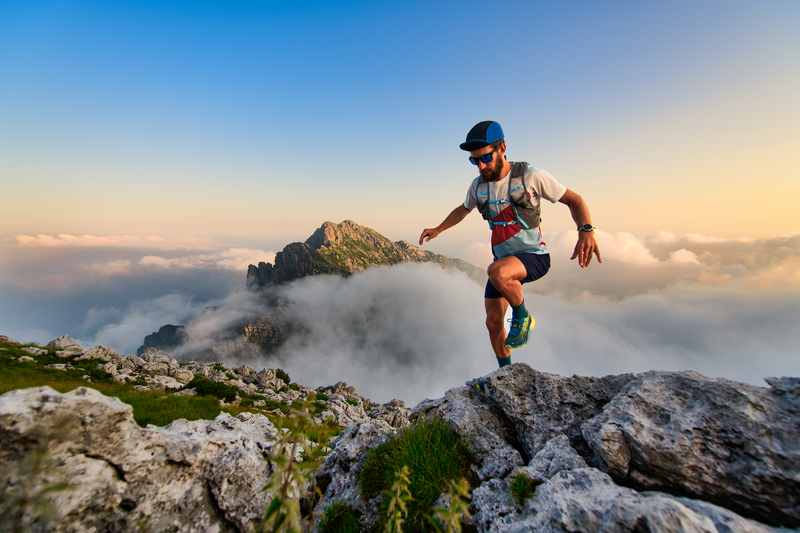
95% of researchers rate our articles as excellent or good
Learn more about the work of our research integrity team to safeguard the quality of each article we publish.
Find out more
REVIEW article
Front. Oncol. , 22 August 2023
Sec. Cancer Metabolism
Volume 13 - 2023 | https://doi.org/10.3389/fonc.2023.1249237
This article is part of the Research Topic Unmasking metabolic dependencies for anti-cancer treatment in cancer View all 11 articles
Currently, immune checkpoint inhibitors (ICIs) are widely considered the standard initial treatment for advanced non-small cell lung cancer (NSCLC) when there are no targetable driver oncogenic alternations. NSCLC tumors that have two alterations in tumor suppressor genes, such as liver kinase B1 (LKB1) and/or Kelch-like ECH-associated protein 1 (KEAP1), have been found to exhibit reduced responsiveness to these therapeutic strategies, as revealed by multiomics analyses identifying immunosuppressed phenotypes. Recent advancements in various biological approaches have gradually unveiled the molecular mechanisms underlying intrinsic reprogrammed metabolism in tumor cells, which contribute to the evasion of immune responses by the tumor. Notably, metabolic alterations in glycolysis and glutaminolysis have a significant impact on tumor aggressiveness and the remodeling of the tumor microenvironment. Since glucose and glutamine are essential for the proliferation and activation of effector T cells, heightened consumption of these nutrients by tumor cells results in immunosuppression and resistance to ICI therapies. This review provides a comprehensive summary of the clinical efficacies of current therapeutic strategies against NSCLC harboring LKB1 and/or KEAP1 mutations, along with the metabolic alterations in glycolysis and glutaminolysis observed in these cancer cells. Furthermore, ongoing trials targeting these metabolic alterations are discussed as potential approaches to overcome the extremely poor prognosis associated with this type of cancer.
The advent of immune checkpoint inhibitor (ICI) therapy has revolutionized the treatment approach for various cancers, including advanced non-small cell lung cancer (NSCLC). Currently, the standard first-line therapy for advanced NSCLC without targetable driver oncogenic alternations consists of multiple treatment regimens involving ICIs, either alone or in combination with platinum-based chemotherapy (1–8). Predictors such as programmed death 1 ligand-1 (PD-L1) tumor proportion scores (TPS) or tumor mutational burden (TMB) are available but insufficient in accurately forecasting the treatment outcome (9–11). In first-line therapies for advanced NSCLC, ICIs as monotherapy, such as pembrolizumab and atezolizumab, have demonstrated clinical benefits primarily in patients with high tumor PD-L1 expression (2, 3). However, several combinations of ICIs and platinum-based chemotherapies have been approved as standard first-line therapies irrespective of TPS, although the effectiveness of these combinations still relies to some extent on the tumor PD-L1 expression status. Nonetheless, even among the subset of patients with high tumor PD-L1 expression, approximately 20–30% initially exhibit resistance to ICIs, either alone or in conjunction with platinum-based chemotherapy (1, 9).
Recent multiomics analyses, including next-generation sequencing-based tests (NGS), have played a crucial role in identifying predictive biomarkers for ICI therapies and uncovering mechanisms of immune evasion in cancer (9, 12–15). Among them, T cell–inflamed gene expression profile and proteogenomic characterization in addition to NGS data analysis have revealed that specific driver mutations in NSCLC exhibit discrete immune phenotypes (16, 17). Notably, two tumor suppressor genes, liver kinase B1 (LKB1) and Kelch-like ECH-associated protein 1 (KEAP1), are associated with inactivating driver mutations that contribute to an immunosuppressed phenotype (18). Somatic mutations in LKB1, encoded by serine/threonine kinase 11 (STK11), occur in approximately 20-25% of lung adenocarcinoma (LUAD), while inactivating mutations in KEAP1 are observed in approximately 10-15% of LUAD (19–21). Several studies using a large number of clinical specimens have also reported a high frequency of co-occurring mutations in STK11 and KEAP1 (22–24). NSCLC with STK11 and/or KEAP1 mutations represents one of most aggressive types of cancer, characterized by resistance to standard cytotoxic chemotherapy or radiotherapy (20, 25–27). However, these tumors also exhibit reduced efficacy to immunotherapy, independent of PD-L1 expression and high TMB (18, 28, 29). This highlights the urgent need for novel therapeutic strategies to effectively treat NSCLC patients with these specific mutations. T-cell infiltration in tumors is known to be relatively weak, and researchers have investigated various factors that contribute to this, such as the secretion of immunosuppressive molecules and impairment of antigen presentation (17, 18). Among these factors, the metabolic reprogramming of glycolysis and glutaminolysis in tumor cells has emerged as a current area of focus for explaining the limited response to immunotherapy (30, 31). The intrinsic metabolic reprogramming of tumor cells, which is essential for tumor growth, also impacts various cells within the tumor microenvironment (TME), leading to immune evasion by the tumor (30–34).
To understand how the inactivation of the two tumor suppressors leads to metabolic reprogramming of glycolysis and glutaminolysis, researchers have gradually uncovered the molecular mechanisms through various biological approaches. These metabolic alterations play a significant role in promoting tumor aggressiveness and reconstructing the TME to support tumor growth (31, 35, 36). In this review, we provide a summary of the current therapeutic strategies and their clinical efficacies against NSCLC with LKB1 and/or KEAP1 inactivation. Furthermore, we delve into the metabolic alterations of glycolysis and glutaminolysis in NSCLC with these mutations, which are associated with ICI resistance, and discuss ongoing trials that target metabolic alterations.
PD-L1 expression on cancer cells is regulated by various mechanisms, including inflammatory cytokines, mechanical signals, and tumor-intrinsic cell signaling. Consequently, there is heterogeneity in the PD-L1 expression levels across tumors (37–40), making them imperfect markers for predicting the response to ICIs. However, during the clinical development of anti-PD-1/PD-L1 antibodies, tumor PD-L1 expression status was used for patient selection based on the observed association between the objective response rate of anti-PD-1 antibody, pembrolizumab, and tumor PD-L1 expression level (41). The KEYNOTE-010 study demonstrated the durable response of pembrolizumab in patients with high tumor PD-L1 expression, leading to subsequent KEYNOTE-024 trial that compared pembrolizumab monotherapy with platinum-based chemotherapy specifically in patients with high tumor PD-L1 expression (2, 42). In these trials, which selected patients based on tumor PD-L1 expression status, the anti-PD-1 antibody showed superior survival outcomes compared to platinum-based chemotherapy, and subsequently, the anti-PD-L1 antibody atezolizumab also demonstrated overall superiority over platinum-based chemotherapy (3, 43) (Figures 1A, B; Supplementary Table 1). Several phase III studies have investigated the clinical efficacy of combining anti-PD-1/PD-L1 antibodies with platinum-based chemotherapy, irrespective of tumor PD-L1 expression, in comparison to platinum-based cytotoxic chemotherapy. These studies, namely, KEYNOTE-189, IMpower150, IMpower130, IMpower132, and KEYNOTE-407, have now become standard first-line options (4, 5, 44–49) (Figures 1A, B; Supplementary Table 1). In addition, the combination of anti-PD-1 antibody and anti-cytotoxic T-lymphocyte-associated protein 4 (CTLA-4) antibody has also demonstrated similar survival superiority. CheckMate 227 and CheckMate 9LA trials showed that the clinical benefits of nivolumab plus ipilimumab and nivolumab plus ipilimumab in combination with platinum-based chemotherapy, respectively, surpassed those of platinum-based chemotherapy alone (7, 50–52). Moreover, in the phase III POSEIDON study, the combination of anti-PD-L1 antibody durvalumab and anti-CTLA-4 antibody tremelimumab, along with platinum-based chemotherapy, recently showed positive results in terms of both progression-free survival (PFS) and overall survival (OS) when compared to platinum-based chemotherapy alone (8) (Figures 1A, B; Supplementary Table 1). These combination regimens involving ICIs have emerged as the leading options for standard first-line therapy in advanced NSCLC cases without targetable drive alterations, regardless of TPS and TMB.
Figure 1 Bar graph comparing control and trial arms in pivotal phase III clinical trials in terms of median progression-free survival (A) and median overall survival (B).
In contrast, most molecular-targeted therapies have become established as the standard first-line treatment for NSCLC cases with epidermal growth factor receptor (EGFR), anaplastic lymphoma kinase (ALK), ROS proto-oncogene 1 (ROS1), B-Raf proto-oncogene (BRAF), and Ret proto-oncogene (RET) alterations, exhibiting over 50% antitumor response rates and long-term PFS (53). A recent significant advancement in molecular-targeted therapy is the approval of sotorasib for second-line treatment in NSCLC cases with Kirsten rat sarcoma viral oncogene homolog (KRAS) G12C mutation, following immunotherapy-based therapies (54). Interestingly, the presence of oncogenic driver gene mutations has been found to have an impact on the efficacy of ICIs in NSCLC. Specifically, EGFR and KRAS mutations have been identified as key factors associated with ICI efficacy. NSCLC patients with KRAS mutations have shown favorable responses to ICIs with or without platinum-based chemotherapy compared to those without KRAS mutations. For instance, in a study involving patients with non-squamous NSCLC treated with pembrolizumab alone or in combination with chemotherapy, those with KRAS mutations had a longer PFS compared to patients with wild-type KRAS (median PFS 16.5 months vs. 8.0 months) (55). Another study also reported that KRAS mutations were significant favorable prognostic factors in NSCLC patients treated with pembrolizumab in combination with carboplatin plus pemetrexed for non-squamous NSCLC or paclitaxel for squamous NSCLC (56). A subgroup analysis of the IMpower150 trial revealed that the combination of atezolizumab, bevacizumab, carboplatin, and paclitaxel (ABCP) showed a greater PFS benefit in the population with KRAS mutations compared to the combination of bevacizumab, carboplatin, and paclitaxel, with hazard ratios (HRs) of 0.42 and 0.65, respectively, in KRAS mutation-positive and KRAS mutation wild-type populations (57).
