- 1Institute for Molecular Bioscience, The University of Queensland, St. Lucia, QLD, Australia
- 2The University of Queensland Frazer Institute, Faculty of Medicine, The University of Queensland, Brisbane, QLD, Australia
- 3School of Biomedical Sciences, University of Queensland, St. Lucia, QLD, Australia
Brain tumors represent the leading cause of disease-related mortality and morbidity in children, with effective treatments urgently required. One factor limiting the effectiveness of systemic therapy is the blood-brain-barrier (BBB), which limits the brain penetration of many anticancer drugs. BBB integrity is often compromised in tumors, referred to as the blood-brain-tumor-barrier (BBTB), and the impact of a compromised BBTB on the therapeutic sensitivity of brain tumors has been clearly shown for a few selected agents. However, the heterogeneity of barrier alteration observed within a single tumor and across distinct pediatric tumor types represents an additional challenge. Herein, we discuss what is known regarding the heterogeneity of tumor-associated vasculature in pediatric brain tumors. We discuss innovative and complementary preclinical model systems that will facilitate real-time functional analyses of BBTB for all pediatric brain tumor types. We believe a broader use of these preclinical models will enable us to develop a greater understanding of the processes underlying tumor-associated vasculature formation and ultimately more efficacious treatment options.
Introduction
The BBB is established through physical and functional interactions of different cell types, referred to as the neurovascular unit (NVU) (1–3), including non-fenestrated endothelial cells (ECs), pericytes, astrocytes and microglia (2–4). In addition to these cellular components, the BBB is further supported by a specialized extracellular matrix (ECM) (5). Here we focus on the structure and function of these BBB components, highlighting complexities within tumor vasculature of pediatric brain cancer and advances in innovative vasculature modelling. For detail into physiological structure and function of NVU components we refer to Table 1. In the context of brain tumors, the BBB is commonly referred to as the blood-brain tumor barrier (BBTB) (3) and is generally thought to be more permeable and ‘leaky’ (3, 35) than the BBB under normal physiological conditions. Leakiness is generally considered to be a consequence of cancer cells disrupting the function or distribution of cells that make up the NVU (36, 37). This relationship is displayed in Figure 1. Clinically, BBB dysfunction in brain tumors is assessed by contrast enhanced magnetic resonance imaging (CE-MRI) using a gadolinium-based contrast agent (38). Studies over the past decade have highlighted that not all pediatric brain tumors alter BBTB similarly. Instead, BBTB function is heterogenous between tumor types as well as within individual tumors (39–42). Understanding BBTB heterogeneity will allow the development of more targeted and effective treatments of distinct tumors. Here we discuss observations made in the most common and also most lethal malignant pediatric brain tumors, Medulloblastoma (MB) and Diffuse Intrinsic Pontine Glioma (DIPG).
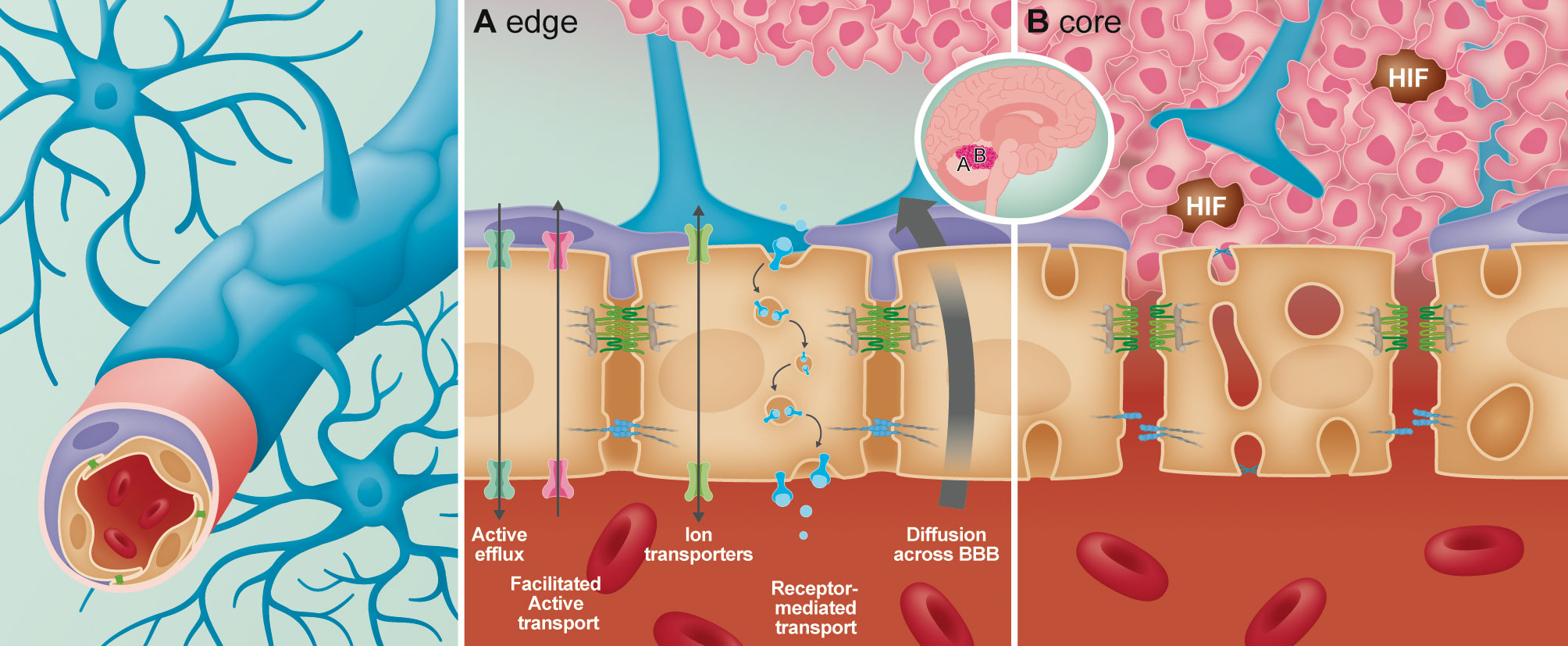
Figure 1 Overview of the structural and anatomical position of the cellular components forming the neurovascular unit (NVU) of the blood-brain-barrier (BBB). In the BBB the endothelial cells act as the interface between the circulating blood and the brain parenchyma. Interlocked with the endothelial cells via “peg-and-socket” connections are the pericytes which aid in maintaining and promoting BBB integrity. Additional components of the NVU are astrocytic endfeet that encapsulate all CNS capillaries for maintenance of the BBB. In the presence of a tumor, heterogeneity of the BBB from the edge (A) to the core (B) has been identified. (A) At the edge of the tumor, the BBB remains intact, with unperturbed transport across the endothelial cells (paracellular and carrier/receptor mediated transcytosis). At the core of the tumor (B), permeability of the BBB increases due to loss of astrocytes and pericyte coverage. In the endothelial cells, junctional integrity diminishes via loss of tight and adherens junctional proteins, while endothelial fenestrations increase leading to enhanced permeability.
Recent findings highlighting the heterogeneity of the BBTB integrity in medulloblastoma
Medulloblastoma (MB) is the most common type of malignant pediatric embryonal tumor that forms in the cerebellum (43). Large scale genomics studies have defined MB into four major subgroups, namely Wingless (WNT), Sonic Hedgehog (SHH), Group 3 (Gp3) and Group 4 (Gp4). These subgroups have been further subdivided into a total of 13 subtypes with distinct molecular and clinical features (43–46). Standard treatment for children greater than three years of age includes surgery, radiation to the cranio-spinal axis and adjuvant chemotherapy (43, 44). WNT-driven MB displays the most favorable prognosis among the four subgroups (43, 44), in part attributed to their robust therapeutic response. CE-MRI studies clearly show variable BBTB integrity across MB subgroups. Solid enhancement indicative of increased BBTB permeability was observed in WNT MB. Heterogeneous contrast enhancement was observed in patients with SHH and Gp3 MB, indicating variable BBTB permeability among regions of the same tumor. Minimal or non-enhancing tumors with an entirely intact BBTB were characteristic of Gp4 (47–49).
