- Department of Anatomy and Cell Biology, Martin Luther University Halle-Wittenberg, Halle (Saale), Germany
Malignant tumors are still a global, heavy health burden. Many tumor types cannot be treated curatively, underlining the need for new treatment targets. In recent years, metastasis associated in colon cancer 1 (MACC1) was identified as a promising biomarker and drug target, as it is promoting tumor migration, initiation, proliferation, and others in a multitude of solid cancers. Here, we will summarize the current knowledge about MACC1-induced tumor cell migration with a special focus on the cytoskeletal and adhesive systems. In addition, a brief overview of several in vitro models used for the analysis of cell migration is given. In this context, we will point to issues with the currently most prevalent models used to study MACC1-dependent migration. Lastly, open questions about MACC1-dependent effects on tumor cell migration will be addressed.
Introduction
Malignant tumors are one of the most common and often deadly health problems throughout the world. Analysis for 2020 estimated 19.3 million new cases of cancer and 10 million deaths from cancer (1). Thus, it is without a doubt still a necessity to better understand the mechanisms of tumor development and expansion and to improve the treatment of cancer. In 2009, the gene metastasis associated in colon cancer 1 (MACC1) was discovered in colorectal cancer (2) and connected to increased metastasis, cell survival, proliferation, and migration. Afterward, MACC1 was quickly found to be involved in tumor formation and migration in a multitude of different solid tumors, including glioblastoma, ovarian carcinoma, gastric cancer, hepatocellular carcinoma, and others (3). Notably, in many instances, MACC1 expression was additionally linked to increased metastasis formation, being the main reason for cancer mortality (3). Interestingly, circulating MACC1 transcripts or protein levels can also serve as predictive markers for tumor progression, as demonstrated in patients with colorectal, pancreatic, gastric, lung, and breast cancer (4–9).
Due to its clinical relevance, MACC1-associated signaling was intensively studied. Thus, many upstream and downstream targets of MACC1 were identified and associated with typical tumor features such as NANOG and OCT4 with dedifferentiation, VEGF, and TWIST1/2 with angiogenesis (3, 10, 11). Notably, in almost all studies, MACC1 expression was associated with a more migratory phenotype (2, 3, 12, 13). Attainment of a migratory phenotype is a key feature in the metastatic cascade and a highly complex phenomenon (14). Cell migration in complex environments necessitates cells to generate propulsive forces, change shapes, form (transient) adhesions with the extracellular matrix (ECM) and neighboring cells, and remodel the extracellular space (15, 16). Consequently, the migratory process is not only highly important during tumor spreading but also involves a multitude of complex processes.
Thus, this review aims to summarize the different downstream effects of MACC1 on tumor migration and how these can be integrated into the cytoskeletal and adhesive systems. Furthermore, we want to point out open questions and additional approaches to elucidate MACC1-dependent effects on cellular migration further.
The cytoskeleton, adhesion, and cell migration
The migration of cells is an essential part of cancer metastasis, depending on the reorganization of the cytoskeleton, cell–cell, and cell–matrix adhesions. Here, only a brief review of the cytoskeleton, adhesion, and its relation to migratory processes is given. For further information, the interested reader is referred to the following reviews (17–31).
The cytoskeleton
The cytoskeleton is a network consisting of actin filaments, microtubules, and intermediate filaments. The (sub-) structures formed by each of those elements are not independent, but coupled, increasing the complexity of the system (17). For this review, we will focus on microtubules and actin due to their crucial role in cell migration and on vimentin as it was often found to be regulated by MACC1 (32–35).
Actin
Actin filaments can form different types of cytoplasmatic structures, such as the actin cortex, the dendritic actin network forming the lamellipodium, and stress fibers.
The lamellipodium is a flat, dendritic actin structure associated with cellular movement in 2D and 3D environments, yet its occurrence in 3D depends on the physical properties of the surrounding cells (36). Actin in the lamellipodium is nucleated by the Arp2/3 complex, quickly creating large protrusions that extend the cells’ exterior by pushing forces created during actin polymerization (37). Arp2/3 is activated via the Scar/WAVE complex which in turn is regulated by the small RhoGTPase Rac1 (38). In a 3D environment, N-WASP was shown to induce ARP2/3 activation, mostly independent of Rac1 (39, 40). In addition to these proteins, others such as anti-capping proteins like Ena/vasodilator-stimulated phosphoprotein (VASP), capping proteins, cross-linkers, and polymerization-limiting factors like arpin are necessary to regulate the formation of the lamellipodium (17). During the expansion of the lamellipodium, new cell–substrate adhesions are formed, increasing the lifetime of the lamellipodium, responsible for a movement in the direction of high cell–ECM adhesiveness (41, 42).
In contrast to the lamellipodium, stress fibers are formed by bundles of parallel or anti-parallel-oriented actin filaments (43). Stress fibers are bundled by cross-linkers such as α-actinin, fascin, and filamin and connected to focal adhesions (17). Anti-parallel stress fibers can additionally contain myosin and, thus, generate contractile forces (44). Given that contractility is regulated by myosin, stress fiber contractility is often regulated similarly, via, e.g., phosphorylation of the regulatory light chain and activation of the myosin light chain kinase either via the small GTPase RhoA or Ca2+, respectively (45). The formation of stress fibers depends on the activation of the formin mDia1 and RhoA (46, 47). Stress fibers do not form protrusions but are thought to generate contractile forces to retract the rear, modify the ECM via generated tension, or transmit traction forces to the substrate for effective cell body translocation (48–50).
The last structure described here is the actin cortex, forming a contractile structure located beneath the plasma membrane. It consists of actin filament bundles, cross-linkers (α-actinin, fascin, etc.), myosin, actin polymerization factors (ARP2/3, mDia1), ERM family members (ezrin, radixin, moesin), and others (51, 52). In terms of migration, the so-called blebbing needs to be mentioned. Blebs are protrusions formed in regions where the actin cortex locally ruptures or is detached from the membrane, so that hydrostatic pressure inside the cell causes small cell protrusions (blebs) (17). Of note, this mode of migration is suitable for migration in low adhesive environments (53–56). Another property of the cortex is its tension, determining cell shape and thus migration regulated by activation of myosin and actin polymerization (57–59).
Microtubules
Microtubules are hollow filaments consisting of α- and β-tubulin. Due to their larger size and organization, microtubules can withstand larger compressive forces than actin and intermediate filaments (60). Thus, microtubules serve as tracks for intracellular transport. Except for some cases, microtubules are mostly regarded as indirect promoters of migration, independent of their mechanical contribution (61–63). As microtubules are polarized, molecular motors can transport cargo directionally along formed tracks. The cargo can contain lipids to increase the surface area for protrusions, secretory proteins, integrins, small GTPases (Rac, CDC42), proteases, etc. (17, 27), all associated with cell migration. Furthermore, mRNA for leading edge components, such as the ARP2/3 complex, profilin, or β-actin, is transported along microtubule tracks (64–66).
Notably, the actin cytoskeleton and microtubules are inherently coupled, due to a multitude of microtubule regulators, such as APC or mDia1, that bind and regulate actin and due to actin–microtubule cross-linking factors such as MACF1 (17, 27). Furthermore, (de-)polymerization of microtubules could be linked to Rac1 or RhoA signaling, respectively, via microtubule-regulated guanine exchange factors (GEFs) (27). Consequently, changes in the regulation of one cytoskeletal network can affect the other directly or indirectly.
Vimentin
Vimentin belongs to the class of type III intermediate filaments, forming homopolymers of vimentin monomers, and it is expressed in most cancer and precursor cells. Vimentin plays an important role in migration. Its upregulation correlates with the epithelial–mesenchymal transition (EMT), associated with metastasis (67). Consequently, motile and invasive cells show higher vimentin expression, and the knockdown of vimentin impairs migration (68). One mechanism of vimentin action is its function as a guiding structure for microtubule growth, necessary for maintaining cell polarity (69). Despite microtubule-associated effects, vimentin co-regulates the organization of the actin cytoskeleton. Thus, vimentin can either directly bind to actin (70) or indirectly via, e.g., plectin (71). In addition, vimentin depletion caused the induction of RhoA and myosin activity and, consequently, stress fiber assembly (72, 73). In astrocytes, vimentin was found to be necessary to maintain cell polarization of leader cells in wound-healing assays via control of forces, attributed to a lower degree of focal adhesion concentration at the cell front (74).
Furthermore, vimentin is involved in nuclear positioning, a key element in cell migration, as the nucleus is the largest and stiffest organelle of the cell (75, 76). Likewise, vimentin supports the cell against compressive stress as experienced during tumor growth, promoting cell migration and invasion (77, 78). In line with this idea, vimentin regulates migration in dense but not in sparse cultures, by induction of a stiffer, less deformable phenotype (79).
Cell adhesions
Cells need anchorage points for migration allowing them to transmit forces. The most common forms are specific cell–cell adhesions and cell–matrix adhesions. Because of the highly complex nature of both adhesive systems, we will focus on integrin-based cell–matrix adhesions and cadherin-mediated cell–cell adhesions.
Cell–matrix adhesions
Cell–matrix adhesions—as referred to here—are considered connections of transmembranous integrins and extracellular matrix components such as collagen, fibronectin, and laminin. These bindings result in the formation of adhesion complexes, connecting the ECM to the actin cytoskeleton. The best-characterized cell–matrix adhesion type is the focal adhesion, containing among others integrins, paxilin, focal adhesion kinase (FAK), talin, vinculin, actin, and actin-regulating proteins (80–83). Notably, these molecules are also associated with cell signaling (especially FAK) and mechanotransduction (talin, vinculin), respectively. The full consensus integrin adhesome contains more than 60 components (84). Integrin-mediated cell–matrix adhesions sense and transmit biochemical signals about the ECM composition and mechanics into the cell interior. Thus, they are responsible for directed cell migration in the direction of more rigid substrates (durotaxis), along chemical gradients (chemotaxis), and in the direction of higher ECM concentration (haptotaxis) (85). Signals are transmitted to the cell interior via activation of, e.g., YAP/TAZ or SRF (86). Further signaling molecules associated with cell–matrix adhesions are FAK, Src, paxillin, and others, all associated with cell migration (24). FAK signaling can promote cell migration via Rac1-induced actin polymerization, using different routes through either PI3K or p130cas/Crk/DOCK180 signaling (87). Furthermore, FAK can suppress stress fiber formation via RhoA inhibition and regulate several GTPase-activating proteins (GAP) and activate N-WASP to facilitate Arp2/3 activation at the leading edge (87).
As cell–matrix adhesions are directly connected to the actin cytoskeleton, it is not surprising that a multitude of interactions between focal adhesions and microtubules and vimentin exist. For example, it was demonstrated that microtubules can target focal adhesions, especially at the rear of the cell, fastening their dissociation (27). On the other hand, microtubules support the formation of focal adhesions at the front via the transport of integrins (88, 89). Similarly, vimentin localizes at focal adhesions (90), directly interacting with integrin subunits (91, 92), and incorporates into forming (nascent) and mature adhesions (93). In addition, vimentin was found to be involved in integrin trafficking to the leading edge (94).
Cell–cell adhesions
Cell–cell adhesions couple neighboring cells, not only functioning as a signaling hub but also introducing mechanical coupling involved in collective cell migration. The most studied class of cell–cell adhesion molecules is cadherins. They are calcium-dependent transmembrane proteins, consisting of multiple members, including N-cadherin, E-cadherin, and P-cadherin, and form homotypic and heterotypic adhesive bonds. Despite cadherins, adhesion complexes contain β-catenin, α-catenin, p120 catenin, vinculin, GEFs, VASP, and others and are connected to the actin cytoskeleton (22, 95). Of note, GEFs, VASP, and others are related to actin remodeling (95). Thus, classical cadherins mechanically connect the actin cytoskeleton of neighboring cells, and therefore, the tension on the actin cytoskeleton can be transferred across multiple cells. The transferred tension can in turn stabilize the adhesion (96–98), resulting in cortical stiffening (98). Cadherin binding also regulates Rac1 and Arp2/3, inhibiting protrusion formation in follower cells during collective migration (99), probably partly dependent on its impact on cortex organization. Cadherin adhesions further alter actin cytoskeleton organization and promote tension by activation of RhoA and Cdc42 (100, 101). Additionally, the application of tension on E-cadherin activates PI3K in EGFR dependence, resulting in integrin-dependent cell–matrix adhesions and ROCK-induced contractility (102). Similarly, P-cadherin was found to promote focal contact formation (103). It is also noteworthy that cadherin types have different functions and associations with (collective) cell migration. E-cadherin and N-cadherin, for example, are involved in EMT and associated with a more (N-cadherin) or less (E-cadherin) migratory phenotype (104). Yet, the exact role of E-cadherin and cell–cell adhesion in cell migration, in general, is still a matter of debate, as E-cadherin was found in migrating tumor cells and collective migration was the dominant form of migration in tumors (21, 105, 106). Similarly, for E-cadherin and P-cadherin, different roles in force transmission were found. While E-cadherin strengthens cell–cell adhesions, P-cadherins regulate the tension a focal adhesion can transmit, but P-cadherin can partially substitute E-cadherin in case of E-cadherin loss (107).
