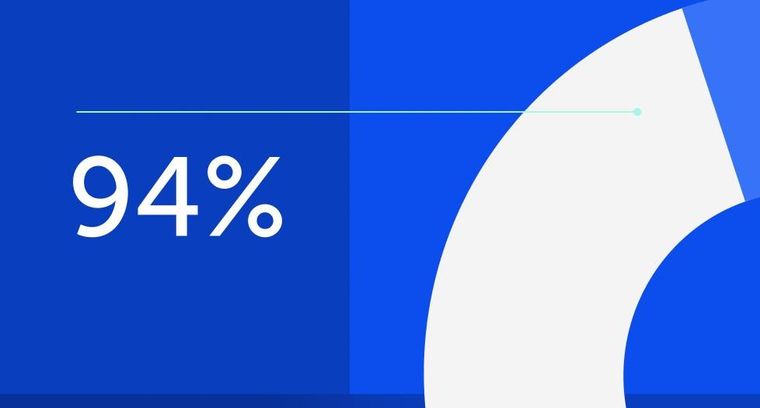
94% of researchers rate our articles as excellent or good
Learn more about the work of our research integrity team to safeguard the quality of each article we publish.
Find out more
REVIEW article
Front. Oncol., 06 July 2023
Sec. Pharmacology of Anti-Cancer Drugs
Volume 13 - 2023 | https://doi.org/10.3389/fonc.2023.1168454
This article is part of the Research TopicAnti-Cancer Drug Delivery: Lipid–Based NanoparticlesView all 6 articles
Tumors of the Central nervous System (CNS) are a spectrum of neoplasms that range from benign lesions to highly malignant and aggressive lesions. Despite aggressive multimodal treatment approaches, the morbidity and mortality are high with dismal survival outcomes in these malignant tumors. Moreover, the non-specificity of conventional treatments substantiates the rationale for precise therapeutic strategies that selectively target infiltrating tumor cells within the brain, and minimize systemic and collateral damage. With the recent advancement of nanoplatforms for biomaterials applications, lipid-based nanoparticulate systems present an attractive and breakthrough impact on CNS tumor management. Lipid nanoparticles centered immunotherapeutic agents treating malignant CNS tumors could convene the clear need for precise treatment strategies. Immunotherapeutic agents can selectively induce specific immune responses by active or innate immune responses at the local site within the brain. In this review, we discuss the therapeutic applications of lipid-based nanoplatforms for CNS tumors with an emphasis on revolutionary approaches in brain targeting, imaging, and drug and gene delivery with immunotherapy. Lipid-based nanoparticle platforms represent one of the most promising colloidal carriers for chemotherapeutic, and immunotherapeutic drugs. Their current application in oncology especially in brain tumors has brought about a paradigm shift in cancer treatment by improving the antitumor activity of several agents that could be used to selectively target brain tumors. Subsequently, the lab-to-clinic transformation and challenges towards translational feasibility of lipid-based nanoplatforms for drug and gene/immunotherapy delivery in the context of CNS tumor management is addressed.
Despite significant improvements in our understanding of the molecular basis of cancer, understanding the natural history of brain tumors is becoming a difficult task Intracranial neoplasms are a group of complex and heterogeneous lesions across the spectrum of benign and malignant tumors having varied treatments and clinical outcomes. The annual mortality rate attributing all malignant and non-malignant brain tumors and other CNS tumors account for about 23.79 per 100,000 as reported by the Central Brain Tumor Registry of the United States (CBTRUS) (1). The recent WHO classification of gliomas has incorporated immunohistochemistry in addition to tumor histology for characterizing gliomas. The present definition classifies diffusely infiltrative astrocytic tumors as being grade II (diffuse astrocytoma), grade III (anaplastic astrocytoma), or grade IV (tumors with microvascular proliferation and/or necrosis in addition to cytological atypia).These tumor have further been classified based on their IDH mutation status (2).
The identifying of regions with the distinctive histology for a particular tumor type is necessary for the categorization of central nervous system tumors since they frequently exhibit a wide range of morphological characteristics. Of all the brain tumors, glial tumors are the most commonly occurring and are categorized into astrocytoma, ependymomas, and oligodendrogliomas (3). Glioblastoma, also termed as Glioblastoma Multiforme (GBM), a Grade IV astrocytoma, constitutes 14% of intracranial tumor and 60% of astrocytic tumors. These tumors arise from glial cells within the central nervous system. Depending on the location of the brain tumor, the growth of tumors manifests as either localized or widespread symptoms, such as irregular headaches accompanied by aphasia and seizures (4). Oligodendrogliomas are another important adult tumor mainly originate from oligodendrocytes and are classified as grade II malignant tumor (5). Ependymomas are uncommon tumors of neuroectodermal origin that develop from ependymal cells in the spinal cord filum terminal, choroid plexus, or white matter of the brain (6). WHO has classified ependymoma grade- I tumors (myxopapillary ependymoma and sub-ependymoma) which are benign, slowly growing lesions. Grade III tumor is anaplastic ependymoma characterized by frequent mitosis, hypercellularity, and endothelial proliferation (7). Today, pediatric brain tumors are the leading cause of cancer-related death in children. One of the most common pediatric brain tumors is medulloblastoma, a primitive neuroectodermal tumor accounting for 20% of brain tumors in children (8). The biologically diverse group of medulloblastoma includes the following four molecular subtypes, namely WNT, Group 3, Group 4, and Sonic Hedgehog (9). The recent understanding of the molecular subgroups of medulloblastoma has resulted in shifting paradigms in not only the therapeutic strategy but also the prognosis of the disease.
The CNS and blood capillaries are connected by an extremely active and selective blood-brain barrier (BBB). It is the biggest barrier to getting drugs into the central nervous system through the blood circulation system. The BBB is a collection of highly specialized cells such as endothelial cells devoid of fenestrations, tight junctions, capillary basement membrane, astrocyte end-feet and pericytes that shields the brain from toxic substances in the blood and provides nutrients for function and helps in maintaining the brain’s homeostasis (10). Thus, even while the BBB is a natural defense mechanism, it also presents a considerable barrier to the systemic transport of many therapeutic medicines to the CNS. Similar to the blood-brain barrier, the blood-tumor barrier (BTB) is found between the microvessels and tumor cells in the brain. BBTB facilitates glioma cell migration to different regions of the brain and helps give nutrition and oxygen to the tumor. Utilizing this leaky BBTB linked to human brain tumors, anticancer drug delivery through nanocarriers may be accomplished by passively diffusing nanoparticles over free pharmaceuticals (11).
Contemporary therapeutics in brain tumors include surgery, radiation, and chemotherapy, each treatment has its own benefits and drawbacks. Combination therapies are effective in maximizing both response and safety, and their regimen is carefully selected based on the patient’s age, health, and life expectancy as well as the rate of tumor progression, and stage of the tumor. Surgery is frequently combined with other therapies including chemotherapy and radiation for high-grade gliomas.
For brain tumors, there have been improvements in the delivery of local therapies. This includes the latest advancements in local therapeutic drug delivery, direct injection, convection-enhanced delivery (CED), and implanting drug-impregnated polymers. These techniques could enhance future therapeutic approaches, such as the implantation of microchips with drugs and local gene therapy (12, 13). Despite the drug being present in a biodegradable polymeric, interstitial diffusion of CW is constrained. The narrow volume of distribution inside the tumor and the peripheral brain tissue, as well as the high and diverse drug concentrations, have resulted in varied short-term therapeutic benefits and toxicity issues that prevent CW from being widely used in therapeutic settings (14).
There is strong evidence to suggest that the “CNS is immune privilege” through the presence of immune cells (15–18). We summarized the key factors/determinants involved in the stimulation/inhibition of CNS immune responses during glioma pathogenesis (Table 1). These immune responses are produced via different mechanisms such as antigen in the brain, antigen presentation and APCs/microglia, T-cell trafficking, antibody penetration, and immunosuppression (immunosuppressive cytokines e.g. transforming growth-ß [TGF-ß], vascular growth factors [VGFs]) etc. Advance research on immune responses within the CNS have demonstrated that drainage of antigens in cervical lymph nodes through non-classical lymphatic pathways with cranial nerves cause induction of various immunological responses (cellular, humoral, innate) thus, resulting in trafficking of activated T-lymphocytes to the brain despite the presence of BBB (39–43). Moreover, antigens gain access through the convective flow of CSF and olfactory nerves across the cribriform plate into the systemic circulation and cervical lymph pathways. Subsequently, these antigens encounter B-lymphocytes and professional APCs in cervical lymph nodes and introduce them to circulating naïve T-cells, and lead to the activation of immune effector mechanisms. Thus, the activated lymphocytes via CNS get recruited at the site of inflammation although the native lymphocytes are unable to cross the BBB.
During normal homeostasis inflammatory immune cells that cross the Virchow-Robin space are constrained in the perivascular space without surpassing the glia limitans (44). However, the integrity of the BBB is compromised during inflammation/diseased conditions, thereby permitting immune cell infiltration into the brain parenchyma (45). The complex interaction between resident immune cells like microglia, recruited macrophages, and lymphocytes from the periphery are key players in immunological responses in the CNS (46). in-vitro and in-vivo studies have shown the role of resident microglia on glioma progression via major histocompatibility complex class-I (MHC-I) and recruitment of CD8+ T-cells (47). Additional studies corroborating prior findings on the role of stimulated microglia as intrinsic immunotherapy candidates through efficient cross-priming of intratumorally injected exogenous antigen (OVA ovalbumin) to naïve CD8+ T-cells have been published (48).
The CNS and blood capillaries are connected by the highly active and selective BBB. It acts as a barrier to drug delivery through the blood circulation system into the CNS. Most of the brain-targeting nanoparticles are given intravenously, which causes some of the drugs to reach other organs and cause toxicity (49). In contrast to systemic administration, local delivery provides several benefits, including avoiding the BBB and improving the therapeutic agent’s local bioavailability without producing systemic toxicity (50). A large spectrum of nano-platforms, including liposomes, polymeric NPs, micelles, protein nanocages, and inorganic NPs, are known for efficient drug delivery to the brain tumor. Nanoparticles enhance drug solubility, extend blood circulation half-life, and regulate drug release when used as drug delivery systems. Even though the BBB is intact, nanoparticles can be decorated with protein receptors and carriers that can mediate the transport of particular ligands and their contents. Additionally, the BBB membrane has a strong affinity for positively charged substances due to its negative charge, which may also cause cells to internalize substances. Therefore, these ligands might act as a conduit for NPs to cross the BBB (51, 52).
Lipid-based or lipidic nanocarriers are widely used as drug delivery systems (DDS) since they have a variety of beneficial qualities, including high drug loading efficiency, low toxicity, biocompatibility, sustained release behavior, protection against drug degradation, stability, and suitability for drug delivery via various routes (53). Lipidic nanocarriers can be divided into several groups according to their physicochemical characteristics and technique of preparation: Liposomes are spherical vesicles with a lipid bilayer made of phospholipids. Niosomes are made of cholesterol and non-ionic surfactants. Transferosomes are similar to liposomes and are made of stabilized lipid matrix. Solid Lipid Nanoparticles have a solid lipid core. Nanostructure lipid carriers (NLCs) have a liquid lipid core encircled by a solid lipid core (54). Self-assembled spherical phospholipid bilayers known as liposomes have drawn a lot of interest as drug delivery vehicles for brain tumor therapy. Major benefits of liposomal drug delivery platforms include improved pharmacokinetic impact and selective accumulation in brain tumors by passive and active targeting. Clinical trials are being conducted on several liposome-based drug delivery systems. In 2011, the beginning of phase I/II clinical trial with 2B3-101 in patients with brain metastases from breast cancer or gliomas (NCT01818713). In this study, PEGylated Liposomal Doxorubicin formulation 2B3-101, coupled with glutathione and specialized transporters on BBB enhanced drug delivery to the brain.
Preclinical studies have shown that incorporating endogenous ligands or monoclonal antibodies onto the liposome surface is primarily attributed to the ability of these ligand to cross the BBB and subsequently bind to receptor overexpressed on GBM cells. Therefore, the improved targeting of GBM cells by these ligand-modified nanoparticles is due to their ability to permeate the BBB and reach the tumor site. A potential method for brain-targeted drug delivery is the active targeting of multifunctional liposomes. These liposomes can be used as targeted drug delivery systems for the treatment of brain tumors because of their simple and large-scale production capability, customizable structure, capacity to cross the BBB and preferred aggregation inside tumor tissue. In the subsequent part of the paper, we have discussed the applications of liposomes as a therapeutic moiety and a theragnostic in immunotherapy for CNS tumors.
