- 1National Clinical Research Center for Obstetrical and Gynecological Diseases; Department of Gynecology, Tongji Hospital, Tongji Medical College, Huazhong University of Science and Technology, Wuhan, Hubei, China
- 2Key Laboratory of Cancer Invasion and Metastasis, Ministry of Education, Wuhan, Hubei, China
Although previous studies have shed light on the etiology of cervical cancer, metastasis of advanced cervical cancer remains the main reason for the poor outcome and high cancer-related mortality rate. Cervical cancer cells closely communicate with immune cells recruited to the tumor microenvironment (TME), such as lymphocytes, tumor-associated macrophages, and myeloid-derived suppressor cells. The crosstalk between tumors and immune cells has been clearly shown to foster metastatic dissemination. Therefore, unraveling the mechanisms of tumor metastasis is crucial to develop more effective therapies. In this review, we interpret several characteristics of the TME that promote the lymphatic metastasis of cervical cancer, such as immune suppression and premetastatic niche formation. Furthermore, we summarize the complex interactions between tumor cells and immune cells within the TME, as well as potential therapeutic strategies to target the TME.
Introduction
Cervical cancer (CC) ranks fourth among all types of cancers in terms of morbidity and mortality in women, with an estimated 604,000 new cases and 342,000 deaths worldwide in 2020. Furthermore, CC is the most commonly diagnosed cancer in 23 countries and the leading cause of cancer-related death in 36 countries (1). Although most patients with early-stage CC recover well through surgery, patients with recurrent or metastatic CC are rarely treated effectively (2, 3). Therefore, patients with advanced CC have a poor prognosis and low survival rate (4, 5). Lymphatic vessels are one of the crucial routes by which CC cells metastasize, and treatment failure is often associated with lymph node metastasis (6). In the past, lymphatic vessels were considered to play a passive role in metastasis, acting merely as a channel for tumor cells to invade. However, growing evidence has suggested that tumor-associated lymphatic vessels can actively participate in tumor-related pathological processes, thereby promoting the lymphatic metastasis of CC (7). On the one hand, peritumoral lymphatic vessels can boost tumor cell lymphatic invasion; on the other hand, tumor-draining lymphatic vessels promote tumor metastasis by increasing pumping and lymph flow, recruiting tumor cells, providing cancer stem cell niches, and modulating antitumor immune responses (8).
The tumor microenvironment (TME) has been an area of active research in recent years, as it exerts an instrumental influence on tumor progression and metastasis (9). The TME is largely composed of immune cells, fibroblasts, endothelial cells, stromal cells, and extracellular matrix (ECM) (10, 11). It is well-accepted that the interactions between tumors and immune cells are more complex and dynamic than previously thought. Many subtypes of immune cells infiltrating the TME also possess potent tumor-promoting abilities (11, 12). However, the impact of the crosstalk between the TME and immune cells on tumor metastasis remains unclear. A better understanding of the interactions between CC cells and immune cell and their roles in lymphatic metastasis will be beneficial for developing alternative therapies. In this review, we summarize the role of the TME in CC and introduce five main characteristics of the TME that promote lymph node metastasis.
Characteristics of the TME
Successful lymphatic metastasis is a consequence of complex processes involving interactions among multiple components in the TME. The progression can be simplified into four steps: the dissemination of locally invasive tumor cells to the lymphatic vessels, the transport of tumor cells through the lymphatic system to the lymph nodes, the settlement of tumor cells in the lymph nodes, and the growth of micrometastases into a detectable mass (13). Observations of metastasis in afferent lymphatic vessels, as well as the specific and early involvement of the tumor-draining lymph nodes have strongly indicated that tumor cells initiate lymph node metastasis by entering tumor-associated lymphatic vessels (14). Interestingly, the lymphatic system does not act as a passive channel for tumors to invade but actively facilitates lymphatic metastasis in the TME (7, 15). The complicated interplay among tumor cells, immune cells, and the local stroma promotes the lymphatic metastasis of CC. At least five key characteristics of the TME favor this process, including immunosuppression, premetastatic niche formation, lymphangiogenesis, epithelial-mesenchymal transition (EMT), and ECM remodeling (Figure 1).
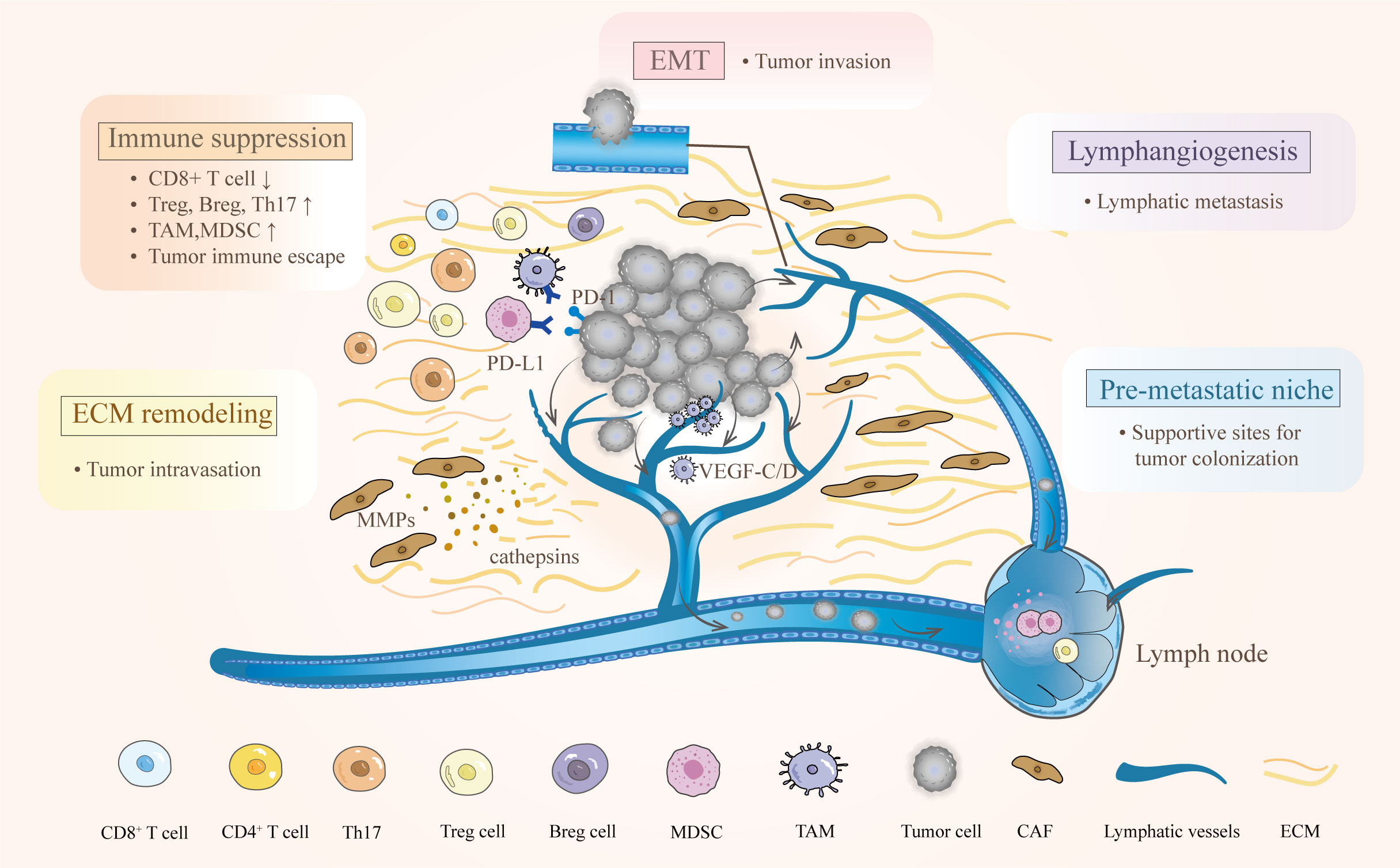
Figure 1 Characteristics of the TME and their effects on lymphatic metastasis in cervical cancer. The complex interactions between cervical cancer and immune cells empower the TME to hamper antitumor immunity. Various bioactive substances derived from primary tumors participate in the regulation of ECM and lymphangiogenesis. After EMT, tumor cells can easily reach and enter the lymphatic vessel. Moreover, creating a premetastatic niche provides supportive sites for metastatic tumor cells.
Immunosuppression
There is a consensus that CC is an immunogenic tumor induced by persistent infection with the high-risk human papillomavirus (HPV) (16). During persistent HPV infection, tumor cells employ a range of strategies for immune evasion. Studies indicate that HPV downregulates the gene expression of C-X-C motif chemokine ligand 14 (CXCL14), the adhesion molecule E-cadherin and Toll-like receptors in host cells, disrupting the recruitment of natural killer (NK) cells, T cells, Langerhans cells and dendritic cells (17, 18). Moreover, HPV impairs the expression of MHC class-I (MHC-I), immunoproteasome subunits, and transporter associated with antigen processing (TAP), interfering with antigen presentation (19). All these strategies enable the virus to escape immunosurveillance and persist for a long time, increasing the risk of lesion progression and malignant transformation.
In addition to the virus, the TME actively participates in tumor proliferation and lymph node metastasis by suppressing antitumor immunity and inducing regulatory and suppressive immune cells. The TME is characterized by an imbalance in T helper (Th) cells in tumor tissues. Normally, CD4+ T cells aid cytotoxic CD8+ T cells in tumor rejection. However, during the progression of the tumor, the Th1 tumor-suppressive phenotype is converted into a Th2 tumor-promoting phenotype, which hinders the activity of CD8+ T cells (12). In fact, reduced intraepithelial infiltration of CD8+ T cells and a significantly decreased CD8+/CD4+ T-cell ratio have been found in CC patients with lymph node metastases (20). Additionally, studies on metastatic tumor-draining lymph nodes in CC have shown that these sites are characterized by suppressor-type cells with significant Th2 polarization and reduced content of effector-type CD8+ T cells (21). Recently, researchers have found that the TME exhibits an immunosuppressive state in CC tissue, characterized by infiltration of exhausted CD8+ T cells and CD163+ CD68+ M2-like macrophages (22). Similarly, another study has demonstrated that the cytotoxic activities of CD8+ T cells are significantly curtailed by high-level expression of checkpoint genes, such as programmed cell death 1 (PD-1), lymphocyte activating 3 (LAG3) and hepatitis A virus cellular receptor 2 (HAVCR2) (23). All these findings suggest the existence of profoundly immunosuppressive microenvironments in primary tumors and tumor-draining lymph nodes, which protect metastatic tumor cells from immune attacks. Consequently, tumor cells are able to migrate to the lymph nodes.
Notably, specific immune cells, including tumor-associated macrophages (TAMs) and myeloid-derived suppressor cells (MDSCs), contribute to the formation of immunosuppressive TME. Increased infiltration of TAMs is related to a more advanced stage and lymph node metastasis in CC (24–26), as is increased infiltration of MDSCs (27, 28). Both of these cell populations can suppress the proliferation and function of effector T cells and induce the recruitment of regulatory T (Treg) cells and regulatory B (Breg) cells (28–30). Additionally, Th17 cells have been found to promote tumor progression by triggering chronic inflammation within the TME. They are attracted by fibroblasts expressing high levels of C-C motif chemokine ligand (CCL20) (31). Lymphatic endothelial cells (LECs) are also reprogrammed into an essential component with immunosuppressive effects in the TME. In a mouse model, exosome-encapsulated microRNA-1468-5p secreted by CC cells could promote programmed cell death ligand 1 (PD-L1) expression in LECs. Then, the LECs impaired CD8+ T-cell immunity by binding to PD-1, enabling CC immune escape (2). Overall, the significant immunosuppressive state offers suitable conditions for tumor proliferation and a window for tumor metastasis.
