- 1Divison of Pharmaceutical Sciences, College of Pharmacy and Pharmaceutical Sciences, Institute of Public Health, Florida A & M University, Tallahassee, FL, United States
- 2John Gnabre Science Research Institute, Baltimore, MD, United States
The presence of microorganism communities (MOCs) comprised of bacteria, fungi, archaea, algae, protozoa, viruses, and the like, are ubiquitous in all living tissue, including plant and animal. MOCs play a significant role in establishing innate and acquired immunity, thereby influencing susceptibility and resistance to disease. This understanding has fostered substantial advancements in several fields such as agriculture, food science/safety, and the development of vaccines/adjuvants, which rely on administering inactivated-attenuated MOC pathogens. Historical evidence dating back to the 1800s, including reports by Drs Busch, Coley, and Fehleisen, suggested that acute febrile infection in response to “specific microbes” could trigger spontaneous tumor remission in humans. This discovery led to the purposeful administration of the same attenuated strains, known as “Coley’s toxin,” marking the onset of the first microbial (pathogen) associated molecular pattern (MAMPs or PAMPs)-based tumor immunotherapy, used clinically for over four decades. Today, these same MAMPS are consumed orally by billions of consumers around the globe, through “specific” mediums (immune boosting “herbal supplements”) as carriers of highly concentrated MOCs accrued in roots, barks, hulls, sea algae, and seeds. The American Herbal Products Association (AHPA) mandates microbial reduction in botanical product processing but does not necessitate the removal of dead MAMP laden microbial debris, which we ingest. Moreover, while existing research has focused on the immune-modulating role of plant phytochemicals, the actual immune-boosting properties might instead reside solely in the plant’s MOC MAMP laden biomass. This assertion is logical, considering that antigenic immune-provoking epitopes, not phytochemicals, are known to stimulate immune response. This review explores a neglected area of research regarding the immune-boosting effects of the herbal microbiome – a presence which is indirectly corroborated by various peripheral fields of study and poses a fundamental question: Given that food safety focuses on the elimination of harmful pathogens and crop science acknowledges the existence of plant microbiomes, what precisely are the immune effects of ingesting MAMPs of diverse structural composition and concentration, and where are these distributed in our botanicals? We will discuss the topic of concentrated edible MAMPs as acid and thermally stable motifs found in specific herbs and how these would activate cognate pattern recognition receptors (PPRs) in the upper gut-associated lymphoid tissue (GALT), including Peyer’s patches and the lamina propria, to boost antibody titers, CD8+ and CD4+ T cells, NK activity, hematopoiesis, and facilitating M2 to M1 macrophage phenotype transition in a similar manner as vaccines. This new knowledge could pave the way for developing bioreactor-grown/heat-inactivated MOC therapies to boost human immunity against infections and improve tumor surveillance.
1 Introduction
Microorganism communities (MOCs), encompassing bacteria, fungi, archaea, algae, protozoa, and viruses, often colloquially referred to as “bugs,” shape both innate and acquired immunity across all living systems, including plants, humans, and even malignant tumors, each of which hosts a unique microbiome. The rapidly evolving role of MOCs in cancer therapy, propelled by advances in metagenomics, has historical roots tracing back to the late 19th century. Physicians, including Busch, Coley, and Fehleisen, observed cancer patients undergoing remarkable spontaneous remission following acute febrile infections (38-40°C) induced by Streptococcus pyogenes (1, 2). These observations inspired collaborations and deliberate attempts to treat cancer patients with live MOCs, sometimes resulting in fatal sepsis. The sucessful approach involved the use of attenuated Streptococcus pyogenes (gram-positive) and Serratia marcescens (gram-negative), constituting the first microbial-associated molecular pattern (MAMP) tumor vaccine immunotherapy, comprised of toll-like receptor (TLRs 2,4) agonists bacterial LPS endotoxin/peptidoglycan (3). Coley’s tumor immunotherapy induced curative remission in approximately 50% of mesodermal embryonic origin cancers (sarcoma, lymphoma, leukemia, kidney, ovarian, and so on) for over 40 years (4). However, this approach was eventually supplanted by advancements in radiation, surgery, and immunosuppressive chemotherapies, which dominated the field of oncology for several decades (5, 6).
There is a resurgence of interest in tumor immunotherapies to achieve the same remission that Coley initially pursued over a century ago (5, 7, 8). However, these therapies must now overcome the immunosuppression imposed by mainstream chemotherapies and painkillers (e.g., cytostatic antineoplastics, opioids, and corticosteroids) (9–14), which destroy hematopoiesis (e.g., cytopenia, neutropenia), and lead to greater risk of infection, further aggravated by use of immunosuppressive antibiotics, and antipyretics (acetaminophen) (15). Infections, whether post-operative or otherwise, continue to be the leading cause of death in cancer patients (16–19). Moreover, the widespread use of synthetic drugs has had a cumulative negative impact on human immune intelligence in civilized societies. The human immune system relies on its capacity to amass a library of data on foreign pathogens, allowing it to eliminate recognized threats swiftly. Initial interaction often involves rapid infection resolution via fever and a robust immune defense.
Modern tendencies towards comfort over enduring sickness, and the overuse of antibiotics, antipyretics (Tylenol), and painkillers, have dulled immune intelligence. To add insult to injury, poor diet and pervasive societal stress, contribute to a widespread dysbiosis of the gut and host-immune dysfunction (effects transferable in utero from mother to child) (20, 21). The “hygiene hypothesis,” posing that minimal exposure to microbes in early life leads to dysbiosis, compounds the problem. This situation has been tied to epidemic non-communicable disorders and human cancers (22–24). Catering to sanitation and comfort is leading to an atrophy of human immune intelligence. As a result, there is an epidemic rise in chronic infections/inflammation which create refractory “T cell exhaustion” and a state of immune suppression, leading to greater susceptibility to various cancers (25–29). Conversely, nurturing immune intelligence through rigorous exposure to MOCs provides the routine cross-reactive targeting needed to destroy self-host malignant cells harboring similar tumor-associated antigens (TAAs) in a manner akin to infection response (30).
Immune suppression manifests itself as a loss of tumor immune surveillance, which is the controlling gateway for all human cancers to establish, develop, and thrive (31–34). In addition, immune suppression increases tumor initiation upon exposure to environmental carcinogens (e.g. pollutants, occupational fumes/dust, ionizing UV radiation (35)) carcinogenic microbes (36, 37), (H pylori), parasites (O viverrine, C Sinensis, and S haematobium) viruses (Epstein–Barr, human papilloma, hepatitis B, C, human herpes type-8, and human T-cell lymphotropic type-1) (38). These factors necessitate exploring new methods to enhance the immune system’s capacity to combat human cancers.
2 Plant medicine: the plant microbiome
Immunological resilience is enhanced through the introduction of MOC antigens, a principle foundational to vaccines (39–43). That said, as of today there is meager research into the study of inactivated/attenuated plant microbiomes in our botanical medicines which contain product specific MOCs (including lethal pathogens) and how they impact human immunity. Plant-specific MOCs, are destroyed during food processing techniques like retort and pasteurization. However, not all MOCs leave a trail of immune provoking microbiome-associated molecular patterns (MAMPs), and their ubiquity varies, issues discussed below. Edible, immune-provoking MAMPs are nature’s concentrated reservoirs of immune stimulants within the plant world. Figure 1 MOCs are ever present as controlling elements in plant health, growth, maturation, and ecological control whereby numerous factors influence the diversity and concentration. These include the plant’s/part phytochemical profile (e.g., anti-biotic, anti-fungal properties), its location and part (e.g., above-ground stems/leaves exposed to UV sterilizing sunlight and rainwater wash, or subsurface parts exposed to different gas compositions and minerals), the plant’s tactile nature (e.g., alginates), MOCs altered during maturity at harvest, and complex interactions during growth, among others. All medicinal herbs and spices contain MOCs, where food safety regulations by the American Herbal products association (AHPA) and National Science Foundation/American National Standards Institute (NSF/ANSI) (44–47) require microbial reduction and third-party testing to establish 1) an absence of viable pathogens and mycotoxins, 2) a residual threshold yield of live non-pathogenic coliform, aerobic plate counts, total yeast & molds reported as colony forming units (CFU)/g (44–49). However, our understanding of which plant microbiomes are immune-boosting and how their residual trail of microbiome/MAMPs impacts health, from pathogenic to non-pathogenic, remains limited. Edible MAMPs, often acid and thermally stable, can act on pattern recognition receptors (PRRs) in the upper gut-associated lymphoid tissue (GALT), Peyer’s patches, and lamina propria, influencing innate and acquired immune systems (44–47, 50). Yet, despite their ubiquity in foods, most studies on the “edible plant microbiome” focus on identifying the taxonomy of live microorganisms present, with little consideration of their potential health implications (51–58).
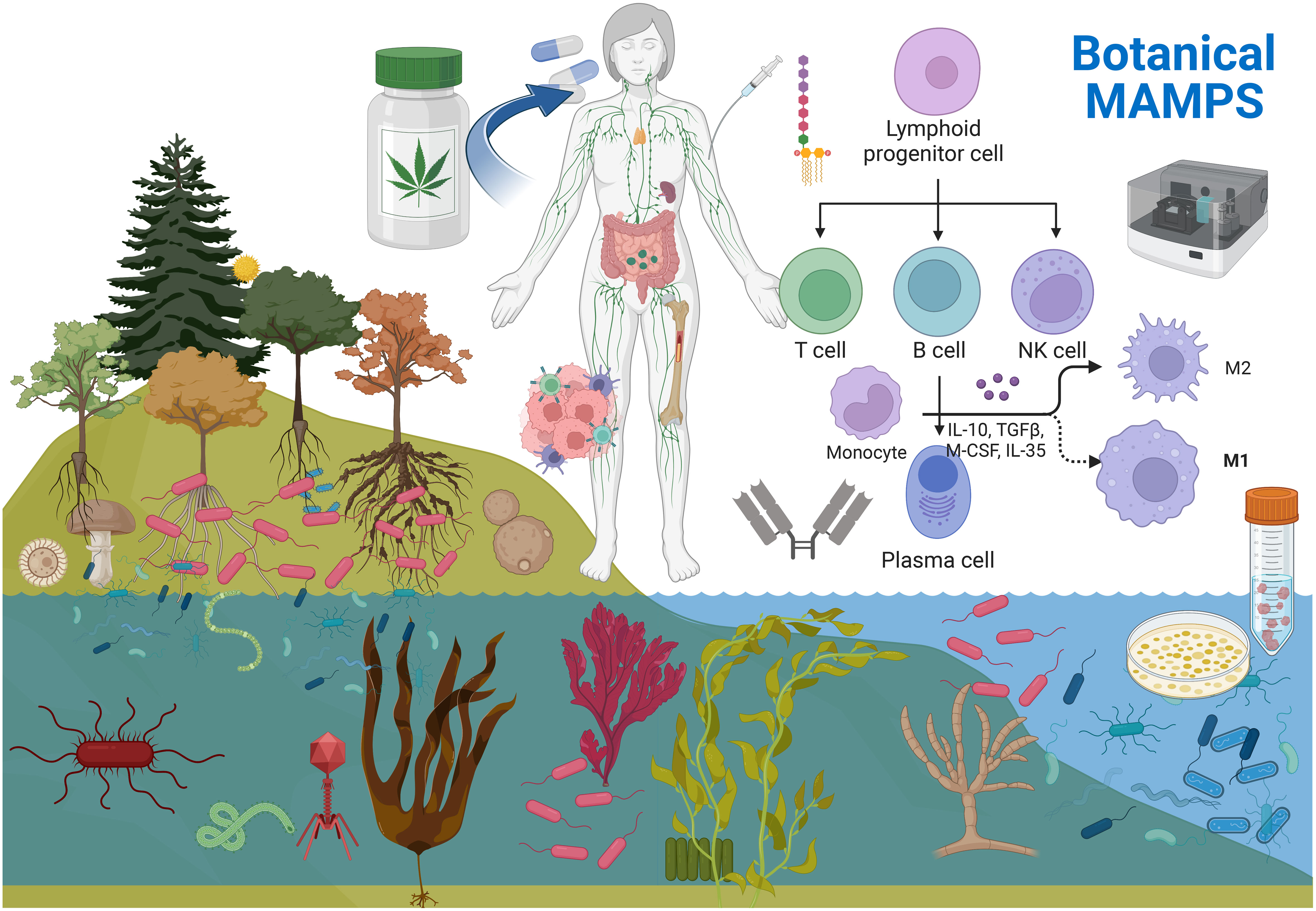
Figure 1 Microorganisms are diverse communities (MOCs) of bacteria, virus, fungus, protozoa etc. being ubiquitous in nature. MOCs are ever present within the richest reservoirs in roots, seeds, and sea algae’s. These MOCs end up in our foods, water and botanical medicines. Botanical products undergo post-harvest sterilization to ensure food safety, where MOCS (inactivated remain) . These MOCS are high in specific over the counter botanicals, few which are rich in MAMPs from gram negative bacteria. These TLR4 activating MAMPs have been inadvertently consumed for thousands of years, which can enhance innate and acquired immunity, while research efforts have been focused predominantly on the therapeutic effects of the plant/ phytochemical constituents. Given the plants microbiome is a unique component within/ but not of the plant - MOCS can be cultivated in a bioreactor / prior to inactivation and evaluated for effects on human health. Created with BioRender.com.