Given the close connection between adherence junctions and the actin cytoskeleton, numerous interactions between cell–matrix interactions, intermediate filaments, and microtubules were found. For example, a formed keratin–cadherin complex was demonstrated to be involved in directional migration (108), while microtubules are involved in the transport of N-cadherin and p120 catenin to adherens junctions (109, 110). Cell–ECM adhesions to fibronectin were found to inhibit the formation of E-cadherin-dependent cell–cell junctions (111). Similarly, β1 integrin binding triggered the scattering and disassembly of cell–cell contacts (112). One explanation may be the binding-induced outside-in signaling of integrins, via FAK and Src that can destabilize cell–cell adhesions (23).
Models to study cell migration
Given the complexity and entanglement of the cytoskeletal and adhesive systems, the choice of the model system and evaluation parameters is highly important to differentiate between different types of effects involved in migration, e.g., effects on cell polarization, cooperativity, and chemotaxis. Thus, we briefly summarize the most popular in vitro migration models and address their advantages and disadvantages. Therefore, we will group the models according to the dimensionality of the system as either 1D, 2D, or 3D and as endpoint or dynamic measurements. A brief summary of the presented models is given in Table 1, and some models are illustrated in Figure 1.
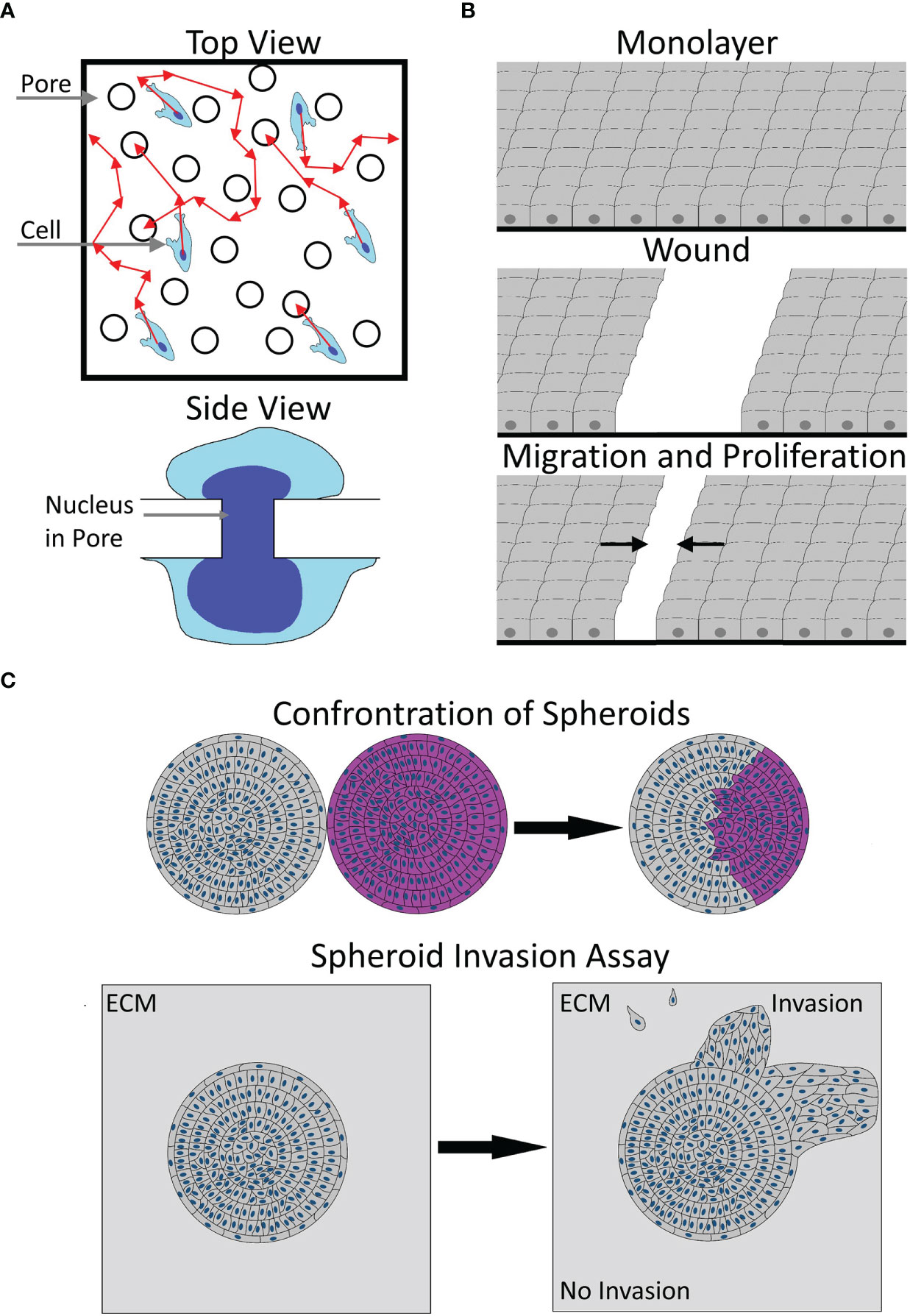
Figure 1 Illustration of migration models. (A) A sketch of the transwell model from the top (top) or side view (bottom). Please note the uneven distribution of pores and unclear cell path (red arrows) before contacting a pore in the top view. The side view shows the necessary, potentially rate-limiting deformation of the nucleus to pass a pore. (B) The working principle of a scratch wound assay, starting from an unscratched monolayer, via wounding, to subsequent wound closure by proliferation and migration. Arrows denote the average direction of migration. (C) Two types of 3D migration models. The top image shows a typical spheroid confrontation assay, bringing the spheroids of two cell populations (gray and pink) into contact, with the subsequent fusion of spheroids and intraspheroidal migration. The bottom image illustrates a typical spheroid invasion assay, showing an embedded spheroid, showing collective and single-cell invasion into the ECM. Arrows illustrate the transition from the start to the end of the experiment.
1D migration can be realized either via thin strips of adhesive substrate surrounded by non-adhesive substrate or via narrow channels that cells can migrate through (113–118). Such models are considered to represent migration along fibers in confinement (119) and are appropriate to either analyze single-cell migration or collective migration of groups of cells in a continuous manner (114, 116). Furthermore, some systems, especially microfluidic ones, can be used to induce long-term stable chemotactic gradients (120). Consequently, 1D systems are suitable to assess chemotaxis, but also migration under confinement. On the other hand, such setups might be complex to implement and analyze. Such approaches might be useful to elucidate the effects of MACC1 on chemotaxis and to decouple the effects on random migration from chemotaxis. Currently, the most prominently used Boyden chamber assays cannot distinguish well between both. Please see below for more details.
Looking at 2D systems, three common models will be discussed: sparsely seeded cells on a 2D substrate, the scratch wound, and the transwell or Boyden chamber assay. While technically not entirely 2D, the dimensionality of transwell assays is closest to a 2D system, rather than 1D or 3D.
The simplest form to analyze migration in 2D is to sparsely seed cells and monitor the movement of individual cells via time-lapse microscopy. Typically, cell speed and the sense of directionality of cells are measured (13, 121, 122). The conduction of such experiments is comparably simple, and surfaces can be modified both in terms of functionalization, via, e.g., ECM components and stiffness (117). Yet, analysis can be more complex. If done manually, the throughput is limited and results may vary between different raters. Automatic approaches allow for a high throughput (121, 123, 124) but are normally optimized to a certain cell appearance and morphology. Thus, they either need significant parameter tuning or might even be unusable for certain cell types, albeit machine learning systems help to overcome this issue (123, 124). As only single cells are analyzed, cell–cell interactions are neglected, but the effects of substrates, interventions and environmental stiffness on cytoskeletal dynamics can be analyzed. Notably, for MACC1, such analysis is mostly missing.
When using the scratch wound assay, cells are seeded to form a dense monolayer, and afterward, an artificial wound is created (125, 126). Notably, the surfaces can be functionalized using ECM components. Afterward, the wound closure is monitored for several days, either as endpoint measurement or in a continuous manner. Typically, the rate of wound closure is used as a proxy for migration (127, 128), albeit a more complex analysis can be performed to obtain additional information (129–132). In the simplest form, conducted as an endpoint assay without complex surface functionalization, the scratch assay is comparably simple to perform and analyze. Yet, the scratch assay has several disadvantages, such as the scratch procedure itself suffers—if done manually—from a large variability (133, 134) and is inducing significant damage to the remaining cells, via factors released from damaged cells (134). Furthermore, the scratch procedure may damage surface modifications (133, 135). To circumvent the abovementioned issues, the cell exclusion assay can be used. In principle, it is identical to the scratch assay except that cells are seeded into a culture vessel containing a block of defined size so that cells cannot enter this area. For the experiment, the block is removed and migration is monitored as mentioned before, assuring that no cell death occurs. Yet, precaution must be taken that none of the cells crawls under the used block (136). Independent of the usage of the scratch or exclusion assay, cell proliferation has to be taken into consideration, as most of these assays are performed for at least 1 day, so the final readout will be a mixture of proliferation and migration (137). While attempts are being made to detect cell divisions in parallel in phase contrast images (138–141), the decoupling of proliferation and migration in these assays remains an issue. Furthermore, if interventions affect cell viability, they cannot be distinguished from migration using the scratch or cell exclusion assay with wound width as a readout (127). While scratch wound assays were used frequently, when analyzing MACC1-dependent migration, they were most often performed as endpoint assays, unable to differentiate between proliferation and migration. The use of more advanced analysis schemes combined with live-cell imaging (129–132) may help to reveal more details regarding MACC1 effects on (collective) cell dynamics, cell polarization, leader cell determination, etc. Furthermore, by simultaneous detection of proliferation events or via inhibition of proliferation (138–142), MACC1-induced effects on proliferation could potentially be decoupled from migration.
The last 2D system discussed here is the transwell or Boyden chamber assay. For the transwell assay, cells are seeded on top of a coated or uncoated porous membrane with a defined pore size, while the culture medium is placed on top and below the cells. Due to this setup, a chemotactic gradient can be generated via the addition of a chemoattractant or repellent to the lower or upper compartment, respectively. As readout for migration, the number of cells migrating from the top to the bottom side of the membrane, the number of cells on the bottom of the lower well, or the sum of both is counted. Normally, cell counting is done manually and only at one defined time point. Therefore, the setup and data analysis can be considered rather simple and quick. Of note, there are modified versions of the Boyden chamber assay that do not need manual counting and allow for a continuous data assessment, e.g., the IncuCyte or xCELLigence systems (12, 143, 144). Yet, for MACC1, the standard Boyden chamber assay was the most used one (see Table 2). On the other hand, the transwell assay has some severe drawbacks: The size of the pores of the membrane has to be chosen carefully, to fit the overall size of the cell and nucleus, as the nucleus and its deformability are often rate-limiting for cell migration (146, 147). Too large pores would in contrast lead to the unspecific dropping of cells through the membrane (136). The gradient generated is neither constant over time nor linear (148, 149). The generated gradients can nearly vanish after as short as 6 h and degrade to 50% of their initial value after ≈1 min (148). Notably, gradient steepness affects chemotactic response (150–152). When seeding cells, care must be taken to obtain a single-cell suspension, as cell aggregates are slower or even unable to migrate through the membrane pores. For obtaining statistically robust results, it is necessary to have a large number of migrating cells, and as most cell types have different migratory capacities, the experimental endpoint needs to be determined for every single-cell type individually (135). Another issue with the transwell assay is the distribution and size of pores on the membrane, which is not even (136, 153), and thus, the amount of random 2D motion of cells on top of the membrane before reaching a pore is undefined and creates additional measurement uncertainty. A last point to be taken into account is the time between seeding and stopping of the experiment. For times above 24 h, proliferation affects the readout, via an increase of cells on the lower membrane and the bottom part of the assay. Thus, for sufficiently long experimental times, the effects of interventions on proliferation cannot be distinguished from migration. Please note that for most migration experiments associated with MACC1, incubation times of 24-48 h were used. Taken together, using the transwell assay, it can be—dependent on the exact setup—highly difficult to elucidate the actual reason for the change of migratory capacity.
For 3D migration models, we limit the description to the spheroid migration/invasion assay. Spheroids are multicellular, spherical objects of one or more cell types, cultured in a low to non-adhesive environment or generated via confinement (154), favoring the formation of cell–cell adhesions instead of cell–matrix adhesions. When reaching a critical diameter of 200-500 µm, spheroids develop oxygen, nutrient, and catabolite gradients and, when growing larger, show necrotic cores, recapitulating several key factors of in vivo tumors (155). For migration/invasion assays, spheroids can be placed on top of a coated surface or embedded in a hydrogel, mimicking the ECM (155). Independent of the exact experimental settings, spheroids can be imaged continuously or at the beginning and end of the experiment only. A typical readout of such migration experiments is the increase in cell-covered area or tracking of individual migrating cells, to measure speed, morphology, or even migration of cells inside the spheroid (156–160). While such a model represents a more physiological approach, it is typically more time-consuming. Notably, the size of the spheroid needs to be controlled tightly, as this affects its composition and thus the gradients inside the spheroid (155). If spheroids are embedded into hydrogels, the stiffness, pore size, and composition of the hydrogel need to be precisely chosen and reproduced, as all affect cell migration (146, 147, 161, 162). Furthermore, not all cell types and lines form spheroids in all assays. Additionally, if live-cell imaging is performed, together with the analysis of the motion of single cells, the analysis might become highly complex (156, 160).
