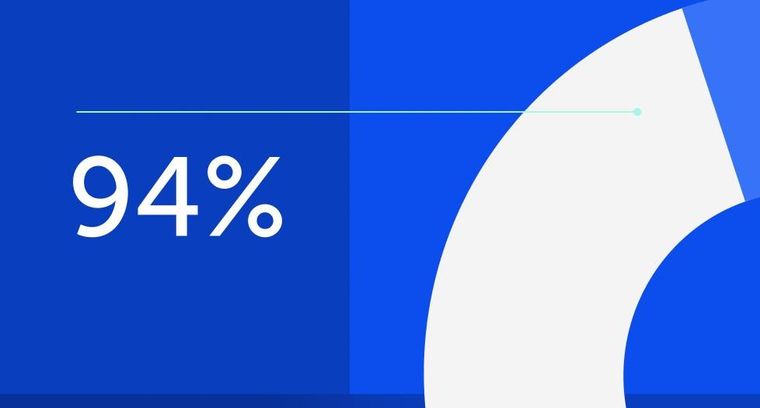
94% of researchers rate our articles as excellent or good
Learn more about the work of our research integrity team to safeguard the quality of each article we publish.
Find out more
MINI REVIEW article
Front. Oncol., 18 January 2023
Sec. Cancer Metabolism
Volume 13 - 2023 | https://doi.org/10.3389/fonc.2023.1058371
This article is part of the Research TopicTherapy Resistance in Tumor Microenvironment: Metabolic Reprogramming and Purinergic SignalingView all 6 articles
Regardless of improved biological insights and therapeutic advances, cancer is consuming multiple lives worldwide. Cancer is a complex disease with diverse cellular, metabolic, and physiological parameters as its hallmarks. This instigates a need to uncover the latest therapeutic targets to advance the treatment of cancer patients. Purines are building blocks of nucleic acids but also function as metabolic intermediates and messengers, as part of a signaling pathway known as purinergic signaling. Purinergic signaling comprises primarily adenosine triphosphate (ATP) and adenosine (ADO), their analogous membrane receptors, and a set of ectonucleotidases, and has both short- and long-term (trophic) effects. Cells release ATP and ADO to modulate cellular function in an autocrine or paracrine manner by activating membrane-localized purinergic receptors (purinoceptors, P1 and P2). P1 receptors are selective for ADO and have four recognized subtypes—A1, A2A, A2B, and A3. Purines and pyrimidines activate P2 receptors, and the P2X subtype is ligand-gated ion channel receptors. P2X has seven subtypes (P2X1–7) and forms homo- and heterotrimers. The P2Y subtype is a G protein-coupled receptor with eight subtypes (P2Y1/2/4/6/11/12/13/14). ATP, its derivatives, and purinoceptors are widely distributed in all cell types for cellular communication, and any imbalance compromises the homeostasis of the cell. Neurotransmission, neuromodulation, and secretion employ fast purinergic signaling, while trophic purinergic signaling regulates cell metabolism, proliferation, differentiation, survival, migration, invasion, and immune response during tumor progression. Thus, purinergic signaling is a prospective therapeutic target in cancer and therapy resistance.
Diverse cellular, molecular, and metabolic adequacies underlie cancer’s pathology (1–3). Aerobic glycolysis or the Warburg effect is a prominent metabolic feature in tumors (4, 5). Cancer cells acquire ATP from glycolysis preferably, in spite of ample oxygen availability and mitochondrial oxidative phosphorylation fitness (6).
Cancer cells are committed to replication and constantly use metabolic pathways to provide biomolecules and genetic material to promote their undifferentiated and aggressive state (7). Cancer cells constantly experience a “metabolic dilemma”: deciding whether to use ATP to facilitate biosynthetic processes/reductive power (NADPH) and biomolecules to synthesize membrane and genetic material (8). Thus, understanding the multifaceted metabolic adaptations in cancer is crucial to develop successful therapeutic approaches.
Purine nucleotides and nucleosides such as adenosine and ATP regulate intracellular energy homeostasis, nucleotide synthesis, and immune response (9, 10). Purines bind and activate plasmalemmal purinoceptors as endogenous ligands in the cell and/or in nearby cells and regulate extracellular signaling known as “purinergic signaling” (11). The Hungarian physiologist Albert Szent-Györgyi first described purines’ extracellular role, after he observed a temporary reduction in heart rate in a guinea pig by purified adenine compounds (12). In 1972, Geoffrey Burnstock proposed ATP as a non-adrenergic, non-cholinergic (NANC) neurotransmitter (11), but it seemed implausible that cells will actively release ATP as a neurotransmitter if it is already a ubiquitous intracellular molecular energy source (13). Adenine nucleotides [ATP, adenosine diphosphate (ADP), and adenosine (ADO)] function as signaling molecules (14) and modulate diverse signaling pathways through specific membrane receptors (15). ATP released through purinergic signaling activates both paracrine and autocrine signaling (16) and ATP hydrolysis further generates additional signaling molecules (17).
Purinergic signaling is a primitive evolutionary system (18), with short-term effects in neurotransmission and neuromodulation (19). Non-neuronal actions of purinergic signaling in mammals include immune response, pain, platelet aggregation, acute inflammation, exocrine and endocrine secretion, and endothelial-mediated vasodilatation (20). Trophic effects of purinergic signaling on cell proliferation, motility, and apoptosis mediate regeneration, wound healing, and cancer (21, 22).
Perivascular sympathetic nerves release ATP as a co-transmitter to cause smooth muscle contraction, whereas endothelial cells release ATP due to shear stress and hypoxia to produce nitric oxide and relaxation (23). Gentle mechanical distortion or hypoxia causes most cell types to release ATP physiologically (Figure 1) (24). Intracellular ATP is released via vesicular exocytosis, specific ATP binding cassette (ABC) transporters, connexin hemichannels, pannexin 1 (Figure 1), calcium homeostasis modulator (CALHM) channels, maxi-ion channels (MACs), volume-regulated ion channels (VRACs), and even purinergic (P2X7) receptors (Figure 1) (25, 26).
Figure 1 Purinergic signaling in cancer: Glycolysis and oxidative phosphorylation (OXPHOS) produce ATP in the cell, and it is released via cellular lysis, pannexin-1 (PNX-1) hemichannels, P2X7R, etc. to extracellular space. Cell membranes have several purine-hydrolyzing enzymes known as ectonucleotidases and include ectonucleotide triphosphate diphosphohydrolases (E-NTPDases/CD39), ectonucleotide pyrophosphatase/phosphodiesterases (E-NPPs), alkaline phosphatases (APs), and ecto-5’-nucleotidases (E-5’-NT/CD73). E-NTPDases hydrolyze exATP to ADP and also ADP to AMP. exATP activates ionotropic P2X receptors (ligand-activated ion channels) and metabotropic P2Y receptors (members of GPCR superfamily). AMP is hydrolyzed to ADO by E-5’-NT and APs. CD39 and CD73 hydrolyze exATP to ADP and AMP to ADO in cancer cells. ADP activates P2Y receptors and ADO activates P1 receptors (member of the GPCR family). ADO is hydrolyzed to inosine (Ino) by ADA or transported back into the cell by nucleoside transporters (NTs). The binding of purines to purinergic receptors turns on/off downstream signaling pathways. P1/P2Y receptor activation alters adenylate cyclase (AC) or phospholipase C-β (PLC-β) activity via different G proteins (Gs and Gq are stimulatory receptors). Activation of P2X receptors induces ion fluxes (Na+, K+, and Ca2+). All these actions alter the levels of secondary messengers like cAMP, inositol 1,4,5-trisphosphate (IP3), and Ca2+. IP3 binds to the IP3R receptors on the endoplasmic reticulum (ER), causing Ca2+ release from the ER. The secondary messengers regulate downstream proteins like protein kinase C (PKC), protein kinase A (PKA), cAMP response element-binding protein (CREB), androgen receptor (AR), mitogen-activated extracellular signal-regulated kinase (MEK), p38, extracellular signal-regulated kinases (ERK), and activating transcription factor 2 (ATF2). Transcription factors CREB, AR, and ATF2 induce gene expression for cancer development and progression.
Extracellular ATP (exATP) either activates purinergic receptors or gets hydrolyzed by ectonucleotidases (E-NTPDases—ectonucleoside triphosphate diphosphohydrolases, CD-73-ecto-59-nucleotidase, ENPP—ectonucleotidepyrophosphatase/phosphodiesterase, AP—alkaline phosphatases, and ecto-5′-nucleotidase) (27–29). Ectonucleotidases thus limit ATP signaling and produce additional ligands for purinergic receptors like ADP to P2Y12 and adenosine to A2AR (A2-adenosine receptors) (30).
ATP has a minor role as a co-transmitter in a healthy human bladder; however, the purinergic component increases to 40% nearly during interstitial cystitis, neurogenic bladder, and outflow obstruction (20, 31). Purinergic signaling has crucial participation in trauma, ischemia, multiple sclerosis, amyotrophic lateral sclerosis, and Alzheimer’s, Parkinson’s, and Huntington’s diseases (25, 32). Purinergic signaling is also reported to be involved in mood disorders, epilepsy, and neuropsychiatric diseases (33, 34). Deregulation of purinergic signaling can lead to neurodegeneration, rheumatic immune diseases, inflammation, and cancer (34, 35). Thus, purinergic agents are being explored to treat disorders of the urinary tract (36, 37), gut (38), bone (39, 40), cardiovascular system (41), kidney (42, 43), reproductive system (44, 45), and cancer (46–51). Selective agonists and antagonists of purinergic receptors play a role in the development of thrombosis, stroke, osteoporosis, kidney failure, and colitis (20, 52, 53), which are orally bio-available and stable in vivo.
Purinergic receptors (purinoceptors) were first described as membrane receptors in 1976 (54), which mediate potent physiological actions of exATP on multiple cell types (55) including stem cells (56–58). Purinoceptors were primarily distinguished into two families—P1 and P2, where P1 mediates responses for adenosine (ADO) and P2 mediates responses for ATP/adenosine 5′-diphosphate (ADP) (59). P1 and P2 receptors mediate neurotransmission and neuromodulation in the central nervous system (CNS) and functions such as memory, feeding, locomotion, and cognition (60).
Early on, two subtypes of the P1 (adenosine) receptor were recognized (61, 62), followed by four subtypes using P1 (adenosine) receptors cloning and characterization (63). The P2 receptor was distinguished into two subtypes—P2X and P2Y—on a pharmacological basis in 1985 (64). In 1993, P2Y was cloned as the first G protein-coupled receptor (65, 66) and two P2X receptors were cloned as ligand-gated ion channels in 1994 (67, 68). Presently, the P2X receptor has seven and the P2Y receptor has eight recognized subtypes, and these receptors are sensitive to both purines and pyrimidines (15, 69, 70). Among the three purinergic receptors, i.e., P1, P2X, and P2Y, P1- and P2Y-mediated cell signaling events have opposite effects in biological systems (71). Almost every cell type expresses specific purinergic receptors and ectonucleotidases, and experiences continuous fluctuations of intracellular ATP (iATP), exATP, and ADO (72). A dissimilar set of purinoceptors and ectonucleotidases in cells generate diverse cellular responses, and a definite set of purinergic signaling components in a particular cell is known as a “purinome” (73).
Selective amplification and cloning were used to isolate complementary DNAs (cDNAs) for two P1 receptor subtypes (A1 and A2) in 1989 (74), followed by the A3 subtype (75). Currently, there are four P1 receptor subtypes—A1, A2A, A2B and A3 (63)—and A1 and A2A receptors have shown polymorphisms (76). P1 receptors are activated by ADO and belong to the G-protein-coupled receptor (GPCR) superfamily (77). P1 receptors are activated by extracellular adenosine (exADO) and AMP, and regulate adenylyl cyclase (AC) (78). A2A and A2B receptors couple to Gs proteins, stimulating increased levels of intracellular cAMP by AC activation, whereas A1 and A3 receptors negatively coupled to AC via Gi/o proteins and inhibit cAMP production (28, 70). Adenosine deaminase (ADA) hydrolyzes exADO and hence regulates ADO-associated signaling, and ADO is transported back into the cytosol by nucleoside transporters (NTs) (49). P1 receptor subtypes arbitrate physiological effects in the central nervous, cardiovascular, and immune systems (79, 80) and many P1-specific agonists and antagonists are identified and in use (Table 1) (133).
P2X receptors are permeable ligand-gated cation channels (LGICs), where homotrimeric or heterotrimeric complexes of P2X1–P2X7 subunits form a trimer ion pore (69, 134, 135). P2X6R can only function as part of a heteromeric complex (136–140), whereas all other P2X subunits can assemble into homomeric or heteromeric ion channels. P2X1–7 receptors’ amino (N-) and carboxyl (C-) termini are intracellular and have binding motifs for protein kinases (69, 141, 142). The intracellular N- and C-termini of P2X receptors are linked to a large extracellular ligand-binding domain (with 10 conserved cysteine residues) using two transmembrane-spanning helices (TM1 and TM2) (143–145). TM1 assists with channel gating, while TM2 lines the ion pore (146).
Cloning of P2X-like receptors suggested ATP as an untimely evolutionary extracellular signaling molecule and a natural ligand for P2X receptors (147). ATP binds in the extracellular domain of P2X receptor and elicits a global conformational change that opens the channel for free cations’ movement in and out of the cell (148, 149).
Alternative splicing of the human P2X7R gene produced different isoforms with diverse cellular responses (150). P2X7R gene isoforms A and B are ubiquitously expressed as functional ion channels (151). P2X7A is a full-length variant, but the P2X7B isoform retains an intron between exons 10 and 11 with a stop codon, thus producing a shorter protein (152, 153). The truncated P2X7B lacks the C-terminal tail, which is crucial to macropore opening; however, P2X7B is still capable of opening the ion channel (153, 154).
P2X receptors mediate fast neurotransmission and at times are located pre-junctionally to promote the amplified release of co-transmitters, such as glutamate in primary afferent neuron terminals in the spinal cord (155). Activation of P2X receptors results in rapid membrane depolarization via Na+ and Ca+2 influx and K+ efflux (Figure 1) (156). P2XRs do not discriminate between Na+ and K+, but prefer the movement of Na+ down its electrochemical gradient at resting membrane potentials (157). P2XRs’ Ca2+ permeability is relative to Na+ (PCa/PNa), but varies due to the subunit makeup of the functional channel (158). Thus, P2XR activation results in Na+-mediated plasma membrane depolarization and an increased free cytosolic Ca2+ concentration ([Ca2+]i), leading to action potential propagation and a myriad of Ca2+-sensitive processes like secretion (159, 160), muscle contraction (161, 162), and cell survival (163, 164). P2X1-, P2X2-, P2X3-, P2X4-, and P2X7-knockout mice and P2X1-overexpressing transgenic mice have multiple diseases associated with P2X receptor polymorphisms (165). Thus, selective P2X agonists and antagonists are available for multiple diseases like neurodegenerative diseases, thrombosis, stroke, osteoporosis, kidney failure, and cancer (20, 52, 53).
P2Y are purinergic G protein-coupled receptors with eight accepted human subtypes: P2Y1, P2Y2, P2Y4, P2Y6, and P2Y11–14 (15, 166), and are stimulated by adenosine and uridine nucleotides and nucleotide sugars. The missing numbers for P2Y receptor correlate to non-mammalian orthologs with a little sequence homology to P2Y receptors, but with no functional proof of receptiveness to nucleotides (147). Based on sequence similarity, P2Y receptors have two distinct subgroups: Gq-coupled receptors sharing a 28%–52% sequence similarity (P2Y1,2,4,6,11) and Gi/o-coupled receptors with a 45%–50% sequence similarity (P2Y12,13,14) (15). Human P2Y1, P2Y12, and P2Y13 are activated by ADP, P2Y2 and P2Y11 are activated by ATP, P2Y2 and P2Y4 are activated by UTP, P2Y6 is activated by UDP, and P2Y14 is activated by UDP-glucose (167). For P2Y1 receptors, ADP is a stronger agonist than ATP (168).
P2Y1, P2Y2, P2Y4, and P2Y6 receptors are coupled to Gq proteins to activate phospholipase C (PLC), increase phosphoinositide level, and mobilize Ca+2 (169). ATP and UTP fully activate P2Y2 receptors; however, ADP and UDP are less effective as agonists (15). UTP is the strongest activator of the human P2Y4 receptor and P2Y6 receptors are UDP receptors (15).
P2Y11 receptor activation by ATP increases both cAMP and inositol triphosphate (IP3), whereas activation by UTP causes calcium mobilization without raising cAMP or IP3 (170). ATP is the preferred native ligand at P2Y11 receptors (170); however, ATPγS is a more efficacious agonist than ATP (15). P2Y12, P2Y13, and P2Y14 coupling to Gi proteins cause inhibition of adenylate cyclase (AC) (15). ADP is the original agonist of the P2Y12 receptor and the P2Y12 receptor activates RhoA and Rho kinase and phosphatidylinositol 3-kinase (PI3-K) via Gαi (171). The P2Y13 receptor can couple to Gs at high ADP concentrations and to G16 and Gi simultaneously (172). Activation of several P2Y receptors stimulates mitogen-activated protein kinase (MAPK), especially extracellular signal-regulated protein kinase 1/2 (ERK1/2) (15).
Purines perform autocrine–paracrine functions in cancer cells by ATP production and release, which causes cell proliferation, tumor growth, and immunosuppression (72). Cancer cells use aerobic glycolysis to synthesize ATP (Warburg effect), lactate production, and consequent extracellular acidification (72). Cancer cells release ATP from pannexin-1 (PANX1) hemichannels, P2X7R, or by cellular lysis and can activate either P2 receptor (Figure 1) (72). ExATP activates P2 receptors in cancer cells (primarily P2X7R and P2Y2R) via an autocrine–paracrine way to encourage proliferation, epithelial-to-mesenchymal transition (EMT), and migration (72). Ectonucleotidases (CD39 and CD73) hydrolyze exATP to ADO and increased ADO inhibits the anti-tumor function of innate immune cells and effector T cells (Teff, CD4+ and CD8+), and monocyte differentiation into associated tumor macrophages 2s (72).
