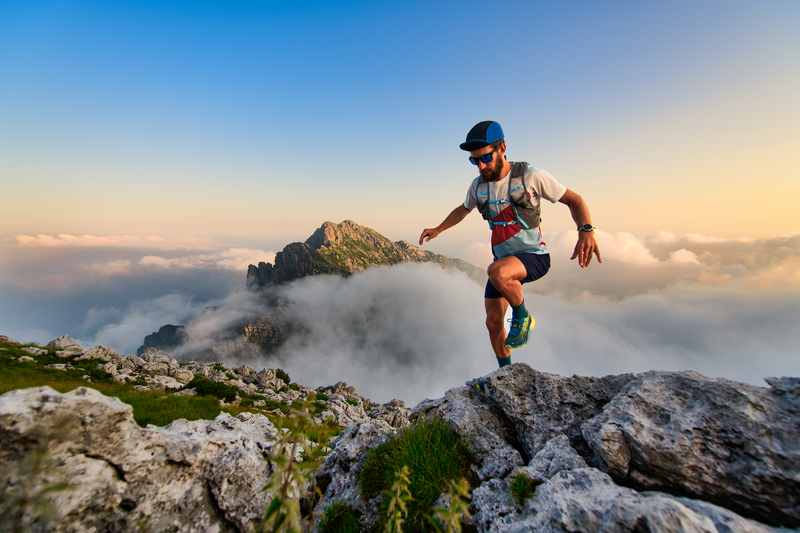
95% of researchers rate our articles as excellent or good
Learn more about the work of our research integrity team to safeguard the quality of each article we publish.
Find out more
REVIEW article
Front. Oncol. , 02 May 2023
Sec. Cancer Metabolism
Volume 13 - 2023 | https://doi.org/10.3389/fonc.2023.1123567
This article is part of the Research Topic Unmasking metabolic dependencies for anti-cancer treatment in cancer View all 11 articles
Cancer-associated cachexia (CAC) is a major characteristic of advanced cancer, associates with almost all types of cancer. Recent studies have found that lipopenia is an important feature of CAC, and it even occurs earlier than sarcopenia. Different types of adipose tissue are all important in the process of CAC. In CAC patients, the catabolism of white adipose tissue (WAT) is increased, leading to an increase in circulating free fatty acids (FFAs), resulting in “ lipotoxic”. At the same time, WAT also is induced by a variety of mechanisms, browning into brown adipose tissue (BAT). BAT is activated in CAC and greatly increases energy expenditure in patients. In addition, the production of lipid is reduced in CAC, and the cross-talk between adipose tissue and other systems, such as muscle tissue and immune system, also aggravates the progression of CAC. The treatment of CAC is still a vital clinical problem, and the abnormal lipid metabolism in CAC provides a new way for the treatment of CAC. In this article, we will review the mechanism of metabolic abnormalities of adipose tissue in CAC and its role in treatment.
Cancer-associated cachexia (CAC) is a “multifactorial syndrome” characterized by increased catabolism, weight loss, and decreased skeletal muscle mass and strength (with or without adipose tissue loss) (1). CAC associates with almost all types of cancer and accounts for a quarter of cancer-related deaths (2). Prevalence of cachexia ranges 50 to 80% in advanced cancer (3). CAC is a continuum with three stages of clinical relevance: precachexia, cachexia, and refractory cachexia. Patients who have more than 5% loss of stable body weight over the past 6 months, or a body-mass index (BMI) less than 20 kg/m² and ongoing weight loss of more than 2%, or sarcopenia and ongoing weight loss of more than 2%, but have not entered the refractory stage, are classified as having cachexia (1). Early CAC can also occur in patients with curable cancer and can be reversed by appropriate treatment (4). However, we often do not diagnose CAC in cancer patients until weight loss has occurred, and there are still few methods for early diagnosis of CAC.
CAC is due to the negative energy balance metabolic changes caused by higher energy demand from the tumor and reduced calorie intake of the host, including inflammation, increased catabolism and excessive energy consumption (5–7). It can lead to multi-organ functional disturbance, which is associated with increased susceptibility to infection, higher incidence of metastasis, decreased response of cancer cells to treatment, decreased quality of life, and poor prognosis (1, 8–11). CAC is affected by endogenous and environmental factors, such as complications, genetic risk factors, gender, age, and anti-tumor treatment (12–14). It has been pointed out that tumor or host derived cytokines can affect the metabolism of CAC (15). Cachexia mainly damages skeletal muscle, adipose tissue, liver, brain, intestine, pancreas, bone, and heart (16). The metabolic disorders can further aggravate this multifactorial syndrome (17).
There are many changes in adipose tissue metabolism in cancer patients. Increasing evidence demonstrates that adipose wasting occurs before muscle loss in the early stage of CAC (18). No matter what the patient’s weight is, fat loss is an adverse prognostic factor for terminal cancer (19). Studies have shown that the changes of adipose tissue morphology and function in CAC patients have important clinical significance, preserving adipose mass and correcting abnormal lipid metabolism in CAC represents a promising therapeutic strategy (20).
However, the knowledge about mechanisms of abnormal lipid metabolism in CAC is still limited. In this review, we summarized the classification and characteristics of adipose tissue and the changes of them in patients with CAC. Finally, we described existing therapeutic approaches and discussed potential new strategies that arose by targeting the link between adipose tissue and cachexia, with a view to providing directions for future clinical treatment of CAC.
Adipose tissue is a large, interactive multi-chamber organ with clear histological and anatomical structure (21). Mature adipocytes account for only one-third of adipose tissue, and the remaining two-thirds of adipose tissue are composed of nerves, blood vessels, fibroblasts, and adipocyte precursor cells (22). It was found that adipose tissue not only played multiple and complex roles in mechanical buffering and energy storage, but also had paracrine and endocrine functions as an important secretory organ (23, 24). It can regulate energy balance and homeostasis in vivo from many aspects, including appetite, inflammation, insulin sensitivity, and lipid metabolism (25). Nowadays, increasing evidence shows that there are changes in lipid metabolism in patients with CAC.
According to its distribution, adipose tissue can be divided into subcutaneous adipose tissue (SCAT) and visceral adipose tissue (VAT), which have different anatomical, metabolic and endocrine characteristics. SCAT accounts for about 80% of total body fat in healthy adults (26). SCAT can be further divided into superficial SCAT and deep SCAT (27). VAT is mainly distributed in the abdominal cavity and retroperitoneum. The metabolic functions of VAT and SCAT are quite different. For example, compared with SCAT, visceral adipocytes have more active metabolism and greater lipolysis activity. Adipocytes of VAT have stronger insulin resistance than those of SCAT (28, 29). At the same time, SCAT is the main source of leptin production (26). Excess energy accumulates in adipocytes of SCAT, which acts as a metabolic pool. Visceral fat accumulation occurs only when SCAT capacity is insufficient or damaged.
According to functional characteristics, adipose tissue can be divided into three types: white adipose tissue (WAT), brown adipose tissue (BAT), or beige adipose tissue.
WAT is the most common type. White adipocytes are the main storage space of triglyceride. The main function of white adipocytes is to store fat and regulate free fatty acids (FFAs). It is mainly composed of large spherical adipocytes, in which single lipid droplets occupy the majority of the cell volume and mainly store energy in the form of triglycerides (21). WAT exists in subcutaneous and visceral tissues, and the increase of WAT quality in viscera is associated with increased metabolic risk (27, 30). WAT has important endocrine and paracrine effects (21, 31, 32).
Unlike WAT, brown adipocytes in BAT contain a large number of mitochondria and scattered lipid droplets. The main function of BAT is energy dissipation, which provides non-shivering thermogenesis to the body during energy-demanding conditions such as exercise, fasting or cold stimulus (33). BAT is mainly located in the interscapular region and perirenal regions of rodents and infants (34, 35), but BAT is normal component of several subcutaneous and visceral depots and is not exclusive to these areas (21). The development and gene characteristics of WAT and BAT are different (36–38). Classic brown adipocytes come from myogenic factor 5 (Myf-5) cell lines, while white adipocytes come from non Myf-5 cell lines (39). Thus, brown adipocytes are labeled with Myf-5 (40) and paired box 7 (41), similar to myogenic precursor cells. BAT contains rich vascular tree and dense capillary network (25). BAT consumes energy in the form of heat production (42–44), which is mainly due to the high level expression of uncoupling protein 1 (UCP1) in mitochondria and its proton leakage pathway (45–47), which is vital to lipid oxidation and thermogenesis. BAT, as an endocrine organ, regulates energy homeostasis by consuming fatty acids and glucose, and plays a key role in carbohydrate and lipid metabolism (47, 48).
Thirdly, there is a type of adipocyte, defined as “Brite” (white brown) (49) or “Beige” (50), which is derived from pre-existing white adipocytes (21). Beige adipocytes have plasticity and can be transformed from WAT by a variety of different pathways (21, 51, 52). The function of beige adipocytes is similar to that of brown adipocytes (49). In patients with CAC, beige adipocytes can develop, expand and activate under multiple environmental stimulation, which is the target of endocrine and paracrine stimulation (53, 54). The formation of beige adipocytes can be triggered by inflammatory mediators (such as interleukin-6 [IL-6] (55)) and tumor-derived compounds (such as parathyroid hormone related protein [PTHrP] (56, 57)). In mouse models, the formation of most beige adipocytes is a strong response to environmental factors, such as long-term low-temperature exposure (35).
The genetic characteristics of brown adipocytes and beige adipocytes partially overlap, except for specific markers, such as zinc finger (Zic1) in cerebellum (58). The common characteristics of brown and beige adipocytes are a large number of lipid droplets and dense accumulation of UCP1 positive mitochondria, although brown adipocytes had higher UCP1 expression level (39). Although brown and beige adipocytes are similar in morphology and biochemistry, they also have some distinct characteristics (35), because they come from different embryonic precursor cells (40). For example, brown adipocytes are mainly located in the interscapular and perirenal regions of rodents, while beige adipocytes exist in various WAT pools, especially in inguinal subcutaneous adipose tissue (59).
All three types of adipose tissue are important in the energy balance disrupted by CAC. WAT and BAT usually have opposite physiological functions. WAT is responsible for energy accumulation of lipid droplets in cells, while BAT is responsible for energy dissipation through heat production. Clinically, browning of WAT and activation of BAT are effective methods to combat obesity and metabolic syndrome, but in CAC we may need to block these mechanisms in order to preserve more adipose tissue. Changes in lipid metabolism under local or systemic stimulation make it a potential cause of CAC. In the case of congenital or acquired lipodystrophy, cachexia or any other severe malnutrition, there is almost total lack of adipose tissue, even severe multiple organ dysfunction results from the lack of leptin and other adipokines.
