- 1Department of Biomedical Engineering, University of Minnesota, Minneapolis, MN, United States
- 2Department of Microbiology and Immunology, Geisel School of Medicine, Dartmouth College, Hanover, NH, United States
- 3Dartmouth Cancer Center, Dartmouth Geisel School of Medicine and Dartmouth Health, Lebanon, NH, United States
- 4Department of Radiology, University of Minnesota, Minneapolis, MN, United States
Energy-based focal therapy (FT) uses targeted, minimally invasive procedures to destroy tumors while preserving normal tissue and function. There is strong emerging interest in understanding how systemic immunity against the tumor can occur with cancer immunotherapy, most notably immune checkpoint inhibitors (ICI). The motivation for combining FT and ICI in cancer management relies on the synergy between the two different therapies: FT complements ICI by reducing tumor burden, increasing objective response rate, and reducing side effects of ICI; ICI supplements FT by reducing local recurrence, controlling distal metastases, and providing long-term protection. This combinatorial strategy has shown promising results in preclinical study (since 2004) and the clinical trials (since 2011). Understanding the synergy calls for understanding the physics and biology behind the two different therapies with distinctive mechanisms of action. In this review, we introduce different types of energy-based FT by covering the biophysics of tissue-energy interaction and present the immunomodulatory properties of FT. We discuss the basis of cancer immunotherapy with the emphasis on ICI. We examine the approaches researchers have been using and the results from both preclinical models and clinical trials from our exhaustive literature research. Finally, the challenges of the combinatory strategy and opportunities of future research is discussed extensively.
1 Introduction
1.1 Overview of cancer treatments
Cancer is the second leading cause of death in the United States (1). Over the past century, numerous cancer treatments have been developed to improve patients’ survival and quality of life. According to NCI and ACS, there are a number of categories (2, 3) of cancer treatments that can be divided into several types, including surgery, radiation therapy, chemotherapy, immunotherapy, targeted therapy, and combination treatments. The U.S. Food and Drug Administration (FDA) has approved standards of care (SOC) to treat particular diseases depending on the type of cancer, the stage of cancer, and the patient’s disease history. SOC refers to a treatment that is accepted by medical experts as a proper treatment for a certain type of disease and that is widely used by healthcare professionals (4).
Surgical resection, chemotherapy, and radiotherapy are the most common SOC choices currently. Surgical resection has remained the first choice for most solid tumor cases. With advanced-stage cancer, chemotherapy, radiation, or both are usually suggested to control symptoms or to reduce the chance of local tumor recurrence and metastasis (5). However, these treatments come with limitations. Not all tumors are eligible for resection due to size, tumor location, or disease stage (6). When chemotherapy and radiation are used instead, the remaining cancerous cells may develop treatment resistance, resulting in failure to treat metastatic diseases in many cases (7). Another serious issue associated with chemotherapy and radiotherapy are side effects due to systemic toxicities and/or local damage to healthy tissues, which can range from hair loss and blood clotting problems to long-term organ damage (8, 9). Therefore, there is a desire for less invasive and more specific treatments that destroy the diseased tissue but have fewer adverse events and shorter recovery times, which has led to treatments such as focal therapies.
Energy-based focal therapy (FT), sometimes referred to as energy-based tumor ablation, is a rapidly growing field in loco-regional therapy (LRT) and interventional oncology (IO) for cancer management. FT uses targeted, minimally invasive procedures, usually performed with the help of image guidance, to treat and/or relieve the symptoms of cancer. It has been used for decades to treat solid tumors by effectively destroying tumors while preserving normal tissue and function so as to reduce side effects and cause minimal pain (10, 11). In addition, focal therapy can be used on unresectable disease, for instance, if the tumor is too large to be safely resected, or if it is intertwined with blood vessels and other vital structures, making safe removal impossible (12).
1.2 FT opportunities in the cancer immunotherapy revolution
The utility of leveraging the immune system to fight tumors has now been robustly validated. Cancer immunotherapy, most notably immune checkpoint inhibitors (ICIs), have definitively established that cancer can be treated very effectively by the immune system without directly eliminating cancer cells (13). ICIs are antibodies that disable molecular immune controls, i.e., checkpoints, that “turn off” antitumor immunity. There is strong interest in understanding the tumor immune microenvironment and how it impacts the response to immunotherapy (14).
While multiple strategies to enhance the response of ICIs have been proposed and studied, FTs have a unique set of advantages for combining with ICIs. Both the applied energy and the cell death caused by the applied energy causes perturbation of the tumor immune microenvironment. The unique ways FT modulate the immune system, especially compared to conventional cancer treatments, have started to be appreciated: FT has long been hypothesized to possess immunomodulatory properties as debulking removes the immunosuppressive tumor burden and consequently enables immune activation (15). By removing a tumor, surgery also eliminates the source of the immunosuppression, but it does not further support antitumor immunity. Unlike surgical resection, FT leaves tumor debris in situ and therefore creates antigens to enhance a locoregional and systemic antitumor immune response (16). Another relevant concept is that FTs support anti-tumor immune responses because of the cell death mechanisms induced by FTs. Unlike chemotherapy and radiation, FT induces cell death by extensive necrosis (16). Necrosis releases intracellular contents containing both the antigen and stimulating signals (among other danger signals) that activate T cells for the adaptive immune response to the FT-treated tumor.
This raises the significant possibility that FT can lead to in-situ tumor vaccination that generates local and systemic antitumor immunity while ICI helps effector T cells overcome immunosuppression in metastatic tumors not exposed to FT. Some recent work suggests that long-lasting systemic immunity against the tumor can occur after FT and ICI combined therapy (17). Therefore, it is crucial to understand the mechanism of action by which FT and ICI modulate the immune system, which is the topic of this review.
1.3 The synergy between FT and ICI
The motivation for combining FT and ICI in cancer management is based on the synergy between the two different therapies with distinctive mechanisms of action, as summarized in Table 1.
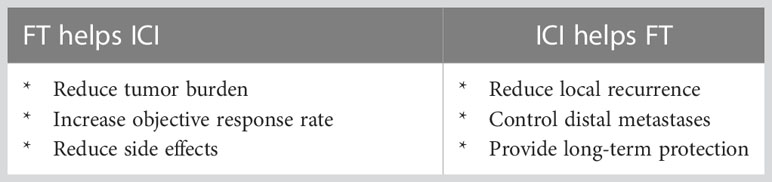
Table 1 The motivation of combinatory strategy relies on the synergy between focal therapy (FT) and immune checkpoint inhibitor (ICI).
In addition to tissue destruction, the immunomodulatory properties of FT have been documented. In recent decades, these immunomodulatory properties have been actively exploited to control cancer after the initial physical destruction (10, 18). FT improving ICI efficacy stems from the theory that FT expands the pool of tumor-specific CD8 effector T cells and reduce tumor immunosuppression, so there is more tumor-specific effector T cells to respond to ICI monotherapy. FT can also reduce ICI treatment-related side effects by improving the efficacy of ICI without increasing dosage.
On the other hand, despite the observation of abscopal effect and immunomodulation following various forms of FT (19–21), the immune response that follows FT alone is usually too modest to cause the system-wide, sustained anti-tumor effect needed to destroy distant metastases. The addition of systemic immunomodulation provided by ICI in the combination treatment has potential for inducing effective long-lasting anti-tumor immunity to combat both local recurrence and metastasis.
This combinatorial strategy has shown promising results in a variety of studies, especially since 2004 (22) and 2011 (23), when the first preclinical study and the first clinical trials were reported, respectively. Since then, more forms of FT and various types of ICI has been evaluated in a wide range of cancer types. More than half of the published preclinical studies and newly launched clinical trials took place within 5 years of the writing of this review. Besides appreciating the explosion of research and the enormous opportunities of the combinatorial strategy of FT and ICI, understanding the synergy also calls for understanding the physics and biology behind this emerging area.
In this review, we start by introducing different types of energy-based focal therapy. In Section 2, we cover the biophysics of FT based on heating and hyperthermia, freezing and electric membrane disruption, and how each utilization of energy leads to focal tissue destruction. In addition, practical and immunomodulatory advantage of energy-based FT compared to conventional cancer therapy is discussed. In Section 3, we present important aspects of immune responses following FT due to its strong association with immunogenic cell death (ICD), as understanding the immunomodulatory effects of FT serves as the foundation of designing and optimizing combinatory approaches with cancer immunotherapy. We continue by introducing cancer immunotherapy (Section 4) with an emphasis on ICI (Section 5) to provide the basis for the synergy between FT and ICI. Later, we examine the approaches and outcomes researchers have been using and the results from both in vivo models (Section 6) and clinical trials (Section 7) from our exhaustive literature research. Finally, the challenges of the combinatory strategy (Section 8) and opportunities of future research (Section 9) are discussed extensively.
2 Energy-based focal therapy (FT) and its clinical applications
Energy-based FT, or focal tissue ablation, is a form of locoregional therapy that relies on energy to destroy tissues without affecting the rest of the body. Cancer focal therapies can be categorized into thermal and nonthermal techniques, depending on the form of energy leading to tissue destruction. Thermal techniques can be high-temperature-based, such as radiofrequency ablation (RFA), microwave ablation (MWA), high-intensity focused ultrasound (HIFU), laser ablation and nanoparticle (NP)-mediated hyperthermia, or low-temperature-based such as cryoablation. Nonthermal techniques include irreversible electroporation (IRE) and histotripsy. Ablation techniques, the source of energy, and their clinical targets are summarized in Table 2.
It should be noted this review focuses on physical energy as the direct and immediate cause of cell death, and we do not cover every form of locoregional therapy. While widely used in clinical settings, some locoregional therapies rely on cell death mechanisms other than energy-cell interaction. Techniques not discussed in this review include but are not limited to (1) ionizing radiation therapy (32), such as external-beam radiation therapy, stereotactic body radiation therapy, selective internal radiation therapy, high dose rate brachytherapy, and transarterial radioembolization; (2) chemical ablation (33), which relies on focal cellular toxicity and protein denaturation to produce cellular necrosis, typically by focal injection of chemical ablation agents such as ethanol, acetic acid, and hypertonic sodium chloride solution; and approaches sometimes called thermochemical ablation and electrolytic ablation, including (3) embolization (34), such as transcatheter arterial chemoembolization and portal vein embolization; (4) local cytotoxic therapy, such as photodynamic therapy (35) and cold atmospheric plasma (36); (5) local chemotherapy, such as electrochemotherapy (37) and local administration of cytotoxic agents (e.g., doxorubicin, benzalkonium chloride, silver nitrate, etc.) (38); and (6) others such as gene electrotransfer (39, 40).
2.1 Heating and hyperthermic focal therapy
Heat has long been studied as a crucial mechanism for cellular damage; different temperature ranges can lead to distinct biological mechanisms. As such, many different energy-based focal ablative techniques exist, as shown in Table 2. In all of these cases, raising the temperature can lead to cell injury and eventually death. For example, as the temperature is raised to 39°C, blood perfusion in tissues will increase to protect tissue, but sustained heating above 42°C can lead to vascular stasis and thrombosis followed by ischemic necrosis and death. At the cell level, temperatures between 41°C and 45°C led to diminished or halted metabolism and impaired DNA repair. Extensive reviews on the subject of hyperthermic cellular and tissue destruction are available (41, 42).
Radiofrequency ablation (RFA) occurs by applying an oscillating electric current (typically between 450 and 500 kHz) to the target tissue through direct placement of one or more interstitial electrodes into the tumor. Tissues further away from the electrode are heated primarily by thermal conduction (43, 44). The delivery of RF energy and resulting lesion size are determined by the tissue electrical conductivity and thermal conduction. During RFA, water vapor, desiccation, and charring can occur, which increases the electrical impedance significantly (43, 45). As a result, RF ablation zones can vary widely according to electrode design (e.g., size, shape, or even internal cooling), electrical control (i.e., voltage, current, and power), local tissue environment (i.e., thermal conduction and blood flow). Different RF probe designs (such as internally cooled wet electrodes) can address desiccation and even decrease electrical impedance (46).
Microwave ablation (MWA) relies on the electromagnetic field generated by an intratumorally placed antenna to generate dielectric heating. The most common frequencies used for microwave ablation are 915 MHz, 2.45 GHz, and broadband frequencies between 1 GHz and 10 GHz. Polar molecules (primarily water) within the tissue continuously realign with the oscillating electromagnetic field, effectively increasing kinetic energy. The ability to cause hyperthermic injury is determined by device design (antenna design, number, and orientation), MW characteristic (power and frequency), tissue electrical properties (dielectric constant, electrical conductivity, and relative permittivity), and tissue thermal properties (43, 47).
High intensity focused ultrasound (HIFU) uses multiple high-intensity non-ionizing ultrasound beams and focuses them on a selected focal area to destroy the target tissue. HIFU systems typically operate in the frequency range of approximately 500 kHz and 7.5 MHz. HIFU causes tissue injury through two primary mechanisms: thermal damage due to absorption of the applied acoustic energy and mechanical damage due to acoustic cavitation. The amount of acoustic energy transferred from the acoustic wave to the tissue is directly proportional to the intensity of the wave and the innate absorption coefficient of the targeted tissue (47). (Micro) bubble can be injected or created during HIFU or tissue boiling. The presence of bubble can enhance heating effect owing to their ability to generate higher harmonics of the exciting frequency and lower the cavitation threshold, allowing lower energies to be used for ablation (48).
Laser ablation, which refers to laser-induced interstitial thermotherapy (LITT) in our context, also known as stereotactic laser ablation (SLA), is performed by implanting a laser catheter into the tumor. It uses high-intensity lasers to generate heat. Heat is generated by optical absorption and is then conducted to the rest of the tissue to shrink or destroy tumors (27). Temperature within the lesion is usually measured throughout the procedure using MRI thermometry. Laser penetration into the tissue is affected by the specific optical properties of the tissue. The effect of laser ablation is influenced by a number of factors: laser light wavelength, laser settings (laser power, laser energy, and treatment time), physical properties of the tissue, and the emission characteristics of the optical applicator (49).
NP-mediated hyperthermia generates heat based on the unique and highly tunable optical or magnetic properties of nanomaterials. Based on the energy source, NP-mediated hyperthermia can be categorized as photothermal therapy (PTT) or magnetic hyperthermia therapy (MHT), also known as magnetic fluid hyperthermia (MFH). PTT involves the application of normally benign light wavelengths (most often NIR light) in combination with efficient photothermal agents (e.g., gold or carbon nanomaterials) that convert the absorbed light to heat (50). MHT relies on magnetic nanoparticles to transform electromagnetic energy from an alternating magnetic field (AMF) to heat (51, 52). In practice, MHT has been studied on pre-clinical models, and has also been used in clinical trials to treat deep-seated, terminal, unresectable tumors, such as glioblastoma multiforme or prostate tumors (53). Due to the limitations of laser light penetration, PTT has been studied in preclinical models and several clinical trials for more superficial cancers, such as breast cancer, melanoma, lung cancer, and colorectal cancer (54).
2.2 Cryogenic focal therapy
Cryoablation relies on cryogenic temperature to cause cold injury and destroy target tissue, as also featured in Table 2. The freezing process results in intracellular and extracellular ice formation, dehydration, and vascular injury. The mechanism of tissue injury varies with the freezing rate and final tissue temperature, as well as tissue susceptibility. A slow rate of freezing favors the formation of extracellular ice crystals, which leads to a hypertonic extracellular environment and osmotic cell shrinkage from fluid shifting out of the cell, increasing cell dehydration and death. Fast freezing to lower temperatures promotes the formation of intracellular ice crystals, which results in direct cell injury because of damage to the cell membrane and organelles (55, 56). Cold-induced vascular injury usually leads to platelet aggregation, microthrombosis, and ischemia, causing further coagulative necrosis. Apoptosis can also occur in some cases in the peripheral zone of sublethal cold temperatures and contributes to tissue damage (55, 56).
Cryoablation is performed using needle-like probes, where rapid cooling is achieved by circulating cryogen (e.g., liquid nitrogen) or by the Joule-Thomson effect (45). Heat transfer in surrounding tissue is governed by passive thermal diffusion (43). During the application of cryoablation, an ice ball is formed, allowing for precise monitoring of the ablation zone by ultrasound, CT, or magnetic resonance imaging. However, the temperature that is necessary for cell lethality is between -20°C and -40°C, which means the lethal isotherm lies inside of the visualized ice ball, making it difficult to destroy tumor completely while not damaging surrounding healthy tissue (57).
2.3 Irreversible electroporation (IRE)
IRE, as also featured in Table 2, applies pulses of electrical fields (thousands of V/cm) that last from nanoseconds to milliseconds to create permanent and lethal nanopores in the cell membrane that disrupt cellular homeostasis and induce cell death (58, 59). IRE relies on the flow of current through tissue to induce cell death; therefore, electrical conductivity is the most important factor determining the distribution of the electric field. In addition, pulse parameters are thought to influence the profile of cell death within targeted tissue and therapeutic outcomes (58).
Unlike thermal ablation techniques, IRE is not susceptible to heat sink effect, which occurs when heat or cold is absorbed by flowing blood or air and carried away from the area of ablation, thereby limiting the effectiveness of ablation when the target lesion is in close proximity to a large blood vessel (45). In addition, IRE can preserve major vascular and ductal structures in the ablated region, offering the benefits of short treatment time and reduced collateral thermal injury (58). These unique advantages make IRE a good option for treating tumors in specific locations (e.g., pancreas).
2.4 Mechanical tissue disruption
Mechanical tissue disruption/destruction, also known as mechanical HIFU ablation, is achieved by exposing tissue to repeated short- (microsecond to millisecond) duration pulses of high-intensity ultrasound with low duty cycles (60). The tissue is fractionated in a controlled manner, compared to thermal destruction by HIFU. There are different methods of mechanical tissue disruption using focused ultrasound with distinctive bio-effects, such as low-intensity (< 1 kw/cm2) focused ultrasound techniques [sometimes refer to ultrasound irradiation (61)], high intensity (1-10 kw/cm2) techniques that increases cell permeability and extremely high-intensity (>10 kw/cm2) focused techniques [for example histotripsy (62), boiling histotripsy (63)] leading to tissue fragmentation. In addition, the collapse of bubbles or gas-filled cavities create an extremely large pressure shock wave capable of inducing fragmentation of tissue into subcellular levels (64).
2.5 Novel energy-based focal therapies
There are numerous energy-based cancer therapies being developed and tested. Some techniques have gained FDA approval, such as tumor treating fields, which rely on mild electrical fields for tumors including glioblastoma multiforme (GBM) (65) to interrupt the cancer cells’ ability to divide. Some of these therapies are in clinical trials, such as cryo-thermal therapy, which combines cooling by LN2 and heating by RFA. Some are still in developmental stages, for example cryoelectrolysis, which combines electrolysis and freezing. However, due to limited knowledge available, their immunological effect and/or their combinatory approach with immunotherapy are not covered in this review.
2.6 Advantages and drawbacks of FT
Focal therapy (FT) has been proposed as a minimally invasive option for treating localized disease with the aim of minimizing the side effects associated with radical treatment while maintaining the oncological benefit of local treatments (66). FT is usually recommended when other common treatments are not appropriate due to tumor size, tumor location, or disease stage. It is useful in relieving pain and slowing disease progression and is often combined with other treatments (as an adjuvant), such as hormone therapy, chemotherapy, radiation therapy, or surgery. The recovery time for FT is typically shorter than for surgery or radiation therapy. In fact, it usually does not require an overnight hospital stay (67, 68). FT can also be used as a salvage or palliative therapy, when other commonly used treatments (e.g., radiation therapy or surgery) fail, or patients cannot tolerate these treatments (69).
FT offers a range of practical advantages over conventional surgical resection, radiation therapy and chemotherapy. Being a cost-effective alternative to surgery, minimally invasive FTs are indicated for a large range of malignancies at an early stage or for those not eligible for surgery. They have a better complication/risk profile than radical surgery and can be used in patients who are not fit enough for or decline radical options (18). When the tumor is not suitable for resection and has limited response to chemo/radiation due to high tumor burden, prior radiation treatment of the site, or drug resistance, an ablation protocol can be a good treatment plan in order to significantly decrease the primary tumor burden and develop systemic anti-cancer immunity. Unlike chemotherapy or radiation therapy, FTs rely on physical energy for the destruction of cancer cells and therefore circumvent the resistance of tumors that received prior treatments, making FTs a great option for repeated treatment (70).
Although focal therapy can offer several advantages compared to traditional treatment, clinical use of FT as a first-line treatment is quite limited. In the clinic, cryosurgery is a first-line treatment in dermatological disorders for early-stage skin cancer, retinoblastoma, and precancerous growths on the skin and cervix (71). Thermal ablation and IRE are not commonly available. The biggest problem preventing FT from being considered SOC is that a large percentage of cancers, including melanoma (72), prostate (73), liver (74), pancreas (75), and kidney (74), treated by FTs still go on to fail by local and systemic recurrence. Even a small amount of residual tumor after focal therapy can lead to treatment failure. Furthermore, long-term safety and efficacy data for FT for various cancers is limited, largely due to the lack of randomized controlled trials comparing specific FTs to SOC therapies in assessing both oncologic and functional outcomes (76).
3 Immunological effects of focal therapy
There is a complex and dynamic interplay between the immune system and cancer. The immune system plays a central role in the balance of cancer progression and cancer suppression. Cancer manifests a variety of mechanisms that manipulate the immune system to support the cancer and suppress antitumor immunity. Adding further complexity, the treatment of cancer can also profoundly modulate the immune system, which has been studied extensively in in vivo models and in clinical studies (77). In short, there are both molecular (Table 3) and cellular (Table 4) modulators of the immune response, as depicted in Figure 1, which we will explore further below.
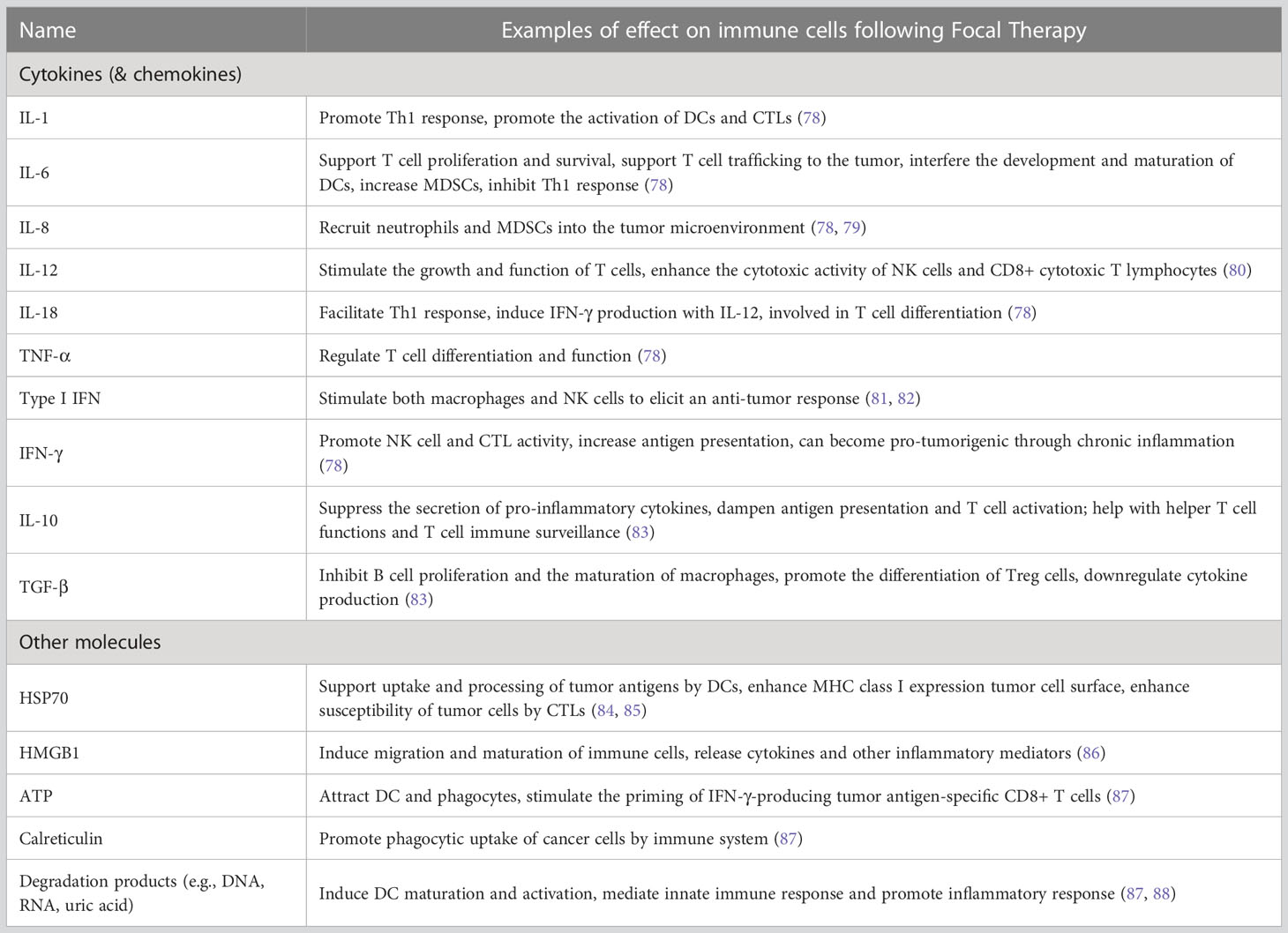
Table 3 Cytokines and other small molecules observed following focal therapy and their immunomodulatory effect.
