- 1Department of Oncology, National Health Commission (NHC) Key Laboratory of Nuclear Technology Medical Transformation (Mianyang Central Hospital), Mianyang Central Hospital, School of Medicine, University of Electronic Science and Technology, Mianyang, China
- 2Department of Oncology, Affiliated Hospital of North Sichuan Medical College, Nanchong, China
Ultra-high dose rate radiotherapy (FLASH-RT) is an external beam radiotherapy strategy that uses an extremely high dose rate (≥40 Gy/s). Compared with conventional dose rate radiotherapy (≤0.1 Gy/s), the main advantage of FLASH-RT is that it can reduce damage of organs at risk surrounding the cancer and retain the anti-tumor effect. An important feature of FLASH-RT is that an extremely high dose rate leads to an extremely short treatment time; therefore, in clinical applications, the steps of radiotherapy may need to be adjusted. In this review, we discuss the selection of indications, simulations, target delineation, selection of radiotherapy technologies, and treatment plan evaluation for FLASH-RT to provide a theoretical basis for future research.
1 Introduction
Cancer is one of the main causes of death, and there were an estimated 19.3 million new cases of cancer and almost 10.0 million deaths from cancer worldwide in 2020 (1). Radiotherapy is an important local treatment strategy for cancer that effectively controls tumor growth and prolongs the patient’s survival time (2, 3). Moreover, radiotherapy can alleviate pain (4), obstruction (5), and bleeding (6) caused by cancer, thereby improving the quality of life of patients with cancer. However, the curative effects of radiotherapy remain limited (7). Insufficient doses to the tumor area may be an important cause of tumor recurrence after radiotherapy (7). Radiotherapy can cause damage to the organs at risk (OAR) around the cancer (8), and the dose of external radical radiotherapy is often limited to 60–70 Gy to avoid unacceptable toxicity (9, 10).
Ultra-high dose rate radiotherapy (FLASH-RT) is an external beam radiotherapy strategy that uses an extremely high dose rate (≥40 Gy/s) (11). Compared with conventional dose rate radiotherapy (COVN-RT) (≤0.1 Gy/s), the main advantage of FLASH-RT is that it can reduce damage to OARs surround the cancer (11) and retain the anti-tumor effect (12); this phenomenon is called the FLASH effect, which suggests that FLASH-RT may widen the treatment window (13). At present, different particle types (photon, electron and proton) are used in FLASH-RT (14). Many current FLASH-RT studies use electron linear accelerators (15, 16); however, due to the low tissue penetration and limited field size of electron beams, they cannot be used for the treatment of deep tumors. As proton beam and photon beam offer the greater tissue penetration depth and, therefore, allow irradiation of deep-seated tumors, they are both considered as the most promising for clinical application (11, 14, 17). Furthermore, due to the presence of the Bragg peak, the proton beam may have a better dose distribution than photon beam, but the cost of a proton beam is more expensive (18). Oxygen depletion is one of the hypotheses for the mechanism of FLASH effect. As a single fraction of FLASH-RT may complete the irradiation in an extremely short time, the oxygen in the tissue is rapidly exhausted, and it is too fast to be supplemented by the circulating blood. This results in a relative hypoxia in the tissue compared with that following COVN-RT (irradiation completed in a few minutes), which may be one of the reasons why FLASH-RT can protect the normal tissue (19). However, the mechanism of the FLASH effect is unclear, and we discussed it in detail in our previous work (20).
Preclinical studies have demonstrated the protective advantages of FLASH-RT in normal tissues of the lungs (12), intestine (21), and brain (22). The first clinical study on FLASH-RT was reported in 2019 (23). In this study, a patient with skin T-cell lymphoma received FLASH-RT (electron, 166.7 Gy/s, a single total dose of 15 Gy); the tumor in the radiation area reached complete remission, and only grade 1 skin toxicity occurred (23). Recently, the results of the FAST-01 clinical study were published; ten patients with bone metastases in the extremities received a total dose of 8 Gy of FLASH-RT (51–61 Gy/s). The pain relief rate was 67% (complete relief rate, 50%), and no grade 3 treatment-related toxicity was observed (24). Owing to the safety and effectiveness of FLASH-RT for the treatment of bone metastasis in the FAST-01 study, the FAST-02 study began to recruit patients in 2022. However, in the FAST-01 study, only metastases in extremities were included, and a single rectangular field (from 7.5 cm × 7.5 cm up to 7.5 cm × 20 cm) was used to treat bone metastases. When the indications were expanded to body tumors with a more complex anatomical structure, it was difficult to meet the clinical requirements. In addition, due to the extremely short irradiation time and limitations on single total dose and dose rate of FLASH-RT, the traditional radiotherapy treatment process may not be applicable to FLASH-RT. Therefore, problems in the clinical implementation of FLASH-RT need to be further explored. In this review, we discuss the problems that need to be considered in the process of FLASH-RT clinical implementation, such as indication selection, stimulation, target delineation, selection of radiotherapy technology, and treatment plan evaluation, to provide a theoretical basis for future research. It should be noted that quality assurance is another important issue in clinical applications of FLASH-RT, which is not discussed in this review.
2 Indication
As reported in current preclinical studies, to trigger the FLASH effect, it may be necessary to simultaneously achieve both ultra-high dose rate (≥40 Gy/s) and large single dose per fraction (19, 25). An in vitro study showed that when a single dose reached 20 Gy, FLASH-RT reduced DNA damage in lung fibroblasts and increased the cell survival rate. When a single dose was <20 Gy, the protective effect of FLASH-RT disappeared (26). Although the single-dose threshold of FLASH-RT to achieve the FLASH effect may be different in different normal tissues (Table 1), we can speculate that in clinical applications, FLASH-RT may only be suitable for high-dose fractionated radiotherapy. Therefore, the experience gained from the current clinical use of stereotactic radiotherapy (SBRT) technology may provide useful experience for the clinical implementation of FLASH-RT, and tumors suitable for SBRT treatment, such as non-metastatic lung cancer (57), liver cancer (58), brain cancer (59), and oligometastases in the lung, liver, may be indications for FLASH-RT (60, 61). However, we need to consider the tumor location and treatment purpose during clinical experiments with FLASH-RT.
First, noncavitary organs may be more suitable for FLASH-RT than cavitary organs. This is because when high-dose fractionated radiotherapy is used for cavitary organ tumors, rapid shrinkage of the tumor may lead to organ perforation (e.g., esophageal perforation), infection, bleeding, or even death (62). The tumor size may also affect the implementation of FLASH-RT because if the tumor size is too large, OARs often have difficulty tolerating a single high-dose irradiation (63). However, further research is needed to determine the limitation of the tumor size on FLASH-RT.