Stimuli-responsive systems are showing promising results for site-specific delivery and release payloads. Many materials have been employed to make carrier systems, including lipids, polymers, and inorganic nanoparticles, all of which have been conferred stimuli-sensitive properties to achieve triggered release. The distinct characteristics of the tumor microenvironment may act as a site for an applied external stimulus (heat or light) or as an endogenous stimulus (pH, redox potential, or distinctive enzyme activity) to induce the regulated release of the drug as depicted in Figure 1 (55).
Figure 1 The stimuli-responsive multifunctional liposomes for drug delivery to CNS tumor cells for effective therapy by killing cancer cells by responding to external and internal stimuli.
Glioblastomas are responsible for local immunosuppression through different immune escape mechanisms for instance, increase expression of co-stimulatory signals (CD80, CD40, CD86), and upregulation of MHC-II molecules etc. These molecules are essential for effective interaction, and communication between glioma cells and the host’s immune cells. Their interactions lead to preclusion of recognition and elimination of tumor cells by T-lymphocytes resulting in local immunosuppression (56–58). Furthermore, glioma tumor microenvironment (TME) abundantly constitutes immunosuppressive players such as TGF-ß, IFN-γ, cytotoxic T-lymphocytes [CTLs]) (59–61), regulatory T-cells (Treg), and dysfunctional NK cells (overexpressed ~12 times more in tumor cells than in the normal cells) etc. Moreover, the recruitment and prolongs survival of these immunosuppressive players supported via activation of high concentration of cytokines and tryptophan catabolic enzyme like indolamine 2,3-dioxygenase-1 (IDO1) thereby aid to sustained the immunosuppression (62–65). The evolutionary investigation in the mechanistic of glioma-associated immunosuppression aids in precise understanding of CNS tumor immunology and designing novel technologies targeting local immunosuppression. For instance, glioma-associated MØ/MG collectively called as GAMs, occupy about 15-30% of tumor mass depending upon the clinical stage of the gliomas (66). The extravasation and increased number of GAMs into the glioma eventually resulted in disruption of the BBB functions, and co-related with histologically aggressive tumors respectively (67). We represented the glioma-associated immunosuppressive microenvironment and its triggers/determinant in the Figure 2.
Figure 2 Schematic representation showing key determinants/triggers responsible for immunosuppressive microenvironment within the CNS tumors.
Further GAMs possess a significant degree of plasticity (M1 and M2 phenotypes) depending upon the type of stimulus (68–70). Various studies based on cell-surface markers and gene profiling at transcription levels are under investigation to gain a greater understanding of the function and potential of GAMs targeted immunotherapies against CNS tumors (71–73). Additionally, glycolysis, hypoxia, nutrient deficiency, and high concentration of lactate impair the effector immune cell functions in the TME contributing to local immunosuppression and subsequently contributing to tumor progression, and invasion. migration, angiogenesis, and resistance to conventional treatment modalities (74).
Cancer immunotherapy is classified into active and passive immunotherapies (75, 76). Since past few decades, anti-cancer immunotherapies have experienced rapid clinical translation such as chimeric antigen receptor (CAR)-T cells therapy (use to enhance effective T-cells responses) targeting three major antigens i.e. human epidermal growth factor receptor-2 (HER2 or ERBB2), EGFRviii, and IL-12 receptor-α2 (IL-13Rα2) for brain tumors (77–79). However, as discussed earlier, the efficacy of cancer immunotherapy is limited by the factors by which cancer cells evade the host immune response via down-regulating surface antigen, and MHC-I expression by infiltrating inflammatory immune cells into the TME, tumor heterogeneity, and subsequently block the effector T-cells priming and activation (80). Current cancer immunotherapy based on activation/stimulation of key players of the immune system (e.g. cytokine therapy, adoptive T-cell transfer) and inhibition/elimination of immunosuppressive markers (e.g. checkpoint blockade [ICB] therapy). However, their clinical success found to be limited in the treatment of solid tumors, and brain cancers with immune response related adverse effects. To overcome these limitations existing immunotherapies with nanotechnology-based brain tumor targeting represent a captivating strategy (Figure 3). Recently, Chen and Cong in 2023 have provided a comprehensive review on the surface-modified nanoparticle platforms in anti-tumor immune response and immunotherapy (81). Furthermore, combination (e.g. chemotherapy, radiation) nano-theranostic and immunotherapy (e.g. macrophage polarization, dendritic cell targeting, NK cell therapies) have been more successful in clinical studies (82–84).
Figure 3 Different type of immunotherapy applications in treating CNS tumors using lipid-based nanocarriers.
Together with the development of smart nano-biomaterials and a series of promising clinical/preclinical studies (e.g. Pegylated liposomal doxorubicin in high-grade gliomas), a significant number of lipid-based nano-drug delivery systems have recently been appeared in anti-glioma clinical trials (85). In the following subsections, we described the recent development and translational barriers to lipid-based nanoplatforms in immunotherapy against CNS tumors.
Various immunogenic factors during apoptosis of tumor cell have been identified to stimulate optimal antigen presentation to the effector T-cells such as damage-associated molecular patterns (DAMPs), calreticulin (CRT, a cell surface pre-apoptotic marker), ATP, and heat shock protein (HSP), and high mobility group box protein-1 (HMGB-1, a post-apoptotic protein), etc. (86). Several research groups have studied the pre-clinical potential of various lipid-based nanoplatforms (liposomes, nanodiscs, lipid-hybrid nanoparticles) for a combination of chemotherapy and immunotherapy against CNS tumors (87). For instance, Kadiyala et al. in 2019 demonstrated the immune-mediated anti-glioma potential of synthetic high-density lipoprotein mimicking nanodiscs (composed of 1,2-dipalmitoyl-sn-glycerol-3-phosphocholine [DPPC], 1,2-dimyristoyl-sn-glycerol-3-phosphocholine [DMPC] and egg sphingomyelin [SM]) loaded with docetaxel (DTX) and oligodeoxynucleotide (CpG a Toll-like receptor-9 agonist) as chemoimmunotherapy (DTX-sHDL-CpG) against glioblastoma. The combination of chemo-drug (DTX) with immunotherapy (CpG) exhibited an improved anti-glioma effect with no appreciable off-target toxicity. Authors further extended this application with adjuvant radiotherapy wherein the DTX-sHDL-CpG with radiotherapy significantly improved the survival benefits (~80% tumor regression) in GBM induced animals. Their findings confirm the role of immune system against tumor progression. Induction of potent cytotoxic CD8α+T cells lymphocytes (CTL) responses responsible for development of robust anti-tumor immunological memory against glioma recurrence (88). Consequently, in 2020 similar research group developed the sHDL nanodiscs containing a cocktail of immune checkpoint blocker anti-PD-L1 CpG, and cysteine-serine-serine (CSS) modified sequence (at N-terminus for conjugation to thiol-modified pyridyl disulfide modified phospholipids i.e. DOPE-PDP) neoantigen peptide as a personalized vaccination against syngeneic GL261 bearing mice model. They found that the nanodiscs platform in combination with a checkpoint blocker has the potential to induce neoantigen-specific CD8α+T cells infiltration into the glioma microenvironment. Collectively, their findings suggested the potential of lipid-based nanoplatform as personalized vaccination for immunotherapy against CNS tumors and other cancers (89). However, the clinical translation of the lipid-based nano platform for chemoimmunotherapy is still in its infancy because of many challenges such as scalability, safety and immunogenicity of the novel biomaterials, optimal ratio, synergistic index, dosing frequency/intervals of chemo-drug and immunotherapeutic to stimulate an immune response, etc. (29),. Thus, these factors need to be resolved for an efficient delivery of small molecules, drugs in CNS therapy using lipid nanoparticles.
Cancer vaccines are typically composed of antigens coupled with an adjuvant and induce de novo response against tumor-specific antigens (90). Local administration of vaccines leads to a cascade of innate inflammatory mediators via the release of DAMPs and chemokines gradient for chemotaxis of APCs at the site of inflammation (91, 92). As a result of the chemotaxis of APCs, antigens were pick-up before migrating to draining lymph nodes followed by their presentation and priming to an activated T-cell response against tumor-specific antigens (93). However, prophylactic and advanced stages of the cancers require multiple boosters over frequent intervals to years to confer adequate protection from immunosuppressive TME (94–96). Therefore, to target the tumor-specific antigens newer technologies need to be developed to harness the immune system in a personalized manner. Most prototype vaccines such as peptide, nucleic acids, mRNA, and DNA-based vaccines are associated with several limitations such as high immunogenicity, lack of stability, and unwanted degradation (97, 98). Alternatively, to prevent degradation and improve their stability before and after cell transfection various delivery vehicles have been developed (99). More recently, Mendez-Gomez and co-workers have developed a lipid nanoparticle multilayer mRNA backbone envelop (mRNA cancer vaccines) for gene transcript in pediatric high-grade glioma. They found localized uptake of RNA-NPs to myeloid cells in the KR158b glioma mouse model. Wherein the mRNA-modified lipid nanocarrier act by reprogramming the TME and inducing tumor-specific immunological response. Subsequently, this selective localization activates the DCs which supplement the regulatory myeloid cell population intratumorally to induce an antigen-recall memory with prolonged survival benefits. Additionally, this lipid nano-formulation received Food and Drug Administration-Investigational New Drug (FDA-IND) approval for a first clinical trial (IND number BB19304) in pediatric high-grade glioma patients (PNOC020 study NCT04573140) (100).
Lipid nanocarriers such as solid lipid nanoparticles and liposomes have been widely studied for the development of a sustained-release vehicle for therapeutic drug and gene delivery against gliomas (101). Various pre-clinical studies have provided a significant amount of toxicological data and offer a more straightforward path for the development of lipid nanocarriers for nucleic acid (e.g. RNA) delivery for further clinical translations (102). These RNA lipid nanoplatforms use the RES organs as more optimal locations for cancer vaccines to induce T-cells priming and APC transfection with antigen-presenting cells (e.g. liver Kupffer cells, splenic macrophages, dendritic cells) which phagocytize the RNA followed by activation of a T-cells against the desired epitope encoded by the mRNA (75). The RNA lipid nanocarriers were further modified with immunostimulatory cytokines encoded by targeted mRNA which are more promising and simple alternatives to dendritic cell-based cancer vaccines. Although lipid nanoplatforms for nucleic acid-based cancer vaccines mimic viremia through type-I interferon signature induction, they could not produce an anti-viral response against a viral antigen but redirect host immunity (innate and adaptive) against tumor mRNA antigenic molecules (103, 104).
Furthermore, the composition, size, and charge of lipid-based nanoparticles play an important role in eliciting immune responses. For instance, polar lipids are one of the major components of various lipid nanoplatforms and are significantly effective in mRNA transfection (105). The polar lipids composed of hydrophilic head groups attached to non-polar tails via linker bonds. The non-polar tail molecules from separate molecules joined to adjacent ones resulting in positively charged hydrophilic head groups that repelled each other and face along opposite sides. Thus, more liposomes join together and form a micelle-like structure having a positively charged outer surface followed by lipid layers either multilayers or single layers, and a positively charged inner core (106). Successively, RNA-loaded lipid nano-vehicles could also be formed by electrostatic interaction/complexation between positively charged lipid nanoparticles and negatively charged nucleic acid (e.g. RNA) (107, 108). An interesting proof-of-concept study of the intranasal DOTAP liposomes targeting the CFTR gene that overexpressed in lung parenchyma of cystic fibrosis (CF) patients demonstrated the safety in the first-in-human trial application (109). Subsequently, several research groups tried the DOTAP liposomes for the delivery of siRNA, and chemotherapeutic agents (paclitaxel and gemcitabine) for patients with advanced CNS tumors (110, 111). Sayour et al. in 2016 demonstrated the application of RNA-liposomal cancer vaccine encapsulated with personalized tumor-derived mRNA which represents tumor-specific transcriptome. This was achieved by isolation and extraction of total RNA (tRNA) from the brain tumor biopsied samples (n=500) to induce anti-tumor response against the murine adoptive cellular therapy for high-grade gliomas. The tRNA contains ribosomal and transfer RNA from which complementary DNA (cDNA) library was generated through RT-PCR on mRNA present from the initial tRNA. The cDNA was then transcribed in-vitro and amplified for the generation of multiple copies of mRNA mediate tumor-specific transfection of APCs. This result in antigen presentation onto the MHC-I/II (immunogenic tumor epitopes) via T-cell receptors (TCRs recognize most foreign and activated tumor epitopes and help the immune system to decide the best line-targets) thereby leading to activation of CD4 and CD8+ T-cells. Although, the study reported that tracking the antigen-specific immune response is more complicated when the immune reactive epitope is unknown (112).
