- 1State Key Laboratory of Oncogenes and Related Genes, Center for Single-Cell Omics, School of Public Health, Shanghai Jiao Tong University School of Medicine, Shanghai, China
- 2College of Medical Technology, Shanghai University of Medicine and Health Sciences, Shanghai, China
Immunotherapies are recently emerged as a new strategy in treating various kinds of cancers which are insensitive to standard therapies, while the clinical application of immunotherapy is largely compromised by the low efficiency and serious side effects. Gut microbiota has been shown critical for the development of different cancer types, and the potential of gut microbiota manipulation through direct implantation or antibiotic-based depletion in regulating the overall efficacy of cancer immunotherapies has also been evaluated. However, the role of dietary supplementations, especially fungal products, in gut microbiota regulation and the enhancement of cancer immunotherapy remains elusive. In the present review, we comprehensively illustrated the limitations of current cancer immunotherapies, the biological functions as well as underlying mechanisms of gut microbiota manipulation in regulating cancer immunotherapies, and the benefits of dietary fungal supplementation in promoting cancer immunotherapies through gut microbiota modulation.
1 Introduction
The concept of cancer immunotherapy was first carried out by clinicians and immunologists centuries ago, but it had not been widely accepted as a practical option against cancer until recently (1). With the achievement of modern biomedical technologies, various types of immunotherapeutic strategies have been developed, which include the well-known “immune checkpoint blockade (ICB)” therapy (2), represented by the antibodies blocking the cytotoxic T-lymphocyte antigen-4 (CTLA-4) and the programmed cell death 1 (PD-1) and its ligand (PD-L1), as well as the “chimeric antigen receptor T cell (CAR-T)” therapy (3). Besides, many other immunotherapies were also proved to be efficient in treating certain types of cancers (4–7). However, the overall response of cancer patients to immunotherapies varies and serious symptoms were frequently observed, which dampened further utilization of this novel approach (8, 9). For instance, immune-related adverse events (irAEs) were frequently observed in patients receiving immune-checkpoint inhibitors (ICIs), characterized by excessive inflammation of multiple organs including skin, liver, lung, gastrointestinal tract, endocrine organs, etc. The incidence of irAEs varies among cohorts due to different type of ICIs and cancers (10), however, according to a retrospective study, 91.3% patients were affected by at least one type of irAE following ICI treatments (11). Cytokine release syndrome (CRS), a systemic inflammatory response, was closely associated with patients receiving CAR-T therapies. It was reported that the overall incidence of CRS can be as high as 90% in patients receiving CAR-T treatments (12). Therefore, a comprehensive understanding of the biology of cancer immunotherapy is required to improve its efficiency as well as to eliminate any potential side effects.
In recent years, the emerging role of gut microbiota in human health was emphasized with the development of multi-omic technologies (13). Previous studies have shown that the disruption of gut microbiota homeostasis contributes to the progression of various diseases (14–17). For cancer immunotherapies, the role of gut microbiota in regulating the efficiency as well as side effects of immunotherapy has also been revealed (18), as certain microbial species or related metabolites were shown closely correlated with the higher responsiveness of cancer patients. Nevertheless, the exact molecular mechanisms that how gut microbiota affect the host’s response to cancer immunotherapies are still under investigation.
Gut microbiota is composed of trillions of residing microbes, which are strongly affected by consumed nutrients (19); therefore, it is a potential way to manipulate gut microbiota through diet intervention to achieve a better outcome for cancer patients receiving immunotherapies (20). Numerous studies have been conducted on the nutritional value of plant or animal natural products as well as their modulation of gut microbiota and tumor immunotherapy (21, 22). Fungal products as a hot topic in recent years have attracted much attention due to their rich nutritional value and regulating functions to human body, of note, the regulatory role of fungal products on gut microbiota and cancer immunotherapies has been revealed (23). In this review, we will focus on the relationship between intake of natural products and gut microbiota modulation, as well as their biological role and underlying mechanism in cancer immunotherapies.
2 Advances and limitations of current cancer immunotherapies
2.1 Immune checkpoint blockade therapy
Compared with previous standards of care including chemotherapy, radiotherapy, and surgery, immunotherapy is a newly introduced approach, while showing significant improvement in the survival as well as the life quality of cancer patients (24). ICB is one of the most revolutionary technologies based on the theory of “immune surveillance” and the discovery of immune checkpoint molecules including CTLA-4 and PD-1, etc., on T cells (25, 26). Signals transduced by CTLA-4 following CD80/86 binding, and PD-1 following PD-L1 binding inhibit the “hyperactivation” of T cells and are important in preventing abnormal immune responses commonly seen in autoimmune diseases (27). However, these signals need to be abrogated to enhance the activity of T cells for the clearance of cancer cells (28). According to previous studies, patients treated with monoclonal antibodies against CTLA-4 or PD-1/PD-L1 resulted in dramatic antitumor responses through upregulation of immune activity (29–31). Mechanistic studies revealed that CTLA-4 or PD-1/PD-L1 blockade significantly enhanced T cell receptor (TCR) signals in tumor-specific T cells which leads to stronger tumor-killing activity (32), the infiltration as well as the survival rate of T cells in tumor microenvironment (TME) were also enhanced accordingly (33, 34). Currently, ICB has been approved for use in various types of cancers including melanoma, non-small cell lung cancer (NSCLC), renal cell carcinoma (RCC), squamous cell carcinoma of head and neck (SCCHN), bladder cancer, merkel cell carcinoma, hepatocellular carcinoma, Hodgkin lymphoma as either first-line or second-line treatment (35). Besides, many other agonistic and antagonistic immune checkpoint modulators which target co-stimulatory factors like 4-1BB, ICOS, GITR, OX-40, CD40, etc., are currently under investigation (36).
Along with the achievements in clinical practice, ICB therapy still has many limitations. One notable challenge is the generally low response across different types of cancers (37). Although the efficiency of anti-PD-1/PD-L1 has been clearly addressed in melanoma and NSCLC, the results from other types of cancers are less promising, and the response of individuals to ICB varies, which suggested the effects of other factors including genetics, environment, behavior, and even gut microbiota on the therapeutical efficiency of ICB (38). Another limitation of ICB is the associated side effects, named irAEs (39). IrAEs are excessive inflammatory responses induced by ICB therapies that multiple organs can be affected, even leading to death in some cases. It was reported that the overall irAEs incidence in ICB is around 70–90% (40). The most common symptoms of irAEs involving the skin, gastrointestinal tract, liver, endocrine organs, and lungs, while it varies among different types of cancers and therapies (41). For instance, colitis is the most common type of irAEs in the gastrointestinal tract, which occurred in 10-20% patients receiving ICI treatments (42). Cutaneous irAEs, including rash, pruritus and vitiligo, are also common-seen side effects in ICI therapies, of which approximately 50% patients were affected (43). The exact mechanisms for irAE development are still under investigation, while it was proposed that the over-activated T cell attacking normal tissue, uncontrolled secretion of cytokines, expansion of autoantibodies and even binding of ICI antibodies to normal tissues (the off-target effect) are responsible for the development of irAEs (44, 45).
Interestingly, it has been revealed that gut microbiota may affect the efficacy of ICB therapy as well as the incidence of associated irAEs (46, 47). Fecal material transplantation (FMT) has been shown effective in improving the overall response to PD-1 therapy in patients with melanoma or epithelial tumors, which indicates a substantial role of gut microbiota in modulating host immune response following PD-1 treatment (48, 49). On the other side, one study on melanoma patients receiving anti-CTLA-4 treatment showed that the enrichment of Bacteroidetes is strongly correlated with less frequency of colitis (50), which was supported by others (46, 47). Despite these findings, there is still an urgent need to improve the efficiency as well as eliminate the side effects of ICB, which relies on a deep understanding of the mechanism of the host response to ICB as well as an illustration of the interplay between host immune response, ICB as well as gut microbiota.
In recent years, bispecific antibodies treatment has been recognized as another promising approach in cancer immunotherapies (51). Notably, bispecific antibodies targeting both PD-1/PD-L1 and TGF-β named YM101 and M7824 were developed and achieved superior effects against cancers by overcoming the anti-PD1/PD-L1 drug resistance induced by TGF-β (52–54). Given the established interactions between gut microbiota and cancer immune responses, it would be also worthwhile to investigate the potential effects as well as underlying mechanisms of gut microbiota on the therapeutical efficiency of bispecific antibodies.
2.2 Chimeric antigen receptor therapy
As mentioned earlier, chimeric antigen receptor T cell (CAR-T) therapy is characterized by the genetic modification of T cells to strengthen their capability against cancer cells. Traditionally, T cell activation depends on the interaction between TCR and specific antigens (including tumor cell-associated antigens) presented by the Major Histocompatibility complex (MHC) on the cell surface, which is frequently down-regulated by tumor cells (55). To overcome this, a chimeric antigen receptor protein (which is composed of the ectodomain of cancer antigen-specific B cells and the intracellular domain of T cells) is designed and artificially expressed in normal T cells from patients to produce the CAR-T cells (56). Compared with the normal T cells, CAR-T cells exhibit much higher affinity as well as stronger killing activity against tumor cells both in vitro and in vivo (57, 58). CAR-T therapy was first developed for treating blood cancers including lymphoma and leukemia and exhibited promising results compared to conventional therapies (59). Currently, CAR-T therapies have been approved for treating various kinds of cancers including relapsed or refractory multiple myeloma, diffuse large B cell lymphoma (DLBCL), high-grade B-cell lymphoma, primary mediastinal large B-cell lymphoma, acute lymphoblastic leukemia (ALL), etc. (60). Additionally, the potential of CAR-T therapy in treating other types of cancers is also evaluated in both clinical and pre-clinical studies (61).