Second, the purpose of treatment (palliative radiotherapy, radical radiotherapy, neoadjuvant radiotherapy, or adjuvant radiotherapy) is also an important factor in the selection of indications. The purpose of palliative radiotherapy is to relieve pain, bleeding, obstruction, and other symptoms at a low total dose, rather than to completely kill the tumor. Palliative radiotherapy is often used in the late stage of cancer, and its main goal is to improve the patient’s quality of life. A low total dose can maximize patient safety; therefore, in the early stage of the clinical implementation of FLASH-RT, palliative treatment should first be selected as an indication, as in the FAST-01 study (24). Follow-up clinical research is needed to explore the efficacy and safety of FLASH-RT in the treatment of pain, tumor hemorrhage, and tumor obstruction caused by tumor metastasis to the trunk. In 2022, FAST-02 was launched, the main inclusion criterion was patients with chest bone metastasis, which will provide important insights for the clinical application of FLASH-RT in palliative treatment. Radical radiotherapy refers to the use of a higher total dose as the main local treatment method to completely kill the tumor, and the main purpose of radical radiotherapy is to cure cancer and prolong the survival time. Because FLASH-RT may widen the treatment window of radiotherapy and a higher target dose could be delivered to the tumor, it is of great relevance to evaluate whether FLASH-RT can improve the anti-tumor effect; therefore, it is of great importance to evaluate the efficacy and safety of FLASH-RT in radical radiotherapy. Neoadjuvant and adjuvant radiotherapies are often used as auxiliary means of surgical treatment before (neoadjuvant radiotherapy) or after (adjuvant radiotherapy) surgery. The main purpose of neoadjuvant or adjuvant radiotherapy is to improve the antitumor effect of surgery and reduce the risk of recurrence. However, in neoadjuvant or adjuvant radiotherapy, irradiation of the lymph node drainage area is typically considered when dealing with regional microscopic tumor spread or incomplete resection (64, 65), which means that the treatment target area is too large to use single high-dose irradiation. However, there were no reports of using FLASH-RT for treatment of lymphatic node drainage areas. In order to determine whether the lymph node drainage area is suitable for FLASH-RT, future research needs to clarify two points: 1) the maximum tolerable dose of OARs around the lymph node drainage area under ultra-high dose rate irradiation condition; 2) the maximum tolerable dose that can be tolerated by OARs large enough to trigger the FLASH effect.
3 Radiotherapy simulation
Radiotherapy simulation is an important preparation step before radiotherapy. Its main purpose is to obtain a repeatable matching three-dimensional computed tomography (CT) image of the patient to meet the target area delineation and implement accurate radiotherapy (66). Bourhis et al. reported the first human FLASH-RT study in 2019. In this study, ultra-high dose rate electron was used treat a patient with cutaneous T-cell lymphoma, and the treatment area is limited by the collimator; therefore, no simulation was conducted (23). FAST-01 study was the first study that reported the experience of FLASH-RT simulation (24). After fitting with an immobilization device, each participant accepted CT simulation imaging for the area(s) encompassing the treatment targets (24). However, FAST-01 study only included patients with bone metastases in the extremities, the target area was fixed, and this experience of FLASH-RT simulation cannot be applied to situations where the target area is movable. Similar to SBRT, the radiotherapy simulation of FLASH-RT requires postural fixation. Thermoplastics is a mature tool that ensures the relative consistency of a patient’s position during positioning and treatment (67). However, movement of internal organs during treatment, such as pharyngeal swallowing activity, respiratory movement, gastrointestinal motility and heartbeat, is an important factor affecting the accuracy of radiotherapy (68–70). Because of the long implementation time of SBRT (minutes to tens of minutes) (71, 72), it is unlikely the movement of organs in the body near the target area can be avoided. Consequently, it is often necessary to expand the volume of the target area to cover the movement track of the tumor target area and avoid the tumor omission (73). CyberKnife is a special treatment platform for SBRT, and its organ tracking function is an important advantage (74). Organ movement can be simulated to the maximum extent using reference marks implanted on the body surface or in vivo. Subsequently, the position is matched using a 6D treatment couch to reduce the volume expansion of the target area caused by organ movement (74). However, the treatment time of FLASH-RT is extremely short (within milliseconds) (11). The organ position may remain unchanged during FLASH-RT irradiation, so FLASH-RT may not need to simulate the dynamic process of organ movement. The problem that needs to be addressed is whether the tumor location is consistent with the initial CT location. Therefore, it may be necessary to implant a target reference object (e.g., a metal marker) into the tumor before simulation. Moreover, an auxiliary device similar to an “adaptive switch” is needed to trigger FLASH-RT immediately when the tumor moves to the target position. By ignoring the effects of organ movement on the target location, FLASH-RT may further narrow the target area to improve normal tissue sparing. The feasibility of implanting markers into tumors and using position matching to trigger FLASH-RT should be verified in subsequent studies. However, marks implantation is an invasive procedure, and although the accuracy of treatment is guaranteed, it may bring risks of pain, bleeding, infection, and tumor metastasis. Therefore, a safer radiotherapy simulation mode may need further exploration.
4 Target delineation
Target delineation is a step for clinicians to determine the scope of the tumor and to delineate the OARs around the target area through clinical physical examination, imaging data, endoscopic data, and tumor biological behavior. This process determines the radiotherapy treatment area (tumor) and protection area (OARs) (75). In traditional radiotherapy, the target areas to be delineated include the gross tumor volume, clinical target volume (CTV), internal target volume (ITV), and planning target volume (76). Considering the extremely short delivery time and dose-rate threshold of FLASH-RT, we propose the following target delineation approaches.
4.1 ITV delineation may not be necessary
The ITV refers to the expanded margin when the position of the CTV is uncertain (mainly due to organ movement, filling, and deformation) (76). Due to the FLASH-RT treatment time being extremely short and internal organ movement in the treatment process having little impact on the target location, once the tumor location is consistent with the initial CT location, FLASH-RT will be triggered. As a result, the ITV may not need to be delineated. Deletion of the ITV may be particularly suitable for FLASH-RT in liver and lung tumors affected by respiratory movement. In future research, more evidence and clinical data are needed to support the feasibility of omitting the ITV. However, when the target area is close to the heart or large blood vessels, and tachycardia is existing simultaneously, the ITV may not be omitted. For example, when using a total dose of 20 Gy and a dose rate of 40 Gy/s to treat a patient with a heart rate of 120 beats/min, the time for a single irradiation is half a second, and the patient’s heart can beat once in half a second, indicating that the heartbeat still affects the position of the tumor target area. A 4D cardiac dual-source CT may be a useful tool to obtain the moving boundaries of the heart and guide the delineation of ITV in this situation (77).
4.2 Addition of the dose rate organs at risk may be needed
As the protective effect of FLASH-RT requires the dose rate of radiotherapy to reach 40 Gy/s or higher, OARs must meet this dose-rate threshold. However, tumors must be killed rather than protected, and the dose rate of the tumor areas does not have to reach the threshold to trigger the FLASH effect. To evaluate the scope of the area to be protected by FLASH-RT more accurately, the dose rate organs at risk (DOARs) may need to be defined to facilitate treatment planning. Considering that a threshold dose is needed to trigger the FLASH effect and that the lowest single dose to trigger the FLASH effect in different OARs may be inconsistent (Table 1), areas in OARs that absorbed doses lower than the threshold dose to trigger the FLASH effect may not be required to reach 40 Gy/s or above. Therefore, it may not be possible to define OARs directly as the DOARs. The DOARs is defined as the area in the OARs that exceeds the minimum dose threshold (i.e., the minimum dose that triggers the FLASH effect) (Figure 1A).
