- Department of Bioactive Material Sciences, Jeonbuk National University, Jeonju, Jeollabuk-do, Republic of Korea
Casein Kinase 1 (CK1) is a family of serine/threonine protein kinases that play a crucial role in various cellular processes, including cell proliferation, survival, and metabolism. The dysregulation of CK1 expression has been implicated in the development and progression of several types of cancer, making it an attractive target for anticancer therapy. In this review, we provide an overview of the current strategies employed to target CK1 for cancer therapy and discuss the future perspectives in this field. We highlight the different approaches, including small molecule inhibitors, RNA interference, genome editing, and immunotherapies, which hold immense potential for targeted modulation of CK1 activity in cancer cells. Furthermore, we discuss the challenges associated with targeting CK1 and propose potential strategies to overcome these hurdles. Overall, targeting CK1 holds great promise as a therapeutic strategy for cancer treatment, and further research in this area is warranted.
1 Introduction
Casein kinase 1 (CK1) has emerged as a promising target for cancer therapy, with growing evidence linking its altered expression and activity to carcinogenesis and cancer progression (1–6). CK1 belongs to a distinct subgroup of the family of Ser/Thr protein kinases, comprising six isoforms in humans: CK1α, γ1, γ2, γ3, δ, and ϵ. It functions as an autonomous monomeric enzyme, independent of cofactors, and its activity can be regulated by the autophosphorylation of carboxyterminal residues (7, 8).
CK1 plays a crucial role in various cellular processes, including the regulation of cell, survival/apoptosis signaling pathways, and cell division (9). Notably, CK1 isoforms have been associated with cell cycle progression (10), chromosome segregation (11), apoptosis (12), DNA repair (13), the circadian rhythm (14), ribosome biogenesis (15), vesicle trafficking (16), and modulation of signaling pathways such as p53 (7), Wnt, Shh (5), and Hippo (17). Recent advances in our understanding of CK1 have shed light on its involvement in protein phosphorylation processes and its impact on signaling pathways mediated by the epidermal growth factor receptor, estrogen receptor, G protein-coupled estrogen receptor (10). Targeting CK1 holds significant promise as a therapeutic strategy for cancer treatment.
The aim of this review is to provide a comprehensive overview of the current strategies employed in targeting CK1 for cancer therapy. It will explore different approaches, including small-molecule inhibitors, RNA interference (RNAi), and immunotherapies. Additionally, it will discuss the challenges associated with the targeting of CK1 and highlight future perspectives in this field. By elucidating the current state of CK1-targeted cancer therapies and outlining potential future directions, this review aims to contribute to the advancement of effective and innovative strategies for cancer treatment.
2 CK1 isoforms and their roles in cancer
CK1 isoforms, including CK1α, CK1δ, CK1ϵ, and CK1γ, play diverse roles in cancer development and progression (7) (Figure 1). Dysregulation of these isoforms contributes to various cancer-related processes, including Wnt/β-catenin signaling, disruption of the circadian rhythm, DNA repair, cell cycle progression, and stability of oncogenic proteins. Targeting specific CK1 isoforms represents a promising avenue for the development of novel anti-cancer agents.
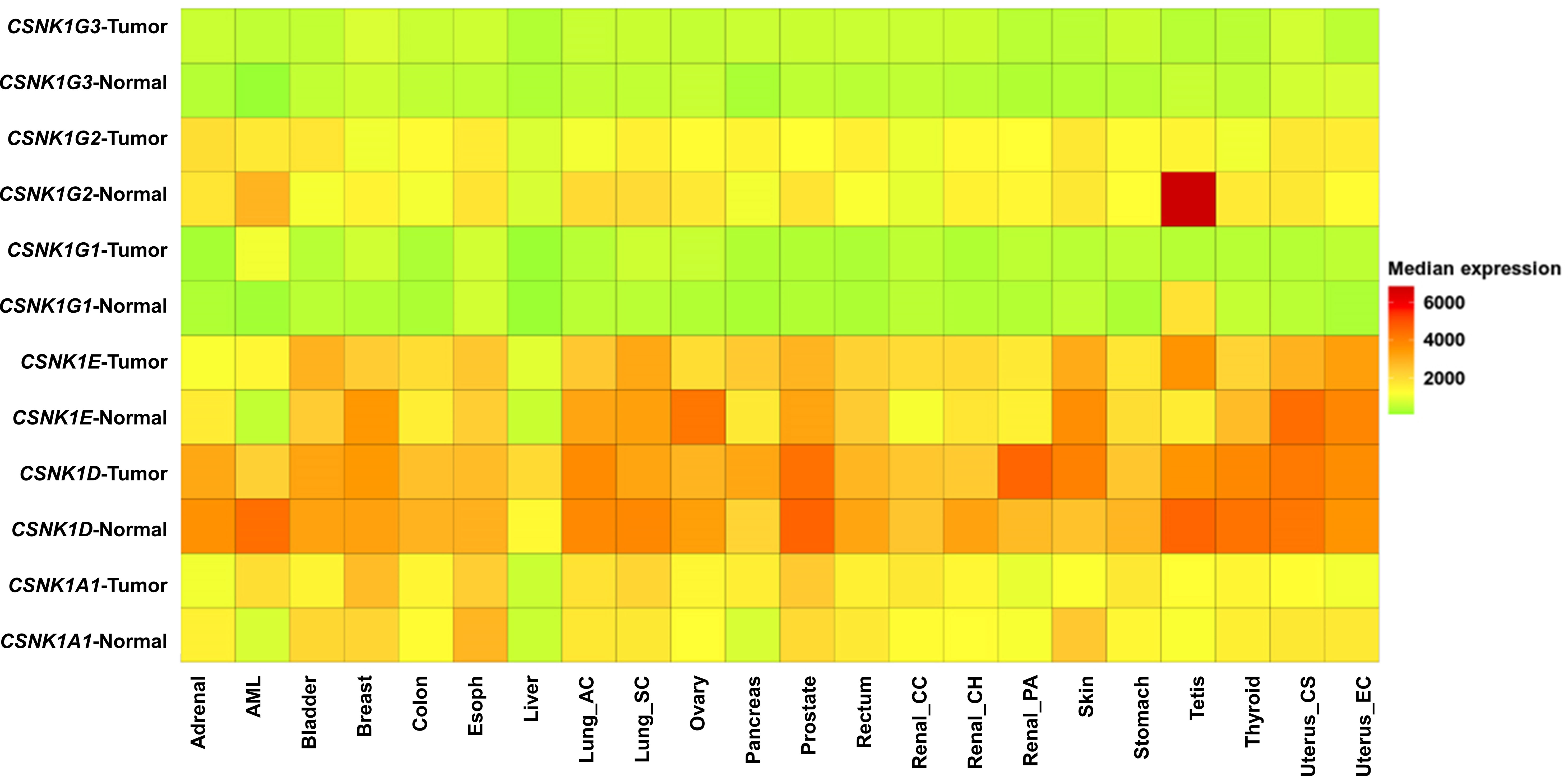
Figure 1 Heatmap comparing gene expression in normal human tissues and matched tumor tissues of various cancer types, including adrenal, acute myeloid leukemia (AML), bladder, breast, colon, esophagus, liver, lung adenocarcinoma (lung_ac), lung squamous cell carcinoma (lung_sc), ovary, pancreas, prostate, rectum, renal clear cell carcinoma (renal_cc), renal chromophobe (renal_ch), renal papillary carcinoma (renal_pa), skin, stomach, testis, thyroid, uterus carcinosarcoma (uterus_cs), and uterus endometrioid carcinoma (uterus_ec). Gene expression data was generated from GTEx, TCGA, and TARGET databases using TNMplot (18). The heatmap represents relative gene expression levels, with higher expression indicated by warmer colors and lower expression by cooler colors.
