- 1Department of Cancer Biology and Therapeutics, Precision Oncology and Multi-Omics Clinic, Genetic Counseling Clinic, Department of Clinical Biochemistry, MVR Cancer Centre and Research Institute, Calicut, Kerala, India
- 2Gastrointestinal Oncology Service, University College London Hospitals National Health Services (NHS) Foundation Trust, London, United Kingdom
- 3Universtiy College London (UCL) Cancer Institute, University College London Hospitals National Health Services (NHS) Foundation Trust, London, United Kingdom
- 4Whittington Health, National Health Services (NHS), London, United Kingdom
Pancreatic ductal adenocarcinoma (PDAC) is the most common exocrine tumor of the pancreas characterized by late diagnosis, adverse overall 5-year survival, a higher propensity for metastatic disease, and lack of efficacy of systemic therapy options. These adverse outcomes can be partly attributed to complex tumor microenvironment (TME). Over the past decade, immunotherapy has revolutionized the management of certain cancers; thus far, the immunologically ‘non-inflamed’ tumor microenvironment in PDACs has proven to be challenging. Indolamine 2,3-dioxygenase 1 (IDO1) is the rate-limiting enzyme in the catabolic pathway of L-Tryptophan, an essential amino acid, that gives rise to the immunosuppressive metabolite Kynurenine. IDO1, Indolamine 2,3-dioxygenase 2 (IDO2), and Tryptophan 2,3-dioxygenase (TDO) are the key enzymes in the tryptophan catabolic pathway but we focus on the role of the predominant enzyme form IDO1 in this review. Nicotinamide phosphoribosyl transferase (iNAMPT) regulates the intracellular concentration of NAD and is upregulated in the tumor. In light of the potential role of IDO1 as a driver of hostile TME in PDAC and NAD+ as a key coenzyme in anti-tumor immune response, this review urges focus on extensive research and initiation of clinical trials using IDO1 and NAMPT inhibitors in pancreatic cancer in the future.
Introduction
Pancreatic tumors, specifically pancreatic ductal adenocarcinoma (PDAC) composing 90% of the exocrine tumors of the pancreas are largely known for diagnosis at later stages, poor overall 5-year survival, a higher propensity for metastatic disease, and poor response to immunotherapy. A multitude of factors is responsible for the grim profile of the disease. As research is underway to uncover the molecular blueprint of PDAC and its unique tumor microenvironment (TME), we intend to review the significant, yet underappreciated intracellular metabolic contribution of tumor cells and TME in rendering PDAC an immune-privileged malignancy (1).
The immune microenvironment of Pancreatic cancer
The tumor microenvironment (TME) influences the malignant process of pancreatic ductal adenocarcinoma (PDAC) more than any other cancer type. Pancreatic TME (2) constitutes a complex interaction between tumor cells, stromal cells such as cancer-associated fibroblasts (CAFs), endothelial cells, perivascular cells, immune cells such as tumor-infiltrating lymphocytes (TILs), tumor-associated macrophages (TAMs), and extracellular matrix elements such as collagen (3, 4), hyaluronic acid (5, 6), fibronectin (7–9), laminin (10–14), and sulfated glycosaminoglycans (15) in an acidic pH (16), resulting in a heavy stromal desmoplastic reaction. In terms of multi-omic approaches, genomic and transcriptomic advances have unearthed the molecular biology of pancreatic cancer to an extent. However, the role of immunotherapy is yet a challenging subject in PDAC. To help uncover newer biomarkers and address the unmet need to comprehend the immunologically ‘non-inflamed’ tumor microenvironment, we require a better understanding of the tumor proteome and metabolome, and the crosstalk between T cells and intermediary molecules that are crucial in signaling pathways and gene regulation (17). The metabolome is the unexcavated effector that executes immune-mediated signals within a malignant cell. From that perspective, a unique ketone Kynurenine has stood out owing to its novel immunoregulatory properties. Indolamine 2,3-dioxygenase 1 (IDO1) is a rate-limiting enzyme in the catabolic pathway of L-tryptophan, an essential amino acid, that gives rise to the immunosuppressive metabolite L-kynurenine. IDO1, Indolamine 2,3-dioxygenase 2 (IDO2), and Tryptophan 2,3-dioxygenase (TDO) are the key enzymes in the pathway, but we focus on the predominant enzyme form IDO1 in this review. The role of Kynurenine in immune privilege and its blood levels in cancer has been studied in cancers of the colon, stomach, breast, and prostate (18). However, its role in the immune microenvironment of Pancreatic cancer is yet to be investigated in-depth. Here we intend to focus solely on the function and duality of immune cells within the pancreatic ‘cold’ TME from the perspective of tumor-metabolomic crosstalk.
The lymphoid and myeloid compartments hold the recipe for an indifferent TME
Pancreatic TME houses a heterogeneous population of immune cells skewed to immune-suppressive functionality than with tumor-inhibiting characteristics. This is initially driven by the unique genomic alterations observed in PDAC.
The interplay of immune cell regulation, TME behavior, and cellular energetics
A harsh TME studded with immunosuppressive or pro-tumor myeloid-derived suppressor cells (MDSCs) and tumor-associated macrophages (TAMs) drives the PDAC cells into extensive metabolic rewiring (19) involving central carbon metabolism, glucose, and glutamine utilization, but generating lesser ATP. This rewiring is predominantly driven by oncogenic KRAS variants. Eventually, the tumor cells and T-cells in the TME compete for glucose as activation of T-cells requires upregulation of GLUT-1 glucose transporter via TCR and Akt activation. As the supply of glucose is diminished along with glutamine and arginine, the T-cells lose functional capacity (20). With an active IDO-1 enzyme breaking down tryptophan to release immunosuppressive metabolites, the immune cells face an added disadvantage. Furthermore, the IDO1- Kynurenine pathway is a de novo source of nicotinamide dinucleotide (NAD) which is metabolized to adenosine which in turn binds to T cell adenosine receptor A2R that inhibits effector T cells and stimulates Tregs. Hypoxic TMEs are under higher influence of this adenosinergic axis activation and immunosuppression. The immunosuppressive-hypoxic environment is further stiffened by intracellular nicotinamide phosphoribosyl transferase (iNAMPT), a crucial enzyme in NAD biosynthesis, via the NAD/SIRT1/HIF-1α axis acts on the mobilization of MDSCs by inhibiting CXCR4 transcription (21). The energetics within the cells define tumor immune escape, the potential for invasiveness, and metastasis.
