- 1Department of Neurosurgery, First Affiliated Hospital of Xinjiang Medical University, Urumqi, China
- 2Department of Four Comprehensive Internal Medicine, The First Affiliated Hospital of Xinjiang Medical University, Xinjiang, China
- 3School of Health Management, Xinjiang Medical University, Urumqi, China
- 4Department of Neurosurgery, Xinjiang Bazhou People’s Hospital, Xinjiang, China
Metastasis is one of the important biological features of malignant tumors and one of the main factors responsible for poor prognosis. Although the widespread application of newer clinical technologies and their continuous development have significantly improved survival in patients with brain metastases, there is no uniform standard of care. More effective therapeutic measures are therefore needed to improve prognosis. Understanding the mechanisms of tumor cell colonization, growth, and invasion in the central nervous system is of particular importance for the prevention and treatment of brain metastases. This process can be plausibly explained by the “seed and soil” hypothesis, which essentially states that tumor cells can interact with various components of the central nervous system microenvironment to produce adaptive changes; it is this interaction that determines the development of brain metastases. As a novel form of intercellular communication, exosomes play a key role in the brain metastasis microenvironment and carry various bioactive molecules that regulate receptor cell activity. In this paper, we review the roles and prospects of brain metastatic tumor cells, the brain metastatic tumor microenvironment, and exosomes in the development and clinical management of brain metastases.
Introduction
In the natural course, brain metastases occur in approximately 20–40% of patients with malignancies. Lung cancers are the most common source of brain metastases (40%–50%), and are followed by cancers of the breast (15%–20%), skin (mainly melanoma; 5%–10%), and gastrointestinal system (4%–6%) (Figure 1) (1). The survival and quality of life of patients with cancer have improved considerably in recent years due to the advent of newer treatment methods, and especially precision therapy. However, owing to the anatomical and physiological peculiarities of the central nervous system (CNS), it is difficult to achieve the desired effect of various treatments; brain metastases are therefore known as the last refuge of malignant tumors (2, 3). The median survival period in patients with untreated brain metastases is 1-2 months, while that of those who have been treated is approximately 6 months (4). The currently available treatments for brain metastases mainly include radiotherapy, systemic chemotherapy, and surgery. In this context, the widespread use of targeted drugs has improved the prognosis of these patients to a certain extent (5). However, the overall prognosis remains unsatisfactory.
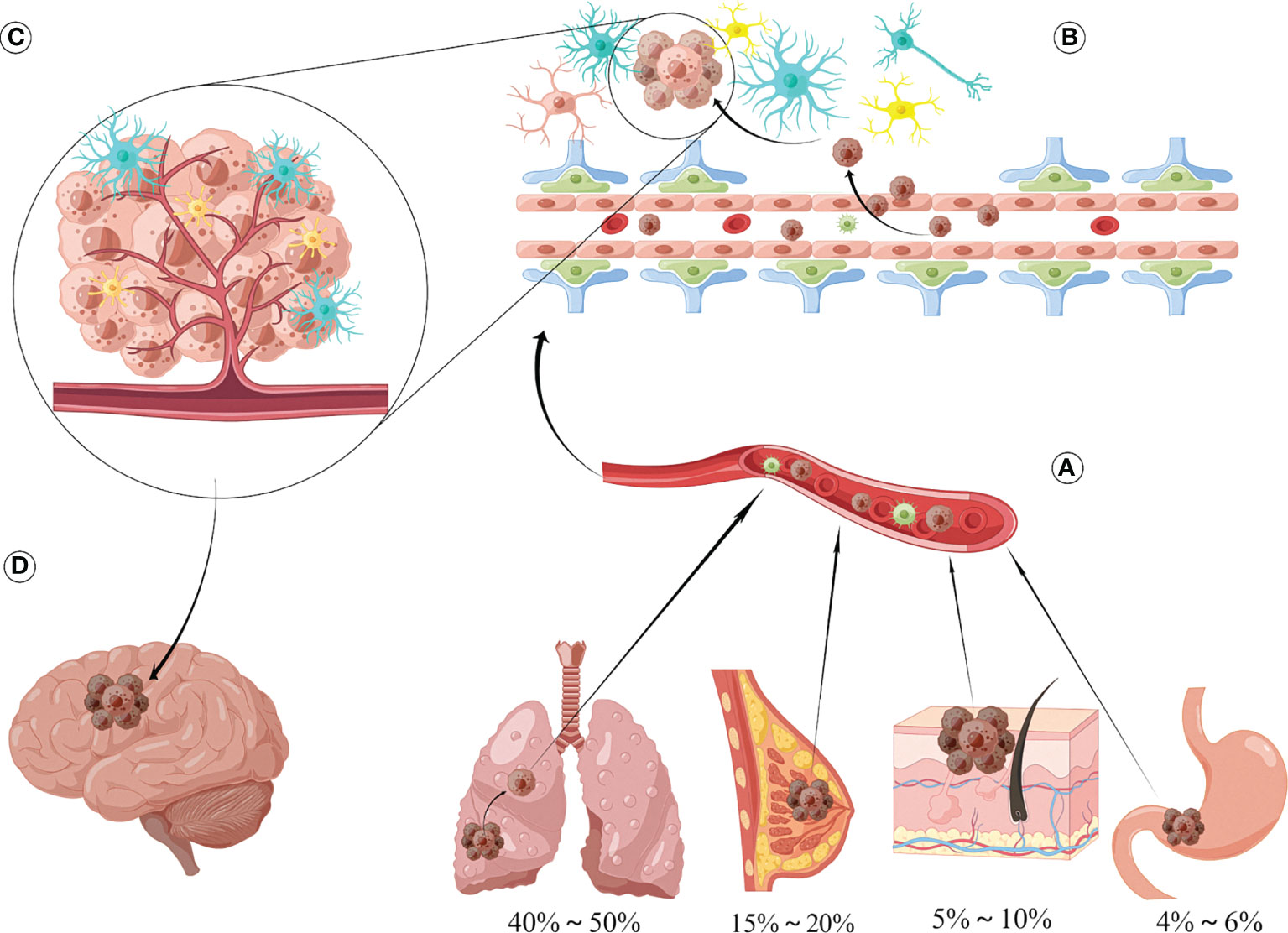
Figure 1 Sources and formation processes of brain metastases. (A) The most common sources of brain metastases are lung cancer (40%-50%), followed by breast cancer (15%-20%), skin cancer (mainly melanoma) accounting for 5%-10%, and gastrointestinal malignancy (4%-6%). (B) Tumor cells and secreted vesicle contents can disrupt the integrity of BBB, thereby promoting tumor metastasis to intracranial, interacting with surrounding astrocytes, microglia/macrophages, and then influencing the biological behavior of brain metastasis through various pathways such as secreting cytokine networks, direct contact and exosomes, and establishing complex networks. (C) Tumor cells can produce mutual adaptive changes with the components of the central nervous system microenvironment, and it is this interaction that determines the occurrence and development of brain metastatic lesions. (D) Metastatic foci appear in the skull, producing obvious mass and edematic effects, which seriously affects the quality of life of patients.
Metastasis of tumor cells is one of the most important features of malignant tumors, and the intra- and inter-cellular molecular mechanisms involved in the metastasis process are considerably complex. These include epithelial-mesenchymal transition, survival of circulating tumor cells in blood vessels, tumor cell dormancy, and tumor cell heterogeneity and stemness, among others. The interaction between tumor and stromal cells, tumor-related angiogenesis, and a series of events related to the tumor microenvironment are also involved (6). The seed and soil hypothesis suggests that a specific tumor cell can only survive in a suitable tumor microenvironment; this explains the occurrence and development of tumor-specific metastasis (7). In this context, exosomes (a type of extracellular vesicles loaded with proteins, nucleic acids, and other signaling molecules) are involved in multiple processes leading to the development of brain metastases (8). Metastatic lesions to the CNS are unique compared to those in other organs (9). Evaluation of the biological characteristics of tumor cells that metastasize to the brain and their interaction with the microenvironment is therefore essential for the prevention and treatment of brain metastases.
Proposal of the tumor microenvironment
The tumor microenvironment was first proposed in 1979, and has been variably termed as the tumor niche or tumor stem cell niche, among others (10). The tumor microenvironment refers to the local homeostatic milieu associated with tumorigenesis and metastasis, and is composed of tumor and non-tumor cells. It mainly includes tumor cells, tumor stem cells, endothelial cells, fibroblasts, immune cells, extracellular matrix structural components (such as collagen and elastin, among others) locally secreted cytokines, peptide growth factors, and other soluble substances (11, 12). It is widely accepted that the tumor microenvironment is a necessary functional unit for protecting and supporting tumorigenesis, development, metastasis, and recurrence; studies are also increasingly demonstrating the vital role of the tumor microenvironment in the evolution of tumors (13). Normal cells reside in a relatively stable internal environment (homeostatic milieu), and follow regulated processes for proliferation, differentiation, apoptosis, and the secretion and expression of related factors (14). Tumorigenesis involves a process that persistently disrupts this balance to alter the equilibrium in the local microenvironment, making it more suitable for tumor cell proliferation (15). Tumor cells proliferate indefinitely, and need to constantly shape an external tissue environment suitable for their growth. This involves the creation of tissue hypoxia and acidosis; formation of interstitial hypertension; and the production of a large number of growth factors, proteolytic enzymes, and immune inflammatory responses (16, 17). The tumor microenvironment provides shelter for metastatic tumor cells, protecting them from differentiation stimulation, apoptosis stimulation, and immune surveillance, thereby improving resistance to radiotherapy and chemotherapy (18). The tumor microenvironment can also induce tumor cell metastasis via secretion of cyclooxygenase-2 (COX2) and epidermal growth factor receptor (EGFR), the factors responsible for the organophilic nature of tumor cell metastasis. Tumor growth may therefore be inhibited by altering the local microenvironment of the tumor (19, 20).
Characteristics of the microenvironment of brain metastases
The microenvironment of brain metastases has the following unique properties compared with that of other tissues (1): the presence of two biological barriers, namely, the blood-brain barrier (BBB) and blood-cerebrospinal fluid barrier (2), lack of immune cells such as lymphocytes and macrophages (microglia play an important role in the immune response) (3), lack of mesenchymal tissues such as fibroblasts (but is rich in astrocytes and oligodendrocytes), and (4) high expression of CNS-specific molecules such as CXCL-12 and neuroserpin (with neutropenia) (21–23). However, the role of the intracranial microenvironment in the development of brain metastases is debated. Previous studies have shown that tumor cells isolated from brain metastases models or co-cultured in vitro in the CNS microenvironment have stronger proliferation, invasion, and metastasis capabilities than the protocellular line (24). However, other studies have shown that astrocytes can secrete plasminogen activators to promote apoptosis of tumor cells; this is not conducive to tumor cell growth (Figure 1) (25). The impact of the intracranial microenvironment on tumor cells (based on the components that constitute the CNS microenvironment and their interaction with tumor cells) has been described below.