Conversely, a meta-analysis of phase III studies comparing ICI monotherapy to docetaxel in the pretreatment setting revealed that ICI monotherapy is less beneficial in NSCLC patients with EGFR mutant compared to those of wild-type (58). However, several clinical trials have shown the clinical benefit of combining ICIs with platinum-based chemotherapy and an anti-vascular endothelial growth factor (VEGF) strategy. In a subset analysis of the IMpower150 trial, the combination of atezolizumab, carboplatin, paclitaxel, and bevacizumab demonstrated longer PFS and OS compared to carboplatin, paclitaxel, and bevacizumab in patients with common EGFR mutations (59). VEGF-A has been found to have an immunosuppressive role by promoting the function of regulatory T-cell and driving the growth of EGFR mutant NSCLC. Therefore, combining ICIs with VEGF-A inhibitors, such as bevacizumab, has emerged as an appealing treatment strategy for EGFR mutant NSCLC after driver-targeted therapy failure (60–62). However, regarding the predictive value of driver oncogenes other than EGFR and KRAS mutations, conclusive evidence has not been established at this stage. Several small retrospective cohort studies have reported the efficacy of ICI monotherapy in NSCLC patients with other diver oncogenic alterations, with response rates ranging from 0% in NSCLC patients with ALK fusion to 24% in NSCLC patients with BRAF mutation (40, 63, 64). Nevertheless, these findings are insufficient to draw definitive conclusions regarding the clinical relevance of ICIs for patients with these driver gene alternations other than EGFR and KRAS. Regarding RET alternations, the ongoing phase III trials comparing the RET inhibitor selpercatinib to other treatments will provide insights into the clinical efficacy of combination therapy involving ICIs for those patients (65).
Recent large-scale profiling studies using NGS in NSCLC have uncovered multiple non-random patterns of driver gene alterations. These patterns often exhibit co-occurrence or mutual exclusivity and are associated with specific driver alterations. One notable example is the co-occurrence of oncogenic driver alterations, such as KRAS and EGFR mutations, along with the inactivation of well-known tumor suppressor genes like tumor protein p53 (TP53), LKB1 (STK11), and KEAP1. These co-occurring patterns have significant biological implications and can influence tumor evolution and progression (18). Furthermore, these co-occurring patterns also impact the clinical efficacies of various therapies, including ICI and cytotoxic chemotherapy. In patients with KRAS-mutant NSCLC who were treated with ICI monotherapies or ICI combination therapies, the response rate was remarkably higher in the group with TP53 co-mutation compared to the group with STK11 co-mutation (28). The median PFS and median OS were reported as 3.0 months and 16.0 months, respectively, for patients with KRAS/TP53 co-mutation (KP group), while it was 1.8 months and 6.4 months for patients with KRAS/STK11 co-mutation (KL group). The underlying biological mechanism explaining the poor efficacy of ICIs in the KL group may be attributed to the immunosuppressive TME caused by LKB1 inactivation followed by STK11 mutation (18). LKB1 inactivation in cancer cells leads to the production of several immunosuppressive cytokines, such as Interleukin (IL)-6, IL-33, chemokine (C-X-C motif) ligand 7, and granulocyte colony-stimulating factor, which contribute to the mobilization of neutrophils (66). Neutrophils play a role in impeding T-cell movement and function, leading to the development of an “immune desert environment” characterized by reduced tumor-infiltrating lymphocytes. The limited efficacy of ICI monotherapies and ICI combined with cytotoxic chemotherapies has been observed in NSCLC patients with STK11 or KEAP1 mutations. In the subgroup analysis of the IMpower150 trial, the KRAS-mutant NSCLC patients and co-occurring STK11 and/or KEAP1 mutations exhibited a significantly shorter median PFS of the combination therapy ABCP compared to those with wild type in both STK11 and KEAP1 (6.0 months vs. 15.2 months) (57) (Supplementary Table 2). In contrast, NSCLC patients with KRAS/TP53 co-mutation had a longer median PFS with ABCP compared to those with KRAS mutations and wild-type TP53 (14.3 months vs. 7.3 months) (57). In the subgroup analysis of the KEYNOTE-189 trial, the overall response rate (ORR) of pembrolizumab in combination with platinum plus pemetrexed was 30.6% in NSCLC patients with STK11 mutation, whereas it was 48.8% in those with STK11 wild type (67) (Supplementary Table 2). Furthermore, in NSCLC patients with KEAP1 mutation, the ORR of pembrolizumab in combination with platinum plus pemetrexed was 35.6% (67). The median PFS for patients with STK11 mutation and those with KEAP1 mutation were 6.1 and 5.1 months (Figures 2A, C), respectively, indicating that the clinical efficacy of ICIs combined with cytotoxic chemotherapy is also limited in NSCLC patients with both gene mutations. However, since STK11 and/or KEAP1 mutations are also associated with poor clinical outcomes to cytotoxic chemotherapy without ICIs, there may still a benefit in adding PD-1/PD-L1 inhibitors to platinum-based chemotherapy even in this population.
Figure 2 Bar graph comparing control and trial arms in subgroup analyses of pivotal clinical trials for NSCLC with STK11 or KEAP1 mutation in terms of median progression-free survival (A, C) and median overall survival (B, D).
To enhance the clinical outcomes of PD-1/PD-L1 inhibitor-based therapy for “immune desert environment” NSCLC caused by STK11 and/or KEAP1 mutations, the addition of CTLA-4 inhibitors to PD-1/PD-L1 inhibitors represents a promising approach. CTLA-4 is expressed on activated T cells upon tumor antigen presentation by dendritic cells. It has a stronger binding affinity to CD80/86 compared to CD28, which is responsible for T-cell activation, thereby suppressing T-cell activation (68). Anti-CTLA-4 antibodies, such as ipilimumab and tremelimumab, block the binding of CTLA-4 to CD80/86, leading to enhanced and sustained T-cell activation (68). The reported clinical benefits of combining PD-1/PD-L1 inhibitors with CTLA-4 inhibitors for NSCLC with STK11 and/or KEAP1 mutations are based on exploratory analyses of phase III clinical trials and involve unstratified univariate analysis with a relatively smaller sample size. In the subgroup analysis of CheckMate 227, the PFS-HR of nivolumab plus ipilimumab compared to platinum-base chemotherapy were 1.04 for patients with STK11 mutation (n = 78) and 0.25 for those with KEAP1 mutation (n = 38) (69) (Figures 2A, C; Supplementary Table 2). In the subgroup analysis of CheckMate 9LA, the PFS-HRs of nivolumab plus ipilimumab with platinum-based chemotherapy compared to platinum-based chemotherapy alone were 0.61 (95%CI: 0.37–1.00) for patients with STK11 mutation and 0.34 (95%CI: 0.14–0.83) for patients with KEAP1 mutation (52) (Figures 2A, C; Supplementary Table 2). Further, in the subgroup analysis of the POSEIDON trial, the PFS-HRs of durvalumab plus tremelimumab with platinum-based chemotherapy compared to platinum-based chemotherapy alone were 0.47 (95%CI: 0.23–0.93) for patients with STK11 mutation (n = 53) and 0.94 (95%CI: 0.33–3.35) for patients with KEAP1 mutation (n = 28) (70) (Figures 2A, C; Supplementary Table 2). The subgroup analyses of these major clinical trials indicate that certain ICI combination therapies may have some degree of effectiveness in treating NSCLC with STK11 or KEAP1 mutations, although their therapeutic benefits are generally limited (Figures 2A–D). Specifically, KRAS-mutant NSCLC with STK11 or KEAP1 mutations tends to have a poorer prognosis, and comprehensive co-mutation analyses in KRAS-mutant NSCLC have not been conducted extensively for other ICI combination therapies except IMpower150 (57). Therefore, for NSCLC cases with these mutations, it is important to continue clinical and molecular analyses and to develop more advanced therapeutic strategies targeting novel therapeutic targets.
Cancer cells have possess a distinct metabolic characteristic known as the Warburg effect, wherein they preferentially utilize the glycolytic pathway for energy production, even in the presence of sufficient oxygen (71–73). This unique glycometabolism trait is characterized by increased glucose uptake and enhanced carbohydrate conversion into lactose. By consuming high amounts of glucose, tumor cells can rapidly proliferate and generate ATP, while also obtaining the necessary glycometabolic intermediates for synthesizing cellular components (73–75). Glucose is not only vital for tumor cell growth but also plays a crucial role in the proliferation and activation of effector T cells. Consequently, intratumoral effector T cells must outcompete tumor cells to acquire glucose (33, 76). Hence, in rapidly growing tumors, high glucose consumption itself may contribute to immunosuppression. In support of this, a study by Zappasodi et al. explored the correlation between tumor immune infiltration and glycolysis of cancer cells in advanced melanoma patients treated with ipilimumab. They discovered that high expression of glucose catabolism genes in melanoma was inversely associated with infiltration of substantial immune cells, suggesting that tumors with low glycolytic activity are more likely to respond to anti-CTLA-4 antibodies (77). Furthermore, lactate dehydrogenase A (LDHA) and monocarboxylate transporter 1 (MCT1), which are key enzymes involved in glycolysis and lactate production, have been found to exhibit an inverse correlation with immune infiltrates even after ipilimumab treatment (77). This suggests that anti-CTLA-4 blockade alone may be insufficient to enhance immune cell infiltration in highly glycolytic tumors.