Furthermore, Liu and co-workers in 2022 have developed the cholesterol and 1,2-dioleoyl-sn-glycerol-3-phosphoethanolamine (DOPE) lipid nanoparticles made up of cationic lipid with different ionizable amine headgroups (BAMPA-O16B, pKa 6.5) for endosomal escape of siRNA in GBM cell lines. Subsequently, they demonstrated that BAMPA-O16B/siRNA lipoplex is highly effective against the simultaneous blockade of two immune inhibitory markers involved in tumor-induced immunosuppression i.e. CD47 (a cancer biomarker overexpressed and prevents tumor cells phagocytosis through interaction with myeloid inhibitory receptor SIRPa) and PD-L1. This combination therapy leads to enhanced adaptive and innate anti-glioma immune responses in orthotopic glioma-bearing mice, due to synergistic activation of T-cell dependent downregulation of overexpressed target gene in the tumor (113). Grafals-Ruiz et al. in 2020 demonstrated the brain targeting peptides (apolipoprotein-E [ApoE] and rabies virus glycoprotein [RVG]) modified gold liposomes (30-50 nm) for siRNA delivery against dysregulated mRNA in GBM. Their study revealed the translational potential of miRNA based liposomal drug delivery for GBM and other CNS diseases (114).
Moreover, several lipid layers can be further modulated by layer-by-layer techniques to effectively traps the RNAs between lipid enveloped and prevent their degradation. However, precise control of the size of the RNA condensed lipid nanoparticles is necessary to avoid unintended biological activity such as transfection efficiency, cellular/intracellular uptake and retention, and toxicity profile (115). Thus, the lipid nano-platform for RNA delivery has great therapeutic potential however, stability and efficacy require more attention for clinical translation. Also, administration of lipid nanoparticles via the alternative route (e.g. intranasal, intrathecal, intraventricular) is highly desirable since these are effective strategies to circumvent the BBB, and can be explored further for future RNA-based nano-therapeutics against CNS tumors.
Cellular immunotherapy involves the administration of living cells (e.g. DCs) stimulating an anti-tumor response to the patients or adoptive transfer of cells (e.g. autologous or allogenic lymphocytes) having intrinsic anti-tumor potential (known as adoptive cell transfer [ACT]) to the patients. Although, the formidable barrier to these cellular therapies is presented by many solid malignancies via their immunosuppressive milieu resulting in impaired anti-tumor immunity (116, 117). Therefore, combination of different cellular therapies is under investigation to combat immunosuppressive TME (118, 119). However, high costs and a significant number of adverse events such as systemic autoimmune toxicity, and resistance to immunotherapy have been reported by many research groups (120–122). Subsequently, nanotechnology could help to solve this problem by using biocompatible, and inexpensive nano-carriers delivering rationally selected combinations of immunotherapeutic into the TME (123).
Recently, Shi and co-workers have demonstrated the T-cell targeting fusogenic liposomes coupled with 2,2,6,6-tetramethylpiperidine (TEMP) neutralizing reactive oxygen species. Fusogenic liposomes have potential in preventing T-cells from oxidative loss and allowing simultaneous MRI imaging. The T-cell’s functionality and proliferation depend on an optimal reducing microenvironment with redox balance between S-S and -SH on its surface membrane, however, the oxidative stress induced by tumor cells causes -S-S and -SH groups oxidation, and T-cells loss their functionality. Moreover, Fusogenic liposomes have a potential to improve the T-cells survival and proliferation, and may further provide opportunities for engineering of T-cells for cancer theranostic applications using lipid nanoarchitecture (124). Collectively, in the following sections we focus on these cellular lipid-based nano-immunotherapies in context of the CNS tumors.
In CAR-T therapy, a chimeric antigen receptor (CAR) is engineered T-cell with intracellular signaling domains for T-cells activation on antigen recognition when exposed to a particular tumor antigen of interest (125). Cancer-specific T-cells are produced via genetically modified T-cells using viral vector-based/non-viral nanoparticle-based systems. Widely used viral vectors (adenoviruses, retroviruses, and lentiviruses) for CNS tumors. Whereas non-viral vectors include various nano-platforms such as lipo/polyplexes, lipid/polymeric nanoparticles, etc. However, non-viral vectors have received greater attention due to their low immunogenicity, safety, efficacy, and ease of modification (126, 127). Recently Ferreras et al. reviewed the challenges and opportunities of CAR-T cell therapy in pediatric CNS tumors with special emphasis on circumventing these challenges through local and controlled delivery of tumor-specific effector immune cells using metal, polymeric, and lipid nanoplatform (128). However, very few studies have explored the role of lipid nanoparticles to enhance CAR-T cell therapy in brain tumors. In 2018, Zhang and their research group demonstrated the application of lipid nanoparticles (egg phosphatidylcholine, cholesterol, PEG (2000)-PE, and DSPE-PEG-maleimide) co-targeting (tumor-targeted peptide [iRGD] and PI3K inhibitor [CAL-101] to reduce the Treg cells and increased the number of effector T-cells) in reshaping the immunosuppressive tumor microenvironment for effective CAR-T cell therapy in a murine model of glioma. Their findings demonstrated that the preconditioning regimen used in the study with targeted nanoparticles transiently reshape the tumor immune microenvironment improving the success rate. Thus, this platform could be explored clinically to improve the CAR-T cell-based immunotherapy for the CNS tumors (129). Moreover, Billingsley and team have reported the orthogonal design of optimized lipid nanoparticle formulation via screening of sequential libraries of ionizable lipids for non-viral messenger (mRNA)-CAR-T cell therapy. The developed system has the advantage to improve mRNA delivery to T-cells with low cytotoxicity. Thus, suggesting the impact of formulation excipient optimization on lipid nanoparticles performance to improve the potency of treatment to deliver mRNA to primary human T-cells with comparable CAR expression by conventional electroporation (EP) method with low cytotoxicity (130).
Conclusively, there is an urgent need for increased translational strategies of these lipid-nanoplatform-based novel CAR-T cells therapy in the glioblastoma’s management. Several research groups have suggested that a judicious balance between the activation of CAR-T cells and cytokines secretions with acceptable toxicity, appropriate pre-clinical model recapitulating the host TME (syngeneic vs humanized mouse models), optimal characterizations (concentration and dosing frequency) of CAR-T cell therapy should be considered for accurate clinical translation to avoid tumor recurrence, toxicities, and resistance (131, 132). Subsequently, mathematical modelling and in-silico models could be useful tool to correlate the CAR-T cell therapy response and tumor regression. Additionally, the selection of T-cell subtypes having non-alloreactive phenotype like memory T-cells, broad therapeutic window of CAR-T cells therapy, and factors affecting it such as the density of tumor-specific antigens is necessary to be considered to enhance CAR-T cell efficacy (133, 134).
Natural killer (NK) cells are innate lymphoid cells located at the epithelial surfaces with selective cytotoxic potential leading to immunosurveillance upon exposure to pathogens or foreign substances (135). Furthermore, NK cells induce immunomodulatory functions by recruiting immune cells such as DCs, and T-cells and secreting cytokines (IFN-γ, TNF-α). However, NK cell therapy still faces considerable challenges in a clinical setting due to immunosuppressive TME that leads to decreased functionality, and poor infiltration and trafficking of NK cells into tumors (136). Various clinical studies have come up with positive outcomes from NK cells in combination with T-cells in pediatric brain tumors. The results from these clinical studies suggested that combinatorial therapies of NK cells with other cellular immunotherapies have the potential to enhance the effectiveness probably due to preventing tumor cells’ immune escape mechanisms (NCT02271711, NCT01804634, NCT02100891). Recently, Siegler et al. in 2017 demonstrated the CAR-T cells engineered NK92 cells as a combination therapy of paclitaxel (PTX) loaded cross-linked multilamellar liposomal vesicles (cMLVs). The liposomes composed of phospholipids i.e. 1,2-dioleoyl-sn-glycerol-3- phosphocholine (DOPC), 1,2-dioleoyl-sn-glycero-3-phospho-(10-rac-glycerol) (DOPG), and 1,2-dioleoyl-sn-glycerol-3-phosphoethanolamine-N-[4-(p-maleimidophenyl) butyramide (maleimide-head group lipid, MPB-PE) and have high specificity, selectivity, homing, and anti-tumor efficacy against human epidermal growth factor receptor-2 (HER2) and CD19 overexpressing solid tumor models (137).
Additionally, to track the NK-cells function as an anti-tumor candidate Yang et al. in 2020 reviewed the recent advances in non-invasive imaging methods like MRI, optical microscopy, and PET/SPECT wherein NK cells were tagged with fluorophores, radioisotopes/radiotracers, and paramagnetic nanoparticles against various cancer. The study addressed the major challenges to nanomaterial-based NK-cell therapy such as safety, and a detailed understanding of the mechanisms of NK cells in immunotherapy that needs further exploration (138). Moreover, due to the complexity of TME, the therapeutic potential of lipid nanoparticle-based NK cell therapy against CNS tumors is still under scrutiny to enhance the clinical effectiveness with acceptable off-target events. We anticipate that multifunctional lipid nanoparticles-NK cells technologies in combination with NK cell biology, and the complex interplay by TME could be targeted via promoting these engineered cells. Thus, the liposomal NK cells platform has a potential for treatment of other solid tumors including CNS tumors.
Glioma stem cells (GSCs) or tumor-initiating cells (TICs) have been reported to be markedly resistant to conventional chemo/radiotherapy. They are responsible for tumor cells infiltration/proliferation, invasion, metastasis, and increase risk of tumor relapse, and mortality. The GSCs-mediated treatment resistance via upregulation of various stem cell markers (e.g. CD133), and ABCB1 transporters (MDR1) etc. were reported in brain tumors (139). Subsequently, high DNA repair ability of GSCs is driven through various mechanisms. For instance, stem cell markers integrin-α6 (receptor for the ECM protein laminin), and nestin (intermediate linker filament protein expresses by oligodendrocytes, astrocytes precursor and differentiated cells during embryogenesis). These stem cells markers were co-expressed with other proteins such a Proliferating Cell Nuclear Antigen (PCNA, an auxiliary protein use by DNA polymerase-δ during replication process), caspase-3, and Vascular Cell Adhesion Molecule-1 (VCAM-1, immunoglobulin mediate cell-cell interactions, and identified as a lineage-specific marker of neural differentiation, progenitor cells, and radial glia in the ventricular zone). They play an important role in maintaining connections between ECM matrix proteins in GSCs to support their propagation, adhesion, and invasion in perivascular niches and confer resistance to DNA alkylating agents and radiotherapy (140–142). In the following section we discussed about several GSCs targeted nanotechnology-based liposomal anti-glioma therapies for GBM treatment
Various liposomal nanoparticles targeting GSCs either alone or in combination with external or internal triggers (e.g. hyperthermia, ultrasound, photodynamic/thermal therapy) have been extensively studied (143, 144). Furthermore, identification GSCs targeting specific stem cell-surface biomarkers (e.g. Aldehyde dehydrogenase [ALDHs], CD44, CD90, CD133), and unique signaling pathways (e.g. Wnt/b-catenin, Notch, TGF-β, Hedgehog) are under investigation to successfully eliminate GSCs to prevent tumor metastasis and recurrence (145). In vitro studies of CD44 targeted lipid nanoparticles modified with hyaluronic acid has been shown to improve therapeutic ratio against GBM. The hyaluronic acid acts as a biomimetic ligand to CD44, selectively targeting CD44 overexpressing glioma cells thereby improving anti-tumor efficacy of doxorubicin (146).
Studies have demonstrated GSCs transdifferentiating into endothelial cells and/or pericytes, and have been reported to promote vasculogenesis (the ability of tumor cells to form the embryonic circulatory system with new blood vessels using pre-existing vasculature) through upregulated VEGF (147, 148). Furthermore, involvement of multiple mechanisms/pathways were studied by many researchers in in-vivo and clinical samples using lineage-specific fluorescence reporter (149–151). For instance, recruitment of GSCs towards the SDF1-CXCR4 axis and subsequent activation by transforming growth factor-β (TGF-β, promoting cancer stemness and tumor metastasis), and hypoxia-inducible factor-1 (HIF-1α, a transcriptional regulator for hypoxia-induced gene expression important in maintaining cell cycle quiescence and promote angiogenesis etc. Their findings demonstrated the elimination of GSCs differentiated pericytes (having the same genetic alteration as glioma cells) helps in tumor regression. These studies advance our understanding of the plasticity of GSCs and may facilitate the designing of GSCs-targeted clinical nano-therapeutic strategies, and drug development to suppress glioma progression. In 2014 Li et al. reported the application of multifunctional liposomes co-loaded with paclitaxel and artemether in reducing the vasculogenic mimicry (VM) through induction of VM channels (composed of genotype-transformed tumor cells supplying nutrients to tumor cells and promoting their recurrence), and activation of apoptotic pathways for GSCs growth inhibition via downregulating expression of MMP-9, HIF-1α, and VEGF against invasive brain tumors (152). Moreover, studies have demonstrated that the overexpression and interaction of tumor biomarkers such as integrin αvβ3 with ECM through tripeptide Arg-Gly-Asp (RGD) exhibited crucial effects on tumor cell proliferation, and invasion. Subsequently, nano-carriers targeting tumor vasculature have been developed that bind to the receptors overamplified during tumor angiogenesis like integrin, VEGF, and VACM-1 etc (153). Consequently, cRGD peptides have been widely investigated as a ligand for integrin using targeted anti-glioma strategies. For instance, Sofias et al. developed liposomes decorated with cRGD and oil-in-water nano-emulsion for ligand-mediated accumulation via immune cell phagocyte hitchhiking in GBM induced mouse models. The authors have demonstrated the real-time nanoparticle targeting kinetics, and their specificity to circulatory, and tumor-homing immune cells (e.g. monocytes, macrophages, neutrophils, and lymphocytes) using PET/CT imaging integrated with flow cytometry and intravital microscopy (154).