Lipid metabolism and adipose tissue mass are regulated by two pathways: lipolysis and lipogenesis. Lipolysis and lipogenesis balance maintain the dynamic balance of adipocytes and regulate the energy balance of CAC patients. Adipose tissue atrophy in cancer patients is attributed to increased lipolysis and lipid oxidation, decreased lipogenesis, impaired fat deposition and lipogenesis, and browning of WAT (60). Compared with non-cancer patients, the volume of adipocytes in cancer patients was smaller, but the total number of adipocytes did not change. Adipocytes isolated from patients with cachexia showed stronger catecholamine and natriuretic peptide-induced lipolysis (61). Weight loss patients also showed more sensitive characteristics to catecholamine signal (62). In addition, compared with cancer patients without cachexia, the expression of UCP1 in adipose tissue of CAC patients is higher, which may lead to adipose tissue atrophy and more heat production (55).
Patients with CAC often show systemic hypermetabolism with reduced energy intake and increased energy consumption, especially the abnormal increase of resting energy expenditure is considered to be the main cause of energy consumption. CAC, which is characterized by adipose tissue loss (60), is the terminal manifestation of metabolic changes in adipose tissue. The metabolic changes of adipose tissue have been proved to play an important role in CAC. In the context of CAC, changes in lipid metabolism and energy consumption have been shown to be harmful (16). In patients with terminal cancer, reduced adipose tissue is associated with poor prognosis (18, 19). In cachexia, fat loss is faster and earlier than lean tissue loss, especially in the period before death.
Adipose tissue contributes to weight loss in starvation, while skeletal muscle and adipose tissue mass decrease significantly in CAC patients. Lipolysis of adipocytes is the main cause of adipose tissue loss in cancer patients, and plays an important role in the course of CAC, and it is not related to malnutrition (63). The mechanism of adipose tissue loss in CAC is believed to be due to increased lipolytic activity and lipid utilization (64), while other mechanisms, such as impaired lipogenesis, may also be one of the reasons (Figure 1). In the experimental model of cachexia, the decrease of adipose tissue appeared before the decrease of skeletal muscle mass and food intake (65). Studies have also shown that the decrease in adipose tissue is due to a significant decrease in adipocyte size resulting from a decrease in fat reserve, rather than a decrease in the number of cells (cell death) (64, 66). Some studies have found that lipogenesis and lipoprotein lipase (LPL) expression and activity have not been significantly down regulated in CAC patients (67, 68). A study identified the marker components of “cachectin” including Ataxin-10, which are sufficient to trigger abnormal fatty acid metabolism and cardiac atrophy. The serum level of Ataxin-10 is significantly increased in CAC patients (69).
Figure 1 The mechanism of adipose tissue loss in CAC. The mechanism of adipose tissue loss in CAC is complicated, and these mechanisms can be roughly divided into increased lipolysis, browning of WAT, increased thermogenesis of BAT, and decreased lipogenesis. Cancer cells produce a variety of cytokines to promote adipose tissue loss, such as IL-6, TNF-α, PTHrP, etc. These cytokines not only promote lipolysis, but also promote WAT browning and upregulation of UCP1 in BAT, ultimately leading to an increase in thermogenesis and energy consumption. At the same time, the crosstalk between adipocyte and immune cells and nervous system also plays an important role in abnormal lipid metabolism of CAC. The loss of adipose tissue has a chain reaction that can produce hyperlipidemia and promote skeletal muscle atrophy, making CAC patients’ condition worse. CAC, cancer-associated cachexia; WAT, white adipose tissue; BAT, brown adipose tissue; FFAs, free fat acids; ATGL, adipose triglyceride lipase; HSL, hormone-sensitive lipase; MGL, monoglyceride lipase; LPL, lipoprotein lipase; FAS, fatty acid synthase; UCP1, uncoupling protein 1; β3-AR, adrenoceptor beta 3; ADP, adenosine diphosphate; ATP, adenosine triphosphate; IL-6, interleukin-6; TNF-α, tumor necrosis factor α; PTHrP, parathyroid hormone related protein; ZAG, zinc-α 2-glycoprotein; PLF-1, proliferin-1; GDF15, growth differentiation factor 15; CIDEA, DNA fragmentation factor like effector A; AMPK, adenosine 5’-monophosphate (AMP)-dependent protein kinase; COX-2, cyclooxygenase 2; GLUT-4, glucose transporter 4.
The reduction of WAT in visceral and subcutaneous tissues plays an important role in CAC (60). High lipolysis is an important feature of cachexia in cancer patients and rodents (70, 71). Lipolysis depends on three kinds of lipases: adipose triglyceride lipase (ATGL), hormone-sensitive lipase (HSL) and monoglyceride lipase (MGL). Increased expression and activity of these three enzymes lead to the decrease of adipose tissue and the increase of circulating FFAs and glycerol (68, 72).
In CAC patients, the high levels of circulating FFAs and glycerin is caused by the involvement of ATGL and HSL in the catabolism of triglyceride in WAT (73). Excess free lipid molecules are “lipotoxic”, leading to cellular dysfunction and even death, insulin resistance in animals and humans, and have side effects on many organs (16, 74, 75). Compared with cancer patients with stable weight, the expression of HSL was increased in patients with CAC (61, 76). A large amount of evidence shows that the overexpression of ATGL and HSL in WAT of CAC patients is related to the decrease of BMI (73). Inhibition of HSL or ATGL expression in mice not only retained WAT, but also reduced the loss of skeletal muscle, which indicated that mutual regulation between adipose tissue and muscle play an important role in CAC (71, 73). CGI-58 can bind and activate ATGL (ATGL is the only lipase activated by CGI-58) (77). G0S2 (G0/S2 switch gene 2) is considered to be the main selective inhibitor of ATGL, which can weaken the effect of ATGL in vitro and in vivo, and regulate triglyceride hydrolysis through this mechanism (78).
In addition, patients with cachexia often have insulin resistance or decreased insulin secretion (79), which may be due to the fact that the body prevents insulin from playing its anti-lipolysis role in CAC. Transcriptome analysis of adipose tissue from patients with gastrointestinal cancer cachexia showed that the expression of related pathways regulating energy conversion was up-regulated (64). Both tumor cells and host immune cells (such as macrophages and lymphocytes) release cytokines or hormones, such as IL-6, tumor necrosis factor α (TNF-α), zinc-α 2-glycoprotein (ZAG, also known as lipid mobilization factor), proliferin-1, catecholamines, and natriuretic peptide, which can promote lipolysis and reduce insulin sensitivity in CAC patients (6, 73, 80, 81). They are involved in the regulation of proinflammatory state, stress response, anorexia, disease behavior, hypermetabolism and accelerated decomposition of protein, muscle and adipose tissue in CAC patients (82). Systemic inflammation and changes in the human immune system are important determinants of this state (83, 84), which suggest that inflammatory cytokines may be biomarkers for the diagnosis of CAC (71).
In mammals, adaptive thermogenesis occurs mainly in brown and beige adipocytes (35). The increase of energy consumption can also be explained by the increased heat production and “browning” of WAT (55, 56). WAT browning refers to the transformation from WAT to BAT, and its name comes from the dark color associated with mitochondria. The gradual transformation of adipose tissue types is an interesting feature of CAC.
This process promotes mitochondrial respiration, leading to thermogenesis rather than ATP synthesis, thus activating lipid mobilization and increasing energy consumption (85). Beige adipocytes, which are phenotypically different from those in WAT and BAT, can appear under severe cold exposure (86), adrenergic stimulation (87) and prostaglandin synthase (cyclooxygenase 2, COX-2) (88). These cells can significantly promote total energy consumption and lead to fat loss (89). Obesity related studies have shown that the central nervous system, especially the hypothalamus, is an important regulatory organ for browning (87). Klaus Felix et al. found that the level of glucagon-like peptide-1 (GLP-1) was increased in pancreatic cancer cachexia patients (90), GLP-1 appears to trigger satiety and inhibit food intake through molecular regulation in the hypothalamus (91). At the molecular level, transcription factors such as peroxisome proliferator activated receptor gamma (PPARγ), PPARγ coactivator 1α (PGC-1α), transcription factor PR domain-containing 16 (PDRM16) and CCAAT enhancer binding protein β (C/EBP-β) regulate the transcription events of differentiation into brown adipocytes (92, 93). This is related to upregulation of UCP1 expression, increased heat production (non-shivering), loss of ATP production and increased energy catabolism (73). WAT atrophy and adipose tissue browning occurred in the early stage of CAC (94). The loss of brown adipocytes may lead to browning of WAT, which indicates that there is a compensatory mechanism between mature brown adipocytes and beige adipocytes (95).
In adipose tissue, proinflammatory factors promote the browning of WAT. With the development of CAC, inflammatory microenvironment and metabolic disorder caused by IL-6, TNF-α and parathyroid related peptide secreted by tumors and host immune system will promote browning of WAT (59, 94). IL-6 promotes systemic metabolism to a certain extent by regulating BAT activation and adipose tissue browning (80). In addition, tumor-derived IL-6 and adrenoceptor beta 3 (β3-AR) activation is associated with CAC mediated adipose tissue browning. Neutralization of IL-6 or β3-AR can significantly improve cachexia (55). The introduction of IL-6 into the brain by adenovirus vector can significantly increase the expression of UCP1 in sympathetic innervated brown adipocytes, but not in denervated brown adipocytes, indicating that IL-6 might activate BAT through β3-AR signaling pathway (96). Although PTHrP treatment did not change tumor size in Lewis mouse lung CAC model, it resulted in CAC related weight loss and skeletal muscle atrophy, and activated beige cells to produce heat. On the contrary, blocking PTHrP with neutralizing antibody can prevent adipose tissue and skeletal muscle atrophy. In addition, PTHrP shares the G-protein coupled receptor signaling pathway with β 3-AR agonists, and upregulates the protein expression level of UCP1 in white and brown adipocytes (56). Therefore, tumor cell-derived IL-6 and PTHrP may play an important role in CAC by activating BAT and/or adipose tissue browning.
