experienced adverse events, the majority of which were self-limiting gastrointestinal problems (84). Nonetheless, a randomized, placebo-controlled trial that evaluated the safety of FMT for active peripheral psoriatic arthritis reported no serious adverse events in patients treated with FMT (85). According to the European consensus on FMT in clinical practice, FMT shows an excellent short-term safety profile, with only a few mild adverse events observed. However, long-term safety data are limited. Therefore, theoretically, FMT may transfer potentially dangerous microbial features as well. In conclusion, given that FMT is a highly effective treatment for NSCLC patients, the benefit-to-risk ratio should be considered for each individual (86).
4.2 Antibiotics and proton pump inhibitors
ATBs not only kill pathogenic bacteria but also alter the diversity and composition of the GM, thereby disrupting gut homeostasis (87–89). In the context of NSCLC, ATBs appear to be associated with a reduction in ICI therapeutic impact (Table 5). Specifically, PFS duration was considerably reduced in patients with NSCLC who were treated with ATBs within 2 months of their initial ICI therapy than in those who had not received ATB treatment within this timeframe (96). Furthermore, the use of ATBs before ICI therapy has been demonstrated to reduce GM diversity, and the presence of Ruminococcaceae in patients with advanced NSCLC resulted in unfavorable outcomes (15). The effectiveness of cancer therapies has also been revealed to be impaired in ATB-treated and GF mice (97).
However, considering that many patients with NSCLC require ATB treatment over the course of this disease, the current focus of research is to determine what factors can reduce the effects of ATBs on ICI therapy. First, the use of ATBs during the early immunotherapy period may be associated with poor therapeutic outcomes. Interesting data from Zitvogel et al. demonstrated that patients receiving ATBs immediately before or after initiating ICI therapy exhibited poor outcomes (26). Similarly, in a trial involving 72 patients with NSCLC who were treated with nivolumab, the early use of ATBs had also been associated with a shorter OS (98). A meta-analysis conducted on patients with NSCLC established that exposure to ATBs shortly before or after ICI therapy had a notably harmful impact, whereas ATB use later in the disease progression had no significant effect on survival (99). Second, patients with NSCLC that possessed a higher ATB-ICI exposure ratio (defined as “number of days of ATB administration/number of days of ICI administration” for the entire immunotherapy period) also exhibited poor outcomes (100). Third, the effect of ATBs on ICI effectiveness varies depending on the PD-L1 expression levels in patients with advanced NSCLC. Specifically, a deleterious effect has been observed in patients with ≥50% PD-L1 expression but not in those with <50% PD-L1 expression (101). Finally, with all other clinically relevant parameters constant, patients who received a single course of ATBs were observed to exhibit a shorter median OS and PFS, whereas those who received multiple courses or prolonged ATB therapy exhibited the worst overall outcomes (95). Gut microbiota plays a crucial role in immune response. The use of antibiotics affects the efficacy of ICIs by altering the gut microbiota. Alkan et al. showed that the use of antibiotics at the start of ICIS was associated with reduced OS, PFS and objective response rate (ORR) in NSCLC patients (102). This suggests that gut microbiota diversity may be one of the factors predicting ICI efficacy. Therefore, cumulative ATB use is not recommended.
Similar to ATB, PPIs are negative prognostic markers in patients with NSCLC who are undergoing immunotherapy. In a clinical study involving 169 individuals, PPI use was associated with shorter PFS in patients with NSCLC that were treated with atezolizumab (91). Additionally, PPI use has been observed to negatively influence ICI efficacy in patients with NSCLC who received atezolizumab + carboplatin + paclitaxel treatment (ACP) or atezolizumab + bevacizumab + carboplatin + paclitaxel treatment (ABCP) but not in those who received bevacizumab + carboplatin + paclitaxel treatment (BCP) (91). Moreover, a meta-analysis indicated that PPI treatment is associated with a reduced PFS and OS in patients with advanced cancer who were treated with ICIs (103). Thus, when not clearly warranted, PPI medication should be discontinued; additionally, the use of histamine H2-receptor antagonists should be investigated as an alternative to PPI treatment.
In conclusion, there is a consensus on the negative effects of ATBs and PPIs on patients with NSCLC who are receiving immunotherapy. Given that the prohibition of ATB use is impractical for certain patients, modulating ATB-related GM dysbiosis may be a viable strategy to improve clinical outcomes. Therefore, the use of targeted antibiotics that allow immunopotentiating bacteria to proliferate have been recently proposed (104). However, the dosage, frequency, and delivery methods for targeted ATBs remain to be elucidated.
4.3 Probiotics, prebiotics, postbiotics, and synbiotics
Recently, probiotics, prebiotics, and postbiotics have gained the attention of clinicians due to their potential to enhance ICI efficacy (Table 6). “Probiotic” was first defined in 1965 (105); nonetheless, the most recent definition of probiotic is as follows: “live microorganisms that, when administered in adequate amounts, confer a health benefit on the host” (106). Prebiotics are fibers that are digestible by individual commensal species; these bacteria metabolize the prebiotics, thereby producing secondary metabolites such as SCFAs (107). Any material released or produced via the metabolic activities of microbes that has a direct or indirect positive impact on the host is regarded as a postbiotic (108). Finally, synbiotics are a mix of prebiotics and probiotics and have been proven advantageous in inflammatory bowel disease; however, their impact on cancer is not yet examined in clinical studies (104).
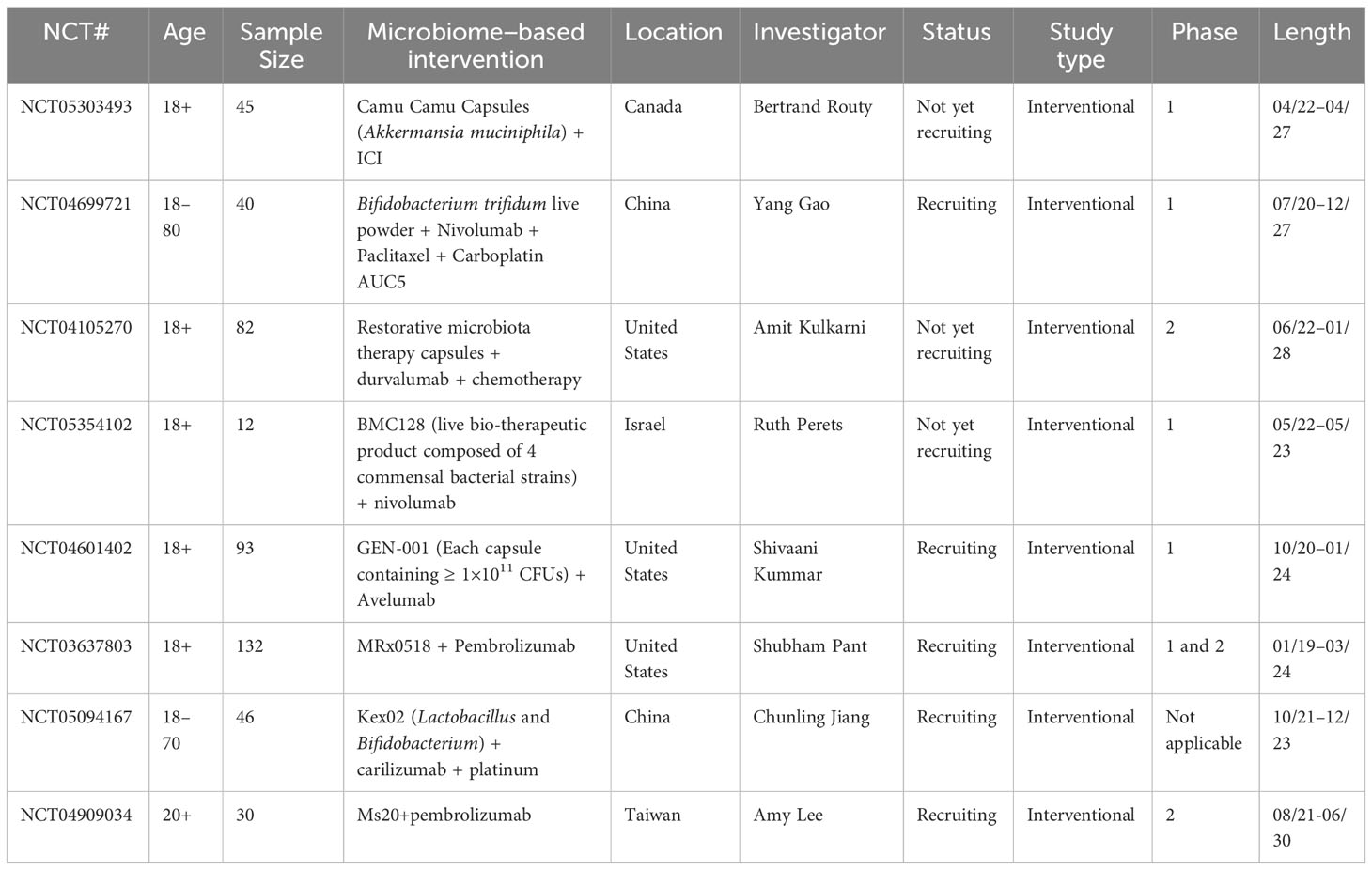
Table 6 Clinical trials for the use of prebiotics, probiotics, postbiotics, and synbiotics in combination with ICIs for the treatment of NSCLC.