Purines in the tumor microenvironment (TME) have gained immense attention, as high concentrations of ATP and ADO in the TME are established biochemical markers of cancer (173, 174). Treatment of pheochromocytoma PC-12 cells with maitotoxin released ATP and activated purinergic receptors (175); mechanical stimulation of Ehrlich ascites tumor cells released ATP (176) and TGF-β stimulation of A549 human lung cancer cells released ATP by exocytosis (177). In vivo ATP monitoring in a tumor-bearing mouse using reporter cells carrying an exATP sensor revealed that exATP is a primary source of exADO in the TME (178). ExADO is copious in microdialysates from tumoral core regions (178) and tumor hypoxia promotes ADO formation by hypoxia-inducible factor 1α/β (HIF-1α/β)-induced CD39 and CD73 expression (179–182). Ectonucleotidases CD39 and CD73 are immune checkpoints in cancer, expressed in cancer cell lines, stromal cells, and immune cells, and modulate ATP and ADO levels in the TME (183). ATP is released through the PANX1 channel and a truncated PANX1 protein (PANX11-89) is overexpressed in metastatic human cancer cell lines (184). PANX11-89 along with wild-type PANX-1 promotes gain of function of channel activity, an increased ATP release, and resistance of cancer cells to mechanical deformation (184). ATP is also released through P2X7R and P2X7R association with NLRP3 inflammasome producing pro-inflammatory cytokines, like IL-1β and IL-18 (185–187). Tissue damage and cell death induced during chemotherapy/radiotherapy produce damage-associated molecular patterns (DAMPs), which are mostly ATP (188). The CD39/CD73 pathway quickly converts this exATP into ADO, and thus, a particular equilibrium of purines in the TME decides the success of a given clinical treatment (189).
P2X7R is the most studied purinergic signaling element for its role as an apoptotic inducer (190). However, P2X7R promoted proliferation in lymphoid cells (191), instead of its previously designated role as a pro-apoptotic and necrotic cationic channel receptor (192). Elevated exATP levels and activated P2X7R had growth-promoting effects via MAPK/ERK kinases and stimulated de novo synthesis of pyrimidine nucleotides (193). P2X7R has a pro-mitotic function in B-cell chronic lymphocytic leukemia, and higher P2X7R expression was seen in patients with an aggressive form of chronic lymphocytic leukemia (194). P2X7R expression promoted prostate and breast tumor progression (195); thus, P2X7R has a dual ability to induce apoptosis and promote cell survival (196).
Increased P2X7R expression is found in breast (195), thyroid (197), mesothelioma (198), ovarian (199), pancreatic (200), colon (201, 202), osteosarcoma cell (111), and liver (203) cancer, and higher P2X7R expression associated with a high tumor grade. Elevated P2X7R expression was originally known to induce apoptosis; however, proliferating and/or survival effects of P2X7 were reported later on (204). P2X7R as a growth-promoting receptor was supported by its exogenic expression in numerous cancer cell lines and tissues, by high amounts of ATP in the TME, and by positively regulating aerobic glycolysis (204). P2X7R stimulates ERK phosphorylation-dependent and -independent intracellular Ca2+ rise (205, 206), the PI3K/AKT/GSK3β/β-catenin pathway, and mTOR/HIF1α/VEGF signaling (199, 207).
P2X7B is a widely studied splice variant of P2X7 in cancer due to its pro-tumoral role and powerful constructive effect on P2X7A (208, 209). Removal of the lengthy carboxy-terminal in P2X7B enables its growth-promoting potential in response to ATP; however, it loses its cytotoxicity linked to the pore formation (208). P2X7B promoted ATP secretion, activated NFATc1 (nuclear factor of activated T cells c1) proliferative pathway, and supported soft agar invasion (208, 209). The role of P2X7B in solid cancer was first reported in human osteosarcoma, where P2X7 isoforms were expressed in osteosarcoma tissues and P2X7B expression was the most prevalent (209). Transfection of Te85 osteosarcoma cells with P2X7B increased proliferation and reduced bone deposition in serum-starving conditions compared to non-transfected cells (209). Bradykinin treatment of human neuroblastoma cell lines (CHP-100, SH-SY5Y) upregulated P2X7B and cell proliferation, suggesting a growth-metastatic stimulus by P2X7B overexpression (210). The P2X7B isoform favored metastasis and resistance in tumor cells (208, 209, 211), caused malignancy of neuroblastoma (NB) cells (210), suppressed autophagy, and induced drug efflux and EMT in NB (212).
P2Y receptor overexpression also promotes cell proliferation and is observed in basal cell and squamous cell carcinoma biopsies (213). cDNA microarray analysis showed higher expression of P2RY2 transcript in gastric cancer biopsies than adjacent healthy tissue (214). P2Y2R promoted cell proliferation in C6 glioma cells via the Ras/Raf/MEK-1 pathway, which was modulated by PLC/PKC and Ca2+ (215).
ADO is the chief product from exATP, and ADO receptor A2BR functions as a cell proliferation promoter in human hepatocellular carcinoma (216), prostate cancer (217), colorectal carcinoma (218), breast cancer (219), oral squamous carcinoma (220), head and neck squamous cell carcinoma (HNSCC) (91), and bladder urothelial carcinoma (221). Pharmacological/genetic inhibition of A2BR decreased cell proliferation in colon carcinoma cells, human oral cancer, and bladder urothelial carcinoma (218, 220, 221).
Contrary to P2Y2R activity, P2X4R expression inhibited proliferation in gastric cancer cell lines (222) and reversed P2X7R-induced proliferation in breast cancers (223). P2X7R is downregulated in endometrial cancer and P2X7R activation-induced apoptosis in endometrial carcinoma cells (224) and inhibited virus-induced skin cancer formation in vivo (225). P2Y2 receptor activation suppressed cell proliferation in endometrial carcinoma cells (HEC-1A and Ishikawa) (226), human esophageal cancer cells (227), human colorectal carcinoma cells (HT29 and Colo320 D) (228), and nasopharyngeal carcinoma cells (229). P2Y6R activity inhibited cell proliferation through store-operated Ca2+ entry (SOCE) and β-catenin (230). Consequently, purinergic signaling positively regulates cell proliferation; however, functional interactions among purinergic receptor subtypes determine the ultimate effect of purinergic signaling in cancer.
HEK293 and ACN (neuroblastoma) cell lines transfected with P2X7R showed an amplified lactate production and cell proliferation, signifying aerobic glycolysis and the Warburg effect (231). P2X7R expression upregulated glycolytic promoters like glucose transporter Glut1, enhanced intracellular glycogen stores, repressed pyruvate dehydrogenase (PDH) activity, and increased protein kinase B (PKB/Akt) phosphorylation and hypoxia-inducible factor 1α (HIF-1α) expression to evade aerobic adaptations (231).
P2X7R accomplishes Warburg and proliferative effect by functioning as an ion channel and a highly conductive non-selective pore (232). In this way, P2X7R stimulated a rise in mitochondria’s resting membrane potential and basal calcium levels (232). P2X1R and P2X7Rs activation in leukemia T cells (Jurkat) caused higher intracellular calcium levels and uncontrolled proliferation, and pharmacological inhibition of these receptors decreased cell proliferation, mitochondrial activity, and calcium signaling (233).
A549 (human non-small cell lung cancer cell line) internalized exATP by clathrin-mediated endocytosis (CME), caveolae-mediated endocytosis, and macropinocytosis (234), which served as glycolytic ATP in cancer cells (235) and favors proliferation, drug resistance, and EMT (234). High exATP and exADO concentrations are typical of neoplastic cells, thus recognizing ADO as a pro-tumoral factor (236). Toll-like receptor 3 (TLR3) is a pro-inflammatory receptor and promoted glucose consumption and lactate release in prostate cancer cell lines via HIF-1α and extracellular activation of A2BR (237). ZM241385 and SCH5826 are A2AR antagonists and repressed proliferation in cancer-associated fibroblasts and human tumor xenografts in mice (87).
Continual proliferation characterizes tumor cells and growth factors stimulate cell proliferation through receptors with intracellular tyrosine kinase domains (238). ATP is not a member of the growth factor family and P2RX7 has no tyrosine kinase domains; however, low concentration of ATP activates P2RX7, cell proliferation (111, 200, 239–241), and kinase activity (242). P2RX7 activated cellular sarcoma tyrosine kinase (c-Src), PI3-K/Akt, MAPKs (ERK1/2, p38, and JNK), and protein kinase C (PKC) (243). P2RX7 activated these kinases in response to ATP, indirectly via Ca2+ or its co-localization with kinases in lipid rafts using a lipid-interaction motif in its C-terminus (amino acids from 574 to 589) (244–247). Reduced P2RX7 expression resulted in the development of various cancers (150, 248–250) and P2RX7 inhibition by shRNA in glioma tissue increased EGF and phosphor-EGF protein expression (251). P2RX7 is not known to inhibit tumor suppressor proteins like TP53 or retinoblastoma-associated (RB), but a death domain is localized in its C-terminus (244).
The ATP/P2RX7 axis can induce or inhibit apoptosis. The ATP/P2RX7 axis promoted p53 protein levels, apoptosis, and necrosis in mesangial (252) and human cervical cancer cells (253), and induced apoptosis in acute myeloid leukemia (AML) (254). Tumors grew faster in p2rx7−/− mice (255, 256), and P2RX7 inhibition induced apoptosis in colorectal cancer cells (241) and the MCF7 breast cancer model (257). P2RX7-induced necrosis released growth factors to advance the proliferation and invasiveness of cancer cells (200) and immunomodulatory cytokines. A non-conformal receptor (nfP2RX7) promotes tumor growth, has calcium channel activity, but is incapable of inducing cell death (258, 259).
Tumors facilitate neoangiogenesis, i.e., growth of novel blood vessels from a prior vasculature for oxygen and nutrients (260). Tumor vasculature is abnormal with hardly any perivascular cells, and this brings hypoxia and acidity in TME, which facilitates tumor aggression (261). Vascular endothelial growth factor (VEGF) couples with VEGF receptor 2 (VEGFR2) to boost endothelial cell propagation (262). P2RX7 promoted VEGF discharge in human monocytes (263, 264) and P2RX7-expressing tumor xenografts of human embryonic kidney cells were found to be more angiogenic than the control cell tumors (239). The ATP/P2RX7 axis stimulates PI3K/Akt and NF-κB pathways to induce VEGF and higher vessel density (113, 239, 265).
Malignant cells invade surrounding and distant tissues, and this metastatic extension of the primary tumor is responsible for approximately 90% of mortality in cancer patients (266). Metastatic stages in a primary tumor involve cell–cell adhesion loss, EMT, anoikis evasion, migration, invasion, mesenchymal–epithelial transition (MET), and embedding as a secondary tumor (267). EMT initiates metastatic stages, as epithelial cells morph into a mesenchymal phenotype with a superior invasive and migration capacity (268). Transforming growth factor-β (TFG-β), Wingless/Int (WNT), and epidermal growth factor (EGF) stimulates EMT promoter transcription factors, i.e., TWIST and SNAIL (269). TWIST and SNAIL cause loss of epithelial marker expression [E-cadherin, zona occludens-1 (ZO-1), and keratins] and induce expression of mesenchymal markers [N-cadherin, metalloproteinases (MMPs), and vimentin], thus promoting metastasis (270).
ATP is the cellular energy currency, but during purinergic signaling, it engages in EMT, migration/invasion, and metastasis in various cancer types (271). High ATP concentrations in lung cancer cell lines favored cell detachment, migration, and invasion, with increased MMPs, vimentin, SNAIL and SLUG expression, and filopodia development and cell protrusions (235). A decrease in E-cadherin and ZO-1 expression by the micropinocytosis of exATP and genetic deletion of sorting nexin-5 (SNX5, a gene involved in intracellular trafficking) reduced cancer cell proliferation and metastasis (235). TGF-β1 elicited lung cancer cells to release ATP and activated P2 receptors (177). TGF-β1 induced remodeling of actin and cell migration by autocrine stimulation of P2X7R and knockdown or pharmacological inhibition of P2X7R suppressed these processes (177). A mutated EGFR in PC9 (human lung adenocarcinoma cell line) caused a constitutive activation of P2X7R and cell migration, even without TGF-β1 (272). AG1478 is an EGFR inhibitor and abolished PC9 cells’ motility and lamellipodium extension, showing a TGF-β1/P2X7R/EGFR cross-signaling during cell migration (272).
P2X7R promoted metastasis in pulmonary (177, 269), prostatic (273), mammary (108), pancreatic (200), glioma (274), osteosarcoma (111), and glioblastoma stem cell (GSC) cancer (275). P2RX7 high expression in MDA-MB-435 cells (an invasive human breast cancer cell line) activated pro-migratory phenotype and invasiveness by releasing extracellular matrix-degrading proteases in vitro and in vivo (276, 277). The ATP/P2X7R axis encouraged an autocrine-mediated TGF-β-instigated cell migration in lung cancer cells (177). In response to ATP, P2X7R promoted cell migration and invasion in pancreatic ductal adenocarcinoma (200), human breast cancer, and colon cancer cells, where the ATP-activated PI3K/Akt/GSK3 pathway mediated E-cadherin expression and EMT (108, 241). In a mammary 4T1 cancer mouse model, P2RX7 expression promoted tumor growth and metastasis and was reversed by P2X7R antagonists (108). Tumors with distant metastasis showed higher expression of P2X7R in broncho-alveolar lavage (278) and more lung tumors were observed in p2rx7−/− mice injected with B16 melanoma cells, suggesting that endogenous P2X7R activity hinders B16 migration and invasion (256). Bradykinin induced P2X7B expression and promoted neuroblastoma metastasis in bone marrow using high exATP levels in the bone marrow as growth, seeding, and anti-apoptosis stimulus (210).
In most breast cancer cell lines (121, 279, 280), the invasive edge of the breast tumor tissue and tumor embolus in lymphatic sinuses express P2Y2R highly, proving the participation of P2Y2R in metastasis (279). Breast cancer cell lines with more exATP are highly metastatic (281), and migration and invasion are moderated through MEK/ERK1/2-dependent signaling pathway activation (121, 279, 282) and ATP-P2Y2R-β-catenin (280). Activation of P2Y2R increases EMT-related gene expression and transactivates a pathway amid P2Y2R and EGFR in ovarian (283) and prostate cancer cells (284).
A1R antagonist 1,3-dipropyl-8-cyclopentylxanthine (DPCPX) reduced proliferation and migration in renal cancer cell (RCC) lines, and tumor growth in vivo, while A1 agonist N6-Cyclopentyladenosine (CPA) rescued RCC cell migration (82). Higher CD73 expression was seen in lymph nodes of HNSCC patients, and CD73 encouraged HNSCC migration and invasion in vitro by A3R activation via the EGF/EGFR signaling pathway (285). CD73 expression promoted mesenchymal phenotype in hepatocellular carcinoma and A2AR activation restored the effect of CD73 knockdown, suggesting an adjuvant treatment of CD73 and A2AR inhibitors (286). Low ADO concentrations reduced migration and the invasive capacity of prostate and breast cancer cell lines (287) and human cervical and ovarian cancer cell lines (288, 289), but enhanced stemness and EMT gene expression in gastric cancer cells by activation of A2AR and the Akt/mTOR pathway (290).
A2BR activation induced a partial EMT through cAMP/PKA and MAPK/ERK transduction pathways in human type II alveolar epithelial cells (A549) (89) and BAY 60-6583 (selective A2BR agonist) counteracted TFG-β-induced EMT in A549 cells (89). ADO augmented migration via the A2BR/AC/PKA/cAMP axis in MDA-MB231 breast cancer cells (219). A2BR antagonist PSB-603 inhibited cell proliferation and transmigration in HNSCC-derived cell lines and MRS1754 (a selective A2BR antagonist) reduced proliferation and migration in RCC 769-P and Caki-1 cell lines (91, 92).
A3R activation detained cancer cell motility and invasive capacity by hampering NADPH oxidase activity and AC/PKA in AT6.1 rat prostate (291). A3R obstruction reduced cell migration and invasion in glioblastoma (GBM) patient-derived primary cultures of stem-like cells and a GBM cell line (292). Collagen architecture modulates ATP : ADP ratio, and this ratio increases in dense extracellular matrices (ECMs) where migration is arrested and decreases in aligned ECMs where migration is eased (293). Thus, the adhesion environment changes the cellular energy necessity, and ATP increases in the ECM assist metastasis (293). ATP is also vital during invadopodia formation, and even in the absence of MMPs, it helps in F-actin network growth (294).
The immune system fights infections and immune surveillance eliminates cancer cells via checkpoint inhibitor (CPI) expression, immunosuppressive factor secretion (295, 296), and Teff cell and natural killer (NK) cells (297). These immune responses against cancer cells are dulled by immune-suppressive cells [regulatory T (Treg) and myeloid-derived suppressor cells (MDSCs)] (298).
Ectonucleotidases mediate ADO accumulation, where CD39/NTPDase1 converts ATP to ADP/AMP and CD73 converts AMP to ADO (299). Tumor cells with activated CD73 favor cell adhesion through EGF and ADO accumulation energizes cell proliferation and metastasis by inducing intracellular cAMP’s downstream signaling through A2A and A2B, leading to immunosuppression (300). exATP evokes a “find me” signal evoking an immune response, and this response is overturned by exATP conversion to ADO by CD39 and CD73 (183, 189, 301). ADO, thus, has an immunosuppressive role and dismantles the anti-tumor immune attack in the TME (183, 189, 301). Conditions like hypoxia, necrosis, and inflammation prime cells to release DAMPs, mainly ATP (187), and exATP employs macrophages, neutrophils, and dendritic cells (DCs) to resolve cellular damage (302–304). Chemotherapeutic agents in AML induced exATP and promoted the upregulation of Treg cells (305), suggesting that ATP mediates the communication of tumor cells and immune system components and modulates the inflammatory response in the TME. Post-anti-cancer therapies, dying cancer cells release ATP and activate P2X7R in DCs, leading to IL-1β secretion via P2X7R-dependent NLRP-3 inflammasome assembly (306, 307), and this suggests the role of NLRP-3 inflammasome in the efficacy of anti-cancer therapies. Hypoxia in cancer cells favors ADO accumulation and CD39 and CD73 overexpression in the TME, by HIF-1 transcription factor activity (179–182).
Ovarian cancer cell lines generated ADO to attract myeloid cells and induce their differentiation to macrophages with a non-inflammatory phenotype (M2-TAM) with CD39 overexpression (308). CD39-expressing TAMs and CD73-expressing stromal fibroblasts collaborated to amplify ADO levels and immune-suppressive effects in ovarian cancer cell lines (308). ADO buildup is deadly for immune surveillance, and CD39/CD73-targeted antibodies promoted immunosuppression in macrophages, DCs, and T cells (309), making these ectonucleotidases the obvious immunity-strengthening targets. Antibodies against CD39 and CD73 improved NK and T-cell cytotoxicity in ovarian cancer cell lines by ADO reduction (310). CD73 expression and ADO levels increase post-focal radiotherapy, and thus, blocking CD73 during focal radiotherapy enhanced DC infiltration and anti-tumor T-cell-dependent responses in breast cancer cells (311).