Astrocytes
Astrocytes are the most abundant glial cells in the CNS. They are activated on stimulation, and appear morphologically hypertrophied; this is accompanied by increased expression of glial fibrillary acidic protein, a marker specific for astrocyte activation (26). Astrocytes perform a variety of functions, which include supporting nerve cells, nourishing nerve tissue, maintaining CNS homeostasis, forming a BBB, and repairing damaged CNS tissue (27). As the most important component of the CNS microenvironment, astrocytes play an important role in the formation of brain metastases (28). Following activation, astrocytes secrete a variety of cytokines that affect the proliferation, invasion, and metastatic ability of tumor cells (29). Studies have suggested that astrocytes can secrete matrix metalloproteinase (MMP)-2 and MMP-9, remove matrix components on the surface of tumor cells and the surrounding matrix, and promote the invasion and metastasis of tumor cells. In this context, MMP-2 and MMP-9 can activate transforming growth factor-β (TGF-β) (30), which in turn regulates cell growth, angiogenesis, and other functions through vascular endothelial growth factor (VEGF). Related clinical data show that patients with MMP-2-positive in situ or metastatic tumors of the brain have shorter survival times (31). A study on melanoma brain metastases found that astrocytes can produce interleukin (IL)-3, CD40L, CXCL 12, interferon-γ, and other cytokines that stimulate melanoma cells; in this context, IL-23 stimulates tumor cells to produce MMP-2, thereby promoting tumor cell proliferation (32). The mechanism of interaction between tumor cells and astrocytes (via cytokine networks) is considerably complex. Studies have shown that tumor cells in the CNS can secrete macrophage migration inhibitory factor, IL-8, and plasminogen activator inhibitor 1, thereby activating astrocytes; activated astrocytes can secrete IL-6 and tumor necrosis factor-α (TNF-α). TNF-α and IL-1β promote tumor cell growth; however, IL-6 receptor expression is down-regulated in these tumors (33, 34). However, as demonstrated by Sierra et al. (35), astrocytes also inhibit the growth of tumor cells. This is mainly mediated by fibrinolytic enzymes in the CNS that cause shedding of Fas ligand from astrocyte membranes; the secreted Fas ligand triggers apoptosis of tumor cells (36).
Astrocytes also protect tumor cells from the cytotoxic effects of chemotherapy. This protection may be mediated by direct contact with tumor cells and gap junctions (GJs); however, fibroblasts do not play a similar role (37–39). In this context, direct connexin 43-mediated intercellular communication between astrocytes and melanoma cells protects the latter against chemotherapy-induced apoptosis (40). Direct contact between astrocytes and tumor cells can also promote the secretion of IL-6 and IL-8 by tumor cells. Astrocytes then produce endothelin 1, which binds to the endothelin receptor of tumor cells to activate the AKT and mitogen-activated protein kinase pathways; this affects the downstream expression of Bcl-2-like protein 1, twist family basic helix-loop-helix transcription factor 1, and glutathione s-transferase alpha 5, thereby protecting the cells against chemotherapy drugs (41). Murphy et al. (42) found that connexin 43 can induce resistance to temozolomide by activating the AKT/AMP-activated protein kinase/mammalian target of rapamycin signaling pathway in malignant gliomas. However, studies have shown that connexin 43-mediated intercellular communication can enhance the cytotoxic effect of chemotherapy drugs in testicular cancer cells; in this context, studies suggest that GJs can transmit certain small molecules to induce tumor cell apoptosis (43). These findings suggest that specific GJ signaling molecules in tumor cells and the microenvironment can affect tumor cell sensitivity to chemotherapy in the CNS; they may block or activate signaling between GJs and offer clinically significant enhancement of chemotherapy drug effects.
As a key factor in the microenvironment, astrocytes can interact with tumor cells to influence the biological behavior of brain metastases via various channels such as cytokine networks and direct contact. The interaction between the two is complex and some mechanisms have not been fully understood; this needs further evaluation (44–46).
Microglia/macrophages
Microglia play an important role in the CNS immune response (47). They belong to the monocyte-macrophage system, and it is difficult to distinguish them from circulating macrophages based on morphological and molecular markers after activation (48). Animal experiments have shown that in metastatic tumor models, microglia/macrophages are mostly derived from circulating monocytes; original intracranial microglia represent a minority. Certain studies therefore refer to activated microglia as microglia/macrophages (49).
The immune system plays an important role in tumorigenesis and development. Tumor macrophages can be divided into two types, namely, M1 and M2; the M2 mononuclear macrophage surface antigens CD163 and CD204 lead to secretion of arginase, IL-10, lipopolysaccharide, interferon γ, and transforming growth factor-β1. Cytokines such as transforming growth factor-β1 promote tumor growth. Conversely, M1 macrophages show high levels of inducible nitric oxide synthase expression and secrete IL-1, IL-12, nitric oxide, and TNF-α, all of which have tumoricidal effect (50). Wei et al. (48) studied the role of microglia/macrophages in glioma. They found that the M2 macrophages in the tumor microenvironment promoted glioma cell invasion, angiogenicity, and the formation of an inhibitory immune microenvironment, resulting in poor prognosis. Microglia/macrophages serve as the most important link for immune function in the CNS. It is therefore essential to identify the types of microglia/macrophages and the mechanisms of their production in brain metastatic tumors; this may help to confirm the relationship between the immune system and tumor cells in the CNS (51).
Data regarding the phenotypic changes of microglia/macrophages in brain metastases and the related mechanisms are lacking. Data regarding their impact on the treatment of tumors are also considerably scarce compared to those on current popular immunotherapy (52). In vitro experiments have shown that zoledronic acid can promote phenotypic changes in CNS microglia/macrophages to inhibit tumor invasion; clinical data also suggest that zoledronic acid can reduce the risk of recurrence in patients with breast cancer (53). However, sufficient clinical evidence is lacking for patients with brain metastases. Further trials are needed to evaluate the effect of microglia/macrophages in the treatment of brain metastasis (52, 54).
Recent research suggests that in addition to astrocytes and microglia, neurons and neurotransmitters play an important role in the occurrence and development of metastases (55). Zeng et al. (56) found that elevated N-methyl-D-aspartic acid (NMDA) receptor expression promotes the development of intracranial metastasis in breast cancer. The process is mediated by a protein subunit of the NMDA receptor, namely, GIuN2B, which is required for synapse formation and alteration of synaptic junction intensity; it is highly expressed in both human and mouse breast cancer cells. NMDA receptors allow calcium ions to enter the cells; this may be involved in the development of some human cancers. Zeng et al. (56) also found that human breast cancer cells express a protein known as neuroligin, which contributes to intercellular adhesion; it typically promotes the formation of synapes between neurons. This suggests that similar to human glioma cells, human breast cancer cells may exploit neuronal machinery to establish synaptic connections. Microscopy of mouse brain tissue samples containing human breast cancer cells has shown that the proteins that pack glutamate into vesicles are in close proximity to NMDA receptors; it also demonstrated the formation of synaptic structures between cancer cells and neurons. Compared with the mice that were injected with breast cancer cells having normal GIuN2B levels, the modified mice produced smaller brain tumors; they also had longer survival times with lower GIuN2B expression. This suggests that GIuN2B-mediated NMDA receptor signaling occurs through the formation of “pseudo” three-way synapses, which promote tumor cell colonization and growth in the brain. Several subsequent studies have shown that brain metastatic tumor cells can establish synaptic connections with neurons by using the molecular mechanisms involved in synapse formation between neurons. Synaptic activity causes depolarization in neurons and facilitates calcium ion flow, which is necessary for cell differentiation, proliferation, and survival. In cancer cells, this process promotes tumor colonization and progression (57, 58).
Blood-brain barrier
The BBB is the structure with which tumor cells first come into contact during the development of brain metastases. It is composed of capillary endothelial cells and the tight connections between them, basal membranes, and dendrites of astrocytes (59). Under physiological circumstances, the BBB maintains CNS homeostasis and has an isolating effect on drugs, toxins, ions, and other substances (60). The tight connections of the BBB are the key to maintaining its integrity. These are composed of transmembrane proteins and surrounding proteins; the transmembrane proteins which constitute the connection between cells comprise occludin, junctional adhesion molecules, and the tight junction protein, claudin (mainly claudin-5 on BBB). The surrounding proteins are distributed on both sides of the tight junction (52, 61–63). Proteins such as the atresia band (zonula occludin [ZO]) and the filamentous actin-binding protein (afadin) maintain BBB stability (64). Animal experiments have shown that a variety of tumor cell lines can successfully pass through the BBB (65), and that the passage of tumor cells through the BBB is the first step in the formation of brain metastatic foci; however, the specific mechanism is not fully understood. On comparing differences in gene expression between brain metastatic lesions and protocellular cells, Bos et al. (66) found that COX2, α2,6-sialyltransferase (ST6Gal-I), and EGF can mediate passage of breast cancer cells through the BBB; they speculated that ST6Gal-I can specifically mediate brain metastasis by promoting acidification of endothelial cell surfaces (67). In patients with colon cancer, a single-nucleotide polymorphism of ST6Gal-I RS1736858 is highly associated with the risk of brain metastasis. The COX2 produced by tumor cells can induce the production of prostaglandins, which promote high expression of MMP-1 in tumor cells and degrade claudin and ZO-1 on the BBB (68). However, Lee et al. (69) suggested that the main source of COX2 was not the tumor cells, but the endothelial cells of the BBB. The neuropeptide, substance P, can also facilitate the passage of tumor cells through the BBB by changing the distribution and location of ZO-1 and claudin-5. In vitro studies have shown that small cell lung cancer cells can secrete placental growth factor after binding to VEGF-1 receptors, activate the extracellular signal-regulated kinase 1/2 pathway, promote occludin phosphorylation, and change the tight connections of the BBB, all of which eventually aid the easy transport of these cancer cells through the BBB (70).
Cell-secreted vesicle contents can also mediate tumor cell-induced destruction of the BBB. Studies have shown that miR-105 secreted by breast cancer cells can be transported across tight junctions via exosomes to destroy the integrity of the BBB; this promotes intracranial metastasis of tumor cells (71). However, some studies suggest that the destruction of the BBB does not only involve the ZO-1 tight junction protein. Tominaga and others found that breast cancer cell-secreted extracellular vesicles can be specifically taken up by endothelial cells of the BBB; miRNA-181c in the extracellular vesicles can inhibit the expression of phosphoinositide-dependent protein kinase 1 on endothelial cells of the BBB. Down-regulation of phosphoinositide-dependent protein kinase 1 can reduce actinin phosphorylation levels and activate cofilin; this causes conformational changes in actin, disrupts the tight connections of the BBB, and prompts breast cancer cells to pass through the BBB. Given the diversity and fragility of the mechanisms by which tumor cells cross the BBB, it may not be a good therapeutic target for resistance to tumor invasion (72, 73). Previous research on the mechanisms of tumor cell penetration of the BBB has mainly focused on breast cancer; studies on other cancers are relatively lacking. The presence of different mechanisms in various tumor cell types therefore warrants further exploration.
Another component of the BBB, namely, vascular endothelial cells, mainly interact with metastatic tumor cells by intercellular adhesion. In the early stages of brain metastasis in non-small cell lung cancer, tumor cells adhere with endothelial cells through VLA-4/VCAM-1, ALCAM/ALCAM, and LFA-1/ICAM-1 binding; these early adhesion molecules can therefore be used as targets to prevent brain metastasis (74). Other studies have shown that non-small cell lung cancer cells that metastasize to the brain have high levels of CD15 expression; they interact with TNF-α-activated CD62E on endothelial cells to mediate adhesion of tumor cells to microvessels (74). In addition, the interaction between tumor and endothelial cells can also promote tumor invasion and angiogenesis. Activation of the Janus kinase-signal transducer and activator of transcription pathway in tumor cells can cause them to secrete VEGF. In the vascular endothelium, this pathway is activated after VEGFR2 binding; this increases MMP-9 secretion and enhances the invasion ability of tumor cells (75).