Humans have consumed plant medicines and their resident MOCs for millennia, attributing the health benefits only to the plant and constituent phytochemicals. However, a small number of research teams have proposed that the health benefits of certain herbs may instead be due to their inactivated microbial biomass after separating the plants bioactive MAMPs from the plant itself (39–41). This theory aligns with the broader field of microbiomic science and the simple logic that the immune system responds aggressively not to phytochemicals or drugs but to the presence of foreign antigenic microorganism debris (MAMPs), the basis for vaccine and adjuvant development. A few research teams have discovered that MOC-MAMP PRR TLR4 agonists are responsible for immune boosting effects in the following herbs; Black walnut, Echinacea Root, Ginseng Root, Alfalfa Seeds (59) Astragalus Root (60, 61), Angelica Root (62), and Wheat, the latter who introduces the concept of edible LPS found in wheat from Pantoea agglomerans as a “modern-day nontoxic Coleys toxin” with limitless capacity when taken orally (63–66). Our recent research corroborates the findings of all of these studies, indicating the immune boosting properties are not of the plant, but rather attributable to its MAMP MOC biomass, while we now expand this list to include 65 out of approximately 2000 OTC products tested containing TLR4 PRR agonists (Publication Pending, Mazzio et al., 2023. Journal of Funcitonal Foods ).
Our research findings directly align with ecological studies reporting similar soil-embedded rhizome microbiomes (67) (e.g., echinacea, stinging nettle, burdock etc.) and high concentrations of gram-negative microbes and biofilms in edible sea kelps and other marine vegetation, including Granulosicoccus antarcticus, hellea balneolensis (Gammaproteobacteria) bacteroidetes and Alphaproteobacteria, these including Fucus vesiculosus, N. leutkeana (Bladderwrack), P. scouleri (Surf Grass), Laminaria setchellii (Kelp) Chondrus crispus (Irish Moss), Laminaria ochroleuca (Kelp/Brown Seaweed), Palmaria palmate (Dulse), etc. (68, 69).
According to our research, approximately 98% of herbs, fruits, and vegetables do not contain bioactive toll-like receptor (TLR4) agonists, which suggests that immune-modulating microbiomes in plants and herbs are not ubiquitous but instead result from natural forces that encourage their accumulation.
2.1 Diverting research focal points
Significant research has been directed toward peripheral topics, often overlooking the potential human health aspect of ingesting inactivated MOCs. None the less, the concept that ingestion of “dead bugs” can impact human health is gradually gaining acceptance, as seen in the emerging fields of para-probiotics (inactivated probiotics) (70–72) development of oral vaccines (73, 74) and with greater understanding as to the anatomy of the human gut microbiome and its PRRs (44–47, 50, 75). Despite this, most of the work carried out on the human microbiome to date has focused on the “living microbiome” (probiotics, prebiotics, and microbiota cultures) as symbionts/pathobionts (76–79). Meanwhile, therapeutic research in plant medicine continues to focus on plant phytochemicals rather than the plant’s inactivated microbiome. Plants contain a diverse array of “phytochemicals” such as flavonoids, phenolics, alkaloids, glycosides, lignans, and triterpenoids, which exhibit a broad range of protective properties, including anti-inflammatory, analgesic, antipyretic, antimalarial, antibacterial, antiprotozoal, antioxidant, antifungal, and antiviral effects (80). Inadvertently, the antimicrobial capabilities of these plant chemicals have been exploited for centuries for the preservation and treatment of infections. Today, the predominant focus on anti-cancer natural medicines, also continues to be on the phytochemicals. Thousands of studies demonstrate phytochemicals to manipulate cellular signaling pathways such as apoptosis (Bcl-2/Bax), oncogene transcription, gene induction, enzyme expression/activity (e.g., topoisomerase, cyclooxygenase, matrix metalloproteases), cell cycle regulation, and modulation of signaling systems associated with rapid tumor growth (e.g., MAPK/ERK pathway, PI3K/AKT/mTORC1), or to activate DNA repair mechanisms (80–85). A similar emphasis on chemicals is observed in research exploring the interaction between microbes and cancer, specifically focusing on secondary metabolites produced by Actinobacteria and Streptomyces spp. These metabolites, which include polyphenols, indolocarbazoles, anthracyclines, halogenated compounds, polyketides, anthracenes, and alkaloids, form the foundation of traditional chemotherapy drugs like doxorubicin, mithramycin, and mitomycin C (86).
While plant chemicals can prevent cancer by attenuating chronic inflammation that initiates cancer (27, 87), managing already-established cancer requires a robust and sharp immune boost, necessitating a pro-inflammatory response.
2.2 MAMPS in anti-tumor immune therapies
The potential to boost the immune system to overpower and destroy a human tumor forms the foundation for future immunotherapy breakthroughs. All tumor immune therapies share a common goal: to “boost,” “activate,” or “reawaken” a dormant host immune system subdued by the tumor’s immunosuppressive barrier to regain control over the mechanisms capable of destroying malignant cells (5, 7, 8); this requires a pro-inflammatory (immune-boosting) response, not an anti-inflammatory response. Therapies (similar to Coley) have thus far explored combination of synthetic hyperthermia (fever) ± inactivated microbes containing potent MAMPS (6, 88, 89), MAMP vaccines (90, 91), exogenous cytokines (IL-2, 15), interferon-alpha (IFNα) or granulocyte-macrophage colony-stimulating factor (GM-CSF)), adoptive T cell therapies with direct targeting of (tumor-associated (TAA)/tumor-specific antigens (TSA)), checkpoint inhibitors which remove/braking systems (mAb), ipilimumab, nivolumab, pembrolizumab, etc.) (92–95) or autologous/allogenic tumor vaccines primed with strong immunological bacterial laden adjuvants and co-stimulatory cytokines (96).
Pharmacodynamically and mechanistically edible MAMPS may do the same, having direct access to the immune system through the gut mucosa. The upper GI can capture (by mucin), identify, respond to, and build an acquired immunity database to foreign antigens while offering 15 times the surface area than the large intestine (97). The upper GI also houses plentiful GALT-/LP PRRs, in epithelial intestinal and immune cells (neutrophils, macrophages/monocytes, dendritic cells, mast cells, T and B lymphocytes), and a highly integrated signaling surface readily acted upon by MAMPS on C-type lectin-like receptors (CLRs) (Dectins, Mincle, and Mcl), nucleotide-binding oligomerization domain (NOD)-like receptors (NLRs), and Toll-like receptors (TLRs) (98). As for TLRs, these are housed in the gut immune plexus on either cell surfaces (TLR1, 2, 4, 5, 6) or/in (endosomes TLR3, 7, 8, 9) (99, 100). Known edible MAMPS that would activate these include food-based bacterial LPS, lipoproteins, zymosan, byglycans, acylated lipopeptides, lipoteichoic acid, peptidoglycans, modulin, dsRNA from bacteria, glycolipids, fibrinogen/fibronectin, heat shock proteins, uric acid, flagellin (TLR5), ssRNA of microbial origin and unmethylated CpG rich DNA (101–104).
Just in the case of TLR agonists alone, these are widely in our foods and vaccines as adjuvants, being pro-inflammatory, with a capacity to boost antibody titers, CD8+ and CD4+ T cells, cytokine levels (IL-12, TNF-α, IFN-γ, IL-6 and type I interferon), chemokines (monocyte chemoattractant protein-1 (MCP-1/CCL2), macrophage inflammatory proteins (MIP-α/1-β) MyD88, IL-1R), and related signaling pathways (IRAK, MAPK) through transcriptional activation of NF-κB and AP-1 (42, 43). PRR TLR adjuvants used in vaccines include agonists of TLR9 (CpG oligodeoxynucleotides (ODN)) - the hepatitis B virus vaccine Heplisav-B, MGN1703 for cancer vaccines; TLR3 (poly-IC/ICLC derivates (Ampligen® Hiltonol®)) for cancer and HIV vaccines, TLR4 (monophosphoryl lipid A derivative agonist AS04, for Cervarix (human HPV)/Hep B (Fendrix®), AS01 for the herpes zoster vaccine Shingrix®, glucopyranosyl Lipid A for influenza vaccines), TLR 5 agonists (flagellin derivatives such as Mobilan or entolimod) or TLR7 (resiquimod (an imidazoquinoline) employed with cancer vaccines (42).
While dead bugs in our botanical medicines have not yet been explored in large, fungal molecules are subject to exhaustive research, as in the case of the peptide fragment zymosan; also known as β-glucan derived from Saccharomyces cerevisiae (105–112). When ingested orally, β-glucans activate (M-cells)/, Peyer’s patches and boost innate and systemic acquired intelligence (107–109). This mechanism of action has direct relevance to tumor immunotherapies given it can elicit sharp responses which foster 1) macrophage phenotype polarization in tumor-associated macrophages (TAMs) to M1 anti-tumor fighter macrophages (110, 111), 2) reduce the load of tumor immune suppressors such as myeloid-derived suppressor cells (MDSC) or Tregs (112) 3) heighten activated CD4(+), CD8(+) T cells and the IL-2 IFN-γ response (113) 4) augment hematopoiesis (106) prompt maturation of neutrophils, macrophages, dendritic cells, and NK cells (Dectin/TLR/complement receptor 3 (CR3), CD11b CD18) (114, 115) and 5) initiate iC3b-opsonized targeting of tumor cells for phagocytosis and degranulation (106, 107, 114, 116), linked to reduced tumor burden in mammals (105, 114, 115, 117–119).
Using edible immune boosters such as zymosan could synergize the efficacy of checkpoint inhibitors, whereby taking the foot off the tumor immunosuppressive barrier (immune checkpoint inhibitors) combined with the second foot on acceleration “immune boosting” would provide therapeutic advantage. (115, 120, 121). Edible MAMPS (zymogen or other) reaching the GI would evoke the transfer of enemy antigenic data through M cells to antigen-presenting cells (APCs), which then travel to the mesenteric lymph node (122) concomitant to lamina propria APC dendritic cell branching to systemic lymphatic vessels (75, 123–125) evoking T cell clonal expansion (126–128). Moreover, oral administration of MAMPS, like LPS, may enter into blood circulation through lipid absorption or chylomicrons by intestinal epithelial cells (50) triggering systemic innate and acquired immune system response as evidenced in mammals (75).
2.3 Are edible PRR-TLR4 agonists endotoxin?
Are we suggesting that edible endotoxin can alter immunity? Yes, the practice of consuming endotoxin has been applied for hundreds - thousands of years, before the discovery of microbes in cultural medicine for example edible spirulina, chlorella, sea moss, and sea kelps. There is enormous confusion surrounding the term “endotoxin” because it is a misnomer. The term “endotoxin” today is an open-ended, vague term to describe all gram-negative microbial cell wall LPS with the lipid A the “ toxin” all alive or dead, all pathogenic or non-pathogenic, and all-inclusive to every type of taxonomic and cell wall polysaccharide variation. The term endotoxin is one term, used to ascribe deadly infectious (sepsis) with multi-organ failure and death, to health products sold OTC taken by billions of consumers across the globe every day, e.g., as previously stated: LPS Arthrospira platensis (Spirulina). Endotoxins are in many cases endo-immunomodulators (not toxins) with beneficial effects justifying their use as peptide-based anti-cancer vaccines adjuvants (101). Many studies show endotoxin in immune-competent cancer models can reverse the negative effects of chemotherapy and shrink/sometimes eradicate human tumors in a manner as described by Coley (129–138) with the ability to boost NK cell activity, activate macrophages and stimulate hematopoiesis of lymphocytes (139–155). And, if MAMP-laden edible products bear resemblance to Coley’s toxin (156), then as longitudinal fibrils contiguous (157) they too could very likely evoke mitogenic B cell activation (158) influence the complement system (156), stimulate macrophages to lyse tumor cells (159) and bestow all benefits known to LPS/TLR4 agonists and their use in anti-cancer medicines when taken orally (63, 65, 66).
This concept is substantiated further by, numerous animal and human studies demonstrating that exposure to LPS in early life can stimulate systemic immunity, being inversely related to the occurrence of hay fever, atopic asthma, and atopic sensitization (160). Moreover, LPS, a standard endotoxin, has been identified as a vital immune system stimulant (161). Specifically, LPS derived from Escherichia coli has been proven to elicit a robust immune response via TLR4 signaling; this, in turn, triggers the release of pro-inflammatory cytokines, leading to an enhanced immune response (162). Immunotherapies that leverage LPS have shown promise in preclinical and clinical studies. For instance, MPL (monophosphoryl lipid A), a derivative of LPS, has been successfully used as an adjuvant in anti-cancer vaccines (162, 163). Despite these advancements, the potential of dead bugs, especially their endotoxins, in therapeutics and immunotherapies remains largely unexplored. The primary barrier has been the conventional perception of endotoxins as harmful substances associated with diseases such as sepsis. Therefore, the potential of dead bugs in therapeutics and immunotherapies warrants further exploration. Future studies should focus on unraveling the specific immune-modulatory mechanisms of endotoxins and other components of dead bugs and their potential applications in treating diseases such as cancer. With technological advancements and an understanding of the gut microbiome, the prospect of leveraging dead bugs in therapeutics is promising.