P2RX7 has an extensive role in immune cells and tumor onset (312). P2RX7 is expressed in human macrophages and DCs to alter cytokine production owing to exATP (313–316). The ATP/P2RX7 axis boosts anti-tumor immune response, as exATP attracts DC precursors to the tumor bed, in the immediacy of dying cells, and promotes their ability to present tumor-associated antigens (317). P2RX7 activation in macrophages and DCs releases NLRP3 to increase IL-1β and IL-18 production and NLRP3 has pro- and anti-tumorigenic roles in cancers based on cytokine quantity (318). AML patients have upregulated P2X7R receptor mRNAs (splice variants A and B) in AML blasts, suggesting P2X7RA and P2X7RB as potential prognostic markers in AML patients (211, 319). Murine B16 melanoma cells express high levels of P2X7R, and its xenotransplantation in wild-type and p2rx7–/– null mice showed accelerated tumor growth in the p2rx7–/–mice (320). Compared to wild-type animals, p2rx7–/– mice tumors showed immunosuppression with a higher number of Treg cells and fitness markers (OX40, PD-1, and CD73) and a declined number of Teff and CD8+ T cells (320). Ectonucleotidase CD73 was expressed highly in macrophages, Treg, and CD8+ Teff, while CD39 was prominent in Teff in tumor-bearing p2rx7–/– mice (320). These CD39/CD73 expression alterations reduced exATP and increased ADO in the TME, causing immunosuppression in p2rx7–/– mice tumors (320). Blocking P2X7 by using A740003 in wild-type mice reduced the growth of tumors, improved the immune response, elevated IFN-γ, and reduced IL-1β levels, without changing exATP levels (320). Thus, P2X7R is a key determinant of anti-tumor immune response due to its effects on immune cell infiltration (320). Leukocyte common antigen (CD45+)-immune cells in the lung adenocarcinoma patients’ TME had lesser P2X7R pore function than CD45+ cells from outside the tumors, suggesting higher P2X7B mRNA levels in the immune cells from the TME than normal lung tissue (321).
P2X7 stimulation restricted tumor suppression by inducing stress-induced premature senescence (SIPS) in tumor-infiltrating lymphocytes (TIL) and transfer of p2rx7-/- CD8 T cells reduced tumor growth with improved survival in lymphopenic mice (322). However, in a mouse melanoma CD8+ T-cell adoptive transfer model, the number of tumor-specific p2rx7-/- CD8+ T declined with an increase in tumor burden, and this was concurrent with decreased proliferation and higher apoptosis (323). In vitro stimulation of P2RX7 via ATP analog BzATP controlled B16 melanoma by CD8+ T cells, suggesting that exATP sensing on CD8+ T cells by P2RX7 diminished melanoma tumors (323). This presents P2X7 as a purinergic checkpoint that may be targeted to enhance anti-tumor response.
A2AR is the foremost P1 causing immunosuppression and its genetic deletion eased tumor eradication by T cells (324). Supplemental oxygenation (hyperoxia) helped CD8+ T cells to infiltrate tumors, regress the tumor, weaken immunosuppression by Treg, and enhance chemokines and cytokine levels, through the hypoxia/ADO/A2AR pathway, and these effects were not shown by A2AR-/- mice (181). A2AR antagonist co-administration improved the efficiency of PD-1 antibodies (325) in reversing the immunosuppression of induced tumors, thus making A2AR an immunotherapy target (326). Tissues lacking A2AR showed improved tumor rejection, diminished immunosuppression, and enhanced IFN-γ secretion by T cells (327). A2AR-mediated ADO signaling ablation matured NK cells, improved anti-tumor immunity, and delayed tumor instigation and growth (328). A2BR works synergically with A2AR in colorectal cancer cells to participate in the NT5E/ADO-dependent immune checkpoint to launch immunosuppression (329). A1R deletion reduced human melanoma cell line and tumor growth in immune-deficient xenografts, upregulated PD-L1 levels, and inactivated co-cultured T cells, which compromised anti-tumor immunity in vivo (330), indicating the critical role of ARs in the anti-tumor immune response.
Cancer stem cells (CSCs) within the tumor are related to cancer metastasis, chemotherapeutic drug resistance, and tumor recurrence (331). CSCs drive tumor spread and preservation due to their stem cell-like properties (332) of self-renewal and multi-lineage differentiation potential (333). Unlike normal tissue renewal, CSCs do not mature and form a core in a tumor to sustain tumor growth (334). CSCs express explicit cell surface markers like CD166+, CD133+, CD44+, CD29+, and CD24+ (335), and the nature and expression level of these markers vary depending on the type of cancer (336). Endogenous ATP release caused a proliferation in various human glioma cell lines (U251MG, U87MG, and U138MG) via both P1 and P2 receptor signaling (337). ATP treatment decreased the number and size of tumor spheres in human U87 or U343 and rat C6 gliomas (338), by upregulating P2X4, P2Y1, and P2Y14 in tumor spheres (338) and reduced the number of Nanog, Oct-4, and CD133+ CSCs (338). Thus, ATP and purinergic receptors are potential pharmacological targets for CSC therapy (338, 339).
Expression of the P2X7 receptor correlates with the degree of cell differentiation and its higher expression and activity are seen in embryonic stem cells (340). A decrease in pluripotency markers suppresses P2X7 receptor expression and activity, and differentiation was induced in quiescent cells upon P2X7 inhibition pharmacologically (340, 341). Sustained P2X7 stimulation in glioblastoma multiforme stem-like cells from primary human tumors decreased CSC proliferation due to cell death and growth arrest (342). This growth arrest induced a quiescent state in the surviving cells and caused cell regrowth and sphere formation 2 weeks after the end of the treatment (342), suggesting P2X7-induced chemoresistance and tumor recurrence.
P2X7B promoted osteosarcoma by a higher expression in osteoblast precursor cells than differentiated osteoblasts (343). ATP analog and P2X7 agonist 2’[3’]-O-[4-benzoylbenzoyl]-ATP (BzATP) increased the expression of P2X7A and B in human GSCs and supported GSC invasion (275). P2X7 antagonist A438079 nullified BzATP effects on P2X7A and P2X7B expression (275).
Dying tumor cells release ATP, making exATP levels multiple times higher in cancer tissues than the analogous normal tissues (178). exATP induces cellular stress and anti-cancer therapy resistance via inflammation, hypoxia, and platelet aggregation (344). Anti-cancer drugs (targeted and chemotherapeutic) increase iATP and cancer cell survival by exATP (345). Sunitinib promoted drug resistance in NSCLC A549 cells by internalizing exATP through CME, caveolae-mediated endocytosis, and macropinocytosis, which elevated iATP levels to 150%–200% of the original iATP concentration (234, 345, 346). Increased iATP levels by exATP is not a momentary effect and the elevation lasts till exATP is present in the TME (346).
Macropinocytosis activation is a hallmark of oncogenic Ras mutations harboring cancers, and macropinocytosis/endocytosis-mediated elevated iATP levels from exATP internalization promote drug resistance (347). p21-activated kinase 1 (PAK1) is an essential enzyme for macropinocytosis, and its inhibition by siRNA knockdown or IPA3 inhibitor resulted in lower iATP levels and survival in exATP- and sunitinib-treated A549 cells (345). Abundant iATP molecules after exATP internalization contend for the ATP binding site on receptor tyrosine kinases (RTKs) with tyrosine kinase inhibitors, inducing phosphorylation and, in turn, activation of downstream signaling events causing drug resistance (345).
Internalized exATP molecules served as an energy supplement, regulated ABC transporter expression levels, and increased cell survival in A549 lung cancer and SK-Hep-1 cells (345), indicating intense effects of exATP on transporter activity of ABC to enhance anti-cancer drug efflux and drug resistance. exATP hastened EMT, mobility, and invasion, increased MMP expression, increased levels of EMT-transcription factors (vimentin, Snail, and Slug), and decreased epithelial markers in lung cancer cells (235). These effects were TGF-β-independent and partially dependent on the purinergic signaling (P2X7) activation (235), suggesting exATP-induced drug resistance via EMT. exATP promotes drug resistance through P2 receptors as an extracellular messenger (72), and hence, the P2X and P2Y receptor families have emerged as potential candidates for chemotherapy resistance (348, 349). ENTPD1/CD39 convert exATP to AMP and activate cAMP-mediated mitochondrial stress response via the CD39/P2RY13/cAMP/PKA axis and aided resistance to cytarabine in AML, proposing exATP and CD39 as key factors in AML chemoresistance (348).
Activated platelets in tumor cells release ADP in the extracellular compartment, and ADP-activated P2Y12 in pancreatic ductal adenocarcinoma (PDAC) increased expression of markers for gemcitabine resistance like human equilibrative nucleoside transporter 1 (hENT1) and cytidine deaminase (CDD) (350). P2Y12 inhibition by ticagrelor blocked the survival of PDAC cells induced by platelet-derived ADP and ATP (350).
P2X7 receptor is bi-functional, and depending on the cell type and level of activation, it can trigger either cell growth or death (191, 194, 351–353). P2X7 overstimulation by mM ATP concentration formed pores and caused cell death in rat peritoneal cells (RPCs) and in P2X7 transfected 1321N1 cells (1321rP2X (7)-11) (354). Low-level stimulation of P2X7 endowed P2X7-expressing cells with proliferative advantage by enhancing aerobic glycolysis, OXPHOS, and biosynthesis (173). P2X7 expression helps cancer cells to invade and metastasize (153, 173), and thus, its antagonists inhibited tumor growth and migration (344). Non-pore functional P2X7 (nfP2X7) is a distinct P2X7 conformation that cannot form a functional pore upon exATP stimulation (259). ATP concentration equivalent to TME drives nfP2X7 expression and survival in tumor cells (259), suggesting a role of nfP2X7 in promoting resistance to apoptosis and chemotherapies.
Some chemotherapeutic agents incite immunogenic cell death (ICD), leading to immunological memory generation and improved relapse-free survival (355). Stressed/dying tumor cells release exATP as a DAMP during ICD (259), and thus, ICD depends on exATP release as well as on its stability in the TME (356). exATP release drives DC recruitment and activation by P2RX7 ligation, generating adaptive immunity against cancer by tumor-derived antigens and IL-1β secretion (306).
P2X7B caused daunorubicin and radiotherapy resistance in P2X7B-overexpressing cells by increasing exATP levels in the TME (211, 357). P2X7B expression increased neuroblastoma chemoresistance through drug efflux via multi-drug resistance protein (MRP)-type transporters, suppressing autophagy, promoting EMT and resistance to retinoids, and retaining stem-like phenotype (212).
exATP communicates with immune cells like monocytes, T cells, B cells, macrophages, eosinophils, and neutrophils to execute immune-activating functions (306). exATP is converted to ADO by CD39 and NT5E/CD73, and while exATP promotes anti-tumor immunity, ADO attenuates it (349, 358). CD39 and CD73 have emerged as potential targets for anti-cancer immunotherapy, as these limit exATP conversion to ADO (355). CD39 blockade augmented exATP/P2X7-mediated pro-inflammatory anti-tumor response and release of IL-18, which facilitated increased infiltration of intra-tumor Teff cells and overturned anti-PD-1 resistance (349). Adenosine-induced signaling diminishes the anti-tumor action of immune cells (macrophages, T cells, B cells, DCs, mast cells, and NKs) and activates Treg cells, creating an immune-suppressive environment to diminish immune-therapy efficacy (344, 359). Tumor cell autophagy is critical for chemotherapy-induced ICD, and it promotes exATP release over adenosine generation (360); thus, purinergic signaling inhibition might improve anti-cancer drug response.
Fe3+ and doxorubicin-releasing nanoparticles depleted exATP via metal ion-triphosphate coordination and sensitized chemotherapy (361), suggesting that exATP depletion may also reduce drug resistance. Macropinocytosis inhibition reduced cellular uptake of exATP and iATP levels, alleviating EMT and drug resistance promoted by exATP (361), suggesting a multifunctional role of ATP as an energy currency, a drug efflux facilitator, and a signal transducer for cell survival and tumor migration/invasion.
Cancer is a complex disease and self-regulation of differentiation, growth, and expansion has allowed cancer cells to succeed biologically over normal cells (266, 362). This review summarizes existing information about the role of purinergic signaling in the attainment and preservation of cancer cell phenotype. Purinergic signaling in the TME exerts autocrine–paracrine actions to alter energy metabolism to facilitate cell survival, proliferation, migration, immunosuppression, and drug resistance in cancer cells. exATP is an emerging inducer and regulator of drug resistance through EMT and CSCs in cancer cells, and thus, targeting exATP will improve drug sensitivity of cancer cells to chemotherapeutic agents (363). Clinical interventions of purinergic signaling agonists and antagonists in various cancer patients are ongoing (Table 1), but are still in their infancy as very few agonists and antagonists are successful and steady in vivo (364). This may be due to the fact that most purinoceptors are ubiquitous and hence there is a problem of redundancy, and selectively targeting specific cell type remains a challenge.
Thus, there is still a lot unknown about purinergic signaling. For example, why do dual actions of exATP and purinoceptors occur in both normal and malignant cells? What is an apparent function of various purinoceptors and the concrete effects mediated by purinoceptors? What is the cell-specific location of ectonucleotidase expression in a tumor and is this expression stromal or in the immune cells? Furthermore, what is the most effective route of administration of ATP or purinoceptor-selective agonists or antagonists in humans, which best imitate the current administration procedure for anti-cancer drugs? Thus, further understanding of purinergic signaling and its interactions with other oncogenic signaling systems is the need of the hour.
JK conceptualized the manuscript, reviewed the literature and wrote the manuscript. SD did the literature search. All authors contributed to the article and approved the submitted version.
The authors declare that the research was conducted in the absence of any commercial or financial relationships that could be construed as a potential conflict of interest.
All claims expressed in this article are solely those of the authors and do not necessarily represent those of their affiliated organizations, or those of the publisher, the editors and the reviewers. Any product that may be evaluated in this article, or claim that may be made by its manufacturer, is not guaranteed or endorsed by the publisher.
1. Singh MS, Tammam SN, Shetab Boushehri MA, Lamprecht A. MDR in cancer: Addressing the underlying cellular alterations with the use of nanocarriers. Pharmacol Modul Tumor Microenviron (2017) 126:2–30. doi: 10.1016/j.phrs.2017.07.023
2. Chakravarthi BVSK, Nepal S, Varambally S. Genomic and epigenomic alterations in cancer. Am J Pathol (2016) 186:1724–35. doi: 10.1016/j.ajpath.2016.02.023
3. Robey RB, Weisz J, Kuemmerle NB, Salzberg AC, Berg A, Brown DG, et al. Metabolic reprogramming and dysregulated metabolism: cause, consequence and/or enabler of environmental carcinogenesis? Carcinogenesis (2015) 36 Suppl 1:S203–231. doi: 10.1093/carcin/bgv037
4. Chen X, Qian Y, Wu S. The warburg effect: evolving interpretations of an established concept. Free Radic Biol Med (2015) 79:253–63. doi: 10.1016/j.freeradbiomed.2014.08.027
5. Liberti MV, Locasale JW. The warburg effect: How does it benefit cancer cells? Trends Biochem Sci (2016) 41:211–8. doi: 10.1016/j.tibs.2015.12.001
6. Warburg O. The metabolism of carcinoma Cells1. J Cancer Res (1925) 9:148–63. doi: 10.1158/jcr.1925.148
7. Israël M, Schwartz L. The metabolic advantage of tumor cells. Mol Cancer (2011) 10:70. doi: 10.1186/1476-4598-10-70
8. Schwartz L, Supuran CT, Alfarouk KO. The warburg effect and the hallmarks of cancer. Anticancer Agents Med Chem (2017) 17:164–70. doi: 10.2174/1871520616666161031143301
9. Cronstein BN, Kramer SB, Weissmann G, Hirschhorn R. Adenosine: a physiological modulator of superoxide anion generation by human neutrophils. J Exp Med (1983) 158:1160–77. doi: 10.1084/jem.158.4.1160
10. Chagoya de Sánchez V. Circadian variations of adenosine and of its metabolism. could adenosine be a molecular oscillator for circadian rhythms? Can J Physiol Pharmacol (1995) 73:339–55. doi: 10.1139/y95-044
12. Drury AN, Szent-Györgyi A. The physiological activity of adenine compounds with especial reference to their action upon the mammalian heart. J Physiol (1929) 68:213–37. doi: 10.1113/jphysiol.1929.sp002608
13. Kushmerick MJ. From crossbridges to metabolism: System biology for energetics. In: Sugi H, editor. Sliding filament mechanism in muscle contraction. Boston, MA: Springer US (2005). p. 171–82.
14. Burnstock G. Purinergic signalling. Br J Pharmacol (2006) 147 Suppl 1:S172–181. doi: 10.1038/sj.bjp.0706429
15. Abbracchio MP, Burnstock G, Boeynaems J-M, Barnard EA, Boyer JL, Kennedy C, et al. International union of pharmacology LVIII: update on the P2Y G protein-coupled nucleotide receptors: from molecular mechanisms and pathophysiology to therapy. Pharmacol Rev (2006) 58:281–341. doi: 10.1124/pr.58.3.3
16. Ostrom RS, Gregorian C, Insel PA. Cellular release of and response to ATP as key determinants of the set-point of signal transduction pathways. J Biol Chem (2000) 275:11735–9. doi: 10.1074/jbc.275.16.11735
17. Zimmermann H. Extracellular ATP and other nucleotides-ubiquitous triggers of intercellular messenger release. Purinergic Signal (2016) 12:25–57. doi: 10.1007/s11302-015-9483-2
18. Verkhratsky A, Burnstock G. Biology of purinergic signalling: Its ancient evolutionary roots, its omnipresence and its multiple functional significance. BioEssays News Rev Mol Cell Dev Biol (2014) 36:697–705. doi: 10.1002/bies.201400024
19. Burnstock G, Knight GE. Cellular distribution and functions of P2 receptor subtypes in different systems. Int Rev Cytol (2004) 240:31–304. doi: 10.1016/S0074-7696(04)40002-3
20. Burnstock G. Pathophysiology and therapeutic potential of purinergic signaling. Pharmacol Rev (2006) 58:58–86. doi: 10.1124/pr.58.1.5
21. Abbracchio MP, Burnstock G. Purinergic signalling: Pathophysiological roles. Jpn J Pharmacol (1998) 78:113–45. doi: 10.1254/jjp.78.113
22. Burnstock G, Verkhratsky A. Long-term (trophic) purinergic signalling: purinoceptors control cell proliferation, differentiation and death. Cell Death Dis (2010) 1:e9. doi: 10.1038/cddis.2009.11
23. Burnstock G. Purinergic signaling and vascular cell proliferation and death. Arterioscler Thromb Vasc Biol (2002) 22:364–73. doi: 10.1161/hq0302.105360
24. Bodin P, Burnstock G. Purinergic signalling: ATP release. Neurochem Res (2001) 26:959–69. doi: 10.1023/a:1012388618693
25. Burnstock G. Physiology and pathophysiology of purinergic neurotransmission. Physiol Rev (2007) 87:659–797. doi: 10.1152/physrev.00043.2006
26. Lazarowski ER, Sesma JI, Seminario-Vidal L, Kreda SM. Molecular mechanisms of purine and pyrimidine nucleotide release. Adv Pharmacol San Diego Calif (2011) 61:221–61. doi: 10.1016/B978-0-12-385526-8.00008-4
27. Yegutkin GG. Enzymes involved in metabolism of extracellular nucleotides and nucleosides: functional implications and measurement of activities. Crit Rev Biochem Mol Biol (2014) 49:473–97. doi: 10.3109/10409238.2014.953627
28. Yegutkin GG. Nucleotide- and nucleoside-converting ectoenzymes: Important modulators of purinergic signalling cascade. Biochim Biophys Acta (2008) 1783:673–94. doi: 10.1016/j.bbamcr.2008.01.024
29. Zimmermann H, Mishra S, Shukla V. Ecto-nucleotidases, molecular properties and functional impact. Real Acad Nac Farm (2009), 73:537–66.