Two G-protein coupled receptors, called FFA receptor 1 (FFA1) and FFA receptor 4 (FFA4), were identified as molecular targets for ω-3 polyunsaturated fatty acids (97, 98). When activated, these receptors can promote a variety of effects, such as increasing insulin sensitivity, inducing adipose tissue browning, promoting analgesia by releasing β-endorphin, controlling energy homeostasis, and reducing food intake (99, 100). Activation of FFA4 results in browning of adipose tissue (99, 101). Lewis lung cancer mouse models lacking the adipose tissue specific PRDM16 showed reduced browning, thermogenesis and lipoatrophy (56). By neutralizing browning promoting hormones, such as PTHrP, the improvement of CAC and the reduction of adipose tissue loss were observed in animal models (56). WAT showed heterogeneity in browning efficiency. Some parts such as visceral adipose tissue is resistant to browning. It has been reported that visceral adipose tissue browning may be a compensatory heat production mechanism (102), but its conversion mechanism is still unclear. Hongmei Yang et al. found that exosomes from Lewis lung carcinoma cells can induce lipolysis in vitro and in vivo by delivering PTHrP, and inhibition of exosome generation prevented the fat loss of tumor bearing mice (103, 104). Furthermore, there have been many studies in recent years confirming that non-coding RNA plays an important role in the browning of WAT. Wenjuan Di et al. found that miR-146b-5p was enriched in cancer-related exosomes, which plays an essential role in WAT browning. miR-146b-5p can directly repress the downstream gene homeodomain‐containing gene C10 (HOXC10), thereby regulating lipolysis (105). Non-coding RNA such as miR-155, miR-425-3p, and miR-182-5p have also been shown to play a role in promoting WAT catabolism and browning in several cancer species (106–108). Further study on the mechanism of systemic metabolic and inflammatory changes leading to the transformation of WAT into BAT can further improve our understanding and treatment of cachexia.
There is a lot of evidence that BAT is activated under different conditions of cachexia. The enhanced heat production of BAT is considered to be one of the main reasons for the increase of resting energy expenditure in cancer patients (72). The activity of BAT was also positively correlated with the stage of cancer (109).
BAT is characterized by high mitochondrial content and increased expression of UCP1. UCP1 regulates body temperature through oxidative phosphorylation of uncoupling ATP, resulting in increased energy consumption, increased heat production, and lipolysis, leading to weight loss and progression of CAC (56, 59, 94). The activation of BAT is mediated by β3-AR, which is activated by the sympathetic nervous system, leading to adipocyte contraction (56). β3-AR was activated and UCP1 expression was increased, which activated the delipidation in BAT (110). Catecholamine signaling in BAT transduction was enhanced in cachexia mice, but blocking the β3-AR by propranolol could prevent the increase of body temperature (111). Catecholamine levels are associated with BAT activity and BMI (112). Moreover, FFAs released by lipolysis are direct activators of UCP1 (33), indicating that enhanced WAT catabolism in CAC will promote BAT activation.
Brown adipose precursor cells expressing early B-cell factor 2 and platelet-derived growth factor receptor α differentiated into mature brown adipocytes (113). The regulation of BAT depends on a variety of cytokines. β1-adrenergic receptor (ADRB1) mediates norepinephrine induced BAT formation (114). The expression of ADRB1 was correlated with the rate of lipolysis in patients with CAC (76), Prep1 is a adipo-osteogenesis regulatory factor, which is related to the increase of BAT density and osteogenesis reduction (115). IL-6 also plays an important role in mediating BAT activation by increasing the expression of UCP1 and activating fatty acid β-oxidation related gene thermogenesis in gastric cancer and colon cancer patients with cachexia (80). With Lewis lung cancer model, it has been proved that tumor-derived PTHrP regulates the expression of genes involved in adipose tissue thermogenesis, lipolytic enzymes and muscle atrophy. These studies suggest that blocking BAT activation may be a way to treat cachexia. However, due to the difficulty of BAT localization in human body, the research progress on BAT is very slow at present.
In the context of CAC, there are communications between adipocytes and a variety of non-adipocytes, which is the key to control energy homeostasis and prevent metabolic diseases. DNA fragmentation factor like effector A (CIDEA) is an important metabolic regulator and apoptosis inducing factor. Adipocyte dysfunction leads to the increase of CIDEA, followed by the degradation of adenosine 5’-monophosphate (AMP)-dependent protein kinase (AMPK) in cachexia adipose tissue (116). By hypothalamic AMPK signal, the secretion of leptin, adiponectin and insulin are controlled (117). When the effect of insulin is enhanced, IL-6 secreted by skeletal muscle will increase. IL-6 acts on muscle contraction also by activating AMPK (117). Meanwhile, IL-6 induces Toll-like receptor-4 gene expression via activation of STAT3, leading to insulin resistance in human skeletal muscle, which further accelerates muscle wasting (118). Lipolysis results in increased FFAs in circulation, and FFAs will eventually enter skeletal muscle and cause muscle atrophy (91). Blocking fatty acid oxidation not only rescued human myotubes, but also improved muscle mass and body weight in CAC models in vivo, which indicates that there may be interaction between adipose tissue and skeletal muscle (119). Rowena Suriben’s study has indicated that growth differentiation factor 15 (GDF15) elicits a lipolytic response in adipose tissue and leads to reduced adipose and muscle mass and function in tumor-bearing mice, inhibiting GDF15-driven lipid mobilization and oxidation can be translated to preservation of skeletal muscle mass (120). GDF15 regulates survival of motor and chipmaker sensory neurons (121), so lipid and skeletal muscle may interact through neurons.
During cachexia, systemic inflammation is one of the main driving forces of fat consumption. Cancer cells secrete a variety of mediators, such as TNF-α, IL-6, IFN-γ, ZAG and PTHrP, which can promote browning (55, 56, 122). TNF-α belongs to cachectin (123), which can be released from adipose tissue and mediates CAC by reducing the expression of glucose transporter 4 (GLUT4), which in turn inhibits glucose transport and adipogenesis (124). In addition, adipose tissue is closely related to inflammatory cells, the cross-talk between immune response and adipose tissue biology has been proved. Macrophages can infiltrate WAT and activate immune responses in cachexia mice. A study has shown that macrophages can regulate WAT browning through paracrine heat shock protein A12A (125). Recently, Hao Xie et al. demonstrated that the immune-sympathetic neutron communication axis is essential for WAT browning in CAC. IL-6 and PTHrP can activate immune cells, including macrophages. Then, the type 2 macrophages will produce neurotrophins and increase polyamine synthesis and secretion. This increases neuronal activity, leading to enhanced local catecholamine synthesis, β-adrenergic stimulation of WAT, and WAT browning (126). Not only that, virus-specific CD8(+) T cells caused morphologic and molecular changes in adipose tissue, which may also lead to cachexia (127). Another study implicates adipocytes as predominantly negative regulators of the surrounding myeloid cells (128). At the same time, studies have shown that the imbalance of the immune system affects the gut microbiota (129), which also plays an important role in CAC. One clinical study has shown that compared with non-cachexic people, Proteobacteria, an unknown genus from the Enterobacteriaceae family, and Veillonella were more abundant among CAC patients (130). Currently, the crosstalk between adipose tissue and other tissues is attracting more and more attention, and the study of these mechanisms may provide us with a more holistic understanding of CAC, so as to develop diagnosis and treatment strategies.
The decrease of fat mass in cancer patients depends on the decrease of lipid deposition and lipogenesis. In the process of lipogenesis in adipose tissue, some fatty acids will be re-esterified to triglycerides, forming a futile cycle, which is mediated by AMPK pathway, and the activity of AMPK pathway in cachexia adipose tissue is decreased (116). The activities of fatty acid synthase (FAS) and LPL in adipose tissue of cancer patients were decreased (131). A large number of animal studies have also shown that the activity of LPL is reduced in cancer (132). LPL hydrolyses free circulating triacylglycerol present in chylomicrons very-low-density lipoproteins (VLDLs), whose decreased activity is associated with increased IL-6 (133). Compared with cancer patients with stable weight, patients with CAC have more adipose tissue oxidation (134). Mice-bearing colon adenocarcinoma showed an increase in LPL activity in the heart and adipose tissue increasing weight loss but decreasing with further weight loss. It is suggested that the initial rise in LPL activity provides more oxidation of fatty acids in cachexia state (7, 135). These evidences suggest that the dysfunction of LPL is related to the occurrence and development of CAC, and testing the activity of LPL may be useful for the diagnosis of cachexia. In the experimental model of CAC, the process of lipogenesis was weakened and the expression of lipogenic transcription factors was decreased, which was related to the decrease of adipocyte size and the higher expression of TNF-α (136–138). Besides, non-coding RNA also affects lipogenesis, Diya Sun et al. found that the expression of miR-410-3p was higher in subcutaneous adipose tissues and serum exosomes of CAC patients, which significantly inhibited adipogenesis and lipid accumulation (139). Additionally, due to anorexia associated with CAC or the difficulty of eating due to cancer, the intake of lipids and other nutrients will be reduced, which will also lead to the reduction of lipogenesis.
However, the research progress in the treatment of CAC and the improvement of patients’ prognosis is relatively slow, there is also no definite treatment plan for the abnormal lipid metabolism of CAC patients. Studies have shown that CAC is often reversible if intervention is carried out in pre-cachexia or cachexia stage (10, 140). The main treatment strategies used in these stages include exercise and nutritional support, as well as the elimination of any direct cause of cancer (such as reduced food intake or malnutrition due to obstruction or compression) (140, 141). Unfortunately, many cases have been diagnosed as refractory cachexia, when CAC is usually irreversible (1, 140). At present, the main researches focus on the treatment of CAC. Several treatment options have been proposed, but have not been clinically confirmed. The treatment combination mainly involves two pathways: the anabolic pathway and the antimetabolic pathway for muscle and fat catabolism (82). Neutralization of metabolic changes is the first task to overcome cachexia, including the control of skeletal muscle protein decomposition rate, as well as the control of liver, lipid and carbohydrate protein metabolism abnormalities.