As a special case, a spheroid confrontation assay can be done. There, cells of one spheroid can migrate into another spheroid of the same or different cell type (155, 159, 160). In principle, similar parameters can be assessed as described before: the infiltration of one spheroid into the other, either as a bulk measurement or on a single-cell basis, and the time of complete fusion (159, 160). All parameters can be considered proxies of cell migration and/or invasion. Usage of the mentioned 3D assays could help to analyze the role of homo- or heterotypical cell–cell interactions during migration in more physiological environments, in terms of dimensionality, stiffness, and chemical composition. Notably, the effect of confinement—drastically altering the motile machinery (163)—can be studied as well. Currently, studies on MACC1 focused on 2D models. Yet, in 2D, cells tend to show drastically different behavior and organization compared with 3D, including morphology, proliferation, cell interactions, and gene expression patterns (161). Thus, the usage of more complex 3D models is expected to yield a rich set of information on MACC1-dependent migration.
MACC1 in tumor migration
Starting with the initial discovery of MACC1, effects on migration were reported (2). Notably, in vivo MACC1 was also enriched in tumor buds and cells at the invasive front of colon carcinoma (164, 165), making it tempting to speculate about the role of MACC1 in leader cell determination during collective invasion. Initially, MACC1-induced effects involved the activation of HGF/cMet signaling (2). The following studies supported these findings (12, 33, 166–170). cMet signaling can induce activation of the Src family members, FAK, small Rho GTPases such as Cdc42 or Rac1, and others, all implied in cell migration (171, 172). A pan-cancer database analysis of MACC1 in 33 cancer types generated a consensus list of 1,896 genes correlating with MACC1 in at least half of the tumor entities. The authors found an enrichment of the consensus list in genes associated with cell junction organization, cell–cell junctions, and regulation of cell adhesion and cell junctions, pointing toward a far larger set of MACC1-associated pathways than HGF/cMet (173). A sketch of the current migration-associated network is shown in Figure 2A, and the potential intervention strategies are shown in Figure 2B. Of note, cMet signaling can induce AKT and ERK signaling (171, 172, 174), both also induced by MACC1 (see below).
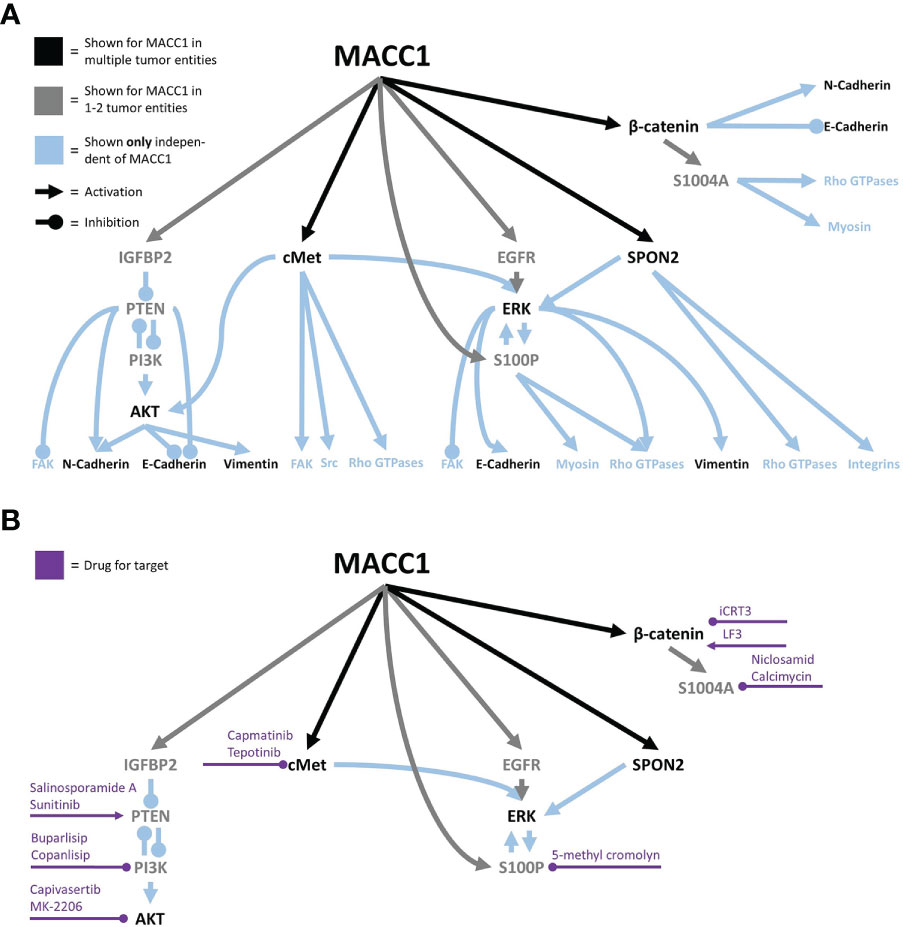
Figure 2 The MACC1 signaling network associated with cell migration (A) and potential intervention points, with the associated drugs (B).
Furthermore, MACC1 expression correlated with intracellular and secreted IGFBP2 (175) that was independently found to be collected by integrins causing inhibition of the tumor suppressor PTEN (176, 177). Increased PTEN expression was found after MACC1 silencing in esophageal carcinoma (178), and in hepatocellular carcinoma, MACC1 induced PI3K activation (179). In line with this, several studies reported associations of MACC1 with AKT activation (33, 143, 166, 178–183), suggesting the following MACC1 signaling: MACC1/IGFBP2/PTEN/PI3K/AKT (175, 178, 179, 184). Yet, MACC1 may affect PI3K/AKT signaling also via direct interactions with YWHAE (14-3-3 epsilon), as confirmed by affinity capture-MS (185). YWHAE was previously found to induce PI3K and AKT activation (186). Additionally, PTEN was reported to suppress cell–matrix adhesion-associated tumor cell migration by inhibition of FAK (187) and further induce N-cadherin and suppress E-cadherin (188), while PI3K/Akt signaling had the opposite effects (189). Interestingly, MACC1 was shown to interact with MARK2 (Microtubule Affinity Regulating Kinase 2) (185), a protein that induced FAK activation, contractility, and stress fiber formation and was involved in cell polarization (190, 191). Another hint suggesting that MACC1 interacts with cell–cell and cell–matrix adhesions was implied by the interaction of MACC1 with SPON2 in colorectal cancer (192). SPON2 is a known ligand for integrins α4 and β2 (193), linked to integrin α5 and β1 signaling (194), and demonstrated to regulate the expression of Rac1 and Rac2 in dendritic cells (195), resulting in the promotion of lamellipodium formation (196). In contrast, no MACC1-dependent change in the distribution of integrins α5 and β1 was found in glioblastoma cells (13). Furthermore, SPON2 was shown to inhibit E-cadherin and N-cadherin in gastric cancer (197). In addition, MACC1 negatively correlated with the expression of α-actinin (34), a cross-linker necessary for the formation and stability of stress fibers (198). Furthermore, a study in ovarian cancer demonstrated lower cell–matrix adhesion to matrigel after MACC1 silencing in a 2-h time frame covering the onset of cell attachment (199). In line with this, in non-small cell lung and gastric cancer, MACC1 correlated with a lower expression of collagen I and fibronectin (34, 167). Yet, two studies in gastric cancer associated MACC1 with higher fibronectin expression (35, 200). Similarly, adhesion to fibronectin and laminin of glioblastoma cells was not altered after MACC1 overexpression for short interaction times of 1 min (13). In summary, there are clear hints on the effects of MACC1 acting on cell–matrix adhesions, but the effects may depend on the tumor type, analyzed integrins, or the ECM component, and consequently, this aspect needs additional research for a clear picture.
Another signaling route affected by MACC1 is the ERK pathway, as demonstrated in multiple tumor entities (168, 169, 180, 182, 183, 201–203). Potentially, ERK activation is induced by MACC1 via sustained EGFR signaling, independent of EGFR expression (201). Moreover, it was demonstrated that the MACC1 target SPON2 can also induce ERK activation in gastric cancer cells (197). Interestingly, ERK1/2 can regulate and is regulated by S100P, which is also induced by MACC1 (204, 205). Previously, S100P was shown to affect myosin II, reduce the number of focal adhesions (206), interact with Cdc42 and Rac1 regulators, and affect cell migration via ezrin binding (207). In addition, ERK co-regulates E-cadherin (208) and was shown to be involved in protrusion formation via induction of actin polymerization at the leading edge (209). ERK was also suspected to be involved in FAK inactivation, regulation of RhoA and myosin II, downregulation of E-cadherin, and upregulation of N-cadherin and vimentin (197, 209, 210).
Looking further downstream, MACC1 was frequently found to induce a mesenchymal phenotype in multiple tumor types, through the measurement of EMT markers, such as increased expression of vimentin and N-cadherin and reduced expression of E-cadherin (32–34, 166, 169, 170, 182, 203, 211–213). Yet, the intracellular organization of the vimentin cytoskeleton does not appear to be altered in glioblastoma cells upon MACC1 overexpression (13). Direct interactions between E-cadherin and MACC1 were described earlier, being another potential way of E-cadherin regulation via MACC1 (214). As discussed before, these molecules are involved in single-cell and collective migration, due to associations with cytoskeletal reorganization and cell–cell coupling. Likewise, MACC1 induced β-catenin expression and phosphorylation, an important signaling and adaptor protein in cell–cell junctions (169, 180, 203, 215–217), negatively regulating E-cadherin (208). As mentioned earlier, MACC1 interacts with YWHAE which was shown to induce lower E-cadherin but higher N-cadherin and vimentin expression (186), being another explanation for the observed effects. Arguments for MACC1 acting on cell–cell adhesions and cytoskeletal organization are supported by a study in HeLa cells showing lowered actin staining upon MACC1 silencing (218) and by experiments on glioblastoma cells showing lower equilibrium cell–cell adhesion after MACC1 overexpression (13). Additionally, α-smooth muscle actin (αSMA) expression, normally expressed in smooth muscles and myofibroblasts, was also positively correlated with MACC1 expression (212). In glioblastoma, MACC1 promoted random motion in 2D, caused by a lower cortical stiffness and accumulation of protrusive actin near the protruding edge (13). Yet, the stiffer and faster random migratory phenotype appears to be tumor type-dependent, as it was not found in colorectal cancer cells (142).
Interestingly, MACC1 also was involved in cytoskeletal organization under metabolic stress in gastric cancer (219). Upon glucose deprivation, gastric cancer cells showed increased formation of stress fibers, caused by DLC3 downregulation and subsequent MACC1 upregulation. Upon silencing MACC1, the induction of stress fibers under metabolic stress was abrogated (219). The same study suggested a MACC1-promoted chemotactic migration along glucose gradients (219). In line with this, MACC1 expression resulted in increased glucose uptake, ATP levels, and lactate production in gastric cancer cells (211). Interestingly, the MACC1 stabilizing long non-coding RNA MACC1-AS is upregulated upon glucose deprivation, further promoting MACC1-induced glycolysis and antioxidant production under metabolic stress (220). Given the fact that the metabolic state and nutrient availability of cells have a large impact on cytoskeletal organization and migration (26, 221), MACC1-induced metabolic adaptation might be another part of the MACC1-regulated migration in vivo.
MACC1 expression not only had intracellular effects but also changed the secretion profile of cells. In colorectal cancer, MACC1 expression was connected to increased S100A4 secretion, induced by the β-catenin/TCF4 axis (216). S1004A was previously shown to regulate non-muscle myosin heavy chain and RhoA, leading to chemotaxis and stress fiber formation (222). Additionally, direct interactions with the actin cytoskeleton were found (223). Of note, extracellular S1004A was demonstrated to induce matrix metalloproteinase (MMP) expression of MMP1, MMP3, MMP9, and MMP13, facilitating the degradation and remodeling of the extracellular matrix and thus its migration (223). In agreement, MACC1 was associated with increased expression of several MMPs, namely MMP2, MMP3, and MMP9 (34, 199, 224–226).
Another study performed in colorectal cancer found MACC1 to affect collective but not single-cell migration (142). Interestingly, MACC1-induced effects were fully abrogated when proliferation was inhibited (142). This study points toward another—potentially very important—aspect of MACC1-induced migration that was previously identified independently on MACC1. Proliferation events can cause both a local and even global fluidization of cell layers and thus permit the reorganization and migration of cells (227–232). In light of these observations, the following questions arise: what part of MACC1 promigratory effects is caused by the classical way via the cytoskeletal and adhesive dynamics, and what proportion is caused by MACC1-induced increased proliferation? These questions become even more relevant, given the large number of assays used to analyze MACC1-dependent migration that cannot distinguish between proliferation and migration, e.g., scratch assays performed as endpoint assays and long-lasting transwell assays (see Table 2).
Inhibiting cancer cell migration and thus ultimately metastasis formation is one approach to fight against cancer. Yet, many clinical trials targeting migration-associated molecules had only limited success, if at all (233, 234). The MACC1-dependent signaling network could be an additional piece to the puzzle helping to bridge the gap between preclinical research and the successful clinical application of anti-migratory drugs. For many MACC1 targets, drugs that are or were in clinical use exist (235–238), albeit for some, available drugs are sparse (see Figure 2B). Additionally, some of them, e.g., 5-methyl cromolyn, were not yet clinically tested to the authors’ knowledge and are generally only poorly investigated in human systems (239). Furthermore, lovastatin and rottlerin were shown to transcriptionally inhibit MACC1, but both have diverse off-targets and are only a little specific (128). Consequently, further research into MACC1-specific drugs may be an additional route to inhibit cancer cell migration.
