- 1Department of Pathology and Microbiology, University of Nebraska Medical Center, Omaha, NE, United States
- 2Eppley Institute for Research in Cancer and Allied Diseases, Omaha, NE, United States
- 3Fred & Pamela Buffett Cancer Center, University of Nebraska Medical Center, Omaha, NE, United States
One of the greatest challenges in improving prostate cancer (PCa) survival is in designing new therapies to effectively target bone metastases. PCa regulation of the bone environment has been well characterized; however, bone-targeted therapies have little impact on patient survival, demonstrating a need for understanding the complexities of the tumor-bone environment. Many factors contribute to creating a favorable microenvironment for prostate tumors in bone, including cell signaling proteins produced by osteoid cells. Specifically, there has been extensive evidence from both past and recent studies that emphasize the importance of chemokine signaling in promoting PCa progression in the bone environment. Chemokine-focused strategies present promising therapeutic options for treating bone metastasis. These signaling pathways are complex, with many being produced by (and exerting effects on) a plethora of different cell types, including stromal and tumor cells of the prostate tumor-bone microenvironment. This review highlights an underappreciated molecular family that should be interrogated for treatment of bone metastatic prostate cancer (BM-PCa).
Introduction
PCa is the second most common cancer, is the second leading cause of cancer death in American men, and over 34,000 men are predicted to die of PCa in 2023 (1). Up to 90% of advanced prostate cancers metastasize to bone, and there are currently no curative therapies for BM-PCa (2). Interactions between BM-PCa cells and cells of the bone microenvironment cooperate to drive tumor growth in bone. Specifically, BM-PCa cells are known to produce factors that alter the normal bone remodeling cycle (3, 4).
During normal bone remodeling, mononuclear cells fuse to form multinuclear osteoclasts which secrete hydrogen ions and hydrolytic enzymes, including proteases such as collagenase and cathepsin k, to induce acid and enzyme-mediated bone resorption (5, 6). This releases bone-sequestered growth factors, including transforming growth factor-beta (TGF-β) and insulin-like growth factor (IGF)-1, and stimulates the differentiation of mesenchymal stem cells into bone-forming osteoblasts, which secrete extracellular matrix proteins and deposit hydroxyapatite mineral to generate new bone matrix (5, 6). These processes of osteolysis and osteogenesis are coupled, with bone resorption promoting the differentiation of MSCs into osteoblasts and osteoblast-derived factors, specifically receptor activator of nuclear factor-kappa B ligand (RANKL), driving osteoclastogenesis. This coupling allows these two processes to balance each other and the net bone mass to remain constant (5, 6).
PCa cells in the bone microenvironment disrupt this balance by: 1) over-stimulating osteoblasts to produce new bone, resulting in the production of a weaker woven type of newly formed bone, and 2) by promoting osteoclastogenesis, which leads to excessive osteolysis and the release of large amounts of bone-sequestered growth factors, such as TGF-β, which promote BM-PCa disease progression (3, 4, 7, 8). This hijacking of the normal bone remodeling process not only drives tumor growth in bone, but also leads to skeletal related adverse events such as pain, spinal cord compression and increased risk of fractures from the excessive bone resorption and increased frailty of cancer-induced bone (2, 9, 10).
Approved therapies, such as bisphosphonates and Denosumab (a monoclonal antibody to RANKL), target osteoclasts either by inducing of osteoclast cell death or by inhibiting osteoclastogenesis, respectively (11, 12). While multiple targeted therapies, including osteoclast-targeted therapies have been designed to block this process of tumor-induced bone remodeling, these have failed to extend patient survival (13). Thus, a greater understanding of the crosstalk between BM-PCa cells and other osteoid cells is necessaru to design effective new therapies.
A unique feature of PCa, in contrast to other types of bone metastasis, excessive osteosclerosis regulated by interations with osteoid cells, such as mesenchymal stromal cells, osteoblasts, and osteocytes. Mesenchymal stromal cells (MSCs) were originally isolated from bone marrow by Friedenstein and characterized as an adherent fibroblast-like cell type capable of maturing into bone-forming cells (14). MSCs have been classified as multipotent adult stem cells capable of differentiating along three mesodermal lineages into adipocytes, osteocytes, and chondrocytes and are therefore also known as mesenchymal stem cells (15–17). Studies have observed significant heterogeneity in populations of MSCs, including differences in growth rates, gene expression, and differentiation potential with variability attributed to donor age, tissue source, culture conditions, and other still unidentified genetic and environmental factors (16, 18). In the bone microenvironment, MSCs are important in the process of osteogenesis by differentiating into osteoblasts. Osteoblasts are active bone matrix-secreting cells. Osteoblasts sit on nonmineralized surfaces in bone and secrete extracellular matrix proteins, hydroxyapatite for matrix mineralization, and growth factors and cytokines that stimulate new bone formation. Eventually, osteoblasts become either bone lining cells or mature osteocytes embedded in the bone matrix they have produced, where they coordinate responses to mechanical stress by stimulating bone resorption and replacement (19).
BM-PCa cells are known to interact with all three of these types of osteoid cells, and many different factors derived from these bone cells have been characterized to drive BM-PCa progression (20–26). While these include a variety of growth factors and cytokines, this review will specifically highlight the impact of a subtype of cytokines known as chemokines, found in abundance in the tumor microenvironment.
Studies show that MSCs, osteoblasts, and osteocytes produce many different types of chemokines, and their expression of several chemokines is altered following exposure to BM-PCa cells (20, 27–29). Despite substantial evidence of the importance of chemokines, the complexity of their functions has made clinical translation difficult. Nonetheless, this review aims to re-introduce the possibility of targeting these chemokines and their receptors in the treatment of BM-PCa patients.
Osteoid-derived chemokines in BM-PCa
Chemokines are small cytokine proteins classically characterized by their ability to induce leukocyte migration via G protein coupled receptor signaling. This large family of signaling ligands currently consists of 50 known ligands and is divided into four families (XC, CXC, CC, CX3C) based on the position of conserved cysteine residues (27, 30, 31). Although initially classified as chemokines based on their chemotactic functions, many of these signaling ligands have roles in several cellular processes, including bone remodeling, angiogenesis, as well as tumor cell proliferation, survival, invasion, migration, stemness, chemoresistance, and immune evasion (32–37). The chemokines discussed in below have been shown to play important roles in driving BM-PCa growth, survival, migration & invasion, chemoresistance, and tumor-induced osteolysis (summarized in Figure 1).
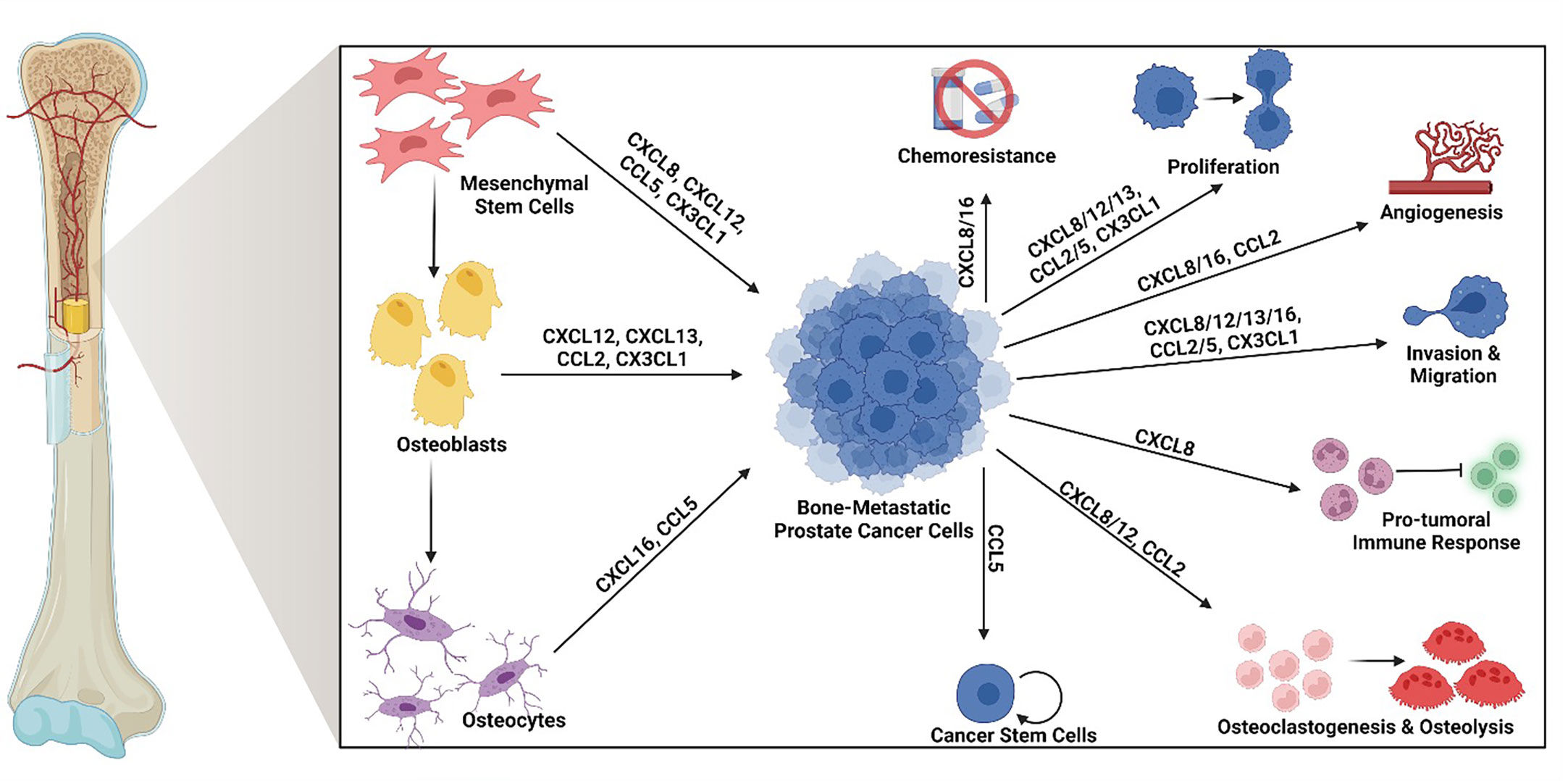
Figure 1 Osteoid cell-derived chemokines and their roles in bone-metastatic prostate cancer. Many chemokines produced by osteoid cells in the bone promote bone-metastatic prostate cancer progression. Specifically, mesenchymal stem cells produce CXCL8, CXCL12, CCL5, and CX3CL1; osteoblasts produce CXCL12, CXCL13, CCL2, and CX3CL1, and osteocytes produce CXCL16 and CCL5. Among these, CXCL8 and CXCL16 have been found to promote chemoresistance. CXCL8, CXCL12, CXCL13, CCL2, CCL5 and CX3CL1 enhance tumor cell proliferation. CXCL8, CXCL16, and CCL2 contribute tumor-induced angiogenesis. CXCL8, CXCL12, CXCL13, CXCL16, CCL2, CCL5, and CX3CL1 induce tumor cell migration and invasion. CXCL8 drives a pro-tumoral immune response, often involving myeloid cell suppression of anti-tumor effector cells. CXCL8, CXCL12, and CCL2 induce osteoclastogenesis and tumor-induced osteolysis. CCL5 increases the population of cancer stem cells. Created by Biorender.com.
CXCL8: CXCL8 (also known as interleukin-8), is a pro-inflammatory chemokine originally identified as a neutrophil chemotactic factor that signals through CXCR1 and CXCR2 to induce neutrophil migration out of the bone marrow and toward sites of injury and inflammation (38, 39). In the context of BM-PCa, CXCL8 was shown to be upregulated in MSCs in response to BM-PCa cells (28). Likewise, CXCL8 also directly promotes osteoclastogenesis (40, 41) in support of extensive evidence that it is important in PCa-induced bone remodeling. Further, in PCa patients, high serum levels of CXCL8 positively correlated with higher Gleason score and disease progression (42, 43). Additionally, one study found high serum CXCL8 correlated with the presence of bone metastases in patients (44). Higher PCa patient serum CXCL8 levels were also associated with an increase in T-cell suppressive myeloid-derived suppressor cells (MDSCs) (45).
Preclinical studies in BM-PCa revealed that CXCL8 promotes in vivo tumor growth and angiogenesis of PC3, an osteolytic PCa cell line derived from a human bone metastasis (46, 47). In vitro studies showed CXCL8 to promote BM-PCa cell migration and PCa-induced osteoclastogenesis (48–50). Another group looking at factors overexpressed by an osteogenic PCa xenograft identified CXCL8, which suggests a role for CXCL8 in osteogenesis in addition to its previously found role in promoting osteolysis (51). In another study, CXCL8 upregulated PCa cell expression of bone sialoprotein (BSP), an extracellular bone matrix protein, and increased PCa invasion and adhesion to bone chips in vitro, showing PCa cells can contribute to bone remodeling by producing bone matrix proteins and that CXCL8 has a role in this process as well as in the process of PCa metastasis to bone (52). The role of CXCL8 in bone remodeling and tumor progression makes it ideal for therapeutic targeting.
CXCL12: CXCL12 (or Stromal Derived Factor-1α (SDF-1α)) is best known for signaling the retention of hematopoietic stem cells and innate immune cells in the bone marrow niche through its receptor CXCR4 (53, 54). Multiple studies have also shown recruitment of osteoclast pre-cursors by CXCL12, which upregulated the secretion of osteolytic enzymes and led to increased bone resorption (55–57). In addition to its role in osteolysis, CXCL12/CXCR4 signaling is important in the differentiation of MSCs into osteoblasts, with targeted genetic deletion of either the receptor CXCR4 or its ligand CXCL12 resulting in impaired osteoblast differentiation and decreased bone formation in mouse models (58–61).
CXCL12 may also have an indirect impact on PCa migration, invasion, and angiogenesis through altered gene expression of matrix metalloproteinases, including of MMP-1, MMP-3, MMP-9, and MMP-14 in PCa cells (62, 63). Overexpression of MMP-1/collagenase-1 in PCa cells has been shown to increase migration in vitro and incidence of metastasis in mouse models (64–66). Genetic silencing of MMP-3, also referred to as stromelysin-1, reduced tumor progression and angiogenesis in mouse models of BM-PCa, and MMP-3 has been shown to play a role in migration in other cancer types, including breast cancer and osteosarcoma (67–69). Inhibition of MMP-9/gelatinase B reduced PCa invasion in vitro and tumor growth, invasion, and angiogenesis in vivo (70–72). MMP-14 is reported to be important in the migration of multiple types of cancer, with mutations in MMP-14 leading to a decrease in PCa migration in vitro and peptide inhibitors of MMP-14 decreasing breast cancer invasion in vitro and metastasis in vivo (73, 74). These studies suggest CXCL12 promotes PCa migration, invasion, and angiogenesis via altered MMP gene expression, in addition to the direct chemotactic effect of CXCL12 on PCa cells.
CXCL12 also plays an important role in PCa in the bone with increased expression of CXCR4 observed in bone-metastatic PCa cell lines and patient samples. Further, in patients with metastatic PCa, high CXCR4 expression correlated with decreased survival (75). Pertinent to BM-PCa, CXCR4 is upregulated in PCa cells cultured with bone stromal cells (76). Additionally, CXCL12 is expressed by osteoblasts and may mediate the increased homing of PCa cells to osteoblast-rich regions of the bone, such as the lateral endocortical surfaces (77, 78). Inhibition of CXCR4 blocked the increase in number of BM-PCa cells found in osteoblast-dense niches in mouse tibia following intracardiac injection, suggesting that CXCL12/CXCR4 signaling may play an important role in PCa metastasis to bone (78). Likewise, in vivo treatment with CXCR4 antagonists reduced intratibial growth and tumor-induced osteolysis of PC3 cells, whereas overexpression of CXCR4 increased PC3 tumor growth in bone and osteolysis (76, 79, 80).