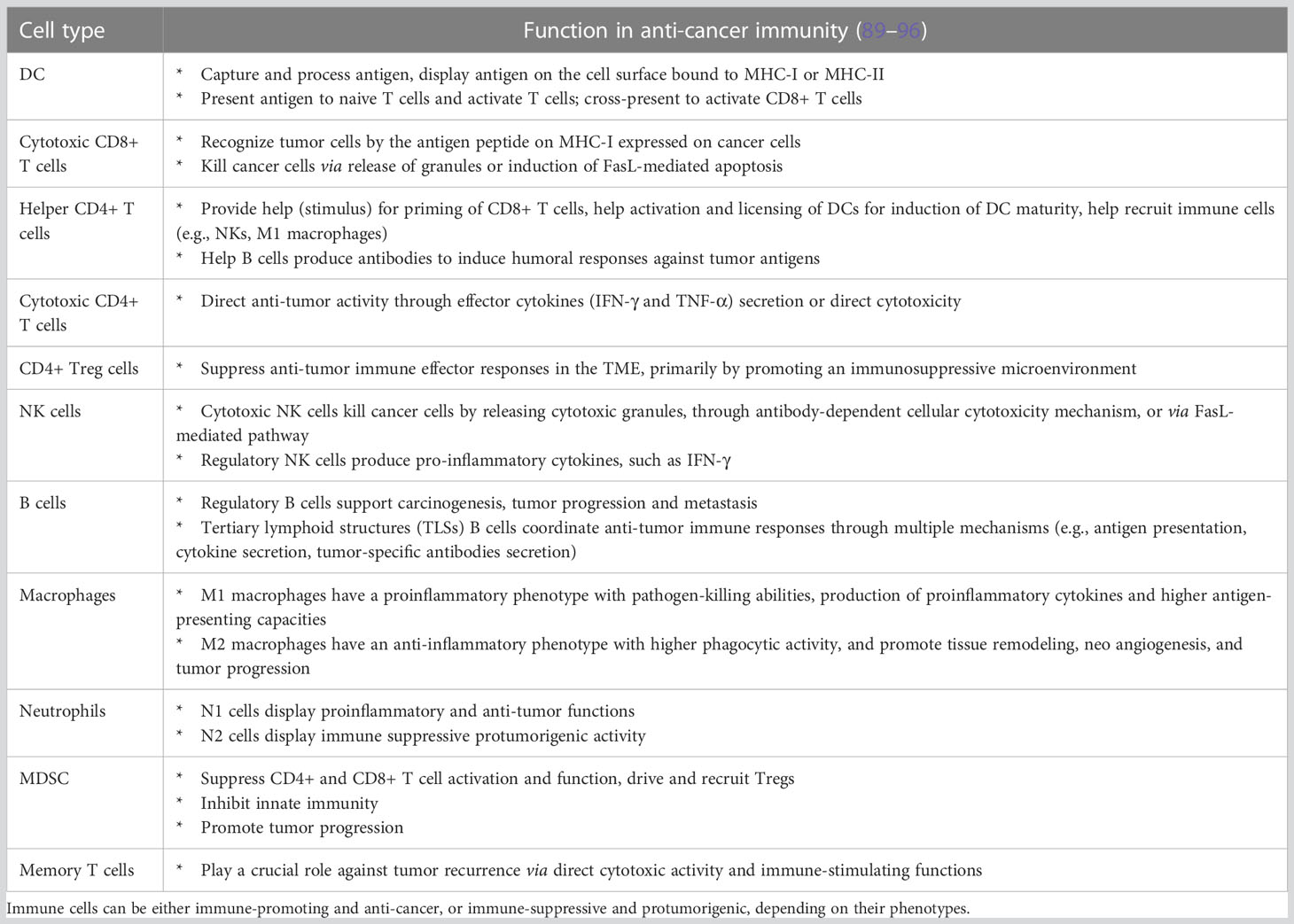
Table 4 Major immune cells involved in FT-induced anti-cancer immune response, based on their respective functional roles.
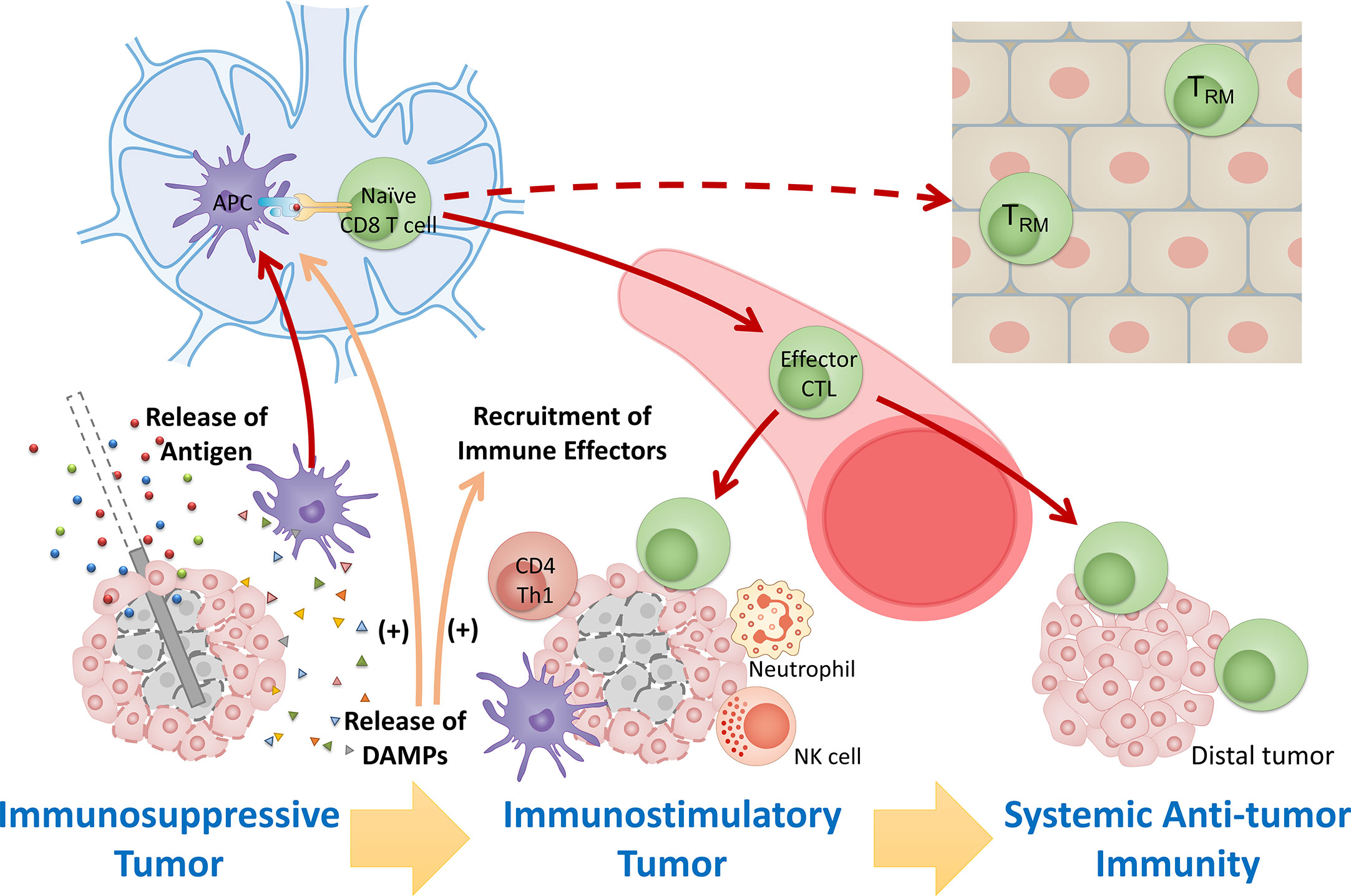
Figure 1 Overview of immunomodulatory effect after FT. Tumor destruction by FT releases large amount of tumor antigens and immunostimulatory DAMPs, leading to antigen presentation and activation of immunity, recruitment of immune effectors, and mitigation of immunosuppression. The response stimulates systemic anti-tumor immunity and induces maintenance of tissue-resident memory cells involved in local immunosurveillance, leading to potential long-lasting protection against cancer.
Cancer focal ablations dramatically change the physical tumor environment and function through the direct or indirect induction of cell death. Each type of ablation causes a distinct type of cell stress and tissue destruction with variable immunological outcomes (16). Focal ablation is set apart from conventional treatment methods (e.g., chemotherapy, surgery) due to its strong association with immunogenic cell death (ICD) and because it is characterized by the tissue injury response and wound healing processes (16, 55, 97). Therefore, FT can lead to a strong anti-tumor immunity by direct stimulation and indirect support involving both adaptive and innate immune response (10). In order to understand how focal therapy impacts the anti-cancer response at the primary tumor and, furthermore, how it can generate an effective abscopal effect and long-term immune protection, the unique features of these complex and variable interactions must be explored.
ICD is cell death characterized by molecular signals that activate innate immune cells, including antigen-presenting cells and this leads to tumor antigen presentation to T cells, activating the adaptive immune system against cancer (87, 98). ICD is mainly mediated by release of damage-associated molecular patterns (DAMPs) (87) and can strongly support the immune system in support of cancer therapy (98). It is worth noting that cancer cell death can be immunogenic or nonimmunogenic and that cell death in tumors without any intervention is common, often due to hypoxia. This type of cell death is not immunostimulatory because of the lack of DAMPs.
In addition to ICD, the immunological effects of focal therapy following acute physical destruction of a tumor are strongly influenced by the details of cell death, tissue injury, inflammation induction, and subsequent wound healing processes. Upon tissue injury, neutrophils and macrophages present in local tissue become activated, and the mast cells release cytokines and vasoactive substances. This results in the recruitment of white blood cells and the initiation of the wound healing process (99). Wound healing is typically divided into four phases: blood clotting (hemostasis), inflammation, tissue growth (cell proliferation), and tissue remodeling (maturation and cell differentiation) (100). Among these stages, inflammation is considered an important reaction after FT and may determine the success or failure of treatment. Inflammation is accompanied by the release of inflammatory mediators, vasodilation, and the migration of leukocytes, mainly neutrophils and macrophages, into the tissue (101). In addition to these various interactions with the immune system, ongoing research is also examining different pathways focal therapies modulate.
Substantial preclinical and clinical studies using different cancer models to investigate how specific focal therapies modulate antitumor immunity have demonstrated that tumor destruction by FT releases large amounts of tumor antigens and immuno-activatory DAMPs, leading to antigen presentation, activation of immunity, and recruitment of immune effectors (10, 16). These immune responses after FT potentially “reset” the tumor microenvironment from an immunosuppressive state that largely excludes invading immune cells to an immunostimulatory state that is more susceptible to local control by the immune system, further stimulating systemic anti-tumor immunity and inducing maintenance of memory cells involved in local immunosurveillance (Figure 1).
In this review, the short-term and long-term immune response initiated by cancer focal therapy are categorized into 6 aspects: (1) exposure of tumor antigens, (2) ICD and release of DAMPs, (3) antigen presentation and activation of immunity, (4) recruitment of immune effectors, (5) modulation of immunosuppressive cell types and (6) maintenance of memory cells. Here we describe the basis of each pathway and the modulations that focal therapies have in common. The DAMPs, cytokines, and chemokines that have been observed following FT together with their primary roles in the immune response against cancer are summarized in Table 3. Major immune cells involved in FT-induced anti-cancer immune response and their functions are summarized in Table 4.
3.1 Tumor antigens
Tumor antigens are proteins or carbohydrates that are recognizable by T cells or B cells as antigens and against which an antitumor immune response can be generated (102, 103). Based on expression profiles, there are two general classes of tumor antigens: tumor-specific antigens (TSAs), which are expressed only by cancer cells, and tumor-associated antigens (TAAs), which represent the mutated counterparts of proteins expressed by normal tissues and can often be expressed by many patient tumors of a given type (103). Most tumor-specific antigens are due to mutations that accumulate in cancer cells and are often termed neoantigens, which are generally specific for a given patients tumors. The expression of cancer antigens reveals the accumulation of a variable number of genetic alterations and the loss of normal cellular regulatory processes, distinguishing cancer cells from normal cells (104). These differences are fundamental for cancer cell recognition and clearance by the adaptive immune system (102).
During tumor focal destruction, when cancer cells are going through mostly necrosis, cellular contents containing tumor antigens are exposed to the extracellular space due to the loss of plasma membrane integrity, the disruption of cell organelles, and the degradation of nucleic acids and proteins (105, 106). Antigens that reside in plasma membrane blebs and apoptotic bodies can also be released after FT when the cells are undergoing apoptosis (106). These antigenic materials enter the local lymphoid drainage in the form of soluble proteins or aggregates (22). There is evidence that tumor-cell derived exosomes contain an enriched amount of tumor antigens (107) and the release of exosomes is increased when tumor cells experience stress, such as hypoxia (108) and heat (109). When antigens enter the bloodstream, they may bind to serum antibodies to form immune complexes, which have been shown to stimulate cross-presentation, CD8+ CTL responses, and cellular tumor immunity (110).
Even though antigens are released and/or exposed by all forms of focal therapy, the quantity and quality of released antigens are very different among focal therapy approaches, leading to variable immunogenicity. For example, cryoablation is considered to induce higher post-ablative immunogenicity compared to high-temperature-based methods (e.g., MWA and RFA) based on the assessment of the serum antigen level and the percentage of antigen-loaded DCs in the draining lymph node, and the general evaluation of inflammatory responses (111–113). A popular explanation is that thermal ablation tends to cause protein denaturation, therefore decreasing the amount of soluble protein accessible to the immune system, as well as reducing the antigenicity of protein (10, 111, 114, 115). Intense heat typically results in coagulation, further hindering the transport of antigenic materials. In contrast, cryogenic methods tend to preserve protein structure without significant denaturation (10, 114). In addition, IRE has been shown to release a substantial quantity of antigen to stimulate immune response (116). As an electrical-based ablation technique, IRE delivers high-voltage electrical pulses to the tumor to cause membrane rupture, induces severe leakage of intracellular contents with less drastic alterations in protein conformation compared to thermal-based methods, and presumably contributes to superior antigen release (58, 116). Furthermore, the preservation of extracellular structures, including lymphatics and blood vessels, after IRE treatment (58) can facilitate the distribution of antigens into circulation and later interaction with APC. However, it is important to avoid oversimplifying the difference in antigen accessibility by looking only at the categorization of FT therapies: comprehensive immunological studies are needed, and the details of focal therapy matter immensely.
3.2 Generation of DAMPs
DAMPs also known as danger-associated molecular patterns and danger signals, are host biomolecules released from or exposed on dying, stressed, or injured cells that act on pattern-recognition receptors to activate the innate and, subsequently, the acquired immune systems (16, 87). The generation of DAMPs is recognized as a prominent immunogenic characteristic of ICD, and DAMPs play a critical role in inflammatory responses (87, 88) and tissue healing after inflammation (88). Therefore, the role of DAMPs in cancer focal therapy immune stimulation is central.
During focal ablation, DAMPs are released from or exposed on the ablated tissue, including tumor cells, stromal cells, endothelial cells, and immune cells, as well as released due to the disruption of local extracellular matrix (117). These DAMPs then bind to phagocytosis receptors, purinergic receptors, and pattern-recognition receptors to initiate a series of reactions such as the local production of inflammatory cytokines (TNF, IL-1, IL-6, and IL-8) from innate immune cells (16, 87). Meanwhile, neutrophils, macrophages, dendritic cells, and other immune cells are recruited to the ablated site and are locally modulated by the DAMPs. The recruited cells will release cytokines and chemokines, which in turn coordinate with local cells to further modulate the immune response, ultimately leading to the activation of anti-cancer immunity (16). To some extent, the release of DAMPs, cytokines, and chemokines following FT could be used to rapidly evaluate the immunogenic effects of a treatment.
Cytokines are widely studied in the immunological response to focal ablation through evaluation of serum levels of cytokines in vivo and clinically. Heat shock proteins, ATP, HMGB1, calreticulin, and end-stage degradation products (such as DNA, RNA, and uric acid) have been largely limited to in vitro study.
Following thermal ablation, increase of pro-inflammatory cytokines (e.g., IL-1, IL-6, IL-8, IL-18, and TNF-α) are common for several hours to days (118–120), while anti-inflammatory cytokines (e.g., IL-10 and TGF-β) are also released. Cryoablation releases a similar category of cytokines, but in different quantity. For example, IL-1, IL-6, and NF-κB-dependent cytokines such as TNF-α are released in higher quantities after cryoablation than after RFA and MWA (114, 121). The large amount of pro-inflammatory mediators after cryoablation may induce a systemic inflammatory response syndrome (SIRS), leading to a phenomenon called cryoshock. Cryoshock can cause severe consequences, including disseminated intravascular coagulopathy, multi-system organ failure, or even death (122). SIRS does not occur when heat-based focal ablation techniques are used (121). After heat treatment, chemokines such as CCL2, CCL4, CCL5, and CCL10, which attract DC and T cells into the tumor, are detected (123). The release of ATP, HMGB1, and degradation products, as well as calreticulin externalization, are often detected after focal treatment is applied to cell suspension (105); however it is unclear how different ablation methods influence the amount of released molecules. Following IRE, ATP- HMGB1-mediated signaling are significantly up-regulated (124), suggesting that IRE might be an effective strategy to induce immunogenic cell death. The elevated expression of HSP 70 has been reported after both thermal ablation and cryoablation (125, 126), while the elevation in the thermal ablation group is much higher than with cryoablation (126). Furthermore, increased serum levels of HSP70 are correlated with better survival in patients treated with RFA (127).
3.3 Antigen presentation and activation of immunity
After the maturation and activation of APCs (particularly DCs) stimulated by DAMPs, dying tumor cells or released antigens are absorbed and processed by APCs, then the activated APCs travel to the draining lymph nodes, where antigens are presented to naive T cells (128). Cross-presentation and cross-priming are critically important for CD8-mediated immune response, in which MHC-I binds peptides derived from exogenous proteins internalized by endocytosis or phagocytosis and then primes naive CD8+ T cells (128). Following this interaction between peptide-MHC-I complexes and TCR, in the presence of costimulatory signals and/or cytokines, naive cytotoxic T cells become activated and proliferate. Following initial proliferation, they migrate into the circulatory system as effector T cells to identify cancer cells and ultimately eradicate tumors (128).
Antigen presentation and the activation of adaptive immunity rely on the accessibility of antigens and activation status of DCs and T cells, which are modulated by immunostimulatory signals such as DAMPs released after FT. This process is typically evaluated through the assessment of activation markers/phenotype change on DCs and T cells, as well as proliferation of T cells in lymph nodes and peripheral blood. Following cryoablation and thermal ablation in mouse models, a significant amount of antigen-positive DCs and an increase of DC activation markers (e.g., CD80/86) within the draining lymph nodes have been demonstrated (113, 129, 130). Increased tumor-specific T cell activation in regional lymph nodes and expanded CD8 T cell populations have also been widely detected after focal ablation (131). Clinically, the changes in T cell population in the peripheral blood of patients with different tumor types following thermal ablation or cryoablation have been evaluated. Peripheral blood CTL numbers and CTL/regulatory T cells (Treg) ratios increased significantly after thermal ablation but remained unchanged after cryoablation (132). In an in vitro study, IRE has shown superior induction of CTL response compared to cryoablation and heat-based treatment (116). Nevertheless, carefully controlled studies evaluating antigen presentation and activation of CTL will productively guide the choice and implementation of focal therapy to improve anti-tumor immune response.
There is a growing interest in the role of CD4+ T cells in anti-tumor immunity (133–135). Th1 type CD4+ cells most likely support a robust anti-tumor immune response, while CD4+ Treg cells are immunosuppressive (96). The influence of FT on CD4+ T cell differentiation and the role of CD4+ T cells in FT-induced immune response is being actively investigated (136, 137). For example, one study found that cryo-thermal therapy, but not RFA, led to a strong neoantigen-specific CD4+ T-cell response that mediated the resistance to tumor challenge (136).
In addition to cellular adaptive immunity, which is mediated by T lymphocytes, humoral immunity, which is an antibody-mediated response, is also involved in FT-induced anti-cancer immunity. The predominance of macrophages for the uptake of ablated tumors is more likely to induce a humoral response involving helper T cells and B cells rather than a cellular response (110).
3.4 Recruitment of immune effectors
After FT, a variety of cell types are recruited to the ablation site owing to injury response, inflammation reaction, and wound healing. These recruited cells can be immune-promoting or immune-suppressive, depending on their functional phenotype, as summarized in Table 4. FT can either promote or suppress anti-tumor immune responses, depending on the population and phenotype of recruited immune cells. Here, we consider immune effectors to be tumor-infiltrating immune cells that support immune recognition and cytotoxic function. The most important populations of immune effectors are tumor-infiltrating lymphocytes (TILs).
TILs including cytotoxic (CD8+) and helper (CD4+) T cells, B cells, and NK cells are emerging as prominent biomarkers in predicting the efficacy and outcome of treatments (138). Tumor-infiltrating CD8+ T cells have been shown to be positively associated with improved cancer prognoses after various forms of FT (18). Most FTs also have been reported to increase NK cell number and/or cytotoxic function (80, 139, 140). An increase of infiltrating Th1 CD4+ T cells and CD4+ CTL has been shown to be associated with enhanced immune response following focal ablation (105, 137). Tumor infiltrating B cells have been shown to positively correlate with overall survival in several solid tumors given its antigen presentation and tumor-killing function (141–143). Although the study of B lymphocytes in the context of FT is not common, there is evidence that HIFU ablation induced distinctive infiltration of B lymphocytes and RFA can lead to a change in the level of Ig secreted by B cells (144, 145).
In addition to TILs, DCs and M1 macrophages are also considered to be critical immune effectors contributing to the activation of TILs. Much evidence suggests that FT can promote DC activation and maturation and macrophage polarization toward M1 (10, 129, 146–148). Note that the historical and simplistic M1/M2 classification has the limitation of describing this transcriptionally dynamic cell type, as mixed phenotypes or populations with different phenotypes coexist. Neutrophil recruitment in FT-treated tumor due to injury response is commonly observed (149). However, little is known about the shift of neutrophil phenotypes (N1 or N2) (150) following FT.
3.5 Modulation of immunosuppressive cell types
Many cell types contribute to the generation of an immunosuppressive tumor microenvironment, including cancer-associated fibroblasts, myeloid-derived suppressor cells (MDSCs), Tregs, and tumor-associated macrophages (TAMs) (151). These cells are generally immune suppressive and protumorigenic. Theoretically, the elimination or reduction of immunosuppressive cell types present in the tumor bed and/or the disruption of tissue barriers can promote tumor infiltration by cytotoxic T lymphocytes, enhance anti-tumor immunity, and foster the formation of immunological memory (16).
Conflicting results have been published on how FT modulates these immunosuppressive cell types, especially for thermal ablation techniques such as RFA. For example, a number of studies have reported the reduced frequency of Treg cells in the tumor and peripheral blood after RFA, thereby promoting antitumor immunity (118, 139, 152, 153). On the contrary, some studies have shown RFA leads to the generation of an immune-suppressive environment, where immunosuppressive cell types are stimulated to proliferate, leading to tumor progression and/or aggressive recurrence (154, 155). One study pointed out that RFA leads to upregulated IL-10 and TGF-β levels, followed by a profoundly increased frequency of Treg cells in peripheral blood in a murine HCC model (156). The difference may rely on the details of RFA and the nature of the tumor.
Other modes of FTs have been more consistent in showing a decrease of immunosuppressive cell types. For instance, IRE decreases Treg cells and MDSC in the tumor bed as well as in peripheral blood (73, 83, 84). Cryoablation has also been demonstrated to induce a decrease of intratumoral Treg cells and MDSCs both in vivo and clinically (157). Cryo-thermal therapy can cause an elevated extracellular release of Hsp70 to induce differentiation of MDSCs into mature DCs, contributing to the relief of MDSC-mediated immunosuppression and ultimately the activation of a strong anti-tumor immune response (158).
3.6 Maintenance of memory cells
The maintenance of memory cells involved in local immunosurveillance is important to initiate a long-lasting antitumor immune response (16). Tissue-resident memory T cells (TRM), a subset of memory CD8+ T cells, are non-recirculating tissue-localized cells crucial for protective immunity against tumor recurrence (159). CD8+ TRM and CD8+ T cells with a TRM phenotype are typically associated with an improved prognosis in the immune response to cancer (159–164). For instance, skin TRM in local immunosurveillance has been shown to be able to promote long-term protection (165). In addition, memory and memory-Like NK cells also possess traits of immunological memory against pathogens and malignancy (166).
However, little is known about how FT contributes to the establishment of endogenous tumor-specific memory cells. A study using TRAMP-C2 prostate cancer model demonstrated an increase of non-recirculating tumor-specific CD8+ T cells in non-lymphoid tissue (NLT) distal to the tumor after IRE treatment, specifically within the salivary gland, contralateral skin, and liver (167). It remains unclear, though, whether NK cells can be modulated by focal ablation. Further studies investigating the function of memory T cells are warranted.
3.7 Additional immunological aspects following Focal therapy
In addition to these above-mentioned 6 aspects of immune response to FT, FT can also modulate the immune system through other pathways, including but not limited to (1) cell infiltration and permeability, such as changes in vascular structure and blood flow and stroma remodeling; (2) modulation of gene expression of cancer cells and immune cells; (3) changes in metabolism, such as tumor hypoxia; and (4) tissue damage and remodeling.