30. Kukulski F, Lévesque SA, Sévigny J. Impact of ectoenzymes on p2 and p1 receptor signaling. Adv Pharmacol San Diego Calif (2011) 61:263–99. doi: 10.1016/B978-0-12-385526-8.00009-6
31. Burnstock G. Handbook of experimental pharmacology. In: Purinergic and pyrimidinergic signalling I–molecular, nervous and urinogenitary system function, Berlin: Springer-Verlag; vol. volume 151/I. (2001).
32. Sperlágh B, Illes P. P2X7 receptor: an emerging target in central nervous system diseases. Trends Pharmacol Sci (2014) 35:537–47. doi: 10.1016/j.tips.2014.08.002
33. Rassendren F, Audinat E. Purinergic signaling in epilepsy. J Neurosci Res (2016) 94:781–93. doi: 10.1002/jnr.23770
34. Burnstock G. Purinergic signalling and disorders of the central nervous system. Nat Rev Drug Discovery (2008) 7:575–90. doi: 10.1038/nrd2605
35. Huang Z, Xie N, Illes P, Di Virgilio F, Ulrich H, Semyanov A, et al. From purines to purinergic signalling: molecular functions and human diseases. Signal Transduct Target Ther (2021) 6:162. doi: 10.1038/s41392-021-00553-z
36. Burnstock G. Therapeutic potential of purinergic signalling for diseases of the urinary tract. BJU Int (2011) 107:192–204. doi: 10.1111/j.1464-410X.2010.09926.x
37. Ryten M, Yang SY, Dunn PM, Goldspink G, Burnstock G. Purinoceptor expression in regenerating skeletal muscle in the mdx mouse model of muscular dystrophy and in satellite cell cultures. FASEB J Off Publ Fed Am Soc Exp Biol (2004) 18:1404–6. doi: 10.1096/fj.03-1175fje
38. Burnstock G. Purinergic receptors as future targets for treatment of functional GI disorders. Gut (2008) 57:1193–4. doi: 10.1136/gut.2008.151134
39. Burnstock G, Arnett TR. Nucleotides and Regulation of Bone Cell Function (1st ed.). CRC Press (2006). doi: 10.1201/9780849333682
40. Orriss IR, Burnstock G, Arnett TR. Purinergic signalling and bone remodelling. Curr Opin Pharmacol (2010) 10:322–30. doi: 10.1016/j.coph.2010.01.003
41. Erlinge D, Burnstock G. P2 receptors in cardiovascular regulation and disease. Purinergic Signal (2008) 4:1–20. doi: 10.1007/s11302-007-9078-7
42. Shirley D, Bailey M, Wildman S, Tam F, Unwin R. Inherited Disorders of Renal Salt Homeostasis. Journal: Seldin and Giebisch’s The Kidney. (2013) 2013:1213–40.
43. Taylor SRJ, Turner CM, Elliott JI, McDaid J, Hewitt R, Smith J, et al. P2X7 deficiency attenuates renal injury in experimental glomerulonephritis. J Am Soc Nephrol JASN (2009) 20:1275–81. doi: 10.1681/ASN.2008060559
44. Calvert RC, Khan MA, Thompson CS, Mikhailidis DP, Burnstock G. A functional study of purinergic signalling in the normal and pathological rabbit corpus cavernosum. BJU Int (2008) 101:1043–7. doi: 10.1111/j.1464-410X.2007.07385.x
45. Gur S, Kadowitz PJ, Abdel-Mageed AB, Kendirci M, Sikka SC, Burnstock G, et al. Management of erectile function by penile purinergic p2 receptors in the diabetic rat. J Urol (2009) 181:2375–82. doi: 10.1016/j.juro.2009.01.002
46. Burnstock G, Di Virgilio F. Purinergic signalling and cancer. Purinergic Signal (2013) 9:491–540. doi: 10.1007/s11302-013-9372-5
47. Shabbir M, Burnstock G. Purinergic receptor-mediated effects of adenosine 5’-triphosphate in urological malignant diseases. Int J Urol Off J Jpn Urol Assoc (2009) 16:143–50. doi: 10.1111/j.1442-2042.2008.02207.x
48. Shabbir M, Thompson C, Jarmulowiczc M, Mikhailidis D, Burnstock G. Effect of extracellular ATP on the growth of hormone-refractory prostate cancer in vivo. BJU Int (2008) 102:108–12. doi: 10.1111/j.1464-410X.2008.07578.x
49. Stagg J, Smyth MJ. Extracellular adenosine triphosphate and adenosine in cancer. Oncogene (2010) 29:5346–58. doi: 10.1038/onc.2010.292
50. White N, Burnstock G. P2 receptors and cancer. Trends Pharmacol Sci (2006) 27:211–7. doi: 10.1016/j.tips.2006.02.004
51. White N, Knight GE, Butler PEM, Burnstock G. An in vivo model of melanoma: treatment with ATP. Purinergic Signal (2009) 5:327–33. doi: 10.1007/s11302-009-9156-0
52. Bartlett R, Stokes L, Sluyter R. The P2X7 receptor channel: recent developments and the use of P2X7 antagonists in models of disease. Pharmacol Rev (2014) 66:638–75. doi: 10.1124/pr.113.008003
53. Burnstock G. Purinergic mechanisms and pain–an update. Eur J Pharmacol (2013) 716:24–40. doi: 10.1016/j.ejphar.2013.01.078
54. Burnstock G. Purinergic receptors. J Theor Biol (1976) 62:491–503. doi: 10.1016/0022-5193(76)90133-8
55. Praetorius HA, Leipziger J. Intrarenal purinergic signaling in the control of renal tubular transport. Annu Rev Physiol (2010) 72:377–93. doi: 10.1146/annurev-physiol-021909-135825
56. Burnstock G, Ulrich H. Purinergic signaling in embryonic and stem cell development. Cell Mol Life Sci CMLS (2011) 68:1369–94. doi: 10.1007/s00018-010-0614-1
57. Delic J, Zimmermann H. Nucleotides affect neurogenesis and dopaminergic differentiation of mouse fetal midbrain-derived neural precursor cells. Purinergic Signal (2010) 6:417–28. doi: 10.1007/s11302-010-9206-7
58. Trujillo CA, Schwindt TT, Martins AH, Alves JM, Mello LE, Ulrich H. Novel perspectives of neural stem cell differentiation: from neurotransmitters to therapeutics. Cytom Part J Int Soc Anal Cytol (2009) 75:38–53. doi: 10.1002/cyto.a.20666
59. Burnstock G. A basis for distinguishing two types of purinergic receptor. In: Straub R. W., Bolis L. (eds) Cell Membrane Receptors for Drugs and Hormones: A Multidisciplinary Approach. New York: Raven Press. pp. 107–118.
60. Burnstock G, Krügel U, Abbracchio MP, Illes P. Purinergic signalling: from normal behaviour to pathological brain function. Prog Neurobiol (2011) 95:229–74. doi: 10.1016/j.pneurobio.2011.08.006
61. Londos C, Cooper DM, Wolff J. Subclasses of external adenosine receptors. Proc Natl Acad Sci U.S.A. (1980) 77:2551–4. doi: 10.1073/pnas.77.5.2551
62. van Calker D, Müller M, Hamprecht B. Adenosine regulates via two different types of receptors, the accumulation of cyclic AMP in cultured brain cells. J Neurochem (1979) 33:999–1005. doi: 10.1111/j.1471-4159.1979.tb05236.x
63. Fredholm BB, IJzerman AP, Jacobson KA, Klotz KN, Linden J. International union of pharmacology. XXV. nomenclature and classification of adenosine receptors. Pharmacol Rev (2001) 53:527–52.
64. Burnstock G, Kennedy C. Is there a basis for distinguishing two types of P2-purinoceptor? Gen Pharmacol (1985) 16:433–40. doi: 10.1016/0306-3623(85)90001-1
65. Lustig KD, Shiau AK, Brake AJ, Julius D. Expression cloning of an ATP receptor from mouse neuroblastoma cells. Proc Natl Acad Sci U.S.A. (1993) 90:5113–7. doi: 10.1073/pnas.90.11.5113
66. Webb TE, Simon J, Krishek BJ, Bateson AN, Smart TG, King BF, et al. Cloning and functional expression of a brain G-protein-coupled ATP receptor. FEBS Lett (1993) 324:219–25. doi: 10.1016/0014-5793(93)81397-i
67. Brake AJ, Wagenbach MJ, Julius D. New structural motif for ligand-gated ion channels defined by an ionotropic ATP receptor. Nature (1994) 371:519–23. doi: 10.1038/371519a0
68. Valera S, Hussy N, Evans RJ, Adami N, North RA, Surprenant A, et al. A new class of ligand-gated ion channel defined by P2x receptor for extracellular ATP. Nature (1994) 371:516–9. doi: 10.1038/371516a0
69. North RA. Molecular physiology of P2X receptors. Physiol Rev (2002) 82:1013–67. doi: 10.1152/physrev.00015.2002
71. Eltzschig HK, Sitkovsky MV, Robson SC. Purinergic signaling during inflammation. N Engl J Med (2012) 367:2322–33. doi: 10.1056/NEJMra1205750
72. Campos-Contreras ADR, Díaz-Muñoz M, Vázquez-Cuevas FG. Purinergic signaling in the hallmarks of cancer. Cells (2020) 9:1612. doi: 10.3390/cells9071612
73. Volonté C, D’Ambrosi N. Membrane compartments and purinergic signalling: the purinome, a complex interplay among ligands, degrading enzymes, receptors and transporters. FEBS J (2009) 276:318–29. doi: 10.1111/j.1742-4658.2008.06793.x
74. Libert F, Parmentier M, Lefort A, Dinsart C, Van Sande J, Maenhaut C, et al. Selective amplification and cloning of four new members of the G protein-coupled receptor family. Science (1989) 244:569–72. doi: 10.1126/science.2541503
75. Zhou QY, Li C, Olah ME, Johnson RA, Stiles GL, Civelli O. Molecular cloning and characterization of an adenosine receptor: The A3 adenosine receptor. Proc Natl Acad Sci U.S.A. (1992) 89:7432–6. doi: 10.1073/pnas.89.16.7432
76. Kumral A, Tuzun F, Yesilirmak DC, Duman N, Ozkan H. Genetic basis of apnoea of prematurity and caffeine treatment response: role of adenosine receptor polymorphisms: genetic basis of apnoea of prematurity. Acta Paediatr Oslo Nor 1992 (2012) 101:e299–303. doi: 10.1111/j.1651-2227.2012.02664.x
77. Fredholm BB, IJzerman AP, Jacobson KA, Linden J, Müller CE. International union of basic and clinical pharmacology. LXXXI. nomenclature and classification of adenosine receptors–an update. Pharmacol Rev (2011) 63:1–34. doi: 10.1124/pr.110.003285
78. Reshkin SJ, Guerra L, Bagorda A, Debellis L, Cardone R, Li AH, et al. Activation of A3 adenosine receptor induces calcium entry and chloride secretion in A6 cells. J Membr Biol (2000) 178:103–13. doi: 10.1007/s002320010018
79. Ledent C, Vaugeois JM, Schiffmann SN, Pedrazzini T, El Yacoubi M, Vanderhaeghen JJ, et al. Aggressiveness, hypoalgesia and high blood pressure in mice lacking the adenosine A2a receptor. Nature (1997) 388:674–8. doi: 10.1038/41771
80. Sun D, Samuelson LC, Yang T, Huang Y, Paliege A, Saunders T, et al. Mediation of tubuloglomerular feedback by adenosine: evidence from mice lacking adenosine 1 receptors. Proc Natl Acad Sci U.S.A. (2001) 98:9983–8. doi: 10.1073/pnas.171317998
81. Dastjerdi MN, Rarani MZ, Valiani A, Mahmoudieh M. The effect of adenosine A1 receptor agonist and antagonist on p53 and caspase 3, 8, and 9 expression and apoptosis rate in MCF-7 breast cancer cell line. Res Pharm Sci (2016) 11:303–10. doi: 10.4103/1735-5362.189301
82. Zhou Y, Tong L, Chu X, Deng F, Tang J, Tang Y, et al. The adenosine A1 receptor antagonist DPCPX inhibits tumor progression via the ERK/JNK pathway in renal cell carcinoma. Cell Physiol Biochem Int J Exp Cell Physiol Biochem Pharmacol (2017) 43:733–42. doi: 10.1159/000481557
83. Hosseinzadeh H, Jaafari MR, Shamsara J. Selective inhibitory effect of adenosine A1 receptor agonists on the proliferation of human tumor cell lines. Iran BioMed J (2008) 12:203–8.
84. O’Donnell EF 3rd, Jang HS, Liefwalker DF, Kerkvliet NI, Kolluri SK. Discovery and mechanistic characterization of a select modulator of AhR-regulated transcription (SMAhRT) with anti-cancer effects. Apoptosis Int J Program Cell Death (2021) 26:307–22. doi: 10.1007/s10495-021-01666-0
85. Bednarska-Szczepaniak K, Krzyżanowski D, Klink M, Nowak M. Adenosine analogues as opposite modulators of the cisplatin resistance of ovarian cancer cells. Anticancer Agents Med Chem (2019) 19:473–86. doi: 10.2174/1871520619666190118113201
86. Fiebich BL, Biber K, Gyufko K, Berger M, Bauer J, van Calker D. Adenosine A2b receptors mediate an increase in interleukin (IL)-6 mRNA and IL-6 protein synthesis in human astroglioma cells. J Neurochem (1996) 66:1426–31. doi: 10.1046/j.1471-4159.1996.66041426.x
87. Mediavilla-Varela M, Luddy K, Noyes D, Khalil FK, Neuger AM, Soliman H, et al. Antagonism of adenosine A2A receptor expressed by lung adenocarcinoma tumor cells and cancer associated fibroblasts inhibits their growth. Cancer Biol Ther (2013) 14:860–8. doi: 10.4161/cbt.25643
88. Tang J, Zou Y, Li L, Lu F, Xu H, Ren P, et al. BAY 60-6583 enhances the antitumor function of chimeric antigen receptor-modified T cells independent of the adenosine A2b receptor. Front Pharmacol (2021) 12:619800. doi: 10.3389/fphar.2021.619800
89. Giacomelli C, Daniele S, Romei C, Tavanti L, Neri T, Piano I, et al. The A(2B) adenosine receptor modulates the epithelial- mesenchymal transition through the balance of cAMP/PKA and MAPK/ERK pathway activation in human epithelial lung cells. Front Pharmacol (2018) 9:54. doi: 10.3389/fphar.2018.00054
90. Mølck C, Ryall J, Failla LM, Coates JL, Pascussi J-M, Heath JK, et al. The A(2b) adenosine receptor antagonist PSB-603 promotes oxidative phosphorylation and ROS production in colorectal cancer cells via adenosine receptor-independent mechanism. Cancer Lett (2016) 383:135–43. doi: 10.1016/j.canlet.2016.09.018
91. Wilkat M, Bast H, Drees R, Dünser J, Mahr A, Azoitei N, et al. Adenosine receptor 2B activity promotes autonomous growth, migration as well as vascularization of head and neck squamous cell carcinoma cells. Int J Cancer (2020) 147:202–17. doi: 10.1002/ijc.32835
92. Yi Y, Zhou Y, Chu X, Zheng X, Fei D, Lei J, et al. Blockade of adenosine A2b receptor reduces tumor growth and migration in renal cell carcinoma. J Cancer (2020) 11:421–31. doi: 10.7150/jca.31245
93. Iannone R, Miele L, Maiolino P, Pinto A, Morello S. Blockade of A2b adenosine receptor reduces tumor growth and immune suppression mediated by myeloid-derived suppressor cells in a mouse model of melanoma. Neoplasia N Y N (2013) 15:1400–9. doi: 10.1593/neo.131748
94. Malki WH, Gouda AM, Ali HEA, Al-Rousan R, Samaha D, Abdalla AN, et al. Structural-based design, synthesis, and antitumor activity of novel alloxazine analogues with potential selective kinase inhibition. Eur J Med Chem (2018) 152:31–52. doi: 10.1016/j.ejmech.2018.04.029
95. Kanno T, Nakano T, Fujita Y, Gotoh A, Nishizaki T. Adenosine induces apoptosis in SBC-3 human lung cancer cells through A(3) adenosine receptor-dependent AMID upregulation. Cell Physiol Biochem Int J Exp Cell Physiol Biochem Pharmacol (2012) 30:666–77. doi: 10.1159/000341447
96. Madi L, Bar-Yehuda S, Barer F, Ardon E, Ochaion A, Fishman P. A3 adenosine receptor activation in melanoma cells: association between receptor fate and tumor growth inhibition. J Biol Chem (2003) 278:42121–30. doi: 10.1074/jbc.M301243200
97. Blad CC, von Frijtag Drabbe Künzel JK, de Vries H, Mulder-Krieger T, Bar-Yehuda S, Fishman P, et al. Putative role of the adenosine A(3) receptor in the antiproliferative action of n (6)-(2-isopentenyl)adenosine. Purinergic Signal (2011) 7:453–62. doi: 10.1007/s11302-011-9244-9
98. Sigdel I, Gupta N, Faizee F, Khare VM, Tiwari AK, Tang Y. Biomimetic microfluidic platforms for the assessment of breast cancer metastasis. Front Bioeng Biotechnol (2021) 9:633671. doi: 10.3389/fbioe.2021.633671
99. Rocha R, Torres Á, Ojeda K, Uribe D, Rocha D, Erices J, et al. The adenosine A3 receptor regulates differentiation of glioblastoma stem-like cells to endothelial cells under hypoxia. Int J Mol Sci (2018) 19:1228. doi: 10.3390/ijms19041228
100. Scarpellino G, Genova T, Quarta E, Distasi C, Dionisi M, Fiorio Pla A, et al. P2X purinergic receptors are multisensory detectors for micro-environmental stimuli that control migration of tumoral endothelium. Cancers (2022) 14:2743. doi: 10.3390/cancers14112743
101. Zhang X, Shi S, Su Y, Yang X, He S, Yang X, et al. Suramin and NF449 are IP5K inhibitors that disrupt inositol hexakisphosphate-mediated regulation of cullin-RING ligase and sensitize cancer cells to MLN4924/pevonedistat. J Biol Chem (2020) 295:10281–92. doi: 10.1074/jbc.RA120.014375
102. Cossu F, Sorrentino L, Fagnani E, Zaffaroni M, Milani M, Giorgino T, et al. Computational and experimental characterization of NF023, a candidate anticancer compound inhibiting cIAP2/TRAF2 assembly. J Chem Inf Model (2020) 60:5036–44. doi: 10.1021/acs.jcim.0c00518
103. Ahmed NM, Youns M, Soltan MK, Said AM. Design, synthesis, molecular modelling, and biological evaluation of novel substituted pyrimidine derivatives as potential anticancer agents for hepatocellular carcinoma. J Enzyme Inhib Med Chem (2019) 34:1110–20. doi: 10.1080/14756366.2019.1612889
104. Kaan TKY, Yip PK, Patel S, Davies M, Marchand F, Cockayne DA, et al. Systemic blockade of P2X3 and P2X2/3 receptors attenuates bone cancer pain behaviour in rats. Brain (2010) 133:2549–64. doi: 10.1093/brain/awq194
105. Hansen RR, Nasser A, Falk S, Baldvinsson SB, Ohlsson PH, Bahl JMC, et al. Chronic administration of the selective P2X3, P2X2/3 receptor antagonist, a-317491, transiently attenuates cancer-induced bone pain in mice. Eur J Pharmacol (2012) 688:27–34. doi: 10.1016/j.ejphar.2012.05.008
106. Maynard JP, Lu J, Vidal I, Hicks J, Mummert L, Ali T, et al. P2X4 purinergic receptors offer a therapeutic target for aggressive prostate cancer. J Pathol (2022) 256:149–63. doi: 10.1002/path.5815
107. He J, Zhou Y, Arredondo Carrera HM, Sprules A, Neagu R, Zarkesh SA, et al. Inhibiting the P2X4 receptor suppresses prostate cancer growth In vitro and In vivo, suggesting a potential clinical target. Cells (2020) 9:2511. doi: 10.3390/cells9112511
108. Xia J, Yu X, Tang L, Li G, He T. P2X7 receptor stimulates breast cancer cell invasion and migration via the AKT pathway. Oncol Rep (2015) 34:103–10. doi: 10.3892/or.2015.3979
109. Liu B-H, Yuan T-M, Huang C-J, Hsu D-T, Chen S-W, Hsiao N-W, et al. DNA Repair proteins as the targets for paroxetine to induce cytotoxicity in gastric cancer cell AGS. Am J Cancer Res (2022) 12:1465–83.
