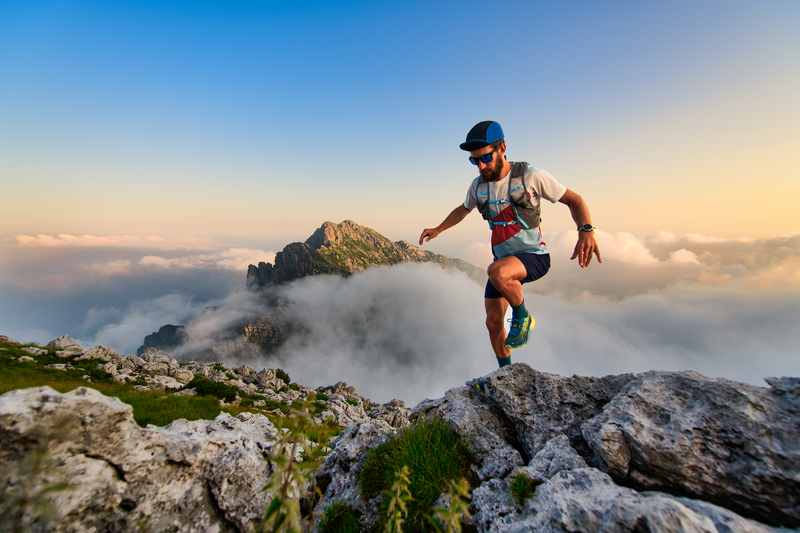
94% of researchers rate our articles as excellent or good
Learn more about the work of our research integrity team to safeguard the quality of each article we publish.
Find out more
REVIEW article
Front. Oncol. , 28 August 2023
Sec. Neuro-Oncology and Neurosurgical Oncology
Volume 13 - 2023 | https://doi.org/10.3389/fonc.2023.1236268
This article is part of the Research Topic Research Progress on Immune Microenvironment and Molecular Mechanism of Glioma View all 9 articles
Gliomas, the most prevalent primary tumors in the central nervous system, are marked by their immunosuppressive properties and consequent poor patient prognosis. Current evidence emphasizes the pivotal role of the tumor microenvironment in the progression of gliomas, largely attributed to tumor-associated macrophages (brain-resident microglia and bone marrow-derived macrophages) that create a tumor microenvironment conducive to the growth and invasion of tumor cells. Yet, distinguishing between these two cell subgroups remains a challenge. Thus, our review starts by analyzing the heterogeneity between these two cell subsets, then places emphasis on elucidating the complex interactions between microglia and glioma cells. Finally, we conclude with a summary of current attempts at immunotherapy that target microglia. However, given that independent research on microglia is still in its initial stages and has many shortcomings at the present time, we express our related concerns and hope that further research will be carried out to address these issues in the future.
The characteristics of brain tumors include high incidence and mortality rates and are usually locally aggressive in growth. Brain tumors are predominantly caused by cancers originating outside the central nervous system (CNS) that have metastasized, while gliomas and meningiomas represent the most frequently occurring primary brain tumor varieties, with gliomas constitute nearly 30% of all primary brain tumors and 80% of all malignancies. Consequently, gliomas are the primary cause of death in patients with primary brain tumors (1). Based on the World Health Organization (WHO) taxonomy, gliomas are classified into four grades based on the presence or absence of mesenchymal features, with WHO grade I indicating the lowest malignancy and grade IV the highest. Generally, grade I/II gliomas are low-grade gliomas (LGG), and grade III/IV gliomas are high-grade gliomas (HGG), which include the most malignant glioblastoma (1, 2). In general, tumor grade is negatively correlated with patient prognosis, with median survival time remaining less than 12 years even for LGG (3). Thus, the prognosis for HGG patients is worse, with a median overall survival (OS) time of approximately 3 years for grade III glioma patients, while the median OS time for grade IV glioma patients is just slightly over a year (4). However, recent discoveries indicate that patients with gliomas carrying isocitrate dehydrogenase (IDH) mutations exhibit a comparatively high survival rate compared to wild-type patients (5). Annually, around 100,000 individuals globally receive a diagnosis of diffuse glioma, but account for less than 5% of new cancer diagnoses, yet possess a high mortality rate. Of these, glioblastoma, a grade IV glioma, is the most lethal and constitutes 70-75% of all diffuse gliomas. Globally, there are significant differences in glioma incidence between Europe and Asia, with age, gender, ethnicity and tumor histology also contributing, in addition to geographically-induced differences in incidence. In addition, the age and gender of the patient also shape the final survival rate (6). The current conventional treatment for adult patients with malignant glioma is a combination of surgical resection, postoperative radiotherapy and chemotherapy. However, this standard treatment regimen has strong limitations in terms of the clinical outcomes it brings to patients. And high-grade gliomas tend to recur, which often prevents complete clinical cure of gliomas (7). At the same time, glioma patients undergoing conventional surgical treatment often cause an increased degree of loss of their own neurological function or the development of new neurological impairment, that is typically linked to an unfavorable ultimate prognosis for the patient (8).
The brain was previously considered an “immune privileged” organ with immunosuppressive properties due to its protection from various immune cells by the blood-brain barrier (BBB) (9). However, as early as 1925, Wilder Penfield first described microglia in the context of glioma and proposed that microglia had a significant function in extracellular matrix (ECM) remodeling (10). And a later research by Bettinger’s team in 2002 suggested that the remodeling effect of microglia on the ECM was pro-tumor, as the migratory capacity of glioma cells was enhanced threefold in the background of the presence of microglia (11). And in a subsequent study, microglia and blood/bone marrow macrophages (BMDMs) were identified as the predominant infiltrating cell population in gliomas, and it was confirmed that microglia and exogenous macrophages could serve as a crucial factor in the immune escape of gliomas (12, 13). Furthermore, it is proposed that gliomas are complex tumors composed of tumor and non-tumor cells, where most of the non-tumor cells are tumor-associated macrophages (TAMs) composed of microglia and exogenous macrophages, and TAMs can account for 30%-40% of the tumor volume, surpassing the quantity of infiltrating lymphocytes within the tumor by a large margin. Thus they become an important component of the tumor microenvironment (TME), providing a tumor-promoting matrix for tumor cell proliferation and invasion. Immune cells and non-tumor cells in the TME create an immunosuppressive microenvironment for glioma via the secretion of multiple cytokines, chemokines, growth factors, and other mediators that contribute substantially to glioma progression (14–16). The TME re-educates TAMs to have different gene expression profiles as well as altered functions, so that there is functional, spatial and temporal heterogeneity of action of TAMs in microglia and blood/bone marrow macrophages (BMDMs) (17).
Therefore, we can conclude that TAMs serve as a crucial factor in the TME and contribute to tumor progression through a close interplay relationship with glioma cells. However, the differences between microglia and BMDMs cell subpopulations and the mechanisms of interactions between microglia subpopulations and gliomas are not clear at present. And based on the poor prognosis of glioma under current conventional treatment, we may appropriately explore new options for microglia-based tumor treatment.
Since the discovery of microglia in the brain by the Spanish neuroscientist Pío del Río Hortega in 1919, there has been a debate about their biological origin to date. The argument for an extracerebral origin was made when some researchers found specific macrophage antigens on the surface of microglia (18). Research on the origin of microglia has never ceased in recent years. In early transplantation experiments with green fluorescent protein (GFP)-labeled bone marrow (BM) hematopoietic cells, GFP-labeled microglia were detected in the cerebellum, striatum, and hippocampus, indicating that peripheral cells may partially replace microglia (19). Later, the Hickey WF team used a model of BM cell transplantation and symbiosis to somewhat answer the question of how microglia maintain themselves in the adult brain. The results showed that BM replaced only perivascular macrophages, but not branched ramified microglia in the brain parenchyma (20). In contrast, the study by Mildner A et al. proposed that the heterogeneitybetween microglia and BMDMs does not stem from prolonged developmental isolation but instead arises from the CNS’s local milieu, and suggested that microglia are transformed from Ly-6ChiCCR2+monocytes as precursor cells. Surprisingly, under protection of the head from radiation, any significant infiltration of cells of bone marrow origin was not observed in the brain, suggesting that this sporadic substitution was due to cell entry resulting from radiation disruption of the BBB with enhanced permeability (21). Recently, a study has used a new non-cleared marrow transplantation (NMT) murine model that precisely differentiate glioma microglia and BMDMs, and from which the two can be distinguished. This model achieves significant levels of peripheral hematopoietic chimerism without BBB disruption or cellular infiltration before tumor implantation when low-dose busulfan is administered prior to bone marrow transplantation (22). In addition, in mouse models of multiple sclerosis and autoimmune encephalitis (EAE), the two cell subpopulations of microglia andexogenous macrophages were indistinguishable from each other and they were recruited from proliferating resident precursors and blood-derived progenitor cells, respectively. Symbiosis and myeloablation was used to replace circulating progenitor cells without affecting CNS-resident microglia, but recruited monocytes disappeared, thus demonstrating that exogenous monocytes don’t ultimately contribute to the resident microglia pool (23).
Previous studies have used several of the specific experimental models described above, including bone marrow (BM) transplantation of syngeneic chimeric mice and sorting models by cell surface specific antigens. It has often been concluded that microglia will be partially replaced by exogenous monocytes or supplemented by blood-derived microglia progenitors. However, when it comes to microglia activation models as well as immune encephalitis models, it was concluded that the complementation or replacement of resident microglia pools by exogenous monocytes was not evident. Until recently fate-mapping studieshave revealed that immature yolk sac progenitor cells are the predominant initial source of microglia in the brain. The survival of the cell subpopulation is enabled by a long life span and a limited number of self-renewals (14, 24). The discovery of an ancestral marker, nestin, confirmed that microglia progenitor cells are not bone marrow hematopoietic cells. Multiple experiments above have demonstrated that microglia are resident in the CNS, but BMDMs is not a significant contributor to the microglia pool and recruited BMDMs do not persist in the CNS (24). However, recently Peng B’s team overturned the previous conclusion that repopulating microglia originated from Nestin-positive precursor cell differentiation by multiple genealogical tracing techniques, as well as the fact that no repopulating microglia of blood origin were found in any of the studies, thus also ruling out the hypothesis of blood origin of repopulating microglia and finally finding that their repopulating microglia originated exclusively from residual microglial cell proliferation (25).
Regardless of the findings of subsequent studies, we can easily conclude that microglia and BMDMs are two separate subpopulations of bone marrow cells, and although there is a small amount of substitution or complementation in the context of blood-brain barrier disruption, the two cell subpopulations should still correspond to different progenitor cells in terms of cellular origin, with obvious origin Heterogeneity of origin.We summarize in Table 1 the heterogeneity that exists between microglia and BMDMs, including the cell origin.
Normally, the quantity of macrophages within the CNS is considerably lower than that of microglia and is mainly concentrated around the perivascular space, meninges, and organs adjacent to the ventricles and choroid plexus, and is seldom observed within the brain parenchyma (50). In the context of glioma, microglia constitute between 13% and 34% of the cellular population within the tumor, are distributed throughout the CNS, and are present in the tumor, the tumor periphery, and the contralateral tumor-free hemisphere. In contrast, macrophages account for a lower proportion of only 4.2-12% in the tumor and peritumor and only about 1% in the contralateral tumor-free hemisphere (26). The genetic lineage tracing approach also confirmed that the spatial and temporal distribution of microglia and BMDMs differed significantly in the tumor, with BMDMs are generally restricted to the interior of the tumor, however microglia were present both inside and outside the tumor, and both were generally enriched near the associated blood vessels inside the tumor, with BMDMs being more enriched than microglia. Microglia-derived TAMs predominate in tumors that have just been diagnosed, yet gradually decrease until they disappear as the glioma progresses. However, in the context of tumor recurrence, especially in the hypoxic tumor environment, the number of monocyte-derived TAMs exceeds this number. In contrast, in the context of BMDMs depletion, the proportion of microglia increases instead, suggesting that to some extent microglia may be regulated by the number of BMDMs occupied (27, 28). Friebel E’s team monitored resident and invasive leukocyte populations in the CNS in the context of glioma by multiple techniques of single cell proteomic analysis, immunofluorescence imaging and gene fate mapping. The results showed that microglia-derived TAMs dominate and are diffusely dispersed throughout the glioma, but they are restricted to the boundary area of the tumor and are not found in the central region of BrM (brain metastases), whereas invading exogenous macrophages dominate the immune microenvironment of BrM (29). The proliferation of both tumor-associated microglia and BMDMs showed increased proliferation in IDH-wt (wild-type) gliomas and BrM, whereas only microglia proliferation was upregulated in IDH-mut (mutant) gliomas. Simultaneously, the microglia to BMDMs ratio varied across different high-grade glioma subtypes (30). This suggests that the type of brain tumor determines the type of subject cell population infiltrating the tumor microenvironment as well as its distribution. Furthermore, in glioma specimens obtained from human patients, immune cells isolated from tumor margins demonstrated elevated expression of genes encoding cytokines (CCL3/4 and TNF) and pro-inflammatory interleukins (IL-1A, IL-1B and IL6-R), whereas immune cells isolated from tumor cores upregulated genes involved in angiogenesis (VEGFA/B) and those encoding pro-inflammatory inhibitors of cytokines (IL1RN). This phenomenon suggesting that TAMs infiltrating the tumor are predominantly pro-tumor phenotypes and also showing that microglia and BMDMs may play relatively different roles in tumor progression (51).
In addition to studies on the origin of the two cell subpopulations and their distribution in the brain, the phenotypic characteristics or markers of microglia have been a subject of heated discussion. Over an extended period, the main method to differentiate between exogenous monocytes and resident microgliain the brain was the CD45 antibody, which is generally considered to be less expressed in microglia (26). It is on the basis of this traditional method of differentiation that it has been suggested that the core cell population in gliomas is exogenous macrophages rather than microglia, as its major cell population was detected to be highly expressing CD45 (52). To explore the unique physiological role of microglia in the tumor microenvironment (TME), early studies have largely relied on the surface protein CD45 as well as cell morphology to differentiate, however, these two features are not specific markers of microglia. In addition, Badie B et al. suggested that microglia in the TME may also upregulate their own CD45 expression, reducing the accuracy of this CD45-based differentiation method (26). This is confirmed by the results of Müller A’s team, who used a new model of head-protected irradiation (HPI), which effectively avoids the effect of total body irradiation (TBI) on the BBB causing massive mononuclear cell infiltration and improves the accuracy of probing for tumor-associated cells. It is proposed that microglia in the context of glioma can increase their own CD45 expression and become part of the fixed expression of CD45 in glioma. This further reveals the obvious inadequacy of previous conventional differentiation methods (31).
At present, many experiments have used new technologies to explore the specific characterization of microglia in order to distinguish them from BMDMs cell subpopulations. Based on flow sorting techniques and transcriptome sequencing, marker molecules specific to primary microglia that are only expressed in their own were identified. Transcription factors, including Cebpe, Rhox5, E2f6, Phf17, Ppargc1b and Hoxc6. lipid metabolism-associated cell membrane molecules (LMACMs) Pap2c, Lpcat3, Lrp8, Stab1 and ion transport proteins (e.g. Slco4a1, Slc30a5, Mcoln3) in addition to the putative efflux cell membrane receptor (PECMR) Mfsd10 is also unique to microglia compared to macrophages (32, 33). Transcriptional level studies of microglia in human and mouse brains by single-cell RNA sequencing identified P2RY12/13, SLC2A5, CX3CR1 and TMEM119 as the most abundant genes (34). In human brain, microglia express P2RY12/13, TMEM119, and CD11b, but express CD45 at a lower level, whereas exogenous macrophages express CD45 and CD11b,with TMEM119 expression remaining significantly characterized throughout the growth of microglia (35). In addition Buttgereit A et al. also identified Sall1 as a microglia signature gene (36). Therefore, we generally consider that the core features of microglia in the brain consist of CX3CR1, TMEM119, P2RY12, etc., and considered them as microglia cell-specific surface markers (35, 37, 38).
Sankowski R et al. found by combined high-throughput experiments that gene expression in tumor-associated microglia was out of homeostasis, with down-regulation of expression of core features and up-regulation of expression of pro-inflammatory and metabolic genes involving SPP1, APOE, CD163 and several type I interferon genes. This high expression was also corroborated by CyTOF (Cytometry by Time-Of-Flight) technique, including upregulation of CD163, TREM2, APOE, HLA-DR and GPR56 (53). In addition, microglia-specific inhibition of Itga4 (CD49D) allows it to also serve as a marker for differentiation between microglia and BMDMs in the context of glioma (39). CD163 expression tends to be positively correlated with patient OS, however the microglia marker CX3CR1 lacks such correlation. Therefore, we can consider CD49D and CD163 as BMDMs markers (40). Fluorescent labeling of them using red fluorescent protein (RFP) and GFP, i.e. macrophages (CCR2-RFP) and microglia (CX3CR1-GFP), can also distinguish to some extent between the two cell subpopulations of microglia and BMDMs (41). However, in the context of glioma, CX3CR1 may no longer be a specific marker of microglia, as Feng X et al. indicated that exogenous monocyte-derived macrophages can also express CX3CR1 (42). This was also confirmed by the study of Yona S et al., which found that blood monocytes express CX3CR1, and its expression elevates when they differentiate into macrophages (43). In HGG, tumor cells can lead to local inflammation that results in the disruption of the integrity of the BBB and leads to monocyte infiltration into gliomas and differentiation into TAMs that are indistinguishable from microglia in the tumor microenvironment, which is one of the possibilities that led to conflicting results in a series of previous studies (23).
Therefore, the current studies aimed at distinguishing microglia from BMDMs cell subpopulations have several limitations. For instance, most studies do not trace the origin of macrophages recruited to the tumor site any further and cannot demonstrate that their target macrophages are single-derived monocytes of exogenous origin. Furthermore, no study has proposed one or more specific markers with absolute convincing power to distinguish between the two. This suggests that in order to distinguish more clearly between the two cell subpopulations, we may need to iterate the current experimental model to find a more realistic experimental approach in humans.
