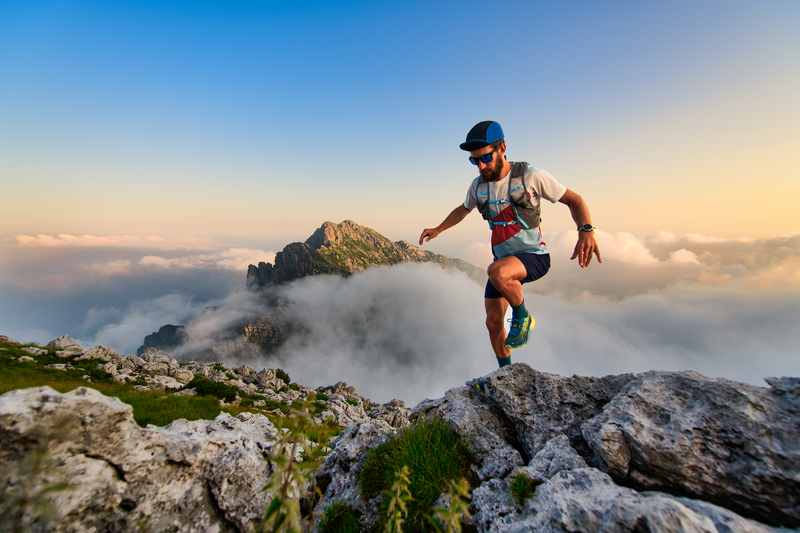
95% of researchers rate our articles as excellent or good
Learn more about the work of our research integrity team to safeguard the quality of each article we publish.
Find out more
EDITORIAL article
Front. Oncol. , 14 March 2023
Sec. Cancer Metabolism
Volume 13 - 2023 | https://doi.org/10.3389/fonc.2023.1155774
This article is part of the Research Topic Understanding Mitochondrial Dynamics and Metabolic Plasticity in Cancer Stem Cells: Recent Advances in Cancer Treatment and Potential Therapeutic Approaches View all 12 articles
Editorial on the Research Topic
Understanding mitochondrial dynamics and metabolic plasticity in cancer stem cells: Recent advances in cancer treatment and potential therapeutic approaches
Mitochondria and mitochondrial homeostasis have been shown to play critical roles in the pathology of various chronic medical conditions including cancer. In cancer, mitochondria are associated with most of the basic cellular mechanisms and signaling events. Being the powerhouse of the cell, mitochondria control cellular energy metabolism and the generation of ATP through oxidative phosphorylation and reactive oxygen species (ROS). Mitochondria are also responsible for the maintenance of cellular homeostasis through controlled cell death. Several factors contribute to the vulnerability of mitochondrial genes to mutation, including the lack of proof-reading mechanism in the replication process, high ROS levels, and the absence of histone proteins (1, 2). This results in a high degree of acquired heterogeneity in tumor microenvironments during disease progression. Due to the direct involvement of mitochondria in cellular events, mitochondrial genes and mitochondrial proteins have immense potential as therapeutic targets and biomarkers for early detection and prognosis.
The mitochondrial DNA (mtDNA) copy number is a direct indication of mitochondrial activity and is known to be associated with cancer by influencing numerous cellular mechanisms. It is also interesting that both increases and decreases in mtDNA copy number alterations are responsible for cancer initiation and progression (3). In adult gliomas, the mtDNA copy number has been evaluated as an age-related predictive marker (4), and, in colon cancer (CRC), it has been reported as a predictor for poor prognosis (5). Moreover, mtDNA copy number variation has been found to be associated with poor prognosis of various other cancers such as cervical cancer, breast cancer, esophageal squamous carcinoma, and chronic lymphocytic leukemia (6–9). Apart from the CNVs, mitochondrial protein expressions were also reported as prognostic biomarkers. Ubiquinol cytochrome c reductase binding protein (UQCRB), the protein responsible for the stabilization of mitochondrial ETC complex-III, was reported as a biomarker for CRC. The overall expression and CNV have been reported to be highly associated with CRC progression (10). In a case-control study of 260 renal cell carcinoma patients and 280 matched control individuals, a decreased mtDNA copy number was reported as a heritable predictive marker for higher cancer incidence (11).