MSCs also produce CXCL12, which can induce PCa cell migration in vitro (62, 63, 77, 81, 82). A previous study suggested a role for CXCL12 in the creation of a pre-metastatic niche in bone through the upregulation of CXCL12 expression in MSCs by PCa exosome-derived pyruvate kinase M2 (PKM2) and demonstrated a CXCR4-dependent increase in metastatic seeding and growth of PC3 cells in mice treated with PCa exosomes (83). Collectively, these findings demonstrate the CXCL12/CXCR4 signaling axis to be a viable target for treating PCa growth in bone.
CCL2: One of the most studied chemokines, CCL2 (or Monocyte Chemoattractant Protein 1 (MCP-1)), was named for its ability to recruit monocytes to sites of injury or infection; however, dendritic cells, memory T cells, basophils, and NK cells can be recruited by this pro-inflammatory chemokine through signaling via its receptor CCR2 (84–90). Several different cell types produce CCL2, including monocytes, endothelial cells, epithelial cells, smooth muscle cells, fibroblasts, and osteoblasts (91–95).
In addition to its role in inflammation, CCL2 also has an important role in bone remodeling. Given that monocytes are precursors for osteoclasts and that CCL2 is important for monocyte recruitment, it is not surprising that CCL2 and CCR2 KO mice have decreased numbers of osteoclasts, which resulted in decreased bone resorption and increased bone mass (96–99). However, other studies suggest the role of CCL2 in bone remodeling may be more complex. One study found that mechanical stress upregulated CCL2 expression in osteoblasts via ERK1/2 signaling and led to increased bone formation in mice, suggesting CCL2 may also be involved in osteogenesis in addition to osteoclastogenesis (95). Osteoblast expression of CCL2 was discovered to be induced by a number of different factors including growth differentiation factor-15 (GDF15), a member of the TGF-β family of ligands that is highly expressed by PCa cells. High serum GDF15 levels are associated with bone metastasis, suggesting that GDF15 may contribute to high levels of CCL2 production by osteoblasts in BM-PCa (29, 100). Another factor produced by PCa cells that can upregulate CCL2 is parathyroid hormone-related protein (PTHrP), which increases CCL2 expression in osteoblasts via the transcription factors C/EBPβ and NFκB (101). These studies suggest that PCa cells in the bone microenvironment manipulate osteoblast expression of CCL2 to drive tumor-induced bone remodeling.
Multiple studies show CCL2 recruits monocytes to BM-PCa tumors and increases the number of tumor-associated osteoclasts, such that CCL2 inhibition decreases tumor-induced bone resorption in mice (102, 103). Recruited monocytes were also found to mature into tumor-associated macrophages (TAMs), which promote cancer progression through multiple mechanisms (102, 104–106), including promotion of tumor proliferation, cancer cell stemness, metastasis, and therapy resistance, while also suppressing anti-tumor immune responses (107–111).
In patients, CCR2 expression levels positively correlate with increasing PCa stage, and CCL2 expression is associated with reduced survival (101, 112). Additionally, serum CCL2 levels were increased in those with bone metastases compared to localized PCa (101). These findings demonstrate that CCL2 may play a particularly key role in PCa bone metastases, where it can contribute to tumor growth, invasion, angiogenesis, bone remodeling, and recruitment of pro-tumoral immune cells.
CCL5: The pro-inflammatory chemokine CCL5, initially named Regulated on Activation, Normal T cell Expressed and Secreted (RANTES), was first identified as a T cell chemoattractant (113). It was later found to recruit monocytes, dendritic cells, NK cells, basophils, eosinophils, and mast cells as well (114–119). CCL5 can bind and signal through many receptors, including CCR1, CCR3, CCR4, and CCR5 (with highest affinity) (120, 121). In addition to its role in inflammation, CCL5 also has a role in bone remodeling and is known to recruit osteoblasts (122). Specifically, total KO of CCL5 in mice results in decreased bone mass, although this phenotype disappears as these mice age, likely through compensatory signaling through other chemokines not identified in this study (99).
Surprisingly, there is little to no evidence of CCL5 in osteoblast function. However, in osteocytes, mechanical pressure was found to upregulate expression of CCL5, which was partially responsible for an increase in BM-PCa in vitro growth and invasion by osteocyte-derived factors (123).
In PCa, multiple cell types have been found to increase tumor cell proliferation, migration, invasion, chemoresistance, and cancer stem cell populations via secretion of CCL5 (124). In vivo, CCR5 inhibitor treatment reduced bone metastasis following intracardiac injection of PCa cells in mice, suggesting CCR5 may be a potential therapeutic target to reduce bone metastasis in PCa patients (125). Further support for the clinical relevance of CCL5 in PCa patients comes from research showing that CCL5 expression is increased in tissue samples from PCa patients compared to healthy controls and that increasing serum CCL5 levels correlate with increasing Gleason score in PCa patients (110). A separate study comparing gene expression from PCa tissue to BPH tissue found increased expression of both CCL5 and CCR5 in PCa samples (126). These findings demonstrate the impact of CCL5 on many processes essential for PCa progression.
CXCL13: CXCL13, also called B Cell Attracting Chemokine 1 (BCA-1), was discovered as a B-cell chemotactic factor important for the homing of B cells to lymphoid organs (127). While CXCL13 was originally found to be derived from dendritic cells and T follicular helper cells, it was later also found to be produced by osteoblasts and human bone marrow endothelial (HBME) cells and to promote MSC osteoblastic differentiation (127–129).
Since its identification, CXCL13 has been revealed to induce PCa proliferation, migration, and invasion (128, 130, 131). Mechanistically, in vitro assays showed that CXCL13 promotes PCa proliferation through c-Jun N-terminal kinase (JNK) signaling and invasion via protein kinase B (Akt) and extracellular signal-regulated kinase (ERK)-1/2 signaling (130). CXCL13 also promoted BM-PCa adhesion to HBME cells in vitro, suggesting a role for CXCL13 in the extravasation of PCa cells during metastasis (127, 128).
Serum CXCL13 levels are increased in patients with PCa compared to healthy controls and patients with benign prostatic hyperplasia (BPH) or high-grade prostatic intraepithelial neoplasia (HGPIN). Notably, CXCL13 is the only ligand for the receptor CXCR5, and CXCR5 expression has been shown to positively correlate with PCa grade (128). CXCL13 expression has also been reported to be upregulated by interleukin 6 (IL-6) through an unknown mechanism, which may be significant in BM-PCa, as IL-6 levels are observed to be higher in PCa patients with bone metastases (128, 132, 133). These studies provide support for an important role for CXCL13 in PCa bone metastases.
CXCL16: CXCL16 is a pro-inflammatory chemokine that can be upregulated by the cytokines interferon-gamma (IFN-γ) and tumor necrosis factor-alpha (TNF-α) and has been shown to recruit CXCR6 expressing T helper 1 (Th1) cells, cytotoxic CD8+ T effector cells, plasma cells, NK cells, and NKT cells (134–140). CXCL16 is produced as a transmembrane protein that is cleaved by a disintegrin and metalloproteinase domain-containing protein 10 (ADAM10) to release the soluble chemokine (135, 141). Its receptor CXCR6, whose only ligand is CXCL16, was previously found to be a co-receptor for HIV infection (142). In some cancers, such as colorectal and renal cancer, CXCL16 is associated with tumor-infiltrating lymphocytes and good prognosis (143–145). However, in PCa, CXCL16 has been linked to cancer progression and metastasis (146). In patients, increased expression of the CXCL16 and CXCR6 were observed in tissue samples of tumors with higher Gleason scores and in metastatic PCa cell lines (147–149).
Several bone stromal cells, including MSCs, monocytes, fibroblasts, and osteocytes have all been shown to express CXCL16 (135, 142, 150–153). In primary tumors, PCa-derived CXCL16 recruits CXCR6-expressing MSCs which upregulate PCa CXCR4 expression, induce EMT markers via CXCL12 secretion, and promote tumor growth in vivo in a subcutaneous model (154). Intracardiac injection of mouse PCa cells into CXCR6 knockout (KO) mice resulted in reduced bone metastasis compared to wildtype mice, suggesting that CXCR6 has a role in the homing of PCa to bone (154). CXCL16 was also found to induce the expression of pro-angiogenic factors such as vascular endothelial growth factor (VEGF) in BM-PCa cells and to promote chemoresistance to docetaxel (147, 155). BM-PCa cell invasion and VEGF secretion was found to be dependent on AKT/Mammalian Target of Rapamycin (mTOR) signaling (154). These studies bring to light the importance of CXCL6/CXCR6 signaling in driving PCa progression by promoting metastasis, angiogenesis, and chemoresistance.
CX3CL1: CX3CL1 (fractalkine), the only identified member of the CX3C family of chemokines, is produced as a membrane bound protein which, and like CXCL16, can undergo proteolytic cleavage to release a soluble chemotactic domain. It is upregulated by IFN-γ and TNF-α and functions as a chemoattractant for Th1 cells, NK cells, and monocytes that express the receptor CX3CR1 (156–159). In the bone, membrane-bound CX3CL1 is detected on BM-MSCs, osteoblasts, and bone marrow endothelial cells, and the soluble form of cleaved CX3CL1 is detected in bone marrow (160).
CX3CL1 has been shown to have an important role in bone resorption as a chemokine that recruits monocyte osteoclast precursors and promotes osteoclastogenesis (157, 161–165). Treatment of mice with CX3CR1 neutralizing antibody decreased bone resorption in vivo, and CX3CR1-deficient pre-osteoclasts failed to differentiate in vitro. Osteoblasts also express the receptor CX3CR1, and CX3CL1 has been shown to have an impact on osteoblast differentiation. CX3CR1-deficient osteoblasts have delayed induction of a key osteoblastic transcription factor runt-related transcription factor 2 (RUNX2), but upregulation of a late osteoblastic transcription factor osterix (OSX), as well as decreased calcium deposition in vitro, suggesting CX3CL1/CX3CR1 signaling regulates the temporal expression of transcription factors required for normal osteoblast differentiation. CX3CR1-deficient osteoblasts also have reduced expression of osteoclastogenic factor RANKL. Treatment of wildtype osteoblasts with recombinant CX3CL1 induces both RUNX2 and OSX but decreases expression of several bone matrix proteins, including osteonectin, osteocalcin, and osteopontin (161). Thus, the impact of CX3CL1/CX3CR1 signaling in osteoblasts is complex, but it clear that this chemokine plays an important role in bone remodeling.
CX3CR1 is also expressed in prostate epithelial cells. CX3CR1 expression is detected in both normal prostate epithelium and PCa tumors, with higher expression observed in PCa cells compared to healthy prostate epithelial cells (160, 166). Levels of soluble CX3CL1 in the bone marrow were found to be androgen regulated, with the potent androgen dihydrotestosterone upregulating cleavage of CX3CL1 from the plasma membrane of bone cells (160). This may be through upregulation of a disintegrin and metalloproteases (ADAM) enzymes, which have been shown to cleave CX3CL1 and to be upregulated by dihydrotestosterone (167–170). CX3CL1 is suggested to play a role in the extravasation of circulating PCa cells during metastasis to bone. It is expressed on the luminal side of bone marrow endothelial cells, and PC3 cells have been found to adhere to HBME cells in vitro under flow conditions recapitulating the shear force measured in microvessels in vivo, with this adhesion blocked by CX3CL1 neutralizing antibody (171). CX3CL1 also induces BM-PCa expression of EMT markers and migration and invasion in vitro (166, 171, 172). Overexpression of CX3CR1 in BM-PCa cells increased proliferation and decreased apoptosis (166). In mice, intracardiac injection of BM-PCa cells overexpressing CX3CR1 led to increased incidence of spinal metastases compared to mice injected with control BM-PCa cells (166). In PCa patients, serum CX3CL1 levels show a positive correlation with the presence of spinal metastases, suggesting a role for CX3CL1 in the process of PCa metastasis to bone (166, 173).
Chemokine regulation of bone cancers and bone metastasis
Although we are aware that PCa can progress to development of visceral and soft tissue metastases, bone is the most frequent site of PCa metastasis. Chemokines have are involved in other bone and bone-metastatic cancers as well, suggesting that they may be key for understanding how to target cancer growth specifically in bone. For example, CXCL8 is involved in tumor-induced osteolysis in bone-metastatic breast cancer, and breast cancer cells upregulated osteoblast expression of CXCL8 (41, 174–177). CXCL8 was also increased in serum samples from lung cancer patients with bone metastases and was involved in lung cancer-induced osteoclastogenesis in vitro (178, 179). In osteosarcoma, CXCL8 promotes migration and activates Akt signaling to promote survival in vitro, and CXCL8 inhibition or knock down (KD) of the receptor CXCR1 decreases lung metastases in vivo (180–182). CXCL8 serum levels in patients with multiple myeloma, a cancer originating in bone marrow plasma cells, were elevated compared to healthy controls, and multiple myeloma cells upregulated CXCL8 expression in bone stromal cells (183–186). In vitro, CXCL8 promoted multiple myeloma cell survival and tumor-induced osteoclastogenesis (185).
CXCL12 has been reported to recruit multiple types of cancer to bone (187–189). In breast cancer cells, angiopoietin-like protein 2 (ANGPTL2) was found to upregulate CXCR4 and promote bone metastasis in an intracardiac bone metastasis mouse model (190). Among breast cancer cell lines, CXCR4 was more highly expressed by bone metastatic cells (191). In multiple myeloma, CXCL12/CXCR4 signaling has been shown to promote proliferation, migration, invasion, metastasis, chemoresistance, and tumor-induced osteoclastogenesis (192–196). In mice, treatment with CXCR4 antagonist mobilized multiple myeloma cells from the bone marrow and induced cancer cell death (197). In osteosarcoma, CXCL12/CXCR4 signaling promotes cancer progression and metastasis, and CXCR4 expression is associated with poor prognosis in patients (198–204).
CXCL13 and CXCR5 are also overexpressed in breast cancer and associated with increasing stage and metastasis (205, 206). CXCL13/CXCR5 signaling promotes osteosarcoma migration and invasion in vitro (207). CXCR5 is also expressed on bone marrow-metastatic neuroblastoma cells, which migrate toward CXCL13 in vitro, suggesting CXCL13/CXCR5 signaling may be involved in neuroblastoma metastasis to bone marrow (208).
As seen in PCa, primary breast cancer tumors recruit CXCR6 expressing MSCs via CXCL16, and the tumor-associated MSCs then secrete other chemokines, such as CCL5 and CXCL10, that promote invasion and metastasis (209). Lung cancer cells also highly express CXCL16/CXCR6, which promote lung cancer cell migration and invasion in vitro and bone metastasis in vivo (210–212). In osteosarcoma, CXCL16 was found to be upregulated and to induce EMT markers and promote growth and invasion in vitro (213, 214).
CCL2 is associated with increased tumor grade and decreased relapse-free survival in breast cancer patients and promotes bone metastasis and osteolysis in mice (215, 216). Breast cancer cells were also found to upregulate osteoblast expression of CCL2, suggesting that breast cancer cells manipulate osteoid cells in the bone microenvironment to promote osteolysis and tumor progression (177, 217). Lung cancer patients with bone metastases have increased serum CCL2, and shRNA KD of CCL2 in non-small cell lung cancer cells reduced intratibial tumor growth and bone resorption in SCID mice (179). CCL2 also promotes osteosarcoma progression, metastasis, and bone resorption (218–221). In multiple myeloma, CCL2 recruits and polarized macrophages to promote chemoresistance through upregulation of MCP-1-induced protein (MCPIP1) (222, 223).