Probiotics, prebiotics, postbiotics, and synbiotics play important roles in assisting immunotherapy in the treatment of NSCLC. A retrospective study involving 294 patients established that probiotic use is associated with favorable clinical outcomes in patients with advanced or recurrent NSCLC who were subjected to anti-PD-1 monotherapy (109). Alternatively, a retrospective study evaluated 118 patients with advanced NSCLC who were treated with ICIs at Kumamoto University Hospital. Significant improvements in PFS and OS were observed in patients who received probiotic Clostridium butyricum therapy, even in those who had previously undergone ATB therapy before ICI therapy (110). Castalagin, the active ingredient in camu-camu, can concentrate microorganisms (Ruminococcaceae and Alistipes) associated with effective immunotherapeutic responses and can enhance the CD8+/FOXP3+CD4+ ratio in the TME. In preclinical ICI-resistant models, castalagin was determined to re-establish the efficacy of anti–PD-1 in NSCLC (111). Further, in addition to improving survival, 3-IAld (a microbial tryptophan catabolite) has been found to protect mice with ICI-induced colitis from intestinal injury by acting on both the host and microbiota (112). In a direct comparison study, the oral application of a combination of four Clostridiales strains (CC4) was observed to outperform anti-PD-1 therapy in mouse models of NSCLC. Specifically, CC4 therapy has been found to suppress tumor growth by increasing the number of IFN-γ+ CD8+ T cells and natural killer cells while reducing the number of PD-L1+ macrophages (113). A meta-analysis determined that probiotics are favorably associated with the OS and PFS of patients with NSCLC that are being treated with ICIs, but had no effect on ORR (114). Similar results have also been reported in other cancer types. For example, CBM588 appeared to increase the PFS of patients with metastatic RCC who were being treated with nivolumab-ipilimumab (115). Additionally, the adjunctive probiotic Lactobacillus rhamnosus probio-M9 was determined to enhance the effect of anti-PD-1 antitumor therapy by repairing antibiotic-disrupted GM in mouse models of colorectal cancer (116). Chen et al. showed that JK5G postbiotics may attenuate immune-related adverse events irAEs and improve quality of life (QoL) and nutritional levels in advanced NSCLC patients receiving ICIs. JK5G postbiotics can also improve gut microbiota structure, increase Faecalibacterium, Ruminococcaceae relative abundance, fecal butyrate concentration, reduce Escherichia-Shigella relative abundance, and improve tumor microenvironment and inflammation (117). A study reveals that probiotic Lactobacillus reuteri (Lr) uses its released dietary tryptophan catabolite indole-3-aldehyde(I3A), it locally promoted the production of interferon-γ by CD8 T cells, thus bolstering ICI (90).
Overall, opinions have been divided regarding the safety of probiotics. Systemic infections, harmful metabolic activities, excessive immunological activation in sensitive individuals, gene transfer, and gastrointestinal side effects are the potential dangers of this therapeutic strategy. On the other hand, critics argue that the overwhelming body of evidence, including a long history of safe probiotic usage and data from clinical trials, alongside animal and in vitro research, supports the understanding that probiotics are generally safe for most individuals (118). In general, more advantages than disadvantages have been observed with regard to probiotic treatment. Ultimately, probiotics, prebiotics, postbiotics, and synbiotics are potentially viable strategies as they are less complicated and presumably safer than FMT, which has been associated with increased pathogen transmission.
4.4 Bacteriophages
Non-bacterial microorganisms in the GM have received less attention than bacteria, despite their potential importance. Bacteriophages, also known as bacterial viruses or phages, are obligate intracellular parasites that rely on the metabolic apparatus of their bacterial host for multiplication (119). Bacteriophages are the most abundant biological entities on Earth (120). Fluckiger et al. established that E. hirae harbors a bacteriophage that can modify immunological responses. After PD-1 inhibition, mice bearing E. hirae bacteriophages have been observed to exhibit an increase in the CD8+ T lymphocyte response. In patients with lung cancer, the presence of bacteriophages in their feces and the expression of a tape measure protein–cross-reactive antigen in tumors has been linked to better survival following PD-1 treatment (121). Dong et al. screened a strain of selective Fusobacterium nucleatum–binding M13 bacteriophages. The introduction of silver nanoparticles onto the surface of the M13 bacteriophages allowed precise scavenging of pro-tumor F. nucleatum; consequently, the host’s immune response was enhanced via APC activation and the tumor-immunosuppressive microenvironment was remodeled. Notably, M13-Ag combined with PD1 inhibitors has been observed to significantly prolong OS in a colorectal cancer mouse model (122). Therefore, the use of bacteriophages to modulate the GM for NSCLC immunotherapy is increasingly gaining importance in both basic and translational research.
4.5 Diet
Diet plays an essential role in bridging the connection between humans and the microbiota. The GM consumes and utilizes ingested nutrients for basic biological activities; therefore, diet is a pivotal determinant of GM structure and function (123, 124). Recent evidence suggests the use of high-fiber diets for better outcomes in cancer immunotherapy. In a prospective cohort of patients with melanoma that were receiving ICI therapy, intake of a fiber-rich whole-grain diet was strongly correlated with increased PFS. Interestingly, PFS was observed to be the highest in the group that consumed a high-fiber diet but did not consume probiotics. The inhibition of intratumoral IFN-γ T cell responses was assumed to be the cause of the worsened response to immunotherapy observed in those on a low-fiber diet (125). Dietary fermentable fiber content alters the ratio of Firmicutes to Bacteroidetes in the gut and the lungs. The GM digests fiber, which increases the concentration of circulating SCFAs and influencing the lung immune environment (126). In addition to high-fiber diets, compelling evidence has suggested that fasting-mimicking diets, long-term calorie restrictions, and ketogenic diets may improve anticancer immunosurveillance during immunotherapy (12, 127–131). Overall, several studies have focused on the influence of diet on immunotherapy for NSCLC (Table 7).
