- 1Department of Nuclear Medicine, Affiliated Hospital of North Sichuan Medical College, North Sichuan Medical College, Nanchong, China
- 2School of Health Management, Xihua University, Chengdu, China
Background: Hepatocellular carcinoma (HCC) is one of the most prevalent and deadly malignant tumors with serious clinical and socioeconomic consequences. Although gene therapy holds great promise in the treatment of hepatoma, its clinical applications are hindered by uncontrolled gene transmission and transcription.
Methods: The pY-ads-8-5HRE-cfosp-IFNG plasmid was constructed and identified by double enzyme digestion and gene sequencing. The expression of pYr-ads-8-5HRE-cfosp-IFNG in HepG2 cells was detected by quantitative PCR. PEI-Fe3O4/pYr-ads-8-5HRE-cfosp-IFNG albumin nanospheres were prepared and characterized. In vitro heating test of magnetic albumin nanospheres in an alternating magnetic field (AMF) was carried out. The therapeutic effect of PEI-Fe3O4/pYr-ads-8-5HRE-cfosp-IFNG albumin nanospheres on hepatocellular carcinoma was investigated by cell and animal experiments. After treatment, mice blood was collected for clinical biochemical analysis and histopathological evaluation of major organs was performed to assess potential adverse effects of treatment.
Results: Double enzyme digestion and gene sequencing showed that the pY-ads-8-5HRE-cfosp-IFNG plasmid was constructed successfully. QPCR results showed that the IFNγ transcript level in the PEI-Fe3O4/pYr-ads-8-5HRE-cfosp-IFNG group was higher than that in the PEI-Fe3O4/pYr-ads-8-cfosp-IFNG group after being treated with hypoxia (P<0.05). TEM revealed that the self-prepared PEI-Fe3O4/pYr-ads-8-5HRE-cfosp-IFNG albumin nanospheres exhibit an approximately spherical or elliptical shape. The hydrodynamic size of the albumin nanospheres was 139.7 nm. The maximum temperature of 0.25 mg/mL solution is stable at about 44°C, which is suitable for tumor thermal therapy without damaging normal tissues. The relative cell inhibition rate of the radiation-gene therapy and MFH combination group was higher than that of other control groups in CCK8 experiment. (P<0.05) Flow cytometry showed that the apoptosis rate and necrosis rate of the combined treatment group were 42.32% and 35.73%, respectively, higher than those of the other groups. (P<0.05) In animal experiments, the mass and volume inhibition rates of the combined treatment group were 66.67% and 72.53%, respectively, higher than those of other control groups. (P<0.05) Clinical biochemical analysis and histopathological evaluation showed no abnormality.
Conclusions: The results indicated the successful construction of the radiation-induced plasmid and demonstrated that the hypoxia enhancer could augment the expression of INFγ in a hypoxia environment. Gene therapy combined with magnetic fluid hyperthermia (MFH) has exhibited excellent outcomes in both cell and animal studies. Our experiments demonstrated that the PEI-Fe3O4/pYr-ads-8-5HRE-cfosp-IFNG albumin nanospheres system is a comprehensive treatment method for hepatoma, which can effectively combine immune genre therapy with hyperthermia.
Introduction
In the ranking of malignant tumor incidence in the world, hepatoma is the fifth. It is also one of the malignant tumors with the highest morbidity and mortality in China. Hepatoma has the characteristics of insidious onset, rapid progression, early recurrence and poor prognosis (1). Many patients with hepatoma are diagnosed at an advanced stage. With the improvement of early diagnosis rate of hepatoma, the improvement of surgery and interventional therapy and the adhibition of comprehensive therapy, the prognosis of patients with early hepatoma is satisfactory. However, the prognosis of patients with middle to advanced hepatoma is still poor.
Hepatoma is a complex, multi-step biological process involving changes in adhesion factors, matrix metalloproteinases, corresponding signal transduction matrix, and related genes. With the deepening of research on molecular signaling pathways and the tumor microenvironment of hepatoma, gene therapy for hepatoma has rapidly developed into a new treatment mode after surgery, radiotherapy, chemotherapy, and interventional therapy.
Gene therapy is a kind of treatment method which uses molecular biology technology to transplant selected genes into patients to correct abnormal gene expression. Gene therapy is mainly applied to remedy illnesses that cause grievous damage to human health, such as infectious diseases, malignant tumors, cardiovascular illnesses, and genetic illnesses (2). At present, many gene therapy methods (such as suicide genes, immune genes, RNA interference, etc.) are increasingly used in tumor therapy and have achieved exciting results. IFNγ, IL-2, and TNFα are widely used in immune gene therapy, and their curative effects are clear. The local transcription and expression of immune genes in cancer cells can increase the immunogenicity of cancer cells, thus stimulating and enhancing the body’s immune response to cancer cells and eliminating them as soon as possible. After the cancer cells are killed, the expression of immune genes also stops, and the initial triggering effect is over. IFNγ kills tumors in two ways. On the one hand, it has a direct anti-tumor effect, which is realized by inducing differentiation and accelerating tumor cell apoptosis, restraining tumor cell proliferation (3, 4). After the cancer cells are killed, the expression of immune genes also stops, and the initial triggering effect is over. IFNγ kills tumors in two ways. On the one hand, it has a direct anti-tumor effect, which is realized by inducing differentiation and accelerating tumor cell apoptosis, restraining tumor cell proliferation (5). Immunogene therapy can be combined with the body’s immune response to treat tumors, making it a worthy research direction of gene therapy for hepatoma.
Radio-gene therapy is the fusion of therapeutic genes with radiation-induced promoters. Upon radiation/radionuclide exposure, the radiation promoter is activated, and the therapeutic gene is expressed. In this process, radiotherapy and gene therapy can synergistically kill tumors, and localization of anti-tumor gene expression can be achieved by local irradiation. Moreover, the dose of radiation in the combination therapy is smaller under the premise of achieving the same anti-tumor effect (6).
However, like traditional gene therapy, this method has some shortcomings, such as issues with specificity and safety. Additionally, the general hypoxic environment of solid tumors can reduce the efficiency of the promoter induced by radiation, which affects the curative effect and application of this therapy (7, 8). Some researchers have found that inserting the hypoxia-response element (HRE) sequence upstream of the gene promoter can effectively solve the aforementioned problems. HRE is an enhancer sequence that could enhance promoter sensitivity and activity in hypoxic environments (9, 10).
Naked DNA is unstable in organism because it is easily degraded by nuclease (11). So far, there are two main gene transfer carriers: viral systems and non-viral systems, each having its own strengths and weaknesses. Viral gene vectors are currently the most efficient ones, but they pose serious biosecurity risks and have some disadvantages, such as strong self-immunogenicity, poor target specificity, and limited gene capacity, which greatly limit their clinical application. Although non-viral vectors avoid significant safety risks, the transfection efficiency of most non-viral vectors is not satisfactory, making it difficult to obtain meaningful target gene expression (12). Nowadays, the most commonly used non-viral carrier transfection methods are liposome transfection and electroporation transfection. Both methods have high transfection efficiency. However, liposomes have high cytotoxicity, which causes them to be quickly cleared by the body. Therefore, the application of liposomes must first solve this problem. Electrotransfection can only be used in vitro, and the gene is only transiently expressed in cells or tissues, so it cannot be used for transfection in vivo (13). Therefore, solving the problem of gene transfer is still the primary challenge in current gene therapy.
In recent years, nanotechnology has received much attention in various fields, including its application in the development of gene transfer vectors (14–18). Nanoparticles-based gene transfer vectors have attracted the attention of scholars (19, 20) due to their low biotoxicity and large surface area (21–25). Compared with traditional vectors, nano-vectors offer several advantages, such as slow and sustained gene release and maintenance of effective concentration for an extended period of time. Moreover, nano-vectors are highly safe and can be repeatedly injected to improve transfection efficiency. Among the various types of magnetic nanoparticles (MNPs), Fe3O4 MNPs are the most frequently used due to their excellent biocompatibility, low immunogenicity, superparamagnetism, and other interesting properties (26). The superparamagnetism effect allows MNPs to target tissues under an applied magnetic field, achieving efficient transfection, which enables targeted gene therapy. Additionally, MNPs can be used for hyperthermia of tumors as they can be heated through magnetic induction in an external magnetic field.
Hyperthermia is a therapeutic method used to treat cancer by destroying cancer cells and making them more sensitive to chemotherapy and radiotherapy. Therefore, it is often used in combination with gene therapy, chemotherapy, and radiotherapy to produce synergistic and supplementary effects. However, achieving uniform heating of the tumor tissue to an ideal temperature without damaging normal tissue during treatment is a challenging technical problem. Magnetic fluid hyperthermia (MFH) is a tumor hyperthermia method that combines magnetic induction heating with nanotechnology. This treatment allows for targeted localization, meaning that only tissues with magnetic nanoparticles experience an increase in temperature under an external magnetic field, while tissues without magnetic nanoparticles are unaffected by heat. As the intake of magnetic nanoparticles in tumor tissues is much higher than that in normal tissues, MFH can destroy tumor tissues while simultaneously protecting normal tissues (27–29).
In some of our previous studies, we chemically prepared Fe2O3 and Fe3O4 magnetic nanoparticles and applied them to the hyperthermia of hepatoma and lung cancer with good results. The Curie temperature of the magnetic fluid can be controlled by adjusting the iron concentration. Fe3O4 nanoparticles can absorb electromagnetic waves below Curie temperature and heat up in alternating magnetic fields (AMF). Once the Curie temperature is reached, Fe3O4 nanoparticles becomes non-magnetic, loses their capacity to take in electromagnetic waves, and the temperature begins to drop. When the temperature is below the Curie temperature, the Fe3O4 magnetic fluid begins to heat up again. Therefore, the temperature is cycled within the range set by Curie temperature consistently, such as 42-44 degrees Celsius, which is the effective hyperthermia temperature for cancer, and this temperature will not cause damage to normal tissues. Thus, we successfully solved the issue of temperature measurement and regulation in tumor hyperthermia, and improved the safety and stability of hyperthermia (23, 30).
Magnetic nanoparticles are coated with an albumin shell called magnetic albumin nanospheres (MANS) (31). Albumin nanospheres are often used as drug carriers, which can lower drug discharge rate and avoid drug inactivation in the course of transfer. Magnetic nanoparticles’ biocompatibility can be improved when albumin is coated with nano-materials. Placing a magnetic field outside the tumor site can make the drug-loaded magnetic albumin nanoparticles focus on tumor site. Thereby enhancing the anti-tumor effect of the antineoplastic drug and reducing the harm to normal tissues (32, 33).
Based on the above, we constructed a eukaryotic recombinant plasmid pYr-ads-8-5HRE-cfosp-IFNG and hypothesized that Fe3O4 nanoparticles could be used as gene carriers to target transport radiation-induced immune genes to tumor tissues in an external magnetic field. Meanwhile, Fe3O4 nanoparticles can also be used as magnetic media for hyperthermia, combining immunogene therapy and hyperthermia for tumor. Thus, we aim to explore an adjustable and precise combination therapy for hepatoma.
