- Department of Neurosurgery, Stanford University School of Medicine, Stanford, CA, United States
Central nervous system (CNS) neoplasms are difficult to treat due to their sensitive location. Over the past two decades, the availability of patient tumor materials facilitated large scale genomic and epigenomic profiling studies, which have resulted in detailed insights into the molecular underpinnings of CNS tumorigenesis. Based on results from these studies, CNS tumors have high molecular and cellular intra-tumoral and inter-tumoral heterogeneity. CNS cancer models have yet to reflect the broad diversity of CNS tumors and patients and the lack of such faithful cancer models represents a major bottleneck to urgently needed innovations in CNS cancer treatment. Pediatric cancer model development is lagging behind adult tumor model development, which is why we focus this review on CNS tumors mutated for BRAFV600E which are more prevalent in the pediatric patient population. BRAFV600E-mutated CNS tumors exhibit high inter-tumoral heterogeneity, encompassing clinically and histopathological diverse tumor types. Moreover, BRAFV600E is the second most common alteration in pediatric low-grade CNS tumors, and low-grade tumors are notoriously difficult to recapitulate in vitro and in vivo. Although the mutation predominates in low-grade CNS tumors, when combined with other mutations, most commonly CDKN2A deletion, BRAFV600E-mutated CNS tumors are prone to develop high-grade features, and therefore BRAFV600E-mutated CNS are a paradigm for tumor progression. Here, we describe existing in vitro and in vivo models of BRAFV600E-mutated CNS tumors, including patient-derived cell lines, patient-derived xenografts, syngeneic models, and genetically engineered mouse models, along with their advantages and shortcomings. We discuss which research gaps each model might be best suited to answer, and identify those areas in model development that need to be strengthened further. We highlight areas of potential research focus that will lead to the heightened predictive capacity of preclinical studies, allow for appropriate validation, and ultimately improve the success of “bench to bedside” translational research.
1 Introduction
1.1 BRAFV600E mutation in central nervous system tumors have diverse prognosis and are candidates for molecular targeted therapy
The MEK-ERK signaling pathway is activated by growth factor-stimulated RAS binding to BRAF, which, when dysregulated, can initiate tumorigenesis by promoting uncontrolled cell growth (1) and cell fate changes (2, 3). Alterations in the BRAF gene, which encodes a central kinase in the MAPK signaling pathway, are prevalent in CNS tumors and are enriched in the pediatric population. BRAF protein is the most frequently mutated serine/threonine kinase in human cancer, with BRAFV600E and KIAA1549::BRAF fusion being significant drivers of cellular transformation in pediatric CNS tumors, with high occurrences in low-grade gliomas (LGGs, up to 35%), and low occurrences in high-grade gliomas (HGGs, ~5% for BRAFV600E) (4, 5). BRAFV600E is a class I mutation, the most common class of mutations in glioma. Through a somatic point mutation at codon V600, valine is substituted by glutamate (BRAFV600E), which is thought to mimic regulatory phosphorylation in the activation segment of the protein kinase domain (6). Consequently, BRAFV600E-mutated kinase monomers constitutively activate the downstream MAPK-ERK signaling pathway, regardless of any external stimuli and independent of upstream Ras (7, 8).
Due to the high incidence of BRAFV600E in several solid cancers, small molecule inhibitors against BRAFV600E have been developed and have been actively tested in the clinic (9–11). These BRAF inhibitors rapidly suppress MAPK signaling but exhibit only transient effects, prompting their subsequent combination with MAPK inhibitors for treatment (12). BRAFV600E and MEK inhibitor combination therapy (e.g., dabrafenib+trametinib) has been tested in the clinic against glioma and clinical response rates of 70% and 33% have been observed amongst adults with low- and high-grade glioma, respectively. Similar response rates in children have led to the recent approval of BRAFV600E inhibitor dabrafenib and MEK inhibitor trametinib for pediatric patients one year of age and older with BRAFV600E-mutated low-grade gliomas (LGG) who require systemic therapy (13–15). Despite these advances, further research is needed to develop new therapies and improve patient outcomes; clinical data shows tumor rebound when treatment is discontinued, and high-grade tumors frequently fail to respond or develop therapy resistance (16). Significant challenges stand in the way of improving current treatments, including that BRAFV600E-mutated pediatric LGG (pLGG) are frequently found with secondary mutations, most commonly CDKN2A deletion, and at lower frequency with single nucleotide variations (SNVs) in NF1, FGFR1, KRAS, and H3FA (5). In addition, 24% of BRAFV600E-mutated adult glioblastoma had mutations in the TERT promoter (17).
Alteration type influences the clinical course and therapy responsiveness of tumors. BRAFV600E altered pLGG lacking tumor suppressor CDKN2A are at high risk for transforming into high-grade tumors when compared with BRAFV600E CDKN2A balanced and BRAF wildtype tumors (18–20). Co-occurring alterations provide novel therapeutic vulnerabilities that can be targeted such as for example CDK4/6 inhibitors that target the p16/RB axis (21). Whether these drugs work synergistically or cooperatively with MAPK pathway inhibitors is not fully explored and additional preclinical testing is needed. Such preclinical testing requires preclinical models for BRAFV600E CNS tumors that capture the genetic alterations found in human tumors. Mechanisms for cooperation of BRAFV600E with CDKN2A loss for malignant progression have been investigated in genetically engineered mouse models (22). Other combinations of other co-occurring alterations (e.g., FGFR, H3FA) and BRAFV600E are not yet available in models.
In conclusion, BRAFV600E is a common kinase activating alteration in human cancer and in CNS with high prevalence in pLGG, whereby patients with BRAFV600E altered tumors are routinely, successfully treated with molecular targeted therapy (BRAF inhibition). A unique feature is that BRAFV600E-mutated hemispheric pLGG tend to progress from low to high grade tumors at which point they become difficult to manage and exhibit therapy resistance to BRAF inhibition (20, 23). Models that recapitulate BRAFV600E pLGG and the progression from low- to high-grade are not available, which is why little is known about their biology and the underlying mechanisms of progression; this will be discussed in detail in the “Developing models for tumor progression from low to high grade is challenging” section in this review.
The clinicopathologic diversity of BRAFV600E-mutated CNS tumors due to diverse co-occurring alterations makes treatment decisions ambiguous and raises the need for preclinical testing of combination therapies of BRAF/MEK inhibitors and therapies targeting co-occurring alterations. Such studies require generation of additional models which represent all types of genetic alterations combined with BRAFV600E in human tumors.
1.2 BRAFV600E -mutated CNS tumors striking inter-tumoral heterogeneity affects clinical prognosis
BRAFV600E CNS tumors are enriched in the pediatric population and occur most frequently in pLGG (17-35% of pLGG) and are found in a diverse range of histopathologic subtypes of glioma. Both pleomorphic xanthoastrocytoma/PXA (40~80%), ganglioglioma/GG (25~45%) and desmoplastic infantile gangioglioma/astrocytoma (DIG/DIA; 45%) are more likely to harbor the mutation than other pLGG, such as pilocytic astrocytoma/PA (5~16%); optic pathway glioma (OPG; ~2%), dysembryoplastic neuroepithelial tumors/DNT (5~10%), infantile LGG (iLGG; 18%), and diffuse pediatric-type LGGs (19, 24–26). BRAFV600E occurs at lower frequency in pHGG (6-14%) a histopathologically and clinical diverse group of tumors (4). Importantly, BRAFV600E expression when coinciding with CDKN2A deletion marks a clinicopathologic subgroup of pLGG at high-risk for progression (5, 18). Consistent with this high risk, BRAFV600E is the most frequent recurrent mutation in secondary pHGGs, occurring in 39% of cases (27).
BRAFV600E-mutated pLGG occurred most frequently in hemispheric regions (56%) but were also common in the diencephalon (29%). Interestingly, BRAFV600E-mutated iLGG which present most frequently in the midline rarely progresses, and the mutation is absent in infantile HGG (iHGG), which is paradoxical to its occurrence in pHGG overall (5, 26). These data suggest that tumor location affects the clinical course of BRAFV600E-mutated gliomas. Further to this point, BRAFV600E-mutated PA are prevalent in diencephalic areas in particular in unresectable hypothalamic/optic pathway PA, in contrast to KIAA1549-BRAF fusion-positive PA, which are prevalent in the cerebellum (24). Amongst these hypothalamic/optic pathway PA, BRAFV600E is more frequently associated with non-progression, and KIAA1549-BRAF fusion was more common in progressive tumors (28).
BRAF-mutated diencephalic LGGs rarely present malignant transformation, but because they are usually unresectable, novel targeted therapy is strongly warranted. Indeed, clinical reports show promising results with BRAFV600E inhibitor therapy in optic pathway glioma (29). Spatial distribution patterns of the BRAFV600E mutation in pediatric, infantile, and adult LGGs (25) and clinical trial results with molecular targeted therapies against BRAF-mutated tumors have been extensively reviewed elsewhere (8, 17, 30, 31). While BRAF-fusion events have been associated with a good prognosis, and SNVs (most prominently BRAFV600E) have been associated with poor prognosis in pediatric LGG when all sites and histologies were included (5), the situation is reversed in BRAF-mutated pediatric diencephalic LGG such as optic pathway/hypothalamic glioma.
In conclusion, BRAFV600E-altered CNS tumors occur in diverse neuroanatomical locations, predominantly in hemispheric areas and BRAFV600E-altered PA exhibit a strong association with extra-cerebellar location. The microenvironment of tumors in distinct locations may determine the prognostic value of the BRAF alteration while responses to BRAF inhibitors appear to be universal. Due to the small study size of diencephalic tumors, further clinical and preclinical analyses are needed to test the neuroanatomical aspects of BRAFV600E-altered CNS tumors.
BRAFV600-mutated tumors present at a wide age range with a median age of 10.6 years in the pediatric population and are also found in adults. Genomic and epigenomic large scale analyses have detected BRAFV600E in several adult CNS astrocytic tumors, including astroblastoma (24%-38%) (8, 25, 32), diffuse astrocytoma (DA; 30~40%), glioblastoma multiforme (GBM; ~ 6%) and astrocytoma (2~5%) (5, 17, 24, 33). Notably, the spectrum of histologic grades, pathology and nature of BRAF mutations in adults contrasted with that in pediatric tumors. In adults with BRAF-mutated CNS tumors, BRAFV600E is most frequent in glioblastoma (~50%), and is common in epitheloid GBM (34, 35), followed by LGG (~22%), and PXA (18%). The mutation is notably absent in tumors with oligodendroglioma histology. Therefore, the histologic spectrum of BRAFV600E CNS tumors is different in pediatric and adult patients. BRAFV600E co-occurs with a similar set of alterations in pediatric and adult tumors, and both patient groups can benefit from BRAF-targeted therapy (17).
In non-CNS solid cancers, BRAFV600E is frequently detected in malignant melanoma, hairy cell leukemia, colorectal carcinoma, and papillary thyroid carcinoma, as well as ovarian and lung tumors (36). Approximately 50% of melanomas harbor a BRAFV600 mutation (37, 38). Melanoma has the highest propensity to form brain metastases of all malignancies (39) and given that melanoma patients have a high incidence (10-40%) of developing metastases in the brain (40), the clinical relevance of BRAF mutational status in melanoma brain metastasis has been studied (41, 42). Evidence showed that patients with BRAFV600E-mutated melanoma were more likely to have CNS involvement than those with BRAF wild-type melanoma (43, 44). Clinical studies showed safety and efficacy of BRAF inhibitor therapy in patients with treated and untreated melanoma brain metastases more recently (45–47). In addition, BRAF and MEK inhibitor combination therapy provided promising clinical results against melanoma brain metastases (48, 49) which have prompted assessing baseline clinical features associated with outcomes (45, 46, 48–51). Genetic interaction studies in genetically engineered mouse models showed that BRAF V600E expression cooperates with Pten tumor suppressor loss to generate metastatic melanoma without CNS involvement (52). Expression of BRAFV600E in Cdkn2aNull mice generates melanomas without metastasis, but AKT1 activation promotes development of CNS metastases in this model (53).
In conclusion, BRAFV600E expression is a driver mutation of CNS tumors that occur in various neuroanatomic locations and exhibit a wide range of age at presentation. In non-CNS cancers BRAFV600E expression is not sufficient to generate CNS metastases and the type of co-occurring alterations determine whether CNS metastases form. More work is needed to unravel how BRAFV600E mutation cooperates with co-alterations to increase invasive properties of tumor cells and generate brain metastasis.
1.3 Variation of BRAFV600E-mutated CNS neoplasms necessitates targeted and tailored strategies
Dysregulated neurodevelopmental programs underlying the distinct susceptibility of stem and progenitor cells to oncogenic alterations cause childhood CNS cancer (54). As stated above, the wide variance of disease location, age at onset, histopathology, and clinical outcome of BRAFV600E-mutated CNS neoplasms is quite striking and quite unique amongst pediatric CNS cancer types. This diversity sets BRAFV600E apart from other point mutations in pediatric CNS cancers, such as the histone 3 variants H3.3 and H3.1, which are strongly associated with unique locations, high grade and more narrow age range, and a uniformly dismal prognosis (55). It suggests that cells at distinct stages and neuroanatomical locations can be susceptible to transformation by this oncogene (55, 56). How a single oncogene can generate such diverse tumors and lead to age-dependent differences of prognosis is unknown. Tumor extrinsic factors, such as the tumor microenvironment and the immune system may play an important role in the development of these tumors and these factors need to be considered as important determinants of tumor subtypes when developing BRAFV600E CNS tumor models. We are far from capturing the diversity of BRAFV600E-mutated CNS tumor’s onset, histopathology, location, and prognosis with in vitro and in vivo models; thus, the underlying mechanisms leading to such diverse tumors remain poorly understood.
This represents a significant research problem. Despite the initial successes of treatment with FDA-approved first-generation small molecule inhibitors of BRAFV600E, such as vemurafenib, dabrafenib, and encorafenib, still therapeutic effects are not durable. Although effective in treating other varieties of BRAFV600E malignancies, tolerance towards BRAFi as a monotherapy has been shown in melanoma and HGG, commonly through (acquired, secondary) reactivation of the MEK pathway in melanoma, and resistance mechanisms are discussed in more detail below. Since treatment efficacy is limited by drug resistance in HGGs and BRAFV600E -mutated LGGs frequently progress to high-grade, novel therapeutic approaches against BRAFV600E -mutated gliomas in general are needed, raising the urgent need for further refinement of BRAFV600E models for preclinical studies and their successful translation.
Here, we aim at providing an overview of mammalian models for BRAFV600E mutated CNS tumors; we list existing models, compare their usefulness for basic mechanistic studies and preclinical approaches, and describe current strategies to analyze and quantify tumor phenotypes and vulnerabilities. The objectives of this review are to categorize available preclinical in vitro and in vivo models of BRAFV600E-mutated CNS tumors. We highlight the applicability of these models for drug screening purposes and managing resistance. We describe their utility for disease characterization, including identifying and characterizing co-occurring mutations for their clinicopathologic characteristics and predictive and prognostic impact. In addition, we make suggestions on how to overcome existing limitations in model development. Preclinical studies should be performed in multiple models to avoid model-specific bias and ensure that preclinical data are robust and successfully translated to the clinic. Fortunately, several types of models are available and will be discussed in greater detail below.
2 In Vitro modeling and drug strategies
2.1 Cancer cell line models
Conventional cancer cell lines have been historically used for therapeutic screening due to their robust and uniform phenotypes and growth behavior as attached monolayer in high-serum culture conditions. Davies et al. identified BRAFV600E mutations in established cancer cell lines DBTRG-05MG and AM38 (6), which subsequently became workhorses for preclinical testing, in particular of small molecule BRAF inhibitors, including PLX4720, a tool compound of Vemurafenib (Table 1). PLX4720 suppressed cell viability in several BRAFV600E altered cell lines and patient-derived orthotopic xenografts (PDoX) from DBTRG-05MG and AM38, with PDoX recapitulating the in vitro sensitivity to BRAF inhibitors (Table 2) including PLX4720 monotherapy (79).