The BBB limits antigen presentation and immune cell infiltration in the normal resting state. In order to enter the CNS parenchymal space in an inflammatory environment, T cells must first pass through the endothelial layer followed by the glial boundary (76). The vascular structure loses its integrity in patients with brain metastases, and may therefore promote other restrictions on the entry of peripheral immune cells. However, the more modern conceptual framework is that the BBB does not break down, but forms a blood-tumor barrier (BTB) instead; lymphocytes can pass through the intact BBB via the chemokine axis and multi-step adhesion process (77). In this context, the BTB has been shown to have heterogeneous permeability (regulated by reactive astrocytes); this may drive variable immune cell infiltration (78). The process of thrombotic inflammation, which has been recently studied in mouse models of acute stroke, provides new insights into the possibility of biological overlap between brain metastases and BBB/BTB immune interfaces. Studies have shown that clots form preferentially in cerebral microvasculature and tumor cells form large metastases at the site of stagnation in blood vessels; cancer cells embedded in the clot have a higher rate of successful extravasation (79). To date, minimal progress has been made on transformation strategies involving the development of BBB/BTB destruction methods, receptor agonists that alter permeability, radiosensitizing nanoparticles, and novel delivery platforms, all of which have been evaluated in phase I clinical trials (80). These focus areas for future research will not only require increased understanding on the BBB/BTB itself, but also specific knowledge of its role in regulating CNS anti-tumor immunity.
Microvasculature in brain metastasis
Adequate blood supply is indispensable for tumor growth. The microvasculature therefore plays an important role in the metastasis and growth of tumor cells (81). Pathological findings from animal models of brain metastases have shown that tumor cells are mostly distributed around the microvasculature within a radial distance of 75 μm; tumor cells located 100 μm away from the microvasculature cannot not survive (82, 83). Kienast et al. (84) traced the fate of all tumor cells in a brain metastases model using fluorescence tracing; they found that the tumor cells that were separated from blood vessels had all died. In this context, Fidler et al. (85) found that the microvasculature of brain metastases has low microvessel density. However, the lumen is characterized by numerous abnormally dilated segments.
VEGF is a key factor in angiogenesis. Earlier experiments have shown that although it is necessary, its presence is not sufficient for the formation of brain metastases (86). Studies have shown VEGF levels in brain metastases tend to be higher than those of primary lesions; the levels also correlate positively with microvessel density (87). In addition to angiogenesis, VEGF can activate a proportion of dormant cells during brain metastasis, prompting proliferation to micrometastases (84). A retrospective analysis showed that the use of bevacizumab can effectively reduce the development of brain metastases in patients with lung cancer without increasing the risk of CNS bleeding (88). However, it should be noted that tumor vasculature formation is affected by many factors; the regulatory role of other factors therefore need to be considered (89).
Other cellular components of the brain metastases microenvironment
Interactions of tumor cells with other cellular components such as oligodendrocytes, circulating immune cells, and CNS interstitial components have been less studied (90). Studies using natural killer cells in animal models of breast cancer or glioma showed that they inhibited the growth of glioma cells and human epidermal growth factor receptor (HER)-positive breast cancer cells. However, in animal models of breast cancer with brain metastases, CD11b-positive myeloid cells have been found to aggregate and form the “soil” for early tumor metastasis; this further releases the inflammatory factors S100A8 and S100A9, inducing tumor cell chemotaxis (91). Cancer associated fibroblasts have been found in human tumors of the CNS; research suggests that these fibroblasts promote tumor cell invasion (7).
Biological characteristics of tumor cells in the brain metastases microenvironment
In the process of tumor metastasis, a series of biological changes occur in cells of distant metastatic foci to enable adaptation to the microenvironment (92–94). The alterations may manifest at the deoxyribonucleic acid (DNA) or epigenetic levels, thereby influencing phenotypic changes in tumor cells (Figure 1). The biological characteristics of brain metastatic tumor cells have been explained from the aspects of genetic alteration, post-translational modification, and metabolic characteristics of brain metastatic tumors (95).
Gene changes in brain metastatic tumor cells
Brastianos et al. (96) examined 86 metastatic brain lesions and their matching primary lesions based on focal point mutations and copy number variations (CNVs), which map the evolutionary tree of tumor cells by calculating the individual cancer cell fraction. They estimated the homology between cells by measuring the number of gene copies near the mutation at the checkpoint, and found that although the tumor cells from the metastases and primary lesion originated from the same ancestor, they had different subclones. They also found homology between subclonal tumor cells from multiple intracranial foci. A series of other related studies (Table 1) have confirmed different genotypic changes such as single nucleotide variations, CNVs, deletion, and amplification, among others, between the primary and metastatic brain lesions. The changes mainly involve activation of multiple cell signaling pathways, apoptosis, and cell adhesion, and partially explain the mechanism of development of brain metastases (100, 101, 103, 104).
Studying the patterns of change and the mechanisms by which they arise may provide promising therapeutic targets for brain metastases (99). In this context, a study on 86 patients with breast cancer showed the presence of clinically relevant therapeutic mutations in metastatic lesions from the brain; these included mutations of HER2, EGFR, the B-Raf proto-oncogene, and AKT. These genes were not detected in the primary lesion. The CNVs of HER1 and HER2 were higher in the metastatic brain lesions than in the primary lesion; however, the CNVs of hormone receptors including the estrogen and progesterone receptors had decreased (105). Genetic alterations such as fibroblast growth factor receptor amplification and B-Raf proto-oncogene and neuroblastoma RAS hotspot mutations can also be detected in brain metastases (Table 1) (98, 106).
Epigenetic changes in the brain metastases microenvironment
A study had compared the whole genome methylation levels of tumor cells in brain metastases of nude mice with those of subcutaneous tumor models of melanoma, lung cancer, stomach cancer, and other cell lines; the methylation levels of a series of transcription factors such as transcription factor 4, purine rich element binding protein B, one cut homeobox 2, estrogen related receptor gamma, nuclear factor IB, and myocyte enhancer factor 2C were found to differ significantly in the brain metastases model. In particular, the difference in transcription factor 4, a transcription factor related to neurodevelopment, was the most obvious (107). Tumor cells that metastasize to the brain have unique gene expression profiles owing to these changes (108). Marzese et al. (109) also observed an inconsistency in methylation levels between brain metastases and extracranial lesions of human melanoma; methylation levels were significantly increased in the promoter range of the homeobox A9 gene (among members of the homeobox family), a transcription component that encodes multiple genes and induces changes in neuro development-related genes (110). In a study on breast cancer, the methylation levels of genes such as polypeptide N-acetylgalactosaminyltransferase 9, coiled-coil domain containing 8, and basonuclin 1 were significantly higher in brain metastases than in the primary lesions; in vitro silencing of the mentioned genes could enhance the invasion ability of tumor cells (Table 1) (111). Changes in methylation levels of tumor cells in the CNS may be responsible for changes in tumor phenotype; however, the mechanism for the changes is not fully understood.
Recent studies have confirmed that micro ribonucleic acids (miRNAs) are one of the key factors affecting protein expression after transcription (112). By comparing miRNA levels between the primary lesion and brain metastases, Zhao et al. (113) identified a group of down-regulated miRNAs in patients with lung cancer; these included miR-145, miR-214, miR-9, and miR-1471. Among these, miR-145 was the most obviously down-regulated. In this context, the down-regulation of miR-145 may promote the proliferation of the A549 and SPC-A1 cell lines in lung cancer. Previous studies have shown that miR-145 can affect the proliferation and invasion of lung cancer cells by participating in the regulation of c-Myc, EGFR, and nudix hydrolase 1 expression (114). MiR-145-5p, another member of the miR-145 family, was also found to be significantly down-regulated in patients with brain metastases from lung cancer; this increased the expression levels of downstream EGFR, octamer-binding transcription factor 4, mucin 1, c-Myc, and tumor protein D52. In this context, the down-regulation of miR-145-5p was caused by initiation of interval methylation (115). MiR-141-3p and miR-200b-3p of the miR-200 family have also been found to be significantly up-regulated in metastatic brain lesions than in primary tumors; they down-regulate zinc finger E-Box binding homeobox 2 expression, thereby affecting the proliferation and invasion ability of tumor cells (116).
Characteristics of tumor cell metabolism in the microenvironment of brain metastases
The CNS has an abundant blood supply, with a blood flow that accounts for 1/5 of the total body volume; blood and energy supplies to the CNS are therefore relatively sufficient (117). Due to the presence of the BBB, the levels of glucose in the interstitial fluid of the CNS are lower than those in the blood. However, it has abundant levels of branched-chain amino acids such as leucine, valine, isoleucine, and glutamic acid (118).
Compared to the invasion and proliferation characteristics of brain metastases, the metabolic characteristics of tumor cells in the CNS have been relatively underexplored (119). Chen et al. (120) compared the levels of protein expression related to energy metabolism between tumor cells in brain and bone metastasis models; they found that unlike common tumor cells which rely predominantly on anaerobic metabolism, tumor cells in the CNS actively demonstrate tricarboxylic acid cycle-oxidative phosphorylation with activation of the pentose phosphate pathway. This may induce resistance of tumor cells to certain antimetabolite chemotherapy drugs such as D-2-deoxyglucose (121). Chen et al. (122) found that breast cancer cells that metastasize to the brain have greater tolerance to low sugar levels than their parent cells; they also express more glutamate dehydrogenase and α-ketoacid dehydrogenase to use the glutamic acid and branched-chain amino acids available in the environment.
In terms of lipid metabolism, Chen et al. (120) found fatty acid-β oxidation-related enzyme profile expression to be higher in the animal brain metastases model than in the bone metastases model. However, the human breast cancer brain metastases tissue chip showed the expression levels of acetyl-CoA oxidase-1 and fatty acid synthase to be higher than those of metastases to other sites. This suggests that the processes of lipid synthesis and catabolism were more active in the metastatic brain lesions (123).
Tumor cells in the brain metastases microenvironment acquire nerve cell properties
Park et al. (107) found that tumor cells in animal models of brain metastasis from lung cancer, melanoma, and colon cancer showed certain characteristics of neuronal cells; the levels of glutamate signaling pathway proteins and neurotransmitter complex proteins such as synaptosomal-associated protein 25 and synaptosomal-associated protein 91 were significantly increased. Similarly, brain metastases models of human breast cancer showed the expression of γ-aminobutyric acid (GABA) receptors and transaminase sources to have increased; this allows tumor cells to use the available GABA in the CNS for various metabolic activities (124, 125). Nygaard et al. (126) found the expression of glutamate-related signaling pathway signaling proteins, glutamate receptor ionotropic AMPA 2 and glutamate metabotropic receptor 4, to have increased in patients with melanoma brain metastases and animal models; this promotes the growth of tumor cells. Studies have also shown that plasmin found in the CNS can induce apoptosis of tumor cells; however, breast and lung cancer cell lines highly express neuroserpin, a neuronal inhibitor of plasminogen activator, thereby evading the pro-apoptotic effect of plasmin (127). This finding may be based on the fact that high levels of chloride in the interstitial fluid have a damaging effect on non-neuronal cells; coupled with the abundant neurotrophic factors, glutamate, and other substances in the CNS, this may induce certain neuronal properties in tumor cells to make them more suitable for survival in the CNS microenvironment (128).