In response to cell demands and environmental conditions, mitochondria can divide (mitochondrial fission) and fuse (mitochondrial fusion) (12). These dynamics have important roles in the pathology of cancer. The balance between fission and fusion is crucial in the maintenance of cellular processes such as energy metabolism, calcium signaling, oxygen sensing, and ROS generation. Most genes regulating mitochondrial fission and fusion are encoded by nuclear genes (13). In solid tumours, mitochondrial fission induces a reduction in the expression of MHC-I, causing immune escape (14) and making it a target to prevent immune evasion. Similarly, in triple negative breast cancer (TNBC) samples, significantly increased mitochondrial fission is associated with poorer survival (15). It is also observed that the survival of TNBC cells increased with a positive feedback loop to mitochondrial fission, by enhancing the Notch- and surviving-mediated pathways (15). On the other hand, Humphries et al. reported that TNBC with increased mitochondrial fission showed reduced metastatic potential (16).
Mitophagy, the elimination of defective mitochondria through autophagy, is considered to be a quality control mechanism, and any defects in mitophagy lead to impairment of mitochondrial functions, creating pathological alterations (17). Dysfunction of mitophagy leads to tumorigenesis (18) and the role of mitophagy varies with tumour progression. In a study using mitochondrial depletion, Yu-Seoun et al., demonstrated that mitochondrial dysfunction can lead to cancer cells acquiring stem cell-like properties (19). Furthermore, the mitochondrial density decreases as a result of increased mitophagy, which results in low reactive oxygen levels and low energy levels in the cells. Consequently, cells that possess stem cell-like quiescence properties survive in hypoxic conditions, resulting in residual cells and relapses of cancer. The majority of chemotherapies target rapidly dividing cells and ROS-producing cells, both of which become ineffective against quiescent cells, resulting in chemo resistance (20).
Metabolic reprogramming is an extremely prevalent feature of cancer cells and is directly linked to mitochondria. Mitochondria are indispensable to cancer cells because of their ability to generate ATP. In breast cancer cells, Lu et al., reported that the overexpression of mitochondrial fission regulator protein (MTFR2) alters the metabolism of glucose. MTFR2 changes oxidative phosphorylation (OXPHOS) into glycolysis in a HIF1α- and HIF2α-dependent manner (21). In hypoxic conditions, cancer cells also switch from OXPHOS to glycolysis. During this switching, anaerobic glycolysis generates lactate as the end product, which assists the cells in reducing ROS levels by utilizing metabolic intermediates such as pyruvate (22). In addition, it has been reported that glycolytic enzymes are upregulated during hypoxia; therefore, inhibiting these enzymes could be a promising way to eradicate residual cells and cancer stem cells (23–25). In addition to switching to glycolysis, the hypoxic microenvironment activates the pentose phosphate pathway (PPP). PPP activation produces NADPH and facilitates cancer cell survival in hypoxic environments by maintaining ROS homeostasis (26, 27).
Notably, mitochondria are the major source of intracellular ROS for a cell. By the oxidation of nucleotides, increased intracellular ROS directly damages nuclear and mitochondrial DNA. Similarly, reduced ROS levels cause cancer cells to enter a quiescent state, preventing them from being damaged by oxidative stress (28). Low levels of ROS are reported in cancer stem cells and metabolically inactive drug resistance cells (1). It has been shown that mitochondria-targeted photodynamic therapies (PDT) are effective in eliminating quiescent cancer stem cells and chemo-resistant cancers that are in a low-energy state (29). Integrated PDT uses the cancer cell’s ROS and metabolic state to convert prodrugs into active photosensitizers or to specifically target photosensitizers to mitochondria, resulting in effective photosensitizer accumulation (30). Upon exposure to irradiation, mitochondria suffer irreversible damage, resulting in cell death.