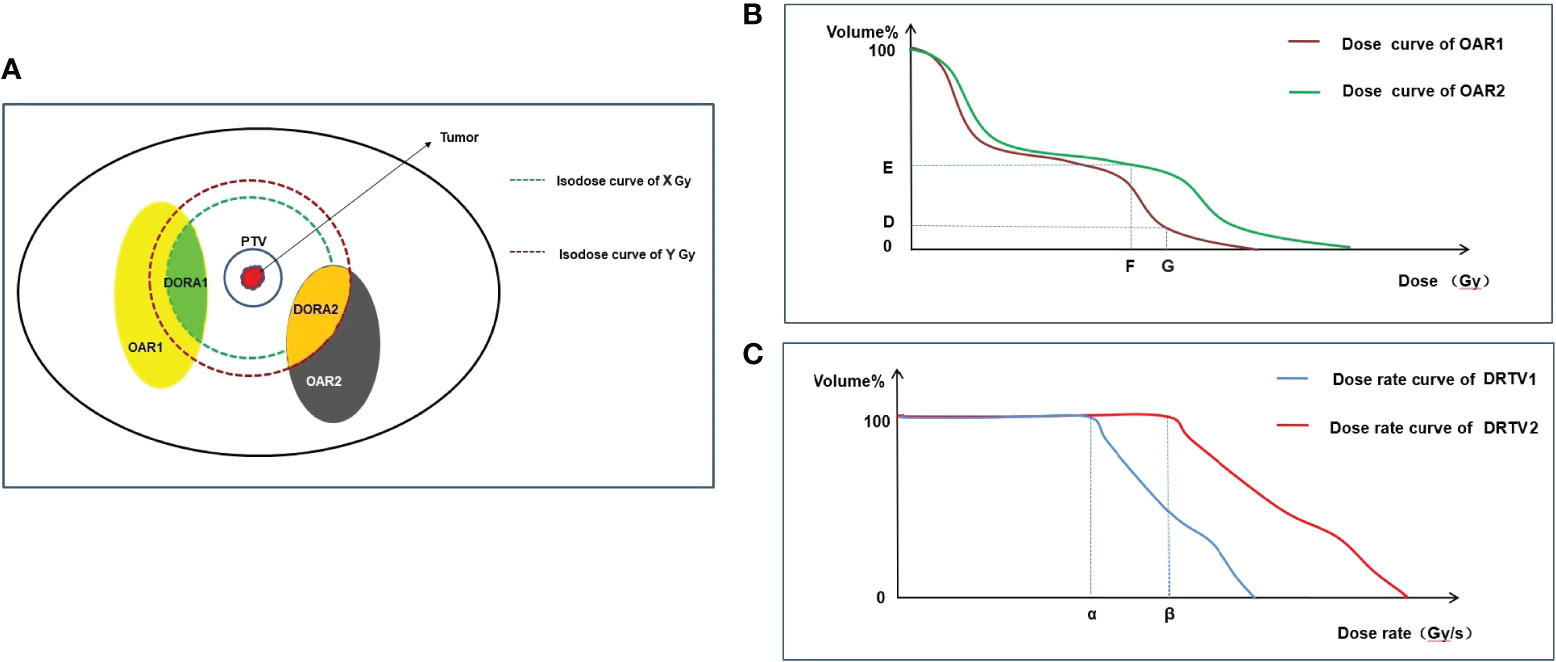
Figure 1 Graphical representation of DOARs delineation and TPE. OAR1 and OAR2 represent two normal organs around tumor (A), respectively. Firstly, dose optimization is performed and evaluated using DVH to meet the following requirements: in OAR1, VG<D%, and in OAR2, VF<E% (B). Secondly, after dose optimization is completed, DOARs are delineated (A). X Gy and Y Gy are the threshold doses that trigger the FLASH effect of OAR1 and OAR2, respectively. In OAR1, the area (green) with dose ≥A Gy may produce the FLASH effect, and the dose rate assessment is required, which is defined as DOAR1. In OAR2, the area (orange) with dose ≥B Gy may produce the FLASH effect, and the dose rate assessment is required, which is defined as DOAR2. Dose rate is evaluated by DRVH (C). α and β are the threshold dose rates that trigger the FLASH effect of OAR1 and OAR2, respectively. To trigger the FLASH effect, the dose rate of αshould cover 100% volume of DOAR1 and the dose rate of β should cover 100% volume of DOAR2. DOARs, dose rate organs at risk; OARs, organs at risk; DVH, dose–volume histogram; DRVH, dose rate–volume histogram; TPE, treatment plan evaluation.
The dose distribution of OARs can only be obtained after the completion of radiotherapy dose optimization. Therefore, the delineation of the DOARs should be sketched after completion of the first treatment plan (dose optimization), and the second step of the treatment plan (dose rate optimization) should be subsequently conducted. However, reaching the dose-rate threshold of FLASH-RT requires extremely high-technology radiotherapy equipment, which results in high costs (78). Adding the DOARs to OARs in the treatment field and selectively meeting the dose-rate threshold of FLASH-RT may further reduce the technical difficulty of the clinical implementation of FLASH-RT. Moreover, the DOARs will facilitate clinicians in evaluating treatment plans in more dimensions; this is discussed in detail in the plan evaluation section.
5 Radiotherapy technology
In traditional radiotherapy, radiotherapy technologies include two-dimensional radiotherapy (2DRT), three-dimensional conformal radiotherapy (3DCRT), intensity-modulated radiotherapy (IMRT), and image-guided radiotherapy (IGRT) (79). In the case of a simple target structure and a few surrounding OARs (e.g., limb bone metastasis), 2DRT or 3DCRT can meet the treatment requirements. However, when the shape of the target area is complex, particularly with a groove structure, 2DRT or 3DCRT often cannot produce a highly conformal dose distribution area (76, 80). Therefore, IMRT is currently the most widely used radiotherapy technique in clinical practice (81). IMRT can form multiple subfields in any direction by moving a multileaf collimator (MLC) to optimize the dose distribution in the target area (82). However, in the process of producing a daughter field, the MLC movement requires sufficient time (83), which prolongs the treatment time and reduces the average dose rate of radiotherapy, resulting in failure to meet the dose-rate threshold of FLASH-RT. Therefore, IMRT is not applicable to FLASH-RT, but 3D-CRT may be a radiotherapy technology suitable for FLASH-RT. At the same time, in the process of FLASH-RT, it is necessary to accurately locate the tumor before treatment; hence, IGRT is necessary, but it is impossible to use IGRT for real-time position adjustment in the treatment because the FLASH-RT treatment time is very short and the time required for image matching and treatment bed movement far exceeds the time of FLASH-RT.
6 Treatment plan evaluation
Treatment plan evaluation (TPE) is an important step before the implementation of radiotherapy. The main purpose of TPE is to evaluate whether the target areas in the radiotherapy plan reach the prescribed dose, the uniformity and conformability of the target area, the dose hot and cold spots in the target area, and whether the dose of OARs exceeds the limit value (76). In traditional radiotherapy, the isodose curve and dose–volume histogram (DVH) are important evaluation tools (84, 85). However, in FLASH-RT, the dose rate is an important physical parameter because a normal tissue protection effect can be achieved only when the dose rate exceeds a threshold. Therefore, we propose that additional tools should be provided when evaluating normal tissues, including the isodose rate curve and dose rate–volume histogram (DRVH).
6.1 Isodose rate curve
The isodose rate curve refers to the curve connected by the voxels that receive the same dose rate in a three-dimensional human-simulated CT image. In traditional radiotherapy, the isodose curve can assist clinicians in evaluating the coverage of OARs at a certain dose in the axial, sagittal, and coronal positions. Take the spinal cord as an example, in order to meet the limit that the maximum dose cannot exceed 50 Gy (conventional fraction) (86), an isodose rate curve of 50 Gy should not include the spinal cord. Similarly, when evaluating the DOARs, the lowest dose rate isodose rate curve that triggers the FLASH effect must include all the DOARs. When evaluating OARs, the dose-rate curve is an important supplement to the dose curve. The dose curve can evaluate the radiation dose of normal tissues to meet the dose limit of traditional radiotherapy on normal tissues; the dose-rate curve can give full play to the technical advantages of FLASH-RT and make use of the protective effect of the FLASH dose rate on normal tissues to protect normal tissues more effectively and reduce the incidence of toxicity. However, because the minimum dose-rate threshold for triggering the FLASH effect in different tissues may be inconsistent (Table 1), the isodose rate curves of interest may also be inconsistent when evaluating different OARs. In future studies, a large number of preclinical and clinical trials are required to determine the minimum dose rate for different OARs.