3 LPS/TRL4 agonists and cancer models
If the administration of gram-negative (non-strain specific) LPS can reduce tumor burden, then why is the literature riddled with contradiction? These conflicting reports appear consistent when organized by model type and route of administration. For example, pro-tumor effects of LPS are consistently reported in (immune-deficient) animal models, or isolated cancer cells in vitro (without an immune coculture) or by assumptions drawn on greater expression levels for TLR4 reported in cancer vs. healthy adjacent tissue (164–175). However, anti-tumor effects of LPS/TLR4 agonists are consistently reported in fully immune competent animals, the majority showing greater survival time, curative remission in a significant % of test groups similar to Coley’s toxin, reduction of adverse effects of chemotherapy and radiation, including resistance (130–133, 176–178), and effects augmented when combined with anti-tumor cytokines (e.g. G-CSF) (134). LPS administration, when injected directly into tumors (immune-competent models) - elicits massive macrophage and neutrophil trafficking (135) evoking nearly complete and total tumor regression consistently reported (136, 137). In humans, oral administration of LPS from wheat (Pantoea agglomerans) shows recovery and remission in 62% of cancer patients (138), whereas other sources (e.g., LPS Alistipes shahii) can achieve a reduction in side effects associated with radiation/chemotherapies (129).
3.1 LPS/TLR4 agonists: mechanism of action
The mechanism by which LPS reduces tumor size remains a topic of continued research, but it is generally thought to be closely tied to its critical role in regulating macrophages. Several steps mediate its anti-tumor effects: 1) LPS first binds to the LPS first binds to protein/sensitizer CD14 (179) 2), both then binding to the TLR4/MD-2 complex (180), 3) which triggers MyD88 signaling, 4) and leads to an IFN-type 1 response, NF-kappaB and activation of TAMs in the TME, thus switching “on” the M1 anti-cancer fighter phenotype (pro-inflammatory, anti-tumorigenic) and overcoming the acquiescent M2 (anti-inflammatory pro-tumorigenic phenotype) (7, 181, 182). Figure 2 In immune-competent animals, the transition from M2 to M1 TAM phenotype is associated with the re-awakening of T-cell mediated adaptive host immune response that recognizes malignant cells However, this transition’s mechanisms are still poorly understood (101, 183–186). M1 TAM phenotypes coincide with increased activation of CD4(+) and CD8(+) T cytotoxic cells, NK cells, higher IFN-gamma, reduction in myeloid-derived suppressor cells, and Tregs, all of which contribute to reducing tumor burden (186–188). These responses are also directly related to the functional relay of TLR4/Myd88 receptor adaptor response (133, 189, 190). For instance, TLR4−/− mice exhibit rapid tumor growth following inoculation (191) which corresponds to a deficiency in CD8+ CD4+ T and activated NK cells (42, 192).
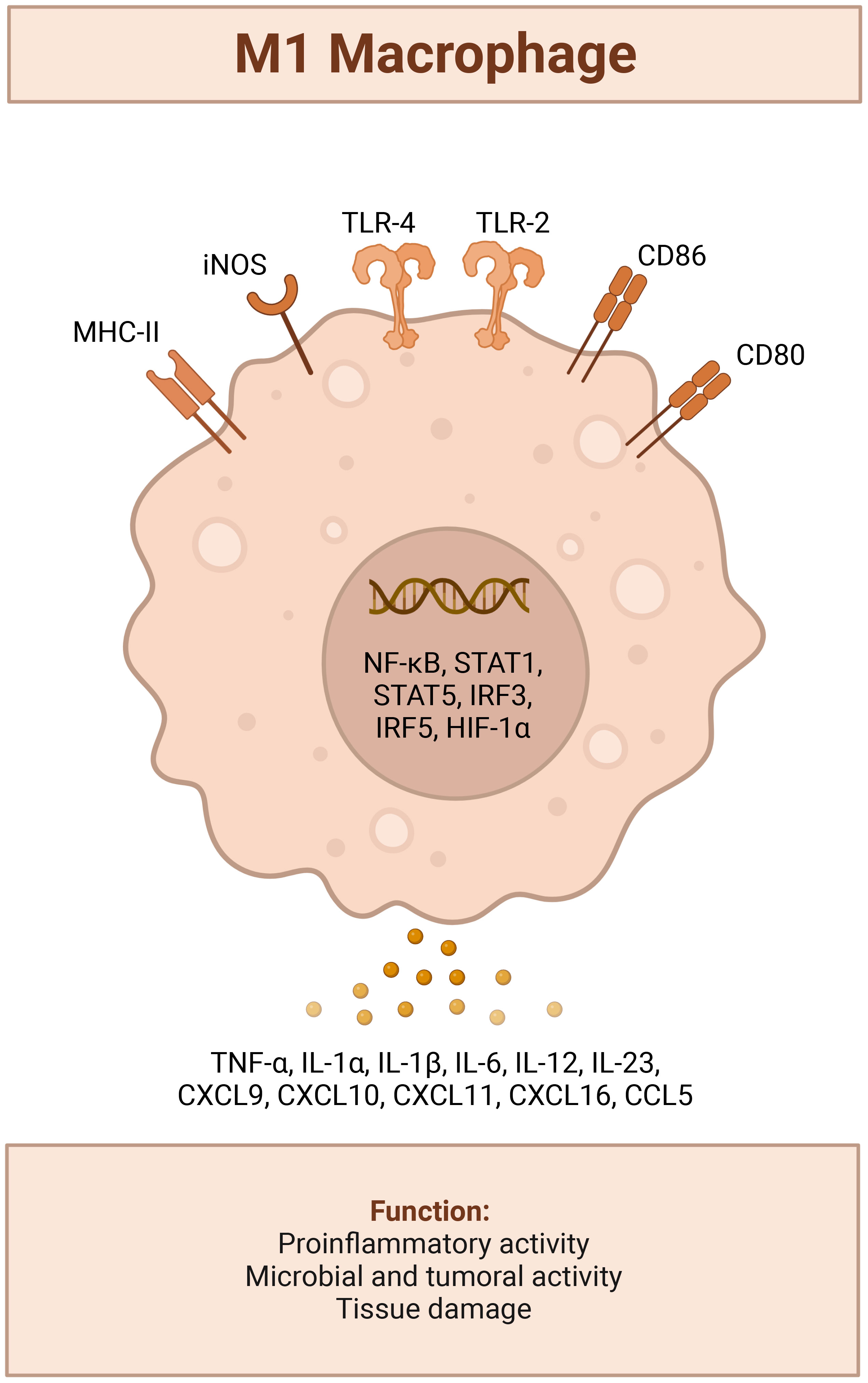
Figure 2 LPS TLR4 activated M1 tumor suppressor Macrophage Phenotype; triggers recruitment of MyD88 to the cytosolic domain, activates mitogen activated protein kinase signaling to elicit translocation of nuclear factor kappa-light-chain enhancer of activated B cells (NF-κB) and upregulation a host of genes involved with leukocyte recruitment (CCL2,CCL6,CCL12, CXCL10, CXCL11,CXCL12,CXCL13), a pro-inflammatory response (IFN-γ, TNF –α, IL-6 or Type I interferons IFN-α and IFN-β), iNOS induction and production of NO2.[(42)] Created with BioRender.com
The factors that maintain the M2 phenotype are still debated, but reportedly involve continuous exposure to cytokines released from the stroma/tumor itself, such as suppressive E-receptor factor, hyaluronan (193–196) or the dominance of gram-negative bacteria in the ‘tumor microbiome,’ as seen in gemcitabine-resistant pancreatic ductal carcinoma (197). The future therapeutic use of LPS/TLR4 agonists could enhance the efficacy of immune checkpoint inhibitors (ICIs) (198–201), increase the effectiveness of monoclonal antibodies (MAbs) (e.g., trastuzumab) (202) and boost the potency of platinum- and taxol-based chemotherapies (203). As of now, TLR4 agonists are primarily used as adjuvants in tumor vaccines (204–207) and in adoptive anti-tumor immunotherapies (208) as well as in dendritic cell-based therapies specific to tumor antigens (209).
3.2 The tumor microbiome: an immune suppressive barrier
The discovery of the tumor microbiome has brought significant challenges to the conceptual basis of tumor vaccines. This discovery creates a model akin to a house within a house. The larger house represents the human microbiome’s role in regulating systemic immunity, while the smaller house symbolizes the tumor microbiome’s role in controlling its local environment. The host microbiome, which largely colonizes the mucosal tissue of the oral/nasal cavity and gastrointestinal, vaginal, and urogenital tracts (97, 210), is sensitive to environmental factors (e.g., drugs, including antibiotics and chemotherapy, prebiotics, probiotics, alcohol, diet, stress aging, and exercise) (211–213).
When the normal flora is overtaken by pathogenic flora, as documented in thousands of studies [indicating changes in the composition of microbial communities in malignant versus healthy non-malignant tissues], it increases the risk of cancer (76, 214–216). Pathogenic flora that contribute to cancer can spread locally (e.g., from the vagina to the cervix (217), or from the respiratory system to the lungs (218)) or distally as bacteria originating in the oral cavity (e.g., Porphyromonas gingivalis, Tannerella forsythia, Veillonella parvula, F. nucleatum, Parvimonas Micra, etc.), have been found associated with cancers of the esophagus (219), GI/colon (220) pancreas (221) and liver (87). Once cancer is established, microorganisms can infiltrate the tumor microenvironment (TME), working synergistically with tumor-infiltrated leukocyte subpopulations (LSPs); tumor-associated macrophages (TAMs), tumor-associated neutrophils (TANs), myeloid-derived suppressor cells (MDSCs), CD4+CD25+Foxp3+Tregs creating a robust and strong immunosuppressive barrier (37, 87, 222, 223).
Overcoming the immunosuppressive barrier circumscribing tumors can be achieved by either the complete obliteration of the tumor microbiome, which can restore immune competence, decrease immune suppressors (MDSCs), and enhance Th1-type CD4+/cytotoxic CD8+ T function (221) or boosting the immune system (using immunotherapies), similar to the original MAMP vaccine by Coley, but with greater specificity (90, 224).
4 MAMPS and chronic inflammation
There is a potential double-edged sword involving the biological effects of LPS/TLR agonists, which on the one hand may boost immune response, and on the other hand are used in experimental models of inflammatory oxidative injury. Generally, repeated administration of LPS in animals is an established model of inflammatory injury to assess the value of anti-inflammatory agents (225, 226). The understudy of the paradox between immune stimulating vs chronic inflammation is a needed area of research given the large number of individuals, globally, who consume daily supplements containing fungal yeast (b-glucan)/microbial MAMP TLR agonists. There are several major plausible outcomes of repeated daily use 1) the establishment of biological tolerance, which can provide resilience to allergies (e.g., pollen) (227), eczema (228), or osteoporosis (229), infection and cancer (230, 231) or 2) chronic inflammation (232, 233) leading to refractory T-cell exhaustion, upregulation of (PD-1)/(PD-L1) axis, MDSCs and enhanced capacity of carcinogen-mediated tumorigenesis (26, 27, 234, 235);this raises an important question: Could repeated oral administration of MAMP-rich herbs like sea moss/kelp and roots result in immune suppression due to chronic inflammation, either of which could potentially initiate human cancers? In this context, promoting the intake of anti-inflammatory phytochemicals might be advisable to reduce the risk of cancer initiation (28, 236). By contrast, repeated TLR-4 stimulation could be linked to chronic infections and inflammatory conditions and may be contraindicated for individuals with autoimmune diseases (237). These are pressing issues that require further investigation for clarity.
5 Conclusion
This review looks into the potential of heat inactivated MOCs, particularly in botanicals, to boost immunity and improve illness outcomes. Specific immune-boosting herbs are abundant with dead microbes, thereby holding significance for drug discovery endeavors to develop oral, edible tumor immunotherapies. Future research should explore the intriguing hypothesis that the health benefits of certain herbs may be due to their inactivated microbial biomass rather than the plant’s phytochemicals; this would not only align with the broader field of microbiome science but also with the simple logic that the immune system reacts to foreign antigenic microorganism debris (MAMPs), the basis for vaccine and adjuvant development. Despite our growing knowledge, significant gaps still need to be discovered. For example, current research primarily focusing on cataloging the live microorganisms in the “edible or medicinal plant microbiome,” are often without considering potential health implications. While we understand which plant microbiomes survive food safety regulations, we need comprehensive knowledge of MAMPS from inactivated MOCs and their immune-boosting potential, including the concentrations and taxonomy, from pathogenic to non-pathogenic.