Materials and methods
Main materials
Restriction enzyme, Trizol, DNA maker and RNasin were purchased from Takara Biomedical Technology (Japan) Co., Ltd. Tryptone, and BSA were purchased from Sigma-Aldrich Co. (USA). HepG2 cells were purchased from the Institute of Biochemistry and Cell Biology, Shanghai Institute of Biological Sciences, Chinese Academy of Sciences. Plasmid extraction kit from Axygen (USA). Fetal bovine serum and DMEM medium were purchased from Gibco BRL Co. (USA); plasmid pDONR223-IFNG, pYr-ads-8-cfosp and pUC57-Simple-5HRE was synthesized by Biotech Co. Ltd., Changsha Ying Run (China).
Construction and identification of pYr-ads-8-5HRE-cfosp-IFNG plasmid
The 5HRE fragment was obtained by double restriction enzyme digestion of NheI and BglII, and the template plasmid was pUC57-5HRE plasmid. Then the 5HRE fragment was ligated with pYr-ads-8-cfosp to construct a plasmid containing 5HRE-cfosp fragment. With pDONR223-IFNG as template, the fragment of IFNG CDS region was amplified by PCR, and inserted into EcoRI-BamHI site of pYr-ads-8-5HRE-cfosp plasmid, and finally the pY-ads-8-5HRE-cfosp-IFNG eukaryotic plasmid was constructed. The constructed plasmid was identified by double enzyme digestion and gene sequencing (Figure 1).
Preparation and characterization of Fe3O4 nanoparticles and PEI-Fe3O4 magnetic nanoparticles
Fe3O4 nanoparticles were synthetized by chemical coprecipitation method. The synthesis steps of Fe3O4 nanoparticles are shown below. A 100 ml solution of FeCl3 with a concentration of 0.1mol/L and a 600ml solution of FeCl2 with a concentration of 0.1mol/L were prepared. The two solutions were then added to a jar and mixed for 10 minutes under nitrogen protection. The PH of the mixed solution was adjusted to 9 with 1.5mol/L ammonia (22).
In the mixture, black sediment rapidly appeared. The suspension was stirred continuously for 30 minutes. After incubation at 90 degrees Celsius for 30 minutes, the jar was placed on a strong permanent magnet. When the black sediment had sunk to the bottom of the jar, the supernatant was discarded. The black sediment was washed three times with deionized water and dried under vacuum. The morphology, structure, and size of Fe3O4 nanoparticles were observed using a high-resolution transmission electron microscope (TEM) (JEM-2100, Jeol, Japan). The hydrated particle size and zeta potential of Fe3O4 were measured using a Zetasizer Nano (Nano ZS, Malvern, UK).
The modification of Fe3O4 nanoparticles with PEI was performed as follows (22): Fe3O4 magnetic fluid was prepared with buffered saline as a solvent at a 4% concentration. The magnetic fluid was dispersed with ultrasound as much as possible. The suspension was stirred on a shaking table at room temperature for 24 h. PEI-Fe3O4 nanoparticles were separated from the suspension using a permanent magnet and washed three times each with deionized water and methanol. The PEI-Fe3O4 nanoparticles were dried in a vacuum drying chamber.
The morphology and size of PEI-Fe3O4 nanoparticles were observed by high-resolution TEM. The hydrated particle size and zeta potential of PEI-Fe3O4 were measured by Zetasizer Nano. Fourier transform infrared spectroscopy (FTIR) (Nicolet IS5, Thermo, USA) was used to verify whether PEI was successfully modified on the surface of Fe3O4 nanoparticles.
Preparation and characteristics of PEI-Fe3O4/pYr-ads-8-5HRE-cfosp-IFNG albumin nanospheres
In our previous experiments, we investigated the optimal mass ratio of PEI-Fe3O4 MNPs to plasmid pYr-ads-8-5HRE-cfosp-IFNG (30). Based on this mass ratio, a 20ml suspension was prepared and allowed to stand for 30 minutes. Then, 100 mg of BSA was added to the suspension stirred. The pH of the suspension was adjusted to 9 using NaOH. Ethanol was slowly dripped into the suspension at a rate of 1 mL/min, followed by the addition of 50 μL of 2.5% glutaraldehyde. The suspension was continuously stirred at room temperature for 24 h. After high-speed centrifugation, the supernatant was discarded, and the nanospheres were washed with deionized water three times to obtain PEI-Fe3O4/pYr-ads-8-5HRE-cfosp-IFNG albumin nanospheres. The nanospheres were characterized by TEM and Zetasizer Nano.
The expression of pYr-ads-8-5HRE-cfosp-IFNG in HepG2 cells was detected by quantitative PCR
HepG2 cells were added to 6-well plates at a density of 3×105 cells/well, and 2ml of cell suspension was added to each well. After 24 hours of incubation at 37°C, when the cell confluence level reached 80%, the cells were divided into 4 groups, and 2ml of following nanospheres and serum-free medium mixture was added to each group, respectively (1): PEI-Fe3O4 nanospheres. (2) PEI-Fe3O4/pDONR223-IFNG nanospheres. (3) PEI-Fe3O4/pYr-ads-8-cfosp-IFNG nanospheres. (4) PEI-Fe3O4/pYr-ads-8-5HRE-cfosp-IFNG nanospheres. Cells were incubated for 4 hours and fresh medium containing serum was added to the cells as a replacement. The cells were then incubated for 24 h at 37°C and exposed to 2Gy of X-ray (RS2000Pro, RAD, USA). The cells were further cultured for 48 h at 37°C under hypoxic conditions (1%O2, 5%CO2 and N2 in equilibrium). Total RNA was extracted from the cells after incubation. Untreated HepG2 cells served as a blank control group. The internal reference gene was GAPDH. Quantitative polymerase chain reaction (qPCR) was used to quantitatively analyzed the expression level of IFNγ gene. The primer sequences were obtained from Nan Jing Jin Sirui Company (China)
Heating test of PEI-Fe3O4/pYr-ads-8-5HRE-cfosp-IFNG albumin nanospheres
The concentration of iron in the PEI-Fe3O4/pYr-ads-8-5HRE-cfosp-IFNG albumin nanospheres was determined using the thiocyanate spectrophotometric method (34). Various doses of PEI-Fe3O4/pYr-ads-8-5HRE-cfosp-IFNG albumin nanospheres were prepared as magnetic fluids with 5 mL normal saline. The iron concentrations in these magnetic fluids were 0.125, 0.25, 0.375, 0.5, 0.625, 0.75, 0.875, and 1.0 mg/mL, respectively. 2 mL of magnetic fluids with different concentrations were added into flat-bottomed vessels. These vessels were placed at the center of the hyperthermia coil of high-frequency AMF (alternating magnetic field) (SP-04C, Shuangping, China). The parameters of high-frequency electromagnetic field, including output power and output circuit, were set to 230kHz and 25A, respectively. The total heating time was 1 hour, and the temperature was measured every 5 minutes.
The therapeutic effect of PEI-Fe3O4/pYr-ads-8-5HRE-cfosp-IFNG albumin nanospheres on hepatoma
CCK8 assay
HepG2 cells were cultured in three 96-well plates with a density of 4×104 cells/well. The cells in the three plates were incubated for 24 h. Subsequently, the cells in each plate were divided into five groups as follows:
(1) The radiation-gene therapy and MFH combination group (PEI-Fe3O4/pYr-ads-8-5HRE-cfosp-IFNG albumin nanospheres were added);
(2) The radiation-gene therapy group (PEI-Fe3O4/pYr-ads-8-5HRE-cfosp-IFNG albumin nanospheres were added);
(3) The MFH group (PEI-Fe3O4/pYr-ads-8-5HRE-cfosp-IFNG albumin nanospheres were added);
(4) The radiation alone group (without nanospheres);
(5) Negative control group (without nanospheres).
PEI-Fe3O4/pYr-ads-8-5HRE-cfosp-IFNG albumin nanospheres were added to the MFH group, the radiation-gene therapy group, and the radiation-gene therapy group. An equal volume of DMEM nutrient solution was added to the radiation alone group and negative control group. The cells were incubated for another 24 hours at 37°C, and then the 96-well plates of the MFH group, the radiation-gene therapy group, and the radiation-gene therapy group were put on a strong permanent magnet (Magneto FACTOR plate) from Germany. All the groups that need to be heated were put on a high-frequency AMF and heated for 1 hour (f=230 kHz, I=25A) (35). All the radiation groups were exposed to 2Gy of X-ray radiation using an X-ray biological irradiator (30).
The three 96-well plates were incubated for 24 h,48 h, and 72 h, respectively. 10 μL of CCK8 solution was added into each well of the plates and incubated at 37 degrees Celsius for 1–4 h. The optical density (OD) of each group at 450 nm was measured by a spectrophotometer (Infinite 50, Tecan, CH). The relative inhibition rate (RIR) of cell proliferation (36) could be calculated using the following formula:
The apoptosis rate of HepG2 cells was analyzed by flow cytometry
HepG2 cells were cultured in 5 culture vials with a density of 3×105 cells/mL. Then, the grouping and treatment were the same as in the CCK8 experiment. At the end of the treatment, the cells were cultured for another 48 hours. The cells were resuspended with 1× binding buffer at a density of 1×106 cells/mL after being washed with cold PBS three times. For each group, 100 μL of cell suspension was poured into a 5 mL tube. 5 μL of fluorescein isothiocyanate Annexin V and 5 μL of propidium iodide (PI) were added to each tube. After the cells were incubated for 15 min at 25°C in the dark, 400 μL of 1× binding buffer was added to each tube. The apoptosis of the cells was analyzed by flow cytometry within 1 hour. (FCM, Vantage SE, BD, USA) (37).
Intracellular analysis of HepG2 cells treated with Fe3O4 nanoparticles by TEM
The intake of Fe3O4 nanoparticles in HepG2 cells was analyzed by TEM (JEM- 200CX, JBOL, Japan). HepG2 cells (4×104) were grown in a 12-well culture plate for 24h and then incubated with Fe3O4 nanoparticles (PEI-Fe3O4/pYr-ads-8-cfosp-IFNG) under standard condition (37°C, 5% CO2). After incubation for 24 hours, the cells were fixed with 2.5% glutaraldehyde for more than 6 hours (4°C). Then, the cells were prepared according to the conventional procedure of transmission electron microscope ultrathin section. The finished samples were observed under TEM at 80 kV.
Animal experiments
BALB/c nude mice (6-weeks-old, weighing 20–22 g), with half male and half female, were obtained from the Animal Experimental Center, Institute of Biochemistry and Cell Biology, Shanghai Academy for Biological Sciences, China. The animal experiments were approved by Ethics Committee of North Sichuan Medical College (NSMC-AEC 2021 [157]) and carried out according to institutional guidelines. The nude mice were raised in the Experimental Center of North Sichuan Medical College. HepG2 cells at a density of 2 ×106 cells were injected subcutaneously into the buttocks of the right hind limbs of the mice.
