- 1Pharmacy Department, Al-kut University College, Kut, Iraq
- 2Department of Medical Laboratory, Affiliated Hospital of Guangdong Medical University, Zhanjiang, China
- 3Department of Radiation Oncology, Melvin and Bren Simon Comprehensive Cancer Center, Indiana University School of Medicine, Indianapolis, IN, United States
- 4Department of Medical Laboratory, School of Laboratory Medicine and Biotechnology, Southern Medical University, Guangzhou, China
- 5Department of Biological Sciences, National University of Medical Sciences (NUMS), Rawalpindi, Pakistan
- 6Department of Clinical Biobank Center, Zhujiang Hospital, Southern Medical University, Guangzhou, China
Low oxygen tension, or hypoxia is the driving force behind tumor aggressiveness, leading to therapy resistance, metastasis, and stemness in solid cancers including breast cancer, which now stands as the leading cause of cancer-related mortality in women. With the great advancements in exploring the regulatory roles of the non-coding genome in recent years, the wide spectrum of hypoxia-responsive genome is not limited to just protein-coding genes but also includes multiple types of non-coding RNAs, such as micro RNAs, long non-coding RNAs, and circular RNAs. Over the years, these hypoxia-responsive non-coding molecules have been greatly implicated in breast cancer. Hypoxia drives the expression of these non-coding RNAs as upstream modulators and downstream effectors of hypoxia inducible factor signaling in the favor of breast cancer through a myriad of molecular mechanisms. These non-coding RNAs then contribute in orchestrating aggressive hypoxic tumor environment and regulate cancer associated cellular processes such as proliferation, evasion of apoptotic death, extracellular matrix remodeling, angiogenesis, migration, invasion, epithelial-to-mesenchymal transition, metastasis, therapy resistance, stemness, and evasion of the immune system in breast cancer. In addition, the interplay between hypoxia-driven non-coding RNAs as well as feedback and feedforward loops between these ncRNAs and HIFs further contribute to breast cancer progression. Although the current clinical implications of hypoxia-driven non-coding RNAs are limited to prognostics and diagnostics in breast cancer, extensive explorations have established some of these hypoxia-driven non-coding RNAs as promising targets to treat aggressive breast cancers, and future scientific endeavors hold great promise in targeting hypoxia-driven ncRNAs at clinics to treat breast cancer and limit global cancer burden.
1 Introduction
1.1 Breast cancer
Despite the substantial improvements in cancer prevention, diagnosis, and treatment over the last few decades, 19.3 million new cases and almost 10 million cancer associated deaths were recorded globally in 2020 (1). Breast cancer is now the most prevalent malignancy worldwide and is the leading cause of cancer related mortality among women (2). The heterogeneous nature of breast cancer has led to its classification into different subtypes based on both clinical and molecular characteristics, with each subtype exhibiting varying biological and pathological traits as well as distinct clinical outcomes (3). In clinical practice, immunohistochemical quantification of Estrogen Receptor (ER), Progesterone Receptor (PR) and Human Epidermal Growth Factor Receptor 2 (HER2) is the gold standard to classify breast cancer into hormone receptor positive (HR+), HER2 positive (HER2+) and triple negative breast cancer (TNBC), whereas transcriptional profiling stratifies breast cancer into five major subtypes, i.e. luminal A, luminal B, HER2 over-expression, basal, and normal-like tumors primarily overlapping clinical subtypes (4). For instance, luminal tumors overlap with the HR+ clinical subtype. These tumors grow slowly but often relapse, and are treated with a combination of endocrine- and chemo-therapy (5). HER2+ breast cancer is relatively aggressive, with a poor prognosis and treatment options revolving around targeting HER2 along with chemotherapy (6). Basal or TNBC represent the most aggressive subtype of breast cancer, with chemotherapy as sole treatment option due to lack of the expression of targetable receptors (7). Unfortunately, therapy resistance is inevitable due to cellular heterogeneity of breast cancer, and conventional treatment options often fall short of tackling almost all aggressive breast cancer cases (8). On top of that, distant metastatic spread further complicates the scenario and is considered incurable, as almost 90% of breast cancer associated deaths are due to metastatic disease (9). Therefore, discovering new drugable targets, developing potent therapies, and identifying innovative therapeutic strategies are indispensable for breast cancer.
1.2 Hypoxia and cancer
Oxygen is the driving force behind cellular metabolism, homeostasis, and survival. During the initial stages of oncogenic transformation, oxygen and nutrients are still readily available to transformed cells because they are in close proximity to normal tissue vasculature in their surroundings (10). As these cells progress to form tumors, low oxygen tension, or hypoxia, starts to prevail in almost all solid tumors, especially in the central core of the mass (11). As a result of uncontrolled proliferation outgrowing surrounding vasculature, oxygen levels markedly plunge in these hypoxic regions, leading to changes in the pH gradient towards an acidic environment (12). Although transformed cells tend to die in the beginning within the hypoxic core as oxygen and glucose deprivation trigger necrosis due to a drop in cellular adenosine triphosphate (ATP) levels (13), these cancer cells adapt quickly to changing microenvironment and orchestrate a hypoxic signaling response to favor cancer cell survival, tumor progression, therapy resistance, metastasis, and cancer stemness overtime (14, 15). The classical hypoxic response involves the stabilization of oxygen-sensitive molecular mediators called hypoxia-inducible factors (HIFs). Under normoxia, HIFs (HIF1α, HIF2α, HIF3α) are continuously hydroxylated by prolyl-4-hydroxylases (PHDs), and these hydoxylated HIFs are under selective pressure to be degraded by an E3 ubiquitin ligase called Von Hippel-Lindau (VHL). Upon oxygen deprivation, HIF1α escapes degradation and binds to form heterodimeric HIF1 transcription factor with constitutively expressed and oxygen insensitive HIF1β subunit. This complex is then translocated into the nucleus where it binds to hypoxia-responsive elements (HREs) in the promoter regions and transcribes hundreds of genes involved in multiple signaling pathways controlling cancer promoting cellular mechanisms such as proliferation, apoptosis, extracellular matrix remodeling, epithelial-to-mesenchymal transition (EMT), and angiogenesis (16). Alternatively, HIF2α can partner with HIF1β upon oxygen deprivation and form the HIF2 transcription factor. Notably, HIF1 and HIF2 transcription factors share multiple common targets alongside unique sets of genes that they individually transcribe (17) (Figure 1). Alternatively, HIF3α may compete with HIF1α and HIF2α to bind with HIF1β, thereby inhibiting HIF-dependent gene regulation (18). In addition, hypoxia may drive a non-classical response as well by regulating gene expression through HIF-independent mechanisms (19, 20). Given its central role in cancer progression, targeting hypoxia and HIF-mediated signaling pathways provides excellent therapeutic option for treating solid tumors such as breast cancer in clinical settings.
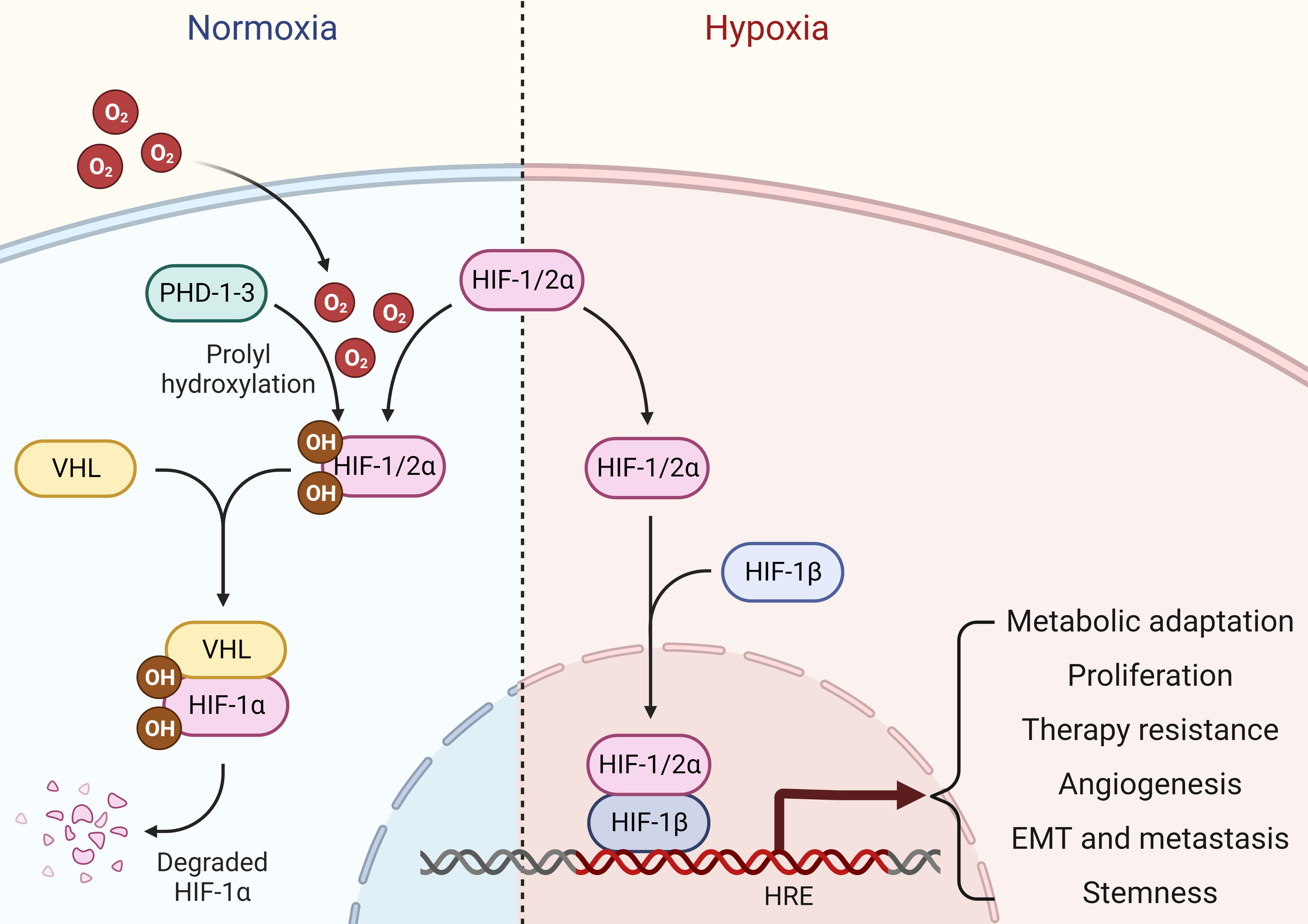
Figure 1 Hypoxia signaling. Under normal oxygen availability, HIF1/2α are hydroxylated by PHDs, which are then ubiquitinated and undergo proteasomal degradation in an E3 ubiquitin ligase VHL-dependent manner. Upon oxygen deprivation, HIF1/2α escapes degradation and binds to form heterodimeric HIF transcription factors with constitutively expressed and oxygen insensitive HIF1β subunit. This complex is then translocated into the nucleus, where it binds to HREs in the promoter regions and stimulates gene transcription that aids cancer cells for metabolic adaptations, proliferation, therapy resistance, angiogenesis, EMT, metastasis and stemness.
1.3 ncRNAs and cancer
Over the last two decades, scientists have discovered the great virtues of the non-coding genome in regulating gene expression. It all started with the identification of small (19-24 nucleotides in length) ncRNAs called microRNAs (miRNAs). These tiny structures are transcribed from genome as primary miRNA (pri-miRNA) which are then processed inside the nucleus by DROSHA and DGCR8 into precursor miRNA (pre-miRNA). Once exported to the cytoplasm by exportin 5 (XPO5), pre-miRNAs are further cleaved by DICER to become mature miRNAs which can preferentially bind to the 3’UTR of their target mRNAs in the miRNA-induced silencing complex (RISC) leading to translational repression or mRNA degradation depending upon miRNA-mRNA binding synergy (21, 22). miRNAs are involved in every cellular and biological process, as they are estimated to regulate almost 60% of the mammalian genome (23). In addition to their classical mRNA targeting function, miRNAs may maintain cellular homeostasis in other ways, such as by inhibiting nuclear ncRNAs, binding and inhibiting proteins, inhibiting mitochondrial transcripts, coding for peptides, triggering transcription, activating the translation of mRNAs, and activating Toll-like receptors (24). Long ncRNAs (lncRNAs) denote another class of regulatory ncRNAs comprising RNA molecules of around 200 nucleotides in length that are not translated into proteins. LncRNAs are distributed throughout the genome and are transcribed and processed in similar fashion to mRNAs, though they tend to form multifaceted 3D structures with the flexibility to rapidly change and perform multiple functions (25). LncRNAs may work in a cis (in proximity to their transcription site) or trans (at distant sites) fashion. Their known functions include, but are not limited to, chromatin modification, chromosomal looping, gene transcription, binding to mRNAs and proteins, interacting with other ncRNAs, protein translation, and paraspeckle formation (26). The third major class of regulatory ncRNAs is circular RNAs (circRNAs) which are joined end-to-end to form loops. They are generated through diversified biogenesis mechanisms such as exon-skipping, intron debranching, intron pairing, and dimerization of RNA binding proteins. CircRNAs play a role in cellular homeostasis by sponging miRNAs, controlling protein translation and binding proteins to regulate their functions (27) (Figure 2). Deregulated expression of miRNAs, lncRNAs, and circRNAs in cancer cells links these ncRNAs to different hallmarks of cancer, such as uncontrolled proliferation, resistance to cell death, invasion and metastasis, angiogenesis, immune evasion, and cancer stemness (28). Notably, unlike most protein-coding genes, a ncRNA may exhibit cancer promoting or inhibiting functions in tissue- and context-dependent manners, thereby adding another level of complexity to our understanding of the true nature of ncRNAs in cancer (29). Recently, multiple other types of ncRNAs such as piwi-interacting RNAs (piRNAs), small nuclear RNAs (snRNAs), and small nucleolar RNAs (snoRNAs), have also been explored to perform key regulatory mechanisms (30), though understanding of their role in cancer biology is still limited.