Conclusion and outlook
In the few years since its discovery, MACC1 has been demonstrated to be a very promising predictive biomarker in a multitude of tumor entities because it induces migration and proliferation among others. On a mechanistic level, effects were mediated very frequently via ubiquitous, major pathways, such as cMet, AKT, or ERK. While several studies elucidated further the downstream effects, the current data and understanding of how MACC1-induced effects are transduced to cytoskeletal or adhesive remodeling in detail remain largely elusive. Further research may help to complete this picture and identify potential targets for treatment. As MACC1 is involved not only in cell migration but also in proliferation, functional assays need to take the pro-proliferative effect of MACC1 into account. Currently, most of the assays employed cannot clearly distinguish between proliferation and migration and partly neglect the MACC1-induced effects on proliferation.
Despite the high effort put into elucidating MACC1-induced effects, several questions regarding its promigratory effects remain open. Downstream targets mediating the currently discovered effects need to be analyzed more precisely, as by now mostly the major signaling routes are identified. As MACC1 was shown to affect cortical tension and some of its downstream targets affect key components of the actin cortex, the question arises if and how MACC1 affects blebbing and thus migration in low adhesive environments. Another question is how 3D migration is altered in MACC1 dependence, under different amounts of confinement, in matrices of varying stiffness and composition. Furthermore, it also appears necessary to elucidate the MACC1-dependent effects on changes in the adhesive system, currently summarized under the broad term EMT, as they largely determine collective migration, a key factor in tumor invasion. This includes substrate dependence of cell–matrix adhesion formation, the strength of cell–cell and cell–matrix adhesions, and consequences on biomechanical properties and collective migration. Further experiments should clarify whether MACC1 additionally affects heterotypic cell–cell interactions with stromal cells and what the consequences are.
Currently, several drugs are under clinical trials which have at least a partial inhibitory effect on cell migration, potentially affecting metastasis formation (234). Given the clear association between MACC1 and poor survival and increased metastasis in so many tumor entities and its tight relation to cell migration and the cytoskeletal or adhesive system, it is likely that targeting MACC1 and analyzing its signal cascades will help to better understand the metastatic processes and develop precise tools to interact with tumor progression.
Author contributions
TH, UH and FD contributed to the writing and review of the manuscript. All authors contributed to the article and approved the submitted version.
Funding
T.H. was supported by the funding program Open Access Publishing by the German Research Foundation (DFG).
Conflict of interest
The authors declare that the research was conducted in the absence of any commercial or financial relationships that could be construed as a potential conflict of interest.
Publisher’s note
All claims expressed in this article are solely those of the authors and do not necessarily represent those of their affiliated organizations, or those of the publisher, the editors and the reviewers. Any product that may be evaluated in this article, or claim that may be made by its manufacturer, is not guaranteed or endorsed by the publisher.
References
1. Ferlay J, Colombet M, Soerjomataram I, Parkin DM, Piñeros M, Znaor A, et al. Cancer statistics for the year 2020: An overview. Int J Cancer (2021) 149:778–89. doi: 10.1002/ijc.33588
2. Stein U, Walther W, Arlt F, Schwabe H, Smith J, Fichtner I, et al. MACC1, a newly identified key regulator of HGF-MET signaling, predicts colon cancer metastasis. Nat Med (2009) 15:59–67. doi: 10.1038/nm.1889
3. Radhakrishnan H, Walther W, Zincke F, Kobelt D, Imbastari F, Erdem M, et al. MACC1–the first decade of a key metastasis molecule from gene discovery to clinical translation. Cancer Metastasis Rev (2019) 37:805–20. doi: 10.1007/s10555-018-9771-8
4. Stein U, Burock S, Herrmann P, Wendler I, Niederstrasser M, Wernecke K-D, et al. Circulating MACC1 transcripts in colorectal cancer patient plasma predict metastasis and prognosis. PloS One (2012) 7:e49249. doi: 10.1371/journal.pone.0049249
5. Wang G, Kang MX, Lu WJ, Chen Y, Zhang B, Wu YL. MACC1: A potential molecule associated with pancreatic cancer metastasis and chemoresistance. Oncol Lett (2012) 4:783–91. doi: 10.3892/ol.2012.784
6. Burock S, Herrmann P, Wendler I, Niederstrasser M, Wernecke KD, Stein U. Circulating metastasis associated in colon cancer 1 transcripts in gastric cancer patient plasma as diagnostic and prognostic biomarker. World J Gastroenterol (2015) 21:333–41. doi: 10.3748/wjg.v21.i1.333
7. Wang Z, Cai M, Weng Y, Zhang F, Meng D, Song J, et al. Circulating MACC1 as a novel diagnostic and prognostic biomarker for nonsmall cell lung cancer. J Cancer Res Clin Oncol (2015) 141:1353–61. doi: 10.1007/s00432-014-1903-0
8. Tan W, Xie X, Li L, Tang H, Ye X, Chen L, et al. Diagnostic and prognostic value of serum MACC1 in breast cancer patients. Oncotarget (2016) 7:84408–15. doi: 10.18632/oncotarget.12910
9. Ashktorab H, Hermann P, Nouraie M, Shokrani B, Lee E, Haidary T, et al. Increased MACC1 levels in tissues and blood identify colon adenoma patients at high risk. J Transl Med (2016) 14:1–9. doi: 10.1186/s12967-016-0971-0
10. Lemos C, Hardt MS, Juneja M, Voss C, Forster S, Jerchow B, et al. MACC1 induces tumor progression in transgenic mice and colorectal cancer patients. via Increased Pluripotency Markers Nanog Oct4. Clin Cancer Res (2016) 22:2812–24. doi: 10.1158/1078-0432.CCR-15-1425
11. Wang L, Lin L, Chen X, Sun L, Liao Y, Huang N, et al. Metastasis-associated in colon cancer-1 promotes vasculogenic mimicry in gastric cancer by upregulating TWIST1/2. Oncotarget (2015) 6:11492–506. doi: 10.18632/oncotarget.3416
12. Hagemann C, Fuchs S, Monoranu CM, Herrmann P, Smith J, Hohmann T, et al. Impact OfMACC1 on human malignant glioma progression and patients’ unfavorable prognosis. Neuro. Oncol (2013) 15:1696–709. doi: 10.1093/neuonc/not136
13. Hohmann T, Hohmann U, Kolbe MR, Dahlmann M, Kobelt D, Stein U. MACC1 driven alterations in cellular biomechanics facilitate cell motility in glioblastoma. Cell Commun Signal (2020) 18:1–13. doi: 10.1186/s12964-020-00566-1
14. Bravo-Cordero JJ, Hodgson L, Condeelis J. Directed cell invasion and migration during metastasis Jose. Curr Opin Cell Biol (2012) 24:077–283. doi: 10.1016/j.ceb.2011.12.004
15. Eble JA, Niland S. The extracellular matrix in tumor progression and metastasis. Clin Exp Metastasis (2019) 36:171–98. doi: 10.1007/s10585-019-09966-1
16. Norden C, Lecaudey V. Collective cell migration: General themes and new paradigms. Curr Opin Genet Dev (2019) 57:54–60. doi: 10.1016/j.gde.2019.06.013
17. Hohmann T, Dehghani F. The cytoskeleton–a complex interacting meshwork. Cells (2019) 8(4):362. doi: 10.3390/cells8040362
18. Aseervatham J. Cytoskeletal remodeling in cancer. Biol (Basel). (2020) 9:1–40. doi: 10.3390/biology9110385
19. Svitkina T. The actin cytoskeleton and actin-based motility. Cold Spring Harb. Perspect Biol (2018) 10:1–21. doi: 10.1101/cshperspect.a018267
20. Venhuizen JH, Zegers MM. Making heads or tails of it: Cell–cell adhesion in cellular and supracellular polarity in collective migration. Cold Spring Harb. Perspect Biol (2017) 9. doi: 10.1101/cshperspect.a027854
21. Venhuizen JH, Jacobs FJC, Span PN, Zegers MM. P120 and e-cadherin: Double-edged swords in tumor metastasis. Semin Cancer Biol (2020) 60:107–20. doi: 10.1016/j.semcancer.2019.07.020
22. Khalil AA, de Rooij J. Cadherin mechanotransduction in leader-follower cell specification during collective migration. Exp Cell Res (2019) 376:86–91. doi: 10.1016/j.yexcr.2019.01.006
23. Hamidi H, Ivaska J. Every step of the way: Integrins in cancer progression and metastasis. Nat Rev Cancer (2018) 18:533–48. doi: 10.1038/s41568-018-0038-z
24. Conway JRW, Jacquemet G. Cell matrix adhesion in cell migration. Essays Biochem (2019) 63:535–51. doi: 10.1042/EBC20190012
25. Datta A, Deng S, Gopal V, Yap KCH, Halim CE, Lye ML, et al. Cytoskeletal dynamics in epithelial-mesenchymal transition: Insights into therapeutic targets for cancer metastasis. Cancers (Basel). (2021) 13:1–27. doi: 10.3390/cancers13081882
26. DeWane G, Salvi AM, DeMali KA. Fueling the cytoskeleton-links between cell metabolism and actin remodeling. J Cell Sci (2021) 134. doi: 10.1242/jcs.248385
27. Garcin C, Straube A. Microtubules in cell migration. Essays Biochem (2019) 63:509–20. doi: 10.1042/EBC20190016
28. Janiszewska M, Primi MC, Izard T. Cell adhesion in cancer: Beyond the migration of single cells. J Biol Chem (2020) 295:2495–505. doi: 10.1074/jbc.REV119.007759
29. Kaszak I, Witkowska-Piłaszewicz O, Niewiadomska Z, Dworecka-Kaszak B, Toka FN, Jurka P. Role of cadherins in cancer–a review. Int J Mol Sci (2020) 21:1–17. doi: 10.3390/ijms21207624
30. Ruggiero C, Lalli E. Targeting the cytoskeleton against metastatic dissemination. Cancer Metastasis Rev (2021) 40:89–140. doi: 10.1007/s10555-020-09936-0
31. Seetharaman S, Etienne-Manneville S. Cytoskeletal crosstalk in cell migration. Trends Cell Biol (2020) 30:720–35. doi: 10.1016/j.tcb.2020.06.004
32. Bai L, Gao Z, Jiang A, Ren S, Wang B. Circ_0101802 functions as a sponge of MiR-1236-3p to facilitate the proliferation, migration and invasion of colorectal cancer via regulating MACC1. J Pharmacol Sci (2021) 147:104–13. doi: 10.1016/j.jphs.2021.06.002
33. Huang N, Wu Z, Lin L, Zhou M, Wang L, Ma H, et al. MiR-338-3p inhibits epithelial-mesenchymal transition in gastric cancer cells by targeting ZEB2 and MACC1/Met/Akt signaling. Oncotarget (2015) 6:15222–34. doi: 10.18632/oncotarget.3835
34. Wang L, Wu Y, Lin L, Liu P, Huang H, Liao W, et al. Metastasis-associated in colon cancer-1 upregulation predicts a poor prognosis of gastric cancer, and promotes tumor cell proliferation and invasion. Int J cancer. (2013) 133:1419–30. doi: 10.1002/ijc.28140
35. Yang T, He W, Cui F, Xia J, Zhou R, Wu Z, et al. MACC1 mediates acetylcholine-induced invasion and migration by human gastric cancer cells. Oncotarget (2016) 7:18085–94. doi: 10.18632/oncotarget.7634
36. Petrie RJ, Yamada KM. At The leading edge of three-dimensional cell migration. J Cell Sci (2012) 125:5917–26. doi: 10.1242/jcs.093732
37. Papalazarou V, Machesky LM. The cell pushes back: The Arp2/3 complex is a key orchestrator of cellular responses to environmental forces. Curr Opin Cell Biol (2021) 68:37–44. doi: 10.1016/j.ceb.2020.08.012
38. Krause M, Gautreau A. Steering cell migration: Lamellipodium dynamics and the regulation of directional persistence. Nat Rev Mol Cell Biol (2014) 15:577–90. doi: 10.1038/nrm3861