Researchers usually characterize macrophages by their bipolar differentiation status, with M1/2 phenotypes are deemed “pro-inflammatory” and “anti-inflammatory”, respectively. This dichotomous approach is widely used in macrophage typing (44). The M2 phenotype can also be subdivided into M2a, M2b and M2c polarization states (54). Microglia, although they have a macrophage-like polarized expression profile, may not be a true binary classification (45). This simplified dichotomy was also followed in several current studies based on TAMs, but transcriptomic analysis in microglia failed to demonstrate this relationship in vivo (46). Therefore, it is controversial whether microglia also exist in this polarized state in vivo (47). Genome-wide expression analysis of TAMs and comparison with expression data of M1, M2a, M2b and M2c polarized macrophages showed only a small overlap. Therefore, a simple dichotomy of M1/2 polarization may not be fully applicable for TAMs and can only be used as a reference classification method to facilitate the use of subsequent studies (48). Experiments by Müller S et al. proven that markers of M1 or M2 activation are able to be expressed simultaneously in the majority of microglia (49). And considering the complexity of transcriptional programming of microglia in gliomas, it goes beyond the simple division of M1/M2 polarization (30). Maas SLN et al. performed the isolation of cell subpopulations with palmitoylated fluorescent protein labeling and fluorescence-activated cell sorting (FACS) and analyzed their transcriptome. The results showed that as microglia approached the tumor, their anti-tumor genes were down-regulated while pro-tumor and immunosuppressive related genes were up-regulated. Therefore, they proposed a more precise scheme than M1/2 polarization classification, which classifies microglia by their proximity to the tumor (55).
The coexistence of microglia promotes earlier glioma cell migration and enhances tumor growth by several-fold. A similar effect was observed in microglia conditioned medium, so Bettinger I et al. suggest that this phenomenon is induced by microglia-secreted substances and is unique, since glioma cell migration is only weakly stimulated in the context of oligodendrocytes and endothelial cells. In addition mediators secreted by tumor cells can also activate microglia leading to a further increase in motility (11). We broadly depict the interactions between microglia and gliomas in Figure 1.
Figure 1 A schematic diagram outlining microglia-glioma cell interactions in the tumor microenvironment. (A) distribution of microglia and BMDMs in the hypoxic tumor microenvironment. (B) cytokines, signaling pathways, and surface receptors involved in microglia-glioma cell interactions. TGFR, transforming growth factor receptor; PD-L1, programmed cell death 1 ligand 1; PDGFR, platelet-derived growth factor receptor; EGFR, epidermal growth factor receptor; CSFR, colony-stimulating factor receptor; TLR, Toll-like receptors; P2X7R, P2X Purinoceptor 7; RAGE, receptor for advanced glycation endproducts. Created with MedPeer (www.medpeer.cn).
Under hypoxic conditions, glioma cells enhance glycolysis to deliver more lactate into the tumor microenvironment (56). Microglia exposure to lactate leads to a significant upregulation of MCT1 gene expression, where MCT1/2 are mainly accountable for lactate uptake (57). The recombinant protein of insulin-like growth factor binding protein 6 (IGFBP6), regulated by lactate levels in tumors, regulates microglia polarization toward the M2 phenotype, in addition to enhancing the capability of tumor cells to migrate and aggregate (58). However, in malignant tumors, enzymatic reactions, called “aerobic glycolysis” or “Warburg Effect”, continue to occur even under conditions of sufficient oxygen, as a result of hypoxia-induced transcription factors HIF-1/2, referred to as “pseudohypoxia” (59). In glioblastoma due to abnormal tumor angiogenesis leads to collapse of some of the proto-microvessels and formation of hypoxic Pseudopalisades regions. Microglia in hypoxic Pseudopalisades exhibit a specific pattern of movement along the fibers of glioma cells and show high phagocytic activity at the necrotic border of Pseudopalisades. The recruitment of microglia to the hypoxic niche is facilitated through HIF-1α downstream targets, and their motility is curtailed by hypoxia and shifted toward a pro-tumor phenotype. Hypoxic microglia infiltrating tumors promote tumor angiogenesis with HIF-1α-mediated upregulation of VEGF-A expression. In addition, macrophage migration inhibitory factor (MIF) is secreted to bind to CXCR4 on tumor cells to activate the Akt pathway to promote angiogenesis (60, 61). Glioma cells, stimulated by CD74, also secrete MIF and inhibit their IFN-γ secretion by phosphorylating the ERK1/2 pathway in microglia, inhibiting the shift of microglia to an anti-tumor phenotype (62). Interaction of tumor cells with microglia leads to high expression of LPA (Lysophosphatidic acid) and ATX (Autotaxin, an enzyme that synthesizes LPA) in microglia, supporting glioma progression and invasion, which is further enhanced in the hypoxic tumor microenvironment (63).
Epigenetic mechanisms can convert cellular signals into enduring cellular responses through phosphorylation, acetylation and DNA methylation, the effects of these epigenetic modifications are mediated by modifications to histones and chromatin (64). Pretreatment of microglia with glioma-conditioned medium (GCM) strongly reduces lipopolysaccharide (LPS)-induced inflammatory gene expression (c-Myc, Mark1), whereby microglia acquire “transcriptional memory”. Hdacs-mediated histone modifications induce microglial polarization, in which HDACs 5 and 9 are the main players. Therefore, histone deacetylase inhibitor (HDACI) can eliminate tumor-induced “transcriptional memory”, block glioma-induced microglia polarization, and restore microglia responsiveness to LPS and inflammatory gene expression (65). In contrast, it was found in microglia conditioned medium (MCM) to promote apoptosis in glioma cells, exhibiting extra cytotoxic effects especially in response to LPS or IFN -γ stimulation. This killing may also be caused by epigenetic modifications mediated by increased secretion of histone proteinase B and nitric oxide (66). Glioma-induced transformation of microglia to a pro-tumor phenotype is also linked to increased acetylation of H4K16 in microglia and enhanced nuclear translocation of the deacetylase SIRT1 (67). pHGGs, including glioblastoma and DIPGs, and pediatric and juvenile HGGs are unique in that H3-K27M mutation occurs in more than half of cases, and the activity of EZH2 (histone-lysine N-methyltransferase Enhancer of zeste homolog 2) is suppressed by the H3-K27M mutation (68). EZH2 is a histone methyltransferase (HMTs) that regulates normal cellular physiological functions by catalyzing the methylation of H3K27 (histone H3 lysine 27) to control the expression of various genes. EZH2 is involved in glioblastoma-induced immunosuppression, it induces IL-10 as well as TGF-β secretion in glioblastoma cells and attenuates microglia phagocytosis and shifts toward the M2 phenotype (69). Lily Keane’s team proposed the notion that inhibition of EZH2 in tumor cells could not have a practical effect, whereas inhibition of EZH2 in microglia may have a beneficial effect. Because EZH2 mediates the inhibition of MHC I/II in microglia, inhibition of EZH2 in microglia restores some of the immunogenicity of the tumor and reinforces the sensitivity to ICB (immune checkpoint blockade). Tazemetostat, an epigenetic drug EZH2 inhibitor currently used for the treatment of lymphoma (Tazemetostat) may be tried as a therapeutic agent for glioma (70).
Self-associated molecular patterns (SAMPs) acts as a modifying factor in tumor cells, block immune responses in their tumor microenvironment and promote the development of immunosuppression. This immunosuppression is primarily mediated by PRRs (pattern recognition receptors) expressed in microglia that recognize DAMPs (damage-associated molecular patterns)and PAMPs (pathogen-associated molecular patterns) (71). DAMPs induces microglia in the area surrounding tumor necrosis to express IL-1β in a TLR-mediated manner, which induces glioma cells to release a range of cytokines such as CXCL8,CCL2, IL-1β and IL-6. Among them, STAT3 and NF-κB pathways are strongly activated so that IL-6 and CXCL8 act synergistically to promote the proliferation of tumor cells (72). Glioma cells secrete S1P (sphingosine-1-phosphate), which functions as an intercellular signaling molecule that recruits microglia to the tumor region and transforms them into a tumor-supportive phenotype by suppressing inflammation mediated by the NF-kB pathway (73). IL-11 secreted by microglia activates STAT3 signaling pathways, leading to increased MYC expression, and MYC overexpression transforms glioma cells into a stem cell state, thereby enhancing tumorigenicity and TMZ resistance (74). The interaction between microglia and tumor cells is a crucial factor that initiates the secretion of IL-6, which leads to hyperpermeability of brain endothelial cells through activation of the JAK (Janus Kinase)/STAT3 pathway, ultimately leading to enhanced BBB permeability, and BBB damage is a critical step in the progression of brain tumors (75). Moreover, PDIA3 (protein disulfide isomerase A3) is expressed on both glioma cells and microglia, where the expression level is higher in microglia than in microglia in tumor peripheral tissues. PDIA3 expression in tumor cells promotes microglia polarization toward the M2 phenotype and releases mediators such as CCL2 and COX-2 to activate the JAK/STAT pathway (76). Damage to the BBB causes aseptic inflammation, causing infiltration of microglia and transition to a pro-inflammatory polarized phenotype (77).
The mTOR (mammalian target of rapamycin) signaling pathway is activated in multiple human neoplasms, which is involved in the crosstalk between microglia and glioma. Activation of the mTOR pathway within glioma-associated microglia is about twice as high as in peripheral tissues. In an in vitro model of glioma at all stages, pharmacologic blocking of mTOR decreases urea and ARG1 levels (considered a biomarker of the M2 polarization state of microglia), decreases the M2 polarization phenotype of microglia by 40% and increases the proportion of the M1 phenotype realizing its direct antitumor effects (78). It has been shown that glioma progenitor cells enhance mTOR signaling in microglia but not in BMDMs via the PI3K (phosphatidylinositol 3 kinase)/Akt (Protein Kinase B) axis. mTOR-mediated control of STAT3 and NF-κB activities promotes an Immune-suppressive microglial phenotype that impedes the proliferation, infiltration, and immunological responses of effector T cells, thus facilitating tumor immune escape and growth (79). Microglia releasing EGF binds to EGFR in glioblastoma activating its downstream PI3K/Akt/mTOR signaling pathway (80, 81). ZDHHC-type palmitoyl acyltransferases, which are aberrantly expressed in gliomas, promote glioma advancement and microglial invasion through the PI3K/Akt pathway. Blockade of ZDHHCs with 2-BP (2-bromopalmitate) promotes apoptosis of tumor cells and also promotes TMZ sensitivity of glioma cells (82). SLIT2 induces migration of ROBO1/2 (Roundabout1/2) receptor-expressing cells, and in malignant gliomas, SLIT2 expression increases with malignancy. SLIT2 promotes microglia chemotaxis and polarization toward a pro-tumor phenotype through ROBO1 and ROBO2-mediated activation of the PI3K pathway. SLIT in the SLIT-ROBO signaling axis can regulate cell motility mediating behaviors associated with tumor aggressiveness by regulating actin and the microtubule cytoskeleton.Tumor growth can be inhibited by inhibiting SLIT2 signaling (83, 84).
It is well known that the Wnt/β-catenin signaling pathway is implicated in the regulation of cell proliferation, migration and apoptosis, and promotes cancer progression (85). CCN4, also referred to as WISP1 (Wnt1-inducible signaling pathway protein-1), is secreted by GSCs to bind with integrin α6β1, thereby activating the Akt pathway to enhance GSC proliferation and microglia viability, creating a tumor-supportive cellular environment (86). In vitro experiments demonstrate that glioma cell-derived Wnt3a triggers the activation of the Wnt/β-catenin pathway in microglia, induces them to express ARG-1 and STI1, shifts them to the M2 phenotype, enhances tumor infiltration, invasion, growth and the crosstalk between microglia and gliomas (87). The proto-oncogene AEG-1, overexpressed in gliomas, is targeted to GSK-3β (glycogen synthase kinase-3β) to activate the Wnt/β-catenin pathway, mediating a pro-tumor phenotypic shift in microglia and reducing the sensitivity of glioma cells to TMZ (88). This also demonstrates that the Wnt/β-catenin pathway is also markedly upregulated in glioma cells. In addition to eliciting a phenotypic shift of immune-suppressive microglia to anti-tumor growth, inhibiting Wnt signaling also exerts a direct inhibitory effect on the growth of glioma cells (89).
CECR1,Cat Eye Syndrome Critical Region Protein 1, modulates microglia polarization and M2-type microglia exhibit sustained high expression of it, especially in malignant glioma. CECR1-induced paracrine effects in M2-type microglia activate MAPK (mitogen-activated protein kinase) signaling and stimulate migration and proliferation of glioma cells (90). Glioma cells activate the MEK (A kinase of MAPK)/ERK (extracellular regulated protein kinases) signaling pathway by secreting cytokines that downregulate the expression of aquaporin 1 (AQP1, a transmembrane glycoprotein involved in tumor angiogenesis) on microglia, attenuate their response to pro-inflammatory factors, and convert them to a pro-tumor phenotype, ultimately promoting tumor progression. The MEK inhibitor Trametinib, an FDA-approved drug for the treatment of lung cancer, inhibits this downregulation of expression, blocks the conversion of microglia to a pro-tumor phenotype, and inhibits glioma growth (91). Tumor-associated microglia promoting glioma cell invasion can be mediated by CSF-1R signaling on gliomas, a pathway involving CSF-1 signaling via ERK that upregulates the expression of dual-regulatory proteins (AREG) in microglia, which are ligands for epithelial growth factor receptor (EGFR) (92). The release of various cytokines such as EGF, PDGFβ and SDF-1α (CXCL12) from microglia activates the Pyk2 and FAK signaling pathways, thereby promoting proliferation and invasion of glioma cells (93). Glioma cells can maintain their own sustained proliferation by reducing Notch signaling, significantly inhibiting the recruitment of anti-tumor immune cell populations, contributing to the formation of anti-inflammatory microglia, and contributing to the immune escape microenvironment of glioma. Furthermore, as a result, gliomas will also gain resistance to microglia re-educating therapy (94).
Chemokines can directly stimulate the migration of diverse immune cells such as microglia in the TME, and May also enhance tumor progression and migration by directly affecting tumor cell proliferation and indirectly by regulating neovascularization and recruiting immunosuppressive cells. The crosstalk between microglia and glioma mainly involves the CC, CXC, and CX3C chemokine families. Chemokine receptors represent a heterogeneous group of GPCRs (G protein-coupled receptors) that tend to be expressed systemically, most commonly on immune cell populations and driven by inflammation. In addition, non-immune cells like endothelial cells are also capable of expressing it (95).
The gene expression of CCR1 receptor and its ligand was strongly upregulated in microglia exposed to glioma conditioned medium probably mediated by CSF-1R signaling, including CCL3/5/6/9,especially CCL3.However, the current study proposes that the CCR1 signaling axis may not be a major factor in microglia chemotaxis (96). Previously, it was generally believed that only macrophages expressed CCR2, but now studies have found that microglia in gliomas can also express it. In a mouse model of CCR2-knockout microglia, it was found that CCR2 deficiency reduced pro-inflammatory molecule expression, phagocytic activity, and migratory capacity and affected the degree of infiltration (97). However, a study by Mizutani M et al. suggested that CCR2 may not be expressed in the mouse model (98). CCL2, referred to as MCP-1 (Monocyte chemotactic protein-1), is secreted by glioma cells and binds to CCR2 expressed on microglia, inducing microglia to recruit to the glioma region and release interleukin-6 (IL-6) thereby promoting the invasion and growth of glioma cells (99, 100). CCL20 secreted by glioma cells induces CCL2 secretion by peritumor microglia (PGAM) and attracts a variety of peripheral immunosuppressive cells via CCR2/4 to participate in the establishment of the immunosuppressive microenvironment of glioma, which includes MDSC (myeloid-derived suppressor cells) and Tregs (regulatory T cells) differentiated from monocytes (101). This allows us to antagonize CCR2 for antitumor purposes, and studies have also confirmed that CCX872, a CCR2 antagonist, increases MS in animal models and further improves MS and OS when used in combination with anti-PD-1 (102). GM-CSF -induced CCL5 secretion by microglia may increase the level of glioma infiltration when tumors progress. The CCL5-CCR5 pathway has been demonstrated to promote tumor invasiveness by activating intracellular calcium cascade (increased calcium levels) and CaMKII (Calcium/Calmodulin Dependent Protein Kinase Kinase II)-dependent matrix metalloproteinase 2 (MMP2) upregulation in tumor cells (103). Venkataramani et al. observed that elevated calcium levels in glioblastoma cells also lead to the re-formation of glioblastoma microtubules thereby causing increased tumor aggressiveness (104). CaMKII has been found to be a contributing factor to ICB resistance by promoting CD8+ T cell depletion and reducing the proliferation of effector CD4+ T cells. And TAMs show high expression levels of CaMKII, which is often linked to a worse prognosis in patients with glioblastoma (105). Blocking the CCR5 receptor reduces microglia migratory capacity and blocks microglia M2 polarization and shift to M1 phenotype by inhibiting AKT pathway and mTOR pathway, respectively. So MRV (Maraviroc), a CCR5 antagonist, has potential as a pharmacological inhibitor (106). In Addition, microglia polarized to the M2 phenotype and upregulated their CCL18 expression in response to IL-4 stimulation. In contrast, CCL18, a chemokine expressed only in the cellular context of humans, is often linked to poor patient prognosis when its high expression inhibits the recruitment and maturation of cytotoxic cells, like dendritic cells or lymphocytes, and disrupts their immune competence, and shows capacity for binding to the CCR8 on glioma cells and promote tumor progression via ACP5 signaling (107, 108).
PDCD10 (Programmed cell death 10) overexpression in glioblastoma promotes microglia recruitment to the tumor core through elevating cell migration capacity and evokes microglia polarization toward a pro-tumor phenotype with CXCL2-CXCR2 axis signaling (109). Microglia and glioma cells secrete stromal-derived factor-1 (SDF-1 or CXCL12), a microglia recruitment molecule that normally binds to its receptor CXCR4, a specific receptor for CXCL12 expressed on microglia, to form the CXCR4-CXCL12 axis that attracts microglia to hypoxic regions of the tumor and mediates tumor proliferation, abnormal angiogenesis, invasion and drug resistance (110–112). CXCL14 secreted by PXA (pleomorphic yellow astrocytoma) cells binds to CXCR4, participates in the construction of the TME, and mediates the tropism of CD8+ T cells in a proportionate manner to the dose, in which the existence of a large number of activated microglia is also found (113). Glioma-secreted CXCL16 binds to CXCR6 on microglia, and the CXCL16-CXCR6 axis mediates the polarization of microglia, promoting a shift to a pro-tumor phenotype and promoting tumor invasion and progression (114).