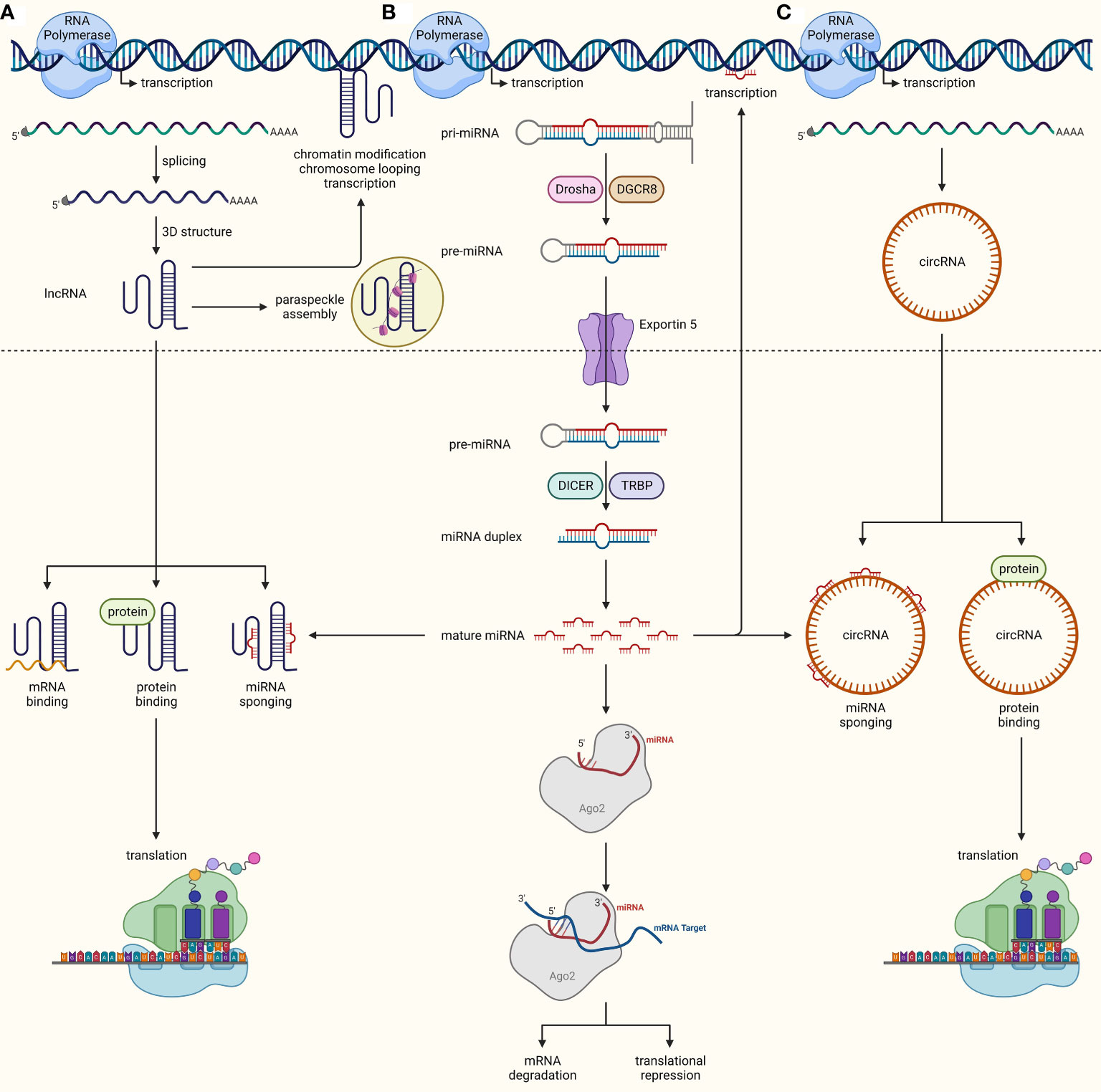
Figure 2 Biogenesis and functions of ncRNAs. (A) lncRNAs are transcribed from the genome similarly to mRNAs, undergo splicing, and adopt complex 3D structures upon maturation inside the nucleus. They can either work inside the nucleus to regulate chromatin modifications, chromosomal looping, DNA transcription, and paraspeckle formation, or they can be exported out to the cytoplasm, where they interact with mRNAs, proteins, and miRNAs, and regulate protein translation and modifications, as well as compete with miRNAs by sponging them off their targets. (B) miRNAs are transcribed as pri-miRNAs from the genome, which are processed into pre-miRNA by DROSHA and DGCR8. These pre-miRNA are then exported out into the cytoplasm through XPO5, where they are transformed into miRNA-duplexes through cleavage by DICER. One strand of duplex is then loaded into RISC, where its seed region binds complementarily to target mRNA, leading to mRNA degradation or translational repression. miRNAs may also aid in transcription or interact with other ncRNAs. (C) CircRNAs mature through diversified biogenesis mechanisms such as exon-skipping, intron debranching, intron pairing, and dimerization of RNA binding proteins. Similar to lncRNAs, circRNAs can also modulate protein translation and function through direct interactions and miRNA inhibition by sponging them off their target.
Here, we aimed to comprehensively review the role of hypoxia-driven ncRNAs in breast cancer and their therapeutic potential in clinics. In this context, we have first discussed the molecular mechanisms through which hypoxia drives the expression of different ncRNAs as upstream modulators and downstream effectors of hypoxia inducible factor (HIF) signaling in favor of breast cancer. In addition, we have also summarized the contribution of these ncRNAs in orchestrating aggressive hypoxic tumor environment by regulating cancer associated cellular processes such as proliferation, evasion of apoptotic death, extracellular matrix remodeling, angiogenesis, migration, invasion, EMT, metastasis, therapy resistance, stemness, and evasion of the immune system in breast cancer. We have reviewed the interplay between hypoxia-driven ncRNAs as well as feedback and feedforward loops between these ncRNAs and HIFs in breast cancer. Later, we have presented the current clinical implications of hypoxia-driven ncRNAs as prognostic and diagnostic markers in breast cancer. Lastly, we have discussed the current developments and challenges in therapeutic strategies as well as future prospects for targeting hypoxia-driven ncRNAs in breast cancer.
2 Hypoxia-driven ncRNAs in breast cancer
In addition to regulating a plethora of protein-coding genes, hypoxia may regulate the expression of different ncRNAs both in HIF-signaling-dependent and independent manners. The underlying molecular mechanisms include transcriptional regulation as well as post-transcriptional regulation such as editing, modification, maturation, stability, localization, and transport of these ncRNAs (31–34). For instance, changes in miRNA editing patterns, particularly if miRNAs are edited in their seed regions, may alter the whole transcriptome, as a single miRNA can target multiple mRNAs at the same time (35). Recently, hypoxia has been suggested as a key external stimulus that can alter the whole transcriptomic profile of cells by inducing miRNA editing in seed regions (36). Hypoxia may affect the processing of miRNA transcripts into mature miRNAs in breast cancer cells by downregulating DICER, the key enzyme involved in miRNA biogenesis (37, 38). Alternatively, epidermal growth factor receptor (EGFR) binds to argonaute 2 (AGO2) and phosphorylates it at Tyr 393 under hypoxia, thereby disrupting the interaction between AGO2 and DICER and resulting in impaired miRNA maturation. This leads to the evasion of multiple oncogenes from miRNA inhibition, thereby contributing to invasion and metastasis in breast cancer (39). In addition, the expression of multiple classes of ncRNAs, including miRNAs, lncRNAs, circRNAs, and snRNAs, is fine-tuned by hypoxia to facilitate cancer progression (34). This section summarizes the contributory roles of ncRNA as upstream modulators and as downstream effectors of hypoxia in orchestrating an aggressive hypoxic tumor environment in breast cancer.
2.1 ncRNAs as upstream modulators of HIF-signaling in breast cancer
The overall hypoxic phenotype is mainly coordinated through oxygen deprivation-driven HIF-dependent signaling. How HIFs are stabilized and activated in the first place to orchestrate the hypoxic tumor microenvironment is of key importance. Multiple ncRNAs, including miRNAs, lncRNAs and circRNAs, are now implicated in the regulation of HIFs upon oxygen deprivation via direct or indirect interactions in breast cancer (Figure 3). For instance, miR-182 aids in hypoxia-driven cancer progression by directly targeting the E3 ubiquitin-protein ligase FBXW7, which preferentially tags HIF1 for degradation. Furthermore, upregulated miR-182 under hypoxia induces vascular endothelial growth factor A (VEGFA) expression downstream of HIF1-signaling to promote angiogenesis in breast tumors (40). miR-24 regulates HIF1α expression specifically in breast cancer stem cells (CSCs) and promotes cancer stemness. Mechanistically, it targets factor inhibiting HIF1α (FIH1), which is a negative regulator of HIF1α, leading to increased expression of HIF1α (41). A well-established oncogenic miRNA, miR-21, may also upregulate HIF1α expression and promote EMT-like features and stemness in breast cancer cells, though the molecular link between miR-21 and elevated HIF1α expression is not established yet (25). Oncoprotein hepatitis B X-interacting protein (HBXIP) escalates tumorigenesis by enhancing HIF1α expression in multiple ways. It targets VHL and disassociates it from HIF1α to stabilize HIF1α expression. As a result, HIF1α may promote miR-183/96/182 cluster expression, out of which miR-183 can also target VHL mRNAs to further inhibit its expression and constitutively promote HIF1α expression and associated cancer progression in a feedback loop mechanism. In addition, these miRNAs may also target key regulators of glucose metabolism, such as synthesis of cytochrome C oxidase 2 (SCO2) and pyruvate dehydrogenase alpha 1 (PDHA1), in favor of cancer progression (42). These findings suggest that multiple oncogenic miRNAs indirectly regulate HIF signaling, and targeting these molecules holds promise to limit hypoxic TME and cancer progression.
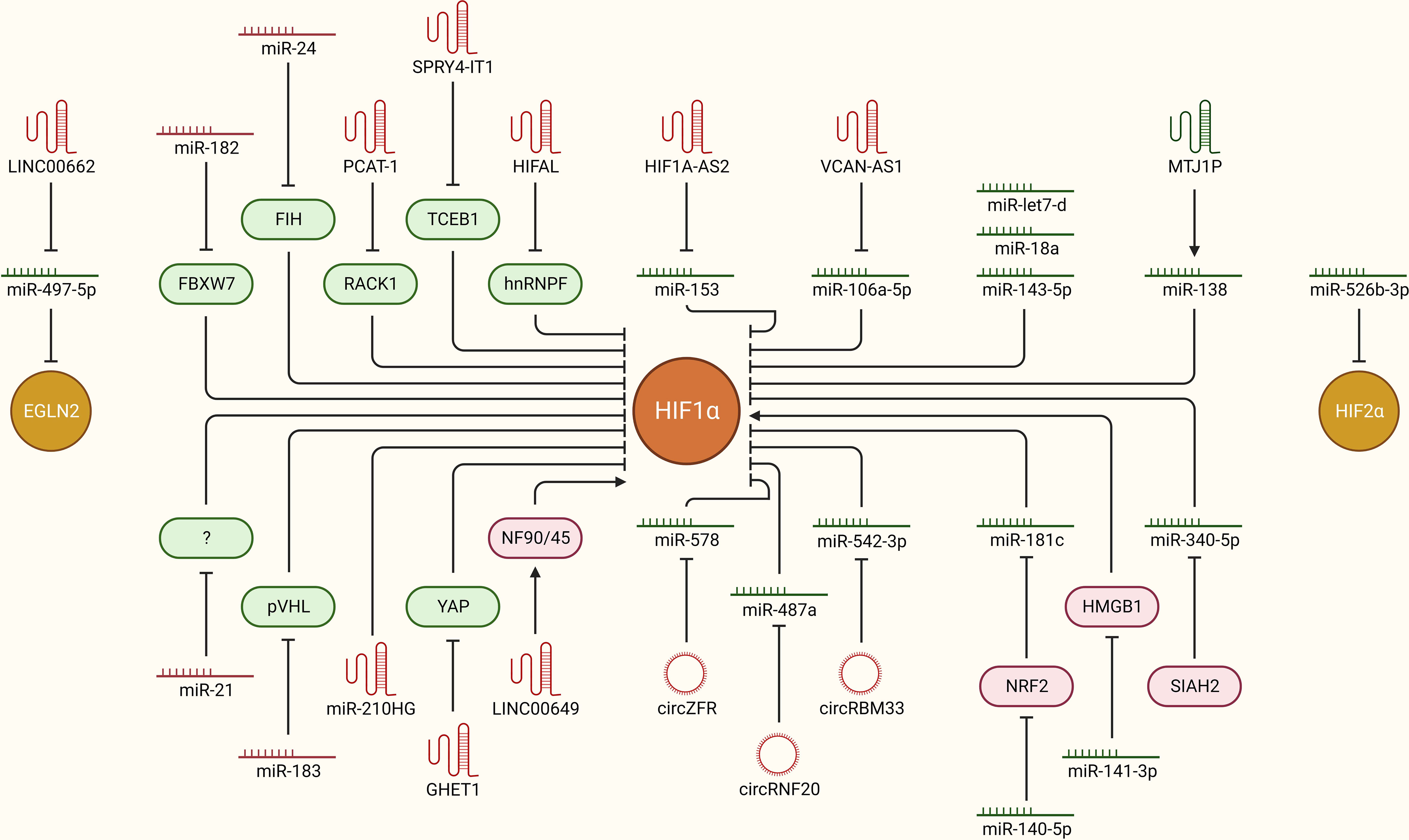
Figure 3 ncRNAs as upstream modulators of HIF-signaling in breast cancer. Hypoxia-driven ncRNAs are implicated in regulating the expression of HIFs to modulate downstream oncogenic signaling pathways in breast cancer. The mechanistic regulation of HIFs downstream of different ncRNAs (miRNAs, lncRNAs, and circRNAs) in breast cancer is summarized. Green: anti-tumor, Red: pro-tumor, arrowhead: activation, blockhead: inhibition.
LncRNA prostate cancer-associated transcript-1 (PCAT-1) is induced upon hypoxia in breast cancer cells and stabilizes HIF1α by directly interacting with the receptor of activated protein C kinase-1 (RACK1) protein and preventing RACK1-mediated degradation of HIF1α. Unsurprisingly, PCAT-1 is upregulated in breast cancer patients and is also associated with clinical parameters such as grade, tumor size, and poor clinical outcome (43). Similarly, high expression of the lncRNA SPRY4 intronic transcript 1 (SPRY4-IT1) is associated with cancer aggressiveness and poor outcome in breast cancer. It promotes cancer cell metastasis by upregulating HIF1α expression. Mechanistically, NF-kB/p65 transactivates SPRY-IT1, which inhibits transcription elongation factor B-polypeptide 1 (TCEB1) expression by promoting Staufen 1 (STAU1) mediated decay of TCEB1 mRNA transcripts. In turn, the activity of E3 ubiquitin ligases decreases, which results in elevated HIF1α expression, leading to increased cancer progression and metastasis (44). HIF-1α anti-sense lncRNA, HIFAL, is also critical in maintaining a hypoxic tumor environment by promoting HIF1α expression in a feedback loop. It first induces prolyl hydroxylation of pyruvate kinase 2 (PKM2) by recruiting PHD3 and later aids in translocating the PKM2/PHD3 complex into the nucleus via binding heterogeneous nuclear ribonucleoprotein F (hnRNPF). Once inside the nucleus, the PKM2/PHD3 complex enhances HIF-1α transactivation, which in turn induces HIFAL transcription to maintain the feed-forward loop. Notably, HIFAL expression is also validated to be associated with an aggressive phenotype and poor clinical outcome in breast cancer patients (45). Higher expression of MIR210HG lncRNA is also associated with poor clinical outcome in breast cancer patients. At the molecular level, hypoxia induced MIR210HG stabilizes HIF1α expression by directly binding its 5’UTR. As a result, increased HIF1α expression promotes glycolytic metabolism and tumorigenic potential in TNBC cells (46). Hypoxia induced lncRNA gastric carcinoma proliferation enhancing transcript 1 (GHET1) is highly expressed in TNBC tissues and is associated with extensive cell proliferation, invasion, and glycolysis. The downstream effects of GHET1 are attributed to the induction of HIF1α expression and activation of Hippo/Yap signaling pathway through the promotion of nuclear translocation of Yes1 associated transcriptional regulator (YAP) (47). LINC00649 stabilizes HIF1α expression and promotes tumor growth and metastasis in breast cancer. Mechanistically, it stabilizes HIF1α mRNA by interacting with the nuclear factor 90 (NF90)-NF45 complex and upregulates HIF1α expression (48). These findings highlight that multiple lncRNAs boost HIF-signaling directly or indirectly upon hypoxia, and targeting these lncRNA can aid to limit cancer progression.