This potential paradigm shift could have significant implications for our understanding of plant-based health and for developing new therapeutic approaches. Furthermore, the historical backdrop of MOCs’ influence on immunity, as indicated by cases of spontaneous tumor remission due to acute febrile illnesses, lays the groundwork for investigating MOCs’ therapeutic potential in cancer immunotherapy. This has the potential to transform our approach to disease prevention and treatment, specifically by using the ability of MOCs to strengthen human defense against pathogens and improve tumor surveillance.
Author contributions
All authors listed have made a substantial, direct, and intellectual contribution to the work, and approved it for publication.
Funding
This project was supported by the FAMU Center of Health disparities research grant, RCMI 5U54MD007582 from NIH.
Conflict of interest
The authors declare that the research was conducted in the absence of any commercial or financial relationships that could be construed as a potential conflict of interest.
Publisher’s note
All claims expressed in this article are solely those of the authors and do not necessarily represent those of their affiliated organizations, or those of the publisher, the editors and the reviewers. Any product that may be evaluated in this article, or claim that may be made by its manufacturer, is not guaranteed or endorsed by the publisher.
References
1. Shah AH, Jusue-Torres I, Ivan ME, Komotar RJ, Kasahara N. Pathogens and glioma: a history of unexpected discoveries ushering in novel therapy. J Neurosurg (2018) 128(4):1139–46. doi: 10.3171/2016.12.JNS162123
3. Vacchelli E, Eggermont A, Sautes-Fridman C, Galon J, Zitvogel L, Kroemer G, et al. Trial watch: toll-like receptor agonists for cancer therapy. Oncoimmunology (2013) 2(8):e25238. doi: 10.4161/onci.25238
4. Hobohm U. Fever and cancer in perspective. Cancer Immunol Immunother (2001) 50(8):391–6. doi: 10.1007/s002620100216
5. McCarthy EF, Iowa Orthop J. The toxins of William b. coley and the treatment of bone and soft-tissue sarcomas. (2006) 26:154–8.
6. Hoption Cann SA, van Netten JP, van Netten C. Dr William coley and tumour regression: a place in history or in the future. Postgrad Med J (2003) 79(938):672–80. doi: 10.1093/postgradmedj/79.938.672
7. Orange M, Reuter U, Hobohm U. Coley's lessons remembered: augmenting mistletoe therapy. Integr Cancer Ther (2016) 15(4):502–11. doi: 10.1177/1534735416649916
8. Cole WH. Spontaneous regression of cancer and the importance of finding its cause. Natl Cancer Inst Monogr (1976) 44:5–9.
9. Bertaglia V, Morelli AM, Solinas C, Aiello MM, Manunta S, Denaro N, et al. Infections in lung cancer patients undergoing immunotherapy and targeted therapy: an overview on the current scenario. Crit Rev Oncol Hematol (2023) 184:103954. doi: 10.1016/j.critrevonc.2023.103954
10. Ruan J, Sun S, Cheng X, Han P, Zhang Y, Sun D. Mitomycin, 5-fluorouracil, leflunomide, and mycophenolic acid directly promote hepatitis b virus replication and expression in vitro. Virol J (2020) 17(1):89. doi: 10.1186/s12985-020-01339-5
11. Snidvongs S, Mehta V. Recent advances in opioid prescription for chronic non-cancer pain. Postgrad Med J (2012) 88(1036):66–72. doi: 10.1136/pgmj.2010.112045
12. Goodman RS, Johnson DB, Balko JM. Corticosteroids and cancer immunotherapy. Clin Cancer Res (2023). doi: 10.1158/1078-0432.CCR-22-3181
13. Vallejo R, Hord ED, Barna SA, Santiago-Palma J, Ahmed S. Perioperative immunosuppression in cancer patients. J Environ Pathol Toxicol Oncol (2003) 22(2):139–46. doi: 10.1615/JEnvPathToxOncol.v22.i2.70
14. Tange S, Scherer MN, Graeb C, Weiss T, Justl M, Frank E, et al. The antineoplastic drug paclitaxel has immunosuppressive properties that can effectively promote allograft survival in a rat heart transplant model. Transplantation (2002) 73(2):216–23. doi: 10.1097/00007890-200201270-00011
16. Worku M, Belay G, Tigabu A. Bacterial profile and antimicrobial susceptibility patterns in cancer patients. PloS One (2022) 17(4):e0266919. doi: 10.1371/journal.pone.0266919
17. Uneno Y, Imura H, Makuuchi Y, Tochitani K, Watanabe N. Pre-emptive antifungal therapy versus empirical antifungal therapy for febrile neutropenia in people with cancer. Cochrane Database Syst Rev (2022) 11(11):CD013604.
18. Rao L, Zhang K, Luo H, He S, Li Y, Liu C, et al. The ability of inflammatory markers to recognize infection in cancer patients with fever at admission. Immunol Res (2022) 70(5):667–77. doi: 10.1007/s12026-022-09299-4
19. Wang WW, Wu L, Lu W, Chen W, Yan W, Qi C, et al. Lipopolysaccharides increase the risk of colorectal cancer recurrence and metastasis due to the induction of neutrophil extracellular traps after curative resection. J Cancer Res Clin Oncol (2021) 147(9):2609–19. doi: 10.1007/s00432-021-03682-8
20. Walker RW, Clemente JC, Peter I, Loos RJF. The prenatal gut microbiome: are we colonized with bacteria in utero? Pediatr Obes (2017) 12 Suppl 1(Suppl 1):3–17. doi: 10.1111/ijpo.12217
21. Grecco GG, Gao Y, Gao H, Liu Y, Atwood BK. Prenatal opioid administration induces shared alterations to the maternal and offspring gut microbiome: a preliminary analysis. Drug Alcohol Depend (2021) 227:108914. doi: 10.1016/j.drugalcdep.2021.108914
22. Alvarez J, Fernandez Real JM, Guarner F, Gueimonde M, Rodriguez JM, Saenz de Pipaon M, et al. Gut microbes and health. Gastroenterol Hepatol (2021) 44(7):519–35. doi: 10.1016/j.gastre.2021.01.002
23. Perkin MR, Strachan DP. The hygiene hypothesis for allergy - conception and evolution. Front Allergy (2022) 3:1051368. doi: 10.3389/falgy.2022.1051368
24. Pfefferle PI, Postigo I, Garn H. Editorial: the immunological implications of the hygiene hypothesis. Front Immunol (2021) 12:732127. doi: 10.3389/fimmu.2021.732127
26. Liu CH, Chen Z, Chen K, Liao FT, Chung CE, Liu X, et al. Lipopolysaccharide-mediated chronic inflammation promotes tobacco carcinogen-induced lung cancer and determines the efficacy of immunotherapy. Cancer Res (2021) 81(1):144–57. doi: 10.1158/0008-5472.CAN-20-1994
27. Di ME, Kahkonen B, Liu CH, Di YP. Lung carcinomas induced by NNK and LPS. Methods Cell Biol (2021) 163:175–85. doi: 10.1016/bs.mcb.2021.01.002
28. Chen CY, Kao CL, Liu CM. The cancer prevention, anti-inflammatory and anti-oxidation of bioactive phytochemicals targeting the TLR4 signaling pathway. Int J Mol Sci (2018) 19(9):1–17. doi: 10.3390/ijms19092729
29. Michels N, van Aart C, Morisse J, Mullee A, Huybrechts I. Chronic inflammation towards cancer incidence: a systematic review and meta-analysis of epidemiological studies. Crit Rev Oncol Hematol (2021) 157:103177. doi: 10.1016/j.critrevonc.2020.103177
30. Tagliamonte M, Buonaguro L. The impact of antigenic molecular mimicry on anti-cancer T-cell immune response. Front Oncol (2022) 12:1009247. doi: 10.3389/fonc.2022.1009247
31. Rama I, Grinyo JM. Malignancy after renal transplantation: the role of immunosuppression. Nat Rev Nephrol (2010) 6(9):511–9. doi: 10.1038/nrneph.2010.102
32. Vial T, Descotes J. Immunosuppressive drugs and cancer. Toxicology (2003) 185(3):229–40. doi: 10.1016/S0300-483X(02)00612-1
33. Penn I. Secondary neoplasms as a consequence of transplantation and cancer therapy. Cancer Detect Prev (1988) 12(1-6):39–57.
34. Stucker F, Ackermann D. [Immunosuppressive drugs - how they work, their side effects and interactions]. Ther Umsch (2011) 68(12):679–86. doi: 10.1024/0040-5930/a000230
35. Schiller JT, Lowy DR. Prospects for preventing cancer with anti-microbial prophylactic vaccines. Cell Host Microbe (2022). doi: 10.1016/j.chom.2022.10.016
36. You L, Zhou J, Xin Z, Hauck JS, Na F, Tang J, et al. Novel directions of precision oncology: circulating microbial DNA emerging in cancer-microbiome areas. Precis Clin Med (2022) 5(1):pbac005. doi: 10.1093/pcmedi/pbac005
37. Seely KD, Morgan AD, Hagenstein LD, Florey GM, Small JM. Bacterial involvement in progression and metastasis of colorectal neoplasia. Cancers (Basel) (2022) 14(4). doi: 10.3390/cancers14041019
38. Hiddemann W. What's new in malignant tumors in acquired immunodeficiency disorders? Pathol Res Pract (1989) 185(6):930–4. doi: 10.1016/S0344-0338(89)80300-0
39. Kumar V, Barrett JE. Toll-like receptors (TLRs) in health and disease: an overview. Handb Exp Pharmacol (2022) 276:1–21. doi: 10.1007/164_2021_568
40. Yan Y, Yao D, Li X. Immunological mechanism and clinical application of PAMP adjuvants. Recent Pat Anticancer Drug Discovery (2021) 16(1):30–43. doi: 10.2174/1574892816666210201114712
41. Ong GH, Lian BSX, Kawasaki T, Kawai T. Exploration of pattern recognition receptor agonists as candidate adjuvants. Front Cell Infect Microbiol (2021) 11:745016. doi: 10.3389/fcimb.2021.745016
42. Luchner M, Reinke S, Milicic A. TLR agonists as vaccine adjuvants targeting cancer and infectious diseases. Pharmaceutics (2021) 13(2). doi: 10.3390/pharmaceutics13020142
43. Rossi O, Mastroeni P. Editorial: pattern recognition receptors at the crosstalk between innate and adaptive immune systems and implications for vaccine development. Front Cell Infect Microbiol (2022) 12:1088029. doi: 10.3389/fcimb.2022.1088029
44. Shi YG, Zhu CM, Li DH, Shi ZY, Gu Q, Chen YW, et al. New horizons in microbiological food safety: ultraefficient photodynamic inactivation based on a Gallic acid derivative and UV-a light and its application with electrospun cyclodextrin nanofibers. J Agric Food Chem (2021) 69(49):14961–74. doi: 10.1021/acs.jafc.1c04827
45. Sholtes KA, Lowe K, Walters GW, Sobsey MD, Linden KG, Casanova LM. Comparison of ultraviolet light-emitting diodes and low-pressure mercury-arc lamps for disinfection of water. Environ Technol (2016) 37(17):2183–8. doi: 10.1080/09593330.2016.1144798
46. Ma L, Yang H, Guan L, Liu X, Zhang T. Risks of antibiotic resistance genes and antimicrobial resistance under chlorination disinfection with public health concerns. Environ Int (2022) 158:106978. doi: 10.1016/j.envint.2021.106978
47. Jefri U, Khan A, Lim YC, Lee KS, Liew KB, Kassab YW, et al. A systematic review on chlorine dioxide as a disinfectant. J Med Life (2022) 15(3):313–8. doi: 10.25122/jml-2021-0180
48. Jovanovic J, Tretiak S, Begyn K, Rajkovic A. Detection of enterotoxigenic psychrotrophic presumptive bacillus cereus and cereulide producers in food products and ingredients. Toxins (Basel) (2022) 14(4). doi: 10.3390/toxins14040289
49. Becker B, Stoll D, Schulz P, Kulling S, Huch M. Microbial contamination of organically and conventionally produced fresh vegetable salads and herbs from retail markets in southwest Germany. Foodborne Pathog Dis (2019) 16(4):269–75. doi: 10.1089/fpd.2018.2541
50. Inagawa H, Kohchi C, Soma G. Oral administration of lipopolysaccharides for the prevention of various diseases: benefit and usefulness. Anticancer Res (2011) 31(7):2431–6.