2.2 High-fidelity mouse models were used for testing combinatorial inhibitor therapy
Like studies in melanoma, where BRAF inhibitor monotherapy shows rapid but transient MAPK pathway inhibition, PLX4720 treatment in glioma cell lines (M-38, DBTRG-05MG and NMC-G1) did not suppress MAPK pathway signaling in a durable way. This prompted testing of vertical dual inhibition of MAPK pathway with BRAF and MEK inhibitors, an approach that has improved response rates in melanoma. Several studies indeed showed that MAPK signaling rebound in glioma can be overcome by adding MEK inhibition, including our work with a BRAFV600E murine glioma cell line (2341) which were derived from an adenovirus-induced tumor in a Cre-inducible mouse model in which BRAFV600E is expressed under the endogenous promoter, and hence under physiologic levels (22) (Figure 1). BRAFV600E inhibitor monotherapy with dabrafenib of 2341 cells recapitulated the initial reduction followed by reactivation of MAPK signaling and accordingly 2341-derived orthotopic glioma transiently respond to BRAFV600E inhibitor monotherapy with initial responses followed by continuous tumor growth. Combining BRAF and MEK inhibitors (dabrafenib+trametinib) suppressed MAPK pathway signaling in a more durable fashion (57, 80). Tumor regression from the combined dual MAPK pathway blockade with BRAF and MEK inhibitors was also confirmed in BT40 PDoX, which are described in greater detail below (81). In addition, combination therapy with PLX4720 + MEK inhibitor mirdametinib (PD0325901) (81) showed durable suppression of the MAPK pathway and this resulted in longer survival of animal subjects, further confirming our earlier data.
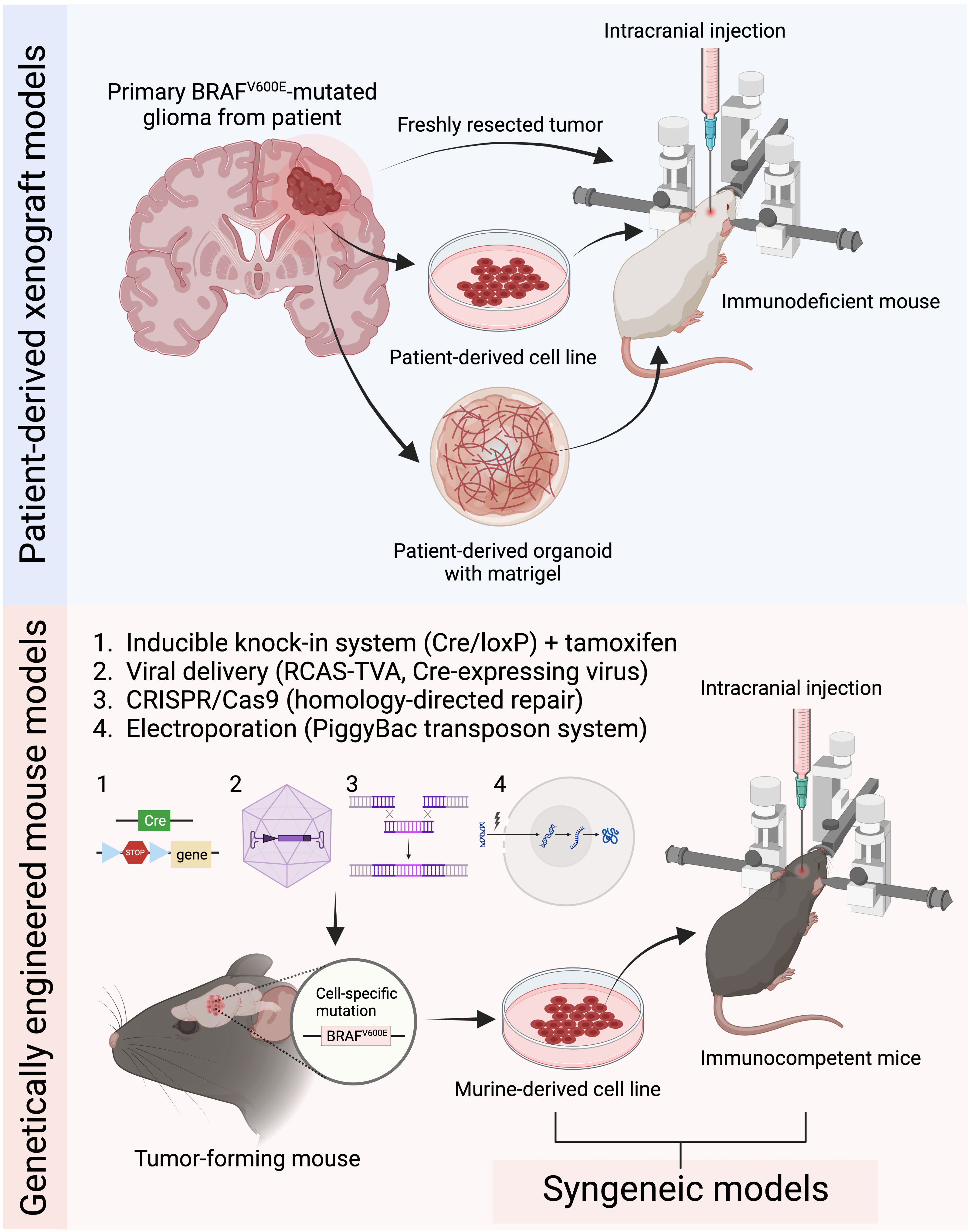
Figure 1 Current common preclinical mouse models for BRAFV600E mutated gliomas. Patient-derived xenograft models are generated by intracranially implanting patient-derived tumor tissue, cells, or organoids into immunodeficient mice. Genetically engineered mouse models (GEMMs) induce tumor development through genetic manipulation using either the inducible knock-in system, virus-based strategies, CRISPR/Cas9 system, or in utero electroporation. Syngeneic models are established by implanting murine-derived tumor cell lines into immunocompetent mice (Figure created with BioRender.com).
These studies combined prompted subsequent clinical evaluation of Vemurafenib against BRAFV600E-mutated gliomas (11) and more recently clinical use of BRAF and MEK inhibitor combination treatment in patients with BRAFV600E-mutated CNS tumors. Clinical responses showed an improvement in response rates with combination therapy over monotherapy (13). These data lead to the recent FDA approval of dabrafenib+trametinib for LGG and provide a paradigm for successful translation of preclinical data based on high-fidelity tumor models, including the 2341 model which expresses BRAFV600E under the endogenous promoter like that in human tumors (57).
Continuous studies into novel combination therapies are important because of therapy resistance to vertical inhibition of MAPK signaling and cross-resistance to other BRAF/MEK inhibitors and BRAF/EGFR inhibitors in the clinic. Combinations investigated are of BRAFV600E inhibitors with cdk4/6 inhibitor PD0332991 (22), PLK1 inhibitor tool compound BI2536 (82), radiation (83), MEK inhibitor AZD6224 + mTOR inhibitor everolimus (80), and EGFR inhibitor HKI-272 (84). These studies have used 2341 in addition to BRAFV600E mutated CDKN2ANull murine glioma cells derived from mice expressing BRAFV600E using the CRE/Lox system under the nestin and GFAP-promoter, as well as established cancer cell lines.
Noteworthy, the 2341 orthotopic model can be implanted in fully immunocompetent mice allowing studies of immunomodulation and immunotherapy in the context of BRAFV600E-mutated gliomas. Using the 2341-BRAFV600E model, we have recently demonstrated that BRAF/MEK inhibition alters the tumor immune infiltrate and sensitizes tumors against immune checkpoint blockade by anti-PD-L1 and anti-CTLA4 treatment, which resulted in better clinical outcomes in mice implanted with BRAFV600E mutated high-grade gliomas (3).
In conclusion, preclinical data obtained using murine glioma models that faithfully recapitulate the human tumors’ genetics and phenotypes have high predictive capacity.
2.3 Patient-derived cell lines and media considerations
Conventional cancer cell lines are customarily grown as adherent monolayers on plastic dishes or laminin covered flasks in high-serum medium (typically supplemented with 10% FBS), for multiple generations. Although they are robust and easy to grow in this manner, the high-serum medium prompts cells to differentiate towards astrocytic phenotype, thus generating homogeneous cell cultures, which is unrepresentative of glioma’s biology. Moreover, the parental tumor of conventional cancer cell lines is frequently unknown and/or uncharacterized. As such, these models are useful for assay optimizations, including commercially available kits, imaging, monitoring morphological changes and optimizing antibody staining conditions. However, 2D monolayer architecture poorly represents patient-specific heterogeneity and affects tumor intrinsic features related to cell signaling, phenotype, cell-to-cell interactions, and drug response. The 2D cell arrangement generates inappropriate cell density and nutrient gradients, unphysiological oxygen levels, underrepresented spatial context, and artificial interactions with extracellular matrix and tumor microenvironment (85). In contrast, patient-derived cell lines (PDCLs) are initiated from tissue obtained during craniotomies, biopsies, and autopsies. Rapidly dissociated surgical samples are typically seeded in low-attachment cell culture flasks, and generate 3D spheres within a few days. Moreover, the culturing medium is serum-free and is commonly supplemented with growth factors (EGF and FGF-2), which perpetuates the stem cell phenotypes frequently observed in glioma cells and establishes robust glioma stem cell (GSC) cultures. Such patient-derived 3D spheroid cultures preserve features of the original tumor better than conventional 2D cancer cells lines (86) because they demonstrate higher proliferation and invasive capacity, high tolerance towards chemoradiation and sensitivity towards T- and NK-mediated cell killing (85, 87). This being said, PDCLs can be used as personalized platforms for drug testing and therapy tailoring (88), such as evaluating the benefit of single BRAFi vs combined with MEK/mTOR/EGFR inhibitors. A side-by-side comparison of patient-derived GBM cells has clearly demonstrated loss of malignant potential, decreased morphologic, and cell type heterogeneity, and divergence of expression profiles in cells grown in 2D differentiating conditions (high serum), versus 3D un-differentiating (serum-free) medium (89). Proliferation rates are frequent measures of drug activity in cell-based assays. These are significantly increased in adherent cells, while 3D spheroid cultures exhibit more stable doubling times even after multiple rounds of passaging. Furthermore, conventional 2D cultures are unreliable for functional analysis, as they undergo genetic drift over time, emphasizing the need for 3D spheroid models of CNS tumors (Table 1).
Our group recently generated a stable spheroid PDCL from a patient with a high-grade BRAFV600E mutated tumor that also carried a TP53 and RB mutation (STN-10049) (3). This cell line was sensitive to the anti-proliferative effects of combined dabrafenib and trametinib treatment in vitro, although a marker for therapy resistant cells CD133 (82) was upregulated with dual MAPK inhibitor treatment, and the patient worsened rapidly despite aggressive chemotherapy and dabrafenib-trametinib treatment (3). Cells derived from the same patient’s tumor at autopsy showed decreased sensitivity to dabrafenib-trametinib, and increased expression of CD133, consistent with the patient’s lack of response and cancer progression on the drug. Thus, patient-derived cells derived from different timepoints (pre- and post-treatment) recapitulate longitudinal the patient response to therapy.
Several PDCLs representing various BRAFV600E-mutated CNS tumor subtypes have been generated and published by individual labs (see Table 1 for a comprehensive list), and these lines are important novel tools available to investigators. Successful culturing and careful expansion of these models while monitoring for retention of driver alterations and genetic drift will ensure deep, comprehensive understanding of the biological and functional impact of BRAFV600E mutation in glioma.
Molecular analyses of BRAFV600E-mutated glioma PDCLs are still scarce (Table 1, Other Alterations Reported), and the congruence of these cell lines with their tumor of origin has yet to be determined. Large scale initiatives such as the Human Cancer Model Initiative (HCMI) in the USA have been launched with the purpose of generating next-generation models, whereby “next-generation” refers to models being clinically annotated and are characterized molecularly and phenotypically for their resemblance to their parental tumor (see HCMI searchable catalogue). HCMI cell lines can be purchased from the American Type Culture Collection (ATCC). As of May 2023, no BRAFV600E-mutated CNS tumor cell lines have been reported in the HCMI searchable catalogue, but the efforts of the HCMI are ongoing, and expected to include more pediatric models soon.
In summary, PDCLs grown under 3D spheroid conditions are superior models of the human disease than conventional cancer cell lines and multiple individual labs. Novel BRAFV600E PDCLs are continuously reported by individual investigators (Table 1) and larger scale model development initiatives and collectively these models will provide an important toolkit for robust preclinical and mechanistic studies of drug resistance and targetability.
2.4 Models for studying and overcoming therapy resistance
2.4.1 Non-CNS cancer
Treatment with FDA-approved first-generation small molecule inhibitors of BRAFV600E, such as vemurafenib, dabrafenib, and encorafenib, showed clinical efficacy in some but not all glioma patients, raising the question how therapy resistance develops and how it can be overcome (90). Much data for therapy resistance mechanisms have been derived from BRAFV600E-mutated melanoma and to a lesser extent colorectal cancer PDCLs and cell lines (Table 2).
Preclinical studies in melanoma are the perfect example of how fundamental and translational findings improved clinical management of this disease. BRAFV600E is prevalent in metastatic melanoma and novel strategies for BRAF and MEK inhibition are continuously developed and trialed to deliver long-term clinical response in patients. Combined BRAF and MEK inhibitors, as compared with BRAF inhibition alone, provided remarkable response rates in melanoma, delayed the emergence of resistance, and reduced toxic effects in patients with BRAFV600E-mutated melanoma (91, 92). Long-term survival analyses in metastatic melanoma showed that while one third of patients demonstrate long-lasting clinical benefit, the majority invariably progress with MAPK pathway inhibition alone (93). Resistance pathways discovered in melanoma can be divided broadly into three groups: RTK hyperactivation/overexpression, secondary MAPK/ERK mutations (ERK1/2 phosphorylation through NRAS mutations, paradoxical MAPK activation, AKT amplification/mutation, CRAF dimerization, BRAF amplification) and other alternative pathways (cyclin D1 induction, PTEN loss, BIM suppression) (73). Comparably, two independent studies of BRAFV600E-mutated melanoma cell lines (conventional and PDCLs) responded distinctively to PLX4032 (also known as RG7204 or RO5185426) (94, 95). Both studies show that treatment response differed among BRAFV600E mutant cells. The diversity in sensitivity could be attributed to the additional co-occurring mutations contributing towards resistance (94) and/or differential expression levels of BRAFV600E (95). Another study compared the sensitivity of A375 and WM115 melanoma cell lines to siRNA-mediated downregulation of BRAFV600E. WM115 are less sensitive to suppressed BRAFV600E expression compared to A375 cells, but they were sensitive to PI3K inhibition. In both models dual inhibition of BRAFV600E and PI3K signaling is more efficient in targeting melanoma cells than monotherapies (96). Altogether these results suggest that because of intra-tumoral heterogeneity, determining therapy solely on BRAF genomic status or single biomarkers seems insufficient. By these means, personalized approaches and rationally designing patient-specific therapies based on molecular signaling alterations evolving during disease progression might grant higher selectivity and longer-lasting effects. One great example of integration of network-based modeling and preclinical research is a study which set out to explore protein datasets (accessed at the Human Protein Atlas) of 353 BRAFV600E and BRAF wildtype, and 372 thyroid carcinoma which were then used to identify patient-specific, co-expressed groups of onco-proteins in each tumor. These onco-proteins constitute a functional and targetable signaling network and a single tumor can harbor several distinct groups of signaling networks. Targeting all distinct “unbalanced” networks is necessary to disintegrate the altered signaling flux in the tumor. Once the 725 tumor samples were mapped based on the altered signaling pattern, Vasudevan et al. showed that their dataset is constituted of 138 different types of tumors, instead of just four (BRAFV600E and BRAF wildtype and melanoma and thyroid cancer). They also showed that signaling patterns are distinct in some BRAFV600E cancers, and that there are communalities with BRAF wildtype melanomas, which collectively confirmed that BRAF genomic status might be insufficient in assigning therapy. These findings were validated in melanoma cell lines (G361, A375 and A2058), which activate distinct signaling networks and required three different drug combinations to eliminate cell growth, despite carrying an identical BRAFV600E mutation. As such, the predicted drug combinations were more efficient in tumor cell eradication, rather than monotherapies or dabrafenib + trametinib, often prescribed in the clinic (97).