Certain lncRNAs and circRNAs can alter cellular transcriptomic profiles and associated phenotype through sponging off miRNAs from their mRNA targets, leading to enhanced expression of a set of genes. So far, a few lncRNAs and circRNAs have been reported to regulate hypoxia through such mechanism in breast cancer. For instance, miR-153 inhibits angiogenesis in breast cancer tissues by directly targeting HIF1α and angiopoietin (ANG1), thereby suppressing the migration and invasion of cancer cells (49). Interestingly, in order to fine-tune the angiogenesis in TNBC, hypoxia-driven ER stress also induces miR-153 expression in an endoribonuclease IRE1-like protein (IRE1A)-X-box binding protein 1 (XBP1) complex-dependent manner, where XBP1 directly binds to miR-153 host gene protein tyrosine phosphatase receptor type N (PTPRN) and promotes its transcription. In return, miR-153 directly targets the HIF1α 3’ UTR and inhibits downstream VEGFA mediated angiogenesis (50). Unfortunately, this whole axis is balanced in favor of increased angiogenesis preferentially through hypoxia mediated induction of the lncRNA HIF1α-anti sense 2 (AS2), which sponges off miR-153 from HIF1α mRNA transcripts (51). LncRNA versican (VCAN)-AS1 promotes breast cancer cell migration, invasion, and EMT by working as a competing endogenous RNA to inhibit miR-106a-5p, which cancer cell progression via targeting signal transducer and activator of transcription 3 (STAT3) and inhibiting the STAT3/HIF1α axis (52). LINC00662 sponges off miR-497-5p from the egl-9 family hypoxia inducible factor 2 (EglN2) and promotes breast cancer cell proliferation, migration, and invasion (53). CircZFR promotes breast cancer progression and metastasis by relieving HIF1α from being targeted by miR-578. It sponges off miR-578 from HIF1α. On the other hand, miR-578 promotes apoptosis by targeting HIF1α (54). CircRNF20 also plays a role in cancer progression through stabilizing HIF1α and downstream signaling in breast cancer cells. Mechanistically, it sponges off miR-487a from mRNAs of HIF1α, which results in HIF1α mediated transcription of hexokinase II (HK2) and promotes glycolysis and proliferation in cancer cells (55). Similarly, miR-542-3p works as a negative regulator of HIF1α, but unfortunately it is downregulated in breast cancer cells through a HIF1α-driven feed-back loop. Mechanistically, increased hypoxia in cancer tissue masks the miR-542-3p mediated inhibition of HIF1α by upregulating circRBM33 via transcriptional activation by HIF1α itself. Resultantly, increased HIF1α expression induces proliferation and promotes glycolysis in cancer cells (56). Overall, multiple lncRNAs and circRNAs work as positive regulators of HIF-signaling by sponging of miRNAs, and targeting these ncRNAs in clinics provide a great avenue to limit hypoxic TME and associated tumor progression in breast cancer patients.
miRNA-mediated tightly controlled regulation of HIFs and other associated signaling factors is key determinant in bringing about the molecular changes behind the normoxia-hypoxia shift upon oxygen deprivation. For instance, HIF1α is a direct target of miR-143-5p, which inhibits tumorigenesis, cancer proliferation, and progression by suppressing HIF1α expression at the post-transcriptional level and solute carrier family 2 member 1 (GLUT1) pathway downstream of HIF1α signaling. Unfortunately, miR-143-5p expression is decreased in breast cancer clinical samples compared to adjacent healthy tissues, suggesting that hypoxia may overcome miR-143-5p induced HIF1α downregulation, though the underlying mechanism is still unexplored (57). Another miRNA, let-7d, also regulates HIF1α expression in breast cancer cells. Loss of let-7d and gain of HIF1α activity are in concordance in patient datasets and clinical breast cancer samples. Increased HIF1α expression and activity due to loss of let-7d leads to elevated expression of platelet-derived growth factors (PDGFA/B), resulting in increased brain metastasis of breast cancer cells (58). Expression of miR-18a from the miR17HG family is downregulated in a cell line-based experimental TNBC model in vitro and in vivo. Upregulation of this miRNA inhibits HIF1α expression through direct targeting and suppresses tumor growth at primary sites as well as metastasis to the lungs (59). Under hypoxic conditions, nuclear factor erythroid 2-like-2 (NRF2) aids in stabilizing HIF1α expression and subsequent downstream signaling by inhibiting miR-181c, which directly targets HIF1α. This supports cancer cells’ metabolic adaptation via heightened glycolysis, aiding in proliferation and survival (60). Alternatively, miR-140-5p may suppress hypoxia-driven tumor growth, angiogenesis, invasion, and metastasis via targeting NRF2, but its expression is downregulated upon hypoxia in breast cancer cells (61). High‐mobility group box protein 1 (HMGB1) works as a positive regulator of hypoxia by directly regulating HIF1α expression. This axis is fine-tuned by miR-141-3p, which post-transcriptionally inhibits HMGB1 expression upstream. Notably, miR-141-3p expression is low in breast cancer tissues and is associated with poor prognosis, but how hypoxia downregulates its expression in the first place is not yet established (62). LncRNA, Metallothionein 1 J, pseudogene (MT1JP), may suppress cancer cell proliferation and migration by transcriptionally activating miR-138, which directly targets HIF1α mRNA transcripts. Unfortunately, expression of both MTJ1P and miR-138 progressively downregulates as TNBC tumors advance towards an aggressive and hypoxic phenotype (63). Hypoxia-induced downregulation of miR-340-5p promotes EMT and metastasis in breast cancer cells by upregulating SIAH2 expression, which directly stabilizes HIF1α expression (64). In addition, miR-526b-3p may suppress hypoxia induced notch signaling and inhibit cancer cell stemness and chemoresistance by directly targeting HIF2α. This sensitizes cancer cells to paclitaxel treatment, as well as attenuates stem cell-like features (65). Although certain ncRNAs have the potential to counteract hypoxia by directly or indirectly regulating HIFs, these molecules are often downregulated in hypoxic, advanced stage breast cancers. Clinical activation of such axes may be fruitful in limiting hypoxia-driven tumor growth and progression in breast cancer.
2.2 ncRNAs as downstream effectors of hypoxia/HIF-signaling in breast cancer
In addition to their above discussed role in boosting HIF-signaling and orchestrating hypoxic TME, multiple ncRNAs are also regulated as the downstream effectors of HIF-signaling to directly endorse breast cancer by taking part in promoting cancer cell proliferation and progression, limiting apoptotic cell death, inducing angiogenesis, stimulating EMT and metastasis, resisting therapeutic interventions, acquiring stem cell-like features, and evading immune system (Figure 4).
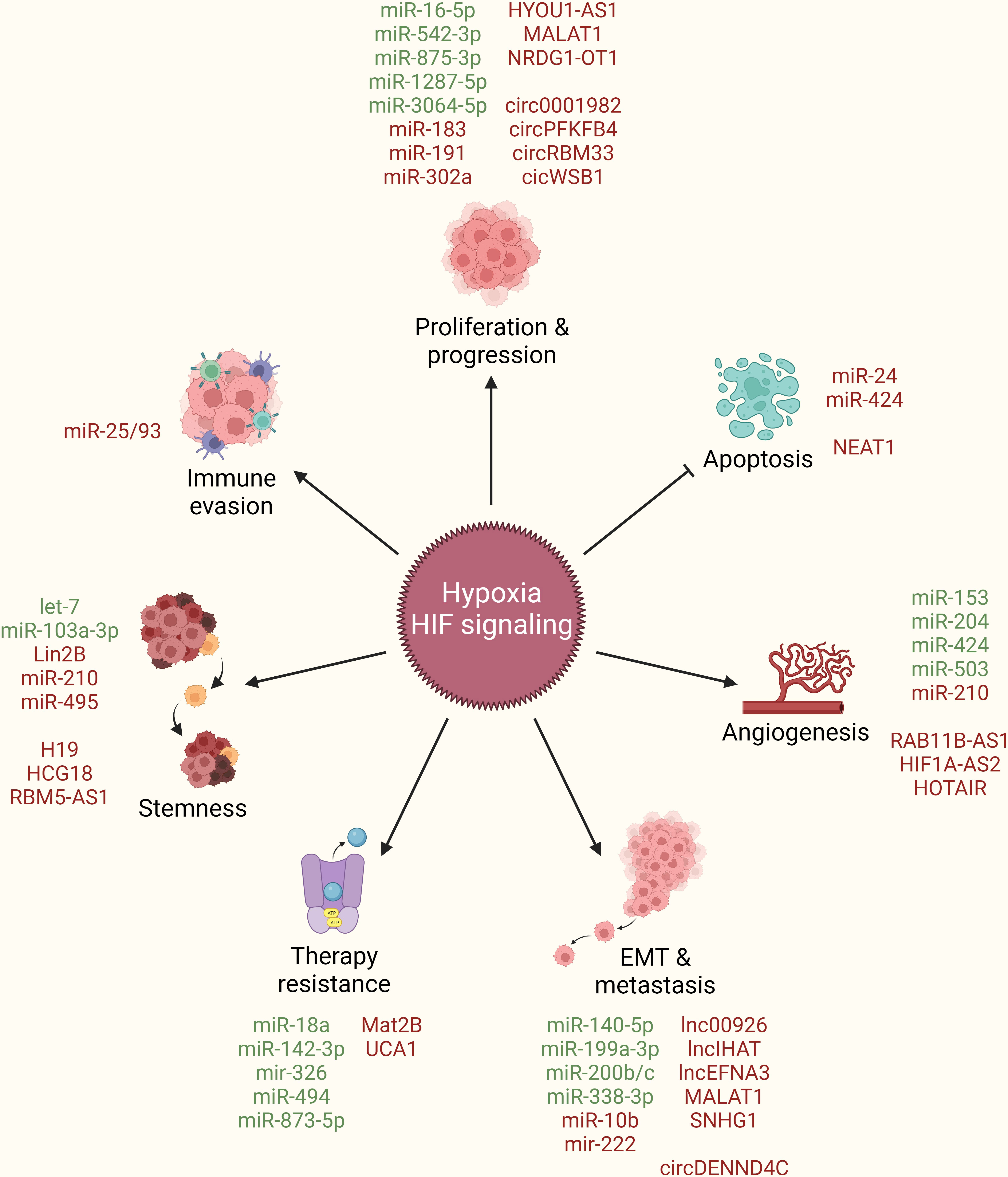
Figure 4 ncRNAs as downstream effectors of hypoxia/HIF-signaling in breast cancer. Hypoxia/HIF-signaling regulate the expression of different ncRNAs (miRNAs, lncRNAs, circRNAs) to promote cancer cell proliferation and progression, limit apoptotic cell death, induce angiogenesis, stimulate EMT and metastasis, resist therapeutic interventions, acquire stem cell-like features, and evade immune system. Green: Inhibited by hypoxia/HIF-signaling, Red: Activated by hypoxia/HIF-signaling, arrowhead: activation, blockhead: inhibition.
2.2.1 Proliferation and progression
Hypoxia regulates the expression of multiple ncRNAs, including miRNAs, lncRNAs, circRNAs, through varying mechanisms to promote breast cancer proliferation and progression. For instance, hypoxia induces the expression of the pro-tumorigenic driver YTH N6-methyladenosine RNA binding protein F1 (YTHDF1), and promotes breast cancer cell proliferation through dual mechanisms; first, by promoting its transcriptional activation in HIF1α-dependent manner, and second, by inhibiting the miR-16-5p-mediated post-transcriptional repression of YTHDF1. In return, YTHDF1 promotes glycolysis by activating PKM2 expression and promotes cancer cell proliferation and migration (66). As mentioned earlier, HIF1α may promote miR-183/96/182 cluster expression in an HBXIP-dependent manner, out of which miR-183 can target VHL mRNAs and inhibit its expression, thereby constitutively promoting HIF1α-driven tumorigenesis and cancer progression in a feedback loop mechanism. In addition, these miRNAs may also target SCO2 and PDHA1 and promote glucose metabolism in favor of cancer progression (42). Hypoxia induces miR-191 expression in HIFs-dependent manner and promotes breast tumor aggressiveness. In turn, miR-191 targets the RNA binding protein HUR and indirectly upregulates transforming growth factor beta 2 (TGFβ2) expression, which leads to upbeat TGFβ signaling and increases the migration of cancer cells (67). Cancer cells tend to decrease proliferation and migrate towards excessive nutrient availability during the early stages of hypoxia. In this line, miR-491 tend to inhibit proliferation by targeting B-cell lymphoma-extra large (Bcl-xl), whereas miR-302a tends to promote cell migration by targeting C-X-C chemokine receptor 4 (CXCR4) expression in cancer cells upon hypoxia (68) though molecular link between HIF-signaling and induction of these miRNAs is not established so far.
The Hpoxia up-regulated 1 (HYOU1) gene is associated with poor clinical outcomes in breast cancer patients. Interestingly, the same gene locus expresses a lncRNA from the opposite strand named HYOU1-AS1, which aids in HYOU1 mediated TNBC cell proliferation and progression through competitively binding to heterogeneous nuclear ribonucleoprotein A1 (hnRNPA1), an RNA binding protein that may post-transcriptionally inhibit HYOU1 mRNA (69). HIF1α transcriptionally activates the lncRNA N-Myc downstream regulated 1-overlapping 1 (NDRG1-OT1) in breast cancer cells, which aids in cancer progression by destabilizing its parent gene NDRG1 both at transcriptional and post-translational levels. At the transcriptional level, it impairs promoter activity by increasing the binding of hnRNPA1 and limiting KH-Type Splicing Regulatory Protein (KHSPR) accumulation at promoter site. At post-translational level, it promotes degradation of NDRG1 via the ubiquitin proteasome pathway (70, 71). lncRNA NDRG1-OT1 may work as a miRNA sponge to particularly inhibit a tumor suppressor miRNA, miR-875-3p, and promote cancer cell proliferation, though functional targets at the downstream of the HIF1α/NDRG1-OT1/miR-875-3p axis have not been established so far (72). Metastasis associated lung adenocarcinoma transcript 1 (MALAT1) is a hypoxia-responsive lncRNA that promotes proliferation and migration in breast cancer cells. Interestingly, MALAT1 can be transcribed in both HIF1α- and HIF2α-dependent manners and stimulate tumor growth and migration by sponging off miR-3064-5p in breast cancer cells, though downstream functional targets of miR-3064-5p have not been identified (73).
Hypoxia promotes breast cancer proliferation and progression by inducing certain circRNAs as well. For instance, upon transcriptional activation through HIF1α, circPFKFB4 binds to damage specific DNA binding protein 1 (DDB1)/DDB2 and aids in cullin-ring ligase-4 (CRL4)-DDB2 ubiquitin ligase assembly, which in turn promotes cancer cell proliferation by specifically increasing the degradation of tumor suppressor p27 (74). circWSB1 expression correlates with poor prognosis and clinical outcome in breast cancer patients. It is also induced in a HIF1α-dependent manner and promotes cancer proliferation by destabilizing p53. It primarily targets ubiquitin specific peptidase 10 (USP10), which no longer deubiquitinates its targets, leading to p53 degradation (75). Hypoxia-driven HIF1α expression transcriptionally activates circRBM33, which aids in further enhancing HIF1a expression by masking miR-542-3p mediated inhibition of HIF1α. Resultantly, increased HIF1α expression induces proliferation through elevated glycolysis in cancer cells (56). Hypoxia upregulates circ0001982, which sponges off miR-1287-5p from its target mucin 19 (MUC19) and promotes cancer cell growth, invasion, and migration through elevated glycolysis in vitro and in vivo (76). Hypoxia also induces circDENND4C expression in a HIF1α-dependent manner to promote proliferation in breast cancer cells (77), though downstream molecular mechanisms remain to be elucidated. Overall, multiple miRNAs, lncRNAs, and circRNAs directly contribute to cancer proliferation and progression downstream of HIF-signaling.