CX3CR1 expression is mainly restricted to microglia, whereas CX3CL1 expression is mostly confined to neurons, and co-expression of CX3CR1 and CX3CL1 promotes microglia aggregation and increased tumor microvessel density through the mediation of CCL2 and matrix metallopeptidase 9 (MMP9) upregulation, contributing to tumor progression and shifting LGG’s to a higher malignant grade (115). In Nf1 optic gliomas, TAMs are mostly CD11bhigh CD45low, and in mouse models with downregulated CX3CR1 expression, yet exhibit delayed tumor growth (116). In contrast, CX3CR1 deficiency causes a dose-dependent overexpression of IL-1β by microglia. This activates the p38 MAPK pathway, upregulation of CCL2 expression, and enhancement of the tumor stem cell (GSC) phenotype, promoting glioma progression (42).
In glioblastoma, CLOCK and its heterodimeric chaperone BMAL1 behave as tumor-promoting factors, directly modulating the NF-κB pathway, thereby regulating glioma cell migration and proliferation, and maintaining GSC stemness. In addition, it enhances the transcriptional expression of OLFML3, a recently discovered chemokine that recruits immunosuppressive microglia to the TME to trigger pro-tumor immunity (117). Olfml3 is also a immediate target gene for all TGF-β isoforms in mouse microglia and is a crucial factor in TGF-β-induced pro-tumor phenotypic transformation of microglia, and also promotes tumor progression by increasing tumor cell invasion and migration capacity. In TME microglia-derived OLFML3 a pro-angiogenic factor distinct from VEGF (vascular endothelial growth factor), which promotes brain microvascular endothelial cell migration and germination mainly through activation of the SMAD1/5/8 signaling pathway (117, 118).
Significant upregulation of PDGF receptor (PDGFR) expression is a recognized marker of aberrations in the glioblastoma subgroup. Glioma cells express PDGFRA, while PDGFRB is predominantly localized in the glioma-associated extracellular matrix. M2-polarized microglia, rather than BMDMs, provoke PDGFRB expression in glioma cells and stimulate their migratory ability, and have a tight interaction with pericytes for intra-tumor angiogenesis. It recruits pericytes into the tumor microvascular system, not in combination with perfused vessels, but in the PDGF signaling pathway that mediates tumor progression (119).
The transmembrane receptor Nrp1 is a co-receptor for VEGF expressed on microglia that, when activated, drives microglia to migrate toward tumor areas and polarize toward the M2 phenotype, and correlates with tumor neovascularization and immunosuppressive microenvironment formation (120). In general, early microglia dominate the tumor, but with increased neovascularization, BMDMs becomes the main infiltrating cell population. However, microglia with downregulated Nrp1 can lead to reduced tumor neovascularization, preventing BMDMs from infiltrating the tumor and eventually reasserting a dominant position (121).
CD204-positive microglia typically accumulate around tumor vessels and in necrotic areas and are in close proximity to podoplanin+ (PDPN) stem cell-like glioblastoma cells. The number of CD204-positive microglia increases with glioma grade and tends to exhibit a pro-tumor phenotype. Furthermore, in gliomas with high CD204 expression, upregulation of genes related to angiogenesis often forecasts a negative prognosis for patients (122, 123).
The binding of RAGE receptors in glioma-associated microglia to various ligands like advanced glycation endproducts (AGEs), S100 proteins and high mobility group box 1 (HMGB1) mediates tumor angiogenesis and promotes glioma invasion and progression (90).
Cannabinoid binding to the CB1R/CB2R inhibits the growth of gliomas. Among them, CB2R expression directly correlates with the malignancy of gliomas, and the selective CB2 agonist JWH133 induces glioma cell apoptosis to induce tumor regression (124). However, one study suggested that JHW133-treated microglia would shift to an M2 immunophenotype with detectable overexpression of CYP2J2, which metabolizes more arachidonic acid in microglia to 11,12-EET (epoxyeicosatrienoic acid), of which 11,12-EET significantly promotes human brain microvascular endothelial cells (HBMECs)-mediated tumor angiogenesis, increasing cancer cell proliferation and preventing apoptosis, thereby promoting glioma progression (125, 126).
P2X7R was highly expressed on glioma-associated microglia and higher than on peritumor microglia, and the quantity of P2X7R was directly linked to the malignancy of glioma. P2X7R expression is also observed on glioma cells and may mediate the regulation of the immune phenotype of microglia and play a tumor-supporting and nutritional role. However, until now, there has been little progress in the development of P2X7R-targeted drugs in glioma. The application of P2X7R agonists and antagonists, such as OxATP, BBG and BzATP, has yielded varying outcomes in glioma models of both animal and human origin. AZ10606120, a potent antagonist of P2X7R, significantly inhibited tumor growth directly through microglia inactivation. It also demonstrated better effects than TMZ, the P2X7R agonist BzATP, and its inhibitors BBG and OxATP (127, 128). In zebrafish models studying brain tumors, high calcium levels in cells can be monitored and dynamically regulated, and calcium-stimulated ATP and P2RY12 signaling mediates the interaction of tumor cells with microglia, ultimately promoting their own proliferation (129). And it has been proposed that the swift closure of the BBB is dependent on the P2RY12-driven chemotactic response of microglia after BBB damage (130). Gliomas, in contrast to other malignancies, are more dependent on cell motility than blood circulation for their invasion and spread, and are therefore more regulated by calcium-dependent signaling in tumor progression. Apparently, not only calcium signaling is regulated in tumor cells, but calcium signaling in microglia is responsible for their activation and motility, especially their P2Y2 receptors, which have an important role in microglial cell chemotaxis (131). The morphological transition and motility patterns of microglia are regulated by purinergic signaling and mediated mainly through P2Y1, P2Y12 and P2Y13 receptors (132). In addition, some P2Y receptors are also implicated in the interplay between microglia and tumors, as an illustration, if selective stimulation of P2Y14 receptors on microglia reduces IL-6 secretion and even tumor cell proliferation (133).
Glioma cells secrete MMP-2 in an inactive state, which requires cleavage to attain an active form, microglia express the enzyme that cleaves MMP-2, MT1-MMP. In the normal brain, microglia generally do not express this enzyme or show only very low expression in cerebral white matter. However, it can be induced by endogenous ligand versican of TLR2 secreted by tumor cells in close contact with tumor cells, and its own TLR2 is activated to induce MT1-MMP expression, accompanied by up-regulated expression of MMP-9, and the magnitude of expression is directly proportional to the malignancy of glioma (134–136). In addition to versican ligands, TLR2-expressing microglia are also activated by glioma-released mediators, such as HMGB1 (high mobility group protein B1), HSP (heat shock protein), and hyaluronic acid stimulation leading to MT1-MMP upregulation, enhancing ECM restructuring which in turn enhances TLR2 signaling, ultimately promoting glioma expansion and invasion, constituting a vicious circle of microglia-glioma crosstalk (137). Minocycline inhibits glioma invasion and progression by attenuating MT1-MMP expression in microglia (138).
However, while minocycline was employed as an adjunctive therapy in clinical trials (NCT02770378, NCT02272270 and NCT01580969), it did not demonstrate actual clinical effects (139). Glioma cells release GDNF (Glial cell line-Derived Neurotraphic Factor), a chemotactic agent that recruits microglia to the tumor region and also induces a shift to a pro-tumor phenotype. This is accompanied by an upregulation of its TLR1 and TLR2 expression and mediates an increase in MMP-9/MMP-14 expression, promoting microglia-mediated glioma progression (140, 141). MHC class II expression is suppressed by TLR2 activation on microglia, limits CD4+ T cell-dependent antitumor immunity, diminishes the antigen presentation ability of microglia, and enhances the immunosuppressive profile of the tumor microenvironment (142). After microglia TLR3 and TLR9 are co-activated, microglia shift to an anti-tumor phenotype and enhance migratory activity to accumulate in glioma tissue, which in turn inhibits glioma cell function by releasing cytokines or directly kills glioma cells by enhancing phagocytic activity of microglia (143). Microglia tend to be highly plastic and are usually transformed to an anti-tumor M1 phenotype mediated by TLR4 activation and IFN-γ stimulation (144). The let-7 miRNA family with conserved sequences is an immunomodulatory factor expressed widely in the brain and is involved in glioma development and progression (145). Let-7 miRNA directly activates TLR7 in microglia, leading to upregulation of MHC I and ICAM1 (CD54) and induction of TNF-α production to inhibit glioma growth (146). Activation of TLR5 in microglia and mediates the release of multiple inflammatory molecules causing inflammatory neuronal damage in the CNS, but in contrast to TLR 2/3/4/7/9, TLR5 is not involved in microglia-glioma interactions (147).
CSF-1 secreted by glioma cells acts on CSF-1R on microglia, which attracts microglia to the tumor and induces a shift to a pro-tumorigenic phenotype, enhancing the crosstalk between microglia and glioma. Simultaneously, stimulating microglia to express EGFR also facilitates tumor cell invasion and growth (80). Nijaguna et al. demonstrated that glioma-secreted CSF-1 stimulates microglia to upregulate IGFBP1 expression levels and that upregulation of IGFBP1 underlies tumor angiogenesis (148). Colony-stimulating factor 2 (CSF-2), which is also called GM-CSF, is a glioma-derived CSF-2 that attracts and induces microglia to polarize into a pro-tumor phenotype. When it is knocked down or depleted, a decrease in glioma cells and apoptotic microglia are found (149). In contrast, in a rat model, stimulation with G-CSF (CSF-3) enhanced microglia proliferation and infiltration of tumors, accompanied by a decrease in TGF-β and IL-10 production and enhanced antigen expression, ultimately improving survival in rats transplanted with hypodifferentiated malignant gliomas by altering the tumor microenvironment (150).
Human interleukin-33 (IL-33), belonging to the IL-1 family, is a cytokine that mediates endogenous cellular alerts and exerts a tumor-promoting effect in various types of tumors, gliomas included (151). IL-33 exists in nuclear and secretory forms; nuclear IL-33 stimulates glioma cells to express inflammatory cytokines (IL-1β, IL-8, IL-6, etc.), and secretory IL-33 is secreted by glioma cells, which activates microglia and drives them to a pro-tumor phenotype; both synergistically accelerate glioma progression (152).
IL-10 secreted by microglia in gliomas is an immunosuppressive cytokine and correlates with the malignancy of gliomas (153). Concurrently, glioma cells can also secrete the immunosuppressive factor interleukin-10 (IL-10) via an autocrine or paracrine pathway and microglial PD-L1 expression is elevated, thus promoting the formation of an immunosuppressive tumor microenvironment (154). In addition, GSC also induces IL-10 secretion from microglia and polarizes microglia to the M2 phenotype, inhibiting microglia phagocytosis (155).
TGF-β is an immunosuppressive factor in gliomas and patient prognosis is linked to the level of its expression. Tumor tissues expressed both TGF-β1 and TGF-β2 isoforms of TGF-β. TGF-β1 secreted by glioma cells activates microglia in tumors and secretes IL-1β through lipoprotein E (ApoE)-mediated NLRP1 inflammasome. TGF-β2 affects brain tumor-initiating cells (BTIC) proliferation and migration and stimulates tumor angiogenesis thus exerting an immunosuppressive function, and the two TGF-β isoforms synergistically promote tumor progression (156, 157). TGF-β1released from tumor-associated microglia promotes the expression of MMP-9 in GSC and maintains the characteristics of glioma stem cells, enhancing the aggressiveness of gliomas (158). Targeted blocking of TGF-β presents a promising therapeutic approach, but it is currently found to be less feasible because of its severe side effects, resulting in acute inflammation and disturbance of immune system balance (159). Liu H et al. proposed that depletion of TGF-β1/TGFBR1 (TGF-β type I Receptor) could reduce the concentration of microglia within tumors and suppress tumor growth (157). The better prognosis of patients with simultaneous low expression of TGFBR1 and PD-L1 is in some agreement with the above-mentioned studies, making the combination of TGF-β1/TGFBR1 inhibitors and PD-1/PD-L1 inhibitors a potential immunotherapeutic strategy for glioma (160). Furthermore, research conducted by Ye XZ and colleagues has indicated that knockdown of TGFBR2 (Transforming Growth Factor Beta Receptor 2) diminishes the invasive potential of glioma cells (158). The use of plasmid-transcribed small hairpin RNA (shRNA) to downregulate TGFBR2 expression also can achieve exactly this effect, i.e., to eliminate the glioblastoma invasive and migratory response induced by microglia-secreted TGF-β (159).
An often-observed mechanism of immune escape in tumors is the upregulation of CD47 (Integrin-associated protein, IAP) expression and evasion of immune surveillance by binding to SIRPα on phagocytes and inhibiting their phagocytic capacity (161). Elevated LRIG2 (Leucine Rich Repeats And Immunoglobulin Like Domains 2) gene expression in glioblastoma cells triggers CD47 upregulation and activates the CD47-SIRPα anti-phagocytic axis (162). CD47-SIRPα signaling prevents excessive microglia phagocytosis, ensuring that microglia engulf specific synapses and avoid others, maintaining normal developmental growth of synapses (163). Microglia were shown to act as effector cells against the action of the CD47-SIRPα axis even in the absence of a background of peripheral macrophages, and the phagocytic capacity of microglia was also stronger than that of macrophages in tumors. This demonstrates that microglia have an anti-tumor effect independent of macrophages (41).
Elevated expression of GPNMB (glycoprotein non-metastatic B) and SPP1 in microglia is linked to an unfavorable prognosis in glioblastoma patients (48). SPP1 (secretory phosphoprotein 1) also identified as OPN (osteopontin). In glioma perivascular ecotone, OPN promotes increased stem cell-like properties (HIF-2α-mediated) and radioresistance of neighboring tumor cells through activation of CD44 signaling and also have a synergistic effect on PDGF-induced aggressive growth of glioblastoma (164). CD44 is a transmembrane glycoprotein involved in intercellular or interactions with the matrix. CD44 in microglia is pivotal for the initiation of the TLR2 pathway and the upregulation of TNF-α, MMP9 and IL-1β expression (165). Additionally, integrin αvβ5 (ITGαvβ5) is highly expressed as a key receptor for OPN on tumor-infiltrating microglia, and the binding of both mediates the recruitment of glioma cells to microglia and polarization to the M2 phenotype, and reduces the direct cytotoxic sensitivity of tumors to CD8+ T cells (166). In gliomas, GSCs are also predominantly clustered in the perivascular niche and are usually surrounded by tumor-associated microglia, positively correlated with the cell density of microglia, suggesting that GSCs may be more adept at recruiting microglia than glioma cells (167).
Fasl is a type II transmembrane glycoprotein known as a death factor, and Fas-FasL binding mediates programmed cell death. Microglia represent the major origin of FasL expression in gliomas and are responsible for immune escape in the glioma microenvironment by promoting apoptosis of T cells that express FasL (168). Dcf1 (Dendritic cell-derived factor 1) also is a membrane protein that significantly contributes to the differentiation of neural stem cells. Available studies indicate that its overexpression in glioma cells can lead to reduced proliferation and invasive capacity of glioma cells, even mediate apoptosis of tumor cells by disrupting mitochondria (169). Dcf1 deficiency has a large impact on microglia function, promoting microglia activation but reducing their migration and phagocytosis (170). During tumor initiation, pre-neoplastic cells also can reduce microglia migration and phagocytosis by altering their Wasla gene and WASL expression, leading to a morphological shift of microglia to amoeboid morphology. WASL plays a critical role in actin cytoskeleton organization and is essential for maintaining microglia core activity during the initiation phase of glioma (171).
The interaction of glioma with microglia induces high expression of SELP and induces microglial polarization towards a pro-tumor phenotype through the SELP-PSGL-1 (P-selectin with P-selectin glycoprotein ligand-1) axis, enhancing their immunosuppressive capacity and promoting tumor progression. Recombinant SELP decreased the phagocytic activity of microglia and reduced the expression of nitric oxide synthase (iNOS) and the release of nitric oxide (NO). Anti-SELP therapy holds potential for enhancing tumor sensitivity to immunotherapeutic approaches and conventional radiotherapy treatments (172). Additionally, Stress-Inducible Phosphoprotein 1 (STIP1), abundantly present in glioma cells, and Stress-Inducible Protein 1 (STI1), released by microglia, significantly contribute to the progression of glioblastoma multiforme, with their expression levels positively associated with the degree of tumor malignancy (173).