A retrospective study by Dingemans et al. included 12 phase II clinical trials involving 11 compounds. These drugs fight CAC through one of the following mechanisms: increase appetite, improve digestion, reduce systemic inflammation, and increase the ratio of muscle synthesis and degradation (142). Many other drugs have entered phase III trials, but it is difficult to achieve multiple clinical endpoints at the same time. Anamorelin, an auxin receptor agonist, has demonstrated its ability to improve lean weight in phase II clinical trials in patients with non-small cell lung cancer and CAC (143). However, the results of this phase II trial and subsequent phase III trial showed that although lean weight was improved, grip strength did not improve (143, 144), which was rejected by the European Drug Administration in 2017. Enobosarm, a selective androgen receptor regulator, showed a significant increase in total lean body weight in the phase II study (145), but it did not produce consistent end-point results in the phase III trial (146, 147). There are many influencing factors in CAC. Current studies cannot fully elucidate the pathophysiological mechanisms of CAC, so as to effectively reduce or reverse all clinical factors of CAC. Therefore, even if the development of many drugs is moving in the right direction, few of them can pass phase II and III studies.
As we mentioned above, lipopenia is an important feature of CAC, and several studies have suggested potential therapeutic strategies for lipid metabolism in CAC, which are summarized in Table 1. Our summary shows that although so many potential strategies have been identified, they are still far from clinical practice. Based on the available evidence, nutritional strategies such as supplementing patients with unsaturated fatty acids and marine phospholipids may be effective (7, 164). Some chemotherapeutic agents, such as cytarabine, promote fat depletion, and we can intervene early in the treatment of patients using these chemotherapeutic agents (165). Exercise training may also reduce inflammation and improve the condition of muscle and adipose tissue in CAC patients (166). What’s more, the application of some new technologies may be helpful to this field. The appearance of 3D bioprinted WAT model is helpful for us to further study and understand the mechanism of adipose tissue metabolism in cachexia (167). Portable biosensors can make it easier for us to monitor lipolysis in patients (168). However, these strategies still need more clinical data to support, the treatment of CAC is still a major challenge for clinicians.
CAC is a chronic disease involving multiple organs and tissues, which requires multi-mode treatment, including drug treatment, nutritional support and physical exercise, so as to better adapt to the complex mechanisms of body consumption. While enhancing the balance of anabolism/catabolism, it can improve the physical condition and improve the quality of life (169, 170). However, the ideal drugs against CAC are still under development.
Studies have shown that adipose tissue is involved in the formation of CAC, and abnormal lipid metabolism plays an important role in the development of CAC (Figure 2). In adipose tissue, WAT guides the system energy production through the balance between lipogenesis and lipolysis. BAT has been found to have important physiological and pathological functions in adults. Browning stimulates the differentiation and thermogenesis of beige adipocytes. These adipose tissues contribute differently to CAC. In the development of CAC, adipose tissue interacts with other cells or organs, showing therapeutic potential. Since abnormal lipid metabolism occurs at the early stage of CAC, correcting abnormal lipid metabolism and appropriately increasing adipose tissue may delay or even prevent the further deterioration of CAC. Therefore, it is also very important to explore related biomarkers to monitor lipid metabolism in cancer patients. In this field, we need to continue to pay attention to and think about the following questions: (1) At present, there is a lack of clinical monitoring indicators of lipid metabolism, and it is difficult for us to identify abnormal fat loss in cancer patients at an early stage. At the same time, the specific changes of each fat depot during fat loss are still not clear. (2) Some studies have shown that appropriate diet control and exercise can increase muscle strength and promote metabolic health in cancer patients. However, there is still a lack of relevant research conclusions on whether these measures are safe for patients with CAC and whether these interventions should be implemented in patients with CAC. (3) The related side effects of anti-tumor therapy may also promote the development of CAC. The effects of these therapies, including chemotherapy, radiotherapy, immunotherapy and targeted therapy, on lipid metabolism remain unclear. (4) Current animal models of CAC may not adequately simulate the actual physiological changes of CAC, many mechanistic details of abnormal lipid metabolism in CAC need to be further explored, and the interaction between different types of cancers and adipose tissue may also be different.
In this review, we systematically summarize the currently known mechanisms related to abnormal lipid metabolism in CAC. Although we have summarized a number of potential treatments for abnormal lipid metabolism in CAC in this review, most of them still require further drug development and clinical validation. CAC is still an unavoidable problem for oncologists. Through this review of abnormal lipid metabolism in CAC, we hope that drugs targeting abnormal lipid metabolism in CAC can be developed in the future, so as to effectively treat CAC and improve the prognosis of patients with CAC.
Abnormal lipid metabolism in CAC can be summarized as increased catabolism and decreased synthesis of white adipose tissue, increased browning of adipose tissue and abnormal activation of brown adipose tissue, and crosstalk between adipose tissue and non-adipose tissue further promotes adipose loss. There are a number of treatments available to prevent lipopenia, such as exercise, appetite promotion, caloric and nutrient supplementation by oral or parenteral nutrition, and NSAIDs and other drugs that may be beneficial to fat preservation, the specific items are listed in Table 1. In clinical practice, these strategies are often used in combination to prevent the progression of CAC, and drugs targeting the above abnormal lipid metabolism need to be developed and explored in the future.
RF: Writing—Original Draft Preparation; LY, ZL: Supervision and writing — Review and Editing. All authors contributed to the article and approved the submitted version.
This work was supported by grants from the National Natural Science Foundation of China [grant numbers 81870299], Guiding Fund of Renmin Hospital of Wuhan University [grant numbers RMYD2018M39].
The authors declare that the research was conducted in the absence of any commercial or financial relationships that could be construed as a potential conflict of interest.
All claims expressed in this article are solely those of the authors and do not necessarily represent those of their affiliated organizations, or those of the publisher, the editors and the reviewers. Any product that may be evaluated in this article, or claim that may be made by its manufacturer, is not guaranteed or endorsed by the publisher.
1. Fearon K, Strasser F, Anker SD, Bosaeus I, Bruera E, Fainsinger RL, et al. Definition and classification of cancer cachexia: an international consensus. Lancet Oncol (2011) 12:489–95. doi: 10.1016/S1470-2045(10)70218-7
2. Laviano A, Meguid MM, Inui A, Muscaritoli M, Rossi-Fanelli F. Therapy insight: cancer anorexia-cachexia syndrome–when all you can eat is yourself. Nat Clin Pract Oncol (2005) 2:158–65. doi: 10.1038/ncponc0112
3. von Haehling S, Anker SD. Prevalence, incidence and clinical impact of cachexia: facts and numbers-update 2014. J Cachexia Sarcopenia Muscle (2014) 5:261–3. doi: 10.1007/s13539-014-0164-8
4. Arends J, Bachmann P, Baracos V, Barthelemy N, Bertz H, Bozzetti F, et al. ESPEN guidelines on nutrition in cancer patients. Clin Nutr (2017) 36:11–48. doi: 10.1016/j.clnu.2016.07.015
5. Baracos VE, Martin L, Korc M, Guttridge DC, Fearon KCH. Cancer-associated cachexia. Nat Rev Dis Primers (2018) 4:17105. doi: 10.1038/nrdp.2017.105
6. Petruzzelli M, Wagner EF. Mechanisms of metabolic dysfunction in cancer-associated cachexia. Genes Dev (2016) 30:489–501. doi: 10.1101/gad.276733.115