Encouragingly, innovative approaches to study therapy resistance in cancer are rapidly emerging, including multi-omics approaches and computational modeling (98), and high-throughput CRISPR screening (99, 100). As such, targeted-exome, single-cell DNA and RNA-sequencing, ATAC- and ChiP-sequencing facilitated biological characterization, biomarker discovery and target identification among various cancer-treatment entities (98). Indeed, the activation of resistance mechanisms, as well as cell-cell interactions occurring in heterogeneous tumor samples/models can be mined by longitudinal sampling accompanied with classical or spatial multi-omics (101). However, the generation of such multidimensional, computationally heavy datasets requires competent teams of bioinformaticians and engines capable of dealing with complex molecular and pathological cues. Currently, there are three bioinformatic approaches available for computational resistance modeling. These include machine learning, network, gene co-expression modeling (network-based) and genome-scale metabolic modeling. Network-based modeling can identify gene co-expression patterns and mechanisms related to resistance. In addition, weighted network analysis indicates the level of significance of the co-expression link between gene pairs. Machine learning methods have a broader applicability regarding the type of datasets. Drug susceptibility and resistance can be predicted by logistic regression, random forest, and deep neural networks. Finally, genome-scale metabolic modeling informs on gene-protein interactions, to ultimately portray the global metabolic dynamics (98). In combination with the CRIPSR screening that enables unbiased evaluation of gene function by manipulating the target genes with CRISPR-Cas9 approach, various molecular mechanisms that confer drug resistance could be revealed (100).
A multi-centric study of BRAF inhibitor-resistant melanoma tissue samples, analyzed after disease progression, most detected secondary genomic alterations in the MAPK/ERK signaling pathway, BRAF copy number gains and BRAF alternative splicing, as mechanistic drivers of resistance. However, ~40% of samples did not provide a clear genetic cause of resistance (102). This set out research into unknown drivers and putative resistance mechanisms in preclinical models. In vitro models revealed secondary genetic resistance mechanisms, similarly, observed in patient tissue, whereby RTK expression was increased, through MAPK/ERK and/or PI3K/Akt signaling (Table 2).
In an in vitro study of resistance, a Luria – Delbrück fluctuation analysis was applied to generate two models of resistance propagated from single-cells, derived from PDCLs (WM989, WM983B): the first resistance condition was represented by a genetic “mutation”, heritable model, which harbors intrinsic capability to tolerate acute doses of vemurafenib (at 1μM). The second is a transient, non-heritable model, for which the resistant state is reversible and is achieved when a subpopulation of primed pre-resistant cells give rise to resistant colonies upon drug treatment, either through survival of resistant cell subpopulations, or epigenetic reprogramming of non-resistant cells. In addition, acquisition of secondary mutations can happen upon which the resistant state becomes irreversible (71). In both cases single cells were isolated, in order to minimize the effect of genetic heterogeneity and expanded for 7-8 divisions (generating approximately one million cells), after which the drug was added and colonies were counted. Thus, a large number of resistant colonies was detected in the heritable (genetic) model. In contrast, in the non-heritable model, there remained a high probability that any pre-resistant cell would result in a resistant colony. Untreated, intrinsically resistant colonies expressed high levels of WNT5A, AXL, EGFR, PDGFRb, APCDD, and JUN.
To examine temporal aspects of epigenetic resistance programs, cells were treated with one 1μM vemurafenib for four weeks, which resulted in an irreversible drug resistant state (71). After one week of vemurafenib treatment, pre-resistant cells expressed only a fraction of resistant genes (AXL, EGFR and NGFR) and after four weeks of treatment cells were fully reprogrammed and expressed the entire panel of resistance genes. ATAC-sequencing of transient cells uncovered broad cellular reprogramming by gain (TEAD, Jun/AP1) and losses (SOX10) of transcription factor occupancy. Once fully resistant, drug “holidays” (intermittent drug treatment) did not affect the resistant phenotype (71). In conclusion, epigenetic changes are sufficient to induce stable resistance to molecular targeted therapy.
Adding vemurafenib at progressively increased concentrations generated resistant sub-lines of M229, M249 and M238, through upregulated PDGFR-B and N-RAS (72). Interestingly, another study showed that vemurafenib-resistant cells develop in vivo and in vitro “drug addiction”, meaning that cells do not proliferate in the absence of the drug. In two PDX models, resistance was established 100 days after continuous administration of vemurafenib, while no resistant sub-clones emerged in the intermittently treated mice. Furthermore, cells derived from the resistant PDX models did not grow in vitro, unless the cell culture media was supplemented with small doses of vemurafenib (74). Similar observations were made in other in vitro studies of BRAFV600E-expressing cell lines (M288, SK-MEL28 and M14). Here, in absence of secondary mutations in NRAS, BRAF, KRAS, HRAS and MEK1, resistance emerged through BRAF amplification (74). These results suggest that dose and treatment schedule modulate therapy responses in patients.
Finally, the era of immunotherapy raised the question of the efficacy of combined BRAFi and immunomodulatory treatments in melanoma. This in vitro trial showed that vemurafenib-resistant sublines of M29 and M238 are cross-resistant to MART CTL and NK-mediated cell killing, pointing to intersecting apoptotic networks involving EGFR, PDGFRa, c-KIT, Met and IGFRb. In addition, researchers generated F5 CTL-resistant melanoma sub-lines through serial exposure to TCR-transgenic CD8+ T cells and found them to be cross-resistant to BRAFi. Interestingly, pretreatment of the resistant (vemurafenib and CTL) clones with HDACi (suberoylanidilide hydroxamic acid, SAHA) for 48 hours reversed their resistance to F5 CTL and NK-killing, but not to BRAFi. SAHA sensitized the cells by increasing the expression levels of proapoptotic regulators, caspases, TNF/TNFR family and death domain proteins (TNFSF10, TNFRSF10B, 11B), and reduction of antiapoptotic signaling (73). These in vitro data suggest that HDACi might overcome acquired dual resistance and immunosensitize BRAFV600E mutated cells to CTL and NK-killing.
In addition to melanoma, colorectal cancer (CRC) cell lines are also used as representative BRAFV600E disease models. In contrast to BRAFV600E melanoma cell lines, CRC cell lines present higher activity of PI3K/AKT pathway and lower levels of phosphorylated MEK, ERK, RSK, cyclin-D1 and Myc (76). Upregulation of ERK/MAPK signaling pathway is a driver mechanism of CRC, whereby cancer progression is warranted by constitutive activation of RAS and BRAF. Driven by the successful inhibition of BRAFV600E in melanoma, dabrafenib and vemurafenib were approved drugs for CRC treatment. However, ERK rebound in CRC is secured mainly by EGFR activation. Although, CRCs are largely inert to BRAFi monotherapy still, combined RAF and EGFR inhibition is shown to have some clinical benefit (78). However, even dual inhibition of RAF/EGFR in CRC can be overcome by wild-type NRAS amplification, which has been identified as a unique adaptive mechanism in CRC, but not in melanoma (78).
Resistant in vitro models commonly established from Colo205, HT-29, VACO432 and RKO cell lines and patient-derived xenografts of progression samples were shown to be sensitive to combined treatment of BRAFi and AKTi (PLX4720/vemurafenib and MK-2206) (76, 77). Tumor regression was also achieved by combining BRAF inhibitor BGB659 with EGFR inhibitor cetuximab in RAS - amplified, vemurafenib - resistant models (78).
2.4.2 CNS tumors
We are only beginning to understand resistance mechanisms in CNS tumors (103), mainly due to the scarcity of resistance models. In a study of BRAFi cellular resistance, we have treated mice carrying BRAFV600E-mutated flank xenografts with BRAFi tool compound PLX4720 for almost a year. Cell isolates from these PDX have shown increased levels of CD133 and Nestin, which suggests upregulation of stem-like cells, but molecular mechanisms of resistance have not been analyzed (82). Patient-matched pairs of cells from before and after treatment are rarely obtained, but are useful tools for studying therapy resistance.
A novel promising therapeutic strategy emerged from using isogenic BRAF inhibitor sensitive (794, AM38, BT40) and resistant (794R, AM38R, BT76) cell lines and treating them with combinations of BRAF inhibitor (vemurafenib) and autophagy inhibitor (chloroquine). Importantly, clinical data support the use of autophagy inhibitors to overcome resistance to BRAF/MEK inhibition, suggesting successful translation of this approach. This study is impactful because it used several distinct models for BRAFV600E-mutated gliomas, including established cancer cell line AM38 as well as patient-derived cell lines (794, B78), a patient-derived xenograft (BT40), as well as patient-derived slice cultures (60) (Table 1). This study is an example of preclinical data derived from multiple models which were followed by rapid clinical responses in BRAF-inhibitor resistant patients. Consistent preclinical data from multiple models are thought to be of higher predictive value than those obtained from a single model, due to model-specific bias.
Another study used patient-derived glioma tissue from paired pre-/post- BRAF/MEK inhibitor treatment to identify treatment-related changes in gene alterations and expression using RNA and DNA sequencing. They performed functional validation of candidates and found that resistance mechanisms are varied and highly PDCL-dependent, further underscoring the importance of using multiple models for functional studies (104). Yet another study generated patient-derived cell lines and xenografts from primary tumors (YMG62P, YMG89P, NGT41P) and recurrent samples (cells collected from CSF: YMG62R, YMG89R, NGT41R) from patients relapsing after dabrafenib+trametinib treatment. Here, refractory cells showed sensitivity to HSP90 in combination to dabrafenib or trametinib treatment (67). Moreover, we have recently isolated tumor cells from a patient with BRAFV600E-mutated high-grade glioma, pre-/post- BRAF+MEK inhibitor combination therapy. We found that post-treatment samples exhibit resistance to BRAF/MEK inhibition, which is accompanied by elevated expression of markers associated with cancer stem cells, differentiation, and chemo- and radiation-therapy resistance, including CD133, in the post-treatment tumor (3).
Given the rarity of such patient-matched samples from before treatment and after treatment when resistance has formed, alternative approaches are needed, such as forcing cells in vitro to acquire resistance using chemical approaches. Chronic treatment with escalating doses of the chosen therapeutic agent or acute, constant doses of the inhibitor until refractory subclones overpopulate the cell culture are useful approaches to induce resistance (Figure 2). As such, adaptive, functional mutations were identified in vemurafenib resistant cell lines (AM38, MAF-794) (103). Moreover, CRISPR-mediated genome editing can be applied to studying drug resistance (intrinsic and acquired), through functional screens and candidate approaches (100).
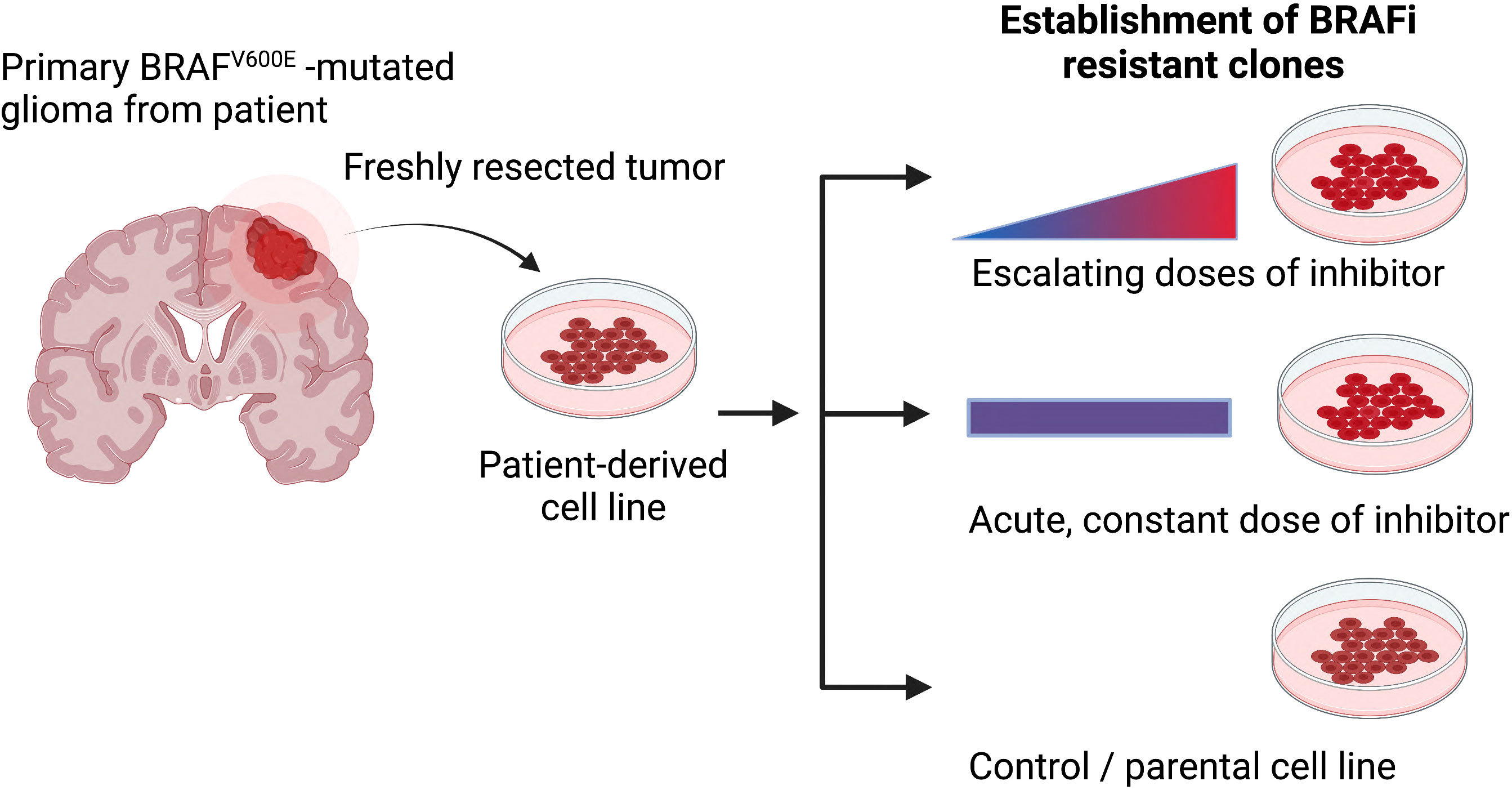
Figure 2 The most common methods for generating drug-resistant cancer cell lines. Primary patient-derived cell lines are treated either with escalating or constant doses of the relevant inhibitor over an extended period of time. Secondary, resistance associated mechanisms can be evaluated when comparing resistance and parental models (Figure created with BioRender.com).
In conclusion, validation of actionable molecules in resistant cells and preclinical studies of novel drug combinations that target these molecules using high-quality platforms are expected to overcome clinical limitations of BRAF/MEK inhibitors. Preclinical models provide unparalleled insights into intrinsic and acquired mechanisms of BRAF/MEK inhibitor resistance and models can be generated under various experimental conditions.
2.5 Patient-derived xenograft (PDX)-derived cell lines
Certain types of CNS tumors, including low-grade glioma, have a high failure rate for developing into patient-derived cell lines. Implanting surgical tissue directly into mice to generate patient-derived xenografts (PDX) and derive cell lines from PDX tissue is a valid alternative approach to culturing cell lines from surgical tissue directly. The PDX-to-cell line approach was used to generate a heterozygous BRAFV600E-mutated cell line BT-40 (63). BT-40 cells exhibited sensitivity to MAPK inhibitors selumetinib and trametinib (64, 105). A MAPK inhibitor library screen against BT-40 expressing a luciferase-based MAPK reporter assay revealed distinct sensitivities to single inhibitors, including next-generation RAF inhibitors, and synergistic effects of different inhibitor classes (106). BT-40 was also used to assess the clinical potential of ulixertinib, a first-in-class ERK inhibitor, and to test combinatorial effects with MEK inhibitors or BH3-mimetics (107).
However, it is unclear how well the low-grade parental tumor phenotype is preserved in BT-40 cells since they have been cultured in serum after passaging them as PDX. An additional caveat of patient-derived orthotopic xenografts (PDoX)-derived cell lines is potential contamination with mouse cells since they are hosted in mice brains; However, the BT-40 cell line had identical short tandem repeat analyses to the parental tumor and was free from mouse cells, as determined by LDH isozyme analyses. Whether BT-40 exhibits a gain of chromosome 7q identical to the xenograft has not been determined (105). In conclusion, PDX serve as an alternative source for cell lines and their ability to recapitulate the parental tumor needs to be monitored as it might change over time.
2.6 Limitations of existing in vitro models
There are clear limitations with existing models, the most prevalent limitation being that most of these models represent high-grade CNS tumors with combined BRAFV600E expression and CDKN2A deletion; progression models that recapitulate progression from low grade to high grade tumors are not available. Combinations of BRAFV600E with other rarer coinciding alterations are rarely represented. CNS tumors display marked inter-tumoral heterogeneity, which emphasizes the need for using multiple cell lines for each tumor class. Unfortunately, there are not enough cell lines available to the community to capture representative patient-to-patient differences. Moreover, despite the relative frequency and poor clinical outcome of BRAFV600E mutant-brain metastases, there is only one published genetically engineered model for BRAFV600E melanoma metastatic to the brain (53, 108), which severely hinders studies of the mechanisms underlying the metastatic process.