LKB1 is recognized as a key metabolic regulator that exerts control over glucose metabolism by inducing the expression of critical genes encoding enzymes involved in glycolysis, gluconeogenesis, aerobic oxidation, and the pentose phosphate pathway. It achieves this regulation by acting on several downstream targets, including the central metabolic sensor called AMP-activated protein kinase (AMPK) (78–82). Under conditions of energy stress, LKB1 directly phosphorylates AMPK, which in turn promotes the activation of catabolic pathways such as glycolysis and fatty acid oxidation. Simultaneously, it suppresses anabolic pathways, including gluconeogenic enzymes, to maintain intracellular ATP levels (81, 82). Furthermore, the LKB1-AMPK axis plays a role in regulating cell growth and division by inhibiting the mammalian target of rapamycin complex 1 (mTORC1), which serves as the central integrator of nutrient and mitogenic signals. Notably, mTORC1 is often activated in cancer cells, contributing to tumor progression (81, 83). When LKB1 function is compromised, these downstream factors become dysregulated, leading to increased glucose uptake and consumption, as well as a metabolic shift toward aerobic glycolysis. Even in benign tumors with LKB1 haploinsufficient, there have been reports of enhanced accumulation of 18F-deoxyglucose on positron emission tomography, indicating that the loss of LKB1 function directly influences glucose metabolic reprogramming (84). Studies using the naturally LKB1-inactivated NSCLC cell line A549 have demonstrated that the activation of hypoxia-inducible factor 1 alpha (HIF-1α), induced by LKB1 inactivation, contributes to the enhancement of the aerobic glycolytic system (85). The absence of LKB1 was found to result in increased HIF-1α expression, which was shown to depend on both mTOR signaling and cellular mitochondrial reactive oxygen species (ROS) levels. Notably, HIF-1α knockdown in LKB1-deficient cell line significantly reduced proliferation under low-glucose conditions, indicating that HIF-1α promotes the growth of NSCLC with LKB1 inactivation even when nutrients are limited (85). Alongside LKB1 inactivation, KRAS mutation, which is the most prevalent oncogenic alteration in tumors with LKB1 inactivation, also leads to heightened glucose uptake and increased glycolytic activity. This is achieved through the upregulation of glucose transporter 1 (GLUT1) and key glycolytic enzymes such as LDHA, hexokinases, and phosphofructokinase 1 (PFK1) (86–88). Mutant KRAS, by upregulating GLUT1 and these glycolytic enzymes, further enhances aerobic glycolysis. Therefore, lung cancer cells with simultaneous LKB1 inactivation and KRAS mutation are likely to exhibit greater glucose uptake and consumption, contributing to their rapid tumor growth and suppression of intratumor effector T-cell activity (Figure 3A).
Figure 3 Overview of glucose and glutamine (Gln) metabolism in KRAS-mutant NSCLC with LKB1 or KEAP1 inactivation. (A) Overview of glucose and Gln metabolism in KRAS-mutant NSCLC with LKB1 inactivation. Glucose is imported by glucose transporter 1 (GLUT1) and is then metabolized by glycolysis into pyruvate. Pyruvate then either enters the tricarboxylic acid (TCA) cycle for ATP synthesis or is converted to lactate by lactate dehydrogenase (LDH). Mutant-KRAS enhances aerobic glycolysis by upregulating GLUT1 and LDH. After exported by monocarboxylate transporter 4 (MCT4), lactate increases extracellular acidification rate of tumor microenvironment (TME) and has diverse effects on various immune cells. Gln is imported by SLC1A5 where it then enters into the mitochondria and is converted to glutamate (Glu) by glutaminase (GLS), which is highly increased in NSCLC with LKB1 inactivation. The released NH4+ during the conversion to Glu is used for the synthesis of the purine/pyrimidine base. Carbamoyl phosphate synthetase 1 (CPS1), which is the first rate-limiting mitochondrial enzyme in the urea cycle, is overexpressed in NSCLC with LKB1 inactivation. Glu is also used for the precursor of glutathione (GSH), which promotes reactive oxygen species (ROS) detoxification. In addition, the excess synthesized Glu is excreted out via xCT/SLC7A11 and is then required for T-cell activation. (B) Overview of glucose and Gln metabolism in KRAS-mutant NSCLC with KEAP1 inactivation. Glucose and Gln metabolism are promoted to the anabolic pathway through interaction with the phosphatidylinositol 3’-kinase/protein kinase B signaling. Gln exchanges Glu for cystine via the antiporter xCT (SLC3A2/SLC7A11), which activated NRF2 target. Both cystine and Gln are used to produce GSH, leading to ROS neutralization. Gln is also used in purine base synthesis, and fewer Gln are used for TCA cycle.
Tumor cells that undergo rapid proliferation stimulate the formation of tumor blood vessels by releasing factors that promote angiogenesis. This process is crucial for acquiring nutrients and oxygen. However, the resulting vasculature is often immature and hyperpermeable, leading to the development of hypoxic regions within the tumor. These hypoxic areas create a barrier that hampers the infiltration of immune cells (89). Moreover, the hypoxic tumor microenvironment contributes to the accumulation of immunosuppressive metabolic byproducts. These metabolic alterations negatively impact the function of effector T cells, while they may have little to no effect or even benefit suppressive immune populations like regulatory T cells (Treg) and suppressive myeloid populations (31, 90). The increased glycolytic activity in tumor leads to the production of large amounts of lactate, which in turn acidifies the extracellular spaces. NSCLC with LKB1 inactivation is associated with an elevated extracellular acidification rate (ECAR), which indicates higher lactate levels. Introducing transient expression of LKB1 in an NSCLC cell line with LKB1 inactivation resulted in a 20% decrease in ECAR (85). Despite its ability to lower pH, lactate has diverse effects on immune cell populations. For instance, it promotes a metabolic shift in Treg to enable their activity in low-glucose environments and induces macrophages to adopt an M2 phenotype, which supports tumor growth (91–93). Notably, the accumulation of lactic acid can suppress the proliferation of CD4+ and CD8+ T cells, as well as inhibit their cytokine production (94). Lactate can deplete intracellular nicotinamide adenine dinucleotide+ (NAD+) levels and impair effector T cells because LDH uses lactate to generate NAD+ hydrogen (NADH). Conversely, Treg can continue to function in high lactate environments where conventional T cells are suppressed due to the NAD+ produced by mitochondrial metabolism (91, 93).
Furthermore, it is recognized that circulating lactate is transported into cells via MCT1 and used as an energy source and substrate for lipogenesis in certain cancer types (95). In an analysis that measured the uptake of metabolic intermediates from tumor samples after labeled glucose infusion in NSCLC patients, elevated lactate labeling was observed, indicating the uptake of lactate in tumors compared to glycolytic metabolites (96). In addition, a xenograft model using an NSCLC cell line with LKB1 inactivation showed increased labeled lactate in the tumor, indicating the uptake of extracellular lactate and its incorporation into the tricarboxylic acid (TCA) cycle as a carbon source (96). This study suggests that lactate plays a crucial role as an energy source in LKB1-inactivated NSCLC. Apart from LDHA/B, elevated levels of the lactate transporter MCT1/4 have also been observed in lung cancer cells with LKB1 inactivation (96, 97), suggesting that intracellular lactate is not only incorporated into the TCA cycle but that extracellular lactate released by neighboring cancer cells can be taken up and incorporated into the TCA cycle as an energy source (Figure 3A).
Glutamine (Gln) is a vital amino acid with significant roles in cellular functions, including energy and biomolecule synthesis, as well as ROS scavenging. Upon cellular uptake, Gln is converted into glutamate (Glu) by the enzyme glutaminase (GLS). It is further converted to α-ketoglutarate, which enters the TCA cycle, generating metabolic intermediates for lipid, nucleic acid, and protein synthesis. In the TME, both tumor cells and infiltrating immune cells have a high demand for Gln, similar to glucose. T-cell activation and proliferation heavily rely on Gln metabolism, and when Gln is insufficient in the TME, the high consumption by tumors can inhibit T-cell activity. Conversely, reduced Gln metabolism in tumors has shown to increase Gln utilization within the TME (34). In a mouse model of colorectal cancer using MC38 tumor-bearing mice, combination therapy of anti-PD-1 monoclonal antibody and a Gln antagonist prodrug, 6-diazo-5-oxo-L-norleucine, resulted in enhanced tumor growth inhibition (98).
Several oncogenes and tumor suppressors play a role in regulating Gln metabolism, and LKB1 inactivation is also implicated in Gln flux regulation. LKB1 ectopic expression in NSCLC cells with LKB1 deficiency led to a decrease in Gln-derived Glu (85). Furthermore, in the LKB1-deficient NSCLC cell line A549, the majority of Gln-derived carbon entered the TCA cycle compared to glucose-derived carbon, in contrast to the cell line with LKB1 ectopic expression (85). Moreover, LKB1-inactivated NSCLC cells exhibit higher levels of GLS expression and more active conversion of Gln to Glu (99). The released NH4+ during this conversion is used for the synthesis of purine/pyrimidine bases, which are essential for rapid cell proliferation. Notably, studies have demonstrated characteristic overexpression of carbamoyl phosphate synthetase 1 (CPS1), the first rate-limiting mitochondrial enzyme in the urea cycle, in a subset of NSCLC with LKB1 inactivation (100, 101). CPS1 plays a vital role in promoting cell growth by increasing the bioavailability of carbamoyl phosphate, an intermediary metabolite required for de novo pyrimidine synthesis. The CPS1 expression is transcriptionally regulated by LKB1 through AMPK, and cases with high CPS1 expression have been associated with poor prognosis, particularly in NSCLC with LKB1 inactivation (100, 101). Thus, LKB1-inactivated lung cancers effectively utilize excess Gln, and the activation of these metabolic pathways may contribute to their high malignancy. Furthermore, oncogenic KRAS has been shown to stimulate Gln catabolism in the mitochondria (87, 88). Since both KRAS and LKB1 regulate metabolism, the co-mutation of these two genes could lead to a unique metabolic phenotype not observed with either mutation alone. In fact, CPS1 plays a pivotal role in maintaining the balance between purine and pyrimidine in NSCLC cells with co-mutated KRAS and LKB1, and the enzyme also provides an alternative pool of carbamoyl phosphate to sustain pyrimidine availability (101). Hence, apart from glucose metabolism, reprogramming of Gln metabolism in tumors harboring co-mutated KRAS and LKB1 likely contributes to aggressive oncological behavior and impacts TME (Figure 3A). Notably, the clinical response to PD-(L)1 inhibition is significantly poorer in NSCLC patients with co-mutated KRAS and STK11 compared to those with only STK11 mutation (102).
Cellular metabolism generates ROS, which need to be scavenged to prevent damage to DNA, RNA, and proteins. Gln metabolism also plays an important role in maintaining oxidative homeostasis. Glu, generated from Gln by the catalytic action of GLS, serves as the precursor of glutathione (GSH), which promotes ROS detoxification (103). GSH, along with thioredoxin, plays a major role in neutralizing ROS and is synthesized through an NADPH-dependent mechanism. Loss of LKB1 activity resulting in metabolic reprogramming leads to elevated ROS levels and metabolic stress, while the conversion of Gln to Glu significantly contributes to ROS neutralization by stimulating the production of GSH (104). Furthermore, due to the increased aerobic glycolysis in cancer cells, metabolites are shunted toward the pentose phosphate pathway (PPP), which aids in ROS scavenging. In LKB1 mutant cell lines, such as A549 and H460 cells, genes associated with the PPP are upregulated, indicating their dependence on this pathway (105). Meanwhile, A549 cells that re-express LKB1 exhibit a higher apoptosis rate under ROS stress compared to control cells (104), suggesting that the upregulation of Gln conversion observed in LKB1-inactivating mutations may confer increased resistance to ROS.