7. Joshi M, Patel BM. The burning furnace: alteration in lipid metabolism in cancer-associated cachexia. Mol Cell Biochem (2022) 477:1709–23. doi: 10.1007/s11010-022-04398-0
8. Prado CM, Bekaii-Saab T, Doyle LA, Shrestha S, Ghosh S, Baracos VE, et al. Skeletal muscle anabolism is a side effect of therapy with the MEK inhibitor: selumetinib in patients with cholangiocarcinoma. Br J Cancer (2012) 106:1583–6. doi: 10.1038/bjc.2012.144
9. Prado CM, Sawyer MB, Ghosh S, Lieffers JR, Esfandiari N, Antoun S, et al. Central tenet of cancer cachexia therapy: do patients with advanced cancer have exploitable anabolic potential? Am J Clin Nutr (2013) 98:1012–9. doi: 10.3945/ajcn.113.060228
10. Fearon K, Arends J, Baracos V. Understanding the mechanisms and treatment options in cancer cachexia. Nat Rev Clin Oncol (2013) 10:90–9. doi: 10.1038/nrclinonc.2012.209
11. Bachmann J, Ketterer K, Marsch C, Fechtner K, Krakowski-Roosen H, Buchler MW, et al. Pancreatic cancer related cachexia: influence on metabolism and correlation to weight loss and pulmonary function. BMC Cancer (2009) 9:255. doi: 10.1186/1471-2407-9-255
12. Dewys WD, Begg C, Lavin PT, Band PR, Bennett JM, Bertino JR, et al. Prognostic effect of weight loss prior to chemotherapy in cancer patients. Eastern cooperative oncology group. Am J Med (1980) 69:491–7. doi: 10.1016/S0149-2918(05)80001-3
13. Hendifar A, Yang D, Lenz F, Lurje G, Pohl A, Lenz C, et al. Gender disparities in metastatic colorectal cancer survival. Clin Cancer Res (2009) 15:6391–7. doi: 10.1158/1078-0432.CCR-09-0877
14. Baracos VE, Reiman T, Mourtzakis M, Gioulbasanis I, Antoun S. Body composition in patients with non-small cell lung cancer: a contemporary view of cancer cachexia with the use of computed tomography image analysis. Am J Clin Nutr (2010) 91:1133S–7S. doi: 10.3945/ajcn.2010.28608C
15. Tisdale MJ. Molecular pathways leading to cancer cachexia. Physiol (Bethesda) (2005) 20:340–8. doi: 10.1152/physiol.00019.2005
16. Argiles JM, Stemmler B, Lopez-Soriano FJ, Busquets S. Inter-tissue communication in cancer cachexia. Nat Rev Endocrinol (2018) 15:9–20. doi: 10.1038/s41574-018-0123-0
17. Porporato PE. Understanding cachexia as a cancer metabolism syndrome. Oncogenesis (2016) 5:e200. doi: 10.1038/oncsis.2016.3
18. Fouladiun M, Korner U, Bosaeus I, Daneryd P, Hyltander A, Lundholm KG. Body composition and time course changes in regional distribution of fat and lean tissue in unselected cancer patients on palliative care–correlations with food intake, metabolism, exercise capacity, and hormones. Cancer (2005) 103:2189–98. doi: 10.1002/cncr.21013
19. Murphy RA, Wilke MS, Perrine M, Pawlowicz M, Mourtzakis M, Lieffers JR, et al. Loss of adipose tissue and plasma phospholipids: relationship to survival in advanced cancer patients. Clin Nutr (2010) 29:482–7. doi: 10.1016/j.clnu.2009.11.006
20. Kershaw JC, Elzey BD, Guo XX, Kim KH. Piceatannol, a dietary polyphenol, alleviates adipose tissue loss in pre-clinical model of cancer-associated cachexia via lipolysis inhibition. Nutrients (2022) 14:2306. doi: 10.3390/nu14112306
22. Avram AS, Avram MM, James WD. Subcutaneous fat in normal and diseased states: 2. anatomy and physiology of white and brown adipose tissue. J Am Acad Dermatol (2005) 53:671–83. doi: 10.1016/j.jaad.2005.05.015
23. Deng T, Lyon CJ, Bergin S, Caligiuri MA, Hsueh WA. Obesity, inflammation, and cancer. Annu Rev Pathol (2016) 11:421–49. doi: 10.1146/annurev-pathol-012615-044359
24. Dahlman I, Elsen M, Tennagels N, Korn M, Brockmann B, Sell H, et al. Functional annotation of the human fat cell secretome. Arch Physiol Biochem (2012) 118:84–91. doi: 10.3109/13813455.2012.685745
25. Cinti S. The adipose organ. Prostaglandins Leukot Essent Fatty Acids (2005) 73:9–15. doi: 10.1016/j.plefa.2005.04.010
26. Wajchenberg BL. Subcutaneous and visceral adipose tissue: their relation to the metabolic syndrome. Endocr Rev (2000) 21:697–738. doi: 10.1210/edrv.21.6.0415
27. Smith SR, Lovejoy JC, Greenway F, Ryan D, deJonge L, de la Bretonne J, et al. Contributions of total body fat, abdominal subcutaneous adipose tissue compartments, and visceral adipose tissue to the metabolic complications of obesity. Metabolism (2001) 50:425–35. doi: 10.1053/meta.2001.21693
28. Abate N, Garg A, Peshock RM, Stray-Gundersen J, Grundy SM. Relationships of generalized and regional adiposity to insulin sensitivity in men. J Clin Invest (1995) 96:88–98. doi: 10.1172/JCI118083
29. Frayn KN. Visceral fat and insulin resistance–causative or correlative? Br J Nutr (2000) 83 Suppl 1:S71–77. doi: 10.1017/S0007114500000982
30. Macotela Y, Emanuelli B, Mori MA, Gesta S, Schulz TJ, Tseng YH, et al. Intrinsic differences in adipocyte precursor cells from different white fat depots. Diabetes (2012) 61:1691–9. doi: 10.2337/db11-1753
31. Romacho T, Elsen M, Rohrborn D, Eckel J. Adipose tissue and its role in organ crosstalk. Acta Physiol (Oxf) (2014) 210:733–53. doi: 10.1111/apha.12246
32. McGown C, Birerdinc A, Younossi ZM. Adipose tissue as an endocrine organ. Clin Liver Dis (2014) 18:41–58. doi: 10.1016/j.cld.2013.09.012
33. Li Y, Li Z, Ngandiri DA, Llerins Perez M, Wolf A, Wang Y. The molecular brakes of adipose tissue lipolysis. Front Physiol (2022) 13:826314. doi: 10.3389/fphys.2022.826314
34. Rosen ED, Spiegelman BM. What we talk about when we talk about fat. Cell (2014) 156:20–44. doi: 10.1016/j.cell.2013.12.012
35. Ikeda K, Maretich P, Kajimura S. The common and distinct features of brown and beige adipocytes. Trends Endocrinol Metab (2018) 29:191–200. doi: 10.1016/j.tem.2018.01.001
36. Giralt M, Villarroya F. White, brown, beige/brite: different adipose cells for different functions? Endocrinology (2013) 154:2992–3000. doi: 10.1210/en.2013-1403
37. Hilton C, Karpe F, Pinnick KE. Role of developmental transcription factors in white, brown and beige adipose tissues. Biochim Biophys Acta (2015) 1851:686–96. doi: 10.1016/j.bbalip.2015.02.003
38. Ussar S, Lee KY, Dankel SN, Boucher J, Haering MF, Kleinridders A, et al. ASC-1, PAT2, and P2RX5 are cell surface markers for white, beige, and brown adipocytes. Sci Transl Med (2014) 6:247ra103. doi: 10.1126/scitranslmed.3008490
39. Wu J, Bostrom P, Sparks LM, Ye L, Choi JH, Giang AH, et al. Beige adipocytes are a distinct type of thermogenic fat cell in mouse and human. Cell (2012) 150:366–76. doi: 10.1016/j.cell.2012.05.016
40. Seale P, Bjork B, Yang W, Kajimura S, Chin S, Kuang S, et al. PRDM16 controls a brown fat/skeletal muscle switch. Nature (2008) 454:961–7. doi: 10.1038/nature07182
41. Lepper C, Fan CM. Inducible lineage tracing of Pax7-descendant cells reveals embryonic origin of adult satellite cells. Genesis (2010) 48:424–36. doi: 10.1002/dvg.20630
42. Schulz TJ, Tseng YH. Emerging role of bone morphogenetic proteins in adipogenesis and energy metabolism. Cytokine Growth Factor Rev (2009) 20:523–31. doi: 10.1016/j.cytogfr.2009.10.019
43. Nicholls DG, Rial E. A history of the first uncoupling protein, UCP1. J Bioenerg Biomembr (1999) 31:399–406. doi: 10.1023/A:1005436121005
44. Klingenberg M, Huang SG. Structure and function of the uncoupling protein from brown adipose tissue. Biochim Biophys Acta (1999) 1415:271–96. doi: 10.1016/S0005-2736(98)00232-6
45. Nicholls DG. The bioenergetics of brown adipose tissue mitochondria. FEBS Lett (1976) 61:103–10. doi: 10.1016/0014-5793(76)81014-9
46. Lean ME. Brown adipose tissue in humans. Proc Nutr Soc (1989) 48:243–56. doi: 10.1079/PNS19890036
47. Townsend KL, Tseng YH. Brown fat fuel utilization and thermogenesis. Trends Endocrinol Metab (2014) 25:168–77. doi: 10.1016/j.tem.2013.12.004
48. Cypess AM, Lehman S, Williams G, Tal I, Rodman D, Goldfine AB, et al. Identification and importance of brown adipose tissue in adult humans. N Engl J Med (2009) 360:1509–17. doi: 10.1056/NEJMoa0810780
49. Petrovic N, Walden TB, Shabalina IG, Timmons JA, Cannon B, Nedergaard J. Chronic peroxisome proliferator-activated receptor gamma (PPARgamma) activation of epididymally derived white adipocyte cultures reveals a population of thermogenically competent, UCP1-containing adipocytes molecularly distinct from classic brown adipocytes. J Biol Chem (2010) 285:7153–64. doi: 10.1074/jbc.M109.053942
50. Ishibashi J, Seale P. Medicine. Beige Can be slimming. Sci (2010) 328:1113–4. doi: 10.1126/science.1190816
51. Rosen ED, Spiegelman BM. Adipocytes as regulators of energy balance and glucose homeostasis. Nature (2006) 444:847–53. doi: 10.1038/nature05483
52. Vitali A, Murano I, Zingaretti MC, Frontini A, Ricquier D, Cinti S. The adipose organ of obesity-prone C57BL/6J mice is composed of mixed white and brown adipocytes. J Lipid Res (2012) 53:619–29. doi: 10.1194/jlr.M018846