51. Soto-Giron MJ, Kim JN, Schott E, Tahmin C, Ishoey T, Mincer TJ, et al. The edible plant microbiome represents a diverse genetic reservoir with functional potential in the human host. Sci Rep (2021) 11(1):24017. doi: 10.1038/s41598-021-03334-4
52. Abdullaeva Y, Manirajan Ambika B, Honermeier B, Schnell S, Cardinale M. Domestication affects the composition, diversity, and co-occurrence of the cereal seed microbiota. J Adv Res (2021) 31:75–86. doi: 10.1016/j.jare.2020.12.008
53. Higgins D, Pal C, Sulaiman IM, Jia C, Zerwekh T, Dowd SE, et al. Application of high-throughput pyrosequencing in the analysis of microbiota of food commodities procured from small and large retail outlets in a U.S. metropolitan area - a pilot study. Food Res Int (2018) 105:29–40. doi: 10.1016/j.foodres.2017.10.057
54. Frohling A, Rademacher A, Rumpold B, Klocke M, Schluter O. Screening of microbial communities associated with endive lettuce during postharvest processing on industrial scale. Heliyon (2018) 4(7):e00671. doi: 10.1016/j.heliyon.2018.e00671
55. Hong S, Yuan X, Yang J, Yang Y, Jv H, Li R, et al. Selection of rhizosphere communities of diverse rotation crops reveals unique core microbiome associated with reduced banana fusarium wilt disease. New Phytol (2023). doi: 10.1111/nph.18816
56. Nakayasu M, Takamatsu K, Yazaki K, Sugiyama A. Plant specialized metabolites in the rhizosphere of tomatoes: secretion and effects on microorganisms. Biosci Biotechnol Biochem (2022) 87(1):13–20. doi: 10.1093/bbb/zbac181
57. Agarussi MCN, Pereira OG, Pimentel FE, Azevedo CF, da Silva VP. Microbiome of rehydrated corn and sorghum grain silages treated with microbial inoculants in different fermentation periods. Sci Rep (2022) 12(1):16864. doi: 10.1038/s41598-022-21461-4
58. Abera S, Shimels M, Tessema T, Raaijmakers JM, Dini-Andreote F. Back to the roots: defining the core microbiome of sorghum bicolor in agricultural field soils from the centre of origin. FEMS Microbiol Ecol (2022) 98(12). doi: 10.1093/femsec/fiac136
59. Pugh ND, Tamta H, Balachandran P, Wu X, Howell J, Dayan FE, et al. The majority of in vitro macrophage activation exhibited by extracts of some immune enhancing botanicals is due to bacterial lipoproteins and lipopolysaccharides. Int Immunopharmacol (2008) 8(7):1023–32. doi: 10.1016/j.intimp.2008.03.007
60. Koehler H, Puchalski K, Ruiz G, Jacobs B, Langland J. The role of Endophytic/Epiphytic bacterial constituents in the immunostimulatory activity of the botanical, astragalus membranaceus. Yale J Biol Med (2020) 93(2):239–50.
61. Denzler KL, Waters R, Jacobs BL, Rochon Y, Langland JO. Regulation of inflammatory gene expression in PBMCs by immunostimulatory botanicals. PloS One (2010) 5(9):e12561. doi: 10.1371/journal.pone.0012561
62. Montenegro D, Kalpana K, Chrissian C, Sharma A, Takaoka A, Iacovidou M, et al. Uncovering potential 'herbal probiotics' in juzen-taiho-to through the study of associated bacterial populations. Bioorg Med Chem Lett (2015) 25(3):466–9. doi: 10.1016/j.bmcl.2014.12.036
63. Nishizawa T, Inagawa H, Oshima H, Okutomi T, Tsukioka D, Iguchi M, et al. Homeostasis as regulated by activated macrophage. i. lipopolysaccharide (LPS) from wheat flour: isolation, purification and some biological activities. Chem Pharm Bull (Tokyo) (1992) 40(2):479–83. doi: 10.1248/cpb.40.479
64. Mizuno D, Soma G. Oral or percutaneous administration of lipopolysaccharide of small molecular size may cure various intractable diseases: a new version of coley's toxin. Mol Biother (1992) 4(4):166–9.
65. Taniguchi Y, Yoshioka N, Nishizawa T, Inagawa H, Kohchi C, Soma G. Utility and safety of LPS-based fermented flour extract as a macrophage activator. Anticancer Res (2009) 29(3):859–64.
66. Kohchi C, Inagawa H, Nishizawa T, Yamaguchi T, Nagai S, Soma G. Applications of lipopolysaccharide derived from pantoea agglomerans (IP-PA1) for health care based on macrophage network theory. J Biosci Bioeng (2006) 102(6):485–96. doi: 10.1263/jbb.102.485
67. Lakshmanan V, Ray P, Craven KD. Rhizosphere sampling protocols for microbiome (16S/18S/ITS rRNA) library preparation and enrichment for the isolation of drought tolerance-promoting microbes. Methods Mol Biol (2017) 1631:349–62. doi: 10.1007/978-1-4939-7136-7_23
68. Miranda K, Weigel BL, Fogarty EC, Veseli IA, Giblin AE, Eren AM, et al. The diversity and functional capacity of microbes associated with coastal macrophytes. mSystems (2022):e0059222. doi: 10.1128/msystems.00592-22
69. Weigel BL, Pfister CA. Oxygen metabolism shapes microbial settlement on photosynthetic kelp blades compared to artificial kelp substrates. Environ Microbiol Rep (2021) 13(2):176–84. doi: 10.1111/1758-2229.12923
70. Taverniti V, Guglielmetti S. The immunomodulatory properties of probiotic microorganisms beyond their viability (ghost probiotics: proposal of paraprobiotic concept). Genes Nutr (2011) 6(3):261–74. doi: 10.1007/s12263-011-0218-x
71. Vallejo-Cordoba B, Castro-Lopez C, Garcia HS, Gonzalez-Cordova AF, Hernandez-Mendoza A. Postbiotics and paraprobiotics: a review of current evidence and emerging trends. Adv Food Nutr Res (2020) 94:1–34. doi: 10.1016/bs.afnr.2020.06.001
72. Fiore W, Arioli S, Guglielmetti S. The neglected microbial components of commercial probiotic formulations. Microorganisms (2020) 8(8). doi: 10.3390/microorganisms8081177
73. Akter S, Park JH, Jung HK. Potential health-promoting benefits of paraprobiotics, inactivated probiotic cells. J Microbiol Biotechnol (2020) 30(4):477–81. doi: 10.4014/jmb.1911.11019
74. Deshpande G, Athalye-Jape G, Patole S. Para-probiotics for preterm neonates-the next frontier. Nutrients (2018) 10(7). doi: 10.3390/nu10070871
75. Iqbal S, Zebeli Q, Mansmann DA, Dunn SM, Ametaj BN. Oral administration of LPS and lipoteichoic acid prepartum modulated reactants of innate and humoral immunity in periparturient dairy cows. Innate Immun (2014) 20(4):390–400. doi: 10.1177/1753425913496125
76. Zhou Y, Zhou C, Zhang A. Gut microbiota in acute leukemia: current evidence and future directions. Front Microbiol (2022) 13:1045497. doi: 10.3389/fmicb.2022.1045497
77. Samanta S. Potential impacts of prebiotics and probiotics on cancer prevention. Anticancer Agents Med Chem (2022) 22(4):605–28. doi: 10.2174/1871520621999201210220442
78. Saeed M, Shoaib A, Kandimalla R, Javed S, Almatroudi A, Gupta R, et al. Microbe-based therapies for colorectal cancer: advantages and limitations. Semin Cancer Biol (2022) 86(Pt 3):652–65. doi: 10.1016/j.semcancer.2021.05.018
79. Zhou A, Tang L, Zeng S, Lei Y, Yang S, Tang B. Gut microbiota: a new piece in understanding hepatocarcinogenesis. Cancer Lett (2020) 474:15–22. doi: 10.1016/j.canlet.2020.01.002
80. Siddiqui AJ, Jahan S, Singh R, Saxena J, Ashraf SA, Khan A, et al. Plants in anticancer drug discovery: from molecular mechanism to chemoprevention. BioMed Res Int (2022) 2022:5425485. doi: 10.1155/2022/5425485
81. Xia Y, Lian S, Khoi PN, Yoon HJ, Joo YE, Chay KO, et al. Chrysin inhibits tumor promoter-induced MMP-9 expression by blocking AP-1 via suppression of ERK and JNK pathways in gastric cancer cells. PloS One (2015) 10(4):e0124007. doi: 10.1371/journal.pone.0124007
82. Park C, Lee WS, Go SI, Nagappan A, Han MH, Hong SH, et al. Morin, a flavonoid from moraceae, induces apoptosis by induction of BAD protein in human leukemic cells. Int J Mol Sci (2014) 16(1):645–59. doi: 10.3390/ijms16010645
83. Bhatia M, Bhalerao M, Cruz-Martins N, Kumar D. Curcumin and cancer biology: focusing regulatory effects in different signalling pathways. Phytother Res (2021) 35(9):4913–29. doi: 10.1002/ptr.7121
84. Upreti S, Pandey SC, Bisht I, Samant M. Evaluation of the target-specific therapeutic potential of herbal compounds for the treatment of cancer. Mol Divers (2022) 26(3):1823–35. doi: 10.1007/s11030-021-10271-x
85. Sahoo A, Mandal AK, Kumar M, Dwivedi K, Singh D. Prospective challenges for patenting and clinical trials of anticancer compounds from natural products: coherent review. Recent Pat Anticancer Drug Discov (2022). doi: 10.2174/1574892818666221104113703
86. Pattnaik S, Imchen M, Kumavath R, Prasad R, Busi S. Bioactive microbial metabolites in cancer therapeutics: mining, repurposing, and their molecular targets. Curr Microbiol (2022) 79(10):300. doi: 10.1007/s00284-022-02990-7
87. Kleef R, Jonas WB, Knogler W, Stenzinger W. Fever, cancer incidence and spontaneous remissions. Neuroimmunomodulation (2001) 9(2):55–64. doi: 10.1159/000049008
88. Zhou L, Zhang M, Fu Q, Li J, Sun H. Targeted near infrared hyperthermia combined with immune stimulation for optimized therapeutic efficacy in thyroid cancer treatment. Oncotarget (2016) 7(6):6878–90. doi: 10.18632/oncotarget.6901
89. Soleimani N, Javadi MM. Future prospects of bacteria-mediated cancer therapies: Affliction or opportunity? Microb Pathog (2022) 172:105795. doi: 10.1016/j.micpath.2022.105795
90. Kokorovic A, Ory J, Saad F. Emerging treatment options for bacillus calmette- guerin-unresponsive non-muscle invasive bladder cancer. Curr Opin Support Palliat Care (2022) 16(1):48–53. doi: 10.1097/SPC.0000000000000587
91. Garcia-Martinez E, Smith M, Buque A, Aranda F, de la Pena FA, Ivars A, et al. Trial watch: Immunostimulation with recombinant cytokines for cancer therapy. Oncoimmunology (2018) 7(6):e1433982. doi: 10.1080/2162402X.2018.1433982
92. Maiorano BA, Maiorano MFP, Ciardiello D, Maglione A, Orditura M, Lorusso D, et al. Beyond platinum, ICIs in metastatic cervical cancer: A systematic review. Cancers (Basel) (2022) 14(23). doi: 10.3390/cancers14235955
93. Borgers JSW, Haanen J. Cellular therapy and cytokine treatments for melanoma. Hematol Oncol Clin North Am (2021) 35(1):129–44. doi: 10.1016/j.hoc.2020.08.014
94. Conlon KC, Miljkovic MD, Waldmann TA. Cytokines in the treatment of cancer. J Interferon Cytokine Res (2019) 39(1):6–21. doi: 10.1089/jir.2018.0019
95. Tagliamonte M, Petrizzo A, Tornesello ML, Buonaguro FM, Buonaguro L. Antigen-specific vaccines for cancer treatment. Hum Vaccin Immunother (2014) 10(11):3332–46. doi: 10.4161/21645515.2014.973317
96. Roberti MP, Rauber C, Kroemer G, Zitvogel L. Impact of the ileal microbiota on colon cancer. Semin Cancer Biol (2022) 86(Pt 2):955–66. doi: 10.1016/j.semcancer.2021.09.016
97. Patin EC, Thompson A, Orr SJ. Pattern recognition receptors in fungal immunity. Semin Cell Dev Biol (2019) 89:24–33. doi: 10.1016/j.semcdb.2018.03.003
98. Oblak A, Jerala R. Toll-like receptor 4 activation in cancer progression and therapy. Clin Dev Immunol (2011) 2011:609579. doi: 10.1155/2011/609579
99. Wolska A, Lech-Maranda E, Robak T. Toll-like receptors and their role in hematologic malignancies. Curr Mol Med (2009) 9(3):324–35. doi: 10.2174/156652409787847182