However, a better understanding of GSCs and their properties could support the development of personalized anti-tumor nanodrug therapies to fulfil unmet clinical needs. For instance, Wang et al. in 2009 has demonstrated the siRNA-modified nano drug delivery platform downregulating expression of HIF1α in animal studies. Their findings revealed the potential of anti-HIF1α siRNA-based integrin targeted multifunctional nanocarrier knockdown of HIF-1α expression in human glioma xenograft mouse models. Furthermore, Sakurai et al. in 2014 reported the pH-sensitive cationic (YSK05) liposomal siRNA nanoparticle (MEND) modified with cRGD for tumor endothelial cells (TECs) gene silencing, and for VEGF as an anti-angiogenic therapy (155). Recently, dual ligands targeted delivery strategies have shown great potential in the delivery of therapeutic agents to tumor cells for CNS tumor treatment as a means of circumventing the BBB compared to a single ligand modification system (129, 156). Recently a combination of integrin αvβ3 and lactoferrin receptors (overexpressed in glioma cells and cerebral microvascular endothelial cells) targeted RGD-modified docetaxel (DTX) pegylated liposomes (RGD-Lf-LP-DTX, 140 nm size) for treatment of glioma have also been developed (157). These dual ligand modification plays an important role in increasing selective retention, and accumulation of the drug in orthotopic brain tumor model. The liposomes had shown anti-glioma effect with significantly improved survival of mice (32 days) compared to control (20 days), single ligand modified liposomes (28days), and unmodified drug-loaded liposomes (21.5 days) (157). Although, novel lipid nanoplatforms are reported to be effective against CNS tumors, interaction between nanoparticles and biological fluids can modulate the pharmacokinetics, brain uptake, and organ distribution thereby dampening of therapeutic effects (158, 159). Therefore, preclinical studies in orthotopic or patient-derived xenograft models (PDX) with robust clinical findings should be undertaken for translation of novel lipid nanoplatform systems against the CNS tumors (160).
The primary treatment method for CNS tumors continues to be conventional surgery together with radiotherapy and concurrent chemotherapy, however, it offers little promise and runs the risk of tumor recurrence. Finding NP-based drug delivery systems that deliver medications directly to the cancer location is urgently needed, given the other numerous drawbacks of this therapeutic strategy. Recent advances in the molecular characterization of brain tumors have led to the development of targeted therapies. Despite the novel therapeutic intervention, the bottleneck arises in the clinical translation of drugs and their derivatives. The most common problems faced during drug delivery are; The inability of the drug to cross the BBB; poor specificity of the drug; short half-life or circulation time and physical and/or chemical interaction of the drug with the circulating proteins and brain tissue components etc (161–164). Therefore, newer and improved drug delivery methods need to be developed for the targeted delivery of the drugs to tumor cells while sparing healthy tissue.
Lipid-based drug delivery systems are promising candidates for addressing these needs for treating brain tumors. Encapsulating drugs in liposomes would be beneficial as they can cross the blood-brain barrier (BBB) more efficiently. However, liposomes can be difficult and expensive to manufacture on a large scale. Therefore, there is a need for alternative lipid-based drug delivery systems that can encapsulate drugs just as well but at a lower cost. Modifications of conventional chemotherapeutics or immune therapeutics have made drug delivery feasible and targetable in recent years in treating C high-grade gliomas (165). The pharmacokinetics of conventional liposomes after intravenous injection are very poor and are characterized by rapid systemic clearanced by plasma proteins and phagocytosis and removal by the liver, spleen, and other reticuloendothelial organs (166) Figure 4 depicts some of the considerations in lipid-based drug delivery systems to the brain.
Figure 4 Considerations in using different types of liposomes 1. Conventional (encapsulated with a chemotherapeutic agent (165, 167–169), 2. Bionic; either cationic or anionic with the drug of interest (108, 170–172), 3. Macromolecule formulated; for example, PEGylated (172), and 4. Next-gen containing targeted ligands towards receptors that are typically overexpressed in case of brain cancers for better clinical utility (166, 173, 174).
The use of liposomes has been linked with lower mortality rates and better patient outcomes in patients with brain tumors, eventually may become a standard mode of anti glioma therapy. Trials comparing chemotherapy and liposome-encapsulated doxorubicin have shown improved response rates and longer progression-free survival time for patients with glioblastoma multiforme (GBM). Another study showed that when delivered by liposomes, the chemotherapeutic agent tamoxifen could cross the blood-brain barrier more effectively than it could on its own. Koukourakis et al. investigated the clinical applications of radionuclide-labeled liposomal doxorubicin in both primary and metastatic brain tumors. radionuclide-labeled liposomal doxorubicin concentrated 13-19 times higher than the normal brain parenchyma. at the targeted tumor site, A Phase I and II trial by Hau et al. evaluated the role of PEGylated doxorubicin in high-grade gliomas and demonstrated moderate efficacy against recurrent gliomas, especially Grade III gliomas. In addition, PEG-DOX proved to be a safe treatment regimen with no significant side effects. The 6-month progression-free survival rate in the PEG-DOX arm was 32% compared to 20% in the standard temozolamide arm. Notably, approximately 5% of patients with Grade IV disease and about 40% of patients with Grade III disease were stable for up to 160 weeks after the initiation of therapy and exhibited long-term survival (175). In addition to better clinical response, a few common adverse effects like hand foot syndrome and stomatitis were observed due to increased circulation time of the formulation.
PEG-Dox liposome (2B3-101) have been clinically evaluated in phase I and II trials in high-grade gliomas (HGG’s). Among 24 HGG patients, 54% had stable disease, and a 3-month progression-free rate of 33% (176). Based on the first-in-human study 2B3-201 was considered clinically safe, with no serious adverse events reported, indicating the marketing potential of this product in the near future. Liposomal irinotecan (nal-IRI) have been evaluated in patients with recurrent high-grade gliomas., Patient treatment was stratified based on the UGT1A1 status. Homozygous WT patients were started at 120 mg/m2 IV with a dose escalation of 60 mg/m2 increments every 3 weeks and in heterozygous patients (HT) dosing began at 60 mg/m2, with dose escalation of 30 mg/m2increments. The MTD achieved was 120mg/m2 and 150 mg/m2 in WT and HT, respectively, with no major side effects reported (177). In another phase I and early efficacy trial, the same formulation was tested among patients with diffuse intrinsic pontine glioma (DIPG) with CED (Convection Enhanced Delivery). NaI-IRI was administered directly into the tumor using CED-post-radiotherapy (178). Similarly in a trial, when NaI-IRI was administered intravenously was considered safe with no anticipated toxicity among GBM patients. Notably, UGT1A1 genotype did not influence PK profile or correlate with toxicity (177).
In 2022, Kasenda and group, for the first time in a phase I trial, clinically assessed the capacity of EGFR-targeted immunoliposomes in delivering the cargo to brain tissue in patients with relapsed EGFR amplified glioblastomas. C225-ILs-dox-PEGylated liposomal doxorubicin containing the Fab fragment of anti-EGFR antibody CC25(Cetuximab), was administered at a dose of 50 mg/m2 intravenously, on day 1 of each cycle, every 28 days Anti-EGFR ILs-dox were infused intravenously with dose escalations of (doxorubicin 5 mg/m2, 10 mg/m2, 20 mg/m2, 30 mg/m2, 40 mg/m2, 50 mg/m2, and 60 mg/m2) once every 4 weeks for a maximum of six cycles in 26 patients. The MTD achieved was50mg/m2.Although no significant side effects were observed, one patient developed severe pneumonitis (179). Table 2 summarizes the ongoing and completed clinical trials utilizing lipid based drug delivery system.
Table 2 Clinical trials – past, present, and future of lipid-based drug delivery systems in CNS tumors.
(Data meta-analyzed using clinicaltrials.gov.in with the search keywords- neural/glial/CNS tumors/lipid/liposomes therapy)
Despite providing a multitude of applications in biology apart from cancer treatment, the delay in clinical translation of liposomes from preclinical to clinical is contributed by many issues such as pharmaceutical development being limited by cost and cumbersome quality assurance protocol (180). The complexity of multifunctional liposomal formulations complicates the larger scale manufacturing, cost, etc, in an industrial setting and Pk/PD, toxicity, and biosafety evaluation in research settings (181, 182). Clinical trials are more complex to account for groups for each entity of multifunctional nanoformulation, the discrepancy in the translation of therapeutic efficacy In vivo to humans (183, 184). Patenting and Copyrights (part of Intellectual property rights) contribute to the cost of development depending upon the complexity of formulations, design, composition, etc which may overshadow the commercial attractiveness, and the multiple patents over a given formulation might call for a need for cross-licensing arrangements (185–188).
For nano-liposomes to translate from a research lab to a clinical setting, challenges still need to be overcome. For instance, the safety and toxicity of liposomal formulations need further optimization, as the chemical method of synthesizing lipid-based NPs utilizes toxic organic solvents which are difficult to remove otherwise; secondly, long-term In vitro and In vivo toxicity and safety evaluation is required in case of nanomaterials due to their slow rate of metabolism, and; thirdly, the clinical translation of polymers, such as PEG mainly addresses such problems as their interaction with cell membranes results in low drug retention, increased toxicity, and rapid clearance from blood circulation. First, it is necessary to decrease the cytotoxic immune response and lengthen blood circulation by altering the surface of cell membranes, such as cationic polymers, peptides, etc. To increase the stability and effective crossing of NPs via the blood-brain barrier In vivo, it is also vital to optimize the size of nanoplatforms. Although new liposomal-based drug delivery systems have been well explored and established in preclinical animal models, these liposomal pharmaceutical products may not provide promising therapeutic effects in clinical trials. Next-generation development of liposomal-based drugs, the comparison of drug circulation time in blood, drug accumulation in tissues, and possible toxicity between conventional vesicles and new classes of liposomes should be investigated in preclinical animal models for better clinical utility.
Due to their heterogeneity, infiltrating nature, and inherent resistance to radiation and chemotherapy, gliomas, are considered the least treatable cancer. Hence, using targeted drug delivery systems that increase drug concentration in tumor tissue while avoiding systemic adverse effects is necessary for developing smart and effective therapeutics. One of the most promising approaches in treating brain tumors is nanomedicine-based delivery of drugs or biologics (immunotherapy). The advantages offered by lipid-based nanotherapeutics, especially the immunoliposomes is depicted in Figure 5. Nevertheless, many hurdles, such as the highly repressed immune microenvironment, the restricted drug transport to the central nervous system, and others have hampered the huge promise of chemotherapy and immunotherapy in these tumors. With the evolution in nanotechnology, drug delivery platforms have been created which can transport not only chemotherapeutics but also biologics like immuno therapeutic agents that work synergistically at the targeted site. The distribution of therapeutic compounds to brain tumor sites can be aided by the development of colloidal nanocarriers such as liposomes that release their payload inside the tumor microenvironment after selectively targeting immune cells.
Figure 5 Schematic representation of limitations in conventional therapeutic management of CNS tumors, and the benefits offered by lipid-based drug delivery systems, with special emphasis on immunoliposomes.
MH and PS share equal authorship. MH, PS and JA contributed to conceptualization, resource collection, and writing the original manuscript. VG contributed to reviewing, editing and supervising. JG did conceptualization, visualization, supervision, reviewing and editing the manuscript. All authors contributed to the article and approved the submitted version.
Open access funding is supported by Advance Centre for Treatment research and education in Cancer, Tata memorial Centre and Homi Bhabha National Institute, Mumbai, India
The authors declare that the research was conducted in the absence of any commercial or financial relationships that could be construed as a potential conflict of interest.
All claims expressed in this article are solely those of the authors and do not necessarily represent those of their affiliated organizations, or those of the publisher, the editors and the reviewers. Any product that may be evaluated in this article, or claim that may be made by its manufacturer, is not guaranteed or endorsed by the publisher.