Nevertheless, there are also notable drawbacks of CAR-T therapy, and one of the most challenging issues is the development of tumor resistance to single antigen targeting CAR constructs. Although the administration of CAR-T cells initially yields high response rates, a significant proportion of patients experienced the loss of target antigen expression either partially or completely, which is known as antigen escape (62). It reported that 70–90% of ALL patients show durable responses to CD19 CAR-T therapy in the initial phase; however, it was followed by the downregulation or loss of CD19 antigen expression in 30–70% of the recurrent proportions (63). Consistently, downregulation of other targets including B-cell maturation antigen (BCMA) was also observed in CAR-T treated multiple myeloma patients (64). Another challenge of CAR-T therapy in clinic is the systemic cytokine release syndrome (CRS), which is characterized by hypotension, cardiac dysfunction, circulatory collapse, respiratory failure, renal failure, multiorgan system failure, etc., and may be life-threatening if not well-controlled (65). Pro-inflammatory IL-1 and IL-6 was identified as the key mediators of CRS in CAR-T therapies; therefore, IL-6/IL-6R blockade has been suggested as potential approaches to eliminate CRS. However, even with the use of tocilizumab, an FDA-approved IL-6R mAb in treating severe CRS, symptoms still persist and eventually lead to patient death (66). To date, an effective strategy against CAR-T therapy-induced CRS is still lacking. In addition, the efficacy of CAR-T therapy on solid tumors is compromised by low ability of tissue infiltration (67), which leads to less promising therapeutic outcomes (68). Localized injection instead of systemic administration was utilized to facilitate tumor infiltration of CAR-T cells, while it is only practical for single tumor lesions/oligometastatic disease (69).
The correlation between gut microbiota and the response/toxicity of CAR-T therapy was recently discovered. According to one cohort study, gut microbiome profile is strongly correlated with response and toxicity following anti-CD19 CAR T cell therapy in B cell malignancy patients, as revealed by distinct bacterial taxa and metabolic pathways in patients treated with/without antibiotics, as well as worse survival and increased neurotoxicity seen in patients exposed to antibiotics (piperacillin/tazobactam, meropenem and imipenem/cilastatin) (70). Nevertheless, there is still largely unknown regarding the role of gut microbiota on CAR-T therapy outcomes and the mechanistic insights are still lacking.
2.3 Other cancer immunotherapies
Beyond the mainstream cancer immunotherapies described above, there are also several other immunotherapies developed or under investigation. IL-2 is a typical example of cytokine therapies and was approved by FDA for treating metastatic renal cell carcinoma in 1992, while the significant toxicities including capillary leak syndrome and multiple organ dysfunction limit the use of IL-2 (71). T cell receptor-engineered T cell (TCR-T) therapy, another subtype of adoptive cell transfer (ACT) therapy just like CAR-T, is characterized by the genetic modification of T cells by implantation with a tumor antigen-specific TCR molecule. The advantage of TCR-T has been well documented in both pre-clinical and clinical studies (72). Beyond that, cancer vaccines and oncolytic virus therapies are also recognized as effective strategies for cancer (4, 5, 61). However, the role of gut microbiota in regulating the efficiency or toxicity of cancer immunotherapies still needs to be addressed.
3 Influence of gut microbiota on cancer immunotherapy
Gut microbiota is a complex community of microorganisms living in digestive tracts, which has the biggest quantities and greatest number of species compared to any other parts of the body (73). It is well recognized that microbes in human gut play fundamental roles in the well-being of the host. Interactions within constituents of the microbiota (bacteria, viruses, and eukaryotes) as well their relationship with the host immune system influence the development of disease in many ways. For example, it protects the host from pathogens by colonizing mucosal surfaces and secreting various antimicrobial substances, which help enhance the immune response (74). In addition, gut microbiota plays a vital role in digestion and metabolism, controlling epithelial cell proliferation and differentiation, regulating insulin resistance, and brain-gut communication (75). With respect to cancer immunotherapies, the composition, biological activity, and metabolic products derived from gut microbiota were shown to have substantial impacts on efficiency as well as side effects of treatments (Figure 1).
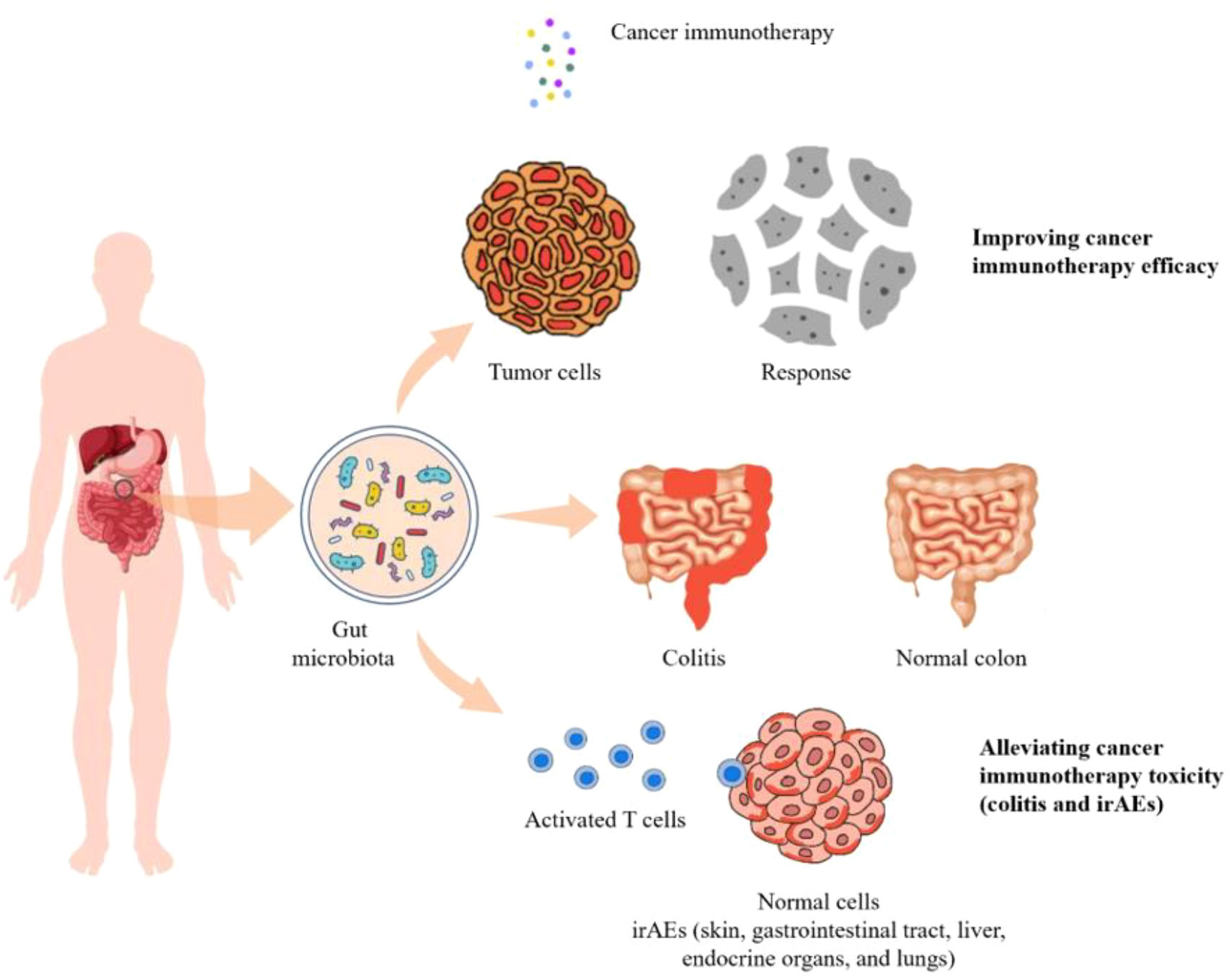
Figure 1 The diversity and composition of the gut microbiome can impact the efficacy as well as the toxicity of cancer immunotherapies including colitis and irAEs.
3.1 Gut microbiota affects the efficiency of cancer immunotherapies
The relationship of gut microbiota and anti-PD-1 efficacy in melanoma has been revealed by previous studies. Sivan et al. examined the subcutaneous growth of B16.SIY melanoma in genetically similar C57BL/6 mice raised in two facilities (76). They found that the tumor growth was more aggressive in one group, which was associated with significantly lower intra-tumoral CD8+ T cell accumulation and was affected by gut microbiota composition. In line with this, Gopalakrishnan et al. examined the gut microbiota of melanoma patients undergoing anti-PD-1 therapy and observed a significant difference in the diversity and composition of the gut microbiota between responders and non-responders (48). Additionally, a retrospective cohort study found that exposure to antibiotics in four weeks before CAR-T therapy might reduce patients survival and increase the incidence of neurotoxicity, which underscored the association between gut microbiota and the efficiency of CAR-T therapy (72). The effects of gut microbiota on CAR-T therapy were also proposed and further supported by the observation of close correlation between gut microbial composition and the response to CAR-T therapy (70, 77–79).
The involvement of specific gut microbial species in cancer immunotherapies has also been accessed. Matson et al. examined the stool samples collected from patients with metastatic melanoma before anti-PD-1 immunotherapy and found that Bifidobacterium longum, Collinsella aerofaciens, and Enterococcus faecium were more abundant in responders, indicating the antitumor effects of Bifidobacterium species in the context of PD-1 immunotherapy (80). Similarly, previous study showed that the antitumor effects of CTLA-4 blockade depend on distinct Bacteroides species, as T cell responses specific for B. thetaiotaomicron or B. fragilis were associated with the efficacy of CTLA-4 blockade (81).
To determine the biological role of gut microbiota in regulating patients’ response to ICB therapy, Davar et al., evaluated the therapeutic efficiency of combined treatment of FMT (from PD-1 responders) and anti-PD-1 administration on patients with PD-1 refractory melanoma (82). It showed that the responders exhibited an increased abundance of taxa that were previously shown to be associated with response to anti–PD-1, increased CD8+ T cell activation, and decreased frequency of IL-8 expressing myeloid cells. Additionally, responders had distinct proteomic and metabolomic signatures, and trans-kingdom network analysis revealed the dominant role of gut microbiota in regulating these changes. These results confirmed the effect of gut microbiota in improving anti-PD-1 efficiency against melanoma. In addition to this, gut microbiota had also been found critical in enhancing the efficacy of PD-1 therapy against other cancer types (83).
The biological role of certain gut microbial species in regulating the efficiency of cancer immunotherapies has been addressed by previous studies. It was revealed that Bifidobacterium species were beneficial in promoting antitumor immune responses during anti-PD-1 treatment (76). The cause-effect relationship between gut colonization of B. fragilis and improved outcome of CTLA-4 blockade therapy has been well demonstrated by fecal material transplantation, and B. fragilis implantation approaches (81). In a similar study, Routy et al., uncovered the role of Akkermansia muciniphila in regulating the response of melanoma patients to anti-PD-1 treatment (49).Oral supplementation with A. muciniphila after fecal material transplantation (FMT) with feces from non-responders significantly restored the efficacy of PD-1 blockade (49). Altogether, these findings highlight the importance of gut microbiota manipulation in improving the efficiency of cancer immunotherapies.