5 Discussion
Overall, this review provides new insights into the importance of the GM in NSCLC immunotherapy. Wide differences in the diversity and composition of the GM have been discovered between patients with NSCLC who responded and failed to respond to ICI therapy. The bidirectional GLA has recently emerged as a distinct two-way interaction between the lungs and the gut, including both microbial and immunological interactions. Specific GM compositions may communicate with innate and adaptive immune cells, ameliorate ICI responses, and alleviate irAEs in NSCLC. Therefore, numerous studies have explored the optimal bacterial environment within the gut that best enhances immunotherapy effectiveness in NSCLC. FMT, ATBs, PPIs, probiotics, prebiotics, postbiotics, synbiotics, bacteriophages, and diet alterations are among the methods currently being investigated.
To date, four major limitations have been identified regarding the use of the GM as a therapeutic strategy. First, the cause-and-effect relationship between GM and immunotherapy in NSCLC remains unclear. Therefore, larger multicenter collaborations and randomized controlled trials should be performed to identify whether a specific signature GM is a common biomarker for enhancing ICI response and preventing irAEs in NSCLC. Second, it is yet to be clarified whether the current findings on the role of the GM in modulating ICI responses in NSCLC animal models and in patients with other tumor types, such as melanoma or colorectal cancer, also apply to human patients with NSCLC. Third, further investigations into the interaction of GM with other immunotherapies, such as CAR-T cell therapy, dendritic cell vaccines, and adoptive cell transfer, in NSCLC are warranted. Finally, it is important to avoid treating diseases in isolation because organs can interact with each other in various ways. The mechanism of the GLA has primarily been elucidated through the relationship between the GM and asthma, chronic obstructive pulmonary disease, and respiratory infections. Therefore, further research is necessary to understand the effects of the lung microbiota on the gut and the role of the GM in the development and treatment of lung cancer. Specifically, evaluating how the GM modulates NSCLC immunotherapy may further elucidate the implications of GLA interactions in this disease.
Potential risks and side effects of FMT include infection, disease transmission, allergic reactions, etc. Patients who receive FMT may develop intestinal or systemic infections and may develop drug or food allergies. The application of FMT in NSCLC immunotherapy is still in the exploratory stage and has not been widely used at present. Potential risks and side effects of antibiotics include disruption of gut microbiota, development of drug resistance, resulting in intestinal problems such as diarrhea and constipation. Long-term use of antibiotics may also lead to the development of resistance, making antibiotics ineffective. Probiotics may cause drug or food allergies and may interact with antibiotics or other medications. In summary, FMT, antibiotics, and probiotics all carry potential risks and side effects in NSCLC immunotherapy. When using these interventions, it is necessary to weigh the pros and cons according to the specific situation of the patient and choose the most appropriate treatment method. Further studies are needed to determine the long-term efficacy and safety of these interventions (132, 133).
ICI efficacy is often limited by treatment resistance and adverse reactions. Therefore, the development of less invasive biomarkers can identify responders and non-responders early in treatment and significantly improve treatment protocols. Ni et al. found that Agathbacter, Blautia, Clostridium and Muribaculacea were more abundant in patients with early NSCLC than in healthy controls. Dysregulation of pathways such as sphingolipid metabolism and sphingolipid signaling pathway may be emerging therapeutic strategies for early NSCLC (134). Zhu et al. showed that the metabolite butyrate of the gut microbiota, promotes the efficacy of anti-PD-1 therapy by modulating T cell receptor (TCR) signaling of cytotoxic CD8 T cells, and the metabolite butyrate may be a very promising therapeutic biomarker for enhancing anti-tumor immunity (135).Sarkar et al. found decreased abundance of Odoribacter, Gordonibacter, Stoquefichus, Escheria-Shigella, and Collinsella genera and increased abundance of Clostridium sensu stricto1 in fecal samples of NSCLC patients receiving anti-PD-1 therapy. In contrast, nonresponders to anti-PD-1 immunotherapy showed increased treatment with Prevotella, Porphyromonas, Streptococcus, and Escherichia-Shigella,as well as decreased abundance of Akkermannia. Gut microbiota have potential utility as a noninvasive biomarker for NSCLC patients to predict response to anti-PD-1 therapy in NSCLC patients and need to be validated in larger prospective studies (136). In the future, it is hoped that gut microbiota and its metabolites will be used as predictive invasiveness biomarkers for response rate to ICI therapy of NSCLC patients, and realize clinical transformation. Therefore, the composition and function of gut microbiota in NSCLC patients should be clarified first, and specific microbial markers related to immunotherapy should be searched. Animal model experiments were conducted to test the effects and side effects of different intervention strategies, and to explore the optimal time and dose of intervention. Finally, testing and validation is performed in clinical trials to determine the optimal treatment regimen. Emerging technologies, such as chip-based gut models, edible capsules for microbiota sampling, and metabolomic analysis may accelerate the development of intervention strategies for gut microbiota.
6 Conclusion
Modulation of gut microbiota homeostasis as a new approach to treating NSCLC patients, this approach improves NSCLC therapeutic outcomes through manipulation of gut microbiota, such as probiotics design, FMT, dietary improvement, and modulation of antibiotics application, but all of these approaches lack target specificity. Gut microbiota provides new strategies for the occurrence, development, diagnosis, treatment and prognosis of NSCLC, however, its clinical application still faces the influence of age, gender, disease status and environmental factors on the gut microbiota, which also varies in different individuals, and the immunoregulatory mechanism of gut microbiota is not yet fully understood, which still needs to be extensively validated by a large number of representative preclinical models and clinical trials. In the future, a comprehensive understanding of the mechanisms of probiotic species and gut microbiota in the host and tumor cells is needed, which will help guide the treatment of NSCLC and improve the prognosis.
Author contributions
HZ: Writing – original draft, Writing – review & editing. ZX: Visualization, Writing – original draft.
Funding
The author(s) declare that no financial support was received for the research, authorship, and/or publication of this article.
Conflict of interest
The authors declare that the research was conducted in the absence of any commercial or financial relationships that could be construed as a potential conflict of interest.
Publisher’s note
All claims expressed in this article are solely those of the authors and do not necessarily represent those of their affiliated organizations, or those of the publisher, the editors and the reviewers. Any product that may be evaluated in this article, or claim that may be made by its manufacturer, is not guaranteed or endorsed by the publisher.