Premetastatic niches
Primary tumors can instruct the formation of microenvironments in distant organs hospitable to tumor cells before arriving at these sites. These predetermined microenvironments are termed premetastatic niches (32, 33). Well-established premetastatic niches with a fertile microenvironment support the survival, seeding, colonization, and outgrowth of metastatic tumor cells, resulting in the formation of micrometastases (33). Evidence suggests that the nearest draining lymph nodes in patients with CC can be prepared early to host metastatic cells (15). To successfully build a premetastatic niche, primary CC cells, MDSCs, and other components in the local stromal microenvironment of the host (or future metastatic organ) work together. However, there needs more research to understand the role of CC cells in establishing the premetastatic niche.
The creation of a premetastatic niche is fostered by mobilized and recruited immune cells. These cells remodel the local microenvironment by secreting inflammatory cytokines, growth factors, and proangiogenic molecules (34). For instance, in mouse models, MDSCs have been shown to contribute to premetastatic niche formation by expressing high levels of proinflammatory chemoattractants such as Cxcl2, S100a8, S100a9, and Bv8 (35). Similarly, in ME180 tumor-bearing rats, MDSCs were found to express S100a8/a9 to create a premetastatic niche in the lymph nodes (36). Before metastasis, Treg cells may also condition lymph nodes to form a metastatic niche. Subsequently, PD-L1+ M2-like macrophages are recruited to facilitate the expansion of the next wave of Treg cells, preparing for further metastatic spread (37).
Changes in the TME, such as lymphangiogenesis and ECM remodeling, establish a supportive microenvironment that may foster the colonization and outgrowth of metastatic tumor cells in secondary sites (33, 38). Periostin (POSTN) has recently been identified as a crucial matricellular protein in lymph node premetastatic niche development. In a preclinical murine model, cancer-associated fibroblast (CAF)-derived POSTN regulated the function of LECs and promoted the implantation of metastatic cells in the lymph nodes (38, 39). Moreover, both in mouse and human CC, lymphangiogenesis is detected in the tumor-draining lymph nodes even before lymphatic metastasis, confirming the presence of a premetastatic niche (8). The role of lymphatic vessels in promoting metastasis via the premetastatic niche has yet to be fully understood. Further analysis of LECs and identification of the key components involved in promoting metastasis via lymphangiogenesis may help to unravel these mysteries.
Tumor lymphangiogenesis
In CC, lymphatic metastasis is one of the major routes of metastasis, within which tumor-associated lymphangiogenesis is an essential event. Tumor lymphangiogenesis involves the migration of endothelial cells into the tumor tissue and the formation of new lymphatic vessels. This process leads to an increase in tumoral lymphatic vessels and enlargement of tumor-draining collecting vessels. The expansion of the lymphatic network promotes tumor migration into the lymphatic circulation and entry into the lymph nodes, where the tumor cells multiply, and potentially facilitate migration to distant organs (14, 40). Clinicopathological studies have shown a positive correlation between lymphatic microvessel density and metastasis (41). As mentioned before, premetastatic lymphangiogenesis in the lymph nodes plays an important role in preparing the site for metastatic dissemination (15). Therefore, lymphangiogenesis and lymphatic remodeling are functionally important in the promotion of tumor metastasis.
Lymphangiogenesis is mediated by various lymphangiogenic factors released by CC cells or host-derived cells within the TME, including vascular endothelial growth factor A (VEGF-A), VEGF-C/D, and angiopoietins. VEGF-C/D promotes tube formation by binding to their receptor VEGFR-3 expressed by LECs (14, 42), while VEGF-A stimulates both angiogenesis and lymphangiogenesis by binding to VEGFR-2 (14). Clinical data indicate elevated VEGF-C expression in peripheral leukocytes and retroperitoneal lymph nodes in CC is associated with tumor progression and lymphatic metastasis (43).
Some tumor-derived factors have been found to promote lymphangiogenesis in CC. For instance, the receptor for activated C-kinase 1 (RACK1), a scaffold protein that participates in many intracellular signal transduction pathways, facilitates tumor invasion and lymphatic tube formation. Upregulated expression of RACK1 promotes lymphangiogenesis via galectin-1 (44), which promotes lymphatic vascular growth and contributes to the maintenance of the lymphatic endothelial phenotype (45). In addition, RACK1 interacts with insulin−like growth factor 1 receptor (IGF1R) to promote lymphangiogenesis via activation of the AKT/mTOR pathway in CC cells (46). Hematological and neurological expressed 1 (HN1), a microtubule-associated protein, induces lymphangiogenesis by activating the NF-κB signaling pathway (47). Likewise, sine oculis homeobox homolog 1 (SIX1) and protein tyrosine phosphatase receptor type M (PTPRM) expressed in tumor cells both promote tumor lymphangiogenesis by inducing increased expression of VEGF-C (48, 49). Moreover, cancer-secreted exosomal microRNA-221-3p transferred to LECs may promote lymphangiogenesis by downregulating vasohibin-1, an endogenous angiogenesis inhibitor (40). CC-secreted exosomal microRNA-1468-5p also promotes lymphangiogenesis by reprogramming LECs (2). As a regulator of the function of Treg cells, the Foxp3 gene has a vital role in forming the immunosuppressive microenvironment. Interestingly, a study indicated that Foxp3 was positively correlated with VEGF-C expression and might be involved in lymph node metastasis of CC by promoting lymphangiogenesis (50). These results indicate that multiple tumor-associated factors play important roles in inducing lymphangiogenesis, leading to the lymphatic metastasis of CC.
Other tumor partners in the TME also contribute to lymphangiogenesis in CC. For example, after being cultured in a conditioned medium from CC cell-macrophage coculture, human LECs formed more tube-like structures in vitro. These TAMs promoted lymphangiogenesis by increasingly expressing VEGF-C and VEGF-A, which was induced by the interactions with surrounding CC cells (26). Tumor-associated LECs actively participate in lymphatic metastasis by highly expressing soluble semaphorin 4C (sSEMA4C), which promotes lymphangiogenesis by activating PlexinB2-ERBB2 signaling in LECs and facilitates tumor proliferation and migration by activating PlexinB2-MET signaling (7). CAFs regulate LEC functions and promote metastatic cell implantation in the lymph nodes by secreting POSTN, which induces VEGF-C-driven lymphangiogenesis (38). Moreover, cancer-associated inflammation is likely to promote lymphangiogenesis and facilitate metastasis. Studies in CC provide evidence for a clinical association between cyclooxygenase-2 (COX-2) and VEGF-C expression. COX-2, an enzyme required for prostaglandin synthesis, is induced by inflammatory signals in various cell types, including myeloid and endothelial cells. As a consequence, prostaglandins induce the expression of VEGF-C and increase tumor lymphangiogenesis (41, 51). Taken together, these data suggest that various molecules in the TME of CC contribute to tumor lymphangiogenesis. However, it is unclear whether the increased quantity of lymphatic vessels and enlarged draining collecting vessels due to lymphangiogenesis and remodeling are prerequisites for lymphatic metastasis, and the mechanisms underlying these events require further elucidation.
EMT
EMT refers to the cellular phenotype change, in which epithelial cells acquire mesenchymal properties and attain motility. The EMT phenotype promotes attachment to the ECM. It enhances the migratory potential of an individual tumor cell by reorganizing actin fibers, which confers mesenchymal traits that are extremely important for tumor cells to efficiently intravasate into basement membranes and lymphatic vessels. In fact, infiltrating tumor cells undergo “partial” or “hybrid” EMT, leading to a mixed phenotype with both epithelial and mesenchymal traits, which favor collective over single-cell infiltration (52). In the tumor context, EMT is induced by various signals, such as transforming growth factor β (TGF-β), WNT/β-catenin, AKT signaling, inflammation, and hypoxia, which activate EMT-associated transcription factors including Snail, Twist, and Zeb family members (49, 53–59). These transcription factors trigger EMT, converge at the promotion of E-cadherin repressors, and mediate phenotypic changes by downregulating epithelial traits while inducing mesenchymal characteristics (60). Ultimately, the expressed cytoskeletal-remodeling and ECM-degrading proteases enable tumor cells to invade the surrounding tissue (61, 62).
Numerous EMT-inducing factors in the TME are associated with an increased risk of metastasis and a worse prognosis. For instance, the elevated expression of fatty acid-binding protein 5 (FABP5), nucleolar and spindle associated protein 1 (NUSAP1), and the oncoprotein cancerous inhibitor of protein phosphatase 2 (CIP2A) in the tumor tissue of CC patients is significantly associated with lymph node metastasis. Furthermore, the effects of knockdown or overexpression of these factors in different CC cell lines demonstrate that these molecules promote EMT by activating NF-κB signaling, Wnt/β-catenin signaling, and MEK/ERK signaling pathway, respectively (63–65). The long noncoding RNAs taurine-upregulated gene 1 (TUG1) and steroid receptor RNA activator (SRA), microRNA-21 and microRNA-663b modulate the EMT process and ultimately promote local and distant metastasis (66–69). Additionally, overexpressed PD-L1 binds directly to integrin β4 (ITGB4) and activates the AKT/GSK3β signaling pathway, consequently inducing the expression of Snail (56). PTPRM promotes EMT via the Src-AKT signaling pathway (49). Indeed, cancer cells are not the only cells that can induce EMT; CAFs also induce EMT by overexpressing TGF-β1 and stromal cell-derived factor 1 (SDF-1), further promoting the growth, invasion, and migration of CC (70). Taken together, these findings indicate that significant EMT in the TME is involved in lymphatic metastasis by promoting tumor adhesion and intravasation into the lymphatic vessel endothelium.
ECM remodeling
Tumor metastasis requires the breakdown of the basement membrane and remodeling of the ECM. The proteolytic cleavage and degradation of ECM proteins are coordinated by a number of enzymes, including serine proteases, matrix metalloproteinases (MMPs) (71–73), and cysteine cathepsins (74). Alterations in these ECM molecules can be observed in the TME during tumor metastasis. For instance, elevated expression of MMPs has been reported in invasive CC tissues compared with normal cervical tissues, especially MMP-3 and MMP-9 (75). MMPs play a role in the degradation of the ECM through direct action. As one of the cysteine proteases, cathepsin L not only reduces cell adhesion through cleavage of E-cadherin but also degrades the ECM to support tumor migration and invasion. Moreover, increased cathepsin L expression in CC tissues correlates with poorer 5-year overall cumulative survival in CC patients (74).