In the tumor microenvironment (TME) hypoxia induces acidification with low pH through the accumulation of lactic acid from glycolysis. The low pH alters the expression of multiple genes that promote cancer cell invasion and metastasis, and inhibits immune cell infiltration into the TME (31). Mitochondria-mediated upregulation of carbonic anhydrase enzyme, responsible for cancer cell survival in acidic environments, is a target for inhibiting cancer progression and metastasis (van Gisbergen et al.). Moreover, at an acidic pH, the immune cells lose their ability to counteract cancer cells. According to a recent study by Yi-Ru et al., decreased mitophagy in T cells leads to the accumulation of depolarized mitochondria and terminal exhaustion of T cells. Notably, T cells treated with nicotinamide riboside recovered mitochondrial fitness and became responsive to PD-1 inhibitors, confirming the role of mitochondrial dynamics in T-cell exhaustion (32). In addition, MDSCs infiltrate tumors by secreting chemokines that are regulated by mitochondria through HIF-1α (28). An immune responsive TME results from targeting mitochondria to impede chemokine production, thereby affecting the recruitment of MDSCs and Tregs.
The transfer of mitochondria between cells of the TME is yet another mechanism for cell survival and immune evasion. Saha et al., with the help of advanced technologies, depicted the nanotube-mediated transfer of mitochondria between cancer cells and immune cells. The cancer cells benefit from this exchange by generating more energy and increasing their cell division, whereas the immune cells become inactive and exhausted from this exchange. A promising strategy to target the TME for cancer treatment is to inhibit nanotube formation to enhance immune therapies (33).
Mitochondria provide resistance against chemotherapeutic agents through a number of different ways. As discussed previously, the most important way is ROS homeostasis. Additionally, ATP-dependent efflux pathways for multidrug resistance (MDR) (34), as well as TME acidifications, are reported to have a mechanistic impact on chemoresistance (35). Besides chemoresistance, the ROS scavenging ability protects cancer cells against radiotherapy (27).
Another therapeutic target is mitochondrial biogenesis in cancer cells. In a bioinformatics-based analysis of lung cancer patients, the key gene for mitochondrial biogenesis, HSPD1, was confirmed as a predictive biomarker (36). Additionally, pharmacological induction of Mfn-2 with Leflunomide increased mitochondrial fusion, with decreased ATP production and tumor growth in pancreatic ductal adenocarcinoma (37). Anti-mitochondrial therapy is a potential approach to target caner progression and metastasis. By inhibiting cancer cell mitochondria, numerous precancerous changes can be targeted. As well as preventing therapy resistance, targeting mitochondria can enhance chemotherapy, radiotherapy, and immunotherapy.
In this Research Topic, we discuss the critical function of mitochondria in cancer cells and TMEs. The mitochondria provide a connection between the cells of the TME and support the survival and progression of cancer cells. By modifying ROS homeostasis, mitochondria control the glycolytic flux and the induction of genes involved in progression and metastasis. Mitochondria in acidic TMEs inhibit immune infiltration, cause immune cell exhaustion, and induce cancer cell immune evasion. In cancer cells, mitochondria provide resistance to chemotherapy, radiotherapy, and immunotherapy. Taken together, mitochondria are crucial targets for drug therapies, and changes in mitochondrial copy number variations and gene expressions function as predictive and prognostic biomarkers.
PD and VSN equally contributed writing, editing, and proof reading. All authors contributed to the article and approved the submitted version.
The authors declare that the research was conducted in the absence of any commercial or financial relationships that could be construed as a potential conflict of interest.