The International Society for Extracellular Vesicles (ISEV) designates EVs as irreproducible microparticles composed of lipid bilayers that are naturally secreted by cells. The diameter of small EVs (sEVs) typically ranges below 200 nm and include the well known exosome and medium or large EVs (mEVs or lEVs) with diameters between 200 and 1000 nm (174). In addition to differences in size, the two subtypes differ in terms of their formation mechanisms and cargo. EVs in general can transport biologically active molecules like nucleic acids, lipids and proteins between cells, consisting of DNA and assorted RNA types (microRNA and mRNA) (175).
glioblastoma patients generally exhibit elevated levels of EVs in their plasma compared to healthy individuals, suggesting that EVs could play a role in the pathogenesis and advancement of gliomas (176). EVs, as substances that mediate intercellular communication, can execute diverse functions during glioma progression including promoting immunosuppression and immune escape, and tumor cells secrete about 10-fold more EVs than normal cells. The interaction between EVs and microglia is further intensified by the hypoxic and acidic milieu of the TME, inducing a shift toward an anti- or pro-tumor immune phenotype in microglia (177). This was confirmed in an in vitro model. Exposure of microglial cells to glioblastoma cell-derived EVs revealed an upregulation of the expression of the pro-tumor factors CXCL1/10, CCL2/CCL5 and IL-6 as well as an increase in their own proliferation. And Spellicy SE et al. also suggested that glioma-derived EVs may exert a selective influence on microglia, which is not evident in other immune cells such as BMDMs (178). Furthermore, overexpression of Cavin1 (polymerase Iand transcript release factor or PTRF) enhances the secretion of pro-tumor type EVs by glioma cells and demonstrates a direct association with the malignancy of glioma patients. Mediators such as IFN-γ/LPS, Wnt3a, and 5-hydroxytryptamine in the TME is able to trigger the release of EVs from microglia (174, 179). GSC transfer lncRNA MALAT1 (nuclear-enriched abundant transcript 2, NEAT2) cells into microglia in an EVs manner and regulate inflammatory responses mediated by the miR-129-5p and HMGB1 cascade to achieve pro-tumor effects (180). Secretion of EVs-mediated miRNA-21 transfer by glioma cells triggers the downregulation of particular mRNA targets in microglia, reprogramming microglia, with more prominent alterations in Btg2, thereby reducing cell cycle protein D1 activity to negatively regulate cell proliferation, leading to enhanced proliferation of microglia and tumor cells and promoting glioma progression (181). M2 phenotype microglia can promote glioma proliferation and migration by delivering miRNA-7239-3p to glioma cells via exosomes, regulating their tumor-associated protein expression (BMAL1 down-regulation, CLOCK up-regulation) and reducing tumor cell apoptosis (182). In contrast to this situation, microglia released sEVs after stimulation with LPS/INF-γ and IL-4 exhibit anti-tumor effects, in addition unstimulated microglia may release more sEVs and also reduce the invasion of glioma cells. This may be mediated by miR-124 in sEVs, causing a decrease in lactate, nitric oxide and glutamate in the microenvironment, inhibiting tumor neovascularization and tumor cell proliferation (183). Externally, miRNA-504 is overexpressed in GSCs and delivered to microglia via EVs, promoting their polarization to the M1 phenotype and exerting a bystander effect on surrounding microglia. In addition, miRNA-504 also inhibits angiogenesis and GSC stemness, which exerts a synergistic tumor suppressive effect (184). Under hypoxic conditions, glioma-derived exosomes mediated their pro-tumor effects by activating STAT3 and inhibiting NF-κB signaling, with miRNA-1246 being the most enriched and also highly concentrated in the CSF of patients (185). WT1 expression is notably elevated in EVs isolated from the CSF of patients with malignant glioma, and glioma-derived EVs with WT1 promote tumor progression by suppressing the expression of the Thbs1 gene(Thbs1 is a negative regulator) and TSP-1 (TSP-1 has anti-tumor angiogenic properties) production in microglia (186). From this, it is natural to think that monitoring EVs in blood or CSF could be a new attempt for clinical biopsy of glioma. One study attempted to detect CSF-derived EVs to diagnose EGFRvIII-positive glioblastoma with a sensitivity of over 60% and a specificity of over 95% (176). Given that EVs are pivotal in the interaction between glioma and microglia, it is possible to consider ways to inhibit tumor progression by modifying or selecting specific cargoes. For example, Fareh M et al. posited that the replication and tumorigenicity of glioma cells could be reduced by modifying them to sustain the expression of miR-302-367 and to be internalized by surrounding glioma cells through paracrine secretion (187). In addition, EVs-based drugs are thought to be effective through the BBB, and then EVs can be used as a platform to inject miRNA-124 into the TME to inhibit glioma cell proliferation and migration. Moreover, by targeting the STAT3 pathway, it can impede microglia polarization toward the M2 phenotype (188, 189).
Patients with glioma often receive conventional radiation therapy after surgery, and although the dose of radiation therapy is positively correlated with patient prognosis, serious complications such as neuroinflammation, gliosis, cerebral atrophy, and cerebral hemorrhage can occur with increasing dose, where neuroinflammation and reactive gliosis are mediated by PGE2 secreted by microglia. In parallel, rising BBB permeability microglia are recruited to enhance the pro-inflammatory microenvironment (190). Despite numerous attempts over an extended period, the approval of new drugs for glioma treatment remains limited. The unique physiological structure of the BBB presents significant challenges to drug development (191). Therefore, until now, the conventional treatment of glioma is still limited to surgical resection, TMZ and radiotherapy, and there are very few other efficient treatment options available, so that the survival outcome for patients with glioma has shown little improvement in recent years. Hence, there is an urgent demand for the development of novel therapeutic approaches that can make a difference to the current situation, and targeted immunotherapy has become a popular area of research (192).We summarize potential antitumor therapies targeting microglia in Table 2.
PD-1, also known as CD279, is an immunosuppressive mediator found in various tumors, gliomas included. PD-L1 is also present in gliomas and correlates with tumor grade (209). Anti-PD-1 therapy in conjunction with radiotherapy has been shown to be effective against glioma in a mouse model (210). Nivolumab is an FDA-approved human IgG4 anti-PD-1 monoclonal antibody that, in conjunction with ipilimumab, is used to treat metastatic melanoma tumors. The effectiveness of this combination therapy for recurrent glioblastoma was assessed in a phase III clinical trial (NCT02017717), but the results suggested that the combination of the two drugs did not confer any survival benefit in terms of overall survival (211). Then for Nivolumab monotherapy, there are also large phase III clinical studies underway (NCT02667587, NCT02617589) for the evaluation of Nivolumab combined with radiotherapy ± TMZ for the management of newly diagnosed glioblastoma patients, where NCT02667587 showed no improvement in patient survival with this regimen (212). One of the contributing factors to the lack of clinical efficacy of this combination of standard RT + anti-PD-1 may be the existence of microglia within the tumor (193). The M1 microglial phenotype contributes to the effectiveness of anti-PD-1 immunotherapy in gliomas, while recruitment and clustering of the M2 phenotype are linked to anti-PD-1 resistance. Additionally, the upregulation of PD-L1 expression in microglia impacts treatment efficacy (213). Immune checkpoint inhibition (ICI) is generally ineffective against glioblastoma, primarily due to its immunosuppressive tumor microenvironment and limited CD8+ T cell infiltration. Furthermore, microglia can inhibit CD8+ T cell cytotoxicity through several mechanisms during tumor development and treatment. Anti-PD-1 can suppress microglia proliferation and induce apoptosis via antibody-dependent cytotoxicity (ADCC). Moreover, anti-PD-1 antibodies can directly penetrate the glioma microenvironment, kill PD-1-expressing microglia, and convert them to an anti-tumor phenotype. Therefore, in gliomas with limited T-cell infiltration, anti-PD-1 therapy may rely on the substitution of T cells with other immune cells, such as microglia, to exert its anti-tumor effects (214, 215). Thus, removal of microglia from gliomas becomes a possibility to improve the anti-PD-1 effect, and the use of a highly selective CSF-1R inhibitor (PLX5622) to remove microglia across the BBB was proposed by Clausi MG et al. (193). Simultaneous treatment with p38 MAPK inhibitors and PD-L1 antibodies effectively reduced PD-L1 levels in microglia residing within glioma microenvironments, leading to a significant decrease in tumor cell proliferation. This combination therapy significantly extended the survival of patients with temozolomide-resistant glioblastoma (194).
All viruses have a specific cellular orientation, and oncolytic viruses are anti-cancer viruses that have a specific orientation towards tumor cells and therefore do not cause damage to normal cells. Oncolytic viruses kill infected cancer cells through various pathways including direct or indirect virus-mediated cytotoxic immune effect mechanisms and regulation of apoptosis, necrosis, and autophagy of tumor cells. In addition, it can also kill uninfected cancer cells indirectly by directly destroying tumor blood vessels (216). Talimogene laherparepvec (T-VEC), a genetically modified herpes simplex virus (HSV), received FDA approval in 2015 as a therapeutic option for patients with metastatic melanoma (217). In addition to this, several other oncolytic viruses, including adenovirus, retroviruses, measles virus, and poliovirus have all demonstrated some antitumor activity in cancer patients (218). DNX-2401 (Delta-24-RGD/tasadenoturev) is an oncolytic adenovirus designed for targeting tumors that is selective for tumor cells and easy to manipulate and has shown significant effects in both murine models and a phase I clinical trial (219).The recombinant oncolytic poliovirus (PVSRIPO) targets the poliovirus receptor CD155, which is frequently overexpressed in tumors and also shows good results after intra-glioma injection (220). In addition, clinical trials investigating oncolytic viruses based on the HSV strain for glioma treatment are currently underway (NCT00028158, NCT03911388, NCT02062827) (221). The efficacy of OV may be influenced by increased microglia aggregation and infiltration in the tumor. This is because microglia can compete with glioma cells for viral uptake both through direct uptake of the virus by endocytosis and the antiviral response generated by the M1 polarization state. In addition, silencing of the OV gene can also be mediated by the STAT pathway, ultimately leading to a decrease in OV titers and inhibition of subsequent replication (222). A recent investigation has additionally verified that a rise in proliferation and infiltration of microglia around the tumor was indeed monitored during PVSRIPO treatment, and suggested that this antiviral inflammatory response may result from PD-L1 immune checkpoint activation on microglia. Therefore, Yang et al. proposed that the combination of PVSRIPO with PD-1/PD-L1 blockade could potentially extend the transient antitumor effect (196). The use of clodronate liposomes (CL) as well as cyclophosphamide depletes tumor microglia and inhibits the release of their antiviral factors to increase OV titers and gene expression, ultimately improving efficacy (197, 198). At the same time, several experiments targeting inhibition of microglia activity have shown a facilitative effect on OV therapy. For example, by using the immunosuppressive factor TGF-β to temporarily suppress tumor microglia and thus enhance the efficacy of OV (223).
Microglia can be invaded by glioma cells mediated by CSF-1R signaling on gliomas, a pathway involving CSF-1 signaling emitted by ERK(extracellular regulated protein kinases) (224). In a murine model, treatment with the CSF-1R inhibitor (PLX3397) resulted in decreased microglia activation and proliferation to prevent glioma cells from invading the brain parenchyma (80). However, PLX3397 was found to hinder the differentiation of monocytes into macrophages, rather than inhibiting monocyte influx into the tumor (28). In the clinical trial (NCT01349036), the drug exhibited effective BBB penetration and acceptable patient tolerability, the preliminary findings of the trial did not demonstrate significant antitumor effects of the inhibitor (225). A phase II clinical trial (NCT01790503) investigated the efficacy of combining PLX3397 with TMZ and radiotherapy, preliminary results of the trial also showed no significant clinical effect (226). Another CSF-1R inhibitor (BLZ945) was also found to impede tumor growth in a murine model. GM-CSF and IFN-γ, secreted by gliomas, sustained the viability of microglia in the presence of CSF-1R inhibition (195). Although CSF-1R inhibitors have shown prolonged overall survival in glioma models, they are also associated with a certain percentage of recurrences. At the same time, the poor effect shown during human clinical trials may be associated with acquired resistance, which may be related to the aforementioned increase in tumor-secreted GM-CSF and IFN-γ and the increased expression of immune checkpoint molecules, including PD-L1. Elevated activation of the phosphatidylinositol 3-kinase (PI3K) pathway, which is instigated by insulin-like growth factor-1 (IGF-1) and the insulin-like growth factor-1 receptor (IGF-1R) on tumor cells, is detectable in recurrent tumors (227, 228).
Natural flavonoids and synthetic derivatives have been previously shown to exhibit clear antitumor activity in malignant tumors such as breast cancer and melanoma (229). A 2017 study by Trevor A Stump et al. proposed that apigenin also has antitumor properties and the ability to promote apoptosis in glioblastoma (230). Flavonoids are generally classified into seven types, of which Apigenin, Rutin, and Quercetin represent flavonoids that can traverse the BBB effectively and impede the progression of glioma via modulation of distinct signaling pathways (231). Apigenin directly affects glioma cell migration by modulating IL-6 levels and also induces a reversal from the M2 polarized phenotype to the M1 phenotype of microglia and enrichment towards the tumor region. This effect is dose-dependent and remains non-toxic in an anti-tumor context (199). Rutin and Quercetin, which modulate the chemotaxis of glioma cells to microglia and induce microglia proliferation and restore their original immune response to inflammation, and modulate HDGF, IGF and GDNF levels to impede the growth and invasiveness of glioma cells (200). Wang M’s team extracted Rutin from purple potato and named purple sweet potato delphinidin-3-rutin (PSPD3R) which, additionally possessing the above-mentioned functions, was found to induce autophagy and apoptosis in glioma cells to achieve inhibition of glioma proliferation (201).
Tumor cells exhibit a heightened requirement for arginine, a semi-essential amino acid that is synthesized via the participation of argininosuccinate synthase (ASS) (232). Targeted arginine-depleting antitumor approaches become a potential possibility, and arginine-depleting agents (arginine deiminase ADI-PEG20) not only markedly suppressed tumor growth in a mouse model of glioma, but also acted synergistically with TMZ to enhance its effect in glioma (202). In addition, ADI-PEG20 augmented the radiosensitivity of glioma cells that are positive for argininosuccinate synthase 1 (ASS1) to ionizing radiation (IR) (i.e., sensitivity to radiotherapy) and also promoted further infiltration of microglia into the tumor, shifting their pro-tumor phenotype to an anti-tumor phenotype and exerting anti-tumor effects (203).
Zoledronate (ZOL)-loaded microglia membrane nanoparticles (ZOL@CNPs), which can target drug ZOL delivery to tumor regions by exploiting the recruitment mechanism of tumor cells to microglia, can inhibit tumor cell proliferation and invasion by increasing M1 phenotype microglia and blocking HIF-1α expression, ultimately achieving TMZ-resistant glioma progression inhibition (233). Polyamindoamine (PAMAM) can effectively cross the BBB and tumor barrier, and surface modification with sugar molecules will enable the dendrimer polymer to expand its microglial cell targeting promising as a targeting vehicle for antitumor drugs. In addition, AD, an amphiphilic PAMAM dendrimer, can complex with siRNA to form stable nanoscale particles, thereby preventing its degradation, reduce its target gene and protein expression after being taken up by microglia. Since this approach does not affect the other normal functions of microglia, it can be expected to be a harmless carrier for siRNA delivery to microglia (234, 235). miRNAs are key regulators of microglia polarization, with miRNA-155 having prominent significance for M1 polarization. Gao X’s team developed a membrane-encapsulated nucleic acid nanogel, Vir-Gel, embedded with a therapeutic miR-155-like virus, which can reprogram microglia from M2 phenotype to M1 phenotype to achieve tumor suppression (204). In addition, either intranasal delivery of a siRNA CD73-loaded cationic nanoemulsion or rAAV2-IL-15 (recombinant adeno-associated virus serotype rAAV2 carrying IL-15) modified microglia, or injection of a hydrogel containing the CXCL12 blocker Plerixafor (AMD3100) into the surgical cavity, can improve the immunosuppressed tumor microenvironment by blocking microglia recruitment and infiltration, thereby inhibiting tumor progression or recurrence (205, 206, 236). Chen C et al. reported an injectable nanocarrier-hydrogel superstructure into the postoperative cavity in which the nanocarrier introduces GSC-targeted CAR genes into the nucleus of microglia, thereby generating GSC-specific chimeric antigen receptor microglia/macrophages (CAR-MΦs) in the surgical cavity to remove residual GSCs and prevent postoperative glioma recurrence (207). Microglia can engage in cell-to-cell communication with numerous cell types in the brain, such as glioma cells, via tunneling nanotubes (TNTs) (237). Using the properties of tunneling nanotubes (TNTs), microglia can be engineered to enhance the targeted delivery of the anticancer drug paclitaxel (PTX). Loading PTX with liposomes (PTX-Lp) and engulfment by microglia circumvents the non-selective cytotoxicity of PTX and gives it the ability to efficiently transmigrate through the BBB (208). Guo L’s team proposed that iron oxide-nanoparticles and the near-infrared fluorescent dye DiD could be used to engineer microglia BV2 as an intraoperative optical imaging agent carrier (DiDBV2-Fe), which could be considered for intraoperative display of tumor boundaries and guidance of surgery given its efficient transmission through the BBB with no significant adverse effects. It may also be superior to commercially available 5-ALA, but there remains a potential clinical immune rejection issue (238).
As a serious primary brain tumor plaguing humans, glioma has a high morbidity and mortality rate, usually with a strong local invasive capacity and a poor overall prognosis for patients. Early investigators believed that this particular physiological structure of the BBB gave the brain its so-called immune privilege, and now, as related studies continue, the significance of TAMs in the TME is increasingly being recognized. An increasing body of ex vivo evidence is also corroborating that it is the tight interplay between TAMs and various cells in the TME that contributes to the distinctive immune-suppressive properties of gliomas. Microglia and BMDMs are the main cellular components that constitute TAMs, and their crosstalk with gliomas deserves further exploration. At this moment, it is challenging to differentiate between BMDMs and microglia in tumors either by cell morphology or by the surface specific antigen CD45, and then it is even more difficult to understand the specific functional roles of the two cell subpopulations. Therefore, in order to facilitate the study of the interactions between microglia and gliomas, we should clarify the heterogeneity of these two cell subpopulations. The heterogeneity between microglia and BMDMs is mainly reflected in the cytological origin, the different temporal and spatial distribution in the tumor, and the specific markers. Although a few microglia are replaced in gliomas with damaged BBB, immature yolk sac progenitor cells are now considered to be the primary microglia initiating cells, which differ from the bone marrow origin of BMDMs. In general, microglia tend to accumulate in the marginal regions of tumors, whereas BMDMs are primarily enriched within tumors, but this distribution changes with tumor progression and recurrence or even tumor type. Both the traditional differentiation method based on the macrophage surface antigen CD45 and the later microglia marker CX3CR1 are deficient because they are expressed in both cell subpopulations and therefore are not specific enough. The current study proposes that TMEM119 and P2RY12 can be considered as markers of microglia while CD49D and CD163 can be considered as markers of BMDMs.
The presence of microglia substantially augments the proliferative and migratory capabilities of glioma cells, suggesting that this may be due to the active mediators secreted by microglia. For example, CSF family, interleukin family, TGF-β, etc. At the same time, EVs sreleased by microglia and glioma cells, along with the activation of relevant surface receptors on both cell types, are pivotal in mediating the crosstalk process. Since the BBB is often disrupted in the context of malignant glioma and its permeability is increased, exogenous macrophages (BMDMs) tend to pass through the BBB to tumor-associated areas and gradually accumulate as a major infiltrating cell subpopulation. Concurrently, tumor-secreted chemokines recruit resident microglia in the brain, which undergo a transformation into a macrophage-like immune phenotype upon activation of multiple signaling pathways, including STAT3, NF-κB, mTOR, PI3K/Akt, and Wnt/β-catenin. This immune phenotype of microglia contributes to tumor progression by exerting an immunosuppressive effect.