Role of exosomes in the brain metastases microenvironment
Exosomes are membranous vesicles with a diameter of between 30-100 nm; they have a lipid bilayer; can be secreted from all kinds of cells; are present in serum, urine, saliva, and other human body fluids; and can be separated and purified by ultracentrifugation, density gradient centrifugation, and other methods (129). Exosomes have a considerably complex composition, and include a variety of lipids, proteins, mRNAs, miRNAs, long non-coding RNAs, circular RNAs, and DNA. Advances in exosome-related research in recent years is gradually revealing the causes for organ propensity of metastatic tumors (102). Hoshino et al. (130) found that tumor exosome integrin expression profiles determine the organ propensity of tumor metastasis; they also found that ingestion of exosomes in the brain can create a pre-metastatic microenvironment for tumor metastasis. This suggests that exosomes can alter the pre-metastatic microenvironment to help target tumor localization (Figure 1). Studies also suggest that CD46 found on endothelial cells of human cerebral microvasculature is a receptor that mediates melanoma exosome uptake; this further confirms the role of exosomes in tumor targeting (131). Exosomes can also help target the localization of tumors by altering the manner by which energy is metabolized. Fong et al. (130) found that cancer cells inhibit glucose uptake by non-tumor cells; they down-regulate pyruvate kinase, and thereby glycolysis, in the pre-metastatic microenvironment by secreting high levels of exosomal miR-122. This suggests that exosomes can also promote tumor brain metastasis by changing glucose uptake in the pre-metastatic microenvironment (Table 2) (134).
The role of exosomes in the proliferation of brain metastases
Stromal cell exosomes promote the proliferation of brain metastases
Phosphatase and Tensin homolog deleted on chromosome ten is a tumor suppressor gene with phosphorylation activity, that regulates the apoptosis and proliferation of tumor cells (140). Zhang et al. (141) found that exosomal miRNA-19 produced by astrocytes can target the inhibition of Phosphatase and Tensin homolog tumor suppressor genes, resulting in increased secretion of chemokine ligand-2 and nuclear factor kappa-B; this promotes the growth of brain metastases. This shows that stromal cell exosomes found in the microenvironment can promote tumor cell growth by carrying miRNAs to influence tumor cell proliferation and inhibit apoptosis (Table 2; Figure 2).
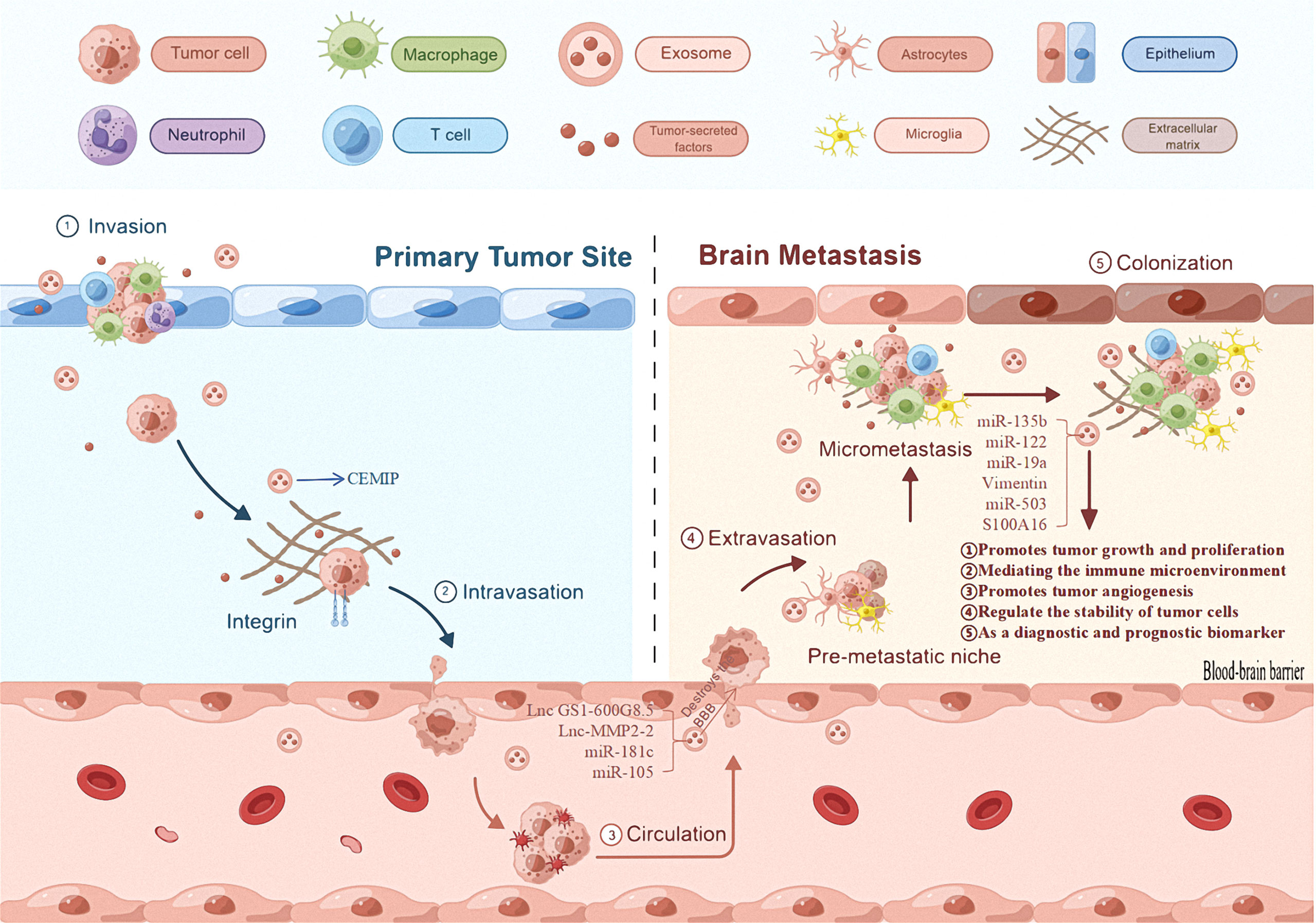
Figure 2 The role of exosomes in brain metastases. The first mechanism of primary tumor exosomes is that they can promote their own progression and metastasis. The second general mechanism is that exosomes derived from primary tumor cells promote the proliferation of brain metastases, regulated immune mechanism to promote tumor cell proliferation, and regulate the stability of tumor cells, and can be useful diagnostic and/or prognostic biomarkers.
Exosome-regulated immune mechanisms promote tumor cell proliferation
Metastatic exosomes can change the microenvironment to promote tumor cell proliferation (142). A study on breast cancer brain metastases found that X-inactive specific transcript deletion increased the secretion of exosomal miR-503, which was transmitted to microglia; this led to M1-M2 transformation and inhibition of T cell proliferation, enabling tumor immune evasion (136, 137). This confirms that tumor cell exosomes can regulate immune cells to enable tumor cell immune escape mechanisms and provide conditions for tumor cell proliferation (Table 2; Figure 2).
Exosomes regulate the stability of tumor cells
A variety of apoptotic mechanisms are often accompanied by a decrease in mitochondrial membrane potential (143). Xu et al. (144) found that exosomes can prevent the loss of mitochondrial membrane potentials through the prohibitin 1 protein present on mitochondrial membranes; tumor cells can therefore tolerate apoptosis in a stressed environment. This indicates that exosomes can regulate tumor cell stability and promote tumor cell proliferation by influencing mitochondrial membrane potential (Figure 2).
Diagnostic significance of exosomes in brain metastases
Liquid biopsy technologies are rapidly gaining attention because of their rapid and non-invasive characteristics. Common techniques for tumor fluid biopsy include traditional circulating tumor cell detection, circulating tumor DNA detection, and tumor cell exosome detection (144, 145).
Advantages of exosomes in diagnosis
Exosome-based diagnosis offers the following advantages: (1) exosomes exist in almost all body fluids, are easier to enrich, and are more sensitive to current detection methods, and (2) they have high stability, allowing a large number of specific proteins to be isolated at temperatures as low as -80°C. However, exosome-related research has started recently and there is a paucity of cumulative data from studies; further in-depth research and analysis is required (146).
Prospects of exosomes in the diagnosis of brain metastases
In recent years, studies have focused on the role of exosomes in brain metastases (147). Camacho et al. studied the miRNA and protein profiles of brain metastasis-competent exosomes (148). Multiple proteins pertaining to cell communication, the cell cycle, and key signaling pathways involved in cell invasion and metastasis are promising biomarkers for brain metastases. Although exosomes are still in their infancy as biomarkers for the diagnosis of brain metastases, they are already in use for the diagnosis of lung cancer. Exosome Diagnostics made a major breakthrough in 2016 with the ExoDx™ Lung (ALK) technology for detection of exosomal miRNA by analysis of blood samples; this is being widely used. Combining exosomal miRNA and circulating tumor DNA analysis can increase diagnostic sensitivity by approximately 3-fold compared to circulating tumor DNA-based diagnosis alone (149).
Exosomes can be used as targets and tools for the treatment of brain metastases
Exosomes are involved in almost all processes of brain metastasis including cell genesis, metastasis, and proliferation. It is possible to target exosomes and the corresponding nucleic acids and proteins to treat the corresponding tumors; this provides new concepts for the treatment of brain metastases (150). In a recent study, Yang et al. (151) found that exosomes secreted by tumor cells contain functional programmed death-1 (PD-L1) protein, which can be transferred to other cells to inhibit T-cell resistance; binding of PD-1 to T cells inhibits anti-tumor immunity and protects the tumor cells. Inhibiting the secretion of PD-L1-containing exosomes by knocking down Rab27a or applying the inhibitor GW4869 can lead to meaningful anti-cancer effects. This offers a major step towards precision and individualized treatment of brain metastases (152).
The BBB has always been the greatest challenge in the treatment of brain metastases. Most traditional chemotherapeutic drugs and large molecule targeted drugs are denied entry by the BBB, thus making the CNS a sanctuary for survival and multiplication of metastatic cells (153). As a drug delivery system, exosomes may effectively address the issue BBB permeability to chemotherapy drugs (154). There are two main types of drug delivery methods for exosomes: exogenous and endogenous (155). Exogenous drug delivery requires the extraction of exosomes from donor cells and the delivery of small molecules (paclitaxel, adriamycin, and curcumin, among others) or gene-based drugs (e.g., small interfering RNA) into the exosome by electroporation (156). The endogenous drug delivery method carries the drug out of the cell via exosome secretion after the drug enters the donor cell; the drug-loaded exosome is finally extracted (157). Reversible protein-protein interaction molecules have been designed for large molecule proteins; these are controlled by blue light to allow integration into the endogenous pathway of exosome production, and are successfully loaded as “cargo proteins” into the new exosomes. This provides an important method for carriage of large molecule proteins by exosomes (158). Yang et al. (159) reported that exosomes derived from endothelial cells of mouse brain microvasculature can effectively deliver antitumor drugs in vivo across the BBB to inhibit tumor growth. This is indicates the advantages and possibilities of exosome treatment for brain metastases.