Increased levels of kynurenine prevent the proliferation of NK cells and T cells by interactions with the aryl hydrocarbon (AhR) receptor. The general control non-deprepressible-2 (GCN2) and mammalian target of rapamycin (mTOR) kinases are also believed to be involved in this effect. Kynurenine via the AhR and FoxP3 transcription factor also urges the differentiation of naïve CD4+ T-cells to T- regulatory cells that are immunosuppressive by nature (22). Tryptophan metabolism birthing kynurenine is a pathway that is a proven generator of one-carbon units for the pancreatic stellate cells (PSCs), a precursor of CAFs to help maintain tumor growth by purine nucleotide synthesis (23). It is unknown if the primary aim of the pathway is providing immune privilege to tissues or generating one-carbon units and maintaining redox balance in the tissue.
There is a notable differential regulation of TH1/TH2 by IDO. Stimulation of IDO activity by positive signals or lack of inhibitory molecules such as DNAX-activation protein 12 (DAP12) appears to decrease TH1 cellular responses. Further, 3-hydroxyanthranilic (3-HAA) and quinolinic acid metabolites of kynurenine have been found to induce selective apoptosis of murine TH1 cells but not of TH2 cells. It could be a specific negative feedback mechanism for TH1 cells (24). Yet, induction of apoptosis of macrophages required >10-fold concentrations of 3-HAA in a study by Fallarino F et al. In addition, this apoptosis is surprisingly not mediated by Fas/Fas ligand and cytochrome c (25). TH17 cells (CD4+IL-17+) are effector T cells found in the pancreatic TME. Along with IL-17A, they are involved in immune regulation. However, He S et al. remarks “the mechanism for regulating the balance of TH17/Treg cells in the tumor microenvironment needs to be further elucidated” (26). We now recognize that anthranilic acid (AA) and 3-hydroxyanthranilic acid (3-HAA) can abolish the function of TH17 cells in a dose-dependent manner (27). The anti-tumor function of TFH cells (Follicular helper T cells) in PDAC is gridlocked by the PD-L1/PD-1 signaling pathway (28). B cells have been implicated in immune tolerance, but the exact mechanism is still under investigation. Regulatory B cells (iBregs) have been found to suppress immune responses via IL-10 which is the cytokine responsible for converting naïve CD4+ T cells to CD4+ CD25+ Foxp3+ Tregs that produced TGF-β. Bregs have also been found to cause T cell apoptosis by cell-to-cell physical contact. Moreover, a CTLA-4 dependent TGFβ/IDO axis in B cells can induce IDO1 and convert them to induced iBregs that could create Tregs, Type 1 T-regulatory cell (Tr1), and TH3 cells which in turn suppress TH1 cell induction (29, 30).
Kynurenine pathway gene expression and immune cell inhibitory checkpoints (T cell signatures) are inversely correlated. Higher Kynurenine and kynurenic acid levels were also found to cause anergy phenotype and CD4+ T-cell exhaustion (Figures 1A, B). Fundamentally, kynurenine has an unmistakable role in cancer immune escape, making IDO1 a potential candidate to assist immunotherapy (31).
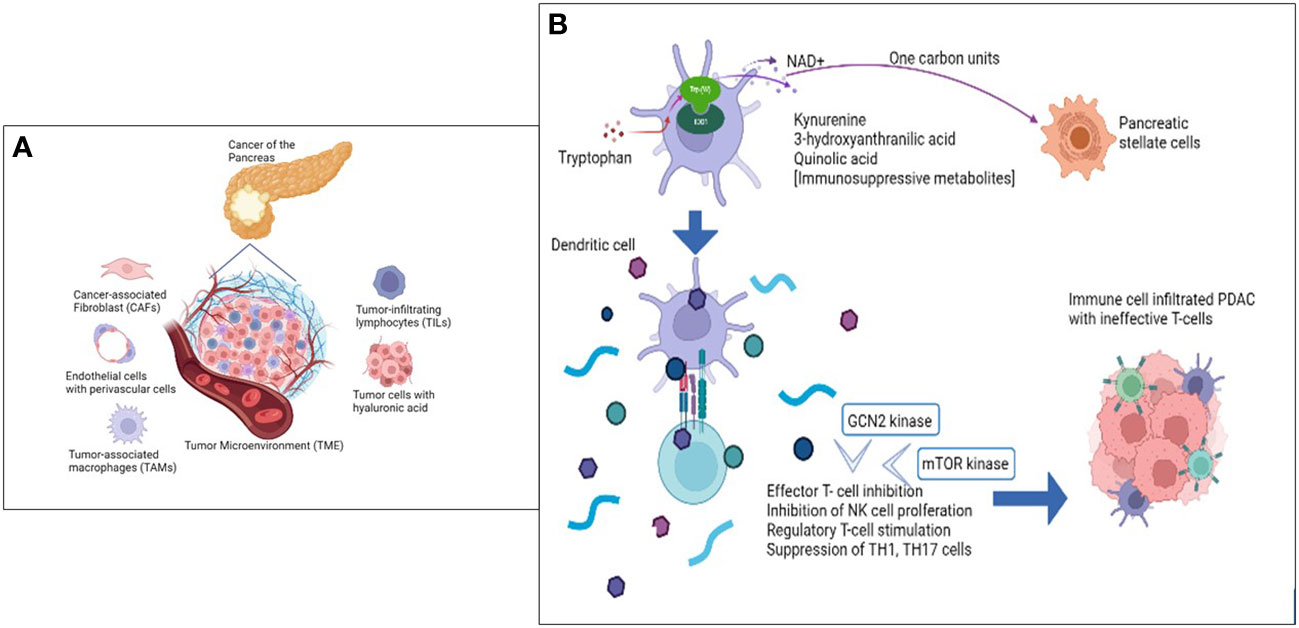
Figure 1 The Tumor microenvironment metabolome is key in PDAC immune modulation. (A) Cellular components of the Tumor Microenvironment (TME) that primarily influence and contribute to ‘non-inflamed’ tumor architecture. (B) Role of Tryptophan and IDO1 enzyme in immune inhibition of PDAC targeting Immune cell repertoire.