2.2.2 Evasion of apoptosis
Urothelial cancer associated 1 (UCA1) is a hypoxia-responsive lncRNA whose expression is upregulated in breast cancer tissues. It is primarily transcribed by HIF1α and promotes cell proliferation by inhibiting apoptotic cell death under hypoxic conditions, though downstream molecular mechanisms are not established yet (78). Alternatively, hypoxia induces the lncRNA nuclear paraspeckle assembly transcript 1 (NEAT1) in a HIF2α-dependent manner and reduces apoptotic cell death in breast cancer cells. Elevated NEAT1 expression in tumor tissues is associated with poor survival in breast cancer patients. Mechanistically, NEAT1 controls gene regulation through the formation of paraspeckle nuclear structures, leading to nuclear retention of certain proteins and RNAs in hypoxic environment. Notably, mRNA transcripts of junction adhesion molecule 1 (JAM1) are among targets of HIF2α/NEAT1-mediated gene regulation (79). Hypoxia induced miR-424 promotes resistance to chemotherapies such as doxorubicin and etoposide by targeting the apoptosis-related tumor suppressor programmed cell death 4 (PDCD4) in breast cancer cells (80). In addition to regulating HIF1α expression through inhibition of FIH1, miR-24 further aids cancer cells by targeting pro-apoptotic BCL2-like 11 (BCL2L11, Bim) to promote resistance towards chemotherapy-driven apoptosis in breast CSCs (41). These studies highlight that hypoxia aids cancer cells to evade apoptosis by regulating multiple ncRNAs downstream of HIF-signaling.
2.2.3 Angiogenesis
In order to access nutrients in bulk, cancer cells tend to initiate angiogenic molecular reprogramming under hypoxic conditions, which not only helps these cells to survive but also aids in the metastatic spread of cancer (81). The hypoxic core of tumor tissue may have differential expression of certain ncRNAs compared to the outer edges, which have an abundance of oxygen. For instance, miR-374a is lower in the center of tumor mass compared to the edges and may be associated with differential and opposite expression of its angiogenic targets VEGFA and vascular cell adhesion molecule 1(VCAM1) in tumor mass (82). Similarly, miR-20a and 20b are also differentially distributed within breast tumor mass, along with the opposite expression patterns of HIF1α and VEGFA in these tumors (83). miR-20b may inhibit VEGFA transcription and translation by simultaneously inhibiting HIF1α, STAT3, and VEGFA expression, thereby decreasing the nuclear accumulation of the HIF1α/STAT3 complex on the VEGFA promoter as well (84). VEGFA is also a direct target of miR-503. HBXIP regulates VEGFA expression and angiogenesis in breast cancer cells by inhibiting miR-503 expression as well as by hyperactivating PI3K/Akt-mediated HIF1α signaling (85). Upon hypoxia, HIF2α specifically induces transcriptional expression of the lncRNA RAB11B-AS1, which favors migration and invasion of cancer cells by inducing angiogenesis. Mechanistically, this lncRNA promotes the recruitment of RNA-polymerase II on the promoters of angiogenic genes such as VEGFA and angiopoietin-like 4 (ANGPTL4) (86). As mentioned earlier, hypoxia-driven ER stress induces miR-153 expression-mediated downregulation of HIF1α to fine-tune VEGFA expression and angiogenesis in TNBC (50), but this axis is counteracted by hypoxia mediated induction of the lncRNA HIF1α-AS2, which targets miR-153 and promotes angiogenesis (51). In vitro 3D culture studies illustrate that hypoxia drives vasculogenic mimicry in cancer cells to form channel-like networks resembling endothelial blood vessels. Independent studies show that miR-204 may inhibit the formation of such vascular channels by targeting focal adhesion kinase (FAK) (87) and CAMP responsive element binding protein 5 (CREB5) (88) under hypoxia. Notably, hypoxia counteracts the effects of miR-204 through the induction of the lncRNA named HOX transcript antisense RNA (HOTAIR), which induces angiogensis in breast cancer cells by sponging-off miR-204 from its targets (87). Certain cancer cells may express the hemostatic glycoprotein Von Willebrand factor (VWF) as well for their own benefits (89). Interestingly, hypoxia-induced Yin and Yang 1 (YY1) transcription factor works as a transcriptional activator of VWF, which in turn promotes angiogenesis and breast cancer cell migration. Although miR-424 works as a negative regulator of VWF expression and post-transcriptionally represses it, hypoxic environment downregulates miR-424 as well to further enhance the expression of VWF and associated angiogenic response (90). Furthermore, cancer cells tend to release excessive amount of exosomes under hypoxic conditions to communicate with surrounding cells. Hypoxic cancer cells preferentially release miR-210 loaded exosomes (91), which can work on other normoxic cancer cells within the TME, and promote VEGFA expression and angiogenesis by targeting vascularization modulators such as Ephrin A3 (EFNA3) and protein tyrosine phosphatase non-receptor type 1 (PTP1B) (92). These findings suggest that HIF-signaling-driven ncRNAs boost angiogenic signaling in the tumor that in turn promotes breast cancer cell survival.
2.2.4 EMT and metastasis
With increasing hypoxia as tumors grow in size and become more aggressive, cancer cells tend to undergo EMT, intravasate into the circulatory system, and extravasate to distant organs to develop metastatic tumors. HIFs and associated signaling mechanisms are already established as key players regulating breast cancer EMT and metastasis (93). In order to fine-tune global miRNA expression patterns, hypoxia silences DICER promoter by inhibiting H3K27me3 demethylases KDM6A/B resulting in reduced miRNA processing. In particular, EMT-related transcription factor zinc finger E-box binding homeobox 1 (ZEB1) gets upregulated due to repressed expression of miR-200 family miRNAs (37). Under hypoxia, HIF1α directly inhibits miR-338-3p transcription, leading to increased expression of its downstream target and EMT-related transcription factor, ZEB2, which then promotes cancer progression, EMT, and metastasis in an NF-kB and PI3K/Akt signaling-dependent manner (94). HIF1α also promotes metastasis in breast cancer through inhibiting miR-140-5p, which regulates NRF2/H-O1 signaling by directly targeting NRF2 expression. Notably, NRF2 may directly regulate HIF1α expression to promote hypoxia and subsequent tumor growth, angiogenesis, invasion, and metastasis in a feedback loop manner (61). JNK signaling stabilizes HIF1α expression to promote transcription of another EMT-related transcription factor, TWIST1, which then transcribes miR-10b to inhibit epithelial markers and escalate EMT and metastasis. Cellular Communication Network Factor 5 (CCN5) may fine-tune this signaling by inhibiting JNK signaling at the top (95). Hypoxia-driven preferential splicing of the methyl-CpG binding domain protein 2 (MBD2) gene to the MBD2a isoform promotes breast cancer metastasis. Mechanistically, HIF1α upregulates miR-222 expression, which in turn inhibits serine and arginine rich splicing factor 2 (SRSF2), the gene controlling alternative splicing of MDB2 transcripts, leading to preferential MDB2a production. As a result, MDB2a transcriptionally activates frizzled class receptor 1 (FZD1) expression and promotes EMT and metastasis in breast cancer cells (96).
Stress, driven by a lack of nutrients, induces the unfolder protein response (UPR) or endoplasmic reticulum (ER) stress response, which is mediated through the ER-localized transmembrane sensor IRE1 and its substrate XBP1. Interestingly, XBP1 interacts with HIF1α and assembles a transcriptional complex to regulate a set of genes, which promote cancer progression in TNBC (97). Notably, MALAT1 is one of the key targets at the downstream of the XBP1-HIF1α axis whose expression correlates with aggressive phenotype, and occurrence of metastasis in breast cancer patients through regulating MYC expression (98). Interestingly, HIF2α, along with HIF-independent mechanisms, also establishes chromatin interactions at the MALAT1 promoter to regulate its transcription and subsequent metastasis in breast cancer cells (99). HIF1α also induces lncRNA induced by hypoxia and abundant in TNBC (lncIHAT) expression to promote cancer cell survival and lung metastasis in vivo. This lncRNA then works in a cis-acting manner and promotes the expression of neighboring oncogenes, including pyruvate dehydrogenase kinase 1 (PDK1) and integrin subunit alpha 6 (ITGA6) (100). HIF1α-AS2 is upregulated in breast cancer tissue compared to adjacent normal tissues and is associated with poor clinical outcome in patients entailing advanced tumor grade, lymph node metastasis, and distant metastasis, along with shorter overall survival (101). Hypoxia also induces transcription of multiple lncRNAs from the EFNA3 locus in HIF-dependent manner, which post-transcriptionally stabilizes EFNA3 and aids in cancer cell extravasation into distant sites to develop metastatic tumors (102). LncRNA small nucleolar RNA host gene 1 (SNHG1) is also regulated by hypoxia. HIF1α may promote SNHG1 expression, which then binds to miR-199a-3p, rescuing its post-transcriptional target TFAM, and leads to breast cancer cell metastasis (103). LINC00926 is associated with good clinical outcome and survival in breast cancer patients. It suppresses proliferation, migration and invasion of breast cancer cells by inhibiting phosphoglycerate kinase 1 (PGK1) expression via promoting STUB1-driven ubiquitination of PGK1. Unfortunately, LINC00826 is downregulated in breast cancer tissue compared to healthy controls, and this downregulation is attributed to hypoxia-driven inhibition of forkhead box O3 (FOXO3A) dependent transcription of LINC00926, which results in advanced tumor growth and lung metastasis in vivo (104). Hypoxia induced circular RNA circDENND4C targets well established tumor suppressor miRNAs, miR-200b and miR-200c (105, 106), and sponges them off of their known targets to promote glycolysis, migration, invasion, and metastasis in breast cancer cells (107). Overall, these studies highlight the role of hypoxia-driven ncRNAs in facilitating breast cancer cells to undergo EMT and metastasize to distant organs.
2.2.5 Therapy resistance
Therapy resistance revolves around the limited intake of drug transport into cancer cells, the acquisition of mechanisms to get rid of the drug, and the activation of alternate signaling cascades to counteract the therapeutic effects of drug (108). Hypoxia inhibits miR-873-5p, thereby promoting the expression of its post transcriptional targets such as multi-drug resistance 1 (MDR1) and pregnane X receptor (PXR), which are involved in resisting drug influx into cancer cells (109). Hypoxic tumor microenvironment leads to inhibition of miR-326 expression in a HIF1α-dependent manner in cancer cells, in turn, promoting ITGA5 expression and hyperactivation of subsequent integrin signaling, resulting in chemotherapy resistance in TNBC cells (110). In hypoxic TNBC cells, HIF1α also drives lysyl oxidase (LOX) expression to regulate the tumor microenvironment via downstream integrin/FAK signaling and, as a result, confers resistance to chemotherapeutic agents. Here, HIF1α directly regulates LOX at the transcriptional level as well as inhibits miR-142-3p expression, which has the potential to simultaneously target HIF1α, LOX, and ITGA5 (111). Hypoxia induces docetaxel resistance in TNBC cells by inhibiting miR-494 in a HIF1α-dependent manner, thereby rescuing Survivin from miR-494 mediated degradation. Pharmacological targeting of HIF1α or overexpressing miR-494 sensitizes TNBC cells to docetaxel treatment in vivo (112). In addition, Hypoxia-induced miR-424 targets the apoptosis-related tumor suppressor PDCD4, rendering breast cancer cells resistant to chemotherapies such as doxorubicin and etoposide. Notably, miR-424 expression negatively correlates with that of PDCD4 in clinical samples of breast cancer as well (80). In in vitro multicellular tumor spheroids, hypoxia-induced lncRNA methionine adenosyl transferase 2 non-catalytic beta subunit (lncMAT2B) promotes cisplatin resistance by elevating DNA damage repair (113). Tamoxifen treatment in ER+ breast cancer cells may induce lncRNA UCA1 expression in a HIF1α-dependent manner, leading to tamoxifen resistance overtime. Mechanistically, UCA1 promotes hypoxic signaling by masking miR-18a mediated degradation of HIF1α through sponging off miR-18a, rendering miR-18a unable to inhibit HIF1α and its other targets involved in cell cycle progression (114). These findings suggest that hypoxia-driven inhibition of tumor suppressor ncRNAs and upregulation of oncogenic ncRNAs play crucial roles in conferring resistance to different therapeutic agents in breast cancer cells (Table 1).
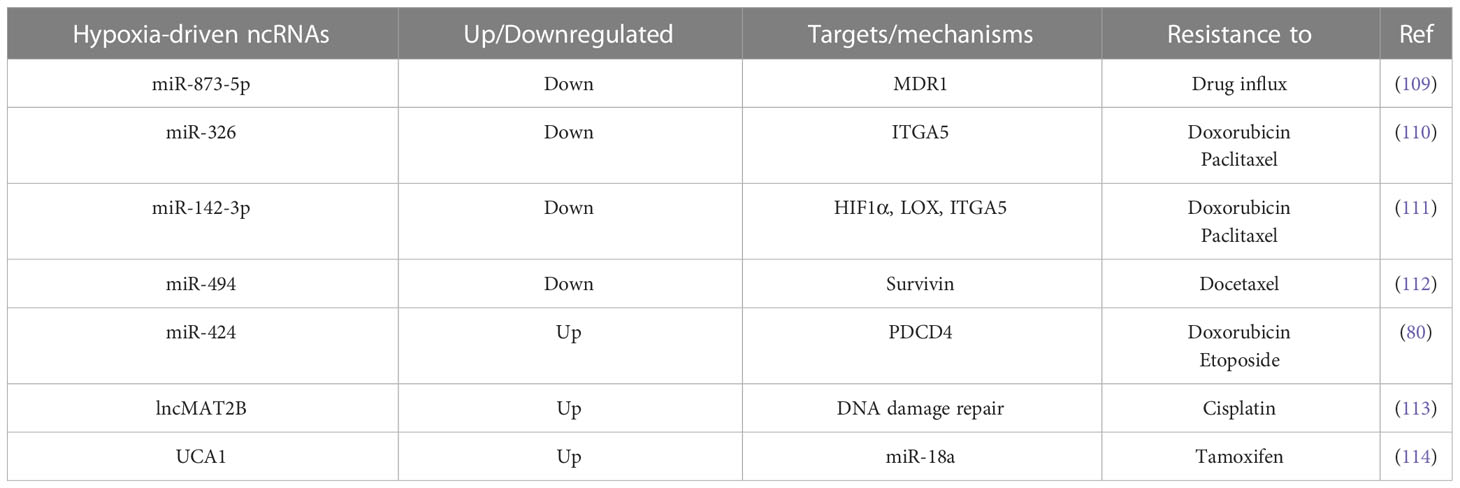
Table 1 List of hypoxia-driven ncRNAs with key targets and mechanisms through which resistance to different therapeutic agents is acquired upon hypoxia.