It has been shown that changes in temperature can cause a wide range of changes to the tumor microenvironment. For example, hyperthermia is usually accompanied by increased blood flow, resulting in increased oxygenation and intense infiltration of inflammatory cells and tumor-infiltrating lymphocytes (TILs) (10, 16). Hyperthermia also increases the surface expression of MICA and MHC-I on tumor cells, making tumor cells more sensitive to lysis by NK cells and CD8+ T cells (168, 169). Hyperthermia also stimulates the functional activity of NK cells, macrophages, and dendritic cells (168). Non-thermal ablation techniques such as IRE have also been shown to modulate the immunosuppressive tumor microenvironment to relieve intratumoral hypoxia, which suppresses immune cells. Several components of the fibrotic stroma were also downregulated, facilitating the infiltration of cytotoxic T lymphocytes (170).
4 Focal therapy combined with immunotherapy
Combining FT with immunotherapy may improve the potential of focal therapy to eliminate established tumors and prevent tumor recurrence. By taking advantage of focal ablation’s ability to induce the activation of an anti-cancer immune response, the efficacy of immunotherapy can be improved. Here, we examine different types of cancer immunotherapies and the status of preclinical and clinical research combining the two forms of cancer treatment.
4.1 Cancer immunotherapy
Cancer immunotherapies basically work through stimulating effector mechanisms and/or counteracting inhibitory and suppressive mechanisms. The NIH categorizes immunotherapy into 5 types: monoclonal antibodies, treatment vaccines, immune system modulators, T cell transfer therapy, and immune checkpoint inhibitors (171), as summarized in Table 5. Immunotherapy has emerged as the fourth pillar of cancer treatment, alongside surgery, radiation, and chemotherapy.
4.2 Current state of focal therapy combined with immunotherapy
The immune responses after focal ablation monotherapy are usually weak and rarely induce clinically relevant antitumor effects. Different modalities of immunotherapy have been combined with focal therapy to stimulate a more robust anti-tumor reaction with the hope of a systemic immune response, as summarized in Table 6. The majority of cancer immunotherapies combined with FT function through stimulating immune response components to augment anti-tumor immunity. Many proof-of-principle, animal, or preclinical studies have shown promising results, demonstrated by the improvement of DC number and/or function, increase of tumor-specific CTL response, tumor growth suppression, enhanced survival, and even inhibited metastasis (105, 157). Hundreds of clinical trials are in progress to test this combinatorial therapy in various cancer types for patients. So far, most publications have been limited to safety and tolerability. For the translation of the results seen in animals and small groups of patients, larger prospective trials need to be designed to first examine safety and efficacy before moving into randomized controlled settings. Finally, large-scale randomized controlled trials are needed to determine the clinical benefit of combined treatment.
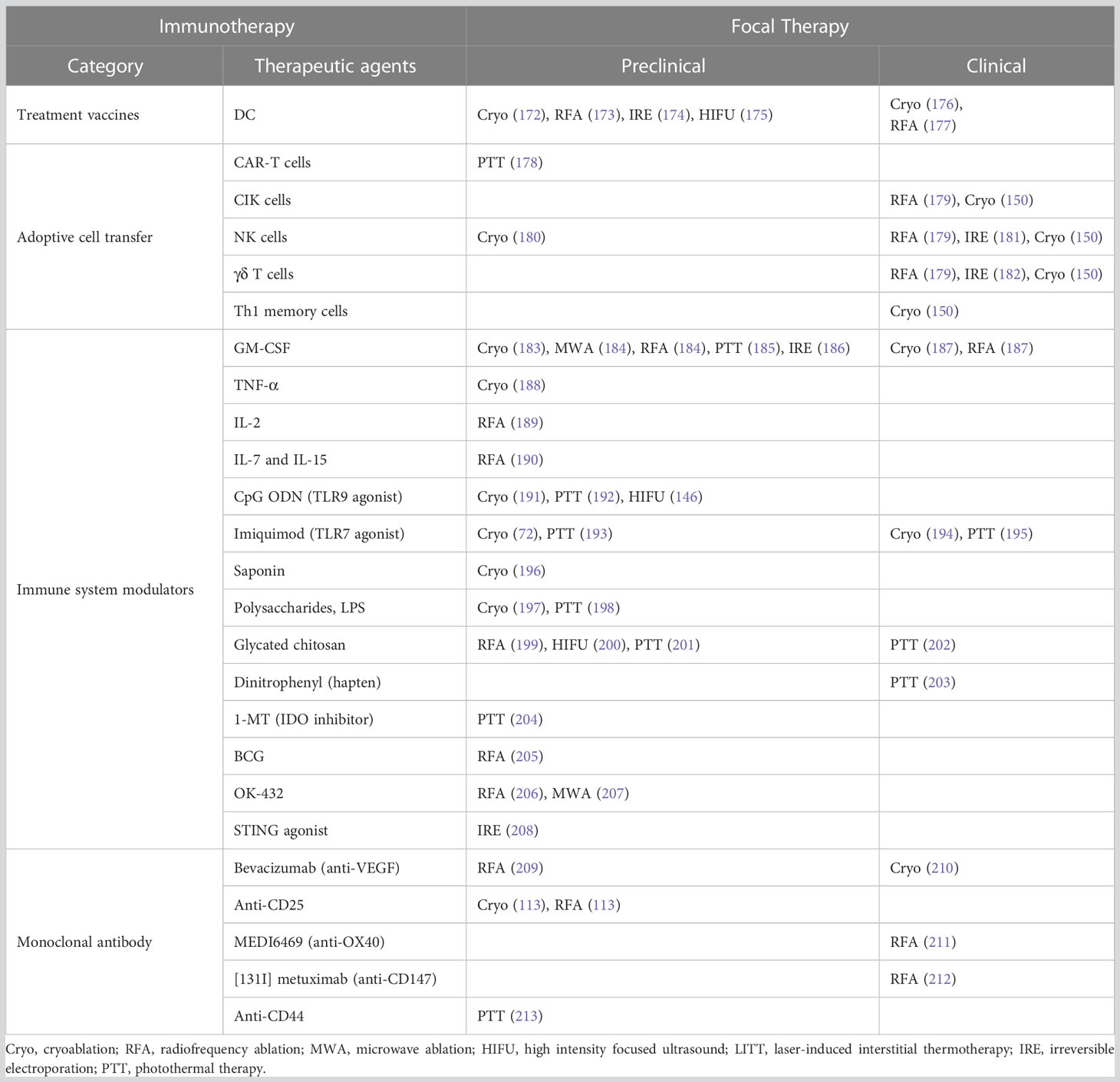
Table 6 Selected examples of studies combining immunotherapy (except for ICIs, which is discussed in section 6) and focal therapy.
5 Immune checkpoint inhibitors (ICIs)
Immune checkpoints are crucial to the immune system for maintaining self-tolerance and modulating the duration and amplitude of physiological immune responses in order to prevent auto-immune disease (214). When checkpoint and partner proteins bind together, they send inhibitory signals to T cells. It is now clear that cancer cells harness immune-checkpoint pathways as an important mechanism of immune resistance (214). Therefore, checkpoint inhibitors that target these receptors or ligands hold promise as cancer treatments. Immune checkpoint blockade removes inhibitory signals to anti-tumor T cells, which enables tumor-specific T cells to overcome regulatory mechanisms and results in a broad enhancement of T cell-mediated immune responses (215).
Among identified immune checkpoints, cytotoxic T-lymphocyte-associated protein (CTLA-4) and Programmed cell death protein 1/Programmed death-ligand 1 (PD-1/PD-L1) are the most common targets in checkpoint blockade therapy. In general, it is perceived that CTLA-4 predominantly regulates early-stage T cell activation, whereas PD1/PD-L1 primarily regulates effector T cell activity within tissue and tumors (215, 216).
5.1 CTLA-4 pathway
During antigen recognition, T cells are activated when T cell receptors (TCRs) bind to antigen displayed in the MHC on APCs in concert with CD28:B7-mediated costimulation (216). CTLA-4 is a CD28 homolog with much higher binding affinity for B7 ligands (217). In resting T cells, CTLA-4 is an intracellular protein; however, after TCR ligation and a costimulatory signal through CD28, CTLA-4 expression is upregulated by translocation to the cell surface and decreased internalization (216). CTLA-4 outcompetes CD28 for binding to critical costimulatory molecules (B7-1/B7-2, also called CD80 and CD86) on APCs and mediates inhibitory signaling into the T cell, resulting in arrest of both activation and proliferation (215). CTLA-4 is also expressed by Tregs constitutively and contributes to their inhibitory functions (217). Anti-CTLA-4 antibodies block the activation of the CTLA-4 pathway, allowing for the activation and proliferation of more T-cell clones and reducing Treg-mediated immunosuppression (215, 217, 218).
5.2 PD-1/PD-L1 pathway
PD-1 is a member of the B7/CD28 family of costimulatory receptors (217). It regulates T-cell function through binding to its ligands, programmed death ligand 1 (PD-L1), and programmed death ligand 2 (PD-L2), which are widely expressed in nonlymphoid tissues such as cancer cells, macrophages, and myeloid cells (215). PD-1 expression on T cells is induced when T cells become activated. Upon engagement with PD-L1 and PD-L2, PD-1 is thought to primarily transmit a negative costimulatory signal through the tyrosine phosphatase SHP2 to attenuate T-cell activation and hinder cytolytic capacity (219). PD-1 expression is a hallmark of “exhausted” T cells (217), which are effector T cells with overexpressed inhibitory receptors and decreased cytokine production and cytolytic activity (220). Unlike CTLA-4, which is confined to T cells, PD-1 is also expressed in non-T lymphocyte subsets, including B cells and NK cells (214). PD-1 pathway blockade restores the activity of anti-tumor T cells that have been turned off, thus boosting an effective immune response (215, 217). Additionally, it has been pointed out that PD-1 is highly expressed on Treg cells, where PD-1 blockade actually amplifies Treg cells thus opposing the immune benefits (221).
5.3 FDA approved ICIs
With ample evidence suggesting that T cell response modulated by checkpoint blockade can promote durable cancer remission, the US Food and Drug Administration (FDA) has approved nine ICIs for targeted diseases (222), as summarized in Table 7.
5.4 Emerging inhibitory checkpoints
In addition to the well-known CTLA-4 and PD-1 pathways, other immune checkpoints such as LAG-3, TIM-3, TIGIT, and VISTA are considered as promising immune therapy targets, and numerous antibodies have been developed to regulate these pathways (223, 224). Recently, the USFDA approved the combination of Nivolumab and the first lymphocyte-activation gene 3 (LAG-3)-blocking antibody, Relatlimab (BMS986016), as treatment for patients with unresectable or metastatic melanoma (Table 7) (225). Opdualag is also being studied in clinical trials of other cancers, including lung, colorectal, and liver cancer (226). Encouraged by the success of Relatlimab, additional drugs targeting LAG-3 and other novel immune checkpoints are being evaluated for the treatment of multiple types of cancers. We present a list of drugs that have entered clinical trial (223, 227–230) in Table 8. As of the writing of this review, hundreds of clinical trials are being carried out to evaluate the efficacy of these novel ICIs as monotherapy or in combination with currently approved ICI drugs.
ICIs can be used in combination for better efficacy and response by targeting different checkpoints simultaneously. The combination of CTLA-4 and PD-1/PD-L1 blockers has been the most extensively researched regimen (231). For example, the combination of ipilimumab plus nivolumab has been approved by the USFDA for the treatment of advanced melanoma, advanced RCC, MSI-H/dMMR metastatic colorectal cancer, HCC, metastatic NSCLC, and malignant pleural mesothelioma (232). In addition to therapies utilizing CTLA-4 and PD-1/PD-L1 blockade, other combinations targeting multiple checkpoints such as LAG-3/PD-L1, TIM-3/PD-1, B7-H3/CTLA-4, and VISTA/PD-1 are undergoing pre-clinical studies and clinical trials (233).
Our knowledge of the fundamental biological roles of these molecules remains limited and, in many cases, is being outpaced by clinical investigation. Deeper understanding of the basic biology is in urgent need for the rational development of new immune checkpoint blockade therapies and combinatory approaches. Meanwhile, ongoing efforts in preclinical and clinical studies are expected to reveal mechanisms of using these novel ICIs to enhance anticancer immunity.
5.5 Limitations of ICI monotherapy
Although ICIs have been used widely and show great promise for improving the outcome of cancer treatment, this therapy is limited by low response rate. Many patients exhibit innate resistance and disease progression. For example, among all cancer types, anti-CTLA-4 drugs have the highest objective radiographic response of 15% in advanced metastatic melanoma (216). Anti-PD-1 ICIs (Pembrolizumab and nivolumab) have high response rate (about 80%) in Hodgkin’s disease, but intermediate response (10-45%) in melanoma, non-small cell lung carcinoma (NSCLC), bladder and urinary tract cancer, and triple-negative breast cancer (TNBC) patients (216, 219).
Immune-related adverse events (irAEs) associated with ICIs is another concern for clinical use, due to overactivation of the immune system in almost any organ of the body. IrAEs can occur at any point along a patient’s treatment course. The most common toxicities during the treatment using CTLA-4-blocking antibodies include enterocolitis, inflammatory hepatitis, and dermatitis. The most common adverse events of anti-PD-1 ICIs are fatigue, diarrhea, rash, and pruritus (216, 234, 235). Serious organ inflammatory toxicities that can be life-threatening are uncommon, but this risk may be higher with combination ICI treatments. ICI toxicity can be mitigated by high doses of corticosteroids to suppress the immune response, but these in turn can attenuate the anti-cancer immunity (216, 234).
Therefore, the evaluation of biomarkers that can predict tumor response to ICI treatment is necessary to avoid overtreatment of ICIs and minimize side effects. Emerging research has focused on identifying predictive markers and combinatory approaches to improve the relatively low response rate of ICIs (236–238).
5.6 Biomarkers to predict ICI therapy response
There are a wide range of biomarkers being used to predict the ICI therapy response. Tumor mutational burden (TMB, the number of somatic, coding, base substitution, and indel mutations per megabase of genome examined) is one of the biomarkers (237). For colorectal cancer (CRC), anti-PD-1 and anti-PD-L1 therapy often generates a durable response in patients with mismatch repair-deficient/microsatellite instability high (dMMR/MSI-H) CRC, which is a tumor subtype with high TMB, compared to failure in microsatellite stable (MSS) CRC, which has a significantly lower TMB (239, 240). Yarchoan et al. have analyzed 27 tumor types or subtypes and observed a significant correlation between the tumor mutational burden and the objective response rate to anti-PD-1 or anti-PD-L1 therapy (P<0.001) (237).
Certain cell surface markers have also been identified as biomarkers for ICI responsiveness. For example, PD-L1 expression has been vital to predicting tumor response to anti-PD-1 or anti-PD-L1 antibodies in melanoma and NSCLC (241, 242). PD-L1 expression is currently used to guide treatment decisions and regulatory approval. However, its expression can be upregulated by several factors including interferon gamma (IFN-γ) (243) and may vary over time and across multiple tumor sites. Upregulation of other inhibitory molecules such as TIM-3, LAG-3, and VISTA can also cause resistance to anti-PD-1/L1 antibody therapy (244). A study examining 45 FDA ICI approvals between 2011 and 2019 across 15 tumor types found that PD-L1 expression was predictive of response to PD-1/L1 ICI in only 28.9% of cases (245), suggesting the complexity and difficulty of identifying putative protein and epigenetic markers for patient stratification and potential therapeutic targeting.
Immune cell infiltration is another important predictive biomarker considering the mechanism of ICI therapy. Generally, a higher number of tumor-infiltrating lymphocytes (TILs) has been a favorable prognostic factor in many types of cancers, including melanoma and colorectal cancer (246, 247).
Taken together, “hot tumors,” characterized by high tumor mutational burden, increased expression of PD-L1 and IFN-γ signaling, and high T-cell infiltration are associated with better ICI efficacy (248, 249). In contrast, for patients with “cold” tumors such as prostate, pancreas, and brain cancers, checkpoint inhibitors are typically ineffective (249).
5.7 The basis of combinatory approach with focal therapy
To improve the benefit of ICI immunotherapy, especially to increase the objective response rate and reduce irAEs, substantial efforts are focused on combination strategies aimed at turning “cold” tumors into “hot” tumors (249) by changing the immunosuppressive TME or targeting other pathways that potentially inhibit the activation of T cells (250).
Immunomodulation by energy-based focal therapy, as we discussed previously, is well aligned with the strategy of combination therapy with ICI to improve tumor immunogenicity (Figure 2). In the following sections, we will discuss preclinical study and clinical trials that utilize this principle and the process in fulfilling the synergy between FT and ICI.
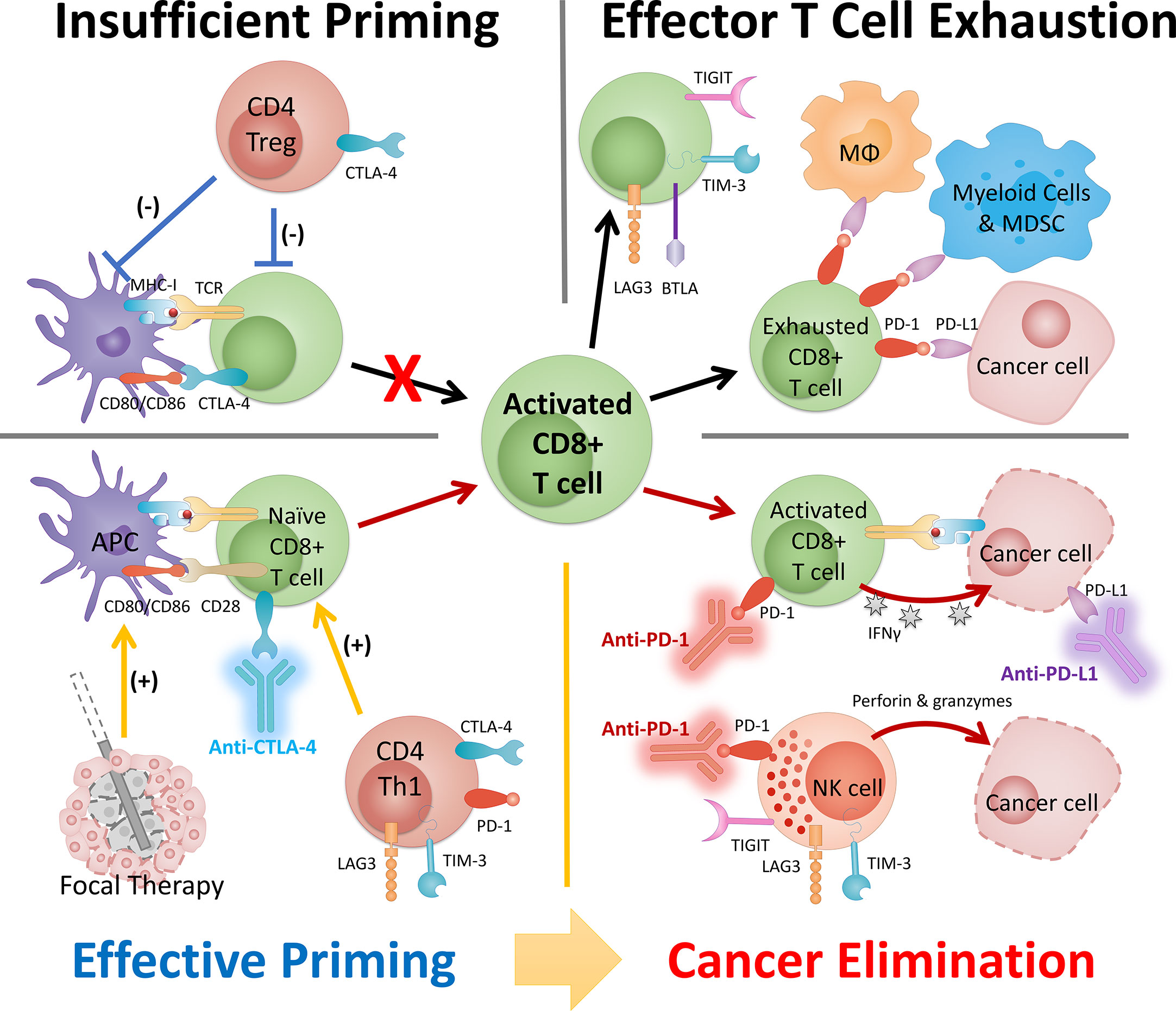
Figure 2 The synergy between FT and ICI serves as the basis of a combinatory approach in cancer management. The success of this combinatory strategy relies heavily on the activated CD8 T cells (i.e., population, location, and phenotype). The status of CD8 T cells is dictated by the cancer property (e.g., tumor type) and disease status and can be strongly modulated by intervention. Insufficient priming can be addressed by focal therapy and early intervention of anti-CTLA-4 and other ICI targeting Th1 CD4 T cells. T cell exhaustion can be mitigated by careful engineering of FT and ICIs (primarily anti-PD-1/PD-L1) that targets pathways preventing T cells to eliminate cancer. MDSC, myeloid-derived suppressor cells; MΦ, macrophage.
6 Preclinical in vivo research combining focal therapy and ICI
6.1 Overview of preclinical approaches
The keywords, database, and inclusion criteria for the literature research are summarized in Supplemental Table S1. A wide range of focal therapies have been combined with ICI therapy in various cancer models in preclinical studies. Melanoma (B16 and its derivatives), breast cancer (4T1 and NDL), HCC (Hepa1-6, H22, etc.), colorectal cancer (CT26 and MC38), prostate cancer (TRAMP, RM-1, and MycCap), and pancreatic cancer (KPC and its variants, KRAS*, etc.) are the most used cancer models to evaluate the benefits of combination therapy. Almost all the cancer models are syngeneic to corresponding mice strains (e.g., C57BL/6, BALB/c, and A/J) and were introduced by injecting cancer cells to form orthotopic or subcutaneous tumors.
The most targeted checkpoints are CTLA-4 and PD-1. ICI monoclonal antibodies, almost all purchased exclusively from Bio X Cell, are typically administered through intraperitoneal injection after FT at 100 or 200 µg per mouse for 3 or 4 total injections (Supplemental Table S2).
To evaluate the performance of combination therapy, researchers usually monitor the growth of the primary tumor and re-challenge long-term surviving mice with the same and/or different tumor cells to see whether this approach could improve primary tumor control and induce long-term protection against specific cancers. Some groups have also used a metastasis model or inoculated contralateral tumor to show that the combinatorial approach could enhance abscopal effect. Following the course of treatment, assessment of the immunomodulatory effect typically focuses on the CD8+ and CD4+ T cells in the tumor, spleen, and lymph nodes. Immunosuppressive cells and cytokines are also analyzed, as summarized in Supplemental Table S2.
6.2 Summary of preclinical findings
Published preclinical results suggest that FT combined with ICI is a promising treatment approach for multiple pre-clinical cancer models, as summarized in Table 9. Most of the research achieved 2 out of 3 of the following: (1) improved primary tumor control, (2) protection from tumor re-challenge, and/or (3) enhanced abscopal effect. It is worth noting that in all the cancer models, complete elimination of primary and systemic cancer is not guaranteed by monotherapy, but the chances have been greatly increased by the combinatory approach.
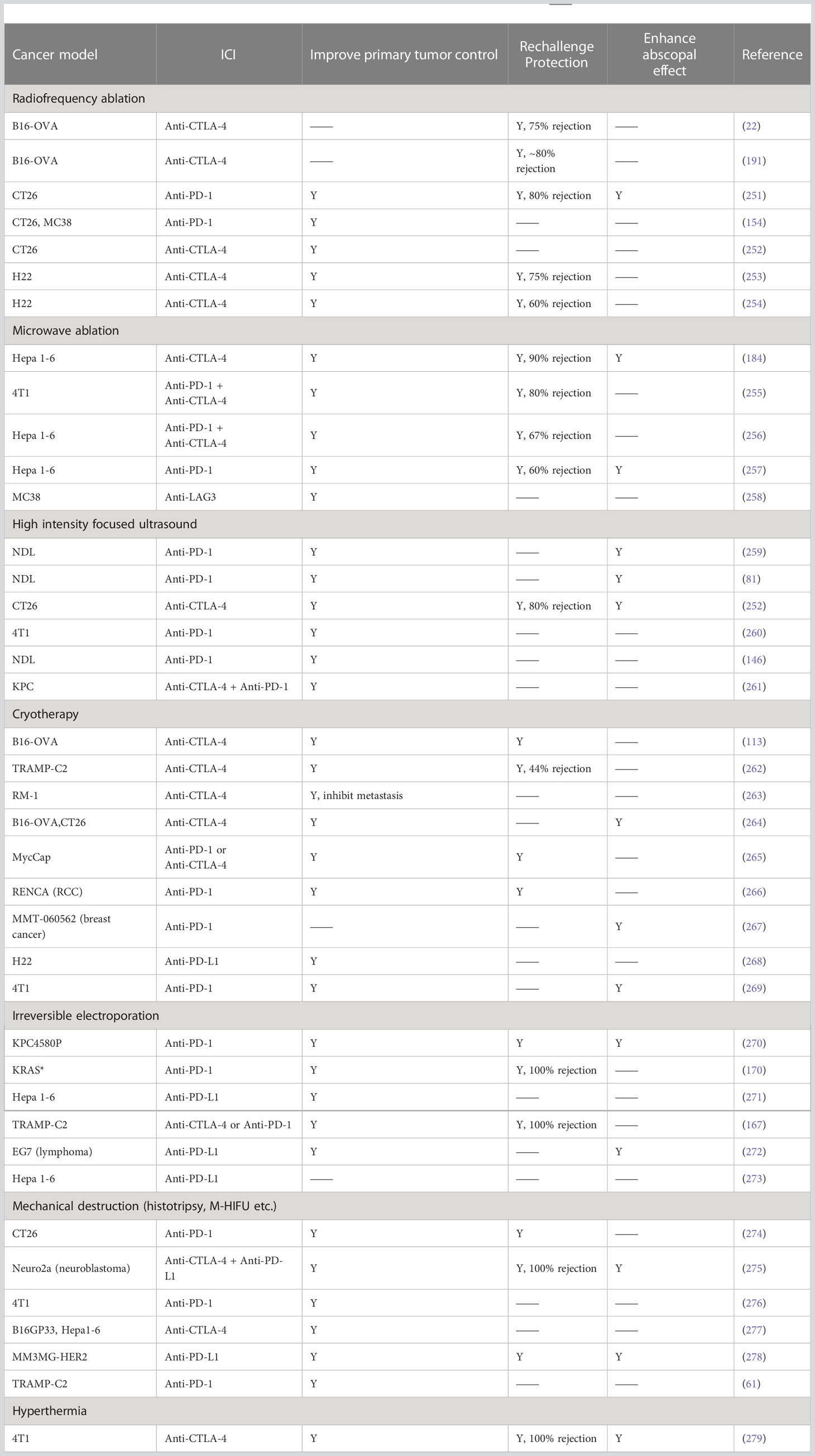
Table 9 Materials and methods of combinatory FT and ICI in preclinical models. Y, results observed. ―, no data.