110. Han Y, Bai C, He X-M, Ren Q-L. P2X7 receptor involved in antitumor activity of atractylenolide I in human cervical cancer cells. Purinergic Signal (2022). doi: 10.1007/s11302-022-09854-6
111. Zhang Y, Cheng H, Li W, Wu H, Yang Y. Highly-expressed P2X7 receptor promotes growth and metastasis of human HOS/MNNG osteosarcoma cells via PI3K/Akt/GSK3β/β-catenin and mTOR/HIF1α/VEGF signaling. Int J Cancer (2019) 145:1068–82. doi: 10.1002/ijc.32207
112. Lee K-H. CaMKII inhibitor KN-62 blunts tumor response to hypoxia by inhibiting HIF-1α in hepatoma cells. Korean J Physiol Pharmacol Off J Korean Physiol Soc Korean Soc Pharmacol (2010) 14:331–6. doi: 10.4196/kjpp.2010.14.5.331
113. Amoroso F, Capece M, Rotondo A, Cangelosi D, Ferracin M, Franceschini A, et al. The P2X7 receptor is a key modulator of the PI3K/GSK3β/VEGF signaling network: evidence in experimental neuroblastoma. Oncogene (2015) 34:5240–51. doi: 10.1038/onc.2014.444
114. Zhang Y, Li F, Wang L, Lou Y. A438079 affects colorectal cancer cell proliferation, migration, apoptosis, and pyroptosis by inhibiting the P2X7 receptor. Biochem Biophys Res Commun (2021) 558:147–53. doi: 10.1016/j.bbrc.2021.04.076
115. Kan LK, Seneviratne S, Drummond KJ, Williams DA, O’Brien TJ, Monif M. P2X7 receptor antagonism inhibits tumour growth in human high-grade gliomas. Purinergic Signal (2020) 16:327–36. doi: 10.1007/s11302-020-09705-2
116. Matyśniak D, Chumak V, Nowak N, Kukla A, Lehka L, Oslislok M, et al. P2X7 receptor: the regulator of glioma tumor development and survival. Purinergic Signal (2022) 18:135–54. doi: 10.1007/s11302-021-09834-2
117. Le HTT, Murugesan A, Ramesh T, Yli-Harja O, Konda Mani S, Kandhavelu M. Molecular interaction of HIC, an agonist of P2Y1 receptor, and its role in prostate cancer apoptosis. Int J Biol Macromol (2021) 189:142–50. doi: 10.1016/j.ijbiomac.2021.08.103
118. Puchałowicz K, Tarnowski M, Tkacz M, Chlubek D, Kłos P, Dziedziejko V. Extracellular adenine nucleotides and adenosine modulate the growth and survival of THP-1 leukemia cells. Int J Mol Sci (2020) 21:4425. doi: 10.3390/ijms21124425
119. Wei Q, Costanzi S, Liu Q-Z, Gao Z-G, Jacobson KA. Activation of the P2Y1 receptor induces apoptosis and inhibits proliferation of prostate cancer cells. Biochem Pharmacol (2011) 82:418–25. doi: 10.1016/j.bcp.2011.05.013
120. Yokdang N, Nordmeier S, Speirs K, Burkin HR, Buxton ILO. Blockade of extracellular NM23 or its endothelial target slows breast cancer growth and metastasis. Integr Cancer Sci Ther (2015) 2:192–200. doi: 10.15761/icst.1000139
121. Chadet S, Jelassi B, Wannous R, Angoulvant D, Chevalier S, Besson P, et al. The activation of P2Y2 receptors increases MCF-7 breast cancer cells migration through the MEK-ERK1/2 signalling pathway. Carcinogenesis (2014) 35:1238–47. doi: 10.1093/carcin/bgt493
122. Oka K, Suzuki T, Onodera Y, Miki Y, Takagi K, Nagasaki S, et al. Nudix-type motif 2 in human breast carcinoma: a potent prognostic factor associated with cell proliferation. Int J Cancer (2011) 128:1770–82. doi: 10.1002/ijc.25505
123. Woods LT, Jasmer KJ, Muñoz Forti K, Shanbhag VC, Camden JM, Erb L, et al. P2Y(2) receptors mediate nucleotide-induced EGFR phosphorylation and stimulate proliferation and tumorigenesis of head and neck squamous cell carcinoma cell lines. Oral Oncol (2020) 109:104808. doi: 10.1016/j.oraloncology.2020.104808
124. Placet M, Arguin G, Molle CM, Babeu J-P, Jones C, Carrier JC, et al. The G protein-coupled P2Y6 receptor promotes colorectal cancer tumorigenesis by inhibiting apoptosis. Biochim Biophys Acta BBA - Mol Basis Dis (2018) 1864:1539–51. doi: 10.1016/j.bbadis.2018.02.008
125. Gendaszewska-Darmach E, Szustak M. Thymidine 5’-o-monophosphorothioate induces HeLa cell migration by activation of the P2Y6 receptor. Purinergic Signal (2016) 12:199–209. doi: 10.1007/s11302-015-9492-1
126. Ma X, Pan X, Wei Y, Tan B, Yang L, Ren H, et al. Chemotherapy-induced uridine diphosphate release promotes breast cancer metastasis through P2Y6 activation. Oncotarget (2016) 7:29036–50. doi: 10.18632/oncotarget.8664
127. Liu X, Riquelme MA, Tian Y, Zhao D, Acosta FM, Gu S, et al. ATP inhibits breast cancer migration and bone metastasis through down-regulation of CXCR4 and purinergic receptor P2Y11. Cancers (2021) 13:4293. doi: 10.3390/cancers13174293
128. Khalid M, Brisson L, Tariq M, Hao Y, Guibon R, Fromont G, et al. Carcinoma-specific expression of P2Y11 receptor and its contribution in ATP-induced purinergic signalling and cell migration in human hepatocellular carcinoma cells. Oncotarget (2017) 8:37278–90. doi: 10.18632/oncotarget.16191
129. Liu C-L, Cheng S-P, Chen M-J, Lin C-H, Chen S-N, Kuo Y-H, et al. Quinolinate phosphoribosyltransferase promotes invasiveness of breast cancer through myosin light chain phosphorylation. Front Endocrinol (2020) 11:621944. doi: 10.3389/fendo.2020.621944
130. Palacios-Acedo AL, Mezouar S, Mège D, Crescence L, Dubois C, Panicot-Dubois L. P2RY12-inhibitors reduce cancer-associated thrombosis and tumor growth in pancreatic cancers. Front Oncol (2021) 11:704945. doi: 10.3389/fonc.2021.704945
131. Wang X, Liu R, Zhu W, Chu H, Yu H, Wei P, et al. UDP-Glucose accelerates SNAI1 mRNA decay and impairs lung cancer metastasis. Nature (2019) 571:127–31. doi: 10.1038/s41586-019-1340-y
132. Tang M, Etokidem E, Lai K. The leloir pathway of galactose metabolism - A novel therapeutic target for hepatocellular carcinoma. Anticancer Res (2016) 36:6265–71. doi: 10.21873/anticanres.11221
133. Jacobson KA, Gao Z-G. Adenosine receptors as therapeutic targets. Nat Rev Drug Discovery (2006) 5:247–64. doi: 10.1038/nrd1983
134. Nicke A, Bäumert HG, Rettinger J, Eichele A, Lambrecht G, Mutschler E, et al. P2X1 and P2X3 receptors form stable trimers: a novel structural motif of ligand-gated ion channels. EMBO J (1998) 17:3016–28. doi: 10.1093/emboj/17.11.3016
135. Stelmashenko O, Lalo U, Yang Y, Bragg L, North RA, Compan V. Activation of trimeric P2X2 receptors by fewer than three ATP molecules. Mol Pharmacol (2012) 82:760–6. doi: 10.1124/mol.112.080903
136. Khakh BS, Burnstock G, Kennedy C, King BF, North RA, Séguéla P, et al. International union of pharmacology. XXIV. current status of the nomenclature and properties of P2X receptors and their subunits. Pharmacol Rev (2001) 53:107–18.
137. Ding Y, Cesare P, Drew L, Nikitaki D, Wood JN. ATP. P2X receptors and pain pathways. J Auton Nerv Syst (2000) 81:289–94. doi: 10.1016/s0165-1838(00)00131-4
138. Robertson SJ, Ennion SJ, Evans RJ, Edwards FA. Synaptic P2X receptors. Curr Opin Neurobiol (2001) 11:378–86. doi: 10.1016/s0959-4388(00)00222-1
139. Dunn PM, Zhong Y, Burnstock G. P2X receptors in peripheral neurons. Prog Neurobiol (2001) 65:107–34. doi: 10.1016/s0301-0082(01)00005-3
140. Kim M, Jiang LH, Wilson HL, North RA, Surprenant A. Proteomic and functional evidence for a P2X7 receptor signalling complex. EMBO J (2001) 20:6347–58. doi: 10.1093/emboj/20.22.6347
141. Alves LA, da Silva JHM, Ferreira DNM, Fidalgo-Neto AA, Teixeira PCN, de Souza CAM, et al. Structural and molecular modeling features of P2X receptors. Int J Mol Sci (2014) 15:4531–49. doi: 10.3390/ijms15034531
142. Rokic MB, Stojilkovic SS. Two open states of P2X receptor channels. Front Cell Neurosci (2013) 7:215. doi: 10.3389/fncel.2013.00215
143. Newbolt A, Stoop R, Virginio C, Surprenant A, North RA, Buell G, et al. Membrane topology of an ATP-gated ion channel (P2X receptor). J Biol Chem (1998) 273:15177–82. doi: 10.1074/jbc.273.24.15177
144. Torres GE, Egan TM, Voigt MM. N-linked glycosylation is essential for the functional expression of the recombinant P2X2 receptor. Biochemistry (1998) 37:14845–51. doi: 10.1021/bi981209g
145. Torres GE, Egan TM, Voigt MM. Topological analysis of the ATP-gated ionotropic [correction of ionotrophic] P2X2 receptor subunit. FEBS Lett (1998) 425:19–23. doi: 10.1016/s0014-5793(98)00179-3
146. Heymann G, Dai J, Li M, Silberberg SD, Zhou H-X, Swartz KJ. Inter- and intrasubunit interactions between transmembrane helices in the open state of P2X receptor channels. Proc Natl Acad Sci U.S.A. (2013) 110:E4045–4054. doi: 10.1073/pnas.1311071110
147. Burnstock G. Purine and purinergic receptors. Brain Neurosci Adv (2018) 2:2398212818817494. doi: 10.1177/2398212818817494
148. Baconguis I, Gouaux E. Structural plasticity and dynamic selectivity of acid-sensing ion channel-spider toxin complexes. Nature (2012) 489:400–5. doi: 10.1038/nature11375
149. Hattori M, Gouaux E. Molecular mechanism of ATP binding and ion channel activation in P2X receptors. Nature (2012) 485:207–12. doi: 10.1038/nature11010
150. Lara R, Adinolfi E, Harwood CA, Philpott M, Barden JA, Di Virgilio F, et al. P2X7 in cancer: From molecular mechanisms to therapeutics. Front Pharmacol (2020) 11:793. doi: 10.3389/fphar.2020.00793
151. Pegoraro A, De Marchi E, Adinolfi E. P2X7 variants in oncogenesis. Cells (2021) 10:189. doi: 10.3390/cells10010189
152. Cheewatrakoolpong B, Gilchrest H, Anthes JC, Greenfeder S. Identification and characterization of splice variants of the human P2X7 ATP channel. Biochem Biophys Res Commun (2005) 332:17–27. doi: 10.1016/j.bbrc.2005.04.087
153. Arnaud-Sampaio VF, Rabelo ILA, Ulrich H, Lameu C. The P2X7 receptor in the maintenance of cancer stem cells, chemoresistance and metastasis. Stem Cell Rev Rep (2020) 16:288–300. doi: 10.1007/s12015-019-09936-w
154. Di Virgilio F, Schmalzing G, Markwardt F. The elusive P2X7 macropore. Trends Cell Biol (2018) 28:392–404. doi: 10.1016/j.tcb.2018.01.005
155. Burnstock G. Chapter 18 - purinergic neurotransmission and nucleotide receptors. In: Robertson D, Biaggioni I, Burnstock G, Low PA, Paton JFR, editors. Primer on the autonomic nervous system, 3rd ed. San Diego: Academic Press (2012). p. 87–93. doi: 10.1016/B978-0-12-386525-0.00018-4
156. Coddou C, Yan Z, Obsil T, Huidobro-Toro JP, Stojilkovic SS. Activation and regulation of purinergic P2X receptor channels. Pharmacol Rev (2011) 63:641–83. doi: 10.1124/pr.110.003129
157. Samways DSK, Li Z, Egan TM. Principles and properties of ion flow in P2X receptors. Front Cell Neurosci (2014) 8:6. doi: 10.3389/fncel.2014.00006
158. Egan TM, Khakh BS. Contribution of calcium ions to P2X channel responses. J Neurosci Off J Soc Neurosci (2004) 24:3413–20. doi: 10.1523/JNEUROSCI.5429-03.2004
159. Khakh BS, Henderson G. Modulation of fast synaptic transmission by presynaptic ligand-gated cation channels. J Auton Nerv Syst (2000) 81:110–21. doi: 10.1016/s0165-1838(00)00111-9
160. Nörenberg W, Göbel I, Meyer A, Cox SL, Starke K, Trendelenburg AU. Stimulation of mouse cultured sympathetic neurons by uracil but not adenine nucleotides. Neuroscience (2001) 103:227–36. doi: 10.1016/s0306-4522(00)00547-9
161. Lamont C, Wier WG. Evoked and spontaneous purinergic junctional Ca2+ transients (jCaTs) in rat small arteries. Circ Res (2002) 91:454–6. doi: 10.1161/01.res.0000035060.98415.4b
162. Brain KL, Cuprian AM, Williams DJ, Cunnane TC. The sources and sequestration of Ca(2+) contributing to neuroeffector Ca(2+) transients in the mouse vas deferens. J Physiol (2003) 553:627–35. doi: 10.1113/jphysiol.2003.049734
163. Di Virgilio F. Purines, purinergic receptors, and cancer. Cancer Res (2012) 72:5441–7. doi: 10.1158/0008-5472.CAN-12-1600
164. Volonté C, Apolloni S, Skaper SD, Burnstock G. P2X7 receptors: channels, pores and more. CNS Neurol Disord Drug Targets (2012) 11:705–21. doi: 10.2174/187152712803581137
165. Caseley EA, Muench SP, Roger S, Mao H-J, Baldwin SA, Jiang L-H. Non-synonymous single nucleotide polymorphisms in the P2X receptor genes: Association with diseases, impact on receptor functions and potential use as diagnosis biomarkers. Int J Mol Sci (2014) 15:13344–71. doi: 10.3390/ijms150813344
166. Abbracchio MP, Boeynaems J-M, Barnard EA, Boyer JL, Kennedy C, Miras-Portugal MT, et al. Characterization of the UDP-glucose receptor (re-named here the P2Y14 receptor) adds diversity to the P2Y receptor family. Trends Pharmacol Sci (2003) 24:52–5. doi: 10.1016/S0165-6147(02)00038-X
167. Jacobson KA, Paoletta S, Katritch V, Wu B, Gao Z-G, Zhao Q, et al. Nucleotides acting at P2Y receptors: Connecting structure and function. Mol Pharmacol (2015) 88:220–30. doi: 10.1124/mol.114.095711
168. Schachter JB, Li Q, Boyer JL, Nicholas RA, Harden TK. Second messenger cascade specificity and pharmacological selectivity of the human P2Y1-purinoceptor. Br J Pharmacol (1996) 118:167–73. doi: 10.1111/j.1476-5381.1996.tb15381.x
169. Erb L, Weisman GA. Coupling of P2Y receptors to G proteins and other signaling pathways. Wiley Interdiscip Rev Membr Transp Signal (2012) 1:789–803. doi: 10.1002/wmts.62
170. White PJ, Webb TE, Boarder MR. Characterization of a Ca2+ response to both UTP and ATP at human P2Y11 receptors: Evidence for agonist-specific signaling. Mol Pharmacol (2003) 63:1356–63. doi: 10.1124/mol.63.6.1356
171. Soulet C, Sauzeau V, Plantavid M, Herbert J, Pacaud P, Payrastre B, et al. Gi-dependent and-independent mechanisms downstream of the P2Y12 ADP-receptor. J Thromb Haemost (2004) 2:135–46. doi: 10.1111/j.1538-7836.2004.00556.x
172. Marteau F, Le Poul E, Communi D, Communi D, Labouret C, Savi P, et al. Pharmacological characterization of the human P2Y13 receptor. Mol Pharmacol (2003) 64:104–12. doi: 10.1124/mol.64.1.104
173. Di Virgilio F, Adinolfi E. Extracellular purines, purinergic receptors and tumor growth. Oncogene (2017) 36:293–303. doi: 10.1038/onc.2016.206
174. Di Virgilio F, Sarti AC, Falzoni S, De Marchi E, Adinolfi E. Extracellular ATP and P2 purinergic signalling in the tumour microenvironment. Nat Rev Cancer (2018) 18:601–18. doi: 10.1038/s41568-018-0037-0
175. Gusovsky F, Daly JW, Yasumoto T, Rojas E. Differential effects of maitotoxin on ATP secretion and on phosphoinositide breakdown in rat pheochromocytoma cells. FEBS Lett (1988) 233:139–42. doi: 10.1016/0014-5793(88)81371-1
176. Pedersen S, Pedersen SF, Nilius B, Lambert IH, Hoffmann EK. Mechanical stress induces release of ATP from Ehrlich ascites tumor cells. Biochim Biophys Acta BBA - Biomembr (1999) 1416:271–84. doi: 10.1016/S0005-2736(98)00228-4