Elegant preclinical studies are consistent with clinical observations, with heterogeneous BBTB permeability observed in various widely used preclinical MB mouse models. High-resolution dynamic CE-MRI analyses was recently used to evaluate the integrity and permeability of BBTB in a murine genetically engineered mouse model (GEMM) of SHH MB and patient-derived orthotopic xenograft (PDOX) models from SHH and Gp3 MB (39). BBTB integrity was highly variable in preclinical models of MB, with heterogeneous contrast enhancement observed in both SHH and Gp3 PDOX MB. The invasive front of SHH PDOX tumors displayed minimal contrast enhancement, indicating clear differences in vascular integrity where tumor tissue meets the brain parenchyma. A completely intact functional BBTB was observed in SHH MB tumors initiated in an independent GEMMs despite significant tumor burden (39, 50), consistent with findings from an additional GEMM model of SHH MB (50). Together, these findings indicate that distinct biological processes govern tumor vascularization in tumors that initiate endogenously (GEMM tumors) compared to ectopic tumor cell engraftment in an adult host mouse. Recently, lineage tracing studies performed in a GEMM of SHH MB showed that Sox2-positive MB cells extend protrusions to directly ensheathe nearby capillaries, similar to astrocytes endfeet, contributing to a more intact BBTB formation and function (51). These Sox2-positive MB cells that construct the BBTB were shown to be mechanoresponsive, with Piezo2-mediated signaling regulating both the state of Sox2-positive MB cells and the BBTB. Knockout of Piezo2 resulted in a compromised BBTB, as shown by intratumoural accumulation of systemically administered 1kDa Cadaverine and 70kDa Dextran (51), and extended survival in response to etoposide treatment compared to tumor-bearing controls. Another study systemically administered 70kDa tetramethylrhodamine (TMR)-dextran to demonstrate that a GEMM model of WNT MB lack a functional BBTB (50), consistent with the solid enhancement observed in patients with WNT MB. Increased BBTB permeability has been suggested to dictate the improved therapeutic response of WNT MB (52). Indeed, functional studies have shown that tumors with a permeable BBTB from a genetically engineered mouse model (GEMM) of WNT MB responded to vincristine, which does not penetrate well into normal brain tissue (52). Disruption of WNT signaling restored BBTB function, blocked delivery of vincristine to the tumor and rendering them resistant to therapy in vivo. Together these studies point to the influence of tumor genotype on the development of an intact BBTB and reinforce that in vivo preclinical models of MB faithfully recapitulate the intratumoral and intertumoral heterogeneity of the BBTB phenotype of primary MB.
Various alterations in a number of components of the NVU is likely contributing to BBTB dysfunction in MB. The degree of BBTB permeability observed by MRI was shown to be correlated to differences in the structural and subcellular features of tumor-associated vasculature (39). Intact tumor-associated vasculature of the GEMM model of SHH MB displayed organized, linear expression of junctional markers CD31 and CLDN5, outlining a continuous vessel structure with extensive astrocytic encircling (39, 51). Basement membrane components, pericyte coverage and tight junction proteins were significantly reduced in this model upon genetic deletion of Piezo2, leading to increased leakage of fluorescent dyes into the brain parenchyma (51). In SHH and Gp3 PDOX models, the BBTB is compromised with abnormal barrier features such as disorganized endothelial cell-cell junctions, minimal astrocyte coverage and the presence of fenestrated, immature endothelium as determined by the expression of fenestrae marker Plvap (53, 54), and the loss of Glucose transporter 1 (Glut1) (53). Similar defects were observed in tumor-associated vasculature of PDOX and GEMM WNT MB, with hemorrhagic, aberrant vascular networks displaying a non-BBB immunophenotype characterized by the ectopic expression of Plvap and the loss Glut1 (52). Transmission and scanning electron microscopy confirmed fenestrated pores connecting the luminal and abluminal compartments of endothelium of tumors from GEMM WNT MB, with disruption of endothelial tight junctions also confirmed (52). These vascular changes were not observed in tumor endothelium from GEMM SHH MB. Transcriptomic analysis also confirmed that this endothelium was more similar to peripheral endothelium with the down-regulation of endothelial tight junctional protein Cldn5 and Glut1, while endothelium from GEMM SHH MB was very similar to normal brain endothelium (52). Decreased pericyte coverage was also observed in tumors from GEMM WNT MB compared to normal coverage in GEMM SHH MB (52).
Whilst the above-mentioned studies have begun to explore functional differences in BBTB integrity and the structural and sub-cellular features of tumor-associated vasculature in MB, very little is known regarding the processes driving tumor vascularization. Several mechanisms of tumor vascularization have been defined including sprouting angiogenesis, intussusceptive angiogenesis, vessel co-option, vasculogenic mimicry and lymphangiogenesis (55). Histological analysis of brain tumor sections implies a role for angiogenesis and vascular mimicry in GEMM and PDOX tumor sections of SHH and Gp3 MB (39), with an earlier study identifying elevated Vascular endothelial growth factor (VEGF), a principal angiogenic factor, in cell line xenograft Gp3 models and Gp3 MB patients (56). Given vasculature architecture has been shown to influence therapeutic response (52, 57), further characterization of tumor vasculature and the processes driving this in MB is necessary to ensure effective treatment of this disease.
An intact BBTB represents a major hurdle in the treatment of Diffuse Midline Glioma
Diffuse Intrinsic Pontine Glioma (DIPG), more recently termed Diffuse Midline Glioma (DMG) (58), is a highly aggressive, lethal pediatric brain tumor that grows diffusely throughout the brainstem (59, 60). Surgical options are limited for DMG patients, largely due to the location of the tumors. Chemotherapy or other targeted therapies have not been shown to significantly improve survival rates for DMG patients (60, 61). One proposed explanation for the failure of systemically delivered therapies in DMG is due to the intact nature of the BBTB (59, 62, 63), as evidenced by the failure of contrast-enhancing agents to penetrate tumor tissue. Histological and molecular analyses of primary DMG, PDOX and in utero electroporation (IUE) mouse models, all revealed a minimal change in vascular phenotype within tumors compared to normal brain (58). The ECs displayed continuous expression junctional proteins CLDN5 and CD31, normal expression of the transporter Glut1 and did not express the pathological marker, Plvap. Extensive coverage by pericytes was also revealed and administration of 10kDa TMR-dextran in the IUE model, showing limited leakage. Together, these findings suggest that blood vessels are unaffected by the presence of DMG cells, possibly explaining why systemic therapy is not efficacious for this disease. Instead, novel delivery technologies such as small lipophilic drugs designed to cross an intact BBTB with minimal active efflux, are likely to be more successful. Another emerging technology is MRI-guided focused ultrasound (MRIg-FUS) that induces transient openings of the BBB by acoustic activation of circulating microbubbles. This technique has been shown to disrupt the BBB in a controllable manner in both animal models and in patients with high grade gliomas (64, 65). More recently, a similar approach was applied to target blood vessels in a PDOX model for DMG, revealing a significant increase in intra-tumoral doxorubicin concentrations and reduced tumor volume through MRIg-FUS (66).