3.2 Gut microbiota affect the toxicities of cancer immunotherapies
Another question regarding gut microbiota in cancer immunotherapies is how the alteration of gut microbes, either in composition or in biological activities, affect the risk of ICB associated toxicities. It showed that the composition of intestinal microbiota can predict whether a patient will develop colitis following treatment with ipilimumab, a monoclonal antibody that blocks CTLA-4 (50). 16S rRNA gene sequencing/16S rDNA sequencing results showed that both the colitis and colitis-free groups had similar microbial compositions before the onset of colitis by ipilimumab administration, however, patients who remained colitis-free following treatment showed a higher proportion of Bacteroidetes phylum. Specifically, Bacteroidaceae, Rikenellaceae, and Barnesiellaceae were more abundant in the feces of colitis resistant patients. Metagenomic sequencing analysis further revealed that 4 microbial modules associated with polyamine transport and B vitamin biosynthesis were more abundant in the microbiota of patients who remained colitis-free. In line with this, gut microbiota can also be used for the prediction of side effect risks in anti-PD-1/PD-L1 therapies. According to an observational study, patients with severe irAEs showed higher abundance of Streptococcus, Paecalibacterium, and Stenotrophomonas, while patients with mild irAEs were enriched in Faecalibacterium and Lachnospiraceae (84). Similarly, another clinical study revealed the distinct compositional differences in gut microbiota between patients who experienced clinically significant or non-significant irAEs (85). In a more comprehensive study led by Dr. Jennifer Wargo, blood, tumor, and gut microbiome of 77 patients with advanced melanoma treated with combined immune checkpoint blockade (CICB) targeting PD-1 and CTLA-4 were profiled, and a significantly higher abundance of Bacteroides intestinalis was found in patients with toxicity characterized by colitis and upregulation of mucosal IL-1β (47). In addition, as mentioned above, exposure to antibiotics was correlated with a high incidence of neurotoxicity in B cell lymphoma and leukemia patients receiving CD19 CAR-T therapy (70). This indirectly confirmed that the gut microbiota could alleviate the related side effects of CAR-T therapy.
As another common side effect of ICB, colitis is commonly treated with immunosuppressive drugs, including corticosteroids and/or agents targeting tumor-necrosis factor-α (TNF-α), all of such have obvious side effects (86). Clinical evidence showing that colitis and inflammatory bowel disease (IBD) can be successfully treated by gut microbiota manipulation aroused the interest to develop new strategies against ICB-induced colitis (87, 88). It revealed that FMT treatment significantly reduced the incidence and severity of colitis in patients receiving ICB therapies, with a relative increase in the proportion of regulatory T-cells within the colonic mucosa area, although the sample number is too limit (n=2) to draw a firm conclusion (46).
Overall, the biological roles of gut microbiota in regulating efficacy as well as toxicity of cancer immunotherapies have been well documented, which is indicative the future development of new strategies in boosting cancer immunotherapy.
4 Dietary fungi in cancer immunotherapies
4.1 Overview of dietary products, gut microbiota, and cancer immunotherapy
With a deeper understanding of gut microbiota, potential ways to optimize gut microbiota in patients and healthy people have also been evaluated recently. Fecal microbiota transplantation (FMT) and single bacteria transplantation (probiotic administration) have achieved promising results in improving the wellness of patients, however, it may be detrimental for patients exposed to the allogenic strains for FMT and make people vulnerable to chronic diseases such as autoimmune disease (AID) (89, 90). Instead, diet intervention or prebiotic supplementation may be more suitable for the general population as it is considered less harmful and easier to be accepted (91). Meanwhile, the regulatory mechanism of diet intervention on gut microbiota is necessary to be elucidated as a pre-requirement. Researchers have been working in this field for decades and many dietary supplements were identified to have “microbiota-modulating” activity. In general, animal-based diets resulted in higher levels of amino acid fermentation products and lower levels of carbohydrate fermentation products, while the levels of amino acid fermentation products were positively correlated with the amounts of putrefactive, bile-tolerant microbes like Bacteroides and Clostridia, saccharolytic microbes were increased as well. In contrast, beneficial bacteria, like Bifidobacteria and Eubacteria were negatively correlated with the consumption of animal products (92). In line with this, high-fat and animal-based diets can promote the growth of Bilophila wadsworthia—a bacteria producing hydrogen sulfide (H2S), which is presumably responsible for GI inflammation (93). Nevertheless, high consumption of polyunsaturated fats fosters Ruminococcus growth inside the gut (94). The role of fiber in plant-based diets has been revealed by several studies. For instance, diets rich in carbohydrates and fiber increase the variety and richness of gut microbiota, characterized by the increase of Bacteroidetes and decrease of Firmicutes/Bacteroidetes ratio (95). High fiber intake also boosted the outgrowth of Firmicutes and Proteobacteria, which were usually low in subjects consuming high-fat diets (96). In contrast to that, the high intake of simple sugar instead of fiber resulted in a substantial outgrowth of Bacteroides (97). Vegetarian diets do not contain any meat or fish while rich in carbohydrates and fiber. Consumption of vegetarian diets led to increased production of short chain fatty acids (SCFA), which is beneficial in preventing GI inflammation and maintaining the homeostasis of microbial flora in the gut (98). Moreover, it was found that protein consumption increases the diversity of gut microbiota; however, the effects vary depending on the source of proteins. Notably, whey and pea protein consumption increased the levels of Bifidobacterium and Lactobacillus, while limiting the growth of Bacteroides fragilis and Clostridium perfringens. In addition, pea protein increased the level of SCFA in GI levels. Meanwhile, an animal-protein-based diet stimulates bile-tolerant anaerobes (90).
Along with the assessment of the role of animal or plant-based diets on gut microbiota, modulating activity of phytochemicals as well as prebiotics on gut microbiota was also evaluated. Phytochemicals, including polyphenols, carotenoids, phytosterols/phytostanols, lignans, alkaloids, have been shown to have positive effects on the modulation of gut microbiota (99). Supplementation of carotenoids such as astaxanthin or retinoic acid help maintains intestinal immune homeostasis by inducing IgA production (100). One study showed that bilberry anthocyanin consumption promoted the efficiency of ICB by modulating the composition of gut microbiota (21). They found that the antitumor efficiency of anti-PD-L1 antibody was enhanced by oral administration of bilberry anthocyanin extracts in a mouse tumor model, which was accompanied by the enrichment of Clostridia and Lactobacillus johnsonii in feces. Prebiotics, including fructooligosaccharides, galactooligosaccharides, soybean oligosaccharides, inulin, etc., exert benefits in the regulation of gut microbiota by increasing the population of commensals like Lactobacillus and Bifidobacterium (100). One study reported that orally administered inulin gel could enhance the antitumor efficacy of α-PD-1 therapy via modulating commensal microorganisms in situ, as well as lead to a potent long-lived antitumor effect via eliciting memory CD8+ T-cell responses (101). Overall, despite interesting findings on natural products from plants and animals, few studies have investigated natural products from dietary fungus, which may also have potential effects on the regulation of composition as well as biological activity of gut microbes.
4.2 Impact of dietary fungi on gut microbiota
Compared with extensive research on the effect of plants or animal-derived diets on gut microbiota regulation, the role of dietary fungus remained unexplored until recently. Lentinula edodes (shiitake) is an edible mushroom enriched with different types of polysaccharides (102), it showed that administration of heteropolysaccharides from Lentinula significantly altered the diversity of microbiota in the small intestine, cecum, colon, and the distal colon (feces) in mice (103). Specifically, the decrease of Bacteroidetes was associated with the increase of Proteobacteria. Of note, Chloroflexi, Gemmatimonadetes, Nitrospirae, and Planctomycetes are exclusively present in treated mice. One study reported that Lentinula edodes by-products (LESDF-3) could promote the production of Bacteroides, indicating the importance of LESDF on the regulation of gut microbiota (104). In line with this, several studies also demonstrated the biological functions of polysaccharides from Cordyceps Sinensis, Auricularia auricular-judae, Ganoderma lucidum, Grifola frondose, Pleurotus ostreatus, Hericium erinaceus, and wild morels in reshaping gut microbiota and immune regulation (105–111). Ganoderma lucidum has long been considered valuable in medication and diet supplementation. According to a recent study, spore oil from Ganoderma lucidum has strong immunoenhancing activity, which was correlated with the elevated abundance of several bacterial genera (Lactobacillus, Turicibacter, and Romboutsia) and species (Lactobacillus_intestinalis and Lactobacillus_reuteri), as well as reduced level of Staphylococcus and Helicobacter (112). Those alterations in gut microbiota further resulted in the secretion of a range of key metabolites such as dopamine, prolyl-glutamine, pentahomomethionine, leucyl-glutamine, L-threonine, stearoylcarnitine, dolichyl β-D-glucosyl phosphate to enhance phagocytosis of macrophages and NK cell cytotoxicity. Inulin, a type of natural polysaccharide that exists in various kinds of natural products has been recognized as a powerful probiotic (113), interestingly, β-glucan from dietary fungus also exhibited comparative effects with inulin (114). Specifically, β-glucan could modulate the structure and composition of gut microbiota by inhibiting the proliferation of harmful gut microbiota while upregulating the abundance of beneficial Bacteroidetes. Furthermore, both β-glucan and inulin could selectively promote the growth of Bifidobacterium. D-glucan from mushrooms also showed similar effects on gut microbiota modulation and thus it can be considered a new type of probiotic. A list of dietary fungi and their roles in modulating gut microbiota is summarized in Table 1.