177. Takai E, Tsukimoto M, Harada H, Sawada K, Moriyama Y, Kojima S. Autocrine regulation of TGF-β1-induced cell migration by exocytosis of ATP and activation of P2 receptors in human lung cancer cells. J Cell Sci (2012) 125:5051–60. doi: 10.1242/jcs.104976
178. Pellegatti P, Raffaghello L, Bianchi G, Piccardi F, Pistoia V, Di Virgilio F. Increased level of extracellular ATP at tumor sites: in vivo imaging with plasma membrane luciferase. PloS One (2008) 3:e2599. doi: 10.1371/journal.pone.0002599
179. Synnestvedt K, Furuta GT, Comerford KM, Louis N, Karhausen J, Eltzschig HK, et al. Ecto-5’-nucleotidase (CD73) regulation by hypoxia-inducible factor-1 mediates permeability changes in intestinal epithelia. J Clin Invest (2002) 110:993–1002. doi: 10.1172/JCI15337
180. Poth JM, Brodsky K, Ehrentraut H, Grenz A, Eltzschig HK. Transcriptional control of adenosine signaling by hypoxia-inducible transcription factors during ischemic or inflammatory disease. J Mol Med Berl Ger (2013) 91:183–93. doi: 10.1007/s00109-012-0988-7
181. Hatfield SM, Kjaergaard J, Lukashev D, Schreiber TH, Belikoff B, Abbott R, et al. Immunological mechanisms of the antitumor effects of supplemental oxygenation. Sci Transl Med (2015) 7:277ra30–277ra30. doi: 10.1126/scitranslmed.aaa1260
182. Sitkovsky MV, Hatfield S, Abbott R, Belikoff B, Lukashev D, Ohta A. Hostile, hypoxia–A2-Adenosinergic tumor biology as the next barrier to overcome for tumor immunologists. Cancer Immunol Res (2014) 2:598–605. doi: 10.1158/2326-6066.CIR-14-0075
183. Allard B, Beavis PA, Darcy PK, Stagg J. Immunosuppressive activities of adenosine in cancer. Curr Opin Pharmacol (2016) 29:7–16. doi: 10.1016/j.coph.2016.04.001
184. Furlow PW, Zhang S, Soong TD, Halberg N, Goodarzi H, Mangrum C, et al. Mechanosensitive pannexin-1 channels mediate microvascular metastatic cell survival. Nat Cell Biol (2015) 17:943–52. doi: 10.1038/ncb3194
185. Gombault A, Baron L, Couillin I. ATP release and purinergic signaling in NLRP3 inflammasome activation. Front Immunol (2012) 3:414. doi: 10.3389/fimmu.2012.00414
186. Mantel A, Harvey V. P2X7/PANX1 as a new target for melanoma? Exp Dermatol (2015) 24:336–7. doi: 10.1111/exd.12633
187. Pelegrin P, Surprenant A. Pannexin-1 mediates large pore formation and interleukin-1β release by the ATP-gated P2X7 receptor. EMBO J (2006) 25:5071–82. doi: 10.1038/sj.emboj.7601378
188. de Leve S, Wirsdörfer F, Jendrossek V. Targeting the immunomodulatory CD73/Adenosine system to improve the therapeutic gain of radiotherapy. Front Immunol (2019) 10:698. doi: 10.3389/fimmu.2019.00698
189. de Andrade Mello P, Coutinho-Silva R, Savio LEB. Multifaceted effects of extracellular adenosine triphosphate and adenosine in the tumor-host interaction and therapeutic perspectives. Front Immunol (2017) 8:1526. doi: 10.3389/fimmu.2017.01526
190. Coutinho-Silva R, Persechini PM, Bisaggio RD, Perfettini JL, Neto AC, Kanellopoulos JM, et al. P2Z/P2X7 receptor-dependent apoptosis of dendritic cells. Am J Physiol (1999) 276:C1139–1147. doi: 10.1152/ajpcell.1999.276.5.C1139
191. Baricordi OR, Ferrari D, Melchiorri L, Chiozzi P, Hanau S, Chiari E, et al. An ATP-activated channel is involved in mitogenic stimulation of human T lymphocytes. Blood (1996) 87:682–90. doi: 10.1182/blood.V87.2.682.bloodjournal872682
192. Humphreys BD, Rice J, Kertesy SB, Dubyak GR. Stress-activated protein kinase/JNK activation and apoptotic induction by the macrophage P2X7 nucleotide receptor. J Biol Chem (2000) 275:26792–8. doi: 10.1074/jbc.M002770200
193. Graves LM, Guy HI, Kozlowski P, Huang M, Lazarowski E, Pope RM, et al. Regulation of carbamoyl phosphate synthetase by MAP kinase. Nature (2000) 403:328–32. doi: 10.1038/35002111
194. Adinolfi E, Melchiorri L, Falzoni S, Chiozzi P, Morelli A, Tieghi A, et al. P2X7 receptor expression in evolutive and indolent forms of chronic b lymphocytic leukemia. Blood (2002) 99:706–8. doi: 10.1182/blood.v99.2.706
195. Slater M, Danieletto S, Gidley-Baird A, Teh LC, Barden JA. Early prostate cancer detected using expression of non-functional cytolytic P2X7 receptors. Histopathology (2004) 44:206–15. doi: 10.1111/j.0309-0167.2004.01798.x
196. Adinolfi E, Callegari MG, Ferrari D, Bolognesi C, Minelli M, Wieckowski MR, et al. Basal activation of the P2X7 ATP receptor elevates mitochondrial calcium and potential, increases cellular ATP levels, and promotes serum-independent growth. Mol Biol Cell (2005) 16:3260–72. doi: 10.1091/mbc.e04-11-1025
197. Solini A, Cuccato S, Ferrari D, Santini E, Gulinelli S, Callegari MG, et al. Increased P2X7 receptor expression and function in thyroid papillary cancer: A new potential marker of the disease? Endocrinology (2008) 149:389–96. doi: 10.1210/en.2007-1223
198. Amoroso F, Salaro E, Falzoni S, Chiozzi P, Giuliani AL, Cavallesco G, et al. P2X7 targeting inhibits growth of human mesothelioma. Oncotarget (2016) 7:49664–76. doi: 10.18632/oncotarget.10430
199. Vázquez-Cuevas FG, Martínez-Ramírez AS, Robles-Martínez L, Garay E, García-Carrancá A, Pérez-Montiel D, et al. Paracrine stimulation of P2X7 receptor by ATP activates a proliferative pathway in ovarian carcinoma cells. J Cell Biochem (2014) 115:1955–66. doi: 10.1002/jcb.24867
200. Giannuzzo A, Pedersen SF, Novak I. The P2X7 receptor regulates cell survival, migration and invasion of pancreatic ductal adenocarcinoma cells. Mol Cancer (2015) 14:203. doi: 10.1186/s12943-015-0472-4
201. Qian F, Xiao J, Hu B, Sun N, Yin W, Zhu J. High expression of P2X7R is an independent postoperative indicator of poor prognosis in colorectal cancer. Hum Pathol (2017) 64:61–8. doi: 10.1016/j.humpath.2017.03.019
202. Calik I, Calik M, Turken G, Ozercan IH. A promising independent prognostic biomarker in colorectal cancer: P2X7 receptor. Int J Clin Exp Pathol (2020) 13:107–21.
203. Asif A, Khalid M, Manzoor S, Ahmad H, Rehman AU. Role of purinergic receptors in hepatobiliary carcinoma in Pakistani population: an approach towards proinflammatory role of P2X4 and P2X7 receptors. Purinergic Signal (2019) 15:367–74. doi: 10.1007/s11302-019-09675-0
204. Di Virgilio F, Ferrari D, Adinolfi E. P2X7: a growth-promoting receptor–implications for cancer. Purinergic Signal (2009) 5:251–6. doi: 10.1007/s11302-009-9145-3
205. Bradford MD, Soltoff SP. P2X7 receptors activate protein kinase d and p42/p44 mitogen-activated protein kinase (MAPK) downstream of protein kinase c. Biochem J (2002) 366:745–55. doi: 10.1042/BJ20020358
206. Stefano L, Rössler OG, Griesemer D, Hoth M, Thiel G. P2X7 receptor stimulation upregulates egr-1 biosynthesis involving a cytosolic Ca2+ rise, transactivation of the EGF receptor and phosphorylation of ERK and elk-1. J Cell Physiol (2007) 213:36–44. doi: 10.1002/jcp.21085
207. Choi JH, Ji YG, Ko JJ, Cho HJ, Lee DH. Activating P2X7 receptors increases proliferation of human pancreatic cancer cells via ERK1/2 and JNK. Pancreas (2018) 47:643–651. doi: 10.1097/MPA.0000000000001055
208. Adinolfi E, Cirillo M, Woltersdorf R, Falzoni S, Chiozzi P, Pellegatti P, et al. Trophic activity of a naturally occurring truncated isoform of the P2X7 receptor. FASEB J Off Publ Fed Am Soc Exp Biol (2010) 24:3393–404. doi: 10.1096/fj.09-153601
209. Giuliani AL, Colognesi D, Ricco T, Roncato C, Capece M, Amoroso F, et al. Trophic activity of human P2X7 receptor isoforms a and b in osteosarcoma. PloS One (2014) 9:e107224. doi: 10.1371/journal.pone.0107224
210. Ulrich H, Ratajczak MZ, Schneider G, Adinolfi E, Orioli E, Ferrazoli EG, et al. Kinin and purine signaling contributes to neuroblastoma metastasis. Front Pharmacol (2018) 9:500. doi: 10.3389/fphar.2018.00500
211. Pegoraro A, Orioli E, De Marchi E, Salvestrini V, Milani A, Di Virgilio F, et al. Differential sensitivity of acute myeloid leukemia cells to daunorubicin depends on P2X7A versus P2X7B receptor expression. Cell Death Dis (2020) 11:876. doi: 10.1038/s41419-020-03058-9
212. Arnaud-Sampaio VF, Bento CA, Glaser T, Adinolfi E, Ulrich H, Lameu C. P2X7 receptor isoform b is a key drug resistance mediator for neuroblastoma. Front Oncol (2022) 12:966404. doi: 10.3389/fonc.2022.966404
213. Greig AVH, Linge C, Healy V, Lim P, Clayton E, Rustin MHA, et al. Expression of purinergic receptors in non-melanoma skin cancers and their functional roles in A431 cells. J Invest Dermatol (2003) 121:315–27. doi: 10.1046/j.1523-1747.2003.12379.x
214. Aquea G, Bresky G, Lancellotti D, Madariaga JA, Zaffiri V, Urzua U, et al. Increased expression of P2RY2, CD248 and EphB1 in gastric cancers from Chilean patients. Asian Pac J Cancer Prev APJCP (2014) 15:1931–6. doi: 10.7314/apjcp.2014.15.5.1931
215. Tu MT, Luo SF, Wang CC, Chien CS, Chiu CT, Lin CC, et al. P2Y(2) receptor-mediated proliferation of C(6) glioma cells via activation of Ras/Raf/MEK/MAPK pathway. Br J Pharmacol (2000) 129:1481–9. doi: 10.1038/sj.bjp.0703182
216. Xiang H-J, Liu Z-C, Wang D-S, Chen Y, Yang Y-L, Dou K-F. Adenosine A(2b) receptor is highly expressed in human hepatocellular carcinoma. Hepatol Res Off J Jpn Soc Hepatol (2006) 36:56–60. doi: 10.1016/j.hepres.2006.06.008
217. Wei Q, Costanzi S, Balasubramanian R, Gao Z-G, Jacobson KA. A2B adenosine receptor blockade inhibits growth of prostate cancer cells. Purinergic Signal (2013) 9:271–80. doi: 10.1007/s11302-012-9350-3
218. Ma D-F, Kondo T, Nakazawa T, Niu D-F, Mochizuki K, Kawasaki T, et al. Hypoxia-inducible adenosine A2B receptor modulates proliferation of colon carcinoma cells. Hum Pathol (2010) 41:1550–7. doi: 10.1016/j.humpath.2010.04.008
219. Fernandez-Gallardo M, González-Ramírez R, Sandoval A, Felix R, Monjaraz E. Adenosine stimulate proliferation and migration in triple negative breast cancer cells. PloS One (2016) 11:e0167445. doi: 10.1371/journal.pone.0167445
220. Kasama H, Sakamoto Y, Kasamatsu A, Okamoto A, Koyama T, Minakawa Y, et al. Adenosine A2b receptor promotes progression of human oral cancer. BMC Cancer (2015) 15:563. doi: 10.1186/s12885-015-1577-2
221. Zhou Y, Chu X, Deng F, Tong L, Tong G, Yi Y, et al. The adenosine A2b receptor promotes tumor progression of bladder urothelial carcinoma by enhancing MAPK signaling pathway. Oncotarget (2017) 8:48755–68. doi: 10.18632/oncotarget.17835
222. Hevia MJ, Castro P, Pinto K, Reyna-Jeldes M, Rodríguez-Tirado F, Robles-Planells C, et al. Differential effects of purinergic signaling in gastric cancer-derived cells through P2Y and P2X receptors. Front Pharmacol (2019) 10:612. doi: 10.3389/fphar.2019.00612
223. Draganov D, Gopalakrishna-Pillai S, Chen Y-R, Zuckerman N, Moeller S, Wang C, et al. Modulation of P2X4/P2X7/Pannexin-1 sensitivity to extracellular ATP via ivermectin induces a non-apoptotic and inflammatory form of cancer cell death. Sci Rep (2015) 5:16222. doi: 10.1038/srep16222
224. Li X, Qi X, Zhou L, Catera D, Rote NS, Potashkin J, et al. Decreased expression of P2X7 in endometrial epithelial pre-cancerous and cancer cells. Gynecol Oncol (2007) 106:233–43. doi: 10.1016/j.ygyno.2007.03.032
225. Fu W, McCormick T, Qi X, Luo L, Zhou L, Li X, et al. Activation of P2X(7)-mediated apoptosis inhibits DMBA/TPA-induced formation of skin papillomas and cancer in mice. BMC Cancer (2009) 9:114. doi: 10.1186/1471-2407-9-114
226. Katzur AC, Koshimizu T, Tomić M, Schultze-Mosgau A, Ortmann O, Stojilkovic SS. Expression and responsiveness of P2Y2 receptors in human endometrial cancer cell lines. J Clin Endocrinol Metab (1999) 84:4085–91. doi: 10.1210/jcem.84.11.6119
227. Maaser K, Höpfner M, Kap H, Sutter AP, Barthel B, von Lampe B, et al. Extracellular nucleotides inhibit growth of human oesophageal cancer cells via P2Y(2)-receptors. Br J Cancer (2002) 86:636–44. doi: 10.1038/sj.bjc.6600100
228. Höpfner M, Maaser K, Barthel B, von Lampe B, Hanski C, Riecken EO, et al. Growth inhibition and apoptosis induced by P2Y2 receptors in human colorectal carcinoma cells: involvement of intracellular calcium and cyclic adenosine monophosphate. Int J Colorectal Dis (2001) 16:154–66. doi: 10.1007/s003840100302
229. Yang G, Zhang S, Zhang Y, Zhou Q, Peng S, Zhang T, et al. The inhibitory effects of extracellular ATP on the growth of nasopharyngeal carcinoma cells via P2Y2 receptor and osteopontin. J Exp Clin Cancer Res CR (2014) 33:53. doi: 10.1186/1756-9966-33-53
230. Wan H, Xie R, Xu J, He J, Tang B, Liu Q, et al. Anti-proliferative effects of nucleotides on gastric cancer via a novel P2Y6/SOCE/Ca(2+)/β-catenin pathway. Sci Rep (2017) 7:2459. doi: 10.1038/s41598-017-02562-x
231. Amoroso F, Falzoni S, Adinolfi E, Ferrari D, Di Virgilio F. The P2X7 receptor is a key modulator of aerobic glycolysis. Cell Death Dis (2012) 3:e370. doi: 10.1038/cddis.2012.105
232. Adinolfi E, Pizzirani C, Idzko M, Panther E, Norgauer J, Di Virgilio F, et al. P2X(7) receptor: Death or life? Purinergic Signal (2005) 1:219–27. doi: 10.1007/s11302-005-6322-x
233. Ledderose C, Woehrle T, Ledderose S, Strasser K, Seist R, Bao Y, et al. Cutting off the power: inhibition of leukemia cell growth by pausing basal ATP release and P2X receptor signaling? Purinergic Signal (2016) 12:439–51. doi: 10.1007/s11302-016-9510-y
234. Qian Y, Wang X, Liu Y, Li Y, Colvin RA, Tong L, et al. Extracellular ATP is internalized by macropinocytosis and induces intracellular ATP increase and drug resistance in cancer cells. Cancer Lett (2014) 351:242–51. doi: 10.1016/j.canlet.2014.06.008
235. Cao Y, Wang X, Li Y, Evers M, Zhang H, Chen X. Extracellular and macropinocytosis internalized ATP work together to induce epithelial-mesenchymal transition and other early metastatic activities in lung cancer. Cancer Cell Int (2019) 19:254. doi: 10.1186/s12935-019-0973-0
236. Murín R, Vidomanová E, Kowtharapu BS, Hatok J, Dobrota D. Role of s-adenosylmethionine cycle in carcinogenesis. Gen Physiol Biophys (2017) 36:513–20. doi: 10.4149/gpb_2017031
237. Cutruzzolà F, Giardina G, Marani M, Macone A, Paiardini A, Rinaldo S, et al. Glucose metabolism in the progression of prostate cancer. Front Physiol (2017) 8:97. doi: 10.3389/fphys.2017.00097
238. Moyer JD, Barbacci EG, Iwata KK, Arnold L, Boman B, Cunningham A, et al. Induction of apoptosis and cell cycle arrest by CP-358,774, an inhibitor of epidermal growth factor receptor tyrosine kinase. Cancer Res (1997) 57:4838–48.