The studies described here have begun to unravel the complexity of BBTB in MB and DMG and the relevance of modifying BBTB function for more effective, targeted treatment. However, before we can do this, a better understanding of the processes underlying tumor vascularization and the tumor-specific changes in NVU composition and BBTB function are urgently required. Next, we describe a range of innovative preclinical models that can be utilized to accelerate this understudied aspect of pediatric brain tumor biology.
Innovative preclinical models to interrogate pediatric brain tumor vasculature
Preclinical mouse PDOX and GEMM models of MB and DMG are widely used as the gold standard for preclinical testing of novel therapeutics (67–69). Studies utilizing these models clearly show that a greater understanding of how tumor cells interact with each other, and their surrounding microenvironment is urgently needed. Further to this, we need to better understand how tumors orchestrate structural and functional changes in their associated vasculature before we can develop effective, targeted therapies for pediatric brain tumors. Such mouse models have further uncovered the important roles for astrocytes (70), pericytes (13, 71, 72) and microglial (73) cells for BBB development and integrity. However, the complex interplay of the NVU in the context of tumor progression and therapy response remains to be determined.
In murine models, non-invasive techniques such as positron emission tomography (PET), computed tomography (CT), and more commonly MRI, have uncovered changes in the BBTB (74). However, longitudinal dynamic high-resolution imaging is required to understand the interactions between the developing tumor and its associated microenvironment. Live imaging technologies have been developed for rodent brains (75, 76), however these approaches are incredibly costly and present with a number of technical challenges due to the location of MB and DMG within the cerebellum and the pons of the brain stem respectively.
Dynamic modelling of tumor-vessel interactions in zebrafish
A vertebrate model that is rapidly developing as a robust model for cancer research is the zebrafish (77–81). A major reason for the expansion of zebrafish studies is the cost-effectiveness of the model due to the large number of offspring that can be obtained from a single mating and lower husbandry costs associated with zebrafish housing. Another great advantage is that zebrafish embryos and larvae are optically transparent and develop ex utero, allowing live visualization of organs and cells during zebrafish development. Although structural similarities of zebrafish and human proteins remain to be determined, whole genome sequencing has identified that approximately 80% of disease associated genes in humans are conserved in zebrafish (82). This finding combined with the ease of genetic manipulation in zebrafish (83–85), led to the development of genetically engineered zebrafish models for a range of cancer types (86). In addition to genetic models, xenografting approaches are also widely applied to study cancer biology in zebrafish. Since the adaptive immune system in zebrafish is fully functional from 28 days post fertilization (dpf) (87, 88), mouse or human cancer cells are tolerated without inflicting an immune response prior to this stage.
The brain is challenging to image in higher vertebrates due to the presence of a thick skull, zebrafish brains are much more accessible and thus researchers can visualize and monitor tumor cell behavior in space and time. The anatomy of zebrafish brains is comparable to that of mammalian brains, with significant homology in molecular signatures and structure of distinct brain regions (89). Notable differences however do exist in terms of size and organization of distinct brain regions (90–92). In terms of the BBB, live imaging of zebrafish vascular transgenic marker lines has been applied to establish that the BBB in zebrafish begins to form at three days post-fertilization (dpf) and is fully functional at 10 dpf (93, 94). The zebrafish NVU is made up of endothelial cells, pericytes and radial glial cells (95). The radial glial cells express key astrocytic markers including Gfap, glutamine synthase and Aqp4 (95) and therefore are considered to perform orthologous roles to astrocytes (95). Transgenic marker lines, labelling distinct cell types of the NVU have been developed (96–99), allowing live visualization of the morphology, abundance, and dynamic behavior of distinct NVU cell types simultaneously.
In the context of brain cancer, zebrafish provide a powerful model to monitor dynamic interactions between tumor cells and NVU cell types, therefore determining what pathological changes might be contributing to BBTB malfunction. To date a small set of genetic zebrafish models have been established to study pediatric brain tumors (100–102). One such model that recapitulates central nervous system primitive neuroectodermal tumors (CNS-PNETs) was developed by Solin and colleagues (102), whereby TALEN-mediated genome editing was applied to inactivate retinoblastoma1 (rb1). When placed on a p53 null background, these rb1 knockout fish developed malformations of the skull and lesions on the eye. Histological analysis of the lesions revealed that the majority resembled CNS-PNET tumors and others were glial-like (102). Others have tested whether oligoneural precursor cells (OPCs) could give rise to CNS-PNETs by overactivated NRAS/MAPK signaling exclusively in these sox10 expressing cells (101). This resulted in the development of large lesions in 6-week old zebrafish with conserved genetic and histologic hallmarks. Since hundreds of zebrafish embryos can be derived from a single paired mating it is highly suited for drug screening, especially when screening water soluble compounds that can be added to the zebrafish water. The authors utilized a screening approach to show that addition of MEK inhibitors to the fish water could effectively inhibit the growth of CNS-PNETs upon orthotopic xenografting (101). Human derived pediatric brain tumor cells have not been studied using zebrafish xenografting, however, tumor cells derived from adult brain cancers have been grafted successfully showing that human cells can utilize the zebrafish brain microenvironment to proliferate and migrate from the initial injection site (87). One challenge to utilizing zebrafish for xenografting is the optimal physiological core temperature of 28°C. However, zebrafish can adapt to changes in temperature. Previous studies have successfully grown zebrafish at temperatures of up to 36°C for xenografting purposes without notable side effects (103–106). Whether changes in temperature might invoke more subtle changes in the biological response to xenografted cells remains to be determined (107, 108).
Nevertheless, we propose that with well-established methods in place to visualize NVU cell types in the zebrafish brain (96–99) and determine BBB permeability (95), the zebrafish model is perfectly positioned to enable in depth studies that will generate new knowledge into the fundamental aspects of pediatric tumor heterogeneity, drug responses, metastatic potential, and alteration of the microenvironment.
Microfluidic tumor-vessel co-culture models
Traditional in vitro cell culture methods have been used in basic research for many years to study mechanisms of cancer cell growth and evaluate drug efficacy. Various pediatric brain cancer cell lines are currently commercially available, with the most widely published MB models such as D283MED, D341MED, D425MED, UW228-2 and DAOY propagated in vitro for decades. As seen with other widely utilised brain cancer cell lines (109), it is increasingly likely that the original molecular features and biological behaviour of tumor cells would have been lost, failing to recapitulate tumour heterogeneity. Additionally, it is well appreciated that these simplistic 2D cell culture systems do not model the spatial, cellular and chemical complexity of tumors and the associated TME (110), limiting the translational utility of these model systems.
3D spheroid models are being increasingly developed for a variety of pediatric brain cancers (111, 112) replicating elements of the tumor microenvironment such as a gradient distribution of nutrients, oxygen, pH, cell-cell and cell- extracellular (ECM) contact (113). 3D tumor spheres derived from a variety of pediatric brain cancers including PDOX models of Gp3 MB (114) and primary biopsy material from MB, Ependymoma, Glioblastoma and Astrocytoma patients (111) have been recently established. Whilst pediatric 3D tumor spheroid cultures represent an important advance for the field, they still lack several essential components of the brain specific TME such as the ECM and the diverse non-cancerous cells including endothelial cells (ECs), pericytes, fibroblasts, immune cells, astrocytes, neurons and microglia (115, 116). Advances in cellular engineering, biomaterials and biofabrication technologies have led to the development of co-culture platforms whereby 3D tumor spheroids can be grown in the context of blood vessels and biologically relevant ECM hydrogels (117). To model the brain endothelium specifically, distinct types of brain ECs have been employed to form so called 3D BBB models. Of particular interest are recent protocols that utilize induced pluripotent stem cell (iPSC) derived brain microvascular ECs (iBMECs) (118–122). This is because these iBMECs when grown with other NVU cell types have been shown to form a tight BBB with physiologically relevant barrier properties as measured by transendothelial electrical resistance (TEER) and extravasation of fluorescent dyes (118–122). The validation of structural and functional features of an intact BBB supports the utility of these models to monitor how tumor cells alter the BBB and how this impacts the efficacy of therapeutics. Further details on the overall benefits and drawbacks of BBB culture have been reviewed elsewhere (123–125).