2.2.6 Stemness
Tumor heterogeneity, attributed to varying degrees of tumor-initiating potential or stemness among cancer cells, facilitates adapting to changing tumor microenvironment, maintaining tumor aggressiveness, and resisting therapeutic interventions. Hypoxia is now recognized as a key contributing factor to cancer stemness (115). For instance, hypoxia is shown to induce embryonic stem cell markers such as OCT4, NANOG, SOX2, KLF4, and cMYC, along with miR-302, in HIF-dependent manner in different cancer cell lines, including those of breast origin (116). Hypoxia-driven miR-210 expression is now well-established as a key regulator of metabolism, cell cycle progression, proliferation, angiogenesis, DNA damage response, and apoptosis in multiple cancer types, including breast cancer (117, 118). This miRNA also promote breast cancer cell stemness through targeting E-cadherin, which is also involved in limiting EMT and metastasis (119). Notably, breast CSCs overexpress the E12/E47 transcription factor to transcriptionally regulate miR-495 expression, which then promotes cell proliferation via DNA damage inducible transcript 4 (REDD1) inhibition and cell invasion via E-cadherin inhibition (120). Carbonic anhydrase IX (CAIX) is a hypoxia marker involved in pH regulation to facilitate cancer cells’ adaptation to the changing environment during hypoxic shift. Interestingly, CAIX enriched cancer cells exhibit CSC markers. Mechanistically, CIAX fine-tunes the double negative feedback loop between LIN28 and let-7 miRNA in favor of LIN28 overexpression, leading to elevated glycolysis through increased expression of PDK1 and PDH, contributing to the acquisition of CSC markers such as NANOG and ALDH1 (121). In addition, lncRNA H19 also targets miRNA let-7 under hypoxic conditions, leading to the release of HIF1α from let-7-mediated suppression, resulting in activation of PDK1 downstream and increased stemness in breast cancer cells (122). HIF1α transcriptionally regulate lncRNA HCG18 to promote cancer stemness and proliferation. Mechanistically, HCG18 sponges off miR-103a-3p from its target ubiquitin-conjugating enzyme E2O (UBE2O). UBE2O progressively promotes cancer growth and progression via upregulating AMPK/mTORC1, which induces HIF1α, completing a positive feedback loop to confer a continuous hypoxic environment favoring cancer cell progression and stemness (123). Hypoxia induced Runx family transcription factor 2 (RUNX2) transcriptionally activates the lncRNA RBM5-AS1 which is involved in maintaining breast cancer stemness through stabilizing the β-catenin-TCF4 transcriptional complex by preventing β-catenin degradation (124). Hypoxia-induced lncRNA KB-1980E6.3 is related to poor prognosis in breast cancer patients. It stabilizes c-Myc mRNA by recruiting insulin-like growth factor 2 mRNA-binding protein 1 (IGF2BP1) and promotes Myc signaling mediated self-renewal in breast CSCs (125). Stromal cells such as tumor associated fibroblasts may induce TGFβ signaling in breast cancer cells to hyperactivate the peroxisome proliferator activated receptor alpha (PPARα)/HIF1α axis, which regulates multiple survival and self-renewal genes including CAIX, apolipoprotein E (APoE), snail family transcriptional repressor 2 (Slug) and interleukin 6 (IL6) to promote cancer stemness. Although PPARγ can inhibit the PPARα/HIF1α axis through upregulating miR-17-5p expression, miR-130b is induced under hypoxia to counter the inhibitory effects of PPARγ on the PPARα/HIF1α axis (126). These findings suggest that HIF-signaling-driven ncRNAs aid cancer cells to acquire stem cell like characteristics.
2.2.7 Immune evasion
Hypoxia regulates immune suppression through the induction of miR-25 and miR-93 in a HIF1α independent manner. Upregulation of these miRNAs under hypoxia establishes an immunosuppressive environment characterized by an increased number of Treg cells, and a decreased population of CD8+ effector T cells. At the molecular level, these miRNAs are induced in a Tet methylcytosine dioxygenase 1 (TET1)-dependent manner and target the lysine acetyltransferase NCOA3 to inhibit transcription of cyclic-GMP-AMP synthase (cGAS), which works as a critical factor for the innate immune system in detecting cytosolic DNA. Notably, upregulated miR-25 and miR-93 expression inversely correlates with cGAS expression in clinical tumor samples and is associated with poor clinical outcome in breast cancer patients (127).
3 Clinical implications of hypoxia-driven ncRNAs in breast cancer
Owing to the fact that ncRNAs are often expressed in tissue- and cancer-type specific manners, tumor tissue-specific differential expression of ncRNAs may serve as prognostic biomarkers in different cancers (128). In relation to this, miR-210 is extensively studied as a hypoxia-driven prognostic ncRNA in breast cancer. It is regulated in HIF1α-dependent manner and correlates well with the hypoxia signature in breast cancer samples. In addition, it is a prognostic factor for poor disease-free and overall survival in breast cancer patients (129). Hypoxia-induced miR-210 may also serve as an independent prognostic factor in TNBC, as it is highly expressed in TNBCs compared to other subtypes of breast cancer. Moreover, it is also associated with poor overall and disease-free survival in TNBC patients (130). Hypoxia induced miR-210-3p may work as a predictive biomarker for docetaxel treatment in breast cancer as it is found to be associated with the worst outcome and poor survival in patient in response to docetaxel treatment in sequential therapy with anthracyclines (131). Furthermore, quantifying exosomal miRNA levels may also work as diagnostic tool as hypoxia not only increases the release of exosomes but also of miR-210 loaded exosomes from breast cancer cells (91). Single nucleotide polymorphisms in miRNA genes (miRSNPs) may profoundly alter global transcriptome compared to genetic polymorphisms in single gene and play an important role in different aspects of cancer biology. For instance, altered targeting of HIF1αN due to miRSNPs in the let-7 family miRNA is associated with predicting complete pathological response towards taxane and platinum based chemotherapeutic treatment in breast cancer patients (132). The hypoxia-related gene signature of twelve different lncRNAs is also proposed as a prognostic model to predict clinical outcomes such as therapeutic response, immune cell infiltration and survival in breast cancer patients (133). In addition, another set of four hypoxia-related lncRNAs is shown as a powerful tool to predict immune cell infiltration, and overall survival in breast cancer patients (134). Moreover, higher expression of hypoxia related circRNF20 aligns well with increased tumor volume and lymphatic metastasis, and predicts poor overall survival in breast cancer patients (55).
The most extraordinary aspect of ncRNAs is their presence in the circulatory system, which has led to their utilization as diagnostic and prognostic biomarkers in detecting cancers, monitoring the disease course, and assessing the therapeutic response over time. Due to their high stability and abundance, clinical quantification of circulating ncRNAs serves as an efficient, non-invasive tool for providing information about tumor growth and treatment efficacy (135). Only a few hypoxia-driven circulatory ncRNAs are reported in breast cancer so far. For instance, a bi-miRNA signature comprising hypoxia-driven oncogenic miR-210 and tumor suppressor miR-152 is proposed as a diagnostic biomarker of breast cancer. In addition, miR-152 plasma levels correlate well with different stages of the disease (136). Similarly, a tri-ncRNA signature comprising AS-ncRNA in the INK4 locus (ANRIL), HIF1α-AS2, and UCA1 may serve as diagnostic biomarker for TNBC as these lncRNAs are frequently upregulated in the plasma of TNBC patients compared to non-TNBC patients (137). Overall, hypoxia-driven ncRNAs, whether tumor-specific or circulating ncRNAs, are potential candidates to be utilized as diagnostic and prognostic biomarkers of disease progression and therapeutic response in breast cancer.
4 Targeting hypoxia-driven ncRNAs in breast cancer
Given their central role behind cancer progression in solid tumors, such as breast cancer, directly targeting HIFs provides an excellent therapeutic option as a treatment for solid tumors in clinical settings (138–140). The scientific community has extensively invested over the years and utilized multiple approaches such as inhibition of HIF mRNA expression, protein synthesis, dimerization, DNA binding, and transcriptional activities to identify potent HIF inhibitors. As a result, multiple HIF targeting inhibitors are now being tested in clinical trials to be approved for commercial use alone or in combination with other therapeutic regimens against multiple advanced stage cancers. Notable mentions include 2ME2 NCD (panzem) that inhibits HIF protein synthesis and transcriptional activity, EZN-2208 that suppresses HIF1 mRNA expression, Vorinostat (SAHA) and antibiotic (geldanamycin)-derived 17-AAG which increase HIF degradation, and HIF2α antagonists PT2385 and PT2977 (141). Targeting downstream effectors of HIF signaling is another promising approach to countering overall hypoxia-driven cancer aggressiveness (142). For instance, limiting hypoxia-driven secretion of heat shock protein 90α (HSP90α) by targeting a specific 115-amino acid long peptide is proposed to inhibit distant metastasis in breast cancer (143). Though still in infancy, a few studies have explored the ways to target hypoxia-driven ncRNAs in breast cancer and have shown that disrupting ncRNA mediated adaptive response to hypoxia my aid in directing hypoxic signaling to work in favor of killing cancer cells. No doubt, miR-210 is the most studied miRNA downstream of hypoxic signaling in cancer. Notably, a small molecule named Targapremir-210 that attaches to the DICER binding sites of pre-miR-210 leading to inhibited production of mature miRNA, resulting in upregulation of its targets. Glycerol-3-phosphate dehydrogenase 1-like enzyme (GPD1L) is identified as a target that not only promotes HIF1α degradation at the upstream through inhibiting PHDs but also triggers apoptosis in hypoxic breast cancer cells both in vitro and in vivo (144). Moreover, a small molecule named “1” inhibits the production of miR-544, specifically under hypoxic conditions. Mechanistically, this molecule directly targets DICER and DROSHA binding sites in pre-miR-544 transcripts, leading to depression of its mRNA targets, and hyperactivates mTOR signaling downstream, resulting in chemosensitivity and apoptotic cell death (145). Advances in nanotechnology based drug delivery options have greatly facilitated the devising of therapeutic strategies to achieve targeted delivery of ncRNAs to cancer cells (146). For instance, combined delivery of tumor suppressor miR-205 and metastasis suppressor miR-182 along with the chemotherapeutic agent doxorubicin using RNA hydrogel-based nanoparticles alleviates hypoxia-driven TNBC progression and metastasis (147). Hypoxia-driven downregulation of miR-340-5p promotes EMT and metastasis in breast cancer cells by upregulating E3 ubiquitin ligase SIAH2, which directly stabilizes HIF1α. Sinomenine, a herbal alkaloid, upregulates miR-340-5p expression and exerts its anticancer activities through inhibiting the SIAH2/HIF1α axis downstream (64). Despite recent advances in the utilization of ncRNA-based therapies, targeting hypoxia-driven ncRNAs in cancer, in general, and in breast cancer, in particular, is still evolving and awaits further biological insights to reach from bench to bedside.
5 Challenges and future prospect
Hypoxia-driven non-coding genome is expanding exponentially, with a lot of different ncRNAs, including miRNAs, lncRNAs, circRNAs being identified as downstream target of hypoxia or HIF-signaling, which also actively contribute to hypoxia-driven cancer progression (148–150). Of note, advances in computational pipelines to detect ncRNAs are playing a pivotal role in providing novel insights into the biogenesis and function of ncRNAs under hypoxic stress (151). Although extensive use of genome-wide approaches to identify ncRNA transcribing regions in the genome is underway, a lot of ncRNAs are still hiding out there in our genome and need to be explored for their contribution to biological processes (152, 153). Moreover, the biological functions of certain ncRNAs, such as piRNAs, snRNAs, and snoRNAs, are still underexplored in cancer biology (30). Therefore, we are still midway towards identifying the true transcriptional spectrum of hypoxia in cancer as well as towards understanding the true nature of ncRNAs in cellular mechanisms, in general, and in hypoxia, in particular. Another level of complexity in understanding the role of hypoxia-driven ncRNAs in breast cancer aggressiveness arises from the fact that certain ncRNAs may regulate and/or get regulated by HIF signaling simultaneously. On top of that, hypoxia may regulate the expression of different ncRNAs in a HIF-independent manner as well.
Tissue-specific expression and circulating levels of certain ncRNAs are now being utilized in clinics for the diagonosis and prognosis of diseases (30, 154). Similarly, tumor tissue specific expression or circulating levels of a bunch of hypoxia-driven ncRNAs are already proposed to be used as diagnostic and prognostic markers of disease progression, tumor stage, survival, therapeutic response, and immune cell infiltration in breast cancer. Of note, hypoxia-driven miR-210 is a prime candidate to become a standard in identifying the extent of hypoxia-driven tumor aggressiveness in different cancers in addition to breast cancer (118). With the advancements in ncRNA-targeted therapies in cancer (155, 156), as well as with the improvements in nanotechnology-based techniques to efficiently deliver such ncRNAs (146), it is need of time that miR-210 targeting TargapremiR-210 also get tested in clinical settings on an urgent basis against hypoxia-driven aggressive, advanced stage, and metastatic solid cancers.
Another underexplored area in HIF biology is how these transcription factors and downstream signaling pathways may behave if activated under normoxia. Initial evidence suggests that miR-101-mediated downregulation of VHL induces HIF1α-driven cell cycle arrest and apoptosis in cancer cells under normoxia (157). It is in part in line with the findings that HIFs may upregulate the expression of tumor suppressor lncRNA mitochondrial oxygen-responsive transcript 1 (MTORT1), which sponges off miR-26a-5p from its targets CREB1 and serine/threonine kinase 4 (STK4), and inhibits cell growth and proliferation in breast cancer cells (158), in contrast to the well-established tumor promoting functions of HIFs under hypoxic conditions. Therefore, exploring the downstream effectors of HIFs, including both protein-coding and ncRNAs, under normoxia as well as modulating HIFs under normoxia may also advance an alternative way to tackle tumor aggressiveness in cancer, in general, and in breast cancer, in particular.
6 Conclusion
Hypoxia is now established as the driving force behind tumor aggressiveness, leading to therapy resistance, metastasis, and stemness in solid tumors, including those of breast cancer. With the great advancements in exploring the regulatory roles of non-coding genome in recent years, hypoxia-responsive genome now encompasses multiple types of ncRNAs, such as miRNAs, lncRNAs, and circRNAs. These RNAs are often transcriptionally regulated downstream of HIFs and contribute to orchestrating aggressive hypoxic tumor environment by working as downstream effectors in cellular processes such as proliferation, evasion of apoptotic death, extracellular matrix remodeling, angiogenesis, migration, invasion, EMT, metastasis, therapy resistance, stemness, and evasion of the immune system in breast cancer. Alternatively, hypoxia drives the expression of certain ncRNAs in a HIF-independent manner as well, which may eventually work as upstream modulators of HIF signaling in breast cancer. Interplay between ncRNAs as well as feedback and feedforward loops between ncRNAs and HIFs further adds to the complexity of understanding hypoxia-driven ncRNAs in cancer, in general and in breast cancer, in particular. Certain hypoxia-driven ncRNAs are already proposed to be used as diagnostic and prognostic markers of disease course in breast cancer. Overcoming the challenges in devising ncRNA-targeted therapies, especially the development of efficient delivery systems in the future, will efficiently aid in the utilization and targeting of hypoxia-driven ncRNAs to treat aggressive hypoxic breast cancer in clinics.