When the transplanted tumor grew to 1 cm in diameter, the mice were divided into five groups, each containing six mice. A multi-point injection strategy was used to inject the required drugs at 3, 6, 9 and 12 points of the transplanted tumor. The specific procedures were as follows:
(1) Radiation-gene therapy and MFH combination group: PEI-Fe3O4/pYr-ads-8-5HRE-cfosp-IFNG albumin nanospheres (plasmid: 10 μg/mouse), combined with high-frequency AMF exposure.
(2) The radiation-gene therapy group: PEI-Fe3O4/pYr-ads-8-5HRE-cfosp-IFNG albumin nanospheres (plaimid: 10 μg/mouse).
(3) MFH group: PEI-Fe3O4/pYr-ads-8-5HRE-cfosp-IFNG albumin nanospheres (plasmid: 10 μg/mouse), heated on a high frequency AMF for 1 hour.
(4) Radiation alone group:1 ml PEI-Fe3O4 albumin nanospheres;
(5) Negative control group:1 ml 0.9% NaCl.
The tumors in groups (1), (3), and (4) received 2 Gy of X-ray radiation under an X-ray biological irradiator every other day, three times in total. The tumors in groups (1) and (3) were heated on a high frequency AMF for 1 hour, with a frequency of 230 kHz and a current of 25A. The temperature of the tumors was measured at multiple points using an infrared thermometer (ZyTemp-TN18, Xingtaiheng, China). After treatment, the nude mice were fed for 6 weeks and then sacrificed. The volume and mass of the tumors were measured. And the tumor mass and volume inhibition rate (38) were calculated according to the following formula.
Long-term safety of Fe3O4 magnetic nanoparticles
Before sacrificing the mice, blood samples were collected in EDTA-coated tubes, and hematological parameters were analyzed immediately using an automatic hematology analyzer (KX-21, Sysmex, Japan), including platelet (PLT), red blood cell (RBC), white blood cell (WBC), and hemoglobin (HGB). Additionally, an automated analyzer platform (Cobas C501, Roche, Chian) was used to measure creatinine (CREA), alkaline phosphatase (ALP), triglycerides (TGs), total cholesterol (TC), high-density lipoprotein (HDL), low-density lipoprotein (LDL), and blood urea nitrogen (UREA). Histopathological evaluations were performed to assess any potential adverse effects of the treatments. After the experiment, the heart, spleen, lung, and kidney of the nude mice were removed and fixed with paraformaldehyde to prepare pathological sections for observation under the microscope.
Statistical analysis
All experiment data were processed by SPSS 25.0 software. P<0.05 was considered statistically significant.
Results
Identification of pYr-ads-8-5HRE-cfosp-IFNG
Because the theoretical structure of p5HRE-cfosp-IFNG is clear, double digestion of the constructed plasmid with EcoRI and MluI restriction enzymes will result in 800 bp fragment and a 5.5 K fragment. Agarose gel electrophoresis verified that the obtained fragments from the digested plasmid were consistent with the predicted outcomes (Figure 2).
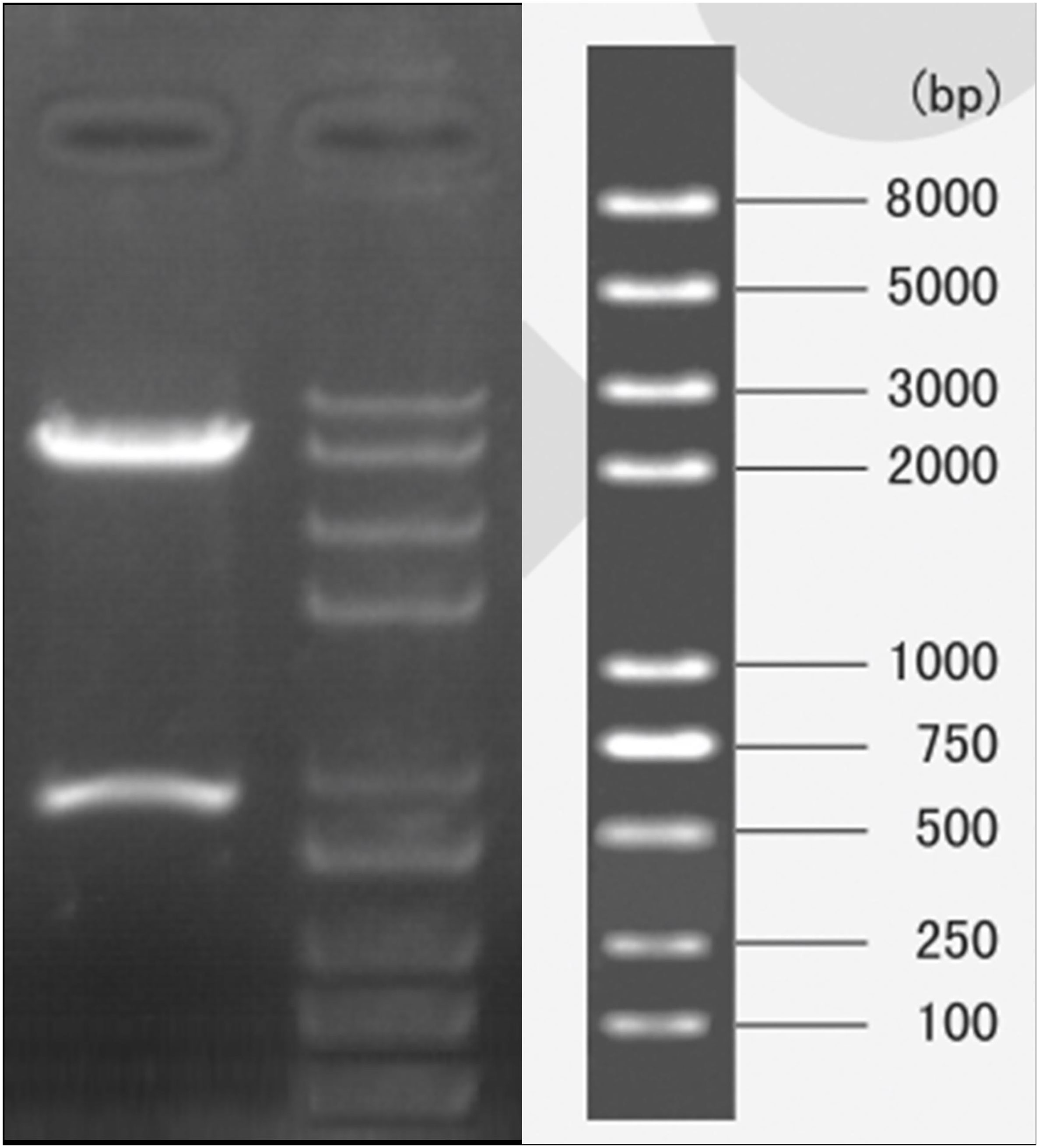
Figure 2 Gel electrophoresis of pYr-5HRE-cfosp-IFNG plasmid after double enzyme digested. Lane M, marker; lane 1, pYr-5HRE -cfosp-IFNG plasmid.
Furthermore, the sequencing results of the self-constructed pYr-5HRE-cfosp-IFNG plasmid were compared to the reference sequence, and the findings are presented in Figure 3. The complete sequence was accurate, indicating successfully synthesis of the pYr-5HRE-cfosp-IFNG plasmid.
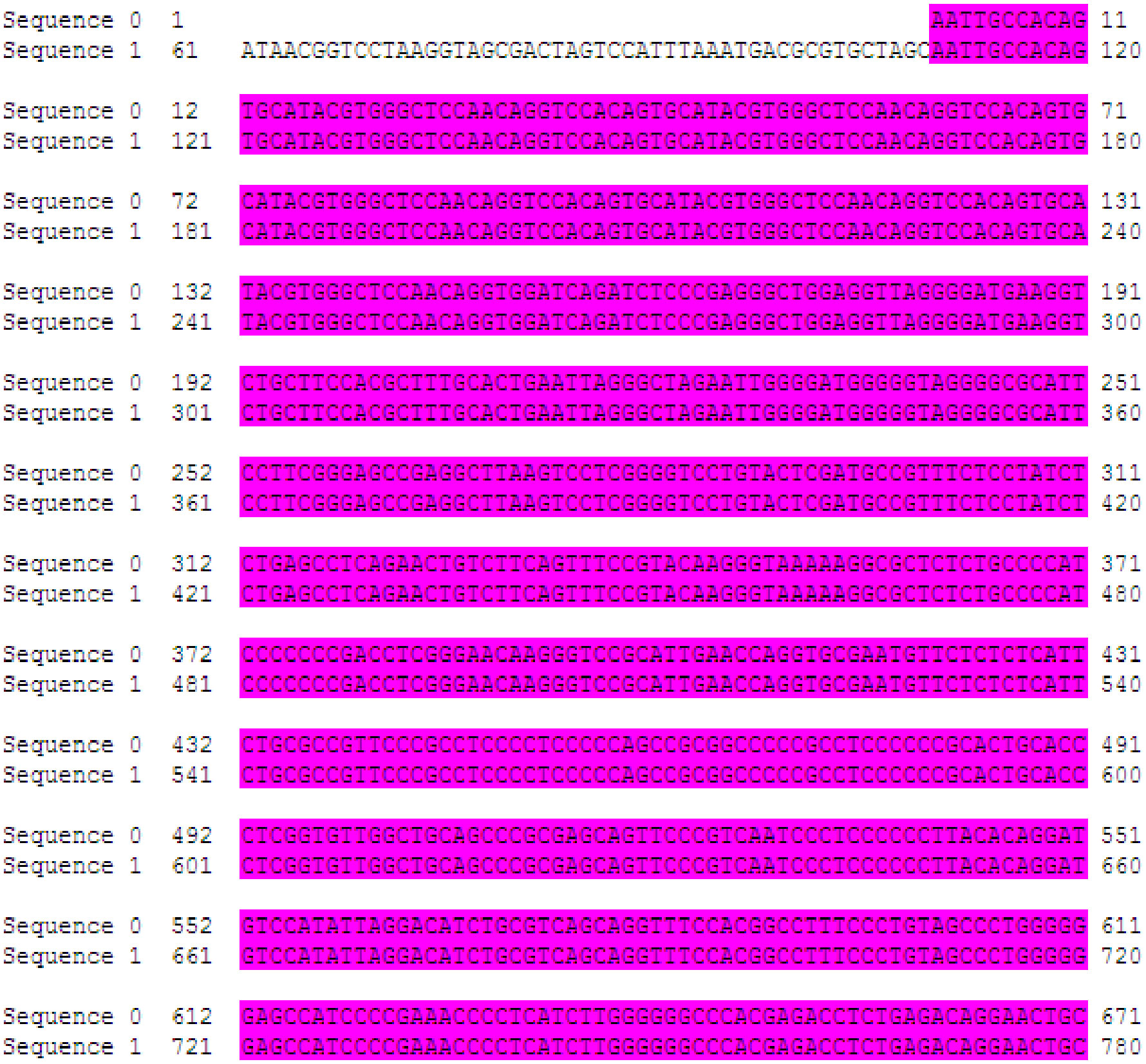
Figure 3 The sequencing results of pYr-5HRE-cfosp-IFNG. Sequence 0, template sequence; sequence 1, pYr-5HRE-cfosp-IFNG. Comparison software used was Dnassit 2.0 (Dnassit, Imola, BO, Italy).