All claims expressed in this article are solely those of the authors and do not necessarily represent those of their affiliated organizations, or those of the publisher, the editors and the reviewers. Any product that may be evaluated in this article, or claim that may be made by its manufacturer, is not guaranteed or endorsed by the publisher.
1. Fulda S, Galluzzi L, Kroemer G. Targeting mitochondria for cancer therapy. Nat Rev Drug Discov (2010) 9(6):447–64. doi: 10.1038/nrd3137
2. Bianchi NO, Bianchi MS, Richard SM. Mitochondrial genome instability in human cancers. Mutat Res (2001) 488(1):9–23. doi: 10.1016/S1383-5742(00)00063-6
3. Abd Radzak SM, Mohd Khair SZN, Ahmad F, Patar A, Idris Z, Mohamed Yusoff AA. Insights regarding mitochondrial DNA copy number alterations in human cancer (Review). Int J Mol Med (2022) 50(2):104. doi: 10.3892/ijmm.2022.5160
4. Sourty B, Dardaud L-M, Bris C, Desquiret-Dumas V, Boisselier B, Basset L, et al. Mitochondrial DNA copy number as a prognostic marker is age-dependent in adult glioblastoma. Neurooncol Adv (2022) 4(1):vdab191. doi: 10.1093/noajnl/vdab191
5. van Osch FHM, Voets AM, Schouten LJ, Gottschalk RWH, Simons CCJM, van Engeland M, et al. Mitochondrial DNA copy number in colorectal cancer: Between tissue comparisons, clinicopathological characteristics and survival. Carcinogenesis (2015) 36(12):1502–10. doi: 10.1093/carcin/bgv151
6. Campa D, Barrdahl M, Santoro A, Severi G, Baglietto L, Omichessan H, et al. Mitochondrial DNA copy number variation, leukocyte telomere length, and breast cancer risk in the European prospective investigation into cancer and nutrition (EPIC) study. Breast Cancer Res (2018) 20(1):29. doi: 10.1186/s13058-018-0955-5
7. Li H, Tian Z, Zhang Y, Yang Q, Shi B, Hou P, et al. Increased copy number of mitochondrial DNA predicts poor prognosis of esophageal squamous cell carcinoma. Oncol Lett (2018) 15(1):1014–20. doi: 10.3892/ol.2017.7416
8. Lan Q, Lim U, Liu C-S, Weinstein SJ, Chanock S, Bonner MR, et al. A prospective study of mitochondrial DNA copy number and risk of non-Hodgkin lymphoma. Blood (2008) 112(10):4247–9. doi: 10.1182/blood-2008-05-157974
9. Warowicka A, Kwasniewska A, Gozdzicka-Jozefiak A. Alterations in mtDNA: A qualitative and quantitative study associated with cervical cancer development. Gynecol Oncol (2013) 129(1):193–8. doi: 10.1016/j.ygyno.2013.01.001
10. Kim H-C, Chang J, Lee HS, Jeong Kwon H. Mitochondrial UQCRB as a new molecular prognostic biomarker of human colorectal cancer. Exp Mol Med (2017) 49(11):e391–1. doi: 10.1038/emm.2017.152
11. Xing J, Chen M, Wood CG, Lin J, Spitz MR, Ma J, et al. Mitochondrial DNA content: Its genetic heritability and association with renal cell carcinoma. JNCI: J Natl Cancer Institute (2008) 100(15):1104–12. doi: 10.1093/jnci/djn213
12. Archer SL. Mitochondrial dynamics — mitochondrial fission and fusion in human diseases. New Engl J Med (2013) 369(23):2236–51. doi: 10.1056/NEJMra1215233
13. Koopman WJH, Willems PHGM, Smeitink JAM. Monogenic mitochondrial disorders. New Engl J Med (2012) 366(12):1132–41. doi: 10.1056/NEJMra1012478