1. Ostrom QT, Patil N, Cioffi G, Waite K, Kruchko C, Barnholtz-Sloan JS. CBTRUS statistical report: primary brain and other central nervous system tumors diagnosed in the united states in 2013–2017. Neuro Oncol (2020) 22(Suppl 1):iv1–96. doi: 10.1093/neuonc/noaa200
2. Louis DN, Perry A, Wesseling P, Brat DJ, Cree IA, Figarella-Branger D, et al. The 2021 WHO classification of tumors of the central nervous system: a summary. Neuro Oncol (2021) 23(8):1231–51. doi: 10.1093/neuonc/noab106
3. Louis DN, Ohgaki H, Wiestler OD, Cavenee WK, Burger PC, Jouvet A, et al. The 2007 WHO classification of tumours of the central nervous system. Acta Neuropathol (2007) 114(2):97–109. doi: 10.1007/s00401-007-0243-4
4. Shieh LT, Guo HR, Chang YK, Lu NM, Ho SY. Clinical implications of multiple glioblastomas: an analysis of prognostic factors and survival to distinguish from their single counterparts. J Formosan Med Assoc (2020) 119(3):728–34. doi: 10.1016/j.jfma.2019.08.024
5. Bou Zerdan M, Assi HI. Oligodendroglioma: a review of management and pathways. Front Mol Neurosci (2021) 14:722396. doi: 10.3389/fnmol.2021.722396
6. Reni M, Gatta G, Mazza E, Vecht C. Ependymoma. Crit Rev Oncology/Hematol (2007) 63(1):81–9. doi: 10.1016/j.critrevonc.2007.03.004
7. Kleihues P, Louis DN, Scheithauer BW, Rorke LB, Reifenberger G, Burger PC, et al. The WHO classification of tumors of the nervous system. J Neuropathol Exp Neurol. (2002) 61(3):215–25. doi: 10.1093/jnen/61.3.215
8. Wang J, Garancher A, Ramaswamy V, Wechsler-Reya RJ. Medulloblastoma: from molecular subgroups to molecular targeted therapies. Annu Rev Neurosci (2018) 41(1):207–32. doi: 10.1146/annurev-neuro-070815-013838
9. Read TA, Hegedus B, Wechsler-Reya R, Gutmann DH. The neurobiology of neurooncology. Ann Neurol (2006) 60(1):3–11. doi: 10.1002/ana.20912
10. Serlin Y, Shelef I, Knyazer B, Friedman A. Anatomy and physiology of the blood-brain barrier. Semin Cell Dev Biol (2015) 38:2–6. doi: 10.1016/j.semcdb.2015.01.002
11. Arvanitis CD, Ferraro GB, Jain RK. The blood–brain barrier and blood–tumour barrier in brain tumours and metastases. Nat Rev Cancer (2020) 20(1):26–41. doi: 10.1038/s41568-019-0205-x
12. Voges J, Reszka R, Gossmann A, Dittmar C, Richter R, Garlip G, et al. Imaging-guided convection-enhanced delivery and gene therapy of glioblastoma. Ann Neurol (2003) 54(4):479–87. doi: 10.1002/ana.10688
13. Lesniak MS, Brem H. Targeted therapy for brain tumours. Nat Rev Drug Discovery (2004) 3(6):499–508. doi: 10.1038/nrd1414
14. Ene CI, Nerva JD, Morton RP, Barkley AS, Barber JK, Ko AL, et al. Safety and efficacy of carmustine (BCNU) wafers for metastatic brain tumors. Surg Neurol Int (2016) 7(Suppl 11):S295–9. doi: 10.4103/2152-7806.181987
15. Gehrmann J, Matsumoto Y, Kreutzberg GW. Microglia: intrinsic immuneffector cell of the brain. Brain Res Brain Res Rev (1995) 20(3):269–87. doi: 10.1016/0165-0173(94)00015-H
16. Serot JM, Foliguet B, Béné MC, Faure GC. Ultrastructural and immunohistological evidence for dendritic-like cells within human choroid plexus epithelium. Neuroreport (1997) 8(8):1995–8. doi: 10.1097/00001756-199705260-00039
17. Owens T, Renno T, Taupin V, Krakowski M. Inflammatory cytokines in the brain: does the CNS shape immune responses? Immunol Today (1994) 15(12):566–71. doi: 10.1016/0167-5699(94)90218-6
18. Piguet V, Diserens AC, Carrel S, Mach JP, de Tribolet N. The immunobiology of human gliomas. Springer Semin Immunopathol (1985) 8(1–2):111–27. doi: 10.1007/BF00197250
19. Roy LO, Poirier MB, Fortin D. Transforming growth factor-beta and its implication in the malignancy of gliomas. Target Oncol (2015) 10(1):1–14. doi: 10.1007/s11523-014-0308-y
20. Nickl-Jockschat T, Arslan F, Doerfelt A, Bogdahn U, Bosserhoff A, Hau P. An imbalance between smad and MAPK pathways is responsible for TGF-beta tumor promoting effects in high-grade gliomas. Int J Oncol (2007) 30(2):499–507. doi: 10.3892/ijo.30.2.499
21. Mimeault M, Batra SK. Hypoxia-inducing factors as master regulators of stemness properties and altered metabolism of cancer- and metastasis-initiating cells. J Cell Mol Med (2013) 17(1):30–54. doi: 10.1111/jcmm.12004
22. Piperi C, Samaras V, Levidou G, Kavantzas N, Boviatsis E, Petraki K, et al. Prognostic significance of IL-8-STAT-3 pathway in astrocytomas: correlation with IL-6, VEGF and microvessel morphometry. Cytokine (2011) 55(3):387–95. doi: 10.1016/j.cyto.2011.05.012
23. Turkowski K, Brandenburg S, Mueller A, Kremenetskaia I, Bungert AD, Blank A, et al. VEGF as a modulator of the innate immune response in glioblastoma. Glia (2018) 66(1):161–74. doi: 10.1002/glia.23234
24. Kunkel P, Müller S, Schirmacher P, Stavrou D, Fillbrandt R, Westphal M, et al. Expression and localization of scatter factor/hepatocyte growth factor in human astrocytomas. Neuro Oncol (2001) 3(2):82–8. doi: 10.1093/neuonc/3.2.82
25. Arrieta O, Garcia E, Guevara P, Garcia-Navarrete R, Ondarza R, Rembao D, et al. Hepatocyte growth factor is associated with poor prognosis of malignant gliomas and is a predictor for recurrence of meningioma. Cancer (2002) 94(12):3210–8. doi: 10.1002/cncr.10594
26. Esencay M, Newcomb EW, Zagzag D. HGF upregulates CXCR4 expression in gliomas via NF-kappaB: implications for glioma cell migration. J Neurooncol (2010) 99(1):33–40. doi: 10.1007/s11060-010-0111-2
27. Wiesenhofer B, Stockhammer G, Kostron H, Maier H, Hinterhuber H, Humpel C. Glial cell line-derived neurotrophic factor (GDNF) and its receptor (GFR-alpha 1) are strongly expressed in human gliomas. Acta Neuropathol (2000) 99(2):131–7. doi: 10.1007/PL00007416
28. Zhang J, Sarkar S, Cua R, Zhou Y, Hader W, Yong VW, et al. And microglia that promotes tumor invasiveness through the CCL2/CCR2/interleukin-6 axis. Carcinogenesis (2012) 33(2):312–9. doi: 10.1093/carcin/bgr289
29. Wang SC, Hong JH, Hsueh C, Chiang CS. Tumor-secreted SDF-1 promotes glioma invasiveness and TAM tropism toward hypoxia in a murine astrocytoma model. Lab Invest. (2012) 92(1):151–62. doi: 10.1038/labinvest.2011.128
30. Zimmer N, Kim E, Sprang B, Leukel P, Khafaji F, Ringel F, et al. GARP as an immune regulatory molecule in the tumor microenvironment of glioblastoma multiforme. Int J Mol Sci (2019) 20(15):3676. doi: 10.3390/ijms20153676
31. Nduom EK, Weller M, Heimberger AB. Immunosuppressive mechanisms in glioblastoma. Neuro Oncol (2015) 17(Suppl 7):vii9–14. doi: 10.1093/neuonc/nov151
32. Mbongue JC, Nicholas DA, Torrez TW, Kim NS, Firek AF, Langridge WHR. The role of indoleamine 2, 3-dioxygenase in immune suppression and autoimmunity. Vaccines (2015) 3(3):703–29. doi: 10.3390/vaccines3030703
33. Zhang M, Song T, Yang L, Chen R, Wu L, Yang Z, et al. Nestin and CD133: valuable stem cell-specific markers for determining clinical outcome of glioma patients. J Exp Clin Cancer Res (2008) 27(1):85. doi: 10.1186/1756-9966-27-85
34. Yan J, Zhao Q, Gabrusiewicz K, Kong LY, Xia X, Wang J, et al. FGL2 promotes tumor progression in the CNS by suppressing CD103+ dendritic cell differentiation. Nat Commun (2019) 10(1):448. doi: 10.1038/s41467-018-08271-x
35. Bloch O, Crane CA, Kaur R, Safaee M, Rutkowski MJ, Parsa AT. Gliomas promote immunosuppression through induction of B7-H1 expression in tumor-associated macrophages. Clin Cancer Res (2013) 19(12):3165–75. doi: 10.1158/1078-0432.CCR-12-3314
36. Pyonteck SM, Akkari L, Schuhmacher AJ, Bowman RL, Sevenich L, Quail DF, et al. CSF-1R inhibition alters macrophage polarization and blocks glioma progression. Nat Med (2013) 19(10):1264–72. doi: 10.1038/nm.3337
37. Liu X, Liu J, Zhao S, Zhang H, Cai W, Cai M, et al. Interleukin-4 is essential for Microglia/Macrophage M2 polarization and long-term recovery after cerebral ischemia. Stroke (2016) 47(2):498–504. doi: 10.1161/STROKEAHA.115.012079
38. Orihuela R, McPherson CA, Harry GJ. Microglial M1/M2 polarization and metabolic states. Br J Pharmacol (2016) 173(4):649–65. doi: 10.1111/bph.13139
39. Street NE, Mosmann TR. Functional diversity of T lymphocytes due to secretion of different cytokine patterns. FASEB J (1991) 5(2):171–7. doi: 10.1096/fasebj.5.2.1825981
40. Hart DN, Fabre JW. Demonstration and characterization of ia-positive dendritic cells in the interstitial connective tissues of rat heart and other tissues, but not brain. J Exp Med (1981) 154(2):347–61. doi: 10.1084/jem.154.2.347
41. Harling-Berg CJ, Park TJ, Knopf PM. Role of the cervical lymphatics in the Th2-type hierarchy of CNS immune regulation. J Neuroimmunol (1999) 101(2):111–27. doi: 10.1016/S0165-5728(99)00130-7
42. Harling-Berg C, Knopf PM, Merriam J, Cserr HF. Role of cervical lymph nodes in the systemic humoral immune response to human serum albumin microinfused into rat cerebrospinal fluid. J Neuroimmunol (1989) 25(2–3):185–93. doi: 10.1016/0165-5728(89)90136-7
43. Harling-Berg CJ, Hallett JJ, Park JT, Knopf PM. Hierarchy of immune responses to antigen in the normal brain. Curr Top Microbiol Immunol (2002) 265:1–22. doi: 10.1007/978-3-662-09525-6_1
44. Cserr HF, Knopf PM. Cervical lymphatics, the blood-brain barrier and the immunoreactivity of the brain: a new view. Immunol Today (1992) 13(12):507–12. doi: 10.1016/0167-5699(92)90027-5
45. Engelhardt B, Ransohoff RM. Capture, crawl, cross: the T cell code to breach the blood-brain barriers. Trends Immunol (2012) 33(12):579–89. doi: 10.1016/j.it.2012.07.004
46. Lowe J, MacLennan KA, Powe DG, Pound JD, Palmer JB. Microglial cells in human brain have phenotypic characteristics related to possible function as dendritic antigen presenting cells. J Pathol (1989) 159(2):143–9. doi: 10.1002/path.1711590209
47. Jarry U, Jeannin P, Pineau L, Donnou S, Delneste Y, Couez D. Efficiently stimulated adult microglia cross-prime naive CD8+ T cells injected in the brain. Eur J Immunol (2013) 43(5):1173–84. doi: 10.1002/eji.201243040
48. Geribaldi-Doldán N, Fernández-Ponce C, Quiroz RN, Sánchez-Gomar I, Escorcia LG, Velásquez EP, et al. The role of microglia in glioblastoma. Front Oncol (2020) 10:603495. doi: 10.3389/fonc.2020.603495
49. Játiva P, Ceña V. Use of nanoparticles for glioblastoma treatment: a new approach. Nanomed (Lond). (2017) 12(20):2533–54. doi: 10.2217/nnm-2017-0223
50. Seo YE, Bu T, Saltzman WM. Nanomaterials for convection-enhanced delivery of agents to treat brain tumors. Curr Opin Biomed Eng (2017) 4:1–12. doi: 10.1016/j.cobme.2017.09.002
51. Zhang TT, Li W, Meng G, Wang P, Liao W. Strategies for transporting nanoparticles across the blood-brain barrier. Biomater Sci (2016) 4(2):219–29. doi: 10.1039/C5BM00383K
52. Xin H, Sha X, Jiang X, Zhang W, Chen L, Fang X. Anti-glioblastoma efficacy and safety of paclitaxel-loading angiopep-conjugated dual targeting PEG-PCL nanoparticles. Biomaterials (2012) 33(32):8167–76. doi: 10.1016/j.biomaterials.2012.07.046
53. Niu X, Chen J, Gao J. Nanocarriers as a powerful vehicle to overcome blood-brain barrier in treating neurodegenerative diseases: focus on recent advances. Asian J Pharm Sci (2019) 14(5):480–96. doi: 10.1016/j.ajps.2018.09.005
54. Tapeinos C, Battaglini M, Ciofani G. Advances in the design of solid lipid nanoparticles and nanostructured lipid carriers for targeting brain diseases. J Control Release (2017) 264:306–32. doi: 10.1016/j.jconrel.2017.08.033
55. Zhu L, Torchilin VP. Stimulus-responsive nanopreparations for tumor targeting. Integr Biol (Camb). (2013) 5(1):96–107. doi: 10.1039/c2ib20135f
56. Lanier LL. NK cell recognition. Annu Rev Immunol (2005) 23:225–74. doi: 10.1146/annurev.immunol.23.021704.115526
57. Friese MA, Platten M, Lutz SZ, Naumann U, Aulwurm S, Bischof F, et al. MICA/NKG2D-mediated immunogene therapy of experimental gliomas. Cancer Res (2003) 63(24):8996–9006.