Discussion
Changes in the biological properties of brain metastatic tumor cells and the interaction between tumor cells and their microenvironment may explain the relatively inefficient metastatic process of tumor cell colonization and growth in the intracranial “soil.” However, it is unclear whether the cranial microenvironment plays a screening or inducing role in the altered biological behavior of tumor cells in metastatic lesions (160). A study that examined genome-wide methylation levels of lung cancer and melanoma cells co-cultured with astrocytes partially replicated the altered methylation profile in animal models of brain metastasis; this suggests that astrocytes can cause changes in methylation levels in tumor cells (161). However, McDermott (162) proposed a model in which CNS microenvironment components such as astrocytes and microglia interacted with tumor cells to produce certain cytokines; these cytokines altered miRNA levels in tumor cells, which in turn affected the expression of the corresponding target genes.
Several studies have shown that tumor cell exosomes are closely related to tumor metastasis. The alteration of the cranial microenvironment and targeted migration of cancer cell exosomes are particularly important for the development of lung cancer brain metastases. In this context, exosomes play an important role in the tumor microenvironment and are directly or indirectly involved in intercellular signal transduction, tumorigenesis, and progression in the tumor microenvironment (163). For example, exosomes secreted by lung cancer cells contain oncogenes and they target the tumor microenvironment, thus promoting tumor progression (164). Exosomes are involved in DNA methylation, histone modification, post-transcriptional regulation, and RNA regulation. The relevant substances delivered by exosomes reflect the state of the cell, and exosomes originating from tumor cells may alter the tumor and promote the expression of tumor suppressor genes in recipient cells. Thus, exosomes in body fluids (including blood) may serve as biomarkers of cancer, and the detection of these biomarkers may be used for diagnosis or prognostic assessment of cancer.
As a target system, exosomes are expected to effectively promote the development of medical oncology. The relationship between exosomes and brain metastases needs to be explored further to understand the intrinsic mechanisms of exosome structure and their interactions with regulatory proteins (165–167). Along with the in-depth study of exosomes in brain metastases, their monitoring can aid the screening of susceptible or high-risk groups, clinical diagnosis, molecular staging, prognosis assessment, recurrence or metastasis prediction, and efficacy evaluation. In particular, the monitoring of exosomes may aid the formulation of brain metastases prevention strategies and the establishment of a risk evaluation system.
Conclusion
In conclusion, the metastatic tumor microenvironment is a complex biological system. The mechanisms of metastasis formation and regulation that are associated with the microenvironment are areas of particular interest in cancer research. Findings indicate that the factors in the microenvironment that promote the formation of metastases are interrelated and interdependent. The proposed “seed and soil” hypothesis provides a broad framework for addressing the growth of brain metastases. As metastasis is almost always closely related to the formation and alteration of the microenvironment in a large number of cancers, continuous research on the microenvironment will improve understanding on the mechanisms of metastasis development and provide new targets for their diagnosis and treatment. Finally, as new therapeutic tools, exosomes are expected to be ideal markers for the early diagnosis of brain metastases and new targets for their treatment.
Author contributions
ZW and YW designed the study; YA and NM wrote the first draft of the manuscript; HQ and WJ prepared the figure; GF prepared the Tables. All authors approved the final version of the manuscript for submission.
Funding
This study was supported by The Xinjiang Uygur Autonomous Regional Cooperative Innovation ProgramGrant (NO. 2021D01A02), Regional Collaborative Innovation Special Project of Xinjiang Uygur Autonomous Region (No.2021E01013).
Conflict of interest
The authors declare that the research was conducted in the absence of any commercial or financial relationships that could be construed as a potential conflict of interest.
Publisher’s note
All claims expressed in this article are solely those of the authors and do not necessarily represent those of their affiliated organizations, or those of the publisher, the editors and the reviewers. Any product that may be evaluated in this article, or claim that may be made by its manufacturer, is not guaranteed or endorsed by the publisher.
References
1. Steeg PS, Camphausen KA, Smith QR. Brain metastases as preventive and therapeutic targets. Nat Rev Cancer. (2011) 11(5):352–63. doi: 10.1038/nrc3053
2. Lowery FJ, Yu D. Brain metastasis: Unique challenges and open opportunities. Biochim Biophys Acta Rev Cancer. (2017) 1867(1):49–57. doi: 10.1016/j.bbcan.2016.12.001
3. Valiente M, Ahluwalia MS, Boire A, Brastianos PK, Goldberg SB, Lee EQ, et al. The evolving landscape of brain metastasis. Trends Cancer. (2018) 4(3):176–96. doi: 10.1016/j.trecan.2018.01.003
4. Eichler AF, Chung E, Kodack DP, Loeffler JS, Fukumura D, Jain RK, et al. The biology of brain metastases-translation to new therapies. Nat Rev Clin Oncol (2011) 8(6):344–56. doi: 10.1038/nrclinonc.2011.58
5. Lee HH, Chen CH, Chuang HY, Huang YW, Huang MY. Brain surgery in combination with tyrosine kinase inhibitor and whole brain radiotherapy for epidermal growth factor receptor-mutant non-small-cell lung cancer with brain metastases. Sci Rep (2019) 9(1):16834. doi: 10.1038/s41598-019-53456-z
6. Fares J, Fares MY, Khachfe HH, Salhab HA, Fares Y. Molecular principles of metastasis: a hallmark of cancer revisited. Signal Transduct Target Ther (2020) 5(1):28. doi: 10.1038/s41392-020-0134-x
7. Valastyan S, Weinberg RA. Tumor metastasis: Molecular insights and evolving paradigms. Cell (2011) 147(2):275–92. doi: 10.1016/j.cell.2011.09.024
8. Ono M, Kosaka N, Tominaga N, Yoshioka Y, Takeshita F, Takahashi RU, et al. Exosomes from bone marrow mesenchymal stem cells contain a microRNA that promotes dormancy in metastatic breast cancer cells. Sci Signal (2014) 7(332):ra63. doi: 10.1126/scisignal.2005231
9. Zhang C, Yu D. Advances in decoding breast cancer brain metastasis. Cancer Metastasis Rev (2016) 35(4):677–84. doi: 10.1007/s10555-016-9638-9
10. Whiteside TL. The tumor microenvironment and its role in promoting tumor growth. Oncogene (2008) 27(45):5904–12. doi: 10.1038/onc.2008.271
11. Witz IP. The tumor microenvironment: the making of a paradigm. Cancer Microenviron. (2009) 2 Suppl 1(Suppl 1):9–17. doi: 10.1007/s12307-009-0025-8
12. Neophytou CM, Panagi M, Stylianopoulos T, Papageorgis P. The role of tumor microenvironment in cancer metastasis: Molecular mechanisms and therapeutic opportunities. Cancers (Basel). (2021) 13(9):2053. doi: 10.3390/cancers13092053
13. Whipple CA. Tumor talk: Understanding the conversation between the tumor and its microenvironment. Cancer Cell Microenviron. (2015) 2:e773. doi: 10.14800/ccm.773
14. Merrell AJ, Stanger BZ. Adult cell plasticity in vivo: de-differentiation and transdifferentiation are back in style. Nat Rev Mol Cell Biol (2016) 17(7):413–25. doi: 10.1038/nrm.2016.24
15. Shalapour S, Karin M. Pas de deux: Control of anti-tumor immunity by cancer-associated inflammation. Immunity (2019) 51(1):15–26. doi: 10.1016/j.immuni.2019.06.021
16. Seimiya T, Otsuka M, Iwata T, Shibata C, Tanaka E, Suzuki T, et al. Emerging roles of exosomal circular RNAs in cancer. Front Cell Dev Biol (2020) 8:568366. doi: 10.3389/fcell.2020.568366
17. Greten FR, Grivennikov SI. Inflammation and cancer: Triggers, mechanisms, and consequences. Immunity (2019) 51(1):27–41. doi: 10.1016/j.immuni.2019.06.025
18. Quail DF, Joyce JA. Molecular pathways: Deciphering mechanisms of resistance to macrophage-targeted therapies. Clin Cancer Res (2017) 23(4):876–84. doi: 10.1158/1078-0432.CCR-16-0133
19. Li F, Liu Y, Chen H, Liao D, Shen Y, Xu F, et al. EGFR and COX-2 protein expression in non-small cell lung cancer and the correlation with clinical features. J Exp Clin Cancer Res (2011) 30(1):27. doi: 10.1186/1756-9966-30-27
20. Sasaki T, Hiroki K, Yamashita Y. The role of epidermal growth factor receptor in cancer metastasis and microenvironment. BioMed Res Int (2013) 2013:546318. doi: 10.1155/2013/546318
21. Luo L, Liu P, Zhao K, Zhao W, Zhang X. The immune microenvironment in brain metastases of non-small cell lung cancer. Front Oncol (2021) 11:698844. doi: 10.3389/fonc.2021.698844
22. Li M, Ransohoff RM. Multiple roles of chemokine CXCL12 in the central nervous system: a migration from immunology to neurobiology. Prog Neurobiol (2008) 84(2):116–31. doi: 10.1016/j.pneurobio.2007.11.003
23. Srinivasan ES, Deshpande K, Neman J, Winkler F, Khasraw M. The microenvironment of brain metastases from solid tumors. Neurooncol Adv (2021) 3(Suppl 5):v121–32. doi: 10.1093/noajnl/vdab121
24. Xing F, Liu Y, Sharma S, Wu K, Chan MD, Lo HW, et al. Activation of the c-met pathway mobilizes an inflammatory network in the brain microenvironment to promote brain metastasis of breast cancer. Cancer Res (2016) 76(17):4970–80. doi: 10.1158/0008-5472.CAN-15-3541
25. Toshniwal PK, Firestone SL, Barlow GH, Tiku ML. Characterization of astrocyte plasminogen activator. J Neurol Sci (1987) 80(2-3):277–87. doi: 10.1016/0022-510x(87)90162-6
26. Ye P, Popken GJ, Kemper A, McCarthy K, Popko B, D'Ercole AJ. Astrocyte-specific overexpression of insulin-like growth factor-I promotes brain overgrowth and glial fibrillary acidic protein expression. J Neurosci Res (2004) 78(4):472–84. doi: 10.1002/jnr.20288
27. Quist E, Trovato F, Avaliani N, Zetterdahl OG, Gonzalez-Ramos A, Hansen MG, et al. Transcription factor-based direct conversion of human fibroblasts to functional astrocytes. Stem Cell Rep (2022). doi: 10.1016/j.stemcr.2022.05.015. S2213-6711(22)00268-5.