These proteolytic enzymes can be secreted by tumor cells. However, the majority are secreted by resident stromal cells, such as cancer-associated myofibroblasts (myCAFs) (76). MMP-1 and MMP-2 secreted by CAFs play vital roles in initiating the invasion of malignant tumors and make tumor intravasation easier by clearing ECM proteins that block access to the endothelium. CAFs also promote the migration of tumor cells that express laminin receptors by facilitating the synthesis of laminin-1, which has been proven to be an efficient chemoattractant (71). Additionally, POSTN and lysyl oxidase-like 1 (LOXL1) overexpressed by CAFs degrade the basement membrane and stromal ECM and initiate the invasion of malignant tumors. Researchers have found that POSTN-expressing CAFs can impair lymphatic endothelial barriers by activating the integrin-FAK/Src/VE-cadherin signaling pathway in lymphatic endothelial cells. The impaired lymphatic endothelial barrier facilitates tumor cell intravasation and transendothelial migration, consequently promoting lymphatic metastasis in CC. Consistently, high stromal POSTN expression is closely associated with lymph node metastasis and low overall survival in CC patients (39). Overall, the abnormal metabolism of ECM components results in disturbed ECM homeostasis in the TME, which promotes stromal reconstruction and influences the infiltration of inflammatory components. Then, active ECM remodeling in the TME offers the necessary prerequisites for local invasion and distant metastasis by clearing the physiological barrier. In summary, the above five characteristics of the TME may promote lymphatic metastasis of CC in different but integrative ways.
Diverse cells and intercellular interactions in the TME
With further investigation of tumor metastasis, the crucial role of the TME has gradually been revealed. Primary tumor-suppressive immune cells can be converted into tumor-promoting cells in the TME. Immune cells display significant diversity and plasticity in response to stimulatory or suppressive cytokines (77). These immune cells, including T cells, B cells, TAMs, and MDSCs that undergo phenotypic changes can exert immunosuppressive effects through their surface receptors and released cytokines, creating a tolerogenic microenvironment in the tumor-draining lymph nodes that allows tumors to grow and metastasize (12) (Figure 2).
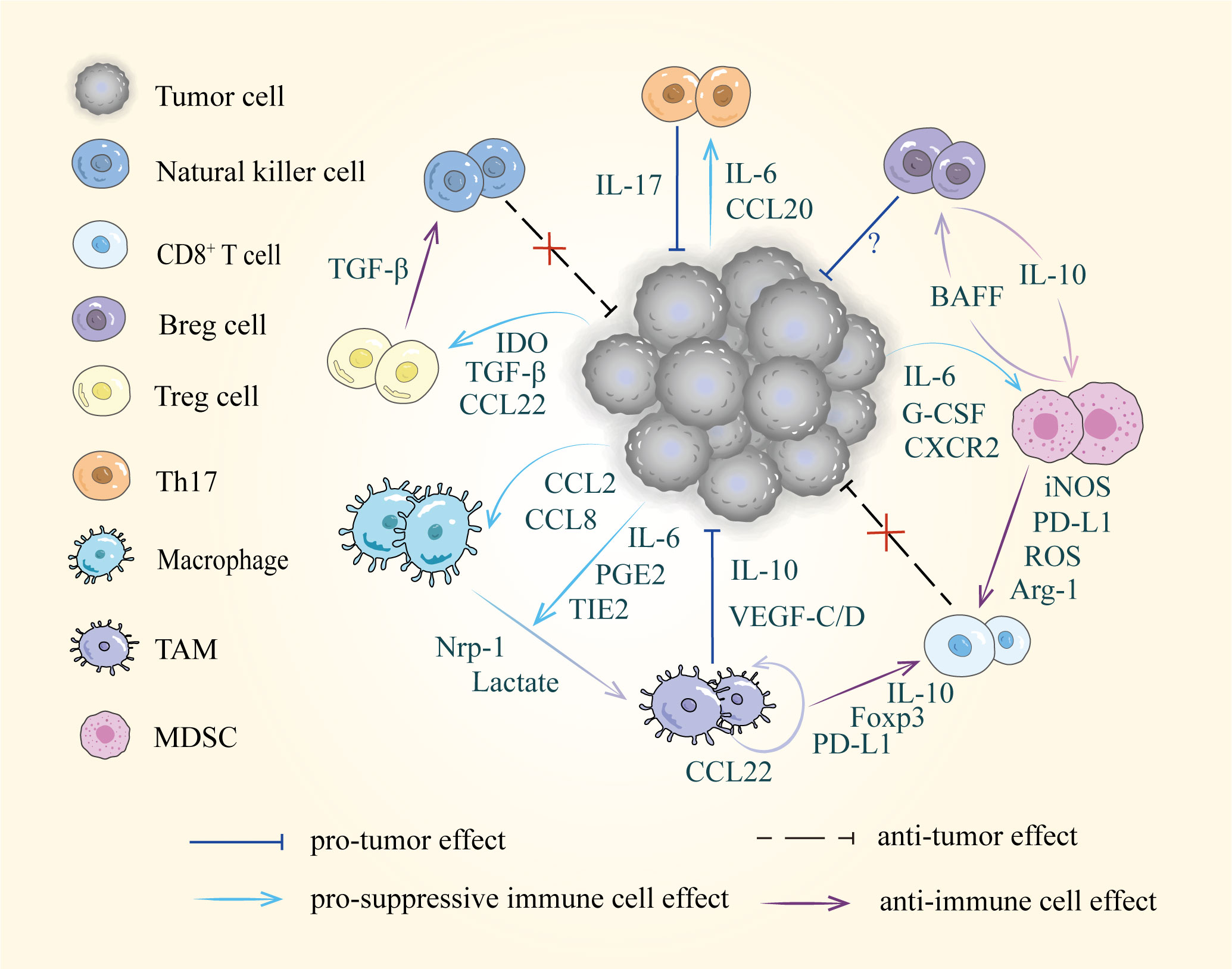
Figure 2 Diverse cells and intercellular interactions in the TME. Tumor cells recruit suppressive immune cells via released factors including cytokines, chemokines, and metabolic products, such as IL-6, CCL22 and IDO etc. Furthermore, these pro-tumor cells disrupt immune surveillance by suppressing the activity of natural killer cells and CD8+ T cells.
T cells
Cellular immunity mediated by T lymphocytes is the cornerstone of antitumor immunity. However, tumor progression and metastasis are usually accompanied by a remarkably compromised antitumor response. The immunosuppressive TME driven by the close interactions between cancer cells and T lymphocytes contributes to tumor progression and forms a barrier that triggers resistance to immunotherapy. As mentioned before, tumor cells not only block the normal function of effector immune cells but also recruit suppressive lymphocytes. The metastatic lymph nodes in CC patients are characterized by the accumulation of PD-L1+ CD14+ antigen-presenting cells (APCs), FOXP3+ Treg cells, and PD-L1+ myeloid cells (37, 78). Furthermore, both CC cells and APCs at the invasive front express indoleamine 2,3-dioxygenase (IDO), which can recruit FOXP3+ Treg cells (79, 80). Tumors also promote Treg cell expansion by secreting exosomes that carry abundant signaling molecules, such as TGF-β, cyclic GMP-AMP synthase, and 2’-3’-cGAMP. Then, these exosomes activate the T cell-intrinsic stimulator of interferon genes (STING) signaling, resulting in immune suppression and poor outcomes in CC patients (81). In addition, the level of Th17 cells gradually increases with tumor progression, which is associated with an advanced clinical stage and lymph node metastases (82, 83). Mechanistically, CC cells indirectly support Th17 cell recruitment by instructing fibroblasts to produce high levels of CCL20 via the CCAAT/enhancer-binding protein β pathway (31). Besides, tumor-derived interleukin 6 (IL-6) supports IL23-mediated Th17 cell expansion (84).
In addition to aiding the immune evasion of tumor cells, immunosuppressive lymphocytes promote tumor proliferation and aggression. For instance, Th17 cells can promote angiogenesis, tumor proliferation, and invasion by secreting IL-17 and activating the NF-κB signaling pathway (85). Treg cells obtained from CC patients were shown to suppress the immune function of natural killer cells through the TGF-β pathway in vitro (86). Collectively, the close interactions between tumors and T cells result in a suppressive or even protumor immune state.
B cells
Compared with T cells, the role of B cells in antitumor immunity remains poorly understood. B cells are principally known for their involvement in humoral immune responses. However, B cells from primary tumors are functionally different from those in the lymph node. In CC tumor-bearing mice, the percentage of B cells in the draining lymph nodes progressively increased with tumor growth. But there were few B cells in the primary tumor, and most of the tumor-infiltrating lymphocytes were T cells. These accumulated B cells were characterized by the overexpression of PD-L1 and the downregulation of MHC II molecules and participated in establishing an immunosuppressive TME. After being shaped by the microenvironment of draining lymph nodes, B cells promoted tumor growth in an IL-10-independent manner (87). More recently, studies on CC patients showed that elevated CD19+ CD5+ CD1d+ Breg cell levels were associated with lymph node metastasis and might be involved in the immunoregulation and inhibition of CD8+ T cells. In vitro, after coculture with Breg cells, the amounts of perforin and granzyme B secreted by CD8+ T cells were significantly decreased (88). Conversely, other studies have revealed an antitumorigenic role for B cells in HPV-related cervical squamous cell carcinomas and a remarkably beneficial impact on patient outcomes (89). Overall, B cells in the TME may possess dual functions. Specifically, B cells within the lymph nodes have potential immunoregulatory properties, and the ones in the primary CC tissue may play a key role in tumor control. However, due to the small amounts of B cells in the primary tumor, they exert limited antitumor effects.
TAMs
In the TME, tumors actively recruit macrophages in various ways. For instance, CCL2 expressed by CC cells is associated with TAMs at the tumor site (90). Similarly, CCL8 derived from cancer cells attracts macrophages by binding to the receptor C-C chemokine receptor 2 (CCR2) on macrophages, which is related to the progression of CC. Notably, in the process of TAM migration, the hypoxia-induced transcription factor ZEB1 activates the transcription of CCL8, which is more important than CCL2 and macrophage colony-stimulating factor (M-CSF) (91). Macrophages possess a certain degree of plasticity that is regulated by microenvironmental stimulation. CC cells induce the polarization of macrophages into M2-like TAMs, which produce smaller amounts of proinflammatory cytokines, such as tumor necrosis factor α (TNF-α), IL-1β, IL-6, and nitrogen oxide (NO), but greater amounts of anti-inflammatory cytokines, such as IL-10 (92, 93). Tumor cells induce M2 polarization in macrophages by secreting various cytokines and growth factors. A blocking study revealed that M2 differentiation was caused by tumor-produced prostaglandin E2 (PGE2) and IL-6. However, TGF-β, IL-10, VEGF, and M-CSF did not play a role (94). Recently, research has confirmed that the nuclear factor of activated T cell 1 (NFATc1), a member of the Wnt family, is highly expressed in the CC microenvironment (95). It promotes M2 polarization of TAMs by regulating IL-10 secretion mediated by the c-myc/PKM2 pathway, thereby promoting tumor growth, invasion, and metastasis (96). Moreover, TAMs can promote their polarization toward M2a macrophages by secreting CCL22 in CC (97). In addition to the abovementioned mechanisms, tumor cells modulate metabolites to induce TAMs. For example, neuropilin-1 (Nrp-1) accumulation in the hypoxic TME educates recruited macrophages to polarize into the M2-phenotype (25). Lactate secreted by CC cells mediates crosstalk between tumor cells and macrophages, which promotes the secretion of IL-1, IL-10, and IL-6, and upregulates the expression of hypoxia-induced factor-1, further promoting a suppressive phenotype (98). Moreover, researchers have observed a direct transfer of tyrosine kinase with immunoglobulin and epidermal growth factor homology domains 2 (TIE2) proteins from TIE2-high CC cells to monocytes and macrophages via exosomes, which promoted increased infiltration of M2-like TAMs (99). All these findings suggest that CC cells in the TME actively recruit normal macrophages and induce them to serve as immunosuppressive TAMs by secreting various cytokines and manipulating metabolites.