The KEAP1-nuclear factor erythroid-derived 2-like 2 (NRF2) pathway plays a crucial role in regulating the cellular response to oxidative stress, and its signaling abnormalities have been observed in various cancer types, including NSCLC (106, 107). In normal conditions, KEAP1 ubiquitinates NRF2, encoded by the NFE2L2 gene, for degradation through ubiquitination. However, under stress conditions, KEAP1 activity is reduced, leading to increased transcription of NRF2 target genes. This activation of NRF2 signaling enhances antioxidant defense against ROS and regulates drug detoxification and immune response (106, 107). In NSCLC, KEAP1 deficiency is commonly observed in LUAD, while activating alterations of NFE2L2 are more prevalent in squamous cell lung carcinoma (~20%), with both alterations being mutually exclusive (108). The constitutive activation of NRF2 signaling in advanced cancer patients diminishes the therapeutic effects of chemotherapy and radiation therapy, as these treatments rely on inducing cell death through DNA replication damage and ROS induction (106, 107). Furthermore, recent studies have revealed that NRF2 activation promotes various metabolic reprogramming processes and is associated with tumor progression in NSCLC, including glutaminolysis (109–111).
Similar to tumors with LKB1 inactivation, tumors harboring KEAP1 mutations increased uptake of Gln from TME, leading to reduced availability of Gln for infiltrating T cells and consequent inhibition of their activation. Activation of NRF2 signaling resulting from KEAP1 inactivation promotes glucose and Gln metabolism toward the anabolic pathway through phosphatidylinositol 3’-kinase/protein kinase B signaling (112). This increased Gln consumption is accompanied by increased expression of the Gln importer SLC1A5 (113). Furthermore, the incorporated Gln exchanges Glu for cystine through the antiporter xCT (SLC3A2/SLC7A11), which is upregulated as a target of NRF2 activation, in a Gln degradation-dependent manner (113, 114). Both cystine and Gln contribute to the production of GSH, thereby enhancing antioxidant activity. In addition, Gln is actively used in purine base synthesis. Therefore, tumors with KEAP1 inactivation may have limited Gln availability for the TCA cycle (Figure 3B). NRF2 knockdown in NSCLC cell lines, such as A549, reduces GSH formation from Gln (104). Furthermore, KEAP1-mutant NSCLC cell lines demonstrate sensitivity to GLS inhibition due to their high dependence on Gln uptake in the culture medium (104). Integrating these findings, the combination of GLS inhibition and immunotherapy may offer a promising therapeutic strategy in KEAP1-inactivated NSCLC. By suppressing Gln uptake, this strategy could potentially activate T cells in the TME while attenuating the antioxidant effect of KEAP1-inactivated tumors. Furthermore, Pranavi et al. found that NSCLC with KEAP1 inactivation exhibits increased dependence on glucose under glucose-limiting conditions, as NRF2-dependent SLC7A11 expression is upregulated, resulting in cytotoxicity related to disulfide stress (115). In addition, they demonstrated the high sensitivity of KEAP1-inactivated NSCLC to GLUT inhibitor (115). These findings suggest that targeting Gln and glucose metabolism could be an attractive therapeutic target in NSCLC cases with KEAP1 inactivation or constitutive activation of NRF2.
Clinical data analysis reveals that lung cancers characterized by simultaneous mutations in LKB1 and KEAP1 exhibit an exceptionally poor prognosis (23). In vitro and in vivo studies have demonstrated that co-occurring mutations of STK11 and KEAP1 in KRAS-mutant NSCLC promote tumor growth and confer enhanced resistance to radiotherapy (116). The co-inactivation of LKB1 and KEAP1 cooperatively promotes metabolic reprogramming in KRAS-mutant tumor, and even in the presence of KEAP1 inactivation, LKB1 inactivation modulates NRF2 activity through increased ROS levels (104). LKB1-mutant cells induce NRF2-dependent Glu cysteine ligase expression, a key enzyme that generates γ-Gly-Gly from Gln and cysteine to increase the GSH pool (104). These results indicate that KRAS-mutant NSCLC with co-inactivation of LKB1 and KEAP1 enhanced Gln dependence compared to KRAS-mutant NSCLC with LKB1 or KEAP1 inactivation. Consistently, KRAS-mutant NSCLC cell lines with co-inactivation of LKB1 and KEAP1 display increased sensitivity to GLS inhibitors compared to other cell lines (104), indicating that targeting glutaminolysis in KRAS-mutant NSCLC with co-inactivation of LKB1 and KEAP1 holds promise as a therapeutic strategy.
In a study conducted by Best et al., distinct metabolic characteristics were observed among KRAS-KEAP1 (KK), KRAS-LKB1 (KL), and KRAS-KEAP1-LKB1 (KKL) mutant LUAD using genetically engineered mouse models (99). In KRAS-mutant LUAD with LKB1 inactivation, the expression of GLS1, an enzyme responsible for metabolizing Gln to Glu, was significantly higher compared to KRAS-mutant NSCLC with co-inactivation of LKB1 and KEAP1. The conversion of Gln to Glu was particularly enhanced in the KL mouse model (Figure 3A). Furthermore, the influx of α-ketoglutaric acid into the TCA cycle was significantly increased in KL mice compared to KK or KKL mice (99). Tumors from KL mice also exhibited a notable increase in orotic acid, which is synthesized during the Gln to Glu conversion process via carbamoyl phosphate. Orotic acid is a precursor of pyrimidine and its synthesis directly affects pyrimidine production (99). Tumors from KL mice also exhibited a notable increase in orotic acid, which is synthesized during the Gln to Glu conversion process via carbamoyl phosphate. Orotic acid is a precursor of pyrimidine and its synthesis directly affects pyrimidine production (117, 118). Increased orotic acid synthesis is closely linked to enhanced nucleic acid synthesis, as nucleotide synthesis is tightly regulated by pyrimidine. In KRAS-mutant LUAD with LKB1, CPS1, an enzyme responsible for carbamoyl phosphate synthesis in the mitochondria, is highly expressed, and the heightened Gln metabolism contributes to rapid tumor growth through increased nucleic acid synthesis (100, 101). Excess Glu synthesized is also released from cancer cells via xCT/SLC7A11 (119, 120). Best et al. demonstrated that the release of Glu from cancer cells is crucial for T-cell activation and clonal expansion of T-cell receptors (99). Therefore, GLS inhibition attenuates CD8+ T-cell activation, suggesting that the combining GLS inhibitors with immunotherapy may not enhance the immune response. Particularly in KL mice, the amount of Glu released from cancer cells was higher, and KKL mice exhibited a similar Glu metabolic pattern to KL mice compared to KK mice (99). These findings suggest that GLS inhibitors may be less effective in KRAS-mutant LUAD with LKB1 inactivation and co-occurring mutations of LKB1 and KEAP1 compared to KRAS-mutant LUAD with KEAP1 inactivation.
To date, subgroup analyses of pivotal clinical trials have shown that current ICI combination regimens have some effectiveness in NSCLC patients with LKB1 or KEAP1 inactivation compared to standard platinum doublet chemotherapies (52, 57, 67, 69, 70). However, their efficacy is not sufficient to significantly improve long-term prognosis compared to NSCLC patients without LKB1 and KEAP1 inactivation (52, 57, 67, 69, 70). This indicates that the combination of anti-PD-1/PD-L1 antibodies with cytotoxic chemotherapies and/or anti-CTLA-4 antibodies is unable to fully restore the dysfunctional state of T cells or NK cells in NSCLC with these mutations. Moreover, the clinical efficacy of most regimens has not yet been analyzed for KRAS-mutant NSCLC with LKB1 or KEAP1 inactivation, which is associated with the poorest prognosis (29, 102). On the other hand, a subgroup analysis of the IMpower150 trial revealed that the trial arm, ABCP regimen, demonstrated superior antitumor effects compared to the control arm in KRAS-mutant NSCLC with STK11 or KEAP1 mutations (57). By normalizing abnormal tumor vasculature, the addition of VEGF-A inhibitors to ICIs can increase the infiltration of effector T cells into tumors (121). Furthermore, since VEGF-A receptors are expressed on various tumor-promoting immune cells, such as Tregs and immature dendritic cells, this combination therapy may have additional effects in converting the intrinsically immunosuppressive TME into an immunosupportive one, even in immune cold subtypes (121). However, further analysis is needed to fully understand the significance of VEGF-A inhibition for immune cold tumors from both basic and clinical perspectives. Regarding molecular-targeted agents for KRAS G12C mutations, sotorasib and adagrasib are now indicated as a second-line treatment following ICI regimens and has expanded the therapeutic options for KRAS-mutant NSCLC patients (54). However, its efficacy is limited in cases of NSCLC with co-mutations of STK11 and KEAP1 (122). Similarly, in NSCLC with EGFR mutations, co-mutations such as TP53 and RB transcriptional corepressor 1 can affect the antitumor effect of EGFR-tyrosine kinase inhibitors (123). Therefore, in addition to targeting oncogenic driver alterations, it is increasingly important to identify inactivating mutations in tumor suppressor genes that can impact the efficacy of immunotherapy and of molecularly targeted agents. In fact, some clinical trials of novel molecularly targeted agents targeting KRAS G12C mutation have included STK11 mutation as a stratification factor (124, 125) (Table 1). These trends underscore the need for novel therapeutic strategies in the treatment of NSCLC with STK11 and/or KEAP1 mutations, as the efficacy of ICIs and molecular targeting agents directly affects patient outcomes.
Concurrent with the advancements in immune checkpoint inhibitors (ICIs) and molecularly targeted therapies, recent fundamental research has uncovered that each driver gene alteration has a cancer-specific impact on the TME through metabolic reprogramming. Specifically, the alteration of glucose and glutamine (Gln) metabolism resulting from LKB1 or KEAP1 inactivation appears to play a significant role in diminishing the effectiveness of current immunotherapies by suppressing the activity of effector T cells. Ongoing clinical trials targeting glucose or Gln metabolism, as depicted in Table 1, aim to develop novel therapies for NSCLC with LKB1 or KEAP1 inactivation (126–128).
One therapeutic strategy being explored involves the addition of metformin, a commonly used medication for type 2 diabetes, to cytotoxic chemotherapy. Accumulating evidence supports the antitumor effects of metformin, as it enhances AMPK-mediated cell growth inhibition and cisplatin-induced apoptosis in LKB1-inactivated NSCLC (135, 136). Interestingly, despite initial reports indicating that metformin requires LKB1 for the regulation of gluconeogenesis in the liver, it demonstrates efficacy in LKB1-inactivated NSCLC (137). Clinical trials targeting Gln metabolism have also been initiated, employing Gln antagonists and oral GLS inhibitors, to explore a new therapeutic approach for NSCLC with LKB1 inactivation or KEAP1 inactivation/NFE2L2 alteration (127, 128) (Table 1). However, the utilization of glutamate (Glu) released from cancer cells by T cells reveals a complex and interconnected relationship between cancer metabolism and immune cells within the TME (99). Furthermore, NSCLC with concurrent STK11 and KEAP1 mutations exhibit distinct Gln metabolism patterns compared to NSCLC with KEAP1 mutation alone, suggesting that the antitumor effects of targeting Gln metabolism may vary among NSCLC subgroups with different mutation co-occurring patterns (99). Therefore, considering the potential impact of diverse metabolic reprogramming based on specific mutation patterns, it will be crucial to assess the response of each mutated subgroup when treated with Gln metabolism inhibitors, either alone or in combination with a PD-(L)1 inhibitor.