Preparation and characterization of the Fe3O4 nanoparticles and PEI -Fe3O4 nanoparticles
According to transmission electron microscopy, the Fe3O4 nanoparticles display a high electron density with a diameter of approximately 10 nm. (Figure 4A) The hydrodynamic size of Fe3O4 nanoparticles was 149.7 nm with a polydispersity index (PdI) of 0.238 (Figure 4B).
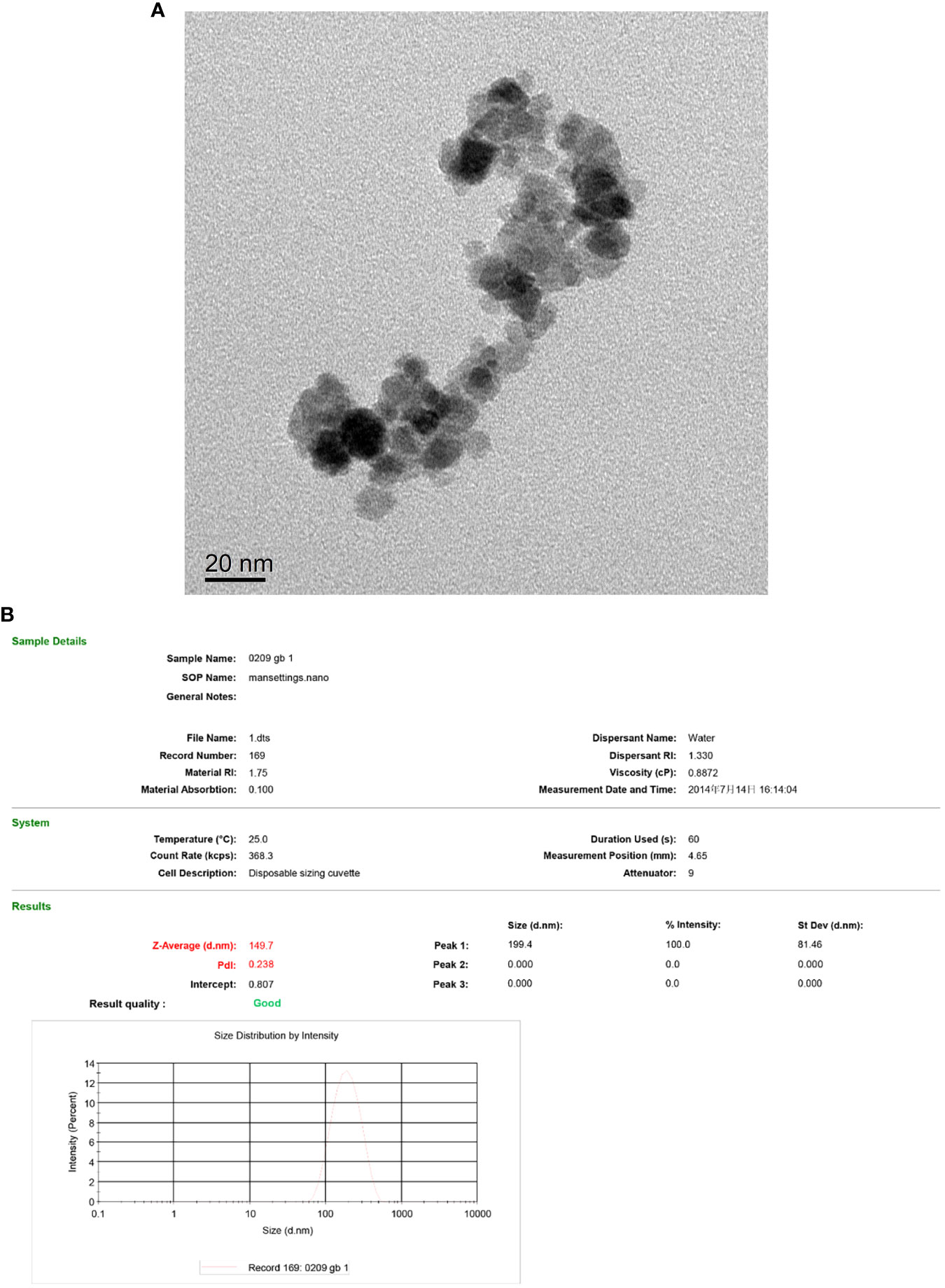
Figure 4 TEM of the Fe3O4 nanoparticles (A) and hydrodynamic diameter distribution of Fe3O4 nanoparticles (B).
The size of PEI-Fe3O4 nanoparticles under TEM was found to be similar to that of Fe3O4, which a diameter of approximately 10 nm (Figure 5). Furthermore, FTIR results revealed the presence of specific peaks at 3,414.9 cm-1, 2,805.2cm-1 and 1,456.8 cm-1, which is consistent with the unique chemical structure of PEI (Figure 6). The zeta potential test results indicated that the surface charge of Fe3O4 nanoparticles at pH 7 was 0 ± 0.7 mV, while the surface charge of PEI - Fe3O4 nanoparticles increased to 40.3 ± 1 mV (Figure 7C).
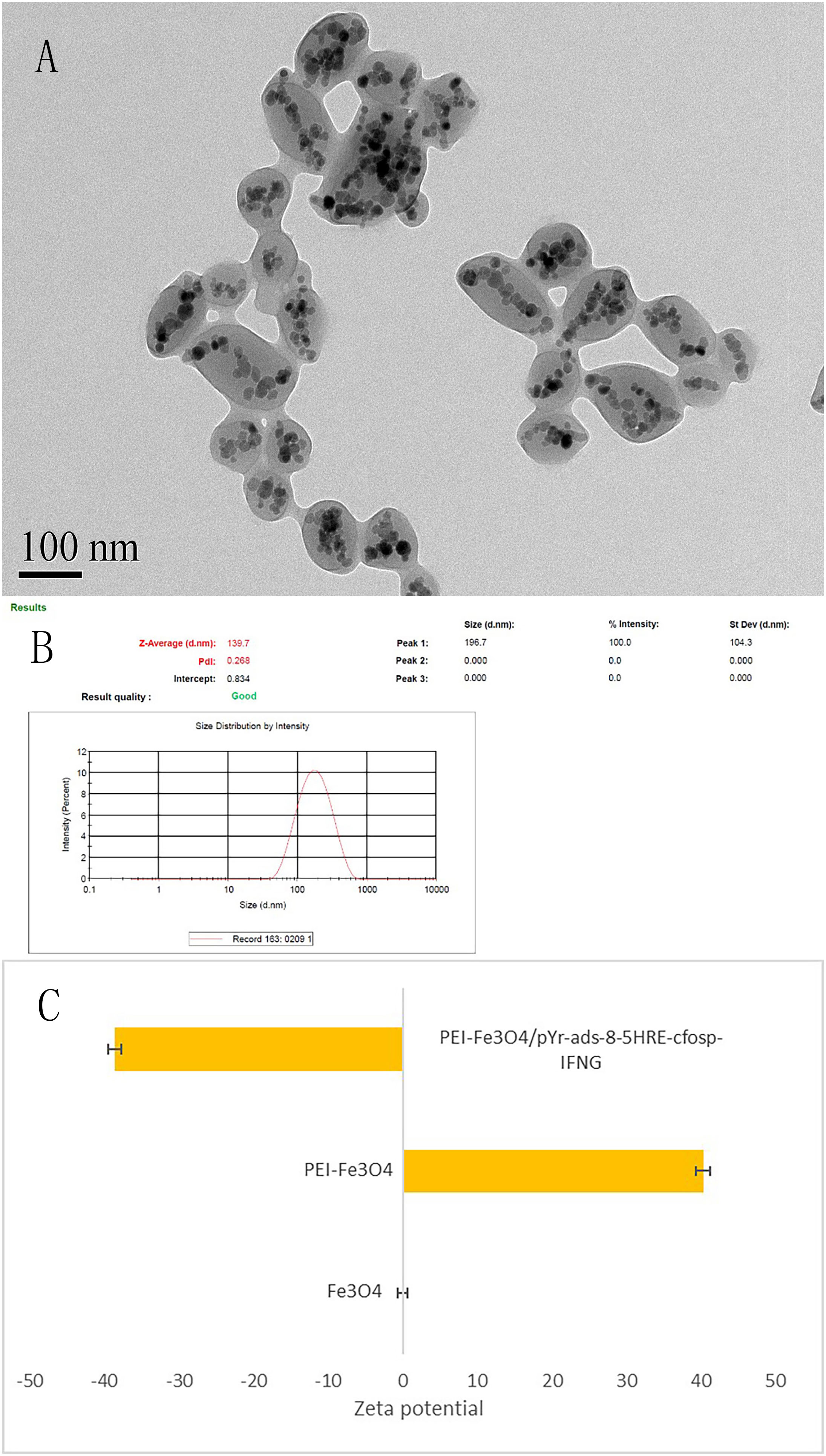
Figure 7 Characterization of PEI-Fe3O4/pYr-ads-8-5HRE-cfosp-IFNG albumin nanospheres. (A) TEM of PEI-Fe3O4/pYr-ads-8-5HRE-cfosp-IFNG albumin nanospheres. (B) Hydrodynamic diameter distribution of PEI-Fe3O4/pYr-ads-8-5HRE-cfosp-IFNG albumin nanospheres. (C) The zeta potential of Fe3O4, PEI-Fe3O4 and PEI-Fe3O4/pYr-ads-8-5HRE-cfosp-IFNG albumin nanospheres in pH7.
Preparation and characteristics of PEI-Fe3O4/pYr-ads-8-5HRE-cfosp-IFNG albumin nanospheres
TEM revealed that the self-prepared PEI-Fe3O4/pYr-ads-8-5HRE-cfosp-IFNG albumin nanospheres exhibit an approximately spherical or elliptical shape. The albumin nanospheres were utilized as encapsulating agents for Fe3O4 nanoparticles with high electron density (Figure 7A). The hydrodynamic size of the albumin nanospheres was 139.7 nm with a polydispersity index (PdI) of 0.268 (Figure 7B). Zeta potential test results indicated that the surface charge of the magnetic albumin nanospheres was -38.6 ± 0.9 mV.
The transcript of pYr-ads-8-5HRE-cfosp-IFNG in HepG2 cells was detected by quantitative PCR
The IFNG mRNA levels in HepG2 cells were detected by qPCR. Figure 8 presents the relative transcript levels of IFNG in each group.
(1) The negative control group;
(2) The PEI-Fe3O4 albumin nanospheres group;
(3) The PEI-Fe3O4/pDONR223-IFNG albumin nanospheres group;
(4) The PEI-Fe3O4/pYr-ads-8-cfosp-IFNG albumin nanospheres group;
(5) The PEI-Fe3O4/pYr-ads-8-5HRE-cfosp-IFNG albumin nanospheres group.