Last, CX3CL1/CX3CR1 play a role in bone metastasis of other cancers, including breast cancer. Specifically, CX3CR1 can be detected on both healthy and malignant breast epithelial cells, with a greater percentage of cells expressing high levels of CX3CR1 in breast cancer tissues and even higher expression in bone metastases (224–226). Breast cancer cells intracardially injected in total CX3CR1 KO mice had reduced bone metastases compared to wildtype mice, and bone metastases in wildtype mice were reduced with CX3CR1 antagonist treatment (224, 225). Spinal metastases in hepatocellular carcinoma patients also have high CX3CL1 and CX3CR1 expression, and CX3CL1 promoted hepatocellular carcinoma cell migration in vitro and spinal metastasis in vivo (227). Additionally, CX3CL1 is associated with spinal metastasis in lung cancer patients and correlates with poor overall survival (228, 229) and CX3CL1 is also involved in metastasis of cancers originating in the bone (230). One study observed that osteosarcoma cells have increased expression of CX3CL1 compared to healthy osteoblasts (231). These studies confirm the importance of these chemokines in the tumor bone microenvironment, not just in BM-PCa, but also in other bone and bone-metastatic cancers.
Chemokine-focused therapy for treating BM-PCa
Chemokines also represent promising new options as PCa biomarkers. Serum CCL2 and CXCL12 were significantly elevated in patients with localized PCa compared to healthy donors, which suggests they may be valuable for diagnosing PCa but not specifically for identifying high grade or metastatic PCa (232, 233). CCL5 has been found to be elevated in the blood of PCa patients and, unlike CCL2, it is associated with higher Gleason grade and metastases (110). CXCL8 was elevated in PCa patients with bone metastases and associated with higher grade tumors and AR loss (44, 234). Serum CXCL13 levels have been found to be elevated in PCa patients and discovered to be a better predictor of PCa than PSA levels (128, 131).
Since the prostate tumor-bone microenvironment is rich in chemokines, blocking pro-tumorigenic signals from chemokines may be an effective treatment for patients with bone metastases. Currently, the only chemokine receptor inhibitors approved for use in patients are a CCR5 antagonist (Maraviroc) to treat HIV, a CCR4 antagonist (Mogamulizumab) to treat mycosis fungoides, and a small molecule CXCR4 antagonist (Plerixafor) (235–237). Plerixafor is currently approved for use in patients with Non-Hodgkin Lymphoma and Multiple Myeloma to mobilize hematopoietic stem cells in preparation for autologous transplant (237). However, both Maraviroc and Plerixafor are currently being tested in clinical trials for multiple types of solid tumors.
A phase 1 clinical trial (NCT01736813) of Maraviroc in colorectal cancer showed it was well tolerated and induced partial responses, including decreased proliferation markers in biopsies and partial remission of lung metastases in previously refractory tumors (238). Another phase 1 clinical trial (NCT03274804) of Maraviroc combined with the immunotherapy Pembrolizumab also reported a safe toxicity profile and prolonged disease stabilization in metastatic colorectal cancer patients (239, 240). This suggests CCR5 antagonist treatment may be a promising approach to reduce tumor growth in other solid tumors in which CCL5 plays a pro-tumoral role, such as PCa, where Maraviroc was shown to reduce bone metastasis of intracardially injected PCa cells in mice (125). Preclinical studies also show promise for CCL5 antagonists in pancreatic cancer and bone-metastatic breast cancer, and a current phase 1 clinical trial (NCT04721301) is investigating the combination of Maraviroc with other immunotherapies in metastatic colorectal and pancreatic cancer patients (241, 242).
Inhibition of CXCR4 also showed promise, with pre-clinical testing of Plerixafor reducing the establishment of bone metastases in bone-metastatic prostate cancer mouse models, yet it failed to impact the growth of pre-established bone metastases (243). This suggests CXCR4 may be particularly important in the process of metastasis to bone, and inhibition may be effective in preventing bone metastasis but ineffective in patients who already have bone metastases. Another pre-clinical study showed Plerixafor sensitized BM-PCa cells to docetaxel, suggesting possible benefits of including CXCR4 inhibitors in combination treatment approaches (244).
Pre-clinical testing of CCL2 inhibition also decreased bone metastatic prostate tumor growth, both alone and in combination with docetaxel (245, 246). Unfortunately, when tested in a clinical trial (NCT00992186), the monoclonal antibody used to target CCL2 failed to induce long-term suppression of serum CCL2, resulting in no effect on tumor growth (247, 248). Therefore, more work needs to be done to develop and test inhibitors of chemokine signaling before these treatments can be moved into the clinic.
Androgen-targeted therapies and chemokines. The current standard-of-care therapies for BM-PCa are androgen-targeted interventions. Several chemokines may be uniquely important in PCa due to roles of androgen signaling in the mechanism of their effect on cancer cells. For example, the pro-migratory effect of CCL5 on PCa cells occurs via suppression of androgen receptor (AR) by inhibition of AR nuclear translocation and is thus an AR-dependent mechanism (249). Some effects of CXCL12 signaling are also AR-dependent. CXCL12 induces PCa cell proliferation by increasing nuclear accumulation of both AR and its co-regulator steroid receptor coactivator 1 (SRC-1) and upregulating AR/SCR-1-responsive genes (250).
There is also evidence of chemokines acting through suppression of androgen signaling. One study reported that coculture of BM-MSCs with PCa cells upregulated their expression of CCL5, which was shown to block AR nuclear translocation and a resultant increase in cancer stem cells and PCa cell invasiveness (251, 252). In a separate study, suppression of AR signaling was determined to mediate CCL5-induced PCa cell migration towards bone stromal cells derived from a PCa patient bone metastasis (249). Likewise, endothelial cell derived CCL5 was shown to downregulate AR and promote PCa cell invasion in vitro and metastasis in vivo (253). These findings suggest that several pro-tumoral mechanisms of osteoid cell-derived chemokines are enhanced by anti-androgen therapies and promote therapy resistance.
Androgen signaling is also involved in the regulation of chemokine expression. One study found that AR signaling suppressed CXCL8 expression, with androgen deprivation therapy leading to upregulation of CXCL8 (254). Another study reported that AR signaling upregulates expression of CXCL13, with an androgen responsive element (ARE) identified in its enhancer region (131). Androgen signaling also upregulates cleavage of membrane-bound CX3CL1 to increase levels of the soluble chemokine (160).
Additionally, studies have demonstrated a role for AR in regulating CCL2 expression, as siRNA targeting of AR in PCa cells was observed to upregulate CCL2 via STAT3 activation by downregulating an AR-inducible protein inhibitor of activated STAT 3 (PIAS3). Another study found AR inhibitors enzalutamide and bicalutamide upregulated CCL2 via STAT3 in PCa cells, leading them to propose the use of CCL2/CCR2 or STAT3 inhibitors in combination with AR inhibitors to block the pro-tumoral effects of ADT-induced CCL2 (255). Therefore, targeting of these androgen-regulated chemokines may be especially effective in PCa patients.
Bone-focused therapies and chemokine regulation. In addition to androgen-focused therapy, BM-PCa patients also receive specific bone-targeted therapies to treat cancer-induced skeletal events that contribute to poor quality of life. Despite the extensive evidence of chemokine functions in the tumor-bone space, research is lacking on the impact of bone-targeted therapies on osteoid cell-derived chemokines. Since both bisphosphonates and denosumab primarily target osteoclasts, the greatest effect of bone-targeted therapies may be a reduction in osteoclast-derived chemokines. Yet, the contribution of osteoclasts to chemokine production in the BM-PCa microenvironment remains poorly studied. Osteoclasts are known to produce large amounts of CCL9 and slightly smaller amounts of other chemokines, including CCL22, CCL25, and CXCL13, but it is not reported whether BM-PCa upregulates any of these in osteoclasts (256). There is also limited research on the roles of some of these chemokines in BM-PCa. A single study reported that CCL22 induces BM-PCa cell migration, and one study observed that CCL25 promotes BM-PCa chemoresistance (257, 258). Further investigation is required to understand both the roles of osteoclast-derived chemokines in BM-PCa and the impact of bone-targeted therapies on those chemokines.
It is also possible that targeting of bone resorption may alter chemokine expression regulated by bone-sequestered growth factors. For example, TGFβ has been shown to upregulate osteoclast CXCL16 expression, which recruits osteoblasts (259). Reducing the amount of tumor-induced osteolysis would likely then result in fewer osteoblasts in the area, which would consequently reduce the levels of osteoblast-derived chemokines.
Additionally, a few studies have identified bisphosphonate treatment specifically to alter chemokine expression in other cancer types. In bronchoalveolar macrophages, the bisphosphonate zoledronate has been observed to promote upregulation of pro-inflammatory chemokines such as CCL2 and CCL5 in response to immune challenge (260). Yet, in osteosarcoma cells zoledronate downregulated CCL2 (219). However, in osteosarcoma and bone-metastatic breast and prostate cancer cells bisphosphonates downregulated CXCR4, which suppressed CXCL12-CXCR4 signaling-induced invasion though this phenomenon needs to be tested in vivo (261–263). These findings suggest bisphosphonates could be targeting BM-PCa by suppressing pro-tumoral signaling through CXCR4 in addition to blocking tumor-induced osteolysis. The impact of bisphosphonates on chemokine production appears to be cell type-specific and remains to be investigated in osteoid cells of the BM-PCa microenvironment.
It is likely that Denosumab, the monoclonal antibody of RANKL, also alters osteoid cell chemokine signaling in BM-PCa; however, the detailed mechanisms have yet to be discovered. RANKL downregulates expression of CCR2 and CCR5 on macrophages and CXCR6 and CX3CR1 on osteoclasts (256, 264, 265). If these receptors on tumor cells are similarly inhibited by RANKL, then inhibition of RANKL by denosumab would increase their expression and enhance pro-tumoral signaling by the osteoid cell-derived chemokines CCL2, CCL5, CXCL16, and CX3CL1. In this case, the addition of chemokine targeted treatments to denosumab treatment may be effective new combination therapies.
Another bone-targeted treatment, radium 223 dichloride, targets alpha radiation specifically to areas of active bone remodeling (266, 267) and will significantly impact osteoid cells, but changes in chemokine signaling have not been characterized. In the lungs, irradiated MSCs promote metastasis via upregulation of CCL5 (268). Other studies have observed CCL2 to be upregulated following radiation (269–271), but this has not been shown in osteoid cells to date. Therefore, while radium 223 likely suppresses tumor-induced bone formation by reducing osteoblast numbers and function, it may also upregulate pro-tumoral chemokines and osteolysis. Thus, the use of chemokine inhibitors and osteoclast-targeted therapies may block pro-tumoral responses to radium 223.
There is a major gap in understanding how bone targeted and standard of care hormonal therapy impacts chemokine signaling in BM-PCa. Additional research on the impact of bone-targeted therapies could further highlight potential combination treatment approaches for BM-PCa patients.
Conclusion
In this review, we have discussed the importance of chemokines produced by osteoid cells that contribute significantly to PCa progression in the bone microenvironment. However, chemokine signaling in the tumor microenvironments is complex, and production of these chemokines by osteoid cells can also induce other cell types, including the cancer cells and recruited immune cells, to produce additional chemokines that can drive PCa progression. Clearly the research discussed in this review only covers a small part of the true impact of osteoid cell-derived chemokines on BM-PCa, as the ripple effect of many of these chemokines on other cell types and signaling pathways in the tumor microenvironment has yet to be elucidated.
Another area requiring further investigation is the dependence of BM-PCa on osteoid cell-derived chemokines since many of these chemokines can also be produced by the BM-PCa cells themselves (154, 254, 272–275). While it is likely the tumor cells simply take advantage of bone cell-produced chemokines to reduce the need to produce their own, it is also possible osteoid cells may primarily produce different isoforms of some of these chemokines which could differ in potency. For example, studies have shown that two different isoforms of CXCL8 are produced by different cell types and differ in potency (176, 276). The endothelial cell-derived isoform of CXCL8 is reported to have reduced potency in recruiting neutrophils compared to the isoform produced by monocytes and other cells (276). In breast cancer, two human cell lines were shown to each produce a different isoform of CXCL8 with a difference in potency between the two (176). Therefore, it is possible there may a difference in potency between PCa-derived and osteoid cell-derived isoforms of these chemokines. It is also possible that osteoid cells may produce more of these chemokines than the PCa tumor cells. In breast cancer, tumor cells were found to produce only picogram quantities of CCL2, whereas osteoblasts produced nanogram quantities of CCL2 (177). Additionally, it has been shown in osteosarcoma, a bone cancer arising from malignant transformation of osteoblasts, that tumor cell-derived CXCL8 can upregulate MSC expression of CXCL8, which then increases osteosarcoma cell expression of CXCL8, creating a positive feedback loop driving tumor progression (180). However, this has not been shown in PCa to date. Nonetheless, osteoid cell-derived chemokines play a key role in the progression of BM-PCa.
In summary, chemokines derived from osteoid cells in the bone microenvironment play key roles in driving PCa metastasis to bone and the progression of BM-PCa and represent promising new therapeutic targets and potential biomarkers.
Author contributions
All authors listed have made a substantial, direct, and intellectual contribution to the work and approved it for publication.
Funding
LC and CJ were supported by an American Cancer Society Research Scholar Grant (RSG-19-127-01-CSM). LC was additionally supported by funding from the National Cancer Institute (1R01CA274605-01). CJ was additionally supported by funding from the National Cancer Institute, T32 Cancer Biology Training Grant (T32CA009476). This publication’s contents are the sole responsibility of the authors and do not necessarily represent the official views of the NCI.
Conflict of interest
The authors declare that the research was conducted in the absence of any commercial or financial relationships that could be construed as a potential conflict of interest.
Publisher’s note
All claims expressed in this article are solely those of the authors and do not necessarily represent those of their affiliated organizations, or those of the publisher, the editors and the reviewers. Any product that may be evaluated in this article, or claim that may be made by its manufacturer, is not guaranteed or endorsed by the publisher.