2.1 CK1α
CK1α is one of the most extensively studied isoforms of CK1 and has been implicated in several malignancies (Figure 2A). It plays a critical role in regulating the Wnt/β-catenin signaling pathway, which is frequently dysregulated in cancer. CK1α phosphorylates β-catenin, leading to its degradation and inhibition of downstream Wnt signaling. Dysregulation of CK1α in cancer leads to abnormal accumulation of β-catenin and activation of Wnt signaling, thus facilitating tumor growth and metastasis (5). CK1α has been shown to play a role in the regulation of the immune response in cancer. CK1α expression is altered in response to interferon-γ (19) and is required for the expression of pseudo-Programmed death-ligand 1 (20, 21), an immune checkpoint protein that is often overexpressed in cancer cells to evade immune surveillance (22).CK1α has also been implicated in the regulation of DNA damage response pathways in cancer cells (23, 24) and in mouse embryonic stem cells (25), suggesting a potential role in promoting genomic instability.
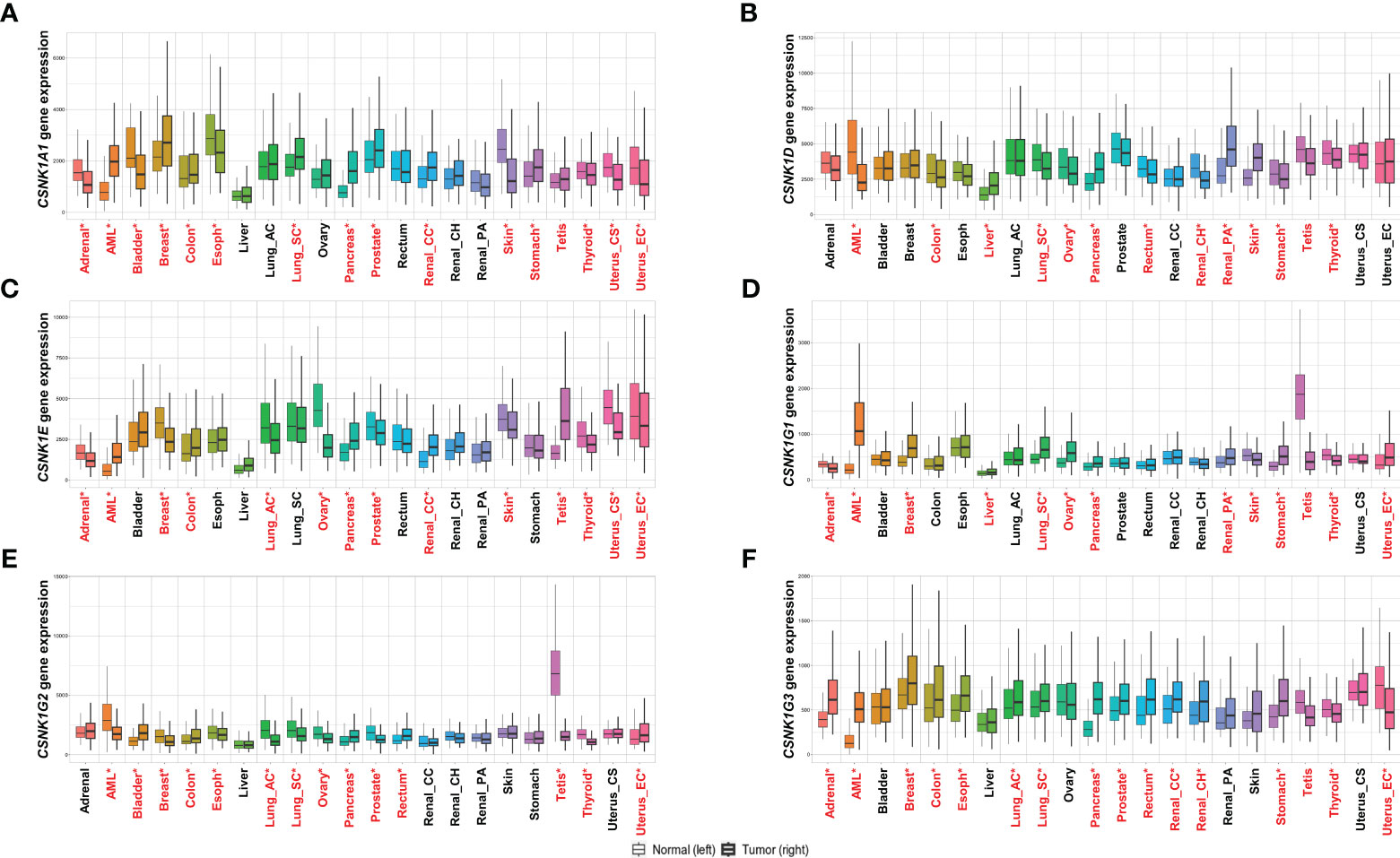
Figure 2 Comparison of the expression of each casein kinase (CKN) isoform encoded gene in humans, including (A) CNSK1A1, (B) CSNK1D, (C) CNSK1E, (D) CSNK1G1, (E) CNSK1G2, and (F) CNSK1G3, in normal and tumor tissues of various cancer types, including adrenal, acute myeloid leukemia (AML), bladder, breast, colon, esophagus, liver, lung adenocarcinoma (lung_ac), lung squamous cell carcinoma (lung_sc), ovary, pancreas, prostate, rectum, renal clear cell carcinoma (renal_cc), renal chromophobe (renal_ch), renal papillary carcinoma (renal_pa), skin, stomach, testis, thyroid, uterus carcinosarcoma (uterus_cs), and uterus endometrioid carcinoma (uterus_ec). Gene expression data was obtained from the GTEx, TCGA, and TARGET databases using TNMplot (18). Significant differences are identified by Mann-Whitney U test are denoted by red asterisks (*P<0.05).