In conclusion, high consumption of glycolysis and glutaminolysis in immune-resistant phenotype tumors, such as NSCLC with LKB1 and/or KEAP1 inactivation, not only contribute to tumor aggressiveness but also impede intratumor T-cell function. The presence of co-occurring mutations in NSCLC leads to distinct metabolic alterations that impact immune cells within TME. These differences in metabolic reprogramming may affect clinical efficacies of current ICI combination regimens and novel agents targeting metabolic enzymes. To develop new therapeutic strategies that target metabolic alterations in combination with ICI regimens for NSCLC with LKB1 and/or KEAP1 inactivation, further extensive analyses on a larger scale will be necessary.
IT, JK, HI, SH, and MM were involved in the initial drafting of the manuscript, data collection, and analysis. They also contributed to the conceptualization of the study, reviewed the manuscript, and provided feedback and edits. All authors have read and given their approval for the final version of the manuscript. IT have demonstrated the dependency of CPS1, a metabolic enzyme, in cell growth, metabolism and prognosis in LKB1-inactivated lung adenocarcinomas. Furthermore, Serglycin secretion, which is a chondroitin sulfate proteoglycan involved in reprograming to an immunosuppressive TME, is epigenetically induced through nicotinamide N-methyltransferase-induced perturbation of methionine metabolism in TTF-1–negative lung adenocarcinoma. These results were published in J Natl Cancer Inst (2017) 109:1-9 and J Natl Cancer Inst (2022) 114:290-301.
IT received support for this research from the Japan Society for the Promotion of Science through Grant-in-Aid for Scientific Research (B) 23H02920.
The authors would like to thank Enago (www.enago.jp) for providing English language editing services.
The authors declare that the research was conducted in the absence of any commercial or financial relationships that could be construed as a potential conflict of interest.
All claims expressed in this article are solely those of the authors and do not necessarily represent those of their affiliated organizations, or those of the publisher, the editors and the reviewers. Any product that may be evaluated in this article, or claim that may be made by its manufacturer, is not guaranteed or endorsed by the publisher.
The Supplementary Material for this article can be found online at: https://www.frontiersin.org/articles/10.3389/fonc.2023.1249237/full#supplementary-material
1. Grant MJ, Herbst RS, Goldberg SB. Selecting the optimal immunotherapy regimen in driver-negative metastatic NSCLC. Nat Rev Clin Oncol (2021) 18:625–44. doi: 10.1038/s41571-021-00520-1
2. Reck M, Rodriguez-Abreu D, Robinson AG, Hui R, Csoszi T, Fulop A, et al. Pembrolizumab versus chemotherapy for PD-L1-positive non-small-cell lung cancer. N Engl J Med (2016) 375:1823–33. doi: 10.1056/NEJMoa1606774
3. Herbst RS, Giaccone G, de Marinis F, Reinmuth N, Vergnenegre A, Barrios CH, et al. Atezolizumab for first-line treatment of PD-L1-selected patients with NSCLC. N Engl J Med (2020) 383:1328–39. doi: 10.1056/NEJMoa1917346
4. Gandhi L, Rodriguez-Abreu D, Gadgeel S, Esteban E, Felip E, De Angelis F, et al. Pembrolizumab plus chemotherapy in metastatic non-small-cell lung cancer. N Engl J Med (2018) 378:2078–92. doi: 10.1056/NEJMoa1801005
5. Socinski MA, Jotte RM, Cappuzzo F, Orlandi F, Stroyakovskiy D, Nogami N, et al. Atezolizumab for first-line treatment of metastatic nonsquamous NSCLC. N Engl J Med (2018) 378:2288–301. doi: 10.1056/NEJMoa1716948
6. Reck M, Schenker M, Lee KH, Provencio M, Nishio M, Lesniewski-Kmak K, et al. Nivolumab plus ipilimumab versus chemotherapy as first-line treatment in advanced non-small-cell lung cancer with high tumour mutational burden: patient-reported outcomes results from the randomised, open-label, phase III CheckMate 227 trial. Eur J Cancer (2019) 116:137–47. doi: 10.1016/j.ejca.2019.05.008
7. Paz-Ares L, Ciuleanu TE, Cobo M, Schenker M, Zurawski B, Menezes J, et al. First-line nivolumab plus ipilimumab combined with two cycles of chemotherapy in patients with non-small-cell lung cancer (CheckMate 9LA): an international, randomised, open-label, phase 3 trial. Lancet Oncol (2021) 22:198–211. doi: 10.1016/S1470-2045(20)30641-0
8. Johnson ML, Cho BC, Luft A, Alatorre-Alexander J, Geater SL, Laktionov K, et al. Durvalumab with or without tremelimumab in combination with chemotherapy as first-line therapy for metastatic non-small-cell lung cancer: the phase III POSEIDON study. J Clin Oncol (2023) 41:1213–27. doi: 10.1200/JCO.22.00975
9. Anagnostou V, Niknafs N, Marrone K, Bruhm DC, White JR, Naidoo J, et al. Multimodal genomic features predict outcome of immune checkpoint blockade in non-small-cell lung cancer. Nat Cancer (2020) 1:99–111. doi: 10.1038/s43018-019-0008-8
10. Gavrielatou N, Shafi S, Gaule P, Rimm DL. PD-L1 expression scoring: noninterchangeable, noninterpretable, neither, or both. J Natl Cancer Inst (2021) 113:1613–4. doi: 10.1093/jnci/djab109
11. Tanaka I, Furukawa T, Morise M. The current issues and future perspective of artificial intelligence for developing new treatment strategy in non-small cell lung cancer: harmonization of molecular cancer biology and artificial intelligence. Cancer Cell Int (2021) 21:454. doi: 10.1186/s12935-021-02165-7
12. Faruki H, Mayhew GM, Serody JS, Hayes DN, Perou CM, Lai-Goldman M. Lung adenocarcinoma and squamous cell carcinoma gene expression subtypes demonstrate significant differences in tumor immune landscape. J Thorac Oncol (2017) 12:943–53. doi: 10.1016/j.jtho.2017.03.010
13. Grenda A, Krawczyk P, Blach J, Chmielewska I, Kubiatowski T, Kieszko S, et al. Tissue microRNA expression as a predictor of response to immunotherapy in NSCLC patients. Front Oncol (2020) 10:563613. doi: 10.3389/fonc.2020.563613
14. Sun J, Zhang Z, Bao S, Yan C, Hou P, Wu N, et al. Identification of tumor immune infiltration-associated lncRNAs for improving prognosis and immunotherapy response of patients with non-small cell lung cancer. J Immunother Cancer (2020) 8(1):e000110. doi: 10.1136/jitc-2019-000110
15. Zheng Y, Tang L, Liu Z. Multi-omics analysis of an immune-based prognostic predictor in non-small cell lung cancer. BMC Cancer (2021) 21:1322. doi: 10.1186/s12885-021-09044-4
16. Cristescu R, Mogg R, Ayers M, Albright A, Murphy E, Yearley J, et al. Pan-tumor genomic biomarkers for PD-1 checkpoint blockade-based immunotherapy. Science (2018) 362:6411. doi: 10.1126/science.aar3593
17. Gillette MA, Satpathy S, Cao S, Dhanasekaran SM, Vasaikar SV, Krug K, et al. Proteogenomic characterization reveals therapeutic vulnerabilities in lung adenocarcinoma. Cell (2020) 182:200–25 e35. doi: 10.1016/j.cell.2020.06.013
18. Skoulidis F, Heymach JV. Co-occurring genomic alterations in non-small-cell lung cancer biology and therapy. Nat Rev Cancer (2019) 19:495–509. doi: 10.1038/s41568-019-0179-8
19. Devarakonda S, Morgensztern D, Govindan R. Genomic alterations in lung adenocarcinoma. Lancet Oncol (2015) 16:e342–51. doi: 10.1016/S1470-2045(15)00077-7
20. Calles A, Sholl LM, Rodig SJ, Pelton AK, Hornick JL, Butaney M, et al. Immunohistochemical loss of LKB1 is a biomarker for more aggressive biology in KRAS-mutant lung adenocarcinoma. Clin Cancer Res (2015) 21:2851–60. doi: 10.1158/1078-0432.CCR-14-3112
21. Cardnell RJ, Behrens C, Diao L, Fan Y, Tang X, Tong P, et al. An integrated molecular analysis of lung adenocarcinomas identifies potential therapeutic targets among TTF1-negative tumors, including DNA repair proteins and Nrf2. Clin Cancer Res (2015) 21:3480–91. doi: 10.1158/1078-0432.CCR-14-3286
22. Marinelli D, Mazzotta M, Scalera S, Terrenato I, Sperati F, D’Ambrosio L, et al. KEAP1-driven co-mutations in lung adenocarcinoma unresponsive to immunotherapy despite high tumor mutational burden. Ann Oncol (2020) 31:1746–54. doi: 10.1016/j.annonc.2020.08.2105
23. Papillon-Cavanagh S, Doshi P, Dobrin R, Szustakowski J, Walsh AM. STK11 and KEAP1 mutations as prognostic biomarkers in an observational real-world lung adenocarcinoma cohort. ESMO Open (2020) 5(2):e000706. doi: 10.1101/2020.01.23.20017566
24. Singh A, Daemen A, Nickles D, Jeon SM, Foreman O, Sudini K, et al. NRF2 activation promotes aggressive lung cancer and associates with poor clinical outcomes. Clin Cancer Res (2021) 27:877–88. doi: 10.1158/1078-0432.CCR-20-1985
25. Binkley MS, Jeon YJ, Nesselbush M, Moding EJ, Nabet BY, Almanza D, et al. KEAP1/NFE2L2 mutations predict lung cancer radiation resistance that can be targeted by glutaminase inhibition. Cancer Discovery (2020) 10:1826–41. doi: 10.1158/2159-8290.CD-20-0282
26. Jeong Y, Hellyer JA, Stehr H, Hoang NT, Niu X, Das M, et al. Role of KEAP1/NFE2L2 mutations in the chemotherapeutic response of patients with non-small cell lung cancer. Clin Cancer Res (2020) 26:274–81. doi: 10.1158/1078-0432.CCR-19-1237