6.2 DRVH
DRVH is a statistical chart that evaluates the proportion of the fixed dose rate volume in the overall volume in DRVH with a dose rate as the horizontal axis and volume percentage as the vertical axis (Figures 1B, C). In traditional radiotherapy, the isodose curve is often only able to determine whether the region of interest is covered by a certain dose curve, but the extent of coverage cannot be provided by the isodose curve; therefore, the DVH tool is needed for further evaluation. DVH is an important evaluation tool for parallel organs (e.g., the lungs and kidneys) (86). For example, V20 (the proportion of the lung volume covered by a dose of >20 Gy to the total lung volume) is significantly associated with the incidence of radiation pneumonia. In TPE of the lung, V20 <30% is used in clinical practice to limit the incidence of radiation-induced lung injury (87). Similarly, the isodose rate curve alone cannot indicate the extent to which the region of interest is covered by the target dose rate curve. Therefore, it may be necessary to introduce DRVH to obtain additional information. More importantly, the dose-rate curve histogram can quantify the coverage of the dose-rate curve of OARs, which is helpful in analyzing the relationship between the dose-rate volume and the incidence of adverse reactions and can guide follow-up clinical practice. Figure 1 is used to illustrate the practical use of DVH and DRVH in TPE. It should be noted that we have only speculated theoretically about the TPE tools of FLASH-RT. The prerequisite for the application of these tools is to clarify the physical conditions that trigger the FLASH effect (dose rate, total single dose), as well as the maximum tolerable dose of OARs under ultra-high dose rate conditions. However, these physical parameters are currently unclear and need to be clarified in future biological experiments.
7 Implement of FLASH-RT
Currently, only two clinical studies have reported the implementation of FLASH-RT (23, 24), however, both of these clinical studies used surface markers for setup and treatment. For surface or limb lesions with fixed tumor locations, this method is acceptable, but for deep tumors, especially those with organ movements, it is necessary to explore a real-time imaging method to ensure that dose is accurately transmitted to the tumor area. Currently, the mature technology used in the implementation of COVN-RT is CBCT image-guided radiotherapy (88). Patients usually need to undergo CBCT scanning after fixation, and the obtained images are matched with CT-simulated images for position registration. An error range of less than 3 mm is often considered an acceptable range (89). However, the slow acquisition time of CBCT images would limit its utility for real-time imaging. Recently, El Naqa et al. proposed that MRI or ultrasound images may be suitable for real-time image guidance of FLASH-RT (90). MRI-guided radiotherapy has become more popular in recent years as it has superior soft tissue contrast and no moving parts for 3D imaging, allowing for real-time motion monitoring (91). Ultrasound provides a more economical, non-invasive, real-time, and radiation free imaging method that can provide (even time-resolved) 3D-US anatomical visualization for selected anatomical positions. Meanwhile, ultrasound has been used in the past for image-guided radiotherapy of prostate cancer and gynecological cancer (92). In future research, it is necessary to further verify the feasibility of applying these two image-guided technologies to FLASH-RT.
8 Conclusions
This review discusses the changes in the future clinical application of FLASH-RT and provides new research directions for the clinical transformation of FLASH-RT. First, we discussed the indications for FLASH-RT and suggested that tumors suitable for SBRT may be suitable for FLASH-RT. Simultaneously, the radiotherapy process may need to be modified (Figure 2). The ITV may not be required for target area delineation, and for normal tissues, DOARs may need to be added. Because of the long modulation time of IMRT, 3D-CRT may be a suitable radiotherapy technology for FLASH-RT. When conducting TPE, it may be necessary to introduce a dose-rate curve and DRVH to fully evaluate whether OARs have received sufficient dose-rate radiation to achieve a better protective effect. However, the clinical application of FLASH-RT requires further clinical trials to ensure better validation and improvement, especially in the treatment of irregularly shaped tumors in the body. It may be challenging to ensure that the radiation dose is accurately transmitted to the tumor target area and achieve sufficient dose rate and total single dose to trigger the FLASH effect.
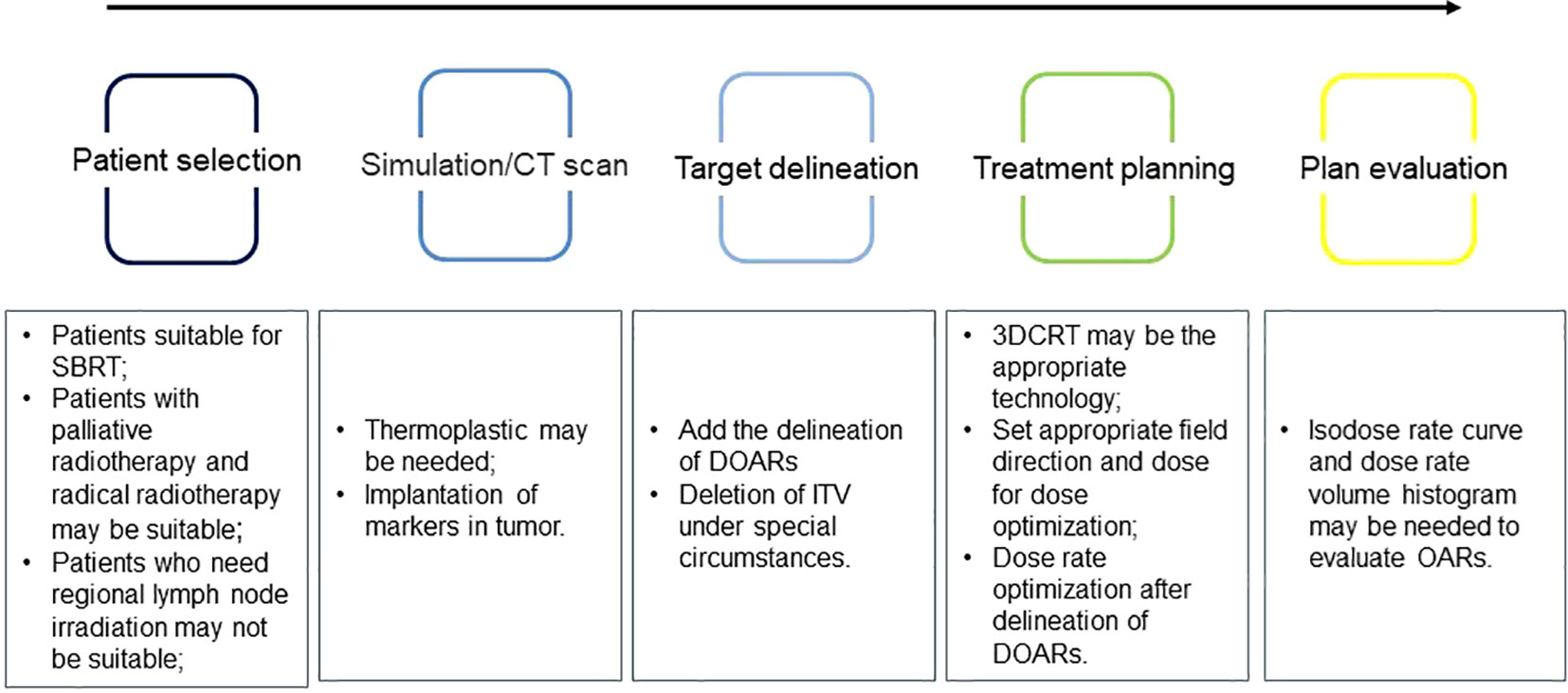
Figure 2 Problems that may need attention during the implementation of ultra-high dose rate radiotherapy.