39. Tang H, Li A, Bi J, Veltman DM, Zech T, Spence HJ, et al. Loss of Scar/WAVE complex promotes n-WASP- and FAK-dependent invasion. Curr Biol (2013) 23:107–17. doi: 10.1016/j.cub.2012.11.059
40. Petrie RJ, Gavara N, Chadwick RS, Yamada KM. Nonpolarized signaling reveals two distinct modes of 3D cell migration. J Cell Biol (2012) 197:439–55. doi: 10.1083/jcb.201201124
41. Petrie RJ, Doyle AD, Yamada KM. Random versus directionally persistent cell migration Ryan. Nat Rev Mol Cell Biol (2009) 10:538–49. doi: 10.1038/nrm2729.Random
42. Gardel ML, Schneider IC, Aratyn-Schaus Y, Waterman CM. Mechanical integration of actin and adhesion dynamics in cell migration. Annu Rev Cell Dev Biol (2010) 176:315–33. doi: 10.1146/annurev.cellbio.011209.122036.Mechanical
43. Naumanen P, Lappalainen P, Hotulainen P. Mechanisms of actin stress fibre assembly. J Microsc. (2008) 231:446–54. doi: 10.1111/j.1365-2818.2008.02057.x
44. Koenderink GH, Paluch EK. Architecture shapes contractility in actomyosin networks. Curr Opin Cell Biol (2018) 50:79–85. doi: 10.1016/j.ceb.2018.01.015
45. Garrido-Casado M, Asensio-Juárez G, Vicente-Manzanares M. Nonmuscle myosin II regulation directs its multiple roles in cell migration and division. Annu Rev Cell Dev Biol (2021) 37:285–310. doi: 10.1146/annurev-cellbio-042721-105528
46. Leung T, Chen XQ, Manser E, Lim L. The P160 RhoA-binding kinase ROK alpha is a member of a kinase family and is involved in the reorganization of the cytoskeleton. Mol Cell Biol (1996) 16:5313–27. doi: 10.1128/MCB.16.10.5313
47. Watanabe N, Madaule P, Reid T, Ishizaki T, Watanabe G, Kakizuka A, et al. P140mDia, a mammalian homolog of drosophila diaphanous, is a target protein for rho small GTPase and is a ligand for profilin. EMBO J (1997) 16:3044–56. doi: 10.1093/emboj/16.11.3044
48. Castella LF, Buscemi L, Godbout C, Meister J-J, Hinz BA. New lock-step mechanism of matrix remodelling based on subcellular contractile events. J Cell Sci (2010) 123:1751–60. doi: 10.1242/jcs.066795
49. Kirfel G, Rigort A, Borm B, Herzog V. Cell migration: Mechanisms of rear detachment and the formation of migration tracks. Eur J Cell Biol (2004) 83:717–24. doi: 10.1078/0171-9335-00421
50. Saraswathibhatla A, Notbohm J. Tractions and stress fibers control cell shape and rearrangements in collective cell migration. Phys Rev X (2020) 10:11016. doi: 10.1103/PhysRevX.10.011016
51. Biro M, Romeo Y, Kroschwald S, Bovellan M, Boden A, Tcherkezian J, et al. Cell cortex composition and homeostasis resolved by integrating proteomics and quantitative imaging. Cytoskeleton (2013) 70:741–54. doi: 10.1002/cm.21142
52. Bovellan M, Romeo Y, Biro M, Boden A, Chugh P, Yonis A, et al. Cellular control of cortical actin nucleation. Curr Biol (2014) 24:1628–35. doi: 10.1016/j.cub.2014.05.069
53. Sroka J, von Gunten M, Dunn GA, Keller HU. Phenotype modulation in non-adherent and adherent sublines of walker carcinosarcoma cells: The role of cell-substratum contacts and microtubules in controlling cell shape, locomotion and cytoskeletal structure. Int J Biochem Cell Biol (2002) 34:882–99. doi: 10.1016/s1357-2725(01)00178-9
54. Blaser H, Eisenbeiss S, Neumann M, Reichman-Fried M, Thisse B, Thisse C, et al. Transition from non-motile behaviour to directed migration during early PGC development in zebrafish. J Cell Sci (2005) 118:4027–38. doi: 10.1242/jcs.02522
55. Trinkaus JP, Lentz TL. Surface specializations of fundulus cells and their relation to cell movements during gastrulation. J Cell Biol (1967) 32:139–53. doi: 10.1083/jcb.32.1.139
56. Paluch EK, Raz E. The role and regulation of blebs in cell migration. Curr Opin Cell Biol (2013) 25:582–90. doi: 10.1016/j.ceb.2013.05.005
57. Chugh P, Clark AG, Smith MB, Cassani DAD, Dierkes K, Ragab A, et al. Actin cortex architecture regulates cell surface tension. Nat Cell Biol (2017) 19:689–97. doi: 10.1038/ncb3525
58. Tinevez J-Y, Schulze U, Salbreux G, Roensch J, Joanny J-F, Paluch E. Role of cortical tension in bleb growth. Proc Natl Acad Sci (2009) 106:18581–6. doi: 10.1073/pnas.0903353106
59. Chugh P, Paluch EK. The actin cortex at a glance. J Cell Sci (2018) 131:jcs186254. doi: 10.1242/jcs.186254
60. Brangwynne CP, MacKintosh FC, Kumar S, Geisse NA, Talbot J, Mahadevan L, et al. Microtubules can bear enhanced compressive loads in living cells because of lateral reinforcement. J Cell Biol (2006) 173:733–41. doi: 10.1083/jcb.200601060
61. Etienne-Manneville S. Microtubules in cell migration. Annu Rev Cell Dev Biol (2013) 29:471–99. doi: 10.1146/annurev-cellbio-101011-155711
62. Lu W, Fox P, Lakonishok M, Davidson MW, Gelfand VI. Initial neurite outgrowth in drosophila neurons is driven by kinesin-powered microtubule sliding. Curr Biol (2013) 23:1018–23. doi: 10.1016/j.cub.2013.04.050
63. Bouchet BP, Noordstra I, van Amersfoort M, Katrukha EA, Ammon YC, ter Hoeve ND, et al. Mesenchymal cell invasion requires cooperative regulation of persistent microtubule growth by SLAIN2 and CLASP1. Dev Cell (2016) 39:708–23. doi: 10.1016/j.devcel.2016.11.009
64. Johnsson AK, Karlsson R. Microtubule-dependent localization of profilin I MRNA to actin polymerization sites in serum-stimulated cells. Eur J Cell Biol (2010) 89:394–401. doi: 10.1016/j.ejcb.2009.10.020
65. Mingle LA. Localization of all seven messenger RNAs for the actin-polymerization nucleator Arp2/3 complex in the protrusions of fibroblasts. J Cell Sci (2005) 118:2425–33. doi: 10.1242/jcs.02371
66. Oleynikov Y, Singer RH. RNA Localization: Different zipcodes, same postman? Trends Cell Biol (1998) 8:381–3. doi: 10.1016/S0962-8924(98)01348-8
67. Usman S, Waseem NH, Nguyen TKN, Mohsin S, Jamal A, Teh MT, et al. Vimentin is at the heart of epithelial mesenchymal transition (Emt) mediated metastasis. Cancers (Basel). (2021) 13:1–26. doi: 10.3390/cancers13194985
68. Battaglia RA, Delic S, Herrmann H, Snider NT. Vimentin on the Move : New developments in cell migration. F1000Research (2018) 7:1–10. doi: 10.12688/f1000research.15967.1
69. Gan Z, Ding L, Burckhardt CJ, Lowery J, Zaritsky A, Sitterley K, et al. Vimentin intermediate filaments template microtubule networks to enhance persistence in cell polarity and directed migration. Cell Syst (2016) 3:252–263.e8. doi: 10.1016/j.cels.2016.08.007
70. Esue O, Carson AA, Tseng Y, Wirtz DA. Direct interaction between actin and vimentin filaments mediated by the tail domain of vimentin. J Biol Chem (2006) 281:30393–9. doi: 10.1074/jbc.M605452200
71. Svitkina TM, Verkhovsky AB, Borisy GB. Plectin sidearms mediate interactions of intermediate filaments with microtubules and other components of the cytoskeleton. Biol Bull (1998) 194:409–10. doi: 10.2307/1543127
72. Gregor M, Osmanagic-Myers S, Burgstaller G, Wolfram M, Fischer I, Walko G, et al. Mechanosensing through focal adhesion-anchored intermediate filaments. FASEB J (2014) 28:715–29. doi: 10.1096/fj.13-231829
73. Jiu Y, Peränen J, Schaible N, Cheng F, Eriksson JE, Krishnan R, et al. Vimentin intermediate filaments control actin stress fiber assembly through GEF-H1 and RhoA. J Cell Sci (2017) 130:892–902. doi: 10.1242/jcs.196881
74. De Pascalis C, Pérez-González C, Seetharaman S, Boëda B, Vianay B, Burute M, et al. Intermediate filaments control collective migration by restricting traction forces and sustaining cell-cell contacts. J Cell Biol (2018) 217:3031–44. doi: 10.1083/jcb.201801162
75. Petrie RJ, Koo H, Yamada KM. Generation of compartmentalized pressure by a nuclear piston governs cell motility in a 3D matrix. Science (2014) 345:1062–5. doi: 10.1126/science.1256965
76. Gundersen GG, Worman HJ. Nuclear positioning. Cell (2013) 152:1376–89. doi: 10.1016/j.cell.2013.02.031
77. Mendez MG, Restle D, Janmey PA. Vimentin enhances cell elastic behavior and protects against compressive stress. Biophys J (2014) 107:314–23. doi: 10.1016/j.bpj.2014.04.050
78. Tse JM, Cheng G, Tyrrell JA, Wilcox-Adelman SA, Boucher Y, Jain RK, et al. Mechanical compression drives cancer cells toward invasive phenotype. Proc Natl Acad Sci U. S. A. (2012) 109:911–6. doi: 10.1073/pnas.1118910109
79. Messica Y, Laser-Azogui A, Volberg T, Elisha Y, Lysakovskaia K, Eils R, et al. The role of vimentin in regulating cell invasive migration in dense cultures of breast carcinoma cells. Nano Lett (2017) 17:6941–8. doi: 10.1021/acs.nanolett.7b03358
80. Kanchanawong P, Shtengel G, Pasapera AM, Ramko EB, Davidson MW, Hess HF, et al. Nanoscale architecture of integrin-based cell adhesions. Nature (2010) 468:580–4. doi: 10.1038/nature09621
81. Case LB, Baird MA, Shtengel G, Campbell SL, Hess HF, Davidson MW, et al. Molecular mechanism of vinculin activation and nanoscale spatial organization in focal adhesions. Nat Cell Biol (2015) 17:880–92. doi: 10.1038/ncb3180
82. Liu J, Wang Y, Goh WI, Goh H, Baird MA, Ruehland S, et al. Talin determines the nanoscale architecture of focal adhesions. Proc Natl Acad Sci U. S. A. (2015) 112:E4864–73. doi: 10.1073/pnas.1512025112
83. Stubb A, Guzmán C, Närvä E, Aaron J, Chew TL, Saari M, et al. Superresolution architecture of cornerstone focal adhesions in human pluripotent stem cells. Nat Commun (2019) 10. doi: 10.1038/s41467-019-12611-w
84. Horton ER, Byron A, Askari JA, Ng DHJ, Millon-Frémillon A, Robertson J, et al. Definition of a consensus integrin adhesome and its dynamics during adhesion complex assembly and disassembly. Nat Cell Biol (2015) 17:1577–87. doi: 10.1038/ncb3257
85. Charras G, Sahai E. Physical influences of the extracellular environment on cell migration. Nat Rev Mol Cell Biol (2014) 15:813–24. doi: 10.1038/nrm3897
86. Cho S, Irianto J, Discher DE. Mechanosensing by the Nucleus : From pathways to scaling relationships. J Cell Biol (2017) 216:1–11. doi: 10.1083/jcb.201610042
87. Zhao X, Guan JL. Focal adhesion kinase and its signaling pathways in cell migration and angiogenesis. Adv Drug Deliv. Rev (2011) 63:610–5. doi: 10.1016/j.addr.2010.11.001
88. Gu Z, Noss EH, Hsu VW, Brenner MB. Integrins traffic rapidly via circular dorsal ruffles and macropinocytosis during stimulated cell migration. J Cell Biol (2011) 193:61–70. doi: 10.1083/jcb.201007003
89. Theisen U, Straube E, Straube A. Directional persistence of migrating cells requires Kif1C-mediated stabilization of trailing adhesions. Dev Cell (2012) 23:1153–66. doi: 10.1016/j.devcel.2012.11.005
90. Danielsson F, Peterson MK, Araújo HC, Lautenschläger F, Gad AKB. Vimentin diversity in health and disease. Cells (2018) 7:1–38. doi: 10.3390/cells7100147
91. Kreis S, Schönfeld H-J, Melchior C, Steiner B, Kieffer N. The intermediate filament protein vimentin binds specifically to a recombinant integrin α2/β1 cytoplasmic tail complex and Co-localizes with native α2/β1 in endothelial cell focal adhesions. Exp Cell Res (2005) 305:110–21. doi: 10.1016/J.YEXCR.2004.12.023
92. Kim J, Yang C, Kim EJ, Jang J, Kim S-J, Kang SM, et al. Vimentin filaments regulate integrin–ligand interactions by binding to the cytoplasmic tail of integrin β3. J Cell Sci (2016) 129:2030–42. doi: 10.1242/jcs.180315