100. Galluzzi L, Vacchelli E, Eggermont A, Fridman WH, Galon J, Sautes-Fridman C, et al. Trial watch: Experimental toll-like receptor agonists for cancer therapy. Oncoimmunology (2012) 1(5):699–716. doi: 10.4161/onci.20696
101. Wolska A, Lech-Maranda E, Robak T. Toll-like receptors and their role in carcinogenesis and anti-tumor treatment. Cell Mol Biol Lett (2009) 14(2):248–72. doi: 10.2478/s11658-008-0048-z
102. Ehrchen JM, Sunderkotter C, Foell D, Vogl T, Roth J. The endogenous toll-like receptor 4 agonist S100A8/S100A9 (calprotectin) as innate amplifier of infection, autoimmunity, and cancer. J Leukoc Biol (2009) 86(3):557–66. doi: 10.1189/jlb.1008647
103. Gulyas D, Kovacs G, Jankovics I, Meszaros L, Lorincz M, Denes B. Effects of the combination of a monoclonal agonistic mouse anti-OX40 antibody and toll-like receptor agonists: Unmethylated CpG and LPS on an MB49 bladder cancer cell line in a mouse model. PloS One (2022) 17(7):e0270802. doi: 10.1371/journal.pone.0270802
104. Vetvicka V, Vetvickova J. Anti-infectious and anti-tumor activities of beta-glucans. Anticancer Res (2020) 40(6):3139–45. doi: 10.21873/anticanres.14295
105. Vetvicka V. Glucan-immunostimulant, adjuvant, potential drug. World J Clin Oncol (2011) 2(2):115–9. doi: 10.5306/wjco.v2.i2.115
106. Geller A, Shrestha R, Yan J. Yeast-derived beta-glucan in cancer: Novel uses of a traditional therapeutic. Int J Mol Sci (2019) 20(15). doi: 10.3390/ijms20153618
107. Xiang D, Sharma VR, Freter CE, Yan J. Anti-tumor monoclonal antibodies in conjunction with beta-glucans: a novel anti-cancer immunotherapy. Curr Med Chem (2012) 19(25):4298–305. doi: 10.2174/092986712802884303
108. Li B, Cai Y, Qi C, Hansen R, Ding C, Mitchell TC, et al. Orally administered particulate beta-glucan modulates tumor-capturing dendritic cells and improves antitumor t-cell responses in cancer. Clin Cancer Res (2010) 16(21):5153–64. doi: 10.1158/1078-0432.CCR-10-0820
109. Liu M, Luo F, Ding C, Albeituni S, Hu X, Ma Y, et al. Dectin-1 activation by a natural product beta-glucan converts immunosuppressive macrophages into an M1- like phenotype. J Immunol (2015) 195(10):5055–65. doi: 10.4049/jimmunol.1501158
110. Xin Y, Ji H, Cho E, Roh KB, You J, Park D, et al. Immune-enhancing effect of water-soluble beta-glucan derived from enzymatic hydrolysis of yeast glucan. Biochem Biophys Rep (2022) 30:101256. doi: 10.1016/j.bbrep.2022.101256
111. Albeituni SH, Ding C, Liu M, Hu X, Luo F, Kloecker G, et al. Yeast-derived particulate beta-glucan treatment subverts the suppression of myeloid-derived suppressor cells (MDSC) by inducing polymorphonuclear MDSC apoptosis and monocytic MDSC differentiation to APC in cancer. J Immunol (2016) 196(5):2167– 80. doi: 10.4049/jimmunol.1501853
112. Ding J, Feng T, Ning Y, Li W, Wu Q, Qian K, et al. Beta-glucan enhances cytotoxic t lymphocyte responses by activation of human monocyte-derived dendritic cells via the PI3K/AKT pathway. Hum Immunol (2015) 76(2-3):146–54. doi: 10.1016/j.humimm.2015.01.009
113. Su Y, Chen L, Yang F, Cheung PCK. Beta-d-glucan-based drug delivery system and its potential application in targeting tumor associated macrophages. Carbohydr Polym (2021) 253:117258. doi: 10.1016/j.carbpol.2020.117258
114. Chan ASH, Kangas TO, Qiu X, Uhlik MT, Fulton RB, Ottoson NR, et al. Imprime PGG enhances anti-tumor effects of tumor-targeting, anti-angiogenic, and immune checkpoint inhibitor antibodies. Front Oncol (2022) 12:869078. doi: 10.3389/fonc.2022.869078
115. Albeituni SH, Yan J. The effects of beta-glucans on dendritic cells and implications for cancer therapy. Anticancer Agents Med Chem (2013) 13(5):689–98. doi: 10.2174/1871520611313050003
116. Taghavi M, Mortaz E, Khosravi A, Vahedi G, Folkerts G, Varahram M, et al. Zymosan attenuates melanoma growth progression, increases splenocyte proliferation and induces TLR-2/4 and TNF-alpha expression in mice. J Inflammation (Lond) (20185) 15. doi: 10.1186/s12950-018-0182-y
117. Geller AE, Shrestha R, Woeste MR, Guo H, Hu X, Ding C, et al. The induction of peripheral trained immunity in the pancreas incites anti-tumor activity to control pancreatic cancer progression. Nat Commun (2022) 13(1):759. doi: 10.1038/s41467-022-28407-4
118. Yoon TJ, Koppula S, Lee KH. The effects of beta-glucans on cancer metastasis. Anticancer Agents Med Chem (2013) 13(5):699–708. doi: 10.2174/1871520611313050004
119. Steimbach L, Borgmann AV, Gomar GG, Hoffmann LV, Rutckeviski R, de Andrade DP, et al. Fungal beta-glucans as adjuvants for treating cancer patients - a systematic review of clinical trials. Clin Nutr (2021) 40(5):3104–13. doi: 10.1016/j.clnu.2020.11.029
120. Bose N, Ottoson NR, Qiu X, Harrison B, Lowe JR, Uhlik MT, et al. Immune pharmacodynamic responses of the novel cancer immunotherapeutic imprime PGG in healthy volunteers. J Immunol (2019) 202(10):2945–56. doi: 10.4049/jimmunol.1801533
121. Mo S, Ru H, Huang M, Cheng L, Mo X, Yan L. Oral-intestinal microbiota in colorectal cancer: Inflammation and immunosuppression. J Inflammation Res (2022) 15:747–59. doi: 10.2147/JIR.S344321
122. Debnath N, Thakur M, Khushboo NP, Gautam V, Kumar Yadav A, et al. Insight of oral vaccines as an alternative approach to health and disease management: An innovative intuition and challenges. Biotechnol Bioeng (2022) 119(2):327–46. doi: 10.1002/bit.27987
123. Stander J, Mbewana S, Meyers AE. Plant-derived human vaccines: Recent developments. BioDrugs (2022). doi: 10.1007/s40259-022-00544-8
124. Paradia PK, Bhavale R, Agnihotri T, Jain A. A review on edible vaccines and biopharmaceutical products from plants. Curr Pharm Biotechnol (2022). doi: 10.2174/1389201023666220803151039
125. Smith KM, Davidson JM, Garside P. T-cell activation occurs simultaneously in local and peripheral lymphoid tissue following oral administration of a range of doses of immunogenic or tolerogenic antigen although tolerized t cells display a defect in cell division. Immunology (2002) 106(2):144–58. doi: 10.1046/j.1365-2567.2002.01427.x
126. Gallichan WS, Rosenthal KL. Long-lived cytotoxic t lymphocyte memory in mucosal tissues after mucosal but not systemic immunization. J Exp Med (1996) 184(5):1879–90. doi: 10.1084/jem.184.5.1879
127. Woolverton CJ, Holt LC, Mitchell D, Sartor RB. Identification and characterization of rat intestinal lamina propria cells: consequences of microbial colonization. Vet Immunol Immunopathol (1992) 34(1-2):127–38. doi: 10.1016/0165-2427(92)90156-k
128. Soma G, Inagawa H. Methods to prevent or treat refractory diseases by focusing on intestinal microbes using LPS and macrophages. Anticancer Res (2015) 35(8):4393–6.
129. Ignacio RMC, Lee ES, Son DS. Potential roles of innate immune chemokine and cytokine network on lipopolysaccharide-based therapeutic approach in ovarian cancer. Immune Netw (2019) 19(3):e22. doi: 10.4110/in.2019.19.e22
130. Okuyama H, Tominaga A, Fukuoka S, Taguchi T, Kusumoto Y, Ono S. Spirulina lipopolysaccharides inhibit tumor growth in a toll-like receptor 4-dependent manner by altering the cytokine milieu from interleukin-17/interleukin-23 to interferon- gamma. Oncol Rep (2017) 37(2):684–94. doi: 10.3892/or.2017.5346
131. Won EK, Zahner MC, Grant EA, Gore P, Chicoine MR. Analysis of the antitumoral mechanisms of lipopolysaccharide against glioblastoma multiforme. Anticancer Drugs (2003) 14(6):457–66. doi: 10.1097/00001813-200307000-00012
132. Vigneron C, Mirouse A, Merdji H, Rousseau C, Cousin C, Alby-Laurent F, et al. Sepsis inhibits tumor growth in mice with cancer through toll-like receptor 4-associated enhanced natural killer cell activity. Oncoimmunology2019 8(11):e1641391. doi: 10.1080/2162402X.2019.1641391
133. Tanaka M, Abe S. Augmented anti-tumour effect of lipopolysaccharide with g-CSF without enhancing body weight loss in mice bearing MH134 hepatoma. Eur J Pharmacol (2022) 934:175206. doi: 10.1016/j.ejphar.2022.175206
134. Janotova T, Jalovecka M, Auerova M, Svecova I, Bruzlova P, Maierova V, et al. The use of anchored agonists of phagocytic receptors for cancer immunotherapy: B16- F10 murine melanoma model. PloS One (2014) 9(1):e85222. doi: 10.1371/journal.pone.0085222
135. Chicoine MR, Won EK, Zahner MC. Intratumoral injection of lipopolysaccharide causes regression of subcutaneously implanted mouse glioblastoma multiforme. Neurosurgery (2001) 48(3):607–14. doi: 10.1097/00006123-200103000-00032
136. Chicoine MR, Zahner M, Won EK, Kalra RR, Kitamura T, Perry A, et al. The in vivo antitumoral effects of lipopolysaccharide against glioblastoma multiforme are mediated in part by toll-like receptor 4. Neurosurgery (2007) 60(2):372–80. doi: 10.1227/01.NEU.0000249280.61761.2E
137. Morishima A, Inagawa H. Clinical effects of orally administered lipopolysaccharide derived from pantoea agglomerans on malignant tumors. Anticancer Res (2016) 36(7):3747–51.
138. Kim JH, Kim DH, Jo S, Cho MJ, Cho YR, Lee YJ, et al. Immunomodulatory functional foods and their molecular mechanisms. Exp Mol Med (2022) 54(1):1–11. doi: 10.1038/s12276-022-00724-0
139. Skjanes K, Aesoy R, Herfindal L, Skomedal H. Bioactive peptides from microalgae: Focus on anti-cancer and immunomodulating activity. Physiol Plant (2021) 173(2):612–23. doi: 10.1111/ppl.13472
140. Ishiguro S, Robben N, Burghart R, Cote P, Greenway S, Thakkar R, et al. Cell wall membrane fraction of chlorella sorokiniana enhances host antitumor immunity and inhibits colon carcinoma growth in mice. Integr Cancer Ther (2020) 19:1534735419900555. doi: 10.1177/1534735419900555
141. Yuan YV, Walsh NA. Antioxidant and antiproliferative activities of extracts from a variety of edible seaweeds. Food Chem Toxicol (2006) 44(7):1144–50. doi: 10.1016/j.fct.2006.02.002
142. Skibola CF, Curry JD, VandeVoort C, Conley A, Smith MT. Brown kelp modulates endocrine hormones in female sprague-dawley rats and in human luteinized granulosa cells. J Nutr (2005) 135(2):296–300. doi: 10.1093/jn/135.2.296
143. Rosen JE, Gardiner P, Saper RB, Pearce EN, Hammer K, Gupta-Lawrence RL, et al. Kelp use in patients with thyroid cancer. Endocrine (2014) 46(1):123–30. doi: 10.1007/s12020-013-0048-2
144. Al-Batshan HA, Al-Mufarrej SI, Al-Homaidan AA, Qureshi MA. Enhancement of chicken macrophage phagocytic function and nitrite production by dietary spirulina platensis. Immunopharmacol Immunotoxicol (2001) 23(2):281–9. doi: 10.1081/iph-100103866
145. Nelson SM, Gao YT, Nogueira LM, Shen MC, Wang B, Rashid A, et al. Diet and biliary tract cancer risk in shanghai, china. PloS One (2017) 12(3):e0173935. doi: 10.1371/journal.pone.0173935
146. Ohno Y, Yoshida O, Oishi K, Okada K, Yamabe H, Schroeder FH. Dietary beta-carotene and cancer of the prostate: a case-control study in kyoto, japan. Cancer Res (1988) 48(5):1331–6.