Author contributions
HHA, KRZ, XX and QH wrote the manuscript. HHA prepared figures. UR reviewed the manuscript. QH and TZ devised the layout and supervised the manuscript. All authors read and approved the final manuscript.
Funding
This work was supported by the Start-up Fund for High-level Talents in Affiliated Hospital of Guangdong Medical University (Grant No. 51301Z20200007), Discipline construction project of Guangdong Medical University (Grant No. 4SG21266P and 4SG21276P), Guangdong Basic and Applied Basic Research Foundation (Grant no. 2023A1515010235), and Basic and Applied Basic Research Project of GuangZhou (Grant no. SL2022A04J00207).
Conflict of interest
The authors declare that the research was conducted in the absence of any commercial or financial relationships that could be construed as a potential conflict of interest.
Publisher’s note
All claims expressed in this article are solely those of the authors and do not necessarily represent those of their affiliated organizations, or those of the publisher, the editors and the reviewers. Any product that may be evaluated in this article, or claim that may be made by its manufacturer, is not guaranteed or endorsed by the publisher.
References
1. Sung H, Ferlay J, Siegel RL. Global Cancer Statistics 2020: GLOBOCAN Estimates of Incidence and Mortality Worldwide for 36 Cancers in 185 Countries. CA Cancer J Clin (2021) 71:209–49. doi: 10.3322/caac.21660
2. Jin H, Du W, Huang W, Yan J, Tang Q, Chen Y, et al. lncRNA and breast cancer: Progress from identifying mechanisms to challenges and opportunities of clinical treatment. Mol Ther Nucleic Acids (2021) 25:613–37. doi: 10.1016/j.omtn.2021.08.005
3. Polyak K. Breast cancer: origins and evolution. J Clin Invest (2007) 117:3155–63. doi: 10.1172/JCI33295
4. Perou CM, Sørlie T, Eisen MB, van de Rijn M, Jeffrey SS, Rees CA, et al. Molecular portraits of human breast tumours. Nature (2000) 406:747–52. doi: 10.1038/35021093
5. Ignatiadis M, Sotiriou C. Luminal breast cancer: from biology to treatment. Nat Rev Clin Oncol (2013) 10:494–506. doi: 10.1038/nrclinonc.2013.124
6. Goutsouliak K, Veeraraghavan J, Sethunath V, De Angelis C, Osborne CK, Rimawi MF, et al. Towards personalized treatment for early stage HER2-positive breast cancer. Nat Rev Clin Oncol (2020) 17:233–50. doi: 10.1038/s41571-019-0299-9
7. Badve S, Dabbs DJ, Schnitt SJ, Baehner FL, Decker T, Eusebi V, et al. Basal-like and triple-negative breast cancers: a critical review with an emphasis on the implications for pathologists and oncologists. Mod Pathol (2011) 24:157–67. doi: 10.1038/modpathol.2010.200
8. Marusyk A, Janiszewska M, Polyak K. Intratumor Heterogeneity: The Rosetta Stone of Therapy Resistance. Cancer Cell (2020) 37:471–84. doi: 10.1016/j.ccell.2020.03.007
9. Harbeck N, Penault-Llorca F, Cortes J, Gnant M, Houssami N, Poortmans P, et al. Breast cancer. Nat Rev Dis Primers (2019) 5:66. doi: 10.1038/s41572-019-0111-2
10. Brahimi-Horn MC, Chiche J, Pouysségur J. Hypoxia and cancer. J Mol Med (Berlin Germany) (2007) 85:1301–7. doi: 10.1007/s00109-007-0281-3
11. Toffoli S, Michiels C. Intermittent hypoxia is a key regulator of cancer cell and endothelial cell interplay in tumours. FEBS J (2008) 275:2991–3002. doi: 10.1111/j.1742-4658.2008.06454.x
12. McKeown SR. Defining normoxia, physoxia and hypoxia in tumours-implications for treatment response. Br J Radiol (2014) 87:20130676. doi: 10.1259/bjr.20130676
13. Carreau A, El Hafny-Rahbi B, Matejuk A, Grillon C, Kieda C. Why is the partial oxygen pressure of human tissues a crucial parameter? Small molecules hypoxia. J Cell Mol Med (2011) 15:1239–53. doi: 10.1111/j.1582-4934.2011.01258.x
14. Singh M, Devi U, Roy S, Gupta PS, Saraf SA, Kaithwas G. Prolyl hydroxylase mediated inhibition of fatty acid synthase to combat tumor growth in mammary gland carcinoma. Breast Cancer (Tokyo Japan) (2016) 23:820–9. doi: 10.1007/s12282-016-0683-6
15. Rani S, Roy S. Regulation of Transactivation at C-TAD Domain of HIF-1α by Factor-Inhibiting HIF-1α (FIH-1): A Potential Target for Therapeutic Intervention in Cancer. Oxid Med Cell Longev (2022) 2022:2407223. doi: 10.1155/2022/2407223
16. Tirpe AA, Gulei D, Ciortea SM. Hypoxia: Overview on Hypoxia-Mediated Mechanisms with a Focus on the Role of HIF Genes. Int J Mol Sci (2019) 20(24):6140. doi: 10.3390/ijms20246140
17. Lee JW, Ko J. Hypoxia signaling in human diseases and therapeutic targets. Exp Mol Med (2019) 51:1–13. doi: 10.1038/s12276-019-0235-1
18. Sørensen BS, Horsman MR. Tumor Hypoxia: Impact on Radiation Therapy and Molecular Pathways. Front Oncol (2020) 10:562. doi: 10.3389/fonc.2020.00562
19. Arsham AM, Howell JJ, Simon MC. A novel hypoxia-inducible factor-independent hypoxic response regulating mammalian target of rapamycin and its targets. J Biol Chem (2003) 278:29655–60. doi: 10.1074/jbc.M212770200
20. Mizukami Y, Kohgo Y, Chung DC. Hypoxia inducible factor-1 independent pathways in tumor angiogenesis. Clin Cancer Res (2007) 13:5670–4. doi: 10.1158/1078-0432.CCR-07-0111
22. Carrington JC, Ambros V. Role of microRNAs in plant and animal development. Science (2003) 301:336–8. doi: 10.1126/science.1085242
23. Friedman RC, Farh KK, Burge CB, Bartel DP. Most mammalian mRNAs are conserved targets of microRNAs. Genome Res (2009) 19:92–105. doi: 10.1101/gr.082701.108
24. Dragomir MP, Knutsen E, Calin GA. SnapShot: Unconventional miRNA Functions. Cell (2018) 174:1038–1038.e1. doi: 10.1016/j.cell.2018.07.040
25. Fabbri M, Girnita L, Varani G, Calin GA. Decrypting noncoding RNA interactions, structures, and functional networks. Genome Res (2019) 29:1377–88. doi: 10.1101/gr.247239.118
26. Quinn JJ, Chang HY. Unique features of long non-coding RNA biogenesis and function. Nat Rev Genet (2016) 17:47–62. doi: 10.1038/nrg.2015.10
27. Hansen TB, Jensen TI, Clausen BH, Bramsen JB, Finsen B, Damgaard CK, et al. Natural RNA circles function as efficient microRNA sponges. Nature (2013) 495:384–8. doi: 10.1038/nature11993
28. Agostini M, Ganini C. The role of noncoding RNAs in epithelial cancer. Cell Death Discov (2020) 6:13. doi: 10.1038/s41420-020-0247-6
29. Anastasiadou E, Jacob LS, Slack FJ. Non-coding RNA networks in cancer. Nat Rev Cancer (2018) 18:5–18. doi: 10.1038/nrc.2017.99
30. Esteller M. Non-coding RNAs in human disease. Nat Rev Genet (2011) 12:861–74. doi: 10.1038/nrg3074
31. Barreca MM, Zichittella C, Alessandro R, Conigliaro A. Hypoxia-Induced Non-Coding RNAs Controlling Cell Viability in Cancer. Int J Mol Sci (2021) 22:1857. doi: 10.3390/ijms22041857
32. Nallamshetty S, Chan SY, Loscalzo J. Hypoxia: a master regulator of microRNA biogenesis and activity. Free Radic Biol Med (2013) 64:20–30. doi: 10.1016/j.freeradbiomed.2013.05.022
33. Peng PH, Hsu KW, Chieh-Yu Lai J, Wu KJ. The role of hypoxia-induced long noncoding RNAs (lncRNAs) in tumorigenesis and metastasis. BioMed J (2021) 44:521–33. doi: 10.1016/j.bj.2021.03.005
34. Choudhry H, Harris AL, McIntyre A. The tumour hypoxia induced non-coding transcriptome. Mol Aspects Med (2016) 47-48:35–53. doi: 10.1016/j.mam.2016.01.003
35. Raza U, Zhang JD, Sahin O. MicroRNAs: master regulators of drug resistance, stemness, and metastasis. J Mol Med (Berlin Germany) (2014) 92:321–36. doi: 10.1007/s00109-014-1129-2
36. Nigita G, Acunzo M, Romano G, Veneziano D, Laganà A, Vitiello M, et al. microRNA editing in seed region aligns with cellular changes in hypoxic conditions. Nucleic Acids Res (2016) 44:6298–308. doi: 10.1093/nar/gkw532
37. van den Beucken T, Koch E, Chu K, Rupaimoole R, Prickaerts P, Adriaens M, et al. Hypoxia promotes stem cell phenotypes and poor prognosis through epigenetic regulation of DICER. Nat Commun (2014) 5:5203. doi: 10.1038/ncomms6203
38. Camps C, Saini HK, Mole DR, Choudhry H, Reczko M, Guerra-Assunção JA, et al. Integrated analysis of microRNA and mRNA expression and association with HIF binding reveals the complexity of microRNA expression regulation under hypoxia. Mol Cancer (2014) 13:28. doi: 10.1186/1476-4598-13-28
39. Shen J, Xia W, Khotskaya YB, Huo L, Nakanishi K, Lim SO, et al. EGFR modulates microRNA maturation in response to hypoxia through phosphorylation of AGO2. Nature (2013) 497:383–7. doi: 10.1038/nature12080
40. Chiang CH, Chu PY, Hou MF, Hung WC. MiR-182 promotes proliferation and invasion and elevates the HIF-1α-VEGF-A axis in breast cancer cells by targeting FBXW7. Am J Cancer Res (2016) 6:1785–98.
41. Roscigno G, Puoti I, Giordano I, Donnarumma E, Russo V, Affinito A, et al. MiR-24 induces chemotherapy resistance and hypoxic advantage in breast cancer. Oncotarget (2017) 8:19507–21. doi: 10.18632/oncotarget.14470
42. Liu F, Zhang W, You X, Liu Y, Li Y, Wang Z, et al. The oncoprotein HBXIP promotes glucose metabolism reprogramming via downregulating SCO2 and PDHA1 in breast cancer. Oncotarget (2015) 6:27199–213. doi: 10.18632/oncotarget.4508
43. Wang J, Chen X, Hu H, Yao M, Song Y, Yang A, et al. PCAT-1 facilitates breast cancer progression via binding to RACK1 and enhancing oxygen-independent stability of HIF-1α. Mol Ther Nucleic Acids (2021) 24:310–24. doi: 10.1016/j.omtn.2021.02.034
44. Zhao L, Jiang L, Zhang M, Zhang Q, Guan Q, Li Y, et al. NF-κB-activated SPRY4-IT1 promotes cancer cell metastasis by downregulating TCEB1 mRNA via Staufen1-mediated mRNA decay. Oncogene (2021) 40:4919–29. doi: 10.1038/s41388-021-01900-8
45. Zheng F, Chen J, Zhang X, Wang Z. The HIF-1α antisense long non-coding RNA drives a positive feedback loop of HIF-1α mediated transactivation and glycolysis. Nature Commun (2021) 12:1341. doi: 10.1038/s41467-021-21535-3
46. Du Y, Wei N, Ma R, Jiang SH, Song D. Long Noncoding RNA MIR210HG Promotes the Warburg Effect and Tumor Growth by Enhancing HIF-1α Translation in Triple-Negative Breast Cancer. Front Oncol (2020) 10:580176. doi: 10.3389/fonc.2020.580176
47. Wang Y, Liu S. LncRNA GHET1 Promotes Hypoxia-Induced Glycolysis, Proliferation, and Invasion in Triple-Negative Breast Cancer Through the Hippo/YAP Signaling Pathway. Front Cell Dev Biol (2021) 9:643515. doi: 10.3389/fcell.2021.643515
48. Zhang J, Du C, Zhang L, Wang Y, Zhang Y, Li J. LncRNA LINC00649 promotes the growth and metastasis of triple-negative breast cancer by maintaining the stability of HIF-1α through the NF90/NF45 complex. Cell Cycle (2022) 21:1034–47. doi: 10.1080/15384101.2022.2040283
49. Liang H, Ge F, Xu Y, Xiao J, Zhou Z, Liu R, et al. miR-153 inhibits the migration and the tube formation of endothelial cells by blocking the paracrine of angiopoietin 1 in breast cancer cells. Angiogenesis (2018) 21:849–60. doi: 10.1007/s10456-018-9630-9
50. Liang H, Xiao J, Zhou Z, Wu J, Ge F, Li Z, et al. Hypoxia induces miR-153 through the IRE1α-XBP1 pathway to fine tune the HIF1α/VEGFA axis in breast cancer angiogenesis. Oncogene (2018) 37:1961–75. doi: 10.1038/s41388-017-0089-8
51. Li L, Wang M, Mei Z, Cao W, Yang Y, Wang Y, et al. lncRNAs HIF1A-AS2 facilitates the up-regulation of HIF-1α by sponging to miR-153-3p, whereby promoting angiogenesis in HUVECs in hypoxia. BioMed Pharmacother (2017) 96:165–72. doi: 10.1016/j.biopha.2017.09.113
52. Du P, Luo K, Li G, Zhu J, Xiao Q. Long non-coding RNA VCAN-AS1 promotes the malignant behaviors of breast cancer by regulating the miR-106a-5p-mediated STAT3/HIF-1α pathway. Bioengineered (2021) 12:5028–44. doi: 10.1080/21655979.2021.1960774
53. Cheng L, Xing Z, Zhang P, Xu W. Long non-coding RNA LINC00662 promotes proliferation and migration of breast cancer cells via regulating the miR-497-5p/EglN2 axis. Acta Biochim Pol (2020) 67:229–37. doi: 10.18388/abp.2020_5203
54. Chen Z, Wang F, Xiong Y, Wang N, Gu Y, Qiu X. CircZFR functions as a sponge of miR-578 to promote breast cancer progression by regulating HIF1A expression. Cancer Cell Int (2020) 20:400. doi: 10.1186/s12935-020-01492-5
55. Cao L, Wang M, Dong Y, Xu B, Chen J, Ding Y, et al. Circular RNA circRNF20 promotes breast cancer tumorigenesis and Warburg effect through miR-487a/HIF-1α/HK2. Cell Death Dis (2020) 11:145. doi: 10.1038/s41419-020-2336-0
56. Jiang Y, Zhang M, Yu D, Hou G, Wu J, Li F. CircRBM33 downregulation inhibits hypoxia-induced glycolysis and promotes apoptosis of breast cancer cells via a microRNA-542-3p/HIF-1α axis. Cell Death Discov (2022) 8:126. doi: 10.1038/s41420-022-00860-6
57. Xu J, Li X, Zhang P, Luo J, Mou E, Liu S. miR-143-5p suppresses breast cancer progression by targeting the HIF-1α-related GLUT1 pathway. Oncol Lett (2022) 23:147. doi: 10.3892/ol.2022.13268
58. Wyss CB, Duffey N, Peyvandi S, Barras D, Martinez Usatorre A. Gain of HIF1 Activity and Loss of miRNA let-7d Promote Breast Cancer Metastasis to the Brain via the PDGF/PDGFR Axis. Cancer Res (2021) 81:594–605. doi: 10.1158/0008-5472.CAN-19-3560
59. Krutilina R, Sun W, Sethuraman A, Brown M, Seagroves TN, Pfeffer LM, et al. MicroRNA-18a inhibits hypoxia-inducible factor 1α activity and lung metastasis in basal breast cancers. Breast Cancer Res (2014) 16:R78. doi: 10.1186/bcr3693