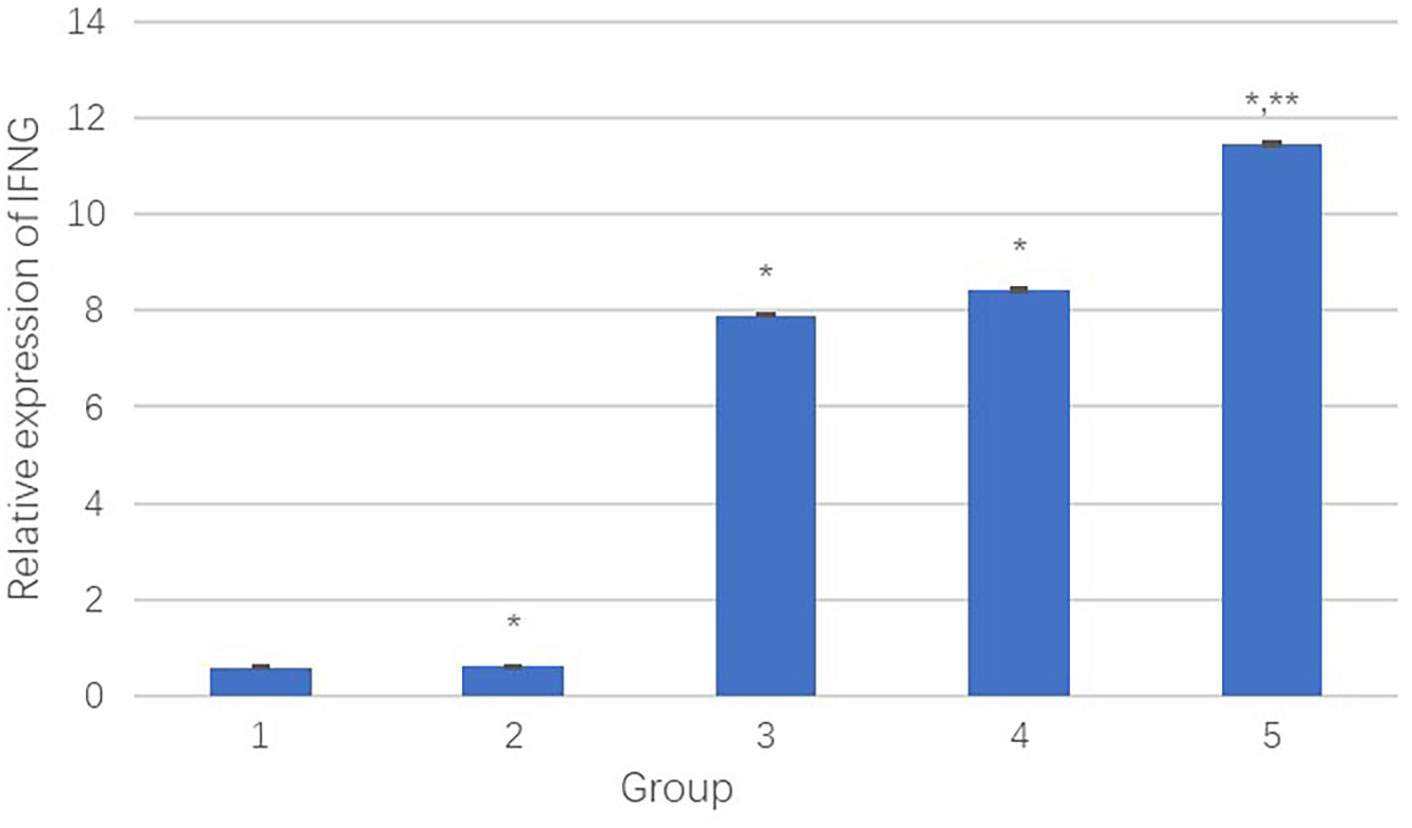
Figure 8 The relative transcript quantity of IFNγ. *Comparison between the experimental group and the negative control group, P<0.05; **Comparison between group 4 and group 5, P<0.05.
Results showed that compared to the negative control group, the PEI-Fe3O4/pDONR223-IFNG group, PEI-Fe3O4/pYr-ads-8-cfosp-IFNG group, and PEI-Fe3O4/pYr-ads-8-5HRE-cfosp-IFNG group had significantly higher IFNγ transcript, while the PEI-Fe3O4 group exhibited few IFNγ transcript. Moreover, the IFNγ transcript level in the PEI-Fe3O4/pYr-ads-8-5HRE-cfosp-IFNG group was higher than that in the PEI-Fe3O4/pYr-ads-8-cfosp-IFNG group after being treated with hypoxia (P<0.05).
Heating test of PEI-Fe3O4/pYr-ads-8-5HRE-cfosp-IFNG albumin nanospheres
Figure 9 shows the temperature rise curve of the PEI-Fe3O4/pYr-ads-8-5HRE-cfosp-IFNG albumin nanospheres at different concentrations. Upon exposed to an alternating magnetic field, the temperature of all concentrations of fluid increases quickly, and remains constant at a certain temperature. The maximum temperature that can be reached is higher for higher concentrations of magnetic fluid. However, the heating rate of solutions with different concentrations is not significantly different. Within the first 5 minutes of heating, the magnetic fluid rapidly heat up, reaching the highest temperature in about 35 minutes, and then maintaining at this temperature. The maximum temperature of 0.25 mg/mL solution is stable at about 44°C, which is suitable for tumor thermal therapy without damaging normal tissues. Therefore, a concentration of 0.25 mg/mL was deemed suitable for the subsequent MFH experiment.
The therapeutic effect of PEI-Fe3O4/pYr-ads-8-5HRE-cfosp-IFNG albumin nanospheres on hepatoma
CCK8 assay
Figure 10 presents the results of the relative inhibition rate of cell proliferation. The difference in relative inhibition rate between the experimental groups and the negative control group was statistically significant. (P<0.05). Table 1 and Figure 10 show the relative inhibition rate of each group at 24h, 48h, and 72h. It can be observed that the combination treatment (Group 1) exhibited the highest relative inhibition rate. Radiation-alone slightly inhibited the growth of HepG2 cells. Both MFH and radiation-gene therapy can significantly restrain the growth of HepG2 cells, but the relative inhibition rate was still lower than that of the combined therapy.
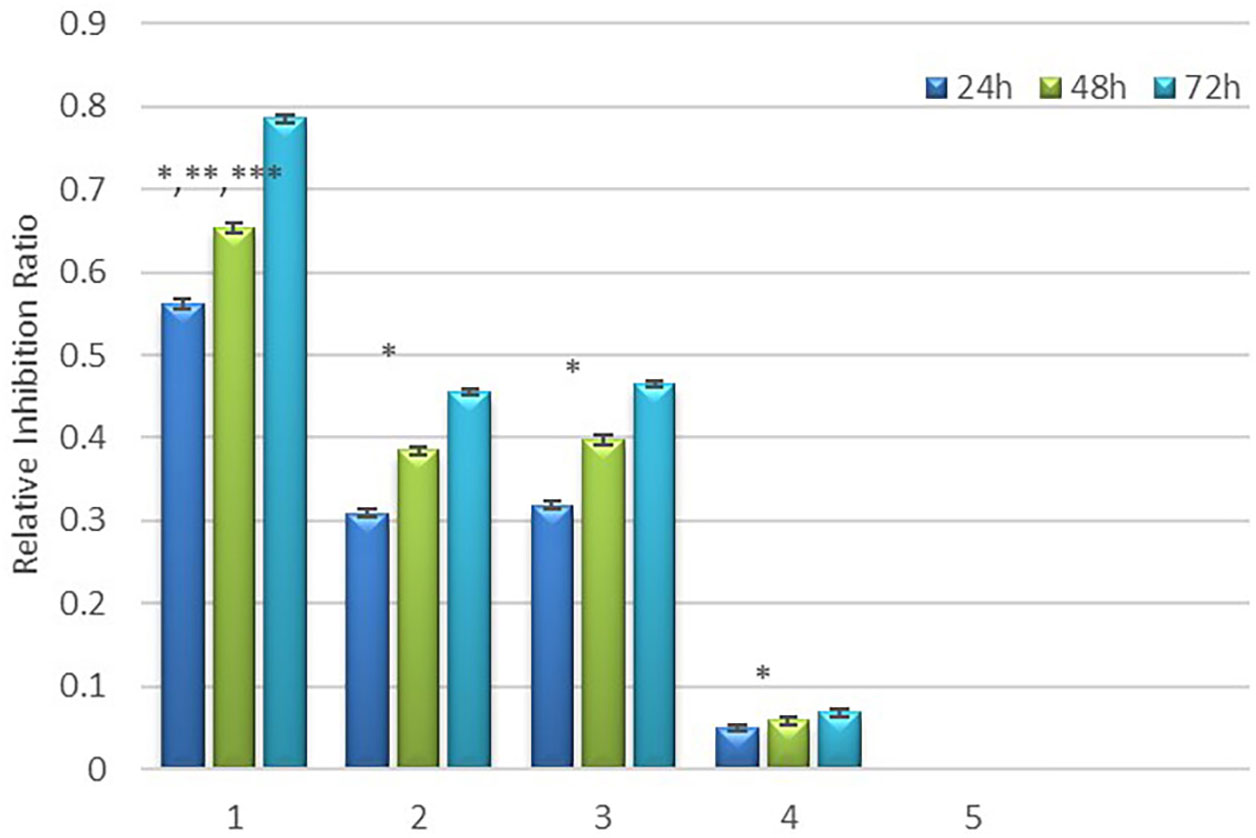
Figure 10 Relative inhibition rate in each group. * Comparison between the experimental group and the negative control group, P<0.05; ** Compared to group 4, P<0.05, *** Compared to group 2 and 3, P<0.05.
Flow cytometry assay
Flow cytometry was employed to analyze whether cell death was due to necrosis or apoptosis. Our results showed different degrees of apoptosis and necrosis in each experimental group (Figure 11). In the combination treatment group, the apoptotic rate and necrotic rate were 42.32% and 35.73%, respectively, indicating that the cell death was mainly due to apoptosis. The apoptotic rate and necrotic rate in the radiation-gene therapy group were 20.40% and19.79%, respectively. The MFH group had apoptotic and necrotic of 20.24% and 24.22%, respectively. The radiation alone group had the lowest rates of apoptotic rate and necrotic cells, at 5.42% and 10.53%, respectively. The combination of radiation-gene therapy and MFH exhibited better efficacy than any other group.