93. Terriac E, Coceano G, Mavajian Z, Hageman TAG, Christ AF, Testa I, et al. Vimentin levels and serine 71 phosphorylation in the control of cell-matrix adhesions, migration speed, and shape of transformed human fibroblasts. Cells (2017) 6:1–13. doi: 10.3390/cells6010002
94. Fortin S, Le Mercier M, Camby I, Spiegl-Kreinecker S, Berger W, Lefranc F, et al. Galectin-1 is implicated in the protein kinase c ϵ/Vimentin-controlled trafficking of integrin-β1 in glioblastoma cells. Brain Pathol (2010) 20:39–49. doi: 10.1111/j.1750-3639.2008.00227.x
95. Pannekoek W-J, De Rooij J, Gloerich M. Open peer review force transduction by cadherin adhesions in morphogenesis. F1000Res (2019) 8:1–14. doi: 10.12688/f1000research.18779.1
96. Yonemura S, Wada Y, Watanabe T, Nagafuchi A, Shibata M. α-catenin as a tension transducer that induces adherens junction development. Nat Cell Biol (2010) 12:533–42. doi: 10.1038/ncb2055
97. Huveneers S, Oldenburg J, Spanjaard E, van der Krogt G, Grigoriev I, Akhmanova A, et al. Vinculin associates with endothelial VE-cadherin junctions to control force-dependent remodeling. J Cell Biol (2012) 196:641–52. doi: 10.1083/jcb.201108120
98. Leckband DE, de Rooij J. Cadherin adhesion and mechanotransduction. Annu Rev Cell Dev Biol (2014) 30:291–315. doi: 10.1146/annurev-cellbio-100913-013212
99. Drees F, Pokutta S, Yamada S, Nelson WJ, Weis WI. α-catenin is a molecular switch that binds e-Cadherin-β-Catenin and regulates actin-filament assembly. Cell (2005) 123:903–15. doi: 10.1016/j.cell.2005.09.021
100. Timmerman I, Heemskerk N, Kroon J, Schaefer A, van Rijssel J, Hoogenboezem M, et al. Correction to “A local VE-cadherin and trio-based signaling complex stabilizes endothelial junctions through Rac1”. J Cell Sci (2015) 128:3041–54. doi: 10.1242/jcs.179424
101. Toret CP, Collins C, Nelson WJ. An elmo-dock complex locally controls rho gtpases and actin remodeling during cadherin-mediated adhesion. J Cell Biol (2014) 207:577–87. doi: 10.1083/jcb.201406135
102. Muhamed I, Wu J, Sehgal P, Kong X, Tajik A, Wang N. Leckband, D.E. e-Cadherin-Mediated force transduction signals regulate global cell mechanics. J Cell Sci (2016) 129:1843–54. doi: 10.1242/jcs.185447
103. Plutoni C, Bazellieres E, Le Borgne-Rochet M, Comunale F, Brugues A, Séveno M, et al. P-cadherin promotes collective cell migration via a Cdc42-mediated increase in mechanical forces. J Cell Biol (2016) 212:199–217. doi: 10.1083/jcb.201505105
104. Loh CY, Chai JY, Tang TF, Wong WF, Sethi G, Shanmugam MK, et al. The e-cadherin and n-cadherin switch in epithelial-to-Mesenchymal transition: Signaling, therapeutic implications, and challenges. Cells (2019) 8. doi: 10.3390/cells8101118
105. Blauth E, Kubitschke H, Gottheil P, Grosser S, Käs JA. Jamming in embryogenesis and cancer progression. Front Phys (2021) 9:666709. doi: 10.3389/fphy.2021.666709
106. Park JA, Kim JH, Bi D, Mitchel JA, Qazvini NT, Tantisira K, et al. Unjamming and cell shape in the asthmatic airway epithelium. Nat Mater (2015) 14:1040–8. doi: 10.1038/nmat4357
107. Bazellières E, Conte V, Elosegui-Artola A, Serra-Picamal X, Bintanel-Morcillo M, Roca-Cusachs P, et al. Control of cell-cell forces and collective cell dynamics by the intercellular adhesome. Nat Cell Biol (2015) 17:409–20. doi: 10.1038/ncb3135
108. Weber GF, Bjerke MA, DeSimone DWA. Mechanoresponsive cadherin-keratin complex directs polarized protrusive behavior and collective cell migration. Dev Cell (2012) 22:104–15. doi: 10.1016/j.devcel.2011.10.013
109. Mary S, Charrasse S, Meriane M, Comunale F, Travo P, Blangy A, et al. Biogenesis of n-Cadherin-Dependent cell-cell contacts in living fibroblasts is a microtubule-dependent kinesin-driven mechanism. Mol Biol Cell (2002) 13:285–301. doi: 10.1091/mbc.01-07-0337
110. Chen X, Kojima SI, Borisy GG, Green KJ. P120 catenin associates with kinesin and facilitates the transport of cadherin-catenin complexes to intercellular junctions. J Cell Biol (2003) 163:547–57. doi: 10.1083/jcb.200305137
111. Borghi N, Lowndes M, Maruthamuthu V, Gardel ML, Nelson WJ. Regulation of cell motile behavior by crosstalk between cadherin- and integrin-mediated adhesions. Proc Natl Acad Sci U. S. A. (2010) 107:13324–9. doi: 10.1073/pnas.1002662107
112. Gimond C, van der Flier A, Van Delft S, Brakebusch C, Kuikman I, Collard JG, et al. Induction of cell scattering by expression of β1 integrins in β1- deficient epithelial cells requires activation of members of the rho family of GTPases and downregulation of cadherin and catenin function. J Cell Biol (1999) 147:1325–40. doi: 10.1083/jcb.147.6.1325
113. Zhang H, Hou R, Xiao P, Xing R, Chen T, Han Y, et al. Single cell migration dynamics mediated by geometric confinement. Colloids Surfaces B Biointerfaces (2016) 145:72–8. doi: 10.1016/j.colsurfb.2016.04.039
114. Schreiber C, Segerer FJ, Wagner E, Roidl A, Rädler JO. Ring-shaped microlanes and chemical barriers as a platform for probing single-cell migration. Sci Rep (2016) 6:1–9. doi: 10.1038/srep26858
115. Doyle AD, Wang FW, Matsumoto K, Yamada KM. One-dimensional topography underlies three-dimensional Fi brillar cell migration. J Cell Biol (2009) 184:481–90. doi: 10.1083/jcb.200810041
116. Desai RA, Gopal SB, Chen S, Chen CS. Contact inhibition of locomotion probabilities drive solitary versus collective cell migration. J R Soc Interface (2013) 10. doi: 10.1098/rsif.2013.0717
117. Pathak A, Kumar S. Independent regulation of tumor cell migration by matrix stiffness and confinement. Proc Natl Acad Sci U. S. A. (2012) 109:10334–9. doi: 10.1073/pnas.1118073109
118. Sentoku M, Hashimoto H, Iida K, Endo M, Yasuda K. Photothermal agarose microfabrication technology for collective cell migration analysis. Micromachines (2021) 12:1–17. doi: 10.3390/mi12091015
119. Doyle AD, Petrie RJ, Kutys ML, Yamada KM. Dimensions in cell migration. Curr Opin Cell Biol (2013) 25:642–9. doi: 10.1016/j.ceb.2013.06.004.Dimensions
120. Sala F, Ficorella C, Osellame R, Käs JA, Martínez Vázquez R. Microfluidic Lab-on-a-Chip for studies of cell migration under spatial confinement. Biosensors (2022) 12:1–19. doi: 10.3390/bios12080604
121. Masuzzo P, Huyck L, Simiczyjew A, Ampe C, Martens L, Van Troys M. An end-to-End software solution for the analysis of high-throughput single-cell migration data. Sci Rep (2017) 7:1–13. doi: 10.1038/srep42383
122. Hohmann T, Grabiec U, Ghadban C, Feese K, Dehghani F. The influence of biomechanical properties and cannabinoids on tumor invasion. Cell Adh. Migr. (2017) 11:54–67. doi: 10.1080/19336918.2016.1183867
123. Maddalena L, Antonelli L, Albu A, Hada A, Guarracino MR. Artificial intelligence for cell segmentation, event detection, and tracking for label-free microscopy imaging. Algorithms (2022) 15:1–22. doi: 10.3390/a15090313
124. Ronneberger O, Fischer P. Brox, t. U-net: Convolutional networks for biomedical image segmentation. Lect. Notes Comput Sci (2015), 234–41. doi: 10.1007/978-3-319-24574-4
125. Tamada M, Perez TD, Nelson WJ, Sheetz MP. Two distinct modes of myosin assembly and dynamics during epithelial wound closure. J Cell Biol (2007) 176:27–33. doi: 10.1083/jcb.200609116
126. Keese CR, Wegener J, Walker SR, Giaever I. Electrical wound-healing assay for cells in vitro. Proc Natl Acad Sci U. S. A. (2004) 101:1554–9. doi: 10.1073/pnas.0307588100
127. Rodriguez LG, Wu X, Guan JL. Wound-healing assay. Methods Mol Biol (2005) 294:23–9. doi: 10.1385/1-59259-860-9:023
128. Juneja M, Kobelt D, Walther W, Voss C, Smith J, Specker E, et al. Statin and rottlerin small-molecule inhibitors restrict colon cancer progression and metastasis. via MACC1. PloS Biol (2017) 15:1–28. doi: 10.1371/journal.pbio.2000784
129. Nnetu KD, Knorr M, Käs J, Zink M. The impact of jamming on boundaries of collectively moving weak-interacting cells. New J Phys (2012) 14. doi: 10.1088/1367-2630/14/11/115012
130. Chepizhko O, Lionetti MC, Malinverno C, Giampietro C, Scita G, Zapperi S, et al. From jamming to collective cell migration through a boundary induced transition. Soft Matter (2018) 14:3774–82. doi: 10.1039/c8sm00128f
131. Vishwakarma M, Di Russo J, Probst D, Schwarz US, Das T, Spatz JP. Mechanical interactions among followers determine the emergence of leaders in migrating epithelial cell collectives. Nat Commun (2018) 9. doi: 10.1038/s41467-018-05927-6
132. Hohmann U, von Widdern JC, Ghadban C, Giudice MC. Lo; lemahieu, g.; cavalcanti-Adam, E.A.; dehghani, f.; hohmann, t. jamming transitions in astrocytes and glioblastoma are induced by cell density and tension. Cells (2023) 12:1–23. doi: 10.3390/cells12010029
133. Kam Y, Guess C, Estrada L, Weidow B, Quaranta VA. Novel circular invasion assay mimics in vivo invasive behavior of cancer cell lines and distinguishes single-cell motility in vitro. BMC Cancer (2008) 8:1–12. doi: 10.1186/1471-2407-8-198
134. Staton CA, Reed MWR, Brown NJA. Critical analysis of current in vitro and in vivo angiogenesis assays. Int J Exp Pathol (2009) 90:195–221. doi: 10.1111/j.1365-2613.2008.00633.x
135. Hulkower KI, Herber RL. Cell migration and invasion assays as tools for drug discovery. Pharmaceutics (2011) 3:107–24. doi: 10.3390/pharmaceutics3010107
136. Kramer N, Walzl A, Unger C, Rosner M, Krupitza G, Hengstschläger M, et al. In vitro cell migration and invasion assays. Mutat Res - Rev Mutat Res (2013) 752:10–24. doi: 10.1016/j.mrrev.2012.08.001
137. Entschladen F, Drell TL IV, Lang K, Masur K, Palm D, Bastian P, et al. Analysis methods of human cell migration. Exp Cell Res (2005) 307:418–26. doi: 10.1016/j.yexcr.2005.03.029
138. Huh S, Eom S, Ker DFE, Weiss L, Kanade T. Mitosis detection of hematopoietic stem cell populations in time-lapse phase-contrast microscopy images. Proc - Int Symp Biomed Imaging (2012) 30:390–3. doi: 10.1109/ISBI.2012.6235566
139. Thirusittampalam K, Hossain MJ, Ghita O, Whelan PFA. Novel framework for cellular tracking and mitosis detection in dense phase contrast microscopy images. IEEE J Biomed Heal Inf (2013) 17:642–53. doi: 10.1109/TITB.2012.2228663
140. Su YT, Lu Y, Liu J, Chen M, Liu AA. Spatio-temporal mitosis detection in time-lapse phase-contrast microscopy image sequences: A benchmark. IEEE Trans Med Imaging (2021) 40:1319–28. doi: 10.1109/TMI.2021.3052854
141. Grah JS, Harrington JA, Koh SB, Pike JA, Schreiner A, Burger M, et al. Mathematical imaging methods for mitosis analysis in live-cell phase contrast microscopy. Methods (2017) 115:91–9. doi: 10.1016/j.ymeth.2017.02.001
142. Hohmann T, Hohmann U, Dahlmann M, Kobelt D, Stein U, Dehghani F. MACC1-induced collective migration is promoted by proliferation rather than single cell biomechanics. Cancers (Basel). (2022) 14:2857. doi: 10.3390/cancers14122857
143. Basti A, Malhan D, Dumbani M, Dahlmann M, Stein U, Relógio A. Core-clock genes regulate proliferation and invasion via a reciprocal interplay with MACC1 in colorectal cancer cells. Cancers (Basel). (2022) 14:1–20. doi: 10.3390/cancers14143458
144. Treese C, Werchan J, von Winterfeld M, Berg E, Hummel M, Timm L, et al. Inhibition of MACC1-induced metastasis in esophageal and gastric adenocarcinomas. Cancers (Basel). (2022) 14:1–16. doi: 10.3390/cancers14071773