References
1. Siegel RL, Miller KD, Fuchs HE, Jemal A. Cancer statistics, 2022. CA Cancer J Clin (2022) 72:7–33. doi: 10.3322/caac.21708
2. Rajpar S, Fizazi K. Bone targeted therapies in metastatic castration-resistant prostate cancer. Cancer J (2013) 19:66–70. doi: 10.1097/PPO.0b013e31827f123e
3. Jin JK, Dayyani F, Gallick GE. Steps in prostate cancer progression that lead to bone metastasis. Int J Cancer (2011) 128:2545–61. doi: 10.1002/ijc.26024
4. Wong SK, Mohamad NV, Giaze TR, Chin KY, Mohamed N, Ima-Nirwana S. Prostate cancer and bone metastases: The underlying mechanisms. Int J Mol Sci (2019) 20(10):2587. doi: 10.3390/ijms20102587
5. Kenkre JS, Bassett J. The bone remodelling cycle. Ann Clin Biochem (2018) 55:308–27. doi: 10.1177/0004563218759371
6. Katsimbri P. The biology of normal bone remodelling. Eur J Cancer Care (Engl) (2017) 26. doi: 10.1111/ecc.12740
7. Hensel J, Thalmann GN. Biology of bone metastases in prostate cancer. Urology (2016) 92:6–13. doi: 10.1016/j.urology.2015.12.039
8. Lin SC, Yu-Lee LY, Lin SH. Osteoblastic factors in prostate cancer bone metastasis. Curr Osteoporos Rep (2018) 16:642–7. doi: 10.1007/s11914-018-0480-6
9. Yong C, Onukwugha E, Mullins CD. Clinical and economic burden of bone metastasis and skeletal-related events in prostate cancer. Curr Opin Oncol (2014) 26:274–83. doi: 10.1097/CCO.0000000000000071
10. Weinfurt KP, Li Y, Castel LD, Saad F, Timbie JW, Glendenning GA, et al. The significance of skeletal-related events for the health-related quality of life of patients with metastatic prostate cancer. Ann Oncol (2005) 16:579–84. doi: 10.1093/annonc/mdi122
11. Jakob T, Tesfamariam YM, Macherey S, Kuhr K, Adams A, Monsef I, et al. Bisphosphonates or RANK-ligand-inhibitors for men with prostate cancer and bone metastases: A network meta-analysis. Cochrane Database Syst Rev (2020) 12:CD013020. doi: 10.1002/14651858.CD013020.pub2
12. Hegemann M, Bedke J, Stenzl A, Todenhofer T. Denosumab treatment in the management of patients with advanced prostate cancer: Clinical evidence and experience. Ther Adv Urol (2017) 9:81–8. doi: 10.1177/1756287216686018
13. Gartrell BA, Coleman R, Efstathiou E, Fizazi K, Logothetis CJ, Smith MR, et al. Metastatic prostate cancer and the bone: Significance and therapeutic options. Eur Urol (2015) 68:850–8. doi: 10.1016/j.eururo.2015.06.039
14. Friedenstein AJ, Chailakhyan RK, Gerasimov UV. Bone marrow osteogenic stem cells: in vitro cultivation and transplantation in diffusion chambers. Cell Tissue Kinet (1987) 20:263–72. doi: 10.1111/j.1365-2184.1987.tb01309.x
15. Bianco P, Robey PG, Simmons PJ. Mesenchymal stem cells: Revisiting history, concepts, and assays. Cell Stem Cell (2008) 2:313–9. doi: 10.1016/j.stem.2008.03.002
16. Phinney DG. Functional heterogeneity of mesenchymal stem cells: implications for cell therapy. J Cell Biochem (2012) 113:2806–12. doi: 10.1002/jcb.24166
17. Ding DC, Shyu WC, Lin SZ. Mesenchymal stem cells. Cell Transplant (2011) 20:5–14. doi: 10.3727/096368910X
18. Elahi KC, Klein G, Avci-Adali M, Sievert KD, MacNeil S, Aicher WK. Human mesenchymal stromal cells from different sources diverge in their expression of cell surface proteins and display distinct differentiation patterns. Stem Cells Int (2016) 2016:5646384. doi: 10.1155/2016/5646384
19. Bilezikian JP, et al. Primer on the metabolic bone diseases and disorders of mineral metabolism. ninth edition. Hoboken NJ, editor. Chichester, West Sussex: Wiley-Blackwell, John Wiley & Sons, Inc Wiley Online Library (2019).
20. Wang W, et al. Prostate cancer promotes a vicious cycle of bone metastasis progression through inducing osteocytes to secrete GDF15 that stimulates prostate cancer growth and invasion. Oncogene (2019) 38(23):4540–59. doi: 10.1038/s41388-019-0736-3
21. Ribelli G, Simonetti S, Iuliani M, Rossi E, Vincenzi B, Tonini G, et al. Osteoblasts promote prostate cancer cell proliferation through androgen receptor independent mechanisms. Front Oncol (2021) 11:789885. doi: 10.3389/fonc.2021.789885
22. Reyes-Moreno C, Sourla A, Choki I, Doillon C, Koutsilieris M. Osteoblast-derived survival factors protect PC-3 human prostate cancer cells from adriamycin apoptosis. Urology (1998) 52:341–7. doi: 10.1016/S0090-4295(98)00182-4
23. Yu-Lee LY, Yu G, Lee YC, Lin SC, Pan J, Pan T, et al. Osteoblast-secreted factors mediate dormancy of metastatic prostate cancer in the bone via activation of the TGFbetaRIII-p38MAPK-pS249/T252RB pathway. Cancer Res (2018) 78:2911–24. doi: 10.1158/0008-5472.CAN-17-1051
24. Hass R. Role of MSC in the tumor microenvironment. Cancers (Basel) (2020) 12(8):2107. doi: 10.3390/cancers12082107
25. Ridge SM, Sullivan FJ, Glynn SA. Mesenchymal stem cells: Key players in cancer progression. Mol Cancer (2017) 16:31. doi: 10.1186/s12943-017-0597-8
26. Riquelme MA, Cardenas ER, Jiang JX. Osteocytes and bone metastasis. Front Endocrinol (Lausanne) (2020) 11:567844. doi: 10.3389/fendo.2020.567844
27. Brylka LJ, Schinke T. Chemokines in physiological and pathological bone remodeling. Front Immunol (2019) 10:2182. doi: 10.3389/fimmu.2019.02182
28. Ridge SM, Bhattacharyya D, Dervan E, Naicker SD, Burke AJ, Murphy JM, et al. Secreted factors from metastatic prostate cancer cells stimulate mesenchymal stem cell transition to a pro-tumourigenic 'activated' state that enhances prostate cancer cell migration. Int J Cancer (2018) 142:2056–67. doi: 10.1002/ijc.31226
29. Siddiqui JA, Seshacharyulu P, Muniyan S, Pothuraju R, Khan P, Vengoji R, et al. GDF15 promotes prostate cancer bone metastasis and colonization through osteoblastic CCL2 and RANKL activation. Bone Res (2022) 10:6. doi: 10.1038/s41413-021-00178-6
30. Hughes CE, Nibbs RJB. A guide to chemokines and their receptors. FEBS J (2018) 285:2944–71. doi: 10.1111/febs.14466
31. Miller MC, Mayo KH. Chemokines from a structural perspective. Int J Mol Sci (2017) 18(10):2088. doi: 10.3390/ijms18102088
32. Gilchrist A. Chemokines and bone. Handb Exp Pharmacol (2020) 262:231–58. doi: 10.1007/164_2020_349
33. Dimberg A. Chemokines in angiogenesis. Curr Top Microbiol Immunol (2010) 341:59–80. doi: 10.1007/82_2010_21
34. Nagarsheth N, Wicha MS, Zou W. Chemokines in the cancer microenvironment and their relevance in cancer immunotherapy. Nat Rev Immunol (2017) 17:559–72. doi: 10.1038/nri.2017.49
35. Mollica Poeta V, Massara M, Capucetti A, Bonecchi R. Chemokines and chemokine receptors: New targets for cancer immunotherapy. Front Immunol (2019) 10:379. doi: 10.3389/fimmu.2019.00379
36. Adekoya TO, Richardson RM. Cytokines and chemokines as mediators of prostate cancer metastasis. Int J Mol Sci (2020) 21(12):4449. doi: 10.3390/ijms21124449
37. Griffith JW, Sokol CL, Luster AD. Chemokines and chemokine receptors: Positioning cells for host defense and immunity. Annu Rev Immunol (2014) 32:659–702. doi: 10.1146/annurev-immunol-032713-120145
38. Baggiolini M, Clark-Lewis I. Interleukin-8, a chemotactic and inflammatory cytokine. FEBS Lett (1992) 307:97–101. doi: 10.1016/0014-5793(92)80909-Z
39. Russo RC, Garcia CC, Teixeira MM, Amaral FA. The CXCL8/IL-8 chemokine family and its receptors in inflammatory diseases. Expert Rev Clin Immunol (2014) 10:593–619. doi: 10.1586/1744666X.2014.894886
40. Pathak JL, Bakker AD, Verschueren P, Lems WF, Luyten FP, Klein-Nulend J, et al. CXCL8 and CCL20 enhance osteoclastogenesis via modulation of cytokine production by human primary osteoblasts. PloS One (2015) 10:e0131041. doi: 10.1371/journal.pone.0131041
41. Bendre M, Gaddy D, Nicholas RW, Suva LJ. Breast cancer metastasis to bone: It is not all about PTHrP. Clin Orthop Relat Res (2003) 415:S39–45. doi: 10.1097/01.blo.0000093844.72468.f4
42. Gwak J, Jeong H, Lee K, Shin JY, Sim T, Na J, et al. SFMBT2-mediated infiltration of preadipocytes and TAMs in prostate cancer. Cancers (Basel) (2020) 12(9):2718. doi: 10.3390/cancers12092718
43. Xie K. Interleukin-8 and human cancer biology. Cytokine Growth Factor Rev (2001) 12:375–91. doi: 10.1016/S1359-6101(01)00016-8
44. Lehrer S, Diamond EJ, Mamkine B, Stone NN, Stock RG. Serum interleukin-8 is elevated in men with prostate cancer and bone metastases. Technol Cancer Res Treat (2004) 3:411. doi: 10.1177/153303460400300501
45. Chi N, Tan Z, Ma K, Bao L, Yun Z. Increased circulating myeloid-derived suppressor cells correlate with cancer stages, interleukin-8 and -6 in prostate cancer. Int J Clin Exp Med (2014) 7:3181–92.
46. Moore BB, Arenberg DA, Stoy K, Morgan T, Addison CL, Morris SB, et al. Distinct CXC chemokines mediate tumorigenicity of prostate cancer cells. Am J Pathol (1999) 154:1503–12. doi: 10.1016/S0002-9440(10)65404-1
47. Aalinkeel R, Nair B, Chen CK, Mahajan SD, Reynolds JL, Zhang H, et al. Nanotherapy silencing the interleukin-8 gene produces regression of prostate cancer by inhibition of angiogenesis. Immunology (2016) 148:387–406. doi: 10.1111/imm.12618
48. Alassaf E, Mueller A. The role of PKC in CXCL8 and CXCL10 directed prostate, breast and leukemic cancer cell migration. Eur J Pharmacol (2020) 886:173453. doi: 10.1016/j.ejphar.2020.173453
49. Humbert P, Brennan MA, De Lima J, Brion R, Adrait A, Charrier C, et al. Apoptotic mesenchymal stromal cells support osteoclastogenesis while inhibiting multinucleated giant cells formation in vitro. Sci Rep (2021) 11:12144. doi: 10.1038/s41598-021-91258-4
50. Lu Y, Cai Z, Xiao G, Keller ET, Mizokami A, Yao Z, et al. Monocyte chemotactic protein-1 mediates prostate cancer-induced bone resorption. Cancer Res (2007) 67:3646–53. doi: 10.1158/0008-5472.CAN-06-1210
51. Lee YC, Cheng CJ, Bilen MA, Lu JF, Satcher RL, Yu-Lee LY, et al. BMP4 promotes prostate tumor growth in bone through osteogenesis. Cancer Res (2011) 71:5194–203. doi: 10.1158/0008-5472.CAN-10-4374
52. Liu B, Xu M, Guo Z, Liu J, Chu X, Jiang H. Interleukin-8 promotes prostate cancer bone metastasis through upregulation of bone sialoprotein. Oncol Lett (2019) 17:4607–13. doi: 10.3892/ol.2019.10138
53. Sugiyama T, Kohara H, Noda M, Nagasawa T. Maintenance of the hematopoietic stem cell pool by CXCL12-CXCR4 chemokine signaling in bone marrow stromal cell niches. Immunity (2006) 25:977–88. doi: 10.1016/j.immuni.2006.10.016
54. Bule P, Aguiar SI, Aires-Da-Silva F, Dias JNR. Chemokine-directed tumor microenvironment modulation in cancer immunotherapy. Int J Mol Sci (2021) 22. doi: 10.3390/ijms22189804
55. Grassi F, Cristino S, Toneguzzi S, Piacentini A, Facchini A, Lisignoli G. CXCL12 chemokine up-regulates bone resorption and MMP-9 release by human osteoclasts: CXCL12 levels are increased in synovial and bone tissue of rheumatoid arthritis patients. J Cell Physiol (2004) 199:244–51. doi: 10.1002/jcp.10445
56. Zannettino AC, Farrugia AN, Kortesidis A, Manavis J, To LB, Martin SK, et al. Elevated serum levels of stromal-derived factor-1alpha are associated with increased osteoclast activity and osteolytic bone disease in multiple myeloma patients. Cancer Res (2005) 65:1700–9. doi: 10.1158/0008-5472.CAN-04-1687
57. Ponte F, Kim HN, Iyer S, Han L, Almeida M, Manolagas SC. Cxcl12 deletion in mesenchymal cells increases bone turnover and attenuates the loss of cortical bone caused by estrogen deficiency in mice. J Bone Miner Res (2020) 35:1441–51. doi: 10.1002/jbmr.4002
58. Zhu W, Liang G, Huang Z, Doty SB, Boskey AL. Conditional inactivation of the CXCR4 receptor in osteoprecursors reduces postnatal bone formation due to impaired osteoblast development. J Biol Chem (2011) 286:26794–805. doi: 10.1074/jbc.M111.250985
59. Guang LG, Boskey AL, Zhu W. Age-related CXC chemokine receptor-4-deficiency impairs osteogenic differentiation potency of mouse bone marrow mesenchymal stromal stem cells. Int J Biochem Cell Biol (2013) 45:1813–20. doi: 10.1016/j.biocel.2013.05.034
60. Shahnazari M, Chu V, Wronski TJ, Nissenson RA, Halloran BP. CXCL12/CXCR4 signaling in the osteoblast regulates the mesenchymal stem cell and osteoclast lineage populations. FASEB J (2013) 27:3505–13. doi: 10.1096/fj.12-225763
61. Tzeng YS, Chung NC, Chen YR, Huang HY, Chuang WP, Lai DM. Imbalanced osteogenesis and adipogenesis in mice deficient in the chemokine Cxcl12/Sdf1 in the bone mesenchymal Stem/Progenitor cells. J Bone Miner Res (2018) 33:679–90. doi: 10.1002/jbmr.3340
62. Chinni SR, Sivalogan S, Dong Z, Filho JC, Deng X, Bonfil RD, et al. CXCL12/CXCR4 signaling activates akt-1 and MMP-9 expression in prostate cancer cells: The role of bone microenvironment-associated CXCL12. Prostate (2006) 66:32–48. doi: 10.1002/pros.20318
63. Singh S, Singh UP, Grizzle WE, Lillard JW Jr. CXCL12-CXCR4 interactions modulate prostate cancer cell migration, metalloproteinase expression and invasion. Lab Invest (2004) 84:1666–76. doi: 10.1038/labinvest.3700181
64. Pulukuri SM, Rao JS. Matrix metalloproteinase-1 promotes prostate tumor growth and metastasis. Int J Oncol (2008) 32:757–65.