Under the influence of tumor-derived factors, the TME is dominated by M2-like TAMs (30). Furthermore, TAMs modulate the immune response to facilitate tumor growth and progression. M2-like macrophages from HPV16-associated tumor-bearing mice were shown to induce a regulatory phenotype in CD8+ T lymphocytes by expressing IL-10 and Foxp3 (30). TAMs also recruit Treg cells by secreting the chemokine CCL22, and high expression of CCL22 is associated with poor outcomes in CC patients (80). PD-L1+ TAMs, which have been identified in different histological subtypes of CC, exert immunosuppressive effects by binding to PD-1 expressed on T lymphocytes (100). Moreover, studies have demonstrated that increased levels of macrophages in the tumor stroma are significantly associated with peritumoral lymphangiogenesis and lymphatic metastasis in CC. TAMs induce lymphangiogenesis by increasing the production of VEGF-C/D and VEGF-A, which stimulate the division of preexisting lymphatic endothelial cells (26, 101). Recently, a novel metastasis-promoting lymphatic pattern was found in CC, wherein TAMs encapsulated lymphatic vessels to form an interconnected network. After the formation of this pattern, the lymphatic vessels acquired an advantageous metastatic capacity. TAMs adjacent to lymphatic vessels could secrete IL-10 within the hypoxic TME, which increased the expression of Sp1 in LECs. As a transcriptional regulator in LECs that contributes to CC metastasis, Sp1 promoted the transactivation of CCL1 to facilitate TAMs and tumor cell recruitment further, forming a positive feedback loop to strengthen the pattern (102). In short, accumulated TAMs not only cooperate with CC cells to develop an immunosuppressive TME but also participate in lymphatic metastasis by inducing lymphangiogenesis.
MDSCs
MDSCs are a group of heterogeneous immature myeloid cells with blocked maturation in cancer. They are one of the main driving forces of immunosuppressive TME (16). According to differences in phenotype and morphology, these cells can be divided into two subsets: granulocytic MDSCs (G-MDSCs), which resemble neutrophils, and monocytic MDSCs (Mo-MDSCs), which are similar to monocytes (103). An increased number of MDSCs in CC patients was first demonstrated in 2014 (104). Since then, a growing number of reports have indicated that the number of MDSCs, especially G-MDSCs, is significantly increased in the peripheral blood (35, 105–107), lymph nodes (36, 78), and tumor tissues of CC patients (108–110), which is associated with visceral or lymph node metastases (35, 78). In mouse models, MDSC depletion by splenectomy or anti-Gr-1-neutralizing antibody administration not only inhibited visceral organ metastasis and premetastatic niche formation but also prolonged the survival of CC-bearing mice (35, 104, 108, 109). In the TME of CC, tumor cells secrete various molecules to recruit MDSCs from immature bone marrow cells, including granulocyte colony-stimulating factor (G-CSF) (35, 36, 107, 108), IL-6 (111), and highly expressed C-X-C chemokine receptor 2 (CXCR2) chemokines, such as CXCL1, CXCL2, CXCL3, CXCL5, and CXCL8 (110).
MDSCs can inhibit T-cell proliferation and cytotoxic function by suppressing IL-2 and interferon γ (IFN-γ) production in CD4+ T cells and IFN-γ production in CD8+ T cells (105, 106, 109). Activated MDSCs secrete immunosuppressive factors, such as inducible nitric oxide synthase (iNOS), reactive oxygen species (ROS), IDO, and arginase I (Arg-1) to inhibit CD8+ T cells (28, 106, 112). It remains unclear by what mechanism and to what degree these factors derived from MDSCs lead to T-cell suppression. However, it is known that these factors lead to abnormal amino acid metabolism and the production of NO, resulting in diminished T-cell proliferation and impaired antigen presentation and recognition by CD8+ T cells (113). Using an HPV-mediated CC mouse model, researchers demonstrated that MDSCs mediated immunosuppressive activity via IL-6/JAK/STAT3 signaling. STAT3 activation mediated by the proinflammatory cytokine IL-6 might be responsible for the expansion of MDSCs, which then accelerated tumor growth (112). Moreover, MDSC-derived IL-6 was reported to be partly involved in stimulating tumor cell proliferation (111, 114). Additionally, MDSCs interact with B lymphocytes in the TME of CC via the B cell activating factor (BAFF) expressed on the surface of MDSCs. By acting on the BAFF receptor expressed by B cells, MDSCs induce B cells to differentiate into Breg cells. Furthermore, IL-10 secreted by Breg cells can promote STAT3 phosphorylation and activate MDSCs, thus establishing a positive feedback loop. The constant differentiation of Breg cells and activation of MDSCs induce an immunosuppressive state and enable tumor immune escape in CC patients (28).
Studies of mouse models have revealed that MDSCs also contribute to premetastatic niche formation (36), tumor angiogenesis (107), and the enhancement of the stem-like properties of cancer cells (108), all of which facilitate tumor metastasis (35). For instance, MDSCs have been reported to enhance the stemness of CC cells by producing PGE2. MDSC depletion inhibited the induction of cancer stem-like cells and enhanced the efficacy of chemotherapy in experimental models of CC (108). MDSCs also stimulate tumor angiogenesis by secreting Bv8, a potent proangiogenic factor (104, 107). In summary, MDSCs utilize several mechanisms to enhance the proliferation and metastasis of CC. After being recruited to the TME, they exert powerful immunosuppressive effects mainly by suppressing T-cell function.
Therapeutic strategies for targeting the TME of cervical cancer
Over the past several years, standard treatments for patients with advanced CC have included radiotherapy and chemotherapy, but their prognosis has been disappointing (115). Therefore, researchers focus on exploring new treatment options, among which immunotherapy is considered promising. The importance of immunotherapy has been highlighted in recent years as further insights into the interactions between tumors and immune cells in the TME have been reported. To date, three immunotherapeutic drugs have been approved by the Food and Drug Administration (FDA) for treating CC: pembrolizumab, tisotumab vedotin, and nivolumab. Furthermore, various immunotherapeutic strategies are being developed to reactivate the antitumor immune responses or remodel the immunosuppressive microenvironment. Therefore, we briefly summarize the progress in therapeutic strategies targeting the TME of CC.
Targeting immune checkpoint molecules
In particular, the upregulation of immune checkpoint molecules in tumor tissues, such as PD-L1 and PD-1, promotes immune escape by downregulating T-cell function. Anti-PD-1 therapies have been demonstrated to enhance the disease-free progression and survival rates of CC patients (116, 117). Although the FDA has approved several anti-PD-L1 antibodies for tumor immunotherapy, none have yet been approved for treating CC. A clinical trial performed with patients with recurrent or metastatic CC indicated that the anti-PD-L1 monoclonal antibody (mAb) socazolimab has durable safety and efficacy (118). Several other trials have demonstrated the therapeutic benefit of immune checkpoint inhibitors (ICIs) in CC, as reviewed in (119, 120). However, ICI monotherapy in CC yields limited durable responses. A few relevant clinical trials are ongoing to find more effective therapies or combination regimens. Recently, a bifunctional agent bintrafusp α was developed to target PD-L1 and TGF-β simultaneously, and one CC patient demonstrated a durable complete response in this phase I clinical trial (121). Rather than solely targeting immunosuppression in the TME or enhancing effector immune cells, combining multiple strategies may achieve better outcomes. The preliminary data of a phase I clinical trial combining an anti-PD-L1 mAb (durvalumab) with anti-cytotoxic T-lymphocyte associated protein 4 (CTLA4) (tremelimumab) and metronomic chemotherapy reported that one CC patient achieved a complete response and four patients achieved stable disease, and a phase II trial is currently ongoing (122). Except for the PD-1/PD-L1 axis and CTLA4, other therapeutic antibodies aiming to activate T cells and eliminate the inhibition of T-cell activation are being tested. For example, single-agent human agonistic mAbs specific for OX40 and 4-1BB, such as ivuxolimab or utomilumab, have demonstrated safety and preliminary antitumor activity in CC patients. OX40 and 4-1BB belong to the tumor necrosis factor receptor superfamily and play important roles in T-cell activation, proliferation, and survival. Costimulating these molecules induces clonal expansion of CD4+ and CD8+ T cells, as well as increased cytotoxicity of T cells (123). Nevertheless, no such treatment has yet been approved for application in CC.
Targeting suppressive immune cells
Due to the existence of suppressive immune cells in the TME, compromised efficacy or resistance to ICI therapy may occur. Therefore, therapeutic approaches targeting suppressive immune cells, such as MDSCs and TAMs, are being tested for their capacity to improve sensitivity to ICIs. In fact, the antitumor effects of anti-PD-1 therapy are enhanced by inhibiting the main chemokine receptor CXCR2 that recruits MDSCs in human pancreatic cancer (124). Research on CC also indicates that treatment with a CXCR2 antagonist weakens the proliferation and migration of CC cells (125). Additionally, the approaches targeting the CSF-1/CSF-1R axis of TAMs are being tested in mouse models. CSF-1R inhibition attenuates the turnover rate of TAMs while increasing the number of CD8+ T cells that infiltrate tumor tissue (126). Although these are results from only cellular and animal experiments, they bring forward new ideas for the treatment of CC. As mentioned before, various metabolites derived from suppressive immune cells, such as Arg-1 and IDO, contribute to the impaired function of effector T cells. Thus. limiting certain metabolites holds promise. Recently, the arginase inhibitor INCB001158 was tested in a clinical trial for metastatic solid tumors treatment (NCT02903914). Another immunosuppressive metabolite, IDO, within the TME is also speculated to be a worthy target for intervention. Treating IL-6 knockout mice with IDO inhibitors was shown to inhibit IDO expression. Furthermore, combination therapy with a therapeutic vaccine resulted in decreased intratumoral polymorphonuclear MDSCs and Treg cells, supporting IL-6 and IDO as immune-metabolic adjuvants for immunotherapies against CC (127). Considering this preclinical research data, it appears that targeting the suppressive immune cells and metabolites within the TME can be used in combination therapies to boost tumor-specific immunity, further improving the therapeutic antitumor effect.
Anti-lymphangiogenesis and anti-inflammatory therapy
Currently, chemotherapy in combination with bevacizumab, an antiangiogenic molecule, is the first-line therapy for recurrent or metastatic CC. In contrast, the search for a therapeutic agent that explicitly inhibits lymphangiogenesis has yet to achieve a breakthrough. As lymphangiogenesis and lymphatic remodeling are functionally important in tumor progression, they represent potential targets for treating metastatic CC. The VEGF-C/VEGFR-3 signaling axis is the most prominent and specific pathway that induces tumor lymphangiogenesis (14, 26, 38, 41). Blocking VEGF-C/VEGFR-3 has been shown to reduce tumor lymphangiogenesis and metastasis in experimental models (128). However, due to the scarcity of relevant studies in CC, the exact effects of targeting lymphangiogenesis are still unclear, and more research is needed.
Since chronic inflammation is another essential contributor to tumorigenesis and metastasis, anti-inflammatory drugs may be a promising tool. The combined effect of nonsteroidal anti-inflammatory drugs with chemotherapy and radiotherapy has increased sensitivity in patients with locally advanced CC. Therapeutic targeting of the COX/PGE2 axis in CC has been reviewed (129). In addition, several anti-inflammatory drug candidates can potentially be therapeutic agents for CC, but further experimental validations are needed (130). Currently, lymphangiogenesis and inflammation-inhibiting therapies are only used as supplements to chemoradiotherapy and immunotherapy. The interactions of various cells in the TME are so complicated that the effect of any single therapy is limited. An increasing number of clinical trials are focusing on novel combination therapies, which are expected to significantly improve the outcome and prognosis of patients with recurrent or metastatic CC.