A quest beyond cellular elements to the realm of the metabolome
Tumor cell machinery utilizing Kynurenine for Immune suppression in the pancreas
In the normal state, mature dendritic cells in lymphoid organs, the vagina, and placental and lung endothelial cells express the enzyme IDO (32). The cellular localization of the enzyme is cytosol (33). Evolutionarily, induction of IDO and generation of kynurenine metabolites have been proposed to be for two reasons: a genome-immune protective mechanism during the phagocyte-induced respiratory burst that generates reactive oxygen species (ROS) including superoxide anion. This event induces the production of IDO enzyme that uses the superoxide anion to break the pyrrole ring of tryptophan, but few anions escape to enter the nucleus. To thwart pathogen invasion, ROS generation inadvertently causes DNA strand damage, thus inducing PARP molecules that are necessary for DNA damage repair (DDR). This process drains the NAD+ pool which is in turn replenished by the IDO-Kynurenine-NAD+ pathway (34). The second evolutionary advantage is that Kynurenine and its metabolites play a significant role in immune privilege. IDO bridges the innate and adaptive immune systems. The adenosine/purinergic pathway, cytotoxic T lymphocyte antigen-4 (CTLA-4) and programmed cell death-1 (PD-L1) aid Kynurenine and metabolites to induce immune privilege in certain sites. This is attained by a) tryptophan exhaustion via induction of GCN2 and suppression of mTOR1 pathways that lead to T cell inhibition, b) induction of TH17 cells and transdifferentiation by dendritic cells and macrophages which are induced by the effect of Kynurenine on aryl hydrocarbon receptor (AhR), c) using PTEN protein to aid in the differentiation of CD4 T cells into Treg cells, d) inhibition of IL-2 that impeded CD4 T cell survival (35). However, the intended metabolic effect is sabotaged when the scenario changes from a healthy state to cancer.
In KPC mice bearing PDAC, restriction of serine has been found to be of no effect. This is postulated to be due to a) increased synthesis of serine de novo, or b) due to tryptophan catabolism offering one-carbon units. The latter is supported by the finding that interstitial fluid analysis shows severe tryptophan depletion (36, 37). Immune cells (T cells and macrophages) are sources of the cytokine IFN-γ (38) which is the sole inducer of the IDO enzyme. As immune cell infiltration in PDAC increases (39), it also increases tryptophan metabolism. In the catabolic pathway, L-tryptophan is initially converted to N-formyl-l-kynurenine by the rate-limiting enzymes. This metabolite is further converted to L-kynurenine which undergoes further downstream catabolism to form xanthurenate, glutaryl-CoA, and picolinate apart from generation of NAD+. Immunomodulation of the TME is however brought about by the intermediary metabolites and compounds formed during Tryptophan catabolism, as elaborated early in the review (Figure 2) (40). Whether the immunosuppressive milieu that follows this pathway activation is the intended effect or the bystander effect, is a crucial and intriguing argument that we are yet to unravel.
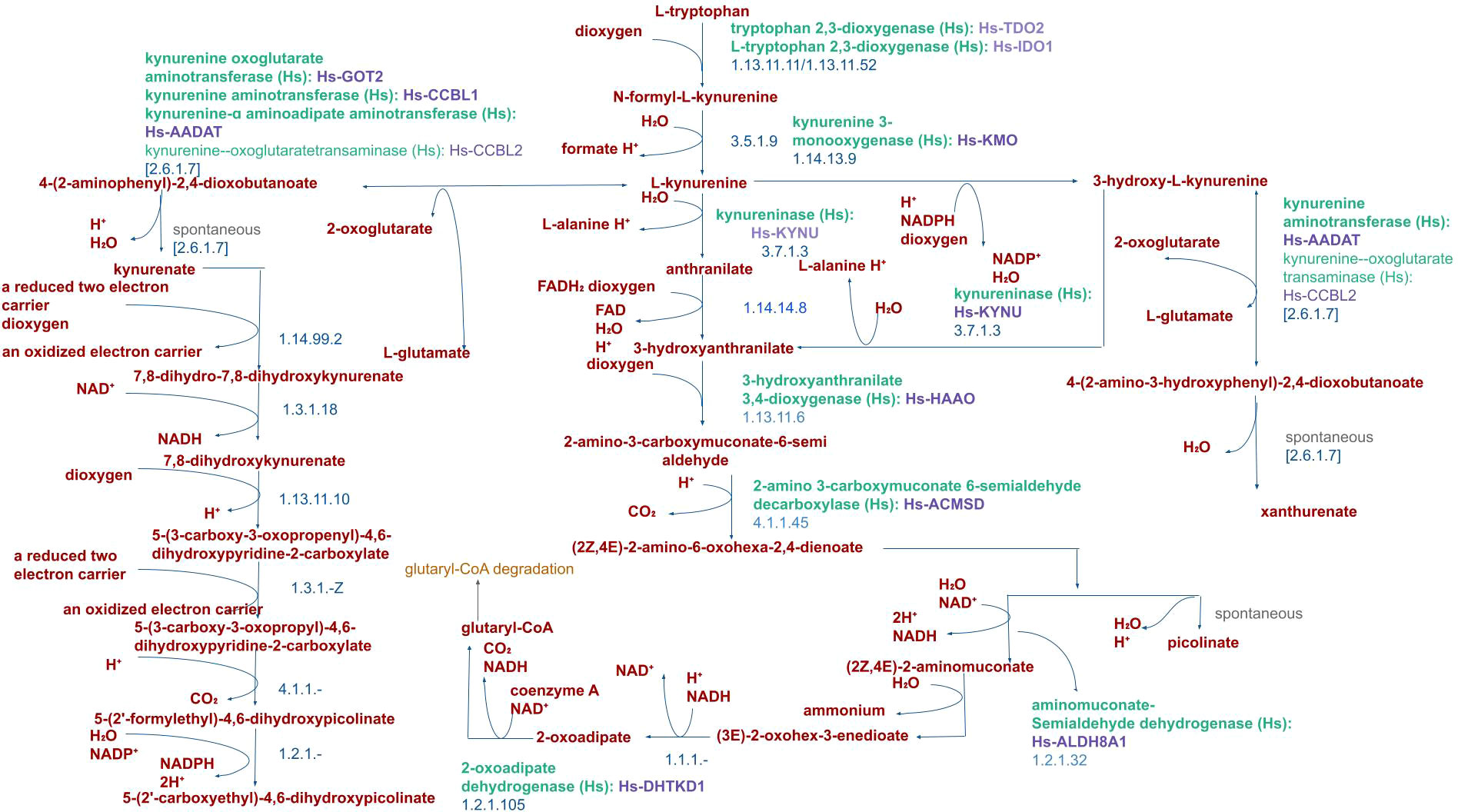
Figure 2 Pathway of Tryptophan catabolism in Humans. Catabolism of L-tryptophan by the human IDO1 enzyme produces L-kynurenine and immunosuppressive metabolites such as 3-hydroxyanthranilic acid (3-HAA) and quinolinic acid metabolites.