65. Fuhrman-Luck RA, Stansfield SH, Stephens CR, Loessner D, Clements JA. Prostate cancer-associated kallikrein-related peptidase 4 activates matrix metalloproteinase-1 and thrombospondin-1. J Proteome Res (2016) 15:2466–78. doi: 10.1021/acs.jproteome.5b01148
66. Chakravarthi BV, Pathi SS, Goswami MT, Cieslik M, Zheng H, Nallasivam S, et al. The miR-124-prolyl hydroxylase P4HA1-MMP1 axis plays a critical role in prostate cancer progression. Oncotarget (2014) 5:6654–69. doi: 10.18632/oncotarget.2208
67. Frieling JS, Li T, Tauro M, Lynch CC. Prostate cancer-derived MMP-3 controls intrinsic cell growth and extrinsic angiogenesis. Neoplasia (2020) 22:511–21. doi: 10.1016/j.neo.2020.08.004
68. Phromnoi K, Yodkeeree S, Anuchapreeda S, Limtrakul P. Inhibition of MMP-3 activity and invasion of the MDA-MB-231 human invasive breast carcinoma cell line by bioflavonoids. Acta Pharmacol Sin (2009) 30:1169–76. doi: 10.1038/aps.2009.107
69. Chen CL, Zhang L, Jiao YR, Zhou Y, Ge QF, Li PC, et al. miR-134 inhibits osteosarcoma cell invasion and metastasis through targeting MMP1 and MMP3 in vitro and in vivo. FEBS Lett (2019) 593:1089–101. doi: 10.1002/1873-3468.13387
70. Xu D, McKee CM, Cao Y, Ding Y, Kessler BM, Muschel RJ. Matrix metalloproteinase-9 regulates tumor cell invasion through cleavage of protease nexin-1. Cancer Res (2010) 70:6988–98. doi: 10.1158/0008-5472.CAN-10-0242
71. London CA, Sekhon HS, Arora V, Stein DA, Iversen PL, Devi GR. A novel antisense inhibitor of MMP-9 attenuates angiogenesis, human prostate cancer cell invasion and tumorigenicity. Cancer Gene Ther (2003) 10:823–32. doi: 10.1038/sj.cgt.7700642
72. Nalla AK, Gorantla B, Gondi CS, Lakka SS, Rao JS, MMP-9 T. uPAR, and cathepsin b inhibits invasion, migration and activates apoptosis in prostate cancer cells. Cancer Gene Ther (2010) 17:599–613. doi: 10.1038/cgt.2010.16
73. Zarrabi K, Dufour A, Li J, Kuscu C, Pulkoski-Gross A, Zhi J, et al. Inhibition of matrix metalloproteinase 14 (MMP-14)-mediated cancer cell migration. J Biol Chem (2011) 286:33167–77. doi: 10.1074/jbc.M111.256644
74. Yan T, Lin Z, Jiang J, Lu S, Chen M, Que H, et al. MMP14 regulates cell migration and invasion through epithelial-mesenchymal transition in nasopharyngeal carcinoma. Am J Transl Res (2015) 7:950–8.
75. Akashi T, Koizumi K, Tsuneyama K, Saiki I, Takano Y, Fuse H. Chemokine receptor CXCR4 expression and prognosis in patients with metastatic prostate cancer. Cancer Sci (2008) 99:539–42. doi: 10.1111/j.1349-7006.2007.00712.x
76. Gravina GL, Mancini A, Muzi P, Ventura L, Biordi L, Ricevuto E, et al. CXCR4 pharmacogical inhibition reduces bone and soft tissue metastatic burden by affecting tumor growth and tumorigenic potential in prostate cancer preclinical models. Prostate (2015) 75:1227–46. doi: 10.1002/pros.23007
77. Taichman RS, Cooper C, Keller ET, Pienta KJ, Taichman NS, McCauley LK. Use of the stromal cell-derived factor-1/CXCR4 pathway in prostate cancer metastasis to bone. Cancer Res (2002) 62:1832–7.
78. Wang N, Docherty FE, Brown HK, Reeves KJ, Fowles AC, Ottewell PD, et al. Prostate cancer cells preferentially home to osteoblast-rich areas in the early stages of bone metastasis: Evidence from in vivo models. J Bone Miner Res (2014) 29:2688–96. doi: 10.1002/jbmr.2300
79. Chinni SR, Yamamoto H, Dong Z, Sabbota A, Bonfil RD, Cher ML. CXCL12/CXCR4 transactivates HER2 in lipid rafts of prostate cancer cells and promotes growth of metastatic deposits in bone. Mol Cancer Res (2008) 6:446–57. doi: 10.1158/1541-7786.MCR-07-0117
80. Sun YX, Schneider A, Jung Y, Wang J, Dai J, Wang J, et al. Skeletal localization and neutralization of the SDF-1(CXCL12)/CXCR4 axis blocks prostate cancer metastasis and growth in osseous sites in vivo. J Bone Miner Res (2005) 20:318–29. doi: 10.1359/JBMR.041109
81. Mognetti B, La Montagna G, Perrelli MG, Pagliaro P, Penna C. Bone marrow mesenchymal stem cells increase motility of prostate cancer cells via production of stromal cell-derived factor-1alpha. J Cell Mol Med (2013) 17:287–92. doi: 10.1111/jcmm.12010
82. Uygur B, Wu WS. SLUG promotes prostate cancer cell migration and invasion via CXCR4/CXCL12 axis. Mol Cancer (2011) 10:139. doi: 10.1186/1476-4598-10-139
83. Dai J, Escara-Wilke J, Keller JM, Jung Y, Taichman RS, Pienta KJ, et al. Primary prostate cancer educates bone stroma through exosomal pyruvate kinase M2 to promote bone metastasis. J Exp Med (2019) 216:2883–99. doi: 10.1084/jem.20190158
84. Charo IF, Ransohoff RM. The many roles of chemokines and chemokine receptors in inflammation. N Engl J Med (2006) 354:610–21. doi: 10.1056/NEJMra052723
85. Singh S, Anshita D, Ravichandiran V. MCP-1: Function, regulation, and involvement in disease. Int Immunopharmacol (2021) 101:107598. doi: 10.1016/j.intimp.2021.107598
86. Deshmane SL, Kremlev S, Amini S, Sawaya BE. Monocyte chemoattractant protein-1 (MCP-1): an overview. J Interferon Cytokine Res (2009) 29:313–26. doi: 10.1089/jir.2008.0027
87. Jimenez F, Quinones MP, Martinez HG, Estrada CA, Clark K, Garavito E, et al. CCR2 plays a critical role in dendritic cell maturation: Possible role of CCL2 and NF-kappa b. J Immunol (2010) 184:5571–81. doi: 10.4049/jimmunol.0803494
88. Zhang HH, Song K, Rabin RL, Hill BJ, Perfetto SP, Roederer M, et al. CCR2 identifies a stable population of human effector memory CD4+ t cells equipped for rapid recall response. J Immunol (2010) 185:6646–63. doi: 10.4049/jimmunol.0904156
89. van Helden MJ, Zaiss DM, Sijts AJ. CCR2 defines a distinct population of NK cells and mediates their migration during influenza virus infection in mice. PloS One (2012) 7:e52027. doi: 10.1371/journal.pone.0052027
90. Iikura M, Miyamasu M, Yamaguchi M, Kawasaki H, Matsushima K, Kitaura M, et al. Chemokine receptors in human basophils: Inducible expression of functional CXCR4. J Leukoc Biol (2001) 70:113–20. doi: 10.1189/jlb.70.1.113
91. Yoshimura T, Robinson EA, Tanaka S, Appella E, Leonard EJ. Purification and amino acid analysis of two human monocyte chemoattractants produced by phytohemagglutinin-stimulated human blood mononuclear leukocytes. J Immunol (1989) 142:1956–62. doi: 10.4049/jimmunol.142.6.1956
92. Standiford TJ, Kunkel SL, Phan SH, Rollins BJ, Strieter RM. Alveolar macrophage-derived cytokines induce monocyte chemoattractant protein-1 expression from human pulmonary type II-like epithelial cells. J Biol Chem (1991) 266:9912–8. doi: 10.1016/S0021-9258(18)92905-4
93. Cushing SD, Berliner JA, Valente AJ, Territo MC, Navab M, Parhami F, et al. Minimally modified low density lipoprotein induces monocyte chemotactic protein 1 in human endothelial cells and smooth muscle cells. Proc Natl Acad Sci U.S.A. (1990) 87:5134–8. doi: 10.1073/pnas.87.13.5134
94. Deng X, Mercer PF, Scotton CJ, Gilchrist A, Chambers RC. Thrombin induces fibroblast CCL2/JE production and release via coupling of PAR1 to galphaq and cooperation between ERK1/2 and rho kinase signaling pathways. Mol Biol Cell (2008) 19:2520–33. doi: 10.1091/mbc.e07-07-0720
95. Yao Z, Chen P, Fan L, Chen P, Zhang X, Yu B. CCL2 is a critical mechano-responsive mediator in crosstalk between osteoblasts and bone mesenchymal stromal cells. FASEB J (2021) 35:e21851. doi: 10.1096/fj.202002808RR
96. Li X, Qin L, Bergenstock M, Bevelock LM, Novack DV, Partridge C. Parathyroid hormone stimulates osteoblastic expression of MCP-1 to recruit and increase the fusion of pre/osteoclasts. J Biol Chem (2007) 282:33098–106. doi: 10.1074/jbc.M611781200
97. Binder NB, Niederreiter B, Hoffmann O, Stange R, Pap T, Stulnig TM, et al. Estrogen-dependent and c-c chemokine receptor-2-dependent pathways determine osteoclast behavior in osteoporosis. Nat Med (2009) 15:417–24. doi: 10.1038/nm.1945
98. Sul OJ, Ke K, Kim WK, Kim SH, Lee SC, Kim HJ, et al. Absence of MCP-1 leads to elevated bone mass via impaired actin ring formation. J Cell Physiol (2012) 227:1619–27. doi: 10.1002/jcp.22879
99. Wintges K, Beil FT, Albers J, Jeschke A, Schweizer M, Claass B, et al. Impaired bone formation and increased osteoclastogenesis in mice lacking chemokine (C-c motif) ligand 5 (Ccl5). J Bone Miner Res (2013) 28:2070–80. doi: 10.1002/jbmr.1937
100. Windrichova J, Fuchsova R, Kucera R, Topolcan O, Fiala O, Finek J, et al. MIC1/GDF15 as a bone metastatic disease biomarker. Anticancer Res (2017) 37:1501–5. doi: 10.21873/anticanres.11477
101. Lu Y, Xiao G, Galson DL, Nishio Y, Mizokami A, Keller ET, et al. PTHrP-induced MCP-1 production by human bone marrow endothelial cells and osteoblasts promotes osteoclast differentiation and prostate cancer cell proliferation and invasion in vitro. Int J Cancer (2007) 121:724–33. doi: 10.1002/ijc.22704
102. Mizutani K, Sud S, McGregor NA, Martinovski G, Rice BT, Craig MJ, et al. The chemokine CCL2 increases prostate tumor growth and bone metastasis through macrophage and osteoclast recruitment. Neoplasia (2009) 11:1235–42. doi: 10.1593/neo.09988
103. Li X, Loberg R, Liao J, Ying C, Snyder LA, Pienta KJ, et al. A destructive cascade mediated by CCL2 facilitates prostate cancer growth in bone. Cancer Res (2009) 69:1685–92. doi: 10.1158/0008-5472.CAN-08-2164
104. Noy R, Pollard JW. Tumor-associated macrophages: From mechanisms to therapy. Immunity (2014) 41:49–61. doi: 10.1016/j.immuni.2014.06.010
105. Chen Y, Song Y, Du W, Gong L, Chang H, Zou Z. Tumor-associated macrophages: An accomplice in solid tumor progression. J BioMed Sci (2019) 26:78. doi: 10.1186/s12929-019-0568-z
106. Zhou J, Tang Z, Gao S, Li C, Feng Y, Zhou X. Tumor-associated macrophages: Recent insights and therapies. Front Oncol (2020) 10:188. doi: 10.3389/fonc.2020.00188
107. Guo Z, Xing Z, Cheng X, Fang Z, Jiang C, Su J, et al. Somatostatin derivate (smsDX) attenuates the TAM-stimulated proliferation, migration and invasion of prostate cancer via NF-kappaB regulation. PloS One (2015) 10:e0124292. doi: 10.1371/journal.pone.0124292
108. Guan H, Peng R, Fang F, Mao L, Chen Z, Yang S, et al. Tumor-associated macrophages promote prostate cancer progression via exosome-mediated miR-95 transfer. J Cell Physiol (2020) 235:9729–42. doi: 10.1002/jcp.29784
109. Shan K, Feng N, Cui J, Wang S, Qu H, Fu G, et al. Resolvin D1 and D2 inhibit tumour growth and inflammation via modulating macrophage polarization. J Cell Mol Med (2020) 24:8045–56. doi: 10.1111/jcmm.15436
110. Huang R, Wang S, Wang N, Zheng Y, Zhou J, Yang B, et al. CCL5 derived from tumor-associated macrophages promotes prostate cancer stem cells and metastasis via activating beta-catenin/STAT3 signaling. Cell Death Dis (2020) 11:234. doi: 10.1038/s41419-020-2435-y
111. Han C, Deng Y, Xu W, Liu Z, Wang T, Wang S, et al. The roles of tumor-associated macrophages in prostate cancer. J Oncol (2022) 2022:8580043. doi: 10.1155/2022/8580043
112. Izumi K, Fang LY, Mizokami A, Namiki M, Li L, Lin WJ, et al. Targeting the androgen receptor with siRNA promotes prostate cancer metastasis through enhanced macrophage recruitment via CCL2/CCR2-induced STAT3 activation. EMBO Mol Med (2013) 5:1383–401. doi: 10.1002/emmm.201202367
113. Schall TJ, Jongstra J, Dyer BJ, Jorgensen J, Clayberger C, Davis MM, et al. A human t cell-specific molecule is a member of a new gene family. J Immunol (1988) 141:1018–25. doi: 10.4049/jimmunol.141.3.1018
114. Schall TJ, Bacon K, Toy KJ, Goeddel DV. Selective attraction of monocytes and t lymphocytes of the memory phenotype by cytokine RANTES. Nature (1990) 347:669–71. doi: 10.1038/347669a0
115. Ma K, Xu W, Shao X, Yanyue L, Xu H, et al. Coimmunization with RANTES plasmid polarized Th1 immune response against hepatitis b virus envelope via recruitment of dendritic cells. Antiviral Res (2007) 76:140–9. doi: 10.1016/j.antiviral.2007.06.012
116. Zhang H, Yang H, Ma W, He S. Induction of IL-13 production and upregulated expression of protease activated receptor-1 by RANTES in a mast cell line. Cytokine (2011) 53:231–8. doi: 10.1016/j.cyto.2010.10.005
117. Alam R, Stafford S, Forsythe P, Harrison R, Faubion D, Lett-Brown MA, et al. RANTES is a chemotactic and activating factor for human eosinophils. J Immunol (1993) 150:3442–8. doi: 10.4049/jimmunol.150.8.3442
118. Kuna P, Alam R, Ruta U, Gorski P. RANTES induces nasal mucosal inflammation rich in eosinophils, basophils, and lymphocytes in vivo. Am J Respir Crit Care Med (1998) 157:873–9. doi: 10.1164/ajrccm.157.3.9610052
119. Maghazachi AA, al-Aoukaty A, Schall TJ. C-c chemokines induce the chemotaxis of NK and IL-2-activated NK cells. role for g proteins. J Immunol (1994) 153:4969–77. doi: 10.4049/jimmunol.153.11.4969
120. Zeng Z, Lan T, Wei Y, Wei X. CCL5/CCR5 axis in human diseases and related treatments. Genes Dis (2022) 9:12–27. doi: 10.1016/j.gendis.2021.08.004