3 In vivo modeling
3.1 In vivo models for BRAFV600E mutated neoplasms
To study the precise role of BRAFV600E mutation in gliomagenesis in vivo, various mouse models of BRAFV600E-mutated glioma have been developed to better understand the complexity of tumor biology. Transgenic or genetically engineered mouse models (GEMMs), syngeneic models, patient-derived xenografts (PDX) in immunocompromised mice, and virus-induced models are commonly used to recapitulate certain biological features of primary patient BRAF-mutated tumors (Table 3). Although these models provide a valuable resource for studying how BRAFV600E is involved in tumor development and recurrence following therapy, each model comes with its own benefits and limitations that need to be carefully considered.
3.2 Patient-derived xenograft models
These models involve the implantation of tissue into the flank and dissociated cells into the brain to obtain orthotopic xenografts (PDoX). Although the growth of PDoX is harder to follow due to their inaccessibility in the brain, they provide a more faithful representation of the tumor microenvironment and invasiveness of CNS tumors than flank tumors. Severe combined immunodeficient (SCID), non-obese/diabetic (NOD)/SCID, athymic nude, and NSG mice are typical hosts for PDX development, due to their impaired immune systems, which facilitates achieving graft survival without risk of short-term rejection. Although these models can mimic certain features of the original human tumors, such as genetic and molecular heterogeneity, the biological aspect of tumor initiation and growth cannot be studied. Moreover, they do not fully capture the immune system’s role in controlling glioma growth and progression, deeming them unsuitable for studying tumor immunology and evaluating immunotherapy response.
Establishing PDX models for BRAFV600E-mutated gliomas can also be time-consuming with a low success rate of engraftment (109) depending on the availability of patient tumor tissue with several factors taken into consideration, such as how the tissue is collected and stored. Several PDX biobanks have been established by individual groups and institutions, including PDX panels from GBM (117), and some specifically for pediatric brain tumors (118, 119). BRAF missense mutations have been identified in these PDX by molecular profiling, but due to the relative scarcity of BRAFV600E altered CNS tumors amongst brain tumor patients, PDX for these tumors are rare. A PDX biobank specifically focused on BRAFV600E-mutated gliomas is currently lacking due to the scarcity of patient material, and the difficulty in retrieving complete patients’ clinical data, pathologies, gene expression profiles, and drug responsiveness (64). However, there is great potential for this to be an authoritative resource for developing new treatments and personalized medicine approaches.
3.3 Syngeneic murine models
These models are created by implanting an established tumor cell line derived from the same species as the host animal. The 2341 cell line of BRAFV600E-mutated and CDKN2A-deleted glioma injected into both immunodeficient (NSG) and immunocompetent (FVB/N) mice have revealed successful engraftment and has been critical for the preclinical assessment of experimental therapeutics (57). The use of syngeneic models of BRAFV600E-mutated glioma enables the study of the immune microenvironment associated with the tumor, and the testing of immunotherapies (3). However, syngeneic models may have limited translational potential since they do not fully recapitulate the biological and clinical features of human gliomas. Species-dependent differences in tumor microenvironment, immune response, tumor heterogeneity and therapeutic response are important factors that need to be taken into account. Multiple models generated from syngeneic mouse tumor lines for a particular type of glioma such as glioblastomas could also be variable in immune phenotypes among tumors, translating these findings into effective immunotherapeutic strategies for human glioma patients could be complex and warrants further research and validation (120). In addition, these models may exhibit inconsistent tumor growth rates, due to a functional immune response in host mice, which may affect the reliability of experimental results and require a large study cohort.
3.4 Inducible modeling systems
Knock-in GEMMs for BRAFV600E-mutated gliomas are alternative models that involve conditional and inducible systems such as the Cre-loxP system to allow the expression of BRAFV600E under the control of the endogenous promoter and enhancer sequences in a cell-specific or time-specific manner upon the activation of Cre recombinase (57, 121), which can be induced by delivering either tamoxifen or Cre-expressing viruses such as adeno-associated viruses and adenoviruses (22, 111). The use of the inducible Cre-loxP system combined with knock-in GEMMs has provided valuable tools for understanding the effects of BRAFV600E mutation in tumor progression in the context of an intact immune system and microenvironment, and for developing potential therapies that specifically target these mutations.
Another type of GEMM for BRAFV600E-mutated gliomas is the replication-competent avian sarcoma-leukosis retrovirus and the corresponding avian leukosis virus A (RCAS-TVA) system, which allows for somatic gene delivery to cells that are engineered to express cell surface receptor TVA using a cell type-specific promoter such as Nestin, which is a common marker for neural stem cells (112, 113). This enables investigation of the tumor cell of origin after BRAFV600E mutation, as well as the role of BRAF in glioma development.
Transposon-based GEMMs have been developed for studying glioma development. These models include the Sleeping Beauty transposon system and the PiggyBac transposon system by which a transposon containing a mutation is integrated into the genomes of targeted cells of interest, resulting in the development of malignant tumors in the brain (122, 123). Specifically, BRAFV600E mutation in neural progenitor cells induced by the binary PiggyBac transposase system has revealed the formation of GG-like tumors only in concert with the activation of Akt/mTOR signaling (115), as well as a hyperexcitable phenotype in neocortical pyramidal neurons with increased neuronal firing frequencies (114). This is also supported by another study showing a causal relationship between BRAFV600E mutations and epileptic seizures, which are often associated with GG and PXA (111) (Table 3).
3.5 Challenges of modeling BRAFV600E mutated gliomas in vivo
The inter-tumoral heterogeneity of BRAFV600E mutated gliomas provides an additional modeling challenge. PXA express reticulin fibers and are therefore thought to originate from transformed subpial fibrous astrocytes (124), whereas GG consisting of mixed neuronal and glial components points to a neural stem cell origin. Since there is an ongoing debate over the cell of origin for gliomas with Nestin-expressing neural stem and progenitor cells (NSCs) and oligodendrocyte progenitor cells (OPCs) being the two major candidates to transform into tumor cells and to shape tumor heterogeneity, it is important to characterize the relative contribution of each population to glioma initiation and progression in the context of BRAFV600E mutation.
Moreover, no studies have yet been conducted to determine the OPC nature of BRAF-mutated tumors using either the RCAS-TVA system or the Cre-lox system with OPC-specific promoters such as PDGFRα or NG2 (Table 3). This is particularly important since BRAFV600E expression in Ink4a/Arf-deleted cells has been shown to increase the proportion of proliferative glial progenitor cells in adult mice in vivo, disrupt asymmetric cell division of OPCs, and increase OPC frequency in vitro at the expense of mature oligodendrocytes and astrocytes, respectively, without altering NSC frequency (3). These data suggest that OPCs are more sensitive to cellular transformation by concurrent BRAFV600E expression and Ink4a/Arf loss than NSCs. These two alterations have yet to be combined in transgenic mouse models with OPC type-specific expression, to address whether OPCs can indeed be the origin of BRAFV600E mutated tumors in vivo. Exploring whether BRAFV600E mutation can also occur in mature astrocytes or oligodendrocytes that de-differentiate into stem or progenitor cells with tumorigenic potential would be intriguing to explore, since both astrocytes and neurons have been shown to function as the cells of origin for gliomas via dedifferentiation (125).
Nevertheless, the cellular origin of BRAF-mutated gliomas remains underappreciated, and further studies are required to understand the cellular and molecular mechanisms underlying the development of these tumors. Distinct cellular origins are just one potential explanation for the phenotypic diversity of BRAFV600E-mutated tumors; alternative explanations such as microenvironmental diversity, and distinct neurodevelopmental origin amongst the tumors with distinct age presentation are important underlying factors to be considered.
4 Challenges for establishing high-fidelity BRAFV600E tumor models
4.1 The challenges of developing models for low-grade gliomas
Establishing robust in vitro models that are representative of low-grade CNS tumors, incuding BRAFV600E -mutated CNS tumors is inherently challenging. Low grade tumors are notoriously difficult to model in vitro and in vivo, likely due to tumor cell differentiation states, slow growth senescence, and microenvironmental signals in culture media that favor outgrowth of normal brain cells. Indeed, certain copy number alterations have been associated with robust in vitro growth, including gain of chromosome 7p, and loss of chromosome 10q. Loss of PTEN (126) and CDKN2A loci (127) have been positively associated with the establishment of PDCLs. While high-grade tumors tend to have a higher frequency of establishing cell lines, it is challenging to maintain the phenotypic intra-tumoral heterogeneity that is so characteristic of high-grade CNS tumors; in addition, certain genetic alterations (ATRX, IDH1, and hTert) tend to get lost in cultured cells presumably due to negative selective pressure from culture conditions (128). Recent studies have successfully approached generating adult LGG marked by IDH1 alterations by picking slow-growing tumor cells and separating them from fast-growing normal fibroblasts, that typically outgrow from mixed patient-derived cultures (127). Moreover, patient-derived organoid cultures produced faithful in vitro models for adult IDH1-mutated LGG (129). Most in vitro BRAFV600E-mutated CNS tumor models created thus far are high-grade models, including 2341, AM38, DBTRG-M05, STN-10049. Encouragingly, some models for LGG are also reported, including BT-40 and IC3635-PXA (see Tables 1, 3). Care must be taken that these models retain their low-grade features mentioned above since it is well-known that tumor cells can spontaneously become more aggressive when passaged in vitro over time.
In conclusion, attempts to generate in vitro models for low-grade CNS tumors have been hampered by negative selection against tumor cells in culture and phenotypic drift to a high-grade tumor over time. Recent studies from adult tumor types have provided new guidance for generating urgently needed robust in vitro models of pediatric CNS tumors in general and BRAFV600E pLGG in particular.
4.2 Developing models for tumor progression from low to high grade is challenging
Adult LGG commonly evolve into higher grade tumors, while malignant transformation occurs less frequently in pLGG, with BRAFV600E-mutated pLGG being an important exception (5, 18, 20, 27). Most in vitro and in vivo models in the field of neuro-oncology are for HGG, and genetically engineered mouse models recapitulating BRAFV600E-mutated LGG and their progression to high-grade are lacking, due to the slow-growing nature of these tumors. Thus, the biological mechanisms underlying the onset of LGG formation remains poorly understood and molecular and cellular mechanisms for progression to a more aggressive clinical course of BRAFV600E-mutated gliomas are unknown. To create an ideal preclinical model for BRAFV600E-mutated LGG that represents the complex and dynamic tumor microenvironment of malignant transformation, the grading and classification of gliomas need to be recapitulated as closely as possible in animal models. As indicated in Figure 3, the WHO grading system consisting of cellular density, nuclear atypia, mitosis, necrosis, and endothelial proliferation is commonly used to evaluate malignancy based on the tumor’s histopathological appearance with high grade tumors having more aggressive microscopic features than low grade tumors (130). High grade tumors often have increased nuclear pleomorphism with variability in shape and size, as well as elongated, large, and hyperchromatic nuclei. They also have increased cellularity, mitotic activity, necrosis, and vascular hyperproliferation. High grade tumors have a higher Ki67 proliferation index indicative of more aggressive tumor growth and they tend to exhibit high immunoreactivity for stem cell markers such as CD133, Nestin, Sox2 and Olig2. However, functional roles and contribution to tumor behavior during progression and treatment response of stem cells in LGG are still being investigated, and therefore the clinical significance of stem cell marker expression in glioma progression remains unclear. Asymmetric divisions of glioma cells regulate adult tumor cell fate and perpetuate a stem-like phenotype and contribute to tumor expansion (82, 131, 132). Regulation of asymmetric division in LGG has yet to be determined.
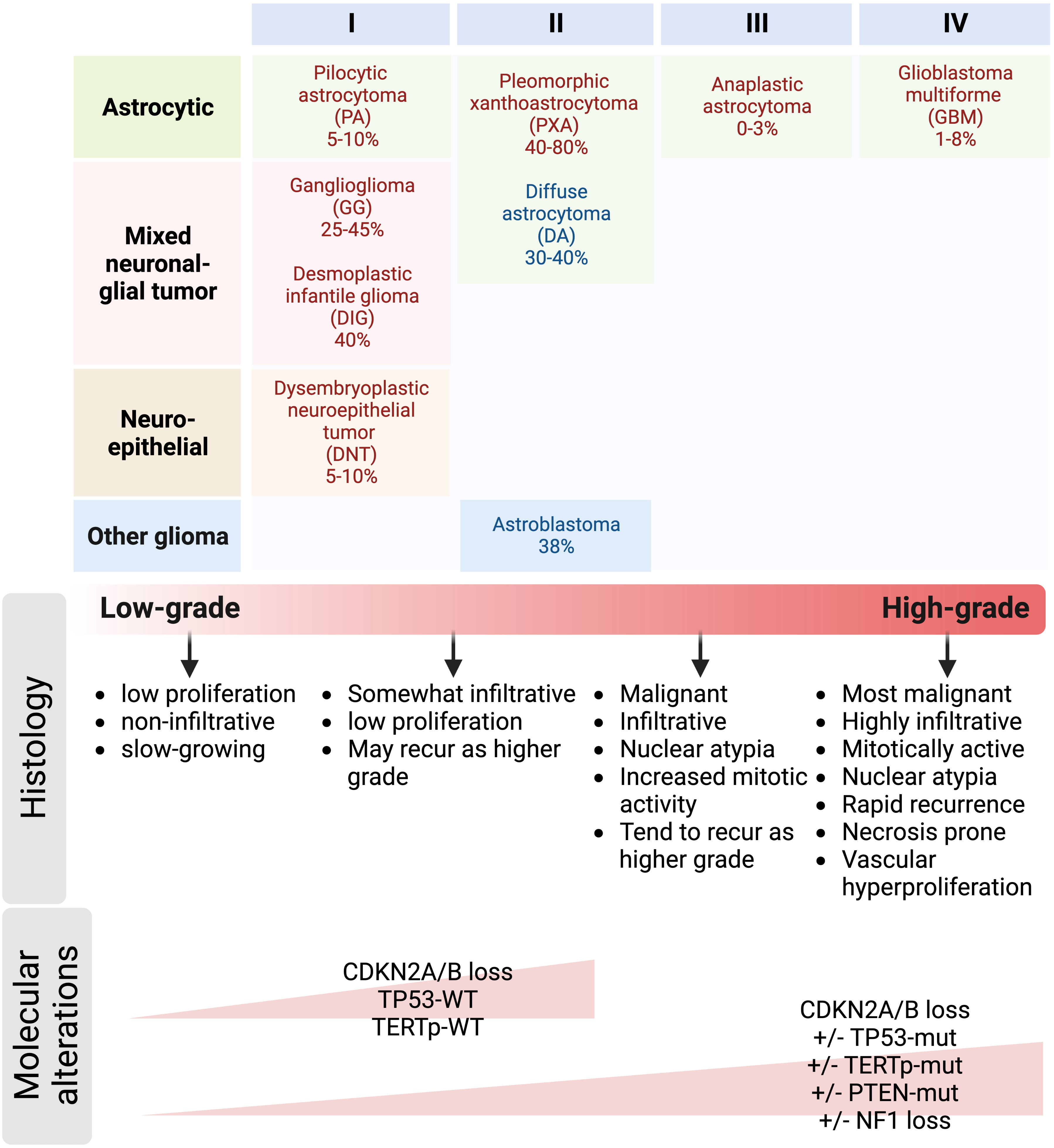
Figure 3 Prevalence of BRAFV600E mutation in pediatric and adult gliomas. Gliomas are classified into tumor subtypes and WHO grades based on both histological and molecular features. Grade 1 and 2 gliomas are often referred to as low-grade gliomas, whereas grade 3 and 4 gliomas are referred to as high-grade and malignant gliomas. Histological characteristics such as nuclear atypia, mitotic activity, necrosis, and endothelial proliferation are often used to differentiate low-grade from high-grade tumors. Key molecular alterations co-occurring with BRAFV600E mutation include CDKN2A/B deletion, mutations in TP53, TERT gene promoter, and PTEN; and NF1 loss can also help distinguish low-grade from high-grade gliomas.