The induced immune response of thermal ablation, most commonly RFA and MWA, is considered incapable of complete eradication of established tumors or durable prevention of disease progression (130). With the addition of ICI, positive prognostics are often observed. Anti-CTLA-4 and RFA in the B16-OVA model have been shown to augment the anti-tumor effect of splenocytes, which resulted in long-lasting tumor protection (22) and regulatory T cell depletion in addition to increased tumor-specific T cell numbers, therefore protecting mice from tumor challenges (113). Anti-PD-1 in tandem with RFA in a colon cancer model has been reported to result in stronger anti-tumor immunity, demonstrated by prolonged survival and reversed immunosuppression in distant lesions (251). Combined ICI and MWA share similar trends: anti-CTLA-4 co-administered with MWA and GM-CSF contribute to 90% secondary tumor rejection in Hepa 1-6 model mice (184). Further studies revealed that the tumor-specific augmentation is supported by NK, CD4+, and CD8+ T cells (255, 256).
Some successes have also been reported on HIFU working well with ICI immunotherapy. Extended survival with partial tumor ablation, abscopal effects, and inhibition of re-challenged tumors have been reported. These observations are correlated with systematically activated DCs and TILs and downregulated Tregs (252, 275).
In contrast to thermal ablation such as RFA and MWA, which relies on a temperature typically above 60 ˚C, local hyperthermia (42.5 ˚C for 20 min) with anti-CTLA-4 was also shown to enhance the anti-tumor response (279). Other studies generate local hyperthermia using photo-absorber dye (photothermal therapy) combined with ICI treatment to stimulate tumor-specific immune responses (35, 36). These studies, based on various heating methods, demonstrate that thermal therapy is capable of magnifying the impact of ICI immunotherapy, especially for treating “cold” tumors.
Cryoablation has been described to exert both immunostimulatory and immunosuppressive effects due to the specific physiological mechanisms of cold injury (105, 130). Some studies show that cryoablation has no effect on the growth of distant secondary tumors or on increased tumor-specific CD8 T cells (130, 262). However, the therapeutic effect of cryoablation is substantially improved with anti-CTLA-4: secondary tumors are significantly slowed or prevented (262, 263). Compared to cryoablation monotherapy, secondary tumors in the combination group were highly infiltrated by CD4 T cells and CD8 T cells, and there was a significant increase in the ratio of intratumoral T effector cells to FoxP3(+) Tregs (262).
Despite the unique advantage of IRE over thermal-based ablation, especially in pancreatic cancer, IRE alone is insufficient to eradicate remote micrometastatic lesions completely, and most patients receiving this therapy develop distant progression (280). Synergizing IRE with ICI immunotherapy to address local and systemic cancer has a huge potential for clinical translation in PDAC. In one example, IRE reverses resistance to immune checkpoint blockade in a murine orthotopic PDAC model with a long-term memory immune response (170). In another example in prostate cancer, IRE and anti-CTLA-4 increased intratumoral tumor-specific T cells and increased tissue-resident CD8+ memory T cells (TRM) in non-lymphoid tissues including skin. Mice that had previously achieved complete remission following dual IRE + anti-CTLA-4 therapy were also 100% protected from secondary tumor challenge (167).
The immunologic effects of mechanical ablation (including mechanical HIFU, boiling histotripsy and cavitation-cloud histotripsy) are less well characterized. This non-thermal ablation process can increase the expression of both CTLA-4 and PD-1 pathway receptors (281), which serves the basis of synergistic effect with ICI.
6.3 Nanoparticle-mediated hyperthermia
In recent years, the number of preclinical studies combining NP-mediated hyperthermia therapy with ICI has increased rapidly, especially with a large number of studies from China, as summarized in Supplemental Table S3, NP-mediated hyperthermia therapy + ICI in vivo research on animals. In preclinical studies, NP-mediated hyperthermia, both photothermal therapy (PTT) and magnetic hyperthermia (MHT), combined with ICI has been demonstrated to enhance therapeutic outcomes, reverse tumor-mediated immunosuppression, result in therapeutic effect for both primary tumors and metastatic lesions, prevent cancer recurrence, and prolong the survival period (282, 283).
As shown in Supplemental Table S3, among numerous nanoparticles used for hyperthermia therapy with ICIs, near-infrared lasers using gold nanoparticles are most common. A wide range of tumor models are used: most of them are orthotopic or subcutaneous syngeneic tumors. Among all the cancer models, the most studied is 4T1 (breast cancer), followed by CT26 (colorectal cancer) and B16 (melanoma). These 3 models make up more than 80% of the preclinical studies on the list. Different from ablative thermal therapy, where temperatures greater than 55°C are common, these NP-mediated hyperthermia therapies typically operate at a lower temperature. Most of the literature has reported enhanced tumor antigen-specific T cell responses, inhibition of Treg cell functions, promoted M1 macrophage differentiation, and improved tumor-infiltrating lymphocytes (284).
Compared to conventional hyperthermia, NP-mediated hyperthermia offers several advantages: in theory, the cancer cells can be selectively targeted and ablated at microprecision scale due to the propensity of NPs to extravasate from the tumor vascular network in some cancers and accumulate in and around cancer cells (285). The construction of NPs can be easily modified according to therapeutic needs; for example, anti-cancer drugs can be linked to or carried by NPs to enhance tumor killing and immune activation. The versatility of multifunctional NPs has enabled the integration of hyperthermia with other treatment approaches such as photodynamic therapy (PDT), chemodynamic therapy (CDT), and even immunotherapy within the same platform.
As of the writing of this review, there has been no clinical trial combining NP-mediated hyperthermia with ICI. The intrinsic limitations of both PTT and MHT are barriers preventing large-scale clinical translation from small animal models, even when combined with immunotherapy. First, uniform and fast energy deposition to the entire tumor remains challenging: the variation of NP concentration within the tissue, the limited tissue penetration depth (a few millimeters) of light for PTT and the poor magnetothermal conversion efficiency for MHT all severely dampen the treatment effect. Second, effective delivery of nanomedicine remains a major challenge. Only a fraction of intravenously administered nanomaterials can be delivered to the tumor regions, while the majority are absorbed by the reticuloendothelial system during blood circulation, followed by clearance from the body (285). At the same time, the biosafety of nanomedicines needs to be assessed systematically in order to prevent severe treatment-related side effects. Most animal studies have utilized intratumoral delivery of the NP agent to guarantee enough NPs located in the tumor, which is not always feasible for clinical cases. Third, the immunomodulatory effects of NP-mediated hyperthermia need to be mechanistically studied and understood. The success of these combination approaches appears to be linked to a combination of factors related to the hyperthermia response and specifics of each nanoparticle platform, necessitating an understanding of the mechanisms of interplay between hyperthermia and nanoparticle design.
7 Clinical research combining focal therapy and ICI
7.1 Clinical approaches of focal therapy and ICI
7.1.1 Overview of clinical trials
The keywords, database, and inclusion and exclusion criteria of the literature research of clinical trials are summarized in Supplemental Table S4. When more than one report of the same study was available, the most recent data (with longer follow-up and/or higher number of patients) were included.
The keyword search identified a total of 55 studies by Sept 15, 2022, as summarized in Table 10. Five were excluded as they were withdrawn or terminated before completion, which would not yield meaningful results. The 50 clinical trials included were sorted by FT type and ICI type, separately. In general, the number of ongoing clinical trials has increased steadily each year since 2011 (Figure 3A). Seven different types of FTs and 11 distinct ICIs have been used in the identified trials. Several studies involved the comparison of different FTs or ICIs, and 10 out of the 50 used more than 1 ICI in the treatment arm.
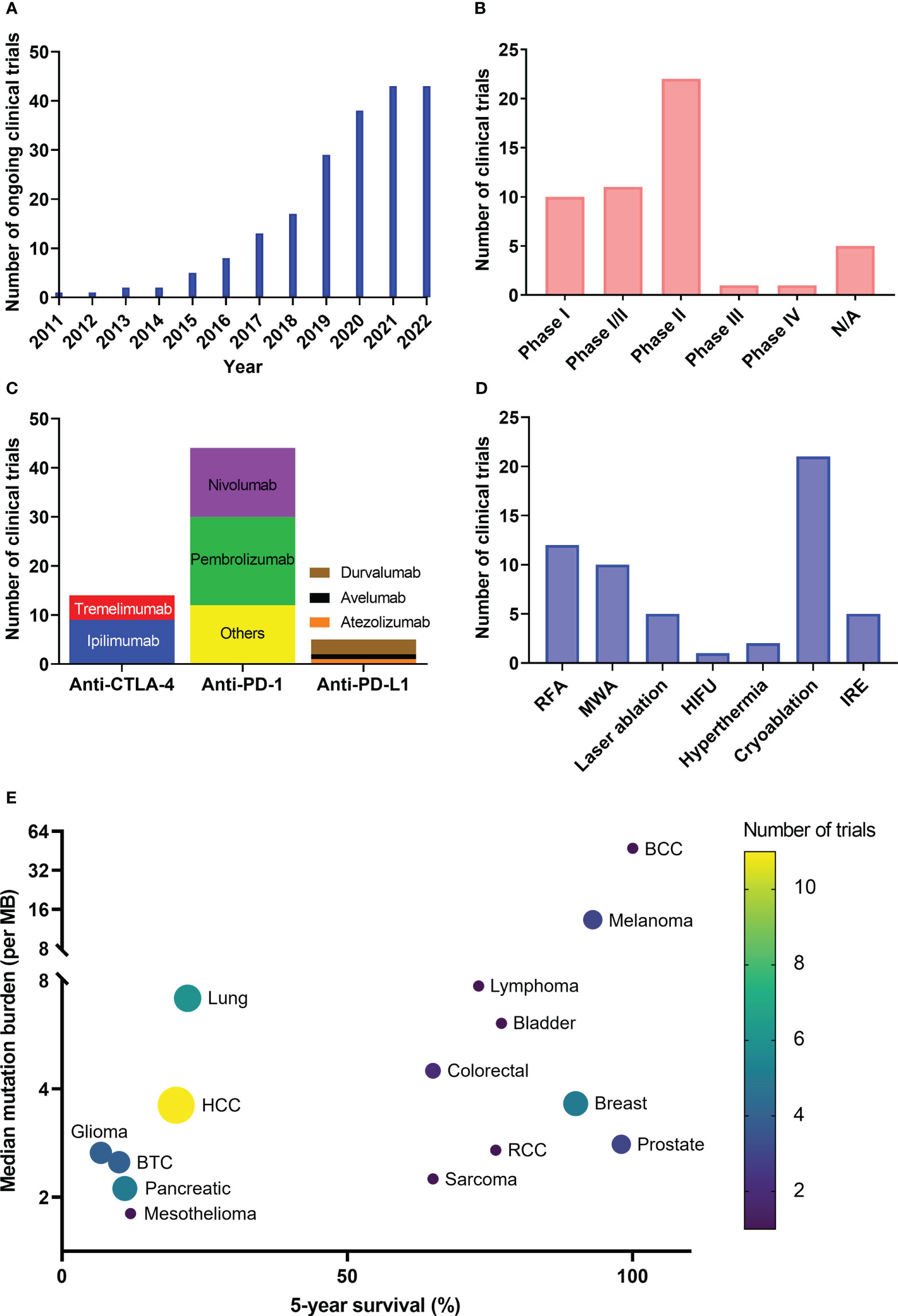
Figure 3 Clinical trial summary. The numbers of clinical trials are grouped by year (A), clinical phase (B), use of ICI drug (C), focal therapy modality (D) and targeted diseases with their tumor mutation burden and 5-year survival (E). BCC, basal cell carcinoma; BTC, biliary tract carcinoma; HCC, hepatocellular carcinoma; RCC, renal cell carcinoma.
The majority of trials were in phase I or II or a combination of phase I and II, enrolling small cohorts, typically fewer than 50 patients. One phase III and one phase IV trial (both outside U.S.) were identified (Figure 3B). As of September 2022, more than half of the trials were still recruiting, and only 11 trials have been completed. In general, most of these studies are in the early phase, and there is indispensable need for randomized phase III trials to confirm the clinical efficacy of these novel combinations.
7.1.2 ICI used in clinical combination therapy
Most of the clinical trials used anti-PD-1 drugs (Pembrolizumab or Nivolumab) or anti-CTLA-4 drugs (Ipilimumab) in combination with FT, which are the first three ICI drugs approved by the FDA. The number of ICIs that are used in combination treatment has been increasing during the past 10 years. Although ipilimumab was the only ICI involved in a clinical trial in 2011, 11 distinct ICIs have been included in clinical trials by 2022 along with the thriving development of ICI drugs worldwide (Figure 3C). Anti-PD-1 antibodies were the most frequently used ICIs across all studies, with Pembrolizumab included in 18/50 trials, followed by Nivolumab (14/50), due to a number of factors including the broad applications (Table 7), the largest market share in ICI (286) and being manufactured by numerous pharmaceutical companies globally.
Dual immunotherapy using an anti-PD-1/L1 antibody and an anti-CTLA-4 antibody with FT was identified in 10/50 trials. Ipilimumab + Pembrolizumab/Nivolumab (7/50) were the most common double-agent immunotherapies along with FT. The application of dual combinations is encouraged by the evidence that combination anti-CTLA-4 plus anti-PD-1 checkpoint blockade has shown enhanced efficacy compared to monotherapy in a wide range of cancer types (287–289). In addition, multiple studies included other immunotherapies such as CIK therapy and dendritic cell injection in order to further enhance anti-tumor immune response.
7.1.3 FT used in clinical combination therapy
Cryoablation, which has been used in 21 trials (19 single FT + 2 more than one FT), has been the most commonly studied FT modality (Figure 3D), followed by RFA, MWA, IRE, LITT, hyperthermia, and HIFU. Also, cryoablation has been used in combination with 8 ICIs reported in this study, probably owing to the solid understanding of cryoinjury and the long history of this technique being applied in numerous diseases (Table 2). Among identified clinical trials, the combination of Pembrolizumab/Ipilimumab and cryoablation was most frequently studied.
The choice of FT in combination for specific disease is largely based on the established clinical benefit of locoregional therapy (LRT) monotherapy, which depends on the properties of the targeted tissue and the FT mechanism, as we reviewed in section 2. For example, RFA, MWA and cryoablations were used on primary liver tumors, while cryoablation was the most frequently studied FT modality to be combined with ICIs for primary breast cancer.
7.1.4 Combination therapies benefits a broader range of cancer patients
The combination of FT and ICI is likely to benefit a broader range of patients than monotherapy, reflected by the widening scope of indications for which this treatment has been tested. In Figure 3E, we map the number of clinical trials on both TMB and 5-year survival for each specific cancer type. The references for mutation burden and survival data are summarized in Supplemental Table S5.
Combination therapies greatly expand the application of ICI, offering opportunities to treat a wider variety of cancer where ICI monotherapy is ineffective. When used alone, ICI therapy has been mostly limited due to low response rates; the FDA-approved indications focus on “hot” tumors with high TMB. Cancer types with higher response rates to ICI largely associated with their TMB, including melanoma, lung cancer, and colorectal cancer, have been treated with combination therapy. In addition, certain cancers with lower ICI response rates due to their relatively low mutation loads, such as prostate cancer, pancreatic cancer, and MSS CRC, are being treated in clinical trials with combination therapy. Still other cancer types, including HCC, BTC, and glioma are benefiting from combination therapy with ICI, mainly owing to the crucial role that LRTs have played in managing these diseases.
LRT monotherapies are usually considered in patients with unresectable local disease, as either a conservative approach (high 5-year survival, such as breast cancer and prostate cancer) or a palliative or even “last-ditch measure” (low 5-year survival, such as glioma and pancreatic cancer), according to NCCN guideline. In comparison, the combination therapies are being evaluated as a first- or second-line treatment: not limited to primary tumors but also used for recurrent and/or metastatic disease.
Taken together, combination therapy shows promise for mitigating the limitations of both ICI and FT monotherapy to expand their application. This strategy not only makes existing monotherapy more effective, but more importantly, opens up possibilities for a broader range of cancers.
7.2 Summary of clinical findings
It is anticipated that the combination of ICIs and FTs can produce synergistic effects, leading to improved outcomes without added toxicities. The primary reported outcomes of clinical studies examined, most commonly, safety and tolerability, survival, response rate, and immune-related biomarkers. The potential benefits of combination therapy include: 1) improved therapeutic effect, 2) enhanced immune response, and 3) reduced adverse events (Table 11). The outcomes of clinical reports are summarized in Supplemental Table S6.
7.2.1 Improved therapeutic effect
The therapeutic effect is improved by an increase of response rate and/or better survival compared to either FT or ICI monotherapy. For example, a phase II study that evaluated subtotal thermal ablation (RFA or MWA) with anti-PD-1 therapy (nivolumab or pembrolizumab) in patients with advanced HCC has shown that additional ablation increases the objective response rate with tolerated toxicity and achieves a relatively better median survival (298). In this study, the complete response rate and partial response rate were increased from 4% to 8% and from 6% to 16%, respectively, when ablation was added to anti-PD-1 monotherapy. Another phase Ib study assessing combined IRE with nivolumab on locally advanced pancreatic adenocarcinoma showed a complete ablation effect in 100% of patients, a mean time to progression (TTP) of 6.3 months, mean progression-free survival (PFS) of 6.8 months (95% CI, 3.5-10.0), and a median overall survival (OS) of 18.0 months (95% CI, 9.2-26.8) (307). Encouraged by the remarkable efficacy, a multicenter, phase II adjuvant trial (NCT03080974) is underway evaluating IRE and nivolumab in patients with locally advanced pancreatic cancer.
7.2.2 Enhanced immune response
An enhanced immune response is typically demonstrated by an elevation in the biomarkers associated with stronger anti-tumor immunity in most of the trials or lessening of immune anergy. Most noticeable is the sustained elevation in intratumoral and/or peripheral CD8 T cells in combination therapy. In one study, cryoablation and tremelimumab treatment led to a significant increase in immune cell infiltration and tertiary lymphoid structures in patients with metastatic clear cell RCC (294). Another phase I/II study using tremelimumab and loco-regional therapy (RFA, cryoablation, or TACE) on HCC patients showed an activation of tumor-specific T cells and a decrease in T-cell clonality on the patients (302). A study involving cryoablation and ipilimumab on breast cancer showed that combination therapy was associated with increases in Th1-type cytokines, an increased frequency of activated T cells and proliferating T cells in PBMC, and an expansion of effector T cells within the tumor relative to regulatory T cells (297).
7.2.3 Reduce adverse effects
Safety and tolerability are the primary outcome for most studies demonstrating the reduction of adverse effects. Among all the reported trials, the combination therapy was well-tolerated, and the toxicity is not increased by the association of ICIs and LRTs. Adverse events were less than or equal to Grade II for most of the studies. Moreover, the ICI dose in combination treatment can be reduced owing to the increase in therapeutic effect and improved response rate, therefore reducing the immune-related adverse effects.
7.2.4 Opportunities in clinical research
More clinical evidence is needed to demonstrate whether the combination of ICIs and FTs is feasible and effective in treating solid tumors and shows advantages over monotherapy and even SOCs. As of the writing of this review, the numbers of completed clinical trials and retrospective clinical studies are still small, especially compared to those in radiation oncology or chemotherapy. Among these studies with combination FT and ICI, the patient selection may be biased; some patients with low TMB (and presumably low response rate to ICI) may have been excluded. In addition, more detailed analysis of immune response is warranted for better understanding of immune modulation by this combined treatment.
There has not been a reported randomized phase III study that compares combination therapy with other treatments including monotherapy (FT or ICI alone). The immune and therapeutic effects of the combination therapy remain to be better understood. Fundamental questions in designing optimal combinatorial treatment regimen need to be answered, as we discuss in our next sections.
8 Challenges for FT and ICI
8.1 Lack of “immunological dosing” of FT
Unlike thermal dosing, the “immunological dosing” of FT, which describes the interaction between the immune system and the FT, is not well defined nor established. Even though there have been comprehensive reviews on the immunomodulation of types of FT such as cryoablation (110), hyperthermia (130), and IRE (308), the quantitative relationship between the exact treatment conditions and the subsequent immune responses (summarized in section 3) have not been well described. For example, even though numerous studies have demonstrated that hyperthermia could provoke robust immune responses and create an immune-favorable TME that sensitizes tumors to immunotherapy (309, 310), the relationship between hypothermia parameters (the elevated temperature, time of elevated temperature, and distribution of temperature) and the extent of immune system activation still needs to be elucidated (283, 311). To be specific, the quantity of antigen being released as a function of tumor volume and treatment condition (e.g., temperature and time for thermal ablation) is yet to be uncovered. The amount of tumor antigen needed to sufficiently prime the immune system is largely unknown, despite its well-recognized importance. In addition, the composition of DAMPs generated following FT is not clear either. Furthermore, a quantitative relationship between the dynamics of DAMPs, and specific FT treatment conditions is largely unknown. Understanding the immune impacts of FT will require careful and extensive mechanism studies that also includes variability based on heterogeneity of tumor types.
The best choice of FT is usually not straightforward. Currently, clinicians are not selecting FT approaches based on the immunological effects of specific FT modalities, in part because such immunologic effects are not yet clear, but by other factors, including the tumor location, tumor size, and disease stage. To date, clinical studies offering direct comparison of the immune response among the different modes of FT have not been conducted. Preclinical comparison among different modes of FT is also still lacking. Another factor that further complicates the issue is that the device and setup of FTs vary among different laboratories: some use a clinical-size probe which is less than ideal for sub-centimeter tumors, while some groups opt to build probes specially for small animal research (312). When FT probes for small animals are used, they are usually not fully characterized: lacking an assessment of the ex vivo and in vivo physical performance of the probes with regards to the biological sequelae of tumor destruction.
Proper dosing in clinical study is key for optimal combination therapy. For example, even though RFA and anti-PD-1 generally works well in most of the cases, the inflammation induced by incomplete RFA can accelerate tumor progression and hinder anti-PD-1 immunotherapy (154). However, studying FT dosing in humans is difficult if not impossible, so careful examination of controlled FT dosing must be studied in small animal models, like rodents, despite their difference in size. Fundamentally, the “immunological dosing” of FT on a tumor should depend on the energy field and its coverage. Even though the energy field (spatiotemporal distribution of physical quantities, such as temperature for thermal therapy and cryoablation, electric field for IRE) has been extensively studied for cell death and tissue destruction, unfortunately the importance of a well-described energy field is largely overlooked in both preclinical and clinical studies: when a tumor is being treated, the direct physical quantities are usually not quantitively prescribed or not being monitored. In addition, for example, when comparing the additive or synergistic effect of combinatory therapy to energy-based focal therapy, controllable and consistent partial ablation is desirable so that additional therapeutic benefit can be quantitatively analyzed by tracking the tumor volume over time from the same baseline. However, such a baseline is not easy to achieve without controlling the energy field. To delineate the energy field of a specific FT, a few steps have to take place (1): the device needs to be well-designed for repeatable delivery of the energy (2), the energy needs to be precisely mapped based on the boundary condition defined by the device and the multi-physics models, and (3) in vivo measurements including monitoring and validating the energy fields need to be taken and compared to the models to ensure the consistency of FT protocols.
8.2 No consensus on the timing of ICI when combined with FT
Despite research advancement toward understanding synergistic combination therapy, the optimal timing of ICI treatment has not been well studied. Little is known about how to design the combinatory regimens to favor therapeutic efficacy, whether before, during or after the FT. Most clinical studies incorporated FTs during ICI treatment. This fact might lead to weakened responses, as some preclinical evidence suggests that checkpoint inhibition before antigen priming can lead to poor anti-tumor effects (313). A popular theory is that ablation should be performed before ICIs because neoantigen-specific T cells induced by interventional therapy can have an improved response to ICI, thus enhancing the anti-tumor immune response. However, some researchers propose that administering ICI therapies should be in a neoadjuvant setting prior to FT (314) and argue that immunotherapy begun before ablation can be curative and can enhance efficacy in the presence of a high tumor burden (259). Moreover, the immunomodulatory effects produced by some ablation treatments may be short-lived, so the timing of sequential treatment of ICIs needs careful planning. Unfortunately, to knowledge, no study was designed with this issue in mind. There is indispensable need for preclinical study to answer the question of how the sequencing of FT and ICI affects optimal anti-tumor response.
In addition to timing, there is a need for preclinical and clinical investigation to optimize the synergistic efficacy of the dosage, frequency, and delivery approach of ICI when combined with FT. Most of the studies adopted dosage regimens based on the previous experience of preclinical studies or previous clinical trials on ICIs alone and adjusted them according to regular evaluation (315), rather than a rigorous optimization process. ICI usually comes with dose-limiting immune-related side effects; these side effects may be mitigated by incorporating FT to enhance the response of ICI, as we summarized previously.