Even with timely surgical resection of the lesion and standard postoperative treatment protocols, patients with glioma still face serious postoperative complications, highlighted by neurological impairment, which can significantly reduce the quality of survival. In particular, grade IV glioblastoma is not only prone to recurrence due to its high malignancy, but may also be resistant to conventional radiotherapy treatment. In addition, HGG such as glioblastoma is also less responsive to immunotherapy such as ICB, which has become popular in recent years. Existing evidence indicates that activated microglia in the tumor microenvironment can inhibit the activity, function, and quantity of tumor-infiltrating lymphocytes (TILs, like CD4+ or CD8+ T cells) with anti-tumor effects. This exacerbates the immune escape of glioma cells and their tolerance to common immunotherapies (such as Anti-PD-1 therapy), and may even eventually lead to patient relapse. However, current research is largely limited to the macroscopic mechanisms between common effector lymphocytes and microglia. The microscopic interaction between the two is not yet well understood, and no specific mechanisms of interaction have been clearly identified to provide new solutions for gliomas. In addition, the interaction between potential tumor-killing cells other than common T cells (B lymphocytes, natural killer cells, dendritic cells, polymorphonuclear neutrophils, γδ T cells, etc.) and microglia remains unclear and requires further research. In particular, it is worth exploring whether microglia will have similar inhibitory effects on these cells, thereby promoting tumor progression. Of course, combination of the relatively mature PD-1/PD-L1 immunotherapy with other treatments has shown promise in improving clinical outcomes. OV treatment regimens, engineered microglia and some potential agents (CSF-1R inhibitor, Flavonoids, Arginine-depleting agents) can also be considered as a new attempt to treat glioma. Based on such findings, then, it is reasonable to hypothesize that the combination of the aforementioned emerging treatments may produce more significant clinical results. However, this also requires more experiments in the future to verify this idea. Therefore, we can reasonably assume that the combination of multiple effective treatments should become a maturing trend in the future.
Whether it is the inability of most experimental drugs to reproduce their effectiveness when entering clinical trials or the lack of analysis of crosstalk studies between microglia and gliomas, We can find that this is due to the fact that there is still a lack of a reliable in vivo and ex vivo experimental model. Because murine models are still the main platform for research and experimentation, mouse and human microglia tend to have different altered gene expression profiles in the glioma setting and the source of cells is often limited to intraoperative collections (which is the period when microglia exert their strongest immunosuppressive effects), which means that the already complex immune microenvironment of the human brain cannot be perfectly reproduced (239, 240). Several teams have made new attempts, simple tissue engineered hydrogel platform models, peripheral blood-derived microglia-like (iMG) cells can serve as a predictor for the microglia in tumors and Microfluidic cell culture assays with full phase detection capability have contributed to the evolution of the experimental model (240–242).
Study conception and design: NL, J-CT, DY. Literature search and review: WS, D-RZ, YW, S-QH, KD. Manuscript writing and revision: NL, J-CT, DY. All authors contributed to the article and approved the submitted version. J-CT and DY contributed equally to this work and share first authorship.
This work was financially supported by the Anhui Province key research and development plan (2022e07020069), the Major natural science research project in universities of Anhui Province (KJ2021ZD0036), the Key project of natural science research in universities in Anhui Province (2022AH050769).
The authors declare that the research was conducted in the absence of any commercial or financial relationships that could be construed as a potential conflict of interest.
All claims expressed in this article are solely those of the authors and do not necessarily represent those of their affiliated organizations, or those of the publisher, the editors and the reviewers. Any product that may be evaluated in this article, or claim that may be made by its manufacturer, is not guaranteed or endorsed by the publisher.
1. Weller M, Wick W, Aldape K, Brada M, Berger M, Pfister SM, et al. Glioma. Nat Rev Dis Primers. (2015) 1:15017. doi: 10.1038/nrdp.2015.17
2. Horbinski C, Berger T, Packer RJ, Wen PY. Clinical implications of the 2021 edition of the WHO classification of central nervous system tumours. Nat Rev Neurol (2022) 18(9):515–29. doi: 10.1038/s41582-022-00679-w
3. Ohgaki H, Kleihues P. Population-based studies on incidence, survival rates, and genetic alterations in astrocytic and oligodendroglial gliomas. J Neuropathol Exp Neurol (2005) 64(6):479–89. doi: 10.1093/jnen/64.6.479
4. Bleeker FE, Molenaar RJ, Leenstra S. Recent advances in the molecular understanding of glioblastoma. J Neurooncol. (2012) 108(1):11–27. doi: 10.1007/s11060-011-0793-0
5. Molenaar RJ, Radivoyevitch T, Maciejewski JP, van Noorden CJ, Bleeker FE. The driver and passenger effects of isocitrate dehydrogenase 1 and 2 mutations in oncogenesis and survival prolongation. Biochim Biophys Acta (2014) 1846(2):326–41. doi: 10.1016/j.bbcan.2014.05.004
6. Molinaro AM, Taylor JW, Wiencke JK, Wrensch MR. Genetic and molecular epidemiology of adult diffuse glioma. Nat Rev Neurol (2019) 15(7):405–17. doi: 10.1038/s41582-019-0220-2
7. Bahadur S, Sahu AK, Baghel P, Saha S. Current promising treatment strategy for glioblastoma multiform: A review. Oncol Rev (2019) 3(2):417. doi: 10.4081/oncol.2019.417
8. Zetterling M, Elf K, Semnic R, Latini F, Engström ER. Time course of neurological deficits after surgery for primary brain tumours. Acta Neurochir (Wien). (2020) 162(12):3005–18. doi: 10.1007/s00701-020-04425-3
9. Engelhardt B, Vajkoczy P, Weller RO. The movers and shapers in immune privilege of the CNS. Nat Immunol (2017) 18(2):123–31. doi: 10.1038/ni.3666
10. Penfield W. Microglia and the process of phagocytosis in gliomas. Am J Pathol (1925) 1(1):77–90.15.
11. Bettinger I, Thanos S, Paulus W. Microglia promote glioma migration. Acta Neuropathol. (2002) 103(4):351–5. doi: 10.1007/s00401-001-0472-x
12. Graeber MB, Scheithauer BW, Kreutzberg GW. Microglia in brain tumors. Glia (2002) 40(2):252–9. doi: 10.1002/glia.10147
13. Glass R, Synowitz M. CNS macrophages and peripheral myeloid cells in brain tumours. Acta Neuropathol. (2014) 128(3):347–62. doi: 10.1007/s00401-014-1274-2
14. Hambardzumyan D, Gutmann DH, Kettenmann H. The role of microglia and macrophages in glioma maintenance and progression. Nat Neurosci (2016) 19(1):20–7. doi: 10.1038/nn.4185
15. Quail DF, Joyce JA. The microenvironmental landscape of brain tumors. Cancer Cell (2017) 31(3):326–41. doi: 10.1016/j.ccell.2017.02.009
16. Lin C, Wang N, Xu C. Glioma-associated microglia/macrophages (GAMs) in glioblastoma: Immune function in the tumor microenvironment and implications for immunotherapy. Front Immunol (2023) 14:1123853. doi: 10.3389/fimmu.2023.1123853
17. Pires-Afonso Y, Niclou SP, Michelucci A. Revealing and harnessing tumour-associated microglia/macrophage heterogeneity in glioblastoma. Int J Mol Sci (2020) 21(3):689. doi: 10.3390/ijms21030689
18. Prinz M, Jung S, Priller J. Microglia biology: one century of evolving concepts. Cell (2019) 179(2):292–311. doi: 10.1016/j.cell.2019.08.053
19. Priller J, Flügel A, Wehner T, Boentert M, Haas CA, Prinz M, et al. Targeting gene-modified hematopoietic cells to the central nervous system: use of green fluorescent protein uncovers microglial engraftment. Nat Med (2001) 7(12):1356–61. doi: 10.1038/nm1201-1356
20. Hickey WF, Kimura H. Perivascular microglial cells of the CNS are bone marrow-derived and present antigen in vivo. Science. (1988) 239(4837):290–2. doi: 10.1126/science.3276004
21. Mildner A, Schmidt H, Nitsche M, Merkler D, Hanisch UK, Mack M, et al. Microglia in the adult brain arise from Ly-6ChiCCR2+ monocytes only under defined host conditions. Nat Neurosci (2007) 10(12):1544–53. doi: 10.1038/nn2015
22. Yu K, Youshani AS, Wilkinson FL, O'Leary C, Cook P, Laaniste L, et al. A nonmyeloablative chimeric mouse model accurately defines microglia and macrophage contribution in glioma. Neuropathol Appl Neurobiol (2019) 45(2):119–40. doi: 10.1111/nan.12489
23. Ajami B, Bennett JL, Krieger C, McNagny KM, Rossi FM. Infiltrating monocytes trigger EAE progression, but do not contribute to the resident microglia pool. Nat Neurosci (2011) 14(9):1142–9. doi: 10.1038/nn.2887
24. Ginhoux F, Prinz M. Origin of microglia: current concepts and past controversies. Cold Spring Harb Perspect Biol (2015) 7(8):a020537. doi: 10.1101/cshperspect.a020537
25. Huang Y, Xu Z, Xiong S, Sun F, Qin G, Hu G, et al. Repopulated microglia are solely derived from the proliferation of residual microglia after acute depletion [published correction appears in Nat Neurosci. (2021)24(2):288]. Nat Neurosci (2018) 21(4):530–40. doi: 10.1038/s41593-018-0090-8
26. Badie B, Schartner JM. Flow cytometric characterization of tumor-associated macrophages in experimental gliomas. Neurosurgery (2000) 46(4):957–62. doi: 10.1097/00006123-200004000-00035
27. Banerjee K, Ratzabi A, Caspit IM, Ganon O, Blinder P, Jung S, et al. Distinct spatiotemporal features of microglia and monocyte-derived macrophages in glioma. Eur J Immunol (2023) 53(4):e2250161. doi: 10.1002/eji.202250161
28. Pombo Antunes AR, Scheyltjens I, Lodi F, Messiaen J, Antoranz A, Duerinck J, et al. Single-cell profiling of myeloid cells in glioblastoma across species and disease stage reveals macrophage competition and specialization. Nat Neurosci (2021) 24(4):595–610. doi: 10.1038/s41593-020-00789-y
29. Friebel E, Kapolou K, Unger S, Núñez NG, Utz S, Rushing EJ, et al. Single-cell mapping of human brain cancer reveals tumor-specific instruction of tissue-invading leukocytes. Cell (2020) 181(7):1626–1642.e20. doi: 10.1016/j.cell.2020.04.055
30. Klemm F, Maas RR, Bowman RL, Kornete M, Soukup K, Nassiri S, et al. Interrogation of the microenvironmental landscape in brain tumors reveals disease-specific alterations of immune cells. Cell (2020) 181(7):1643–1660.e17. doi: 10.1016/j.cell.2020.05.007
31. Müller A, Brandenburg S, Turkowski K, Müller S, Vajkoczy P. Resident microglia, and not peripheral macrophages, are the main source of brain tumor mononuclear cells. Int J Cancer. (2015) 137(2):278–88. doi: 10.1002/ijc.29379
32. Beutner C, Linnartz-Gerlach B, Schmidt SV, Beyer M, Mallmann MR, Staratschek-Jox A, et al. Unique transcriptome signature of mouse microglia. Glia (2013) 61(9):1429–42. doi: 10.1002/glia.22524
33. Lannes N, Eppler E, Etemad S, Yotovski P, Filgueira L. Microglia at center stage: a comprehensive review about the versatile and unique residential macrophages of the central nervous system. Oncotarget (2017) 8(69):114393–413. doi: 10.18632/oncotarget.23106
34. Masuda T, Sankowski R, Staszewski O, Böttcher C, Amann L, Sagar , et al. Spatial and temporal heterogeneity of mouse and human microglia at single-cell resolution [published correction appears in Nature. (2019) 568(7751):E4]. Nature (2019) 566(7744):388–92. doi: 10.1038/s41586-019-0924-x
35. Bennett ML, Bennett FC, Liddelow SA, Ajami B, Zamanian JL, Fernhoff NB, et al. New tools for studying microglia in the mouse and human CNS. Proc Natl Acad Sci U S A. (2016) 113(12):E1738–46. doi: 10.1073/pnas.1525528113
36. Buttgereit A, Lelios I, Yu X, Vrohlings M, Krakoski NR, Gautier EL, et al. Sall1 is a transcriptional regulator defining microglia identity and function. Nat Immunol (2016) 17(12):1397–406. doi: 10.1038/ni.3585
37. Gautier EL, Shay T, Miller J, Greter M, Jakubzick C, Ivanov S, et al. Gene-expression profiles and transcriptional regulatory pathways that underlie the identity and diversity of mouse tissue macrophages. Nat Immunol (2012) 13(11):1118–28. doi: 10.1038/ni.2419
38. Butovsky O, Jedrychowski MP, Moore CS, Cialic R, Lanser AJ, Gabriely G, et al. Identification of a unique TGF-β-dependent molecular and functional signature in microglia. Nat Neurosci (2014) 17(1):131–43. doi: 10.1038/nn.359
39. Bowman RL, Klemm F, Akkari L, Pyonteck SM, Sevenich L, Quail DF, et al. Macrophage ontogeny underlies differences in tumor-specific education in brain Malignancies. Cell Rep (2016) 17(9):2445–59. doi: 10.1016/j.celrep.2016.10.052
40. Lai AY, Dhami KS, Dibal CD, Todd KG. Neonatal rat microglia derived from different brain regions have distinct activation responses. Neuron Glia Biol (2011) 7(1):5–16. doi: 10.1017/S1740925X12000154
41. Hutter G, Theruvath J, Graef CM, Zhang M, Schoen MK, Manz EM, et al. Microglia are effector cells of CD47-SIRPα antiphagocytic axis disruption against glioblastoma. Proc Natl Acad Sci U S A. (2019) 116(3):997–1006. doi: 10.1073/pnas.1721434116
42. Feng X, Szulzewsky F, Yerevanian A, Chen Z, Heinzmann D, Rasmussen RD, et al. Loss of CX3CR1 increases accumulation of inflammatory monocytes and promotes gliomagenesis. Oncotarget (2015) 6(17):15077–94. doi: 10.18632/oncotarget.3730
43. Yona S, Kim KW, Wolf Y, Mildner A, Varol D, Breker M, et al. Fate mapping reveals origins and dynamics of monocytes and tissue macrophages under homeostasis [published correction appears in Immunity. Immunity (2013) 38(1):79–91. doi: 10.1016/j.immuni.2012.12.001
44. Xue J, Schmidt SV, Sander J, Draffehn A, Krebs W, Quester I, et al. Transcriptome-based network analysis reveals a spectrum model of human macrophage activation. Immunity (2014) 40(2):274–88. doi: 10.1016/j.immuni.2014.01.006
45. Marinelli S, Basilico B, Marrone MC, Ragozzino D. Microglia-neuron crosstalk: Signaling mechanism and control of synaptic transmission. Semin Cell Dev Biol (2019) 94:138–51. doi: 10.1016/j.semcdb.2019.05.017
46. Wes PD, Holtman IR, Boddeke EW, Möller T, Eggen BJ. Next generation transcriptomics and genomics elucidate biological complexity of microglia in health and disease. Glia (2016) 64(2):197–213. doi: 10.1002/glia.22866
47. Ransohoff RM. A polarizing question: do M1 and M2 microglia exist? Nat Neurosci (2016) 19(8):987–91. doi: 10.1038/nn.4338
48. Szulzewsky F, Pelz A, Feng X, Synowitz M, Markovic D, Langmann T, et al. Glioma-associated microglia/macrophages display an expression profile different from M1 and M2 polarization and highly express Gpnmb and Spp1. PloS One (2015) 10(2):e0116644. doi: 10.1371/journal.pone.0116644
49. Müller S, Kohanbash G, Liu SJ, Alvarado B, Carrera D, Bhaduri A, et al. Single-cell profiling of human gliomas reveals macrophage ontogeny as a basis for regional differences in macrophage activation in the tumor microenvironment. Genome Biol (2017) 18(1):234. doi: 10.1186/s13059-017-1362-4
50. Li Q, Barres BA. Microglia and macrophages in brain homeostasis and disease. Nat Rev Immunol (2018) 18(4):225–42. doi: 10.1038/nri.2017.125
51. Darmanis S, Sloan SA, Croote D, Mignardi M, Chernikova S, Samghababi P, et al. Single-cell RNA-seq analysis of infiltrating neoplastic cells at the migrating front of human glioblastoma. Cell Rep (2017) 21(5):1399–410. doi: 10.1016/j.celrep.2017.10.030
52. Parney IF, Waldron JS, Parsa AT. Flow cytometry and in vitro analysis of human glioma-associated macrophages. Laboratory investigation. J Neurosurg (2009) 110(3):572–82. doi: 10.3171/2008.7.JNS08475
53. Sankowski R, Böttcher C, Masuda T, Geirsdottir L, Sagar, Sindram E, et al. Mapping microglia states in the human brain through the integration of high-dimensional techniques [published correction appears in Nat Neurosci. (2022) 25(7):975]. Nat Neurosci (2019) 22(12):2098–110. doi: 10.1038/s41593-019-0532-y
54. Mantovani A, Sica A, Sozzani S, Allavena P, Vecchi A, Locati M. The chemokine system in diverse forms of macrophage activation and polarization. Trends Immunol (2004) 25(12):677–86. doi: 10.1016/j.it.2004.09.015
55. Maas SLN, Abels ER, Van De Haar LL, Zhang X, Morsett L, Sil S, et al. Glioblastoma hijacks microglial gene expression to support tumor growth. J Neuroinflammation. (2020) 17(1):120. doi: 10.1186/s12974-020-01797-2