To further improve the classification of gliomas due to the limitations of the histological grading system such as subjectivity with inter-observer variabilities and intra-tumor heterogeneity (133), molecular markers are often integrated into a more clinically relevant grading scheme. Some alterations such as CDKN2A/B loss, PTEN, EGFR, NF1, and TERT promoter mutations have been shown to be associated with very poor clinical outcomes in patients with BRAF-altered gliomas (17). However, some studies reported conflicting results regarding the prognostic value of some molecular markers (133), emphasizing the importance of understanding the molecular mechanisms underlying glioma progression. This poses another challenge in generating preclinical models of LGG due to the difficulty in recapitulating the widespread genomic and epigenomic effects of BRAF alterations. Despite the mechanistic challenges in creating an ideal mouse model of BRAFV600E-mutated LGG, it is feasible using the genetic engineering techniques with the following factors considered: 1) cell type-specific BRAFV600E mutation induced by either Cre-loxP or RCAS-TVA system, 2) selection of the appropriate genetic background of the mouse which can influence the development and progression of tumors, 3) monitoring of tumor progression by MRI to detect the presence and growth of tumors, 4) validation of the model by comparing its histopathological and molecular characteristics to human BRAF-mutated LGG, and 5) the validated mouse model can be used for preclinical research to test drugs to better understand the underlying biological mechanisms of BRAF-mutated LGG and this will pave the way for the identification of therapeutic targets to block tumor progression.
In conclusion, LGG models are needed to better understand the role for stem cells and asymmetric division in tumor formation and progression. Due to the complexity of generating a mouse model that accurately recapitulates the genetic background, intra-tumoral heterogeneity, and tumor microenvironment that all closely resemble those of human tumors, multiple models may need to be generated and evaluated before an ideal model is established.
4.3 Loss of oncogenic alterations in vitro
Recurring oncogenic alterations are difficult to maintain in vitro, including IDH1 mutations, which are reportedly frequently lost in PDCLs grown under standard serum-containing conditions (134). One study recently reported successfully generating several IDH1 mutated glioma cell lines by growing them under serum-free conditions and selecting cells manually based on their tumor cell morphology and separating them physically from the non-tumor associated cells that are IDH-wildtype (127). Such labor-intensive approaches might not be necessary when attempting to culture BRAFV600E-mutated PDCLs, since BRAFV600E expression was maintained in culture and upon xenografting in vivo, suggesting that cells remain dependent on activated MAPK pathway signaling (3). Notably, one study reports an increase in allelic frequency (from 28% in the patient tumor) to 70% in PDoX, and up to 69% in PDoX-derived cell lines, indicating that BRAFV600E plays an important role in tumor progression (109). In conclusion, BRAFV600E expression might be required for tumor cell growth and therefore maintained in culture. Whether BRAFV600E expression provides a selective disadvantage or advantage in cultured cells has yet to be systematically explored.
4.4 Modeling an intact immune system and microenvironment
4.4.1 Syngeneic and humanized mouse models
Given the key physiological and pathological processes in mice differ substantially from those in humans and most of the traditional mouse models are immunocompromised, humanized mouse models engineered to carry human genes, cells or tissues (usually patient-derived or human cell line-derived xenografts) have been developed, and especially those mice with functional human immune components are an attractive alternative for understanding human cancer immunology and for testing immunotherapies, therefore leveraging their value in translation research. These humanized mouse models involve stable engraftment of either human CD34+ hematopoietic stem cells that produce multi-lineage human immune cells or human peripheral blood monocyte cells, and when combined with orthotopic implantation of patient-derived or human cell line-derived xenografts, human-specific biological processes during tumorigenesis in an immunological context can be studied. In addition, knock-in humanized mouse models are also available to better understand the anti-tumor response of immune checkpoint inhibitors (ICIs) targeting human-specific molecules in fully immunocompetent mice.
For example, since the combination of BRAF and MEK inhibition with immune checkpoint blockade by anti-PD-L1 and anti-CTLA4 treatment exhibited clinical benefit in mice implanted with BRAFV600E-mutated high-grade gliomas (3), the generation and characterization of knock-in humanized mouse models that express both the BRAFV600E mutation and human PD-1 and CTLA-4 molecules would allow researchers to test the efficacy of combination therapies targeting both the BRAFV600E mutation and immune checkpoint molecules in a more human-like setting. Indeed, the double-humanized PD-1 and CLTA-4 knock-in mouse model has been validated (135); in conjunction with mouse models of BRAFV600E mutated glioma, this enables the studies of molecular and immunological mechanisms of ICI response to glioma, as well as the studies of tumor-immune interactions.
5 A critical comparison of existing mouse models of BRAFV600E-mutated glioma
Existing mouse models of BRAFV600E-mutated glioma in the literature are summarized in Table 1. Different mouse models have shown varying median survival times, ranging from 45-170 days (Table 3) (22, 109). This suggests that the effects of BRAFV600E expression are modulated by additional factors that impact tumor formation.
With forced full-length expression of BRAFV600E using either RCAS virus or the Cre/Lox system, respectively, robust tumors were only formed when combined with other oncogenic mutations such as CDKN2A deletion, Akt overexpression, and/or p53 deficiency (22, 112, 115). Tumors with BRAFV600E expression and CDKN2A deficiency remained in an undifferentiated state with one study using the Cre/Lox system showing strong immunoreactivity for GFAP, Olig2, and Nestin, whereas in another study using the RCAS system, tumors with the same genetic mutations exhibited positive immunoreactivity for Nestin, but not GFAP and Olig2 (22, 112) (Table 3). In addition, the former study revealed that BRAFV600E CDKN2ANull tumors tend to proliferate and invade throughout the cerebral hemisphere and white matter tracts, while the latter study did not show evidence of diffuse infiltration of tumor cells. These histopathological differences could be explained by differences in expression levels, since the CRE/Lox-driven model drives BRAFV600E expression from the endogenous promoter, while the RCAS model uses an unphysiological promoter. Despite these differences both studies consistently demonstrated that BRAFV600E expression requires additional mutations to induce formation of high-grade lesions.
Importantly, overexpression of a truncated BRAF kinase domain carrying the V600E mutation (BRAF VE kin) but lacking the autoinhibitory domain in mice by itself can induce slow growth of tumors resembling human PA (113). This was attributed to the increased protein abundance of phosphorylated Erk in BRAF VE kin, resulting in stronger MAPK activation that was sufficient to promote tumorigenicity without further oncogenic mutations. Cases-Cunillera et al. later used this truncated BRAFV600E-containing kinase domain in the piggyBac transposon system to demonstrate that this truncated variant alone indeed induced tumors, but with oligodendroglial and PLNTY-like features.
In conclusion, the phenotypic spectrum of BRAFV600E-positive mouse CNS tumors is influenced by the type of mouse model used. A major modulating factor is the regulation of BRAFV600E expression and whether it is driven by an exogenous or the endogenous promoter, which presumably causes variance in expression levels of the mutant kinase leading to differential signaling strengths. The presence of the BRAF autoinhibitory domain and additional oncogenic mutation(s) and the presence or absence of negative regulators of BRAF (e.g., Sred), and negative feedback loops are additional potentially modulatory factors of tumor phenotypes (Table 3).
6 Enhancing mouse models of BRAFV600E mutated glioma for preclinical studies
Since mouse models of glioma provide an important platform for testing potential therapies and investigating pathological mechanisms underlying tumorigenesis, the usefulness of these models for preclinical studies can be further enhanced by combining them with a variety of advanced technologies and tools, which are briefly reviewed below.
6.1 Non-invasive imaging techniques
One such technology is non-invasive imaging techniques including magnetic resonance imaging (MRI) and bioluminescence imaging (BLI), which enable the visualization and monitoring of tumor growth and assessment of tumor response to treatments in mice. MRI allows the identification of tumor size, shape, location, and invasion into surrounding tissues, whereas easily accessible and commonly used BLI can only monitor the growth of tumor cells that have been engineered to express luciferase, an enzyme that produces light that can be measured throughout BLI when the luciferin substrate is given. However, in contrast to MRI, there are some limitations of BLI that need to be seriously considered: 1) it has limited penetration depth of light so tumor cells located deep in the brain could be hard to detect, 2) it has a limited dynamic range, making it difficult to accurately measure tumor growth changes; the bioluminescence signal measured from tumor cells can sometimes saturate at high cell densities at later stages, and 3) unreliable quantification of BLI signal due to variations in the expression of the luciferase reporter gene in tumor cells. Indeed, a recently published study has demonstrated that the discrepancy in the evaluation of tumor growth by BLI and MRI was attributed to the instability in luciferase expression in the viral construct (136). In contrast, MRI allows for more accurate detection and measurement of tumor size and location in the brain, enabling researchers to perform longitudinal studies, where the tumor growth and progression can be monitored by analyzing changes in the tumor size and shape over time. As a result, BLI should ideally be used in conjunction with MRI if feasible at several time points to obtain complementary information for the accurate interpretive analysis of tumor growth and response to therapy in preclinical studies.
In terms of the assessment of the response to BRAF/MEK inhibitor combination therapy, biochemical analyses for evaluating kinase inhibitor activity in the brain are a time-consuming process that often involves tissue dissection, which may lead to the disruption of the activity being measured. Novel kinase-modulated bioluminescence indicators enable noninvasive imaging of signaling strength and could help tracking the activity of target kinases in response to targeted drug treatment (137). Such approaches represent valuable tools for improving drug discovery against BRAFV600E mutated glioma and they might help with developing biomarkers which are urgently needed to stratify patients and identify those that will need additional treatments in addition to BRAFi+MEKi combination therapy.
6.2 Behavioral consequences of BRAFV600E mutated gliomagenesis
Accumulating evidence suggests that BRAFV600E-mutated gliomas are associated with an increased risk of epilepsy and seizure, which can have a range of behavioral consequences based on their frequency and severity. As indicated in Table 3, some studies using mouse models revealed that BRAFV600E mutation arising from progenitor cells during brain development leads to the acquisition of intrinsic epileptogenic properties in neuronal lineage cells and tumorigenic properties in glial lineage cells. This somatic mutation contributes to epileptogenesis by upregulating the expression of the RE1-silencing transcription factor which can repress a subset of genes coded for ion channels and receptors that are crucial to neuronal function (111, 138). It has also been demonstrated that BRAFV600E mutation in neural progenitor cells results in a hyperexcitable neuronal phenotype (114). Since the BRAFV600E mutation has been strongly linked to changes in neuronal excitability, it would be intriguing to determine whether there is a cognitive impairment as a result of glioma-associated epilepsy induced by BRAFV600E mutation. Nevertheless, further studies are required to better understand the relationship between BRAFV600E -mutated gliomas and epilepsy and the underlying mechanisms and potential therapeutic implications. Currently, behavioral assessment in mouse models of BRAFV600E-mutated glioma that involves testing of motor and cognitive functions is lacking and thus is important to explore. This will also validate whether these preclinical models can mimic human patients’ cognitive decline and epileptogenic properties.
6.3 Single-cell sequencing technologies
Intra-tumoral heterogeneity has been strongly implicated in affecting treatment response and conferring resistance in glioma with the underlying cellular and molecular mechanisms remaining fully unknown (139). Furthermore, the molecular responses of high versus low grade tumor cells carrying the BRAFV600E mutation to BRAF and MEK inhibition have not been investigated in detail and thus the differential responses in patients with LGG and HGG to these therapies are poorly understood (13). Recent advances in single-cell sequencing technology would help address these questions since it allows for the integrative analysis of the genome, transcriptome, epigenome, proteome, and/or metabolome-based characterization of the individual cell among cancer cells within a tumor. Single-cell transcriptomic analysis of A375 (BRAFV600E mutant) melanoma cell line described functional and phenotypic changes during development of drug resistance towards targeted therapy. Overall, four different treatment conditions were applied to the A375 cells (Vemurafenib monotherapy, V + Cobimetinib, V + trametinib, and untreated control). ScRNAseq revealed that the initial events of mono-drug resistance include loss of differentiated antigen presenting and neural crest-like cells and enrichment of undifferentiated, heterogeneous, highly proliferative stromal-like cells. Double-agent resistance featured variable amounts of dedifferentiated stromal cells because of heterogeneous effects of therapies on cellular states. In addition, specific survival machinery related to MAPK, p53 and PI3K/Akt reactivation and pluripotency induce distinctive cell populations (140).
Clonal barcoding is a powerful single-cell technique that allows researchers to track clonal evolution trajectories of tumor cells and to identify genetic changes and chromosomal aberrations that drive tumor growth and progression, or tumor cell resistance over time. A recently developed “CAPTURE” single-cell barcoding approach has been utilized in combination with an in-depth multi-omics analysis to reveal the clonal dynamics of BRAFV600E melanoma cells’ response to vemurafenib and identify novel targetable vulnerabilities of resistant clones such as oxidative phosphorylation and E2F pathways (141). This approach can be applied to study treatment resistance in the context of BRAFV600E mutated gliomas and will help uncover new mechanisms of tumor cell resistance in the brain. Another cell barcoding strategy was applied to study rare subpopulations, by cloning lentiviral library in which the barcode sequence is incorporated in the 3` untranslated region of the green fluorescence protein (GFP). Through sequencing, barcodes present in resistant samples were used to identify and trace rare, primed cells in the untreated samples. In this way, rare, drug-naive precursors were enriched by FACS and characterized and potential mechanisms of resistance were identified. Interestingly, shortly after drug treatment, these cells highly expressed phosphorylated ERK which confirmed earlier studies that BRAF inhibitor effects on MAPK signaling are only transient (142).
In conclusion, single-cell barcoding can be applied to study treatment resistance in the context of BRAFV600E mutated gliomas and once applied to the brain will help uncover new mechanisms of tumor cell resistance.
7 Future perspectives
Model organisms are important substitutes for human studies to facilitate and expedite translation of preclinical studies. Approaches below should be useful for generating next-generation mouse models that better recapitulate the hallmarks and diversity of human cancer (sex, age, race, grade, molecular and clinical features) including gliomas harbored with BRAFV600E mutation.
7.1 CRISPR-Cas9 gene editing
CRISPR-Cas9 gene editing technology is now used more often in combination with other systems for developing glioma mouse models (143). Oldrini et al. combined the CRISPR-Cas9 system with the RCAS-TVA system to generate more precisely targeted glioma mouse models for biological and preclinical research including the one for BRAF-mutated glioma by inducing gain-of-function BRAFV637E mutation (orthologous to human BRAFV600E) in NSCs (116). However, the CRISPR-Cas9 system suffers from a major limitation of off-target effects, which remain to be addressed.
7.2 3D organoid cultures
Recently, 3D organoid culture systems generated from induced pluripotent stem cells (iPSCs), human embryonic stem cells (hESCs), or patient-derived adult stem cells from cancerous tissues have been developed to better capture complex molecular and cellular heterogeneity. In the search for pre-clinical glioma models, remarkable advancements have been made in creating organoid models for gliomas, such as neoplastic cerebral organoids (neoCORs), GLICO, and patient-derived glioblastoma organoids (GBOs) (144–148). These models retain many key features of primary patient tumors, providing accurate models for studying cellular and molecular interactions among and within tumors and thus represent new advanced ex vivo models for disease modeling, omics analysis, genetic manipulation, and personalized drug screening. However, the lack of vasculature, stromal and immune cells in these organoids makes it difficult to study tumor-immune microenvironment interactions. Nonetheless, the exciting organoid technology holds tremendous potential to significantly change our understanding of BRAFV600E-mutated glioma tumor biology.
7.3 Hydrogels and 3D cell culture
Due to low engraftment rates and inconsistent tumor growth of PDX and syngeneic models, various hydrogels have been developed to improve the reliability of mouse models. Although Matrigel is commonly used as a gold-standard hydrogel for 3D cell culture and experimental models (149), batch-to-batch variability in the complex composition of Matrigel can lead to inconsistencies in experimental results, especially when comparing data between different studies using different batches of Matrigel. Therefore, alternative hydrogel scaffolds such as the biomimetic EKGel, tissue-derived extracellular matrix hydrogels, and alginate-based hydrogels have been developed to overcome these limitations (150, 151). Applying one of these advanced hydrogels for culturing BRAFV600E-mutated organoids or for encapsulating BRAFV600E-mutated tumor cells upon intracranial delivery can improve the consistency of models of BRAFV600E mutated gliomas.