References
1. Siegel RL, Miller KD, Fuchs HE, Jemal A. Cancer statistics, 2022. CA Cancer J Clin (2022) 72(1):7–33. doi: 10.3322/caac.21708
2. Relli V, Trerotola M, Guerra E, Alberti S. Abandoning the notion of non-small cell lung cancer. Trends Mol Med (2019) 25(7):585–94. doi: 10.1016/j.molmed.2019.04.012
3. Boyero L, Sanchez-Gastaldo A, Alonso M, Francisco Noguera-Ucles J, Molina-Pinelo S, Bernabe-Caro R. Primary and acquired resistance to immunotherapy in lung cancer: unveiling the mechanisms underlying of immune checkpoint blockade therapy. Cancers (2020) 12(12):3729. doi: 10.3390/cancers12123729
4. Wang M, Herbst RS, Boshoff C. Toward personalized treatment approaches for non-small-cell lung cancer. Chin J Lung Cancer (2022) 25(3):c1–c18. doi: 10.1038/s41591-021-01450-2
5. Osmani L, Askin F, Gabrielson E, Li QK. Current WHO guidelines and the critical role of immunohistochemical markers in the subclassification of non-small cell lung carcinoma (NSCLC): Moving from targeted therapy to immunotherapy. Semin Cancer Biol (2018) 52:103–9. doi: 10.1016/j.semcancer.2017.11.019
6. Suresh K, Naidoo J, Lin CT, Danoff S. Immune checkpoint immunotherapy for non-small cell lung cancer benefits and pulmonary toxicities. Chest (2018) 154(6):1416–23. doi: 10.1016/j.chest.2018.08.1048
7. Inarrairaegui M, Melero I, Sangro B. Immunotherapy of hepatocellular carcinoma: facts and hopes. Clin Cancer Res (2018) 24(7):1518–24. doi: 10.1158/1078-0432.Ccr-17-0289
8. Ren S, Xiong X, You H, Shen J, Zhou P. The combination of immune checkpoint blockade and angiogenesis inhibitors in the treatment of advanced non-small cell lung cancer. Front Immunol (2021) 12:689132. doi: 10.3389/fimmu.2021.689132
9. Remon J, Passiglia F, Ahn M-J, Barlesi F, Forde PM, Garon EB, et al. Immune checkpoint inhibitors in thoracic Malignancies: review of the existing evidence by an IASLC expert panel and recommendations. J Thorac Oncol (2020) 15(6):914–47. doi: 10.1016/j.jtho.2020.03.006
10. Pardoll DM. The blockade of immune checkpoints in cancer immunotherapy. Nat Rev Cancer (2012) 12(4):252–64. doi: 10.1038/nrc3239
11. Shaikh FY, Gills JJ, Mohammad F, White JR, Stevens CM, Ding H, et al. Murine fecal microbiota transfer models selectively colonize human microbes and reveal transcriptional programs associated with response to neoadjuvant checkpoint inhibitors. Cancer Immunol Immunother (2022) 71(10):2405–20. doi: 10.1007/s00262-022-03169-6
12. Park EM, Chelvanambi M, Bhutiani N, Kroemer G, Zitvogel L, Wargo JA. Targeting the gut and tumor microbiota in cancer. Nat Med (2022) 28(4):690–703. doi: 10.1038/s41591-022-01779-2
13. Franzin R, Netti GS, Spadaccino F, Porta C, Gesualdo L, Stallone G, et al. The use of immune checkpoint inhibitors in oncology and the occurrence of AKI: where do we stand? Front Immunol (2020) 11:574271. doi: 10.3389/fimmu.2020.574271
14. Passaro A, Brahmer J, Antonia S, Mok T, Peters S. Managing resistance to immune checkpoint inhibitors in lung cancer: treatment and novel strategies. J Clin Oncol (2022) 40(6):598–+. doi: 10.1200/jco.21.01845
15. Hakozaki T, Richard C, Elkrief A, Hosomi Y, Benlaifaoui M, Mimpen I, et al. The gut microbiome associates with immune checkpoint inhibition outcomes in patients with advanced non-small cell lung cancer. Cancer Immunol Res (2020) 8(10):1243–50. doi: 10.1158/2326-6066.Cir-20-0196
16. Kumar P, Brazel D, DeRogatis J, Valerin JBG, Whiteson K, Chow WA, et al. The cure from within? a review of the microbiome and diet in melanoma. Cancer Metastasis Rev (2022) 41(2):261–80. doi: 10.1007/s10555-022-10029-3
17. Gill SR, Pop M, DeBoy RT, Eckburg PB, Turnbaugh PJ, Samuel BS, et al. Metagenomic analysis of the human distal gut microbiome. Science (2006) 312(5778):1355–9. doi: 10.1126/science.1124234
18. Eckburg PB, Bik EM, Bernstein CN, Purdom E, Dethlefsen L, Sargent M, et al. Diversity of the human intestinal microbial flora. Science (2005) 308(5728):1635–8. doi: 10.1126/science.1110591
19. Tremaroli V, Backhed F. Functional interactions between the gut microbiota and host metabolism. Nature (2012) 489(7415):242–9. doi: 10.1038/nature11552
20. Zhou C-B, Zhou Y-L, Fang J-Y. Gut microbiota in cancer immune response and immunotherapy. Trends Cancer (2021) 7(7):647–60. doi: 10.1016/j.trecan.2021.01.010
21. Jenkins RW, Barbie DA, Flaherty KT. Mechanisms of resistance to immune checkpoint inhibitors. Br J Cancer (2018) 118(1):9–16. doi: 10.1038/bjc.2017.434
22. Zhang C, Wang J, Sun Z, Cao Y, Mu Z, Ji X. Commensal microbiota contributes to predicting the response to immune checkpoint inhibitors in non-small-cell lung cancer patients. Cancer Sci (2021) 112(8):3005–17. doi: 10.1111/cas.14979
23. Lu Y, Yuan X, Wang M, He Z, Li H, Wang J, et al. Gut microbiota influence immunotherapy responses: mechanisms and therapeutic strategies. J Hematol Oncol (2022) 15(1):47. doi: 10.1186/s13045-022-01273-9
24. Newsome RC, Gharaibeh RZ, Pierce CM, da Silva WV, Paul S, Hogue SR, et al. Interaction of bacterial genera associated with therapeutic response to immune checkpoint PD-1 blockade in a United States cohort. Genome Med (2022) 14(1):35. doi: 10.1186/s13073-022-01037-7
25. Lee S-H, Cho S-Y, Yoon Y, Park C, Sohn J, Jeong J-J, et al. Bifidobacterium bifidum strains synergize with immune checkpoint inhibitors to reduce tumour burden in mice. Nat Microbiol (2021) 6(3):277–+. doi: 10.1038/s41564-020-00831-6
26. Routy B, Le Chatelier E, Derosa L, Duong CPM, Alou MT, Daillere R, et al. Gut microbiome influences efficacy of PD-1-based immunotherapy against epithelial tumors. Science (2018) 359(6371):91–+. doi: 10.1126/science.aan3706
27. Hall LJ, Robinson SD. Bacterial strains augment cancer therapeutics. Nat Microbiol (2021) 6(3):275–6. doi: 10.1038/s41564-021-00871-6
28. Sivan A, Corrales L, Hubert N, Williams JB, Aquino-Michaels K, Earley ZM, et al. Commensal Bifidobacterium promotes antitumor immunity and facilitates anti-PD-L1 efficacy. Science (2015) 350(6264):1084–9. doi: 10.1126/science.aac4255