2.2 CK1δ and CK1ϵ
CK1δ and CK1ϵ are closely related isoforms that share a high degree of sequence homology. Both isoforms have been associated with carcinogenesis and cancer progression (Figures 2B, C). CK1δ and CK1ϵ participate in the regulation of the circadian rhythm, and the disruption of circadian clock genes has been linked to an increased risk of cancer. Dysregulated CK1δ and CK1ϵ activity can perturb the normal circadian rhythm, leading to disturbances in cellular processes, including DNA repair, cell cycle progression, and apoptosis, which contribute to tumorigenesis.
CK1δ continues to be an important target for cancer therapy, particularly in triple-negative breast cancer (26, 27). Rosenberg et al. demonstrated that CSNK1D was overexpressed in human breast cancers and established a link between CK1δ and Wnt/β-catenin signaling pathway, whereby inhibition CK1δ blocked the localization of β-catenin and the transcriptional activity of T cell factor, resulting in the apoptosis of breast cancer cells (26). In colorectal cancer, CK1 and expression of CK1δ and ε are altered compared to the normal bowel epithelium, and high CK1ε expression is significantly correlated with prolonged patient survival. Mutations within exon 3 of CK1δ have also been detected in colorectal tumors (28). CK1ε has also been implicated in the regulation of immune responses in cancer. The study by Zhou et al. found that CK1ε interacted with and phosphorylated TRAF3, which promoted the production of type I interferon, a key component of the antiviral immune response. The phosphorylation of TRAF3 by CK1 ε led to the recruitment of another kinase called TBK1 to TRAF3, suggesting that CK1 plays a significant role in regulating the innate immune response to viral infections and provides a novel mechanism of immunoregulation (29). CK1ε has also been shown to play a role in the regulation of the Hippo signaling pathway, a key pathway involved in the regulation of cell proliferation and apoptosis. Its aberrant expression has been identified in a variety of cancers, including breast and ovarian cancers (7, 30). CK1ε plays a crucial role in tumor cell proliferation via interacting and phosphorylating 4E-BP1 at T41 and T50, which is essential for 4E-BP1 inactivation, increasing mRNA translation, and cell proliferation (31). Moreover, CK1δ and CK1ϵ have been implicated in modulating the stability and activity of key oncogenic proteins, such as p53, c-Myc, and NF-κB, further highlighting their roles in cancer biology.
2.3 CK1γ
CK1γ1,2,3 are less well-characterized isoforms compared to CK1α, CK1δ, and CK1ϵ. However, emerging evidence suggests its involvement in cancer-related processes (9, 32). The role of CK1γ in cancer remains unclear, although recent studies have suggested a potential role in the regulation of autophagy (9), a process by which cells recycle damaged or unwanted components. CK1γ has been implicated in the regulation of cell cycle progression and mitotic spindle formation, which are crucial for maintaining genomic stability. Dysregulation of CK1γ has been observed in different types of cancer, including breast (9) and lung cancer (4) (Figures 2D–F). CK1γ has also been shown to regulate the activity of the transcription factor NF-κB, a key regulator of immune responses, suggesting a potential role in the regulation of immune surveillance in cancer.
Understanding the specific roles of each CK1 isoform in cancer is essential for the development of isoform-specific therapeutic strategies. Targeting individual isoforms can help modulate key signaling pathways, restore circadian rhythm, and disrupt interactions with oncogenic proteins, thus inhibiting tumor growth and metastasis. However, additional research is needed to elucidate the precise functions of the CK1 isoforms in diverse types of cancer and to develop isoform-specific inhibitors with optimal efficacy and safety profiles. Validating the potency of specific CK1 isoforms in defined tumor types will be crucial in the future, as their roles can vary and can sometimes exert opposite effects. This validation will enhance our understanding and enable the development of targeted therapies tailored to specific tumors.
3 Small molecule inhibitors targeting CK1
3.1 Development of CK1-specific inhibitors
The development of CK1-specific inhibitors has been driven by the need for isoform-selective targeting to maximize therapeutic efficacy and minimize off-target effects. High-throughput screening, studies of structure-activity relationships, and virtual screening techniques have facilitated the identification and optimization of small molecules with high affinity and selectivity for CK1 isoforms. Several chemical scaffolds, including arylimidazoles, benzimidazoles, benzothiazoles, isoxazoles, purines, pyrazoles, BTX-A51, CKI-7, D4476, IC261, pyrido[3,4-g]quinazoline, PF-4800567, PF-670462, and TG003, have been explored as CK1 inhibitors (33).