8 Conclusions
Preclinical testing of novel therapeutic compounds rarely translates successfully to the clinic due to lack of reproducible cancer models (152). Many promising new anti-cancer treatments are available, especially immunotherapies, that have yet to be assessed preclinically against BRAFV600E mutated CNS malignancies owing to the few preclinical models available. Preclinical results need to be validated in more than one model of a specific tumor type to heighten their predictive capacity. As all the current and next generation in vitro, ex vivo, and in vivo mouse models have their own individual advantages and disadvantages, selecting the most appropriate model depends on the experimental hypotheses. In order to create models that represent the glioma subtypes found in patients harbored with BRAFV600E mutation, they must be rigorously characterized by genomic, transcriptomic, histopathological, and clinical analyses. Such detailed characterizations are necessary to reveal the level of congruency with the according human disease amongst the distinct models. By using different strategies in combination, clinically relevant mouse models that more closely reflect the complexity and histopathological and genetic diversity of BRAFV600E mutated gliomas can be established. It is important to recognize, however, that markers and features of human tumors are not going to be fully recapitulated in mouse models, due to the obvious differences amongst mice and mice, including in life span, metabolism and physiology, brain microenvironment, and immune responses. Although it has been shown that BRAFV600E alteration is retained in cancer models, molecular data of existing BRAFV600E-mutated glioma models are scarce. Thus, it is not known how well these models recapitulate certain pathways activated in human disease, an important question that needs to be addressed is to decide about the utility of each model for answering specific questions.
Although each model has its own distinct strengths and weaknesses, glioma modeling needs to be further refined to accurately mimic the complexity of tumor heterogeneity in human patients and to benefit future clinical translation. Future efforts need to be directed at comparing model organism responses using association analyses, machine learning, pathway enrichment, or meta-analyses, as well as at generating a larger repertoire of preclinical models of BRAFV600E-mutated CNS neoplasms that capture the diversity of BRAFV600E-mutated CNS neoplasms. The availability and accessibility of next-generation mouse models for BRAFV600E-mutated gliomas will have great potential to accelerate our understanding of glioma biology and the development of novel therapeutic strategies.
Author contributions
YLX wrote the manuscript, created the tables and the figures, and reviewed the final manuscript. DP wrote the manuscript, created the tables, and reviewed the final manuscript. CP conceived the content, wrote the manuscript, created the tables, reviewed and approved the final version of the manuscript. All authors contributed to the article and approved the submitted version.
Funding
BRAF LGG consortium research fund (CP), Stanford Cancer Institute-Goldman Sachs Foundation Cancer Research Fund (CP), Stanford Prevention Research Center (CP), National Institutes of Health (R21 NS099836; R01 CA201537; R01 NS080619; R01 NS0950985, R01 CA164746; U54 CA261717) (CP).
Acknowledgments
We thank Christine Plant for editing and submission of the manuscript. Figures were created with BioRender.com.
Conflict of interest
The authors declare that the research was conducted in the absence of any commercial or financial relationships that could be construed as a potential conflict of interest.
Publisher’s note
All claims expressed in this article are solely those of the authors and do not necessarily represent those of their affiliated organizations, or those of the publisher, the editors and the reviewers. Any product that may be evaluated in this article, or claim that may be made by its manufacturer, is not guaranteed or endorsed by the publisher.
References
1. Wan PT, Garnett MJ, Roe SM, Lee S, Niculescu-Duvaz D, Good VM, et al. Mechanism of activation of the RAF-ERK signaling pathway by oncogenic mutations of B-RAF. Cell (2004) 116(6):855–67. doi: 10.1016/s0092-8674(04)00215-6
2. Li S, Mattar P, Dixit R, Lawn SO, Wilkinson G, Kinch C, et al. RAS/ERK signaling controls proneural genetic programs in cortical development and gliomagenesis. J Neurosci (2014) 34(6):2169–90. doi: 10.1523/JNEUROSCI.4077-13.2014
3. Park J-W, Grossauer S, Wang W, Xing YL, Koeck K, Garcia CA, et al. Clinically relevant concurrent BRAF and MEK inhibition alters differentiation states and sensitizes BRAF V600E-mutated high-grade gliomas to immune checkpoint blockade. bioRxiv (2023) 2002:526065. doi: 10.1101/2023.02.03.526065
4. Mackay A, Burford A, Carvalho D, Izquierdo E, Fazal-Salom J, Taylor KR, et al. Integrated molecular meta-Analysis of 1,000 pediatric high-Grade and diffuse intrinsic pontine glioma. Cancer Cell (2017) 32(4):520–537.e525. doi: 10.1016/j.ccell.2017.08.017
5. Ryall S, Zapotocky M, Fukuoka K, Nobre L, Guerreiro Stucklin A, Bennett J, et al. Integrated molecular and clinical analysis of 1,000 pediatric low-Grade gliomas. Cancer Cell (2020) 37(4):569–583.e565. doi: 10.1016/j.ccell.2020.03.011
6. Davies H, Bignell GR, Cox C, Stephens P, Edkins S, Clegg S, et al. Mutations of the BRAF gene in human cancer. Nature (2002) 417(6892):949–54. doi: 10.1038/nature00766
7. Cantwell-Dorris ER, O'Leary JJ, Sheils OM. BRAFV600E: implications for carcinogenesis and molecular therapy. Mol Cancer Ther (2011) 10(3):385–94. doi: 10.1158/1535-7163.MCT-10-0799
8. Andrews LJ, Thornton ZA, Saincher SS, Yao IY, Dawson S, McGuinness LA, et al. Prevalence of BRAFV600 in glioma and use of BRAF Inhibitors in patients with BRAFV600 mutation-positive glioma: systematic review. Neuro Oncol (2022) 24(4):528–40. doi: 10.1093/neuonc/noab247
9. Kaley T, Touat M, Subbiah V, Hollebecque A, Rodon J, Lockhart AC, et al. BRAF inhibition in BRAF(V600)-mutant gliomas: results from the VE-BASKET study. J Clin Oncol (2018) 36(35):3477–84. doi: 10.1200/JCO.2018.78.9990
10. Hargrave DR, Bouffet E, Tabori U, Broniscer A, Cohen KJ, Hansford JR, et al. Efficacy and safety of dabrafenib in pediatric patients with BRAF V600 mutation-positive relapsed or refractory low-grade glioma: results from a phase I/IIa study. Clin Cancer Res (2019) 25(24):7303–11. doi: 10.1158/1078-0432.CCR-19-2177
11. Nicolaides T, Nazemi KJ, Crawford J, Kilburn L, Minturn J, Gajjar A, et al. Phase I study of vemurafenib in children with recurrent or progressive BRAF(V600E) mutant brain tumors: Pacific Pediatric Neuro-Oncology Consortium study (PNOC-002). Oncotarget (2020) 11(21):1942–52. doi: 10.18632/oncotarget.27600
12. Berzero G, Bellu L, Baldini C, Ducray F, Guyon D, Eoli M, et al. Sustained tumor control with MAPK inhibition in BRAF V600-mutant adult glial and glioneuronal tumors. Neurology (2021) 97(7):e673–83. doi: 10.1212/WNL.0000000000012330
13. Wen PY, Stein A, van den Bent M, De Greve J, Wick A, de Vos F, et al. Dabrafenib plus trametinib in patients with BRAF(V600E)-mutant low-grade and high-grade glioma (ROAR): a multicentre, open-label, single-arm, phase 2, basket trial. Lancet Oncol (2022) 23(1):53–64. doi: 10.1016/S1470-2045(21)00578-7
14. FDA. FDA approves dabrafenib with trametinib for pediatric patients with low-grade glioma with a BRAF V600E mutation U.S. Food & Drug administration (2023). Available at: https://www.fda.gov/drugs/resources-information-approved-drugs/fda-approves-dabrafenib-trametinib-pediatric-patients-low-grade-glioma-braf-v600e-mutation.
15. Subbiah V, Kreitman RJ, Wainberg ZA, Gazzah A, Lassen U, Stein A, et al. Dabrafenib plus trametinib in BRAFV600E-mutated rare cancers: the phase 2 ROAR trial. Nat Med (2023) 29:1103–1112. doi: 10.1038/s41591-023-02321-8
16. Schreck KC, Guajardo A, Lin DDM, Eberhart CG, Grossman SA. Concurrent BRAF/MEK inhibitors in BRAF V600-mutant high-grade primary brain tumors. J Natl Compr Canc Netw (2018) 16(4):343–7. doi: 10.6004/jnccn.2017.7052
17. Schreck KC, Langat P, Bhave VM, Li T, Woodward E, Pratilas CA, et al. Integrated molecular and clinical analysis of BRAF-mutant glioma in adults. NPJ Precis Oncol (2023) 7(1):23. doi: 10.1038/s41698-023-00359-y
18. Lassaletta A, Zapotocky M, Mistry M, Ramaswamy V, Honnorat M, Krishnatry R, et al. Therapeutic and prognostic implications of BRAF V600E in pediatric low-Grade gliomas. J Clin Oncol (2017) 35(25):2934–41. doi: 10.1200/JCO.2016.71.8726
19. Ryall S, Arnoldo A, Krishnatry R, Mistry M, Khor K, Sheth J, et al. Multiplex detection of pediatric low-grade glioma signature fusion transcripts and duplications using the nanoString nCounter system. J Neuropathol Exp Neurol (2017) 76(7):562–70. doi: 10.1093/jnen/nlx042
20. Nobre L, Zapotocky M, Ramaswamy V, Ryall S, Bennett J, Alderete D, et al. Outcomes of BRAF V600E pediatric gliomas treated with targeted BRAF inhibition. JCO Precis Oncol (2020) 4:561–71. doi: 10.1200/PO.19.00298
21. Michaud K, Solomon DA, Oermann E, Kim JS, Zhong WZ, Prados MD, et al. Pharmacologic inhibition of cyclin-dependent kinases 4 and 6 arrests the growth of glioblastoma multiforme intracranial xenografts. Cancer Res (2010) 70(8):3228–38. doi: 10.1158/0008-5472.CAN-09-4559
22. Huillard E, Hashizume R, Phillips JJ, Griveau A, Ihrie RA, Aoki Y, et al. Cooperative interactions of BRAFV600E kinase and CDKN2A locus deficiency in pediatric Malignant astrocytoma as a basis for rational therapy. Proc Natl Acad Sci U.S.A. (2012) 109(22):8710–5. doi: 10.1073/pnas.1117255109
23. Sturm D, Pfister SM, Jones DTW. Pediatric gliomas: current concepts on diagnosis, biology, and clinical management. J Clin Oncol (2017) 35(21):2370–7. doi: 10.1200/JCO.2017.73.0242
24. Schindler G, Capper D, Meyer J, Janzarik W, Omran H, Herold-Mende C, et al. Analysis of BRAF V600E mutation in 1,320 nervous system tumors reveals high mutation frequencies in pleomorphic xanthoastrocytoma, ganglioglioma and extra-cerebellar pilocytic astrocytoma. Acta Neuropathol (2011) 121(3):397–405. doi: 10.1007/s00401-011-0802-6
25. Behling F, Schittenhelm J. Oncogenic BRAF alterations and their role in brain tumors. Cancers (Basel) (2019) 11(6):794. doi: 10.3390/cancers11060794
26. Guerreiro Stucklin AS, Ryall S, Fukuoka K, Zapotocky M, Lassaletta A, Li C, et al. Alterations in ALK/ROS1/NTRK/MET drive a group of infantile hemispheric gliomas. Nat Commun (2019) 10(1):4343. doi: 10.1038/s41467-019-12187-5
27. Mistry M, Zhukova N, Merico D, Rakopoulos P, Krishnatry R, Shago M, et al. BRAF mutation and CDKN2A deletion define a clinically distinct subgroup of childhood secondary high-grade glioma. J Clin Oncol (2015) 33(9):1015–22. doi: 10.1200/JCO.2014.58.3922
28. Li X, Moreira DC, Bag AK, Qaddoumi I, Acharya S, Chiang J. The clinical and molecular characteristics of progressive hypothalamic/optic pathway pilocytic astrocytoma. Neuro Oncol (2023) 25(4):750–60. doi: 10.1093/neuonc/noac241
29. Upadhyaya SA, Robinson GW, Harreld JH, Klimo PD Jr., Hoehn ME, Orr BA, et al. Marked functional recovery and imaging response of refractory optic pathway glioma to BRAFV600E inhibitor therapy: a report of two cases. Childs Nerv Syst (2018) 34(4):605–10. doi: 10.1007/s00381-018-3739-4
30. Rayi A, Alnahhas I, Ong S, Giglio P, Puduvalli VK. Targeted therapy for BRAF mutant brain tumors. Curr Treat Options Oncol (2021) 22(11):105. doi: 10.1007/s11864-021-00901-9
31. Manoharan N, Liu KX, Mueller S, Haas-Kogan DA, Bandopadhayay P. Pediatric low-grade glioma: Targeted therapeutics and clinical trials in the molecular era. Neoplasia (2023) 36:100857. doi: 10.1016/j.neo.2022.100857
32. Lehman NL, Hattab EM, Mobley BC, Usubalieva A, Schniederjan MJ, McLendon RE, et al. Morphological and molecular features of astroblastoma, including BRAFV600E mutations, suggest an ontological relationship to other cortical-based gliomas of children and young adults. Neuro Oncol (2017) 19(1):31–42. doi: 10.1093/neuonc/now118
33. Behling F, Barrantes-Freer A, Skardelly M, Nieser M, Christians A, Stockhammer F, et al. Frequency of BRAF V600E mutations in 969 central nervous system neoplasms. Diagn Pathol (2016) 11(1):55. doi: 10.1186/s13000-016-0506-2
34. Kleinschmidt-DeMasters BK, Aisner DL, Birks DK, Foreman NK. Epithelioid GBMs show a high percentage of BRAF V600E mutation. Am J Surg Pathol (2013) 37(5):685–98. doi: 10.1097/PAS.0b013e31827f9c5e
35. Korshunov A, Chavez L, Sharma T, Ryzhova M, Schrimpf D, Stichel D, et al. Epithelioid glioblastomas stratify into established diagnostic subsets upon integrated molecular analysis. Brain Pathol (2018) 28(5):656–62. doi: 10.1111/bpa.12566
36. Zaman A, Wu W, Bivona TG. Targeting oncogenic BRAF: past, present, and future. Cancers (Basel) (2019) 11(8):1197. doi: 10.3390/cancers11081197
37. Cancer Genome Atlas, N. Genomic classification of cutaneous melanoma. Cell (2015) 161(7):1681–96. doi: 10.1016/j.cell.2015.05.044
38. Hayward NK, Wilmott JS, Waddell N, Johansson PA, Field MA, Nones K, et al. Whole-genome landscapes of major melanoma subtypes. Nature (2017) 545(7653):175–80. doi: 10.1038/nature22071
39. Barnholtz-Sloan JS, Sloan AE, Davis FG, Vigneau FD, Lai P, Sawaya RE. Incidence proportions of brain metastases in patients diagnosed (1973 to 2001) in the Metropolitan Detroit Cancer Surveillance System. J Clin Oncol (2004) 22(14):2865–72. doi: 10.1200/JCO.2004.12.149
40. Lamba N, Aizer AA. The evolving role of systemic therapy and local, brain-directed treatment in patients with melanoma and brain metastases. Neuro Oncol (2021) 23(11):1816–7. doi: 10.1093/neuonc/noab194
41. Lamba N, Wen PY, Aizer AA. Epidemiology of brain metastases and leptomeningeal disease. Neuro Oncol (2021) 23(9):1447–56. doi: 10.1093/neuonc/noab101
42. Long GV, Menzies AM, Nagrial AM, Haydu LE, Hamilton AL, Mann GJ, et al. Prognostic and clinicopathologic associations of oncogenic BRAF in metastatic melanoma. J Clin Oncol (2011) 29(10):1239–46. doi: 10.1200/JCO.2010.32.4327
43. Jakob JA, Bassett RL Jr., Ng CS, Curry JL, Joseph RW, Alvarado GC, et al. NRAS mutation status is an independent prognostic factor in metastatic melanoma. Cancer (2012) 118(16):4014–23. doi: 10.1002/cncr.26724
44. Maxwell R, Garzon-Muvdi T, Lipson EJ, Sharfman WH, Bettegowda C, Redmond KJ, et al. BRAF-V600 mutational status affects recurrence patterns of melanoma brain metastasis. Int J Cancer (2017) 140(12):2716–27. doi: 10.1002/ijc.30241
45. Falchook GS, Long GV, Kurzrock R, Kim KB, Arkenau TH, Brown MP, et al. Dabrafenib in patients with melanoma, untreated brain metastases, and other solid tumours: a phase 1 dose-escalation trial. Lancet (2012) 379(9829):1893–901. doi: 10.1016/S0140-6736(12)60398-5
46. Long GV, Trefzer U, Davies MA, Kefford RF, Ascierto PA, Chapman PB, et al. Dabrafenib in patients with Val600Glu or Val600Lys BRAF-mutant melanoma metastatic to the brain (BREAK-MB): a multicentre, open-label, phase 2 trial. Lancet Oncol (2012) 13(11):1087–95. doi: 10.1016/S1470-2045(12)70431-X
47. McArthur GA, Maio M, Arance A, Nathan P, Blank C, Avril MF, et al. Vemurafenib in metastatic melanoma patients with brain metastases: an open-label, single-arm, phase 2, multicentre study. Ann Oncol (2017) 28(3):634–41. doi: 10.1093/annonc/mdw641
48. Davies MA, Saiag P, Robert C, Grob JJ, Flaherty KT, Arance A, et al. Dabrafenib plus trametinib in patients with BRAF(V600)-mutant melanoma brain metastases (COMBI-MB): a multicentre, multicohort, open-label, phase 2 trial. Lancet Oncol (2017) 18(7):863–73. doi: 10.1016/S1470-2045(17)30429-1
49. Long GV, Flaherty KT, Stroyakovskiy D, Gogas H, Levchenko E, de Braud F, et al. Dabrafenib plus trametinib versus dabrafenib monotherapy in patients with metastatic BRAF V600E/K-mutant melanoma: long-term survival and safety analysis of a phase 3 study. Ann Oncol (2017) 28(7):1631–9. doi: 10.1093/annonc/mdx176
50. Luke JJ, Flaherty KT, Ribas A, Long GV. Targeted agents and immunotherapies: optimizing outcomes in melanoma. Nat Rev Clin Oncol (2017) 14(8):463–82. doi: 10.1038/nrclinonc.2017.43
51. Wilmott JS, Tawbi H, Engh JA, Amankulor NM, Shivalingam B, Banerjee H, et al. Clinical features associated with outcomes and biomarker analysis of dabrafenib plus trametinib treatment in patients with BRAF-mutant melanoma brain metastases. Clin Cancer Res (2023) 29(3):521–31. doi: 10.1158/1078-0432.CCR-22-2581
52. Dankort D, Curley DP, Cartlidge RA, Nelson B, Karnezis AN, Damsky WE Jr., et al. Braf(V600E) cooperates with Pten loss to induce metastatic melanoma. Nat Genet (2009) 41(5):544–52. doi: 10.1038/ng.356
53. Cho JH, Robinson JP, Arave RA, Burnett WJ, Kircher DA, Chen G, et al. AKT1 activation promotes development of melanoma metastases. Cell Rep (2015) 13(5):898–905. doi: 10.1016/j.celrep.2015.09.057
54. Filbin M, Monje M. Developmental origins and emerging therapeutic opportunities for childhood cancer. Nat Med (2019) 25(3):367–76. doi: 10.1038/s41591-019-0383-9
55. Jones C, Karajannis MA, Jones DTW, Kieran MW, Monje M, Baker SJ, et al. Pediatric high-grade glioma: biologically and clinically in need of new thinking. Neuro Oncol (2017) 19(2):153–61. doi: 10.1093/neuonc/now101
56. Filbin MG, Tirosh I, Hovestadt V, Shaw ML, Escalante LE, Mathewson ND, et al. Developmental and oncogenic programs in H3K27M gliomas dissected by single-cell RNA-seq. Science (2018) 360(6386):331–5. doi: 10.1126/science.aao4750
57. Grossauer S, Koeck K, Murphy NE, Meyers ID, Daynac M, Truffaux N, et al. Concurrent MEK targeted therapy prevents MAPK pathway reactivation during BRAFV600E targeted inhibition in a novel syngeneic murine glioma model. Oncotarget (2016) 7(46):75839–53. doi: 10.18632/oncotarget.12419
58. Mueller S, Hashizume R, Yang X, Kolkowitz I, Olow AK, Philips J, et al. Targeting Wee1 for the treatment of pediatric high-grade gliomas. Neuro Oncol (2014) 16(3):352–60. doi: 10.1093/neuonc/not220
59. Izumi I, Mineura K, Watanabe K, Kowada M. Establishment of the two glioma cell lines: YH and AM. Hum Cell (1994) 7(2):101–5.