28. Sirkisoon SR, Wong GL, Aguayo NR, Doheny DL, Zhu D, Regua AT, et al. Breast cancer extracellular vesicles-derived miR-1290 activates astrocytes in the brain metastatic microenvironment via the FOXA2→CNTF axis to promote progression of brain metastases. Cancer Lett (2022) 540:215726. doi: 10.1016/j.canlet.2022.215726
29. Deng J, Zhang Q, Lu L, Fan C. Long noncoding RNA DLGAP1-AS1 promotes the aggressive behavior of gastric cancer by acting as a ceRNA for microRNA-628-5p and raising astrocyte elevated gene 1 expression. Cancer Manag Res (2020) 12:2947–60. doi: 10.2147/CMAR.S246166
30. Shi G, Zhang Z. Rap2B promotes the proliferation and migration of human glioma cells via activation of the ERK pathway. Oncol Lett (2021) 21(4):314. doi: 10.3892/ol.2021.12575
31. Thanabalasundaram G, Schneidewind J, Pieper C, Galla HJ. The impact of pericytes on the blood-brain barrier integrity depends critically on the pericyte differentiation stage. Int J Biochem Cell Biol (2011) 43(9):1284–93. doi: 10.1016/j.biocel.2011.05.002
32. Klein A, Schwartz H, Sagi-Assif O, Meshel T, Izraely S, Ben Menachem S, et al. Astrocytes facilitate melanoma brain metastasis via secretion of IL-23. J Pathol (2015) 23(6):116–27. doi: 10.1002/path.4509
33. Seike T, Fujita K, Yamakawa Y, Kido MA, Takiguchi S, Teramoto N, et al. Interaction between lung cancer cells a nd astrocytes via specific in flammatory cytokines in the microenvironment of brain metastasis. Clin Exp Metastasis (2011) 28(1):13–25. doi: 10.1007/s10585-010-9354-8
34. Noda M, Seike T, Fujita K, Yamakawa Y, Kido M, Iguchi H. Role of immune cells in brain metastasis of lung cancer cells and neuron-tumor cell interaction., 243–51. doi: 10.1007/s11055-011-9406-9
35. Sierra A, Price JE, García-Ramirez M, Méndez O, López L, Fabra A, et al. Astrocyte-derived cytokines contribute to the metastatic brain specificity of breast cancer cells. Lab Invest (1997) 77(4):357–68.
36. Xiao Y, Liu T, Liu X, Zheng L, Yu D, Zhang Y, et al. Total astragalus saponins attenuates CVB3-induced viral myocarditis through inhibiting expression of tumor necrosis factor α and fas ligand. Cardiovasc Diagn Ther (2019) 9(4):337–45. doi: 10.21037/cdt.2019.07.11
37. Wikman H, Lamszus K, Detels N, Uslar L, Wrage M, Benner C, et al. Relevance of PTEN loss in brain metastasis formation in breast cancer patients. Breast Cancer Res (2012) 14(2):R49. doi: 10.1186/bcr3150
38. Lin Q, Balasubramanian K, Fan D, Kim SJ, Guo L, Wang H, et al. Reactive astrocytes protect melanoma cells from chemotherapy by sequestering intracellular calcium through gap junction communication channels. Neoplasia Int J Oncol Res (2010) 12(9):748–54. doi: 10.1593/neo.10602
39. Kim SJ, Kim JS, Park ES, Lee JS, Lin Q, Langley RR, et al. Astrocytes upregulate survival genes in tumor cells and induce protection from chemotherapy. Neoplasia (2011) 13(3):286–98. doi: 10.1158/1538-7445.am10-3428
40. Jung HS, Verwilst P, Sharma A, Shin J, Sessler JL, Kim JS. Organic molecule-based photothermal agents: an expanding photothermal therapy universe. Chem Soc Rev (2018) 47(7):2280–97. doi: 10.1039/c7cs00522a
41. Kim SJ, Lee HJ, Kim MS, Choi HJ, He J, Wu Q, et al. Macitentan, a dual endothelin receptor antagonist, in combination with temozolomide leads to glioblastoma regression and long-term survival in mice. Clin Cancer Res (2015) 21(20):4630–41. doi: 10.1158/1078-0432.CCR-14-3195
42. Murphy SF, Varghese RT, Lamouille S, Guo S, Pridham KJ, Kanabur P, et al. Connexin 43 inhibition sensitizes chemoresistant glioblastoma cells to temozolomide. Cancer Res (2016) 76(1):139–49. doi: 10.1158/0008-5472.CAN-15-1286
43. Hong X, Wang Q, Yang Y, Zheng S, Tong X, Zhang S, et al. Gap junctions propagate opposite effects in normal and tumor testicular cells in response to cisplatin. Cancer Lett (2012) 317(2):165–71. doi: 10.1016/j.canlet.2011.11.019
44. Shipman L. Microenvironment: Astrocytes silence PTEN to promote brain metastasis. Nat Rev Cancer (2015) 15(12):695. doi: 10.1038/nrc4045
45. Yu D. PTEN loss by exosomal microRNA primes brain metastasis outgrowth. Cancer Res (2017) 77:BS2–2-BS2-2. doi: 10.1158/1538-7445.SABCS16-BS2-2
46. Uslar L. Clinical relevance of allelic imbalances on chromosome 10q in brain metastases formation in breast cancer patients. (2012) 10:4707.
47. Watters JJ, Schartner JM, Badie B. Microglia function in brain tumors. J Neurosci Res (2005) 81(3):447–55. doi: 10.1002/jnr.20485
48. Wei J, Gabrusiewicz K, Heimberger A. The controversial role of microglia in malignant gliomas. Clin Dev Immunol (2013) 2013:285246. doi: 10.1155/2013/285246
49. Perry VH, Teeling J. Microglia and macrophages of the central nervous system: the contribution of microglia priming and systemic inflammation to chronic neurodegeneration. Semin Immunopathol (2013) 35(5):601–12. doi: 10.1007/s00281-013-0382-8
50. Allavena P, Sica A, Garlanda C. The yin-yang of tumor-associated macrophages in neoplastic progression and immune sur veillance. Immunol Rev (2008) 222:155–61. doi: 10.1111/j.1600-065x.2008.00607.x
51. Whiteside TL, Demaria S, Rodriguez-Ruiz ME, Zarour HM, Melero I. Emerging opportunities and challenges in cancer immunotherapy. Clin Cancer Res (2016) 22(8):1845–55. doi: 10.1158/1078-0432.CCR-16-0049
52. Herz J, Filiano AJ, Smith A, Yogev N, Kipnis J. Myeloid cells in the central nervous system. Immunity (2017) 46(6):943–56. doi: 10.1016/j.immuni.2017.06.007
53. Rietkotter E, Menck K, Bleckmann A, Farhat K, Schaffrinski M, Schulz M, et al. Zoledronic acid inhibits macrophage/microglia-assisted breast cancer cell invasion. Oncotarget (2013) 4(9):1449–60. doi: 10.18632/oncotarget.1201
54. Gnant M. Bisphosphonates in the prevention of disease recurrence: current results and ongoing trials. Curr Cancer Drug Targets (2009) 9(7):824–33. doi: 10.2174/156800909789760267
55. Venkataramani V, Tanev DI, Strahle C, Studier-Fischer A, Fankhauser L, Kessler T, et al. Glutamatergic synaptic input to glioma cells drives brain tumour progression. Nature (2019) 573(7775):532–8. doi: 10.1038/s41586-019-1564-x
56. Zeng Q, Michael IP, Zhang P, Saghafinia S, Knott G, Jiao W, et al. Synaptic proximity enables NMDAR signalling to promote brain metastasis. Nature (2019) 573(7775):526–31. doi: 10.1038/s41586-019-1576-6
57. Barria A. Dangerous liaisons as tumour cells form synapses with neurons. Nature (2019) 573(7775):499–501. doi: 10.1038/d41586-019-02746-7
58. Venkatesh HS, Johung TB, Caretti V, Noll A, Tang Y, Nagaraja S, et al. Neuronal activity promotes glioma growth through neuroligin-3 secretion. Cell (2015) 161(4):803–16. doi: 10.1016/j.cell.2015.04.012
59. Jassam YN, Izzy S, Whalen M, McGavern DB, El Khoury J. Neuroimmunology of traumatic brain injury: Time for a paradigm shift. Neuron (2017) 95(6):1246–65. doi: 10.1016/j.neuron.2017.07.010
60. Tominaga N, Hagiwara K, Kosaka N, Honma K, Nakagama H, Ochiya T. RPN2-mediated glycosylation of tetraspanin CD63 regulates breast cancer cell malignancy. Mol Cancer. (2014) 13:134. doi: 10.1186/1476-4598-13-134
61. Aldape K, Brindle KM, Chesler L, Chopra R, Gajjar A, Gilbert MR, et al. Challenges to curing primary brain tumours. Nat Rev Clin Oncol (2019) 16(8):509–20. doi: 10.1038/s41571-019-0177-5
62. Ribeiro DE, Oliveira-Giacomelli Á, Glaser T, Arnaud-Sampaio VF, Andrejew R, Dieckmann L, et al. Hyperactivation of P2X7 receptors as a culprit of COVID-19 neuropathology. Mol Psychiatry (2021) 26(4):1044–59. doi: 10.1038/s41380-020-00965-3
63. Sweeney MD, Sagare AP, Zlokovic BV. Blood-brain barrier breakdown in Alzheimer disease and other neurodegenerative disorders. Nat Rev Neurol (2018) 14(3):133–50. doi: 10.1038/nrneurol.2017.188
64. Jia W, Lu R, Martin TA, Jiang WG. The role of claudin-5 in blood-brain barrier (BBB) and brain metastases (review). Mol Med Rep (2014) 9(3):779–85. doi: 10.3892/mmr.2013.1875
65. Lu W, Bucana CD, Schroit AJ. Pathogenesis and vascular integrity of breast cancer brain metastasis. Int J Cancer (2007) 120(5):1023–6. doi: 10.1002/ijc.22388
66. Bos PD, Zhang XH, Nadal C, Shu W, Gomis RR, Nguyen DX, et al. Genes that mediate breast cancer metastasis to the brain. Nature (2009) 459(7249):1005–9. doi: 10.1038/NATURE08021
67. Cardinal T, Pangal D, Strickland BA, Newton P, Mahmoodifar S, Mason J, et al. Anatomical and topographical variations in the distribution of brain metastases based on primary cancer origin and molecular subtypes: a systematic review. Neurooncol Adv (2021) 4(1):vdab170. doi: 10.1093/noajnl/vdab170
68. Wu K, Fukuda K, Xing F, Zhang Y, Sharma S, Liu Y, et al. Roles of the cyclooxygenase 2 matrix metalloproteinase 1 pathway in brain metastasis of breast cancer. J Biol Chem (2015) 290(15):9842–54. doi: 10.1074/jbc.M114.602185
69. Lee KY, Kim YJ, Yoo H, Lee SH, Park JB, Kim HJ, et al. Human brain endothelial cell-derived COX-2 facilitates extravasation of breast cancer cells across the bloodbrain barrier. Anticancer Res (2011) 31(12):4307–13. doi: 10.1016/j.canrad.2011.03.008
70. Li B, Wang C, Zhang Y, Zhao XY, Huang B, Wu PF, et al. Elevated PLGF contributes to small-cell lung cancer brain metastasis. Oncogene (2013) 32(24):2952–62. doi: 10.1038/onc.2012.313