Hypoxia and Kynurenine-dependent immune modulation conundrum in PDAC
Hypoxia as an independent factor of tumor aggressiveness is pronounced in Pancreatic cancer when compared to other tumor types. An elevated hypoxia-inducible factor-1 (HIF-1) is an ominous marker for poor prognosis and metastatic events due to the activation of epithelial to mesenchymal transition (EMT). The extensive desmoplastic reaction seen in pancreatic tumor tissue is also directly related to hypoxia (41). Bao B et al. (42) have studied pancreatic tumor cells to uncover molecular mechanisms of hypoxia and discovered that hypoxia-induced increased levels of VEGF, IL-6, and gene expression of cancer stem cell signature genes Nanog, Oct4, and EZH2 that are implicated in tumor invasiveness and aggressive biology. In addition, the pancreatospheres showed increased expression of miR-21 and miR-210. Vieira NF et al. (43) have shown that tissue expression of the two micro-RNAs combined with CA 19-9 had a diagnostic accuracy of 100% in PDAC. The introduction of an inhibitory molecule, a curcumin-derived novel analogue (CDF) revealed a reversal of the hypoxia-induced molecular signature in the tumor cells (42). Intracellular and tumoral hypoxia instinctively shifts the cellular metabolism from oxidative phosphorylation (OXPHOS) yielding high ATP to anaerobic glycolysis with the formation of lactate, bypass of the tricarboxylic acid cycle, and loss of ATP. Genomic factors such as KRAS mutations (KRAS/MEK/ERK signaling pathway), AMPK signaling pathway, Wnt-β catenin pathway, NFAT5, PDK1, LDHA, and P4HA1 alongside the epigenomic regulators UHRF1/SIRT4 axis, LSD1, and miR-124 are implicated in shifting the metabolic balance to an anaerobic glycolytic cellular phenotype that helps in improved malignant potential. The actions of the above factors are mediated by the sustenance of HIF-α under a hypoxic environment leading to the transcription of genes involved in enhancing tumor invasiveness and spread (44).
Hypoxia (1% O2) in dendritic cells (DC) further leads to the induction of indolamine 2,3-dioxygenase dependent on the adenosine A3 receptor as shown by Xiang Song et al. (45) Although a stabilized HIF-α inhibits IDO enzyme of the tryptophan metabolic pathway (46) in glioblastoma cells (47), the immunosuppressive Kynurenine, and its metabolites have an independent mechanistic role in the TME of tissues such as PDAC. This has been proven by a study conducted by Witkiewicz AK et al. (48) in 36 patients with PDAC and five pancreatic cancer cell lines. All samples revealed an increased expression of the IDO enzyme. Functionally active variants of the IDO2 gene were also detected in patients. This corroborates the significant role of Kynurenine and its metabolites in immune modulation in the biology of pancreatic cancer. The kynurenine pathway and mitochondrial metabolism are related via superoxide generation and mitochondrial entry of one-carbon units into the TCA cycle via α-ketoadipate. Though hypoxia and superoxide ions were not found to induce IDO1 expression, when cell cultures were transferred from a monolayer to an AI 3D culture, it was found that the IDO1-dependent kynurenine pathway was dramatically switched on. Predictably, kynurenine efflux increased and the same could be abolished by an IDO1 inhibitor and a JAK1 inhibitor (23). Overall, the contribution of the IDO-Kynurenine-NAD+ pathway in immune modulation, cell proliferation, and metastatic promotion is likely undermined in cancer of the pancreas.
Genomic and transcriptomic influences of IDO-Kyn pathway in PDAC
Pancreatic cancer, specifically PDAC has variants in key genes that aid in malignant transformation and metastasis. The most common genes carrying pathogenic mutations or variations are KRAS, TP53, SMAD4, and CDKN2A. Even though consistent across 50-90% of samples, we are yet to defeat the outcome using targeted therapy, except for renewed optimism with KRAS G12C inhibitors. Lesser common pathogenic variants within the DNA Damage Repair (DDR) pathway or in the BRCA1 or BRCA2 genes however respond to platinum-based chemotherapy and Poly (ADP-ribose) polymerase inhibitors (PARPi) as evidenced by the POLO trial (19). The extensive effect of KRAS mutations on the metabolome of PDAC has been discussed earlier (44). Furthermore, intact p53 was found to mitigate IDO1 expression in lung cancer thereby preventing metastasis (49) hence loss of function of TP53 is likely to induce IDO1 expression. Overall, every omic variation inadvertently affects the tumor metabolome.