145. Li Z, Guo T, Fang L, Li N, Wang X, Wang P, et al. MACC1 overexpression in carcinoma-associated fibroblasts induces the invasion of lung adenocarcinoma cells via paracrine signalling. Int J Oncol (2019) 54:1367–75. doi: 10.3892/ijo.2019.4702
146. Calero-Cuenca FJ, Janota CS, Gomes ER. Dealing with the nucleus during cell migration. Curr Opin Cell Biol (2018) 50:35–41. doi: 10.1016/j.ceb.2018.01.014
147. Wolf K, te Lindert M, Krause M, Alexander S, te Riet J, Willis AL, et al. Physical limits of cell migration: Control by ECM space and nuclear deformation and tuning by proteolysis and traction force. J Cell Biol (2013) 201:1069–84. doi: 10.1083/jcb.201210152
148. Ferreira MM, Dewi RE, Heilshorn SC. Microfluidic analysis of extracellular matrix-BFGF crosstalk on primary human myoblast chemoproliferation, chemokinesis, and chemotaxis. Integr Biol (United Kingdom) (2015) 7:569–79. doi: 10.1039/c5ib00060b
149. Eccles SA, Box C, Court W. Cell Migration/Invasion assays and their application in cancer drug discovery. Biotechnol Annu Rev (2005) 11:391–421. doi: 10.1016/S1387-2656(05)11013-8
150. Fuller D, Chen W, Adler M, Groisman A, Levine H, Rappel WJ, et al. External and internal constraints on eukaryotic chemotaxis. Proc Natl Acad Sci U. S. A. (2010) 107:9656–9. doi: 10.1073/pnas.0911178107
151. Isbister CM, Mackenzie PJ, To KCW, O’Connor TP. Gradient steepness influences the pathfinding decisions of neuronal growth cones in vivo. J Neurosci (2003) 23:193–202. doi: 10.1523/jneurosci.23-01-00193.2003
152. Song L, Nadkarni SM, Bödeker HU, Beta C, Bae A, Franck C, et al. Dictyostelium discoideum chemotaxis: Threshold for directed motion. Eur J Cell Biol (2006) 85:981–9. doi: 10.1016/j.ejcb.2006.01.012
153. Zhang C, Barrios MP, Alani RM, Cabodi M, Wong JYA. Microfluidic transwell to study chemotaxis. Exp Cell Res (2016) 342:159–65. doi: 10.1016/j.yexcr.2016.03.010
154. Lee KH, Kim TH. Recent advances in multicellular tumor spheroid generation for drug screening. Biosensors (2021) 11. doi: 10.3390/bios11110445
155. Katt ME, Placone AL, Wong AD, Xu ZS, Searson PC. In vitro tumor models: Advantages, disadvantages, variables, and selecting the right platform. Front Bioeng. Biotechnol (2016) 4:12. doi: 10.3389/fbioe.2016.00012
156. Haeger A, Krause M, Wolf K, Friedl P. Cell jamming: Collective invasion of mesenchymal tumor cells imposed by tissue confinement. Biochim Biophys Acta - Gen Subj. (2014) 1840:2386–95. doi: 10.1016/j.bbagen.2014.03.020
157. Vinci M, Gowan S, Boxall F, Patterson L, Zimmermann M, Court W, et al. Advances in establishment and analysis of three-dimensional tumor spheroid-based functional assays for target validation and drug evaluation. BMC Biol (2012) 10. doi: 10.1186/1741-7007-10-29
158. Becker KN, Pettee KM, Sugrue A, Reinard KA, Schroeder JL, Eisenmann KM. The cytoskeleton effectors rho-kinase (ROCK) and mammalian diaphanous-related (MDia) formin have dynamic roles in tumor microtube formation in invasive glioblastoma cells. Cells (2022) 11. doi: 10.3390/cells11091559
159. Fuhs T, Wetzel F, Fritsch AW, Li X, Stange R, Pawlizak S, et al. Rigid tumours contain soft cancer cells. Nat Phys (2022) 18:1510–9. doi: 10.1038/s41567-022-01755-0
160. Grosser S, Lippoldt J, Oswald L, Merkel M, Sussman DM, Renner F, et al. Cell and nucleus shape as an indicator of tissue fluidity in carcinoma. Phys Rev X (2021) 11:1–24. doi: 10.1103/PhysRevX.11.011033
161. Rodrigues J, Heinrich MA, Teixeira LM, Prakash J. 3D In vitro model (R)Evolution: Unveiling tumor–stroma interactions. Trends Cancer (2021) 7:249–64. doi: 10.1016/j.trecan.2020.10.009
162. Taubenberger AV, Girardo S, Träber N, Fischer-Friedrich E, Kräter M, Wagner K, et al. 3D microenvironment stiffness regulates tumor spheroid growth and mechanics. via P21 ROCK. Adv Biosyst (2019) 3:1–16. doi: 10.1002/adbi.201900128
163. Balzer EM, Tong Z, Paul CD, Hung WC, Stroka KM, Boggs AE, et al. Physical confinement alters tumor cell adhesion and migration phenotypes. FASEB J (2012) 26:4045–56. doi: 10.1096/fj.12-211441
164. Barbazan J, Dunkel Y, Li H, Nitsche U, Janssen KP, Messer K, et al. Prognostic impact of modulators of G proteins in circulating tumor cells from patients with metastatic colorectal cancer. Sci Rep (2016) 6:1–12. doi: 10.1038/srep22112
165. Koelzer VH, Herrmann P, Zlobec I, Karamitopoulou E, Lugli A, Stein U. Heterogeneity analysis of metastasis associated in colon cancer 1 (MACC1) for survival prognosis of colorectal cancer patients: A retrospective cohort study. BMC Cancer (2015) 15:1–11. doi: 10.1186/s12885-015-1150-z
166. Pan T, Chen W, Yuan X, Shen J, Qin C, Wang L. MiR-944 inhibits metastasis of gastric cancer by preventing the epithelial–mesenchymal transition via MACC1/Met/AKT signaling. FEBS Open Bio (2017) 7:905–14. doi: 10.1002/2211-5463.12215
167. Shi W, Song J, Wang W, Zhang Y, Zheng S. MACC-1 antibody target therapy suppresses growth and migration of non-small cell lung cancer. Mol Med Rep (2017) 16:7329–36. doi: 10.3892/mmr.2017.7517
168. Zhang R, Shi H, Chen Z, Wu Q, Ren F, Huang H. Effects of metastasis-associated in colon cancer 1 inhibition by small hairpin RNA on ovarian carcinoma OVCAR-3 cells. J Exp Clin Cancer Res (2011) 30:1–12. doi: 10.1186/1756-9966-30-83
169. Zhen T, Dai S, Li H, Yang Y, Kang L, Shi H, et al. MACC1 promotes carcinogenesis of colorectal cancer via-catenin signaling pathway. Oncotarget (2014) 5:3756–69. doi: 10.18632/oncotarget.1993
170. Zhang C, Li H, Wang J, Zhang J, Hou X. MicroRNA−338−3p suppresses cell proliferation, migration and invasion in human malignant melanoma by targeting MACC1. Exp Ther Med (2019) 18:997–1004. doi: 10.3892/etm.2019.7644
171. Spina A, De Pasquale V, Cerulo G, Cocchiaro P, Della Morte R, Avallone L, et al. HGF/c-MET axis in tumor microenvironment and metastasis formation. Biomedicines (2015) 3:71–88. doi: 10.3390/biomedicines3010071
172. Gherardi E, Birchmeier W, Birchmeier C, Woude G. Vande targeting MET in cancer: Rationale and progress. Nat Rev Cancer (2012) 12:89–103. doi: 10.1038/nrc3205
173. Budczies J, Kluck K, Walther W, Stein U. Decoding and targeting the molecular basis of MACC1-driven metastatic spread: Lessons from big data mining and clinical-experimental approaches. Semin Cancer Biol (2020) 60:365–79. doi: 10.1016/j.semcancer.2019.08.010
174. Organ SL, Tsao M-S. An overview of the c-MET signaling pathway. Ther Adv Med Oncol (2011) 3:S7–S19. doi: 10.1177/1758834011422556
175. Haschemi R, Kobelt D, Steinwarz E, Schlesinger M, Stein U, Bendas G. Insulin-like growth factor binding protein-2 (IGFBP2) is a key molecule in the MACC1-mediated platelet communication and metastasis of colorectal cancer cells. Int J Mol Sci (2021) 22. doi: 10.3390/ijms222212195
176. Perks CM, Vernon EG, Rosendahl AH, Tonge D, Holly JMP. IGF-II and IGFBP-2 differentially regulate PTEN in human breast cancer cells. Oncogene (2007) 26:5966–72. doi: 10.1038/sj.onc.1210397
177. Mehrian-Shai R, Chen CD, Shi T, Horvath S, Nelson SF, Reichardt JKV, et al. Insulin growth factor-binding protein 2 is a candidate biomarker for PTEN status and PI3K/Akt pathway activation in glioblastoma and prostate cancer. Proc Natl Acad Sci U. S. A. (2007) 104:5563–8. doi: 10.1073/pnas.0609139104
178. Qian LQ, Li XQ, Ye PH, Su HY, Wang G, Liu Y, et al. Downregulation of MACC1 inhibits the viability, invasion and migration and induces apoptosis in esophageal carcinoma cells through the phosphatase and tensin Homolog/Phosphoinositide 3-Kinase/Protein kinase b signaling pathway. Oncol Lett (2017) 14:4897–905. doi: 10.3892/ol.2017.6790
179. Zhang YM, Wu QM, Chang LY, Liu JC. MiR-34a and MiR-125a-5p inhibit proliferation and metastasis but induce apoptosis in hepatocellular carcinoma cells via repressing the MACC1- mediated PI3K/AKT/MTOR pathway. Neoplasma (2020) 67:1042–53. doi: 10.4149/neo
180. Meng F, Li H, Shi H, Yang Q, Zhang F, Yang Y, et al. MACC1 down-regulation inhibits proliferation and tumourigenicity of nasopharyngeal carcinoma cells through akt/β-catenin signaling pathway. PloS One (2013) 8. doi: 10.1371/journal.pone.0060821
181. Wang J, Wang W, Cai H, Du B, Zhang L, Ma W, et al. MACC1 facilitates chemoresistance and cancer stem cell-like properties of colon cancer cells through the PI3K/AKT signaling pathway. Mol Med Rep (2017) 16:8747–54. doi: 10.3892/mmr.2017.7721
182. Zhang K, Tian F, Zhang Y, Zhu Q, Xue N, Zhu H, et al. MACC1 is involved in the regulation of proliferation, colony formation, invasion ability, cell cycle distribution, apoptosis and tumorigenicity by altering akt signaling pathway in human osteosarcoma. Tumor Biol (2014) 35:2537–48. doi: 10.1007/s13277-013-1335-5
183. Zhou X, Xu CJ, Wang JX, Dai T, Ye YP, Cui YM, et al. Metastasis-associated in colon cancer-1 associates with poor prognosis and promotes cell invasion and angiogenesis in human cervical cancer. Int J Gynecol. Cancer (2015) 25:1353–63. doi: 10.1097/IGC.0000000000000524
184. Carracedo A, Pandolfi PP. The PTEN-PI3K pathway: Of feedbacks and cross-talks. Oncogene (2008) 27:5527–41. doi: 10.1038/onc.2008.247
185. Huttlin EL, Bruckner RJ, Navarrete-Perea J, Cannon JR, Baltier K, Gebreab F, et al. Dual proteome-scale networks reveal cell-specific remodeling of the human interactome. Cell (2021) 184:3022–3040.e28. doi: 10.1016/j.cell.2021.04.011
186. Li X, Wang C, Wang S, Hu Y, Jin S, Liu O, et al. YWHAE as an HE4 interacting protein can influence the malignant behaviour of ovarian cancer by regulating the PI3K/AKT and MAPK pathways. Cancer Cell Int (2021) 21:1–18. doi: 10.1186/s12935-021-01989-7
187. Gu J, Tamura M, Yamada KM. Tumor suppressor PTEN inhibits integrin- and growth factor-mediated mitogen-activated protein (MAP) kinase signaling pathways. J Cell Biol (1998) 143:1375–83. doi: 10.1083/jcb.143.5.1375
188. Hao L, Ha JR, Kuzel P, Garcia E, Persad S. Cadherin switch from e- to n-cadherin in melanoma progression is regulated by the PI3K/PTEN pathway through twist and snail. Br J Dermatol (2012) 166:1184–97. doi: 10.1111/j.1365-2133.2012.10824.x
189. Xu W, Yang Z, Lu NA. New role for the PI3K/Akt signaling pathway in the epithelial-mesenchymal transition. Cell Adhes. Migr. (2015) 9:317–24. doi: 10.1080/19336918.2015.1016686
190. Pasapera AM, Heissler SM, Eto M, Nishimura Y, Fischer RS, Thiam HR, et al. MARK2 regulates directed cell migration through modulation of myosin II contractility and focal adhesion organization. Curr Biol (2022) 32:2704–2718.e6. doi: 10.1016/j.cub.2022.04.088
191. Nishimura Y, Applegate K, Davidson MW, Danuser G, Waterman CM. Automated screening of microtubule growth dynamics identifies MARK2 as a regulator of leading edge microtubules downstream of RAC1 in migrating cells. PloS One (2012) 7. doi: 10.1371/journal.pone.0041413
192. Schmid F, Wang Q, Huska MR, Andrade-Navarro MA, Lemm M, Fichtner I, et al. SPON2, a newly identified target gene of MACC1, drives colorectal cancer metastasis in mice and is prognostic for colorectal cancer patient survival. Oncogene (2016) 35:5942–52. doi: 10.1038/onc.2015.451