Although 3D BBB-tumor co-culture models have not yet been implemented to study tumor spheroids derived from pediatric brain cancer, data from adult brain tumors supports the feasibility of this approach (126, 127). For glioblastoma, tumor spheres were grown alongside an iPSC-derived BBB to test combination therapies. Vincristine and doxorubicin, two anti-cancer drugs that do not cross the BBB, were added to the 3D vessel in combination with mannitol and gintonin, to temporally open the BBB (128). Drug uptake was significantly improved within the 3D glioblastoma spheroid in combinations with mannitol and gintonin addition which induces BBB permeability (128). With platforms and applications of organ-on-a-chip models now widely accepted for a variety of diseases (129–134), it is imperative that these models are adapted and transitioned for the study of pediatric brain cancer. These innovative in vitro platforms in conjunction with animal models will be highly important to better understand the molecular mechanism of such ailments and providing novel therapeutics that have been thoroughly tested to target tumors in the presence of a heterogeneous BBTB.
Discussion
Ineffective drug delivery is thought to be a contributing factor underlying the failure of novel therapeutic strategies in early phase clinical studies after demonstrating significant preclinical anti-tumor efficacy. Understanding the plasticity of the BBTB by utilizing complementary pre-clinical models will help to overcome one of the biggest barriers to effective intratumoral drug penetration. This review has summarized recent discoveries that emphasize the relevance of BBTB heterogeneity in pediatric brain tumors. We propose that, in order to better understand how BBTB differences arise and what the functional consequences are for treatment, multi-disciplinary approaches that utilize innovative pre-clinical models hold great potential.
Author contributions
EM, SD-M, SS, LG and AL conceptualized the idea of this review and co-wrote the manuscript. All authors contributed to the article and approved the submitted version.
Funding
This work is supported by research grants from the Children’s Hospital Foundation (LG, AL, SS, Grant number ACR0162020), Brainchild Foundation (LG, AL, SS) and DIPG collaborative (LG, AL, SS).
Acknowledgments
We thank Nick Valmas for assistance in generating the schematic illustration of the BBTB (Figure 1). We thank Prof. B. Wainwright for his mentorship of the research program that produced this manuscript.
Conflict of interest
The authors declare that the research was conducted in the absence of any commercial or financial relationships that could be construed as a potential conflict of interest.
The handling editor RE declared a past co-authorship with the author LG.
Publisher’s note
All claims expressed in this article are solely those of the authors and do not necessarily represent those of their affiliated organizations, or those of the publisher, the editors and the reviewers. Any product that may be evaluated in this article, or claim that may be made by its manufacturer, is not guaranteed or endorsed by the publisher.
References
1. Iadecola C. The neurovascular unit coming of age: A journey through neurovascular coupling in health and disease. Neuron (2017) 96(1):17–42. doi: 10.1016/j.neuron.2017.07.030
2. Sweeney MD, Zhao Z, Montagne A, Nelson AR, Zlokovic BV. Blood-brain barrier: From physiology to disease and back. Physiol Rev (2019) 99(1):21–78. doi: 10.1152/physrev.00050.2017
3. Daneman R, Prat A. The blood-brain barrier. Cold Spring Harb Perspect Biol (2015) 7(1):a020412. doi: 10.1101/cshperspect.a020412
4. Obermeier B, Daneman R, Ransohoff RM. Development, maintenance and disruption of the blood-brain barrier. Nat Med (2013) 19(12):1584–96. doi: 10.1038/nm.3407
5. Baeten KM, Akassoglou K. Extracellular matrix and matrix receptors in blood-brain barrier formation and stroke. Dev Neurobiol (2011) 71(11):1018–39. doi: 10.1002/dneu.20954
6. Stamatovic SM, Keep RF, Andjelkovic AV. Brain endothelial cell-cell junctions: how to "open" the blood brain barrier. Curr Neuropharmacol (2008) 6(3):179–92. doi: 10.2174/157015908785777210
7. McConnell HL, Mishra A. Cells of the blood–brain barrier: An overview of the neurovascular unit in health and disease. In: Stone N, editor. The blood-brain barrier: Methods and protocols. New York, NY: Springer US (2022). p. 3–24.
8. Ben-Zvi A, Lacoste B, Kur E, Andreone BJ, Mayshar Y, Yan H, et al. Mfsd2a is critical for the formation and function of the blood–brain barrier. Nature (2014) 509(7501):507–11. doi: 10.1038/nature13324
9. Villaseñor R, Lampe J, Schwaninger M, Collin L. Intracellular transport and regulation of transcytosis across the blood–brain barrier. Cell Mol Life Sci (2019) 76(6):1081–92. doi: 10.1007/s00018-018-2982-x
10. Langen UH, Ayloo S, Gu C. Development and cell biology of the blood-brain barrier. Annu Rev Cell Dev Biol (2019) 35(1):591–613. doi: 10.1146/annurev-cellbio-100617-062608
11. Pulgar VM. Transcytosis to cross the blood brain barrier, new advancements and challenges. Front Neurosci (2019) 12. doi: 10.3389/fnins.2018.01019
12. Barar J, Rafi MA, Pourseif MM, Omidi Y. Blood-brain barrier transport machineries and targeted therapy of brain diseases. Bioimpacts (2016) 6(4):225–48. doi: 10.15171/bi.2016.30
13. Armulik A, Genove G, Mae M, Nisancioglu MH, Wallgard E, Niaudet C, et al. Pericytes regulate the blood-brain barrier. Nature (2010) 468(7323):557–61. doi: 10.1038/nature09522
14. Cabezas R, Avila M, Gonzalez J, El-Bachá RS, Báez E, García-Segura LM, et al. Astrocytic modulation of blood brain barrier: perspectives on parkinson's disease. Front Cell Neurosci (2014) 8:211. doi: 10.3389/fncel.2014.00211
15. Kim Y, Park J, Choi YK. The role of astrocytes in the central nervous system focused on BK channel and heme oxygenase metabolites: A review. Antioxid (Basel) (2019) 8(5):1–19. doi: 10.3390/antiox8050121
16. Sofroniew MV, Vinters HV. Astrocytes: biology and pathology. Acta Neuropathol (2010) 119(1):7–35. doi: 10.1007/s00401-009-0619-8
17. Liu LR, Liu JC, Bao JS, Bai QQ, Wang GQ. Interaction of microglia and astrocytes in the neurovascular unit. Front Immunol (2020) 11:1024. doi: 10.3389/fimmu.2020.01024
18. Ronaldson PT, Davis TP. Regulation of blood–brain barrier integrity by microglia in health and disease: A therapeutic opportunity. J Cereb Blood Flow Metab (2020) 40(1_suppl):S6–S24. doi: 10.1177/0271678X20951995
19. Herndon JM, Tome ME, Davis TP. Chapter 9 - development and maintenance of the blood–brain barrier. In: Caplan LR, Biller J, Leary MC, Lo EH, Thomas AJ, Yenari M, editors. Primer on cerebrovascular diseases, 2nd ed. San Diego: Academic Press (2017). p. 51–6.