60. Mulcahy Levy JM, Zahedi S, Griesinger AM, Morin A, Davies KD, Aisner DL, et al. Autophagy inhibition overcomes multiple mechanisms of resistance to BRAF inhibition in brain tumors. Elife (2017) 6:e19671. doi: 10.7554/eLife.19671
61. Kruse CA, Mitchell DH, Kleinschmidt-DeMasters BK, Franklin WA, Morse HG, Spector EM, et al. Characterization of a continuous human glioma cell line DBTRG-05MG: growth kinetics, karyotype, receptor expression, and tumor suppressor gene analyses. In Vitro Cell Dev Biol (1992) 28A(9-10):609–14. doi: 10.1007/BF02631035
62. Naruo K, Seko C, Kuroshima K, Matsutani E, Sasada R, Kondo T, et al. Novel secretory heparin-binding factors from human glioma cells (glia-activating factors) involved in glial cell growth. Purification Biol properties. J Biol Chem (1993) 268(4):2857–64.
63. Kolb EA, Gorlick R, Houghton PJ, Morton CL, Neale G, Keir ST, et al. Initial testing (stage 1) of AZD6244 (ARRY-142886) by the pediatric preclinical testing program. Pediatr Blood Cancer (2010) 55(4):668–77. doi: 10.1002/pbc.22576
64. Li F, Bondra KM, Ghilu S, Studebaker A, Liu Q, Michalek JE, et al. Regulation of TORC1 by MAPK signaling determines sensitivity and acquired resistance to trametinib in pediatric BRAFV600E brain tumor models. Clin Cancer Res (2022) 28(17):3836–49. doi: 10.1158/1078-0432.Ccr-22-1052
65. Levy JM, Thompson JC, Griesinger AM, Amani V, Donson AM, Birks DK, et al. Autophagy inhibition improves chemosensitivity in BRAF(V600E) brain tumors. Cancer Discovery (2014) 4(7):773–80. doi: 10.1158/2159-8290.CD-14-0049
66. Thompson EM, Brown M, Dobrikova E, Ramaswamy V, Taylor MD, McLendon R, et al. Poliovirus receptor (CD155) expression in pediatric brain tumors mediates oncolysis of medulloblastoma and pleomorphic xanthoastrocytoma. J Neuropathol Exp Neurol (2018) 77(8):696–702. doi: 10.1093/jnen/nly045
67. Sasame J, Ikegaya N, Kawazu M, Natsumeda M, Hayashi T, Isoda M, et al. HSP90 inhibition overcomes resistance to molecular targeted therapy in BRAFV600E-mutant high-grade glioma. Clin Cancer Res (2022) 28(11):2425–39. doi: 10.1158/1078-0432.Ccr-21-3622
68. Kanemaru Y, Natsumeda M, Okada M, Saito R, Kobayashi D, Eda T, et al. Dramatic response of BRAF V600E-mutant epithelioid glioblastoma to combination therapy with BRAF and MEK inhibitor: establishment and xenograft of a cell line to predict clinical efficacy. Acta Neuropathol Commun (2019) 7(1):119. doi: 10.1186/s40478-019-0774-7
69. Sievert AJ, Lang SS, Boucher KL, Madsen PJ, Slaunwhite E, Choudhari N, et al. Paradoxical activation and RAF inhibitor resistance of BRAF protein kinase fusions characterizing pediatric astrocytomas [published correction appears in proc natl acad sci u s a. 2013 may 21;110(21):8750]. Proc Natl Acad Sci U.S.A. (2013) 110(15):5957–62. doi: 10.1073/pnas.1219232110
70. Gabler L, Lötsch D, Kirchhofer D, van Schoonhoven, Schmidt HM, Mayr L, et al. TERT expression is susceptible to BRAF and ETS-factor inhibition in BRAFV600E/TERT promoter double-mutated glioma. Acta Neuropathol Commun (2019) 7(1):128. doi: 10.1186/s40478-019-0775-6
71. Shaffer SM, Dunagin MC, Torborg SR, Torre EA, Emert B, Krepler C, et al. Rare cell variability and drug-induced reprogramming as a mode of cancer drug resistance. Nature (2017) 546(7658):431–5. doi: 10.1038/nature22794
72. Nazarian R, Shi H, Wang Q, Kong X, Koya RC, Lee H, et al. Melanomas acquire resistance to B-RAF(V600E) inhibition by RTK or N-RAS upregulation. Nature (2010) 468(7326):973–7. doi: 10.1038/nature09626
73. Jazirehi AR, Nazarian R, Torres-Collado AX, Economou JS. Aberrant apoptotic machinery confers melanoma dual resistance to BRAF(V600E) inhibitor and immune effector cells: immunosensitization by a histone deacetylase inhibitor. Am J Clin Exp Immunol (2014) 3(1):43–56.
74. Das Thakur M, Salangsang F, Landman AS, Sellers WR, Pryer NK, Levesque MP, et al. Modelling vemurafenib resistance in melanoma reveals a strategy to forestall drug resistance. Nature (2013) 494(7436):251–5. doi: 10.1038/nature11814
75. Kavran AJ, Stuart SA, Hayashi KR, Basken JM, Brandhuber BJ, Ahn NG. Intermittent treatment of BRAFV600E melanoma cells delays resistance by adaptive resensitization to drug rechallenge. Proc Natl Acad Sci U S A. (2022) 119(12):e2113535119. doi: 10.1073/pnas.2113535119
76. Mao M, Tian F, Mariadason JM, Tsao CC, Lemos R Jr., Dayyani F, et al. Resistance to BRAF inhibition in BRAF-mutant colon cancer can be overcome with PI3K inhibition or demethylating agents. Clin Cancer Res (2013) 19(3):657–67. doi: 10.1158/1078-0432.Ccr-11-1446
77. Yang H, Higgins B, Kolinsky K, Packman K, Bradley WD, Lee RJ, et al. Antitumor activity of BRAF inhibitor vemurafenib in preclinical models of BRAF-mutant colorectal cancer. Cancer Res (2012) 72(3):779–89. doi: 10.1158/0008-5472.CAN-11-2941
78. Yaeger R, Yao Z, Hyman DM, Hechtman JF, Vakiani E, Zhao H, et al. Mechanisms of acquired resistance to BRAF V600E inhibition in colon cancers converge on RAF dimerization and are sensitive to its inhibition. Cancer Res (2017) 77(23):6513–23. doi: 10.1158/0008-5472.Can-17-0768
79. Nicolaides TP, Li H, Solomon DA, Hariono S, Hashizume R, Barkovich K, et al. Targeted therapy for BRAFV600E Malignant astrocytoma. Clin Cancer Res (2011) 17(24):7595–604. doi: 10.1158/1078-0432.CCR-11-1456
80. Olow A, Mueller S, Yang X, Hashizume R, Meyerowitz J, Weiss W, et al. BRAF status in personalizing treatment approaches for pediatric gliomas. Clin Cancer Res (2016) 22(21):5312–21. doi: 10.1158/1078-0432.CCR-15-1101
81. Zhang J, Yao TW, Hashizume R, Hariono S, Barkovich KJ, Fan QW, et al. Combined BRAF(V600E) and MEK blockade for BRAF(V600E)-mutant gliomas. J Neurooncol (2017) 131(3):495–505. doi: 10.1007/s11060-016-2333-4
82. Lerner RG, Grossauer S, Kadkhodaei B, Meyers I, Sidorov M, Koeck K, et al. Targeting a plk1-controlled polarity checkpoint in therapy-resistant glioblastoma-propagating cells. Cancer Res (2015) 75(24):5355–66. doi: 10.1158/0008-5472.CAN-14-3689
83. Dasgupta T, Olow AK, Yang X, Hashizume R, Nicolaides TP, Tom M, et al. Survival advantage combining a BRAF inhibitor and radiation in BRAF V600E-mutant glioma. J Neurooncol (2016) 126(3):385–93. doi: 10.1007/s11060-015-1939-2
84. Yao TW, Zhang J, Prados M, Weiss WA, James CD, Nicolaides T. EGFR blockade prevents glioma escape from BRAFV600E targeted therapy. Oncotarget (2015) 6(26):21993–2005. doi: 10.18632/oncotarget.4014
85. Paolillo M, Comincini S, Schinelli S. In vitro glioblastoma models: A journey into the third dimension. Cancers (Basel) (2021) 13(10):2449. doi: 10.3390/cancers13102449
86. Kim SC, Cho YE, Shin YK, Yu HJ, Chowdhury T, Kim S, et al. Patient-derived glioblastoma cell lines with conserved genome profiles of the original tissue. Sci Data (2023) 10(1):448. doi: 10.1038/s41597-023-02365-y
87. Avril T, Vauleon E, Hamlat A, Saikali S, Etcheverry A, Delmas C, et al. Human glioblastoma stem-like cells are more sensitive to allogeneic NK and T cell-mediated killing compared with serum-cultured glioblastoma cells. Brain Pathol (2012) 22(2):159–74. doi: 10.1111/j.1750-3639.2011.00515.x
88. Ratliff M, Kim H, Qi H, Kim M, Ku B, Azorin DD, et al. Patient-derived tumor organoids for guidance of personalized drug therapies in recurrent glioblastoma. Int J Mol Sci (2022) 23(12):6572. doi: 10.3390/ijms23126572
89. Lee J, Kotliarova S, Kotliarov Y, Li A, Su Q, Donin NM, et al. Tumor stem cells derived from glioblastomas cultured in bFGF and EGF more closely mirror the phenotype and genotype of primary tumors than do serum-cultured cell lines. Cancer Cell (2006) 9(5):391–403. doi: 10.1016/j.ccr.2006.03.030
90. Di Nunno V, Gatto L, Tosoni A, Bartolini S, Franceschi E. Implications of BRAF V600E mutation in gliomas: Molecular considerations, prognostic value and treatment evolution. Front Oncol (2022) 12:1067252. doi: 10.3389/fonc.2022.1067252
91. Flaherty KT, Infante JR, Daud A, Gonzalez R, Kefford RF, Sosman J, et al. Combined BRAF and MEK inhibition in melanoma with BRAF V600 mutations. N Engl J Med (2012) 367(18):1694–703. doi: 10.1056/NEJMoa1210093
92. Long GV, Stroyakovskiy D, Gogas H, Levchenko E, de Braud F, Larkin J, et al. Combined BRAF and MEK inhibition versus BRAF inhibition alone in melanoma. N Engl J Med (2014) 371(20):1877–88. doi: 10.1056/NEJMoa1406037
93. Robert C, Grob JJ, Stroyakovskiy D, Karaszewska B, Hauschild A, Levchenko E, et al. Five-Year outcomes with dabrafenib plus trametinib in metastatic melanoma. N Engl J Med (2019) 381(7):626–36. doi: 10.1056/NEJMoa1904059
94. Sondergaard JN, Nazarian R, Wang Q, Guo D, Hsueh T, Mok S, et al. Differential sensitivity of melanoma cell lines with BRAFV600E mutation to the specific Raf inhibitor PLX4032. J Transl Med (2010) 8:39. doi: 10.1186/1479-5876-8-39
95. Al Hashmi M, Sastry KS, Silcock L, Chouchane L, Mattei V, James N, et al. Differential responsiveness to BRAF inhibitors of melanoma cell lines BRAF V600E-mutated. J Transl Med (2020) 18(1):192. doi: 10.1186/s12967-020-02350-8
96. He H, Nan X, Liu S, Zhang L, Yang Z, Wu Y, et al. Anticancer effects of combinational treatment with BRAF(V600E) siRNA and PI3K pathway inhibitors in melanoma cell lines harboring BRAF(V600E). Oncol Lett (2018) 16(1):632–42. doi: 10.3892/ol.2018.8614
97. Vasudevan S, Flashner-Abramson E, Alkhatib H, Roy Chowdhury S, Adejumobi IA, Vilenski D, et al. Overcoming resistance to BRAF(V600E) inhibition in melanoma by deciphering and targeting personalized protein network alterations. NPJ Precis Oncol (2021) 5(1):50. doi: 10.1038/s41698-021-00190-3
98. Jung HD, Sung YJ, Kim HU. Omics and computational modeling approaches for the effective treatment of drug-resistant cancer cells. Front Genet (2021) 12:742902. doi: 10.3389/fgene.2021.742902
99. Han K, Jeng EE, Hess GT, Morgens DW, Li A, Bassik MC. Synergistic drug combinations for cancer identified in a CRISPR screen for pairwise genetic interactions. Nat Biotechnol (2017) 35(5):463–74. doi: 10.1038/nbt.3834
100. Shirani-Bidabadi S, Tabatabaee A, Tavazohi N, Hariri A, Aref AR, Zarrabi A, et al. CRISPR technology: A versatile tool to model, screen, and reverse drug resistance in cancer. Eur J Cell Biol (2023) 102(2):151299. doi: 10.1016/j.ejcb.2023.151299
101. Vandereyken K, Sifrim A, Thienpont B, Voet T. Methods and applications for single-cell and spatial multi-omics. Nat Rev Genet (2023) 24(8):494–515. doi: 10.1038/s41576-023-00580-2
102. Luebker SA, Koepsell SA. Diverse mechanisms of BRAF inhibitor resistance in melanoma identified in clinical and preclinical studies. Front Oncol (2019) 9:268. doi: 10.3389/fonc.2019.00268
103. Lehmann R, Rayner BS, Ziegler DS. Resistance mechanisms in BRAF(V600E) paediatric high-grade glioma and current therapeutic approaches. Front Oncol (2022) 12:1031378. doi: 10.3389/fonc.2022.1031378
104. Schreck KC, Morin A, Zhao G, Allen AN, Flannery P, Glantz M, et al. Deconvoluting mechanisms of acquired resistance to RAF inhibitors in BRAF(V600E)-mutant human glioma. Clin Cancer Res (2021) 27(22):6197–208. doi: 10.1158/1078-0432.Ccr-21-2660
105. Studebaker A, Bondra K, Seum S, Shen C, Phelps DA, Chronowski C, et al. Inhibition of MEK confers hypersensitivity to X-radiation in the context of BRAF mutation in a model of childhood astrocytoma. Pediatr Blood Cancer (2015) 62(10):1768–74. doi: 10.1002/pbc.25579
106. Usta D, Sigaud R, Buhl JL, Selt F, Marquardt V, Pauck D, et al. A cell-based MAPK reporter assay reveals synergistic MAPK pathway activity suppression by MAPK inhibitor combination in BRAF-driven pediatric low-grade glioma cells. Mol Cancer Ther (2020) 19(8):1736–50. doi: 10.1158/1535-7163.Mct-19-1021