56. Talasila KM, Røsland GV, Hagland HR, Eskilsson E, Flønes IH, Fritah S, et al. The angiogenic switch leads to a metabolic shift in human glioblastoma. Neuro Oncol (2017) 19(3):383–93. doi: 10.1093/neuonc/now175
57. Moreira TJ, Pierre K, Maekawa F, Repond C, Cebere A, Liljequist S, et al. Enhanced cerebral expression of MCT1 and MCT2 in a rat ischemia model occurs in activated microglial cells. J Cereb Blood Flow Metab (2009) 29(7):1273–83. doi: 10.1038/jcbfm.2009.50
58. Longhitano L, Vicario N, Forte S, Giallongo C, Broggi G, Caltabiano R, et al. Lactate modulates microglia polarization via IGFBP6 expression and remodels tumor microenvironment in glioblastoma. Cancer Immunol Immunother. (2023) 72(1):1–20. doi: 10.1007/s00262-022-03215-3
59. Pillai SR, Damaghi M, Marunaka Y, Spugnini EP, Fais S, Gillies RJ. Causes, consequences, and therapy of tumors acidosis. Cancer Metastasis Rev (2019) 38(1-2):205–22. doi: 10.1007/s10555-019-09792-7
60. Erbani J, Boon M, Akkari L. Therapy-induced shaping of the glioblastoma microenvironment: Macrophages at play. Semin Cancer Biol (2022) 86(Pt 3):41–56. doi: 10.1016/j.semcancer.2022.05.003
61. Saavedra-López E, Roig-Martínez M, Cribaro GP, Casanova PV, Gallego JM, Pérez-Vallés A, et al. Phagocytic glioblastoma-associated microglia and macrophages populate invading pseudopalisades. Brain Commun (2019) 2(1):fcz043. doi: 10.1093/braincomms/fcz043
62. Ghoochani A, Schwarz MA, Yakubov E, Engelhorn T, Doerfler A, Buchfelder M, et al. MIF-CD74 signaling impedes microglial M1 polarization and facilitates brain tumorigenesis. Oncogene (2016) 35(48):6246–61. doi: 10.1038/onc.2016.160
63. Amaral RF, Geraldo LHM, Einicker-Lamas M, E Spohr TCLS, Mendes F, Lima FRS. Microglial lysophosphatidic acid promotes glioblastoma proliferation and migration via LPA1 receptor. J Neurochem (2021) 156(4):499–512. doi: 10.1111/jnc.15097
64. Kouzarides T. Chromatin modifications and their function. Cell (2007) 128(4):693–705. doi: 10.1016/j.cell.2007.02.005
65. Maleszewska M, Steranka A, Smiech M, Kaza B, Pilanc P, Dabrowski M, et al. Sequential changes in histone modifications shape transcriptional responses underlying microglia polarization by glioma. Glia (2021) 69(1):109–23. doi: 10.1002/glia.23887
66. Hwang SY, Yoo BC, Jung JW, Oh ES, Hwang JS, Shin JA, et al. Induction of glioma apoptosis by microglia-secreted molecules: The role of nitric oxide and cathepsin B. Biochim Biophys Acta (2009) 1793(11):1656–68. doi: 10.1016/j.bbamcr.2009.08.011
67. Saidi D, Cheray M, Osman AM, Stratoulias V, Lindberg OR, Shen X, et al. Glioma-induced SIRT1-dependent activation of hMOF histone H4 lysine 16 acetyltransferase in microglia promotes a tumor supporting phenotype. Oncoimmunology (2017) 7(2):e1382790. doi: 10.1080/2162402X.2017.1382790
68. Bender S, Tang Y, Lindroth AM, Hovestadt V, Jones DT, Kool M, et al. Reduced H3K27me3 and DNA hypomethylation are major drivers of gene expression in K27M mutant pediatric high-grade gliomas. Cancer Cell (2013) 24(5):660–72. doi: 10.1016/j.ccr.2013.10.006
69. Yin Y, Qiu S, Li X, Huang B, Xu Y, Peng Y. EZH2 suppression in glioblastoma shifts microglia toward M1 phenotype in tumor microenvironment. J Neuroinflammation. (2017) 14(1):220. doi: 10.1186/s12974-017-0993-4
70. Keane L, Cheray M, Saidi D, Friess L, Gonzalez-Rodriguez P, et al. Inhibition of microglial EZH2 leads to anti-tumoral effects in pediatric diffuse midline gliomas. Neurooncol Adv (2021) 3(1):vdab096. doi: 10.1093/noajnl/vdab096
71. RodrÍguez E, Schetters STT, van Kooyk Y. The tumour glyco-code as a novel immune checkpoint for immunotherapy. Nat Rev Immunol (2018) 18(3):204–11. doi: 10.1038/nri.2018.3
72. Kai K, Komohara Y, Esumi S, Fujiwara Y, Yamamoto T, Uekawa K, et al. Macrophage/microglia-derived IL-1β induces glioblastoma growth via the STAT3/NF-κB pathway. Hum Cell (2022) 35(1):226–37. doi: 10.1007/s13577-021-00619-8
73. Arseni L, Sharma R, Mack N, et al. Sphingosine-1-phosphate recruits macrophages and microglia and induces a pro-tumorigenic phenotype that favors glioma progression. Cancers (Basel). (2023) 15(2):479. doi: 10.3390/cancers15020479
74. Li J, Kaneda MM, Ma J, Shepard RM, Patel K, Koga T, et al. PI3Kγ inhibition suppresses microglia/TAM accumulation in glioblastoma microenvironment to promote exceptional temozolomide response. Proc Natl Acad Sci U S A. (2021) 118(16):e2009290118. doi: 10.1073/pnas.2009290118
75. Couto M, Coelho-Santos V, Santos L, Fontes-Ribeiro C, Silva AP, Gomes CMF. The interplay between glioblastoma and microglia cells leads to endothelial cell monolayer dysfunction via the interleukin-6-induced JAK2/STAT3 pathway. J Cell Physiol (2019) 234(11):19750–60. doi: 10.1002/jcp.28575
76. Chiavari M, Ciotti GMP, Canonico F, Altieri F, Lacal PM, Graziani G, et al. PDIA3 expression in glioblastoma modulates macrophage/microglia pro-tumor activation. Int J Mol Sci (2020) 21(21):8214. doi: 10.3390/ijms21218214
77. Zhang Y, Wang J, Ghobadi SN, Zhou H, Huang A, Gerosa M, et al. Molecular identity changes of tumor-associated macrophages and microglia after magnetic resonance imaging-guided focused ultrasound-induced blood-brain barrier opening in a mouse glioblastoma model. Ultrasound Med Biol (2023) 49(5):1082–90. doi: 10.1016/j.ultrasmedbio.2022.12.006
78. Lisi L, Ciotti GMP, Chiavari M, Pizzoferrato M, Mangiola A, Kalinin S, et al. Phospho-mTOR expression in human glioblastoma microglia-macrophage cells. Neurochem Int (2019) 129:104485. doi: 10.1016/j.neuint.2019.104485
79. Dumas AA, Pomella N, Rosser G, Guglielmi L, Vinel C, Millner TO, et al. Microglia promote glioblastoma via mTOR-mediated immunosuppression of the tumour microenvironment. EMBO J (2020) 39(15):e103790. doi: 10.15252/embj.2019103790
80. Coniglio SJ, Eugenin E, Dobrenis K, Stanley ER, West BL, Symons MH, et al. Microglial stimulation of glioblastoma invasion involves epidermal growth factor receptor (EGFR) and colony stimulating factor 1 receptor (CSF-1R) signaling. Mol Med (2012) 18(1):519–27. doi: 10.2119/molmed.2011.00217
81. Li X, Wu C, Chen N, Gu H, Yen A, Cao L, et al. PI3K/Akt/mTOR signaling pathway and targeted therapy for glioblastoma. Oncotarget (2016) 7(22):33440–50. doi: 10.18632/oncotarget.7961
82. Tang F, Yang C, Li FP, Yu DH, Pan ZY, Wang ZF, et al. Palmitoyl transferases act as potential regulators of tumor-infiltrating immune cells and glioma progression. Mol Ther Nucleic Acids (2022) 28:716–31. doi: 10.1016/j.omtn.2022.04.030
83. Geraldo LH, Xu Y, Jacob L, Pibouin-Fragner L, Rao R, Maissa N, et al. SLIT2/ROBO signaling in tumor-associated microglia and macrophages drives glioblastoma immunosuppression and vascular dysmorphia. J Clin Invest. (2021) 31(16):e141083. doi: 10.1172/JCI141083
84. Blockus H, Chédotal A. Slit-robo signaling. Development (2016) 143(17):3037–44. doi: 10.1242/dev.132829
85. Moon RT, Kohn AD, De Ferrari GV, Kaykas A. WNT and beta-catenin signalling: diseases and therapies. Nat Rev Genet (2004) 5(9):691–701. doi: 10.1038/nrg1427
86. Tao W, Chu C, Zhou W, Huang Z, Zhai K, Fang X, et al. Dual Role of WISP1 in maintaining glioma stem cells and tumor-supportive macrophages in glioblastoma. Nat Commun (2020) 11(1):3015. doi: 10.1038/s41467-020-16827-z
87. Matias D, Dubois LG, Pontes B, Rosário L, Ferrer VP, Balça-Silva J, et al. GBM-derived wnt3a induces M2-like phenotype in microglial cells through Wnt/β-catenin signaling. Mol Neurobiol (2019) 56(2):1517–30. doi: 10.1007/s12035-018-1150-5
88. Li J, Sun Y, Sun X, Zhao X, Ma Y, Wang Y, et al. AEG-1 silencing attenuates M2-polarization of glioma-associated microglia/macrophages and sensitizes glioma cells to temozolomide. Sci Rep (2021) 11(1):17348. doi: 10.1038/s41598-021-96647-3
89. Fan D, Yue Q, Chen J, Wang C, Yu R, Jin Z, et al. Reprogramming the immunosuppressive microenvironment of IDH1 wild-type glioblastoma by blocking Wnt signaling between microglia and cancer cells. Oncoimmunology (2021) 10(1):1932061. doi: 10.1080/2162402X.2021.1932061
90. Chen X, Zhang L, Zhang IY, Liang J, Wang H, Ouyang M, et al. RAGE expression in tumor-associated macrophages promotes angiogenesis in glioma. Cancer Res (2014) 74(24):7285–97. doi: 10.1158/0008-5472.CAN-14-1240
91. Zhu C, Mustafa D, Zheng PP, van der Weiden M, Sacchetti A, Brandt M, et al. Activation of CECR1 in M2-like TAMs promotes paracrine stimulation-mediated glial tumor progression. Neuro Oncol (2017) 19(5):648–59. doi: 10.1093/neuonc/now251
92. Hu F, Huang Y, Semtner M, Zhao K, Tan Z, Dzaye O, et al. Down-regulation of Aquaporin-1 mediates a microglial phenotype switch affecting glioma growth. Exp Cell Res (2020) 396(2):112323. doi: 10.1016/j.yexcr.2020.112323
93. Nuñez RE, Del Valle MM, Ortiz K, Almodovar L, Kucheryavykh L. Microglial cytokines induce invasiveness and proliferation of human glioblastoma through pyk2 and FAK activation. Cancers (Basel). (2021) 13(24):6160. doi: 10.3390/cancers13246160
94. Parmigiani E, Ivanek R, Rolando C, Hafen K, Turchinovich G, Lehmann FM, et al. Interferon-γ resistance and immune evasion in glioma develop via Notch-regulated co-evolution of Malignant and immune cells. Dev Cell (2022) 57(15):1847–1865.e9. doi: 10.1016/j.devcel.2022.06.006
95. Takacs GP, Flores-Toro JA, Harrison JK. Modulation of the chemokine/chemokine receptor axis as a novel approach for glioma therapy. Pharmacol Ther (2021) 222:107790. doi: 10.1016/j.pharmthera.2020.107790
96. Zeren N, Afzal Z, Morgan S, Marshall G, Uppiliappan M, Merritt J, et al. The chemokine receptor CCR1 mediates microglia stimulated glioma invasion. Int J Mol Sci (2023) 24(6):5136. doi: 10.3390/ijms24065136
97. Bungert AD, Urbantat RM, Jelgersma C, Bekele BM, Mueller S, Mueller A, et al. Myeloid cell subpopulations compensate each other for Ccr2-deficiency in glioblastoma. Neuropathol Appl Neurobiol (2023) 49(1):e12863. doi: 10.1111/nan.12863
98. Mizutani M, Pino PA, Saederup N, Charo IF, Ransohoff RM, Cardona AE. The fractalkine receptor but not CCR2 is present on microglia from embryonic development throughout adulthood. J Immunol (2012) 188(1):29–36. doi: 10.4049/jimmunol.1100421
99. Platten M, Kretz A, Naumann U, Aulwurm S, Egashira K, Isenmann S, et al. Monocyte chemoattractant protein-1 increases microglial infiltration and aggressiveness of gliomas. Ann Neurol (2003) 54(3):388–92. doi: 10.1002/ana.10679
100. Saederup N, Cardona AE, Croft K, Mizutani M, Cotleur AC, Tsou CL, et al. Correction: selective chemokine receptor usage by central nervous system myeloid cells in CCR2-red fluorescent protein knock-in mice. PloS One (2017) 12(4):e0176931. doi: 10.1371/journal.pone.0176931
101. Caponegro MD, Oh K, Madeira MM, Radin D, Sterge N, Tayyab M, et al. A distinct microglial subset at the tumor-stroma interface of glioma. Glia (2021) 69(7):1767–81. doi: 10.1002/glia.23991
102. Flores-Toro JA, Luo D, Gopinath A, Sarkisian MR, Campbell JJ, Charo IF, et al. CCR2 inhibition reduces tumor myeloid cells and unmasks a checkpoint inhibitor effect to slow progression of resistant murine gliomas. Proc Natl Acad Sci U S A. (2020) 117(2):1129–38. doi: 10.1073/pnas.1910856117
103. Yu-Ju Wu C, Chen CH, Lin CY, Feng LY, Lin YC, Wei KC, et al. CCL5 of glioma-associated microglia/macrophages regulates glioma migration and invasion via calcium-dependent matrix metalloproteinase 2. Neuro Oncol (2020) 22(2):253–66. doi: 10.1093/neuonc/noz189
104. Venkataramani V, Yang Y, Schubert MC, Reyhan E, Tetzlaff SK, Wißmann N, et al. Glioblastoma hijacks neuronal mechanisms for brain invasion. Cell (2022) 185(16):2899–2917.e31. doi: 10.1016/j.cell.2022.06.054
105. Tomaszewski WH, Waibl-Polania J, Chakraborty M, Perera J, Ratiu J, Miggelbrink A, et al. Neuronal CaMKK2 promotes immunosuppression and checkpoint blockade resistance in glioblastoma. Nat Commun (2022) 13(1):6483. doi: 10.1038/s41467-022-34175-y
106. Laudati E, Currò D, Navarra P, Lisi L. Blockade of CCR5 receptor prevents M2 microglia phenotype in a microglia-glioma paradigm. Neurochem Int (2017) 108:100–8. doi: 10.1016/j.neuint.2017.03.002
107. Meng F, Li W, Li C, Gao Z, Guo K, Song S. CCL18 promotes epithelial-mesenchymal transition, invasion and migration of pancreatic cancer cells in pancreatic ductal adenocarcinoma. Int J Oncol (2015) 46(3):1109–20. doi: 10.3892/ijo.2014.2794
108. Huang Y, Motta E, Nanvuma C, Kuhrt LD, Yuan Y, Xia P, et al. Microglia/macrophage-derived human CCL18 promotes glioma progression via CCR8-ACP5 axis analyzed in humanized slice model. Cell Rep (2022) 39(2):110670. doi: 10.1016/j.celrep.2022.110670
109. Zhang Q, Wang J, Yao X, Wu S, Tian W, Gan C, et al. Programmed cell death 10 mediated CXCL2-CXCR2 signaling in regulating tumor-associated microglia/macrophages recruitment in glioblastoma. Front Immunol (2021) 12:637053. doi: 10.3389/fimmu.2021.637053
110. Warrington NM, Woerner BM, Daginakatte GC, Dasgupta B, Perry A, Gutmann DH, et al. Spatiotemporal differences in CXCL12 expression and cyclic AMP underlie the unique pattern of optic glioma growth in neurofibromatosis type 1. Cancer Res (2007) 67(18):8588–95. doi: 10.1158/0008-5472.CAN-06-2220
111. Wang SC, Hong JH, Hsueh C, Chiang CS. Tumor-secreted SDF-1 promotes glioma invasiveness and TAM tropism toward hypoxia in a murine astrocytoma model. Lab Invest. (2012) 92(1):151–62. doi: 10.1038/labinvest.2011.128
112. Chatterjee S, Behnam Azad B, Nimmagadda S. The intricate role of CXCR4 in cancer. Adv Cancer Res (2014) 124:31–82. doi: 10.1016/B978-0-12-411638-2.00002-1
113. Kumar A, Mohamed E, Tong S, Chen K, Mukherjee J, Lim Y, et al. CXCL14 promotes a robust brain tumor-associated immune response in glioma. Clin Cancer Res (2022) 28(13):2898–910. doi: 10.1158/1078-0432.CCR-21-2830
114. Lepore F, D’Alessandro G, Antonangeli F, Santoro A, Esposito V, Limatola C, et al. CXCL16/CXCR6 axis drives microglia/macrophages phenotype in physiological conditions and plays a crucial role in glioma. Front Immunol (2018) 9:2750. doi: 10.3389/fimmu.2018.02750
115. Lee S, Latha K, Manyam G, Yang Y, Rao A, Rao G. Role of CX3CR1 signaling in Malignant transformation of gliomas. Neuro Oncol (2020) 22(10):1463–73. doi: 10.1093/neuonc/noaa075
116. Pong WW, Higer SB, Gianino SM, Emnett RJ, Gutmann DH. Reduced microglial CX3CR1 expression delays neurofibromatosis-1 glioma formation. Ann Neurol (2013) 73(2):303–8. doi: 10.1002/ana.23813
117. Chen P, Hsu WH, Chang A, et al. Circadian regulator CLOCK recruits immune-suppressive microglia into the GBM tumor microenvironment. Cancer Discovery (2020) 10(3):371–81. doi: 10.1158/2159-8290.CD-19-0400
118. Joseph LM, Toedebusch RG, Debebe E, Tan Z, Lan Z, Zhou A, et al. Microglia-derived olfactomedin-like 3 is a potent angiogenic factor in primary mouse brain endothelial cells: A novel target for glioblastoma. Int J Mol Sci (2022) 23(23):14613. doi: 10.3390/ijms232314613
119. Wallmann T, Zhang XM, Wallerius M, Bolin S, Joly AL, Sobocki C, et al. Microglia induce PDGFRB expression in glioma cells to enhance their migratory capacity. iScience (2018) 9:71–83. doi: 10.1016/j.isci.2018.10.011
120. Smith GT, Radin DP, Tsirka SE. From protein-protein interactions to immune modulation: Therapeutic prospects of targeting Neuropilin-1 in high-grade glioma. Front Immunol (2022) 13:958620. doi: 10.3389/fimmu.2022.958620
121. Miyauchi JT, Caponegro MD, Chen D, Choi MK, Li M, Tsirka SE. Deletion of neuropilin 1 from microglia or bone marrow-derived macrophages slows glioma progression. Cancer Res (2018) 78(3):685–94. doi: 10.1158/0008-5472.CAN-17-1435
122. Sørensen MD, Dahlrot RH, Boldt HB, Hansen S, Kristensen BW. Tumour-associated microglia/macrophages predict poor prognosis in high-grade gliomas and correlate with an aggressive tumour subtype. Neuropathol Appl Neurobiol (2018) 44(2):185–206. doi: 10.1111/nan.12428
123. Sørensen MD, Kristensen BW. Tumour-associated CD204+ microglia/macrophages accumulate in perivascular and perinecrotic niches and correlate with an interleukin-6-enriched inflammatory profile in glioblastoma. Neuropathol Appl Neurobiol (2022) 48(2):e12772. doi: 10.1111/nan.12772
124. Sánchez C, de Ceballos ML, Gomez del Pulgar T, Rueda D, Corbacho C, Velasco G, et al. Inhibition of glioma growth in vivo by selective activation of the CB(2) cannabinoid receptor. Cancer Res (2001) 61(15):5784–9.