3.2 Mechanisms of action
Small-molecule inhibitors targeting CK1 exert their effects through multiple mechanisms. The dominant molecular and cellular mechanisms that contribute to the effects of CK1 inhibition in preclinical models and clinical trials have not been fully elucidated. These mechanisms encompass both cell-intrinsic processes and intricate interactions with the tumor microenvironment and cell-to-cell communication. CK1 serves as a critical regulator of various signaling pathways, including Wnt, Hedgehog, and Yap/Taz (5, 7, 8, 34). A comprehensive understanding of these mechanisms will provide valuable insights into the therapeutic implications of CK1 inhibition and will pave the way for the development of targeted interventions in cancer therapy. A common mechanism is the direct inhibition of CK1 kinase activity by binding to the ATP-binding pocket of the kinase domain (35). These inhibitors compete with ATP for binding, thus preventing the phosphorylation of CK1 substrates. Another mechanism involves allosteric modulation of CK1 activity by binding to distinct sites outside the ATP-binding pocket, inducing conformational changes that inhibit kinase activity (36). Additionally, some inhibitors promote protein degradation by targeting specific CK1 substrates, leading to the down-regulation of oncogenic signaling pathways.
3.3 Efficacy in clinical studies
In the clinical setting, the development of CK1 inhibitors is still in its preliminary stages. Despite the absence of selective CK1 inhibitors in clinical trials, umbralisib, a dual inhibitor of PI3Kδ and CK1ϵ, is currently being evaluated in clinical trials for patients with chronic lymphocytic leukemia (CLL) (37) and non-Hodgkin lymphomas (NHL) (38). CK1ϵ has been observed to be upregulated in CLL, and inhibition of CK1ϵ has been demonstrated to prevent chemotaxis of cancer cells in CLL (39). Additionally, CK1ϵ inhibition has also displayed encouraging results in preclinical investigations involving various hematological malignancies, such as NHL, myelodysplastic syndrome (MDS), acute myeloid leukemia (AML), and multiple myeloma (MM) (40). The activation of the p53 pathway by inhibition of CK1α has shown promising preclinical activities in MDS, AML, and MM, leading to the establishment of clinical trials for the first CK1α inhibitor (BTX-A51, NCT04243785). The results from these studies will provide valuable insights into the therapeutic potential of CK1 inhibitors in a clinical setting.
3.4 Limitations of small molecule inhibitors
Inhibitor resistance is a common challenge in cancer treatment, including kinase inhibitors (KIs) (41). Mutations that impair drug binding or interfere with necessary conformational changes can lead to resistance to KIs. For example, the T315I mutation confers resistance to certain Bcr-Abl tyrosine kinase inhibitors (TKIs) (42). To overcome resistance, third-generation TKIs like ponatinib have been developed, but they may have safety concerns (43, 44). Dysregulation of signaling pathways is a common mechanism contributing to drug resistance. Phospho-proteomics analysis has revealed that compensatory pathways, such as epithelial-to-mesenchymal transition and cell adhesion, contribute to resistance in hematological malignancies patients treated with multi-KIs sorafenib (45). Distinct types of inhibitors target specific binding sites, such as type III (within ATP pocket) and type IV (substrate-binding domain) inhibitors, and physiological mechanisms of kinases. These inhibitors have been approved by the FDA for cancer treatment (41).
4 RNA interference-based approaches and genome editing technologies
RNA interference (RNAi) has emerged as a powerful tool for silencing specific genes, including CSNK1, offering a potential therapeutic avenue for cancer treatment. RNAi-based approaches utilize small RNA molecules, such as small interfering RNAs (siRNAs) or short hairpin RNAs (shRNAs), to specifically target and degrade CSNK1 mRNA, thereby reducing the expression of CK1 protein.
The application of RNAi-based approaches to inhibit CSNK1 has several advantages. First, it enables precise and selective targeting of CSNK1, allowing isoform-specific down-regulation. This isoform specificity is crucial, as different CSNK1 isoforms may have distinct functions and contributions to tumorigenesis in diverse types of cancer (Figure 2). By selectively silencing specific isoforms, RNAi-based strategies can effectively disrupt CK1-mediated signaling pathways implicated in cancer progression (46). Second, RNAi-based approaches offer the potential for systemic administration and broad delivery to various tumor sites. The development of efficient delivery systems, such as nanoparticles or viral vectors, has improved the delivery and stability of RNAi molecules, enabling their effective transport to tumor cells (47, 48). This systemic delivery approach enhances the feasibility and applicability of RNAi-based therapeutics for the inhibition of CSNK1 in cancer treatment (49). Moreover, the combinatory potential of RNAi-based approaches with other treatment modalities holds promise for enhanced therapeutic efficacy. By targeting CSNK1 along with other key signaling molecules or pathways, synergistic effects may be achieved, leading to more profound and sustained anti-tumor responses. Additionally, combining RNAi-based approaches with conventional chemotherapy, radiotherapy, or targeted therapies will potentially overcome drug resistance and improve treatment outcomes.
However, several challenges need to be addressed to fully exploit the potential of RNAi-based approaches for the inhibition of CSNK1 in cancer therapy. Efficient delivery of RNAi molecules to tumor cells while minimizing off-target effects remains a critical hurdle. Further optimization of delivery systems, including improving stability, cellular uptake, and intracellular localization, is necessary. Furthermore, the identification of reliable and predictive biomarkers for patient stratification and monitoring of treatment response is essential for the successful implementation of RNAi-based therapies. Biomarkers that can indicate CK1 expression levels, isoform-specific alterations, or downstream pathway activity will facilitate personalized treatment strategies and improve patient outcomes.
In addition to RNAi-based approaches, the use of genome editing technologies, such as CRISPR/Cas9, holds immense potential for targeted modifications of the CSNK1 gene. The remarkable efficiency and simplicity of CRISPR/Cas9 enable precise DNA cleavage at specific sites within the CSNK1 gene, facilitating the manipulation of CK1 expression and function with high precision. By introducing double-stranded breaks, CRISPR/Cas9 enables targeted gene disruption, knockout, or insertion of specific sequences to modulate CSNK1 expression or function (50). Similarly, zinc finger nucleases (ZFNs), transcription-activator-like effector nucleases (TALENs) (51), Homing Endonucleases (52), and CRISPR-Cpf1 (53) offer alternative platforms for site-specific DNA cleavage and gene editing, allowing precise modifications of the CSNK1 gene.