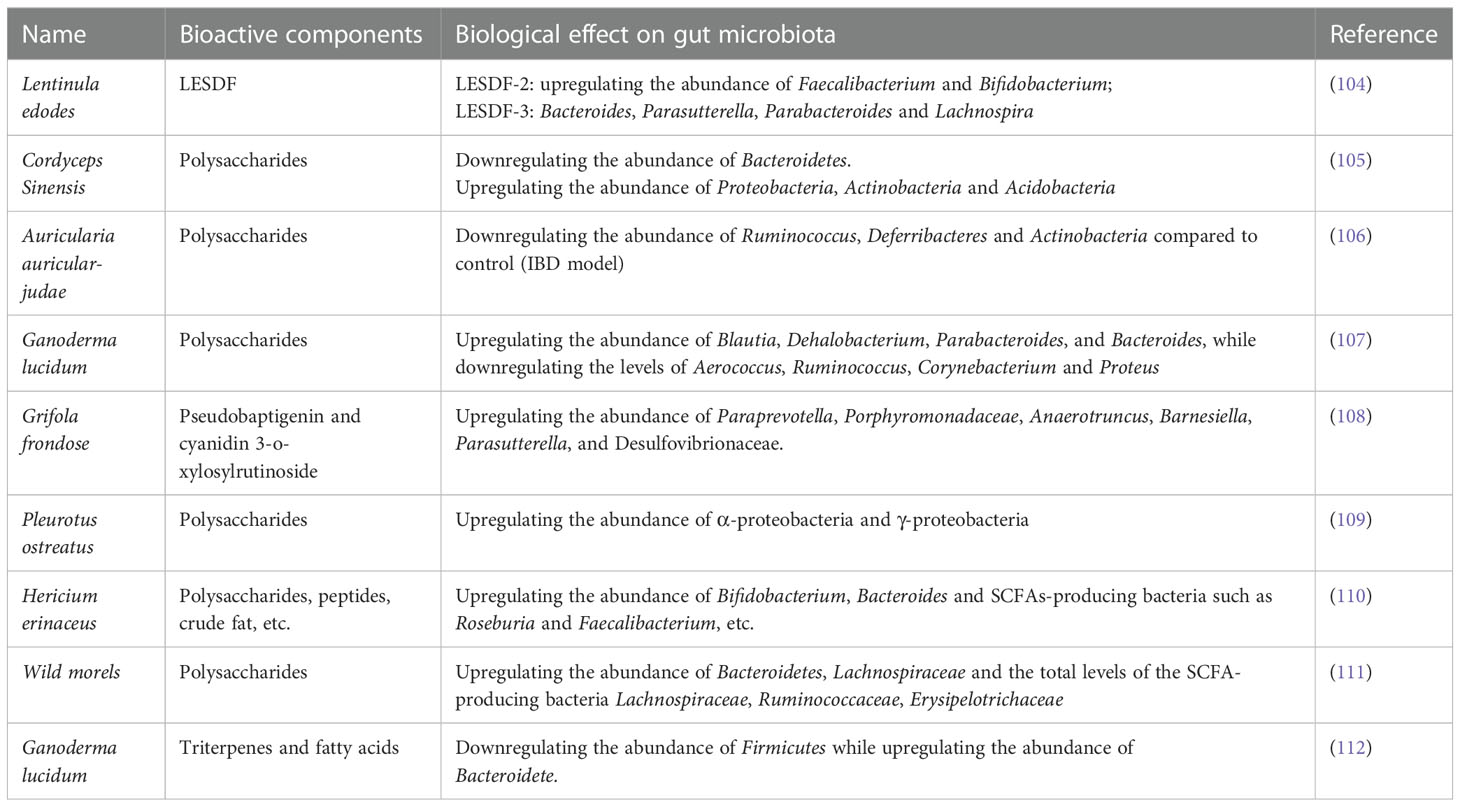
Table 1 List of dietary fungi, the bioactive components, and their role in modulating gut microbiota.
4.3 Impact of dietary fungi on cancer immunotherapy
Despite the well-known effects of plant-based or animal-based diets on cancer immunotherapy, the relationship between dietary fungus and the efficiency of cancer immunotherapy remains elusive. Previous studies have investigated the role of key components from dietary fungus in cancer immune regulation. The main component of the fungal cell wall is β-Glucan, which has been reported to function as potent immunomodulators to enhance antitumor immune responses by regulating differentiation and function of monocytic myeloid-derived suppressor cells (MDSCs) (115). Consistently, polysaccharides from Agaricus blazei Murill stimulated MDSC differentiation from M2 to M1 type, which mediates inhibition of tumor immune evasion via the Toll-like receptor 2 (TLR2) (116). It was later revealed that natural killer (NK) cells, macrophages, and dendritic cells are responsible in mediating fungal products originated antitumor immune response. According to one study, Agaricus bisporus polysaccharides MH751906 exerted immunotherapeutic effect on colon cancer by activating gut-residing NK cells, and these activated NK cells played a stable role in killing human colon cancer cells (117). In line with this, another study reported that Boletus edulis RNA could also stimulate NK cells against myelogenous leukemia (118). In addition, one study indicated that polysaccharides from Luchnum boosted antitumor immune responses by resetting tumor-associated macrophages (TAMs) from the “pro-tumor” M2 to the “antitumor” M1 phenotype (119). Another study uncovered the immunomodulatory activity of polysaccharide−protein complex from Polyporus rhinocerus, exerting antitumor effects by activating macrophage-mediated host immune response (120). Ganoderma lucidum polysaccharides could partially or completely antagonize the suppression of B16F10 melanoma cells on the viability of peritoneal macrophages, suggesting its potential role in cancer immunotherapy (121). Following study found that the antitumor effect of Ganoderma lucidum was from the stimulation of dendritic cell maturation and initiation of the adaptive immune response towards T helper 1 (Th1) polarization in vivo (122). Both studies indicated the immunomodulatory mechanism mediated by Ganoderma lucidum in the antitumor process. Additionally, polysaccharopeptide (PSP) extracted from Coriolus versicolor showed immunotherapeutic effects against tumors via strengthening the phagocytosis of macrophages, increasing the expression of cytokines and chemokines, as well as stimulating the infiltration of both dendritic cells and T-cells into tumors (123).
The exact role of dietary fungi in cancer immunotherapy is still largely unknown except for a few studies reported the relevant effects. One study revealed Cordyceps militaris polysaccharide converted immunosuppressive macrophages into M1 phenotype and activated T lymphocytes by inhibiting the PD-1/PD-L1 axis between TAMs and T lymphocytes, which may improve the effectiveness of anti-PD-1/PD-L1 immunotherapy (124). Another study showed that Ginseng polysaccharides altered the gut microbiota and kynurenine/tryptophan ratio, potentiating the antitumor effect of PD-1/PD-L1 immunotherapy (125), which elucidated the axis of action of fungal polysaccharides through the gut microbiota to strengthen the antitumor effects of ICB. In addition to enhancing the therapeutic effect of ICB, taking fungal products has also been reported to improve the efficacy of cancer vaccines. Oral ingestion of Lentinula edodes mycelia extracts could enhance the antitumor activity of peptide vaccine, which indicated that Lentinus edodes extracts play a vital role in cancer immunotherapy (126). Collectively, it is of particular interest to comprehensively understand the role as well as the molecular mechanism of dietary fungus in cancer immunotherapy, and the involvement of gut microbiota in this process also needs to be further addressed. A list of dietary fungi and their roles in modulating host immune response against cancers is summarized in Table 2.
4.4 Clinical studies for dietary fungi on cancer immunotherapy
To date, a few clinical studies have been conducted to evaluate the antitumor activities of fungal products and the underlying mechanisms. It showed that Grifola frondose could inhibit lung or breast cancer metastasis and decrease the size of tumors (127), which was achieved by increasing NK cell activity and promoting Th1 response, while with the reduced Th2 activity. Another study showed that Ganoderma lucidum polysaccharide has antitumor effects on various types of advanced-stage cancer, which was achieved by stimulating host immune response, including the increased secretion of IL-2, IL-6, IFN-γ, and enhanced NK cell activity, whereas the concentration of IL-1β and TNF-α was reduced compared with baseline (128). According to a study conducted by Zhao et al., 48 breast cancer patients were treated with Ganoderma lucidum spore powder, and it was shown that the concentrations of TNF-α and IL-6 in the serum of patients after treatment decreased significantly, which was accompanied by reduced tumor burden (129). In addition, Pleurotus cornucopiae (Oyster mushroom, Tamogitake) was also found to have antitumor effects through host immune regulation. Tanaka et al. conducted a clinical trial to investigate the antitumor activity of P. cornucopiae, and it was found that the levels of serum IFN-γ and IL-12 increased along with NK cell activity, suggesting the involvement of Th1 immune response in P. cornucopiae directed antitumor activity (130). The antitumor effects on adenocarcinoma as well as the immunomodulation activity of active hexose correlated compound (AHCC, obtained from Lentinus edodes of Basidiomycete mush) was also evaluated clinically, it showed that AHCC treatment led to the increase of neutrophils, the ratio of CD3+/CD4+, CD4+/CD8+, CD3+/CD16+/CD56+ NK cells were also increased accordingly, while the number of lymphocytes and monocytes were reduced (131). In addition, a phase I trial demonstrated the antitumor effect of Agaricus bisporus on prostate cancer by modulating IL-15 level and MDSC activity (132). Recently, several clinical trials are underway to evaluate the antitumor effect of additional fungal extracts and to explore the underlying immunomodulatory mechanisms, while the results have not been reported yet (133). Overall, the antitumor effects of fungal products as well as the molecular mechanisms are well documented, however, most conclusions are drawn only based on small samples. Furthermore, though clinical studies have validated that fungal products can enhance the antitumor effects of chemotherapy and radiotherapy (133), clinical evidence regarding the efficacy of fungal products in cancer immunotherapies is still lacking. Therefore, additional trials are required to investigate the clinical effect of fungal products on cancer immunotherapies. A list of current clinical studies investigating the effects of dietary fungi in different cancers is summarized in Table 3.