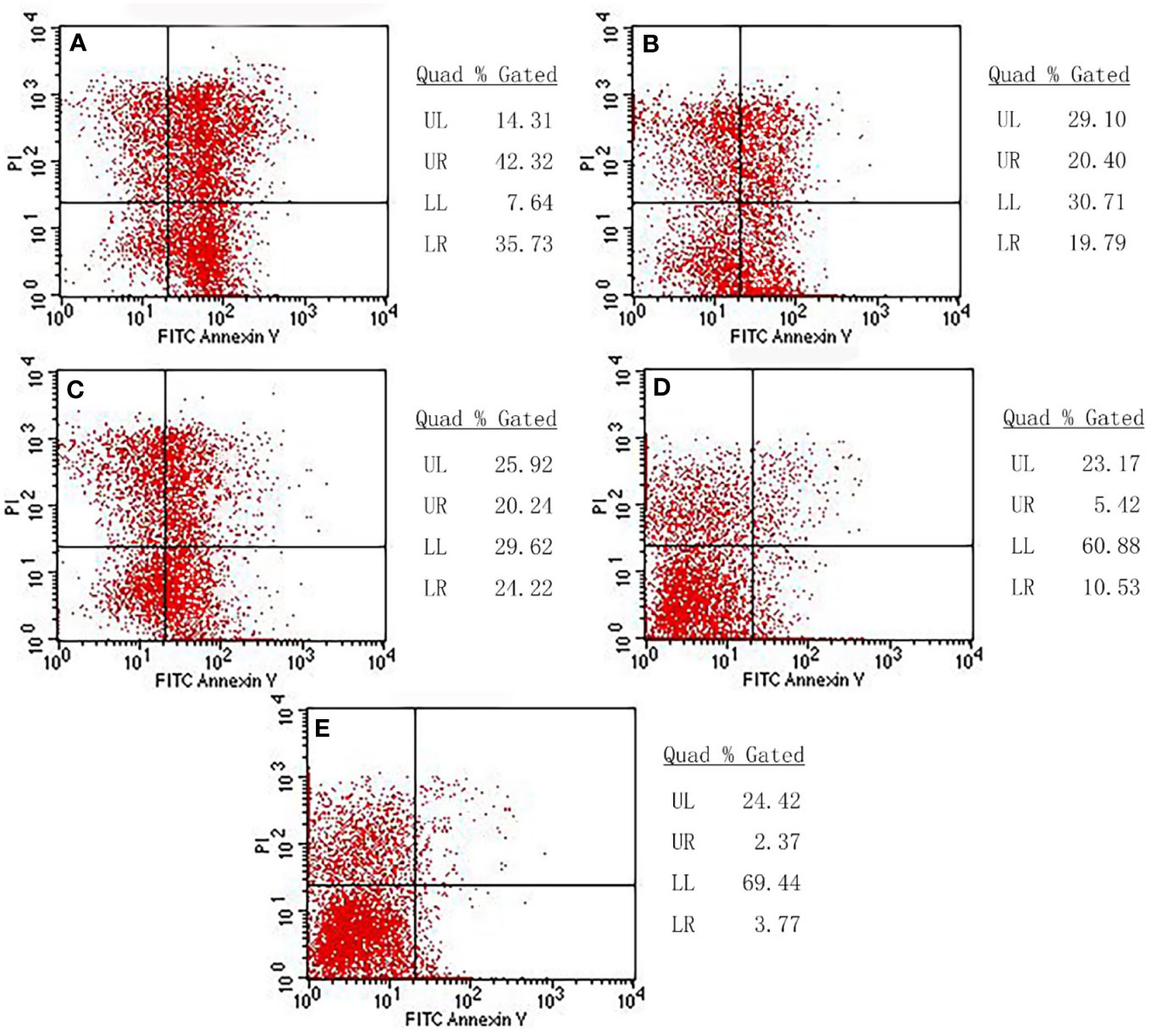
Figure 11 The apoptotic rate and necrotic rate of each group. (A) Radiation-gene therapy and MFH combination group (B) Radiation-gene therapy group; (C) MFH group; (D) Radiation alone group; (E) Negative control group.
Intracellular analysis of HepG2 cells treated with Fe3O4 nanoparticles by TEM
Fe3O4 nanoparticles successfully internalized by HepG2 cells, as shown in Figure 12. The majority of the nanoparticles were localized in lysosomes.
Animal experiments (in vivo experiments of combination therapy)
The tumor mass and volume inhibition rates of the radiation-gene therapy and MFH combination group were 66.67% and72.53%, respectively. which were significantly higher than those of the other groups (Table 2). The combination treatment of radiation-gene therapy and MFH showed a significant inhibitory effect on tumor growth. Radiation alone, MFH alone, and radiation-gene therapy alone also showed inhibitory effects on tumor growth, but the inhibition rates were weaker than those of the combination treatment group. However, there was no significant change in the tumor mass and volume inhibition rate in the radiation alone group, which was not statistically significant when compared with the negative control group.
Long-term safety of Fe3O4 magnetic nanoparticles
During drug treatment, the potential toxic side effects of anti-tumor nanoparticles are important considerations for further clinical application. To assess the biosafety of our treatment, six weeks after treatment. The mice were investigated for potential side effects before they were sacrificed. Clinical biochemistry analysis, including platelet (PLT), red blood cell (RBC), white blood cell (WBC), hemoglobin (HGB), creatinine (CREA), alkaline phosphatase (ALP), triglycerides (TGs), total cholesterol (TC), high-density lipoprotein (HDL), low-density lipoprotein (LDL) and blood urea nitrogen (UREA), were performed (Figure 13). The results showed that these indexes did not significantly change during the treatment, indicating that the liver and kidney functions of nude mice were not impaired by the treatment. Moreover, no obvious pathological injury or inflammatory lesions were found in the tissue sections of the heart, lung, spleen and kidney of the five groups of nude mice, further indicating the biosafety of Fe3O4 magnetic nanoparticles.
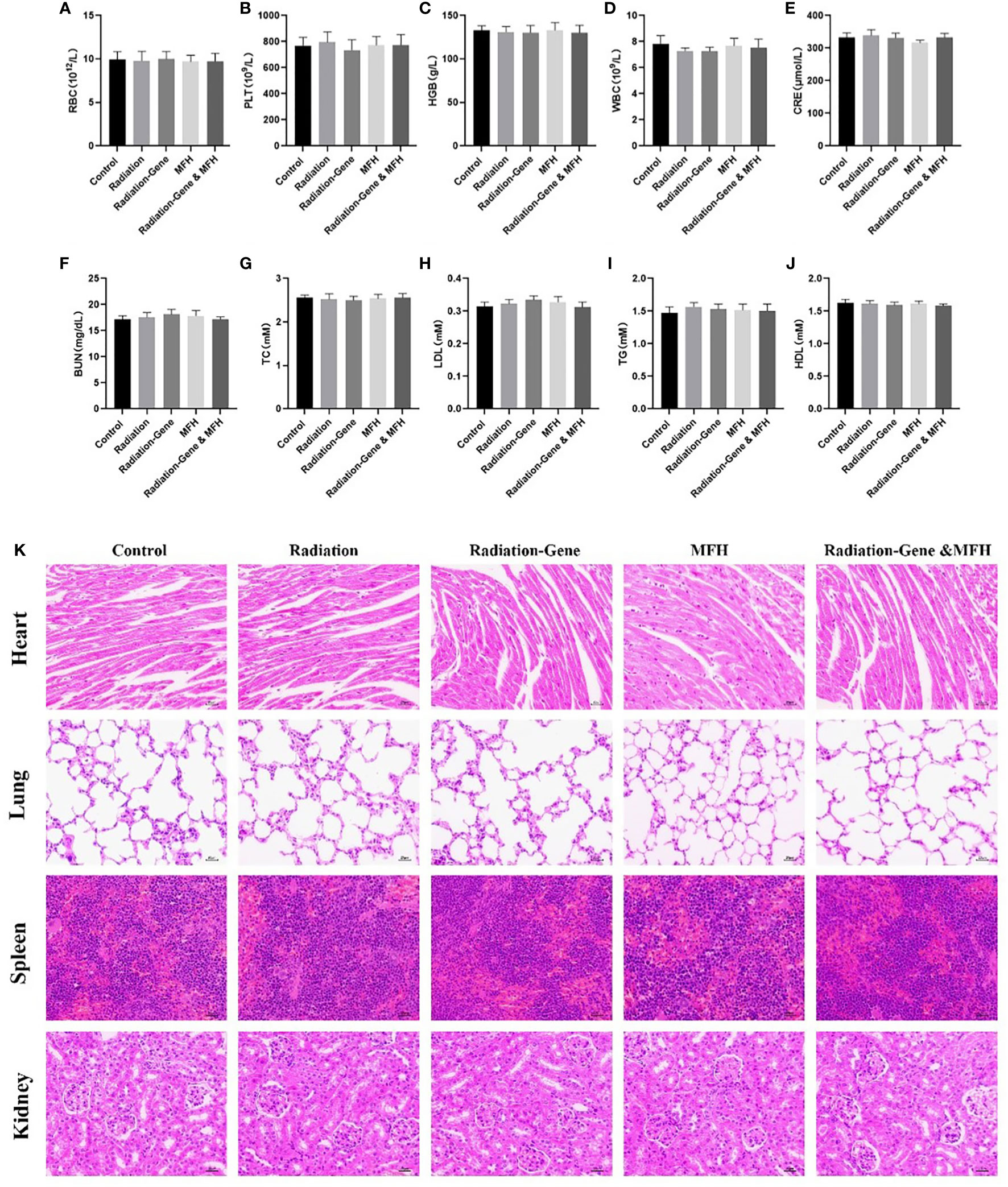
Figure 13 Long term safety of nanoparticle after treatment with different formulations. (A–J) RBC, PLT, HGB, WBC, CRE, BUN, TC, LDL, TG, HDL. (K) Histological evaluation of organs (heart, lung, spleen and kidney) from HCC mice two months post treatments.
Discussion
Cancer remains one of the most severe diseases facing humankind. As far as current medical knowledge goes, there is no single treatment capable of curing cancer. Recent studies have shown that multi-mode therapy is often more effective than single-mode therapy in inhibiting tumors (39–41). In our study, we investigated the combined treatment of immunogene therapy and magnetic fluid hyperthermia using Fe3O4 nanoparticles as the medium on cancer, which demonstrated strong synergies.
Hyperthermia is a common adjuvant treatment for cancer and is used in various cancers. Increasing the temperature of tumor tissue to 40-43 degrees Celsius can effectively induce tumor cell death (42). The hyperthermia of tumor with nano-magnetic fluid involves directly inject MNPs into malignant tumor and heating them in AMF. This method has the advantages of accuracy, targeting, and temperature control and avoids overheating the normal tissues around the tumor (43). To ensure that the liquid is dispersed in the tumor tissues and prevent overflow of the liquid, we adopted a multi-point injection strategy, injecting the magnetic nano-composite materials into the 3rd, 6th, 9th, and 12th points of each tumor. Tumors are more sensitive to heating than normal tissues because of their unique internal microenvironment, such as hypoxia, vascular disorder, acidosis, and hypoperfusion (44). Therefore, hyperthermia is a promising treatment for tumors (45, 46). In our research, the tumor inhibition rate of the MFH group was the second highest, confirming the great potential of MFH in the treatment of solid tumors.