147. Gupta D, Silva M, Radziun K, Martinez DC, Hill CJ, Marshall J, et al. Fucoidan inhibition of osteosarcoma cells is species and molecular weight dependent. Mar Drugs (2020) 18(2). doi: 10.3390/md18020104
148. Bae H, Lee JY, Yang C, Song G, Lim W. Fucoidan derived from fucus vesiculosus inhibits the development of human ovarian cancer via the disturbance of calcium homeostasis, endoplasmic reticulum stress, and angiogenesis. Mar Drugs (2020) 18(1). doi: 10.3390/md18010045
149. Zhang J, Riby JE, Conde L, Grizzle WE, Cui X, Skibola CF. A fucus vesiculosus extract inhibits estrogen receptor activation and induces cell death in female cancer cell lines. BMC Complement Altern Med (2016) 16:151. doi: 10.1186/s12906-016-1129-6
150. Blaszczak W, Lach MS, Barczak W, Suchorska WM. Fucoidan exerts anticancer effects against head and neck squamous cell carcinoma in vitro. Molecules (2018) 23(12). doi: 10.3390/molecules23123302
151. Shen HY, Li LZ, Xue KC, Hu DD, Gao YJ. Antitumor activity of fucoidan in anaplastic thyroid cancer via apoptosis and anti-angiogenesis. Mol Med Rep 201715(5):2620–4. doi: 10.3892/mmr.2017.6338
152. Geisen U, Zenthoefer M, Peipp M, Kerber J, Plenge J, Manago A, et al. Molecular mechanisms by which a fucus vesiculosus extract mediates cell cycle inhibition and cell death in pancreatic cancer cells. Mar Drugs (2015) 13(7):4470–91. doi: 10.3390/md13074470
153. Zhang W, An EK, Park HB, Hwang J, Dhananjay Y, Kim SJ, et al. Ecklonia cava fucoidan has potential to stimulate natural killer cells in vivo. Int J Biol Macromol (2021) 185:111–21. doi: 10.1016/j.ijbiomac.2021.06.045
154. Park AY, Nafia I, Stringer DN, Karpiniec SS, Fitton JH. Fucoidan independently enhances activity in human immune cells and has a cytostatic effect on prostate cancer cells in the presence of nivolumab. Mar Drugs (2021) 20(1). doi: 10.3390/md20010012
155. Gewurz H, Mergenhagen SE, Nowotny A, Phillips JK. Interactions of the complement system with native and chemically modified endotoxins. J Bacteriol 196895(2):397–405. doi: 10.1128/jb.95.2.397-405.1968
156. Acker G. Ultrastructural study of lipopolysaccharide and of polymyxin b-induced changes of the outer membrane of serratia marcescens. Zentralbl Bakteriol Orig A (1977) 239(2):213–30.
157. Frank S, Specter S, Nowotny A, Friedman H. Immunocycte stimulation in vitro by nontoxic bacterial lipopolysaccharide derivatives. J Immunol (1977) 119(3):855–60.
158. Doe WF, Yang ST, Morrison DC, Betz SJ, Henson PM. Macrophage stimulation by bacterial lipopolysaccharides. II. evidence for differentiation signals delivered by lipid a and by a protein rich fraction of lipopolysaccharides. J Exp Med 148(2):557–68:1978. doi: 10.1084/jem.148.2.557
159. Braun-Fahrlander C, Riedler J, Herz U, Eder W, Waser M, Grize L, et al. Environmental exposure to endotoxin and its relation to asthma in school-age children. N Engl J Med (2002) 347(12):869–77. doi: 10.1056/NEJMoa020057
160. Ishikawa M, Tanno K, Sasaki M, Takayanagi Y, Sasaki K. Antidotal effect of lipopolysaccharide against acetaminophen-induced mortality in mice. Pharmacol Toxicol (1990) 67(5):387–91. doi: 10.1111/j.1600-0773.1990.tb00850.x
161. Heine H, Zamyatina A. Therapeutic targeting of TLR4 for inflammation, infection, and cancer: A perspective for disaccharide lipid a mimetics. Pharm (Basel) (2022) 16(1). doi: 10.3390/ph16010023
162. Romerio A, Peri F. Increasing the chemical variety of small-Molecule-Based TLR4 modulators: An overview. Front Immunol (2020) 11:1210. doi: 10.3389/fimmu.2020.01210
163. Ju H, Hu Z, Lu Y, Wu Y, Zhang L, Wei D, et al. TLR4 activation leads to anti- EGFR therapy resistance in head and neck squamous cell carcinoma. Am J Cancer Res (2020) 10(2):454–72.
164. Zhou S, Du R, Wang Z, Shen W, Gao R, Jiang S, et al. TLR4 increases the stemness and is highly expressed in relapsed human hepatocellular carcinoma. Cancer Med (2019) 8(5):2325–37. doi: 10.1002/cam4.2070
165. Che F, Yin J, Quan Y, Xie X, Heng X, Du Y, et al. TLR4 interaction with LPS in glioma CD133+ cancer stem cells induces cell proliferation, resistance to chemotherapy and evasion from cytotoxic t lymphocyte-induced cytolysis. Oncotarget (2017) 8(32):53495–507. doi: 10.18632/oncotarget.18586
166. Peyret V, Nazar M, Martin M, Quintar AA, Fernandez EA, Geysels RC, et al. Functional toll-like receptor 4 overexpression in papillary thyroid cancer by MAPK/ERK-induced ETS1 transcriptional activity. Mol Cancer Res (2018) 16(5):833–45. doi: 10.1158/1541-7786.MCR-17-0433
167. Zu Y, Ping W, Deng T, Zhang N, Fu X, Sun W. Lipopolysaccharide-induced toll-like receptor 4 signaling in esophageal squamous cell carcinoma promotes tumor proliferation and regulates inflammatory cytokines expressionDis esophagus. (2017) 30(2):1–8. doi: 10.1111/dote.12466
168. Tang S, Lian X, Cheng H, Guo J, Ni D, Huang C, et al. Bacterial lipopolysaccharide augmented malignant transformation and promoted the stemness in prostate cancer epithelial cells. J Inflamm Res (2021) 14:5849–62. doi: 10.2147/JIR.S332943
169. Lai FB, Liu WT, Jing YY, Yu GF, Han ZP, Yang X, et al. Lipopolysaccharide supports maintaining the stemness of CD133(+) hepatoma cells through activation of the NF-kappaB/HIF-1alpha pathway. Cancer Lett (2016) 378(2):131–41. doi: 10.1016/j.canlet.2016.05.014
170. Wang GC, Huang TR, Wang KY, Wu ZL, Xie JB, Zhang HL, et al. Inflammation induced by lipopolysaccharide advanced androgen receptor expression and epithelial-mesenchymal transition progress in prostatitis and prostate cancer. Transl Androl Urol (2021) 10(11):4275–87. doi: 10.21037/tau-21-964
171. Zhou B, Zhou H, Ling S, Guo D, Yan Y, Zhou F, et al. Activation of PAR2 or/and TLR4 promotes SW620 cell proliferation and migration via phosphorylation of ERK1/2. Oncol Rep (2011) 25(2):503–11. doi: 10.3892/or.2010.1077
172. Takazawa Y, Kiniwa Y, Ogawa E, Uchiyama A, Ashida A, Uhara H, et al. Toll-like receptor 4 signaling promotes the migration of human melanoma cells. Tohoku J Exp Med (2014) 234(1):57–65. doi: 10.1620/tjem.234.57
173. Rousseau MC, Hsu RY, Spicer JD, McDonald B, Chan CH, Perera RM, et al. Lipopolysaccharide-induced toll-like receptor 4 signaling enhances the migratory ability of human esophageal cancer cells in a selectin-dependent manner. Surgery (2013) 154(1):69–77. doi: 10.1016/j.surg.2013.03.006
174. Hu J, Shi B, Liu X, Jiang M, Yuan C, Jiang B, et al. Activation of toll-like receptor 4 reverses tumor differentiation in human glioma U251 cells via notch pathway. Int Immunopharmacol (2018) 64:33–41. doi: 10.1016/j.intimp.2018.08.019
175. Garay RP, Viens P, Bauer J, Normier G, Bardou M, Jeannin JF, et al. Cancer relapse under chemotherapy: why TLR2/4 receptor agonists can help. Eur J Pharmacol (2007) 563(1-3):1–17. doi: 10.1016/j.ejphar.2007.02.018
176. Pan C, Wu Q, Wang S, Mei Z, Zhang L, Gao X, et al. Combination with toll-like receptor 4 (TLR4) agonist reverses GITR agonism mediated M2 polarization of macrophage in hepatocellular carcinoma. Oncoimmunology (2022) 11(1):2073010. doi: 10.1080/2162402X.2022.2073010
177. Liu Z, Lei X, Li X, Cai JM, Gao F, Yang YY. Toll-like receptors and radiation protection. Eur Rev Med Pharmacol Sci (2018) 22(1):31–9. doi: 10.26355/eurrev_201801_14097
178. Jiang Z, Georgel P, Du X, Shamel L, Sovath S, Mudd S, et al. CD14 is required for MyD88-independent LPS signaling. Nat Immunol (2005) 6(6):565–70. doi: 10.1038/ni1207
179. Resman N, Vasl J, Oblak A, Pristovsek P, Gioannini TL, Weiss JP, et al. Essential roles of hydrophobic residues in both MD-2 and toll-like receptor 4 in activation by endotoxin. J Biol Chem (2009) 284(22):15052–60. doi: 10.1074/jbc.M901429200
180. Muller E, Christopoulos PF, Halder S, Lunde A, Beraki K, Speth M, et al. Toll-like receptor ligands and interferon-gamma synergize for induction of antitumor M1 macrophages. Front Immunol (2017) 8:1383. doi: 10.3389/fimmu.2017.01383
181. Calvano JE, Agnese DM, Um JY, Goshima M, Singhal R, Coyle SM, et al. Modulation of the lipopolysaccharide receptor complex (CD14, TLR4, MD-2) and toll- like receptor 2 in systemic inflammatory response syndrome-positive patients with and without infection: relationship to tolerance. Shock (2003) 20(5):415–9. doi: 10.1097/01.shk.0000092269.01859.44
182. Wang K, Xiong J, Lu Y, Wang L, Tian T. SENP1-KLF4 signalling regulates LPS-induced macrophage M1 polarization. J (2023) 290(1):209–24. doi: 10.1111/febs.16589
183. Evans L, Milward K, Attanoos R, Clayton A, Errington R, Tabi Z. Macrophage plasticity and function in the lung tumour microenvironment revealed in 3D heterotypic spheroid and explant models. Biomedicines (2021) 9(3). doi: 10.3390/biomedicines9030302
184. Chauhan V, Kanwar SS. Lipopeptide(s) associated with human microbiome as potent cancer drug. Semin Cancer Biol (2021) 70:128–33. doi: 10.1016/j.semcancer.2020.06.012
185. Ghochikyan A, Pichugin A, Bagaev A, Davtyan A, Hovakimyan A, Tukhvatulin A, et al. Targeting TLR-4 with a novel pharmaceutical grade plant derived agonist, Immunomax(R), as a therapeutic strategy for metastatic breast cancer. J Transl Med (2014) 12:322. doi: 10.1186/s12967-014-0322-y
186. Koido S, Homma S, Okamoto M, Namiki Y, Takakura K, Takahara A, et al. Combined TLR2/4-activated dendritic/tumor cell fusions induce augmented cytotoxic t lymphocytes. PloS One (2013) 8(3):e59280. doi: 10.1371/journal.pone.0059280
187. Lin YS, Huang LD, Lin CH, Huang PH, Chen YJ, Wong FH, et al. In vitro and in vivo anticancer activity of a synthetic glycolipid as toll-like receptor 4 (TLR4) activator. J Biol Chem (2011) 286(51):43782–92. doi: 10.1074/jbc.M111.285171
188. Akazawa T, Masuda H, Saeki Y, Matsumoto M, Takeda K, Tsujimura K, et al. Adjuvant-mediated tumor regression and tumor-specific cytotoxic response are impaired in MyD88-deficient mice. Cancer Res (2004) 64(2):757–64. doi: 10.1158/0008-5472.can-03-1518
189. de Queiroz N, Marinho FV, de Araujo A, Fahel JS, Oliveira SC. MyD88- dependent BCG immunotherapy reduces tumor and regulates tumor microenvironment in bladder cancer murine model. Sci Rep (2021) 11(1):15648. doi: 10.1038/s41598-021-95157-6
190. Ahmed A, Wang JH, Redmond HP. Silencing of TLR4 increases tumor progression and lung metastasis in a murine model of breast cancer. Ann Surg Oncol (2013) 20 Suppl 3:S389–96. doi: 10.1245/s10434-012-2595-9
191. Yin W, Li Y, Song Y, Zhang J, Wu C, Chen Y, et al. CCRL2 promotes antitumor t- cell immunity via amplifying TLR4-mediated immunostimulatory macrophage activation. Proc Natl Acad Sci USA (2021) 118(16). doi: 10.1073/pnas.2024171118
192. Zhang Y, Zhang Z, Chen L, Zhang X. Tumor cells-derived conditioned medium induced pro-tumoral phenotypes in macrophages through calcium-nuclear factor kappaB interaction. BMC Cancer (2022) 22(1):1327. doi: 10.1186/s12885-022-10431-8
193. Cameron DJ. Inhibitory factors derived from human tumors: isolation of factors which suppress macrophage mediated cytotoxicity. Int J Immunopharmacol (1983) 5(4):345–52. doi: 10.1016/0192-0561(83)90038-3
194. Mytar B, Woloszyn M, Szatanek R, Baj-Krzyworzeka M, Siedlar M, Ruggiero I, et al. Tumor cell-induced deactivation of human monocytes. J Leukoc Biol (2003) 74(6):1094–101. doi: 10.1189/jlb.0403140
195. Oh SK, Ross S, Walker J, Zeisel S. Role of a SER immune suppressor in immune surveillance. Immunology (1988) 64(1):73–9.