Nanomaterial-based photodynamic therapy
Nanomaterial-based photodynamic therapy (PDT) has emerged as a novel noninvasive and highly selective treatment for cancer. Photosensitizers enriched in tumor sites are activated by light to sensitize endogenous molecular oxygen and generate cytotoxic ROS, which induces apoptosis or necrosis in tumor cells. However, PDT efficiency is significantly limited because ROS-mediated therapy heavily depends on O2, while TME is hypoxic. A novel nanoagent, copper ferrite nanospheres (CFNs), has been reported to exhibit enhanced ROS production. CFNs reduce the hypoxia and antioxidant activity of tumors by catalyzing the high content of H2O2 in the TME to produce O2 and consume glutathione, improving the effects of PDT. Moreover, CFNs have shown considerable anticancer effects in vitro and in vivo. After treating xenograft CC cell (U14) tumor models with CFNs, tumor tissues were damaged, and tumor growth was apparently inhibited (131). Recently, an oxygen-independent supramolecular photodynamic agent was developed to oxidize water to generate highly cytotoxic hydroxyl radicals directly. This agent exhibited superb photocytotoxicity even under severely hypoxic environments and excellent antitumor efficacy in HeLa tumor-bearing mouse models (132).
In addition to nanoparticles that kill cancer cells via ROS, other nanoparticles with different functions have been developed. A synthetic therapeutic antibody based on molecularly imprinted nanoparticles (MIP-NPs) has been designed to abrogate cell-cell adhesion. The abolishment of cell adhesion by these antibodies inhibits primary tumor growth and metastatic progression, although the mechanism is not completely understood. Remarkably, when applied to CC (HeLa) cells, MIP-NPs disrupted preformed tumor spheroids and inhibited cancer cell invasion in vitro. Moreover, the dense ECM in the TME makes it difficult for drugs to penetrate tumors. MIPs, an antibody against cadherins, can help to loosen cells to allow more effective drug penetration (133). Because of the high tumor interstitial pressure (TIP), delivering nanodrugs to tumors can be challenging. Considering this situation, researchers fabricated the photocatalytic drug AWS@M, which effectively reduced TIP levels, to enhance intratumoral drug delivery and inhibit tumor growth in U14 tumor-bearing mice (134). Nanomaterials are a prospective agent in tumor therapy, with new nanoagents constantly being developed to achieve excellent biocompatibility and higher selectivity. The combination of multiple approaches, including immunotherapy, targeted therapy, and PDT, is expected to improve tumor-targeting efficiency and capability and bring hope to cancer patients.
Conclusion and future perspectives
Over the past decade, the field has reached a consensus on the critical roles of the TME in tumor progression and metastasis. Generally, CC cells actively recruit immunosuppressive cells, such as Th17 cells, Treg cells, TAMs, and MDSCs, into the TME. These cells hamper immunosurveillance and weaken therapeutic effects by participating in multiple pathological processes. Recent studies have shown that tumor-derived exosomes mediate nonrandom dissemination patterns by biasing metastasis to different target organs due to their affinity for specific recipient cells. However, evidence in CC is still scarce, and it is difficult to determine how CC cells preferentially metastasize to the lymph nodes. Many significant issues regarding premetastatic niche formation, dynamics, and lymphangiogenesis in CC remain unanswered. Regarding the mechanism research of tumor-immune cell interactions involved in the lymphatic metastasis of CC, some limitations must be addressed. First, most preclinical studies have not specifically tested metastatic CC or with lymphatic metastasis as an end-point. Of note, most CC-related tumor biology studies have explored only the effects of certain types of immune cells in tumor progression. An in-depth exploration of the underlying mechanisms is still needed. Second, the degree to which lymphangiogenesis directly contributes to lymphatic metastasis and the mechanism through which this occurs needs to be defined. Third, due to the significant heterogeneity among tumor cells and immune cells in the TME, as well as the multidirectional and pleiotropic effects of cytokines and growth factors, it is difficult to determine the roles played by individual immune cell subsets in the overall tumor progression process.
In summary, a part of the complex interactions between tumors and various immune cells has been gradually unveiled, but a large part remains unknown, and more research is needed. A better understanding of the mechanisms driving tumor lymphatic metastasis will contribute to more precise targeting of vital pathways or molecules, enabling better clinical outcomes for CC patients.
Author contributions
MW conceived the concept and made the final approval of manuscript. YL investigated literatures and wrote the manuscript. XG, YH, and BW revised the manuscript. XZ, QZ, LX, and YC designed the figures. All authors listed made substantial, direct intellectual contributions to the work, and approved it for publication. All authors contributed to the article and approved the submitted version.
Funding
This work was supported by the National Key R & D Program (the 14th Five-Year Plan) (No. 2022YFC2704000).
Acknowledgments
The authors thank all the reviewers for their constructive evaluations which have improved this manuscript.
Conflict of interest
The authors declare that the research was conducted in the absence of any commercial or financial relationships that could be construed as a potential conflict of interest.
Publisher’s note
All claims expressed in this article are solely those of the authors and do not necessarily represent those of their affiliated organizations, or those of the publisher, the editors and the reviewers. Any product that may be evaluated in this article, or claim that may be made by its manufacturer, is not guaranteed or endorsed by the publisher.
References
1. Sung H, Ferlay J, Siegel RL, Laversanne M, Soerjomataram I, Jemal A, et al. Global cancer statistics 2020: GLOBOCAN estimates of incidence and mortality worldwide for 36 cancers in 185 countries. CA Cancer J Clin (2021) 71:209–49. doi: 10.3322/caac.21660
2. Zhou C, Wei W, Ma J, Yang Y, Liang L, Zhang Y, et al. Cancer-secreted exosomal miR-1468-5p promotes tumor immune escape via the immunosuppressive reprogramming of lymphatic vessels. Mol Ther (2021) 29:1512–28. doi: 10.1016/j.ymthe.2020.12.034
3. Bray F, Ferlay J, Soerjomataram I, Siegel RL, Torre LA, Jemal A. Global cancer statistics 2018: GLOBOCAN estimates of incidence and mortality worldwide for 36 cancers in 185 countries. CA Cancer J Clin (2018) 68:394–424. doi: 10.3322/caac.21492
4. Li H, Wu X, Cheng X. Advances in diagnosis and treatment of metastatic cervical cancer. J Gynecol Oncol (2016) 27:e43. doi: 10.3802/jgo.2016.27.e43
5. Peng J, Liu X, Li C, Gao M, Wang H. Sema4C modulates the migration of primary tumor−associated lymphatic endothelial cells via an ERK−mediated pathway. Exp Ther Med (2021) 22:1102. doi: 10.3892/etm.2021.10535
6. Huang B, Fang F. Progress in the study of lymph node metastasis in early-stage cervical cancer. Curr Med Sci (2018) 38:567–74. doi: 10.1007/s11596-018-1915-0
7. Wei J-C, Yang J, Liu D, Wu M-F, Qiao L, Wang J-N, et al. Tumor-associated lymphatic endothelial cells promote lymphatic metastasis by highly expressing and secreting SEMA4C. Clin Cancer Res (2017) 23:214–24. doi: 10.1158/1078-0432.CCR-16-0741
8. Karaman S, Detmar M. Mechanisms of lymphatic metastasis. J Clin Invest (2014) 124:922–8. doi: 10.1172/JCI71606
9. Peña-Romero AC, Orenes-Piñero E. Dual effect of immune cells within tumour microenvironment: pro- and anti-tumour effects and their triggers. Cancers (Basel) (2022) 14:1681. doi: 10.3390/cancers14071681
10. Hinshaw DC, Shevde LA. The tumor microenvironment innately modulates cancer progression. Cancer Res (2019) 79:4557–66. doi: 10.1158/0008-5472.CAN-18-3962
11. Anderson NM, Simon MC. The tumor microenvironment. Curr Biol (2020) 30:R921–5. doi: 10.1016/j.cub.2020.06.081
12. Smith HA, Kang Y. The metastasis-promoting roles of tumor-associated immune cells. J Mol Med (2013) 91:411–29. doi: 10.1007/s00109-013-1021-5
13. Cao Y. Emerging mechanisms of tumour lymphangiogenesis and lymphatic metastasis. Nat Rev Cancer (2005) 5:735–43. doi: 10.1038/nrc1693
14. Dieterich LC, Detmar M. Tumor lymphangiogenesis and new drug development. Adv Drug Delivery Rev (2016) 99:148–60. doi: 10.1016/j.addr.2015.12.011
15. Balsat C, Blacher S, Herfs M, Van de Velde M, Signolle N, Sauthier P, et al. A specific immune and lymphatic profile characterizes the pre-metastatic state of the sentinel lymph node in patients with early cervical cancer. Oncoimmunology (2017) 6:e1265718. doi: 10.1080/2162402X.2016.1265718
16. Mabuchi S, Sasano T. Myeloid-derived suppressor cells as therapeutic targets in uterine cervical and endometrial cancers. Cells (2021) 10:1073. doi: 10.3390/cells10051073
17. Cicchini L, Westrich JA, Xu T, Vermeer DW, Berger JN, Clambey ET, et al. Suppression of antitumor immune responses by human papillomavirus through epigenetic downregulation of CXCL14. mBio (2016) 7:e00270–16. doi: 10.1128/mBio.00270-16