In our study, the tumor inhibition rate was significantly increased when MFH was combined with immunogene therapy. However, the mechanism underlying the synergistic effect of the combination therapy has not been investigated in this study. The following possibilities are speculated: hyperthermia in combination therapy of tumors was considered to influence the susceptibility to other manipulations mostly through microenvironmental factors (47). High temperature can destroy the biological integrity of the cell membrane and increase its permeability, which is conducive to the penetration and absorption of chemical drugs and gene transfer. The interior of solid tumors is often anoxic due to vascular disorder, while the anoxic cells are usually acidic and nutrient-poor, making them more vulnerable to heat damage. However, high temperature can temporarily improve the oxygen and blood supply to the tumor area, helping drugs to enter the tumor and improving the radiation susceptivity of tumor cells (48). Our next research goal is to explore the molecular mechanism of hyperthermia combined with immunogene therapy for hepatoma and to elucidate why combined therapy is superior to single therapy. This will guide the clinical selection of a more targeted and individualized treatment plan.
MNPs can not only be used as the medium of magnetic hyperthermia, but also as gene carriers in this study. Nanomaterials are used in many areas of biomedicine, including drug/gene therapy, diagnostic imaging (as contrast agents), and biological scaffolds. This is due to the advantages of nanomaterials such as high biosafety, complex surface functionalization, and easy preparation (49). MNPs have been used as gene vectors due to their good biocompatibility and large specific surface area, especially their ability to magnetically target to target tissues, which plays an important role in gene transfer (50, 51). Since DNA carries a negative charge, we connect PEI on the surface of Fe3O4-MNPs. PEI carries a positive charge and can bind to negatively charged DNA molecules. Thus, the plasmid can be effectively carried into HepG2 cells. Figure 6 shows specific peaks at 3,414.9 cm-1, 2,805.2cm-1 and 1,456.8 cm-1, which are consistent with the special chemical structure of PEI. This indicates that PEI has successfully modified the surface of nano Fe3O4. Magnetic nanoparticles are easy to agglomerate because of their small particle size. Therefore, in this study, the particle size of MNPs measured by TEM is smaller than that detected by Zetasizer Nano. The Zetasizer Nano measurement shows the hydrodynamic size of the MNPs. However, when we coated the magnetic nanoparticles with albumin, the diameter of the nanoparticles observed by TEM was not very different from that measured by DLS. MNPs modified with albumin have better dispersion. More importantly, albumin is a substance already in the human body. Wrapping magnetic nanomaterials in albumin can improve the safety of nanomaterials and effectively avoid the removal of foreign substances by the body (52, 53).
Using nano-magnetic materials as a carrier, we combined MFH and radiation-induced immunogene therapy to explore a new therapy model for hepatoma. In our research, in addition to the combined treatment group, an experimental group was also set up for each single treatment method for comparison. The consequences of our study showed that the tumor inhibition rate of combination therapy was better than that of single therapy in both cell and animal experiments. As mentioned above, we still have a lot of work to do on the molecular mechanisms. Understanding the deeper issues is helpful in guiding the formulation and selection of clinical treatment. However, this study still has high reference value and research significance in exploring the comprehensive treatment of hepatoma.
Conclusion
The results presented in this study demonstrate the successful synthesis and preparation of PEI-Fe3O4/pYr-ads-8-5HRE-cfosp-IFNG albumin nanospheres for targeted delivery of IFNG to hepatoma cells. The identification of pYr-ads-8-5HRE-cfosp-IFNG confirmed the successful synthesis of the plasmid. The characterization of Fe3O4 nanoparticles and PEI-Fe3O4 nanoparticles indicated their appropriate size and surface charge for subsequent experiments. The transcript levels of IFNG in HepG2 cells were detected by qPCR, and the results showed that the IFNG transcript levels in the PEI-Fe3O4/pYr-ads-8-5HRE-cfosp-IFNG group were higher than those in the PEI-Fe3O4/pYr-ads-8-cfosp-IFNG group after hypoxia treatment, suggesting that the addition of the HRE promoter enhanced the therapeutic effect of IFNG in hypoxic conditions. The results of in vitro and in vivo experiments showed that the relative inhibition rate of cell proliferation was significantly higher in the PEI-Fe3O4/pYr-ads-8-5HRE-cfosp-IFNG albumin nanospheres group compared to the other groups, including the negative control group and the PEI-Fe3O4/pDONR223-IFNG group. The results suggested that the targeted delivery of IFNG by the PEI-Fe3O4/pYr-ads-8-5HRE-cfosp-IFNG albumin nanospheres was effective in inhibiting the proliferation of hepatoma cells. These findings suggest the potential of using targeted delivery of IFNG by nanocarriers for the treatment of hepatoma.
Data availability statement
The original contributions presented in the study are included in the article/supplementary material. Further inquiries can be directed to the corresponding author.
Ethics statement
The animal study was reviewed and approved by Ethics Committee of North Sichuan Medical College.
Author contributions
HZ: performed experiments, analyzed data, interpreted results of experiments, drafted manuscript, edited and revised manuscript; SL: analyzed data, approved final version of manuscript; FC: performed experiments, analyzed data; XM: approved final version of manuscript, analyzed data; ML: conceived and designed research. All authors contributed to the article and approved the submitted version.
Funding
This project was support by the Natural Science Foundation for Young Scholars of Sichuan Province (2022NSFSC1587) and Doctoral research Foundation of North Sichuan Medical College (CBY22-QDA14).
Conflict of interest
The authors declare that the research was conducted in the absence of any commercial or financial relationships that could be construed as a potential conflict of interest.
Publisher’s note
All claims expressed in this article are solely those of the authors and do not necessarily represent those of their affiliated organizations, or those of the publisher, the editors and the reviewers. Any product that may be evaluated in this article, or claim that may be made by its manufacturer, is not guaranteed or endorsed by the publisher.
References
1. Ma HY, Ao YK, Liu WB, Cao GW. A bibliometric analysis on cohort study of liver cancer in China. Zhonghua Liu Xing Bing Xue Za Zhi (2020) 41(1):115–9. doi: 10.3760/cma.j.issn.0254-6450.2020.01.021
2. Tang R, Xu Z. Gene therapy: A double-edged sword with great powers. Mol Cell Biochem (2020) 474(1-2):73–81. doi: 10.1007/s11010-020-03834-3
3. Tecalco-Cruz AC, Cortés-González CC, Cruz-Ramos E, Ramírez Jarquín J O, Romero-Mandujano A K, Sosa-Garrocho M, et al. Interplay between interferon-stimulated gene 15/ISGylation and interferon gamma signaling in breast cancer cells. Cell Signalling (2019) 54:91–101. doi: 10.1016/j.cellsig.2018.11.021
4. Zaidi MR. The interferon-gamma paradox in cancer. J Interferon Cytokine Res (2014) 39(1):30–38. doi: 10.1089/jir.2018.0087
5. Han P, Chandrasekhar G, Yu H, Wang E. Gene regulatory network rewiring in the immune cells associated with cancer. (2017) 8:308. doi: 10.3390/genes8110308
6. Khalifa J, Franois S, Rancoule C, Riccobono D, Magné N, Drouet M, et al. Gene therapy and cell therapy for the management of radiation damages to healthy tissues: Rationale and early results. Cancer/Radiothérapie (2019) 23:449–65. doi: 10.1016/j.canrad.2019.06.002
7. Kai Z, Yang S, Geng J, Gong X, Gong W M, Shen L, et al. Combination of anginex gene therapy and radiation decelerates the growth and pulmonary metastasis of human osteosarcoma xenografts. Cancer Med (2018) 7(6):2518–29. doi: 10.1002/cam4.1476
8. Wu C, Du X, Jia B, Zhang CM, Li WF, Liu TC, et al. A transformable gold nanocluster aggregate-based synergistic strategy for potentiated radiation/gene cancer therapy. J Materials Chem B (RSC Publishing) (2021) 9:2314–22. doi: 10.1039/D0TB02986F
9. Slemc L, Kunej T. Transcription factor HIF1A: downstream targets, associated pathways, polymorphic hypoxia response element (HRE) sites, and initiative for standardization of reporting in scientific literature. Tumor Biol (2016) 37:14851–61. doi: 10.1007/s13277-016-5331-4
10. Cui XY, Skretting G, Tinholt M, Stavik B, Dahm AEA, Sahlberg KKS, et al. A novel hypoxia response element regulates oxygen-related repression of tissue factor pathway inhibitor in the breast cancer cell line MCF-7. Thromb Res (2017) 157:111–6. doi: 10.1016/j.thromres.2017.07.013
11. Shibata T, Giaccia AJ, Brown JM. Development of a hypoxia-responsive vector for tumor-specific gene therapy. Gene Ther (2000) 7:403–98. doi: 10.1038/sj.gt.3301124
12. Zu H, Gao D. Non-viral vectors in gene therapy: Recent development, challenges, and prospects. AAPS J (2021) 23:1–12. doi: 10.1208/s12248-021-00608-7
13. Jafari SS, Shafighi M, Beltraminelli H, Geiser T, Hunger RE, Gazdhar A, et al. Improvement of flap necrosis in a rat random skin flap model by In vivo electroporation-mediated HGF gene transfer. Plast Reconstructive Surg (2017) 139:1116e. doi: 10.1097/PRS.0000000000003259
14. Cheng FY, Chan CH, Wang BJ, Yeh YL, Wang YJ, Chiu HW, et al. The oxygen-generating calcium peroxide-modified magnetic nanoparticles attenuate hypoxia-induced chemoresistance in triple-negative breast cancer. Cancers (2021) 13:606. doi: 10.3390/cancers13040606
15. Liang N, Liu L, Li P, Xu Y, Hou Y, Peng , et al. Efficient isolation and quantification of circulating tumor cells in non-small cell lung cancer patients using peptide-functionalized magnetic nanoparticles. J Thorac Oncol (2021) 16:S483. doi: 10.21037/jtd-20-1026A
16. Shan X, Yu W, Ni X, Xu T, Lei C, Liu Z, et al. Effect of chitosan magnetic nanoparticles loaded with Ang2-siRNA plasmids on the growth of melanoma xenografts in nude mice. Cancer Manage Res (2020) 12:7475–85. doi: 10.2147/CMAR.S250479
17. Chekhonin VP, Abakumov MA, Mazhuga AG, Bagdinova AN, Demikhov EI, Demikhov TE, et al. Relaxation properties of contrast media for MRI based on iron oxide nanoparticles in different magnetic fields. Bull Exp Biol Med (2019) 167:97–9. doi: 10.1007/s10517-019-04469-1
18. Chouhan RS, Horvat M, Ahmed J, Alhokbany N, Alshehri SM, Gandhi S, et al. Magnetic nanoparticles-a multifunctional potential agent for diagnosis and therapy. Cancers (2021) 13:2213. doi: 10.3390/cancers13092213
19. Nam KC, Han YS, Lee JM, Kim SC, Cho G, Park BJ, et al. Photo-functionalized magnetic nanoparticles as a nanocarrier of photodynamic anticancer agent for biomedical theragnostics. Cancers (2020) 12:571. doi: 10.3390/cancers12030571
20. Roeth AA, Slabu I, Kessler A, Engelmann U, Baumann M, Schmitz-Rode T, et al. Local treatment of pancreatic cancer with magnetic nanoparticles. HPB (2019) 21:S868–9. doi: 10.1016/j.hpb.2019.10.959
21. Wang X, Yu B, Wu Y, Wu Y, Lee RJ, Lee LJ, et al. Efficient downregulation of CDK4 by novel lipid nanoparticle-mediated siRNA delivery. Anticancer Res (2011) 31:1619–26.