5 Conclusion
With the advanced achievement of high-throughput multi-omic technologies including microbial amplicon, meta-genomic, meta-transcriptomic, metabolomic and interatomic analysis in recent years, the biological relevance of gut microbiota on human health has been well recognized. Preclinical studies demonstrated the diverse functions of gut microbe in regulating host immune homeostasis, which is beneficial to protect against many diseases, and in particular, the improvement of cancer immunotherapies. Clinical studies again demonstrated gut microbiota modulating strategies in promoting the outcome of patients. Despite the discovery of various beneficial or harmful microbes/metabolites, however, the underlying mechanism remains unclear, which dampens further development of precise manipulation of gut microbiota. FMT has been proven to be effective in preclinical and clinical studies, while concerns regarding the safety of transferring fecal materials still exist. In 2019, it was reported that 2 patients receiving FMT treatment developed invasive infections caused by multidrug-resistant organisms (MDRO) and one of the patients eventually died (134). FDA of United Stated warned about potential risk of serious infections caused by MDRO related to the investigational use of FMT (135). Therefore, it is necessary to keep alert to FMT therapy–induced adverse events in further clinical investigation. Diet interventions, on the side, are much less concerning as it has been long accepted as common sense, but the effectiveness in gut microbiota modulation has not been well addressed. To solve this problem, studies have tried to identify the “key gradients” of daily diet or supplements, and polysaccharides, or “diet fiber” was eventually demonstrated to be essential for promoting the growth of commensals and maintaining a healthy gut environment; saturated acid from animal products, on the side, were shown negatively impact the gut microbiota characterized by the outgrowth of harmful microbes and production of pro-inflammatory factors. Regardless, it remains elusive for the effects of many other components, the underlying mechanism has yet to be clarified as well.
Immunotherapies have been demonstrated successful in treating various types of cancers and the improvement of patients’ well beings. Meanwhile, the effectiveness varies across individuals along with serious side effects such as neurotoxicity, cytokine release syndrome, colitis, etc. Since gut microbiota was shown closely related to clinical features of cancer patients upon treatment, it is applicable to predict the outcome of any individuals by accessing their gut microbiota. In fact, previous studies have demonstrated the efficiency of such strategies. Beyond that, gut microbiota has also been shown important in regulating the immune response of the host, which highlights its potential role in cancer immunotherapies. To achieve this, many studies have revealed the biological functions of the specific gut microbe and/or metabolites in boosting cancer immunotherapies both pre-clinically and clinically by FMT or single strain administration. Nevertheless, how diet intervention affects the behavior of gut microbiota and how is it related to cancer immunotherapy remains unclear. These questions need to be addressed by molecular identification of effective factors from diet and the validation of their functions by pre-clinical and clinical interventions.
Fungi have long been used in diet and medications, while their effects on gut microbiota had not been noticed until recently (102). Like plant or animal products, edible fungi contain various types of nutrients which can be recognized by gut microbes. As mentioned, the roles of dietary fungi on gut microbiota were discovered and the effective components were also pinpointed in recent studies. However, systemic screenings of fungi-derived components on gut microbiota are still lacking, which is the pre-requirement for a comprehensive understanding of the interplays between dietary fungi and gut microbes, and more studies are needed to identify the underlying mechanism. Ultimately, investigations of dietary fungi and gut microbiota will pave the way to developing therapies against cancer in combination with current immunotherapeutic approaches.
Author contributions
YW, DS, HW, and XL made substantial contributions to the design, data collection, and writing of this manuscript. RW and TL assisted in data evaluation and helpful discussion. HW and XL supervised the project. All authors contributed to the article and approved the submitted version.
Funding
This study was supported by grants from the National Natural Science Foundation of China (82030099, 81972820), the Natural Science Foundation of Shanghai (22ZR1435900), the National Key R&D Program of China (2018YFC2000700), the Science and Technology Commission of Shanghai Municipality (22DZ2303000), Innovative research team of high-level local universities in Shanghai and Shanghai Jiao Tong University Key Program of Medical Engineering (YG2021ZD01).
Conflict of interest
The authors declare that the research was conducted in the absence of any commercial or financial relationships that could be construed as a potential conflict of interest.
Publisher’s note
All claims expressed in this article are solely those of the authors and do not necessarily represent those of their affiliated organizations, or those of the publisher, the editors and the reviewers. Any product that may be evaluated in this article, or claim that may be made by its manufacturer, is not guaranteed or endorsed by the publisher.
References
1. Waldman AD, Fritz JM, Lenardo MJ. A guide to cancer immunotherapy: From T cell basic science to clinical practice. Nat Rev Immunol (2020) 20(11):651–68. doi: 10.1038/s41577-020-0306-5
2. Postow MA, Callahan MK, Wolchok JD. Immune checkpoint blockade in cancer therapy. J Clin Oncol (2015) 33(17):1974–82. doi: 10.1200/JCO.2014.59.4358
3. June CH, O’Connor RS, Kawalekar OU, Ghassemi S, Milone MC. Car T cell immunotherapy for human cancer. Science (2018) 359(6382):1361–5. doi: 10.1126/science.aar6711
4. Wang RF, Rosenberg SA. Human tumor antigens for cancer vaccine development. Immunol Rev (1999) 170:85–100. doi: 10.1111/j.1600-065X.1999.tb01331.x
5. Fukuhara H, Ino Y, Todo T. Oncolytic virus therapy: A new era of cancer treatment at dawn. Cancer Sci (2016) 107(10):1373–9. doi: 10.1111/cas.13027
6. Zhao Q, Jiang Y, Xiang S, Kaboli PJ, Shen J, Zhao Y, et al. Engineered tcr-T cell immunotherapy in anticancer precision medicine: Pros and cons. Front Immunol (2021) 12:658753. doi: 10.3389/fimmu.2021.658753
7. Fujii S, Shimizu K, Okamoto Y, Kunii N, Nakayama T, Motohashi S, et al. Nkt cells as an ideal anti-tumor immunotherapeutic. Front Immunol (2013) 4:409. doi: 10.3389/fimmu.2013.00409
8. Tan S, Li D, Zhu X. Cancer immunotherapy: Pros, cons and beyond. BioMed Pharmacother (2020) 124:109821. doi: 10.1016/j.biopha.2020.109821
9. Kennedy LB, Salama AKS. A review of cancer immunotherapy toxicity. CA: A Cancer J Clin (2020) 70(2):86–104. doi: 10.3322/caac.21596
10. Martins F, Sofiya L, Sykiotis GP, Lamine F, Maillard M, Fraga M, et al. Adverse effects of immune-checkpoint inhibitors: Epidemiology, management and surveillance. Nat Rev Clin Oncol (2019) 16(9):563–80. doi: 10.1038/s41571-019-0218-0
11. Thapa B, Roopkumar J, Kim AS, Gervaso L, Patil PD, Calabrese C, et al. Incidence and clinical pattern of immune related adverse effects (Irae) due to immune checkpoint inhibitors (Ici). J Clin Oncol (2019) 37(15_suppl):e14151–e. doi: 10.1200/JCO.2019.37.15_suppl.e14151
12. Yan Z, Zhang H, Cao J, Zhang C, Liu H, Huang H, et al. Characteristics and risk factors of cytokine release syndrome in chimeric antigen receptor T cell treatment. Front Immunol (2021) 12. doi: 10.3389/fimmu.2021.611366
13. Fan Y, Pedersen O. Gut microbiota in human metabolic health and disease. Nat Rev Microbiol (2021) 19(1):55–71. doi: 10.1038/s41579-020-0433-9
14. Belkaid Y, Hand TW. Role of the microbiota in immunity and inflammation. Cell (2014) 157(1):121–41. doi: 10.1016/j.cell.2014.03.011
15. Pascale A, Marchesi N, Marelli C, Coppola A, Luzi L, Govoni S, et al. Microbiota and metabolic diseases. Endocrine (2018) 61(3):357–71. doi: 10.1007/s12020-018-1605-5
16. Maiuolo J, Gliozzi M, Musolino V, Carresi C, Scarano F, Nucera S, et al. The contribution of gut microbiota-brain axis in the development of brain disorders. Front Neurosci (2021) 15:616883. doi: 10.3389/fnins.2021.616883
17. Cheng WY, Wu C-Y, Yu J. The role of gut microbiota in cancer treatment: Friend or foe? Gut (2020) 69(10):1867. doi: 10.1136/gutjnl-2020-321153
18. Zhou C-B, Zhou Y-L, Fang J-Y. Gut microbiota in cancer immune response and immunotherapy. Trends Cancer (2021) 7(7):647–60. doi: 10.1016/j.trecan.2021.01.010
19. Leeming ER, Johnson AJ, Spector TD, Le Roy CI. Effect of diet on the gut microbiota: Rethinking intervention duration. Nutrients (2019) 11(12):2862. doi: 10.3390/nu11122862