These genome editing technologies will provide opportunities to investigate the functional consequences of CSNK1 modulation and uncover the intricate roles of CK1 in cancer development and progression. By introducing specific mutations or alterations in CSNK1, researchers will be able to elucidate the impact of these modifications on downstream signaling pathways and cellular processes involved in cancer. In addition, these techniques will enable the exploration of potential therapeutic targets and the development of personalized treatment strategies based on specific genetic alterations of CSNK1.
5 Immunotherapies targeting CK1
Although the roles of CK1 in immunology are not yet fully understood, it is increasingly recognized that CK1 plays a significant role in the modulation of immune responses, particularly through its involvement in the Wnt signaling pathway (7, 29, 40, 54, 55). This emerging knowledge presents an exciting opportunity to explore the potential of developing immunotherapeutic approaches targeting CK1.
The Wnt signaling pathway is known to be critical in various aspects of the regulation of immune responses, including T cell development, differentiation, and activation (56–58). CK1, as a key regulator of the Wnt pathway, influences immune cell function and can impact the outcome of immune responses (59). Therefore, investigating the precise role of CK1 in immune regulation and exploring its potential as a target for immunotherapy holds great promise. Immunotherapies targeting CK1 could offer novel strategies to modulate immune responses in the context of cancer and other immune-related diseases (39). By selectively targeting CK1, it may be possible to manipulate the Wnt signaling pathway and enhance immune cell activation or suppress immune tolerance mechanisms, leading to improved anti-tumor or immune modulatory effects.
Immunotherapies targeting CK1 have several advantages. They have high specificity, allowing for selective targeting of CK1 overexpressing tumors while sparing healthy tissues. Immunotherapies can also elicit potent and durable anti-tumor responses, providing long-term control of cancer progression. In addition, they offer the possibility of systemic treatment, enabling the eradication of both primary and metastatic tumors. However, several challenges need to be addressed to optimize immunotherapies targeting CK1. One key challenge is identifying reliable biomarkers that accurately predict CK1 expression or isoform-specific alterations in tumors. These biomarkers will aid in patient selection, treatment stratification, and monitoring of therapy response. Additionally, overcoming the immunosuppressive tumor microenvironment is crucial to achieving successful immunotherapy. Strategies to improve T cell infiltration, modulate immune checkpoints, or reprogram the tumor microenvironment to promote immune responses are actively being explored.
Additional research is needed to elucidate the specific functions of CK1 in immune cells and to understand its intricate involvement in the regulation of the immune response. This knowledge will guide the development of innovative immunotherapeutic approaches, such as CK1 inhibitors or CK1-specific antibodies, which can selectively modulate CK1 activity in immune cells.
6 Challenges and future perspectives
One major challenge is the isoform-specific targeting of CK1. As different CK1 isoforms can have distinct functions and contributions to tumorigenesis, it is crucial to develop strategies that selectively inhibit isoforms involved in relevant to specific types of tumors. This requires a deeper understanding of the isoform-specific roles of CK1 in different cancers and the development of selective inhibitors or modulators for each isoform. Another challenge lies in the development of effective delivery systems for CK1-targeted therapies. Efficient delivery to tumor cells while minimizing off-target effects is critical to therapeutic success. Further advancements in delivery technologies, such as the development of nanoparticle-based systems or targeted drug delivery approaches, are necessary to ensure the precise and effective delivery of CK1 inhibitors to tumor tissues.
Combination therapies involving CK1 inhibitors or immunotherapies with other treatment modalities can enhance therapeutic efficacy and overcome resistance mechanisms. Combining CK1-targeted therapies with conventional chemotherapy, radiotherapy, immune checkpoint inhibitors, or other targeted agents can synergistically inhibit multiple signaling pathways and improve anti-tumor immune responses. Rational design and optimization of combination regimens that rely on mechanistic insights and preclinical studies will be crucial to harnessing the full potential of CK1-targeted therapies in the clinic.
The identification of predictive biomarkers is essential for optimal patient selection and treatment monitoring in CK1-targeted therapies. Biomarkers can help stratify patients who are most likely to benefit from CK1 inhibitors or immunotherapies and provide information on response and treatment resistance. Potential biomarkers may include the determination of CK1 expression, isoform-specific signatures, or alterations in downstream signaling molecules. The development of robust and reliable biomarkers through comprehensive genomic profiling, proteomic analyses, or non-invasive imaging techniques will facilitate personalized treatment strategies and improve patient outcomes.
Looking ahead, the future perspectives of targeting CK1 for cancer therapy appear to be promising. Continued research efforts are required to deepen our understanding of the intricate mechanisms underlying CK1 dysregulation in cancer and its crosstalk with other signaling pathways. This will enable the identification of new therapeutic targets and the development of innovative strategies to overcome treatment resistance and improve patient outcomes.
7 Conclusions
While the pursuit of CK1 as a therapeutic target in cancer therapy holds great promise, it confronts noteworthy challenges. A pivotal obstacle lies in the imperative need for isoform-specific targeting of CK1. Given the divergent functionalities of distinct CK1 isoforms and their variable contributions to tumorigenesis, the development of strategies that can selectively inhibit isoforms relevant to specific tumor types becomes imperative. Moreover, the specter of undesired side effects, off-target repercussions, and the emergence of drug resistance looms as potential impediments in CK1-targeted therapy. In response to these challenges, RNAi-based and genome editing approaches emerge as valuable strategies. These methodologies have demonstrated their utility by enriching our comprehension of the pathological intricacies pervasive across various malignancies, and they have excelled in uncovering novel drug targets and elucidating the molecular underlying the adaptive responses following kinase inhibition. Although it is undeniably formidable, with continued research and development, the potential of CK1-targeted therapies can be harnessed to improve patient outcomes and contribute to the evolving landscape of cancer therapies.
Author contributions
LNH: conceptualization, investigation, and writing – original draft. S-JL: project administration, resources, supervision, and writing – review & editing. All authors contributed to the preparation of the article and have approved the submitted version.