The novel genes altered in PDAC have specific functions to execute in the immunological milieu of the TME. Further, Cullis J et al. describe the immunologistics of KRAS variations in pancreatic cancer cell lines. Oncogenic KRAS signaling leads to activation of a plethora of downstream molecules such as TGF-β, GM-CSF, IL-10, IL-6, and the ELR+ CXC chemokines CXCL1, CXCL2, CXCL5, and CXCL8. These independently influence immunomodulation of the TME by not only influencing recruitment and differentiation of immune cells but also by inducing immune suppression and promoting tumor growth (50). KRAS mutations were also found to downregulate MHC Class I and inhibit immunostimulatory IL-18 to evade immune cell attacks in cancer cell lines (51). Wild-type TP53 has been shown to increase T cell infiltration in PDAC whereas mutant TP53 eliminates suppression on IL-6 and induces NF-κB and TNF-α signaling promoting metastasis. TP53 knockout mice also showed T cell differentiation into TH17 cells that play a role in immune privilege as described earlier in this review. Further, p53 influences the PDAC microenvironment through a vast network of microRNAs, namely miR-34a, miR-21, miR-203, miR-128, miR-192, miR-200a, miR-200c, miR- miR-29. MDM2 that suppresses action of TP53 is in turn regulated by miR-29, miR-125a, miR-143, miR-145, and miR-365. miR-145 and miR-135 are specifically influence cellular glycolysis and TCA cycle to promote metastasis and growth of PDAC cells. Interestingly, gain of function (GOF) mutations in TP53 inhibit p73 preventing binding of nuclear factor-Y transcription factor (NF-Y) which increases gene expression of PDGFR-β that is required for development of the fibrotic PDAC TME (52). SMAD4 is a target of TGF-β and its loss is observed in more than half of PDAC cases. Principe DR et al. show that samples with a loss of SMAD4 had lower T cell infiltrates irrespective of addition of neoadjuvant chemotherapy and cell culture from human pancreatic cancer cell lines showed a reduction in IFN-γ. SMAD4 loss also impaired function of members of the CCL/CXCL chemokine family and Interleukin cytokine family. The role of SMAD4 in modulating the immunogenicity of PDAC and pivoting the efficacy of immunotherapy is indisputable (53).
Therapeutic potential of metabolome and clinical trials
Tryptophan and IDO-mediated immunosuppression within tumor cells and draining lymph nodes place IDO as a potential target to reverse suppression and augment immune-mediated anti-tumor interventions (54–56). Clinical trials have been investigating IDO1 inhibitors with positive and negative associations as results. Garber et al. (57) describe the enzyme and its activities in cancer as a “black box”. It is imperative that we have a better understanding of enzymology, molecular biology, and TME characteristics to employ successful IDO1 inhibitors in the clinic. The keyword ‘IDO1’ returns 47 clinical trial results from https://clinicaltrials.gov/.The list of active, recruiting, enrolling by invitation, and completed trials (n=25) are listed in Table 1.
Epacadostat is the most investigated IDO1 inhibitor in cancer. However, there is only one study on metastatic pancreatic cancer [Epacadostat, Pembrolizumab, and CRS-207, With or Without CY/GVAX Pancreas in Patients With Metastatic Pancreas Cancer (NCT03006302)]. As described in this review, IDO inhibitors may also have to be combined with serine and glycine restriction to block one-carbon unit generation and reversal of immune suppression. Inclusion of the molecular profile of tumor cells and variables influencing TME for example Immunoscore may help with a better-targeted selection of patients for Randomised Controlled Trials (RCTs) that are more likely to give results.
Owing to unimpressive Phase III trials of IDO1 inhibitors as single agents, Shao J et al. described a novel method wherein the inhibitor was loaded onto hyaluronic acid-modified nanomaterial graphene oxide (HA-GO) in conjunction with ongoing CAR-T cell therapy. The authors found that by inhibiting IDO so, CAR-T cells were more efficacious in vivo and in vitro (58). The safety and toxicity profile of combinatorial therapies are still being investigated, but the future is promising.
NAD+ being a crucial coenzyme cofactor generated by the kynurenine pathway, it is only right to investigate potential inhibitors. NAMPT regulates intracellular NAD concentration hence NAMPT inhibitors FK866/APO866, CHS-828, KPT-9274, and OT-82 are undergoing Phase I/II clinical trials (59–61).
Conclusion
Pancreatic cancer is a poster child for unsuccessful targeted or immune checkpoint inhibitor therapeutic strategies. Javadrashid D et al. (62) highlight the challenges and have compiled the myriad factors influencing pancreatic cancer and the immune microenvironment. Efforts are to be made to improve survival outcomes by exploring novel treatment molecules and protocols, apart from standard of care. This review explores a novel avenue by highlighting the power of metabolomic influence, specifically the tryptophan metabolic cascade over the tumor immune microenvironment of PDAC. The immunosuppression offered by the downstream actions of IDO1 is credited to the activation of GCN2, inhibition of mTOR pathways which consequently steer tryptophan degradation, and Kynurenine pathway metabolites induced AhR activation (30). It is evident that the effector T cell repertoire is significantly restrained and incapacitated by Kynurenine and allied metabolites leading to immune suppression of the TME. IDO1 and NAMPT inhibitors as combinatorial therapy is postulated to be lucrative in the therapeutics of solid tumors. It is therefore clinically essential to expand the investigation of these agents into PDAC, a classic example of immune-transformed (63) and challenged cancer.
Author contributions
All authors listed have made a direct and intellectual contribution to the work and approved it for publication.
Acknowledgment
The authors acknowledge Ms Febin Huda PP and Ms Pravitha KS for helping with manuscript formatting, sourcing, drafting of the Table and formatting the figure of tryptophan metabolism.
Conflict of interest
The authors declare that the research was conducted in the absence of any commercial or financial relationships that could be construed as a potential conflict of interest.
Publisher’s note
All claims expressed in this article are solely those of the authors and do not necessarily represent those of their affiliated organizations, or those of the publisher, the editors and the reviewers. Any product that may be evaluated in this article, or claim that may be made by its manufacturer, is not guaranteed or endorsed by the publisher.