8.3 Gaps between preclinical models and clinical trials
Most research in this field is currently still in the proof-of-principle phase. Preclinical research largely precedes clinical studies in terms of optimizing treatment approaches and understanding immune system modulation. However, we should recognize the gaps between preclinical models and clinical trials, especially considering differences in (1) the cancer models (2), the size difference, and (3) the drug delivery approaches.
The immune effect is highly dependent on not only the cell death by FT, but also the type of tumor in the experimental mouse model. The extent to which preclinical research can be generalized to humans is largely limited by the heterogeneity of tumor types, animal models, and ablation methodologies. The mouse subcutaneous tumor model has prominent advantages, allowing treatment of a large number of animals with easy handling and housing and lower costs than other mammalian species, and also offering access to an array of assays, reagents and genetically engineered mice that facilitate mechanistic understanding (70). However, there is divergence between mouse and human immune systems, and the immune microenvironment of subcutaneous tissue is significantly different from those in other organs. In addition, the inoculated tumor model using syngeneic cancer cell lines cultured in monolayters for many generations may not represent the real tumor structure and components. Some researchers have proposed more experimentation with orthotopic tumor models to elucidate which organ and disease are suitable for this promising treatment strategy (79).
The size of mice becomes another disadvantage for investigation of local therapies and drug delivery, due to the disparities in scale. On small animal cancer models, the tumors being treated by FT are usually small (a few millimeters) and spherical. Clinical devices, designed to treat tumors at centimeter scale, are usually too big for the tumors on mice, so customized devices have to be used for preclinical research. Monoclonal antibodies are each unique, so ICI drugs for mice are inherently different than for humans, making it difficult to translate the treatment effect from mouse to human. Scaling up the setup and dose of FTs and ICIs remains a big challenge. The size difference may also have an impact on the ICI timing and dosing.
The difference in drug delivery methods between animals and humans also becomes a challenge in translational research. ICI drugs are typically infused intravenously in clinical studies while different drug delivery methods, such as intraperitoneal and intratumoral injection, are typically used in animal studies. Nonetheless, studies of syngeneic cell lines or genetically engineered mouse models have supported progress to date on cancer immunotherapy and that situation is not likely to change in the near future.
9 Future directions for combination therapy of FT with ICI
T cell responses that recognize and eradicate cancer cells are an important part of the cancer-immunity cycle (316), as shown in Figure 4. The generation of immunity to cancer is a cyclical process that can be self-propagating, leading to complete eradication of cancer. The cycle has several major steps that have both immune-stimulatory and inhibitory factors. In order to keep its positive cyclic process progressing, the goal of the therapeutic strategy is to ensure the passage along the steps in the cancer-immunity cycle are free from checkpoint(s). Therefore, the strategy with FT and ICI is the modulation of the cancer-immunity cycle to improve immediate- or long-term response. To accomplish this, the most effective approach is to selectively target the step or steps where the cycle is blocked or checked.
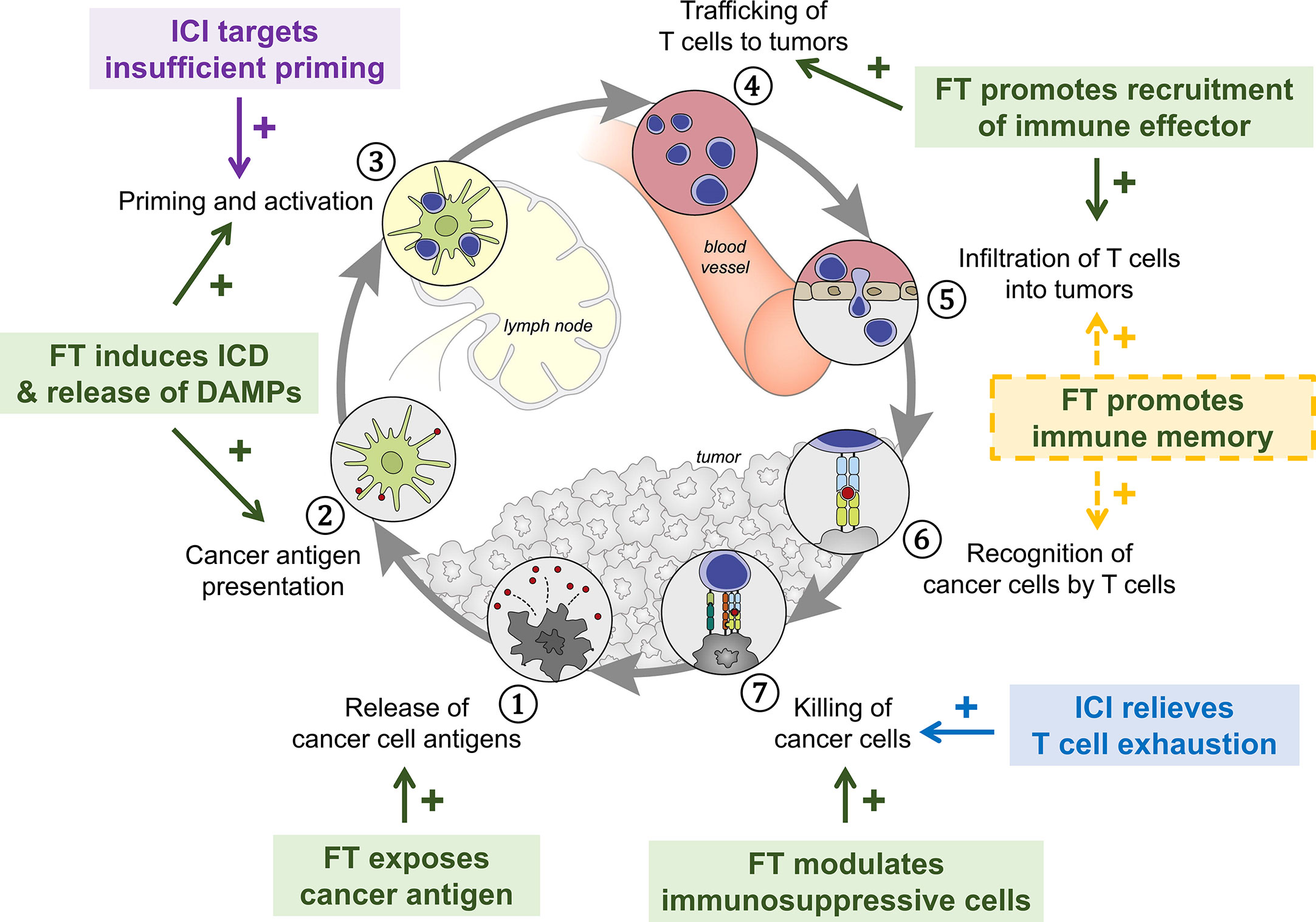
Figure 4 The intervention of focal therapy (FT) and immune checkpoint inhibitor (ICI) in the cancer-immunity cycle. The cycle has several major steps that have both immune-stimulatory and inhibitory factors. The immune response to the FT can be harnessed to amplify and broaden T cell responses while the ICI can target the immune regulatory feedback mechanisms that halt the development or limit the immunity. Working together, FT and ICI can facilitate the maintenance of central, effector and tissue-resident memory CD8 T cells to promote long-term immunosurveillance and protection. ICD, immunogenic cell death; DAMPs, danger-associated molecular patterns. Figure adapted from original figure by Chen et al. (316) with permission.
To design an effective approach involving FT and ICI, the following 3 steps are needed. First, the cancer immune microenvironment must be assessed to identity the “bottlenecks” of the cycle. Second, with the knowledge of the limiting steps, the prescription of FT and/or ICI needs to target the stimulatory and inhibitory factors within each step, without introducing additional bottlenecks to the system. Finally, auxiliary immunotherapy and adjuvant therapy can be prescribed to better address the bottlenecks and stimulate antitumor immunity.
9.1 Identifying the bottlenecks
For a tumor to exist or even to progress, the crucial steps of the cancer-immunity cycles leading to cancer elimination must be arrested. To determine the bottlenecks, the tumor immune microenvironment must be fully assessed before the combination therapy, as the microenvironment of cancer varies among tumors and patients. Predictive biomarkers that are present at baseline prior to treatment initiation and predict ICI response can be evidence of stimulatory signal or inhibitory/suppressive factors. Such factors include the genetics of the cancer cells that dictate the mutation burden and the attributes (i.e., population, phenotype, and the location) of immune cells.
Equally important are the preemptive biomarkers, which are generated upon treatment initiation. Biomarkers associated with good prognoses can be evidence of effective treatment that alleviates the bottlenecks in the cancer-immunity cycle. Biomarkers contrary to prediction can be signs of failed strategy and will require changes of strategy. One challenge, however, is that some biomarkers cannot be detected in peripheral blood and will require tumor biopsy. Other challenges involve elusive data from systematic comparisons of the immunogenic effects of different modalities of focal therapy. The immune cell populations (both the immune effectors and regulators) and immunological features following FT, as monotherapy or with ICI, in addition to their comparisons to conventional treatment, remain largely unknown.
9.2 Prescribing the immunomodulation
The summary of potential solutions to the bottlenecks is presented in Table 12. Optimally, limiting step(s) of a given cancer condition should be identified before prescribing the potential solution that specifically targets each step. Designing the FT+ICI strategy to achieve the listed solutions is crucial for the success of the cancer therapy. Auxiliary therapy (e.g., other forms of immunotherapy, anti-angiogenic therapy) can be used to augment the immunomodulatory effects by FT and/or ICI to alleviate the limits on each of the seven steps.
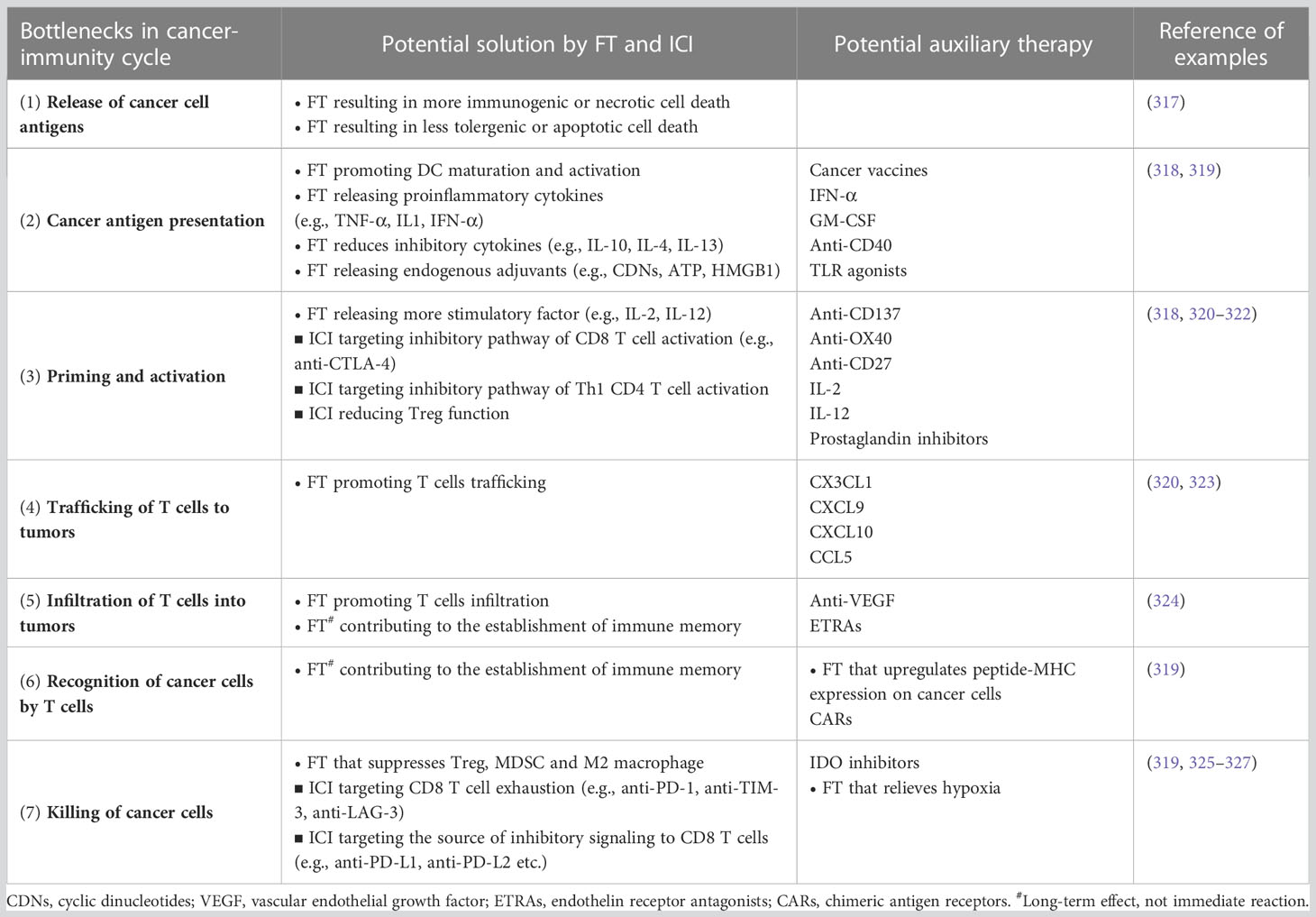
Table 12 Potential solutions to the bottlenecks in the major steps that arrest the self-propagating cancer-immunity cycle by combinatory focal therapy (FT) and immune checkpoint inhibitor (ICI).
Author contributions
MJ, SF, and QS contribute to the conception, MJ and QS were involved in acquisition, analysis, or interpretation of data; MJ, SF, and QS drafted the work and/or substantively revised it. All authors contributed to the article and approved the submitted version.
Acknowledgments
The authors thank John C Bischof for valuable comments on the manuscript. The work receives editing service from Ellen Morgan. The authors thank helpful discussion with Samira Azarin, Brandon Burbach, Yoji Shimizu, Joseph Vallin and members of BioHeat Mass Transfer (BHMT) Laboratory. MJ and QS were supported by Institute for Engineering in Medicine at the University of Minnesota.
Conflict of interest
The authors declare that the research was conducted in the absence of any commercial or financial relationships that could be construed as a potential conflict of interest.
Publisher’s note
All claims expressed in this article are solely those of the authors and do not necessarily represent those of their affiliated organizations, or those of the publisher, the editors and the reviewers. Any product that may be evaluated in this article, or claim that may be made by its manufacturer, is not guaranteed or endorsed by the publisher.
Supplementary material
The Supplementary Material for this article can be found online at: https://www.frontiersin.org/articles/10.3389/fonc.2023.1153066/full#supplementary-material
References
1. Siegel RL, Miller KD, Jemal A. Cancer statistics, 2019. CA-A Cancer J FOR Clin (2019) 69(1):7–34. doi: 10.3322/caac.21551
2. cancer.gov. Types of cancer treatment. Available at: https://www.cancer.gov/about-cancer/treatment/types.
3. cancer.org. Types of cancer treatment. Available at: https://www.cancer.org/treatment/treatments-and-side-effects/treatment-types.html.
4. cancer.net. Standard of care. Available at: https://www.cancer.net/standard-care.
5. cancer.org. Managing advanced cancer. American cancer society. Available at: https://www.cancer.org/treatment/understanding-your-diagnosis/advanced-cancer/managing-symptoms.html.
6. Lim M, Xia YX, Bettegowda C, Weller M. Current state of immunotherapy for glioblastoma. Nat Rev Clin Oncol (2018) 15(7):422–42. doi: 10.1038/s41571-018-0003-5
7. Harrison L, Blackwell K. Hypoxia and anemia: factors in decreased sensitivity to radiation therapy and chemotherapy? Oncologist (2004) 9 Suppl 5:31–40. doi: 10.1634/theoncologist.9-90005-31
8. cancer.org. Chemotherapy side effects. Available at: https://www.cancer.org/treatment/treatments-and-side-effects/treatment-types/chemotherapy/chemotherapy-side-effects.htmlaccessed.
9. cancer.gov. Radiation therapy side effects. Available at: https://www.cancer.gov/about-cancer/treatment/types/radiation-therapy/side-effects.
10. Chu KF, Dupuy DE. Thermal ablation of tumours: biological mechanisms and advances in therapy. Nat Rev Cancer (2014) 14(3):199–208. doi: 10.1038/nrc3672
11. Baust JG, Bischof JC, Jiang-Hughes S, Polascik TJ, Rukstalis DB, Gage AA, et al. Re-purposing cryoablation: a combinatorial 'therapy' for the destruction of tissue. Prostate Cancer Prostatic Dis (2015) 18(2):87–95. doi: 10.1038/pcan.2014.54
12. Mahmoudi K, Bouras A, Bozec D, Ivkov R, Hadjipanayis C. Magnetic hyperthermia therapy for the treatment of glioblastoma: a review of the therapy's history, efficacy and application in humans. Int J Hyperthermia (2018) 34(8):1316–28. doi: 10.1080/02656736.2018.1430867
13. Khalil DN, Smith EL, Brentjens RJ, Wolchok JD. The future of cancer treatment: immunomodulation, CARs and combination immunotherapy. Nat Rev Clin Oncol (2016) 13(5):273–90. doi: 10.1038/nrclinonc.2016.25
14. Binnewies M, Roberts EW, Kersten K, Chan V, Fearon DF, Merad M, et al. Understanding the tumor immune microenvironment (TIME) for effective therapy. Nat Med (2018) 24(5):541–50. doi: 10.1038/s41591-018-0014-x
15. Johnson CB, Win SY. Combination therapy with PD-1/PD-L1 blockade: an overview of ongoing clinical trials. Oncoimmunology (2018) 7(4):e1408744. doi: 10.1080/2162402X.2017.1408744
16. Kepp O, Marabelle A, Zitvogel L, Kroemer G. Oncolysis without viruses - inducing systemic anticancer immune responses with local therapies. Nat Rev Clin Oncol (2020) 17(1):49–64. doi: 10.1038/s41571-019-0272-7
17. van den Bijgaart RJE, Schuurmans F, Futterer JJ, Verheij M, Cornelissen LAM, Adema GJ. Immune modulation plus tumor ablation: adjuvants and antibodies to prime and boost anti-tumor immunity in situ. Front Immunol (2021) 12:617365. doi: 10.3389/fimmu.2021.617365
18. Bastianpillai C, Petrides N, Shah T, Guillaumier S, Ahmed HU, Arya M. Harnessing the immunomodulatory effect of thermal and non-thermal ablative therapies for cancer treatment. Tumor Biol (2015) 36(12):9137–46. doi: 10.1007/s13277-015-4126-3
20. Evans SS, Repasky EA, Fisher DT. Fever and the thermal regulation of immunity: the immune system feels the heat. Nat Rev Immunol (2015) 15(6):335–49. doi: 10.1038/nri3843
21. Geboers B, Scheffer HJ, Graybill PM, Ruarus AH, Nieuwenhuizen S, Puijk RS, et al. High-voltage electrical pulses in oncology: irreversible electroporation, electrochemotherapy, gene electrotransfer, electrofusion, and electroimmunotherapy. Radiology (2020) 295(2):254–72. doi: 10.1148/radiol.2020192190
22. den Brok M, Sutmuller RPM, van der Voort R, Bennink EJ, Figdor CG, Ruers TJM, et al. In situ Tumor ablation creates an antigen source for the generation of antitumor immunity. Cancer Res (2004) 64(11):4024–9. doi: 10.1158/0008-5472.CAN-03-3949
23. Center, M. S. K. C. Pre-operative, single-dose ipilimumab and/or cryoablation in early Stage/Resectable breast cancer (2011). Available at: https://ClinicalTrials.gov/show/NCT01502592.
24. Brace CL. Microwave tissue ablation: biophysics, technology, and applications. Crit Rev Biomed Eng (2010) 38(1):65–78. doi: 10.1615/critrevbiomedeng.v38.i1.60
25. Tatli S, Tapan U, Morrison PR, Silverman SG. Radiofrequency ablation: technique and clinical applications. Diagn Interventional Radiol (2012) 18(5):508–16. doi: 10.4261/1305-3825.DIR.5168-11.1
26. Focused ultrasound foundation. state of the field report 2022; fusfoundation.org. Available at: https://cdn.fusfoundation.org/2022/11/01111233/Focused-Ultrasound-Foundation_State-of-the-Field-Report-2022_Nov1.pdf.
28. Tatli S, Acar M, Tuncali K, Morrison PR, Silverman S. Percutaneous cryoablation techniques and clinical applications. Diagn Interventional Radiol (2010) 16(1):90–5. doi: 10.4261/1305-3825.DIR.1922-08.0
29. Narayanan G. Irreversible electroporation. Semin Interventional Radiol (2015) 32(4):349–55. doi: 10.1055/s-0035-1564706
30. Schena E, Saccomandi P, Fong Y. Laser ablation for cancer: past, present and future. J Funct Biomaterials (2017) 8(2):19. doi: 10.3390/jfb8020019
31. Liu H, Song CE, Zhang BZ, Luo R, Yang JJ. Research trends and areas of focus on cryoablation and oncology: a bibliometric analysis from 2001 to 2020. Medicine (2022) 101(52):e32513. doi: 10.1097/MD.0000000000032513
32. Inchingolo R, Posa A, Mariappan M, Spiliopoulos S. Locoregional treatments for hepatocellular carcinoma: current evidence and future directions. World J Gastroenterol (2019) 25(32):4614–28. doi: 10.3748/wjg.v25.i32.4614
33. Saldanha DF, Khiatani VL, Carrillo TC, Yap FY, Bui JT, Knuttinen MG, et al. Current tumor ablation technologies: basic science and device review. Semin interventional Radiol (2010) 27(3):247–54. doi: 10.1055/s-0030-1261782
34. Makary MS, Khandpur U, Cloyd JM, Mumtaz K, Dowell JD. Locoregional therapy approaches for hepatocellular carcinoma: recent advances and management strategies. Cancers (2020) 12(7):1914. doi: 10.3390/cancers12071914
35. Robertson CA, Evans DH, Abraharnse H. Photodynamic therapy (PDT): a short review on cellular mechanisms and cancer research applications for PDT. J Photochem Photobiol B-Biology (2009) 96(1):1–8. doi: 10.1016/j.jphotobiol.2009.04.001
36. Keidar M, Shashurin A, Volotskova O, Stepp MA, Srinivasan P, Sandler A, et al. Cold atmospheric plasma in cancer therapy. Phys Plasmas (2013) 20(5):057101. doi: 10.1063/1.4801516
37. Yarmush ML, Golberg A, Sersa G, Kotnik T, Miklavcic D. Electroporation-based technologies for medicine: principles, applications, and challenges. Annu Rev Biomed Eng (2014) 16:295–320. doi: 10.1146/annurev-bioeng-071813-104622
38. Hunter WL, Burt HM, Machan L. Local delivery of chemotherapy: a supplement to existing cancer treatments - a case for surgical pastes and coated stents. Advanced Drug Delivery Rev (1997) 26(2-3):199–207. doi: 10.1016/S0169-409X(97)00035-5
39. Aurisicchio L, Mancini R, Ciliberto G. Cancer vaccination by electro-gene-transfer. Expert Rev Vaccines (2013) 12(10):1127–37. doi: 10.1586/14760584.2013.836903
40. Heller R, Heller LC. Gene electrotransfer clinical trials. In: Huang L, Liu D, Wagner E, editors. Nonviral vectors for gene therapy: Physical methods and medical translation (Advances in genetics v. 89) (2015) Amsterdam: Academic Press. p. 235–62.
41. Hall EJ, Roizin-Towle L. Biological effects of heat. Cancer Res (Chicago Ill.) (1984) 44(10):4708s.
43. Hinshaw JL, Lubner MG, Ziemlewicz TJ, Lee FT, Brace CL. Percutaneous tumor ablation tools: microwave, radiofrequency, or cryoablation-what should you use and why? Radiographics (2014) 34(5):1344–62. doi: 10.1148/rg.345140054
44. Salati U, Barry A, Chou FY, Ma R, Liu DM. State of the ablation nation: a review of ablative therapies for cure in the treatment of hepatocellular carcinoma. Future Oncol (2017) 13(16):1437–48. doi: 10.2217/fon-2017-0061
45. Knavel EM, Brace CL. Tumor ablation: common modalities and general practices. Techniques Vasc International Radiol (2013) 16(4):192–200. doi: 10.1053/j.tvir.2013.08.002
46. Kim JH, Kim PN, Won HJ, Shin YM. Percutaneous radiofrequency ablation using internally cooled wet electrodes for the treatment of hepatocellular carcinoma. Am J Roentgenology (2012) 198(2):471–6. doi: 10.2214/AJR.11.6583
47. Singal A. The comparative assessment of clinical ablative therapies: effects on physiological and biomechanical properties of contractile tissues in response to therapeutic doses. (2014). Retrieved from the University of Minnesota Digital Conservancy. https://hdl.handle.net/11299/168310.
48. Farny CH, Holt RG, Roy RA. The correlation between bubble-enhanced HIFU heating and cavitation power. IEEE Trans Biomed Eng (2010) 57(1):175–84. doi: 10.1109/tbme.2009.2028133
49. Muller GJ, Roggan A. Laser-induced interstitial thermotherapy. Bellingham, Wash: SPIE Optical Engineering Press (1995).