71. Zhou W, Fong MY, Min Y, Somlo G, Liu L, Palomares MR, et al. Cancer-secreted miR-105 destroys vascular endothelial barriers to promote metastasis. Cancer Cell (2014) 25(4):501–15. doi: 10.1016/j.ccr.2014.03.007
72. Tominaga N, Kosaka N, Ono M, Katsuda T, Yoshioka Y, Tamura K, et al. Brain metastatic cancer cells release microR NA-181c-containing extracellular vesicles capable of destructing blood-brain barrier. Nat Commun (2015) 6:6716. doi: 10.1038/ncomms7716
73. Erickson MA, Banks WA. Neuroimmune axes of the blood-brain barriers and blood-brain interfaces: Bases for physiological regulation, disease states, and pharmacological interventions. Pharmacol Rev (2018) 70(2):278–314. doi: 10.1124/pr.117.014647
74. Soto MS, Serres S, Anthony DC, Sibson NR. Functional role of endothelial adhesion molecules in the early stages of brain metastasis. Neuro Oncol (2014) 16(4):540–51. doi: 10.1093/neuonc/not222
75. Jassam SA, Maherally Z, Smith JR, Ashkan K, Roncaroli F, Fillmore HL, et al. TNF-α enhancement of CD62E mediates adhesion of non–small cell lung cancer cells to brain endothelium via CD15 in lung-brain metastasis. Neuro-Oncology (2016) 18(5):679–90. doi: 10.1093/neuonc/nov248
76. Xie J, Shen Z, Anraku Y, Kataoka K, Chen X. Nanomaterial-based blood-brain-barrier (BBB) crossing strategies. Biomaterials (2019) 224:119491. doi: 10.1016/j.biomaterials.2019.119491
77. Lee HT, Xue J, Chou PC, Zhou A, Yang P, Conrad CA, et al. Stat3 orchestrates interaction between endothelial and tumor cells and inhibition of Stat3 suppresses brain metastasis of breast cancer cells. Oncotarget (2015) 6(12):10016–29. doi: 10.18632/oncotarget.3540
78. Mehrabian H, Detsky J, Soliman H, Sahgal A, Stanisz GJ. Advanced magnetic resonance imaging techniques in management of brain metastases. Front Oncol (2019) 9:440. doi: 10.3389/fonc.2019.00440
79. de Vries NA, Buckle T, Zhao J, Beijnen JH, Schellens JH, et al. Restricted brain penetration of the tyrosine kinase inhibitor erlotinib due to the drug transporters p-gp and BCRP. Invest New Drugs (2012) 30(2):443–9. doi: 10.1007/s10637-010-9569-1
80. Pardridge WM. Drug transport across the blood-brain barrier. J Cereb Blood Flow Metab (2012) 32(11):1959–72. doi: 10.1038/jcbfm.2012.126
81. Hulin JA, Gubareva EA, Jarzebska N, Rodionov RN, Mangoni AA, Tommasi S. Inhibition of dimethylarginine dimethylaminohydrolase (DDAH) enzymes as an emerging therapeutic strategy to target angiogenesis and vasculogenic mimicry in cancer. Front Oncol (2020) 9:1455. doi: 10.3389/fonc.2019.01455
82. Al Tameemi W, Dale TP, Al-Jumaily RMK, Forsyth NR. Hypoxia-modified cancer cell metabolism. Front Cell Dev Biol (2019) 7:4. doi: 10.3389/fcell.2019.00004
83. Langley RR, Fidler IJ. The biology of brain metastasis. Clin Chem (2013) 59(1):180–9. doi: 10.1097/ppo.0000000000000126
84. Kienast Y, von Baumgarten L, Fuhrmann M, Klinkert WE, Goldbrunner R, Herms J, et al. Real-time imaging reveals the single steps of brain metastasis formation. Nat Med (2010) 16(1):116–22. doi: 10.1038/nm.2072
85. Fidler IJ, Yano S, Zhang RD, Fujimaki T, Bucana CD. The seed and soil hy pothesis: vascularisation and brain metastases. Lancet Oncol (2002) 3(1):53–7. doi: 10.1016/s1470-2045(01)00622-2
86. Yano S, Shinohara H, Herbst RS, Kuniyasu H, Bucana CD, Ellis LM, et al. Expression of vascular endothelial growth factor is necessary but not sufficient for production and growth of brain metastasis. Cancer Res (2000) 60(17):4959–67.
87. Jubb AM, Cesario A, Ferguson M, Congedo MT, Gatter KC, Lococo F, et al. Vascular phenotypes in primary non-small cell lung carcinomas and matched brain metastases. Br J Cancer (2011) 104(12):1877–81. doi: 10.1038/bjc.2011.147
88. Fu Y, Hu J, Du N, Jiao S, Li F, Li X, et al. Bevacizu mab plus chemot herapy versus chemotherapy alone for preventing brain metastasis derived from advanced lung cancer. J Chemother (2015) 28(3):218–24. doi: 10.1179/1973947815Y.0000000045
89. Sandler A, Hirsh V, Reck M, von Pawel J, Akerley W, Johnson DH, et al. A n evidence-based review of the incidence of CNS bleeding with anti-VEGF therapy in non-small cell lung cancer patients with brain metastases. Lung Cancer (2012) 78(1):1–7. doi: 10.1016/j.lungcan.2012.07.004
90. Lee SJ, Kang WY, Yoon Y, Jin JY, Song HJ, Her JH, et al. Natural killer (NK) cells inhibit systemic metastasis of glioblastoma cells and have therapeutic effects against glioblastomas in the brain. BMC Cancer (2015) 15(1):1011. doi: 10.1186/s12885-015-2034-y
91. Chisari A, Golán I, Campisano S, Gélabert C, Moustakas A, Sancho P, et al. Glucose and amino acid metabolic dependencies linked to stemness and metastasis in different aggressive cancer types. Front Pharmacol (2021) 12:723798. doi: 10.3389/fphar.2021.723798
92. Rondeau G, Abedinpour P, Desai P, Baron VT, Borgstrom P, Welsh J. Effects of different tissue microenvironments on gene expression in breast cancer cells. PloS One (2014) 9(7):e101160. doi: 10.1371/journal.pone.0101160
93. Ding L, Ellis MJ, Li S, Larson DE, Chen K, Wallis JW, et al. Genome remodelling in a basal-like breast cancer metastasis and xenograft. Nature (2010) 464(7291):999–1005. doi: 10.1038/nature08989
94. Brastianos PK, Carter SL, Santagata S, Cahill DP, Taylor-Weiner A, Jones RT, et al. Genomic characterization of brain metastases reveals branched evolution and potential therapeutic targets. Cancer Discovery (2015) 5(11):1164–77. doi: 10.1158/2159-8290.CD-15-0369
95. Li F, Sun L, Zhang S. Acquirement of DNA copy number variations in non-small cell lung cancer metastasis to the brain. Oncol Rep (2015) 34(4):1701–7. doi: 10.3892/or.2015.4188
96. Chen G, Chakravarti N, Aardalen K, Lazar AJ, Tetzlaff MT, Wubbenhorst B, et al. Molecular profiling of patient-matched brain and extracranial melanoma metastases implicates the PI3K pathway as a therapeutic target. Clin Cancer Res (2014) 20(21):5537–46. doi: 10.1158/1078-0432.CCR-13-3003
97. Ferguson SD, Zheng S, Xiu J, Zhou S, Khasraw M, Brastianos PK, et al. Profiles of brain metastases: Prioritization of therapeutic targets. Int J Cancer. (2018) 143(11):3019–26. doi: 10.1002/ijc.31624
98. Bollig-Fischer A, Michelhaugh SK, Wijesinghe P, Dyson G, Kruger A, Palanisamy N, et al. Cytogenomic profiling of breast cancer brain metastases reveals potential for repurposing targeted the rapeutics. Oncotarget (2015) 6(16):14614–24. doi: 10.18632/oncotarget.3786
99. Preusser M, Berghoff AS, Berger W, Ilhan-Mutlu A, Dinhof C, Widhalm G, et al. High rate of FGFR1 amplifications in brain metastases of squamous and non-squamous lung cancer. Lung Cancer. (2014) 83(1):83–9. doi: 10.1016/j.lungcan.2013.10.004
100. Lo Nigro C, Vivenza D, Monteverde M, Lattanzio L, Gojis O, Garrone O, et al. High frequency of complex TP53 mutations in CNS metastases from breast cancer. Br J Cancer (2012) 106(2):397–404. doi: 10.1038/bjc.2011.464
101. Gaedcke J, Traub F, Milde S, Wilkens L, Stan A, Ostertag H, et al. Predominance of the basal type and HER-2/neu type in brain metastasis from breast cancer. Mod Pathol (2007) 20(8):864–70. doi: 10.1038/modpathol.3800830
102. Kuroda H, Tachikawa M, Yagi Y, Umetsu M, Nurdin A, Miyauchi EY, et al. Cluster of differentiation 46 is the major receptor in human blood-brain barrier endothelial cells for uptake of exosomes derived from brain-metastatic melanoma cells (SK-Mel-28). Mol Pharm (2019) 16(1):292–304. doi: 10.1021/acs.molpharmaceut.8b00985
103. Arai T, Ichimura K, Hirakawa K, Yuasa Y. DNA Amplifications and elevated expression of proto-oncogene in addition to altered DNA ploidy in metastatic brain tumors. Clin Exp Metastasis (1994) 12(4):267–75. doi: 10.1007/BF01753833
105. Park ES, Kim SJ, Kim SW, Yoon SL, Leem SH, Kim SBC, et al. Cross-species hybridization of microarrays for studying tumor transcriptome of brain metastasis. Proc Natl Acad Sci U S A. (2011) 108(42):17456–61. doi: 10.1073/pnas.1114210108
106. Bleckmann A, Siam L, Klemm F, Rietkötter E, Wegner C, Kramer F, et al. Nuclear LEF1/TCF4 correlate with poor prognosis but not with nuclear β-catenin in cerebral metastasis of lung adenocarcinomas. Clin Exp Metastasis. (2013) 30(4):471–82. doi: 10.1007/s10585-012-9552-7
107. Marzese DM, Scolyer RA, Huynh JL, Huang SK, Hirose H, Chong KK, et al. Epigenome-wide DNA methylation landscape of melanoma progression to brain metastasis reveals aberrations on homeobox d cluster associated with prognosis. Hum Mol Genet (2014) 23(1):226–38. doi: 10.1093/hmg/ddt420
108. Pangeni RP, Channathodiyil P, Huen DS, Eagles LW, Johal BK, Pasha D, et al. The GALNT9, BNC1 and CCDC8 genes are frequently epigenetically dysregulated in breast tumours that metastasise to the brain. Clin Epigenetics. (2015) 7(1):57. doi: 10.1186/s13148-015-0089-x
109. Li T, Lian H, Li H, Xu Y, Zhang H. HY5 regulates light-responsive transcription of microRNA163 to promote primary root elongation in arabidopsis seedlings. J Integr Plant Biol (2021) 63(8):1437–50. doi: 10.1111/jipb.13099
110. Zhao C, Xu Y, Zhang Y, Tan W, Xue J, Yang Z, et al. Downregulation of miR-145 contributes to lung adenocarcinoma cell growth to form brain metastases. Oncol Rep (2013) 30(5):2027–34. doi: 10.3892/or.2013.2728
111. Cho WC, Chow AS, Au JS. Restoration of tumour suppressor hsa-miR-145 inhibits cancer cell growth in lung adenocarcinoma patients with epidermal growth factor receptor mutation. Eur J Cancer. (2009) 45(12):2197–206. doi: 10.1016/j.ejca.2009.04.039