29. Vetizou M, Pitt JM, Daillere R, Lepage P, Waldschmitt N, Flament C, et al. Anticancer immunotherapy by CTLA-4 blockade relies on the gut microbiota. Science (2015) 350(6264):1079–+. doi: 10.1126/science.aad1329
30. Griffin ME, Espinosa J, Becker JL, Luo JD, Caroll TS, Jha JK, et al. Enterococcus peptidoglycan remodeling promotes checkpoint inhibitor cancer immunotherapy. Science (2021) 373(6558):1040–6. doi: 10.1126/science.abc9113
31. Mager LF, Burkhard R, Pett N, Cooke NCA, Brown K, Ramay H, et al. Microbiome-derived inosine modulates response to checkpoint inhibitor immunotherapy. Science (2020) 369(6510):1481–+. doi: 10.1126/science.abc3421
32. Di Modica M, Gargari G, Regondi V, Bonizzi A, Arioli S, Belmonte B, et al. Gut microbiota condition the therapeutic efficacy of trastuzumab in HER2-positive breast cancer. Cancer Res (2021) 81(8):2195–206. doi: 10.1158/0008-5472.Can-20-1659
33. Derosa L, Routy B, Fidelle M, Iebba C, Alla L, Pasolli E, et al . Gut bacteria composition drives primary resistance to cancer immunotherapy in renal cell carcinoma patients. Eur Urol (2020) 78(2):195–206. doi: 10.1016/j.eururo.2020.04.044
34. Lee B, Lee J, Woo M-Y, Lee MJ, Shin H-J, Kim K, et al. Modulation of the gut microbiota alters the tumour-suppressive efficacy of tim-3 pathway blockade in a bacterial species- and host factor-dependent manner. Microorganisms (2020) 8(9):1395. doi: 10.3390/microorganisms8091395
35. Blake SJ, James J, Ryan FJ, Caparros-Martin J, Eden GL, Tee YC, et al. The immunotoxicity, but not anti-tumor efficacy, of anti-CD40 and anti-CD137 immunotherapies is dependent on the gut microbiota. Cell Rep Med (2021) 2(12):100464. doi: 10.1016/j.xcrm.2021.100464
36. Elinav E, Garrett WS, Trinchieri G, Wargo J. The cancer microbiome. Nat Rev Cancer (2019) 19(7):371–6. doi: 10.1038/s41568-019-0155-3
37. Grenda A, Iwan E, Krawczyk P, Frak M, Chmielewska I, Bomba A, et al. Attempting to identify bacterial allies in immunotherapy of NSCLC patients. Cancers (2022) 14(24):6250. doi: 10.3390/cancers14246250
38. Hakozaki T, Nolin-Lapalme A, Kogawa M, Okuma Y, Nakamura S, Moreau-Amaru D, et al. Cancer cachexia among patients with advanced non-small-cell lung cancer on immunotherapy: an observational study with exploratory gut microbiota analysis. Cancers (2022) 14(21):5405. doi: 10.3390/cancers14215405
39. Derosa L, Routy B, Thomas AM, Iebba V, Zalcman G, Friard S, et al. Intestinal Akkermansia muciniphila predicts clinical response to PD-1 blockade in patients with advanced non-small-cell lung cancer. Nat Med (2022) 28(2):315–+. doi: 10.1038/s41591-021-01655-5
40. Huang J, Liu D, Wang Y, Liu L, Li J, Yuan J, et al. Ginseng polysaccharides alter the gut microbiota and kynurenine/tryptophan ratio, potentiating the antitumour effect of antiprogrammed cell death 1/programmed cell death ligand 1 (anti-PD-1/PD-L1) immunotherapy. Gut (2022) 71(4):734–45. doi: 10.1136/gutjnl-2020-321031
41. He D, Li X, An R, Wang L, Wang Y, Zheng S, et al. Response to PD-1-based immunotherapy for non-small cell lung cancer altered by gut microbiota. Oncol Ther (2021) 9(2):647–57. doi: 10.1007/s40487-021-00171-3
42. Song P, Yang D, Wang H, Cui X, Si X, Zhang X, et al. Relationship between intestinal flora structure and metabolite analysis and immunotherapy efficacy in Chinese NSCLC patients. Thorac Cancer (2020) 11(6):1621–32. doi: 10.1111/1759-7714.13442
43. Jin Y, Dong H, Xia L, Yang Y, Zhu Y, Shen Y, et al. The diversity of gut microbiome is associated with favorable responses to anti-programmed death 1 immunotherapy in chinese patients with NSCLC. J Thorac Oncol (2019) 14(8):1378–89. doi: 10.1016/j.jtho.2019.04.007
44. Romeo M, Iadanza G, Martini G, Ciardiello D, Cipullo M, Ventriglia L, et al. GUT MICROBIOTA CORRELATES WITH ANTITUMOR ACTIVITY IN PATIENTS WITH MCRC AND NSCLC TREATED WITH CETUXIMAB PLUS AVELUMAB. Digest Liver Dis (2023) 55:S79–80. doi: 10.1016/S1590-8658(23)00236-0
45. Li Y, Wang K, Zhang Y, Yang J, Wu Y, Zhao M. Revealing a causal relationship between gut microbiota and lung cancer: a Mendelian randomization study. Front Cell Infect Microbiol (2023) 13:1200299. doi: 10.3389/fcimb.2023.1200299
46. Oster P, Vaillant L, Riva E, McMillan B, Begka C, Truntzer C, et al. Helicobacter pylori infection has a detrimental impact on the efficacy of cancer immunotherapies. Gut (2022) 71(3):457–66. doi: 10.1136/gutjnl-2020-323392
47. Nagasaka M, Sexton R, Alhasan R, Rahman S, Asfar AS, Sukari A. Gut microbiome and response to checkpoint inhibitors in non-small cell lung cancer-A review. Crit Rev Oncol Hematol (2020) 145:102841. doi: 10.1016/j.critrevonc.2019.102841
48. Shankar B, Zhang J, Naqash AR, Forde PM, Feliciano JL, Marrone KA, et al. Multisystem immune-related adverse events associated with immune checkpoint inhibitors for treatment of non-small cell lung cancer. JAMA Oncol (2020) 6(12):1952–6. doi: 10.1001/jamaoncol.2020.5012
49. Yin J, Wu Y, Yang X, Gan L, Xue J. Checkpoint inhibitor pneumonitis induced by anti-PD-1/PD-L1 therapy in non-small-cell lung cancer: occurrence and mechanism. Front Immunol (2022) 13:830631. doi: 10.3389/fimmu.2022.830631
50. Tang L, Wang J, Lin N, Zhou Y, He W, Liu J, et al. Immune checkpoint inhibitor-associated colitis: from mechanism to management. Front Immunol (2021) 12:800879. doi: 10.3389/fimmu.2021.800879
51. Khan S, Gerber DE. Autoimmunity, checkpoint inhibitor therapy and immune-related adverse events: A review. Semin Cancer Biol (2020) 64:93–101. doi: 10.1016/j.semcancer.2019.06.012
52. Chau J, Yadav M, Liu B, Furqan M, Dai Q, Shahi S, et al. Prospective correlation between the patient microbiome with response to and development of immune-mediated adverse effects to immunotherapy in lung cancer. BMC Cancer (2021) 21(1):808. doi: 10.1186/s12885-021-08530-z
53. Liu X, Tang H, Zhou Q, Zeng Y, Lu B, Chen D, et al. Gut microbiota composition in patients with advanced Malignancies experiencing immune-related adverse events. Front Immunol (2023) 14:1109281. doi: 10.3389/fimmu.2023.1109281