Data availability statement
The original contributions presented in the study are included in the article/supplementary material. Further inquiries can be directed to the corresponding author.
Author contributions
BL and MF draft the manuscript, TN, YL, HX and WT participated in the data review and collection for the study. XD conceived of the study, revised and prepared the manuscript. All authors contributed to the article and approved the submitted version.
Funding
This work was financially supported by the National Natural Science Foundation of China Academy of Engineering Physics and jointly set up "NSAF" joint fund (grant no. U2330122) and Natural Science Foundation of Sichuan Province (grant no. 2022NSFSC0849).
Conflict of interest
The authors declare that the research was conducted in the absence of any commercial or financial relationships that could be construed as a potential conflict of interest.
Publisher’s note
All claims expressed in this article are solely those of the authors and do not necessarily represent those of their affiliated organizations, or those of the publisher, the editors and the reviewers. Any product that may be evaluated in this article, or claim that may be made by its manufacturer, is not guaranteed or endorsed by the publisher.
References
1. Ferlay J, Colombet M, Soerjomataram I, Parkin DM, Pineros M, Znaor A, et al. Cancer statistics for the year 2020: An overview. Int J Cancer (2021) 149:778–89. doi: 10.1002/ijc.33588
2. Chen Z, Dominello MM, Joiner MC, Burmeister JW. Proton versus photon radiation therapy: A clinical review. Front Oncol (2023) 13:1133909. doi: 10.3389/fonc.2023.1133909
3. Bhide SA, Nutting CM. Advances in radiotherapy for head and neck cancer. Oral Oncol (2010) 46(6):439–41. doi: 10.1016/j.oraloncology.2010.03.005
4. Ito K, Saito T, Nakamura N, Imano N, Hoskin P. Stereotactic body radiotherapy versus conventional radiotherapy for painful bone metastases: a systematic review and meta-analysis of randomized controlled trials. Radiat Oncol (2022) 17(1):156. doi: 10.1186/s13014-022-02128-w
5. Murakami N, Nakagawa K, Yamashita H, Nagawa H. Palliative radiation therapy for advanced gastrointestinal cancer. Digestion (2008) 77 Suppl 1:29–35. doi: 10.1159/000111485
6. Viani GA, Arruda CV, Hamamura AC, Faustino AC, Danelichen AFB, Matsuura FK, et al. Palliative radiotherapy for gastric cancer: Is there a dose relationship between bleeding response and radiotherapy? Clinics (Sao Paulo) (2020) 75:e1644. doi: 10.6061/clinics/2020/e1644
7. Olivares-Urbano MA, Griñán-Lisón C, Marchal JA, Núñez MI. CSC radioresistance: A therapeutic challenge in improving the effectiveness of radiotherapy in cancer. Cells (2020) 9(7):1651. doi: 10.3390/cells9071651
8. Montay-Gruel P, Meziani L, Yakkala C, Vozenin MC. Expanding the therapeutic index of radiation therapy with normal tissue protection. Br J Radiol (2019) 92(1093):20180008. doi: 10.1259/bjr.20180008
9. Perez CA, Pajak TF, Rubin P, Simpson JR, Mohiuddin M, Brady LW, et al. Long-term observations of failure patterns in patients with unresectable non-oat cell carcinoma of the lungs treated with definitive radiotherapy. Rep by Radiat Ther Oncol Group Cancer (1987) 59(11):1874–81. doi: 10.1002/1097-0142(19870601)59:11<1874::aid-cncr2820591106>3.0.co;2-z
10. Eisbruch A, Harris J, Garden AS, Chao CK, Straube W, Harari PM, et al. A multi-institutional trial of accelerated hypofractionated intensity-modulated radiation therapy for early stage oropharyngeal cancer (RTOG 00-22). Int J Radiat Oncol Biol Phys (2010) 76(5):1333–8. doi: 10.1016/j.ijrobp.2009.04.011
11. Lin B, Gao F, Yang Y, Wu D, Zhang Y, Feng G. et al. FLASH Radiother: History Future Front Oncol (2021) 11:644400. doi: 10.3389/fonc.2021.644400
12. Favaudon V, Caplier L, Monceau V, Pouzoulet F, Sayarath M, Fouillade C, et al. Ultrahigh-doserate FLASH irradiation increases the differential response between normal and tumor tissues in mice [published correction appears in Sci Transl Med. 2019 Dec 18;11(523)]. Sci Transl Med (2014) 6(245):245ra93. doi: 10.1126/scitranslmed.3008973
13. Vozenin MC, Bourhis J, Durante M. Towards the clinical translation of FLASH radiotherapy. Nat Rev Clin Oncol (2022) 19(12):791–803. doi: 10.1038/s41571-022-00697-z
14. Hageman E, Che PP, Dahele M, Slotman BJ, Sminia P. Radiobiological aspects of FLASH radiotherapy. Biomolecules (2022) 12(10):1376. doi: 10.3390/biom12101376
15. Lempart M, Blad B, Adrian G, Bäc S, Knöös T, Ceberg C, et al. Modifying a clinical linear accelerator for delivery of ultra-high dose rate irradiation. Radiother Oncol (2019) 139:40–5. doi: 10.1016/j.radonc.2019.01.031
16. de Kruijff RM. FLASH radiotherapy: ultra-high dose rates to spare healthy tissue. Int J Radiat Biol (2020) 96(4):419–23. doi: 10.1080/09553002.2020.1704912
17. Montay-Gruel P, Corde S, Laissue JA, Bazalova-Carter M. FLASH radiotherapy with photon beams. Med Phys (2022) 49(3):2055–67. doi: 10.1002/mp.15222
18. Yuan TZ, Zhan ZJ, Qian CN. New frontiers in proton therapy: applications in cancers. Cancer Commun (Lond) (2019) 39(1):61. doi: 10.1186/s40880-019-0407-3
19. Weiss H, Epp ER, Heslin JM, Ling CC, Santomasso A. Oxygen depletion in cells irradiated at ultra-high dose-rates and at conventional dose-rates. Int J Radiat Biol Relat Stud Phys Chem Med (1974) 26(1):17–29. doi: 10.1080/09553007414550901
20. Lin B, Huang D, Gao F, Yang Y, Wu D, Zhang Y, et al. Mechanisms of FLASH effect. Front Oncol (2022) 12:995612. doi: 10.3389/fonc.2022.995612
21. Ruan JL, Lee C, Wouters S, Tullis IDC, Verslegers M, Mysara M, et al. Irradiation at ultrahigh (FLASH) Dose Rates Reduces Acute Normal Tissue Toxicity in the Mouse Gastrointestinal System. Int J Radiat Oncol Biol Phys (2021) 111(5):1250–61. doi: 10.1016/j.ijrobp.2021.08.004
22. Montay-Gruel P, Markarian M, Allen BD, Baddour JD, Giedzinski E, Jorge PG, et al. Ultrahigh dose- rate FLASH Irradiation Limits Reactive Gliosis in the Brain. Radiat Res (2020) 194(6):636–45. doi: 10.1667/RADE-20-00067.1
23. Bourhis J, Sozzi WJ, Jorge PG, Gaide O, Bailat C, Duclos F, et al. Treatment of the first patient with FLASH-radiotherapy radiotherapy. Radiother Oncol (2019) 139:18–22. doi: 10.1016/j.radonc.2019.06.019