58. Facoetti A, Nano R, Zelini P, Morbini P, Benericetti E, Ceroni M, et al. Human leukocyte antigen and antigen processing machinery component defects in astrocytic tumors. Clin Cancer Res (2005) 11(23):8304–11. doi: 10.1158/1078-0432.CCR-04-2588
59. Hadaschik EN, Enk AH. TGF-β1-induced regulatory T cells. Hum Immunol (2015) 76(8):561–4. doi: 10.1016/j.humimm.2015.06.015
60. Gorelik L, Flavell RA. Immune-mediated eradication of tumors through the blockade of transforming growth factor-beta signaling in T cells. Nat Med (2001) 7(10):1118–22. doi: 10.1038/nm1001-1118
61. Ahmadzadeh M, Rosenberg SA. TGF-beta 1 attenuates the acquisition and expression of effector function by tumor antigen-specific human memory CD8 T cells. J Immunol (2005) 174(9):5215–23. doi: 10.4049/jimmunol.174.9.5215
62. Catalano M, D’Alessandro G, Trettel F, Limatola C. Role of infiltrating Microglia/Macrophages in glioma. Adv Exp Med Biol (2020) 1202:281–98. doi: 10.1007/978-3-030-30651-9_14
63. Schartner JM, Hagar AR, Van Handel M, Zhang L, Nadkarni N, Badie B. Impaired capacity for upregulation of MHC class II in tumor-associated microglia. Glia (2005) 51(4):279–85. doi: 10.1002/glia.20201
64. Wainwright DA, Balyasnikova IV, Chang AL, Ahmed AU, Moon KS, Auffinger B, et al. IDO expression in brain tumors increases the recruitment of regulatory T cells and negatively impacts survival. Clin Cancer Res (2012) 18(22):6110–21. doi: 10.1158/1078-0432.CCR-12-2130
65. Prendergast GC, Malachowski WJ, Mondal A, Scherle P, Muller AJ. Indoleamine 2,3-dioxygenase and its therapeutic inhibition in cancer. Int Rev Cell Mol Biol (2018) 336:175–203. doi: 10.1016/bs.ircmb.2017.07.004
66. Hambardzumyan D, Gutmann DH, Kettenmann H. The role of microglia and macrophages in glioma maintenance and progression. Nat Neurosci (2016) 19(1):20–7. doi: 10.1038/nn.4185
67. Morris CS, Esiri MM. Immunocytochemical study of macrophages and microglial cells and extracellular matrix components in human CNS disease. 1. Gliomas J Neurol Sci (1991) 101(1):47–58. doi: 10.1016/0022-510X(91)90017-2
68. Gabrusiewicz K, Rodriguez B, Wei J, Hashimoto Y, Healy LM, Maiti SN, et al. Glioblastoma-infiltrated innate immune cells resemble M0 macrophage phenotype. JCI Insight. (2016) 1(2):e85841. doi: 10.1172/jci.insight.85841
69. Wierzba-Bobrowicz T, Kuchna I, Matyja E. Reaction of microglial cells in human astrocytomas (preliminary report). Folia Neuropathol (1994) 32(4):251–2.
70. Nishie A, Ono M, Shono T, Fukushi J, Otsubo M, Onoue H, et al. Macrophage infiltration and heme oxygenase-1 expression correlate with angiogenesis in human gliomas. Clin Cancer Res (1999) 5(5):1107–13.
71. Mieczkowski J, Kocyk M, Nauman P, Gabrusiewicz K, Sielska M, Przanowski P, et al. Down-regulation of IKKβ expression in glioma-infiltrating microglia/macrophages is associated with defective inflammatory/immune gene responses in glioblastoma. Oncotarget (2015) 6(32):33077–90. doi: 10.18632/oncotarget.5310
72. Mantovani A, Sozzani S, Locati M, Allavena P, Sica A. Macrophage polarization: tumor-associated macrophages as a paradigm for polarized M2 mononuclear phagocytes. Trends Immunol (2002) 23(11):549–55. doi: 10.1016/S1471-4906(02)02302-5
73. Szulzewsky F, Arora S, de Witte L, Ulas T, Markovic D, Schultze JL, et al. Human glioblastoma-associated microglia/monocytes express a distinct RNA profile compared to human control and murine samples. Glia (2016) 64(8):1416–36. doi: 10.1002/glia.23014
74. Grabowski MM, Sankey EW, Ryan KJ, Chongsathidkiet P, Lorrey SJ, Wilkinson DS, et al. Immune suppression in gliomas. J Neurooncol (2021) 151(1):3–12. doi: 10.1007/s11060-020-03483-y
75. Galluzzi L, Vacchelli E, Bravo-San Pedro JM, Buqué A, Senovilla L, Baracco EE, et al. Classification of current anticancer immunotherapies. Oncotarget (2014) 5(24):12472–508. doi: 10.18632/oncotarget.2998
76. Pires ES, D’Souza RS, Needham MA, Herr AK, Jazaeri AA, Li H, et al. Membrane associated cancer-oocyte neoantigen SAS1B/ovastacin is a candidate immunotherapeutic target for uterine tumors. Oncotarget (2015) 6(30):30194–211. doi: 10.18632/oncotarget.4734
77. Ahmed N, Brawley V, Hegde M, Bielamowicz K, Kalra M, Landi D, et al. HER2-specific chimeric antigen receptor-modified virus-specific T cells for progressive glioblastoma: a phase 1 dose-escalation trial. JAMA Oncol (2017) 3(8):1094–101. doi: 10.1001/jamaoncol.2017.0184
78. O’Rourke DM, Nasrallah MP, Desai A, Melenhorst JJ, Mansfield K, Morrissette JJD, et al. A single dose of peripherally infused EGFRvIII-directed CAR T cells mediates antigen loss and induces adaptive resistance in patients with recurrent glioblastoma. Sci Transl Med (2017) 9(399):eaaa0984. doi: 10.1126/scitranslmed.aaa0984
79. Brown CE, Alizadeh D, Starr R, Weng L, Wagner JR, Naranjo A, et al. Regression of glioblastoma after chimeric antigen receptor T-cell therapy. N Engl J Med (2016) 375(26):2561–9. doi: 10.1056/NEJMoa1610497
80. Sampson JH, Gunn MD, Fecci PE, Ashley DM. Brain immunology and immunotherapy in brain tumours. Nat Rev Cancer (2020) 20(1):12–25. doi: 10.1038/s41568-019-0224-7
81. Chen J, Cong X. Surface-engineered nanoparticles in cancer immune response and immunotherapy: current status and future prospects. Biomed Pharmacother (2023) 157:113998. doi: 10.1016/j.biopha.2022.113998
82. Savitsky K, Yu X. Combined strategies for tumor immunotherapy with nanoparticles. Clin Transl Oncol (2019) 21(11):1441–9. doi: 10.1007/s12094-019-02081-3
83. Guido C, Baldari C, Maiorano G, Mastronuzzi A, Carai A, Quintarelli C, et al. Nanoparticles for diagnosis and target therapy in pediatric brain cancers. Diagnostics (2022) 12(1):173. doi: 10.3390/diagnostics12010173
84. Hou Y, Bu W, Ai H, Lu ZR, Lammers T. Stimuli-responsive nanotheranostics. Adv Healthcare Mater (2021) 10(5):2100243. doi: 10.1002/adhm.202100243
85. Rodallec A, Sicard G, Fanciullino R, Benzekry S, Lacarelle B, Milano G, et al. Turning cold tumors into hot tumors: harnessing the potential of tumor immunity using nanoparticles. Expert Opin Drug Metab Toxicol (2018) 14(11):1139–47. doi: 10.1080/17425255.2018.1540588
86. Kroemer G, Galassi C, Zitvogel L, Galluzzi L. Immunogenic cell stress and death. Nat Immunol (2022) 23(4):487–500. doi: 10.1038/s41590-022-01132-2
87. Zhao Y, Chen Z, Li Q, Cao X, Huang Q, Shi L, et al. Polymer-Reinforced Liposomes Amplify Immunogenic Cell Death-Associated Antitumor Immunity for Photodynamic-Immunotherapy. Advanced Functional Materials [Internet] (2022) 32(52):2209711. doi: 10.1002/adfm.202209711
88. Kadiyala P, Li D, Nuñez FM, Altshuler D, Doherty R, Kuai R, et al. High density lipoprotein-mimicking nanodiscs for chemo-immunotherapy against glioblastoma multiforme. ACS Nano (2019) 13(2):1365–84. doi: 10.1021/acsnano.8b06842
89. Scheetz L, Kadiyala P, Sun X, Son S, Hassani Najafabadi A, Aikins M, et al. Synthetic high-density lipoprotein nanodiscs for personalized immunotherapy against gliomas. Clin Cancer Res (2020) 26(16):4369–80. doi: 10.1158/1078-0432.CCR-20-0341
90. Chiang CLL, Kandalaft LE, Coukos G. Adjuvants for enhancing the immunogenicity of whole tumor cell vaccines. Int Rev Immunol (2011) 30(2–3):150–82. doi: 10.3109/08830185.2011.572210
91. Aaes TL, Kaczmarek A, Delvaeye T, De Craene B, De Koker S, Heyndrickx L, et al. Vaccination with necroptotic cancer cells induces efficient anti-tumor immunity. Cell Rep (2016) 15(2):274–87. doi: 10.1016/j.celrep.2016.03.037
92. Steinman RM. Dendritic cells and the control of immunity: enhancing the efficiency of antigen presentation. Mt Sinai J Med (2001) 68(3):160–6.