53. Celi FS, Le TN, Ni B. Physiology and relevance of human adaptive thermogenesis response. Trends Endocrinol Metab (2015) 26:238–47. doi: 10.1016/j.tem.2015.03.003
54. Boström P, Wu J, Jedrychowski MP, Korde A, Ye L, Lo JC, et al. A PGC1-α-dependent myokine that drives brown-fat-like development of white fat and thermogenesis. Nature (2012) 481:463–8. doi: 10.1038/nature10777
55. Petruzzelli M, Schweiger M, Schreiber R, Campos-Olivas R, Tsoli M, Allen J, et al. A switch from white to brown fat increases energy expenditure in cancer-associated cachexia. Cell Metab (2014) 20:433–47. doi: 10.1016/j.cmet.2014.06.011
56. Kir S, White JP, Kleiner S, Kazak L, Cohen P, Baracos VE, et al. Tumour-derived PTH-related protein triggers adipose tissue browning and cancer cachexia. Nature (2014) 513:100–4. doi: 10.1038/nature13528
57. Kir S, Komaba H, Garcia AP, Economopoulos KP, Liu W, Lanske B, et al. PTH/PTHrP receptor mediates cachexia in models of kidney failure and cancer. Cell Metab (2016) 23:315–23. doi: 10.1016/j.cmet.2015.11.003
58. Walden TB, Hansen IR, Timmons JA, Cannon B, Nedergaard J. Recruited vs. nonrecruited molecular signatures of brown, "brite," and white adipose tissues. Am J Physiol Endocrinol Metab (2012) 302:E19–31. doi: 10.1152/ajpendo.00249.2011
59. Kir S, Spiegelman BM. Cachexia & brown fat: a burning issue in cancer. Trends Cancer (2016) 2:461–3. doi: 10.1016/j.trecan.2016.07.005
60. Ebadi M, Mazurak VC. Evidence and mechanisms of fat depletion in cancer. Nutrients (2014) 6:5280–97. doi: 10.3390/nu6115280
61. Agustsson T, Ryden M, Hoffstedt J, van Harmelen V, Dicker A, Laurencikiene J, et al. Mechanism of increased lipolysis in cancer cachexia. Cancer Res (2007) 67:5531–7. doi: 10.1158/0008-5472.CAN-06-4585
62. Drott C, Persson H, Lundholm K. Cardiovascular and metabolic response to adrenaline infusion in weight-losing patients with and without cancer. Clin Physiol (1989) 9:427–39. doi: 10.1111/j.1475-097X.1989.tb00997.x
63. Ryden M, Agustsson T, Laurencikiene J, Britton T, Sjolin E, Isaksson B, et al. Lipolysis–not inflammation, cell death, or lipogenesis–is involved in adipose tissue loss in cancer cachexia. Cancer (2008) 113:1695–704. doi: 10.1002/cncr.23802
64. Dahlman I, Mejhert N, Linder K, Agustsson T, Mutch DM, Kulyte A, et al. Adipose tissue pathways involved in weight loss of cancer cachexia. Br J Cancer (2010) 102:1541–8. doi: 10.1038/sj.bjc.6605665
65. Byerley LO, Lee SH, Redmann S, Culberson C, Clemens M, Lively MO. Evidence for a novel serum factor distinct from zinc alpha-2 glycoprotein that promotes body fat loss early in the development of cachexia. Nutr Cancer (2010) 62:484–94. doi: 10.1080/01635580903441220
66. Mracek T, Stephens NA, Gao D, Bao Y, Ross JA, Ryden M, et al. Enhanced ZAG production by subcutaneous adipose tissue is linked to weight loss in gastrointestinal cancer patients. Br J Cancer (2011) 104:441–7. doi: 10.1038/sj.bjc.6606083
67. Zechner R, Zimmermann R, Eichmann TO, Kohlwein SD, Haemmerle G, Lass A, et al. FAT SIGNALS–lipases and lipolysis in lipid metabolism and signaling. Cell Metab (2012) 15:279–91. doi: 10.1016/j.cmet.2011.12.018
68. Arner P, Langin D. Lipolysis in lipid turnover, cancer cachexia, and obesity-induced insulin resistance. Trends Endocrinol Metab (2014) 25:255–62. doi: 10.1016/j.tem.2014.03.002
69. Schäfer M, Oeing CU, Rohm M, Baysal-Temel E, Lehmann LH, Bauer R, et al. Ataxin-10 is part of a cachexokine cocktail triggering cardiac metabolic dysfunction in cancer cachexia. Mol Metab (2016) 5:67–78. doi: 10.1016/j.molmet.2015.11.004
70. Zuijdgeest-van Leeuwen SD, van den Berg JW, Wattimena JL, van der Gaast A, Swart GR, Wilson JH, et al. Lipolysis and lipid oxidation in weight-losing cancer patients and healthy subjects. Metabolism (2000) 49:931–6. doi: 10.1053/meta.2000.6740
71. Das SK, Eder S, Schauer S, Diwoky C, Temmel H, Guertl B, et al. Adipose triglyceride lipase contributes to cancer-associated cachexia. Science (2011) 333:233–8. doi: 10.1126/science.1198973
72. Tisdale MJ. Mechanisms of cancer cachexia. Physiol Rev (2009) 89:381–410. doi: 10.1152/physrev.00016.2008
73. Dalal S. Lipid metabolism in cancer cachexia. Ann Palliat Med (2019) 8:13–23. doi: 10.21037/apm.2018.10.01
74. Unger RH. Lipotoxic diseases. Annu Rev Med (2002) 53:319–36. doi: 10.1146/annurev.med.53.082901.104057
75. Savage DB, Petersen KF, Shulman GI. Disordered lipid metabolism and the pathogenesis of insulin resistance. Physiol Rev (2007) 87:507–20. doi: 10.1152/physrev.00024.2006
76. Cao DX, Wu GH, Yang ZA, Zhang B, Jiang Y, Han YS, et al. Role of beta1-adrenoceptor in increased lipolysis in cancer cachexia. Cancer Sci (2010) 101:1639–45. doi: 10.1111/j.1349-7006.2010.01582.x
77. Schweiger M, Schreiber R, Haemmerle G, Lass A, Fledelius C, Jacobsen P, et al. Adipose triglyceride lipase and hormone-sensitive lipase are the major enzymes in adipose tissue triacylglycerol catabolism. J Biol Chem (2006) 281:40236–41. doi: 10.1074/jbc.M608048200
78. Yang X, Lu X, Lombes M, Rha GB, Chi YI, Guerin TM, et al. The G(0)/G(1) switch gene 2 regulates adipose lipolysis through association with adipose triglyceride lipase. Cell Metab (2010) 11:194–205. doi: 10.1016/j.cmet.2010.02.003
79. Rofe AM, Bourgeois CS, Coyle P, Taylor A, Abdi EA. Altered insulin response to glucose in weight-losing cancer patients. Anticancer Res (1994) 14:647–50.
80. Han J, Meng Q, Shen L, Wu G. Interleukin-6 induces fat loss in cancer cachexia by promoting white adipose tissue lipolysis and browning. Lipids Health Dis (2018) 17:14. doi: 10.1186/s12944-018-0657-0
81. Nguyen TD, Miyatake Y, Yoshida T, Kawahara H, Hanayama R. Tumor-secreted proliferin-1 regulates adipogenesis and lipolysis in cachexia. Int J Cancer (2021) 148:1982–92. doi: 10.1002/ijc.33418
82. Argiles JM, Busquets S, Stemmler B, Lopez-Soriano FJ. Cancer cachexia: understanding the molecular basis. Nat Rev Cancer (2014) 14:754–62. doi: 10.1038/nrc3829
83. Purcell SA, Elliott SA, Baracos VE, Chu QS, Prado CM. Key determinants of energy expenditure in cancer and implications for clinical practice. Eur J Clin Nutr (2016) 70:1230–8. doi: 10.1038/ejcn.2016.96
84. Vazeille C, Jouinot A, Durand JP, Neveux N, Boudou-Rouquette P, Huillard O, et al. Relation between hypermetabolism, cachexia, and survival in cancer patients: a prospective study in 390 cancer patients before initiation of anticancer therapy. Am J Clin Nutr (2017) 105:1139–47. doi: 10.3945/ajcn.116.140434
85. Abdullahi A, Jeschke MG. White adipose tissue browning: a double-edged sword. Trends Endocrinol Metab (2016) 27:542–52. doi: 10.1016/j.tem.2016.06.006
86. Loncar D, Bedrica L, Mayer J, Cannon B, Nedergaard J, Afzelius BA, et al. The effect of intermittent cold treatment on the adipose tissue of the cat. apparent transformation from white to brown adipose tissue. J Ultrastruct Mol Struct Res (1986) 97:119–29. doi: 10.1016/S0889-1605(86)80012-X
87. Cao L, Choi EY, Liu X, Martin A, Wang C, Xu X, et al. White to brown fat phenotypic switch induced by genetic and environmental activation of a hypothalamic-adipocyte axis. Cell Metab (2011) 14:324–38. doi: 10.1016/j.cmet.2011.06.020
88. Vegiopoulos A, Muller-Decker K, Strzoda D, Schmitt I, Chichelnitskiy E, Ostertag A, et al. Cyclooxygenase-2 controls energy homeostasis in mice by de novo recruitment of brown adipocytes. Science (2010) 328:1158–61. doi: 10.1126/science.1186034
89. Yoneshiro T, Aita S, Matsushita M, Kayahara T, Kameya T, Kawai Y, et al. Recruited brown adipose tissue as an antiobesity agent in humans. J Clin Invest (2013) 123:3404–8. doi: 10.1172/JCI67803
90. Felix K, Fakelman F, Hartmann D, Giese NA, Gaida MM, Schnölzer M, et al. Identification of serum proteins involved in pancreatic cancer cachexia. Life Sci (2011) 88:218–25. doi: 10.1016/j.lfs.2010.11.011
91. Poulia KA, Sarantis P, Antoniadou D, Koustas E, Papadimitropoulou A, Papavassiliou AG, et al. Pancreatic cancer and cachexia-metabolic mechanisms and novel insights. Nutrients (2020) 12:1543. doi: 10.3390/nu12061543
92. Ohno H, Shinoda K, Spiegelman BM, Kajimura S. PPARγ agonists induce a white-to-brown fat conversion through stabilization of PRDM16 protein. Cell Metab (2012) 15:395–404. doi: 10.1016/j.cmet.2012.01.019
93. Seale P, Kajimura S, Yang W, Chin S, Rohas LM, Uldry M, et al. Transcriptional control of brown fat determination by PRDM16. Cell Metab (2007) 6:38–54. doi: 10.1016/j.cmet.2007.06.001
94. Daas SI, Rizeq BR, Nasrallah GK. Adipose tissue dysfunction in cancer cachexia. J Cell Physiol (2018) 234:13–22. doi: 10.1002/jcp.26811
95. Schulz TJ, Huang P, Huang TL, Xue R, McDougall LE, Townsend KL, et al. Brown-fat paucity due to impaired BMP signalling induces compensatory browning of white fat. Nature (2013) 495:379–83. doi: 10.1038/nature11943