239. Adinolfi E, Raffaghello L, Giuliani AL, Cavazzini L, Capece M, Chiozzi P, et al. Expression of P2X7 receptor increases in vivo tumor growth. Cancer Res (2012) 72:2957–69. doi: 10.1158/0008-5472.CAN-11-1947
240. Haanes KA, Schwab A, Novak I. The P2X7 receptor supports both life and death in fibrogenic pancreatic stellate cells. PloS One (2012) 7:e51164. doi: 10.1371/journal.pone.0051164
241. Zhang W-J, Luo C, Huang C, Pu F-Q, Zhu J-F, Zhu Z-M. PI3K/Akt/GSK-3β signal pathway is involved in P2X7 receptor-induced proliferation and EMT of colorectal cancer cells. Eur J Pharmacol (2021) 899:174041. doi: 10.1016/j.ejphar.2021.174041
242. Janho Dit Hreich S, Benzaquen J, Hofman P, Vouret-Craviari V. To inhibit or to boost the ATP/P2RX7 pathway to fight cancer-that is the question. Purinergic Signal (2021) 17:619–31. doi: 10.1007/s11302-021-09811-9
243. Kopp R, Krautloher A, Ramírez-Fernández A, Nicke A. P2X7 interactions and signaling - making head or tail of it. Front Mol Neurosci (2019) 12:183. doi: 10.3389/fnmol.2019.00183
244. Denlinger LC, Fisette PL, Sommer JA, Watters JJ, Prabhu U, Dubyak GR, et al. Cutting edge: the nucleotide receptor P2X7 contains multiple protein- and lipid-interaction motifs including a potential binding site for bacterial lipopolysaccharide. J Immunol Baltim Md 1950 (2001) 167:1871–6. doi: 10.4049/jimmunol.167.4.1871
245. Gonnord P, Delarasse C, Auger R, Benihoud K, Prigent M, Cuif MH, et al. Palmitoylation of the P2X7 receptor, an ATP-gated channel, controls its expression and association with lipid rafts. FASEB J Off Publ Fed Am Soc Exp Biol (2009) 23:795–805. doi: 10.1096/fj.08-114637
246. Robinson LE, Shridar M, Smith P, Murrell-Lagnado RD. Plasma membrane cholesterol as a regulator of human and rodent P2X7 receptor activation and sensitization. J Biol Chem (2014) 289:31983–94. doi: 10.1074/jbc.M114.574699
247. Murrell-Lagnado RD. Regulation of P2X purinergic receptor signaling by cholesterol. Curr Top Membr (2017) 80:211–32. doi: 10.1016/bs.ctm.2017.05.004
248. Benzaquen J, Heeke S, Janho Dit Hreich S, Douguet L, Marquette CH, Hofman P, et al. Alternative splicing of P2RX7 pre-messenger RNA in health and diseases: Myth or reality? BioMed J (2019) 42:141–54. doi: 10.1016/j.bj.2019.05.007
249. Feng L, Sun X, Csizmadia E, Han L, Bian S, Murakami T, et al. Vascular CD39/ENTPD1 directly promotes tumor cell growth by scavenging extracellular adenosine triphosphate. Neoplasia N Y N (2011) 13:206–16. doi: 10.1593/neo.101332
250. Feng Y-H, Li X, Wang L, Zhou L, Gorodeski GI. A truncated P2X7 receptor variant (P2X7-j) endogenously expressed in cervical cancer cells antagonizes the full-length P2X7 receptor through hetero-oligomerization. J Biol Chem (2006) 281:17228–37. doi: 10.1074/jbc.M602999200
251. Fang J, Chen X, Zhang L, Chen J, Liang Y, Li X, et al. P2X7R suppression promotes glioma growth through epidermal growth factor receptor signal pathway. Int J Biochem Cell Biol (2013) 45:1109–20. doi: 10.1016/j.biocel.2013.03.005
252. Schulze-Lohoff E, Hugo C, Rost S, Arnold S, Gruber A, Brüne B, et al. Extracellular ATP causes apoptosis and necrosis of cultured mesangial cells via P2Z/P2X7 receptors. Am J Physiol (1998) 275:F962–971. doi: 10.1152/ajprenal.1998.275.6.F962
253. Mello P de A, Filippi-Chiela EC, Nascimento J, Beckenkamp A, Santana DB, Kipper F, et al. Adenosine uptake is the major effector of extracellular ATP toxicity in human cervical cancer cells. Mol Biol Cell (2014) 25:2905–18. doi: 10.1091/mbc.E14-01-0042
254. Salvestrini V, Orecchioni S, Talarico G, Reggiani F, Mazzetti C, Bertolini F, et al. Extracellular ATP induces apoptosis through P2X7R activation in acute myeloid leukemia cells but not in normal hematopoietic stem cells. Oncotarget (2017) 8:5895–908. doi: 10.18632/oncotarget.13927
255. Hofman P, Cherfils-Vicini J, Bazin M, Ilie M, Juhel T, Hébuterne X, et al. Genetic and pharmacological inactivation of the purinergic P2RX7 receptor dampens inflammation but increases tumor incidence in a mouse model of colitis-associated cancer. Cancer Res (2015) 75:835–45. doi: 10.1158/0008-5472.CAN-14-1778
256. Adinolfi E, Capece M, Franceschini A, Falzoni S, Giuliani AL, Rotondo A, et al. Accelerated tumor progression in mice lacking the ATP receptor P2X7. Cancer Res (2015) 75:635–44. doi: 10.1158/0008-5472.CAN-14-1259
257. Tan C, Han LI, Zou L, Luo C, Liu A, Sheng X, et al. Expression of P2X7R in breast cancer tissue and the induction of apoptosis by the gene-specific shRNA in MCF-7 cells. Exp Ther Med (2015) 10:1472–8. doi: 10.3892/etm.2015.2705
258. Barden J, Yuksel A, Pedersen J, Danieletto S, Delprado W. Non-functional P2X7: a novel and ubiquitous target in human cancer. J Clin Cell Immunol (2014) 5:2155–9899. doi: 10.4172/2155-9899.1000237
259. Gilbert SM, Oliphant CJ, Hassan S, Peille AL, Bronsert P, Falzoni S, et al. ATP in the tumour microenvironment drives expression of nfP2X(7), a key mediator of cancer cell survival. Oncogene (2019) 38:194–208. doi: 10.1038/s41388-018-0426-6
260. Folkman J. Tumor angiogenesis: therapeutic implications. N Engl J Med (1971) 285:1182–6. doi: 10.1056/NEJM197111182852108
261. Konerding MA, Fait E, Gaumann A. 3D microvascular architecture of pre-cancerous lesions and invasive carcinomas of the colon. Br J Cancer (2001) 84:1354–62. doi: 10.1054/bjoc.2001.1809
262. Shibuya M. Vascular endothelial growth factor (VEGF) and its receptor (VEGFR) signaling in angiogenesis: A crucial target for anti- and pro-angiogenic therapies. Genes Cancer (2011) 2:1097–105. doi: 10.1177/1947601911423031
263. Hill LM, Gavala ML, Lenertz LY, Bertics PJ. Extracellular ATP may contribute to tissue repair by rapidly stimulating purinergic receptor X7-dependent vascular endothelial growth factor release from primary human monocytes. J Immunol Baltim Md 1950 (2010) 185:3028–34. doi: 10.4049/jimmunol.1001298
264. Wei W, Ryu JK, Choi HB, McLarnon JG. Expression and function of the P2X(7) receptor in rat C6 glioma cells. Cancer Lett (2008) 260:79–87. doi: 10.1016/j.canlet.2007.10.025
265. Yang C, Shi S, Su Y, Tong J-S, Li L. P2X7R promotes angiogenesis and tumour-associated macrophage recruitment by regulating the NF-κB signalling pathway in colorectal cancer cells. J Cell Mol Med (2020) 24:10830–41. doi: 10.1111/jcmm.15708
266. Hanahan D, Weinberg RA. Hallmarks of cancer: the next generation. Cell (2011) 144:646–74. doi: 10.1016/j.cell.2011.02.013
267. Fife CM, McCarroll JA, Kavallaris M. Movers and shakers: cell cytoskeleton in cancer metastasis. Br J Pharmacol (2014) 171:5507–23. doi: 10.1111/bph.12704
268. Hay ED. An overview of epithelio-mesenchymal transformation. Acta Anat (Basel) (1995) 154:8–20. doi: 10.1159/000147748
269. Lu W, Kang Y. Epithelial-mesenchymal plasticity in cancer progression and metastasis. Dev Cell (2019) 49:361–74. doi: 10.1016/j.devcel.2019.04.010
270. Lambert AW, Weinberg RA. Linking EMT programmes to normal and neoplastic epithelial stem cells. Nat Rev Cancer (2021) 21:325–38. doi: 10.1038/s41568-021-00332-6
271. Martínez-Ramírez AS, Díaz-Muñoz M, Butanda-Ochoa A, Vázquez-Cuevas FG. Nucleotides and nucleoside signaling in the regulation of the epithelium to mesenchymal transition (EMT). Purinergic Signal (2017) 13:1–12. doi: 10.1007/s11302-016-9550-3
272. Takai E, Tsukimoto M, Harada H, Kojima S. Autocrine signaling via release of ATP and activation of P2X7 receptor influences motile activity of human lung cancer cells. Purinergic Signal (2014) 10:487–97. doi: 10.1007/s11302-014-9411-x
273. Qiu Y, Li W-H, Zhang H-Q, Liu Y, Tian X-X, Fang W-G. P2X7 mediates ATP-driven invasiveness in prostate cancer cells. PloS One (2014) 9:e114371. doi: 10.1371/journal.pone.0114371
274. Ji Z, Xie Y, Guan Y, Zhang Y, Cho K-S, Ji M, et al. Involvement of P2X(7) receptor in proliferation and migration of human glioma cells. BioMed Res Int (2018) 2018:8591397. doi: 10.1155/2018/8591397
275. Ziberi S, Zuccarini M, Carluccio M, Giuliani P, Ricci-Vitiani L, Pallini R, et al. Upregulation of epithelial-To-Mesenchymal transition markers and P2X7 receptors is associated to increased invasiveness caused by P2X7 receptor stimulation in human glioblastoma stem cells. Cells (2019) 9:85. doi: 10.3390/cells9010085
276. Jelassi B, Chantôme A, Alcaraz-Pérez F, Baroja-Mazo A, Cayuela ML, Pelegrin P, et al. P2X(7) receptor activation enhances SK3 channels- and cystein cathepsin-dependent cancer cells invasiveness. Oncogene (2011) 30:2108–22. doi: 10.1038/onc.2010.593
277. Jelassi B, Anchelin M, Chamouton J, Cayuela ML, Clarysse L, Li J, et al. Anthraquinone emodin inhibits human cancer cell invasiveness by antagonizing P2X7 receptors. Carcinogenesis (2013) 34:1487–96. doi: 10.1093/carcin/bgt099
278. Schmid S, Kübler M, Korcan Ayata C, Lazar Z, Haager B, Hoßfeld M, et al. Altered purinergic signaling in the tumor associated immunologic microenvironment in metastasized non-small-cell lung cancer. Lung Cancer Amst Neth (2015) 90:516–21. doi: 10.1016/j.lungcan.2015.10.005
279. Qiu Y, Liu Y, Li W-H, Zhang H-Q, Tian X-X, Fang W-G. P2Y2 receptor promotes the migration and invasion of breast cancer cells via EMT-related genes snail and e-cadherin. Oncol Rep (2018) 39:138–50. doi: 10.3892/or.2017.6081
280. Zhang J-L, Liu Y, Yang H, Zhang H-Q, Tian X-X, Fang W-G. ATP-P2Y2-β-catenin axis promotes cell invasion in breast cancer cells. Cancer Sci (2017) 108:1318–27. doi: 10.1111/cas.13273
281. Eun SY, Ko YS, Park SW, Chang KC, Kim HJ. P2Y2 nucleotide receptor-mediated extracellular signal-regulated kinases and protein kinase c activation induces the invasion of highly metastatic breast cancer cells. Oncol Rep (2015) 34:195–202. doi: 10.3892/or.2015.3972
282. Chen L, He H, Li H, Zheng J, Heng W, You J, et al. ERK1/2 and p38 pathways are required for P2Y receptor-mediated prostate cancer invasion. Cancer Lett (2004) 215:239–47. doi: 10.1016/j.canlet.2004.05.023
283. Martínez-Ramírez AS, Garay E, García-Carrancá A, Vázquez-Cuevas FG. The P2RY2 receptor induces carcinoma cell migration and EMT through cross-talk with epidermal growth factor receptor. J Cell Biochem (2016) 117:1016–26. doi: 10.1002/jcb.25390
284. Li W-H, Qiu Y, Zhang H-Q, Tian X-X, Fang W-G. P2Y2 receptor and EGFR cooperate to promote prostate cancer cell invasion via ERK1/2 pathway. PloS One (2015) 10:e0133165. doi: 10.1371/journal.pone.0133165
285. Ren Z-H, Lin C-Z, Cao W, Yang R, Lu W, Liu Z-Q, et al. CD73 is associated with poor prognosis in HNSCC. Oncotarget (2016) 7:61690–702. doi: 10.18632/oncotarget.11435
286. Ma X-L, Shen M-N, Hu B, Wang B-L, Yang W-J, Lv L-H, et al. CD73 promotes hepatocellular carcinoma progression and metastasis via activating PI3K/AKT signaling by inducing Rap1-mediated membrane localization of P110β and predicts poor prognosis. J Hematol OncolJ Hematol Oncol (2019) 12:37. doi: 10.1186/s13045-019-0724-7
287. Virtanen SS, Kukkonen-Macchi A, Vainio M, Elima K, Härkönen PL, Jalkanen S, et al. Adenosine inhibits tumor cell invasion via receptor-independent mechanisms. Mol Cancer Res MCR (2014) 12:1863–74. doi: 10.1158/1541-7786.MCR-14-0302-T
288. Zhou P, Zhi X, Zhou T, Chen S, Li X, Wang L, et al. Overexpression of ecto-5’-nucleotidase (CD73) promotes T-47D human breast cancer cells invasion and adhesion to extracellular matrix. Cancer Biol Ther (2007) 6:426–31. doi: 10.4161/cbt.6.3.3762
289. Martínez-Ramírez AS, Díaz-Muñoz M, Battastini AM, Campos-Contreras A, Olvera A, Bergamin L, et al. Cellular migration ability is modulated by extracellular purines in ovarian carcinoma SKOV-3 cells. J Cell Biochem (2017) 118:4468–78. doi: 10.1002/jcb.26104
290. Shi L, Wu Z, Miao J, Du S, Ai S, Xu E, et al. Adenosine interaction with adenosine receptor A2a promotes gastric cancer metastasis by enhancing PI3K-AKT-mTOR signaling. Mol Biol Cell (2019) 30:2527–34. doi: 10.1091/mbc.E19-03-0136
291. Jajoo S, Mukherjea D, Watabe K, Ramkumar V. Adenosine A(3) receptor suppresses prostate cancer metastasis by inhibiting NADPH oxidase activity. Neoplasia N Y N (2009) 11:1132–45. doi: 10.1593/neo.09744
292. Torres Á, Erices JI, Sanchez F, Ehrenfeld P, Turchi L, Virolle T, et al. Extracellular adenosine promotes cell migration/invasion of glioblastoma stem-like cells through A(3) adenosine receptor activation under hypoxia. Cancer Lett (2019) 446:112–22. doi: 10.1016/j.canlet.2019.01.004
293. Zanotelli MR, Goldblatt ZE, Miller JP, Bordeleau F, Li J, VanderBurgh JA, et al. Regulation of ATP utilization during metastatic cell migration by collagen architecture. Mol Biol Cell (2018) 29:1–9. doi: 10.1091/mbc.E17-01-0041
294. Kelley LC, Chi Q, Cáceres R, Hastie E, Schindler AJ, Jiang Y, et al. Adaptive f-actin polymerization and localized ATP production drive basement membrane invasion in the absence of MMPs. Dev Cell (2019) 48:313–328.e8. doi: 10.1016/j.devcel.2018.12.018
295. Zhang Q, Zhu B, Li Y. Resolution of cancer-promoting inflammation: A new approach for anticancer therapy. Front Immunol (2017) 8:71. doi: 10.3389/fimmu.2017.00071
296. Shalapour S, Karin M. Pas de deux: Control of anti-tumor immunity by cancer-associated inflammation. Immunity (2019) 51:15–26. doi: 10.1016/j.immuni.2019.06.021
297. Miller JS, Lanier LL. Natural killer cells in cancer immunotherapy. Annu Rev Cancer Biol (2019) 3:77–103. doi: 10.1146/annurev-cancerbio-030518-055653
298. Gabrilovich DI, Nagaraj S. Myeloid-derived suppressor cells as regulators of the immune system. Nat Rev Immunol (2009) 9:162–74. doi: 10.1038/nri2506
299. Dwyer KM, Kishore BK, Robson SC. Conversion of extracellular ATP into adenosine: a master switch in renal health and disease. Nat Rev Nephrol (2020) 16:509–24. doi: 10.1038/s41581-020-0304-7
300. Allard B, Allard D, Buisseret L, Stagg J. The adenosine pathway in immuno-oncology. Nat Rev Clin Oncol (2020) 17:611–29. doi: 10.1038/s41571-020-0382-2
301. Whiteside TL. Targeting adenosine in cancer immunotherapy: a review of recent progress. Expert Rev Anticancer Ther (2017) 17:527–35. doi: 10.1080/14737140.2017.1316197
302. Chekeni FB, Elliott MR, Sandilos JK, Walk SF, Kinchen JM, Lazarowski ER, et al. Pannexin 1 channels mediate “find-me” signal release and membrane permeability during apoptosis. Nature (2010) 467:863–7. doi: 10.1038/nature09413
303. Elliott MR, Chekeni FB, Trampont PC, Lazarowski ER, Kadl A, Walk SF, et al. Nucleotides released by apoptotic cells act as a find-me signal to promote phagocytic clearance. Nature (2009) 461:282–6. doi: 10.1038/nature08296
304. Trautmann A. Extracellular ATP in the immune system: more than just a “danger signal”. Sci Signal (2009) 2:pe6. doi: 10.1126/scisignal.256pe6
305. Lecciso M, Ocadlikova D, Sangaletti S, Trabanelli S, De Marchi E, Orioli E, et al. ATP release from chemotherapy-treated dying leukemia cells elicits an immune suppressive effect by increasing regulatory T cells and tolerogenic dendritic cells. Front Immunol (2017) 8:1918. doi: 10.3389/fimmu.2017.01918
306. Ghiringhelli F, Apetoh L, Tesniere A, Aymeric L, Ma Y, Ortiz C, et al. Activation of the NLRP3 inflammasome in dendritic cells induces IL-1beta-dependent adaptive immunity against tumors. Nat Med (2009) 15:1170–8. doi: 10.1038/nm.2028
307. Aymeric L, Apetoh L, Ghiringhelli F, Tesniere A, Martins I, Kroemer G, et al. Tumor cell death and ATP release prime dendritic cells and efficient anticancer immunity. Cancer Res (2010) 70:855–8. doi: 10.1158/0008-5472.CAN-09-3566
308. Montalbán Del Barrio I, Penski C, Schlahsa L, Stein RG, Diessner J, Wöckel A, et al. Adenosine-generating ovarian cancer cells attract myeloid cells which differentiate into adenosine-generating tumor associated macrophages - a self-amplifying, CD39- and CD73-dependent mechanism for tumor immune escape. J Immunother Cancer (2016) 4:49. doi: 10.1186/s40425-016-0154-9
309. Perrot I, Michaud H-A, Giraudon-Paoli M, Augier S, Docquier A, Gros L, et al. Blocking antibodies targeting the CD39/CD73 immunosuppressive pathway unleash immune responses in combination cancer therapies. Cell Rep (2019) 27:2411–2425.e9. doi: 10.1016/j.celrep.2019.04.091
310. Häusler SF, Del Barrio IM, Diessner J, Stein RG, Strohschein J, Hönig A, et al. Anti-CD39 and anti-CD73 antibodies A1 and 7G2 improve targeted therapy in ovarian cancer by blocking adenosine-dependent immune evasion. Am J Transl Res (2014) 6:129–39.