Funding
This work was supported by a National Research Foundation (NRF) grant funded by the Korean government (MSIT) (No. NRF-2021R1A2C1005980). This study was also supported by Jeonbuk National University, Republic of Korea. Mr. Long Ngo Hoang was supported by the Brain Korea 21 program at the Department of Bioactive Material Sciences.
Conflict of interest
The authors declare that the research was conducted in the absence of any commercial or financial relationships that could be construed as a potential conflict of interest.
Publisher’s note
All claims expressed in this article are solely those of the authors and do not necessarily represent those of their affiliated organizations, or those of the publisher, the editors and the reviewers. Any product that may be evaluated in this article, or claim that may be made by its manufacturer, is not guaranteed or endorsed by the publisher.
References
1. Bar I, Merhi A, Larbanoix L, Constant M, Haussy S, Laurent S, et al. Silencing of casein kinase 1 delta reduces migration and metastasis of triple negative breast cancer cells. Oncotarget (2018) 9(56):30821–36. doi: 10.18632/oncotarget.25738
2. Siri M, Behrouj H, Dastghaib S, Zamani M, Likus W, Rezaie S, et al. Casein kinase-1-alpha inhibitor (D4476) sensitizes microsatellite instable colorectal cancer cells to 5-fluorouracil via authophagy flux inhibition. Arch Immunol Ther Exp (Warsz) (2021) 69(1):26. doi: 10.1007/s00005-021-00629-2
3. Dang F, Nie L, Zhou J, Shimizu K, Chu C, Wu Z, et al. Inhibition of ck1epsilon potentiates the therapeutic efficacy of cdk4/6 inhibitor in breast cancer. Nat Commun (2021) 12(1):5386. doi: 10.1038/s41467-021-25700-6
4. Lantermann AB, Chen D, McCutcheon K, Hoffman G, Frias E, Ruddy D, et al. Inhibition of casein kinase 1 alpha prevents acquired drug resistance to erlotinib in egfr-mutant non-small cell lung cancer. Cancer Res (2015) 75(22):4937–48. doi: 10.1158/0008-5472.CAN-15-1113
5. Shen C, Nayak A, Melendez RA, Wynn DT, Jackson J, Lee E, et al. Casein kinase 1alpha as a regulator of wnt-driven cancer. Int J Mol Sci (2020) 21(16):5940. doi: 10.3390/ijms21165940
6. Mazzoldi EL, Pasto A, Ceppelli E, Pilotto G, Barbieri V, Amadori A, et al. Casein kinase 1 delta regulates cell proliferation, response to chemotherapy and migration in human ovarian cancer cells. Front Oncol (2019) 9:1211(1211). doi: 10.3389/fonc.2019.01211
7. Schittek B, Sinnberg T. Biological functions of casein kinase 1 isoforms and putative roles in tumorigenesis. Mol Cancer (2014) 13(1):231. doi: 10.1186/1476-4598-13-231
8. Jiang J. Ck1 in developmental signaling: hedgehog and wnt. Curr Top Dev Biol (2017) 123:303–29. doi: 10.1016/bs.ctdb.2016.09.002
9. Nguyen Hoang AT, Hoe K-L, Lee S-J. Csnk1g2 differently sensitizes tamoxifen-induced decrease in pi3k/akt/mtor/S6k and erk signaling according to the estrogen receptor existence in breast cancer cells. PloS One (2021) 16(4):e0246264doi: 10.1371/journal.pone.0246264
10. Hoang LN, Lee SJ. Casein kinase 1 controls the shuttling of epidermal growth factor receptor and estrogen receptor in endometrial carcinoma induced by breast cancer hormonal therapy: relevance of gper1/src. Cell Signal (2023) 108:110733. doi: 10.1016/j.cellsig.2023.110733
11. Phadnis N, Cipak L, Polakova S, Hyppa RW, Cipakova I, Anrather D, et al. Casein kinase 1 and phosphorylation of cohesin subunit rec11 (Sa3) promote meiotic recombination through linear element formation. PloS Genet (2015) 11(5):e1005225. doi: 10.1371/journal.pgen.1005225
12. Nguyen Hoang AT, Lee H, Lee SJ. Casein kinase I inhibitor D4476 influences autophagy and apoptosis in chloroquine-induced adult retinal pigment epithelial-19 cells. Exp Eye Res (2022) 218:109004. doi: 10.1016/j.exer.2022.109004
13. Greer YE, Gao B, Yang Y, Nussenzweig A, Rubin JS. Lack of casein kinase 1 delta promotes genomic instability - the accumulation of DNA damage and down-regulation of checkpoint kinase 1. PloS One (2017) 12(1):e0170903. doi: 10.1371/journal.pone.0170903
14. Beale AD, Kruchek E, Kitcatt SJ, Henslee EA, Parry JSW, Braun G, et al. Casein kinase 1 underlies temperature compensation of circadian rhythms in human red blood cells. J Biol Rhythms (2019) 34(2):144–53. doi: 10.1177/0748730419836370
15. Brina D, Miluzio A, Ricciardi S, Biffo S. Eif6 anti-association activity is required for ribosome biogenesis, translational control and tumor progression. Bba-Gene Regul Mech (2015) 1849(7):830–5. doi: 10.1016/j.bbagrm.2014.09.010
16. Goto A, Sakai S, Mizuike A, Yamaji T, Hanada K. Compartmentalization of casein kinase 1 gamma csnk1g controls the intracellular trafficking of ceramide. iScience (2022) 25(7):104624. doi: 10.1016/j.isci.2022.104624
17. Fulford AD, Holder MV, Frith D, Snijders AP, Tapon N, Ribeiro PS. Casein kinase 1 family proteins promote slimb-dependent expanded degradation. Elife (2019) 8:e46592. doi: 10.7554/eLife.46592
18. Bartha A, Gyorffy B. Tnmplot.Com: A web tool for the comparison of gene expression in normal, tumor and metastatic tissues. Int J Mol Sci (2021) 22(5):2622. doi: 10.3390/ijms22052622