125. Lei X, Chen X, Quan Y, Tao Y, Li J. Targeting CYP2J2 to enhance the anti-glioma efficacy of cannabinoid receptor 2 stimulation by inhibiting the pro-angiogenesis function of M2 microglia. Front Oncol (2020) 10:574277. doi: 10.3389/fonc.2020.574277
126. Jiang JG, Ning YG, Chen C, Ma D, Liu ZJ, Yang S, et al. Cytochrome p450 epoxygenase promotes human cancer metastasis. Cancer Res (2007) 67(14):6665–74. doi: 10.1158/0008-5472.CAN-06-3643
127. Kan LK, Williams D, Drummond K, O’Brien T, Monif M. The role of microglia and P2X7 receptors in gliomas. J Neuroimmunol. (2019) 332:138–46. doi: 10.1016/j.jneuroim.2019.04.010
128. Kan LK, Seneviratne S, Drummond KJ, Williams DA, O’Brien TJ, Monif M. P2X7 receptor antagonism inhibits tumour growth in human high-grade gliomas. Purinergic Signal (2020) 16(3):327–36. doi: 10.1007/s11302-020-09705-2
129. Chia K, Keatinge M, Mazzolini J, Sieger D. Brain tumours repurpose endogenous neuron to microglia signalling mechanisms to promote their own proliferation. Elife (2019) 8:e46912. doi: 10.7554/eLife.46912
130. Lou N, Takano T, Pei Y, Xavier AL, Goldman SA, Nedergaard M. Purinergic receptor P2RY12-dependent microglial closure of the injured blood-brain barrier. Proc Natl Acad Sci U S A. (2016) 113(4):1074–9. doi: 10.1073/pnas.1520398113
131. Wypych D, Pomorski P. Calcium signaling in glioma cells: the role of nucleotide receptors. Adv Exp Med Biol (2020) 1202:67–86. doi: 10.1007/978-3-030-30651-9_4
132. Milior G, Morin-Brureau M, Chali F, Le Duigou C, Savary E, Huberfeld G, et al. Distinct P2Y receptors mediate extension and retraction of microglial processes in epileptic and peritumoral human tissue. J Neurosci (2020) 40(7):1373–88. doi: 10.1523/JNEUROSCI.0218-19.2019
133. Curet MA, Watters JJ. P2Y14 receptor activation decreases interleukin-6 production and glioma GL261 cell proliferation in microglial transwell cultures. J Neurooncol. (2018) 137(1):23–31. doi: 10.1007/s11060-017-2700-9
134. Wolf SA, Boddeke HW, Kettenmann H. Microglia in physiology and disease. Annu Rev Physiol (2017) 79:619–43. doi: 10.1146/annurev-physiol-022516-034406
135. Hu F, Ku MC, Markovic D, Dzaye O, Lehnardt S, Synowitz M, et al. Glioma-associated microglial MMP9 expression is upregulated by TLR2 signaling and sensitive to minocycline. Int J Cancer. (2014) 135(11):2569–78. doi: 10.1002/ijc.28908
136. Markovic DS, Vinnakota K, Chirasani S, Synowitz M, Raguet H, Stock K, et al. Gliomas induce and exploit microglial MT1-MMP expression for tumor expansion. Proc Natl Acad Sci U S A. (2009) 106(30):12530–5. doi: 10.1073/pnas.0804273106
137. Vinnakota K, Hu F, Ku MC, Kiwit J, Synowitz M, Glass R, et al. Toll-like receptor 2 mediates microglia/brain macrophage MT1-MMP expression and glioma expansion. Neuro Oncol (2013) 15(11):1457–68. doi: 10.1093/neuonc/not115
138. Markovic DS, Vinnakota K, van Rooijen N, Matyash V, Pohlmann A, Waiczies S, et al. Minocycline reduces glioma expansion and invasion by attenuating microglial MT1-MMP expression. Brain Behav Immun (2011) 25(4):624–8. doi: 10.1016/j.bbi.2011.01.015
139. Gutmann DH, Kettenmann H. Microglia/brain macrophages as central drivers of brain tumor pathobiology. Neuron (2019) 104(3):442–9. doi: 10.1016/j.neuron.2019.08.028
140. Ku MC, Wolf SA, Respondek D, Matyash V, Pohlmann A, Waiczies S, et al. GDNF mediates glioblastoma-induced microglia attraction but not astrogliosis. Acta Neuropathol. (2013) 125(4):609–20. doi: 10.1007/s00401-013-1079-8
141. Huang Y, Zhang B, Haneke H, Haage V, Lubas M, Yuan Y, et al. Glial cell line-derived neurotrophic factor increases matrix metallopeptidase 9 and 14 expression in microglia and promotes microglia-mediated glioma progression. J Neurosci Res (2021) 99(4):1048–63. doi: 10.1002/jnr.24768
142. Qian J, Luo F, Yang J, Liu J, Liu R, Wang L, et al. TLR2 promotes glioma immune evasion by downregulating MHC class II molecules in microglia. Cancer Immunol Res (2018) 6(10):1220–33. doi: 10.1158/2326-6066.CIR-18-0020
143. Huang Y, Zhang Q, Lubas M, Yuan Y, Yalcin F, Efe IE, et al. Synergistic toll-like receptor 3/9 signaling affects properties and impairs glioma-promoting activity of microglia. J Neurosci (2020) 40(33):6428–43. doi: 10.1523/JNEUROSCI.0666-20.2020
144. Mantovani A, Sozzani S, Locati M, Allavena P, Sica A. Macrophage polarization: tumor-associated macrophages as a paradigm for polarized M2 mononuclear phagocytes. Trends Immunol (2002) 23(11):549–55. doi: 10.1016/s1471-4906(02)02302-5
145. Gilles ME, Slack FJ. Let-7 microRNA as a potential therapeutic target with implications for immunotherapy. Expert Opin Ther Targets. (2018) 22(11):929–39. doi: 10.1080/14728222.2018.1535594
146. Buonfiglioli A, Efe IE, Guneykaya D, Ivanov A, Huang Y, Orlowski E, et al. let-7 microRNAs regulate microglial function and suppress glioma growth through toll-like receptor 7. Cell Rep (2019) 29(11):3460–3471.e7. doi: 10.1016/j.celrep.2019.11.029
147. Fuku M, Hinkelmann L, Kuhrt LD, Efe IE, Kumbol V, Buonfiglioli A, et al. Activation of Toll-like receptor 5 in microglia modulates their function and triggers neuronal injury. Acta Neuropathol Commun (2020) 8(1):159. doi: 10.1186/s40478-020-01031-3
148. Nijaguna MB, Patil V, Urbach S, Shwetha SD, Sravani K, Hegde AS, et al. Glioblastoma-derived macrophage colony-stimulating factor (MCSF) induces microglial release of insulin-like growth factor-binding protein 1 (IGFBP1) to promote angiogenesis. J Biol Chem (2015) 290(38):23401–15. doi: 10.1074/jbc.M115.664037
149. Sielska M, Przanowski P, Pasierbińska M, Wojnicki K, Poleszak K, Wojtas B, et al. Tumour-derived CSF2/granulocyte macrophage colony stimulating factor controls myeloid cell accumulation and progression of gliomas. Br J Cancer. (2020) 123(3):438–48. doi: 10.1038/s41416-020-0862-2
150. Zaitsev S, Sharma HS, Sharma A, Manzhulo I, Polevshchikov A, Kudriavtsev I, et al. Pro-inflammatory modification of cancer cells microsurroundings increases the survival rates for rats with low differentiated Malignant glioma of brain. Int Rev Neurobiol (2020) 151:253–79. doi: 10.1016/bs.irn.2020.03.027
151. Shen JX, Liu J, Zhang GJ. Interleukin-33 in Malignancies: friends or foes? Front Immunol (2018) 9:3051. doi: 10.3389/fimmu.2018.03051
152. De Boeck A, Ahn BY, D’Mello C, Lun X, Menon SV, Alshehri MM, et al. Glioma-derived IL-33 orchestrates an inflammatory brain tumor microenvironment that accelerates glioma progression. Nat Commun (2020) 11(1):4997. doi: 10.1038/s41467-020-18569-4
153. Wagner S, Czub S, Greif M, Vince GH, Süss N, Kerkau S, et al. Microglial/macrophage expression of interleukin 10 in human glioblastomas. Int J Cancer. (1999) 82(1):12–6. doi: 10.1002/(sici)1097-0215(19990702)82:1<12::aid-ijc3>3.0.co;2-o
154. Bloch O, Crane CA, Kaur R, Safaee M, Rutkowski MJ, Parsa AT. Gliomas promote immunosuppression through induction of B7-H1 expression in tumor-associated macrophages. Clin Cancer Res (2013) 19(12):3165–75. doi: 10.1158/1078-0432.CCR-12-3314
155. Wu A, Wei J, Kong LY, Wang Y, Priebe W, Qiao W, et al. Glioma cancer stem cells induce immunosuppressive macrophages/microglia. Neuro Oncol (2010) 12(11):1113–25. doi: 10.1093/neuonc/noq082
156. Seliger C, Meyer AL, Renner K, Leidgens V, Moeckel S, Jachnik B, et al. Metformin inhibits proliferation and migration of glioblastoma cells independently of TGF-β2. Cell Cycle (2016) 15(13):1755–66. doi: 10.1080/15384101.2016.1186316
157. Liu H, Sun Y, Zhang Q, Jin W, Gordon RE, Zhang Y, et al. Pro-inflammatory and proliferative microglia drive progression of glioblastoma. Cell Rep (2021) 36(11):109718. doi: 10.1016/j.celrep.2021.109718
158. Ye XZ, Xu SL, Xin YH, Yu SC, Ping YF, Chen L, et al. Tumor-associated microglia/macrophages enhance the invasion of glioma stem-like cells via TGF-β1 signaling pathway. J Immunol (2012) 189(1):444–53. doi: 10.4049/jimmunol.1103248
159. Wesolowska A, Kwiatkowska A, Slomnicki L, Dembinski M, Master A, Sliwa M, et al. Microglia-derived TGF-beta as an important regulator of glioblastoma invasion–an inhibition of TGF-beta-dependent effects by shRNA against human TGF-beta type II receptor. Oncogene (2008) 27(7):918–30. doi: 10.1038/sj.onc.1210683
160. Luo W, Quan Q, Jiang J, Peng R. An immune and epithelial-mesenchymal transition-related risk model and immunotherapy strategy for grade II and III gliomas. Front Genet (2023) 13:1070630. doi: 10.3389/fgene.2022.1070630
161. Jaiswal S, Jamieson CH, Pang WW, Park CY, Chao MP, Majeti R, et al. CD47 is upregulated on circulating hematopoietic stem cells and leukemia cells to avoid phagocytosis. Cell (2009) 138(2):271–85. doi: 10.1016/j.cell.2009.05.046
162. Gholamin S, Mitra SS, Feroze AH, Liu J, Kahn SA, Zhang M, et al. Disrupting the CD47-SIRPα anti-phagocytic axis by a humanized anti-CD47 antibody is an efficacious treatment for Malignant pediatric brain tumors. Sci Transl Med (2017) 9(381):eaaf2968. doi: 10.1126/scitranslmed.aaf2968
163. Lehrman EK, Wilton DK, Litvina EY, Welsh CA, Chang ST, Frouin A, et al. CD47 protects synapses from excess microglia-mediated pruning during development. Neuron (2018) 100(1):120–134.e6. doi: 10.1016/j.neuron.2018.09.017
164. Pietras A, Katz AM, Ekström EJ, Wee B, Halliday JJ, Pitter KL, et al. Osteopontin-CD44 signaling in the glioma perivascular niche enhances cancer stem cell phenotypes and promotes aggressive tumor growth. Cell Stem Cell (2014) 14(3):357–69. doi: 10.1016/j.stem.2014.01.005
165. Ivanova EL, Costa B, Eisemann T, Lohr S, Boskovic P, Eichwald V, et al. CD44 expressed by myeloid cells promotes glioma invasion. Front Oncol (2022) 12:969787. doi: 10.3389/fonc.2022.969787
166. Wei J, Marisetty A, Schrand B, Gabrusiewicz K, Hashimoto Y, Ott M, et al. Osteopontin mediates glioblastoma-associated macrophage infiltration and is a potential therapeutic target. J Clin Invest. (2019) 129(1):137–49. doi: 10.1172/JCI121266
167. Yi L, Xiao H, Xu M, Ye X, Hu J, Li F, et al. Glioma-initiating cells: a predominant role in microglia/macrophages tropism to glioma. J Neuroimmunol. (2011) 232(1-2):75–82. doi: 10.1016/j.jneuroim.2010.10.011
168. Badie B, Schartner J, Prabakaran S, Paul J, Vorpahl J. Expression of Fas ligand by microglia: possible role in glioma immune evasion. J Neuroimmunol. (2001) 120(1-2):19–24. doi: 10.1016/s0165-5728(01)00361-7
169. Xie Y, Li Q, Yang Q, Yang M, Zhang Z, Zhu L, et al. Overexpression of DCF1 inhibits glioma through destruction of mitochondria and activation of apoptosis pathway. Sci Rep (2014) 4:3702. doi: 10.1038/srep03702
170. Wang J, Li J, Wang Q, Kong Y, Zhou F, Li Q, et al. Dcf1 deficiency attenuates the role of activated microglia during neuroinflammation. Front Mol Neurosci (2018) 11:256. doi: 10.3389/fnmol.2018.00256
171. Mazzolini J, Le Clerc S, Morisse G, Coulonges C, Zagury JF, Sieger D. Wasl is crucial to maintain microglial core activities during glioblastoma initiation stages. Glia (2022) 70(6):1027–51. doi: 10.1002/glia.24154
172. Yeini E, Ofek P, Pozzi S, Albeck N, Ben-Shushan D, Tiram G, et al. P-selectin axis plays a key role in microglia immunophenotype and glioblastoma progression. Nat Commun (2021) 12(1):1912. doi: 10.1038/s41467-021-22186-0
173. Carvalho da Fonseca AC, Wang H, Fan H, Chen X, Zhang I, Zhang L, et al. Increased expression of stress inducible protein 1 in glioma-associated microglia/macrophages. J Neuroimmunol. (2014) 274(1-2):71–7. doi: 10.1016/j.jneuroim.2014.06.021
174. Balakrishnan A, Roy S, Fleming T, Leong HS, Schuurmans C. The emerging role of extracellular vesicles in the glioma microenvironment: biogenesis and clinical relevance. Cancers (Basel). (2020) 12(7):1964. doi: 10.3390/cancers12071964
175. Catalano M, Serpe C, Limatola C. Microglial extracellular vesicles as modulators of brain microenvironment in glioma. Int J Mol Sci (2022) 23(21):13165. doi: 10.3390/ijms232113165
176. Osti D, Del Bene M, Rappa G, Santos M, Matafora V, Richichi C, et al. Clinical significance of extracellular vesicles in plasma from glioblastoma patients. Clin Cancer Res (2019) 25(1):266–76. doi: 10.1158/1078-0432.CCR-18-1941
177. Chen QY, Gao B, Tong D, Huang C. Crosstalk between extracellular vesicles and tumor-associated macrophage in the tumor microenvironment. Cancer Lett (2023) 552:215979. doi: 10.1016/j.canlet.2022.215979
178. Spellicy SE, Stice SL. Tissue and stem cell sourced extracellular vesicle communications with microglia. Stem Cell Rev Rep (2021) 17(2):357–68. doi: 10.1007/s12015-020-10011-y
179. Wang L, Yang C, Wang Q, Liu Q, Wang Y, Zhou J, et al. Homotrimer cavin1 interacts with caveolin1 to facilitate tumor growth and activate microglia through extracellular vesicles in glioma. Theranostics (2020) 10(15):6674–94. doi: 10.7150/thno.45688
180. Yang J, Sun G, Hu Y, Yang J, Shi Y, Liu H, et al. Extracellular Vesicle lncRNA Metastasis-Associated Lung Adenocarcinoma Transcript 1 Released From Glioma Stem Cells Modulates the Inflammatory Response of Microglia After Lipopolysaccharide Stimulation Through Regulating miR-129-5p/High Mobility Group Box-1 Protein Axis. Front Immunol (2020) 10:3161. doi: 10.3389/fimmu.2019.03161
181. Abels ER, Maas SLN, Nieland L, Wei Z, Cheah PS, Tai E, et al. Glioblastoma-associated microglia reprogramming is mediated by functional transfer of extracellular miR-21. Cell Rep (2019) 28(12):3105–3119.e7. doi: 10.1016/j.celrep.2019.08.036
182. Li X, Guan J, Jiang Z, Cheng S, Hou W, Yao J, et al. Microglial exosome miR-7239-3p promotes glioma progression by regulating circadian genes. Neurosci Bull (2021) 37(4):497–510. doi: 10.1007/s12264-020-00626-z
183. Serpe C, Monaco L, Relucenti M, Iovino L, Familiari P, Scavizzi F, et al. Microglia-Derived Small Extracellular Vesicles Reduce Glioma Growth by Modifying Tumor Cell Metabolism and Enhancing Glutamate Clearance through miR-124. Cells (2021) 10(8):2066. doi: 10.3390/cells10082066
184. Bier A, Hong X, Cazacu S, Goldstein H, Rand D, Xiang C, et al. miR-504 modulates the stemness and mesenchymal transition of glioma stem cells and their interaction with microglia via delivery by extracellular vesicles. Cell Death Dis (2020) 11(10):899. doi: 10.1038/s41419-020-03088-3
185. Qian M, Wang S, Guo X, Wang J, Zhang Z, Qiu W, et al. Hypoxic glioma-derived exosomes deliver microRNA-1246 to induce M2 macrophage polarization by targeting TERF2IP via the STAT3 and NF-κB pathways. Oncogene (2020) 39(2):428–42. doi: 10.1038/s41388-019-0996-y
186. Tsutsui T, Kawahara H, Kimura R, Dong Y, Jiapaer S, Sabit H, et al. Glioma-derived extracellular vesicles promote tumor progression by conveying WT1. Carcinogenesis (2020) 41(9):1238–45. doi: 10.1093/carcin/bgaa052
187. Fareh M, Almairac F, Turchi L, Burel-Vandenbos F, Paquis P, Fontaine D, et al. Cell-based therapy using miR-302-367 expressing cells represses glioblastoma growth. Cell Death Dis (2017) 8(3):e2713. doi: 10.1038/cddis.2017.117
188. Hong S, You JY, Paek K, Park J, Kang SJ, Han EH, et al. Inhibition of tumor progression and M2 microglial polarization by extracellular vesicle-mediated microRNA-124 in a 3D microfluidic glioblastoma microenvironment. Theranostics (2021) 11(19):9687–704. doi: 10.7150/thno.60851