27. Jeong Y, Hoang NT, Lovejoy A, Stehr H, Newman AM, Gentles AJ, et al. Role of KEAP1/NRF2 and TP53 mutations in lung squamous cell carcinoma development and radiation resistance. Cancer Discovery (2017) 7:86–101. doi: 10.1158/2159-8290.CD-16-0127
28. Skoulidis F, Goldberg ME, Greenawalt DM, Hellmann MD, Awad MM, Gainor JF, et al. STK11/LKB1 mutations and PD-1 inhibitor resistance in KRAS-mutant lung adenocarcinoma. Cancer Discovery (2018) 8:822–35. doi: 10.1158/2159-8290.CD-18-0099
29. Arbour KC, Jordan E, Kim HR, Dienstag J, Yu HA, Sanchez-Vega F, et al. Effects of co-occurring genomic alterations on outcomes in patients with KRAS-mutant non-small cell lung cancer. Clin Cancer Res (2018) 24:334–40. doi: 10.1158/1078-0432.CCR-17-1841
30. Sugiura A, Rathmell JC. Metabolic barriers to T cell function in tumors. J Immunol (2018) 200:400–7. doi: 10.4049/jimmunol.1701041
31. DePeaux K, Delgoffe GM. Metabolic barriers to cancer immunotherapy. Nat Rev Immunol (2021) 21:785–97. doi: 10.1038/s41577-021-00541-y
32. Nabe S, Yamada T, Suzuki J, Toriyama K, Yasuoka T, Kuwahara M, et al. Reinforce the antitumor activity of CD8(+) T cells via glutamine restriction. Cancer Sci (2018) 109:3737–50. doi: 10.1111/cas.13827
33. Cascone T, McKenzie JA, Mbofung RM, Punt S, Wang Z, Xu C, et al. Increased tumor glycolysis characterizes immune resistance to adoptive T cell therapy. Cell Metab (2018) 27:977–87 e4. doi: 10.1016/j.cmet.2018.02.024
34. Leone RD, Zhao L, Englert JM, Sun IM, Oh MH, Sun IH, et al. Glutamine blockade induces divergent metabolic programs to overcome tumor immune evasion. Science (2019) 366:1013–21. doi: 10.1126/science.aav2588
35. Choi H, Na KJ. Different glucose metabolic features according to cancer and immune cells in the tumor microenvironment. Front Oncol (2021) 11:769393. doi: 10.3389/fonc.2021.769393
36. Arner EN, Rathmell JC. Metabolic programming and immune suppression in the tumor microenvironment. Cancer Cell (2023) 41:421–33. doi: 10.1016/j.ccell.2023.01.009
37. Lee SJ, Jang BC, Lee SW, Yang YI, Suh SI, Park YM, et al. Interferon regulatory factor-1 is prerequisite to the constitutive expression and IFN-gamma-induced upregulation of B7-H1 (CD274). FEBS Lett (2006) 580:755–62. doi: 10.1016/j.febslet.2005.12.093
38. Miyazawa A, Ito S, Asano S, Tanaka I, Sato M, Kondo M, et al. Regulation of PD-L1 expression by matrix stiffness in lung cancer cells. Biochem Biophys Res Commun (2018) 495:2344–9. doi: 10.1016/j.bbrc.2017.12.115
39. Altorki NK, Markowitz GJ, Gao D, Port JL, Saxena A, Stiles B, et al. The lung microenvironment: an important regulator of tumour growth and metastasis. Nat Rev Cancer (2019) 19:9–31. doi: 10.1038/s41568-018-0081-9
40. Tanaka I, Morise M. Current immunotherapeutic strategies targeting the PD-1/PD-L1 axis in non-small cell lung cancer with oncogenic driver mutations. Int J Mol Sci (2021) 23(1):245. doi: 10.3390/ijms23010245
41. Herbst RS, Baas P, Kim DW, Felip E, Perez-Gracia JL, Han JY, et al. Pembrolizumab versus docetaxel for previously treated, PD-L1-positive, advanced non-small-cell lung cancer (KEYNOTE-010): a randomised controlled trial. Lancet (2016) 387:1540–50. doi: 10.1016/S0140-6736(15)01281-7
42. Reck M, Rodriguez-Abreu D, Robinson AG, Hui R, Csoszi T, Fulop A, et al. Five-year outcomes with pembrolizumab versus chemotherapy for metastatic non-small-cell lung cancer with PD-L1 tumor proportion score >/= 50. J Clin Oncol (2021) 39:2339–49. doi: 10.1200/JCO.21.00174
43. Jassem J, de Marinis F, Giaccone G, Vergnenegre A, Barrios CH, Morise M, et al. Updated overall survival analysis from IMpower110: atezolizumab versus platinum-based chemotherapy in treatment-naive programmed death-ligand 1-selected NSCLC. J Thorac Oncol (2021) 16:1872–82. doi: 10.1016/j.jtho.2021.06.019
44. Garassino MC, Gadgeel S, Speranza G, Felip E, Esteban E, Domine M, et al. Pembrolizumab plus pemetrexed and platinum in nonsquamous non-small-cell lung cancer: 5-year outcomes from the phase 3 KEYNOTE-189 study. J Clin Oncol (2023) 41(11):JCO2201989. doi: 10.1200/JCO.22.01989
45. Socinski MA, Nishio M, Jotte RM, Cappuzzo F, Orlandi F, Stroyakovskiy D, et al. IMpower150 final overall survival analyses for atezolizumab plus bevacizumab and chemotherapy in first-line metastatic nonsquamous NSCLC. J Thorac Oncol (2021) 16:1909–24. doi: 10.1016/j.jtho.2021.07.009
46. West H, McCleod M, Hussein M, Morabito A, Rittmeyer A, Conter HJ, et al. Atezolizumab in combination with carboplatin plus nab-paclitaxel chemotherapy compared with chemotherapy alone as first-line treatment for metastatic non-squamous non-small-cell lung cancer (IMpower130): a multicentre, randomised, open-label, phase 3 trial. Lancet Oncol (2019) 20:924–37. doi: 10.1016/S1470-2045(19)30167-6
47. Nishio M, Barlesi F, West H, Ball S, Bordoni R, Cobo M, et al. Atezolizumab plus chemotherapy for first-line treatment of nonsquamous NSCLC: results from the randomized phase 3 IMpower132 trial. J Thorac Oncol (2021) 16:653–64. doi: 10.1016/j.jtho.2020.11.025
48. Paz-Ares L, Luft A, Vicente D, Tafreshi A, Gumus M, Mazieres J, et al. Pembrolizumab plus chemotherapy for squamous non-small-cell lung cancer. N Engl J Med (2018) 379:2040–51. doi: 10.1056/NEJMoa1810865
49. Novello S, Kowalski DM, Luft A, Gumus M, Vicente D, Mazieres J, et al. Pembrolizumab plus chemotherapy in squamous non-small-cell lung cancer: 5-year update of the phase III KEYNOTE-407 study. J Clin Oncol (2023) 41(11):1999-2006. doi: 10.1200/JCO.22.01990
50. Hellmann MD, Paz-Ares L, Bernabe Caro R, Zurawski B, Kim SW, Carcereny Costa E, et al. Nivolumab plus ipilimumab in advanced non-small-cell lung cancer. N Engl J Med (2019) 381:2020–31. doi: 10.1056/NEJMoa1910231
51. Brahmer JR, Lee JS, Ciuleanu TE, Bernabe Caro R, Nishio M, Urban L, et al. Five-year survival outcomes with nivolumab plus ipilimumab versus chemotherapy as first-line treatment for metastatic non-small-cell lung cancer in checkMate 227. J Clin Oncol (2023) 41:1200–12. doi: 10.1200/JCO.22.01503
52. Paz-Ares LG, Ciuleanu T-E, Cobo M, Bennouna J, Schenker M, Cheng Y, et al. First-line nivolumab plus ipilimumab with chemotherapy versus chemotherapy alone for metastatic NSCLC in checkMate 9LA: 3-year clinical update and outcomes in patients with brain metastases or select somatic mutations. J Thorac Oncol (2023) 18:204–22. doi: 10.1016/j.jtho.2022.10.014
53. Tan AC, Tan DSW. Targeted therapies for lung cancer patients with oncogenic driver molecular alterations. J Clin Oncol (2022) 40:611–25. doi: 10.1200/JCO.21.01626
54. Skoulidis F, Li BT, Dy GK, Price TJ, Falchook GS, Wolf J, et al. Sotorasib for lung cancers with KRAS p.G12C mutation. N Engl J Med (2021) 384:2371–81. doi: 10.1056/NEJMoa2103695
55. Shen Y, Li J, Qiang H, Lei Y, Chang Q, Zhong R, et al. A retrospective study for prognostic significance of type II diabetes mellitus and hemoglobin A1c levels in non-small cell lung cancer patients treated with pembrolizumab. Transl Lung Cancer Res (2022) 11:1619–30. doi: 10.21037/tlcr-22-493
56. Li Q, Zhou Q, Zhao S, Wu P, Shi P, Zeng J, et al. KRAS mutation predict response and outcome in advanced non-small cell lung carcinoma without driver alterations receiving PD-1 blockade immunotherapy combined with platinum-based chemotherapy: a retrospective cohort study from China. Transl Lung Cancer Res (2022) 11:2136–47. doi: 10.21037/tlcr-22-655
57. West HJ, McCleland M, Cappuzzo F, Reck M, Mok TS, Jotte RM, et al. Clinical efficacy of atezolizumab plus bevacizumab and chemotherapy in KRAS-mutated non-small cell lung cancer with STK11, KEAP1, or TP53 comutations: subgroup results from the phase III IMpower150 trial. J Immunother Cancer (2022) 10(2):e003027. doi: 10.1136/jitc-2021-003027
58. Wu YL, Cheng Y, Zhou X, Lee KH, Nakagawa K, Niho S, et al. Dacomitinib versus gefitinib as first-line treatment for patients with EGFR-mutation-positive non-small-cell lung cancer (ARCHER 1050): a randomised, open-label, phase 3 trial. Lancet Oncol (2017) 18:1454–66. doi: 10.1016/S1470-2045(17)30608-3
59. Nogami N, Barlesi F, Socinski MA, Reck M, Thomas CA, Cappuzzo F, et al. IMpower150 final exploratory analyses for atezolizumab plus bevacizumab and chemotherapy in key NSCLC patient subgroups with EGFR mutations or metastases in the liver or brain. J Thorac Oncol (2022) 17:309–23. doi: 10.1016/j.jtho.2021.09.014
60. Palazon A, Tyrakis PA, Macias D, Velica P, Rundqvist H, Fitzpatrick S, et al. An HIF-1alpha/VEGF-A axis in cytotoxic T cells regulates tumor progression. Cancer Cell (2017) 32:669–83 e5. doi: 10.1016/j.ccell.2017.10.003
61. Tanaka I, Morise M, Miyazawa A, Kodama Y, Tamiya Y, Gen S, et al. Potential benefits of bevacizumab combined with platinum-based chemotherapy in advanced non-small-cell lung cancer patients with EGFR mutation. Clin Lung Cancer (2020) 21:273–80 e4. doi: 10.1016/j.cllc.2020.01.011
62. Kumagai S, Koyama S, Nishikawa H. Antitumour immunity regulated by aberrant ERBB family signalling. Nat Rev Cancer (2021) 21:181–97. doi: 10.1038/s41568-020-00322-0