54. Zeng Y, Shi Q, Liu X, Tang H, Lu B, Zhou Q, et al. Dynamic gut microbiota changes in patients with advanced Malignancies experiencing secondary resistance to immune checkpoint inhibitors and immune-related adverse events. Front Oncol (2023) 13:1144534. doi: 10.3389/fonc.2023.1144534
55. Thompson NA, Stewart GD, Welsh SJ, Doherty GJ, Robinson MJ, Neville BA, et al. The MITRE trial protocol: a study to evaluate the microbiome as a biomarker of efficacy and toxicity in cancer patients receiving immune checkpoint inhibitor therapy. BMC Cancer (2022) 22(1):99. doi: 10.1186/s12885-021-09156-x
56. Andrews MC, Duong CPM, Gopalakrishnan V, Iebba V, Chen W-S, Derosa L, et al. Gut microbiota signatures are associated with toxicity to combined CTLA-4 and PD-1 blockade. Nat Med (2021) 27(8):1432–+. doi: 10.1038/s41591-021-01406-6
57. McCulloch JA, Davar D, Rodrigues RR, Badger JH, Fang JR, Cole AM, et al. Intestinal microbiota signatures of clinical response and immune-related adverse events in melanoma patients treated with anti-PD-1. Nat Med (2022) 28(3):545–+. doi: 10.1038/s41591-022-01698-2
58. Mao J, Wang D, Long J, Yang X, Lin J, Song Y, et al. Gut microbiome is associated with the clinical response to anti-PD-1 based immunotherapy in hepatobiliary cancers. J Immunother Cancer (2021) 9(12):e003334. doi: 10.1136/jitc-2021-003334
59. Tan B, Chen M-j, Guo Q, Tang H, Li Y, Jia X-m, et al. Clinical-radiological characteristics and intestinal microbiota in patients with pancreatic immune-related adverse events. Thorac Cancer (2021) 12(12):1814–23. doi: 10.1111/1759-7714.13990
60. Cheng T-Y, Chang C-C, Luo C-S, Chen K-Y, Yeh Y-K, Zheng J-Q, et al. Targeting lung-gut axis for regulating pollution particle-mediated inflammation and metabolic disorders. Cells (2023) 12(6):901. doi: 10.3390/cells12060901
61. Georgiou K, Marinov B, Farooqi AA, Gazouli M. Gut microbiota in lung cancer: where do we stand? Int J Mol Sci (2021) 22(19):10429. doi: 10.3390/ijms221910429
62. Zorn AM, Wells JM. Vertebrate endoderm development and organ formation. Annu Rev Cell Dev Biol (2009) 25:221–51. doi: 10.1146/annurev.cellbio.042308.113344
63. Budden KF, Gellatly SL, Wood DLA, Cooper MA, Morrison M, Hugenholtz P, et al. Emerging pathogenic links between microbiota and the gut-lung axis. Nat Rev Microbiol (2017) 15(1):55–63. doi: 10.1038/nrmicro.2016.142
64. Tulic MK, Piche T, Verhasselt V. Lung-gut cross-talk: evidence, mechanisms and implications for themucosal inflammatory diseases. Clin Exp Allergy (2016) 46(4):519–28. doi: 10.1111/cea.12723
65. Barcik W, Boutin RCT, Sokolowska M, Finlay BB. The role of lung and gut microbiota in the pathology of asthma. Immunity (2020) 52(2):241–55. doi: 10.1016/j.immuni.2020.01.007
66. Hufnagl K, Pali-Schoell I, Roth-Walter F, Jensen-Jarolim E. Dysbiosis of the gut and lung microbiome has a role in asthma. Semin Immunopathol (2020) 42(1):75–93. doi: 10.1007/s00281-019-00775-y
67. He Y, Wen Q, Yao F, Xu D, Huang Y, Wang J. Gut-lung axis: The microbial contributions and clinical implications. Crit Rev Microbiol (2017) 43(1):81–95. doi: 10.1080/1040841x.2016.1176988
68. Huang Y, Mao K, Chen X, M.-a. Sun T, Li W, Usher N, et al. S1P-dependent interorgan trafficking of group 2 innate lymphoid cells supports host defense. Science (2018) 359(6371):114–9. doi: 10.1126/science.aam5809
69. Ruane D, Brane L, Reis BS, Cheong C, Poles J, Do Y, et al. Lung dendritic cells induce migration of protective T cells to the gastrointestinal tract. J Exp Med (2013) 210(9):1871–88. doi: 10.1084/jem.20122762
70. Southam DS, Dolovich M, O’Bryne PM, Inman MD. Distribution of intranasal instillations in mice: effects of volume, time, body position, and anesthesia. Am J Physiology-Lung Cell Mol Physiol (2002) 282(4):L833–9. doi: 10.1152/ajplung.00173.2001
71. Bienenstock J, McDermott M, Befus D, O’Neill M. A common mucosal immunologic system involving the bronchus, breast and bowel. Adv Exp Med Biol (1978) 107:53–9. doi: 10.1007/978-1-4684-3369-2_7
72. Koboziev I, Karlsson F, Grisham MB. Gut-associated lymphoid tissue, T cell trafficking, and chronic intestinal inflammation. Ann N Y Acad Sci (2010) 1207(Suppl 1):E86–93. doi: 10.1111/j.1749-6632.2010.05711.x
73. Hwang JY, Randall TD, Silva-Sanchez A. Inducible bronchus-associated lymphoid tissue: taming inflammation in the lung. Front Immunol (2016) 7:258. doi: 10.3389/fimmu.2016.00258
74. Derosa L, Routy B, Desilets A, Daillere R, Terrisse S, Kroemer G, et al. Microbiota-centered interventions: the next breakthrough in immuno-oncology? Cancer Discovery (2021) 11(10):2396–412. doi: 10.1158/2159-8290.Cd-21-0236
75. Vilela de Oliveira GL, Simao Oliveira CN, Pinzan CF, Vilela de Salis LV, de Barros Cardoso CR. Microbiota modulation of the gut-lung axis in COVID-19. Front Immunol (2021) 12:635471. doi: 10.3389/fimmu.2021.635471
76. Mirzaei R, Afaghi A, Babakhani S, Sohrabi MR, Hosseini-Fard SR, Babolhavaeji K, et al. Role of microbiota-derived short-chain fatty acids in cancer development and prevention. Biomed Pharmacother (2021) 139:111619 doi: 10.1016/j.biopha.2021.111619
77. Fidelle M, Rauber C, Alves Costa Silva C, Tian A-L, Lahmar I, de la Varende A-LM, et al. A microbiota-modulated checkpoint directs immunosuppressive intestinal T cells into cancers. Sci (New York N.Y.) (2023) 380(6649):eabo2296–eabo2296. doi: 10.1126/science.abo2296
78. Chelvanambi M, Wargo JA. MAdCAM-1: a newly identified microbial ‘gut check’ for T cells. Trends Immunol (2023) 44(8):568–70. doi: 10.1016/j.it.2023.06.007
79. Chen D, Wu J, Jin D, Wang B, Cao H. Fecal microbiota transplantation in cancer management: Current status and perspectives. Int J Cancer (2019) 145(8):2021–31. doi: 10.1002/ijc.32003
80. Eiseman B, Silen W, Bascom GS, Kauvar AJ. Fecal enema as an adjunct in the treatment of pseudomembranous enterocolitis. Surgery (1958) 44(5):854–9.