19. Liu J, Carvalho LP, Bhattacharya S, Carbone CJ, Kumar KG, Leu NA, et al. Mammalian casein kinase 1alpha and its leishmanial ortholog regulate stability of ifnar1 and type I interferon signaling. Mol Cell Biol (2009) 29(24):6401–12. doi: 10.1128/MCB.00478-09
20. Bozatzi P, Sapkota GP. The fam83 family of proteins: from pseudo-plds to anchors for ck1 isoforms. Biochem Soc Trans (2018) 46(3):761–71. doi: 10.1042/BST20160277
21. Bozatzi P, Dingwell KS, Wu KZ, Cooper F, Cummins TD, Hutchinson LD, et al. Paws1 controls wnt signalling through association with casein kinase 1alpha. EMBO Rep (2018) 19(4):e44807. doi: 10.15252/embr.201744807
22. Dermani FK, Samadi P, Rahmani G, Kohlan AK, Najafi R. Pd-1/pd-L1 immune checkpoint: potential target for cancer therapy. J Cell Physiol (2019) 234(2):1313–25. doi: 10.1002/jcp.27172
23. Sperling AS, Guerra VA, Kennedy JA, Yan Y, Hsu JI, Wang F, et al. Lenalidomide promotes the development of tp53-mutated therapy-related myeloid neoplasms. Blood (2022) 140(16):1753–63. doi: 10.1182/blood.2021014956
24. Chen L, Li C, Pan Y, Chen J. Regulation of P53-mdmx interaction by casein kinase 1 alpha. Mol Cell Biol (2005) 25(15):6509–20. doi: 10.1128/MCB.25.15.6509-6520.2005
25. Carreras Puigvert J, von Stechow L, Siddappa R, Pines A, Bahjat M, Haazen LC, et al. Systems biology approach identifies the kinase csnk1a1 as a regulator of the DNA damage response in embryonic stem cells. Sci Signal (2013) 6(259):ra5–ra. doi: 10.1126/scisignal.2003208
26. Rosenberg LH, Lafitte M, Quereda V, Grant W, Chen W, Bibian M, et al. Therapeutic targeting of casein kinase 1delta in breast cancer. Sci Transl Med (2015) 7(318):318ra202. doi: 10.1126/scitranslmed.aac8773
27. Denkert C, Liedtke C, Tutt A, von Minckwitz G. Molecular alterations in triple-negative breast cancer-the road to new treatment strategies. Lancet (2017) 389(10087):2430–42. doi: 10.1016/S0140-6736(16)32454-0
28. Richter J, Ullah K, Xu P, Alscher V, Blatz A, Peifer C, et al. Effects of altered expression and activity levels of ck1delta and varepsilon on tumor growth and survival of colorectal cancer patients. Int J Cancer (2015) 136(12):2799–810. doi: 10.1002/ijc.29346
29. Zhou Y, He C, Yan D, Liu F, Liu H, Chen J, et al. The kinase ck1varepsilon controls the antiviral immune response by phosphorylating the signaling adaptor traf3. Nat Immunol (2016) 17(4):397–405. doi: 10.1038/ni.3395
30. Rodriguez N, Yang J, Hasselblatt K, Liu S, Zhou Y, Rauh-Hain JA, et al. Casein kinase I epsilon interacts with mitochondrial proteins for the growth and survival of human ovarian cancer cells. EMBO Mol Med (2012) 4(9):952–63. doi: 10.1002/emmm.201101094
31. Shin S, Wolgamott L, Roux PP, Yoon SO. Casein kinase 1epsilon promotes cell proliferation by regulating mrna translation. Cancer Res (2014) 74(1):201–11. doi: 10.1158/0008-5472.CAN-13-1175
32. Lee SY, Kim H, Li CM, Kang J, Najafov A, Jung M, et al. Casein kinase-1gamma1 and 3 stimulate tumor necrosis factor-induced necroptosis through ripk3. Cell Death Dis (2019) 10(12):923. doi: 10.1038/s41419-019-2146-4
33. Li SS, Dong YH, Liu ZP. Recent advances in the development of casein kinase 1 inhibitors. Curr Med Chem (2021) 28(8):1585–604. doi: 10.2174/0929867327666200713185413
34. Knippschild U, Kruger M, Richter J, Xu P, Garcia-Reyes B, Peifer C, et al. The ck1 family: contribution to cellular stress response and its role in carcinogenesis. Front Oncol (2014) 4:96. doi: 10.3389/fonc.2014.00096
35. Rodrigues RP, da Silva CHTP. Discovery of potential neurodegenerative inhibitors in alzheimer’s disease by casein kinase 1 structure-based virtual screening. Med Chem Res (2017) 26(12):3274–85. doi: 10.1007/s00044-017-2020-9
36. Mashhoon N, DeMaggio AJ, Tereshko V, Bergmeier SC, Egli M, Hoekstra MF, et al. Crystal structure of a conformation-selective casein kinase-1 inhibitor. J Biol Chem (2000) 275(26):20052–60. doi: 10.1074/jbc.M001713200
37. Davids MS, Kim HT, Nicotra A, Savell A, Francoeur K, Hellman JM, et al. Umbralisib in combination with ibrutinib in patients with relapsed or refractory chronic lymphocytic leukaemia or mantle cell lymphoma: A multicentre phase 1-1b study. Lancet Haematol (2019) 6(1):e38–47. doi: 10.1016/S2352-3026(18)30196-0
38. Nastoupil LJ, Lunning MA, Vose JM, Schreeder MT, Siddiqi T, Flowers CR, et al. Tolerability and activity of ublituximab, umbralisib, and ibrutinib in patients with chronic lymphocytic leukaemia and non-hodgkin lymphoma: A phase 1 dose escalation and expansion trial. Lancet Haematol (2019) 6(2):e100–e9. doi: 10.1016/S2352-3026(18)30216-3
39. Janovska P, Verner J, Kohoutek J, Bryjova L, Gregorova M, Dzimkova M, et al. Casein kinase 1 is a therapeutic target in chronic lymphocytic leukemia. Blood (2018) 131(11):1206–18. doi: 10.1182/blood-2017-05-786947
40. Janovska P, Normant E, Miskin H, Bryja V. Targeting casein kinase 1 (Ck1) in hematological cancers. Int J Mol Sci (2020) 21(23):9026. doi: 10.3390/ijms21239026