63. Mazieres J, Drilon A, Lusque A, Mhanna L, Cortot AB, Mezquita L, et al. Immune checkpoint inhibitors for patients with advanced lung cancer and oncogenic driver alterations: results from the IMMUNOTARGET registry. Ann Oncol (2019) 30:1321–8. doi: 10.1093/annonc/mdz167
64. Dantoing E, Piton N, Salaun M, Thiberville L, Guisier F. Anti-PD1/PD-L1 immunotherapy for non-small cell lung cancer with actionable oncogenic driver mutations. Int J Mol Sci (2021) 22(12):6288. doi: 10.3390/ijms22126288
65. McCoach CE, Rolfo C, Drilon A, Lacouture M, Besse B, Goto K, et al. Hypersensitivity reactions to selpercatinib treatment with or without prior immune checkpoint inhibitor therapy in patients with NSCLC in LIBRETTO-001. J Thorac Oncol (2022) 17:768–78. doi: 10.1016/j.jtho.2022.02.004
66. Koyama S, Akbay EA, Li YY, Aref AR, Skoulidis F, Herter-Sprie GS, et al. STK11/LKB1 deficiency promotes neutrophil recruitment and proinflammatory cytokine production to suppress T-cell activity in the lung tumor microenvironment. Cancer Res (2016) 76:999–1008. doi: 10.1158/0008-5472.CAN-15-1439
67. Garassino MC, Gadgeel S, Novello S, Halmos B, Felip E, Speranza G, et al. Associations of tissue tumor mutational burden and mutational status with clinical outcomes with pembrolizumab plus chemotherapy versus chemotherapy for metastatic NSCLC. JTO Clin Res Rep (2023) 4:100431. doi: 10.1016/j.jtocrr.2022.100431
68. Waldman AD, Fritz JM, Lenardo MJ. A guide to cancer immunotherapy: from T cell basic science to clinical practice. Nat Rev Immunol (2020) 20:651–68. doi: 10.1038/s41577-020-0306-5
69. RaMalingam SS, Balli D, Ciuleanu T-E, Pluzanski A, Lee J-S. M. Schenker ea. 4O Nivolumab (NIVO) + ipilimumab (IPI) versus chemotherapy (chemo) as first-line (1L) treatment for advanced NSCLC (aNSCLC) in CheckMate 227 part 1: Efficacy by KRAS, STK11, and KEAP1 mutation status. Ann Oncol (2021) 32:S1375–S6. doi: 10.1016/j.annonc.2021.10.020
70. Peters S, Cho BC, Luft A, Alatorre-Alexander J, Geater SL, Lim S-W, et al. OA15.04 association between KRAS/STK11/KEAP1 mutations and outcomes in POSEIDON: durvalumab ± Tremelimumab + Chemotherapy in mNSCLC. J Thorac Oncol (2022) 17:S39–41. doi: 10.1016/j.jtho.2022.07.073
71. Vander Heiden MG, Cantley LC, Thompson CB. Understanding the Warburg effect: the metabolic requirements of cell proliferation. Science (2009) 324:1029–33. doi: 10.1126/science.1160809
72. DeBerardinis RJ, Chandel NS. We need to talk about the Warburg effect. Nat Metab (2020) 2:127–9. doi: 10.1038/s42255-020-0172-2
73. Bose S, Zhang C, Le A. Glucose metabolism in cancer: the warburg effect and beyond. Adv Exp Med Biol (2021) 1311:3–15. doi: 10.1007/978-3-030-65768-0_1
74. Pavlova NN, Thompson CB. The emerging hallmarks of cancer metabolism. Cell Metab (2016) 23:27–47. doi: 10.1016/j.cmet.2015.12.006
75. Bartman CR, Weilandt DR, Shen Y, Lee WD, Han Y, TeSlaa T, et al. Slow TCA flux and ATP production in primary solid tumours but not metastases. Nature (2023) 614:349–57. doi: 10.1038/s41586-022-05661-6
76. Cerezo M, Rocchi S. Cancer cell metabolic reprogramming: a keystone for the response to immunotherapy. Cell Death Dis (2020) 11:964. doi: 10.1038/s41419-020-03175-5
77. Zappasodi R, Serganova I, Cohen IJ, Maeda M, Shindo M, Senbabaoglu Y, et al. CTLA-4 blockade drives loss of T(reg) stability in glycolysis-low tumours. Nature (2021) 591:652–8. doi: 10.1038/s41586-021-03326-4
78. Shackelford DB, Abt E, Gerken L, Vasquez DS, Seki A, Leblanc M, et al. LKB1 inactivation dictates therapeutic response of non-small cell lung cancer to the metabolism drug phenformin. Cancer Cell (2013) 23:143–58. doi: 10.1016/j.ccr.2012.12.008
79. Yang R, Li SW, Chen Z, Zhou X, Ni W, Fu DA, et al. Role of INSL4 signaling in sustaining the growth and viability of LKB1-inactivated lung cancer. J Natl Cancer Inst (2019) 111:664–74. doi: 10.1093/jnci/djy166
80. Zhang CS, Hawley SA, Zong Y, Li M, Wang Z, Gray A, et al. Fructose-1,6-bisphosphate and aldolase mediate glucose sensing by AMPK. Nature (2017) 548:112–6. doi: 10.1038/nature23275
81. Lin SC, Hardie DG. AMPK: sensing glucose as well as cellular energy status. Cell Metab (2018) 27:299–313. doi: 10.1016/j.cmet.2017.10.009
82. Jiang S, Wang Y, Luo L, Shi F, Zou J, Lin H, et al. AMP-activated protein kinase regulates cancer cell growth and metabolism via nuclear and mitochondria events. J Cell Mol Med (2019) 23:3951–61. doi: 10.1111/jcmm.14279
83. van Veelen W, Korsse SE, van de Laar L, Peppelenbosch MP. The long and winding road to rational treatment of cancer associated with LKB1/AMPK/TSC/mTORC1 signaling. Oncogene (2011) 30:2289–303. doi: 10.1038/onc.2010.630
84. Shackelford DB, Vasquez DS, Corbeil J, Wu S, Leblanc M, Wu CL, et al. mTOR and HIF-1alpha-mediated tumor metabolism in an LKB1 mouse model of Peutz-Jeghers syndrome. Proc Natl Acad Sci U.S.A. (2009) 106:11137–42. doi: 10.1073/pnas.0900465106
85. Faubert B, Vincent EE, Griss T, Samborska B, Izreig S, Svensson RU, et al. Loss of the tumor suppressor LKB1 promotes metabolic reprogramming of cancer cells via HIF-1alpha. Proc Natl Acad Sci U S A (2014) 111:2554–9. doi: 10.1073/pnas.1312570111
86. Sasaki H, Shitara M, Yokota K, Hikosaka Y, Moriyama S, Yano M, et al. Overexpression of GLUT1 correlates with Kras mutations in lung carcinomas. Mol Med Rep (2012) 5:599–602. doi: 10.3892/mmr.2011.736
87. Pupo E, Avanzato D, Middonti E, Bussolino F, Lanzetti L. KRAS-driven metabolic rewiring reveals novel actionable targets in cancer. Front Oncol (2019) 9:848. doi: 10.3389/fonc.2019.00848
88. Mukhopadhyay S, Vander Heiden MG, McCormick F. The Metabolic Landscape of RAS-Driven Cancers from biology to therapy. Nat Cancer (2021) 2:271–83. doi: 10.1038/s43018-021-00184-x
89. Singleton DC, Macann A, Wilson WR. Therapeutic targeting of the hypoxic tumour microenvironment. Nat Rev Clin Oncol (2021) 18:751–72. doi: 10.1038/s41571-021-00539-4
90. Sasidharan Nair V, Saleh R, Toor SM, Cyprian FS, Elkord E. Metabolic reprogramming of T regulatory cells in the hypoxic tumor microenvironment. Cancer Immunol Immunother (2021) 70:2103–21. doi: 10.1007/s00262-020-02842-y
91. Angelin A, Gil-de-Gomez L, Dahiya S, Jiao J, Guo L, Levine MH, et al. Foxp3 reprograms T cell metabolism to function in low-glucose, high-lactate environments. Cell Metab (2017) 25:1282–93 e7. doi: 10.1016/j.cmet.2016.12.018
92. Haas R, Smith J, Rocher-Ros V, Nadkarni S, Montero-Melendez T, D’Acquisto F, et al. Lactate regulates metabolic and pro-inflammatory circuits in control of T cell migration and effector functions. PloS Biol (2015) 13:e1002202. doi: 10.1371/journal.pbio.1002202
93. Watson MJ, Vignali PDA, Mullett SJ, Overacre-Delgoffe AE, Peralta RM, Grebinoski S, et al. Metabolic support of tumour-infiltrating regulatory T cells by lactic acid. Nature (2021) 591:645–51. doi: 10.1038/s41586-020-03045-2
94. Fischer K, Hoffmann P, Voelkl S, Meidenbauer N, Ammer J, Edinger M, et al. Inhibitory effect of tumor cell-derived lactic acid on human T cells. Blood (2007) 109:3812–9. doi: 10.1182/blood-2006-07-035972
95. Payen VL, Mina E, Van Hee VF, Porporato PE, Sonveaux P. Monocarboxylate transporters in cancer. Mol Metab (2020) 33:48–66. doi: 10.1016/j.molmet.2019.07.006
96. Faubert B, Li KY, Cai L, Hensley CT, Kim J, Zacharias LG, et al. Lactate metabolism in human lung tumors. Cell (2017) 171:358–71 e9. doi: 10.1016/j.cell.2017.09.019
97. Bourouh M, Marignani PA. The tumor suppressor kinase LKB1: metabolic nexus. Front Cell Dev Biol (2022) 10:881297. doi: 10.3389/fcell.2022.881297
98. Rais R, Lemberg KM, Tenora L, Arwood ML, Pal A, Alt J, et al. Discovery of DRP-104, a tumor-targeted metabolic inhibitor prodrug. Sci Adv (2022) 8:eabq5925. doi: 10.1126/sciadv.abq5925
99. Best SA, Gubser PM, Sethumadhavan S, Kersbergen A, Negron Abril YL, Goldford J, et al. Glutaminase inhibition impairs CD8 T cell activation in STK11-/Lkb1-deficient lung cancer. Cell Metab (2022) 34:874–87 e6. doi: 10.1016/j.cmet.2022.04.003
100. Celiktas M, Tanaka I, Tripathi SC, Fahrmann JF, Aguilar-Bonavides C, Villalobos P, et al. Role of CPS1 in cell growth, metabolism and prognosis in LKB1-inactivated lung adenocarcinoma. J Natl Cancer Inst (2017) 109:1–9. doi: 10.1093/jnci/djw231
101. Kim J, Hu Z, Cai L, Li K, Choi E, Faubert B, et al. CPS1 maintains pyrimidine pools and DNA synthesis in KRAS/LKB1-mutant lung cancer cells. Nature (2017) 546:168–72. doi: 10.1038/nature22359
102. Ricciuti B, Arbour KC, Lin JJ, Vajdi A, Vokes N, Hong L, et al. Diminished efficacy of programmed death-(Ligand)1 inhibition in STK11- and KEAP1-mutant lung adenocarcinoma is affected by KRAS mutation status. J Thorac Oncol (2022) 17:399–410. doi: 10.1016/j.jtho.2021.10.013
103. Cruzat V, Macedo Rogero M, Noel Keane K, Curi R, Newsholme P. Glutamine: metabolism and immune function, supplementation and clinical translation. Nutrients (2018) 10(11):1564. doi: 10.3390/nu10111564
104. Galan-Cobo A, Sitthideatphaiboon P, Qu X, Poteete A, Pisegna MA, Tong P, et al. LKB1 and KEAP1/NRF2 pathways cooperatively promote metabolic reprogramming with enhanced glutamine dependence in KRAS-mutant lung adenocarcinoma. Cancer Res (2019) 79:3251–67. doi: 10.1158/0008-5472.CAN-18-3527