50. Chatterjee DK, Diagaradjane P, Krishnan S. Nanoparticle-mediated hyperthermia in cancer therapy. Ther Delivery (2011) 2(8):1001–14. doi: 10.4155/tde.11.72
51. Chang D, Lim M, Goos J, Qiao RR, Ng YY, Mansfeld FM, et al. Biologically targeted magnetic hyperthermia: potential and limitations. Front Pharmacol (2018) 9:831. doi: 10.3389/fphar.2018.00831
52. Qin ZP, Etheridge M, Bischof JC. Nanoparticle heating: nanoscale to bulk effects of electromagnetically heated iron oxide and gold for biomedical applications. In: Energy-Based treatment of tissue and assessment VI. SPIE (2011).
53. Liu X, Zhang Y, Wang Y, Zhu W, Li G, Ma X, et al. Comprehensive understanding of magnetic hyperthermia for improving antitumor therapeutic efficacy. Theranostics (2020) 10(8):3793–815. doi: 10.7150/thno.40805
54. Li XS, Lovell JF, Yoon J, Chen XY. Clinical development and potential of photothermal and photodynamic therapies for cancer. Nat Rev Clin Oncol (2020) 17(11):657–74. doi: 10.1038/s41571-020-0410-2
55. Hoffmann NE, Bischof JC. The Cryobiology of cryosurgical injury. Urology (2002) 60(2 Suppl 1):40–9. doi: 10.1016/s0090-4295(02)01683-7
56. Gage AA, Baust J. Mechanisms of tissue injury in cryosurgery. Cryobiology (1998) 37(3):171–86. doi: 10.1006/cryo.1998.2115
57. Mala T, Samset E, Aurdal L, Gladhaug I, Edwin B, Soreide O. Magnetic resonance imaging-estimated three-dimensional temperature distribution in liver cryolesions: a study of cryolesion characteristics assumed necessary for tumor ablation. Cryobiology (2001) 43(3):268–75. doi: 10.1006/cryo.2001.2351
58. Jiang CL, Davalos RV, Bischof JCA. Review of basic to clinical studies of irreversible electroporation therapy. IEEE Trans Biomed Eng (2015) 62(1):4–20. doi: 10.1109/TBME.2014.2367543
59. Aycock KN, Davalos RV. Irreversible electroporation: background, theory, and review of recent developments in clinical oncology. Bioelectricity (2019) 1(4):214–34. doi: 10.1089/bioe.2019.0029
60. Hoogenboom M, Eikelenboom D, den Brok MH, Heerschap A, Futterer JJ, Adema GJ. Mechanical high-intensity focused ultrasound destruction of soft tissue: working mechanisms and physiologic effects. Uultrasound Med Biol (2015) 41(6):1500–17. doi: 10.1016/j.ultrasmedbio.2015.02.006
61. Hayashi F, Shigemura K, Maeda K, Hiraoka A, Maeshige N, Ooya T, et al. Combined treatment with ultrasound and immune checkpoint inhibitors for prostate cancer. J Clin Med (2022) 11(9):2448. doi: 10.3390/jcm11092448
62. Roberts WW. Development and translation of histotripsy: current status and future directions. Curr Opin Urol (2014) 24(1):104–10. doi: 10.1097/MOU.0000000000000001
63. Pahk KJ, Shin CH, Bae IY, Yang Y, Kim SH, Pahk K, et al. Boiling histotripsy-induced partial mechanical ablation modulates tumour microenvironment by promoting immunogenic cell death of cancers. Sci Rep (2019) 9:9050. doi: 10.1038/s41598-019-45542-z
64. Brujan EA, Ikeda T, Matsumoto Y. On the pressure of cavitation bubbles. Exp Thermal Fluid Sci (2008) 32(5):1188–91. doi: 10.1016/j.expthermflusci.2008.01.006
65. Benson L. Tumor treating fields technology: alternating electric field therapy for the treatment of solid tumors. Semin Oncol Nurs (2018) 34(2):137–50. doi: 10.1016/j.soncn.2018.03.005
66. Marra G, Valerio M, Emberton M, Heidenreich A, Crook JM, Bossi A, et al. Salvage local treatments after focal therapy for prostate cancer. Eur Urol Oncol (2019) 2(5):526–38. doi: 10.1016/j.euo.2019.03.008
67. Oshowo A, Gillams A, Harrison E, Lees WR, Taylor I. Comparison of resection and radiofrequency ablation for treatment of solitary colorectal liver metastases. Br J Surg (2003) 90(10):1240–3. doi: 10.1002/bjs.4264
68. Clevelandclinic. Available at: https://my.clevelandclinic.org/health/treatments/16903-cryoablation.
69. van Son M, Peters M, Moerland M, Kerkmeijer L, Lagendijk J, van Zyp JV. Focal salvage treatment of radiorecurrent prostate cancer: a narrative review of current strategies and future perspectives. Cancers (2018) 10(12):480. doi: 10.3390/cancers10120480
70. Greten TF, Mauda-Havakuk M, Heinrich B, Korangy F, Wood BJ. Combined locoregional-immunotherapy for liver cancer. J Hepatol (2019) 70(5):999–1007. doi: 10.1016/j.jhep.2019.01.027
71. cancer.gov. Cryosurgery in cancer treatment. Available at: https://www.cancer.gov/about-cancer/treatment/types/surgery/cryosurgery-fact-sheet (Accessed 2019 June 29).
72. Redondo P, del Olmo J, de Cerio ALD, Inoges S, Marquina M, Melero I, et al. Imiquimod enhances the systemic immunity attained by local cryosurgery destruction of melanoma lesions. J Invest Dermatol (2007) 127(7):1673–80. doi: 10.1038/sj.jid.5700777
73. Lindner U, Trachtenberg J, Lawrentschuk N. Focal therapy in prostate cancer: modalities, findings and future considerations. Nat Rev Urol (2010) 7(10):562–71. doi: 10.1038/nrurol.2010.142
74. Goldberg SN, Gazelle GS, Mueller PR. Thermal ablation therapy for focal malignancy: a unified approach to underlying principles, techniques, and diagnostic imaging guidance. AJR Am J Roentgenol (2000) 174(2):323–31. doi: 10.2214/ajr.174.2.1740323
75. Vincent A, Herman J, Schulick R, Hruban RH, Goggins M. Pancreatic cancer. Lancet (2011) 378(9791):607–20. doi: 10.1016/S0140-6736(10)62307-0
76. El-Baz AS, Pareek G, Suri JS. Prostate cancer imaging : an engineering and clinical perspective. Boca Raton, FL: CRC Press (2019).
77. Hanahan D, Weinberg RA. Hallmarks of cancer: the next generation. Cell (2011) 144(5):646–74. doi: 10.1016/j.cell.2011.02.013
78. Takaki H, Cornelis F, Kako Y, Kobayashi K, Kamikonya N, Yamakado K. Thermal ablation and immunomodulation: from preclinical experiments to clinical trials. Diagn Interventional Imaging (2017) 98(9):651–9. doi: 10.1016/j.diii.2017.04.008
79. Takaki H, Cornelis FH. Can the combination of ablation and immunomodulation become the breakthrough of cancer treatment? Diagn Interventional Imaging (2018) 99(3):121–2. doi: 10.1016/j.diii.2018.02.002
80. Yu MX, Pan H, Che N, Li L, Wang C, Wang Y, et al. Microwave ablation of primary breast cancer inhibits metastatic progression in model mice via activation of natural killer cells. Cell Mol Immunol (2021) 18(9):2153–64. doi: 10.1038/s41423-020-0449-0
81. Chavez M, Silvestrini MT, Ingham ES, Fite BZ, Mahakian LM, Tam SM, et al. Distinct immune signatures in directly treated and distant tumors result from TLR adjuvants and focal ablation. Theranostics (2018) 8(13):3611–28. doi: 10.7150/thno.25613
82. Zitvogel L, Galluzzi L, Kepp O, Smyth MJ, Kroemer G. Type I interferons in anticancer immunity. Nat Rev Immunol (2015) 15(7):405–14. doi: 10.1038/nri3845
83. Taylor A, Verhagen J, Blaser K, Akdis M, Akdis CA. Mechanisms of immune suppression by interleukin-10 and transforming growth factor-beta: the role of T regulatory cells. Immunology (2006) 117(4):433–42. doi: 10.1111/j.1365-2567.2006.02321.x
84. Srivastava P. Interaction of heat shock proteins with peptides and antigen presenting cells: chaperoning of the innate and adaptive immune responses. Annu Rev Immunol (2002) 20:395–425. doi: 10.1146/annurev.immunol.20.100301.064801
85. Dressel R, Lubbers M, Walter L, Herr W, Gunther E. Enhanced susceptibility to cytotoxic T lymphocytes without increase of MHC class I antigen expression after conditional overexpression of heat shock protein 70 in target cells. Eur J Immunol (1999) 29(12):3925–35. doi: 10.1002/(SICI)1521-4141(199912)29:12<3925::AID-IMMU3925>3.0.CO;2-S
86. Kepp O, Tesniere A, Zitvogel L, Kroemer G. The immunogenicity of tumor cell death. Curr Opin Oncol (2009) 21(1):71–6. doi: 10.1097/CCO.0b013e32831bc375
87. Krysko DV, Garg AD, Kaczmarek A, Krysko O, Agostinis P, Vandenabeele P. Immunogenic cell death and DAMPs in cancer therapy. Nat Rev Cancer (2012) 12(12):860–75. doi: 10.1038/nrc3380
88. Venereau E, Ceriotti C, Bianchi ME. DAMPs from cell death to new life. Front Immunol (2015) 6:422. doi: 10.3389/fimmu.2015.00422
89. Marciscano AE, Anandasabapathy N. The role of dendritic cells in cancer and anti-tumor immunity. Semin Immunol (2021) 52:101481. doi: 10.1016/j.smim.2021.101481
90. Cendrowicz E, Sas Z, Bremer E, Rygiel TP. The role of macrophages in cancer development and therapy. Cancers (2021) 13(8):1946. doi: 10.3390/cancers13081946
91. Farhood B, Najafi M, Mortezaee K. CD8(+) cytotoxic T lymphocytes in cancer immunotherapy: a review. J Cell Physiol (2019) 234(6):8509–21. doi: 10.1002/jcp.27782
92. Kinker GS, Vitiello GAF, Ferreira WAS, Chaves AS, de Lima VCC, Medina TDB. Cell orchestration of anti-tumor immune responses: a matter of cell localization and communication. Front Cell Dev Biol (2021) 9:678127. doi: 10.3389/fcell.2021.678127
93. Okla K, Farber DL, Zou WP. Tissue-resident memory T cells in tumor immunity and immunotherapy. J Exp Med (2021) 218(4):e20201605. doi: 10.1084/jem.20201605
94. Ostrand-Rosenberg S, Fenselau C. Myeloid-derived suppressor cells: immune-suppressive cells that impair antitumor immunity and are sculpted by their environment. J Immunol (2018) 200(2):422–31. doi: 10.4049/jimmunol.1701019
95. Wu SY, Fu T, Jiang YZ, Shao ZM. Natural killer cells in cancer biology and therapy. Mol Cancer (2020) 19(1):120. doi: 10.1186/s12943-020-01238-x
96. Tay RE, Richardson EK, Toh HC. Revisiting the role of CD4(+)T cells in cancer immunotherapy-new insights into old paradigms. Cancer Gene Ther (2021) 28(1-2):5–17. doi: 10.1038/s41417-020-0183-x
97. Godwin BL, Coad JE. Healing responses following cryothermic and hyperthermic tissue ablation. In: Energy-Based treatment of tissue and assessment V. SPIE (2009).
98. Kroemer G, Galluzzi L, Kepp O, Zitvogel L. Immunogenic cell death in cancer therapy. Annu Rev Immunol (2013) 31:51–72. doi: 10.1146/annurev-immunol-032712-100008
99. Peled ZM, Chin GS, Liu W, Galliano R, Longaker MT. Response to tissue injury. Clinics Plast Surg (2000) 27(4):489.
100. Stadelmann WK, Digenis AG, Tobin GR. Physiology and healing dynamics of chronic cutaneous wounds. Am J Surg (1998) 176(2A):26S–38S. doi: 10.1016/S0002-9610(98)00183-4
101. Maletzki C, Emmrich J. Inflammation and immunity in the tumor environment. Digestive Dis (2010) 28(4-5):574–8. doi: 10.1159/000321062
102. Coulie PG, Van den Eynde BJ, van der Bruggen P, Boon T. Tumour antigens recognized by T lymphocytes: at the core of cancer immunotherapy. Nat Rev Cancer (2014) 14(2):135–46. doi: 10.1038/nrc3670
103. Buonaguro L, Petrizzo A, Tornesello ML, Buonaguro FM. Translating tumor antigens into cancer vaccines. Clin Vaccine Immunol (2011) 18(1):23–34. doi: 10.1128/CVI.00286-10
104. Tian TH, Olson S, Whitacre JM, Harding A. The origins of cancer robustness and evolvability. Integr Biol (2011) 3(1):17–30. doi: 10.1039/c0ib00046a
105. Slovak R, Ludwig JM, Gettinger SN, Herbst RS, Kim HS. Immuno-thermal ablations - boosting the anticancer immune response. J Immunother Cancer (2017) 5:78. doi: 10.1186/s40425-017-0284-8
106. Brock RM, Beitel-White N, Davalos RV, Allen IC. Starting a fire without flame: the induction of cell death and inflammation in electroporation-based tumor ablation strategies. Front Oncol (2020) 10:1235. doi: 10.3389/fonc.2020.01235
107. Wolfers J, Lozier A, Raposo G, Regnault A, Thery C, Masurier C, et al. Tumor-derived exosomes are a source of shared tumor rejection antigens for CTL cross-priming. Nat Med (2001) 7(3):297–303. doi: 10.1038/85438
108. King HW, Michael MZ, Gleadle JM. Hypoxic enhancement of exosome release by breast cancer cells. BMC Cancer (2012) 12:421. doi: 10.1186/1471-2407-12-421
109. Chen TY, Guo J, Yang MJ, Zhu XH, Cao XT. Chemokine-containing exosomes are released from heat-stressed tumor cells via lipid raft-dependent pathway and act as efficient tumor vaccine. J Immunol (2011) 186(4):2219–28. doi: 10.4049/jimmunol.1002991
110. Sabel MS. Cryo-immunology: a review of the literature and proposed mechanisms for stimulatory versus suppressive immune responses. Cryobiology (2009) 58(1):1–11. doi: 10.1016/j.cryobiol.2008.10.126
111. Jansen MC, van Hillegersberg R, Schoots IG, Levi M, Beek JF, Crezee H, et al. Cryoablation induces greater inflammatory and coagulative responses than radiofrequency ablation or laser induced thermotherapy in a rat liver model. Surgery (2010) 147(5):686–95. doi: 10.1016/j.surg.2009.10.053
112. Ravindranath MH, Wood TF, Soh D, Gonzales A, Muthugounder S, Perez C, et al. Cryosurgical ablation of liver tumors in colon cancer patients increases the serum total ganglioside level and then selectively augments antiganglioside IgM. Cryobiology (2002) 45(1):10–21. doi: 10.1016/s0011-2240(02)00102-5
113. den Brok MH, Sutmuller RP, Nierkens S, Bennink EJ, Frielink C, Toonen LW, et al. Efficient loading of dendritic cells following cryo and radiofrequency ablation in combination with immune modulation induces anti-tumour immunity. Br J Cancer (2006) 95(7):896–905. doi: 10.1038/sj.bjc.6603341
114. Chapman WC, Debelak JP, Wright Pinson C, Washington MK, Atkinson JB, Venkatakrishnan A, et al. Hepatic cryoablation, but not radiofrequency ablation, results in lung inflammation. Ann Surg (2000) 231(5):752–61. doi: 10.1097/00000658-200005000-00016
115. Gravante G, Sconocchia G, Ong SL, Dennison AR, Lloyd DM. Immunoregulatory effects of liver ablation therapies for the treatment of primary and metastatic liver malignancies. Liver Int (2009) 29(1):18–24. doi: 10.1111/j.1478-3231.2008.01915.x
116. Shao Q, O'Flanagan S, Lam T, Roy P, Pelaez F, Burbach BJ, et al. Engineering T cell response to cancer antigens by choice of focal therapeutic conditions. Int J Hyperthermia (2019) 36(1):130–8. doi: 10.1080/02656736.2018.1539253
117. Cervone F, Ausubel FM, De Lorenzo G. Enhancing immunity by engineering DAMPs. Oncotarget (2015) 6(30):28523–4. doi: 10.18632/oncotarget.5251
118. Fietta AM, Morosini M, Passadore I, Cascina A, Draghi P, Dore R, et al. Systemic inflammatory response and downmodulation of peripheral CD25(+)Foxp3(+) T-regulatory cells in patients undergoing radiofrequency thermal ablation for lung cancer. Hum Immunol (2009) 70(7):477–86. doi: 10.1016/j.humimm.2009.03.012
119. Erinjeri JP, Thomas CT, Samoilia A, Fleisher M, Gonen M, Sofocleous CT, et al. Image-guided thermal ablation of tumors increases the plasma level of interleukin-6 and interleukin-10. J Vasc Interventional Radiol (2013) 24(8):1105–12. doi: 10.1016/j.jvir.2013.02.015
120. Zhao J, Li Q, Muktiali M, Ren B, Hu Y, Li D, et al. Effect of microwave ablation treatment of hepatic malignancies on serum cytokine levels. BMC Cancer (2020) 20(1):812. doi: 10.1186/s12885-020-07326-x
121. Ahmad F, Gravante G, Bhardwaj N, Strickland A, Basit R, West K, et al. Changes in interleukin-1 beta and 6 after hepatic microwave tissue ablation compared with radiofrequency, cryotherapy and surgical resections. Am J Surg (2010) 200(4):500–6. doi: 10.1016/j.amjsurg.2009.12.025
122. Washington K, Debelak JP, Gobbell C, Sztipanovits DR, Shyr Y, Olson S, et al. Hepatic cryoablation-induced acute lung injury: histopathologic findings. J Surg Res (2001) 95(1):1–7. doi: 10.1006/jsre.2000.5976
123. Chen TY, Guo J, Han CF, Yang MKJ, Cao XT. Heat shock protein 70, released from heat-stressed tumor cells, initiates antitumor immunity by inducing tumor cell chemokine production and activating dendritic cells via TLR4 pathway. J Immunol (2009) 182(3):1449–59. doi: 10.4049/jimmunol.182.3.1449
124. Ringel-Scaia VM, Beitel-White N, Lorenzo MF, Brock RM, Huie KE, Coutermarsh-Ott S, et al. High-frequency irreversible electroporation is an effective tumor ablation strategy that induces immunologic cell death and promotes systemic anti-tumor immunity. EBioMedicine (2019) 44:112–25. doi: 10.1016/j.ebiom.2019.05.036
125. Schueller G, Kettenbach J, Sedivy R, Stift A, Friedl J, Gnant M, et al. Heat shock protein expression induced by percutaneous radiofrequency ablation of hepatocellular carcinoma in vivo. Int J Oncol (2004) 24(3):609–13. doi: 10.3892/ijo.24.3.609
126. Kroeze SGC, van Melick HHE, Nijkamp MW, Kruse FK, Kruijssen LWJ, van Diest PJ, et al. Incomplete thermal ablation stimulates proliferation of residual renal carcinoma cells in a translational murine model. BJU Int (2012) 110(6B):E281–6. doi: 10.1111/j.1464-410X.2012.11261.x
127. Haen SP, Gouttefangeas C, Schmidt D, Boss A, Clasen S, von Herbay A, et al. Elevated serum levels of heat shock protein 70 can be detected after radiofrequency ablation. Cell Stress Chaperones (2011) 16(5):495–504. doi: 10.1007/s12192-011-0261-y
128. Blum JS, Wearsch PA, Cresswell P. Pathways of antigen processing. Annu Rev Immunol (2013) 31:443–73. doi: 10.1146/annurev-immunol-032712-095910
129. He XZ, Wang QF, Han S, Wang HQ, Ye YY, Zhu ZY, et al. Cryo-ablation improves anti-tumor immunity through recovering tumor educated dendritic cells in tumor-draining lymph nodes. Drug Design Dev Ther (2015) 9:1449–58. doi: 10.2147/DDDT.S76592
130. Haen SP, Pereira PL, Salih HR, Rammensee HG, Gouttefangeas C. More than just tumor destruction: immunomodulation by thermal ablation of cancer. Clin Dev Immunol (2011) 2011:160250. doi: 10.1155/2011/160250
131. Sabel MS. The interrelationship between cryoablation, the immune response and the tumor microenvironment: stimulatory and suppressive effects. In: Keisari Y, editor. Tumor ablation: effects on systemic and local anti-tumor immunity and on other tumor-microenvironment interactions. Dordrecht: Springer Netherlands (2013). p. 77–107.