121. Blanpain C, Buser R, Power CA, Edgerton M, Buchanan C, Mack M, et al. A chimeric MIP-1alpha/RANTES protein demonstrates the use of different regions of the RANTES protein to bind and activate its receptors. J Leukoc Biol (2001) 69:977–85. doi: 10.1189/jlb.69.6.977
122. Yano S, Mentaverri R, Kanuparthi D, Bandyopadhyay S, Rivera A, Brown EM, et al. Functional expression of beta-chemokine receptors in osteoblasts: Role of regulated upon activation, normal t cell expressed and secreted (RANTES) in osteoblasts and regulation of its secretion by osteoblasts and osteoclasts. Endocrinology (2005) 146:2324–35. doi: 10.1210/en.2005-0065
123. Sottnik JL, Dai J, Zhang H, Campbell B, Keller ET. Tumor-induced pressure in the bone microenvironment causes osteocytes to promote the growth of prostate cancer bone metastases. Cancer Res (2015) 75:2151–8. doi: 10.1158/0008-5472.CAN-14-2493
124. Huang R, Guo L, Gao M, Li J, Xiang S. Research trends and regulation of CCL5 in prostate cancer. Onco Targets Ther (2021) 14:1417–27. doi: 10.2147/OTT.S279189
125. Sicoli D, Jiao X, Ju X, Velasco-Velazquez M, Ertel A, Addya S, et al. CCR5 receptor antagonists block metastasis to bone of v-src oncogene-transformed metastatic prostate cancer cell lines. Cancer Res (2014) 74:7103–14. doi: 10.1158/0008-5472.CAN-14-0612
126. Konig JE, Senge T, Allhoff EP, Konig W. Analysis of the inflammatory network in benign prostate hyperplasia and prostate cancer. Prostate (2004) 58:121–9. doi: 10.1002/pros.10317
127. Legler DF, Loetscher M, Roos RS, Clark-Lewis I, Baggiolini M, Moser B. B cell-attracting chemokine 1, a human CXC chemokine expressed in lymphoid tissues, selectively attracts b lymphocytes via BLR1/CXCR5. J Exp Med (1998) 187:655–60. doi: 10.1084/jem.187.4.655
128. Singh S, Singh R, Sharma PK, Singh UP, Rai SN, Chung LW, et al. Serum CXCL13 positively correlates with prostatic disease, prostate-specific antigen and mediates prostate cancer cell invasion, integrin clustering and cell adhesion. Cancer Lett (2009) 283:29–35. doi: 10.1016/j.canlet.2009.03.022
129. Tian F, Ji XL, Xiao WA, Wang B, Wang F. CXCL13 promotes osteogenic differentiation of mesenchymal stem cells by inhibiting miR-23a expression. Stem Cells Int (2015) 2015:632305. doi: 10.1155/2015/632305
130. El-Haibi CP, Singh R, Sharma PK, Singh S, Lillard JW Jr. CXCL13 mediates prostate cancer cell proliferation through JNK signalling and invasion through ERK activation. Cell Prolif (2011) 44:311–9. doi: 10.1111/j.1365-2184.2011.00757.x
131. Fan L, Zhu Q, Liu L, Zhu C, Huang H, Lu S, et al. CXCL13 is androgen-responsive and involved in androgen induced prostate cancer cell migration and invasion. Oncotarget (2017) 8:53244–61. doi: 10.18632/oncotarget.18387
132. Tumminello FM, Badalamenti G, Incorvaia L, Fulfaro F, D'Amico C, Leto G. Serum interleukin-6 in patients with metastatic bone disease: Correlation with cystatin c. Med Oncol (2009) 26:10–5. doi: 10.1007/s12032-008-9070-2
133. Shariat SF, Andrews B, Kattan MW, Kim J, Wheeler TM, Slawin KM. Plasma levels of interleukin-6 and its soluble receptor are associated with prostate cancer progression and metastasis. Urology (2001) 58:1008–15. doi: 10.1016/S0090-4295(01)01405-4
134. Kim CH, Kunkel EJ, Boisvert J, Johnston B, Campbell JJ, Genovese MC, et al. Bonzo/CXCR6 expression defines type 1-polarized t-cell subsets with extralymphoid tissue homing potential. J Clin Invest (2001) 107:595–601. doi: 10.1172/JCI11902
135. Abel S, Hundhausen C, Mentlein R, Schulte A, Berkhout TA, Broadway N, et al. The transmembrane CXC-chemokine ligand 16 is induced by IFN-gamma and TNF-alpha and shed by the activity of the disintegrin-like metalloproteinase ADAM10. J Immunol (2004) 172:6362–72. doi: 10.4049/jimmunol.172.10.6362
136. Sato T, Thorlacius H, Johnston B, Staton TL, Xiang W, Littman DR, et al. Role for CXCR6 in recruitment of activated CD8+ lymphocytes to inflamed liver. J Immunol (2005) 174:277–83. doi: 10.4049/jimmunol.174.1.277
137. Yamauchi R, Tanaka M, Kume N, Minami M, Kawamoto T, Togi K, et al. Upregulation of SR-PSOX/CXCL16 and recruitment of CD8+ t cells in cardiac valves during inflammatory valvular heart disease. Arterioscler Thromb Vasc Biol (2004) 24:282–7. doi: 10.1161/01.ATV.0000114565.42679.c6
138. Paust S, Gill HS, Wang BZ, Flynn MP, Moseman EA, Senman B, et al. Critical role for the chemokine receptor CXCR6 in NK cell-mediated antigen-specific memory of haptens and viruses. Nat Immunol (2010) 11:1127–35. doi: 10.1038/ni.1953
139. Kim CH, Johnston B, Butcher EC. Trafficking machinery of NKT cells: Shared and differential chemokine receptor expression among v alpha 24(+)V beta 11(+) NKT cell subsets with distinct cytokine-producing capacity. Blood (2002) 100:11–6. doi: 10.1182/blood-2001-12-0196
140. Nakayama T, Hieshima K, Izawa D, Tatsumi Y, Kanamaru A, Yoshie O. Cutting edge: Profile of chemokine receptor expression on human plasma cells accounts for their efficient recruitment to target tissues. J Immunol (2003) 170:1136–40. doi: 10.4049/jimmunol.170.3.1136
141. Gough PJ, Garton KJ, Wille PT, Rychlewski M, Dempsey PJ, Raines EW. A disintegrin and metalloproteinase 10-mediated cleavage and shedding regulates the cell surface expression of CXC chemokine ligand 16. J Immunol (2004) 172:3678–85. doi: 10.4049/jimmunol.172.6.3678
142. Matloubian M, David A, Engel S, Ryan JE, Cyster JG. A transmembrane CXC chemokine is a ligand for HIV-coreceptor bonzo. Nat Immunol (2000) 1:298–304. doi: 10.1038/79738
143. Hojo S, Koizumi K, Tsuneyama K, Arita Y, Cui Z, Shinohara K, et al. High-level expression of chemokine CXCL16 by tumor cells correlates with a good prognosis and increased tumor-infiltrating lymphocytes in colorectal cancer. Cancer Res (2007) 67:4725–31. doi: 10.1158/0008-5472.CAN-06-3424
144. Gutwein P, Schramme A, Sinke N, Abdel-Bakky MS, Voss B, Obermuller N, et al. Tumoural CXCL16 expression is a novel prognostic marker of longer survival times in renal cell cancer patients. Eur J Cancer (2009) 45:478–89. doi: 10.1016/j.ejca.2008.10.023
145. Morris MR, Gentle D, Abdulrahman M, Clarke N, Brown M, Kishida T, et al. Functional epigenomics approach to identify methylated candidate tumour suppressor genes in renal cell carcinoma. Br J Cancer (2008) 98:496–501. doi: 10.1038/sj.bjc.6604180
146. Ha HK, Lee W, Park HJ, Lee SD, Lee JZ, Chung MK. Clinical significance of CXCL16/CXCR6 expression in patients with prostate cancer. Mol Med Rep (2011) 4:419–24. doi: 10.3892/mmr.2011.446
147. Wang J, Lu Y, Wang J, Koch AE, Zhang J, Taichman RS. CXCR6 induces prostate cancer progression by the AKT/mammalian target of rapamycin signaling pathway. Cancer Res (2008) 68:10367–76. doi: 10.1158/0008-5472.CAN-08-2780
148. Lu Y, Wang J, Xu Y, Koch AE, Cai Z, Chen X, et al. CXCL16 functions as a novel chemotactic factor for prostate cancer cells in vitro. Mol Cancer Res (2008) 6:546–54. doi: 10.1158/1541-7786.MCR-07-0277
149. Singh R, Kapur N, Mir H, Singh N, Lillard JW Jr, Singh S. CXCR6-CXCL16 axis promotes prostate cancer by mediating cytoskeleton rearrangement via ezrin activation and alphavbeta3 integrin clustering. Oncotarget (2016) 7:7343–53. doi: 10.18632/oncotarget.6944
150. Ikeda T, Nishita M, Hoshi K, Honda T, Kakeji Y, Minami Y. Mesenchymal stem cell-derived CXCL16 promotes progression of gastric cancer cells by STAT3-mediated expression of Ror1. Cancer Sci (2020) 111:1254–65. doi: 10.1111/cas.14339
151. Hu W, Zhen X, Xiong B, Wang B, Zhang W, Zhou W. CXCR6 is expressed in human prostate cancer in vivo and is involved in the in vitro invasion of PC3 and LNCap cells. Cancer Sci (2008) 99:1362–9. doi: 10.1111/j.1349-7006.2008.00833.x
152. Shimaoka T, Kume N, Minami M, Hayashida K, Kataoka H, Kita T, et al. Molecular cloning of a novel scavenger receptor for oxidized low density lipoprotein, SR-PSOX, on macrophages. J Biol Chem (2000) 275:40663–6. doi: 10.1074/jbc.C000761200
153. Wilbanks A, Zondlo SC, Murphy K, Mak S, Soler D, Langdon P, et al. Expression cloning of the STRL33/BONZO/TYMSTRligand reveals elements of CC, CXC, and CX3C chemokines. J Immunol (2001) 166:5145–54. doi: 10.4049/jimmunol.166.8.5145
154. Jung Y, Kim JK, Shiozawa Y, Wang J, Mishra A, Joseph J, et al. Recruitment of mesenchymal stem cells into prostate tumours promotes metastasis. Nat Commun (2013) 4:1795. doi: 10.1038/ncomms2766
155. Kapur N, Mir H, Sonpavde GP, Jain S, Bae S, Lillard JW Jr, et al. Prostate cancer cells hyper-activate CXCR6 signaling by cleaving CXCL16 to overcome effect of docetaxel. Cancer Lett (2019) 454:1–13. doi: 10.1016/j.canlet.2019.04.001
156. Bazan JF, Bacon KB, Hardiman G, Wang W, Soo K, Rossi D, et al. A new class of membrane-bound chemokine with a CX3C motif. Nature (1997) 385:640–4. doi: 10.1038/385640a0
157. Koizumi K, et al. Role of CX3CL1/fractalkine in osteoclast differentiation and bone resorption. J Immunol (2009) 183(12):7825–31. doi: 10.4049/jimmunol.0803627
158. Ludwig A, Berkhout T, Moores K, Groot P, Chapman G. Fractalkine is expressed by smooth muscle cells in response to IFN-gamma and TNF-alpha and is modulated by metalloproteinase activity. J Immunol (2002) 168:604–12. doi: 10.4049/jimmunol.168.2.604
159. Fraticelli P, Sironi M, Bianchi G, D'Ambrosio D, Albanesi C, Stoppacciaro A, et al. Fractalkine (CX3CL1) as an amplification circuit of polarized Th1 responses. J Clin Invest (2001) 107:1173–81. doi: 10.1172/JCI11517
160. Jamieson WL, Shimizu S, D'Ambrosio JA, Meucci O, Fatatis A. CX3CR1 is expressed by prostate epithelial cells and androgens regulate the levels of CX3CL1/fractalkine in the bone marrow: potential role in prostate cancer bone tropism. Cancer Res (2008) 68:1715–22. doi: 10.1158/0008-5472.CAN-07-1315
161. Hoshino A, Ueha S, Hanada S, Imai T, Ito M, Yamamoto K, et al. Roles of chemokine receptor CX3CR1 in maintaining murine bone homeostasis through the regulation of both osteoblasts and osteoclasts. J Cell Sci (2013) 126:1032–45. doi: 10.1242/dev.097550
162. Han KH, Ryu JW, Lim KE, Lee SH, Kim Y, Hwang CS, et al. Vascular expression of the chemokine CX3CL1 promotes osteoclast recruitment and exacerbates bone resorption in an irradiated murine model. Bone (2014) 61:91–101. doi: 10.1016/j.bone.2013.12.032
163. Imai T, Yasuda N. Therapeutic intervention of inflammatory/immune diseases by inhibition of the fractalkine (CX3CL1)-CX3CR1 pathway. Inflammation Regener (2016) 36:9. doi: 10.1186/s41232-016-0017-2
164. Matsuura T, et al. Involvement of CX3CL1 in the migration of osteoclast precursors across osteoblast layer stimulated by interleukin-1ss. J Cell Physiol (2017) 232(7):1739–45. doi: 10.1002/jcp.25577
165. Muraoka S, Kaneko K, Motomura K, Nishio J, Nanki T. CX3CL1/fractalkine regulates the differentiation of human peripheral blood monocytes and monocyte-derived dendritic cells into osteoclasts. Cytokine (2021) 146:155652. doi: 10.1016/j.cyto.2021.155652
166. Liu P, Liang Y, Jiang L, Wang H, Wang S, Dong J. CX3CL1/fractalkine enhances prostate cancer spinal metastasis by activating the Src/FAK pathway. Int J Oncol (2018) 53:1544–56. doi: 10.3892/ijo.2018.4487
167. Garton KJ, Gough PJ, Blobel CP, Murphy G, Greaves DR, Dempsey PJ, et al. Tumor necrosis factor-alpha-converting enzyme (ADAM17) mediates the cleavage and shedding of fractalkine (CX3CL1). J Biol Chem (2001) 276:37993–8001. doi: 10.1074/jbc.M106434200
168. Tsou CL, Haskell CA, Charo IF. Tumor necrosis factor-alpha-converting enzyme mediates the inducible cleavage of fractalkine. J Biol Chem (2001) 276:44622–6. doi: 10.1074/jbc.M107327200
169. McCulloch DR, Akl P, Samaratunga H, Herington AC, Odorico DM. Expression of the disintegrin metalloprotease, ADAM-10, in prostate cancer and its regulation by dihydrotestosterone, insulin-like growth factor i, and epidermal growth factor in the prostate cancer cell model LNCaP. Clin Cancer Res (2004) 10:314–23. doi: 10.1158/1078-0432.CCR-0846-3
170. McCulloch DR, Harvey M, Herington AC. The expression of the ADAMs proteases in prostate cancer cell lines and their regulation by dihydrotestosterone. Mol Cell Endocrinol (2000) 167:11–21. doi: 10.1016/S0303-7207(00)00305-1
171. Shulby SA, Dolloff NG, Stearns ME, Meucci O, Fatatis A. CX3CR1-fractalkine expression regulates cellular mechanisms involved in adhesion, migration, and survival of human prostate cancer cells. Cancer Res (2004) 64:4693–8. doi: 10.1158/0008-5472.CAN-03-3437
172. Tang J, Xiao L, Cui R, Li D, Zheng X, Zhu L, et al. CX3CL1 increases invasiveness and metastasis by promoting epithelial-to-mesenchymal transition through the TACE/TGF-alpha/EGFR pathway in hypoxic androgen-independent prostate cancer cells. Oncol Rep (2016) 35:1153–62. doi: 10.3892/or.2015.4470