107. Sigaud R, Rösch L, Gatzweiler C, Benzel J, von Soosten L, Peterziel H, et al. The first-in-class ERK inhibitor ulixertinib shows promising activity in mitogen-activated protein kinase (MAPK)-driven pediatric low-grade glioma models. Neuro Oncol (2023) 25(3):566–79. doi: 10.1093/neuonc/noac183
108. Miarka L, Valiente M. Animal models of brain metastasis. Neurooncol Adv (2021) 3(Suppl 5):v144–56. doi: 10.1093/noajnl/vdab115
109. Kogiso M, Qi L, Lindsay H, Huang Y, Zhao X, Liu Z, et al. Xenotransplantation of pediatric low grade gliomas confirms the enrichment of BRAF V600E mutation and preservation of CDKN2A deletion in a novel orthotopic xenograft mouse model of progressive pleomorphic xanthoastrocytoma. Oncotarget (2017) 8(50):87455–71. doi: 10.18632/oncotarget.20713
110. Thompson EM, Landi D, Ashley D, Keir ST, Bigner D, Ahn NG. Bevacizumab, irinotecan, temozolomide, tyrosine kinase inhibition, and MEK inhibition are effective against pleomorphic xanthoastrocytoma regardless of V600E status. J Neurooncol. (2018) 140(2):261–8. doi: 10.1007/s11060-018-2975-5
111. Koh HY, Kim SH, Jang J, Kim H, Han S, Lim JS, et al. BRAF somatic mutation contributes to intrinsic epileptogenicity in pediatric brain tumors. Nat Med (2018) 24(11):1662–8. doi: 10.1038/s41591-018-0172-x
112. Robinson JP, VanBrocklin MW, Guilbeault AR, Signorelli DL, Brandner S, Holmen SL. Activated BRAF induces gliomas in mice when combined with Ink4a/Arf loss or Akt activation. Oncogene (2010) 29(3):335–44. doi: 10.1038/onc.2009.333
113. Gronych J, Korshunov A, Bageritz J, Milde T, Jugold M, Hambardzumyan D, et al. An activated mutant BRAF kinase domain is sufficient to induce pilocytic astrocytoma in mice. J Clin Invest (2011) 121(4):1344–8. doi: 10.1172/JCI44656
114. Goz RU, Akgul G, LoTurco JJ. BRAFV600E expression in neural progenitors results in a hyperexcitable phenotype in neocortical pyramidal neurons. J Neurophysiol (2020) 123(6):2449–64. doi: 10.1152/jn.00523.2019
115. Cases-Cunillera S, van Loo KMJ, Pitsch J, Quatraccioni A, Sivalingam S, Salomoni P, et al. Heterogeneity and excitability of BRAFV600E-induced tumors is determined by Akt/mTOR-signaling state and Trp53-loss. Neuro Oncol (2022) 24(5):741–54. doi: 10.1093/neuonc/noab268
116. Oldrini B, Curiel-García Á, Marques C, Matia V, Uluçkan Ö, Graña-Castro O, et al. Somatic genome editing with the RCAS-TVA-CRISPR-Cas9 system for precision tumor modeling. Nat Commun (2018) 9(1):1466. doi: 10.1038/s41467-018-03731-w
117. Vaubel RA, Tian S, Remonde D, Schroeder MA, Mladek AC, Kitange GJ, et al. Genomic and phenotypic characterization of a broad panel of patient-Derived xenografts reflects the diversity of glioblastoma. Clin Cancer Res (2020) 26(5):1094–104. doi: 10.1158/1078-0432.Ccr-19-0909
118. Brabetz S, Leary SES, Grobner SN, Nakamoto MW, Seker-Cin H, Girard EJ, et al. A biobank of patient-derived pediatric brain tumor models. Nat Med (2018) 24(11):1752–61. doi: 10.1038/s41591-018-0207-3
119. Smith KS, Xu K, Mercer KS, Boop F, Klimo P, DeCupyere M, et al. Patient-derived orthotopic xenografts of pediatric brain tumors: a St. Jude resource. Acta Neuropathol (2020) 140(2):209–25. doi: 10.1007/s00401-020-02171-5
120. Khalsa JK, Cheng N, Keegan J, Chaudry A, Driver J, Bi WL, et al. Immune phenotyping of diverse syngeneic murine brain tumors identifies immunologically distinct types. Nat Commun (2020) 11(1):3912. doi: 10.1038/s41467-020-17704-5
121. Pritchard C, Carragher L, Aldridge V, Giblett S, Jin H, Foster C, et al. Mouse models for BRAF-induced cancers. Biochem Soc Trans (2007) 35(Pt 5):1329–33. doi: 10.1042/bst0351329
122. Rad R, Rad L, Wang W, Cadinanos J, Vassiliou G, Rice S, et al. PiggyBac transposon mutagenesis: a tool for cancer gene discovery in mice. Science (2010) 330(6007):1104–7. doi: 10.1126/science.1193004
123. Genovesi LA, Ng CG, Davis MJ, Remke M, Taylor MD, Adams DJ, et al. Sleeping Beauty mutagenesis in a mouse medulloblastoma model defines networks that discriminate between human molecular subgroups. Proc Natl Acad Sci U.S.A. (2013) 110(46):E4325–4334. doi: 10.1073/pnas.1318639110
124. Shaikh N, Brahmbhatt N, Kruser TJ, Kam KL, Appin CL, Wadhwani N, et al. Pleomorphic xanthoastrocytoma: a brief review. CNS Oncol (2019) 8(3):Cns39. doi: 10.2217/cns-2019-0009
125. Friedmann-Morvinski D, Bushong EA, Ke E, Soda Y, Marumoto T, Singer O, et al. Dedifferentiation of neurons and astrocytes by oncogenes can induce gliomas in mice. Science (2012) 338(6110):1080–4. doi: 10.1126/science.1226929
126. Chen R, Nishimura MC, Bumbaca SM, Kharbanda S, Forrest WF, Kasman IM, et al. A hierarchy of self-renewing tumor-initiating cell types in glioblastoma. Cancer Cell (2010) 17(4):362–75. doi: 10.1016/j.ccr.2009.12.049
127. Verheul C, Ntafoulis I, Kers TV, Hoogstrate Y, Mastroberardino PG, Barnhoorn S, et al. Generation, characterization, and drug sensitivities of 12 patient-derived IDH1-mutant glioma cell cultures. Neurooncol Adv (2021) 3(1):vdab103. doi: 10.1093/noajnl/vdab103
128. Balvers RK, Dirven CM, Leenstra S, Lamfers ML. Malignant glioma in vitro models: on the utilization of stem-like cells. Curr Cancer Drug Targets (2017) 17(3):255–66. doi: 10.2174/1568009616666160813191809
129. Abdullah KG, Bird CE, Buehler JD, Gattie LC, Savani MR, Sternisha AC, et al. Establishment of patient-derived organoid models of lower-grade glioma. Neuro Oncol (2022) 24(4):612–23. doi: 10.1093/neuonc/noab273
130. Zhang S, William C. Educational case: histologic and molecular features of diffuse gliomas. Acad Pathol (2020) 7:2374289520914021. doi: 10.1177/2374289520914021
131. Sugiarto S, Persson AI, Munoz EG, Waldhuber M, Lamagna C, Andor N, et al. Asymmetry-defective oligodendrocyte progenitors are glioma precursors. Cancer Cell (2011) 20(3):328–40. doi: 10.1016/j.ccr.2011.08.011
132. Gomez-Lopez S, Lerner RG, Petritsch C. Asymmetric cell division of stem and progenitor cells during homeostasis and cancer. Cell Mol Life Sci (2014) 71(4):575–97. doi: 10.1007/s00018-013-1386-1
133. Komori T. Grading of adult diffuse gliomas according to the 2021 WHO Classification of Tumors of the Central Nervous System. Lab Invest (2022) 102(2):126–33. doi: 10.1038/s41374-021-00667-6
134. Piaskowski S, Bienkowski M, Stoczynska-Fidelus E, Stawski R, Sieruta M, Szybka M, et al. Glioma cells showing IDH1 mutation cannot be propagated in standard cell culture conditions. Br J Cancer (2011) 104(6):968–70. doi: 10.1038/bjc.2011.27
135. Dovedi SJ, Elder MJ, Yang C, Sitnikova SI, Irving L, Hansen A, et al. Design and efficacy of a monovalent bispecific PD-1/CTLA4 antibody that enhances CTLA4 blockade on PD-1(+) activated T cells. Cancer Discovery (2021) 11(5):1100–17. doi: 10.1158/2159-8290.CD-20-1445
136. Bausart M, Bozzato E, Joudiou N, Koutsoumpou X, Manshian B, Preat V, et al. Mismatch between bioluminescence imaging (BLI) and MRI when evaluating glioblastoma growth: lessons from a study where BLI suggested "Regression" while MRI showed "Progression". Cancers (Basel) (2023) 15(6):1919. doi: 10.3390/cancers15061919
137. Wu Y, Walker JR, Westberg M, Ning L, Monje M, Kirkland TA, et al. Kinase-modulated bioluminescent indicators enable noninvasive imaging of drug activity in the brain. ACS Cent Sci (2023) 9(4):719–32. doi: 10.1021/acscentsci.3c00074
138. McClelland S, Brennan GP, Dubé C, Rajpara S, Iyer S, Richichi C, et al. The transcription factor NRSF contributes to epileptogenesis by selective repression of a subset of target genes. Elife (2014) 3:e01267. doi: 10.7554/eLife.01267
139. Andor N, Graham TA, Jansen M, Xia LC, Aktipis CA, Petritsch C, et al. Pan-cancer analysis of the extent and consequences of intratumor heterogeneity. Nat Med (2016) 22(1):105–13. doi: 10.1038/nm.3984
140. Schmidt M, Mortensen LS, Loeffler-Wirth H, Kosnopfel C, Krohn K, Binder H, et al. Single-cell trajectories of melanoma cell resistance to targeted treatment. Cancer Biol Med (2021) 19(1):56–73. doi: 10.20892/j.issn.2095-3941.2021.0267
141. Zhang ZY, Ding Y, Ezhilarasan R, Lhakhang T, Wang Q, Yang J, et al. Lineage-coupled clonal capture identifies clonal evolution mechanisms and vulnerabilities of BRAF(V600E) inhibition resistance in melanoma. Cell Discovery (2022) 8(1):102. doi: 10.1038/s41421-022-00462-7
142. Emert BL, Cote CJ, Torre EA, Dardani IP, Jiang CL, Jain N, et al. Variability within rare cell states enables multiple paths toward drug resistance. Nat Biotechnol (2021) 39(7):865–76. doi: 10.1038/s41587-021-00837-3
143. Chen S, Sanjana NE, Zheng K, Shalem O, Lee K, Shi X, et al. Genome-wide CRISPR screen in a mouse model of tumor growth and metastasis. Cell (2015) 160(6):1246–60. doi: 10.1016/j.cell.2015.02.038
144. Hubert CG, Rivera M, Spangler LC, Wu Q, Mack SC, Prager BC, et al. A three-dimensional organoid culture system derived from human glioblastomas recapitulates the hypoxic gradients and cancer stem cell heterogeneity of tumors found in vivo. Cancer Res (2016) 76(8):2465–77. doi: 10.1158/0008-5472.Can-15-2402
145. Bian S, Repic M, Guo Z, Kavirayani A, Burkard T, Bagley JA, et al. Genetically engineered cerebral organoids model brain tumor formation. Nat Methods (2018) 15(8):631–9. doi: 10.1038/s41592-018-0070-7
146. Ogawa J, Pao GM, Shokhirev MN, Verma IM. Glioblastoma model using human cerebral organoids. Cell Rep (2018) 23(4):1220–9. doi: 10.1016/j.celrep.2018.03.105
147. Linkous A, Balamatsias D, Snuderl M, Edwards L, Miyaguchi K, Milner T, et al. Modeling patient-derived glioblastoma with cerebral organoids. Cell Rep (2019) 26(12):3203–3211.e3205. doi: 10.1016/j.celrep.2019.02.063
148. Jacob F, Salinas RD, Zhang DY, Nguyen PTT, Schnoll JG, Wong SZH, et al. A patient-derived glioblastoma organoid model and biobank recapitulates inter- and intra-tumoral heterogeneity. Cell (2020) 180(1):188–204.e122. doi: 10.1016/j.cell.2019.11.036
149. Benton G, Arnaoutova I, George J, Kleinman HK, Koblinski J. Matrigel: from discovery and ECM mimicry to assays and models for cancer research. Adv Drug Delivery Rev (2014) 79-80:3–18. doi: 10.1016/j.addr.2014.06.005
150. Correa S, Grosskopf AK, Lopez Hernandez H, Chan D, Yu AC, Stapleton LM, et al. Translational applications of hydrogels. Chem Rev (2021) 121(18):11385–457. doi: 10.1021/acs.chemrev.0c01177
151. Grosskopf AK, Labanieh L, Klysz DD, Roth GA, Xu P, Adebowale O, et al. Delivery of CAR-T cells in a transient injectable stimulatory hydrogel niche improves treatment of solid tumors. Sci Adv (2022) 8(14):eabn8264. doi: 10.1126/sciadv.abn8264
Keywords: BRAF V600E, brain tumor models, therapy resistance, melanoma metastases, low-grade glioma, tumor heterogeneity, tumor progression, tumor imaging
Citation: Xing YL, Panovska D and Petritsch CK (2023) Successes and challenges in modeling heterogeneous BRAFV600E mutated central nervous system neoplasms. Front. Oncol. 13:1223199. doi: 10.3389/fonc.2023.1223199
Received: 15 May 2023; Accepted: 18 September 2023;
Published: 18 October 2023.
Edited by:
Wassim Abou-Kheir, American University of Beirut, LebanonReviewed by:
Yukitomo Ishi, Hokkaido University, JapanMassimo Zollo, University of Naples Federico II, Italy
Liangxue Zhou, Sichuan University, China
Copyright © 2023 Xing, Panovska and Petritsch. This is an open-access article distributed under the terms of the Creative Commons Attribution License (CC BY). The use, distribution or reproduction in other forums is permitted, provided the original author(s) and the copyright owner(s) are credited and that the original publication in this journal is cited, in accordance with accepted academic practice. No use, distribution or reproduction is permitted which does not comply with these terms.
*Correspondence: Claudia K. Petritsch, Y3BldHJpQHN0YW5mb3JkLmVkdQ==