132. Takaki H, Imai N, Thomas CT, Yamakado K, Yarmohammadi H, Ziv E, et al. Changes in peripheral blood T-cell balance after percutaneous tumor ablation. Minimally Invasive Ther Allied Technol (2017) 26(6):331–7. doi: 10.1080/13645706.2017.1310737
133. Tran E, Turcotte S, Gros A, Robbins PF, Lu YC, Dudley ME, et al. Cancer immunotherapy based on mutation-specific CD4+T cells in a patient with epithelial cancer. Science (2014) 344(6184):641–5. doi: 10.1126/science.1251102
134. Linnemann C, van Buuren MM, Bies L, Verdegaal EME, Schotte R, Calis JJA, et al. High-throughput epitope discovery reveals frequent recognition of neo-antigens by CD4(+) T cells in human melanoma. Nat Med (2015) 21(1):81–5. doi: 10.1038/nm.3773
135. Kreiter S, Vormehr M, van de Roemer N, Diken M, Lower M, Diekmann J, et al. Mutant MHC class II epitopes drive therapeutic immune responses to cancer. Nature (2015) 520(7549):692–U269. doi: 10.1038/nature14426
136. Peng P, Hu HM, Liu P, Xu LX. Neoantigen-specific CD4(+)T-cell response is critical for the therapeutic efficacy of cryo-thermal therapy. J Immunother Cancer (2020) 8(2):e000421. doi: 10.1136/jitc-2019-000421
137. Wang SB, Xiong Y, Lin JT, Chen ZZ, Dai LP, Chen JH. Changes of CD4(+) T-cell subsets after radiofrequency ablation in lung cancer and its significance. J Cancer Res Ther (2016) 12(7):C166–70. doi: 10.4103/0973-1482.200609
138. Hendry S, Salgado R, Gevaert T, Russell PA, John T, Thapa B, et al. Assessing tumor-infiltrating lymphocytes in solid tumors: a practical review for pathologists and proposal for a standardized method from the international immunooncology biomarkers working group: part 1: assessing the host immune response, TILs in invasive breast carcinoma and ductal carcinoma in situ, metastatic tumor deposits and areas for further research. Adv Anatomic Pathol (2017) 24(5):235–51. doi: 10.1097/PAP.0000000000000162
139. White SB, Zhang ZL, Chen J, Gogineni VR, Larson AC. Early immunologic response of irreversible electroporation versus cryoablation in a rodent model of pancreatic cancer. J Vasc Interventional Radiol (2018) 29(12):1764–9. doi: 10.1016/j.jvir.2018.07.009
140. Mo ZL, Lu HL, Mo SW, Fu XM, Chang SW, Yue J. Ultrasound-guided radiofrequency ablation enhances natural killer-mediated antitumor immunity against liver cancer. Oncol Lett (2018) 15(5):7014–20. doi: 10.3892/ol.2018.8231
141. Shi JY, Gao Q, Wang ZC, Zhou J, Wang XY, Min ZH, et al. Margin-infiltrating CD20(+) BCells display an atypical memory phenotype and correlate with favorable prognosis in hepatocellular carcinoma. Clin Cancer Res (2013) 19(21):5994–6005. doi: 10.1158/1078-0432.CCR-12-3497
142. Dieu-Nosjean MC, Antoine M, Danel C, Heudes D, Wislez M, Poulot V, et al. Long-term survival for patients with non-small-cell lung cancer with intratumoral lymphoid structures. J Clin Oncol (2008) 26(27):4410–7. doi: 10.1200/JCO.2007.15.0284
143. Nelson BH. CD20(+) b cells: the other tumor-infiltrating lymphocytes. J Immunol (2010) 185(9):4977–82. doi: 10.4049/jimmunol.1001323
144. Lu P, Zhu XQ, Xu ZL, Zhou Q, Zhang J, Wu F. Increased infiltration of activated tumor-infiltrating lymphocytes after high intensity focused ultrasound ablation of human breast cancer. Surgery (2009) 145(3):286–93. doi: 10.1016/j.surg.2008.10.010
145. Li YF, Wang DD, Li XG. The blood cells in NSCLC and the changes after RFA. Int J Hyperthermia (2020) 37(1):753–62. doi: 10.1080/02656736.2020.1782486
146. Fite BZ, Wang J, Kare AJ, Ilovitsh A, Chavez M, Ilovitsh T, et al. Immune modulation resulting from MR-guided high intensity focused ultrasound in a model of murine breast cancer. Sci Rep (2021) 11(1):927. doi: 10.1038/s41598-020-80135-1
147. He CB, Sun SX, Zhang Y, Xie FX, Li SP. The role of irreversible electroporation in promoting M1 macrophage polarization via regulating the HMGB1-RAGE-MAPK axis in pancreatic cancer. Oncoimmunology (2021) 10(1):1897295. doi: 10.1080/2162402X.2021.1897295
148. Zhu J, Lou Y, Liu P, Xu LSX. Tumor-related HSP70 released after cryo-thermal therapy targeted innate immune initiation in the antitumor immune response. Int J Hyperthermia (2020) 37(1):843–53. doi: 10.1080/02656736.2020.1788173
149. Wang J. Neutrophils in tissue injury and repair. Cell Tissue Res (2018) 371(3):531–9. doi: 10.1007/s00441-017-2785-7
150. Yakkala C, Chiang CL, Kandalaft L, Denys A, Duran R. Cryoablation and immunotherapy: an enthralling synergy to confront the tumors. Front Immunol (2019) 10:2283. doi: 10.3389/fimmu.2019.02283
151. Lorenzo-Sanz L, Munoz P. Tumor-infiltrating immunosuppressive cells in cancer-cell plasticity, tumor progression and therapy response. Cancer Microenviron (2019) 12(2-3):119–32. doi: 10.1007/s12307-019-00232-2
152. Scheffer HJ, Stam AGM, Geboers B, Vroomen L, Ruarus A, de Bruijn B, et al. Irreversible electroporation of locally advanced pancreatic cancer transiently alleviates immune suppression and creates a window for antitumor T cell activation. Oncoimmunology (2019) 8(11):1652532. doi: 10.1080/2162402X.2019.1652532
153. He CB, Wang J, Sun SX, Zhang Y, Li SP. Immunomodulatory effect after irreversible electroporation in patients with locally advanced pancreatic cancer. J Oncol (2019) 2019:9346017. doi: 10.1155/2019/9346017
154. Shi LR, Wang JJ, Ding NH, Zhang Y, Zhu YB, Dong SL, et al. Inflammation induced by incomplete radiofrequency ablation accelerates tumor progression and hinders PD-1 immunotherapy. Nat Commun (2019) 10:5421. doi: 10.1038/s41467-019-13204-3
155. Ruzzenente A, de Manzoni G, Molfetta M, Pachera S, Genco B, Donataccio M, et al. Rapid progression of hepatocellular carcinoma after radiofrequency ablation. World J Gastroenterol (2004) 10(8):1137–40. doi: 10.3748/wjg.v10.i8.1137
156. Huang S, Qu N, Men Y, Liu Z. Effects of thermal ablation on Treg/Th17 in hepatocellular carcinoma of mice. Eur J Inflamm (2019) 17:2058739219832489. doi: 10.1177/2058739219832489
157. Aarts BM, Klompenhouwer EG, Rice SL, Imani F, Baetens T, Bex A, et al. Cryoablation and immunotherapy: an overview of evidence on its synergy. Insights into Imaging (2019) 10(1):53. doi: 10.1186/s13244-019-0727-5
158. Zhu J, Zhang Y, Zhang AL, He K, Liu P, Xu LX. Cryo-thermal therapy elicits potent anti-tumor immunity by inducing extracellular Hsp70-dependent MDSC differentiation. Sci Rep (2016) 6:27136. doi: 10.1038/srep27136
159. Enamorado M, Iborra S, Priego E, Cueto FJ, Quintana JA, Martinez-Cano S, et al. Enhanced anti-tumour immunity requires the interplay between resident and circulating memory CD8(+) T cells. Nat Commun (2017) 8:16073. doi: 10.1038/ncomms16073
160. Park SL, Buzzai A, Rautela J, Hor JL, Hochheiser K, Effern M, et al. Tissue-resident memory CD8(+) T cells promote melanoma-immune equilibrium in skin. Nature (2019) 565(7739):366. doi: 10.1038/s41586-018-0812-9
161. Malik BT, Byrne KT, Vella JL, Zhang P, Shabaneh TB, Steinberg SM, et al. Resident memory T cells in the skin mediate durable immunity to melanoma. Sci Immunol (2017) 2(10):eaam6346. doi: 10.1126/sciimmunol.aam6346
162. Rosato PC, Wijeyesinghe S, Stolley JM, Nelson CE, Davis RL, Manlove LS, et al. Virus-specific memory T cells populate tumors and can be repurposed for tumor immunotherapy. Nat Commun (2019) 10:567. doi: 10.1038/s41467-019-08534-1
163. Savas P, Virassamy B, Ye CZ, Salim A, Mintoff CP, Caramia F, et al. Single-cell profiling of breast cancer T cells reveals a tissue-resident memory subset associated with improved prognosis. Nat Med (2018) 24(7):986–93. doi: 10.1038/s41591-018-0078-7
164. Djenidi F, Adam J, Goubar A, Durgeau A, Meurice G, de Montpreville V, et al. CD8(+) CD103(+) tumor-infiltrating lymphocytes are tumor-specific tissue-resident memory T cells and a prognostic factor for survival in lung cancer patients. J Immunol (2015) 194(7):3475–86. doi: 10.4049/jimmunol.1402711
165. Molodtsov A, Turk MJ. Tissue resident CD8 memory T cell responses in cancer and autoimmunity. Front Immunol (2018) 9:2810. doi: 10.3389/fimmu.2018.02810
166. Peng H, Tian Z. Natural killer cell memory: progress and implications. Front Immunol (2017) 8:1143. doi: 10.3389/fimmu.2017.01143
167. Burbach BJ, O'Flanagan SD, Shao Q, Young KM, Slaughter JR, Rollins MR, et al. Irreversible electroporation augments checkpoint immunotherapy in prostate cancer and promotes tumor antigen-specific tissue-resident memory CD8+ T cells. Nat Commun (2021) 12(1):3862. doi: 10.1038/s41467-021-24132-6
168. Toraya-Brown S, Fiering S. Local tumour hyperthermia as immunotherapy for metastatic cancer. Int J Hyperthermia (2014) 30(8):531–9. doi: 10.3109/02656736.2014.968640
169. Repasky EA, Evans SS, Dewhirst MW. Temperature matters! and why it should matter to tumor immunologists. Cancer Immunol Res (2013) 1(4):210–6. doi: 10.1158/2326-6066.CIR-13-0118
170. Zhao J, Wen X, Tian L, Li T, Xu C, Wen X, et al. Irreversible electroporation reverses resistance to immune checkpoint blockade in pancreatic cancer. Nat Commun (2019) 10(1):899. doi: 10.1038/s41467-019-08782-1
171. Cancer.gov. Immunotherapy to treat cancer. Available at: https://www.cancer.gov/about-cancer/treatment/types/immunotherapy.
172. Machlenkin A, Goldberger O, Tirosh B, Paz A, Volovitz I, Bar-Haim E, et al. Combined dendritic cell cryotherapy of tumor induces systemic antimetastatic immunity. Clin Cancer Res (2005) 11(13):4955–61. doi: 10.1158/1078-0432.CCR-04-2422
173. Nakagawa H, Mizukoshi E, Iida N, Terashima T, Kitahara M, Marukawa Y, et al. In vivo Immunological antitumor effect of OK-432-stimulated dendritic cell transfer after radiofrequency ablation. Cancer Immunol Immunother (2014) 63(4):347–56. doi: 10.1007/s00262-013-1514-7
174. Yang J, Eresen A, Shangguan J, Ma Q, Yaghmai V, Zhang Z. Irreversible electroporation ablation overcomes tumor-associated immunosuppression to improve the efficacy of DC vaccination in a mice model of pancreatic cancer. Oncoimmunology (2021) 10(1):1875638. doi: 10.1080/2162402X.2021.1875638
175. Deng J, Zhang Y, Feng J, Wu F. Dendritic cells loaded with ultrasound-ablated tumour induce in vivo specific antitumour immune responses. Ultrasound Med Biol (2010) 36(3):441–8. doi: 10.1016/j.ultrasmedbio.2009.12.004
176. Niu LZ, Li JL, Zeng JY, Mu F, Liao MT, Yao F, et al. Combination treatment with comprehensive cryoablation and immunotherapy in metastatic hepatocellular cancer. World J Gastroenterol (2013) 19(22):3473–80. doi: 10.3748/wjg.v19.i22.3473
177. Chi KW, Liu SD, Li CP, Kuo HP, Wang YS, Chao Y, et al. Combination of conformal radiotherapy and intratumoral injection of adoptive dendritic cell immunotherapy in refractory hepatoma. J Immunother (2005) 28(2):129–35. doi: 10.1097/01.cji.0000154248.74383.5e
178. Chen Q, Hu QY, Dukhovlinova E, Chen GJ, Ahn S, Wang C, et al. Photothermal therapy promotes tumor infiltration and antitumor activity of CAR T cells. Advanced Materials (2019) 31(23):e1900192. doi: 10.1002/adma.201900192
179. Cui JW, Wang NY, Zhao HJ, Jin HF, Wang GJ, Niu C, et al. Combination of radiofrequency ablation and sequential cellular immunotherapy improves progression-free survival for patients with hepatocellular carcinoma. Int J Cancer (2014) 134(2):342–51. doi: 10.1002/ijc.28372
180. Lin M, Liang S, Wang X, Liang Y, Zhang M, Chen J, et al. Cryoablation combined with allogenic natural killer cell immunotherapy improves the curative effect in patients with advanced hepatocellular cancer. Oncotarget (2017) 8(47):81967–77. doi: 10.18632/oncotarget.17804
181. Alnaggar M, Lin M, Mesmar A, Liang SZ, Qaid A, Xu KC, et al. Allogenic natural killer cell immunotherapy combined with irreversible electroporation for stage IV hepatocellular carcinoma: survival outcome. Cell Physiol Biochem (2018) 48(5):1882–93. doi: 10.1159/000492509
182. Lin M, Zhang XY, Liang SZ, Luo HH, Alnaggar M, Liu AH, et al. Irreversible electroporation plus allogenic V gamma 9V delta 2 T cells enhances antitumor effect for locally advanced pancreatic cancer patients. Signal Transduction Targeted Ther (2020) 5(1):215. doi: 10.1038/s41392-020-00260-1
183. Thakur A, Littrup P, Paul EN, Adam B, Heilbrun LK, Lum LG. Induction of specific cellular and humoral responses against renal cell carcinoma after combination therapy with cryoablation and granulocyte-macrophage colony stimulating factor: a pilot study. J Immunotherapy (2011) 34(5):457–67. doi: 10.1097/CJI.0b013e31821dcba5
184. Chen ZB, Shen SQ, Peng BG, Tao JP. Intratumoural GM-CSF microspheres and CTLA-4 blockade enhance the antitumour immunity induced by thermal ablation in a subcutaneous murine hepatoma model. Int J Hyperthermia (2009) 25(5):374–82. doi: 10.1080/02656730902976807
185. Ye XY, Liang X, Chen Q, Miao QW, Chen XL, Zhang XD, et al. Surgical tumor-derived personalized photothermal vaccine formulation for cancer immunotherapy. ACS Nano (2019) 13(3):2956–68. doi: 10.1021/acsnano.8b07371
186. Au JT, Mittra A, Song TJ, Cavnar M, Jun K, Carson J, et al. Irreversible electroporation facilitates gene transfer of a GM-CSF plasmid with a local and systemic response. Surgery (2013) 154(3):496–503. doi: 10.1016/j.surg.2013.06.005
187. Domingo-Musibay E, Heun JM, Nevala WK, Callstrom M, Atwell T, Galanis E, et al. Endogenous heat-shock protein induction with or without radiofrequency ablation or cryoablation in patients with stage IV melanoma. Oncologist (2017) 22(9):1026. doi: 10.1634/theoncologist.2017-0060
188. Jiang J, Goel R, Schmechel S, Vercellotti G, Forster C, Bischof J. Pre-conditioning cryosurgery: cellular and molecular mechanisms and dynamics of TNF-alpha enhanced cryotherapy in an. Vivo prostate Cancer Model system. Cryobiol (2010) 61(3):280–8. doi: 10.1016/j.cryobiol.2010.09.006
189. Johnson EE, Yamane BH, Buhtoiarov IN, Lum HD, Rakhmilevich AL, Mahvi DM, et al. Radiofrequency ablation combined with KS-IL2 immunocytokine (EMD 273066) results in an enhanced antitumor effect against murine colon adenocarcinoma. Clin Cancer Res (2009) 15(15):4875–84. doi: 10.1158/1078-0432.CCR-09-0110
190. Habibi M, Kmieciak M, Graham L, Morales JK, Bear HD, Manjili MH. Radiofrequency thermal ablation of breast tumors combined with intralesional administration of IL-7 and IL-15 augments anti-tumor immune responses and inhibits tumor development and metastasis. Breast Cancer Res Treat (2009) 114(3):423–31. doi: 10.1007/s10549-008-0024-3
191. den Brok M, Sutmuller RPM, Nierkens S, Bennink EJ, Toonen LWJ, Figdor CG, et al. Synergy between in situ cryoablation and TLR9 stimulation results in a highly effective in vivo dendritic cell vaccine. Cancer Res (2006) 66(14):7285–92. doi: 10.1158/0008-5472.CAN-06-0206
192. Guo L, Yan DD, Yang D, Li Y, Wang X, Zalewski O, et al. Combinatorial photothermal and immuno cancer therapy using chitosan-coated hollow copper sulfide nanoparticles. ACS Nano (2014) 8(6):5670–81. doi: 10.1021/nn5002112
193. Chen Q, Xu LG, Liang C, Wang C, Peng R, Liu Z. Photothermal therapy with immune-adjuvant nanoparticles together with checkpoint blockade for effective cancer immunotherapy. Nat Commun (2016) 7:13193. doi: 10.1038/ncomms13193
194. Bassukas ID, Gamvroulia C, Zioga A, Nomikos K, Fotika C. Cryosurgery during topical imiquimod: a successful combination modality for lentigo maligna. Int J Dermatol (2008) 47(5):519–21. doi: 10.1111/j.1365-4632.2008.03562.x
195. Li X, Naylor MF, Le H, Nordquist RE, Teague TK, Howard CA, et al. Clinical effects of in situ photoimmunotherapy on late-stage melanoma patients a preliminary study. Cancer Biol Ther (2010) 10(11):1081–7. doi: 10.4161/cbt.10.11.13434
196. den Brok MH, Nierkens S, Wagenaars JA, Ruers TJ, Schrier CC, Rijke EO, et al. Saponin-based adjuvants create a highly effective anti-tumor vaccine when combined with in situ tumor destruction. Vaccine (2012) 30(4):737–44. doi: 10.1016/j.vaccine.2011.11.080
197. Urano M, Tanaka C, Sugiyama Y, Miya K, Saji S. Antitumor effects of residual tumor after cryoablation: the combined effect of residual tumor and a protein-bound polysaccharide on multiple liver metastases in a murine model. Cryobiology (2003) 46(3):238–45. doi: 10.1016/s0011-2240(03)00039-7
198. Li Y, Li XS, Doughty A, West C, Wang L, Zhou FF, et al. Phototherapy using immunologically modified carbon nanotubes to potentiate checkpoint blockade for metastatic breast cancer. Nanomedicine-Nanotechnology Biol Med (2019) 18:44–53. doi: 10.1016/j.nano.2019.02.009
199. Chiu HY, Leu JD, Chang CY, Lee YJ, Chen WR. Combination of radiofrequency ablation and glycated chitosan as treatment on a syngeneic breast tumor model. Anticancer Res (2017) 37(6):2965–74. doi: 10.21873/anticanres.11650
200. Chen YL, Wang CY, Yang FY, Wang BS, Chen JY, Lin LT, et al. Synergistic effects of glycated chitosan with high-intensity focused ultrasound on suppression of metastases in a syngeneic breast tumor model. Cell Death Dis (2014) 5:e1178. doi: 10.1038/cddis.2014.159
201. Zhou F, Wu S, Song S, Chen WR, Resasco DE, Xing D. Antitumor immunologically modified carbon nanotubes for photothermal therapy. Biomaterials (2012) 33(11):3235–42. doi: 10.1016/j.biomaterials.2011.12.029
202. Li XS, Ferrel GL, Guerra MC, Hode T, Lunn JA, Adalsteinsson O, et al. Preliminary safety and efficacy results of laser immunotherapy for the treatment of metastatic breast cancer patients. Photochemical Photobiological Sci (2011) 10(5):817–21. doi: 10.1039/c0pp00306a
203. Chen DJ, Li XS, Zhao H, Fu Y, Kang HR, Yao FF, et al. Dinitrophenyl hapten with laser immunotherapy for advanced malignant melanoma: a clinical study. Oncol Lett (2017) 13(3):1425–31. doi: 10.3892/ol.2016.5530
204. Zhang D, Zhang J, Li Q, Song AX, Li ZH, Luan YX. Cold to hot: rational design of a minimalist multifunctional photo-immunotherapy nanoplatform toward boosting immunotherapy capability. ACS Appl Materials Interfaces (2019) 11(36):32633–46. doi: 10.1021/acsami.9b09568
205. Hamamoto S, Okuma T, Yamamoto A, Kageyama K, Ueki A, Matsuoka T, et al. Combination radiofrequency ablation and local injection of the immunostimulant bacillus calmette-guerin induces antitumor immunity in the lung and at a distant VX2 tumor in a rabbit model. J Vasc Interventional Radiol (2015) 26(2):271–8. doi: 10.1016/j.jvir.2014.09.002
206. Hamamoto S, Okuma T, Yamamoto A, Kageyama K, Takeshita T, Sakai Y, et al. Radiofrequency ablation and immunostimulant OK-432: combination therapy enhances systemic antitumor immunity for treatment of VX2 lung tumors in rabbits. Radiology (2013) 267(2):405–13. doi: 10.1148/radiol.13120249
207. Li L, Wang W, Pan H, Ma G, Shi XY, Xie H, et al. Microwave ablation combined with OK-432 induces Th1-type response and specific antitumor immunity in a murine model of breast cancer. J Trans Med (2017) 15:23. doi: 10.1186/s12967-017-1124-9
208. Go EJ, Yang H, Chon HJ, Yang D, Ryu W, Kim DH, et al. Combination of irreversible electroporation and STING agonist for effective cancer immunotherapy. Cancers (2020) 12(11):3123. doi: 10.3390/cancers12113123
209. Ueki A, Okuma T, Hamamoto S, Kageyama K, Murai K, Miki Y. Combination therapy involving radiofrequency ablation and targeted chemotherapy with bevacizumab plus paclitaxel and cisplatin in a rabbit VX2 lung tumor model. BMC Res Notes (2018) 11(1):251. doi: 10.1186/s13104-018-3358-x
210. Gaitanis G, Bassukas ID. Intralesional bevacizumab as in-add adjuvant to immunocryosurgery for locally advanced basal cell carcinoma. J Eur Acad Dermatol Venereology (2014) 28(8):1117–21. doi: 10.1111/jdv.12327
211. ClinicalTrials.gov. Anti-OX40 antibody (MEDI6469) in patients with metastatic colorectal cancer. Available at: https://ClinicalTrials.gov/show/NCT02559024.
212. Bian H, Zheng JS, Nan G, Li R, Chen C, Hu CX, et al. Randomized trial of [131I] metuximab in treatment of hepatocellular carcinoma after percutaneous radiofrequency ablation. J Natl Cancer Institute (2014) 106(9):dju239. doi: 10.1093/jnci/dju239
213. Nagaya T, Friedman J, Maruoka Y, Ogata F, Okuyama S, Clavijo PE, et al. Host immunity following near-infrared photoimmunotherapy is enhanced with PD-1 checkpoint blockade to eradicate established antigenic tumors. Cancer Immunol Res (2019) 7(3):401–13. doi: 10.1158/2326-6066.CIR-18-0546
214. Pardoll DM. The blockade of immune checkpoints in cancer immunotherapy. Nat Rev Cancer (2012) 12(4):252–64. doi: 10.1038/nrc3239
215. Wei SC, Duffy CR, Allison JP. Fundamental mechanisms of immune checkpoint blockade therapy. Cancer Discov (2018) 8(9):1069–86. doi: 10.1158/2159-8290.CD-18-0367
216. Ribas A, Wolchok Jedd D. Cancer immunotherapy using checkpoint blockade. Science (2018) 359(6382):1350–5. doi: 10.1126/science.aar4060
217. Buchbinder EI, Desai A. CTLA-4 and PD-1 pathways similarities, differences, and implications of their inhibition. Am J Clin Oncology-Cancer Clin Trials (2016) 39(1):98–106. doi: 10.1097/COC.0000000000000239
218. Liu Y, Zheng P. How does an anti-CTLA-4 antibody promote cancer immunity? Trends Immunol (2018) 39(12):953–6. doi: 10.1016/j.it.2018.10.009
219. Darvin P, Toor SM, Nair VS, Elkord E. Immune checkpoint inhibitors: recent progress and potential biomarkers. Exp Mol Med (2018) 50:1–11. doi: 10.1038/s12276-018-0191-1
221. Kamada T, Togashi Y, Tay C, Ha D, Sasaki A, Nakamura Y, et al. PD-1(+) regulatory T cells amplified by PD-1 blockade promote hyperprogression of cancer. Proc Natl Acad Sci U. S. A (2019) 116(20):9999–10008. doi: 10.1073/pnas.1822001116
222. fda.gov. Drug approvals and databases (2022). Available at: https://www.fda.gov/drugs/development-approval-process-drugs/drug-approvals-and-databases.
223. Donini C, D'Ambrosio L, Grignani G, Aglietta M, Sangiolo D. Next generation immune-checkpoints for cancer therapy. J Thorac Dis (2018) 10(Suppl 13):S1581–601. doi: 10.21037/jtd.2018.02.79
224. Diesendruck Y, Benhar I. Novel immune check point inhibiting antibodies in cancer therapy-opportunities and challenges. Drug Resistance Updates (2017) 30:39–47. doi: 10.1016/j.drup.2017.02.001
225. fda.gov. FDA Approves opdualag for unresectable or metastatic melanoma. U.S. food and drug administration (2022). Available at: https://www.fda.gov/drugs/resources-information-approved-drugs/fda-approves-opdualag-unresectable-or-metastatic-melanoma.
226. cancer.org. Opdualag becomes first FDA-approved immunotherapy to target LAG-3. national cancer institute (2022). Available at: https://www.cancer.gov/news-events/cancer-currents-blog/2022/fda-opdualag-melanoma-lag-3.
227. Chauhan SKS, Koehl U, Kloess S. Harnessing NK cell checkpoint-modulating immunotherapies. Cancers (2020) 12(7):1807. doi: 10.3390/cancers12071807
228. He X, Xu C. Immune checkpoint signaling and cancer immunotherapy. Cell Res (2020) 30(8):660–9. doi: 10.1038/s41422-020-0343-4
229. Torphy RJ, Schulick RD, Zhu YW. Newly emerging immune checkpoints: promises for future cancer therapy. Int J Mol Sci (2017) 18(12):2642. doi: 10.3390/ijms18122642
230. Qin S, Xu LP, Yi M, Yu SN, Wu KM, Luo SX. Novel immune checkpoint targets: moving beyond PD-1 and CTLA-4. Mol Cancer (2019) 18(1):155. doi: 10.1186/s12943-019-1091-2
231. Rotte A. Combination of CTLA-4 and PD-1 blockers for treatment of cancer. J Exp Clin Cancer Res (2019) 38:255. doi: 10.1186/s13046-019-1259-z
232. Opdivo FDA approval history. drugs.com. Available at: https://www.drugs.com/history/opdivo.html.
233. Khair DO, Bax HJ, Mele S, Crescioli S, Pellizzari G, Khiabany A, et al. Combining immune checkpoint inhibitors: established and emerging targets and strategies to improve outcomes in melanoma. Front Immunol (2019) 10:453. doi: 10.3389/fimmu.2019.00453
234. Michot JM, Bigenwald C, Champiat S, Collins M, Carbonnel F, Postel-Vinay S, et al. Immune-related adverse events with immune checkpoint blockade: a comprehensive review. Eur J Cancer (2016) 54:139–48. doi: 10.1016/j.ejca.2015.11.016
235. Sullivan RJ, Weber JS. Immune-related toxicities of checkpoint inhibitors: mechanisms and mitigation strategies. Nat Rev Drug Discov (2022) 21(7):495–508. doi: 10.1038/s41573-021-00259-5
236. Shields BD, Mahmoud F, Taylor EM, Byrum SD, Sengupta D, Koss B, et al. Indicators of responsiveness to immune checkpoint inhibitors. Sci Rep (2017) 7:807. doi: 10.1038/s41598-017-01000-2
237. Yarchoan M, Hopkins A, Jaffee EM. Tumor mutational burden and response rate to PD-1 inhibition. New Engl J Med (2017) 377(25):2500–1. doi: 10.1056/NEJMc1713444
238. Hopkins AM, Rowland A, Kichenadasse G, Wiese MD, Gurney H, McKinnon RA, et al. Predicting response and toxicity to immune checkpoint inhibitors using routinely available blood and clinical markers. Br J Cancer (2017) 117(7):913–20. doi: 10.1038/bjc.2017.274
239. Le DT, Uram JN, Wang H, Bartlett BR, Kemberling H, Eyring AD, et al. PD-1 blockade in tumors with mismatch-repair deficiency. New Engl J Med (2015) 372(26):2509–20. doi: 10.1056/NEJMoa1500596
240. Le DT, Durham JN, Smith KN, Wang H, Bartlett BR, Aulakh LK, et al. Mismatch repair deficiency predicts response of solid tumors to PD-1 blockade. Science (2017) 357(6349):409–13. doi: 10.1126/science.aan6733
241. Daud AI, Wolchok JD, Robert C, Hwu WJ, Weber JS, Ribas A, et al. Programmed death-ligand 1 expression and response to the anti-programmed death 1 antibody pembrolizumab in melanoma. J Clin Oncol (2016) 34(34):4102. doi: 10.1200/JCO.2016.67.2477
242. Gandhi L, Rodriguez-Abreu D, Gadgeel S, Esteban E, Felip E, De Angelis F, et al. Pembrolizumab plus chemotherapy in metastatic non-Small-Cell lung cancer. New Engl J Med (2018) 378(22):2078–92. doi: 10.1056/NEJMoa1801005
243. Abiko K, Matsumura N, Hamanishi J, Horikawa N, Murakami R, Yamaguchi K, et al. IFN-gamma from lymphocytes induces PD-L1 expression and promotes progression of ovarian cancer. Br J Cancer (2015) 112(9):1501–9. doi: 10.1038/bjc.2015.101
244. Nakamura Y. Biomarkers for immune checkpoint inhibitor-mediated tumor response and adverse events. Front Med (2019) 6:119. doi: 10.3389/fmed.2019.00119
245. Davis AA, Patel VG. The role of PD-L1 expression as a predictive biomarker: an analysis of all US food and drug administration (FDA) approvals of immune checkpoint inhibitors. J Immunother Cancer (2019) 7(1):278. doi: 10.1186/s40425-019-0768-9
246. Naito Y, Saito K, Shiiba K, Ohuchi A, Saigenji K, Nagura A, et al. CD8(+) T cells infiltrated within cancer cell nests as a prognostic factor in human colorectal cancer. Cancer Res (1998) 58(16):3491–4.