189. Figueroa JM, Skog J, Akers J, Li H, Komotar R, Jensen R, et al. Detection of wild-type EGFR amplification and EGFRvIII mutation in CSF-derived extracellular vesicles of glioblastoma patients. Neuro Oncol (2017) 19(11):1494–502. doi: 10.1093/neuonc/nox085
190. Hwang SY, Jung JS, Kim TH, Lim SJ, Oh ES, Kim JY, et al. Ionizing radiation induces astrocyte gliosis through microglia activation. Neurobiol Dis (2006) 21(3):457–67. doi: 10.1016/j.nbd.2005.08.006
191. Oberoi RK, Parrish KE, Sio TT, Mittapalli RK, Elmquist WF, Sarkaria JN. Strategies to improve delivery of anticancer drugs across the blood-brain barrier to treat glioblastoma. Neuro Oncol (2016) 18(1):27–36. doi: 10.1093/neuonc/nov164
192. Nabors LB, Portnow J, Ammirati M, Baehring J, Brem H, Butowski N, et al. NCCN guidelines insights: central nervous system cancers, version 1.2017. J Natl Compr Canc Netw (2017) 15(11):1331–45. doi: 10.6004/jnccn.2017.0166
193. Clausi MG, Stessin AM, Zhao Z, Tsirka SE, Ryu S. Neuroinflammatory changes of the normal brain tissue in cured mice following combined radiation and anti-PD-1 blockade therapy for glioma. Sci Rep (2021) 11(1):5057. doi: 10.1038/s41598-021-84600-3
194. Dang W, Xiao J, Ma Q, Miao J, Cao M, Chen L, et al. Combination of p38 MAPK inhibitor with PD-L1 antibody effectively prolongs survivals of temozolomide-resistant glioma-bearing mice via reduction of infiltrating glioma-associated macrophages and PD-L1 expression on resident glioma-associated microglia. Brain Tumor Pathol (2021) 38(3):189–200. doi: 10.1007/s10014-021-00404-3
195. Pyonteck SM, Akkari L, Schuhmacher AJ, Bowman RL, Sevenich L, Quail DF, et al. CSF-1R inhibition alters macrophage polarization and blocks glioma progression. Nat Med (2013) 19(10):1264–72. doi: 10.1038/nm.3337
196. Yang Y, Brown MC, Zhang G, Stevenson K, Mohme M, Kornahrens R, et al. Polio virotherapy targets the Malignant glioma myeloid infiltrate with diffuse microglia activation engulfing the CNS. Neuro Oncol (2023), noad052. doi: 10.1093/neuonc/noad052
197. Fulci G, Dmitrieva N, Gianni D, Fontana EJ, Pan X, Lu Y, et al. Depletion of peripheral macrophages and brain microglia increases brain tumor titers of oncolytic viruses. Cancer Res (2007) 67(22):11092. doi: 10.1158/0008-5472.CAN-07-1063
198. Fulci G, Breymann L, Gianni D, Kurozomi K, Rhee SS, Yu J, et al. Cyclophosphamide enhances glioma virotherapy by inhibiting innate immune responses. Proc Natl Acad Sci U S A. (2006) 103(34):12873–8. doi: 10.1073/pnas.0605496103
199. Coelho PLC, Amparo JAO, da Silva AB, da Silva KC, Braga-de-Souza S, Barbosa PR, et al. Apigenin from Croton betulaster Müll restores the immune profile of microglia against glioma cells. Phytother Res (2019) 33(12):3191–202. doi: 10.1002/ptr.6491
200. da Silva AB, Cerqueira Coelho PL, das Neves Oliveira M, Oliveira JL, Oliveira Amparo JA, da Silva KC, et al. The flavonoid rutin and its aglycone quercetin modulate the microglia inflammatory profile improving antiglioma activity. Brain Behav Immun (2020) 85:170–85. doi: 10.1016/j.bbi.2019.05.003
201. Wang M, Liu K, Bu H, Cong H, Dong G, Xu N, et al. Purple sweet potato delphinidin-3-rutin represses glioma proliferation by inducing miR-20b-5p/Atg7-dependent cytostatic autophagy. Mol Ther Oncolytics. (2022) 26:314–29. doi: 10.1016/j.omto.2022.07.007
202. Przystal JM, Hajji N, Khozoie C, Renziehausen A, Zeng Q, Abaitua F, et al. Efficacy of arginine depletion by ADI-PEG20 in an intracranial model of GBM. Cell Death Dis (2018) 9(12):1192. doi: 10.1038/s41419-018-1195-4
203. Hajji N, Garcia-Revilla J, Soto MS, Perryman R, Symington J, Quarles CC, et al. Arginine deprivation alters microglial polarity and synergizes with radiation to eradicate non-arginine-auxotrophic glioblastoma tumors. J Clin Invest. (2022) 132(6):e142137. doi: 10.1172/JCI142137
204. Gao X, Li S, Ding F, Liu X, Wu Y, Li J, et al. A virus-mimicking nucleic acid nanogel reprograms microglia and macrophages for glioblastoma therapy. Adv Mater (2021) 33(9):e2006116. doi: 10.1002/adma.202006116
205. Azambuja JH, Schuh RS, Michels LR, Iser IC, Beckenkamp LR, Roliano GG, et al. Blockade of CD73 delays glioblastoma growth by modulating the immune environment. Cancer Immunol Immunother. (2020) 69(9):1801–12. doi: 10.1007/s00262-020-02569-w
206. Mormino A, Bernardini G, Cocozza G, Corbi N, Passananti C, Santoni A, et al. Enriched environment cues suggest a new strategy to counteract glioma: engineered rAAV2-IL-15 microglia modulate the tumor microenvironment. Front Immunol (2021) 12:730128. doi: 10.3389/fimmu.2021.730128
207. Chen C, Jing W, Chen Y, Wang G, Abdalla M, Gao L, et al. Intracavity generation of glioma stem cell-specific CAR macrophages primes locoregional immunity for postoperative glioblastoma therapy. Sci Transl Med (2022) 14(656):eabn1128. doi: 10.1126/scitranslmed.abn1128
208. Du Y, Yang Z, Sun Q, Lin M, Wang R, Peng Y, et al. Engineered microglia potentiate the action of drugs against glioma through extracellular vesicles and tunneling nanotubes. Adv Healthc Mater (2021) 10(9):e2002200. doi: 10.1002/adhm.202002200
209. Parsa AT, Waldron JS, Panner A, Crane CA, Parney IF, Barry JJ, et al. Loss of tumor suppressor PTEN function increases B7-H1 expression and immunoresistance in glioma. Nat Med (2007) 13(1):84–8. doi: 10.1038/nm1517
210. Zeng J, See AP, Phallen J, Jackson CM, Belcaid Z, Ruzevick J, et al. Anti-PD-1 blockade and stereotactic radiation produce long-term survival in mice with intracranial gliomas. Int J Radiat Oncol Biol Phys (2013) 86(2):343–9. doi: 10.1016/j.ijrobp.2012.12.025
211. Omuro A, Vlahovic G, Lim M, Sahebjam S, Baehring J, Cloughesy T, et al. Nivolumab with or without ipilimumab in patients with recurrent glioblastoma: results from exploratory phase I cohorts of CheckMate 143. Neuro Oncol (2018) 20(5):674–86. doi: 10.1093/neuonc/nox208
212. Lim M, Weller M, Idbaih A, Steinbach J, Finocchiaro G, Raval RR, et al. Phase III trial of chemoradiotherapy with temozolomide plus nivolumab or placebo for newly diagnosed glioblastoma with methylated MGMT promoter. Neuro Oncol (2022) 24(11):1935–49. doi: 10.1093/neuonc/noac116
213. Wang C, Chen Q, Chen M, Guo S, Hou P, Zou Y, et al. Interaction of glioma-associated microglia/macrophages and anti-PD1 immunotherapy. Cancer Immunol Immunother. (2023) 72(6):1685–98. doi: 10.1007/s00262-022-03358-3
214. Rao G, Latha K, Ott M, Sabbagh A, Marisetty A, Ling XG, et al. Anti-PD-1 induces M1 polarization in the glioma microenvironment and exerts therapeutic efficacy in the absence of CD8 cytotoxic T cells. Clin Cancer Res (2020) 26(17):4699–712. doi: 10.1158/1078-0432.CCR-19-4110
215. Tu S, Lin X, Qiu J, Zhou J, Wang H, Hu S, et al. Crosstalk between tumor-associated microglia/macrophages and CD8-positive T cells plays a key role in glioblastoma. Front Immunol (2021) 12:650105. doi: 10.3389/fimmu.2021.650105
216. Russell SJ, Peng KW, Bell JC. Oncolytic virotherapy. Nat Biotechnol (2012) 30(7):658–70. doi: 10.1038/nbt.2287
217. Andtbacka RH, Kaufman HL, Collichio F, Amatruda T, Senzer N, Chesney J, et al. Talimogene laherparepvec improves durable response rate in patients with advanced melanoma. J Clin Oncol (2015) 33(25):2780–8. doi: 10.1200/JCO.2014.58.3377
218. Lawler SE, Speranza MC, Cho CF, Chiocca EA. Oncolytic viruses in cancer treatment: A review. JAMA Oncol (2017) 3(6):841–9. doi: 10.1001/jamaoncol.2016.2064
219. Lang FF, Conrad C, Gomez-Manzano C, Yung WKA, Sawaya R, Weinberg JS, et al. Phase I study of DNX-2401 (Delta-24-RGD) oncolytic adenovirus: replication and immunotherapeutic effects in recurrent Malignant glioma. J Clin Oncol (2018) 36(14):1419–27. doi: 10.1200/JCO.2017.75.8219
220. Desjardins A, Gromeier M, Herndon JE 2nd, Beaubier N, Bolognesi DP, Friedman AH, et al. Recurrent glioblastoma treated with recombinant poliovirus. N Engl J Med (2018) 379(2):150–61. doi: 10.1056/NEJMoa1716435
221. Alessandrini F, Menotti L, Avitabile E, Appolloni I, Ceresa D, Marubbi D, et al. Eradication of glioblastoma by immuno-virotherapy with a retargeted oncolytic HSV in a preclinical model. Oncogene (2019) 38(23):4467–79. doi: 10.1038/s41388-019-0737-2
222. Blitz SE, Kappel AD, Gessler FA, Klinger NV, Arnaout O, Lu Y, et al. Tumor-associated macrophages/microglia in glioblastoma oncolytic virotherapy: A double-edged sword. Int J Mol Sci (2022) 23(3):1808. doi: 10.3390/ijms23031808
223. Han J, Chen X, Chu J, Xu B, Meisen WH, Chen L, et al. TGFβ Treatment enhances glioblastoma virotherapy by inhibiting the innate immune response. Cancer Res (2015) 75(24):5273–82. doi: 10.1158/0008-5472.CAN-15-0894
224. Coniglio SJ, Segall JE. Microglial-stimulation of glioma invasion involves the EGFR ligand amphiregulin. PloS One (2021) 16(11):e0260252. doi: 10.1371/journal.pone.0260252
225. Butowski N, Colman H, De Groot JF, Omuro AM, Nayak L, Wen PY, et al. Orally administered colony stimulating factor 1 receptor inhibitor PLX3397 in recurrent glioblastoma: an Ivy Foundation Early Phase Clinical Trials Consortium phase II study. Neuro Oncol (2016) 18(4):557–64. doi: 10.1093/neuonc/nov245
226. ClinicalTrials.gov. A phase 1b/2 study of PLX3397 + Radiation therapy + Temozolomide in patients with newly diagnosed glioblastoma (2020). Available at: https://clinicaltrials.gov/ct2/show/NCT01790503 (Accessed June 3, 2023).
227. Barca C, Foray C, Hermann S, Herrlinger U, Remory I, Laoui D, et al. The colony stimulating factor-1 receptor (CSF-1R)-mediated regulation of microglia/macrophages as a target for neurological disorders (Glioma, stroke). Front Immunol (2021) 12:787307. doi: 10.3389/fimmu.2021.787307
228. Quail DF, Bowman RL, Akkari L, Kunze RA, Heinze CE, Huseman ED, et al. The tumor microenvironment underlies acquired resistance to CSF-1R inhibition in gliomas. Science (2016) 352(6288):aad3018. doi: 10.1126/science.aad3018
229. Cárdenas M, Marder M, Blank VC, Roguin LP. Antitumor activity of some natural flavonoids and synthetic derivatives on various human and murine cancer cell lines. Bioorg Med Chem (2006) 14(9):2966–71. doi: 10.1016/j.bmc.2005.12.021
230. Stump TA, Santee BN, Williams LP, Soares JRP, da Silva KC, Santana MR, et al. The antiproliferative and apoptotic effects of apigenin on glioblastoma cells. J Pharm Pharmacol (2017) 69(7):907–16. doi: 10.1111/jphp.12718
231. do Nascimento RP, Dos Santos BL, Amparo JAO, Ji Q, Zhang B, Mei Q, et al. Neuroimmunomodulatory properties of flavonoids and derivates: A potential action as adjuvants for the treatment of glioblastoma. Pharmaceutics (2022) 14(1):116. doi: 10.3390/pharmaceutics14010116
232. Haines RJ, Pendleton LC, Eichler DC. Argininosuccinate synthase: at the center of arginine metabolism. Int J Biochem Mol Biol (2011) 2(1):8–23.
233. Qiao S, Cheng Y, Liu M, Ji Q, Zhang B, Mei Q, et al. Chemoattractants driven and microglia based biomimetic nanoparticle treating TMZ-resistant glioblastoma multiforme. J Control Release. (2021) 336:54–70. doi: 10.1016/j.jconrel.2021.06.015
234. Ellert-Miklaszewska A, Ochocka N, Maleszewska M, Ding L, Laurini E, Jiang Y, et al. Efficient and innocuous delivery of small interfering RNA to microglia using an amphiphilic dendrimer nanovector. Nanomedicine (Lond). (2019) 14(18):2441–58. doi: 10.2217/nnm-2019-0176
235. Sharma R, Liaw K, Sharma A, Jimenez A, Chang M, Salazar S, et al. Glycosylation of PAMAM dendrimers significantly improves tumor macrophage targeting and specificity in glioblastoma. J Control Release. (2021) 337:179–92. doi: 10.1016/j.jconrel.2021.07.018
236. Zhang Y, You H, Wang Y, Chen Q, Guo Q, Chu Y, et al. A micro-environment regulator for filling the clinical treatment gap after a glioblastoma operation. Adv Healthc Mater (2022) 11(3):e2101578. doi: 10.1002/adhm.202101578
237. Khattar KE, Safi J, Rodriguez AM, Vignais ML. Intercellular communication in the brain through tunneling nanotubes. Cancers (Basel). (2022) 14(5):1207. doi: 10.3390/cancers14051207
238. Guo L, Zhang X, Wei R, Li G, Sun B, Zhang H, et al. Engineering microglia as intraoperative optical imaging agent vehicles potentially for fluorescence-guided surgery in gliomas. Biomater Sci (2020) 8(4):1117–26. doi: 10.1039/c9bm01388a
239. Szulzewsky F, Arora S, de Witte L, Ulas T, Markovic D, Schultze JL, et al. Human glioblastoma-associated microglia/monocytes express a distinct RNA profile compared to human control and murine samples. Glia (2016) 64(8):1416–36. doi: 10.1002/glia.23014
240. Gu R, Zhang X, Zhang G, Tao T, Yu H, Liu L, et al. Probing the bi-directional interaction between microglia and gliomas in a tumor microenvironment on a microdevice. Neurochem Res (2017) 42(5):1478–87. doi: 10.1007/s11064-017-2204-1
241. Chen JE, Lumibao J, Leary S, Sarkaria JN, Steelman AJ, Gaskins HR, et al. Crosstalk between microglia and patient-derived glioblastoma cells inhibit invasion in a three-dimensional gelatin hydrogel model. J Neuroinflammation. (2020) 17(1):346. doi: 10.1186/s12974-020-02026-6
242. Tanaka S, Ohgidani M, Hata N, Inamine S, Sagata N, Shirouzu N, et al. CD206 expression in induced microglia-like cells from peripheral blood as a surrogate biomarker for the specific immune microenvironment of neurosurgical diseases including glioma. Front Immunol (2021) 12:670131. doi: 10.3389/fimmu.2021.670131
Keywords: interaction, microglia¸ glioma, microenvironment, heterogeneity, and immunotherapy
Citation: Tao J-C, Yu D, Shao W, Zhou D-R, Wang Y, Hou S-Q, Deng K and Lin N (2023) Interactions between microglia and glioma in tumor microenvironment. Front. Oncol. 13:1236268. doi: 10.3389/fonc.2023.1236268
Received: 07 June 2023; Accepted: 14 August 2023;
Published: 28 August 2023.
Edited by:
Wei Hua, Fudan University, ChinaReviewed by:
Alessandro Poggi, San Martino Hospital (IRCCS), ItalyCopyright © 2023 Tao, Yu, Shao, Zhou, Wang, Hou, Deng and Lin. This is an open-access article distributed under the terms of the Creative Commons Attribution License (CC BY). The use, distribution or reproduction in other forums is permitted, provided the original author(s) and the copyright owner(s) are credited and that the original publication in this journal is cited, in accordance with accepted academic practice. No use, distribution or reproduction is permitted which does not comply with these terms.
*Correspondence: Ning Lin, bGluMjAwNzUxMkB2aXAuMTYzLmNvbQ==
†These authors have contributed equally to this work and share first authorship
Disclaimer: All claims expressed in this article are solely those of the authors and do not necessarily represent those of their affiliated organizations, or those of the publisher, the editors and the reviewers. Any product that may be evaluated in this article or claim that may be made by its manufacturer is not guaranteed or endorsed by the publisher.
Research integrity at Frontiers
Learn more about the work of our research integrity team to safeguard the quality of each article we publish.