20. Matson V, Gajewski TF. Dietary modulation of the gut microbiome as an immunoregulatory intervention. Cancer Cell (2022) 40(3):246–8. doi: 10.1016/j.ccell.2022.02.014
21. Wang L, Jiang G, Jing N, Liu X, Li Q, Liang W, et al. Bilberry anthocyanin extracts enhance anti-Pd-L1 efficiency by modulating gut microbiota. Food Funct (2020) 11(4):3180–90. doi: 10.1039/d0fo00255k
22. Spencer CN, McQuade JL, Gopalakrishnan V, McCulloch JA, Vetizou M, Cogdill AP, et al. Dietary fiber and probiotics influence the gut microbiome and melanoma immunotherapy response. Science (2021) 374(6575):1632–40. doi: 10.1126/science.aaz7015
23. Ayeka PA. Potential of mushroom compounds as immunomodulators in cancer immunotherapy: A review. Evidence-Based Complementary Altern Med (2018) 2018:7271509. doi: 10.1155/2018/7271509
24. Mellman I, Coukos G, Dranoff G. Cancer immunotherapy comes of age. Nature (2011) 480(7378):480–9. doi: 10.1038/nature10673
25. Kim R, Emi M, Tanabe K. Cancer immunoediting from immune surveillance to immune escape. Immunology (2007) 121(1):1–14. doi: 10.1111/j.1365-2567.2007.02587.x
26. Pardoll DM. The blockade of immune checkpoints in cancer immunotherapy. Nat Rev Cancer (2012) 12(4):252–64. doi: 10.1038/nrc3239
27. Orabona C, Mondanelli G, Puccetti P, Grohmann U. Immune checkpoint molecules, personalized immunotherapy, and autoimmune diabetes. Trends Mol Med (2018) 24(11):931–41. doi: 10.1016/j.molmed.2018.08.005
28. Zarour HM. Reversing T-cell dysfunction and exhaustion in cancer. Clin Cancer Res (2016) 22(8):1856–64. doi: 10.1158/1078-0432.CCR-15-1849
29. Rotte A. Combination of ctla-4 and pd-1 blockers for treatment of cancer. J Exp Clin Cancer Res (2019) 38(1):255. doi: 10.1186/s13046-019-1259-z
30. Forde PM, Chaft JE, Smith KN, Anagnostou V, Cottrell TR, Hellmann MD, et al. Neoadjuvant pd-1 blockade in resectable lung cancer. N Engl J Med (2018) 378(21):1976–86. doi: 10.1056/NEJMoa1716078
31. Gao J, Navai N, Alhalabi O, Siefker-Radtke A, Campbell MT, Tidwell RS, et al. Neoadjuvant pd-L1 plus ctla-4 blockade in patients with cisplatin-ineligible operable high-risk urothelial carcinoma. Nat Med (2020) 26(12):1845–51. doi: 10.1038/s41591-020-1086-y
32. Parry RV, Chemnitz JM, Frauwirth KA, Lanfranco AR, Braunstein I, Kobayashi SV, et al. Ctla-4 and pd-1 receptors inhibit T-cell activation by distinct mechanisms. Mol Cell Biol (2005) 25(21):9543–53. doi: 10.1128/MCB.25.21.9543-9553.2005
33. Nagasaki J, Inozume T, Sax N, Ariyasu R, Ishikawa M, Yamashita K, et al. Pd-1 blockade therapy promotes infiltration of tumor-attacking exhausted T cell clonotypes. Cell Rep (2022) 38(5):110331. doi: 10.1016/j.celrep.2022.110331
34. Bengsch F, Knoblock DM, Liu A, McAllister F, Beatty GL. Ctla-4/Cd80 pathway regulates T cell infiltration into pancreatic cancer. Cancer Immunol Immunother (2017) 66(12):1609–17. doi: 10.1007/s00262-017-2053-4
35. Lee JB, Kim HR, Ha SJ. Immune checkpoint inhibitors in 10 years: Contribution of basic research and clinical application in cancer immunotherapy. Immune Netw (2022) 22(1):e2. doi: 10.4110/in.2022.22.e2
36. Zila N, Hoeller C, Paulitschke V. Novel immune checkpoints beyond pd-1 in advanced melanoma. memo - Magazine Eur Med Oncol (2021) 14(2):135–42. doi: 10.1007/s12254-021-00699-0
37. Fujiwara Y, Mittra A, Naqash AR, Takebe N. A review of mechanisms of resistance to immune checkpoint inhibitors and potential strategies for therapy. Cancer Drug Resistance (2020) 3(3):252–75. doi: 10.20517/cdr.2020.11
38. Zhao B, Zhao H, Zhao J. Efficacy of pd-1/Pd-L1 blockade monotherapy in clinical trials. Ther Adv Med Oncol (2020) 12:1758835920937612. doi: 10.1177/1758835920937612
39. Das S, Johnson DB. Immune-related adverse events and anti-tumor efficacy of immune checkpoint inhibitors. J ImmunoTher Cancer (2019) 7(1):306. doi: 10.1186/s40425-019-0805-8
40. Michot JM, Bigenwald C, Champiat S, Collins M, Carbonnel F, Postel-Vinay S, et al. Immune-related adverse events with immune checkpoint blockade: A comprehensive review. Eur J Cancer (2016) 54:139–48. doi: 10.1016/j.ejca.2015.11.016
41. Geisler AN, Phillips GS, Barrios DM, Wu J, Leung DYM, Moy AP, et al. Immune checkpoint inhibitor-related dermatologic adverse events. J Am Acad Dermatol (2020) 83(5):1255–68. doi: 10.1016/j.jaad.2020.03.132
42. Dougan M. Checkpoint blockade toxicity and immune homeostasis in the gastrointestinal tract. Front Immunol (2017) 8. doi: 10.3389/fimmu.2017.01547
43. Villadolid J, Amin A. Immune checkpoint inhibitors in clinical practice: Update on management of immune-related toxicities. Trans Lung Cancer Res (2015) 4(5):560–75. doi: 10.3978/j.issn.2218-6751.2015.06.06
44. Sullivan RJ, Weber JS. Immune-related toxicities of checkpoint inhibitors: Mechanisms and mitigation strategies. Nat Rev Drug Discovery (2022) 21(7):495–508. doi: 10.1038/s41573-021-00259-5
45. Lozano AX, Chaudhuri AA, Nene A, Bacchiocchi A, Earland N, Vesely MD, et al. T Cell characteristics associated with toxicity to immune checkpoint blockade in patients with melanoma. Nat Med (2022) 28(2):353–62. doi: 10.1038/s41591-021-01623-z
46. Wang Y, Wiesnoski DH, Helmink BA, Gopalakrishnan V, Choi K, DuPont HL, et al. Fecal microbiota transplantation for refractory immune checkpoint inhibitor-associated colitis. Nat Med (2018) 24(12):1804–8. doi: 10.1038/s41591-018-0238-9
47. Andrews MC, Duong CPM, Gopalakrishnan V, Iebba V, Chen WS, Derosa L, et al. Gut microbiota signatures are associated with toxicity to combined ctla-4 and pd-1 blockade. Nat Med (2021) 27(8):1432–41. doi: 10.1038/s41591-021-01406-6
48. Gopalakrishnan V, Spencer CN, Nezi L, Reuben A, Andrews MC, Karpinets TV, et al. Gut microbiome modulates response to anti-Pd-1 immunotherapy in melanoma patients. Science (2018) 359(6371):97–103. doi: 10.1126/science.aan4236
49. Routy B, Le Chatelier E, Derosa L, Duong CPM, Alou MT, Daillère R, et al. Gut microbiome influences efficacy of pd-1-Based immunotherapy against epithelial tumors. Science (2018) 359(6371):91–7. doi: 10.1126/science.aan3706
50. Dubin K, Callahan MK, Ren B, Khanin R, Viale A, Ling L, et al. Intestinal microbiome analyses identify melanoma patients at risk for checkpoint-Blockade-Induced colitis. Nat Commun (2016) 7:10391. doi: 10.1038/ncomms10391
51. Ordóñez-Reyes C, Garcia-Robledo JE, Chamorro DF, Mosquera A, Sussmann L, Ruiz-Patiño A, et al. Bispecific antibodies in cancer immunotherapy: A novel response to an old question. Pharmaceutics (2022) 14(6):1243. doi: 10.3390/pharmaceutics14061243
52. Yi M, Zhang J, Li A, Niu M, Yan Y, Jiao Y, et al. The construction, expression, and enhanced anti-tumor activity of Ym101: A bispecific antibody simultaneously targeting tgf-β and pd-L1. J Hematol Oncol (2021) 14(1):27. doi: 10.1186/s13045-021-01045-x
53. Yi M, Wu Y, Niu M, Zhu S, Zhang J, Yan Y, et al. Anti-Tgf-β/Pd-L1 bispecific antibody promotes T cell infiltration and exhibits enhanced antitumor activity in triple-negative breast cancer. J ImmunoTher Cancer (2022) 10(12):e005543. doi: 10.1136/jitc-2022-005543
54. Knudson KM, Hicks KC, Luo X, Chen JQ, Schlom J, Gameiro SR. M7824, a novel bifunctional anti-Pd-L1/Tgfβ trap fusion protein, promotes anti-tumor efficacy as monotherapy and in combination with vaccine. Oncoimmunology (2018) 7(5):e1426519. doi: 10.1080/2162402X.2018.1426519
55. Zagzag D, Salnikow K, Chiriboga L, Yee H, Lan L, Ali MA, et al. Downregulation of major histocompatibility complex antigens in invading glioma cells: Stealth invasion of the brain. Lab Invest (2005) 85(3):328–41. doi: 10.1038/labinvest.3700233
56. Sadelain M, Brentjens R, Rivière I. The basic principles of chimeric antigen receptor design. Cancer Discovery (2013) 3(4):388–98. doi: 10.1158/2159-8290.CD-12-0548
57. Irving BA, Weiss A. The cytoplasmic domain of the T cell receptor zeta chain is sufficient to couple to receptor-associated signal transduction pathways. Cell (1991) 64(5):891–901. doi: 10.1016/0092-8674(91)90314-O
58. Makita S, Yoshimura K, Tobinai K. Clinical development of anti-Cd19 chimeric antigen receptor T-cell therapy for b-cell non-Hodgkin lymphoma. Cancer Sci (2017) 108(6):1109–18. doi: 10.1111/cas.13239
59. Zhao Z, Chen Y, Francisco NM, Zhang Y, Wu M. The application of car-T cell therapy in hematological malignancies: Advantages and challenges. Acta Pharm Sin B (2018) 8(4):539–51. doi: 10.1016/j.apsb.2018.03.001
60. Sengsayadeth S, Savani BN, Oluwole O, Dholaria B. Overview of approved car-T therapies, ongoing clinical trials, and its impact on clinical practice. eJHaem (2022) 3(S1):6–10. doi: 10.1002/jha2.338
61. Zarezadeh Mehrabadi A, Roozbahani F, Ranjbar R, Farzanehpour M, Shahriary A, Dorostkar R, et al. Overview of the pre-clinical and clinical studies about the use of car-T cell therapy of cancer combined with oncolytic viruses. World J Surg Oncol (2022) 20(1):16. doi: 10.1186/s12957-021-02486-x