20. Armulik A, Abramsson A, Betsholtz C. Endothelial/pericyte interactions. Circ Res (2005) 97(6):512–23. doi: 10.1161/01.RES.0000182903.16652.d7
21. Liu Q, Yang Y, Fan X. Microvascular pericytes in brain-associated vascular disease. Biomed Pharmacother (2020) 121:109633. doi: 10.1016/j.biopha.2019.109633
22. Sweeney MD, Ayyadurai S, Zlokovic BV. Pericytes of the neurovascular unit: key functions and signaling pathways. Nat Neurosci (2016) 19(6):771–83. doi: 10.1038/nn.4288
23. Brown LS, Foster CG, Courtney J-M, King NE, Howells DW, Sutherland BA. Pericytes and neurovascular function in the healthy and diseased brain. Front Cell Neurosci (2019) 13. doi: 10.3389/fncel.2019.00282
24. Attwell D, Mishra A, Hall CN, O’Farrell FM, Dalkara T. What is a pericyte? J Cereb Blood Flow Metab (2016) 36(2):451–5. doi: 10.1177/0271678X15610340
25. Kang R, Gamdzyk M, Lenahan C, Tang J, Tan S, Zhang JH. The dual role of microglia in blood-brain barrier dysfunction after stroke. Curr Neuropharmacol (2020) 18(12):1237–49. doi: 10.2174/1570159X18666200529150907
26. Liebner S, Dijkhuizen RM, Reiss Y, Plate KH, Agalliu D, Constantin G. Functional morphology of the blood–brain barrier in health and disease. Acta Neuropathol (2018) 135(3):311–36. doi: 10.1007/s00401-018-1815-1
27. Nimmerjahn A, Kirchhoff F, Helmchen F. Resting microglial cells are highly dynamic surveillants of brain parenchyma in vivo. Science (2005) 308(5726):1314–8. doi: 10.1126/science.1110647
28. Salter MW, Stevens B. Microglia emerge as central players in brain disease. Nat Med (2017) 23(9):1018–27. doi: 10.1038/nm.4397
29. Davalos D, Grutzendler J, Yang G, Kim JV, Zuo Y, Jung S, et al. ATP mediates rapid microglial response to local brain injury in vivo. Nat Neurosci (2005) 8(6):752–8. doi: 10.1038/nn1472
30. LeBleu VS, MacDonald B, Kalluri R. Structure and function of basement membranes. Exp Biol Med (2007) 232(9):1121–9. doi: 10.3181/0703-MR-72
31. Xu L, Nirwane A, Yao Y. Basement membrane and blood-brain barrier. Stroke Vasc Neurol (2018) 4(2):78–82. doi: 10.1136/svn-2018-000198
32. Yurchenco PD, Patton BL. Developmental and pathogenic mechanisms of basement membrane assembly. Curr Pharm Des (2009) 15(12):1277–94. doi: 10.2174/138161209787846766
33. Thomsen MS, Routhe LJ, Moos T. The vascular basement membrane in the healthy and pathological brain. J Cereb Blood Flow Metab (2017) 37(10):3300–17. doi: 10.1177/0271678X17722436
34. Morrissey MA, Sherwood DR. An active role for basement membrane assembly and modification in tissue sculpting. J Cell Sci (2015) 128(9):1661–8. doi: 10.1242/jcs.168021
35. Pollack IF, Agnihotri S, Broniscer A. Childhood brain tumors: current management, biological insights, and future directions. J Neurosurg Pediatr (2019) 23(3):261–73. doi: 10.3171/2018.10.PEDS18377
36. Steeg PS. The blood–tumour barrier in cancer biology and therapy. Nat Rev Clin Oncol (2021) 18(11):696–714. doi: 10.1038/s41571-021-00529-6
37. Arvanitis CD, Ferraro GB, Jain RK. The blood–brain barrier and blood–tumour barrier in brain tumours and metastases. Nat Rev Cancer (2020) 20(1):26–41. doi: 10.1038/s41568-019-0205-x
38. Cha S. Neuroimaging in neuro-oncology. Neurotherapeutics (2009) 6(3):465–77. doi: 10.1016/j.nurt.2009.05.002
39. Genovesi LA, Puttick S, Millar A, Kojic M, Ji P, Lagendijk AK, et al. Patient-derived orthotopic xenograft models of medulloblastoma lack a functional blood-brain barrier. Neuro Oncol (2021) 23(5):732–42. doi: 10.1093/neuonc/noaa266
40. Salloum R, McConechy MK, Mikael LG, Fuller C, Drissi R, DeWire M, et al. Characterizing temporal genomic heterogeneity in pediatric high-grade gliomas. Acta Neuropathol Commun (2017) 5(1):78. doi: 10.1186/s40478-017-0479-8
41. He C, Xu K, Zhu X, Dunphy PS, Gudenas B, Lin W, et al. Patient-derived models recapitulate heterogeneity of molecular signatures and drug response in pediatric high-grade glioma. Nat Commun (2021) 12(1):4089. doi: 10.1038/s41467-021-24168-8
42. Hoffman M, Gillmor AH, Kunz DJ, Johnston MJ, Nikolic A, Narta K, et al. Intratumoral genetic and functional heterogeneity in pediatric glioblastoma. Cancer Res (2019) 79(9):2111–23. doi: 10.1158/0008-5472.CAN-18-3441
43. Northcott PA, Robinson GW, Kratz CP, Mabbott DJ, Pomeroy SL, Clifford SC, et al. Medulloblastoma. Nat Rev Dis Primers (2019) 5(1):11. doi: 10.1038/s41572-019-0063-6
44. Juraschka K, Taylor MD. Medulloblastoma in the age of molecular subgroups: a review. J Neurosurg Pediatr (2019) 24(4):353–63. doi: 10.3171/2019.5.PEDS18381
45. Cavalli FMG, Remke M, Rampasek L, Peacock J, Shih DJH, Luu B, et al. Intertumoral heterogeneity within medulloblastoma subgroups. Cancer Cell (2017) 31(6):737–54.e6. doi: 10.1016/j.ccell.2017.05.005
46. Hovestadt V, Ayrault O, Swartling FJ, Robinson GW, Pfister SM, Northcott PA. Medulloblastomics revisited: biological and clinical insights from thousands of patients. Nat Rev Cancer (2020) 20(1):42–56. doi: 10.1038/s41568-019-0223-8
47. Lastowska M, Jurkiewicz E, Trubicka J, Daszkiewicz P, Drogosiewicz M, Malczyk K, et al. Contrast enhancement pattern predicts poor survival for patients with non-WNT/SHH medulloblastoma tumours. J Neurooncol (2015) 123(1):65–73. doi: 10.1007/s11060-015-1779-0
48. Perreault S, Ramaswamy V, Achrol AS, Chao K, Liu TT, Shih D, et al. MRI Surrogates for molecular subgroups of medulloblastoma. AJNR Am J Neuroradiol (2014) 35(7):1263–9. doi: 10.3174/ajnr.A3990
49. Yeom KW, Mobley BC, Lober RM, Andre JB, Partap S, Vogel H, et al. Distinctive MRI features of pediatric medulloblastoma subtypes. AJR Am J Roentgenol (2013) 200(4):895–903. doi: 10.2214/AJR.12.9249
50. Girard E, Ditzler S, Lee D, Richards A, Yagle K, Park J, et al. Efficacy of cabazitaxel in mouse models of pediatric brain tumors. Neuro Oncol (2015) 17(1):107–15. doi: 10.1093/neuonc/nou163
51. Chen X, Momin A, Wanggou S, Wang X, Min H-K, Dou W, et al. Mechanosensitive brain tumor cells construct blood-tumor barrier to mask chemosensitivity. Neuron (2022) 111:30–48. doi: 10.1093/neuonc/noac209.144
52. Phoenix TN, Patmore DM, Boop S, Boulos N, Jacus MO, Patel YT, et al. Medulloblastoma genotype dictates blood brain barrier phenotype. Cancer Cell (2016) 29(4):508–22. doi: 10.1016/j.ccell.2016.03.002