93. Sabado RL, Bhardwaj N. Cancer immunotherapy: dendritic-cell vaccines on the move. Nature (2015) 519(7543):300–1. doi: 10.1038/nature14211
94. Hinrichs CS, Rosenberg SA. Exploiting the curative potential of adoptive T-cell therapy for cancer. Immunol Rev (2014) 257(1):56–71. doi: 10.1111/imr.12132
95. Finn OJ. The dawn of vaccines for cancer prevention. Nat Rev Immunol (2018) 18(3):183–94. doi: 10.1038/nri.2017.140
96. Fecci PE, Sweeney AE, Grossi PM, Nair SK, Learn CA, Mitchell DA, et al. Systemic anti-CD25 monoclonal antibody administration safely enhances immunity in murine glioma without eliminating regulatory T cells. Clin Cancer Res (2006) 12(14 Pt 1):4294–305. doi: 10.1158/1078-0432.CCR-06-0053
97. Beelman CA, Parker R. Degradation of mRNA in eukaryotes. Cell (1995) 81(2):179–83. doi: 10.1016/0092-8674(95)90326-7
98. Falcone D, Andrews DW. Both the 5’ untranslated region and the sequences surrounding the start site contribute to efficient initiation of translation in vitro. Mol Cell Biol (1991) 11(5):2656–64. doi: 10.1128/mcb.11.5.2656-2664.1991
99. Hoekstra D, Rejman J, Wasungu L, Shi F, Zuhorn I. Gene delivery by cationic lipids: in and out of an endosome. Biochem Soc Trans (2007) 35(Pt 1):68–71. doi: 10.1042/BST0350068
100. Mendez-Gomez H, McGuiness J, Grippin A, Weidert F, Carrera-Justiz S, Mitchell D, et al. Immuu-13.Customizable multi-lamellar RNA-nanoparticles for paediatric glioma. Neuro Oncol (2021) 23(Suppl 1):i29–30. doi: 10.1093/neuonc/noab090.120
101. Wu M, Fan Y, Lv S, Xiao B, Ye M, Zhu X. Vincristine and temozolomide combined chemotherapy for the treatment of glioma: a comparison of solid lipid nanoparticles and nanostructured lipid carriers for dual drugs delivery. Drug Deliv (2016) 23(8):2720–5. doi: 10.3109/10717544.2015.1058434
102. Witika BA, Bassey KE, Demana PH, Siwe-Noundou X, Poka MS. Current advances in specialised niosomal drug delivery: manufacture, characterization and drug delivery applications. Int J Mol Sci (2022) 23(17):9668. doi: 10.3390/ijms23179668
103. Luiz MT, Tofani LB, Araújo VHS, Di Filippo LD, Duarte JL, Marchetti JM, et al. Gene therapy based on lipid nanoparticles as non-viral vectors for glioma treatment. Curr Gene Ther (2021) 21(5):452–63. doi: 10.2174/1566523220999201230205126
104. De Vries J, Figdor C. Immunotherapy: cancer vaccine triggers antiviral-type defences. Nature (2016) 534(7607):329–31. doi: 10.1038/nature18443
105. Joshi S, Singh-Moon RP, Wang M, Chaudhuri DB, Holcomb M, Straubinger NL, et al. Transient cerebral hypoperfusion assisted intraarterial cationic liposome delivery to brain tissue. J Neurooncol (2014) 118(1):73–82. doi: 10.1007/s11060-014-1421-6
106. Joshi S, Singh-Moon R, Wang M, Chaudhuri DB, Ellis JA, Bruce JN, et al. Cationic surface charge enhances early regional deposition of liposomes after intracarotid injection. J Neurooncol (2014) 120(3):489–97. doi: 10.1007/s11060-014-1584-1
107. Vorbrodt AW. Ultracytochemical characterization of anionic sites in the wall of brain capillaries. J Neurocytol (1989) 18(3):359–68. doi: 10.1007/BF01190839
108. Vieira DB, Gamarra LF. Getting into the brain: liposome-based strategies for effective drug delivery across the blood–brain barrier. Int J Nanomed (2016) 11:5381–414. doi: 10.2147/IJN.S117210
109. Porteous DJ, Dorin JR, McLachlan G, Davidson-Smith H, Davidson H, Stevenson BJ, et al. Evidence for safety and efficacy of DOTAP cationic liposome mediated CFTR gene transfer to the nasal epithelium of patients with cystic fibrosis. Gene Ther (1997) 4(3):210–8. doi: 10.1038/sj.gt.3300390
110. Mai Y, Guo J, Zhao Y, Ma S, Hou Y, Yang J. Intranasal delivery of cationic liposome-protamine complex mRNA vaccine elicits effective anti-tumor immunity. Cell Immunol (2020) 354:104143. doi: 10.1016/j.cellimm.2020.104143
111. Dhaliwal HK, Fan Y, Kim J, Amiji MM. Intranasal delivery and transfection of mRNA therapeutics in the brain using cationic liposomes. Mol Pharm (2020) 17(6):1996–2005. doi: 10.1021/acs.molpharmaceut.0c00170
112. Sayour EJ, De Leon G, Pham C, Grippin A, Kemeny H, Chua J, et al. Systemic activation of antigen-presenting cells via RNA-loaded nanoparticles. Oncoimmunology (2017) 6(1):e1256527. doi: 10.1080/2162402X.2016.1256527
113. Liu S, Liu J, Li H, Mao K, Wang H, Meng X, et al. An optimized ionizable cationic lipid for brain tumor-targeted siRNA delivery and glioblastoma immunotherapy. Biomaterials (2022) 287:121645. doi: 10.1016/j.biomaterials.2022.121645
114. Grafals-Ruiz N, Rios-Vicil CI, Lozada-Delgado EL, Quiñones-Díaz BI, Noriega-Rivera RA, Martínez-Zayas G, et al. Brain targeted gold liposomes improve RNAi delivery for glioblastoma. Int J Nanomed (2020) 15:2809–28. doi: 10.2147/IJN.S241055
115. Eygeris Y, Gupta M, Kim J, Sahay G. Chemistry of lipid nanoparticles for RNA delivery. Acc Chem Res (2022) 55(1):2–12. doi: 10.1021/acs.accounts.1c00544
116. Ostrand-Rosenberg S, Fenselau C. Myeloid-derived suppressor cells: immune-suppressive cells that impair antitumor immunity and are sculpted by their environment. J Immunol (2018) 200(2):422–31. doi: 10.4049/jimmunol.1701019
117. Davoodzadeh Gholami M, Kardar GA, Saeedi Y, Heydari S, Garssen J, Falak R. Exhaustion of T lymphocytes in the tumor microenvironment: significance and effective mechanisms. Cell Immunol (2017) 322:1–14. doi: 10.1016/j.cellimm.2017.10.002
118. Heczey A, Louis CU, Savoldo B, Dakhova O, Durett A, Grilley B, et al. CAR T cells administered in combination with lymphodepletion and PD-1 inhibition to patients with neuroblastoma. Mol Ther (2017) 25(9):2214–24. doi: 10.1016/j.ymthe.2017.05.012
119. Huang W, Luo S, Burgess R, Yi YH, Huang GF, Huang RP. New insights into the tumor microenvironment utilizing protein array technology. Int J Mol Sci (2018) 19(2):559. doi: 10.3390/ijms19020559
120. Kourie HR, Klastersky JA. Side-effects of checkpoint inhibitor-based combination therapy. Curr Opin Oncol (2016) 28(4):306–13. doi: 10.1097/CCO.0000000000000295
121. Ramos RN, Piaggio E, Romano E. Mechanisms of resistance to immune checkpoint antibodies. Handb Exp Pharmacol (2018) 249:109–28. doi: 10.1007/164_2017_11
122. Zhou WT, Jin WL. B7-H3/CD276: an emerging cancer immunotherapy. Front Immunol. (2021) 12:701006. doi: 10.3389/fimmu.2021.701006
123. Riley RS, June CH, Langer R, Mitchell MJ. Delivery technologies for cancer immunotherapy. Nat Rev Drug Discovery (2019) 18(3):175–96. doi: 10.1038/s41573-018-0006-z
124. Shi C, Zhang Q, Yao Y, Zeng F, Du C, Nijiati S, et al. Targeting the activity of T cells by membrane surface redox regulation for cancer theranostics. Nat Nanotechnol (2023) 18(1):86–97. doi: 10.1038/s41565-022-01261-7
125. Benmebarek MR, Karches CH, Cadilha BL, Lesch S, Endres S, Kobold S. Killing mechanisms of chimeric antigen receptor (CAR) T cells. Int J Mol Sci (2019) 20(6):1283. doi: 10.3390/ijms20061283
126. Wagner J, Wickman E, DeRenzo C, Gottschalk S. CAR T cell therapy for solid tumors: bright future or dark reality? Mol Ther (2020) 28(11):2320–39. doi: 10.1016/j.ymthe.2020.09.015
127. Akhavan D, Alizadeh D, Wang D, Weist MR, Shepphird JK, Brown CE. CAR T cells for brain tumors: lessons learned and road ahead. Immunol Rev (2019) 290(1):60–84. doi: 10.1111/imr.12773
128. Sanz-Ortega L, Rojas JM, Barber DF. Improving tumor retention of effector cells in adoptive cell transfer therapies by magnetic targeting. Pharmaceutics (2020) 12(9):812. doi: 10.3390/pharmaceutics12090812
129. Zhang F, Stephan SB, Ene CI, Smith TT, Holland EC, Stephan MT. Nanoparticles that reshape the tumor milieu create a therapeutic window for effective T-cell therapy in solid malignancies. Cancer Res (2018) 78(13):3718–30. doi: 10.1158/0008-5472.CAN-18-0306
130. Billingsley MM, Hamilton AG, Mai D, Patel SK, Swingle KL, Sheppard NC, et al. Orthogonal design of experiments for optimization of lipid nanoparticles for mRNA engineering of CAR T cells. Nano Lett (2022) 22(1):533–42. doi: 10.1021/acs.nanolett.1c02503
131. Zhu X, Prasad S, Gaedicke S, Hettich M, Firat E, Niedermann G. Patient-derived glioblastoma stem cells are killed by CD133-specific CAR T cells but induce the T cell aging marker CD57. Oncotarget (2015) 6(1):171–84. doi: 10.18632/oncotarget.2767
132. Walker AJ, Majzner RG, Zhang L, Wanhainen K, Long AH, Nguyen SM, et al. Tumor antigen and receptor densities regulate efficacy of a chimeric antigen receptor targeting anaplastic lymphoma kinase. Mol Ther (2017) 25(9):2189–201. doi: 10.1016/j.ymthe.2017.06.008
133. Tantalo DG, Oliver AJ, von Scheidt B, Harrison AJ, Mueller SN, Kershaw MH, et al. Understanding T cell phenotype for the design of effective chimeric antigen receptor T cell therapies. J Immunother Cancer (2021) 9(5):e002555. doi: 10.1136/jitc-2021-002555
134. Watanabe K, Kuramitsu S, Posey AD, June CH. Expanding the therapeutic window for CAR T cell therapy in solid tumors: the knowns and unknowns of CAR T cell biology. Front Immunol (2018) 9:2486. doi: 10.3389/fimmu.2018.02486
135. Sedgwick AJ, Ghazanfari N, Constantinescu P, Mantamadiotis T, Barrow AD. The role of NK cells and innate lymphoid cells in brain cancer. Front Immunol (2020) 11:1549. doi: 10.3389/fimmu.2020.01549
136. da Silva LHR, Catharino LCC, da Silva VJ, Evangelista GCM, Barbuto JAM. The war is on: the immune system against glioblastoma-how can NK cells drive this battle? Biomedicines (2022) 10(2):400. doi: 10.3390/biomedicines10020400
137. Siegler EL, Kim YJ, Chen X, Siriwon N, Mac J, Rohrs JA, et al. Combination cancer therapy using chimeric antigen receptor-engineered natural killer cells as drug carriers. Mol Ther (2017) 25(12):2607–19. doi: 10.1016/j.ymthe.2017.08.010
138. Yang L, DeBusk LM, Fukuda K, Fingleton B, Green-Jarvis B, Shyr Y, et al. Expansion of myeloid immune suppressor Gr+CD11b+ cells in tumor-bearing host directly promotes tumor angiogenesis. Cancer Cell (2004) 6(4):409–21. doi: 10.1016/j.ccr.2004.08.031
139. Hong IS, Jang GB, Lee HY, Nam JS. Targeting cancer stem cells by using the nanoparticles. Int J Nanomed (2015) 10(Spec Iss):251–60. doi: 10.2147/IJN.S88310
140. Lathia JD, Mack SC, Mulkearns-Hubert EE, Valentim CLL, Rich JN. Cancer stem cells in glioblastoma. Genes Dev (2015) 29(12):1203–17. doi: 10.1101/gad.261982.115
141. Nakada M, Nambu E, Furuyama N, Yoshida Y, Takino T, Hayashi Y, et al. Integrin α3 is overexpressed in glioma stem-like cells and promotes invasion. Br J Cancer (2013) 108(12):2516–24. doi: 10.1038/bjc.2013.218
142. Zarnescu O, Brehar FM, Bleotu C, Gorgan RM. Co-Localization of PCNA, VCAM-1 and caspase-3 with nestin in xenografts derived from human anaplastic astrocytoma and glioblastoma multiforme tumor spheres. Micron (2011) 42(8):793–800. doi: 10.1016/j.micron.2011.04.005
143. Prabhu S, Goda JS, Mutalik S, Mohanty BS, Chaudhari P, Rai S, et al. A polymeric temozolomide nanocomposite against orthotopic glioblastoma xenograft: tumor-specific homing directed by nestin. Nanoscale (2017) 9(30):10919–32. doi: 10.1039/C7NR00305F