24. Mascia AE, Daugherty EC, Zhang Y, Lee E, Xiao Z, Sertorio M, et al. Proton FLASH radiotherapy for the treatment of symptomatic bone metastases: the FAST-01 nonrandomized trial. JAMA Oncol (2023) 9(1):62–9. doi: 10.1001/jamaoncol.2022.5843
25. Rothwell BC, Kirkby NF, Merchant MJ, Chadwick AL, Lowe M, Mackay RI, et al. Determination of the parameter space for effective oxygen depletion in FLASH radiation therapy. Phys Med Biol (2021) 66(5):055020. doi: 10.1088/1361-6560/abe2ea
26. Buonanno M, Grilj V, Brenner DJ. Biological effects of FLASH dose-dependent protons on normal cells. Radiother Oncol (2019) 139:51–5. doi: 10.1016/j.radonc.2019.02.009
27. Fouillade C, Curras-Alonso S, Giuranno L, Quelennec E, Heinrich S, Bonnet-Boissinot S, et al. FLASH irradiation spares lung progenitor cells and limits the incidence of radio-induced senescence. Clin Cancer Res (2020) 26(6):1497–506. doi: 10.1158/1078-0432.CCR-19-1440
28. Gao F, Yang Y, Zhu H, Wang J, Xiao D, Zhou Z, et al. First demonstration of the FLASH effect with ultrahigh dose rate high-energy X-rays. Radiother Oncol (2022) 166:44–50. doi: 10.1016/j.radonc.2021.11.004
29. Adrian G, Konradsson E, Beyer S, Wittrup A, Butterworth KT, McMahon SJ, et al. Cancer cells can exhibit a sparing FLASH effect at low doses under normoxic in vitro-conditions. Front Oncol (2021) 11:686142. doi: 10.3389/fonc.2021.686142
30. Guo Z, Buonanno M, Harken A, Zhou G, Hei TK. Mitochondrial damage response and fate of normal cells exposed to FLASH irradiation with protons. Radiat Res (2022) 197(6):569–82. doi: 10.1667/RADE-21-00181.1
31. Montay-Gruel P, Petersson K, Jaccard M, Boivin G, Germond JF, Petit B, et al. Irradiation in a flash: Unique sparing of memory in mice after whole brain irradiation with dose rates above 100Gy/s. Radiother Oncol (2017) 124(3):365–9. doi: 10.1016/j.radonc.2017.05.003
32. Montay-Gruel P, Bouchet A, Jaccard M, Patin D, Serduc R, Aim W, et al. X-rays can trigger the FLASH effect: Ultra-high dose-rate synchrotron light source prevents normal brain injury after whole brain irradiation in mice. Radiother Oncol (2018) 129(3):582–8. doi: 10.1016/j.radonc.2018.08.016
33. Simmons DA, Lartey FM, Schüler E, Rafat M, King G, Kim A, et al. Reduced cognitive deficits after FLASH irradiation of whole mouse brain are associated with less hippocampal dendritic spine loss and neuroinflammation. Radiother Oncol (2019) 139:4–10. doi: 10.1016/j.radonc.2019.06.006
34. Montay-Gruel P, Acharya MM, Petersson K, Alikhani L, Yakkala C, Allen BD, et al. Long-term neurocognitive benefits of FLASH radiotherapy driven by reduced reactive oxygen species [published correction appears in Proc Natl Acad Sci U S A. 2020 Oct 13;117(41):25946-25947]. Proc Natl Acad Sci U S A (2019) 116(22):10943–51. doi: 10.1073/pnas.1901777116
35. Alaghband Y, Cheeks SN, Allen BD, Montay-Gruel P, Doan NL, Petit B, et al. Neuroprotection of radiosensitive juvenile mice by ultra-high dose rate FLASH irradiation. Cancers (Basel) (2020) 12(6):1671. doi: 10.3390/cancers12061671
36. Allen BD, Acharya MM, Montay-Gruel P, Jorge PG, Bailat C, Petit B, et al. Maintenance of tight junction integrity in the absence of vascular dilation in the brain of mice exposed to ultra-high-dose-rate FLASH irradiation. Radiat Res (2020) 194(6):625–35. doi: 10.1667/RADE-20-00060.1
37. Montay-Gruel P, Acharya MM, Gonçalves Jorge P, Petit B, Petridis IG, Fuchs P, et al. Hypofractionated FLASH-RT as an effective treatment against glioblastoma that reduces neurocognitive side effects in mice. Clin Cancer Res (2021) 27(3):775–84. doi: 10.1158/1078-0432.CCR-20-0894
38. Dokic I, Meister S, Bojcevski J, Tessonnier T, Walsh D, Knoll M, et al. Neuroprotective Effects of Ultra-High Dose Rate FLASH Bragg Peak Proton Irradiation [published online ahead of print, 2022 Feb 20]. Int J Radiat Oncol Biol Phys (2022) 113(3):614–623. doi: 10.1016/j.ijrobp.2022.02.020
39. Levy K, Natarajan S, Wang J, Chow S, Eggold JT, Loo PE, et al. Abdominal FLASH irradiation reduces radiation-induced gastrointestinal toxicity for the treatment of ovarian cancer in mice. Sci Rep (2020) 10(1):21600. doi: 10.1038/s41598-020-78017-7
40. Kim MM, Verginadis II, Goia D, Haertter A, Shoniyozov K, Zou W, et al. Comparison of FLASH proton entrance and the spread-out bragg peak dose regions in the sparing of mouse intestinal crypts and in a pancreatic tumor model. Cancers (Basel) (2021) 13(16):4244. doi: 10.3390/cancers13164244
41. Shi X, Yang Y, Zhang W, Wang J, Xiao D, Ren H, et al. FLASH X-ray spares intestinal crypts from pyroptosis initiated by cGAS-STING activation upon radioimmunotherapy. Proc Natl Acad Sci U S A (2022) 119(43):e2208506119. doi: 10.1073/pnas.2208506119
42. Zhu H, Xie D, Yang Y, Huang S, Gao X, Peng Y, et al. Radioprotective effect of X-ray abdominal FLASH irradiation: Adaptation to oxidative damage and inflammatory response may be benefiting factors. Med Phys (2022) 49(7):4812–22. doi: 10.1002/mp.15680
43. Zhang Q, Gerweck LE, Cascio E, Gu L, Yang Q, Dong X, et al. Absence of tissue-sparing effects in partial proton FLASH irradiation in murine intestine. Cancers (Basel) (2023) 15(8):2269. doi: 10.3390/cancers15082269
44. Vozenin MC, De Fornel P, Petersson K, Favaudon V, Jaccard M, Germond JF, et al. The advantage of FLASH radiotherapy confirmed in mini-pig and cat-cancer patients. Clin Cancer Res (2019) 25(1):35–42. doi: 10.1158/1078-0432.CCR-17-3375
45. Soto LA, Casey KM, Wang J, Blaney A, Manjappa R, Breitkreutz D, et al. FLASH Irradiation Results in Reduced Severe Skin Toxicity Compared to Conventional-Dose-Rate Irradiation [published correction appears in Radiat Res. 2022 Feb 1;197(2):207]. Radiat Res (2020) 194(6):618–24. doi: 10.1667/RADE-20-00090
46. Singers Sørensen B, Krzysztof Sitarz M, Ankjærgaard C, Johansen J, Andersen CE, Kanouta E, et al. In vivo validation and tissue sparing factor for acute damage of pencil beam scanning proton FLASH. Radiother Oncol (2022) 167:109–15. doi: 10.1016/j.radonc.2021.12.022