References
1. Rubin SJS, Sojwal RS, Gubatan J, Rogalla S. The tumor immune microenvironment in pancreatic ductal adenocarcinoma: neither hot nor cold. Cancers (Basel) (2022) 14(17):4236. doi: 10.3390/cancers14174236
2. Ferrara B, Pignatelli C, Cossutta M, Citro A, Courty J, Piemonti L. The extracellular matrix in pancreatic cancer: description of a complex network and promising therapeutic options. Cancers (Basel) (2021) 13(17):4442. doi: 10.3390/cancers13174442
3. Weniger M, Honselmann KC, Liss AS. The extracellular matrix and pancreatic cancer: a complex relationship. Cancers (Basel) (2018) 10(9):316. doi: 10.3390/cancers10090316
4. Cox TR, Erler JT. Molecular pathways: connecting fibrosis and solid tumor metastasis. Clin Cancer Res (2014) 20(14):3637–43. doi: 10.1158/1078-0432.CCR-13-1059
5. Tavianatou AG, Caon I, Franchi M, Piperigkou Z, Galesso D, Karamanos NK. Hyaluronan: molecular size-dependent signaling and biological functions in inflammation and cancer. FEBS J (2019) 286(15):2883–908. doi: 10.1111/febs.14777
6. Bohaumilitzky L, Huber AK, Stork EM, Wengert S, Woelfl F, Boehm H. A trickster in disguise: hyaluronan's ambivalent roles in the matrix. Front Oncol (2017) 7:242. doi: 10.3389/fonc.2017.00242
7. Hosein AN, Brekken RA, Maitra A. Pancreatic cancer stroma: an update on therapeutic targeting strategies. Nat Rev Gastroenterol Hepatol (2020) 17(8):487–505. doi: 10.1038/s41575-020-0300-1
8. Gopal S, Veracini L, Grall D, Butori C, Schaub S, Audebert S, et al. Fibronectin-guided migration of carcinoma collectives. Nat Commun (2017) 8:14105. doi: 10.1038/ncomms14105
9. Attieh Y, Clark AG, Grass C, Richon S, Pocard M, Mariani P, et al. Cancer-associated fibroblasts lead tumor invasion through integrin-β3-dependent fibronectin assembly. J Cell Biol (2017) 216(11):3509–20. doi: 10.1083/jcb.201702033
10. Yang C, Liu Z, Zeng X, Wu Q, Liao X, Wang X, et al. Evaluation of the diagnostic ability of laminin gene family for pancreatic ductal adenocarcinoma. Aging (Albany NY). (2019) 11(11):3679–703. doi: 10.18632/aging.102007
11. Okada Y, Nishiwada S, Yamamura K, Sho M, Baba H, Takayama T, et al. Identification of laminin γ2 as a prognostic and predictive biomarker for determining response to gemcitabine-based therapy in pancreatic ductal adenocarcinoma. Eur J Cancer (2021) 146:125–34. doi: 10.1016/j.ejca.2020.12.031
12. Huang C, Chen J. Laminin−332 mediates proliferation, apoptosis, invasion, migration and epithelial−to−mesenchymal transition in pancreatic ductal adenocarcinoma. Mol Med Rep (2021) 23(1):11. doi: 10.3892/mmr.2020.11649
13. Chen J, Zhang H, Luo J, Wu X, Li X, Zhao X, et al. Overexpression of α3, β3 and γ2 chains of laminin-332 is associated with poor prognosis in pancreatic ductal adenocarcinoma. Oncol Lett (2018) 16(1):199–210. doi: 10.3892/ol.2018.8678
14. Huanwen W, Zhiyong L, Xiaohua S, Xinyu R, Kai W, Tonghua L. Intrinsic chemoresistance to gemcitabine is associated with constitutive and laminin-induced phosphorylation of FAK in pancreatic cancer cell lines. Mol Cancer (2009) 8:125. doi: 10.1186/1476-4598-8-125
15. Quail DF, Joyce JA. Microenvironmental regulation of tumor progression and metastasis. Nat Med (2013) 19(11):1423–37. doi: 10.1038/nm.3394
16. Lucero-Acuña A, Guzmán R. Nanoparticle encapsulation and controlled release of a hydrophobic kinase inhibitor: three stage mathematical modeling and parametric analysis. Int J Pharm (2015) 494(1):249–57. doi: 10.1016/j.ijpharm.2015.07.049
17. Karamitopoulou E. Tumour microenvironment of pancreatic cancer: immune landscape is dictated by molecular and histopathological features. Br J Cancer (2019) 121(1):5–14. doi: 10.1038/s41416-019-0479-5
18. Walczak K, Wnorowski A, Turski WA, Plech T. Kynurenic acid and cancer: facts and controversies. Cell Mol Life Sci (2020) 77(8):1531–50. doi: 10.1007/s00018-019-03332-w
19. Yamamoto K, Iwadate D, Kato H, Nakai Y, Tateishi K, Fujishiro M. Targeting the metabolic rewiring in pancreatic cancer and its tumor microenvironment. Cancers (Basel) (2022) 14(18):4351. doi: 10.3390/cancers14184351
20. Shyer JA, Flavell RA, Bailis W. Metabolic signaling in T cells. Cell Res (2020) 30:649–59. doi: 10.1038/s41422-020-0379-5
21. Audrito V, Managò A, Gaudino F, Sorci L, Messana VG, Raffaelli N, et al. NAD-biosynthetic and consuming enzymes as central players of metabolic regulation of innate and adaptive immune responses in cancer. Front Immunol (2019) 10:1720. doi: 10.3389/fimmu.2019.01720
22. Kim M, Tomek P. Tryptophan: a rheostat of cancer immune escape mediated by immunosuppressive enzymes IDO1 and TDO. Front Immunol (2021) 12:636081. doi: 10.3389/fimmu.2021.636081