311. Wennerberg E, Spada S, Rudqvist N-P, Lhuillier C, Gruber S, Chen Q, et al. CD73 blockade promotes dendritic cell infiltration of irradiated tumors and tumor rejection. Cancer Immunol Res (2020) 8:465–78. doi: 10.1158/2326-6066.CIR-19-0449
312. Chen DS, Mellman I. Oncology meets immunology: the cancer-immunity cycle. Immunity (2013) 39:1–10. doi: 10.1016/j.immuni.2013.07.012
313. Hickman SE, el Khoury J, Greenberg S, Schieren I, Silverstein SC. P2Z adenosine triphosphate receptor activity in cultured human monocyte-derived macrophages. Blood (1994) 84:2452–6. doi: 10.1182/blood.V84.8.2452.2452
314. Ferrari D, Chiozzi P, Falzoni S, Dal Susino M, Melchiorri L, Baricordi OR, et al. Extracellular ATP triggers IL-1 beta release by activating the purinergic P2Z receptor of human macrophages. J Immunol Baltim Md 1950 (1997) 159:1451–8. doi: 10.4049/jimmunol.159.3.1451
315. Berchtold S, Ogilvie AL, Bogdan C, Mühl-Zürbes P, Ogilvie A, Schuler G, et al. Human monocyte derived dendritic cells express functional P2X and P2Y receptors as well as ecto-nucleotidases. FEBS Lett (1999) 458:424–8. doi: 10.1016/s0014-5793(99)01197-7
316. Ferrari D, La Sala A, Chiozzi P, Morelli A, Falzoni S, Girolomoni G, et al. The P2 purinergic receptors of human dendritic cells: identification and coupling to cytokine release. FASEB J Off Publ Fed Am Soc Exp Biol (2000) 14:2466–76. doi: 10.1096/fj.00-0031com
317. Ma Y, Adjemian S, Mattarollo SR, Yamazaki T, Aymeric L, Yang H, et al. Anticancer chemotherapy-induced intratumoral recruitment and differentiation of antigen-presenting cells. Immunity (2013) 38:729–41. doi: 10.1016/j.immuni.2013.03.003
318. Hamarsheh S, Zeiser R. NLRP3 inflammasome activation in cancer: A double-edged sword. Front Immunol (2020) 11:1444. doi: 10.3389/fimmu.2020.01444
319. Feng W, Yang X, Wang L, Wang R, Yang F, Wang H, et al. P2X7 promotes the progression of MLL-AF9 induced acute myeloid leukemia by upregulation of Pbx3. Haematologica (2021) 106:1278–89. doi: 10.3324/haematol.2019.243360
320. De Marchi E, Orioli E, Pegoraro A, Sangaletti S, Portararo P, Curti A, et al. The P2X7 receptor modulates immune cells infiltration, ectonucleotidases expression and extracellular ATP levels in the tumor microenvironment. Oncogene (2019) 38:3636–50. doi: 10.1038/s41388-019-0684-y
321. Benzaquen J, Dit Hreich SJ, Heeke S, Juhel T, Lalvee S, Bauwens S, et al. P2RX7B is a new theranostic marker for lung adenocarcinoma patients. Theranostics (2020) 10:10849–60. doi: 10.7150/thno.48229
322. Romagnani A, Rottoli E, Mazza EMC, Rezzonico-Jost T, De Ponte Conti B, Proietti M, et al. P2X7 receptor activity limits accumulation of T cells within tumors. Cancer Res (2020) 80:3906–19. doi: 10.1158/0008-5472.CAN-19-3807
323. Wanhainen KM, Peng C, Zhou MH, Macedo B de G, O’Flanagan S, Yang T, et al. P2RX7 enhances tumor control by CD8+ T cells in adoptive cell therapy. Cancer Immunol Res (2022) 10:871–84. doi: 10.1158/2326-6066.CIR-21-0691
324. Ohta A, Gorelik E, Prasad SJ, Ronchese F, Lukashev D, Wong MKK, et al. A2A adenosine receptor protects tumors from antitumor T cells. Proc Natl Acad Sci U.S.A. (2006) 103:13132–7. doi: 10.1073/pnas.0605251103
325. Chen L, Han X. Anti-PD-1/PD-L1 therapy of human cancer: Past, present, and future. J Clin Invest (2015) 125:3384–91. doi: 10.1172/JCI80011
326. Beavis PA, Milenkovski N, Henderson MA, John LB, Allard B, Loi S, et al. Adenosine receptor 2A blockade increases the efficacy of anti-PD-1 through enhanced antitumor T-cell responses. Cancer Immunol Res (2015) 3:506–17. doi: 10.1158/2326-6066.CIR-14-0211
327. Kjaergaard J, Hatfield S, Jones G, Ohta A, Sitkovsky M. A(2A) adenosine receptor gene deletion or synthetic A(2A) antagonist liberate tumor-reactive CD8(+) T cells from tumor-induced immunosuppression. J Immunol Baltim Md 1950 (2018) 201:782–91. doi: 10.4049/jimmunol.1700850
328. Young A, Ngiow SF, Gao Y, Patch A-M, Barkauskas DS, Messaoudene M, et al. A2AR adenosine signaling suppresses natural killer cell maturation in the tumor microenvironment. Cancer Res (2018) 78:1003–16. doi: 10.1158/0008-5472.CAN-17-2826
329. Yu M, Guo G, Huang L, Deng L, Chang C-S, Achyut BR, et al. CD73 on cancer-associated fibroblasts enhanced by the A(2B)-mediated feedforward circuit enforces an immune checkpoint. Nat Commun (2020) 11:515. doi: 10.1038/s41467-019-14060-x
330. Liu H, Kuang X, Zhang Y, Ye Y, Li J, Liang L, et al. ADORA1 inhibition promotes tumor immune evasion by regulating the ATF3-PD-L1 axis. Cancer Cell (2020) 37:324–339.e8. doi: 10.1016/j.ccell.2020.02.006
331. Vermeulen L, Sprick MR, Kemper K, Stassi G, Medema JP. Cancer stem cells–old concepts, new insights. Cell Death Differ (2008) 15:947–58. doi: 10.1038/cdd.2008.20
332. Elshamy WM, Duhé RJ. Overview: cellular plasticity, cancer stem cells and metastasis. Cancer Lett (2013) 341:2–8. doi: 10.1016/j.canlet.2013.06.020
333. Vermeulen L, Todaro M, de Sousa Mello F, Sprick MR, Kemper K, Perez Alea M, et al. Single-cell cloning of colon cancer stem cells reveals a multi-lineage differentiation capacity. Proc Natl Acad Sci U.S.A. (2008) 105:13427–32. doi: 10.1073/pnas.0805706105
334. Sell S. On the stem cell origin of cancer. Am J Pathol (2010) 176:2584–494. doi: 10.2353/ajpath.2010.091064
335. Podberezin M, Wen J, Chang C-CJ. Cancer stem cells: A review of potential clinical applications. Arch Pathol Lab Med (2013) 137:1111–6. doi: 10.5858/arpa.2012-0494-RA
336. O’Connor ML, Xiang D, Shigdar S, Macdonald J, Li Y, Wang T, et al. Cancer stem cells: A contentious hypothesis now moving forward. Cancer Lett (2014) 344:180–7. doi: 10.1016/j.canlet.2013.11.012
337. Morrone FB, Jacques-Silva MC, Horn AP, Bernardi A, Schwartsmann G, Rodnight R, et al. Extracellular nucleotides and nucleosides induce proliferation and increase nucleoside transport in human glioma cell lines. J Neurooncol (2003) 64:211–8. doi: 10.1023/a:1025699932270
338. Ledur PF, Villodre ES, Paulus R, Cruz LA, Flores DG, Lenz G. Extracellular ATP reduces tumor sphere growth and cancer stem cell population in glioblastoma cells. Purinergic Signal (2012) 8:39–48. doi: 10.1007/s11302-011-9252-9
339. Paredes-Gamero EJ, Nogueira-Pedro A, Miranda A, Justo GZ. Hematopoietic modulators as potential agents for the treatment of leukemia. Front Biosci Elite Ed (2013) 5:130–40. doi: 10.2741/e602
340. Glaser T, de Oliveira SLB, Cheffer A, Beco R, Martins P, Fornazari M, et al. Modulation of mouse embryonic stem cell proliferation and neural differentiation by the P2X7 receptor. PloS One (2014) 9:e96281. doi: 10.1371/journal.pone.0096281
341. Wu P-Y, Lin Y-C, Chang C-L, Lu H-T, Chin C-H, Hsu T-T, et al. Functional decreases in P2X7 receptors are associated with retinoic acid-induced neuronal differentiation of neuro-2a neuroblastoma cells. Cell Signal (2009) 21:881–91. doi: 10.1016/j.cellsig.2009.01.036
342. D’Alimonte I, Nargi E, Zuccarini M, Lanuti P, Di Iorio P, Giuliani P, et al. Potentiation of temozolomide antitumor effect by purine receptor ligands able to restrain the in vitro growth of human glioblastoma stem cells. Purinergic Signal (2015) 11:331–46. doi: 10.1007/s11302-015-9454-7
343. Carluccio M, Zuccarini M, Ziberi S, Giuliani P, Morabito C, Mariggiò MA, et al. Involvement of P2X7 receptors in the osteogenic differentiation of mesenchymal Stromal/Stem cells derived from human subcutaneous adipose tissue. Stem Cell Rev Rep (2019) 15:574–89. doi: 10.1007/s12015-019-09883-6
344. Feng L-L, Cai Y-Q, Zhu M-C, Xing L-J, Wang X. The yin and yang functions of extracellular ATP and adenosine in tumor immunity. Cancer Cell Int (2020) 20:110. doi: 10.1186/s12935-020-01195-x
345. Wang X, Li Y, Qian Y, Cao Y, Shriwas P, Zhang H, et al. Extracellular ATP, as an energy and phosphorylating molecule, induces different types of drug resistances in cancer cells through ATP internalization and intracellular ATP level increase. Oncotarget (2017) 8:87860–77. doi: 10.18632/oncotarget.21231
346. Qian Y, Wang X, Li Y, Cao Y, Chen X. Extracellular ATP a new player in cancer metabolism: NSCLC cells internalize ATP In vitro and In vivo using multiple endocytic mechanisms. Mol Cancer Res MCR (2016) 14:1087–96. doi: 10.1158/1541-7786.MCR-16-0118
347. Commisso C, Davidson SM, Soydaner-Azeloglu RG, Parker SJ, Kamphorst JJ, Hackett S, et al. Macropinocytosis of protein is an amino acid supply route in ras-transformed cells. Nature (2013) 497:633–7. doi: 10.1038/nature12138
348. Aroua N, Boet E, Ghisi M, Nicolau-Travers M-L, Saland E, Gwilliam R, et al. Extracellular ATP and CD39 activate cAMP-mediated mitochondrial stress response to promote cytarabine resistance in acute myeloid leukemia. Cancer Discovery (2020) 10:1544–65. doi: 10.1158/2159-8290.CD-19-1008
349. Li X-Y, Moesta AK, Xiao C, Nakamura K, Casey M, Zhang H, et al. Targeting CD39 in cancer reveals an extracellular ATP- and inflammasome-driven tumor immunity. Cancer Discovery (2019) 9:1754–73. doi: 10.1158/2159-8290.CD-19-0541
350. Elaskalani O, Falasca M, Moran N, Berndt MC, Metharom P. The role of platelet-derived ADP and ATP in promoting pancreatic cancer cell survival and gemcitabine resistance. Cancers (2017) 9:142. doi: 10.3390/cancers9100142
351. Surprenant A, Rassendren F, Kawashima E, North RA, Buell G. The cytolytic P2Z receptor for extracellular ATP identified as a P2X receptor (P2X7). Science (1996) 272:735–8. doi: 10.1126/science.272.5262.735
352. Di Virgilio F, Bronte V, Collavo D, Zanovello P. Responses of mouse lymphocytes to extracellular adenosine 5’-triphosphate (ATP). lymphocytes with cytotoxic activity are resistant to the permeabilizing effects of ATP. J Immunol Baltim Md 1950 (1989) 143:1955–60.
353. Di Virgilio F, Chiozzi P, Falzoni S, Ferrari D, Sanz JM, Venketaraman V, et al. Cytolytic P2X purinoceptors. Cell Death Differ (1998) 5:191–9. doi: 10.1038/sj.cdd.4400341
354. Chen Y-W, Donnelly-Roberts DL, Namovic MT, Gintant GA, Cox BF, Jarvis MF, et al. Pharmacological characterization of P2X7 receptors in rat peritoneal cells. Inflammation Res Off J Eur Histamine Res Soc Al (2005) 54:119–26. doi: 10.1007/s00011-004-1332-7
355. Kepp O, Loos F, Liu P, Kroemer G. Extracellular nucleosides and nucleotides as immunomodulators. Immunol Rev (2017) 280:83–92. doi: 10.1111/imr.12571
356. Galluzzi L, Buqué A, Kepp O, Zitvogel L, Kroemer G. Immunogenic cell death in cancer and infectious disease. Nat Rev Immunol (2017) 17:97–111. doi: 10.1038/nri.2016.107
357. Zanoni M, Sarti AC, Zamagni A, Cortesi M, Pignatta S, Arienti C, et al. Irradiation causes senescence, ATP release, and P2X7 receptor isoform switch in glioblastoma. Cell Death Dis (2022) 13:80. doi: 10.1038/s41419-022-04526-0
358. Regateiro FS, Cobbold SP, Waldmann H. CD73 and adenosine generation in the creation of regulatory microenvironments. Clin Exp Immunol (2013) 171:1–7. doi: 10.1111/j.1365-2249.2012.04623.x
359. Ohta A. A metabolic immune checkpoint: Adenosine in tumor microenvironment. Front Immunol (2016) 7:109. doi: 10.3389/fimmu.2016.00109
360. Pietrocola F, Pol J, Kroemer G. Fasting improves anticancer immunosurveillance via autophagy induction in malignant cells. Cell Cycle Georget Tex (2016) 15:3327–8. doi: 10.1080/15384101.2016.1224797
361. Luo Y, Qiao B, Zhang P, Yang C, Cao J, Yuan X, et al. TME-activatable theranostic nanoplatform with ATP burning capability for tumor sensitization and synergistic therapy. Theranostics (2020) 10:6987–7001. doi: 10.7150/thno.44569
362. Hanahan D, Weinberg RA. The hallmarks of cancer. Cell (2000) 100:57–70. doi: 10.1016/s0092-8674(00)81683-9
363. Vultaggio-Poma V, Sarti AC, Di Virgilio F. Extracellular ATP: A feasible target for cancer therapy. Cells (2020) 9:2496. doi: 10.3390/cells9112496
Keywords: purinergic signaling, ATP, ADO, P1, P2X, P2Y, cancer
Citation: Kaur J and Dora S (2023) Purinergic signaling: Diverse effects and therapeutic potential in cancer. Front. Oncol. 13:1058371. doi: 10.3389/fonc.2023.1058371
Received: 30 September 2022; Accepted: 02 January 2023;
Published: 18 January 2023.
Edited by:
Michele Zanoni, Scientific Institute of Romagna for the Study and Treatment of Tumors (IRCCS), ItalyReviewed by:
Anna Pegoraro, University of Ferrara, ItalyCopyright © 2023 Kaur and Dora. This is an open-access article distributed under the terms of the Creative Commons Attribution License (CC BY). The use, distribution or reproduction in other forums is permitted, provided the original author(s) and the copyright owner(s) are credited and that the original publication in this journal is cited, in accordance with accepted academic practice. No use, distribution or reproduction is permitted which does not comply with these terms.
*Correspondence: Jasmeet Kaur, amFzbWVldDIza0BnbWFpbC5jb20=
Disclaimer: All claims expressed in this article are solely those of the authors and do not necessarily represent those of their affiliated organizations, or those of the publisher, the editors and the reviewers. Any product that may be evaluated in this article or claim that may be made by its manufacturer is not guaranteed or endorsed by the publisher.
Research integrity at Frontiers
Learn more about the work of our research integrity team to safeguard the quality of each article we publish.