18. Hasan UA, Zannetti C, Parroche P, Goutagny N, Malfroy M, Roblot G, et al. The human papillomavirus type 16 E7 oncoprotein induces a transcriptional repressor complex on the toll-like receptor 9 promoter. J Exp Med (2013) 210:1369–87. doi: 10.1084/jem.20122394
19. Georgopoulos NT, Proffitt JL, Blair GE. Transcriptional regulation of the major histocompatibility complex (MHC) class I heavy chain, TAP1 and LMP2 genes by the human papillomavirus (HPV) type 6b, 16 and 18 E7 oncoproteins. Oncogene (2000) 19:4930–5. doi: 10.1038/sj.onc.1203860
20. Piersma SJ, Jordanova ES, van Poelgeest MIE, Kwappenberg KMC, van der Hulst JM, Drijfhout JW, et al. High number of intraepithelial CD8+ tumor-infiltrating lymphocytes is associated with the absence of lymph node metastases in patients with Large early-stage cervical cancer. Cancer Res (2007) 67:354–61. doi: 10.1158/0008-5472.CAN-06-3388
21. Battaglia A, Buzzonetti A, Baranello C, Ferrandina G, Martinelli E, Fanfani F, et al. Metastatic tumour cells favour the generation of a tolerogenic milieu in tumour draining lymph node in patients with early cervical cancer. Cancer Immunol Immunother (2009) 58:1363–73. doi: 10.1007/s00262-008-0646-7
22. Li C, Hua K. Dissecting the single-cell transcriptome network of immune environment underlying cervical premalignant lesion, cervical cancer and metastatic lymph nodes. Front Immunol (2022) 13:897366. doi: 10.3389/fimmu.2022.897366
23. Gu M, He T, Yuan Y, Duan S, Li X, Shen C. Single-cell RNA sequencing reveals multiple pathways and the tumor microenvironment could lead to chemotherapy resistance in cervical cancer. Front Oncol (2021) 11:753386. doi: 10.3389/fonc.2021.753386
24. Guo F, Kong W, Zhao G, Cheng Z, Ai L, Lv J, et al. The correlation between tumor-associated macrophage infiltration and progression in cervical carcinoma. Biosci Rep (2021) 41:BSR20203145. doi: 10.1042/BSR20203145
25. Chen X-J, Wu S, Yan R-M, Fan L-S, Yu L, Zhang Y-M, et al. The role of the hypoxia-Nrp-1 axis in the activation of M2-like tumor-associated macrophages in the tumor microenvironment of cervical cancer. Mol Carcinog (2019) 58:388–97. doi: 10.1002/mc.22936
26. Ding H, Cai J, Mao M, Fang Y, Huang Z, Jia J, et al. Tumor-associated macrophages induce lymphangiogenesis in cervical cancer via interaction with tumor cells. APMIS (2014) 122:1059–69. doi: 10.1111/apm.12257
27. Liang Y, Wang W, Zhu X, Yu M, Zhou C. Inhibition of myeloid-derived suppressive cell function with all-trans retinoic acid enhanced anti-PD-L1 efficacy in cervical cancer. Sci Rep (2022) 12:9619. doi: 10.1038/s41598-022-13855-1
28. Jianyi D, Haili G, Bo Y, Meiqin Y, Baoyou H, Haoran H, et al. Myeloid-derived suppressor cells cross-talk with B10 cells by BAFF/BAFF-r pathway to promote immunosuppression in cervical cancer. Cancer Immunol Immunother (2022) 72:87–9. doi: 10.1007/s00262-022-03226-0
29. Fleming V, Hu X, Weber R, Nagibin V, Groth C, Altevogt P, et al. Targeting myeloid-derived suppressor cells to bypass tumor-induced immunosuppression. Front Immunol (2018) 9:398. doi: 10.3389/fimmu.2018.00398
30. Lepique AP, Daghastanli KRP, Cuccovia IM, Villa LL. HPV16 tumor associated macrophages suppress antitumor T cell responses. Clin Cancer Res (2009) 15:4391–400. doi: 10.1158/1078-0432.CCR-09-0489
31. Walch-Rückheim B, Mavrova R, Henning M, Vicinus B, Kim Y-J, Bohle RM, et al. Stromal fibroblasts induce CCL20 through IL6/C/EBPβ to support the recruitment of Th17 cells during cervical cancer progression. Cancer Res (2015) 75:5248–59. doi: 10.1158/0008-5472.CAN-15-0732
32. Peinado H, Zhang H, Matei IR, Costa-Silva B, Hoshino A, Rodrigues G, et al. Pre-metastatic niches: organ-specific homes for metastases. Nat Rev Cancer (2017) 17:302–17. doi: 10.1038/nrc.2017.6
33. Liu Y, Cao X. Characteristics and significance of the pre-metastatic niche. Cancer Cell (2016) 30:668–81. doi: 10.1016/j.ccell.2016.09.011
34. Kitamura T, Qian B-Z, Pollard JW. Immune cell promotion of metastasis. Nat Rev Immunol (2015) 15:73–86. doi: 10.1038/nri3789
35. Sasano T, Mabuchi S, Kozasa K, Kuroda H, Kawano M, Takahashi R, et al. The highly metastatic nature of uterine Cervical/Endometrial cancer displaying tumor-related leukocytosis: clinical and preclinical investigations. Clin Cancer Res (2018) 24:4018–29. doi: 10.1158/1078-0432.CCR-17-2472
36. Mabuchi S, Komura N, Sasano T, Shimura K, Yokoi E, Kozasa K, et al. Pretreatment tumor-related leukocytosis misleads positron emission tomography-computed tomography during lymph node staging in gynecological malignancies. Nat Commun (2020) 11:1364. doi: 10.1038/s41467-020-15186-z
37. Heeren AM, de Boer E, Bleeker MCG, Musters RJP, Buist MR, Kenter GG, et al. Nodal metastasis in cervical cancer occurs in clearly delineated fields of immune suppression in the pelvic lymph catchment area. Oncotarget (2015) 6:32484–93. doi: 10.18632/oncotarget.5398
38. Gillot L, Lebeau A, Baudin L, Pottier C, Louis T, Durré T, et al. Periostin in lymph node pre-metastatic niches governs lymphatic endothelial cell functions and metastatic colonization. Cell Mol Life Sci (2022) 79:295. doi: 10.1007/s00018-022-04262-w
39. Wei W, Chen X, Liang L, Yu L, Wu X, Zhou C, et al. Periostin + cancer-associated fibroblasts promote lymph node metastasis by impairing the lymphatic endothelial barriers in cervical squamous cell carcinoma. Mol Oncol (2021) 15:210–27. doi: 10.1002/1878-0261.12837
40. Zhou C-F, Ma J, Huang L, Yi H-Y, Zhang Y-M, Wu X-G, et al. Cervical squamous cell carcinoma-secreted exosomal miR-221-3p promotes lymphangiogenesis and lymphatic metastasis by targeting VASH1. Oncogene (2019) 38:1256–68. doi: 10.1038/s41388-018-0511-x
41. Liu H, Xiao J, Yang Y, Liu Y, Ma R, Li Y, et al. COX-2 expression is correlated with VEGF-c, lymphangiogenesis and lymph node metastasis in human cervical cancer. Microvasc Res (2011) 82:131–40. doi: 10.1016/j.mvr.2011.04.011
42. Secker GA, Harvey NL. VEGFR signaling during lymphatic vascular development: from progenitor cells to functional vessels: VEGFR signaling during lymphatic vascular development. Dev Dyn (2015) 244:323–31. doi: 10.1002/dvdy.24227
43. Huang Yw, Zhou Y, Lan C-Y, Wang Y, Feng Y-L, Luo R-Z, et al. Tumor-induced VEGF-c overexpression in retroperitoneal lymph nodes in VX2 carcinoma-bearing rabbits. Drug Des Devel Ther (2015) 9:5949–56. doi: 10.2147/DDDT.S89810
44. Wu H, Song S, Yan A, Guo X, Chang L, Xu L, et al. RACK1 promotes the invasive activities and lymph node metastasis of cervical cancer via galectin-1. Cancer Lett (2020) 469:287–300. doi: 10.1016/j.canlet.2019.11.002
45. Williams SP, Odell AF, Karnezis T, Farnsworth RH, Gould CM, Li J, et al. Genome-wide functional analysis reveals central signaling regulators of lymphatic endothelial cell migration and remodeling. Sci Signal (2017) 10:eaal2987. doi: 10.1126/scisignal.aal2987
46. Xu L, Li J, Tursun M, Hai Y, Tursun H, Mamtimin B, et al. Receptor for activated c kinase 1 promotes cervical cancer lymph node metastasis via the glycolysis−dependent AKT/mTOR signaling. Int J Oncol (2022) 61:83. doi: 10.3892/ijo.2022.5373
47. Chen J, Qiu J, Li F, Jiang X, Sun X, Zheng L, et al. HN1 promotes tumor associated lymphangiogenesis and lymph node metastasis via NF-κB signaling activation in cervical carcinoma. Biochem Biophys Res Commun (2020) 530:87–94. doi: 10.1016/j.bbrc.2020.05.062
48. Liu D, Li L, Zhang X-X, Wan D-Y, Xi B-X, Hu Z, et al. SIX1 promotes tumor lymphangiogenesis by coordinating TGFβ signals that increase expression of VEGF-c. Cancer Res (2014) 74:5597–607. doi: 10.1158/0008-5472.CAN-13-3598
49. Liu P, Zhang C, Liao Y, Liu J, Huang J, Xia M, et al. High expression of PTPRM predicts poor prognosis and promotes tumor growth and lymph node metastasis in cervical cancer. Cell Death Dis (2020) 11:687. doi: 10.1038/s41419-020-02826-x
50. Tang J, Yang Z, Wang Z, Li Z, Li H, Yin J, et al. Foxp3 is correlated with VEGF-c expression and lymphangiogenesis in cervical cancer. World J Surg Onc (2017) 15:173. doi: 10.1186/s12957-017-1221-5
51. Zeng C, Chen L, Yang Z, Sun S. The close correlation between heparanase and COX-2 expression in lymphangiogenesis of cervical cancer. Med Oncol (2014) 31:314. doi: 10.1007/s12032-014-0314-z
52. Taki M, Abiko K, Ukita M, Murakami R, Yamanoi K, Yamaguchi K, et al. Tumor immune microenvironment during epithelial–mesenchymal transition. Clin Cancer Res (2021) 27:4669–79. doi: 10.1158/1078-0432.CCR-20-4459
53. Zhou Y, Huang Y, Cao X, Xu J, Zhang L, Wang J, et al. WNT2 promotes cervical carcinoma metastasis and induction of epithelial-mesenchymal transition. PloS One (2016) 11:e0160414. doi: 10.1371/journal.pone.0160414
54. Huang Y, Huang H, Li M, Zhang X, Liu Y, Wang Y. MicroRNA-374c-5p regulates the invasion and migration of cervical cancer by acting on the Foxc1/snail pathway. BioMed Pharmacother (2017) 94:1038–47. doi: 10.1016/j.biopha.2017.07.150
55. Wang B, Zhang H, Wei L, Li Y. Expression of dickkopf-1 and Twist2 in cervical squamous cell carcinoma and their correlation with vasculogenic mimicry. J Healthc Eng (2022) 2022:1–8. doi: 10.1155/2022/9288476
56. Wang S, Li J, Xie J, Liu F, Duan Y, Wu Y, et al. Programmed death ligand 1 promotes lymph node metastasis and glucose metabolism in cervical cancer by activating integrin β4/SNAI1/SIRT3 signaling pathway. Oncogene (2018) 37:4164–80. doi: 10.1038/s41388-018-0252-x
57. Ma Y, Zheng X, Zhou J, Zhang Y, Chen K. ZEB1 promotes the progression and metastasis of cervical squamous cell carcinoma via the promotion of epithelial-mesenchymal transition. Int J Clin Exp Pathol (2015) 8:11258–67.