81. Ooijevaar RE, Terveer EM, Verspaget HW, Kuijper EJ, Keller JJ. Clinical application and potential of fecal microbiota transplantation. In: Klotman ME, editor. Annual review of medicine. California, USA: Annual reviews. (2019). 70:335–51. doi: 10.1146/annurev-med-111717-122956
82. Davar D, Dzutsev AK, McCulloch JA, Rodrigues RR, Chauvin J-M, Morrison RM, et al. Fecal microbiota transplant overcomes resistance to anti-PD-1 therapy in melanoma patients. Science (2021) 371(6529):595–+. doi: 10.1126/science.abf3363
83. Baruch EN, Youngster I, Ben-Betzalel G, Ortenberg R, Lahat A, Katz L, et al. Fecal microbiota transplant promotes response in immunotherapy-refractory melanoma patients. Science (2021) 371(6529):602–+. doi: 10.1126/science.abb5920
84. Reinshagen M, Stallmach A. Multidonor intensive faecal microbiota transplantation for active ulcerative colitis: a randomised placebo-controlled trial. Z Fur Gastroenterol (2017) 55(8):779–80. doi: 10.1055/s-0043-103830
85. Kragsnaes MS, Kjeldsen J, Horn HC, Munk HL, Pedersen JK, Just SA, et al. Safety and efficacy of faecal microbiota transplantation for active peripheral psoriatic arthritis: an exploratory randomised placebo-controlled trial. Ann Rheumatic Dis (2021) 80(9):1158–67. doi: 10.1136/annrheumdis-2020-219511
86. Cammarota G, Ianiro G, Tilg H, Rajilic-Stojanovic M, Kump P, Satokari R, et al. European consensus conference on faecal microbiota transplantation in clinical practice. Gut (2017) 66(4):569–80. doi: 10.1136/gutjnl-2016-313017
87. Ianiro G, Tilg H, Gasbarrini A. Antibiotics as deep modulators of gut microbiota: between good and evil. Gut (2016) 65(11):1906–15. doi: 10.1136/gutjnl-2016-312297
88. Karakan T, Ozkul C, Kupeli Akkol E, Bilici S, Sobarzo-Sanchez E, Capasso R. Gut-brain-microbiota axis: antibiotics and functional gastrointestinal disorders. Nutrients (2021) 13(2):389. doi: 10.3390/nu13020389
89. Manichanh C, Reeder J, Gibert P, Varela E, Llopis M, Antolin M, et al. Reshaping the gut microbiome with bacterial transplantation and antibiotic intake. Genome Res (2010) 20(10):1411–9. doi: 10.1101/gr.107987.110
90. Bender MJ, McPherson AC, Phelps CM, Pandey SP, Laughlin CR, Shapira JH, et al. Dietary tryptophan metabolite released by intratumoral Lactobacillus reuteri facilitates immune checkpoint inhibitor treatment. Cell (2023) 186(9):1846–+. doi: 10.1016/j.cell.2023.03.011
91. Chalabi M, Cardona A, Nagarkar DR, Scala AD, Gandara DR, Rittmeyer A, et al. Efficacy of chemotherapy and atezolizumab in patients with non-small-cell lung cancer receiving antibiotics and proton pump inhibitors: pooled post hoc analyses of the OAK and POPLAR trials. Ann Oncol (2020) 31(4):525–31. doi: 10.1016/j.annoc.2020.01.006
92. Cortellini A, Tucci M, Adamo V, Stucci LS, Russo A, Tanda ET, et al. Integrated analysis of concomitant medications and oncological outcomes from PD-1/PD-L1 checkpoint inhibitors in clinical practice. J Immunother Cancer (2020) 8(2):e001361. doi: 10.1136/jitc-2020-001361
93. Derosa L, Hellmann MD, Spaziano M, Halpenny D, Fidelle M, Rizvi H, et al. Negative association of antibiotics on clinical activity of immune checkpoint inhibitors in patients with advanced renal cell and non-small-cell lung cancer. Ann Oncol (2018) 29(6):1437–44. doi: 10.1093/annonc/mdy103
94. Castello A, Rossi S, Toschi L, Lopci E. Impact of antibiotic therapy and metabolic parameters in non-small cell lung cancer patients receiving checkpoint inhibitors. J Clin Med (2021) 10(6):1251. doi: 10.3390/jcm10061251
95. Tinsley N, Graham DM, Cook N. Cumulative antibiotic use significantly decreases efficacy of checkpoint inhibitors in patients with advanced cancer-reply to the editors. Ann Trans Med (2020) 8(4):139. doi: 10.21037/atm.2019.12.06
96. Fang C, Fang W, Xu L, Gao F, Hou Y, Zou H, et al. Distinct functional metagenomic markers predict the responsiveness to anti-PD-1 therapy in chinese non-small cell lung cancer patients. Front Oncol (2022) 12:837525. doi: 10.3389/fonc.2022.837525
97. Iida N, Dzutsev A, Stewart CA, Smith L, Bouladoux N, Weingarten RA, et al. Commensal bacteria control cancer response to therapy by modulating the tumor microenvironment. Science (2013) 342(6161):967–70. doi: 10.1126/science.1240527
98. Ouaknine Krief J, Helly de Tauriers P, Dumenil C, Neveux N, Dumoulin J, Giraud V, et al. Role of antibiotic use, plasma citrulline and blood microbiome in advanced non-small cell lung cancer patients treated with nivolumab. J Immunother Cancer (2019) 7(1):176–6. doi: 10.1186/s40425-019-0658-1
99. Lurienne L, Cervesi J, Duhalde L, de Gunzburg J, Andremont A, Zalcman G, et al. NSCLC immunotherapy efficacy and antibiotic use: A systematic review and meta-analysis. J Thorac Oncol (2020) 15(7):1147–59. doi: 10.1016/j.jtho.2020.03.002
100. Galli G, Triulzi T, Proto C, Signorelli D, Imbimbo M, Poggi M, et al. Association between antibiotic-immunotherapy exposure ratio and outcome in metastatic non small cell lung cancer. Lung Cancer (2019) 132:72–8. doi: 10.1016/j.lungcan.2019.04.008
101. Ochi N, Ichihara E, Takigawa N, Harada D, Inoue K, Shibayama T, et al. The effects of antibiotics on the efficacy of immune checkpoint inhibitors in patients with non-small-cell lung cancer differ based on PD-L1 expression. Eur J Cancer (2021) 149:73–81. doi: 10.1016/j.ejca.2021.02.040
102. Alkan G, Oztas NS, Turna ZH, Ozguroglu M. Effect of antibiotic treatment on immune checkpoint inhibitor efficacy in patients with advanced non-small cell lung cancer. Ann Oncol (2022) 33(7):S1082–2. doi: 10.1016/j.annonc.2022.07.1291
103. Lopes S, Pabst L, Dory A, Klotz M, Gourieux B, Michel B, et al. Do proton pump inhibitors alter the response to immune checkpoint inhibitors in cancer patients? A meta-analysis. Front Immunol (2023) 14:1070076. doi: 10.3389/fimmu.2023.1070076
104. Lee KA, Shaw HM, Bataille V, Nathan P, Spector TD. Role of the gut microbiome for cancer patients receiving immunotherapy: Dietary and treatment implications. Eur J Cancer (2020) 138:149–55. doi: 10.1016/j.ejca.2020.07.026
105. Tsai Y-L, Lin T-L, Chang C-J, Wu T-R, Lai W-F, Lu C-C, et al. Probiotics, prebiotics and amelioration of diseases. J Biomed Sci (2019) 26(1):3. doi: 10.1186/s12929-018-0493-6
106. Hill C, Guarner F, Reid G, Gibson GR, Merenstein DJ, Pot B, et al. The International Scientific Association for Probiotics and Prebiotics consensus statement on the scope and appropriate use of the term probiotic. Nat Rev Gastroenterol Hepatol (2014) 11(8):506–14. doi: 10.1038/nrgastro.2014.66
107. Schwartz DJ, Rebeck ON, Dantas G. Complex interactions between the microbiome and cancer immune therapy. Crit Rev Clin Lab Sci (2019) 56(8):567–85. doi: 10.1080/10408363.2019.1660303
108. Zolkiewicz J, Marzec A, Ruszczynski M, Feleszko W. Postbiotics-A step beyond pre- and probiotics. Nutrients (2020) 12(8):2189. doi: 10.3390/nu12082189
109. Takada K, Shimokawa M, Takamori S, Shimamatsu S, Hirai F, Tagawa T, et al. Clinical impact of probiotics on the efficacy of anti-PD-1 monotherapy in patients with nonsmall cell lung cancer: A multicenter retrospective survival analysis study with inverse probability of treatment weighting. Int J Cancer (2021) 149(2):473–82. doi: 10.1002/ijc.33557