47. Velalopoulou A, Karagounis IV, Cramer GM, Kim MM, Skoufos G, Goia D, et al. FLASH proton radiotherapy spares normal epithelial and mesenchymal tissues while preserving sarcoma response. Cancer Res (2021) 81(18):4808–21. doi: 10.1158/0008-5472.CAN-21-1500
48. Gaide O, Herrera F, Jeanneret Sozzi W, Gonçalves Jorge P, Kinj R, Bailat C, et al. Comparison of ultra-high versus conventional dose rate radiotherapy in a patient with cutaneous lymphoma. Radiother Oncol (2022) 174:87–91. doi: 10.1016/j.radonc.2021.12.045
49. Miles D, Sforza D, Wong JW, Gabrielson K, Aziz K, Mahesh M, et al. FLASH Effects Induced by Orthovoltage X-Rays [published online ahead of print, 2023 Jun 25]. Int J Radiat Oncol Biol Phys (2023) 117(4):1018–27. doi: 10.1016/j.ijrobp.2023.06.006
50. Bozhenko VK, Ivanov AV, Kulinich TM, Smirnov VP, Shishkin AM, Solodky VA. Comparison of biological effects of γ-radiation of low and ultra-high dose rate on lymphocytes and cultured human Malignant lymphoma cells. Bull Exp Biol Med (2019) 166(6):785–7. doi: 10.1007/s10517-019-04440-0
51. Jin JY, Gu A, Wang W, Oleinick NL, Machtay M, Spring Kong FM. Ultra-high dose rate effect on circulating immune cells: A potential mechanism for FLASH effect? Radiother Oncol (2020) 149:55–62. doi: 10.1016/j.radonc.2020.04.054
52. Beyreuther E, Brand M, Hans S, Hideghéty K, Karsch L, Leßmann E, et al. Feasibility of proton FLASH effect tested by zebrafish embryo irradiation. Radiother Oncol (2019) 139:46–50. doi: 10.1016/j.radonc.2019.06.024
53. Ohsawa D, Hiroyama Y, Kobayashi A, Kusumoto T, Kitamura H, Hojo S, et al. DNA strand break induction of aqueous plasmid DNA exposed to 30 MeV protons at ultra-high dose rate. J Radiat Res (2022) 63(2):255–60. doi: 10.1093/jrr/rrab114
54. Eggold JT, Chow S, Melemenidis S, Wang J, Natarajan S, Loo PE, et al. Abdominopelvic FLASH irradiation improves PD-1 immune checkpoint inhibition in preclinical models of ovarian cancer. Mol Cancer Ther (2022) 21(2):371–81. doi: 10.1158/1535-7163.MCT-21-0358
55. Karsch L, Pawelke J, Brand M, Hans S, Hideghéty K, Jansen J, et al. Beam pulse structure and dose rate as determinants for the flash effect observed in zebrafish embryo. Radiother Oncol (2022) 173:49–54. doi: 10.1016/j.radonc.2022.05.025
56. Cuitiño MC, Fleming JL, Jain S, Cetnar A, Ayan AS, Woollard J, et al. Comparison of gonadal toxicity of single-fraction ultra-high dose rate and conventional radiation in mice. Adv Radiat Oncol (2023) 8(4):101201. doi: 10.1016/j.adro.2023.101201
57. Owen D, Sio TT. Stereotactic body radiotherapy (SBRT) for central and ultracentral nodenegative lung tumors. J Thorac Dis (2020) 12(11):7024–31. doi: 10.21037/jtd-2019-cptn-01
58. Shampain KL, Hackett CE, Towfighi S, Aslam A, Masch WR, Harris AC, et al. SBRT for HCC: Overview of the technique and treatment response assessment. Abdom Radiol (NY) (2021) 46(8):3615–24. doi: 10.1007/s00261-021-03107-7
59. Singh R, Didwania P, Lehrer EJ, Trifiletti DM, Sheehan JP. Repeat stereotactic radiosurgery for locally recurrent brain metastases previously treated with stereotactic radiosurgery: A systematic review and meta-analysis of its efficacy and safety. J Radiosurg SBRT (2022) 8(1):1–10.
60. Ahmed KA, Torres-Roca JF. Stereotactic body radiotherapy for management of oligometastatic diseases. Cancer Control (2016) 23(1):21–9. doi: 10.1177/107327481602300105
61. Khan M, Garg R, Gui C, Lee Y, Sahgal A, Mossa-Basha M, et al. Neuroimaging and Stereotactic Body Radiation Therapy (SBRT) for spinal metastases. Top Magn Reson Imag (2019) 28(2):85–96. doi: 10.1097/RMR.0000000000000199
62. Chen HY, Ma XM, Ye M, Hou YL, Xie HY, Bai YR. Esophageal perforation during or after conformal radiotherapy for esophageal carcinoma. J Radiat Res (2014) 55(5):940–7. doi: 10.1093/jrr/rru031
63. Shaw E, Scott C, Souhami L, Dinapoli R, Kline R, Loeffler J, et al. Single-dose radiosurgical treatment of recurrent previously irradiated primary brain tumors and brain metastases: Final report of the RTOG protocol 90-05. Int J Radiat Oncol Biol Phys (2000) 47(2):291–8. doi: 10.1016/S0360-3016(99)00507-6
64. Kreinbrink PJ, Mierzwa ML, Huth B, Redmond KP, Wise-Draper TM, Casper K, et al. Adjuvant radiation and cetuximab improved local control in head and neck cutaneous squamous cell carcinoma: A Phase II study. Head Neck (2021) 43(11):3408–16. doi: 10.1002/hed.26835
65. Furusawa A, Takekuma M, Mori K, Usami T, Kondo E, Nishio S, et al. A randomized phase III trial of adjuvant chemotherapy versus concurrent chemoradiotherapy for postoperative cervical cancer: the Japanese Gynecologic Oncology Group study (JGOG1082). Int J Gynecol Cancer (2021) 31(4):623–6. doi: 10.1136/ijgc-2020-002344
66. Aird EG, Conway J. CT simulation for radiotherapy treatment planning. Br J Radiol (2002) 75(900):937–49. doi: 10.1259/bjr.75.900.750937
67. Sakai Y, Tanooka M, Okada W, Sano K, Nakamura K, Shibata M, et al. Characteristics of the bolus created using thermoplastic sheets for postmastectomy radiation therapy. Radiol Phys Technol (2021) 14(2):179–85. doi: 10.1007/s12194-021-00618-2
68. Ford S, Gollins S, Hobson P, Vyas S. Structural displacements during swallowing in patients with early laryngeal cancers and other early primary cancers of the head and neck. Dysphagia (2009) 24(2):127–36. doi: 10.1007/s00455-008-9163-2
69. Jeon H, Ki Y, Kim DW, Kim W, Nam J, Kim D, et al. Influence of respiratory movement during postmastectomy radiotherapy on targets and heart for breast cancer [published online ahead of print, June 2, 2022]. Asia Pac J Clin Oncol (2022) 19(2):e54–9. doi: 10.1111/ajco.13773
70. Righetto R, Fracchiolla F, Widesott L, Lorentini S, Dionisi F, Rombi B, et al. Technical challenges in the treatment of mediastinal lymphomas using proton pencil beam scanning and deep inspiration breath holding. Radiother Oncol (2022) 169:43–50. doi: 10.1016/j.radonc.2022.02.015
71. Serra M, De Martino F, Savino F, D’Alesio V, Arrichiello C, Quarto M, et al. SBRT for localized prostate cancer: CyberKnife versus VMAT-FFF, a dosimetric study. Life (Basel) (2022) 12(5):711. doi: 10.3390/life12050711