41. Lee PY, Yeoh Y, Low TY. A recent update on small-molecule kinase inhibitors for targeted cancer therapy and their therapeutic insights from mass spectrometry-based proteomic analysis. FEBS J (2023) 290(11):2845–64. doi: 10.1111/febs.16442
42. Barouch-Bentov R, Sauer K. Mechanisms of drug resistance in kinases. Expert Opin Investig Drugs (2011) 20(2):153–208. doi: 10.1517/13543784.2011.546344
43. Gainor JF, Chabner BA. Ponatinib: accelerated disapproval. Oncologist (2015) 20(8):847–8. doi: 10.1634/theoncologist.2015-0253
44. O’Hare T, Shakespeare WC, Zhu X, Eide CA, Rivera VM, Wang F, et al. Ap24534, a pan-bcr-abl inhibitor for chronic myeloid leukemia, potently inhibits the T315i mutant and overcomes mutation-based resistance. Cancer Cell (2009) 16(5):401–12. doi: 10.1016/j.ccr.2009.09.028
45. Dazert E, Colombi M, Boldanova T, Moes S, Adametz D, Quagliata L, et al. Quantitative proteomics and phosphoproteomics on serial tumor biopsies from a sorafenib-treated hcc patient. Proc Natl Acad Sci USA (2016) 113(5):1381–6. doi: 10.1073/pnas.1523434113
46. Mansoori B, Sandoghchian Shotorbani S, Baradaran B. Rna interference and its role in cancer therapy. Adv Pharm Bull (2014) 4(4):313–21. doi: 10.5681/apb.2014.046
47. Tavares AJ, Poon W, Zhang YN, Dai Q, Besla R, Ding D, et al. Effect of removing kupffer cells on nanoparticle tumor delivery. Proc Natl Acad Sci USA (2017) 114(51):E10871–E80. doi: 10.1073/pnas.1713390114
48. Look T, Puca E, Buhler M, Kirschenbaum D, De Luca R, Stucchi R, et al. Targeted delivery of tumor necrosis factor in combination with ccnu induces a T cell-dependent regression of glioblastoma. Sci Transl Med (2023) 15(697):eadf2281. doi: 10.1126/scitranslmed.adf2281
49. Chen X, Mangala LS, Rodriguez-Aguayo C, Kong X, Lopez-Berestein G, Sood AK. Rna interference-based therapy and its delivery systems. Cancer Metastasis Rev (2018) 37(1):107–24. doi: 10.1007/s10555-017-9717-6
50. Zhao W, Siegel D, Biton A, Tonqueze OL, Zaitlen N, Ahituv N, et al. Crispr-cas9-mediated functional dissection of 3’-utrs. Nucleic Acids Res (2017) 45(18):10800–10. doi: 10.1093/nar/gkx675
51. Gersbach CA, Perez-Pinera P. Activating human genes with zinc finger proteins, transcription activator-like effectors and crispr/cas9 for gene therapy and regenerative medicine. Expert Opin Ther Targets (2014) 18(8):835–9. doi: 10.1517/14728222.2014.913572
52. Xu X, Hulshoff MS, Tan X, Zeisberg M, Zeisberg EM. Crispr/cas derivatives as novel gene modulating tools: possibilities and in vivo applications. Int J Mol Sci (2020) 21(9):3038. doi: 10.3390/ijms21093038
53. Liu Y, Han J, Chen Z, Wu H, Dong H, Nie G. Engineering cell signaling using tunable crispr-cpf1-based transcription factors. Nat Commun (2017) 8(1):2095. doi: 10.1038/s41467-017-02265-x
54. Suryawanshi A, Tadagavadi RK, Swafford D, Manicassamy S. Modulation of inflammatory responses by wnt/beta-catenin signaling in dendritic cells: A novel immunotherapy target for autoimmunity and cancer. Front Immunol (2016) 7:460. doi: 10.3389/fimmu.2016.00460
55. Kurihara T, Sakurai E, Toyomoto M, Kii I, Kawamoto D, Asada T, et al. Alleviation of behavioral hypersensitivity in mouse models of inflammatory pain with two structurally different casein kinase 1 (Ck1) inhibitors. Mol Pain (2014) 10:17. doi: 10.1186/1744-8069-10-17
56. Ma B, Hottiger MO. Crosstalk between wnt/B-catenin and nf-Kb signaling pathway during inflammation. Front Immunol (2016) 7:378. doi: 10.3389/fimmu.2016.00378
57. Vallée A, Lecarpentier Y. Crosstalk between peroxisome proliferator-activated receptor gamma and the canonical wnt/B-catenin pathway in chronic inflammation and oxidative stress during carcinogenesis. Front Immunol (2018) 9:745. doi: 10.3389/fimmu.2018.00745
58. Li X, Xiang Y, Li F, Yin C, Li B, Ke X. Wnt/beta-catenin signaling pathway regulating T cell-inflammation in the tumor microenvironment. Front Immunol (2019) 10:2293. doi: 10.3389/fimmu.2019.02293
Keywords: casein kinase 1, cancer therapy, clinical strategy, gene targeting, immunotherapy, RNA interference
Citation: Long NH and Lee S-J (2023) Targeting casein kinase 1 for cancer therapy: current strategies and future perspectives. Front. Oncol. 13:1244775. doi: 10.3389/fonc.2023.1244775
Received: 23 June 2023; Accepted: 07 September 2023;
Published: 08 November 2023.
Edited by:
Yun Dai, First Affiliated Hospital of Jilin University, ChinaReviewed by:
Todd Strochlic, College of Medicine, Drexel University, United StatesCopyright © 2023 Long and Lee. This is an open-access article distributed under the terms of the Creative Commons Attribution License (CC BY). The use, distribution or reproduction in other forums is permitted, provided the original author(s) and the copyright owner(s) are credited and that the original publication in this journal is cited, in accordance with accepted academic practice. No use, distribution or reproduction is permitted which does not comply with these terms.
*Correspondence: Sook-Jeong Lee, c2pAamJudS5hYy5rcg==