105. Martin-Bernabe A, Cortes R, Lehmann SG, Seve M, Cascante M, Bourgoin-Voillard S. Quantitative proteomic approach to understand metabolic adaptation in non-small cell lung cancer. J Proteome Res (2014) 13:4695–704. doi: 10.1021/pr500327v
106. Rojo de la Vega M, Chapman E, Zhang DD. NRF2 and the hallmarks of cancer. Cancer Cell (2018) 34:21–43. doi: 10.1016/j.ccell.2018.03.022
107. Hayes JD, Dinkova-Kostova AT, Tew KD. Oxidative stress in cancer. Cancer Cell (2020) 38:167–97. doi: 10.1016/j.ccell.2020.06.001
108. Cancer Genome Atlas Research N. Comprehensive genomic characterization of squamous cell lung cancers. Nature (2012) 489:519–25. doi: 10.1038/nature11404
109. Zhao J, Lin X, Meng D, Zeng L, Zhuang R, Huang S, et al. Nrf2 mediates metabolic reprogramming in non-small cell lung cancer. Front Oncol (2020) 10:578315. doi: 10.3389/fonc.2020.578315
110. Tanaka I, Dayde D, Tai MC, Mori H, Solis LM, Tripathi SC, et al. SRGN-triggered aggressive and immunosuppressive phenotype in a subset of TTF-1-negative lung adenocarcinomas. J Natl Cancer Inst (2022) 114:290–301. doi: 10.1093/jnci/djab183
111. Fahrmann JF, Tanaka I, Irajizad E, Mao X, Dennison JB, Murage E, et al. Mutational activation of the NRF2 pathway upregulates kynureninase resulting in tumor immunosuppression and poor outcome in lung adenocarcinoma. Cancers (Basel) (2022) 14(10):2543. doi: 10.3390/cancers14102543
112. Mitsuishi Y, Taguchi K, Kawatani Y, Shibata T, Nukiwa T, Aburatani H, et al. Nrf2 redirects glucose and glutamine into anabolic pathways in metabolic reprogramming. Cancer Cell (2012) 22:66–79. doi: 10.1016/j.ccr.2012.05.016
113. Romero R, Sayin VI, Davidson SM, Bauer MR, Singh SX, LeBoeuf SE, et al. Keap1 loss promotes Kras-driven lung cancer and results in dependence on glutaminolysis. Nat Med (2017) 23:1362–8. doi: 10.1038/nm.4407
114. Hassanein M, Hoeksema MD, Shiota M, Qian J, Harris BK, Chen H, et al. SLC1A5 mediates glutamine transport required for lung cancer cell growth and survival. Clin Cancer Res (2013) 19:560–70. doi: 10.1158/1078-0432.CCR-12-2334
115. Koppula P, Olszewski K, Zhang Y, Kondiparthi L, Liu X, Lei G, et al. KEAP1 deficiency drives glucose dependency and sensitizes lung cancer cells and tumors to GLUT inhibition. iScience (2021) 24:102649. doi: 10.1016/j.isci.2021.102649
116. Sitthideatphaiboon P, Galan-Cobo A, Negrao MV, Qu X, Poteete A, Zhang F, et al. STK11/LKB1 mutations in NSCLC are associated with KEAP1/NRF2-dependent radiotherapy resistance targetable by glutaminase inhibition. Clin Cancer Res (2021) 27:1720–33. doi: 10.1158/1078-0432.CCR-20-2859
117. Brosnan ME, Brosnan JT. Orotic acid excretion and arginine metabolism. J Nutr (2007) 137:1656S–61S. doi: 10.1093/jn/137.6.1656S
118. Wang Y, Bai C, Ruan Y, Liu M, Chu Q, Qiu L, et al. Coordinative metabolism of glutamine carbon and nitrogen in proliferating cancer cells under hypoxia. Nat Commun (2019) 10:201. doi: 10.1038/s41467-018-08033-9
119. Ji X, Qian J, Rahman SMJ, Siska PJ, Zou Y, Harris BK, et al. xCT (SLC7A11)-mediated metabolic reprogramming promotes non-small cell lung cancer progression. Oncogene (2018) 37:5007–19. doi: 10.1038/s41388-018-0307-z
120. Jyotsana N, Ta KT, DelGiorno KE. The role of cystine/glutamate antiporter SLC7A11/xCT in the pathophysiology of cancer. Front Oncol (2022) 12:858462. doi: 10.3389/fonc.2022.858462
121. Fukumura D, Kloepper J, Amoozgar Z, Duda DG, Jain RK. Enhancing cancer immunotherapy using antiangiogenics: opportunities and challenges. Nat Rev Clin Oncol (2018) 15:325–40. doi: 10.1038/nrclinonc.2018.29
122. Negrao MV, Araujo HA, Lamberti G, Cooper AJ, Akhave NS, Zhou T, et al. Co-mutations and KRAS G12C inhibitor efficacy in advanced NSCLC. Cancer Discov. (2023) 13(7):1556–71. doi: 10.1158/2159-8290.CD-22-1420
123. Offin M, Chan JM, Tenet M, Rizvi HA, Shen R, Riely GJ, et al. Concurrent RB1 and TP53 Alterations Define a Subset of EGFR-Mutant Lung Cancers at risk for Histologic Transformation and Inferior Clinical Outcomes. J Thorac Oncol (2019) 14:1784–93. doi: 10.1016/j.jtho.2019.06.002
124. Amgen. A Study of Sotorasib (AMG 510) in Participants With Stage IV NSCLC Whose Tumors Harbor a KRAS p.G12C Mutation in Need of First-line Treatment (2022). Available at: https://classic.clinicaltrials.gov/show/NCT04933695.
125. Inc. MT. Phase 1/2 study of MRTX849 in patients with cancer having a KRAS G12C mutation KRYSTAL-1 (2019). Available at: https://classic.clinicaltrials.gov/show/NCT03785249.
126. Garassino M, Fondazione IRCCS Istituto Nazionale dei Tumori M. Metformin plus/minus fasting mimicking diet to target the metabolic vulnerabilities of LKB1-inactive lung adenocarcinoma (2018). Available at: https://classic.clinicaltrials.gov/show/NCT03709147.
127. Institute NC. Testing whether cancers with specific mutations respond better to glutaminase inhibitor, telaglenastat hydrochloride, anti-cancer treatment, beGIN study (2019). Available at: https://classic.clinicaltrials.gov/show/NCT03872427.
128. Dracen Pharmaceuticals I. Study to investigate DRP-104 in adults with advanced solid tumors (2020). Available at: https://classic.clinicaltrials.gov/show/NCT04471415.
129. ClinicalTrials.gov. Canadian profiling and targeted agent utilization trial (CAPTUR). Available at: https://www.clinicaltrials.gov/study/NCT03297606 (Accessed June, 2023).
130. ClinicalTrials.gov. A study to investigate the safety, tolerability, and preliminary anti-tumor activity of bemcentinib in combination with pembrolizumab plus pemetrexed and carboplatin in adult participants with untreated non-squamous non-small cell lung cancer. Available at: https://www.clinicaltrials.gov/study/NCT05469178 (Accessed June, 2023).
131. ClinicalTrials.gov. A Phase Ib Study to Evaluate the Safety and Preliminary Efficacy of IL6-receptor Antibody Sarilumab in Combination With antiPD1 Antibody Cemiplimab for Patients With Non-small Cell Lung Cancer. Available at: https://www.clinicaltrials.gov/study/NCT05704634 (Accessed June, 2023).
132. ClinicalTrials.gov. Study of MGY825 in patients with advanced non-small cell lung cancer. Available at: https://www.clinicaltrials.gov/study/NCT05275868 (Accessed June, 2023).
133. ClinicalTrials.gov. Study of efficacy and safety of JDQ443 single-agent as first-line treatment for patients with locally advanced or metastatic KRAS G12C- mutated non-small cell lung cancer with a PD-L1 expression < 1% or a PD-L1 expression ≥ 1% and an STK11 co-mutation. Available at: https://www.clinicaltrials.gov/study/NCT05445843 (Accessed June, 2023).
134. ClinicalTrials.gov. A Study of JAB-21822 in Advanced or Metastatic NSCLC With KRAS p.G12C and STK11 Co-mutation and Wild-type KEAP1. Available at: https://www.clinicaltrials.gov/study/NCT05276726 (Accessed June, 2023).
135. Guo Q, Liu Z, Jiang L, Liu M, Ma J, Yang C, et al. Metformin inhibits growth of human non-small cell lung cancer cells via liver kinase B-1-independent activation of adenosine monophosphate-activated protein kinase. Mol Med Rep (2016) 13:2590–6. doi: 10.3892/mmr.2016.4830
136. Moro M, Caiola E, Ganzinelli M, Zulato E, Rulli E, Marabese M, et al. Metformin enhances cisplatin-induced apoptosis and prevents resistance to cisplatin in co-mutated KRAS/LKB1 NSCLC. J Thorac Oncol (2018) 13:1692–704. doi: 10.1016/j.jtho.2018.07.102
Keywords: immune checkpoint blockade, NSCLC, LKB1, KEAP1, metabolic barriers, glycolysis, glutaminolysis, PD-1/PD-L1 inhibitors
Citation: Tanaka I, Koyama J, Itoigawa H, Hayai S and Morise M (2023) Metabolic barriers in non-small cell lung cancer with LKB1 and/or KEAP1 mutations for immunotherapeutic strategies. Front. Oncol. 13:1249237. doi: 10.3389/fonc.2023.1249237
Received: 28 June 2023; Accepted: 08 August 2023;
Published: 22 August 2023.
Edited by:
Satyendra Chandra Tripathi, All India Institute of Medical Sciences Nagpur, IndiaReviewed by:
Makoto Kobayashi, Fukushima Medical University, JapanCopyright © 2023 Tanaka, Koyama, Itoigawa, Hayai and Morise. This is an open-access article distributed under the terms of the Creative Commons Attribution License (CC BY). The use, distribution or reproduction in other forums is permitted, provided the original author(s) and the copyright owner(s) are credited and that the original publication in this journal is cited, in accordance with accepted academic practice. No use, distribution or reproduction is permitted which does not comply with these terms.
*Correspondence: Ichidai Tanaka, aWNoaWRhaUBtZWQubmFnb3lhLXUuYWMuanA=
Disclaimer: All claims expressed in this article are solely those of the authors and do not necessarily represent those of their affiliated organizations, or those of the publisher, the editors and the reviewers. Any product that may be evaluated in this article or claim that may be made by its manufacturer is not guaranteed or endorsed by the publisher.
Research integrity at Frontiers
Learn more about the work of our research integrity team to safeguard the quality of each article we publish.