23. Yamamoto AC, Falcone M, Huerta Uribe A, Zhang T, Athineos D, Pietzke M, et al. Immune-regulated IDO1-dependent tryptophan metabolism is source of one-carbon units for pancreatic cancer and stellate cells. Mol Cell (2021) 81(11):2290–2302.e7. doi: 10.1016/j.molcel.2021.03.019
24. Xu H, Zhang GX, Ciric B, Rostami A. IDO: a double-edged sword for T(H)1/T(H)2 regulation. Immunol Lett (2008) 121(1):1–6. doi: 10.1016/j.imlet.2008.08.008
25. Fallarino F, Grohmann U, Vacca C, Bianchi R, Orabona C, Spreca A, et al. T Cell apoptosis by tryptophan catabolism. Cell Death Differ (2002) 9(10):1069–77. doi: 10.1038/sj.cdd.4401073
26. He S, Fei M, Wu Y, Zheng D, Wan D, Wang L, et al. Distribution and clinical significance of Th17 cells in the tumor microenvironment and peripheral blood of pancreatic cancer patients. Int J Mol Sci (2011) 12(11):7424–37. doi: 10.3390/ijms12117424
27. Desvignes L, Ernst JD. Interferon-gamma-responsive nonhematopoietic cells regulate the immune response to mycobacterium tuberculosis. Immunity (2009) 31(6):974–85. doi: 10.1016/j.immuni.2009.10.007
28. Lin X, Ye L, Wang X, Liao Z, Dong J, Yang Y, et al. Follicular helper T cells remodel the immune microenvironment of pancreatic cancer via secreting CXCL13 and IL-21. Cancers (Basel) (2021) 13(15):3678. doi: 10.3390/cancers13153678
29. Nouël A, Pochard P, Simon Q, Ségalen I, Le Meur Y, Pers JO, et al. B-cells induce regulatory T cells through TGF-β/IDO production in a CTLA-4 dependent manner. J Autoimmun (2015) 59:53–60. doi: 10.1016/j.jaut.2015.02.004
30. Krupa A, Kowalska I. The kynurenine pathway-new linkage between innate and adaptive immunity in autoimmune endocrinopathies. Int J Mol Sci (2021) 22(18):9879. doi: 10.3390/ijms22189879
31. Rad Pour S, Morikawa H, Kiani NA, Yang M, Azimi A, Shafi G, et al. Exhaustion of CD4+ T-cells mediated by the kynurenine pathway in melanoma. Sci Rep (2019) 9(1):12150. doi: 10.1038/s41598-019-48635-x
32. Abd El-Fattah EE. IDO/kynurenine pathway in cancer: possible therapeutic approaches. J Transl Med (2022) 20(1):347. doi: 10.1186/s12967-022-03554-w
33. Gaudet P, Livstone MS, Lewis SE, Thomas PD. Phylogenetic-based propagation of functional annotations within the gene ontology consortium. Brief Bioinform (2011) 12(5):449–62. doi: 10.1093/bib/bbr042
34. Moffett JR, Namboodiri MA. Tryptophan and the immune response. Immunol Cell Biol (2003) 81(4):247–65. doi: 10.1046/j.1440-1711.2003.t01-1-01177.x
35. Routy JP, Routy B, Graziani GM, Mehraj V. The kynurenine pathway is a double-edged sword in immune-privileged sites and in cancer: implications for immunotherapy. Int J Tryptophan Res (2016) 9:67–77. doi: 10.4137/IJTR.S38355
36. Maddocks ODK, Athineos D, Cheung EC, Lee P, Zhang T, van den Broek NJF, et al. Modulating the therapeutic response of tumours to dietary serine and glycine starvation. Nature (2017) 544(7650):372–6. doi: 10.1038/nature22056
37. Sullivan MR, Danai LV, Lewis CA, Chan SH, Gui DY, Kunchok T, et al. Quantification of microenvironmental metabolites in murine cancers reveals determinants of tumor nutrient availability. Elife (2019) 8:e44235. doi: 10.7554/eLife.44235
38. Gao Y, Yang W, Pan M, Scully E, Girardi M, Augenlicht LH, et al. Gamma delta T cells provide an early source of interferon gamma in tumor immunity. J Exp Med (2003) 198(3):433–42. doi: 10.1084/jem.20030584
39. Xu C, Sui S, Shang Y, Yu Z, Han J, Zhang G, et al. The landscape of immune cell infiltration and its clinical implications of pancreatic ductal adenocarcinoma. J Adv Res (2020) 24:139–48. doi: 10.1016/j.jare.2020.03.009
40. Caspi R, Billington R, Keseler IM, Kothari A, Krummenacker M, Midford PE, et al. The MetaCyc database of metabolic pathways and enzymes - a 2019 update. Nucleic Acids Res (2020) 48(D1):D445–53. doi: 10.1093/nar/gkz862
41. Erkan M, Kurtoglu M, Kleeff J. The role of hypoxia in pancreatic cancer: a potential therapeutic target? Expert Rev Gastroenterol Hepatol (2016) 10(3):301–16. doi: 10.1586/17474124.2016.1117386
42. Bao B, Ali S, Ahmad A, Azmi AS, Li Y, Banerjee S, et al. Hypoxia-induced aggressiveness of pancreatic cancer cells is due to increased expression of VEGF, IL-6 and miR-21, which can be attenuated by CDF treatment. PloS One (2012) 7(12):e50165. doi: 10.1371/journal.pone.0050165
43. Vieira NF, Serafini LN, Novais PC, Neto FSL, Cirino MLA, Kemp R, et al. The role of circulating miRNAs and CA19-9 in pancreatic cancer diagnosis. Oncotarget (2021) 12(17):1638–50. doi: 10.18632/oncotarget.28038
44. Tao J, Yang G, Zhou W, Qiu J, Chen G, Luo W, et al. Targeting hypoxic tumor microenvironment in pancreatic cancer. J Hematol Oncol (2021) 14(1):14. doi: 10.1186/s13045-020-01030-w
45. Song X, Zhang Y, Zhang L, Song W, Shi L. Hypoxia enhances indoleamine 2,3-dioxygenase production in dendritic cells. Oncotarget (2018) 9(14):11572–80. doi: 10.18632/oncotarget.24098