60. Lee S, Hallis SP, Jung KA, Ryu D, Kwak MK. Impairment of HIF-1α-mediated metabolic adaption by NRF2-silencing in breast cancer cells. Redox Biol (2019) 24:101210. doi: 10.1016/j.redox.2019.101210
61. Mahajan M, Sitasawad S. miR-140-5p Attenuates Hypoxia-Induced Breast Cancer Progression by Targeting Nrf2/HO-1 Axis in a Keap1-Independent Mechanism. Cells (2021) 11(1):12. doi: 10.3390/cells11010012
62. Sun S, Ma J, Xie P, Wu Z, Tian X. Hypoxia-responsive miR-141-3p is involved in the progression of breast cancer via mediating the HMGB1/HIF-1α signaling pathway. J Gene Med (2020) 22:. doi: 10.1002/jgm.3230
63. Wang G, Dong Y, Liu H, Ji N, Cao J, Liu A, et al. (lncRNA) metallothionein 1 J, pseudogene (MT1JP) is downregulated in triple-negative breast cancer and upregulates microRNA-138 (miR-138) to downregulate hypoxia-inducible factor-1α (HIF-1α). Bioengineered (2022) 13:13718–27. doi: 10.1080/21655979.2022.2077906
64. Song L, Tang L. Sinomenine Inhibits Vasculogenic Mimicry and Migration of Breast Cancer Side Population Cells via Regulating miR-340-5p/SIAH2 Axis. Biomed Res Int (2022) 2022:4914005. doi: 10.1155/2022/4914005
65. Liu JH, Li WT, Yang Y, Qi YB, Cheng Y, Wu JH. MiR-526b-3p Attenuates Breast Cancer Stem Cell Properties and Chemoresistance by Targeting HIF-2α/Notch Signaling. Front Oncol (2021) 11:696269. doi: 10.3389/fonc.2021.696269
66. Yao X, Li W, Li L. YTHDF1 upregulation mediates hypoxia-dependent breast cancer growth and metastasis through regulating PKM2 to affect glycolysis. Cell Death Dis (2022) 13:258. doi: 10.1038/s41419-022-04711-1
67. Nagpal N, Ahmad HM, Chameettachal S, Sundar D, Ghosh S, Kulshreshtha R. HIF-inducible miR-191 promotes migration in breast cancer through complex regulation of TGFβ-signaling in hypoxic microenvironment. Sci Rep (2015) 5:9650. doi: 10.1038/srep09650
68. Zhang M, Gao CE, Chen WL, Tang YY, Nie JY, Shen LD, et al. Opposite response to hypoxia by breast cancer cells between cell proliferation and cell migration: A clue from microRNA expression profile. Oncol Lett (2018) 15:2771–80. doi: 10.3892/ol.2017.7636
69. Hao A, Wang Y, Zhang X, Li J, Li Y, Li D, et al. Long non-coding antisense RNA HYOU1-AS is essential to human breast cancer development through competitive binding hnRNPA1 to promote HYOU1 expression. Biochim Biophys Acta Mol Cell Res (2021) 1868:118951. doi: 10.1016/j.bbamcr.2021.118951
70. Lin HC, Yeh CC, Chao LY, Tsai MH, Chen HH, Chuang EY, et al. The hypoxia-responsive lncRNA NDRG-OT1 promotes NDRG1 degradation via ubiquitin-mediated proteolysis in breast cancer cells. Oncotarget (2018) 9:10470–82. doi: 10.18632/oncotarget.23732
71. Yeh CC, Luo JL, Nhut Phan N, Cheng YC, Chow LP, Tsai MH, et al. Different effects of long noncoding RNA NDRG1-OT1 fragments on NDRG1 transcription in breast cancer cells under hypoxia. RNA Biol (2018) 15:1487–98. doi: 10.1080/15476286.2018.1553480
72. Chao HH, Luo JL, Hsu MH, Chen LH, Lu TP, Tsai MH, et al. Regulatory mechanisms and function of hypoxia-induced long noncoding RNA NDRG1-OT1 in breast cancer cells. Cell Death Dis (2022) 13:807. doi: 10.1038/s41419-022-05253-2
73. Shih CH, Chuang LL, Tsai MH, Chen LH, Chuang EY, Lu TP, et al. Hypoxia-Induced MALAT1 Promotes the Proliferation and Migration of Breast Cancer Cells by Sponging MiR-3064-5p. Front Oncol (2021) 11:658151. doi: 10.3389/fonc.2021.658151
74. Chen H, Yang R, Xing L, Wang B, Liu D, Ou X, et al. Hypoxia-inducible CircPFKFB4 Promotes Breast Cancer Progression by Facilitating the CRL4(DDB2) E3 Ubiquitin Ligase-mediated p27 Degradation. Int J Biol Sci (2022) 18:3888–907. doi: 10.7150/ijbs.72842
75. Yang R, Chen H, Xing L, Wang B, Hu M, Ou X, et al. Hypoxia-induced circWSB1 promotes breast cancer progression through destabilizing p53 by interacting with USP10. Mol Cancer (2022) 21:88. doi: 10.1186/s12943-022-01567-z
76. Qiu Z, Wang L, Liu H. Hsa_circ_0001982 promotes the progression of breast cancer through miR-1287-5p/MUC19 axis under hypoxia. World J Surg Oncol (2021) 19:161. doi: 10.1186/s12957-021-02273-8
77. Liang G, Liu Z, Tan L, Su AN, Jiang WG, Gong C. HIF1α-associated circDENND4C Promotes Proliferation of Breast Cancer Cells in Hypoxic Environment. Anticancer Res (2017) 37:4337–43. doi: 10.21873/anticanres.11827
78. Choudhry H. UCA1 Overexpression Promotes Hypoxic Breast Cancer Cell Proliferation and Inhibits Apoptosis via HIF-1α Activation. J Oncol (2021) 2021:5512156. doi: 10.1155/2021/5512156
79. Choudhry H, Albukhari A, Morotti M, Haider S, Moralli D, Smythies J, et al. Tumor hypoxia induces nuclear paraspeckle formation through HIF-2α dependent transcriptional activation of NEAT1 leading to cancer cell survival. Oncogene (2015) 34:4482–90. doi: 10.1038/onc.2014.378
80. Zhang D, Shi Z, Li M, Mi J. Hypoxia-induced miR-424 decreases tumor sensitivity to chemotherapy by inhibiting apoptosis. Cell Death Dis (2014) 5:e1301. doi: 10.1038/cddis.2014.240
81. Krock BL, Skuli N, Simon MC. Hypoxia-induced angiogenesis: good and evil. Genes Cancer (2011) 2:1117–33. doi: 10.1177/1947601911423654
82. Li JY, Zhang Y, Zhang WH, Jia S, Kang Y, Tian R. Effects of differential distribution of microvessel density, possibly regulated by miR-374a, on breast cancer prognosis. Asian Pac J Cancer Prev (2013) 14:1715–20. doi: 10.7314/APJCP.2013.14.3.1715
83. Li JY, Zhang Y, Zhang WH, Jia S, Kang Y, Zhu XY. Differential distribution of miR-20a and miR-20b may underly metastatic heterogeneity of breast cancers. Asian Pac J Cancer Prev (2012) 13:1901–6. doi: 10.7314/APJCP.2012.13.5.1901
84. Cascio S, D'Andrea A, Ferla R, Surmacz E, Gulotta E, Amodeo V, et al. miR-20b modulates VEGF expression by targeting HIF-1 alpha and STAT3 in MCF-7 breast cancer cells. J Cell Physiol (2010) 224:242–9. doi: 10.1002/jcp.22126
85. Wen Y, Chen R, Zhu C, Qiao H, Liu Y, Ji H, et al. MiR-503 suppresses hypoxia-induced proliferation, migration and angiogenesis of endothelial progenitor cells by targeting Apelin. Peptides (2018) 105:58–65. doi: 10.1016/j.peptides.2018.05.008
86. Niu Y, Bao L, Chen Y, Wang C. HIF2-Induced Long Noncoding RNA RAB11B-AS1 Promotes Hypoxia-Mediated Angiogenesis and Breast Cancer Metastasis. Cancer Res (2020) 80:964–75. doi: 10.1158/0008-5472.CAN-19-1532
87. Lozano-Romero A, Astudillo-de la Vega H, Terrones-Gurrola M, Marchat LA. HOX Transcript Antisense RNA HOTAIR Abrogates Vasculogenic Mimicry by Targeting the AngiomiR-204/FAK Axis in Triple Negative Breast Cancer Cells. Noncoding RNA (2020) 6(2):19. doi: 10.3390/ncrna6020019
88. Contreras-Sanzón E, Palma-Flores C, Flores-Pérez A, Salinas-Vera YM, Silva-Cázares MB, Marchat LA, et al. MicroRNA-204/CREB5 axis regulates vasculogenic mimicry in breast cancer cells. Cancer biomark (2022) 35:47–56. doi: 10.3233/CBM-210457
89. O'Sullivan JM, Preston RJS, Robson T, O'Donnell JS. Emerging Roles for von Willebrand Factor in Cancer Cell Biology. Semin Thromb Hemost (2018) 44:159–66. doi: 10.1055/s-0037-1607352
90. Tao Q, Lu Y, Qi Y, Yu D, Gu J, Zhu Y, et al. Hypoxia promotes the expression of Von Willebrand factor in breast cancer cells by up-regulating the transcription factor YY1 and down-regulating the hsa-miR-424. Eur J Pharmacol (2022) 934:175308. doi: 10.1016/j.ejphar.2022.175308
91. King HW, Michael MZ, Gleadle JM. Hypoxic enhancement of exosome release by breast cancer cells. BMC Cancer (2012) 12:421. doi: 10.1186/1471-2407-12-421
92. Jung KO, Youn H, Lee CH, Kang KW, Chung JK. Visualization of exosome-mediated miR-210 transfer from hypoxic tumor cells. Oncotarget (2017) 8:9899–910. doi: 10.18632/oncotarget.14247
93. Lu X, Kang Y. Hypoxia and hypoxia-inducible factors: master regulators of metastasis. Clin Cancer Res (2010) 16:5928–35. doi: 10.1158/1078-0432.CCR-10-1360
94. He J, Wang J, Li S, Li T, Chen K, Zhang S. Hypoxia-inhibited miR-338-3p suppresses breast cancer progression by directly targeting ZEB2. Cancer Sci (2020) 111:3550–63. doi: 10.1111/cas.14589
95. Haque I, Banerjee S, Mehta S, De A, Majumder M, Mayo MS, et al. Cysteine-rich 61-connective tissue growth factor-nephroblastoma-overexpressed 5 (CCN5)/Wnt-1-induced signaling protein-2 (WISP-2) regulates microRNA-10b via hypoxia-inducible factor-1α-TWIST signaling networks in human breast cancer cells. J Biol Chem (2011) 286:43475–85. doi: 10.1074/jbc.M111.284158
96. Liu Z, Sun L. Hypoxia-Induced Suppression of Alternative Splicing of MBD2 Promotes Breast Cancer Metastasis via Activation of FZD1. Cancer Res (2021) 81:1265–78. doi: 10.1158/0008-5472.CAN-20-2876
97. Chen X, Iliopoulos D, Zhang Q, Tang Q, Greenblatt MB, Hatziapostolou M, et al. XBP1 promotes triple-negative breast cancer by controlling the HIF1α pathway. Nature (2014) 508:103–7. doi: 10.1038/nature13119
98. Xiping Z, Bo C, Shifeng Y, Feijiang Y, Hongjian Y, Qihui C, et al. Roles of MALAT1 in development and migration of triple negative and Her-2 positive breast cancer. Oncotarget (2018) 9:2255–67. doi: 10.18632/oncotarget.23370
99. Stone JK, Kim JH. Hypoxia induces cancer cell-specific chromatin interactions and increases MALAT1 expression in breast cancer cells. J Biol Chem (2019) 294:11213–24. doi: 10.1074/jbc.RA118.006889
100. Chen L, Bao L, Niu Y, Wang JE. LncIHAT Is Induced by Hypoxia-Inducible Factor 1 and Promotes Breast Cancer Progression. Mol Cancer Res (2021) 19:678–87. doi: 10.1158/1541-7786.MCR-20-0383
101. Wang Y, Zhang G, Han J. HIF1A-AS2 predicts poor prognosis and regulates cell migration and invasion in triple-negative breast cancer. J Cell Biochem (2019) 120:10513–8. doi: 10.1002/jcb.28337
102. Gómez-Maldonado L, Tiana M, Roche O, Prado-Cabrero A, Jensen L, Fernandez-Barral A, et al. EFNA3 long noncoding RNAs induced by hypoxia promote metastatic dissemination. Oncogene (2015) 34:2609–20. doi: 10.1038/onc.2014.200
103. Zuo Y, Qu C, Tian Y, Wen Y, Xia S, Ma M. The HIF-1/SNHG1/miR-199a-3p/TFAM axis explains tumor angiogenesis and metastasis under hypoxic conditions in breast cancer. Biofactors (2021) 47:444–60. doi: 10.1002/biof.1702
104. Chu Z, Huo N, Zhu X, Liu H, Cong R, Ma L, et al. FOXO3A-induced LINC00926 suppresses breast tumor growth and metastasis through inhibition of PGK1-mediated Warburg effect. Mol Ther (2021) 29:2737–53. doi: 10.1016/j.ymthe.2021.04.036
105. Cavallari I, Ciccarese F. The miR-200 Family of microRNAs: Fine Tuners of Epithelial-Mesenchymal Transition and Circulating Cancer Biomarkers. Cancers (Basel) (2021) 13(23):5874. doi: 10.3390/cancers13235874
106. Mutlu M, Raza U, Saatci Ö., Eyüpoğlu E, Yurdusev E, Şahin Ö.miR-200c: a versatile watchdog in cancer progression, EMT, and drug resistance. J Mol Med (Berlin Germany) (2016) 94:629–44 doi: 10.1007/s00109-016-1420-5
107. Ren S, Liu J, Feng Y, Li Z, He L, Li L, et al. Knockdown of circDENND4C inhibits glycolysis, migration and invasion by up-regulating miR-200b/c in breast cancer under hypoxia. J Exp Clin Cancer Res (2019) 38:388. doi: 10.1186/s13046-019-1398-2
108. Labrie M, Brugge JS, Mills GB. Therapy resistance: opportunities created by adaptive responses to targeted therapies in cancer. Nat Rev Cancer (2022) 22:323–39. doi: 10.1038/s41568-022-00454-5
109. Duan Y, Bai X, Yang J, Zhou Y, Gu W, Liu G, et al. Exposure to High-Altitude Environment Is Associated with Drug Transporters Change: microRNA-873-5p-Mediated Alteration of Function and Expression Levels of Drug Transporters under Hypoxia. Drug Metab Dispos (2022) 50:174–86. doi: 10.1124/dmd.121.000681
110. Assidicky R, Tokat UM, Tarman IO, Saatci O, Ersan PG, Raza U, et al. Targeting HIF1-alpha/miR-326/ITGA5 axis potentiates chemotherapy response in triple-negative breast cancer. Breast Cancer Res Treat (2022) 193:331–48. doi: 10.1007/s10549-022-06569-5
111. Saatci O, Kaymak A, Raza U. Targeting lysyl oxidase (LOX) overcomes chemotherapy resistance in triple negative breast cancer. Nat Commun (2020) 11:2416. doi: 10.1038/s41467-020-16199-4
112. Li H, Sun X, Li J, Liu W, Pan G, Mao A, et al. Hypoxia induces docetaxel resistance in triple-negative breast cancer via the HIF-1α/miR-494/Survivin signaling pathway. Neoplasia (2022) 32:100821. doi: 10.1016/j.neo.2022.100821
113. García-Venzor A, Mandujano-Tinoco EA, Ruiz-Silvestre A, Sánchez JM, Lizarraga F, Zampedri C, et al. lncMat2B regulated by severe hypoxia induces cisplatin resistance by increasing DNA damage repair and tumor-initiating population in breast cancer cells. Carcinogenesis (2020) 41:1485–97. doi: 10.1093/carcin/bgaa078
114. Li X, Wu Y, Liu A, Tang X. Long non-coding RNA UCA1 enhances tamoxifen resistance in breast cancer cells through a miR-18a-HIF1α feedback regulatory loop. Tumour Biol (2016) 37:14733–43. doi: 10.1007/s13277-016-5348-8
115. Yun Z, Lin Q. Hypoxia and Regulation of Cancer Cell Stemness. In: Koumenis C, Hammond E, Giaccia A, editors. Tumor Microenvironment and Cellular Stress. New York, NY: Springer New York (2014). p. 41–53.