53. Zhao Z, Nelson AR, Betsholtz C, Zlokovic BV. Establishment and dysfunction of the blood-brain barrier. Cell (2015) 163(5):1064–78. doi: 10.1016/j.cell.2015.10.067
54. Guo L, Zhang H, Hou Y, Wei T, Liu J. Plasmalemma vesicle-associated protein: A crucial component of vascular homeostasis. Exp Ther Med (2016) 12(3):1639–44. doi: 10.3892/etm.2016.3557
55. Hillen F, Griffioen AW. Tumour vascularization: sprouting angiogenesis and beyond. Cancer Metastasis Rev (2007) 26(3-4):489–502. doi: 10.1007/s10555-007-9094-7
56. Thompson EM, Keir ST, Venkatraman T, Lascola C, Yeom KW, Nixon AB, et al. The role of angiogenesis in group 3 medulloblastoma pathogenesis and survival. Neuro Oncol (2017) 19(9):1217–27. doi: 10.1093/neuonc/nox033
57. Griffith JI, Rathi S, Zhang W, Zhang W, Drewes LR, Sarkaria JN, et al. Addressing BBB heterogeneity: A new paradigm for drug delivery to brain tumors. Pharmaceutics (2020) 12(12):1–38. doi: 10.3390/pharmaceutics12121205
58. Wei X, Meel MH, Breur M, Bugiani M, Hulleman E, Phoenix TN. Defining tumor-associated vascular heterogeneity in pediatric high-grade and diffuse midline gliomas. Acta Neuropathol Commun (2021) 9(1):142. doi: 10.1186/s40478-021-01243-1
59. Warren K. Diffuse intrinsic pontine glioma: poised for progress. Front Oncol (2012) 2(205). doi: 10.3389/fonc.2012.00205
60. Hennika T, Becher OJ. Diffuse intrinsic pontine Glioma:Time for cautious optimism. J Child Neurol (2016) 31(12):1377–85. doi: 10.1177/0883073815601495
61. Jones C, Karajannis MA, Jones DTW, Kieran MW, Monje M, Baker SJ, et al. Pediatric high-grade glioma: biologically and clinically in need of new thinking. Neuro Oncol (2017) 19(2):153–61. doi: 10.1093/neuonc/now101
62. Hoffman LM, Veldhuijzen van Zanten SEM, Colditz N, Baugh J, Chaney B, Hoffmann M, et al. Clinical, radiologic, pathologic, and molecular characteristics of long-term survivors of diffuse intrinsic pontine glioma (DIPG): A collaborative report from the international and European society for pediatric oncology DIPG registries. J Clin Oncol (2018) 36(19):1963–72. doi: 10.1200/JCO.2017.75.9308
63. Warren KE. Beyond the Blood:Brain barrier: The importance of central nervous system (CNS) pharmacokinetics for the treatment of CNS tumors, including diffuse intrinsic pontine glioma. Front Oncol (2018) 8(239). doi: 10.3389/fonc.2018.00239
64. Anastasiadis P, Gandhi D, Guo Y, Ahmed AK, Bentzen SM, Arvanitis C, et al. Localized blood-brain barrier opening in infiltrating gliomas with MRI-guided acoustic emissions-controlled focused ultrasound. Proc Natl Acad Sci U.S.A. (2021) 118(37):1–11. doi: 10.1073/pnas.2103280118
65. Brighi C, Reid L, White AL, Genovesi LA, Kojic M, Millar A, et al. MR-guided focused ultrasound increases antibody delivery to nonenhancing high-grade glioma. Neurooncol Adv (2020) 2(1):vdaa030. doi: 10.1093/noajnl/vdaa030
66. Ishida J, Alli S, Bondoc A, Golbourn B, Sabha N, Mikloska K, et al. MRI-Guided focused ultrasound enhances drug delivery in experimental diffuse intrinsic pontine glioma. J Controlled Release (2021) 330:1034–45. doi: 10.1016/j.jconrel.2020.11.010
67. Wu X, Northcott PA, Croul S, Taylor MD. Mouse models of medulloblastoma. Chin J Cancer (2011) 30(7):442–9. doi: 10.5732/cjc.011.10040
68. Roussel MF, Stripay JL. Modeling pediatric medulloblastoma. Brain Pathol (2020) 30(3):703–12. doi: 10.1111/bpa.12803
69. Welby JP, Kaptzan T, Wohl A, Peterson TE, Raghunathan A, Brown DA, et al. Current murine models and new developments in H3K27M diffuse midline gliomas. Front Oncol (2019) 9. doi: 10.3389/fonc.2019.00092
70. Katz AM, Amankulor NM, Pitter K, Helmy K, Squatrito M, Holland EC. Astrocyte-specific expression patterns associated with the PDGF-induced glioma microenvironment. PloS One (2012) 7(2):e32453–e. doi: 10.1371/journal.pone.0032453
71. Villaseñor R, Kuennecke B, Ozmen L, Ammann M, Kugler C, Grüninger F, et al. Region-specific permeability of the blood-brain barrier upon pericyte loss. J Cereb Blood Flow Metab (2017) 37(12):3683–94. doi: 10.1177/0271678X17697340
72. Daneman R, Zhou L, Kebede AA, Barres BA. Pericytes are required for blood–brain barrier integrity during embryogenesis. Nature (2010) 468(7323):562–6. doi: 10.1038/nature09513
73. Markovic DS, Vinnakota K, Chirasani S, Synowitz M, Raguet H, Stock K, et al. Gliomas induce and exploit microglial MT1-MMP expression for tumor expansion. Proc Natl Acad Sci U S A (2009) 106(30):12530–5. doi: 10.1073/pnas.0804273106
74. Eklund L, Bry M, Alitalo K. Mouse models for studying angiogenesis and lymphangiogenesis in cancer. Mol Oncol (2013) 7(2):259–82. doi: 10.1016/j.molonc.2013.02.007
75. Park J-H, Sun W, Cui M. High-resolution in vivo imaging of mouse brain through the intact skull. Proc Natl Acad Sci (2015) 112(30):9236–41. doi: 10.1073/pnas.1505939112
76. Urban BE, Xiao L, Chen S, Yang H, Dong B, Kozorovitskiy Y, et al. In vivo superresolution imaging of neuronal structure in the mouse brain. IEEE Trans BioMed Eng (2018) 65(1):232–8. doi: 10.1109/TBME.2017.2773540
77. Letrado P, de Miguel I, Lamberto I, Díez-Martínez R, Oyarzabal J. Zebrafish: Speeding up the cancer drug discovery process. Cancer Res (2018) 78(21):6048–58. doi: 10.1158/0008-5472.CAN-18-1029
78. Stoletov K, Klemke R. Catch of the day: zebrafish as a human cancer model. Oncogene (2008) 27(33):4509–20. doi: 10.1038/onc.2008.95
79. Durinikova E, Buzo K, Arena S. Preclinical models as patients' avatars for precision medicine in colorectal cancer: past and future challenges. J Exp Clin Cancer Res (2021) 40(1):185. doi: 10.1186/s13046-021-01981-z
80. Xiao J, Glasgow E, Agarwal S. Zebrafish xenografts for drug discovery and personalized medicine. Trends Cancer (2020) 6(7):569–79. doi: 10.1016/j.trecan.2020.03.012
81. Weiss JM, Lumaquin-Yin D, Montal E, Suresh S, Leonhardt CS, White RM. Shifting the focus of zebrafish toward a model of the tumor microenvironment. eLife (2022) 11:e69703. doi: 10.7554/eLife.69703
82. Howe K, Clark MD, Torroja CF, Torrance J, Berthelot C, Muffato M, et al. The zebrafish reference genome sequence and its relationship to the human genome. Nature (2013) 496(7446):498–503. doi: 10.1038/nature12111