173. Liu W, Bian C, Liang Y, Jiang L, Qian C, Dong J. CX3CL1: A potential chemokine widely involved in the process spinal metastases. Oncotarget (2017) 8:15213–9. doi: 10.18632/oncotarget.14773
174. Bendre MS, Margulies AG, Walser B, Akel NS, Bhattacharrya S, Skinner RA, et al. Tumor-derived interleukin-8 stimulates osteolysis independent of the receptor activator of nuclear factor-kappaB ligand pathway. Cancer Res (2005) 65:11001–9. doi: 10.1158/0008-5472.CAN-05-2630
175. Bendre MS, Montague DC, Peery T, Akel NS, Gaddy D, Suva LJ. Interleukin-8 stimulation of osteoclastogenesis and bone resorption is a mechanism for the increased osteolysis of metastatic bone disease. Bone (2003) 33:28–37. doi: 10.1016/S8756-3282(03)00086-3
176. Kamalakar A, Bendre MS, Washam CL, Fowler TW, Carver A, Dilley JD, et al. Circulating interleukin-8 levels explain breast cancer osteolysis in mice and humans. Bone (2014) 61:176–85. doi: 10.1016/j.bone.2014.01.015
177. Kinder M, Chislock E, Bussard KM, Shuman L, Mastro AM. Metastatic breast cancer induces an osteoblast inflammatory response. Exp Cell Res (2008) 314:173–83. doi: 10.1016/j.yexcr.2007.09.021
178. Hsu YL, Hung JY, Ko YC, Hung CH, Huang MS, Kuo PL. Phospholipase d signaling pathway is involved in lung cancer-derived IL-8 increased osteoclastogenesis. Carcinogenesis (2010) 31:587–96. doi: 10.1093/carcin/bgq030
179. Cai Z, Chen Q, Chen J, Lu Y, Xiao G, Wu Z, et al. Monocyte chemotactic protein 1 promotes lung cancer-induced bone resorptive lesions in vivo. Neoplasia (2009) 11:228–36. doi: 10.1593/neo.81282
180. Kawano M, Tanaka K, Itonaga I, Iwasaki T, Tsumura H. Interaction between human osteosarcoma and mesenchymal stem cells via an interleukin-8 signaling loop in the tumor microenvironment. Cell Commun Signal (2018) 16:13. doi: 10.1186/s12964-018-0225-2
181. Gross AC, Cam H, Phelps DA, Saraf AJ, Bid HK, Cam M, et al. IL-6 and CXCL8 mediate osteosarcoma-lung interactions critical to metastasis. JCI Insight (2018) 3(16):e99791. doi: 10.1172/jci.insight.99791
182. Du L, Han X-G, Tu B, Wang M-Q, Qiao H, Zhang S-H, et al. CXCR1/Akt signaling activation induced by mesenchymal stem cell-derived IL-8 promotes osteosarcoma cell anoikis resistance and pulmonary metastasis. Cell Death Dis (2018) 9:714. doi: 10.1038/s41419-018-0745-0
183. Allegra A, Pace E, Tartarisco G, V. Innao DISE, Allegra AG, et al. Changes in serum interleukin-8 and sRAGE levels in multiple myeloma patients. Anticancer Res (2020) 40:1443–9. doi: 10.21873/anticanres.14086
184. Kohsari M, Khadem-Ansari MH, Rasmi Y, Sayyadi H. Serum levels of interleukin-8 and soluble interleukin-6 receptor in patients with stage-i multiple myeloma: A case-control study. Asian Pac J Cancer Prev (2020) 21:127–32. doi: 10.31557/APJCP.2020.21.1.127
185. Herrero AB, Garcia-Gomez A, Garayoa M, Corchete LA, Hernandez JM, San Miguel J, et al. Effects of IL-8 up-regulation on cell survival and osteoclastogenesis in multiple myeloma. Am J Pathol (2016) 186:2171–82. doi: 10.1016/j.ajpath.2016.04.003
186. Kuku I, Bayraktar MR, Kaya E, Erkurt MA, Bayraktar N, Cikim K, et al. Serum proinflammatory mediators at different periods of therapy in patients with multiple myeloma. Mediators Inflammation (2005) 2005:171–4. doi: 10.1155/MI.2005.171
187. Wang J, Loberg R, Taichman RS. The pivotal role of CXCL12 (SDF-1)/CXCR4 axis in bone metastasis. Cancer Metastasis Rev (2006) 25:573–87. doi: 10.1007/s10555-006-9019-x
188. Benslimane-Ahmim Z, Pereira J, Lokajczyk A, Dizier B, Galy-Fauroux I, Fischer AM, et al. Osteoprotegerin regulates cancer cell migration through SDF-1/CXCR4 axis and promotes tumour development by increasing neovascularization. Cancer Lett (2017) 395:11–9. doi: 10.1016/j.canlet.2017.02.032
189. Guo F, Wang Y, Liu J, Mok SC, Xue F, Zhang W. CXCL12/CXCR4: A symbiotic bridge linking cancer cells and their stromal neighbors in oncogenic communication networks. Oncogene (2016) 35:816–26. doi: 10.1038/onc.2015.139
190. Masuda T, Endo M, Yamamoto Y, Odagiri H, Kadomatsu T, Nakamura T, et al. ANGPTL2 increases bone metastasis of breast cancer cells through enhancing CXCR4 signaling. Sci Rep (2015) 5:9170. doi: 10.1038/srep09170
191. Kang Y, Siegel PM, Shu W, Drobnjak M, Kakonen SM, Cordon-Cardo C, et al. A multigenic program mediating breast cancer metastasis to bone. Cancer Cell (2003) 3:537–49. doi: 10.1016/S1535-6108(03)00132-6
192. Sanz-Rodriguez F, Hidalgo A, Teixido J. Chemokine stromal cell-derived factor-1alpha modulates VLA-4 integrin-mediated multiple myeloma cell adhesion to CS-1/fibronectin and VCAM-1. Blood (2001) 97:346–51. doi: 10.1182/blood.V97.2.346
193. Roccaro AM, Mishima Y, Sacco A, Moschetta M, Tai YT, Shi J, et al. CXCR4 regulates extra-medullary myeloma through epithelial-Mesenchymal-Transition-like transcriptional activation. Cell Rep (2015) 12:622–35. doi: 10.1016/j.celrep.2015.06.059
194. Waldschmidt JM, Simon A, Wider D, Muller SJ, Follo M, Ihorst G, et al. CXCL12 and CXCR7 are relevant targets to reverse cell adhesion-mediated drug resistance in multiple myeloma. Br J Haematol (2017) 179:36–49. doi: 10.1111/bjh.14807
195. Aggarwal R, Ghobrial IM, Roodman GD. Chemokines in multiple myeloma. Exp Hematol (2006) 34:1289–95. doi: 10.1016/j.exphem.2006.06.017
196. Manier S, Sacco A, Leleu X, Ghobrial IM, Roccaro AM. Bone marrow microenvironment in multiple myeloma progression. J BioMed Biotechnol (2012) 2012:157496. doi: 10.1155/2012/157496
197. Liu SH, Gu Y, Pascual B, Yan Z, Hallin M, Zhang C, et al. A novel CXCR4 antagonist IgG1 antibody (PF-06747143) for the treatment of hematologic malignancies. Blood Adv (2017) 1:1088–100. doi: 10.1182/bloodadvances.2016003921
198. Li B, Wang Z, Wu H, Xue M, Lin P, Wang S, et al. Epigenetic regulation of CXCL12 plays a critical role in mediating tumor progression and the immune response in osteosarcoma. Cancer Res (2018) 78:3938–53. doi: 10.1158/0008-5472.CAN-17-3801
199. Perissinotto E, Cavalloni G, Leone F, Fonsato V, Mitola S, Grignani G, et al. Involvement of chemokine receptor 4/stromal cell-derived factor 1 system during osteosarcoma tumor progression. Clin Cancer Res (2005) 11:490–7. doi: 10.1158/1078-0432.490.11.2
200. Sheng G, Gao Y, Yang Y, Wu H. Osteosarcoma and metastasis. Front Oncol (2021) 11:780264. doi: 10.3389/fonc.2021.780264
201. Ren Z, Liang S, Yang J, Han X, Shan L, Wang B, et al. Coexpression of CXCR4 and MMP9 predicts lung metastasis and poor prognosis in resected osteosarcoma. Tumour Biol (2016) 37:5089–96. doi: 10.1007/s13277-015-4352-8
202. Yang C, Tian Y, Zhao F, Chen Z, Su P, Li Y, et al. Bone microenvironment and osteosarcoma metastasis. Int J Mol Sci (2020) 21. doi: 10.3390/ijms21196985
203. Neklyudova O, Arlt MJE, Brennecke P, Thelen M, Gvozdenovic A, Kuzmanov A, et al. Altered CXCL12 expression reveals a dual role of CXCR4 in osteosarcoma primary tumor growth and metastasis. J Cancer Res Clin Oncol (2016) 142:1739–50. doi: 10.1007/s00432-016-2185-5
204. Oda Y, Yamamoto H, Tamiya S, Matsuda S, Tanaka K, Yokoyama R, et al. CXCR4 and VEGF expression in the primary site and the metastatic site of human osteosarcoma: Analysis within a group of patients, all of whom developed lung metastasis. Mod Pathol (2006) 19:738–45. doi: 10.1038/modpathol.3800587
205. Panse J, Friedrichs K, Marx A, Hildebrandt Y, Luetkens T, Barrels K, et al. Chemokine CXCL13 is overexpressed in the tumour tissue and in the peripheral blood of breast cancer patients. Br J Cancer (2008) 99:930–8. doi: 10.1038/sj.bjc.6604621
206. Mitkin NA, Hook CD, Schwartz AM, Biswas S, Kochetkov DV, Muratova AM, et al. p53-dependent expression of CXCR5 chemokine receptor in MCF-7 breast cancer cells. Sci Rep (2015) 5:9330. doi: 10.1038/srep09330
207. Liu JF, Lee CW, Lin CY, Chao CC, Chang TM, Han CK, et al. CXCL13/CXCR5 interaction facilitates VCAM-1-Dependent migration in human osteosarcoma. Int J Mol Sci (2020) 21(17):6095. doi: 10.3390/ijms21176095
208. Airoldi I, Cocco C, Morandi F, Prigione I, Pistoia V. CXCR5 may be involved in the attraction of human metastatic neuroblastoma cells to the bone marrow. Cancer Immunol Immunother (2008) 57:541–8. doi: 10.1007/s00262-007-0392-2
209. Chaturvedi P, Gilkes DM, Takano N, Semenza GL. Hypoxia-inducible factor-dependent signaling between triple-negative breast cancer cells and mesenchymal stem cells promotes macrophage recruitment. Proc Natl Acad Sci U.S.A. (2014) 111:E2120–9. doi: 10.1073/pnas.1406655111
210. Mir H, Singh R, Kloecker GH, Lillard JW Jr, Singh S. CXCR6 expression in non-small cell lung carcinoma supports metastatic process via modulating metalloproteinases. Oncotarget (2015) 6:9985–98. doi: 10.18632/oncotarget.3194
211. Hu W, Liu Y, Zhou W, Si L, Ren L. CXCL16 and CXCR6 are coexpressed in human lung cancer in vivo and mediate the invasion of lung cancer cell lines in vitro. PloS One (2014) 9:e99056. doi: 10.1371/journal.pone.0099056
212. Ajona D, Zandueta C, Corrales L, Moreno H, Pajares MJ, Ortiz-Espinosa S, et al. Blockade of the complement C5a/C5aR1 axis impairs lung cancer bone metastasis by CXCL16-mediated effects. Am J Respir Crit Care Med (2018) 197:1164–76. doi: 10.1164/rccm.201703-0660OC
213. Ma Y, Xu X, Luo M. CXCR6 promotes tumor cell proliferation and metastasis in osteosarcoma through the akt pathway. Cell Immunol (2017) 311:80–5. doi: 10.1016/j.cellimm.2016.11.001
214. Zhang F, Huang W, Sheng M, Liu T. MiR-451 inhibits cell growth and invasion by targeting CXCL16 and is associated with prognosis of osteosarcoma patients. Tumour Biol (2015) 36:2041–8. doi: 10.1007/s13277-014-2811-2
215. Steiner JL, Murphy EA. Importance of chemokine (CC-motif) ligand 2 in breast cancer. Int J Biol Markers (2012) 27:e179–85. doi: 10.5301/JBM.2012.9345
216. Lu X, Kang Y. Chemokine (C-c motif) ligand 2 engages CCR2+ stromal cells of monocytic origin to promote breast cancer metastasis to lung and bone. J Biol Chem (2009) 284:29087–96. doi: 10.1074/jbc.M109.035899
217. Zhu J, Jia X, Xiao G, Kang Y, Partridge NC, Qin L. EGF-like ligands stimulate osteoclastogenesis by regulating expression of osteoclast regulatory factors by osteoblasts: Implications for osteolytic bone metastases. J Biol Chem (2007) 282:26656–65. doi: 10.1074/jbc.M705064200
218. Regan DP, Chow L, Das S, Haines L, Palmer E, Kurihara JN, et al. Losartan blocks osteosarcoma-elicited monocyte recruitment, and combined with the kinase inhibitor toceranib, exerts significant clinical benefit in canine metastatic osteosarcoma. Clin Cancer Res (2022) 28:662–76. doi: 10.1158/1078-0432.CCR-21-2105
219. Ohba T, Cole HA, Cates JM, Slosky DA, Haro H, Ando T, et al. Bisphosphonates inhibit osteosarcoma-mediated osteolysis via attenuation of tumor expression of MCP-1 and RANKL. J Bone Miner Res (2014) 29:1431–45. doi: 10.1002/jbmr.2182
220. Liu JF, Chen PC, Chang TM, Hou CH. Monocyte chemoattractant protein-1 promotes cancer cell migration via c-Raf/MAPK/AP-1 pathway and MMP-9 production in osteosarcoma. J Exp Clin Cancer Res (2020) 39:254. doi: 10.1186/s13046-020-01756-y
221. Chen Q, Sun W, Liao Y, Zeng H, Shan L, Yin F, et al. Monocyte chemotactic protein-1 promotes the proliferation and invasion of osteosarcoma cells and upregulates the expression of AKT. Mol Med Rep (2015) 12:219–25. doi: 10.3892/mmr.2015.3375
222. Xu R, Li Y, Yan H, Zhang E, Huang X, Chen Q, et al. CCL2 promotes macrophages-associated chemoresistance via MCPIP1 dual catalytic activities in multiple myeloma. Cell Death Dis (2019) 10:781. doi: 10.1038/s41419-019-2012-4
223. Li Y, Zheng Y, Li T, Wang Q, Qian J, Lu Y, et al. Chemokines CCL2, 3, 14 stimulate macrophage bone marrow homing, proliferation, and polarization in multiple myeloma. Oncotarget (2015) 6:24218–29. doi: 10.18632/oncotarget.4523
224. Jamieson-Gladney WL, Zhang Y, Fong AM, Meucci O, Fatatis A. The chemokine receptor CX(3)CR1 is directly involved in the arrest of breast cancer cells to the skeleton. Breast Cancer Res (2011) 13:R91. doi: 10.1186/bcr3016
225. Shen F, Zhang Y, Jernigan DL, Feng X, Yan J, Garcia FU, et al. Novel small-molecule CX3CR1 antagonist impairs metastatic seeding and colonization of breast cancer cells. Mol Cancer Res (2016) 14:518–27. doi: 10.1158/1541-7786.MCR-16-0013
226. Liang Y, Yi L, Liu P, Jiang L, Wang H, Hu A, et al. CX3CL1 involves in breast cancer metastasizing to the spine via the Src/FAK signaling pathway. J Cancer (2018) 9:3603–12. doi: 10.7150/jca.26497
227. Sun C, Hu A, Wang S, Tian B, Jiang L, Liang Y, et al. ADAM17-regulated CX3CL1 expression produced by bone marrow endothelial cells promotes spinal metastasis from hepatocellular carcinoma. Int J Oncol (2020) 57:249–63. doi: 10.3892/ijo.2020.5045