247. Taylor RC, Patel A, Panageas KS, Busam KJ, Brady MS. Tumor-infiltrating lymphocytes predict sentinel lymph node positivity in patients with cutaneous melanoma. J Clin Oncol (2007) 25(7):869–75. doi: 10.1200/JCO.2006.08.9755
248. Hegde PS, Karanikas V, Evers S. The where, the when, and the how of immune monitoring for cancer immunotherapies in the era of checkpoint inhibition. Clin Cancer Res (2016) 22(8):1865–74. doi: 10.1158/1078-0432.CCR-15-1507
249. Galon J, Bruni D. Approaches to treat immune hot, altered and cold tumours with combination immunotherapies. Nat Rev Drug Discov (2019) 18(3):197–218. doi: 10.1038/s41573-018-0007-y
250. Varayathu H, Sarathy V, Thomas BE, Mufti SS, Naik R. Combination strategies to augment immune check point inhibitors efficacy-implications for translational research. Front Oncol (2021) 11:559161. doi: 10.3389/fonc.2021.559161
251. Shi LR, Chen LJ, Wu CP, Zhu YB, Xu B, Zheng X, et al. PD-1 blockade boosts radiofrequency ablation-elicited adaptive immune responses against tumor. Clin Cancer Res (2016) 22(5):1173–84. doi: 10.1158/1078-0432.CCR-15-1352
252. Han X, Wang R, Xu J, Chen Q, Liang C, Chen J, et al. In situ Thermal ablation of tumors in combination with nano-adjuvant and immune checkpoint blockade to inhibit cancer metastasis and recurrence. Biomaterials (2019) 224:119490. doi: 10.1016/j.biomaterials.2019.119490
253. Zhang L, Xu LC, Wang Y, Liu XF, Zhang MM, Li WT. Antitumor immunity augmented by combining radiofrequency ablation with anti-CTLA-4 therapy in a subcutaneous murine hepatoma model. J Vasc Interventional Radiol (2020) 31(7):1178–86. doi: 10.1016/j.jvir.2020.01.022
254. Zhang L, Wang J, Jiang JH, Zhang MM, Shen JL. CTLA-4 blockade suppresses progression of residual tumors and improves survival after insufficient radiofrequency ablation in a subcutaneous murine hepatoma model. Cardiovasc Interventional Radiol (2020) 43(9):1353–61. doi: 10.1007/s00270-020-02505-6
255. Zhu J, Yu M, Chen L, Kong P, Li L, Ma G, et al. Enhanced antitumor efficacy through microwave ablation in combination with immune checkpoints blockade in breast cancer: a pre-clinical study in a murine model. Diagn Interventional Imaging (2018) 99(3):135–42. doi: 10.1016/j.diii.2017.12.011
256. Duan X, Wang M, Han X, Ren J, Huang G, Ju S, et al. Combined use of microwave ablation and cell immunotherapy induces nonspecific immunity of hepatocellular carcinoma model mice. Cell Cycle (2020) 19(24):3595–607. doi: 10.1080/15384101.2020.1853942
257. Huang SJ, Li TQ, Chen Y, Liu JC, Wang YL, Yang CT, et al. Microwave ablation combined with anti-PD-1 therapy enhances systemic antitumor immunity in a multitumor murine model of Hepa1-6. Int J Hyperthermia (2022) 39(1):278–86. doi: 10.1080/02656736.2022.2032406
258. Shao D, Chen YP, Huang H, Liu YT, Chen JJ, Zhu DW, et al. LAG3 blockade coordinates with microwave ablation to promote CD8(+) T cell-mediated anti-tumor immunity. J Trans Med (2022) 20(1):433. doi: 10.1186/s12967-022-03646-7
259. Silvestrini MT, Ingham ES, Mahakian LM, Kheirolomoom A, Liu Y, Fite BZ, et al. Priming is key to effective incorporation of image-guided thermal ablation into immunotherapy protocols. JCI Insight (2017) 2(6):e90521. doi: 10.1172/jci.insight.90521
260. Sheybani ND, Witter AR, Thim EA, Yagita H, Bullock TNJ, Price RJ. Combination of thermally ablative focused ultrasound with gemcitabine controls breast cancer via adaptive immunit. J Immunother Cancer (2020) 8(2):e001008. doi: 10.1136/jitc-2020-001008
261. Mouratidis PXE, Costa M, Rivens I, Repasky EE, ter Haar G. Pulsed focused ultrasound can improve the anti-cancer effects of immune checkpoint inhibitors in murine pancreatic cancer. J Ther Soc Interface (2021) 18(180):20210266. doi: 10.1098/rsif.2021.0266
262. Waitz R, Solomon SB, Petre EN, Trumble AE, Fasso M, Norton L, et al. Potent induction of tumor immunity by combining tumor cryoablation with anti-CTLA-4 therapy. Cancer Res (2012) 72(2):430–9. doi: 10.1158/0008-5472.CAN-11-1782
263. Li F, Guo Z, Yu H, Zhang X, Si T, Liu C, et al. Anti-tumor immunological response induced by cryoablation and anti-CTLA-4 antibody in an in vivo RM-1 cell prostate cancer murine model. Neoplasma (2014) 61(6):659–71. doi: 10.4149/neo_2014_081
264. Kudo-Saito C, Fuwa T, Kawakami Y. Targeting ALCAM in the cryo-treated tumour microenvironment successfully induces systemic anti-tumour immunity. Eur J Cancer (2016) 62:54–61. doi: 10.1016/j.ejca.2016.04.013
265. Benzon B, Glavaris SA, Simons BW, Hughes RM, Ghabili K, Mullane P, et al. Combining immune check-point blockade and cryoablation in an immunocompetent hormone sensitive murine model of prostate cancer. Prostate Cancer Prostatic Dis (2018) 21(1):126–36. doi: 10.1038/s41391-018-0035-z
266. Zhu C, Lin S, Liang J, Zhu Y. PD-1 blockade enhances the anti-tumor immune response induced by cryoablation in a murine model of renal cell carcinoma. Cryobiology (2019) 87:86–90. doi: 10.1016/j.cryobiol.2019.01.015
267. Annen R, Kato S, Demura S, Miwa S, Yokka A, Shinmura K, et al. Tumor-specific immunoenhancing effects after local cryoablation for metastatic bone tumor in a mouse model. Int J Mol Sci (2022) 23(16):9445. doi: 10.3390/ijms23169445
268. Tan J, Liu T, Fan W, Wei J, Zhu B, Liu Y, et al. Anti-PD-L1 antibody enhances curative effect of cryoablation via antibody-dependent cell-mediated cytotoxicity mediating PD-L1highCD11b+ cells elimination in hepatocellular carcinoma. Acta Pharm Sin B (2022) 13:632–47. doi: 10.1016/j.apsb.2022.08.006
269. Yu Z-P, Sun X-W, He Y-P, Gu J, Jin Y. PD-1 monoclonal antibodies enhance the cryoablation-induced antitumor immune response: a breast cancer murine model research. Int J Hyperthermia (2023) 40(1):2164625. doi: 10.1080/02656736.2022.2164625
270. Narayanan JSS, Ray P, Hayashi T, Whisenant TC, Vicente D, Carson DA, et al. Irreversible electroporation combined with checkpoint blockade and TLR7 stimulation induces antitumor immunity in a murine pancreatic cancer model. Cancer Immunol Res (2019) 7(10):1714–26. doi: 10.1158/2326-6066.CIR-19-0101
271. Qian JJ, Chen TC, Wu QC, Zhou L, Zhou WH, Wu LM, et al. Blocking exposed PD-L1 elicited by nanosecond pulsed electric field reverses dysfunction of CD8(+) T cells in liver cancer. Cancer Lett (2020) 495:1–11. doi: 10.1016/j.canlet.2020.09.015
272. Babikr F, Wan JB, Xu AZ, Wu ZJ, Ahmed S, Freywald A, et al. Distinct roles but cooperative effect of TLR3/9 agonists and PD-1 blockade in converting the immunotolerant microenvironment of irreversible electroporation-ablated tumors. Cell Mol Immunol (2021) 18(12):2632–47. doi: 10.1038/s41423-021-00796-4
273. Shi XJ, O'Neill C, Wang XT, Chen YJ, Yu YX, Tan M, et al. Irreversible electroporation enhances immunotherapeutic effect in the off-target tumor in a murine model of orthotopic HCC. Am J Cancer Res (2021) 11(6):3304–19.
274. Bulner S, Prodeus A, Gariepy J, Hynynen K, Goertz DE. ENHANCING CHECKPOINT INHIBITOR THERAPY WITH ULTRASOUND STIMULATED MICROBUBBLES. Ultrasound Med Biol (2019) 45(2):500–12. doi: 10.1016/j.ultrasmedbio.2018.10.002
275. Eranki A, Srinivasan P, Ries M, Kim A, Lazarski CA, Rossi CT, et al. High-intensity focused ultrasound (HIFU) triggers immune sensitization of refractory murine neuroblastoma to checkpoint inhibitor therapy. Clin Cancer Res (2020) 26(5):1152–61. doi: 10.1158/1078-0432.CCR-19-1604
276. Nam GH, Pahk KJ, Jeon S, Park HJ, Kim GB, Oh SJ, et al. Investigation of the potential immunological effects of boiling histotripsy for cancer treatment. Advanced Ther (2020) 3(8). doi: 10.1002/adtp.201900214
277. Qu SB, Worlikar T, Felsted AE, Ganguly A, Beems MV, Hubbard R, et al. Non-thermal histotripsy tumor ablation promotes abscopal immune responses that enhance cancer immunotherapy. J Immunother Cancer (2020) 8(1):e000200. doi: 10.1136/jitc-2019-000200
278. Abe S, Nagata H, Crosby EJ, Inoue Y, Kaneko K, Liu CX, et al. Combination of ultrasound-based mechanical disruption of tumor with immune checkpoint blockade modifies tumor microenvironment and augments systemic antitumor immunity. J Immunother Cancer (2022) 10(1):e003717. doi: 10.1136/jitc-2021-003717
279. Ibuki Y, Takahashi Y, Tamari K, Minami K, Seo Y, Isohashi F, et al. Local hyperthermia combined with CTLA-4 blockade induces both local and abscopal effects in a murine breast cancer model. Int J Hyperthermia (2021) 38(1):363–71. doi: 10.1080/02656736.2021.1875059
280. Ruarus AH, Vroomen L, Geboers B, van Veldhuisen E, Puijk RS, Nieuwenhuizen S, et al. Percutaneous irreversible electroporation in locally advanced and recurrent pancreatic cancer (PANFIRE-2): a multicenter, prospective, single-arm, phase II study. Radiology (2020) 294(1):212–20. doi: 10.1148/radiol.2019191109
281. Hendricks-Wenger A, Hutchison R, Vlaisavljevich E, Allen IC. Immunological effects of histotripsy for cancer therapy. Front Oncol (2021) 11:681629. doi: 10.3389/fonc.2021.681629
282. Liu Y, Crawford BM, Vo-Dinh T. Gold nanoparticles-mediated photothermal therapy and immunotherapy. Immunotherapy (2018) 10(13):1175–88. doi: 10.2217/imt-2018-0029
283. Chang MY, Hou ZY, Wang M, Li CX, Lin J. Recent advances in hyperthermia therapy-based synergistic immunotherapy. Advanced Materials (2021) 33(4):e2004788. doi: 10.1002/adma.202004788
284. Li ZH, Deng J, Sun JH, Ma YL. Hyperthermia targeting the tumor microenvironment facilitates immune checkpoint inhibitors. Front Immunol (2020) 11:595207. doi: 10.3389/fimmu.2020.595207
285. Wilhelm S, Tavares AJ, Dai Q, Ohta S, Audet J, Dvorak HF, et al. Analysis of nanoparticle delivery to tumours. Nat Rev Materials (2016) 1(5):16014. doi: 10.1038/natrevmats.2016.14
286. Immune Checkpoint Inhibitors Markets, 2020-2025: PD-1 Inhibitors Segment is Expected to Hold the Largest Market Share - ResearchAndMarkets.com. NewsRX LLC, Vol. 53 (2020).
287. Motzer RJ, Tannir NM, McDermott DF, Frontera OA, Melichar B, Choueiri TK, et al. Nivolumab plus ipilimumab versus sunitinib in advanced renal-cell carcinoma. New Engl J Med (2018) 378(14):1277–90. doi: 10.1056/NEJMoa1712126
288. Overman MJ, Lonardi S, Wong KYM, Lenz HJ, Gelsomino F, Aglietta M, et al. Durable clinical benefit with nivolumab plus ipilimumab in DNA mismatch repair-Deficient/Microsatellite instability-high metastatic colorectal cancer. J Clin Oncol (2018) 36(8):773. doi: 10.1200/JCO.2017.76.9901
289. Postow M, Larkin J, Wolchok JD, Chiarion-Sileni V, Hodi FS, Rutkowski P, et al. Pooled 3-year overall survival data from phase II and phase III trials of nivolumab (NIVO) combined with ipilimumab (IPI) in advanced melanoma. J Immunother Cancer (2017) 5.
290. Kim DW, Haymaker C, McQuail N, Sirmans E, Spencer C, Glitza I, et al. Pilot study of intratumoral (IT) cryoablation (cryo) in combination with systemic checkpoint blockade in patients with metastatic melanoma (MM). J Immunother Cancer (2015) 3(Suppl 2):P137. doi: 10.1186/2051-1426-3-S2-P137
291. Mooradian M, Fintelmann FJ, Kaufman HE, Mino-Kenudson M, Barth JL, Lawless A, et al. The use of cryoablation to overcome resistance to PD-1 blockade in unresectable melanoma. J Clin Oncol (2021) 39(15_suppl):9538–8. doi: 10.1200/JCO.2021.39.15_suppl.9538
292. Comen EA, Bryce Y, Page DB, Solomon SB, Rodine M, Abaya CD, et al. Preoperative checkpoint inhibition (CPI) and cryoablation (Cryo) in women with early-stage breast cancer (ESBC). J Clin Oncol (2019) 37(15_suppl):592–2. doi: 10.1200/JCO.2019.37.15_suppl.592
293. Wetzel R, Monge B MC, Xie C, Mabry-Hrones D, Webb S, Akoth E, et al. A pilot study of the combination of checkpoint inhibition with ablation in subjects with biliary tract cancer. J Clin Oncol (2021) 39(15_suppl):e16150. doi: 10.1200/JCO.2021.39.15_suppl.e16150
294. Campbell MT, Matin SF, Tam AL, Sheth RA, Ahrar K, Tidwell RS, et al. Pilot study of tremelimumab with and without cryoablation in patients with metastatic renal cell carcinoma. Nat Commun (2021) 12(1):6375. doi: 10.1038/s41467-021-26415-4
295. Ross AE, Hurley PJ, Tran PT, Rowe SP, Benzon B, Neal TO, et al. A pilot trial of pembrolizumab plus prostatic cryotherapy for men with newly diagnosed oligometastatic hormone-sensitive prostate cancer. Prostate Cancer Prostatic Dis (2020) 23(1):184–93. doi: 10.1038/s41391-019-0176-8
296. Thomsen LCV, Kristoffersen EK, Melve GK, Biermann M, Helle SI, Azeem W, et al. A prospective phase I trial of dendritic cell-based cryoimmunotherapy in metastatic castration-resistant prostate cancer. J Clin Oncol (2020) 38(15_suppl):3029. doi: 10.1200/JCO.2020.38.15_suppl.3029
297. McArthur HL, Diab A, Page DB, Yuan J, Solomon SB, Sacchini V, et al. A pilot study of preoperative single-dose ipilimumab and/or cryoablation in women with early-stage breast cancer with comprehensive immune profiling. Clin Cancer Res (2016) 22(23):5729–37. doi: 10.1158/1078-0432.CCR-16-0190
298. Lyu N, Kong Y, Li X, Mu L, Deng H, Chen H, et al. Ablation reboots the response in advanced hepatocellular carcinoma with stable or atypical response during PD-1 therapy: a proof-of-Concept study. Front Oncol (2020) 10:580241. doi: 10.3389/fonc.2020.580241
299. Shi L, Zhou C, Long X, Li H, Chen C, Peng C, et al. 949P thermal ablation plus toripalimab in patients with advanced hepatocellular carcinoma: phase I results from a multicenter, open-label, controlled phase I/II trial (IR11330). Ann Oncol (2021) 32:S826. doi: 10.1016/j.annonc.2021.08.169
300. Monge B MC, Xie C, McVey J, Mabry-Hrones D, Duffy A, Wood BJ, et al. Long-term survival of combined ablation therapy and tremelimumab with or without durvalumab in advanced hepatocellular carcinoma. J Clin Oncol (2020) 38(15_suppl):e16689. doi: 10.1200/JCO.2020.38.15_suppl.e16689
301. Segal NH, Kemeny NE, Cercek A, Reidy DL, Raasch PJ, Warren P, et al. Non-randomized phase II study to assess the efficacy of pembrolizumab (Pem) plus radiotherapy (RT) or ablation in mismatch repair proficient (pMMR) metastatic colorectal cancer (mCRC) patients. J Clin Oncol (2016) 34(15_suppl):3539. doi: 10.1200/JCO.2016.34.15_suppl.3539
302. Agdashian D, ElGindi M, Xie C, Sandhu M, Pratt D, Kleiner DE, et al. The effect of anti-CTLA4 treatment on peripheral and intra-tumoral T cells in patients with hepatocellular carcinoma. Cancer Immunol Immunotherapy (2019) 68(4):599–608. doi: 10.1007/s00262-019-02299-8
303. Duffy AG, Ulahannan SV, Makorova-Rusher O, Rahma O, Wedemeyer H, Pratt D, et al. Tremelimumab in combination with ablation in patients with advanced hepatocellular carcinoma. J Hepatol (2017) 66(3):545–51. doi: 10.1016/j.jhep.2016.10.029
304. Xie C, Duffy AG, Mabry-Hrones D, Wood B, Levy E, Krishnasamy V, et al. Tremelimumab in combination with microwave ablation in patients with refractory biliary tract cancer. Hepatology (2019) 69(5):2048–60. doi: 10.1002/hep.30482
305. Hormigo A, Mandeli J, Ghatan S, Hadjipanayis C, Yong R, Kim-Schulze S, et al. Abstract CT231: clinical study of the safety and tolerability of laser interstitial thermal therapy and avelumab for recurrent glioblastoma. Cancer Res (Chicago Ill.) (2020) 80(16_Supplement):CT231. doi: 10.1158/1538-7445.AM2020-CT231
306. Qiao G, Wang X, Zhou X, Morse MA, Wu J, Wang S, et al. Immune correlates of clinical benefit in a phase I study of hyperthermia with adoptive T cell immunotherapy in patients with solid tumors. Int J Hyperthermia (2019) 36(1):74–82. doi: 10.1080/02656736.2019.1647350
307. O'Neill C, Hayat T, Hamm J, Healey M, Zheng Q, Li Y, et al. A phase 1b trial of concurrent immunotherapy and irreversible electroporation in the treatment of locally advanced pancreatic adenocarcinoma. Surgery (2020) 168(4):610–6. doi: 10.1016/j.surg.2020.04.057
308. Zhang NA, Li ZQ, Han X, Zhu ZY, Li ZJ, Zhao Y, et al. Irreversible electroporation: an emerging immunomodulatory therapy on solid tumors. Front Immunol (2022) 12:811726. doi: 10.3389/fimmu.2021.811726
309. van der Meel R, Sulheim E, Shi Y, Kiessling F, Mulder WJM, Lammers T. Smart cancer nanomedicine. Nat Nanotechnol (2019) 14(11):1007–17. doi: 10.1038/s41565-019-0567-y
310. Shi Y, Lammers T. Combining nanomedicine and immunotherapy. Accounts Chem Res (2019) 52(6):1543–54. doi: 10.1021/acs.accounts.9b00148
311. Moy AJ, Tunnell JW. Combinatorial immunotherapy and nanoparticle mediated hyperthermia. Advanced Drug Deliv Rev (2017) 114:175–83. doi: 10.1016/j.addr.2017.06.008
312. Ranjbartehrani P, Etheridge M, Ramadhyani S, Natesan H, Bischof J, Shao Q. Characterization of miniature probes for cryosurgery, thermal ablation, and irreversible electroporation on small animals. Advanced Ther (2022) 5(5):2100212. doi: 10.1002/adtp.202100212
313. Restrepo CR, Field DH, Kim AY. Current state of combination of locoregional therapies with immune checkpoint inhibition. J Vasc Interventional Radiol (2020) 31(11):1740. doi: 10.1016/j.jvir.2020.07.011
314. Abdo J, Cornell DL, Mittal SK, Agrawal DK. Immunotherapy plus cryotherapy: potential augmented abscopal effect for advanced cancers. Front Oncol (2018) 8:85. doi: 10.3389/fonc.2018.00085
315. Chang XF, Lu XF, Guo JH, Teng GJ. Interventional therapy combined with immune checkpoint inhibitors: emerging opportunities for cancer treatment in the era of immunotherapy. Cancer Treat Rev (2019) 74:49–60. doi: 10.1016/j.ctrv.2018.08.006
316. Chen DS, Mellman I. Oncology meets immunology: the cancer-immunity cycle. Immunity (2013) 39(1):1–10. doi: 10.1016/j.immuni.2013.07.012
317. Ferguson TA, Choi JY, Green DR. Armed response: how dying cells influence T-cell functions. Immunol Rev (2011) 241:77–88. doi: 10.1111/j.1600-065X.2011.01006.x
318. Lippitz BE. Cytokine patterns in patients with cancer: a systematic review. Lancet Oncol (2013) 14(6):E218–28. doi: 10.1016/S1470-2045(12)70582-X
319. Rosenberg SA. Cancer immunotherapy comes of age. Nat Clin Pract Oncol (2005) 2(3):115–5. doi: 10.1038/ncponc0101
320. Franciszkiewicz K, Boissonnas A, Boutet M, Combadiere C, Mami-Chouaib F. Role of chemokines and chemokine receptors in shaping the effector phase of the antitumor immune response. Cancer Res (2012) 72(24):6325–32. doi: 10.1158/0008-5472.CAN-12-2027
321. Riella LV, Paterson AM, Sharpe AH, Chandraker A. Role of the PD-1 pathway in the immune response. Am J Transplant (2012) 12(10):2575–87. doi: 10.1111/j.1600-6143.2012.04224.x
322. So T, Lee SW, Croft M. Tumor necrosis factor/tumor necrosis factor receptor family members that positively regulate immunity. Int J Hematol (2006) 83(1):1–11. doi: 10.1532/IJH97.05120
323. Peng WY, Liu CW, Xu CY, Lou YY, Chen JQ, Yang Y, et al. PD-1 blockade enhances T-cell migration to tumors by elevating IFN-gamma inducible chemokines. Cancer Res (2012) 72(20):5209–18. doi: 10.1158/0008-5472.CAN-12-1187
324. Franciszkiewicz K, Le Floc'h A, Boutet M, Vergnon I, Schmitt A, Mami-Chouaib F. CD103 or LFA-1 engagement at the immune synapse between cytotoxic T cells and tumor cells promotes maturation and regulates T-cell effector functions. Cancer Res (2013) 73(2):617–28. doi: 10.1158/0008-5472.CAN-12-2569
325. Chen DS, Irving BA, Hodi FS. Molecular pathways: next-generation immunotherapy-inhibiting programmed death-ligand 1 and programmed death-1. Clin Cancer Res (2012) 18(24):6580–7. doi: 10.1158/1078-0432.CCR-12-1362
326. Greaves P, Gribben JG. The role of B7 family molecules in hematologic malignancy. Blood (2013) 121(5):734–44. doi: 10.1182/blood-2012-10-385591
Keywords: cancer, focal therapy, tumor ablation, immunotherapy, immune checkpoint, immunomodulation
Citation: Jiang M, Fiering S and Shao Q (2023) Combining energy-based focal ablation and immune checkpoint inhibitors: preclinical research and clinical trials. Front. Oncol. 13:1153066. doi: 10.3389/fonc.2023.1153066
Received: 28 January 2023; Accepted: 12 April 2023;
Published: 01 May 2023.
Edited by:
Yueyong Xiao, Chinese People’s Liberation Army General Hospital, ChinaReviewed by:
Bostjan Markelc, Institute of Oncology Ljubljana, SloveniaEvan Unger, NuvOx Pharma, United States
Nana Zhang, Xi’an Jiaotong University, China
Copyright © 2023 Jiang, Fiering and Shao. This is an open-access article distributed under the terms of the Creative Commons Attribution License (CC BY). The use, distribution or reproduction in other forums is permitted, provided the original author(s) and the copyright owner(s) are credited and that the original publication in this journal is cited, in accordance with accepted academic practice. No use, distribution or reproduction is permitted which does not comply with these terms.
*Correspondence: Qi Shao, shaox070@umn.edu