62. Majzner RG, Mackall CL. Tumor antigen escape from car T-cell therapy. Cancer Discovery (2018) 8(10):1219–26. doi: 10.1158/2159-8290.CD-18-0442
63. Sterner RC, Sterner RM. Car-T cell therapy: Current limitations and potential strategies. Blood Cancer J (2021) 11(4):69. doi: 10.1038/s41408-021-00459-7
64. García-Guerrero E, Sierro-Martínez B, Pérez-Simón JA. Overcoming chimeric antigen receptor (Car) modified T-cell therapy limitations in multiple myeloma. Front Immunol (2020) 11:1128. doi: 10.3389/fimmu.2020.01128
65. Frey N, Porter D. Cytokine release syndrome with chimeric antigen receptor T cell therapy. Biol Blood Marrow Transplant (2019) 25(4):e123–e7. doi: 10.1016/j.bbmt.2018.12.756
66. Giavridis T, van der Stegen SJC, Eyquem J, Hamieh M, Piersigilli A, Sadelain M. Car T cell-induced cytokine release syndrome is mediated by macrophages and abated by il-1 blockade. Nat Med (2018) 24(6):731–8. doi: 10.1038/s41591-018-0041-7
67. Marofi F, Motavalli R, Safonov VA, Thangavelu L, Yumashev AV, Alexander M, et al. Car T cells in solid tumors: Challenges and opportunities. Stem Cell Res Ther (2021) 12(1):81. doi: 10.1186/s13287-020-02128-1
68. Qian S, Villarejo-Campos P, Guijo I, Hernández-Villafranca S, García-Olmo D, González-Soares S, et al. Update for advance car-T therapy in solid tumors, clinical application in peritoneal carcinomatosis from colorectal cancer and future prospects. Front Immunol (2022) 13. doi: 10.3389/fimmu.2022.841425
69. Li H, Wang Z, Ogunnaike EA, Wu Q, Chen G, Hu Q, et al. Scattered seeding of car T cells in solid tumors augments anticancer efficacy. Natl Sci Rev (2021) 9(3):nwab172. doi: 10.1093/nsr/nwab172
70. Smith M, Dai A, Ghilardi G, Amelsberg KV, Devlin SM, Pajarillo R, et al. Gut microbiome correlates of response and toxicity following anti-Cd19 car T cell therapy. Nat Med (2022) 28(4):713–23. doi: 10.1038/s41591-022-01702-9
71. Jiang T, Zhou C, Ren S. Role of il-2 in cancer immunotherapy. Oncoimmunology (2016) 5(6):e1163462. doi: 10.1080/2162402X.2016.1163462
72. Tsimberidou A-M, Van Morris K, Vo HH, Eck S, Lin Y-F, Rivas JM, et al. T-Cell receptor-based therapy: An innovative therapeutic approach for solid tumors. J Hematol Oncol (2021) 14(1):102. doi: 10.1186/s13045-021-01115-0
73. Thursby E, Juge N. Introduction to the human gut microbiota. Biochem J (2017) 474(11):1823–36. doi: 10.1042/BCJ20160510
74. Cheng HY, Ning MX, Chen DK, Ma WT. Interactions between the gut microbiota and the host innate immune response against pathogens. Front Immunol (2019) 10:607. doi: 10.3389/fimmu.2019.00607
75. Aziz Q, Doré J, Emmanuel A, Guarner F, Quigley EM. Gut microbiota and gastrointestinal health: Current concepts and future directions. Neurogastroenterol Motil (2013) 25(1):4–15. doi: 10.1111/nmo.12046
76. Sivan A, Corrales L, Hubert N, Williams JB, Aquino-Michaels K, Earley ZM, et al. Commensal bifidobacterium promotes antitumor immunity and facilitates anti-Pd-L1 efficacy. Science (2015) 350(6264):1084–9. doi: 10.1126/science.aac4255
77. Abid MB, Shah NN, Maatman TC, Hari PN. Gut microbiome and car-T therapy. Exp Hematol Oncol (2019) 8(1):31. doi: 10.1186/s40164-019-0155-8
78. Schubert ML, Rohrbach R, Schmitt M, Stein-Thoeringer CK. The potential role of the intestinal micromilieu and individual microbes in the immunobiology of chimeric antigen receptor T-cell therapy. Front Immunol (2021) 12:670286. doi: 10.3389/fimmu.2021.670286
79. Innao V, Allegra AG, Musolino C, Allegra A. New frontiers about the role of human microbiota in immunotherapy: The immune checkpoint inhibitors and car T-cell therapy era. Int J Mol Sci (2020) 21(23):8902. doi: 10.3390/ijms21238902
80. Matson V, Fessler J, Bao R, Chongsuwat T, Zha Y, Alegre ML, et al. The commensal microbiome is associated with anti-Pd-1 efficacy in metastatic melanoma patients. Science (2018) 359(6371):104–8. doi: 10.1126/science.aao3290
81. Vétizou M, Pitt JM, Daillère R, Lepage P, Waldschmitt N, Flament C, et al. Anticancer immunotherapy by ctla-4 blockade relies on the gut microbiota. Science (2015) 350(6264):1079–84. doi: 10.1126/science.aad1329
82. Davar D, Dzutsev AK, McCulloch JA, Rodrigues RR, Chauvin JM, Morrison RM, et al. Fecal microbiota transplant overcomes resistance to anti-Pd-1 therapy in melanoma patients. Science (2021) 371(6529):595–602. doi: 10.1126/science.abf3363
83. Wu J, Wang S, Zheng B, Qiu X, Wang H, Chen L. Modulation of gut microbiota to enhance effect of checkpoint inhibitor immunotherapy. Front Immunol (2021) 12. doi: 10.3389/fimmu.2021.669150
84. Liu W, Ma F, Sun B, Liu Y, Tang H, Luo J, et al. Intestinal microbiome associated with immune-related adverse events for patients treated with anti-Pd-1 inhibitors, a real-world study. Front Immunol (2021) 12:756872. doi: 10.3389/fimmu.2021.756872
85. Hakozaki T, Richard C, Okuma Y, Derosa L, Elkrief A, Zitvogel L, et al. Gut microbiome to predict efficacy and immune-related toxicities in patients with advanced non-small cell lung cancer treated with anti-Pd-1/Pd-L1 antibody-based immunotherapy. J Clin Oncol (2020) 38(15_suppl):3095–. doi: 10.1200/JCO.2020.38.15_suppl.3095
86. Som A, Mandaliya R, Alsaadi D, Farshidpour M, Charabaty A, Malhotra N, et al. Immune checkpoint inhibitor-induced colitis: A comprehensive review. World J Clin cases (2019) 7(4):405–18. doi: 10.12998/wjcc.v7.i4.405
87. Chen P, Xu H, Tang H, Zhao F, Yang C, Kwok LY, et al. Modulation of gut mucosal microbiota as a mechanism of probiotics-based adjunctive therapy for ulcerative colitis. Microb Biotechnol (2020) 13(6):2032–43. doi: 10.1111/1751-7915.13661
88. Eindor-Abarbanel A, Healey GR, Jacobson K. Therapeutic advances in gut microbiome modulation in patients with inflammatory bowel disease from pediatrics to adulthood. Int J Mol Sci (2021) 22(22):12506. doi: 10.3390/ijms222212506
89. Smith MB, Kelly C, Alm EJ. Policy: How to regulate faecal transplants. Nature (2014) 506(7488):290–1. doi: 10.1038/506290a
90. Marrs T, Walter J. Pros and cons: Is faecal microbiota transplantation a safe and efficient treatment option for gut dysbiosis? Allergy (2021) 76(7):2312–7. doi: 10.1111/all.14750
91. Clancy AK, Gunaratne AW, Borody TJ. Dietary management for faecal microbiota transplant: An international survey of clinical and research practice, knowledge and attitudes. Front Nutr (2021) 8:653653. doi: 10.3389/fnut.2021.653653
92. Shortt C, Hasselwander O, Meynier A, Nauta A, Fernández EN, Putz P, et al. Systematic review of the effects of the intestinal microbiota on selected nutrients and non-nutrients. Eur J Nutr (2018) 57(1):25–49. doi: 10.1007/s00394-017-1546-4
93. Ojeda P, Bobe A, Dolan K, Leone V, Martinez K. Nutritional modulation of gut microbiota - the impact on metabolic disease pathophysiology. J Nutr Biochem (2016) 28:191–200. doi: 10.1016/j.jnutbio.2015.08.013
94. Szczyrek M, Bitkowska P, Chunowski P, Czuchryta P, Krawczyk P, Milanowski J. Diet, microbiome, and cancer immunotherapy-a comprehensive review. Nutrients (2021) 13(7):2217. doi: 10.3390/nu13072217
95. Beam A, Clinger E, Hao L. Effect of diet and dietary components on the composition of the gut microbiota. Nutrients (2021) 13(8):2795. doi: 10.3390/nu13082795
96. Cronin P, Joyce SA, O’Toole PW, O’Connor EM. Dietary fibre modulates the gut microbiota. Nutrients (2021) 13(5):1655. doi: 10.3390/nu13051655
97. Satokari R. High intake of sugar and the balance between pro- and anti-inflammatory gut bacteria. Nutrients (2020) 12(5):1348. doi: 10.3390/nu12051348
98. Tomova A, Bukovsky I, Rembert E, Yonas W, Alwarith J, Barnard ND, et al. The effects of vegetarian and vegan diets on gut microbiota. Front Nutr (2019) 6:47. doi: 10.3389/fnut.2019.00047
99. Dingeo G, Brito A, Samouda H, Iddir M, La Frano MR, Bohn T. Phytochemicals as modifiers of gut microbial communities. Food Funct (2020) 11(10):8444–71. doi: 10.1039/D0FO01483D
100. Lyu Y, Wu L, Wang F, Shen X, Lin D. Carotenoid supplementation and retinoic acid in immunoglobulin a regulation of the gut microbiota dysbiosis. Exp Biol Med (Maywood) (2018) 243(7):613–20. doi: 10.1177/1535370218763760
101. Han K, Nam J, Xu J, Sun X, Huang X, Animasahun O, et al. Generation of systemic antitumour immunity Via the in situ modulation of the gut microbiome by an orally administered inulin gel. Nat BioMed Eng (2021) 5(11):1377–88. doi: 10.1038/s41551-021-00749-2
102. Valverde ME, Hernández-Pérez T, Paredes-López O. Edible mushrooms: Improving human health and promoting quality life. Int J Microbiol (2015) 2015:376387. doi: 10.1155/2015/376387
103. Xu X, Zhang X. Lentinula edodes-derived polysaccharide alters the spatial structure of gut microbiota in mice. PloS One (2015) 10(1):e0115037. doi: 10.1371/journal.pone.0115037
104. Xue Z, Ma Q, Chen Y, Lu Y, Wang Y, Jia Y, et al. Structure characterization of soluble dietary fiber fractions from mushroom lentinula edodes (Berk.) pegler and the effects on fermentation and human gut microbiota in vitro. Food Res Int (2020) 129:108870. doi: 10.1016/j.foodres.2019.108870