144. Orza A, Soriţău O, Tomuleasa C, Olenic L, Florea A, Pana O, et al. Reversing chemoresistance of malignant glioma stem cells using gold nanoparticles. Int J Nanomed (2013) 8:689–702. doi: 10.2147/IJN.S37481
145. Glaser T, Han I, Wu L, Zeng X. Targeted nanotechnology in glioblastoma multiforme. Front Pharmacol (2017) 8:166. doi: 10.3389/fphar.2017.00166
146. Hayward SL, Wilson CL, Kidambi S. Hyaluronic acid-conjugated liposome nanoparticles for targeted delivery to CD44 overexpressing glioblastoma cells. Oncotarget (2016) 7(23):34158–71. doi: 10.18632/oncotarget.8926
147. Bao S, Wu Q, Sathornsumetee S, Hao Y, Li Z, Hjelmeland AB, et al. Stem cell-like glioma cells promote tumor angiogenesis through vascular endothelial growth factor. Cancer Res (2006) 66(16):7843–8. doi: 10.1158/0008-5472.CAN-06-1010
148. Bergers G, Song S. The role of pericytes in blood-vessel formation and maintenance. Neuro Oncol (2005) 7(4):452–64. doi: 10.1215/S1152851705000232
149. Jhaveri N, Chen TC, Hofman FM. Tumor vasculature and glioma stem cells: contributions to glioma progression. Cancer Lett (2016) 380(2):545–51. doi: 10.1016/j.canlet.2014.12.028
150. Yoshida D, Kim K, Noha M, Teramoto A. Hypoxia inducible factor 1-alpha regulates of platelet derived growth factor-b in human glioblastoma cells. J Neurooncol (2006) 76(1):13–21. doi: 10.1007/s11060-005-3279-0
151. Hovinga KE, Shimizu F, Wang R, Panagiotakos G, van der Heijden M, Moayedpardazi H, et al. Inhibition of notch signaling in glioblastoma targets cancer stem cells via an endothelial cell intermediate. Stem Cells (2010) 28(6):1019–29. doi: 10.1002/stem.429
152. Li XY, Zhao Y, Sun MG, Shi JF, Ju RJ, Zhang CX, et al. Multifunctional liposomes loaded with paclitaxel and artemether for treatment of invasive brain glioma. Biomaterials (2014) 35(21):5591–604. doi: 10.1016/j.biomaterials.2014.03.049
153. Lei W, Yang C, Wu Y, Ru G, He X, Tong X, et al. Nanocarriers surface engineered with cell membranes for cancer targeted chemotherapy. J Nanobiotechnol (2022) 20:45. doi: 10.1186/s12951-022-01251-w
154. Sofias AM, Toner YC, Meerwaldt AE, van Leent MMT, Soultanidis G, Elschot M, et al. Tumor targeting by αvβ3-Integrin-Specific lipid nanoparticles occurs via phagocyte hitchhiking. ACS Nano (2020) 14(7):7832–46. doi: 10.1021/acsnano.9b08693
155. Sakurai Y, Hatakeyama H, Sato Y, Hyodo M, Akita H, Ohga N, et al. RNAi-mediated gene knockdown and anti-angiogenic therapy of RCCs using a cyclic RGD-modified liposomal-siRNA system. J Control Release (2014) 173:110–8. doi: 10.1016/j.jconrel.2013.10.003
156. Su Z, Xing L, Chen Y, Xu Y, Yang F, Zhang C, et al. Lactoferrin-modified poly(ethylene glycol)-grafted BSA nanoparticles as a dual-targeting carrier for treating brain gliomas. Mol Pharm (2014) 11(6):1823–34. doi: 10.1021/mp500238m
157. Qi N, Zhang S, Zhou X, Duan W, Gao D, Feng J, et al. Combined integrin αvβ3 and lactoferrin receptor targeted docetaxel liposomes enhance the brain targeting effect and anti-glioma effect. J Nanobiotechnology. (2021) 19:446. doi: 10.12669/pjms.38.1.4396
158. Monopoli MP, Aberg C, Salvati A, Dawson KA. Biomolecular coronas provide the biological identity of nanosized materials. Nat Nanotechnol (2012) 7(12):779–86. doi: 10.1038/nnano.2012.207
159. Salvati A, Pitek AS, Monopoli MP, Prapainop K, Bombelli FB, Hristov DR, et al. Transferrin-functionalized nanoparticles lose their targeting capabilities when a biomolecule corona adsorbs on the surface. Nat Nanotechnol (2013) 8(2):137–43. doi: 10.1038/nnano.2012.237
160. Saenz del Burgo L, Hernández RM, Orive G, Pedraz JL. Nanotherapeutic approaches for brain cancer management. Nanomedicine (2014) 10(5):905–19. doi: 10.1016/j.nano.2013.10.001
161. Dong X. Current strategies for brain drug delivery. Theranostics (2018) 8(6):1481–93. doi: 10.7150/thno.21254
162. Pardridge WM. Drug transport across the blood–brain barrier. J Cereb Blood Flow Metab (2012) 32(11):1959–72. doi: 10.1038/jcbfm.2012.126
163. Ahlawat J, Guillama Barroso G, Masoudi Asil S, Alvarado M, Armendariz I, Bernal J, et al. Nanocarriers as potential drug delivery candidates for overcoming the blood–brain barrier: challenges and possibilities. ACS Omega (2020) 5(22):12583–95. doi: 10.1021/acsomega.0c01592
164. Bellettato CM, Scarpa M. Possible strategies to cross the blood–brain barrier. Ital J Pediatr (2018) 44(2):131. doi: 10.1186/s13052-018-0563-0
165. Juhairiyah F, de Lange ECM. Understanding drug delivery to the brain using liposome-based strategies: studies that provide mechanistic insights are essential. AAPS J (2021) 23(6):114. doi: 10.1208/s12248-021-00648-z
166. Newton HB. Advances in strategies to improve drug delivery to brain tumors. Expert Rev Neurother (2006) 6(10):1495–509. doi: 10.1586/14737175.6.10.1495
167. Akbarzadeh A, Rezaei-Sadabady R, Davaran S, Joo SW, Zarghami N, Hanifehpour Y, et al. Liposome: classification, preparation, and applications. Nanoscale Res Lett (2013) 8(1):102. doi: 10.1186/1556-276X-8-102
168. Gao J, Wang Z, Liu H, Wang L, Huang G. Liposome encapsulated of temozolomide for the treatment of glioma tumor: preparation, characterization and evaluation. Drug Discovery Ther (2015) 9(3):205–12. doi: 10.5582/ddt.2015.01016
169. Wu S, Li G, Li X, Lin C, Yu D, Luan S, et al. Transport of glial cell line-derived neurotrophic factor into liposomes across the blood-brain barrier: in vitro and in vivo studies. Int J Mol Sci (2014) 15(3):3612–23. doi: 10.3390/ijms15033612
170. Morille M, Passirani C, Vonarbourg A, Clavreul A, Benoit JP. Progress in developing cationic vectors for non-viral systemic gene therapy against cancer. Biomaterials (2008) 29(24–25):3477–96. doi: 10.1016/j.biomaterials.2008.04.036
171. Bender HR, Kane S, Zabel MD. Delivery of therapeutic siRNA to the CNS using cationic and anionic liposomes. J Vis Exp (2016) 113):54106. doi: 10.3791/54106
172. Yang C, Li Y, Yang Y, Chen Z. Overview of strategies to improve therapy against tumors using natural killer cell. J Immunol Res (2020) 2020:8459496. doi: 10.1155/2020/8459496
173. Mora M, Sagristá ML, Trombetta D, Bonina FP, De Pasquale A, Saija A. Design and characterization of liposomes containing long-chain n-acylPEs for brain delivery: penetration of liposomes incorporating GM1 into the rat brain. Pharm Res (2002) 19(10):1430–8. doi: 10.1023/A:1020440229102
174. Gaillard PJ, Appeldoorn CCM, Dorland R, van Kregten J, Manca F, Vugts DJ, et al. Pharmacokinetics, brain delivery, and efficacy in brain tumor-bearing mice of glutathione pegylated liposomal doxorubicin (2B3-101). PloS One (2014) 9(1):e82331. doi: 10.1371/journal.pone.0082331
175. Hau P, Fabel K, Baumgart U, Rümmele P, Grauer O, Bock A, et al. Pegylated liposomal doxorubicin-efficacy in patients with recurrent high-grade glioma. Cancer (2004) 100(6):1199–207. doi: 10.1002/cncr.20073
176. Brandsma D, Stalpers L, Taal W, Sminia P, van den Bent MJ. Clinical features, mechanisms, and management of pseudoprogression in malignant gliomas. Lancet Oncol (2008) 9(5):453–61. doi: 10.1016/S1470-2045(08)70125-6
177. Clarke JL, Molinaro AM, Cabrera JR, DeSilva AA, Rabbitt JE, Prey J, et al. A phase 1 trial of intravenous liposomal irinotecan in patients with recurrent high-grade glioma. Cancer Chemother Pharmacol (2017) 79(3):603–10. doi: 10.1007/s00280-017-3247-3
178. PhD SM MD. a phase I and early efficacy study of convection enhanced delivery of irinotecan liposome injection using real time imaging with gadolinium in children with diffuse intrinsic pontine glioma (2023). Available at: https://clinicaltrials.gov/ct2/show/study/NCT03086616.
179. Kasenda B, König D, Manni M, Ritschard R, Duthaler U, Bartoszek E, et al. Targeting immunoliposomes to EGFR-positive glioblastoma. ESMO Open (2022) 7(1):100365. doi: 10.1016/j.esmoop.2021.100365
180. Sercombe L, Veerati T, Moheimani F, Wu SY, Sood AK, Hua S. Advances and challenges of liposome assisted drug delivery. Front Pharmacol (2015) 6:286. doi: 10.3389/fphar.2015.00286
181. Teli MK, Mutalik S, Rajanikant GK. Nanotechnology and nanomedicine: going small means aiming big. Curr Pharm Des (2010) 16(16):1882–92. doi: 10.2174/138161210791208992
182. Tinkle S, McNeil SE, Mühlebach S, Bawa R, Borchard G, Barenholz YC, et al. Nanomedicines: addressing the scientific and regulatory gap. Ann N Y Acad Sci (2014) 1313:35–56. doi: 10.1111/nyas.12403
183. Allen TM, Cullis PR. Liposomal drug delivery systems: from concept to clinical applications. Adv Drug Delivery Rev (2013) 65(1):36–48. doi: 10.1016/j.addr.2012.09.037
184. Narang AS, Chang RK, Hussain MA. Pharmaceutical development and regulatory considerations for nanoparticles and nanoparticulate drug delivery systems. J Pharm Sci (2013) 102(11):3867–82. doi: 10.1002/jps.23691
185. Murday JS, Siegel RW, Stein J, Wright JF. Translational nanomedicine: status assessment and opportunities. Nanomedicine (2009) 5(3):251–73. doi: 10.1016/j.nano.2009.06.001
186. Roberts SF, Fischhoff MA, Sakowski SA, Feldman EL. Perspective: transforming science into medicine: how clinician-scientists can build bridges across research’s “valley of death”. Acad Med (2012) 87(3):266–70. doi: 10.1097/ACM.0b013e3182446fa3
187. Satalkar P, Elger BS, Hunziker P, Shaw D. Challenges of clinical translation in nanomedicine: a qualitative study. Nanomedicine (2016) 12(4):893–900. doi: 10.1016/j.nano.2015.12.376
Keywords: CNS tumors, immunotherapy, lipid-based nanoparticles, blood-brain barrier, clinical trail
Citation: Hegde MM, Sandbhor P, J. A, Gota V and Goda JS (2023) Insight into lipid-based nanoplatform-mediated drug and gene delivery in neuro-oncology and their clinical prospects. Front. Oncol. 13:1168454. doi: 10.3389/fonc.2023.1168454
Received: 17 February 2023; Accepted: 16 June 2023;
Published: 06 July 2023.
Edited by:
Jyotsnendu Giri, Indian Institute of Technology Hyderabad, IndiaReviewed by:
Haigang Wu, Henan University, ChinaCopyright © 2023 Hegde, Sandbhor, J., Gota and Goda. This is an open-access article distributed under the terms of the Creative Commons Attribution License (CC BY). The use, distribution or reproduction in other forums is permitted, provided the original author(s) and the copyright owner(s) are credited and that the original publication in this journal is cited, in accordance with accepted academic practice. No use, distribution or reproduction is permitted which does not comply with these terms.
*Correspondence: Jayant S. Goda, amdvZGFAYWN0cmVjLmdvdi5pbg==; Z29kYWpheWFudHNhc3RyaUBnbWFpbC5jb20=; Vikram Gota, dmdvdGFAYWN0cmVjLmdvdi5pbg==
†These authors share first authorship
Disclaimer: All claims expressed in this article are solely those of the authors and do not necessarily represent those of their affiliated organizations, or those of the publisher, the editors and the reviewers. Any product that may be evaluated in this article or claim that may be made by its manufacturer is not guaranteed or endorsed by the publisher.
Research integrity at Frontiers
Learn more about the work of our research integrity team to safeguard the quality of each article we publish.