22. Shundo C, Zhang H, Nakanishi T, Osaka T. Cytotoxicity evaluation of magnetite (Fe3O4) nanoparticles in mouse embryonic stem cells. Colloids Surfaces B (2012) 97:221–5. doi: 10.1016/j.colsurfb.2012.04.003
23. Asimeng BO, Nyankson E, Efavi JK, Amarkai AN, Manu GP, Tiburu E. Characterization and inhibitory effects of magnetic iron oxide nanoparticles synthesized from plant extracts on HeLa cells. Int J Biomaterials (2020) 11:1–11. doi: 10.1155/2020/2630735
24. Zhang T, Li G, Miao Y, Lu JJ, Gong NQ, Zhang YF, et al. Magnetothermal regulation of in vivo protein corona formation on magnetic nanoparticles for improved cancer nanotherapy. Biomaterials (2021) 276:121021. doi: 10.1016/j.biomaterials.2021.121021
25. Lazaro-Carrillo A, Calero M, Aires A, Cortajarena AL, Simões BM, Latorre A, et al. Tailored functionalized magnetic nanoparticles to target breast cancer cells including cancer stem-like cells. Cancers (2020) 12:1397. doi: 10.3390/cancers12061397
26. Zhao S. Tmod-16. multifunctional nanoparticles used for magnetic Resonance/Nir fluorescence dual-modal imaging and chemotherapy of glioma. Neuro Oncol (2019) 6:vi266. doi: 10.1093/neuonc/noz175.1115
27. Jordan A, Wust P, Scholz R, Tesche B, Fahling H, Mitrovics T, et al. Cellular uptake of magnetic fiuid particles and their effects on human adeno carcinoma cells exposed to AC magnetic fields in vitro, int. J. Hyperthermia (1996) 12:705–22. doi: 10.3109/02656739609027678
28. Dahaghin A, Emadiyanrazavi S, Haghpanahi M, Salimibani M, Bahreinizad H, Eivazzadeh-Keihan R, et al. A comparative study on the effects of increase in injection sites on the magnetic nanoparticles hyperthermia. Journal of Drug Delivery Science and Technology (2021) 63:102542. doi: 10.1016/j.jddst.2021.102542
29. Liu D, Hong Y, Li Y, Hu C, Yip TC, Yu WK, et al. Targeted destruction of cancer stem cells using multifunctional magnetic nanoparticles that enable combined hyperthermia and chemotherapy. Theranostics (2020) 10:1181–96. doi: 10.7150/thno.38989
30. Zhang H, Hou X, Lin M, Wang L, Li HB, Yuan CY, et al. The study on the preparation and characterization of gene-loaded immunomagnetic albumin nanospheres and their anti-cell proliferative effect combined with magnetic fluid hyperthermia on GLC-82 cells. Drug Des Devel Ther (2015) 9:6445–60. doi: 10.2147/DDDT.S93481
31. Hornok V. Serum albumin nanoparticles: Problems and prospects. Polymers (2021) 13:3759. doi: 10.3390/polym13213759
32. Hemlata, Gupta S, Tejavath KK. ROS-mediated apoptosis induced by BSA nanospheres encapsulated with fruit extract of cucumis prophetarum in various human cancer cell lines. ACS omega (2021) 6:10383–95. doi: 10.1021/acsomega.1c00755
33. Lotfalian S, Nematollahzadeh A, Ghasemi S. Hierarchically structured protein-based hollow-nanospheres for drug delivery. Reactive Funct Polymers (2021) 160:104821. doi: 10.1016/j.reactfunctpolym.2021.104821
34. Samimifar M. Spectrophotometric determination of cyanide in aqueous samples after its conversion to thiocyanate and complexation to ferric-thiocyanate. J Appl Res Water Wastewater (2019) 6:126–30. doi: 10.22126/arww.2019.1410
35. Raouf I, Khalid S, Khan A, Lee J, Kim HS, Kim MH, et al. A review on numerical modeling for magnetic nanoparticle hyperthermia: Progress and challenges. J Thermal Biol (2020) 91:102644. doi: 10.1016/j.jtherbio.2020.102644
36. Saber S, Nasr M, Saad AS, Mourad AAE, Gobba NA, Shata A, et al. Albendazole-loaded cubosomes interrupt the ERK1/2-HIF-1α-p300/CREB axis in mice intoxicated with diethylnitrosamine: A new paradigm in drug repurposing for the inhibition of hepatocellular carcinoma progression. Biomedicine Pharmacotherapy (2021) 142:112029. doi: 10.1016/j.biopha.2021.112029
37. Cao Y, Wang J, Tian H, Fu GH. Mitochondrial ROS accumulation inhibiting JAK2/STAT3 pathway is a critical modulator of CYT997-induced autophagy and apoptosis in gastric cancer. J Exp Clin Cancer Res (2020) 39:1–15. doi: 10.1186/s13046-020-01621-y
38. Tian H, Zhang M, Jin GX, Jiang Y, Luan Y X. Cu-MOF chemodynamic nanoplatform via modulating glutathione and H2O2 in tumor microenvironment for amplified cancer therapy. J Colloid Interface Sci (2021) 587:358–66. doi: 10.1016/j.jcis.2020.12.028
39. Han Y, Ma R, Cao G, Liu H, He LL, Tang L, et al. Combined treatment of cinobufotalin and gefitinib exhibits potent efficacy against lung cancer. Evidence-Based Complementary Altern Med (2021) 2021:1–9. doi: 10.1155/2021/7782963
40. Petrenko M, Güttler A, Funtan A, Kebler J, Emmerich D, Paschke R, et al. Combined 3-o-acetylbetulin treatment and carbonic anhydrase IX inhibition results in additive effects on human breast cancer cells. Chemico-Biological Interact (2021) 333:109326. doi: 10.1016/j.cbi.2020.109326
41. Li C, Tan L, Zhou W. Meta-analysis of clinical efficacy of metformin combined with chemotherapy in the treatment of ovarian cancer. J Clin Med Res (2022) 3:25–30. doi: 10.32629/jcmr.v3i1.709
42. Wust P, Hildebrandt B, Sreenivasa G, Rau B, Gellermann J, Riess H, et al. Hyperthermia in combined treatment of cancer. Lancet Oncol (2002) 3:487–97. doi: 10.1016/S1470-2045(02)00818-5
43. Johannsen M, Thiesen B, Wust P, Jordan A. Magnetic nanoparticle hyperthermia for prostate cancer. Int J Hyperthermia (2010) 26:790–5. doi: 10.3109/02656731003745740
44. Lu Y, Rivera-Rodriguez A, Tay ZW, Hensley D, Fung K, Colson C, et al. Combining magnetic particle imaging and magnetic fluid hyperthermia for localized and image-guided treatment. Int J Hyperthermia (2020) 37:141–54. doi: 10.1080/02656736.2020.1853252
45. Westermann A, Mella O, van der Zee J, Jones EL, Van Der Steen-Bannasik E, Koper P, et al. Long-term survival data of triple modality treatment of stage IIB–III–IVA cervical cancer with the combination of radiotherapy, chemotherapy and hyperthermia–an update. Int J Hyperthermia (2012) 28:549–53. doi: 10.3109/02656736.2012.673047
46. Vaupel PW, Kelleher DK. Blood flow and associated pathophysiology of uterine cervix cancers: characterisation and relevance for localised hyperthermia. Int J Hyperthermia (2012) 28:518–27. doi: 10.3109/02656736.2012.699134
47. Shen L, Gao B, He K, Xiao WH. Apoptosis of hepatoma cell line HepG2 induced by the combination use of GNRs@ SiO2-FA and 125I seeds: an experimental study. J Interventional Radiol (2015), 236–41.
48. Mortezaee K, Narmani A, Salehi M, Bagheri H, Farhood B, Haghi-Aminjan H, et al. Synergic effects of nanoparticles-mediated hyperthermia in radiotherapy/chemotherapy of cancer. Life Sci (2021) 269:119020. doi: 10.1016/j.lfs.2021.119020
49. Li RN, Da XH, Li X, Lu YS, Gu FF, Liu Y, et al. Functionalized magnetic nanoparticles for drug delivery in tumor therapy*. Chin Phys B (2021) 30:017502. doi: 10.1088/1674-1056/abb3e6
50. Amani A, Alizadeh MR, Yaghoubi H, Ebrahimi HA. Design and fabrication of novel multi-targeted magnetic nanoparticles for gene delivery to breast cancer cells. J Drug Delivery Sci Technol (2021) 61:102151. doi: 10.1016/j.jddst.2020.102151
51. Bi Q, Song X, Hu A, Luo TY, Jin RR, Ai H, et al. Magnetofection: Magic magnetic nanoparticles for efficient gene delivery. Chin Chem Lett (2020) 31:3041–6. doi: 10.1016/j.cclet.2020.07.030
52. Hasanpoor Z, Mostafaie A, Nikokar I, Hassan ZM. Curcumin-human serum albumin nanoparticles decorated with PDL1 binding peptide for targeting PDL1-expressing breast cancer cells. Int J Biol Macromolecules (2020) 159:137–53. doi: 10.1016/j.ijbiomac.2020.04.130
Keywords: hyperthermia, combination therapy, magnetic nanoparticles, nano-drug, gene circuit
Citation: Zhang H, Li S, Chen F, Ma X and Liu M (2023) The therapeutic effect of PEI-Fe3O4/pYr-ads-8-5HRE-cfosp-IFNG albumin nanospheres combined with magnetic fluid hyperthermia on hepatoma. Front. Oncol. 13:1080519. doi: 10.3389/fonc.2023.1080519
Received: 01 February 2023; Accepted: 24 March 2023;
Published: 05 April 2023.
Edited by:
François Chevalier, CEA Saclay, FranceReviewed by:
Bhupendra Gopalbhai Prajapati, Ganpat University, IndiaIordana Astefanoaei, Alexandru Ioan Cuza University, Romania
Copyright © 2023 Zhang, Li, Chen, Ma and Liu. This is an open-access article distributed under the terms of the Creative Commons Attribution License (CC BY). The use, distribution or reproduction in other forums is permitted, provided the original author(s) and the copyright owner(s) are credited and that the original publication in this journal is cited, in accordance with accepted academic practice. No use, distribution or reproduction is permitted which does not comply with these terms.
*Correspondence: Mingying Liu, a2FrYXJ5ZUAxNjMuY29t