72. Zeverino M, Marguet M, Zulliger C, Durham A, Jumeau R, Herrera F, et al. Novel inverse planning optimization algorithm for robotic radiosurgery: First clinical implementation and dosimetric evaluation. Phys Med (2019) 64:230–7. doi: 10.1016/j.ejmp.2019.07.020
73. Zhang S, Lv B, Zheng X, Li Y, Ge W, Zhang L, et al. Dosimetric study of deep-learning-guided ITV prediction in conebeam CT for lung stereotactic body radiotherapy. Front Public Health (2022) 10:860135. doi: 10.3389/fpubh.2022.860135
74. Dvorak P, Knybel L, Dudas D, Benyskova P, Cvek J. Stereotactic ablative radiotherapy of ventricular tachycardia using tracking: optimized target definition workflow. Front Cardiovasc Med (2022) 9:870127. doi: 10.3389/fcvm.2022.870127
75. Ng WT, Chow JCH, Beitler JJ, Corry J, Mendenhall W, Lee AWM, et al. Current radiotherapy considerations for nasopharyngeal carcinoma. Cancer (baseline) (2022) 14(23):5773. doi: 10.3390/cancers14235773
76. Hodapp N. [The ICRU Report 83: prescribing, recording and reporting photon-beam intensitymodulated radiation therapy (IMRT)]. Strahlentherapie und Onkologie (2012) 188(1):97–9. doi: 10.1007/s00066-011-0015-x
77. Bahig H, de Guise J, Vu T, Chartrand-Lefebvre C, Blais D, Lebeau M, et al. Analysis of pulmonary vein antrums motion with cardiac contraction using dual-source computed tomography. Cureus (2016) 8(7):e712. doi: 10.7759/cureus.712
78. Kirkby KJ, Kirkby NF, Burnet NG, Owen H, Mackay RI, Crellin A, et al. Heavy charged particle beam therapy and related new radiotherapy technologies: Clinical potential, physics, and technical developments required to deliver benefits to patients with cancer. Br J Radiol (2020) 93(1116):20200247. doi: 10.1259/bjr.20200247
79. Guo XQ, Mao RH, Liu B, Ge H. Study on esophageal cancer radiotherapy dosimetry and position verification for volumetric modulated arc therapy. Asian J Surg (2023) 46(1):120–5. doi: 10.1016/j.asjsur.2022.02.001
80. Donovan EM, Bleackley NJ, Evans PM, Reise SF, Yarnold JR. Dose position and dose-volume histogram analysis of standard wedge and intensity-modulated treatments in breast radiotherapy. Br J Radiol (2002) 75(900):967–73. doi: 10.1259/bjr.75.900.750967
81. Chiavassa S, Bessieres I, Edouard M, Mathot M, Moignier A. Complexity metrics for IMRT and VMAT plans: a review of current literature and applications. Br J Radiol (2019) 92(1102):20190270. doi: 10.1259/bjr.20190270
82. Hong CS, Ju SG, Kim M, Kim JI, Kim JM, Suh TS, et al. Dosimetric effects of the multileaf collimator leaf width on intensity-modulated radiotherapy for head and neck cancer. Med Phys (2014) 41(2):021712. doi: 10.1118/1.4860155
83. Chen D, Cai SB, Soon YY, Cheo T, Vellayappan B, Tan CW, et al. Dosimetric comparison between Intensity Modulated Radiation Therapy (IMRT) vs dual arc Volumetric Arc Therapy (VMAT) for nasopharyngeal cancer (NPC): Systematic review & meta-analysis [published online ahead of print, 2022 Nov 28]. J Med Imaging Radiat Sci (2022) 54(1):167–177. doi: 10.1016/j.jmir.2022.10.195
84. Fan SM, Chen W, Xiong L, Xia Y, Xie YB, Chen J. Magnetic resonance diffusion tensor imaging study of rhesus optic nerve radiation injury caused by a single dose/fractionation scheme stereotactic radiosurgery at an early stage. J Neuroradiol (2016) 43(3):207–13. doi: 10.1016/j.neurad.2015.10.003
85. Wu J, Song H, Li J, Tang B, Wu F. Evaluation of flattening filter-free and flattening filter dosimetric and radiobiological criteria for lung SBRT: A volume-based analysis. Front Oncol (2023) 13:1108142. doi: 10.3389/fonc.2023.1108142
86. Kong FM, Ritter T, Quint DJ, Senan S, Gaspar LE, Komaki RU, et al. Consideration of dose limits for organs at risk of thoracic radiotherapy: atlas for lung, proximal bronchial tree, esophagus, spinal cord, ribs, and brachial plexus. Int J Radiat Oncol Biol Phys (2011) 81(5):1442–57. doi: 10.1016/j.ijrobp.2010.07.1977
87. Yafeng L, Jing W, Jiawei Z, Yingru X, Xin Z, Danting L, et al. Construction and verification of a radiation pneumonia prediction model based on multiple parameters. Cancer Control (2021) 28:10732748211026671. doi: 10.1177/10732748211026671
88. De Los Santos J, Popple R, Agazaryan N, Bayouth JE, Bissonnette JP, Bucci MK, et al. Image guided radiation therapy (IGRT) technologies for radiation therapy localization and delivery [published online ahead of print 2013/05/15]. Int J Radiat Oncol Biol Phys (2013) 87(1):33–45. doi: 10.1016/j.ijrobp.2013.02.021
89. Turner A, McGuffin M, Au P. An investigation of the feasibility and utility of a low-dose cone-beam computed tomography scan protocol for head and neck cancer patients. J Med Imaging Radiat Sci (2015) 46(2):141–7. doi: 10.1016/j.jmir.2014.10.005
90. El Naqa I, Pogue BW, Zhang R, Oraiqat I, Parodi K. Image guidance for FLASH radiotherapy. Med Phys (2023) 50(5):3244. doi: 10.1002/mp.15662
91. Zhang M, Zou W, Teo B-KK. Image guidance in proton therapy for lung cancer. Transl Lung Cancer Res (2018) 7:160–70. doi: 10.21037/tlcr.2018.03.26
Keywords: FLASH-RT, clinical application, simulations, target delineation, treatment plan evaluation
Citation: Lin B, Fan M, Niu T, Liang Y, Xu H, Tang W and Du X (2023) Key changes in the future clinical application of ultra-high dose rate radiotherapy. Front. Oncol. 13:1244488. doi: 10.3389/fonc.2023.1244488
Received: 22 June 2023; Accepted: 09 October 2023;
Published: 24 October 2023.
Edited by:
Rongxiao Zhang, Dartmouth College, United StatesReviewed by:
Tianyuan Dai, Dartmouth College, United StatesRaees Tonse, Baptist Hospital of Miami, United States
Copyright © 2023 Lin, Fan, Niu, Liang, Xu, Tang and Du. This is an open-access article distributed under the terms of the Creative Commons Attribution License (CC BY). The use, distribution or reproduction in other forums is permitted, provided the original author(s) and the copyright owner(s) are credited and that the original publication in this journal is cited, in accordance with accepted academic practice. No use, distribution or reproduction is permitted which does not comply with these terms.
*Correspondence: Xiaobo Du, ZHV4aWFvYm8yMDA1QDEyNi5jb20=
†These authors have contributed equally to this work