83. Kalueff AV, Stewart AM, Gerlai R. Zebrafish as an emerging model for studying complex brain disorders. Trends Pharmacol Sci (2014) 35(2):63–75. doi: 10.1016/j.tips.2013.12.002
84. Hruscha A, Krawitz P, Rechenberg A, Heinrich V, Hecht J, Haass C, et al. Efficient CRISPR/Cas9 genome editing with low off-target effects in zebrafish. Development (2013) 140(24):4982–7. doi: 10.1242/dev.099085
85. Amsterdam A, Hopkins N. Mutagenesis strategies in zebrafish for identifying genes involved in development and disease. Trends Genet (2006) 22(9):473–8. doi: 10.1016/j.tig.2006.06.011
86. Hason M, Bartůněk P. Zebrafish models of cancer-new insights on modeling human cancer in a non-mammalian vertebrate. Genes (Basel) (2019) 10(11):1–30. doi: 10.3390/genes10110935
87. Wehmas LC, Tanguay RL, Punnoose A, Greenwood JA. Developing a novel embryo-larval zebrafish xenograft assay to prioritize human glioblastoma therapeutics. Zebrafish (2016) 13(4):317–29. doi: 10.1089/zeb.2015.1170
88. Lam SH, Chua HL, Gong Z, Lam TJ, Sin YM. Development and maturation of the immune system in zebrafish, danio rerio: a gene expression profiling, in situ hybridization and immunological study. Dev Comp Immunol (2004) 28(1):9–28. doi: 10.1016/S0145-305X(03)00103-4
89. Kozol RA, Abrams AJ, James DM, Buglo E, Yan Q, Dallman JE. Function over form: Modeling groups of inherited neurological conditions in zebrafish. Front Mol Neurosci (2016) 9:55. doi: 10.3389/fnmol.2016.00055
90. Mueller T, Wullimann MF. An evolutionary interpretation of teleostean forebrain anatomy. Brain Behav Evol (2009) 74(1):30–42. doi: 10.1159/000229011
91. Tropepe V, Sive HL. Can zebrafish be used as a model to study the neurodevelopmental causes of autism? Genes Brain Behav (2003) 2(5):268–81. doi: 10.1034/j.1601-183X.2003.00038.x
92. Haynes EM, Ulland TK. Eliceiri KW. a model of discovery: The role of imaging established and emerging non-mammalian models in neuroscience. Front Mol Neurosci (2022) 15. doi: 10.3389/fnmol.2022.867010
93. Quinonez-Silvero C, Hubner K, Herzog W. Development of the brain vasculature and the blood-brain barrier in zebrafish. Dev Biol (2020) 457(2):181–90. doi: 10.1016/j.ydbio.2019.03.005
94. Jeong J-Y, Kwon H-B, Ahn J-C, Kang D, Kwon S-H, Park JA, et al. Functional and developmental analysis of the blood–brain barrier in zebrafish. Brain Res Bull (2008) 75(5):619–28. doi: 10.1016/j.brainresbull.2007.10.043
95. O'Brown NM, Pfau SJ, Gu C. Bridging barriers: a comparative look at the blood-brain barrier across organisms. Genes Dev (2018) 32(7-8):466–78. doi: 10.1101/gad.309823.117
96. Ando K, Fukuhara S, Izumi N, Nakajima H, Fukui H, Kelsh RN, et al. Clarification of mural cell coverage of vascular endothelial cells by live imaging of zebrafish. Development (2016) 143(8):1328–39. doi: 10.1242/dev.132654
97. Lawson ND, Weinstein BM. In vivo imaging of embryonic vascular development using transgenic zebrafish. Dev Biol (2002) 248(2):307–18. doi: 10.1006/dbio.2002.0711
98. Xie J, Farage E, Sugimoto M, Anand-Apte B. A novel transgenic zebrafish model for blood-brain and blood-retinal barrier development. BMC Dev Biol (2010) 10(1):76. doi: 10.1186/1471-213X-10-76
99. Johnson K, Barragan J, Bashiruddin S, Smith CJ, Tyrrell C, Parsons MJ, et al. Gfap-positive radial glial cells are an essential progenitor population for later-born neurons and glia in the zebrafish spinal cord. Glia (2016) 64(7):1170–89. doi: 10.1002/glia.22990
100. Casey MJ, Stewart RA. Pediatric cancer models in zebrafish. Trends Cancer (2020) 6(5):407–18. doi: 10.1016/j.trecan.2020.02.006
101. Modzelewska K, Boer Elena F, Mosbruger Timothy L, Picard D, Anderson D, Miles Rodney R, et al. MEK inhibitors reverse growth of embryonal brain tumors derived from oligoneural precursor cells. Cell Rep (2016) 17(5):1255–64. doi: 10.1016/j.celrep.2016.09.081
102. Solin SL, Shive HR, Woolard KD, Essner JJ, McGrail M. Rapid tumor induction in zebrafish by TALEN-mediated somatic inactivation of the retinoblastoma1 tumor suppressor rb1. Sci Rep (2015) 5(1):13745. doi: 10.1038/srep13745
103. Zhang B, Shimada Y, Kuroyanagi J, Nishimura Y, Umemoto N, Nomoto T, et al. Zebrafish xenotransplantation model for cancer stem-like cell study and high-throughput screening of inhibitors. Tumor Biol (2014) 35(12):11861–9. doi: 10.1007/s13277-014-2417-8
104. van der Ent W, Burrello C, Teunisse AFAS, Ksander BR, van der Velden PA, Jager MJ, et al. Modeling of human uveal melanoma in zebrafish xenograft embryos. Invest Ophthalmol Visual Sci (2014) 55(10):6612–22. doi: 10.1167/iovs.14-15202
105. Bansal N, Bartucci M, Yusuff S, Davis S, Flaherty K, Huselid E, et al. BMI-1 targeting interferes with patient-derived tumor-initiating cell survival and tumor growth in prostate cancer. Clin Cancer Res (2016) 22(24):6176–91. doi: 10.1158/1078-0432.CCR-15-3107
106. Jung D-W, Oh E-S, Park S-H, Chang Y-T, Kim C-H, Choi S-Y, et al. A novel zebrafish human tumor xenograft model validated for anti-cancer drug screening. Mol BioSyst (2012) 8(7):1930–9. doi: 10.1039/c2mb05501e
107. Cabezas-Sáinz P, Pensado-López A, Sáinz B Jr., Sánchez L. Modeling cancer using zebrafish xenografts: Drawbacks for mimicking the human microenvironment. Cells (2020) 9(9):1–29. doi: 10.3390/cells9091978
108. Cabezas-Sainz P, Guerra-Varela J, Carreira MJ, Mariscal J, Roel M, Rubiolo JA, et al. Improving zebrafish embryo xenotransplantation conditions by increasing incubation temperature and establishing a proliferation index with ZFtool. BMC Cancer (2018) 18(1):3. doi: 10.1186/s12885-017-3919-8
109. Allen M, Bjerke M, Edlund H, Nelander S, Westermark B. Origin of the U87MG glioma cell line: Good news and bad news. Sci Trans Med (2016) 8(354):354re3–re3. doi: 10.1126/scitranslmed.aaf6853
110. Valkenburg KC, de Groot AE, Pienta KJ. Targeting the tumour stroma to improve cancer therapy. Nat Rev Clin Oncol (2018) 15(6):366–81. doi: 10.1038/s41571-018-0007-1
111. Tang-Schomer MD, Chandok H, Wu W-B, Lau CC, Bookland MJ, George J. 3D patient-derived tumor models to recapitulate pediatric brain tumors in vitro. Trans Oncol (2022) 20:101407. doi: 10.1016/j.tranon.2022.101407