105. Chen L, Zhang L, Wang W, Qiu W, Liu L, Ning A, et al. Polysaccharides isolated from cordyceps sinensis contribute to the progression of Nash by modifying the gut microbiota in mice fed a high-fat diet. PloS One (2020) 15(6):e0232972. doi: 10.1371/journal.pone.0232972
106. Zhao D, Dai W, Tao H, Zhuang W, Qu M, Chang YN. Polysaccharide isolated from auricularia auricular-judae (Bull.) prevents dextran sulfate sodium-induced colitis in mice through modulating the composition of the gut microbiota. J Food Sci (2020) 85(9):2943–51. doi: 10.1111/1750-3841.15319
107. Chen M, Xiao D, Liu W, Song Y, Zou B, Li L, et al. Intake of ganoderma lucidum polysaccharides reverses the disturbed gut microbiota and metabolism in type 2 diabetic rats. Int J Biol Macromol (2020) 155:890–902. doi: 10.1016/j.ijbiomac.2019.11.047
108. Deng J, Guo W, Guo J, Li Y, Zhou W, Lv W, et al. Regulatory effects of a grifola frondosa extract rich in pseudobaptigenin and cyanidin-3-O-Xylosylrutinoside on glycolipid metabolism and the gut microbiota in high-fat diet-fed rats. J Funct Foods (2020) 75:104230. doi: 10.1016/j.jff.2020.104230
109. Song X, Feng Z, Tan J, Wang Z, Zhu W. Dietary administration of pleurotus ostreatus polysaccharides (Pops) modulates the non-specific immune response and gut microbiota diversity of apostichopus japonicus. Aquacult Rep (2021) 19:100578. doi: 10.1016/j.aqrep.2020.100578
110. Xie XQ, Geng Y, Guan Q, Ren Y, Guo L, Lv Q, et al. Influence of short-term consumption of hericium erinaceus on serum biochemical markers and the changes of the gut microbiota: A pilot study. Nutrients (2021) 13(3):1008. doi: 10.3390/nu13031008
111. Huo W, Qi P, Cui L, Zhang L, Dai L, Liu Y, et al. Polysaccharide from wild morels alters the spatial structure of gut microbiota and the production of short-chain fatty acids in mice. Biosci Microbiota Food Health (2020) 39(4):219–26. doi: 10.12938/bmfh.2020-018
112. Wu X, Cao J, Li M, Yao P, Li H, Xu W, et al. An integrated microbiome and metabolomic analysis identifies immunoenhancing features of ganoderma lucidum spores oil in mice. Pharmacol Res (2020) 158:104937. doi: 10.1016/j.phrs.2020.104937
113. Vandeputte D, Falony G, Vieira-Silva S, Wang J, Sailer M, Theis S, et al. Prebiotic inulin-type fructans induce specific changes in the human gut microbiota. Gut (2017) 66(11):1968. doi: 10.1136/gutjnl-2016-313271
114. Wang H, Chen G, Li X, Zheng F, Zeng X. Yeast β-glucan, a potential prebiotic, showed a similar probiotic activity to inulin. Food Funct (2020) 11(12):10386–96. doi: 10.1039/d0fo02224a
115. Tian J, Ma J, Ma K, Guo H, Baidoo SE, Zhang Y, et al. β-glucan enhances antitumor immune responses by regulating differentiation and function of monocytic myeloid-derived suppressor cells. Eur J Immunol (2013) 43(5):1220–30. doi: 10.1002/eji.201242841
116. Liu Y, Zhang L, Zhu X, Wang Y, Liu W, Gong W. Polysaccharide agaricus blazei murill stimulates myeloid derived suppressor cell differentiation from M2 to M1 type, which mediates inhibition of tumour immune-evasion Via the toll-like receptor 2 pathway. Immunology (2015) 146(3):379–91. doi: 10.1111/imm.12508
117. El-Deeb NM, Ibrahim OM, Mohamed MA, Farag MMS, Farrag AA, El-Aassar MR. Alginate/κ-carrageenan oral microcapsules loaded with agaricus bisporus polysaccharides Mh751906 for natural killer cells mediated colon cancer immunotherapy. Int J Biol Macromolecules (2022) 205:385–95. doi: 10.1016/j.ijbiomac.2022.02.058
118. Lemieszek MK, Nunes F, Sawa-Wejksza K, Rzeski W. A king bolete, boletus edulis (Agaricomycetes), rna fraction stimulates proliferation and cytotoxicity of natural killer cells against myelogenous leukemia cells. Int J Med Mushrooms (2017) 19(4):347–53. doi: 10.1615/IntJMedMushrooms.v19.i4.50
119. Zong S, Li J, Ye Z, Zhang X, Yang L, Chen X, et al. Lachnum polysaccharide suppresses S180 sarcoma by boosting anti-tumor immune responses and skewing tumor-associated macrophages toward M1 phenotype. Int J Biol Macromol (2020) 144:1022–33. doi: 10.1016/j.ijbiomac.2019.09.179
120. Liu C, Chen J, Chen L, Huang X, Cheung PC. Immunomodulatory activity of polysaccharide-protein complex from the mushroom sclerotia of polyporus rhinocerus in murine macrophages. J Agric Food Chem (2016) 64(16):3206–14. doi: 10.1021/acs.jafc.6b00932
121. Lu J, Sun LX, Lin ZB, Duan XS, Ge ZH, Xing EH, et al. Antagonism by ganoderma lucidum polysaccharides against the suppression by culture supernatants of B16f10 melanoma cells on macrophage. Phytother Res (2014) 28(2):200–6. doi: 10.1002/ptr.4980
122. Wang CL, Lu CY, Hsueh YC, Liu WH, Chen CJ. Activation of antitumor immune responses by ganoderma formosanum polysaccharides in tumor-bearing mice. Appl Microbiol Biotechnol (2014) 98(22):9389–98. doi: 10.1007/s00253-014-6027-6
123. Dou H, Chang Y, Zhang L. Coriolus versicolor polysaccharopeptide as an immunotherapeutic in China. Prog Mol Biol Transl Sci (2019) 163:361–81. doi: 10.1016/bs.pmbts.2019.03.001
124. Bi S, Huang W, Chen S, Huang C, Li C, Guo Z, et al. Cordyceps militaris polysaccharide converts immunosuppressive macrophages into M1-like phenotype and activates T lymphocytes by inhibiting the pd-L1/Pd-1 axis between tams and T lymphocytes. Int J Biol Macromol (2020) 150:261–80. doi: 10.1016/j.ijbiomac.2020.02.050
125. Huang J, Liu D, Wang Y, Liu L, Li J, Yuan J, et al. Ginseng polysaccharides alter the gut microbiota and Kynurenine/Tryptophan ratio, potentiating the antitumour effect of antiprogrammed cell death 1/Programmed cell death ligand 1 (Anti-Pd-1/Pd-L1) immunotherapy. Gut (2022) 71(4):734–45. doi: 10.1136/gutjnl-2020-321031
126. Tanaka K, Ishikawa S, Matsui Y, Kawanishi T, Tamesada M, Harashima N, et al. Combining a peptide vaccine with oral ingestion of lentinula edodes mycelia extract enhances anti-tumor activity in B16 melanoma-bearing mice. Cancer Immunol Immunother (2012) 61(11):2143–52. doi: 10.1007/s00262-012-1275-8
127. Kodama N, Komuta K, Nanba H. Effect of maitake (Grifola frondosa) d-fraction on the activation of nk cells in cancer patients. J Med Food (2003) 6(4):371–7. doi: 10.1089/109662003772519949
128. Gao Y, Zhou S, Jiang W, Huang M, Dai X. Effects of ganopoly (a ganoderma lucidum polysaccharide extract) on the immune functions in advanced-stage cancer patients. Immunol Invest (2003) 32(3):201–15. doi: 10.1081/IMM-120022979
129. Zhao H, Zhang Q, Zhao L, Huang X, Wang J, Kang X. Spore powder of ganoderma lucidum improves cancer-related fatigue in breast cancer patients undergoing endocrine therapy: A pilot clinical trial. Evid Based Complement Alternat Med (2012) 2012:809614. doi: 10.1155/2012/809614
130. Tanaka A, Nishimura M, Sato Y, Sato H, Nishihira J. Enhancement of the Th1-phenotype immune system by the intake of oyster mushroom (Tamogitake) extract in a double-blind, placebo-controlled study. J Tradit Complement Med (2016) 6(4):424–30. doi: 10.1016/j.jtcme.2015.11.004
131. Del Buono A, Bonucci M, Pugliese S, D’orta A, Fioranelli M. Polysaccharide from lentinus edodes for integrative cancer treatment: Immunomodulatory effects on lymphocyte population. WCRJ (2016) 3(1):1–7. https://www.wcrj.net/article/652
132. Twardowski P, Kanaya N, Frankel P, Synold T, Ruel C, Pal SK, et al. A phase I trial of mushroom powder in patients with biochemically recurrent prostate cancer: Roles of cytokines and myeloid-derived suppressor cells for agaricus bisporus-induced prostate-specific antigen responses. Cancer (2015) 121(17):2942–50. doi: 10.1002/cncr.29421
133. Panda SK, Luyten W. Medicinal mushrooms: Clinical perspective and challenges. Drug Discovery Today (2022) 27(2):636–51. doi: 10.1016/j.drudis.2021.11.017
134. DeFilipp Z, Bloom PP, Torres Soto M, Mansour MK, Sater MRA, Huntley MH, et al. Drug-resistant e. coli bacteremia transmitted by fecal microbiota transplant. New Engl J Med (2019) 381(21):2043–50. doi: 10.1056/NEJMoa1910437
Keywords: cancer, immunotherapy, gut microbiota, dietary intervention, dietary fungi
Citation: Wei Y, Song D, Wang R, Li T, Wang H and Li X (2023) Dietary fungi in cancer immunotherapy: From the perspective of gut microbiota. Front. Oncol. 13:1038710. doi: 10.3389/fonc.2023.1038710
Received: 07 September 2022; Accepted: 27 February 2023;
Published: 08 March 2023.
Edited by:
Junmin Zhang, Lanzhou University, ChinaCopyright © 2023 Wei, Song, Wang, Li, Wang and Li. This is an open-access article distributed under the terms of the Creative Commons Attribution License (CC BY). The use, distribution or reproduction in other forums is permitted, provided the original author(s) and the copyright owner(s) are credited and that the original publication in this journal is cited, in accordance with accepted academic practice. No use, distribution or reproduction is permitted which does not comply with these terms.
*Correspondence: Xiaoguang Li, bGl4Z0BzaHNtdS5lZHUuY24=; Hui Wang, aHVpd2FuZ0BzaHNtdS5lZHUuY24=
†These authors have contributed equally to this work