96. Li G, Klein RL, Matheny M, King MA, Meyer EM, Scarpace PJ. Induction of uncoupling protein 1 by central interleukin-6 gene delivery is dependent on sympathetic innervation of brown adipose tissue and underlies one mechanism of body weight reduction in rats. Neuroscience (2002) 115:879–89. doi: 10.1016/S0306-4522(02)00447-5
97. Briscoe CP, Tadayyon M, Andrews JL, Benson WG, Chambers JK, Eilert MM, et al. The orphan G protein-coupled receptor GPR40 is activated by medium and long chain fatty acids. J Biol Chem (2003) 278:11303–11. doi: 10.1074/jbc.M211495200
98. Hirasawa A, Tsumaya K, Awaji T, Katsuma S, Adachi T, Yamada M, et al. Free fatty acids regulate gut incretin glucagon-like peptide-1 secretion through GPR120. Nat Med (2005) 11:90–4. doi: 10.1038/nm1168
99. Quesada-Lopez T, Cereijo R, Turatsinze JV, Planavila A, Cairo M, Gavalda-Navarro A, et al. The lipid sensor GPR120 promotes brown fat activation and FGF21 release from adipocytes. Nat Commun (2016) 7:13479. doi: 10.1038/ncomms13479
100. Oliveira V, Marinho R, Vitorino D, Santos GA, Moraes JC, Dragano N, et al. Diets containing α-linolenic (ω3) or oleic (ω9) fatty acids rescues obese mice from insulin resistance. Endocrinology (2015) 156:4033–46. doi: 10.1210/en.2014-1880
101. Schilperoort M, van Dam AD, Hoeke G, Shabalina IG, Okolo A, Hanyaloglu AC, et al. The GPR120 agonist TUG-891 promotes metabolic health by stimulating mitochondrial respiration in brown fat. EMBO Mol Med (2018) 10:e8047. doi: 10.15252/emmm.201708047
102. Yang X, Sui W, Zhang M, Dong M, Lim S, Seki T, et al. Switching harmful visceral fat to beneficial energy combustion improves metabolic dysfunctions. JCI Insight (2017) 2:e89044. doi: 10.1172/jci.insight.89044
103. Hu W, Ru Z, Xiao W, Xiong Z, Wang C, Yuan C, et al. Adipose tissue browning in cancer-associated cachexia can be attenuated by inhibition of exosome generation. Biochem Biophys Res Commun (2018) 506:122–9. doi: 10.1016/j.bbrc.2018.09.139
104. Hu W, Xiong H, Ru Z, Zhao Y, Zhou Y, Xie K, et al. Extracellular vesicles-released parathyroid hormone-related protein from Lewis lung carcinoma induces lipolysis and adipose tissue browning in cancer cachexia. Cell Death Dis (2021) 12:134. doi: 10.1038/s41419-020-03382-0
105. Di W, Zhang W, Zhu B, Li X, Tang Q, Zhou Y. Colorectal cancer prompted adipose tissue browning and cancer cachexia through transferring exosomal miR-146b-5p. J Cell Physiol (2021) 236:5399–410. doi: 10.1002/jcp.30245
106. Liu A, Pan W, Zhuang S, Tang Y, Zhang H. Cancer cell-derived exosomal miR-425-3p induces white adipocyte atrophy. Adipocyte (2022) 11:487–500. doi: 10.1080/21623945.2022.2108558
107. Ding Z, Sun D, Han J, Shen L, Yang F, Sah S, et al. Novel noncoding RNA CircPTK2 regulates lipolysis and adipogenesis in cachexia. Mol Metab (2021) 53:101310. doi: 10.1016/j.molmet.2021.101310
108. Liu Y, Wang M, Deng T, Liu R, Ning T, Bai M, et al. Exosomal miR-155 from gastric cancer induces cancer-associated cachexia by suppressing adipogenesis and promoting brown adipose differentiation via C/EPBβ. Cancer Biol Med (2022) 19:1301–14. doi: 10.20892/j.issn.2095-3941.2021.0220
109. Huang YC, Chen TB, Hsu CC, Li SH, Wang PW, Lee BF, et al. The relationship between brown adipose tissue activity and neoplastic status: an (18)F-FDG PET/CT study in the tropics. Lipids Health Dis (2011) 10:238. doi: 10.1186/1476-511X-10-238
110. Tsoli M, Moore M, Burg D, Painter A, Taylor R, Lockie SH, et al. Activation of thermogenesis in brown adipose tissue and dysregulated lipid metabolism associated with cancer cachexia in mice. Cancer Res (2012) 72:4372–82. doi: 10.1158/0008-5472.CAN-11-3536
111. Brooks SL, Neville AM, Rothwell NJ, Stock MJ, Wilson S. Sympathetic activation of brown-adipose-tissue thermogenesis in cachexia. Biosci Rep (1981) 1:509–17. doi: 10.1007/BF01121584
112. Wang Q, Zhang M, Ning G, Gu W, Su T, Xu M, et al. Brown adipose tissue in humans is activated by elevated plasma catecholamines levels and is inversely related to central obesity. PloS One (2011) 6:e21006. doi: 10.1371/journal.pone.0021006
113. Wang W, Kissig M, Rajakumari S, Huang L, Lim HW, Won KJ, et al. Ebf2 is a selective marker of brown and beige adipogenic precursor cells. Proc Natl Acad Sci USA (2014) 111:14466–71. doi: 10.1073/pnas.1412685111
114. Lee YH, Petkova AP, Konkar AA, Granneman JG. Cellular origins of cold-induced brown adipocytes in adult mice. FASEB J (2015) 29:286–99. doi: 10.1096/fj.14-263038
115. Maroni G, Panetta D, Luongo R, Krishnan I, La Rosa F, Campani D, et al. The role of Prep1 in the regulation of mesenchymal stromal cells. Int J Mol Sci (2019) 20:3639. doi: 10.3390/ijms20153639
116. Rohm M, Schafer M, Laurent V, Ustunel BE, Niopek K, Algire C, et al. An AMP-activated protein kinase-stabilizing peptide ameliorates adipose tissue wasting in cancer cachexia in mice. Nat Med (2016) 22:1120–30. doi: 10.1038/nm.4171
117. Schnyder S, Handschin C. Skeletal muscle as an endocrine organ: PGC-1α, myokines exercise. Bone (2015) 80:115–25. doi: 10.1016/j.bone.2015.02.008
118. Cannon TY, Guttridge D, Dahlman J, George JR, Lai V, Shores C, et al. The effect of altered toll-like receptor 4 signaling on cancer cachexia. Arch Otolaryngol Head Neck Surg (2007) 133:1263–9. doi: 10.1001/archotol.133.12.1263
119. Fukawa T, Yan-Jiang BC, Min-Wen JC, Jun-Hao ET, Huang D, Qian CN, et al. Excessive fatty acid oxidation induces muscle atrophy in cancer cachexia. Nat Med (2016) 22:666–71. doi: 10.1038/nm.4093
120. Suriben R, Chen M, Higbee J, Oeffinger J, Ventura R, Li B, et al. Antibody-mediated inhibition of GDF15-GFRAL activity reverses cancer cachexia in mice. Nat Med (2020) 26:1264–70. doi: 10.1038/s41591-020-0945-x
121. Strelau J, Strzelczyk A, Rusu P, Bendner G, Wiese S, Diella F, et al. Progressive postnatal motoneuron loss in mice lacking GDF-15. J Neurosci (2009) 29:13640–8. doi: 10.1523/JNEUROSCI.1133-09.2009
122. Elattar S, Dimri M, Satyanarayana A. The tumor secretory factor ZAG promotes white adipose tissue browning and energy wasting. FASEB J (2018) 32:4727–43. doi: 10.1096/fj.201701465RR
123. Uversky VN, El-Baky NA, El-Fakharany EM, Sabry A, Mattar EH, Uversky AV, et al. Functionality of intrinsic disorder in tumor necrosis factor-α and its receptors. FEBS J (2017) 284:3589–618. doi: 10.1111/febs.14182
124. Choe SS, Huh JY, Hwang IJ, Kim JI, Kim JB. Adipose tissue remodeling: its role in energy metabolism and metabolic disorders. Front Endocrinol (Lausanne) (2016) 7:30. doi: 10.3389/fendo.2016.00030
125. Cheng H, Qi T, Zhang X, Kong Q, Min X, Mao Q, et al. Deficiency of heat shock protein A12A promotes browning of white adipose tissues in mice. Biochim Biophys Acta Mol Basis Dis (2019) 1865:1451–9. doi: 10.1016/j.bbadis.2019.02.017
126. Xie H, Heier C, Meng X, Bakiri L, Pototschnig I, Tang Z, et al. An immune-sympathetic neuron communication axis guides adipose tissue browning in cancer-associated cachexia. Proc Natl Acad Sci USA (2022) 119:e2112840119. doi: 10.1073/pnas.2112840119
127. Baazim H, Schweiger M, Moschinger M, Xu H, Scherer T, Popa A, et al. CD8(+) T cells induce cachexia during chronic viral infection. Nat Immunol (2019) 20:701–10. doi: 10.1038/s41590-019-0397-y
128. Naveiras O, Nardi V, Wenzel PL, Hauschka PV, Fahey F, Daley GQ. Bone-marrow adipocytes as negative regulators of the haematopoietic microenvironment. Nature (2009) 460:259–63. doi: 10.1038/nature08099
129. Jia H, Lyu W, Hirota K, Saito E, Miyoshi M, Hohjoh H, et al. Eggshell membrane modulates gut microbiota to prevent murine pre-cachexia through suppression of T helper cell differentiation. J Cachexia Sarcopenia Muscle (2022) 13:2088–101. doi: 10.1002/jcsm.13019
130. Ubachs J, Ziemons J, Soons Z, Aarnoutse R, van Dijk DPJ, Penders J, et al. Gut microbiota and short-chain fatty acid alterations in cachectic cancer patients. J Cachexia Sarcopenia Muscle (2021) 12:2007–21. doi: 10.1002/jcsm.12804
131. Notarnicola M, Miccolis A, Tutino V, Lorusso D, Caruso MG. Low levels of lipogenic enzymes in peritumoral adipose tissue of colorectal cancer patients. Lipids (2012) 47:59–63. doi: 10.1007/s11745-011-3630-5
132. Lopez-Soriano J, Argiles JM, Lopez-Soriano FJ. Lipid metabolism in rats bearing the yoshida AH-130 ascites hepatoma. Mol Cell Biochem (1996) 165:17–23. doi: 10.1007/BF00229741
133. Greenberg AS, Nordan RP, McIntosh J, Calvo JC, Scow RO, Jablons D. Interleukin 6 reduces lipoprotein lipase activity in adipose tissue of mice in vivo and in 3T3-L1 adipocytes: a possible role for interleukin 6 in cancer cachexia. Cancer Res (1992) 52:4113–6.