193. Jia W, Li H, He YW. The extracellular matrix protein mindin serves as an integrin ligand and is critical for inflammatory cell recruitment. Blood (2005) 106:3854–9. doi: 10.1182/blood-2005-04-1658
194. Zhang YL, Li Q, Yang XM, Fang F, Li J, Wang YH, et al. Spon2 promotes M1-like macrophage recruitment and inhibits hepatocellular carcinoma metastasis by distinct integrin–rho gtpase–hippo pathways. Cancer Res (2018) 78:2305–17. doi: 10.1158/0008-5472.CAN-17-2867
195. Li H, Oliver T, Jia W, He YW. Efficient dendritic cell priming of T lymphocytes depends on the extracellular matrix protein mindin. EMBO J (2006) 25:4097–107. doi: 10.1038/sj.emboj.7601289
196. Kuramitsu S, Masuda T, Hu Q, Tobo T, Yashiro M, Fujii A, et al. Cancer-associated fibroblast-derived spondin-2 promotes motility of gastric cancer cells. Cancer Genomics Proteomics (2021) 18:521–9. doi: 10.21873/CGP.20277
197. Lu H, Feng Y, Hu Y, Guo Y, Liu Y, Mao Q, et al. Spondin 2 promotes the proliferation, migration and invasion of gastric cancer cells. J Cell Mol Med (2020) 24:98–113. doi: 10.1111/jcmm.14618
198. Feng Y, Ngu H, Alford SK, Ward M, Yin F, Longmore GD. α-Actinin1 and 4 tyrosine phosphorylation is critical for stress fiber establishment, maintenance and focal adhesion maturation. Exp Cell Res (2013) 319:1124–35. doi: 10.1016/j.yexcr.2013.02.009
199. Sheng XJ, Li Z, Sun M, Wang ZH, Zhou DM, Li JQ, et al. MACC1 induces metastasis in ovarian carcinoma by upregulating hepatocyte growth factor receptor c-MET. Oncol Lett (2014) 8:891–7. doi: 10.3892/ol.2014.2184
200. Xia J, Wang H, Huang H, Sun L, Dong S, Huang N, et al. Elevated Orai1 and STIM1 expressions upregulate MACC1 expression to promote tumor cell proliferation, metabolism, migration, and invasion in human gastric cancer. Cancer Lett (2016) 381:31–40. doi: 10.1016/j.canlet.2016.07.014
201. Imbastari F, Dahlmann M, Sporbert A, Mattioli CC, Mari T, Scholz F, et al. MACC1 regulates clathrin-mediated endocytosis and receptor recycling of transferrin receptor and EGFR in colorectal cancer. Cell Mol Life Sci (2021) 78:3525–42. doi: 10.1007/s00018-020-03734-1
202. Kobelt D, Perez-Hernandez D, Fleuter C, Dahlmann M, Zincke F, Smith J, et al. The newly identified MEK1 tyrosine phosphorylation target MACC1 is druggable by approved MEK1 inhibitors to restrict colorectal cancer metastasis. Oncogene (2021) 40:5286–301. doi: 10.1038/s41388-021-01917-z
203. Zhang R, Shi H, Ren F, Feng W, Cao Y, Li G, et al. MicroRNA-338-3p suppresses ovarian cancer cells growth and metastasis: Implication of Wnt/Catenin beta and MEK/ERK signaling pathways. J Exp Clin Cancer Res (2019) 38:1–13. doi: 10.1186/s13046-019-1494-3
204. Schmid F, Dahlmann M, Röhrich H, Kobelt D, Hoffmann J, Burock S, et al. Calcium-binding protein S100P is a new target gene of MACC1, drives colorectal cancer metastasis and serves as a prognostic biomarker. Br J Cancer (2022) 127:675–85. doi: 10.1038/s41416-022-01833-3
205. Gibadulinova A, Tothova V, Pastorek J, Pastorekova S. Transcriptional regulation and functional implication of S100P in cancer. Amino Acids (2011) 41:885–92. doi: 10.1007/s00726-010-0495-5
206. Du M, Wang G, Ismail TM, Gross S, Fernig DG, Barraclough R, et al. S100P dissociates myosin IIA filaments and focal adhesion sites to reduce cell adhesion and enhance cell migration. J Biol Chem (2012) 287:15330–44. doi: 10.1074/jbc.M112.349787
207. Gross SR, Sin CGT, Barraclough R, Rudland PS. Joining S100 proteins and migration: For better or for worse, in sickness and in health. Cell Mol Life Sci (2014) 71:1551–79. doi: 10.1007/s00018-013-1400-7
208. Zhu X, Wang X, Gong Y. Deng, j. e-cadherin on epithelial–mesenchymal transition in thyroid cancer. Cancer Cell Int (2021) 21:1–15. doi: 10.1186/s12935-021-02344-6
209. Samson SC, Khan AM, Mendoza MC. ERK signaling for cell migration and invasion. Front Mol Biosci (2022) 9:998475. doi: 10.3389/fmolb.2022.998475
210. Olea-Flores M, Zuñiga-Eulogio MD, Mendoza-Catalán MA, Rodríguez-Ruiz HA, Castañeda-Saucedo E, Ortuño-Pineda C, et al. Extracellular-signal regulated kinase: A central molecule driving epithelial–mesenchymal transition in cancer. Int J Mol Sci (2019) 20:1–32. doi: 10.3390/ijms20122885
211. Wan T, Zheng J, Yao R, Yang S, Zheng W, Zhou P. LncRNA DDX11-AS1 accelerates hepatocellular carcinoma progression via the MiR-195-5p/MACC1 pathway. Ann Hepatol (2021) 20:100258. doi: 10.1016/j.aohep.2020.09.003
212. Chen S, Zong ZH, Wu DD, Sun KX, Liu BL, Zhao Y. The role of metastasis-associated in colon cancer 1 (MACC1) in endometrial carcinoma tumorigenesis and progression. Mol Carcinog. (2017) 56:1361–71. doi: 10.1002/mc.22599
213. Cheng H, Zhou L, Long Y, Xiang J, Chen L. MACC1 is associated with epithelial–mesenchymal transition and can predict poor prognosis in nasopharyngeal carcinoma. Front Oncol (2021) 11:644120. doi: 10.3389/fonc.2021.644120
214. Guo Z, Neilson LJ, Zhong H, Murray PS, Zanivan S. Zaidel-bar, r. e-cadherin interactome complexity and robustness resolved by quantitative proteomics. Sci Signal (2014) 7. doi: 10.1126/scisignal.2005473
215. Guo L, Ou S, Ma X, Zhang S, Lai Y. MACC1 silencing inhibits cell proliferation and induces cell apoptosis of lung adenocarcinoma cells through the β-catenin pathway. Neoplasma (2018) 65:552–60. doi: 10.4149/neo
216. Kortüm B, Radhakrishnan H, Zincke F, Sachse C, Burock S, Keilholz U, et al. Combinatorial treatment with statins and niclosamide prevents CRC dissemination by unhinging the MACC1-β-Catenin-S100A4 axis of metastasis. Oncogene (2022) 41:4446–58. doi: 10.1038/s41388-022-02407-6
217. Wang L, Sun Y, Yi J, Wang X, Liang J, Pan Z, et al. Targeting H19 by lentivirus-mediated RNA interference increases A549 cell migration and invasion. Exp Lung Res (2016) 42:346–53. doi: 10.1080/01902148.2016.1223229
218. Chai H, Yang Y. Effects of MACC1 SiRNA on biological behaviors of HeLa. Arch Gynecol. Obstet. (2014) 289:1271–80. doi: 10.1007/s00404-013-3126-z
219. Lin L, Liu Y, Pan C, Zhang J, Zhao Y, Shao R, et al. Gastric cancer cells escape metabolic stress via the DLC3/MACC1 axis. Theranostics (2019) 9:2100–14. doi: 10.7150/thno.29538
220. Zhao Y, Liu Y, Lin L, Huang Q, He W, Zhang S, et al. The LncRNA MACC1-AS1 promotes gastric cancer cell metabolic plasticity via AMPK/Lin28 mediated MRNA stability of MACC1. Mol Cancer (2018) 17:1–16. doi: 10.1186/s12943-018-0820-2
221. Hruska AM, Yang H, Leggett SE, Guo M, Wong IY. Mechanobiology of collective cell migration in 3D microenvironments. arXiv (2022), 1–23. doi: 10.48550/arXiv.2202.02849
222. Claus W. Heizmann Calcium-binding proteins of the EF-hand superfamily from basics to medical applications. Methods Mol Biol (2019) 1929:157–86. doi: 10.1007/978-1-4939-9030-6_11
223. Sack U, Stein U. Wnt up your mind - intervention strategies for S100A4-induced metastasis in colon cancer. Gen Physiol Biophys (2009) 28:F55–64.
224. Tang J, Chen JX, Chen L, Tang JY, Cui Z, Liu CH, et al. Metastasis associated in colon cancer 1 (MACC1) promotes growth and metastasis processes of colon cancer cells. Eur Rev Med Pharmacol Sci (2016) 20:2825–34.
225. Gao J, Ding F, Liu Q, Yao Y. Knockdown of MACC1 expression suppressed hepatocellular carcinoma cell migration and invasion and inhibited expression of MMP2 and MMP9. Mol Cell Biochem (2013) 376:21–32. doi: 10.1007/s11010-012-1545-y
226. Ma L, Zhou Y, Luo X, Gao H, Deng X, Jiang Y. Long non-coding RNA XIST promotes cell growth and invasion through regulating MiR-497/MACC1 axis in gastric cancer. Oncotarget (2017) 8:4125–35. doi: 10.18632/oncotarget.13670
227. Devany J, Sussman DM, Yamamoto T, Manning ML, Gardel ML. Cell cycle–dependent active stress drives epithelia remodeling. Proc Natl Acad Sci U. S. A. (2021) 118. doi: 10.1073/pnas.1917853118
228. Rossen NS, Tarp JM, Mathiesen J, Jensen MH, Oddershede LB. Long-range ordered vorticity patterns in living tissue induced by cell division. Nat Commun (2014) 5:1–7. doi: 10.1038/ncomms6720
229. Doostmohammadi A, Thampi SP, Saw TB, Lim CT, Ladoux B, Yeomans JM. Celebrating soft matter’s 10th anniversary: Cell division: A source of active stress in cellular monolayers. Soft Matter (2015) 11:7328–36. doi: 10.1039/c5sm01382h
230. Czajkowski M, Sussman DM, Marchetti MC, Manning ML. Glassy dynamics in models of confluent tissue with mitosis and apoptosis. Soft Matter (2019) 15:9133–49. doi: 10.1039/c9sm00916g
231. Matoz-Fernandez DA, Martens K, Sknepnek R, Barrat JL, Henkes S. Cell division and death inhibit glassy behaviour of confluent tissues. Soft Matter (2017) 13:3205–12. doi: 10.1039/c6sm02580c
232. Ranft J, Basan M, Elgeti J, Joanny JF, Prost J, Jülicher F. Fluidization of tissues by cell division and apoptosis. Proc Natl Acad Sci U. S. A. (2010) 107:20863–8. doi: 10.1073/pnas.1011086107
233. Bergonzini C, Kroese K, Zweemer AJM, Danen EHJ. Targeting integrins for cancer therapy - disappointments and opportunities. Front Cell Dev Biol (2022) 10:863850. doi: 10.3389/fcell.2022.863850
234. Raudenská M, Petrláková K, Juriňáková T, Leischner Fialová J, Fojtů M, Jakubek M, et al. Engine shutdown: Migrastatic strategies and prevention of metastases. Trends Cancer (2023) xx:1–16. doi: 10.1016/j.trecan.2023.01.001
235. Martorana F, Motta G, Pavone G, Motta L, Stella S, Vitale SR, et al. AKT inhibitors: New weapons in the fight against breast cancer? Front Pharmacol (2021) 12:662232. doi: 10.3389/fphar.2021.662232
236. Mishra R, Patel H, Alanazi S, Kilroy MK, Garrett JT. PI3K inhibitors in cancer: Clinical implications and adverse effects. Int J Mol Sci (2021) 22. doi: 10.3390/ijms22073464
237. Boosani CS, Agrawal DK. PTEN modulators: A patent review. Expert Opin Ther Pat. (2013) 23:569–80. doi: 10.1517/13543776.2013.768985
238. Zhang Y, Wang X. Targeting the wnt/β-catenin signaling pathway in cancer. J Hematol Oncol (2020) 13:1–16. doi: 10.1186/s13045-020-00990-3
Keywords: MACC1, migration, adhesion, cytoskeleton, cancer
Citation: Hohmann T, Hohmann U and Dehghani F (2023) MACC1-induced migration in tumors: Current state and perspective. Front. Oncol. 13:1165676. doi: 10.3389/fonc.2023.1165676
Received: 14 February 2023; Accepted: 14 March 2023;
Published: 27 March 2023.
Edited by:
Zhi Sheng, Virginia Tech, United StatesReviewed by:
Wolfgang Walther, Charité Universitätsmedizin Berlin, GermanyRobert Shoemaker, National Cancer Institute (NIH), United States
Copyright © 2023 Hohmann, Hohmann and Dehghani. This is an open-access article distributed under the terms of the Creative Commons Attribution License (CC BY). The use, distribution or reproduction in other forums is permitted, provided the original author(s) and the copyright owner(s) are credited and that the original publication in this journal is cited, in accordance with accepted academic practice. No use, distribution or reproduction is permitted which does not comply with these terms.
*Correspondence: Tim Hohmann, VGltLmhvaG1hbm5AbWVkaXppbi51bmktaGFsbGUuZGU=