46. Adeyemi OS, Awakan OJ, Afolabi LB, Rotimi DE, Oluwayemi E, Otuechere CA, et al. Hypoxia and the kynurenine pathway: implications and therapeutic prospects in alzheimer's disease. Oxid Med Cell Longev (2021) 2021:5522981. doi: 10.1155/2021/5522981
47. Schmidt SK, Ebel S, Keil E, Woite C, Ernst JF, Benzin AE, et al. Regulation of IDO activity by oxygen supply: inhibitory effects on antimicrobial and immunoregulatory functions. PloS One (2013) 8(5):e63301. doi: 10.1371/journal.pone.0063301
48. Witkiewicz AK, Costantino CL, Metz R, Muller AJ, Prendergast GC, Yeo CJ, et al. Genotyping and expression analysis of IDO2 in human pancreatic cancer: a novel, active target. J Am Coll Surg (2009) 208(5):781–7; discussion 787-9. doi: 10.1016/j.jamcollsurg.2008.12.018
49. Tang D, Yue L, Yao R, Zhou L, Yang Y, Lu L, et al. P53 prevent tumor invasion and metastasis by down-regulating IDO in lung cancer. Oncotarget (2017) 8(33):54548–57. doi: 10.18632/oncotarget.17408
50. Cullis J, Das S, Bar-Sagi D. Kras and tumor immunity: friend or foe? Cold Spring Harb Perspect Med (2018) 8(9):a031849. doi: 10.1101/cshperspect.a031849
51. Pereira F, Ferreira A, Reis CA, Sousa MJ, Oliveira MJ, Preto A. KRAS as a modulator of the inflammatory tumor microenvironment: therapeutic implications. Cells (2022) 11(3):398. doi: 10.3390/cells11030398
52. McCubrey JA, Yang LV, Abrams SL, Steelman LS, Follo MY, Cocco L, et al. Effects of TP53 mutations and miRs on immune responses in the tumor microenvironment important in pancreatic cancer progression. Cells (2022) 11(14):2155. doi: 10.3390/cells11142155
53. Principe DR, Underwood PW, Kumar S, Timbers KE, Koch RM, Trevino JG, et al. Loss of SMAD4 is associated with poor tumor immunogenicity and reduced PD-L1 expression in pancreatic cancer. Front Oncol (2022) 12:806963. doi: 10.3389/fonc.2022.806963
54. Opitz CA, Litzenburger UM, Sahm F, Ott M, Tritschler I, Trump S, et al. An endogenous tumour-promoting ligand of the human aryl hydrocarbon receptor. Nature (2011) 478(7368):197–203. doi: 10.1038/nature10491
55. Liu X, Shin N, Koblish HK, Yang G, Wang Q, Wang K, et al. Selective inhibition of IDO1 effectively regulates mediators of antitumor immunity. Blood (2010) 115(17):3520–30. doi: 10.1182/blood-2009-09-246124
56. Wainwright DA, Dey M, Chang A, Lesniak MS. Targeting tregs in malignant brain cancer: overcoming IDO. Front Immunol (2013) 4:116. doi: 10.3389/fimmu.2013.00116
57. Garber K. A new cancer immunotherapy suffers a setback. Science (2018) 360(6389):588. doi: 10.1126/science.360.6389.588
58. Shao J, Hou L, Liu J, Liu Y, Ning J, Zhao Q, et al. Indoleamine 2,3-dioxygenase 1 inhibitor-loaded nanosheets enhance CAR-T cell function in esophageal squamous cell carcinoma. Front Immunol (2021) 12:661357. doi: 10.3389/fimmu.2021.661357
59. Navas LE, Carnero A. Nicotinamide adenine dinucleotide (NAD) metabolism as a relevant target in cancer. Cells (2022) 11(17):2627. doi: 10.3390/cells11172627
60. Galli U, Colombo G, Travelli C, Tron GC, Genazzani AA, Grolla AA. Recent advances in NAMPT inhibitors: a novel immunotherapic strategy. Front Pharmacol (2020) 11:656. doi: 10.3389/fphar.2020.00656
61. Wei Y, Xiang H, Zhang W. Review of various NAMPT inhibitors for the treatment of cancer. Front Pharmacol (2022) 13:970553. doi: 10.3389/fphar.2022.970553
62. Javadrashid D, Baghbanzadeh A, Derakhshani A, Leone P, Silvestris N, Racanelli V, et al. Pancreatic cancer signaling pathways, genetic alterations, and tumor microenvironment: the barriers affecting the method of treatment. Biomedicines (2021) 9(4):373. doi: 10.3390/biomedicines9040373
Keywords: pancreatic cancer, metabolome, immune microenvironment, Indolamine 2,3-dioxygenase 1, Kynurenine, NAD, epacadostat, NAMPT inhibitor
Citation: Anu RI, Shiu K-K and Khan KH (2023) The immunomodulatory role of IDO1-Kynurenine-NAD+ pathway in switching cold tumor microenvironment in PDAC. Front. Oncol. 13:1142838. doi: 10.3389/fonc.2023.1142838
Received: 12 January 2023; Accepted: 01 June 2023;
Published: 30 June 2023.
Edited by:
Deborah A. Altomare, University of Central Florida, United StatesReviewed by:
Botao Wang, Tianjin Medical University, ChinaAntonella Argentiero, National Cancer Institute Foundation (IRCCS), Italy
Paul David, University Hospital Erlangen, Germany
Copyright © 2023 Anu, Shiu and Khan. This is an open-access article distributed under the terms of the Creative Commons Attribution License (CC BY). The use, distribution or reproduction in other forums is permitted, provided the original author(s) and the copyright owner(s) are credited and that the original publication in this journal is cited, in accordance with accepted academic practice. No use, distribution or reproduction is permitted which does not comply with these terms.
*Correspondence: R. I. Anu, ZHJhbnVAYWx1bW5pLmhhcnZhcmQuZWR1