112. Roper SJ, Linke F, Scotting PJ, Coyle B. 3D spheroid models of paediatric SHH medulloblastoma mimic tumour biology, drug response and metastatic dissemination. Sci Rep (2021) 11(1):4259. doi: 10.1038/s41598-021-83809-6
113. Brüningk SC, Rivens I, Box C, Oelfke U, ter Haar G. 3D tumour spheroids for the prediction of the effects of radiation and hyperthermia treatments. Sci Rep (2020) 10(1):1653. doi: 10.1038/s41598-020-58569-4
114. Brabetz S, Leary SES, Gröbner SN, Nakamoto MW, Şeker-Cin H, Girard EJ, et al. A biobank of patient-derived pediatric brain tumor models. Nat Med (2018) 24(11):1752–61. doi: 10.1038/s41591-018-0207-3
115. Quail DF, Joyce JA. The microenvironmental landscape of brain tumors. Cancer Cell (2017) 31(3):326–41. doi: 10.1016/j.ccell.2017.02.009
116. Northcott JM, Dean IS, Mouw JK, Weaver VM. Feeling stress: The mechanics of cancer progression and aggression. Front Cell Dev Biol (2018) 6:17. doi: 10.3389/fcell.2018.00017
117. Sontheimer-Phelps A, Hassell BA, Ingber DE. Modelling cancer in microfluidic human organs-on-chips. Nat Rev Cancer (2019) 19(2):65–81. doi: 10.1038/s41568-018-0104-6
118. Vatine GD, Barrile R, Workman MJ, Sances S, Barriga BK, Rahnama M, et al. Human iPSC-derived blood-brain barrier chips enable disease modeling and personalized medicine applications. Cell Stem Cell (2019) 24(6):995–1005.e6. doi: 10.1016/j.stem.2019.05.011
119. Park TE, Mustafaoglu N, Herland A, Hasselkus R, Mannix R, FitzGerald EA, et al. Hypoxia-enhanced blood-brain barrier chip recapitulates human barrier function and shuttling of drugs and antibodies. Nat Commun (2019) 10(1):2621. doi: 10.1038/s41467-019-10588-0
120. Linville RM, DeStefano JG, Sklar MB, Xu Z, Farrell AM, Bogorad MI, et al. Human iPSC-derived blood-brain barrier microvessels: validation of barrier function and endothelial cell behavior. Biomaterials (2019) 190(191):24–37. doi: 10.1016/j.biomaterials.2018.10.023
121. Grifno GN, Farrell AM, Linville RM, Arevalo D, Kim JH, Gu L, et al. Tissue-engineered blood-brain barrier models via directed differentiation of human induced pluripotent stem cells. Sci Rep (2019) 9(1):13957. doi: 10.1038/s41598-019-50193-1
122. Workman MJ, Svendsen CN. Recent advances in human iPSC-derived models of the blood-brain barrier. Fluids Barriers CNS (2020) 17(1):30. doi: 10.1186/s12987-020-00191-7
123. Wolff A, Antfolk M, Brodin B, Tenje M. In vitro blood–brain barrier models–an overview of established models and new microfluidic approaches. J Pharm Sci (2015) 104(9):2727–46. doi: 10.1002/jps.24329
124. Raut S, Bhalerao A, Noorani B, Cucullo L. In vitro models of the blood–brain barrier. In: Stone N, editor. The blood-brain barrier: Methods and protocols. New York, NY: Springer US (2022). p. 25–49.
125. Alcendor DJ, Block Iii FE, Cliffel DE, Daniels JS, Ellacott KLJ, Goodwin CR, et al. Neurovascular unit on a chip: implications for translational applications. Stem Cell Res Ther (2013) 4(1):S18. doi: 10.1186/scrt379
126. Peng B, Hao S, Tong Z, Bai H, Pan S, Lim KL, et al. Blood-brain barrier (BBB)-on-a-chip: a promising breakthrough in brain disease research. Lab Chip (2022) 22(19):3579–602. doi: 10.1039/D2LC00305H
127. DePalma TJ, Sivakumar H, Skardal A. Strategies for developing complex multi-component in vitro tumor models: Highlights in glioblastoma. Adv Drug Deliv Rev (2022) 180:114067. doi: 10.1016/j.addr.2021.114067
128. Seo S, Nah S-Y, Lee K, Choi N, Kim HN. Triculture model of In vitro BBB and its application to study BBB-associated chemosensitivity and drug delivery in glioblastoma. Adv Funct Mater (2022) 32(10):2106860. doi: 10.1002/adfm.202106860
129. Nguyen D-HT, Lee E, Alimperti S, Norgard RJ, Wong A, Lee JJ-K, et al. A biomimetic pancreatic cancer on-chip reveals endothelial ablation via ALK7 signaling. Sci Adv (2019) 5(8):eaav6789. doi: 10.1126/sciadv.aav6789
130. Kutys ML, Polacheck WJ, Welch MK, Gagnon KA, Koorman T, Kim S, et al. Uncovering mutation-specific morphogenic phenotypes and paracrine-mediated vessel dysfunction in a biomimetic vascularized mammary duct platform. Nat Commun (2020) 11(1):3377. doi: 10.1038/s41467-020-17102-x
131. Ma Q, Ma H, Xu F, Wang X, Sun W. Microfluidics in cardiovascular disease research: state of the art and future outlook. Microsyst Nanoeng (2021) 7(1):19. doi: 10.1038/s41378-021-00245-2
132. Miny L, Maisonneuve BGC, Quadrio I, Honegger T. Modeling neurodegenerative diseases using In vitro compartmentalized microfluidic devices. Front Bioeng Biotechnol (2022) 10:919646. doi: 10.3389/fbioe.2022.919646
133. Ainscough AJ, Smith TJ, Haensel M, Rhodes CJ, Fellows A, Whitwell HJ, et al. An organ-on-chip model of pulmonary arterial hypertension identifies a BMPR2-SOX17-prostacyclin signalling axis. Commun Biol (2022) 5(1):1192. doi: 10.1038/s42003-022-04169-z
Keywords: medulloblastoma, pediatric brain tumor, blood brain barrier, neurovascular unit, zebrafish, endothelial cells, microfluidics
Citation: Morris EK, Daignault-Mill S, Stehbens SJ, Genovesi LA and Lagendijk AK (2023) Addressing blood-brain-tumor-barrier heterogeneity in pediatric brain tumors with innovative preclinical models. Front. Oncol. 13:1101522. doi: 10.3389/fonc.2023.1101522
Received: 17 November 2022; Accepted: 06 January 2023;
Published: 26 January 2023.
Edited by:
Raelene Endersby, University of Western Australia, AustraliaReviewed by:
Shuhua Bai, Husson University, United StatesTimothy Phoenix, University of Cincinnati, United States
Copyright © 2023 Morris, Daignault-Mill, Stehbens, Genovesi and Lagendijk. This is an open-access article distributed under the terms of the Creative Commons Attribution License (CC BY). The use, distribution or reproduction in other forums is permitted, provided the original author(s) and the copyright owner(s) are credited and that the original publication in this journal is cited, in accordance with accepted academic practice. No use, distribution or reproduction is permitted which does not comply with these terms.
*Correspondence: Laura A. Genovesi, bC5nZW5vdmVzaUB1cS5lZHUuYXU=; Anne K. Lagendijk, YS5sYWdlbmRpamtAdXEuZWR1LmF1