134. Catanese S, Beuchel CF, Sawall T, Lordick F, Brauer R, Scholz M, et al. Biomarkers related to fatty acid oxidative capacity are predictive for continued weight loss in cachectic cancer patients. J Cachexia Sarcopenia Muscle (2021) 12:2101–10. doi: 10.1002/jcsm.12817
135. Briddon S, Beck SA, Tisdale MJ. Changes in activity of lipoprotein lipase, plasma free fatty acids and triglycerides with weight loss in a cachexia model. Cancer Lett (1991) 57:49–53. doi: 10.1016/0304-3835(91)90062-M
136. Bing C, Russell S, Becket E, Pope M, Tisdale MJ, Trayhurn P, et al. Adipose atrophy in cancer cachexia: morphologic and molecular analysis of adipose tissue in tumour-bearing mice. Br J Cancer (2006) 95:1028–37. doi: 10.1038/sj.bjc.6603360
137. Batista ML Jr., Neves RX, Peres SB, Yamashita AS, Shida CS, Farmer SR, et al. Heterogeneous time-dependent response of adipose tissue during the development of cancer cachexia. J Endocrinol (2012) 215:363–73. doi: 10.1530/JOE-12-0307
138. Tsoli M, Schweiger M, Vanniasinghe AS, Painter A, Zechner R, Clarke S, et al. Depletion of white adipose tissue in cancer cachexia syndrome is associated with inflammatory signaling and disrupted circadian regulation. PloS One (2014) 9:e92966. doi: 10.1371/journal.pone.0092966
139. Sun D, Ding Z, Shen L, Yang F, Han J, Wu G. miR-410-3P inhibits adipocyte differentiation by targeting IRS-1 in cancer-associated cachexia patients. Lipids Health Dis (2021) 20:115. doi: 10.1186/s12944-021-01530-9
140. Vigano A, Del Fabbro E, Bruera E, Borod M. The cachexia clinic: from staging to managing nutritional and functional problems in advanced cancer patients. Crit Rev Oncog (2012) 17:293–303. doi: 10.1615/CritRevOncog.v17.i3.70
141. Blum D, Stene GB, Solheim TS, Fayers P, Hjermstad MJ, Baracos VE, et al. Validation of the consensus-definition for cancer cachexia and evaluation of a classification model–a study based on data from an international multicentre project (EPCRC-CSA). Ann Oncol (2014) 25:1635–42. doi: 10.1093/annonc/mdu086
142. Dingemans AM, de Vos-Geelen J, Langen R, Schols AM. Phase II drugs that are currently in development for the treatment of cachexia. Expert Opin Investig Drugs (2014) 23:1655–69. doi: 10.1517/13543784.2014.942729
143. Takayama K, Katakami N, Yokoyama T, Atagi S, Yoshimori K, Kagamu H, et al. Anamorelin (ONO-7643) in Japanese patients with non-small cell lung cancer and cachexia: results of a randomized phase 2 trial. Support Care Cancer (2016) 24:3495–505. doi: 10.1007/s00520-016-3144-z
144. Temel JS, Abernethy AP, Currow DC, Friend J, Duus EM, Yan Y, et al. Anamorelin in patients with non-small-cell lung cancer and cachexia (ROMANA 1 and ROMANA 2): results from two randomised. double-blind phase 3 trials Lancet Oncol (2016) 17:519–31. doi: 10.1016/S1470-2045(15)00558-6
145. Dobs AS, Boccia RV, Croot CC, Gabrail NY, Dalton JT, Hancock ML, et al. Effects of enobosarm on muscle wasting and physical function in patients with cancer: a double-blind, randomised controlled phase 2 trial. Lancet Oncol (2013) 14:335–45. doi: 10.1016/S1470-2045(13)70055-X
146. Stewart Coats AJ, Ho GF, Prabhash K, von Haehling S, Tilson J, Brown R, et al. Espindolol for the treatment and prevention of cachexia in patients with stage III/IV non-small cell lung cancer or colorectal cancer: a randomized, double-blind, placebo-controlled, international multicentre phase II study (the ACT-ONE trial). J Cachexia Sarcopenia Muscle (2016) 7:355–65. doi: 10.1002/jcsm.12126
147. Molfino A, Amabile MI, Rossi Fanelli F, Muscaritoli M. Novel therapeutic options for cachexia and sarcopenia. Expert Opin Biol Ther (2016) 16:1239–44. doi: 10.1080/14712598.2016.1208168
148. Stene GB, Helbostad JL, Balstad TR, Riphagen II, S. Kaasa LM. Oldervoll, effect of physical exercise on muscle mass and strength in cancer patients during treatment–a systematic review. Crit Rev Oncol Hematol (2013) 88:573–93. doi: 10.1016/j.critrevonc.2013.07.001
149. Khosravi N, Stoner L, Farajivafa V, Hanson ED, training E. Circulating cytokine levels and immune function in cancer survivors: a meta-analysis. Brain Behav Immun (2019) 81:92–104. doi: 10.1016/j.bbi.2019.08.187
150. Lai V, George J, Richey L, Kim HJ, Cannon T, Shores C, et al. Results of a pilot study of the effects of celecoxib on cancer cachexia in patients with cancer of the head, neck, and gastrointestinal tract. Head Neck (2008) 30:67–74. doi: 10.1002/hed.20662
151. Solheim TS, Fearon KC, Blum D, Kaasa S. Non-steroidal anti-inflammatory treatment in cancer cachexia: a systematic literature review. Acta Oncol (2013) 52:6–17. doi: 10.3109/0284186X.2012.724536
152. Mocellin MC, Fernandes R, Chagas TR, Trindade E. A meta-analysis of n-3 polyunsaturated fatty acids effects on circulating acute-phase protein and cytokines in gastric cancer. Clin Nutr (2018) 37:840–50. doi: 10.1016/j.clnu.2017.05.008
153. Abe K, Uwagawa T, Haruki K, Takano Y, Onda S, Sakamoto T, et al. Effects of ω-3 fatty acid supplementation in patients with bile duct or pancreatic cancer undergoing chemotherapy. Anticancer Res (2018) 38:2369–75. doi: 10.21873/anticanres.12485
154. Riccardi D, das Neves RX, de Matos-Neto EM, Camargo RG, Lima J, Radloff K, et al. Plasma lipid profile and systemic inflammation in patients with cancer cachexia. Front Nutr (2020) 7:4. doi: 10.3389/fnut.2020.00004
155. Hendifar A, Akinsola R, Muranaka H, Osipov A, Thomassian S, Moshayedi N, et al. Gut microbiome and pancreatic cancer cachexia: an evolving relationship. World J Gastrointest Oncol (2022) 14:1218–26. doi: 10.4251/wjgo.v14.i7.1218
156. Mansson JV, Alves FD, Biolo A, Souza GC. Use of ghrelin in cachexia syndrome: a systematic review of clinical trials. Nutr Rev (2016) 74:659–69. doi: 10.1093/nutrit/nuw029
157. Neary NM, Small CJ, Wren AM, Lee JL, Druce MR, Palmieri C, et al. Ghrelin increases energy intake in cancer patients with impaired appetite: acute, randomized, placebo-controlled trial. J Clin Endocrinol Metab (2004) 89:2832–6. doi: 10.1210/jc.2003-031768
158. Maltoni M, Nanni O, Scarpi E, Rossi D, Serra P, Amadori D. High-dose progestins for the treatment of cancer anorexia-cachexia syndrome: a systematic review of randomised clinical trials. Ann Oncol (2001) 12:289–300. doi: 10.1023/A:1011156811739
159. Wilson HE, Stanton DA, Rellick S, Geldenhuys W, Pistilli EE. Breast cancer-associated skeletal muscle mitochondrial dysfunction and lipid accumulation is reversed by PPARG. Am J Physiol Cell Physiol (2021) 320:C577–c590. doi: 10.1152/ajpcell.00264.2020
160. Auger C, Knuth CM, Abdullahi A, Samadi O, Parousis A, Jeschke MG. Metformin prevents the pathological browning of subcutaneous white adipose tissue. Mol Metab (2019) 29:12–23. doi: 10.1016/j.molmet.2019.08.011
161. Palus S, von Haehling S, Flach VC, Tschirner A, Doehner W, Anker SD, et al. Simvastatin reduces wasting and improves cardiac function as well as outcome in experimental cancer cachexia. Int J Cardiol (2013) 168:3412–8. doi: 10.1016/j.ijcard.2013.04.150
162. Lu S, Li Y, Shen Q, Zhang W, Gu X, Ma M, et al. Carnosol and its analogues attenuate muscle atrophy and fat lipolysis induced by cancer cachexia. J Cachexia Sarcopenia Muscle (2021) 12:779–95. doi: 10.1002/jcsm.12710
163. Chae J, Kim JS, Choi ST, Lee SG, Ojulari OV, Kang YJ, et al. Farrerol induces cancer cell death via ERK activation in SKOV3 cells and attenuates TNF-α-Mediated lipolysis. Int J Mol Sci (2021) 22:9400. doi: 10.3390/ijms22179400
164. Yang YH, Hao YM, Liu XF, Gao X, Wang BZ, Takahashi K, et al. Docosahexaenoic acid-enriched phospholipids and eicosapentaenoic acid-enriched phospholipids inhibit tumor necrosis factor-alpha-induced lipolysis in 3T3-L1 adipocytes by activating sirtuin 1 pathways. Food Funct (2021) 12:4783–96. doi: 10.1039/D1FO00157D
165. Brendle C, Stefan N, Stef I, Ripkens S, Soekler M, la Fougère C, et al. Impact of diverse chemotherapeutic agents and external factors on activation of brown adipose tissue in a large patient collective. Sci Rep (2019) 9:1901. doi: 10.1038/s41598-018-37924-6
166. Solheim TS, Laird BJA, Balstad TR, Bye A, Stene G, Baracos V, et al. Cancer cachexia: rationale for the MENAC (Multimodal-exercise, nutrition and anti-inflammatory medication for cachexia) trial. BMJ Support Palliat Care (2018) 8:258–65. doi: 10.1136/bmjspcare-2017-001440
167. Xue W, Yu SY, Kuss M, Kong Y, Shi W, Chung S, et al. 3D bioprinted white adipose model for in vitro study of cancer-associated cachexia induced adipose tissue remodeling. Biofabrication (2022) 14:034106. doi: 10.1088/1758-5090/ac6c4b
168. Degrelle SA, Delile S, Moog S, Mouisel E, O'Gorman D, Moro C, et al. DietSee: an on-hand, portable, strip-type biosensor for lipolysis monitoring via real-time amperometric determination of glycerol in blood. Anal Chim Acta (2021) 1155:338358. doi: 10.1016/j.aca.2021.338358
169. Ebner N, Anker SD, von Haehling S. Recent developments in the field of cachexia, sarcopenia, and muscle wasting: highlights from the 11th cachexia conference. J Cachexia Sarcopenia Muscle (2019) 10:218–25. doi: 10.1002/jcsm.12408
Keywords: lipid metabolism, adipose tissue, cancer, cachexia, therapy strategy
Citation: Fang R, Yan L and Liao Z (2023) Abnormal lipid metabolism in cancer-associated cachexia and potential therapy strategy. Front. Oncol. 13:1123567. doi: 10.3389/fonc.2023.1123567
Received: 14 December 2022; Accepted: 17 April 2023;
Published: 02 May 2023.
Edited by:
Anna Sebestyén, Semmelweis University, HungaryReviewed by:
Parul Singh, National Institutes of Health (NIH), United StatesCopyright © 2023 Fang, Yan and Liao. This is an open-access article distributed under the terms of the Creative Commons Attribution License (CC BY). The use, distribution or reproduction in other forums is permitted, provided the original author(s) and the copyright owner(s) are credited and that the original publication in this journal is cited, in accordance with accepted academic practice. No use, distribution or reproduction is permitted which does not comply with these terms.
*Correspondence: Zhengkai Liao, emxpYW9Ad2h1LmVkdS5jbg==; Ling Yan, bGluZ3lhbkB3aHUuZWR1LmNu
Disclaimer: All claims expressed in this article are solely those of the authors and do not necessarily represent those of their affiliated organizations, or those of the publisher, the editors and the reviewers. Any product that may be evaluated in this article or claim that may be made by its manufacturer is not guaranteed or endorsed by the publisher.
Research integrity at Frontiers
Learn more about the work of our research integrity team to safeguard the quality of each article we publish.