58. Liao Y, Huang J, Liu P, Zhang C, Liu J, Xia M, et al. Downregulation of LNMAS orchestrates partial EMT and immune escape from macrophage phagocytosis to promote lymph node metastasis of cervical cancer. Oncogene (2022) 41:1931–43. doi: 10.1038/s41388-022-02202-3
59. Bakir B, Chiarella AM, Pitarresi JR, Rustgi AK. EMT, MET, plasticity, and tumor metastasis. Trends Cell Biol (2020) 30:764–76. doi: 10.1016/j.tcb.2020.07.003
60. Thiery JP, Acloque H, Huang RYJ, Nieto MA. Epithelial-mesenchymal transitions in development and disease. Cell (2009) 139:871–90. doi: 10.1016/j.cell.2009.11.007
61. Sinha D, Saha P, Samanta A, Bishayee A. Emerging concepts of hybrid epithelial-to-Mesenchymal transition in cancer progression. Biomolecules (2020) 10:1561. doi: 10.3390/biom10111561
62. Saxena K, Jolly MK, Balamurugan K. Hypoxia, partial EMT and collective migration: emerging culprits in metastasis. Transl Oncol (2020) 13:100845. doi: 10.1016/j.tranon.2020.100845
63. Zhang C, Liao Y, Liu P, Du Q, Liang Y, Ooi S, et al. FABP5 promotes lymph node metastasis in cervical cancer by reprogramming fatty acid metabolism. Theranostics (2020) 10:6561–80. doi: 10.7150/thno.44868
64. Wu Y, Gu T-T, Zheng P-S. CIP2A cooperates with h-ras to promote epithelial–mesenchymal transition in cervical-cancer progression. Cancer Lett (2015) 356:646–55. doi: 10.1016/j.canlet.2014.10.013
65. Li H, Zhang W, Yan M, Qiu J, Chen J, Sun X, et al. Nucleolar and spindle associated protein 1 promotes metastasis of cervical carcinoma cells by activating wnt/β-catenin signaling. J Exp Clin Cancer Res (2019) 38:33. doi: 10.1186/s13046-019-1037-y
66. Hu Y, Sun X, Mao C, Guo G, Ye S, Xu J, et al. Upregulation of long noncoding RNA TUG1 promotes cervical cancer cell proliferation and migration. Cancer Med (2017) 6:471–82. doi: 10.1002/cam4.994
67. Zhang L, Zhan X, Yan D, Wang Z. Circulating MicroRNA-21 is involved in lymph node metastasis in cervical cancer by targeting RASA1. Int J Gynecol Cancer (2016) 26:810–6. doi: 10.1097/IGC.0000000000000694
68. Eoh K, Paek J, Kim S, Kim H, Lee H, Lee S, et al. Steroid receptor RNA activator (SRA), induces tumor proliferation and invasion through the NOTCH pathway in cervical cancer cell lines. Oncol Rep (2017) 38:3481–8. doi: 10.3892/or.2017.6023
69. You X, Wang Y, Meng J, Han S, Liu L, Sun Y, et al. Exosomal miR−663b exposed to TGF−β1 promotes cervical cancer metastasis and epithelial−mesenchymal transition by targeting MGAT3. Oncol Rep (2021) 45:12. doi: 10.3892/or.2021.7963
70. Xiao L, Zhu H, Shu J, Gong D, Zheng D, Gao J. Overexpression of TGF-β1 and SDF-1 in cervical cancer-associated fibroblasts promotes cell growth, invasion and migration. Arch Gynecol Obstet (2022) 305:179–92. doi: 10.1007/s00404-021-06137-0
71. Fullár A, Dudás J, Oláh L, Hollósi P, Papp Z, Sobel G, et al. Remodeling of extracellular matrix by normal and tumor-associated fibroblasts promotes cervical cancer progression. BMC Cancer (2015) 15:256. doi: 10.1186/s12885-015-1272-3
72. Kaewprag J, Umnajvijit W, Ngamkham J, Ponglikitmongkol M. HPV16 oncoproteins promote cervical cancer invasiveness by upregulating specific matrix metalloproteinases. PloS One (2013) 8:e71611. doi: 10.1371/journal.pone.0071611
73. Maity G, Sen T, Chatterjee A. Laminin induces matrix metalloproteinase-9 expression and activation in human cervical cancer cell line (SiHa). J Cancer Res Clin Oncol (2011) 137:347–57. doi: 10.1007/s00432-010-0892-x
74. Mao Z, Sang M-M, Chen C, Zhu W-T, Gong Y-S, Pei D. CSN6 promotes the migration and invasion of cervical cancer cells by inhibiting autophagic degradation of cathepsin l. Int J Biol Sci (2019) 15:1310–24. doi: 10.7150/ijbs.32987
75. Arguello-Ramirez J, Perez-Cardenas E, Delgado-Chavez R, Solorza-Luna G, Villa-Trevino S, Arenas-Huertero F. Matrix metalloproteinases-2, -3, and -9 secreted by explants of benign and malignant lesions of the uterine cervix. Int J Gynecol Cancer (2004) 14:333–40. doi: 10.1111/j.1048-891x.2004.014218.x
76. Ou Z, Lin S, Qiu J, Ding W, Ren P, Chen D, et al. Single-nucleus RNA sequencing and spatial transcriptomics reveal the immunological microenvironment of cervical squamous cell carcinoma. Adv Sci (Weinh) (2022) 9:2203040. doi: 10.1002/advs.202203040
77. Galli SJ, Borregaard N, Wynn TA. Phenotypic and functional plasticity of cells of innate immunity: macrophages, mast cells and neutrophils. Nat Immunol (2011) 12:1035–44. doi: 10.1038/ni.2109
78. Heeren AM, Koster BD, Samuels S, Ferns DM, Chondronasiou D, Kenter GG, et al. High and interrelated rates of PD-L1+CD14+ antigen-presenting cells and regulatory T cells mark the microenvironment of metastatic lymph nodes from patients with cervical cancer. Cancer Immunol Res (2015) 3:48–58. doi: 10.1158/2326-6066.CIR-14-0149
79. Nakamura T, Shima T, Saeki A, Hidaka T, Nakashima A, Takikawa O, et al. Expression of indoleamine 2, 3-dioxygenase and the recruitment of Foxp3-expressing regulatory T cells in the development and progression of uterine cervical cancer. Cancer Sci (2007) 98:874–81. doi: 10.1111/j.1349-7006.2007.00470.x
80. Wang Q, Schmoeckel E, Kost BP, Kuhn C, Vattai A, Vilsmaier T, et al. Higher CCL22+ cell infiltration is associated with poor prognosis in cervical cancer patients. Cancers (2019) 11:2004. doi: 10.3390/cancers11122004
81. Ni H, Zhang H, Li L, Huang H, Guo H, Zhang L, et al. T Cell-intrinsic STING signaling promotes regulatory T cell induction and immunosuppression by upregulating FOXP3 transcription in cervical cancer. J Immunother Cancer (2022) 10:e005151. doi: 10.1136/jitc-2022-005151
82. Zhang Y, Ma D, Zhang Y, Tian Y, Wang X, Qiao Y, et al. The imbalance of Th17/Treg in patients with uterine cervical cancer. Clin Chim Acta (2011) 412:894–900. doi: 10.1016/j.cca.2011.01.015
83. Hou F, Li Z, Ma D, Zhang W, Zhang Y, Zhang T, et al. Distribution of Th17 cells and Foxp3-expressing T cells in tumor-infiltrating lymphocytes in patients with uterine cervical cancer. Clin Chim Acta (2012) 413:1848–54. doi: 10.1016/j.cca.2012.07.012
84. Walch-Rückheim B, Ströder R, Theobald L, Pahne-Zeppenfeld J, Hegde S, Kim Y-J, et al. Cervical cancer–instructed stromal fibroblasts enhance IL23 expression in dendritic cells to support expansion of Th17 cells. Cancer Res (2019) 79:1573–86. doi: 10.1158/0008-5472.CAN-18-1913
85. Lv Q, Wu K, Liu F, Wu W, Chen Y, Zhang W. Interleukin−17A and heparanase promote angiogenesis and cell proliferation and invasion in cervical cancer. Int J Oncol (2018) 53:1809–17. doi: 10.3892/ijo.2018.4503
86. Chang W-C, Li C-H, Chu L-H, Huang P-S, Sheu B-C, Huang S-C. Regulatory T cells suppress natural killer cell immunity in patients with human cervical carcinoma. Int J Gynecol Cancer (2016) 26:156–62. doi: 10.1097/IGC.0000000000000578
87. Tang A, Dadaglio G, Oberkampf M, Di Carlo S, Peduto L, Laubreton D, et al. B cells promote tumor progression in a mouse model of HPV-mediated cervical cancer: b cells promote tumor progression in a mouse model of cervical cancer. Int J Cancer (2016) 139:1358–71. doi: 10.1002/ijc.30169
88. Chen Z, Zhu Y, Du R, Pang N, Zhang F, Dong D, et al. Role of regulatory b cells in the progression of cervical cancer. Mediators Inflammation (2019) 2019:6519427. doi: 10.1155/2019/6519427
89. Kim SS, Shen S, Miyauchi S, Sanders PD, Franiak-Pietryga I, Mell L, et al. B cells improve overall survival in HPV-associated squamous cell carcinomas and are activated by radiation and PD-1 blockade. Clin Cancer Res (2020) 26:3345–59. doi: 10.1158/1078-0432.CCR-19-3211
90. Zijlmans HJ, Fleuren GJ, Baelde HJ, Eilers PH, Kenter GG, Gorter A. The absence of CCL2 expression in cervical carcinoma is associated with increased survival and loss of heterozygosity at 17q11.2. J Pathol (2006) 208:507–17. doi: 10.1002/path.1918
91. Chen X-J, Deng Y-R, Wang Z-C, Wei W-F, Zhou C-F, Zhang Y-M, et al. Hypoxia-induced ZEB1 promotes cervical cancer progression via CCL8-dependent tumour-associated macrophage recruitment. Cell Death Dis (2019) 10:508. doi: 10.1038/s41419-019-1748-1
92. Pedraza-Brindis EJ, Sánchez-Reyes K, Hernández-Flores G, Bravo-Cuellar A, Jave-Suárez LF, Aguilar-Lemarroy A, et al. Culture supernatants of cervical cancer cells induce an M2 phenotypic profile in THP-1 macrophages. Cell Immunol (2016) 310:42–52. doi: 10.1016/j.cellimm.2016.07.001
93. Sánchez-Reyes K, Bravo-Cuellar A, Hernández-Flores G, Lerma-Díaz JM, Jave-Suárez LF, Gómez-Lomelí P, et al. Cervical cancer cell supernatants induce a phenotypic switch from U937-derived macrophage-activated M1 state into M2-like suppressor phenotype with change in toll-like receptor profile. BioMed Res Int (2014) 2014:1–11. doi: 10.1155/2014/683068
94. Heusinkveld M, de Vos van Steenwijk PJ, Goedemans R, Ramwadhdoebe TH, Gorter A, Welters MJP, et al. M2 macrophages induced by prostaglandin e 2 and IL-6 from cervical carcinoma are switched to activated M1 macrophages by CD4 + Th1 cells. J Immunol (2011) 187:1157–65. doi: 10.4049/jimmunol.1100889
95. Zhou Y, Liu H, Wang J, Wang X, Qian L, Xu F, et al. ΔNp63α exerts antitumor functions in cervical squamous cell carcinoma. Oncogene (2020) 39:905–21. doi: 10.1038/s41388-019-1033-x
96. Tan J, Yang L, Zhao H, Ai Y, Ren L, Zhang F, et al. The role of NFATc1/c-myc/PKM2/IL-10 axis in activating cervical cancer tumor-associated M2 macrophage polarization to promote cervical cancer progression. Exp Cell Res (2022) 413:113052. doi: 10.1016/j.yexcr.2022.113052
97. Wang Q, Sudan K, Schmoeckel E, Kost BP, Kuhn C, Vattai A, et al. CCL22-polarized TAMs to M2a macrophages in cervical cancer In vitro model. Cells (2022) 11:2027. doi: 10.3390/cells11132027
98. Stone SC, Rossetti RAM, Alvarez KLF, Carvalho JP, Margarido PFR, Baracat EC, et al. Lactate secreted by cervical cancer cells modulates macrophage phenotype. J Leukoc Biol (2019) 105:1041–54. doi: 10.1002/JLB.3A0718-274RR