196. Guenther M, Gil L, Surendran SA, Palm MA, Heinemann V, von Bergwelt- Baildon M, et al. Bacterial lipopolysaccharide as a negative predictor of adjuvant gemcitabine efficacy in pancreatic cancer. JNCI Cancer Spectr (2022) 6(3). doi: 10.1093/jncics/pkac039
197. Jeong Y, Kim GB, Ji Y, Kwak GJ, Nam GH, Hong Y, et al. Dendritic cell activation by an e. coli-derived monophosphoryl lipid a enhances the efficacy of PD-1 blockade. Cancer Lett (2020) 472:19–28. doi: 10.1016/j.canlet.2019.12.012
198. Farias A, Soto A, Puttur F, Goldin CJ, Sosa S, Gil C, et al. A TLR4 agonist improves immune checkpoint blockade treatment by increasing the ratio of effector to regulatory cells within the tumor microenvironment. Sci Rep (2021) 11(1):15406. doi: 10.1038/s41598-021-94837-7
199. Yin H, Pu N, Chen Q, Zhang J, Zhao G, Xu X, et al. Gut-derived lipopolysaccharide remodels tumoral microenvironment and synergizes with PD-L1 checkpoint blockade via TLR4/MyD88/AKT/NF-kappaB pathway in pancreatic cancer. Cell Death Dis (2021) 12(11):1033. doi: 10.1038/s41419-021-04293-4
200. Wang S, Astsaturov IA, Bingham CA, McCarthy KM, von Mehren M, Xu W, et al. Effective antibody therapy induces host-protective antitumor immunity that is augmented by TLR4 agonist treatment. Cancer Immunol Immunother (2012) 61(1):49–61. doi: 10.1007/s00262-011-1090-7
201. Wang YL, Peng HH, Su SY, Lin CT. Combined immunotherapy (OK-432, IL-2) with chemotherapy decrease the recurrence rate in advanced ovarian cancer. Reprod Sci (2019) 26(2):244–9. doi: 10.1177/1933719118768684
202. Yang D, Luo X, Lian Q, Gao L, Wang C, Qi X, et al. Fully synthetic tn-based three-component cancer vaccine using covalently linked TLR4 ligand MPLA and iNKT cell agonist KRN-7000 as built-in adjuvant effectively protects mice from tumor development. Acta Pharm Sin B (2022) 12(12):4432–45. doi: 10.1016/j.apsb.2022.05.028
203. Dariushnejad H, Ghorbanzadeh V, Akbari S, Hashemzadeh P. Design of a novel recombinant multi-epitope vaccine against triple-negative breast cancer. Iran BioMed J (2022) 26(2):160–74. doi: 10.52547/ibj.26.2.160
204. Oba MS, Teramukai S, Ohashi Y, Ogawa K, Maehara Y, Sakamoto J. The efficacy of adjuvant immunochemotherapy with OK-432 after curative resection of gastric cancer: an individual patient data meta-analysis of randomized controlled trials. Gastric Cancer (2016) 19(2):616–24. doi: 10.1007/s10120-015-0489-9
205. Heine H, Adanitsch F, Peternelj TT, Haegman M, Kasper C, Ittig S, et al. Tailored modulation of cellular pro-inflammatory responses with disaccharide lipid a mimetics. Front Immunol (2021) 12:631797. doi: 10.3389/fimmu.2021.631797
206. Nelson MH, Bowers JS, Bailey SR, Diven MA, Fugle CW, Kaiser AD, et al. Toll-like receptor agonist therapy can profoundly augment the antitumor activity of adoptively transferred CD8(+) t cells without host preconditioning. J Immunother Cancer (2016) 4:6. doi: 10.1186/s40425-016-0110-8
207. Park HJ, Jang GY, Kim YS, Park JH, Lee SE, Vo MC, et al. A novel TLR4 binding protein, 40S ribosomal protein S3, has potential utility as an adjuvant in a dendritic cell-based vaccine. J Immunother Cancer (2019) 7(1):60. doi: 10.1186/s40425-019-0539-7
208. Grover K, Gregory S, Gibbs JF, Emenaker NJ. A discussion of the gut microbiome's development, determinants, and dysbiosis in cancers of the esophagus and stomach. J Gastrointest Oncol (2021) 12(Suppl 2):S290–300. doi: 10.21037/jgo-2019-gi-07
209. Sanidad KZ, Wang G, Panigrahy A, Zhang G. Triclosan and triclocarban as potential risk factors of colitis and colon cancer: Roles of gut microbiota involved. Sci Total Environ (2022) 842:156776. doi: 10.1016/j.scitotenv.2022.156776
210. Siddiqui R, Mungroo MR, Alharbi AM, Alfahemi H, Khan NA. The use of gut microbial modulation strategies as interventional strategies for ageing. Microorganisms (2022) 10(9). doi: 10.3390/microorganisms10091869
211. Sampsell K, Wang W, Ohland C, Mager LF, Pett N, Lowry DE, et al. Exercise and prebiotic fiber provide gut microbiota-driven benefit in a survivor to germ-free mouse translational model of breast cancer. Cancers (Basel) (2022) 14(11). doi: 10.3390/cancers14112722
212. Zhang Z, Feng H, Qiu Y, Xu Z, Xie Q, Ding W, et al. Dysbiosis of gastric mucosal fungal microbiota in the gastric cancer microenvironment. J Immunol Res (2022) 2022:6011632. doi: 10.1155/2022/6011632
213. Zhao H, Li D, Liu J, Zhou X, Han J, Wang L, et al. Bifidobacterium breve predicts the efficacy of anti-PD-1 immunotherapy combined with chemotherapy in chinese NSCLC patients. Cancer Med (2022). doi: 10.1002/cam4.5312
214. Sarkar P, Malik S, Banerjee A, Datta C, Pal DK, Ghosh A, et al. Differential microbial signature associated with benign prostatic hyperplasia and prostate cancer. Front Cell Infect Microbiol (2022) 12:894777. doi: 10.3389/fcimb.2022.894777
215. Rokos T, Holubekova V, Kolkova Z, Hornakova A, Pribulova T, Kozubik E, et al. Is the physiological composition of the vaginal microbiome altered in high-risk HPV infection of the uterine cervix? Viruses (2022) 14(10). doi: 10.3390/v14102130
216. Richardson BN, Lin J, Buchwald ZS, Bai J. Skin microbiome and treatment- related skin toxicities in patients with cancer: A mini-review. Front Oncol (2022) 12:924849. doi: 10.3389/fonc.2022.924849
217. Li X, Zhu S, Zhang T, Chen X. Association between oral microflora and gastrointestinal tumors (Review). Oncol Rep (2021) 46(2). doi: 10.3892/or.2021.8111
218. Rojas-Tapias DF, Brown EM, Temple ER, Onyekaba MA, Mohamed AMT, Duncan K, et al. Inflammation-associated nitrate facilitates ectopic colonization of oral bacterium veillonella parvula in the intestine. Nat Microbiol (2022) 7(10):1673–85. doi: 10.1038/s41564-022-01224-7
219. Pfisterer N, Lingens C, Heuer C, Dang L, Neesse A, Ammer-Herrmenau C. The microbiome in PDAC-vantage point for future therapies? Cancers (Basel) (2022) 14(23). doi: 10.3390/cancers14235974
220. Silveira MAD, Bilodeau S, Greten TF, Wang XW, Trinchieri G. The gut-liver axis: host microbiota interactions shape hepatocarcinogenesis. Trends Cancer (2022) 8(7):583–97. doi: 10.1016/j.trecan.2022.02.009
221. Vitorino M, Alpuim Costa D, Vicente R, Caleca T, Santos C. Local breast microbiota: A "New" player on the block. Cancers (Basel) (2022) 14(15). doi: 10.3390/cancers14153811
222. Vitorino M, Costa Alpuim D, Vicente R, Caleca T, Santos C. Local breast microbiota: a "New" player on the block. Cancers (Basel) (2022) 14(15). doi: 10.3390/cancers14153811
223. Segal BH, Giridharan T, Suzuki S, Khan ANH, Zsiros E, Emmons TR, et al. Neutrophil interactions with T cells, platelets, endothelial cells, and of course tumor cells. Immunol Rev (2022). doi: 10.1111/imr.13178
224. Sell S. Cancer immunotherapy: breakthrough or "deja vu, all over again"? Tumour Biol (2017) 39(6):1010428317707764. doi: 10.1177/1010428317707764
225. Puljic R, Benediktus E, Plater-Zyberk C, Baeuerle PA, Szelenyi S, Brune K, et al. Lipopolysaccharide-induced lung inflammation is inhibited by neutralization of GM-CSF. Eur J Pharmacol (2007) 557(2-3):230–5. doi: 10.1016/j.ejphar.2006.11.023
226. Kasahara DI, Poynter ME, Othman Z, Hemenway D, van der Vliet A. Acrolein inhalation suppresses lipopolysaccharide-induced inflammatory cytokine production but does not affect acute airways neutrophilia. J Immunol (2008) 181(1):736–45. doi: 10.4049/jimmunol.181.1.736
227. Amano S, Inagawa H, Nakata Y, Ohmori M, Kohchi C, Soma G. Oral administration of lipopolysaccharide of acetic acid bacteria protects pollen allergy in a murine model. Anticancer Res (2015) 35(8):4509–14.
228. Nakai K, Kubota Y, Soma GI, Kohchi C. The effect of lipopolysaccharide-containing moisturizing cream on skin care in patients with mild atopic dermatitis. In Vivo (2019) 33(1):109–14. doi: 10.21873/invivo.11446
229. Nakata K, Nakata Y, Inagawa H, Nakamoto T, Yoshimura H, Soma G. Pantoea agglomerans lipopolysaccharide maintains bone density in premenopausal women: a randomized, double-blind, placebo-controlled trial. Food Sci Nutr (2014) 2(6):638–46. doi: 10.1002/fsn3.145
230. Javmen A, Nemeikaite-Ceniene A, Bratchikov M, Grigiskis S, Grigas F, Jonauskiene I, et al. Beta-glucan from saccharomyces cerevisiae induces IFN-gamma production in vivo in BALB/c mice. In Vivo (2015) 29(3):359–63.
231. Ryan MT, Collins CB, O'Doherty JV, Sweeney T. Effects of dietary beta-glucans supplementation on cytokine expression in porcine liver. J Anim Sci (2012) 90 Suppl 4:40–2. doi: 10.2527/jas.53763
232. Kronborg G. Lipopolysaccharide (LPS), LPS-immune complexes and cytokines as inducers of pulmonary inflammation in patients with cystic fibrosis and chronic pseudomonas aeruginosa lung infection. APMIS Suppl (1995) 50:1–30.
233. Li Y, Zhao J, Shao H, Jia W, Su D, Liu T. Preventive effect of total flavonoids of trollius altaicus on a chronic obstructive pulmonary disease rat model based on the TLR4/NF-kappaB pathway. Ann Transl Med (2022) 10(4):222. doi: 10.21037/atm-22-331
234. Melkamu T, Qian X, Upadhyaya P, O'Sullivan MG, Kassie F. Lipopolysaccharide enhances mouse lung tumorigenesis: a model for inflammation-driven lung cancer. Vet Pathol (2013) 50(5):895–902. doi: 10.1177/0300985813476061
235. Li S, Xu X, Jiang M, Bi Y, Xu J, Han M. Lipopolysaccharide induces inflammation and facilitates lung metastasis in a breast cancer model via the prostaglandin E2-EP2 pathway. Mol Med Rep (2015) 11(6):4454–62. doi: 10.3892/mmr.2015.3258
236. Coutinho-Wolino KS, Almeida PP, Mafra D, Stockler-Pinto MB. Bioactive compounds modulating toll-like 4 receptor (TLR4)-mediated inflammation: pathways involved and future perspectives. Nutr Res (2022) 107:96–116. doi: 10.1016/j.nutres.2022.09.001
Keywords: edible microbiome, bugs as drugs, herbal microbiome, immune boosting, edible vaccine, immunotherapies, cancer, plant microbiome
Citation: Mazzio E, Barnes A, Badisa R, Council S and Soliman KFA (2023) Plants against cancer: the immune-boosting herbal microbiome: not of the plant, but in the plant. Basic concepts, introduction, and future resource for vaccine adjuvant discovery. Front. Oncol. 13:1180084. doi: 10.3389/fonc.2023.1180084
Received: 05 March 2023; Accepted: 30 May 2023;
Published: 31 July 2023.
Edited by:
Ming Kuang, The First Affiliated Hospital of Sun Yat-sen University, ChinaReviewed by:
Pratibha Pandey, Noida Institute of Engineering and Technology (NIET), IndiaDeepika Sharma, Institute of Nano Science and Technology (INST), India
Copyright © 2023 Mazzio, Barnes, Badisa, Council and Soliman. This is an open-access article distributed under the terms of the Creative Commons Attribution License (CC BY). The use, distribution or reproduction in other forums is permitted, provided the original author(s) and the copyright owner(s) are credited and that the original publication in this journal is cited, in accordance with accepted academic practice. No use, distribution or reproduction is permitted which does not comply with these terms.
*Correspondence: Karam F. A. Soliman, a2FyYW0uc29saW1hbkBmYW11LmVkdQ==