110. Tomita Y, Ikeda T, Sakata S, Saruwatari K, Sato R, Iyama S, et al. Association of probiotic clostridium butyricum therapy with survival and response to immune checkpoint blockade in patients with lung cancer. Cancer Immunol Res (2020) 8(10):1236–42. doi: 10.1158/2326-6066.Cir-20-0051
111. Messaoudene M, Pidgeon R, Richard C, Ponce M, Diop K, Benlaifaoui M, et al. A natural polyphenol exerts antitumor activity and circumvents anti-PD-1 resistance through effects on the gut microbiota. Cancer Discovery (2022) 12(4):1070–87. doi: 10.1158/2159-8290.Cd-21-0808
112. Renga G, Nunzi E, Pariano M, Puccetti M, Bellet MM, Pieraccini G, et al. Optimizing therapeutic outcomes of immune checkpoint blockade by a microbial tryptophan metabolite. J Immunother Cancer (2022) 10(3):e003725. doi: 10.1136/jitc-2021-003725
113. Montalban-Arques A, Katkeviciute E, Busenhart P, Bircher A, Wirbel J, Zeller G, et al. Commensal Clostridiales strains mediate effective anti-cancer immune response against solid tumors. Cell Host Microbe (2021) 29(10):1573–+. doi: 10.1016/j.chom.2021.08.001
114. Wan L, Wu C, Wu Q, Luo S, Liu J, Xie X. Impact of probiotics use on clinical outcomes of immune checkpoint inhibitors therapy in cancer patients. Cancer Med (2023) 12(2):1841–9. doi: 10.1002/cam4.4994
115. Dizman N, Meza L, Bergerot P, Alcantara M, Dorff T, Lyou Y, et al. Nivolumab plus ipilimumab with or without live bacterial supplementation in metastatic renal cell carcinoma: a randomized phase 1 trial. Nat Med (2022) 28(4):704–+. doi: 10.1038/s41591-022-01694-6
116. Gao G, Ma T, Zhang T, Jin H, Li Y, Kwok L-Y, et al. Adjunctive Probiotic Lactobacillus rhamnosus Probio-M9 Administration Enhances the Effect of Anti-PD-1 Antitumor Therapy via Restoring Antibiotic-Disrupted Gut Microbiota. Front Immunol (2021) 12:772532. doi: 10.3389/fimmu.2021.772532
117. Chen M, Ma L, Yu H, Huang S, Zhang J, Gong J, et al. JK5G postbiotics attenuate immune-related adverse events in NSCLC patients by regulating gut microbiota: a randomized controlled trial in China. Front Oncol (2023) 13:1155592. doi: 10.3389/fonc.2023.1155592
118. Doron S, Snydman DR. Risk and safety of probiotics. Clin Infect Dis (2015) 60:S129–34. doi: 10.1093/cid/civ085
119. Baaziz H, Baker ZR, Franklin HC, Hsu BB. Rehabilitation of a misbehaving microbiome: phages for the remodeling of bacterial composition and function. Iscience (2022) 25(4):104146. doi: 10.1016/j.isci.2022.104146
120. Gorski A, Jonczyk-Matysiak E, Lusiak-Szelachowska M, Miedzybrodzki R, Weber-Dabrowska B, Borysowski J. Bacteriophages targeting intestinal epithelial cells: a potential novel form of immunotherapy. Cell Mol Life Sci (2018) 75(4):589–95. doi: 10.1007/s00018-017-2715-6
121. Fluckiger A, Daillere R, Sassi M, Sixt BS, Liu P, Loos F, et al. Cross-reactivity between tumor MHC class I-restricted antigens and an enterococcal bacteriophage. Science (2020) 369(6506):936–+. doi: 10.1126/science.aax0701
122. Dong X, Pan P, Zheng D-W, Bao P, Zeng X, Zhang X-Z. Bioinorganic hybrid bacteriophage for modulation of intestinal microbiota to remodel tumor-immune microenvironment against colorectal cancer. Sci Adv (2020) 6(20):eaba1590. doi: 10.1126/sciadv.aba1590
123. Jaspers E. “You are, what You eat” Man and Microbe - a Recipe for Success. Deutsche Lebensmittel-Rundschau (2015) 111(11):465–70.
124. Gentile CL, Weir TL. The gut microbiota at the intersection of diet and human health. Science (2018) 362(6416):776–80. doi: 10.1126/science.aau5812
125. Spencer CN, McQuade JL, Gopalakrishnan V, McCulloch JA, Vetizou M, Cogdill AP, et al. Dietary fiber and probiotics influence the gut microbiome and melanoma immunotherapy response. Science (2021) 374(6575):1632–+. doi: 10.1126/science.aaz7015
126. Trompette A, Gollwitzer ES, Yadava K, Sichelstiel AK, Sprenger N, Ngom-Bru C, et al. Gut microbiota metabolism of dietary fiber influences allergic airway disease and hematopoiesis. Nat Med (2014) 20(2):159–66. doi: 10.1038/nm.3444
127. Nencioni A, Caffa I, Cortellino S, Longo VD. Fasting and cancer: molecular mechanisms and clinical application. Nat Rev Cancer (2018) 18(11):707–19. doi: 10.1038/s41568-018-0061-0
128. Ringel AE, Drijvers JM, Baker GJ, Catozzi A, Garcia-Canaveras JC, Gassaway BM, et al. Obesity shapes metabolism in the tumor microenvironment to suppress anti-tumor immunity. Cell (2020) 183(7):1848–+. doi: 10.1016/j.cell.2020.11.009
129. Ferrere G, Alou MT, Liu P, Goubet A-G, Fidelle M, Kepp O, et al. Ketogenic diet and ketone bodies enhance the anticancer effects of PD-1 blockade. JCI Insight (2021) 6(2):e145207. doi: 10.1172/jci.insight.145207
130. Vernieri C, Fuca G, Ligorio F, Huber V, Vingiani A, Iannelli F, et al. Fasting- mimicking diet is safe and reshapes metabolism and antitumor immunity in patients with cancer. Cancer Discovery (2022) 12(1):90–107. doi: 10.1158/2159-8290.Cd-21-0030
131. Dai X, Bu X, Gao Y, Guo J, Hu J, Jiang C, et al. Energy status dictates PD-L1 protein abundance and anti-tumor to enable blockade. Mol Cell (2021) 81(11):2317–+. doi: 10.1016/j.molcel.2021.03.037
132. Shah H, Ng TL. A narrative review from gut to lungs: non-small cell lung cancer and the gastrointestinal microbiome. Trans Lung Cancer Res (2023) 12(4):909–26. doi: 10.21037/tlcr-22-595
133. Rocco D, Della Gravara L, Ragone A, Sapio L, Naviglio S, Gridelli C. Prognostic factors in advanced non-small cell lung cancer patients treated with immunotherapy. Cancers (2023) 15(19):4684. doi: 10.3390/cancers15194684
134. Ni B, Kong X, Yan Y, Fu B, Zhou F, Xu S. Combined analysis of gut microbiome and serum metabolomics reveals novel biomarkers in patients with early-stage non-small cell lung cancer. Front Cell Infect Microbiol (2023) 13:1091825. doi: 10.3389/fcimb.2023.1091825
135. Zhu X, Li K, Liu G, Wu R, Zhang Y, Wang S, et al. Microbial metabolite butyrate promotes anti-PD-1 antitumor efficacy by modulating T cell receptor signaling of cytotoxic CD8 T cell. Gut Microbes (2023) 15(2):2249143. doi: 10.1080/19490976.2023.2249143
Keywords: gut microbiota, immunotherapy, gut-lung axis, non-small cell lung cancer, fecal microbiota transplant, immune checkpoint inhibitors
Citation: Zhang H and Xu Z (2023) Gut–lung axis: role of the gut microbiota in non-small cell lung cancer immunotherapy. Front. Oncol. 13:1257515. doi: 10.3389/fonc.2023.1257515
Received: 12 July 2023; Accepted: 03 November 2023;
Published: 22 November 2023.
Edited by:
Laura Ridolfi, Scientific Institute of Romagna for the Study and Treatment of Tumors (IRCCS), ItalyReviewed by:
Dan Li, National Institutes of Health (NIH), United StatesSajad Karampoor, Iran University of Medical Sciences, Iran
Rasoul Mirzaei, Pasteur Institute of Iran (PII), Iran
Copyright © 2023 Zhang and Xu. This is an open-access article distributed under the terms of the Creative Commons Attribution License (CC BY). The use, distribution or reproduction in other forums is permitted, provided the original author(s) and the copyright owner(s) are credited and that the original publication in this journal is cited, in accordance with accepted academic practice. No use, distribution or reproduction is permitted which does not comply with these terms.
*Correspondence: Huaiyuan Zhang, WmhhbmdodWFpeXVhbjIwMjNAMTI2LmNvbQ==; Ziyuan Xu, eHV6aXl1YW5Abmp1Y20uZWR1LmNu