99. Du S, Qian J, Tan S, Li W, Liu P, Zhao J, et al. Tumor cell-derived exosomes deliver TIE2 protein to macrophages to promote angiogenesis in cervical cancer. Cancer Lett (2022) 529:168–79. doi: 10.1016/j.canlet.2022.01.005
100. Heeren AM, Punt S, Bleeker MC, Gaarenstroom KN, van der Velden J, Kenter GG, et al. Prognostic effect of different PD-L1 expression patterns in squamous cell carcinoma and adenocarcinoma of the cervix. Mod Pathol (2016) 29:753–63. doi: 10.1038/modpathol.2016.64
101. Schoppmann SF, Birner P, Stöckl J, Kalt R, Ullrich R, Caucig C, et al. Tumor-associated macrophages express lymphatic endothelial growth factors and are related to peritumoral lymphangiogenesis. Am J Pathol (2002) 161:947–56. doi: 10.1016/S0002-9440(10)64255-1
102. Chen X-J, Wei W-F, Wang Z-C, Wang N, Guo C-H, Zhou C-F, et al. A novel lymphatic pattern promotes metastasis of cervical cancer in a hypoxic tumour-associated macrophage-dependent manner. Angiogenesis (2021) 24:549–65. doi: 10.1007/s10456-020-09766-2
103. Dou A, Fang J. Heterogeneous myeloid cells in tumors. Cancers (2021) 13:3772. doi: 10.3390/cancers13153772
104. Mabuchi S, Matsumoto Y, Kawano M, Minami K, Seo Y, Sasano T, et al. Uterine cervical cancer displaying tumor-related leukocytosis: a distinct clinical entity with radioresistant feature. J Natl Cancer Inst (2014) 106:dju147–147. doi: 10.1093/jnci/dju147
105. Liang Y, Lü B, Zhao P, Lü W. Increased circulating GrMyeloid-derived suppressor cells correlated with tumor burden and survival in locally advanced cervical cancer patient. J Cancer (2019) 10:1341–8. doi: 10.7150/jca.29647
106. Wu L, Liu H, Guo H, Wu Q, Yu S, Qin Y, et al. Circulating and tumor−infiltrating myeloid−derived suppressor cells in cervical carcinoma patients. Oncol Lett (2018) 15:9507–15. doi: 10.3892/ol.2018.8532
107. Kawano M, Mabuchi S, Matsumoto Y, Sasano T, Takahashi R, Kuroda H, et al. The significance of G-CSF expression and myeloid-derived suppressor cells in the chemoresistance of uterine cervical cancer. Sci Rep (2015) 5:18217. doi: 10.1038/srep18217
108. Kuroda H, Mabuchi S, Yokoi E, Komura N, Kozasa K, Matsumoto Y, et al. Prostaglandin E2 produced by myeloid-derived suppressive cells induces cancer stem cells in uterine cervical cancer. Oncotarget (2018) 9:36317–30. doi: 10.18632/oncotarget.26347
109. Shimura K, Mabuchi S, Komura N, Yokoi E, Kozasa K, Sasano T, et al. Prognostic significance of bone marrow FDG uptake in patients with gynecological cancer. Sci Rep (2021) 11:2257. doi: 10.1038/s41598-021-81298-1
110. Kim KH, Sim NS, Chang JS, Kim YB. Tumor immune microenvironment in cancer patients with leukocytosis. Cancer Immunol Immunother (2020) 69:1265–77. doi: 10.1007/s00262-020-02545-4
111. Lee B-R, Kwon B-E, Hong E-H, Shim A, Song J-H, Kim H-M, et al. Interleukin-10 attenuates tumour growth by inhibiting interleukin-6/signal transducer and activator of transcription 3 signalling in myeloid-derived suppressor cells. Cancer Lett (2016) 381:156–64. doi: 10.1016/j.canlet.2016.07.012
112. Galliverti G, Wullschleger S, Tichet M, Murugan D, Zangger N, Horton W, et al. Myeloid cells orchestrate systemic immunosuppression, impairing the efficacy of immunotherapy against HPV+ cancers. Cancer Immunol Res (2020) 8:131–45. doi: 10.1158/2326-6066.CIR-19-0315
113. Raber PL, Thevenot P, Sierra R, Wyczechowska D, Halle D, Ramirez ME, et al. Subpopulations of myeloid-derived suppressor cells impair T cell responses through independent nitric oxide-related pathways: T cell suppression by MDSC subpopulations in cancer. Int J Cancer (2014) 134:2853–64. doi: 10.1002/ijc.28622
114. Kozasa K, Mabuchi S, Matsumoto Y, Kuroda H, Yokoi E, Komura N, et al. Estrogen stimulates female cancer progression by inducing myeloid-derived suppressive cells: investigations on pregnant and non-pregnant experimental models. Oncotarget (2019) 10:1887–902. doi: 10.18632/oncotarget.26711
115. Kagabu M, Nagasawa T, Sato C, Fukagawa Y, Kawamura H, Tomabechi H, et al. Immunotherapy for uterine cervical cancer using checkpoint inhibitors: future directions. Int J Mol Sci (2020) 21:2335. doi: 10.3390/ijms21072335
116. Colombo N, Dubot C, Lorusso D, Caceres MV, Hasegawa K, Shapira-Frommer R, et al. Pembrolizumab for persistent, recurrent, or metastatic cervical cancer. N Engl J Med (2021) 385:1856–67. doi: 10.1056/NEJMoa2112435
117. Naumann RW, Hollebecque A, Meyer T, Devlin M-J, Oaknin A, Kerger J, et al. Safety and efficacy of nivolumab monotherapy in recurrent or metastatic cervical, vaginal, or vulvar carcinoma: results from the phase I/II CheckMate 358 trial. J Clin Oncol (2019) 37:2825–34. doi: 10.1200/JCO.19.00739
118. An J, Tang J, Li BX, Xiong H, Qiu H, Luo L, et al. Efficacy and safety of the anti-PD-L1 mAb socazolimab for recurrent or metastatic cervical cancer: a phase I dose-escalation and expansion study. Clin Cancer Res (2022) 28:5098–106. doi: 10.1158/1078-0432.CCR-22-1280
119. Ferrall L, Lin KY, Roden RBS, Hung C-F, Wu T-C. Cervical cancer immunotherapy: facts and hopes. Clin Cancer Res (2021) 27:4953–73. doi: 10.1158/1078-0432.CCR-20-2833
120. Maiorano BA, Maiorano MFP, Ciardiello D, Maglione A, Orditura M, Lorusso D, et al. Beyond platinum, ICIs in metastatic cervical cancer: a systematic review. Cancers (Basel) (2022) 14:5955. doi: 10.3390/cancers14235955
121. Lind H, Gameiro SR, Jochems C, Donahue RN, Strauss J, Gulley JL, et al. Dual targeting of TGF-β and PD-L1 via a bifunctional anti-PD-L1/TGF-βRII agent: status of preclinical and clinical advances. J Immunother Cancer (2020) 8:e000433. doi: 10.1136/jitc-2019-000433
122. Vicier C, Isambert N, Cropet C, Hamimed M, Osanno L, Legrand F, et al. MOVIE: a phase I, open-label, multicenter study to evaluate the safety and tolerability of metronomic vinorelbine combined with durvalumab plus tremelimumab in patients with advanced solid tumors. ESMO Open (2022) 7:100646. doi: 10.1016/j.esmoop.2022.100646
123. Hamid O, Chiappori AA, Thompson JA, Doi T, Hu-Lieskovan S, Eskens FALM, et al. First-in-human study of an OX40 (ivuxolimab) and 4-1BB (utomilumab) agonistic antibody combination in patients with advanced solid tumors. J Immunother Cancer (2022) 10:e005471. doi: 10.1136/jitc-2022-005471
124. Steele CW, Karim SA, Leach JDG, Bailey P, Upstill-Goddard R, Rishi L, et al. CXCR2 inhibition profoundly suppresses metastases and augments immunotherapy in pancreatic ductal adenocarcinoma. Cancer Cell (2016) 29:832–45. doi: 10.1016/j.ccell.2016.04.014
125. Sun J, Yuan J. Chemokine (C-X-C motif) ligand 1/chemokine (C-X-C motif) receptor 2 autocrine loop contributes to cellular proliferation, migration and apoptosis in cervical cancer. Bioengineered (2022) 13:7579–91. doi: 10.1080/21655979.2022.2036896
126. Strachan DC, Ruffell B, Oei Y, Bissell MJ, Coussens LM, Pryer N, et al. CSF1R inhibition delays cervical and mammary tumor growth in murine models by attenuating the turnover of tumor-associated macrophages and enhancing infiltration by CD8+ T cells. Oncoimmunology (2013) 2:e26968. doi: 10.4161/onci.26968
127. Pagni RL, Souza P da C, Pegoraro R, Porchia BFMM, da Silva JR, Aps LR de MM, et al. Interleukin-6 and indoleamine-2,3-dioxygenase as potential adjuvant targets for papillomavirus-related tumors immunotherapy. Front Immunol (2022) 13:1005937. doi: 10.3389/fimmu.2022.1005937
128. He Y, Kozaki K-i, Karpanen T, Koshikawa K, Yla-Herttuala S, Takahashi T, et al. Suppression of tumor lymphangiogenesis and lymph node metastasis by blocking vascular endothelial growth factor receptor 3 signaling. J Natl Cancer Inst (2002) 94:819–25. doi: 10.1093/jnci/94.11.819
129. García-Quiroz J, Vázquez-Almazán B, García-Becerra R, Díaz L, Avila E. The interaction of human papillomavirus infection and prostaglandin E2 signaling in carcinogenesis: a focus on cervical cancer therapeutics. Cells (2022) 11:2528. doi: 10.3390/cells11162528
130. Kori M, Arga KY, Mardinoglu A, Turanli B. Repositioning of anti-inflammatory drugs for the treatment of cervical cancer Sub-types. Front Pharmacol (2022) 13:884548. doi: 10.3389/fphar.2022.884548
131. Liu Y, Zhen W, Jin L, Zhang S, Sun G, Zhang T, et al. All-in-One theranostic nanoagent with enhanced reactive oxygen species generation and modulating tumor microenvironment ability for effective tumor eradication. ACS Nano (2018) 12:4886–93. doi: 10.1021/acsnano.8b01893
132. Teng K-X, Niu L-Y, Yang Q-Z. Supramolecular photosensitizer enables oxygen-independent generation of hydroxyl radicals for photodynamic therapy. J Am Chem Soc (2023), jacs.2c11868. doi: 10.1021/jacs.2c11868
133. Medina Rangel PX, Moroni E, Merlier F, Gheber LA, Vago R, Tse Sum Bui B, et al. Chemical antibody mimics inhibit cadherin-mediated cell–cell adhesion: a promising strategy for cancer therapy. Angew Chem Int Ed (2020) 59:2816–22. doi: 10.1002/anie.201910373
Keywords: cervical cancer, tumor microenvironment, immune cells, lymphatic metastasis, lymphangiogenesis
Citation: Li Y, Gao X, Huang Y, Zhu X, Chen Y, Xue L, Zhu Q, Wang B and Wu M (2023) Tumor microenvironment promotes lymphatic metastasis of cervical cancer: its mechanisms and clinical implications. Front. Oncol. 13:1114042. doi: 10.3389/fonc.2023.1114042
Received: 02 December 2022; Accepted: 28 April 2023;
Published: 10 May 2023.
Edited by:
Tiziana Schioppa, University of Brescia, ItalyReviewed by:
Luisa Pedro, The Open University, United KingdomYael Raz Yana, University of California, Los Angeles, United States
Copyright © 2023 Li, Gao, Huang, Zhu, Chen, Xue, Zhu, Wang and Wu. This is an open-access article distributed under the terms of the Creative Commons Attribution License (CC BY). The use, distribution or reproduction in other forums is permitted, provided the original author(s) and the copyright owner(s) are credited and that the original publication in this journal is cited, in accordance with accepted academic practice. No use, distribution or reproduction is permitted which does not comply with these terms.
*Correspondence: Mingfu Wu, wu_mingfu@tjh.tjmu.edu.cn