14. Lei X, Lin H, Wang J, Ou Z, Ruan Y, Sadagopan A, et al. Mitochondrial fission induces immunoescape in solid tumors through decreasing MHC-I surface expression. Nat Commun (2022) 13(1):3882. doi: 10.1038/s41467-022-31417-x
15. Chen L, Zhang J, Lyu Z, Chen Y, Ji X, Cao H, et al. Positive feedback loop between mitochondrial fission and notch signaling promotes survivin-mediated survival of TNBC cells. Cell Death Dis (2018) 9(11):1050. doi: 10.1038/s41419-017-0127-z
16. Humphries BA, Cutter AC, Buschhaus JM, Chen Y-C, Qyli T, Palagama DSW, et al. Enhanced mitochondrial fission suppresses signaling and metastasis in triple-negative breast cancer. Breast Cancer Res (2020) 22(1):60. doi: 10.1186/s13058-020-01301-x
17. Youle RJ, van der Bliek AM. Mitochondrial fission, fusion, and stress. Science (2012) 337(6098):1062–5. doi: 10.1126/science.1219855
18. Chang JY, Yi H-S, Kim H-W, Shong M. Dysregulation of mitophagy in carcinogenesis and tumor progression. Biochim Biophys Acta (BBA) - Bioenergetics (2017) 1858(8):633–40. doi: 10.1016/j.bbabio.2016.12.008
19. Han Y-S, Yi E-Y, Jegal M-E, Kim Y-J. Cancer stem-like phenotype of mitochondria dysfunctional Hep3B hepatocellular carcinoma cell line. Cells (2021) 10(7). doi: 10.3390/cells10071608
20. Blagosklonny MV. Carcinogenesis, cancer therapy and chemoprevention. Cell Death Differ (2005) 12(6):592–602. doi: 10.1038/sj.cdd.4401610
21. Lu G, Yuanhui L, Wang T, Lin W, Lu J, Ma Y, et al. Mitochondrial fission regulator 2 (MTFR2) promotes growth, migration, invasion and tumour progression in breast cancer cells. Aging (2019) 11(22):10203–19. doi: 10.18632/aging.102442
22. Vaupel P, Multhoff G. Revisiting the warburg effect: historical dogma versus current understanding. J Physiol (2021) 599(6):1745–57. doi: 10.1113/JP278810
23. Chang C-W, Lo J-F, Wang XW. Roles of mitochondria in liver cancer stem cells. Differentiation (2019) 107:35–41. doi: 10.1016/j.diff.2019.04.001
24. Song K, Kwon H, Han C, Zhang J, Dash S, Lim K, et al. Active glycolytic metabolism in CD133(+) hepatocellular cancer stem cells: regulation by MIR-122. Oncotarget (2015) 6(38). doi: 10.18632/oncotarget.5812
25. Hur W, Ryu JY, Kim HU, Hong SW, Lee EB, Lee SY, et al. Systems approach to characterize the metabolism of liver cancer stem cells expressing CD133. Sci Rep (2017) 7(1):45557. doi: 10.1038/srep45557
26. Paredes F, Williams HC, San Martin A. Metabolic adaptation in hypoxia and cancer. Cancer Lett (2021) 502:133–42. doi: 10.1016/j.canlet.2020.12.020
27. Li P, Zhang D, Shen L, Dong K, Wu M, Ou Z, et al. Redox homeostasis protects mitochondria through accelerating ROS conversion to enhance hypoxia resistance in cancer cells. Sci Rep (2016) 6(1):22831. doi: 10.1038/srep22831
28. Chiu DK-C, Xu IM-J, Lai RK-H, Tse AP-W, Wei LL, Koh H-Y, et al. Hypoxia induces myeloid-derived suppressor cell recruitment to hepatocellular carcinoma through chemokine (C-c motif) ligand 26. Hepatology (2016) 64(3):797–813. doi: 10.1002/hep.28655
29. Darvin P, Aneesh C, Shankara NV, Chandrasekhar L, Thomas Maliakkal R, John SSM, et al. Mitochondria targeted redox GFP reveals time and dose dependent onset and progression of mitochondrial oxidation with diverging cell death decisions during photodynamic therapy. Photodiagnosis Photodyn Ther (2020) 31:101921. doi: 10.1016/j.pdpdt.2020.101921