228. Li S, Liu S, Xun J, Zhao F. Expression of CX3CL1 and CCL28 in spinal metastases of lung adenocarcinoma and their correlation with clinical features and prognosis. J Healthc Eng (2022) 2022:2580419. doi: 10.1155/2022/2580419
229. Liu Y, Ma H, Dong T, Yan Y, Sun L, Wang W. Clinical significance of expression level of CX3CL1-CX3CR1 axis in bone metastasis of lung cancer. Clin Transl Oncol (2021) 23:378–88. doi: 10.1007/s12094-020-02431-6
230. Wang FR, Xu SH, Wang BM, Wang F. MiR-485-5p inhibits metastasis and proliferation of osteosarcoma by targeting CX3CL1. Eur Rev Med Pharmacol Sci (2018) 22(21):7197–204. doi: 10.26355/eurrev_201811_16253
231. Liu JF, Tsao YT, Hou CH. Fractalkine/CX3CL1 induced intercellular adhesion molecule-1-dependent tumor metastasis through the CX3CR1/PI3K/Akt/NF-kappaB pathway in human osteosarcoma. Oncotarget (2017) 8:54136–48. doi: 10.18632/oncotarget.11250
232. Tsaur I, Noack A, Makarevic J, Oppermann E, Waaga-Gasser AM, Gasser M, et al. CCL2 chemokine as a potential biomarker for prostate cancer: A pilot study. Cancer Res Treat (2015) 47:306–12. doi: 10.4143/crt.2014.015
233. Dehghani M, Kianpour S, Zangeneh A, Mostafavi-Pour Z. CXCL12 modulates prostate cancer cell adhesion by altering the levels or activities of beta1-containing integrins. Int J Cell Biol (2014) 2014:981750. doi: 10.1155/2014/981750
234. Maynard JP, Ertunc O, Kulac I, Baena-Del Valle JA, De Marzo AM, Sfanos KS. IL8 expression is associated with prostate cancer aggressiveness and androgen receptor loss in primary and metastatic prostate cancer. Mol Cancer Res (2020) 18:153–65. doi: 10.1158/1541-7786.MCR-19-0595
235. Lieberman-Blum SS, Fung HB, Bandres JC. Maraviroc: A CCR5-receptor antagonist for the treatment of HIV-1 infection. Clin Ther (2008) 30:1228–50. doi: 10.1016/S0149-2918(08)80048-3
236. Carter NJ, Keating GM. Maraviroc. Drugs (2007) 67:2277–88. doi: 10.2165/00003495-200767150-00010
237. Miao M, De Clercq E, Li G. Clinical significance of chemokine receptor antagonists. Expert Opin Drug Metab Toxicol (2020) 16:11–30. doi: 10.1080/17425255.2020.1711884
238. Halama N, Zoernig I, Berthel A, Kahlert C, Klupp F, Suarez-Carmona M, et al. Tumoral immune cell exploitation in colorectal cancer metastases can be targeted effectively by anti-CCR5 therapy in cancer patients. Cancer Cell (2016) 29:587–601. doi: 10.1016/j.ccell.2016.03.005
239. Haag GM, Halama N, Springfeld C, Grün B, Apostolidis L, Zschaebitz S, et al. Combined PD-1 inhibition (Pembrolizumab) and CCR5 inhibition (Maraviroc) for the treatment of refractory microsatellite stable (MSS) metastatic colorectal cancer (mCRC): First results of the PICCASSO phase i trial. J Clin Oncol (2020) 38:3010–0. doi: 10.1200/JCO.2020.38.15_suppl.3010
240. Haag GM, Springfeld C, Grun B, Apostolidis L, Zschabitz S, Dietrich M, et al. Pembrolizumab and maraviroc in refractory mismatch repair proficient/microsatellite-stable metastatic colorectal cancer - the PICCASSO phase i trial. Eur J Cancer (2022) 167:112–22. doi: 10.1016/j.ejca.2022.03.017
241. Pervaiz A, Zepp M, Mahmood S, Ali DM, Berger MR, Adwan H. CCR5 blockage by maraviroc: A potential therapeutic option for metastatic breast cancer. Cell Oncol (Dordr) (2019) 42:93–106. doi: 10.1007/s13402-018-0415-3
242. Huang H, Zepp M, Georges RB, Jarahian M, Kazemi M, Eyol E, et al. The CCR5 antagonist maraviroc causes remission of pancreatic cancer liver metastasis in nude rats based on cell cycle inhibition and apoptosis induction. Cancer Lett (2020) 474:82–93. doi: 10.1016/j.canlet.2020.01.009
243. Conley-LaComb MK, et al. Pharmacological targeting of CXCL12/CXCR4 signaling in prostate cancer bone metastasis. Mol Cancer (2016) 15(1):68. doi: 10.1186/s12943-016-0552-0
244. Domanska UM, Timmer-Bosscha H, Nagengast WB, Oude Munnink TH, Kruizinga RC, Ananias HJ, et al. CXCR4 inhibition with AMD3100 sensitizes prostate cancer to docetaxel chemotherapy. Neoplasia (2012) 14:709–18. doi: 10.1593/neo.12324
245. Loberg RD, Ying C, Craig M, Day LL, Sargent E, Neeley C, et al. Targeting CCL2 with systemic delivery of neutralizing antibodies induces prostate cancer tumor regression in vivo. Cancer Res (2007) 67:9417–24. doi: 10.1158/0008-5472.CAN-07-1286
246. Kirk PS, Koreckij T, Nguyen HM, Brown LG, Snyder LA, Vessella RL, et al. Inhibition of CCL2 signaling in combination with docetaxel treatment has profound inhibitory effects on prostate cancer growth in bone. Int J Mol Sci (2013) 14:10483–96. doi: 10.3390/ijms140510483
247. Pienta KJ, Machiels JP, Schrijvers D, Alekseev B, Shkolnik M, Crabb SJ, et al. Phase 2 study of carlumab (CNTO 888), a human monoclonal antibody against CC-chemokine ligand 2 (CCL2), in metastatic castration-resistant prostate cancer. Invest New Drugs (2013) 31:760–8. doi: 10.1007/s10637-012-9869-8
248. Brana I, Calles A, LoRusso PM, Yee LK, Puchalski TA, Seetharam S, et al. Carlumab, an anti-C-C chemokine ligand 2 monoclonal antibody, in combination with four chemotherapy regimens for the treatment of patients with solid tumors: an open-label, multicenter phase 1b study. Target Oncol (2015) 10:111–23. doi: 10.1007/s11523-014-0320-2
249. Urata S, Izumi K, Hiratsuka K, Maolake A, Natsagdorj A, Shigehara K, et al. C-c motif ligand 5 promotes migration of prostate cancer cells in the prostate cancer bone metastasis microenvironment. Cancer Sci (2018) 109:724–31. doi: 10.1111/cas.13494
250. Kasina S, Macoska JA. The CXCL12/CXCR4 axis promotes ligand-independent activation of the androgen receptor. Mol Cell Endocrinol (2012) 351:249–63. doi: 10.1016/j.mce.2011.12.015
251. Luo J, Lee SO, Cui Y, Yang R, Li L, Chang C. Infiltrating bone marrow mesenchymal stem cells (BM-MSCs) increase prostate cancer cell invasion via altering the CCL5/HIF2alpha/androgen receptor signals. Oncotarget (2015) 6:27555–65. doi: 10.18632/oncotarget.4515
252. Luo J, Ok Lee S, Liang L, Huang CK, Li L, Wen S, et al. Infiltrating bone marrow mesenchymal stem cells increase prostate cancer stem cell population and metastatic ability via secreting cytokines to suppress androgen receptor signaling. Oncogene (2014) 33:2768–78. doi: 10.1038/onc.2013.233
253. Zhao R, Bei X, Yang B, Wang X, Jiang C, Shi F, et al. Endothelial cells promote metastasis of prostate cancer by enhancing autophagy. J Exp Clin Cancer Res (2018) 37:221. doi: 10.1186/s13046-018-0884-2
254. Lin TH, Izumi K, Lee SO, Lin WJ, Yeh S, Chang C. Anti-androgen receptor ASC-J9 versus anti-androgens MDV3100 (Enzalutamide) or casodex (Bicalutamide) leads to opposite effects on prostate cancer metastasis via differential modulation of macrophage infiltration and STAT3-CCL2 signaling. Cell Death Dis (2013) 4:e764. doi: 10.1038/cddis.2013.270
255. Lean JM, Murphy C, Fuller K, Chambers TJ. CCL9/MIP-1gamma and its receptor CCR1 are the major chemokine ligand/receptor species expressed by osteoclasts. J Cell Biochem (2002) 87:386–93. doi: 10.1002/jcb.10319
256. Maolake A, Izumi K, Shigehara K, Natsagdorj A, Iwamoto H, Kadomoto S, et al. Tumor-associated macrophages promote prostate cancer migration through activation of the CCL22-CCR4 axis. Oncotarget (2017) 8:9739–51. doi: 10.18632/oncotarget.14185
257. Sharma PK, Singh R, Novakovic KR, Eaton JW, Grizzle WE, Singh S. CCR9 mediates PI3K/AKT-dependent antiapoptotic signals in prostate cancer cells and inhibition of CCR9-CCL25 interaction enhances the cytotoxic effects of etoposide. Int J Cancer (2010) 127:2020–30. doi: 10.1002/ijc.25219
258. Ota K, Quint P, Weivoda MM, Ruan M, Pederson L, Westendorf JJ, et al. Transforming growth factor beta 1 induces CXCL16 and leukemia inhibitory factor expression in osteoclasts to modulate migration of osteoblast progenitors. Bone (2013) 57:68–75. doi: 10.1016/j.bone.2013.07.023
259. Munoz MA, Fletcher EK, Skinner OP, Jurczyluk J, Kristianto E, Hodson MP, et al. Bisphosphonate drugs have actions in the lung and inhibit the mevalonate pathway in alveolar macrophages. Elife (2021) 10. doi: 10.1016/j.bone.2013.07.023
260. Byrum ML, Pondenis HC, Fredrickson RL, Wycislo KL, Fan TM. Downregulation of CXCR4 expression and functionality after zoledronate exposure in canine osteosarcoma. J Vet Intern Med (2016) 30:1187–96. doi: 10.1111/jvim.14257
261. Denoyelle C, Hong L, Vannier JP, Soria J, Soria C. New insights into the actions of bisphosphonate zoledronic acid in breast cancer cells by dual RhoA-dependent and -independent effects. Br J Cancer (2003) 88:1631–40. doi: 10.1038/sj.bjc.6600925
262. Miwa S, Mizokami A, Keller ET, Taichman R, Zhang J, Namiki M. The bisphosphonate YM529 inhibits osteolytic and osteoblastic changes and CXCR-4-induced invasion in prostate cancer. Cancer Res (2005) 65:8818–25. doi: 10.1158/0008-5472.CAN-05-0540
263. Li C, Zhao J, Sun L, Yao Z, Liu R, Huang J, et al. RANKL downregulates cell surface CXCR6 expression through JAK2/STAT3 signaling pathway during osteoclastogenesis. Biochem Biophys Res Commun (2012) 429:156–62. doi: 10.1016/j.bbrc.2012.10.122
264. Saitoh Y, Koizumi K, Sakurai H, Minami T, Saiki I. RANKL-induced down-regulation of CX3CR1 via PI3K/Akt signaling pathway suppresses Fractalkine/CX3CL1-induced cellular responses in RAW264.7 cells. Biochem Biophys Res Commun (2007) 364:417–22. doi: 10.1016/j.bbrc.2007.09.137
265. Suominen MI, Wilson T, Kakonen SM, Scholz A. The mode-of-Action of targeted alpha therapy radium-223 as an enabler for novel combinations to treat patients with bone metastasis. Int J Mol Sci (2019) 20. doi: 10.3390/ijms20163899
266. Morris MJ, Corey E, Guise TA, Gulley JL, Kevin Kelly W, Quinn DI, et al. Radium-223 mechanism of action: Implications for use in treatment combinations. Nat Rev Urol (2019) 16:745–56. doi: 10.1038/s41585-019-0251-x
267. Zheng Z, Jia S, Shao C, Shi Y. Irradiation induces cancer lung metastasis through activation of the cGAS-STING-CCL5 pathway in mesenchymal stromal cells. Cell Death Dis (2020) 11:326. doi: 10.1038/s41419-020-2546-5
268. Wang L, Jiang J, Chen Y, Jia Q, Chu Q. The roles of CC chemokines in response to radiation. Radiat Oncol (2022) 17:63. doi: 10.1186/s13014-022-02038-x
269. Su L, Dong Y, Wang Y, Wang Y, Guan B, Lu Y, et al. Potential role of senescent macrophages in radiation-induced pulmonary fibrosis. Cell Death Dis (2021) 12:527. doi: 10.1038/s41419-021-03811-8
270. Fujimori A, Okayasu R, Ishihara H, Yoshida S, Eguchi-Kasai K, Nojima K, et al. Extremely low dose ionizing radiation up-regulates CXC chemokines in normal human fibroblasts. Cancer Res (2005) 65:10159–63. doi: 10.1158/0008-5472.CAN-05-2015
271. Vaday GG, Peehl DM, Kadam PA, Lawrence DM. Expression of CCL5 (RANTES) and CCR5 in prostate cancer. Prostate (2006) 66:124–34. doi: 10.1002/pros.20306
272. Guo Y, Zang Y, Lv L, Cai F, Qian T, Zhang G, et al. IL8 promotes proliferation and inhibition of apoptosis via STAT3/AKT/NFkappaB pathway in prostate cancer. Mol Med Rep (2017) 16:9035–42. doi: 10.3892/mmr.2017.7747
273. Jung Y, Cackowski FC, Yumoto K, Decker AM, Wang J, Kim JK, et al. CXCL12gamma promotes metastatic castration-resistant prostate cancer by inducing cancer stem cell and neuroendocrine phenotypes. Cancer Res (2018) 78:2026–39. doi: 10.1158/0008-5472.CAN-17-2332
274. Gruber HE, Marrero E, Ingram JA, Hoelscher GL, Hanley EN Jr. The chemokine, CXCL16, and its receptor, CXCR6, are constitutively expressed in human annulus fibrosus and expression of CXCL16 is up-regulated by exposure to IL-1ss in vitro. Biotech Histochem (2017) 92:7–14. doi: 10.1080/10520295.2016.1237672
275. Zhang J, Lu Y, Pienta KJ. Multiple roles of chemokine (C-c motif) ligand 2 in promoting prostate cancer growth. J Natl Cancer Inst (2010) 102:522–8. doi: 10.1093/jnci/djq044
Keywords: bone, prostate, cancer, metastasis, chemokine, therapy
Citation: Johnson CS and Cook LM (2023) Osteoid cell-derived chemokines drive bone-metastatic prostate cancer. Front. Oncol. 13:1100585. doi: 10.3389/fonc.2023.1100585
Received: 16 November 2022; Accepted: 07 March 2023;
Published: 21 March 2023.
Edited by:
Leigh Ellis, Cedars Sinai Medical Center, United StatesReviewed by:
Kouji Izumi, Kanazawa University, JapanSerk In Park, Korea University, Republic of Korea
Copyright © 2023 Johnson and Cook. This is an open-access article distributed under the terms of the Creative Commons Attribution License (CC BY). The use, distribution or reproduction in other forums is permitted, provided the original author(s) and the copyright owner(s) are credited and that the original publication in this journal is cited, in accordance with accepted academic practice. No use, distribution or reproduction is permitted which does not comply with these terms.
*Correspondence: Leah M. Cook, bGVhaC5jb29rQHVubWMuZWR1
†ORCID: Catherine S. Johnson, orcid.org/0000-0002-1679-180X
Leah M. Cook, orcid.org/0000-0002-9996-7419