116. Mathieu J, Zhang Z, Zhou W, Wang AJ, Heddleston JM, Pinna CM, et al. HIF induces human embryonic stem cell markers in cancer cells. Cancer Res (2011) 71:4640–52. doi: 10.1158/0008-5472.CAN-10-3320
117. Zhang Y, Yan J, Wang L, Dai H, Li N, Hu W, et al. HIF-1α Promotes Breast Cancer Cell MCF-7 Proliferation and Invasion Through Regulating miR-210. Cancer Biother Radiopharm (2017) 32:297–301. doi: 10.1089/cbr.2017.2270
118. Ivan M, Huang X. miR-210: fine-tuning the hypoxic response. Adv Exp Med Biol (2014) 772:205–27. doi: 10.1007/978-1-4614-5915-6_10
119. Tang T, Yang Z, Zhu Q, Wu Y, Sun K, Alahdal M, et al. Up-regulation of miR-210 induced by a hypoxic microenvironment promotes breast cancer stem cells metastasis, proliferation, and self-renewal by targeting E-cadherin. FASEB J (2018) 32:6965–81. doi: 10.1096/fj.201801013R
120. Hwang-Verslues WW, Chang PH, Wei PC, Yang CY, Huang CK, Kuo WH, et al. miR-495 is upregulated by E12/E47 in breast cancer stem cells, and promotes oncogenesis and hypoxia resistance via downregulation of E-cadherin and REDD1. Oncogene (2011) 30:2463–74. doi: 10.1038/onc.2010.618
121. Gibadulinova A, Bullova P, Strnad H, Pohlodek K. CAIX-Mediated Control of LIN28/let-7 Axis Contributes to Metabolic Adaptation of Breast Cancer Cells to Hypoxia. Int J Mol Sci (2020) 21(12):4299. doi: 10.3390/ijms21124299
122. Peng F, Wang JH, Fan WJ, Meng YT, Li MM, Li TT, et al. Glycolysis gatekeeper PDK1 reprograms breast cancer stem cells under hypoxia. Oncogene (2018) 37:1062–74. doi: 10.1038/onc.2017.368
123. Liu X, Qiao K, Zhu K, Li X, Zhao C, Li J, et al. Long Noncoding RNA HCG18 Promotes Malignant Phenotypes of Breast Cancer Cells via the HCG18/miR-103a-3p/UBE2O/mTORC1/HIF-1α-Positive Feedback Loop. Front Cell Dev Biol (2021) 9:675082. doi: 10.3389/fcell.2021.675082
124. Li X, Yang J, Ni R, Chen J, Zhou Y, Song H, et al. Hypoxia-induced lncRNA RBM5-AS1 promotes tumorigenesis via activating Wnt/β-catenin signaling in breast cancer. Cell Death Dis (2022) 13:95. doi: 10.1038/s41419-022-04536-y
125. Zhu P, He F, Hou Y, Tu G, Li Q, Jin T, et al. A novel hypoxic long noncoding RNA KB-1980E6.3 maintains breast cancer stem cell stemness via interacting with IGF2BP1 to facilitate c-Myc mRNA stability. Oncogene (2021) 40:1609–27. doi: 10.1038/s41388-020-01638-9
126. Papi A, Storci G, Guarnieri T, De Carolis S, Bertoni S, Avenia N, et al. Peroxisome proliferator activated receptor-α/hypoxia inducible factor-1α interplay sustains carbonic anhydrase IX and apoliprotein E expression in breast cancer stem cells. PloS One (2013) 8:e54968. doi: 10.1371/journal.pone.0054968
127. Wu MZ, Cheng WC, Chen SF, Nieh S, O'Connor C, Liu CL, et al. miR-25/93 mediates hypoxia-induced immunosuppression by repressing cGAS. Nat Cell Biol (2017) 19:1286–96. doi: 10.1038/ncb3615
128. Grimaldi A, Zarone MR, Irace C, Zappavigna S, Lombardi A, Kawasaki H, et al. Non-coding RNAs as a new dawn in tumor diagnosis. Semin Cell Dev Biol (2018) 78:37–50. doi: 10.1016/j.semcdb.2017.07.035
129. Camps C, Buffa FM, Colella S, Moore J, Sotiriou C, Sheldon H, et al. hsa-miR-210 Is induced by hypoxia and is an independent prognostic factor in breast cancer. Clin Cancer Res (2008) 14:1340–8. doi: 10.1158/1078-0432.CCR-07-1755
130. Toyama T, Kondo N, Endo Y, Sugiura H, Yoshimoto N, Iwasa M, et al. High expression of microRNA-210 is an independent factor indicating a poor prognosis in Japanese triple-negative breast cancer patients. Jpn J Clin Oncol (2012) 42:256–63. doi: 10.1093/jjco/hys001
131. Pasculli B, Barbano R. Hsa-miR-210-3p expression in breast cancer and its putative association with worse outcome in patients treated with Docetaxel. Sci Rep (2019) 9:14913. doi: 10.1038/s41598-019-51581-3
132. Du Y, Zhou L, Lin Y, Yin K, Yin W, Lu J. Polymorphisms in microRNA let-7 binding sites of the HIF1AN and CLDN12 genes can predict pathologic complete response to taxane- and platinum-based neoadjuvant chemotherapy in breast cancer. Ann Transl Med (2019) 7:138. doi: 10.21037/atm.2019.04.26
133. Gu P, Zhang L, Wang R, Ding W, Wang W, Liu Y, et al. Development and Validation of a Novel Hypoxia-Related Long Noncoding RNA Model With Regard to Prognosis and Immune Features in Breast Cancer. Front Cell Dev Biol (2021) 9:796729. doi: 10.3389/fcell.2021.796729
134. Zhao Y, Liu L, Zhao J, Du X, Yu Q, Wu J, et al. Construction and Verification of a Hypoxia-Related 4-lncRNA Model for Prediction of Breast Cancer. Int J Gen Med (2021) 14:4605–17. doi: 10.2147/IJGM.S322007
135. Anfossi S, Babayan A, Pantel K, Calin GA. Clinical utility of circulating non-coding RNAs - an update. Nat Rev Clin Oncol (2018) 15:541–63. doi: 10.1038/s41571-018-0035-x
136. Lopes BC, Braga CZ, Ventura FV, de Oliveira JG, Kato-Junior EM, Bordin-Junior NA, et al. miR-210 and miR-152 as Biomarkers by Liquid Biopsy in Invasive Ductal Carcinoma. J Pers Med (2021) 11(1):31. doi: 10.3390/jpm11010031
137. Liu M, Xing LQ, Liu YJ. A three-long noncoding RNA signature as a diagnostic biomarker for differentiating between triple-negative and non-triple-negative breast cancers. Med (Baltimore) (2017) 96:e6222. doi: 10.1097/MD.0000000000006222
138. Giaccia A, Siim BG, Johnson RS. HIF-1 as a target for drug development. Nat Rev Drug Discovery (2003) 2:803–11. doi: 10.1038/nrd1199
139. Semenza GL. Targeting HIF-1 for cancer therapy. Nat Rev Cancer (2003) 3:721–32. doi: 10.1038/nrc1187
140. Powis G, Kirkpatrick L. Hypoxia inducible factor-1alpha as a cancer drug target. Mol Cancer Ther (2004) 3:647–54. doi: 10.1158/1535-7163.647.3.5
141. Fallah J, Rini BI. HIF Inhibitors: Status of Current Clinical Development. Curr Oncol Rep (2019) 21:6. doi: 10.1007/s11912-019-0752-z
142. Wilson WR, Hay MP. Targeting hypoxia in cancer therapy. Nat Rev Cancer (2011) 11:393–410. doi: 10.1038/nrc3064
143. Sahu D, Zhao Z, Tsen F, Cheng CF, Park R, Situ AJ, et al. A potentially common peptide target in secreted heat shock protein-90α for hypoxia-inducible factor-1α-positive tumors. Mol Biol Cell (2012) 23:602–13. doi: 10.1091/mbc.e11-06-0575
144. Costales MG, Haga CL, Velagapudi SP, Childs-Disney JL, Phinney DG, Disney MD. Small Molecule Inhibition of microRNA-210 Reprograms an Oncogenic Hypoxic Circuit. J Am Chem Soc (2017) 139:3446–55. doi: 10.1021/jacs.6b11273
145. Haga CL, Velagapudi SP, Strivelli JR, Yang WY, Disney MD, Phinney DG. Small Molecule Inhibition of miR-544 Biogenesis Disrupts Adaptive Responses to Hypoxia by Modulating ATM-mTOR Signaling. ACS Chem Biol (2015) 10:2267–76. doi: 10.1021/acschembio.5b00265
146. Mendes BB, Conniot J, Avital A, Yao D, Jiang X, Zhou X, et al. Nanodelivery of nucleic acids. Nat Rev Methods Primers (2022) 2:24. doi: 10.1038/s43586-022-00104-y
147. Wang W, Liu X, Ding L, Jin HJ, Li X. RNA Hydrogel Combined with MnO(2) Nanoparticles as a Nano-Vaccine to Treat Triple Negative Breast Cancer. Front Chem (2021) 9:797094. doi: 10.3389/fchem.2021.797094
148. Kuo TC, Kung HJ, Shih JW. Signaling in and out: long-noncoding RNAs in tumor hypoxia. J Biomed Sci (2020) 27:59. doi: 10.1186/s12929-020-00654-x
149. Maldonado V, Melendez-Zajgla J. The Role of Hypoxia-Associated Long Non-Coding RNAs in Breast Cancer. Cells (2022) 11(10):1679. doi: 10.3390/cells11101679
150. Sawai S, Wong PF. Hypoxia-regulated microRNAs: the molecular drivers of tumor progression. Crit Rev Biochem Mol Biol (2022) 57:351–76. doi: 10.1080/10409238.2022.2088684
151. Di Liddo A, de Oliveira Freitas Machado C, Fischer S, Ebersberger S, Heumüller AW, Weigand JE, et al. A combined computational pipeline to detect circular RNAs in human cancer cells under hypoxic stress. J Mol Cell Biol (2019) 11:829–44. doi: 10.1093/jmcb/mjz094
152. Huang YE, Zhou S, Liu H, Zhou X, Yuan M, Hou F, et al. Identification of drug resistance associated ncRNAs based on comprehensive heterogeneous network. Life Sci (2020) 243:117256. doi: 10.1016/j.lfs.2020.117256
153. Baptista B, Riscado M, Queiroz JA, Pichon C, Sousa F. Non-coding RNAs: Emerging from the discovery to therapeutic applications. Biochem Pharmacol (2021) 189:114469. doi: 10.1016/j.bcp.2021.114469
154. Anfossi S, Babayan A, Pantel K, Calin GA. Clinical utility of circulating non-coding RNAs — an update. Nat Rev Clin Oncol (2018) 15:541–63. doi: 10.1038/s41571-018-0035-x
155. Misso G, Di Martino MT, De Rosa G, Farooqi AA, Lombardi A, Campani V, et al. Mir-34: a new weapon against cancer? Mol Ther Nucleic Acids (2014) 3:e194. doi: 10.1038/mtna.2014.47
156. Hong DS, Kang Y-K, Borad M, Sachdev J, Ejadi S, Lim HY, et al. Phase 1 study of MRX34, a liposomal miR-34a mimic, in patients with advanced solid tumours. Br J Cancer (2020) 122:1630–7. doi: 10.1038/s41416-020-0802-1
157. Liu N, Xia WY, Liu SS, Chen HY, Sun L, Liu MY, et al. MicroRNA-101 targets von Hippel-Lindau tumor suppressor (VHL) to induce HIF1α mediated apoptosis and cell cycle arrest in normoxia condition. Sci Rep (2016) 6:20489. doi: 10.1038/srep20489
Keywords: hypoxia, HIFs, breast cancer, ncRNA, miRNAs, lncRNAs, circRNAs
Citation: H. Al-Zuaini H, Rafiq Zahid K, Xiao X, Raza U, Huang Q and Zeng T (2023) Hypoxia-driven ncRNAs in breast cancer. Front. Oncol. 13:1207253. doi: 10.3389/fonc.2023.1207253
Received: 17 April 2023; Accepted: 06 July 2023;
Published: 31 July 2023.
Edited by:
Subhadeep Roy, National Institute of Pharmaceutical Education and Research, Kolkata, IndiaReviewed by:
Rakeeb Ahmad Mir, Central University of Kashmir, IndiaXiwang Zheng, First Hospital of Shanxi Medical University, China
Manjari Singh, Assam University, India
Copyright © 2023 H. Al-Zuaini, Rafiq Zahid, Xiao, Raza, Huang and Zeng. This is an open-access article distributed under the terms of the Creative Commons Attribution License (CC BY). The use, distribution or reproduction in other forums is permitted, provided the original author(s) and the copyright owner(s) are credited and that the original publication in this journal is cited, in accordance with accepted academic practice. No use, distribution or reproduction is permitted which does not comply with these terms.
*Correspondence: Tao Zeng, emVuZ3RAc211LmVkdS5jbg==; Qiyuan Huang, MTU0ODQ3ODEyMkBxcS5jb20=