30. Zhang ZJ, Wang K-P, Mo J-G, Xiong L, Wen Y. Photodynamic therapy regulates fate of cancer stem cells through reactive oxygen species. World J Stem Cells (2020) 12(7):562–84. doi: 10.4252/wjsc.v12.i7.562
31. White KA, Grillo-Hill BK, Barber DL. Cancer cell behaviors mediated by dysregulated pH dynamics at a glance. J Cell Sci (2017) 130(4):663–9. doi: 10.1242/jcs.195297
32. Yu Y-R, Imrichova H, Wang H, Chao T, Xiao Z, Gao M, et al. Disturbed mitochondrial dynamics in CD8+ TILs reinforce T cell exhaustion. Nat Immunol (2020) 21(12):1540–51. doi: 10.1038/s41590-020-0793-3
33. Saha T, Dash C, Jayabalan R, Khiste S, Kulkarni A, Kurmi K, et al. Intercellular nanotubes mediate mitochondrial trafficking between cancer and immune cells. Nat Nanotech (2022) 17(1):98–106. doi: 10.1038/s41565-021-01000-4
34. Ji L, Li H, Gao P, Shang G, Zhang DD, Zhang N, et al. Nrf2 pathway regulates multidrug-Resistance-Associated protein 1 in small cell lung cancer. PloS One (2013) 8(5):e63404. doi: 10.1371/journal.pone.0063404
35. Bohloli M, Atashi A, Soleimani M, Kaviani S, Anbarlou A. Investigating effects of acidic pH on proliferation, invasion and drug-induced apoptosis in lymphoblastic leukemia. Cancer Microenviron (2016) 9(2):119–26. doi: 10.1007/s12307-016-0187-0
36. Sotgia F, Lisanti MP. Mitochondrial markers predict survival and progression in non-small cell lung cancer (NSCLC) patients: Use as companion diagnostics. Oncotarget (2017) 8(40). doi: 10.18632/oncotarget.19677
Keywords: mitochondrial dynamics, mitophagy, mitochondrial immune evasion, cancer stem cell (CSC), hypoxic microenvironment
Citation: Darvin P and Sasidharan Nair V (2023) Editorial: Understanding mitochondrial dynamics and metabolic plasticity in cancer stem cells: Recent advances in cancer treatment and potential therapeutic approaches. Front. Oncol. 13:1155774. doi: 10.3389/fonc.2023.1155774
Received: 31 January 2023; Accepted: 07 March 2023;
Published: 14 March 2023.
Edited and Reviewed by:
Ubaldo Emilio Martinez-Outschoorn, Thomas Jefferson University, United StatesCopyright © 2023 Darvin and Sasidharan Nair. This is an open-access article distributed under the terms of the Creative Commons Attribution License (CC BY). The use, distribution or reproduction in other forums is permitted, provided the original author(s) and the copyright owner(s) are credited and that the original publication in this journal is cited, in accordance with accepted academic practice. No use, distribution or reproduction is permitted which does not comply with these terms.
*Correspondence: Pramod Darvin, cHJhbW9kZGFydmluQHJnY2IucmVzLmlu; Varun Sasidharan Nair, dmFydW5zYXNpZGhhcmFuLm5haXJAaGVsbWhvbHR6LWh6aS5kZQ==
Disclaimer: All claims expressed in this article are solely those of the authors and do not necessarily represent those of their affiliated organizations, or those of the publisher, the editors and the reviewers. Any product that may be evaluated in this article or claim that may be made by its manufacturer is not guaranteed or endorsed by the publisher.
Research integrity at Frontiers
Learn more about the work of our research integrity team to safeguard the quality of each article we publish.