112. Cho WC, Chow AS, Au JS. MiR-145 inhibits cell proliferation of human lung adenocarcinoma by targeting EGFR and NUDT1. RNA Biol (2011) 8(1):125–31. doi: 10.4161/rna.8.1.14259
113. Donzelli S, Mori F, Bellissimo T, Sacconi A, Casini B, Frixa T, et al. Epigenetic silencing of miR-145-5p contributes to brain metastasis. Oncotarget (2015) 6(34):35183–201. doi: 10.18632/oncotarget.5930
114. Minn YK, Lee DH, Hyung WJ, Kim JE, Choi J, Yang SH, et al. MicroRNA-200 family members and ZEB2 are associated with brain metastasis in gastric adenocarcinoma. Int J Oncol (2014) 45(6):2403–10. doi: 10.3892/ijo.2014.2680
115. Fellows LK, Boutelle MG, Fillenz M. Extracellular brain glucose levels reflect local neuronal activity: a microdialysis study in awake, freely moving rats. J Neurochem (1992) 59(6):2141–7. doi: 10.1111/j.1471-4159.1992.tb10105.x
116. Daikhin Y, Yudkoff M. Compartmentation of brain glutamate metabolism in neurons and glia. J Nutr (2000) 130(4S Suppl):1026S–31S. doi: 10.1093/jn/130.4.1026S
117. Lau D, Wadhwa H, Sudhir S, Chang AC, Jain S, Chandra A, et al. Role of c-Met/β1 integrin complex in the metastatic cascade in breast cancer. JCI Insight (2021) 6(12):e138928. doi: 10.1172/jci.insight.138928
118. Chen EI, Hewel J, Krueger JS, Tiraby C, Weber MR, Kralli A, et al. Adaptation of energy metabolism in breast cancer brain metastases. Cancer Res (2007) 67(4):1472–86. doi: 10.1158/0008-5472.CAN-06-3137
119. Gurung RB, Gong SY, Dhakal D, Le TT, Jung NR, Jung HJ, et al. Synthesis of curcumin glycosides with enhanced anticancer properties using one-pot multienzyme glycosylation technique. J Microbiol Biotechnol (2017) 27(9):1639–48. doi: 10.4014/jmb.1701.01054
120. Chen J, Lee HJ, Wu X, Huo L, Kim SJ, Xu L, et al. Gain of glucose-independent growth upon metastasis of breast cancer cells to the brain. Cancer Res (2015) 75(3):554–65. doi: 10.1158/0008-5472.CAN-14-2268
121. Jung YY, Kim HM, Koo JS. Expression of lipid metabolism-related proteins in metastatic breast cancer. PloS One (2015) 10(9):e0137204. doi: 10.1371/journal.pone.0137204
122. Dahn ML, Walsh HR, Dean CA, Giacomantonio MA, Fernando W, Murphy JP, et al. Metabolite profiling reveals a connection between aldehyde dehydrogenase 1A3 and GABA metabolism in breast cancer metastasis. Metabolomics (2022) 18(1):9. doi: 10.1007/s11306-021-01864-6
123. Ran L, Hong T, Xiao X, Xie L, Zhou J, Wen G. GABARAPL1 acts as a potential marker and promotes tumor proliferation and metastasis in triple negative breast cancer. Oncotarget (2017) 8(43):74519–26. doi: 10.18632/oncotarget.20159
124. Nygaard V, Prasmickaite L, Vasiliauskaite K, Clancy T, Hovig E. Melanoma brain colonization involves the emergence of a brain-adaptive phenotype. Oncoscience (2014) 1(1):82–94. doi: 10.18632/oncoscience.11
125. Beasley KD, Toms SA. The molecular pathobiology of metastasis to the brain: a review. Neurosurg Clin N Am (2011) 22(1):7–v. doi: 10.1016/j.nec.2010.08.009
126. Termini J, Neman J, Jandial R. Role of the neural niche in brain metastatic cancer. Cancer Res (2014) 74(15):4011–5. doi: 10.1158/0008-5472.CAN-14-1226
127. Aili Y, Maimaitiming N, Mahemuti Y, Qin H, Wang Y, Wang Z. Liquid biopsy in central nervous system tumors: the potential roles of circulating miRNA and exosomes. Am J Cancer Res (2020) 10(12):4134–50. doi: 10.3389/fonc.2021.686369
128. Aili Y, Maimaitiming N, Mahemuti Y, Qin H, Wang Y, Wang Z. The role of exosomal miRNAs in glioma: Biological function and clinical application. Front Oncol (2021) 11:686369. doi: 10.3389/fonc.2021.686369
129. Hoshino A, Costa-Silva B, Shen TL, Rodrigues G, Hashimoto A, Tesic Mark M, et al. Tumour exosome integrins determine organotropic metastasis. Nature (2015) 527(7578):329–35. doi: 10.1038/nature15756
130. Fong MY, Zhou W, Liu L, Alontaga AY, Chandra M, Ashby J, et al. Breast-cancer-secreted miR-122 reprograms glucose metabolism in premetastatic niche to promote metastasis. Nat Cell Biol (2015) 17(2):183–94. doi: 10.1038/ncb3094
131. Cao M, Isaac R, Yan W, Ruan X, Jiang L, Wan Y, et al. Cancer-cell-secreted extracellular vesicles suppress insulin secretion through miR-122 to impair systemic glucose homeostasis and contribute to tumour growth. Nat Cell Biol (2022) 24(6):954–67. doi: 10.1038/s41556-022-00919-7
132. Umezu T, Tadokoro H, Azuma K, Yoshizawa S, Ohyashiki K, Ohyashiki JH, et al. Exosomal miR-135b shed from hypoxic multiple myeloma cells enhances angiogenesis by targeting factor-inhibiting HIF-1. Blood (2014) 124(25):3748. doi: 10.1182/blood-2014-05-576116
133. Wu D, Shihua D, Li L, Liu T, Zhang T, Li J, et al. TGF-β1-mediated exosomal lnc-MMP2-2 increases blood–brain barrier permeability via the miRNA-1207-5p/EPB41L5 axis to promote non-small cell lung cancer brain metastasis. Cell Death Dis (2021) 12(10):1–1. doi: 10.1038/s41419-021-04072-1
134. Zhang L, Zhang S, Yao J, Lowery FJ, Zhang Q, Huang WC. Microenvironment-induced PTEN loss by exosomal microRNA primes brain metastasis outgrowth. Nature (2015) 527(7576):100–4. doi: 10.1038/nature15376
135. Satelli A, Li S. Vimentin in cancer and its potential as a molecular target for cancer therapy. Cell Mol Life Sci (2011) 68(18):3033–46. doi: 10.1007/s00018-011-0735-1
136. Xing F, Liu Y, Wu SY, Wu K, Sharma S, Mo YY, et al. Loss of XIST in breast cancer activates MSN-c-Met and reprograms microglia via exosomal miRNA to promote brain metastasis. Cancer Res (2018) 78(15):4316–30. doi: 10.1158/0008-5472.CAN-18-1102
137. Xu Z-H, Miao Z-W, Jiang QZ, Gan DX, Wei XG, Xue XZ, et al. Brain microvascular endothelial cell exosome-mediated S100A16 up-regulation confers small-cell lung cancer cell survival in brain. FASEB J Off Publ Fed Am Societies Exp Biol (2018) 33(2):1742–54. doi: 10.1096/fj.201800428R
138. Rodrigues G, Hoshino A, Kenific CM, Matei IR, Steiner L, Freitas D, et al. Tumour exosomal CEMIP protein promotes cancer cell colonization in brain metastasis. Nat Cell Biol (2019) 21:1403–12. doi: 10.1038/s41556-019-0404-4
139. Tămaş F, Bălaşa R, Manu D, Gyorki G, Chinezu R, Tămaş C, et al. The importance of small extracellular vesicles in the cerebral metastatic process. Int J Mol Sci (2022) 23(3):1449. doi: 10.3390/ijms23031449
140. Zhu Y, Tao Z, Chen Y, Lin S, Zhu M, Ji W, et al. Exosomal MMP-1 transfers metastasis potential in triple-negative breast cancer through PAR1-mediated EMT. Breast Cancer Res Treat (2022) 193(1):65–81. doi: 10.1007/s10549-022-06514-6
141. Meng L, Song K, Li S, Kang Y. Exosomes: Small vesicles with important roles in the development, metastasis and treatment of breast cancer. Membranes (Basel). (2022) 12(8):775. doi: 10.3390/membranes12080775
142. Ingenito F, Roscigno G, Affinito A, Nuzzo S, Scognamiglio I, Quintavalle C, et al. The role of exo-miRNAs in cancer: A focus on therapeutic and diagnostic applications. Int J Mol Sci (2019) 20(19):4687. doi: 10.3390/ijms20194687
143. Penfornis P, Vallabhaneni KC, Whitt J, Pochampally RJ, et al. Extracellular vesicles as carriers of microRNA, proteins andlipids in tumor microenvironment. Int J Cancer (2016) 138(1):14–21. doi: 10.1002/ijc.29417
144. Maroto R, Zhao Y, Jamaluddin M, Popov VL, Wang H, Kalubowilage M, et al. Effects of storage temperature on airway exosome integrity for diagnostic and functional analyses. J Extracell Vesicles (2017) 6(1):1359478. doi: 10.1080/20013078.2017.1359478
145. Zhang N, He F, Li T, Chen J, Jiang L, Ouyang XP. Role of exosomes in brain diseases. Front Cell Neurosci (2021) 15:743353. doi: 10.3389/fncel.2021.743353
146. Camacho L, Guerrero P, Marchetti D. MicroRNA and protein profiling of brain metastasis competent cellderived exosomes. PloS One (2013) 8(9):e73790. doi: 10.1371/journal.pone.0073790
147. Yu W, Hurley J, Roberts D, Chakrabortty SK, Enderle D, Noerholm M, et al. Exosome-based liquid biopsies in cancer: opportunities and challenges. Ann Oncol (2021) 32(4):466–77. doi: 10.1016/j.annonc.2021.01.074
148. Sheridan C. Exosome cancer diagnostic reaches market. Nat Biotechnol (2016) 34(4):359–60. doi: 10.1038/nbt0416-359
149. Yang Y, Li CW, Chan LC, Wei Y, Hsu JM, Xia W, et al. Exosomal PD-L1harbors active defense function to suppress T cell killing ofbreast cancer cells and promote tumor growth. Cell Res (2018) 28(8):862–4. doi: 10.1038/s41422-018-0060-4
150. Li J, Liu K, Liu Y, Xu Y, Zhang F, Yang H, et al. Exosomes mediate the cell-to-cell transmission of IFN-a-induced antiviral activity. Nat Immunol (2013) 14(8):793–803. doi: 10.1038/ni.2647
151. Toyokawa G, Seto T, Takenoyama M, Ichinose Y. Insights into brain metastasis in patients with ALK+ lung cancer: is the brain truly a sanctuary? Cancer Metastasis Rev (2015) 34(4):797–805. doi: 10.1007/s10555-015-9592-y
152. Arab S. Exosomes: Novel bio-inspired nanocarriers for efficient targeting of glioblastoma tumor cells. J Babol Univ Med Sci (2020) 23(1):16–22.
153. Alvarez-erviti L, Seow Y, Yin HF, Betts C, Lakhal S, Wood MJ, et al. Deliveryof siRNA to the mouse brain by systemic injection of targeted exosomes. Nat Biotechnol (2011) 29(4):341–5. doi: 10.1038/nbt.1807
154. Pascucci L, Cocce V, Bonomi A, Ami D, Ceccarelli P, Ciusani E, et al. Paclitaxel is incorporated by mesenchymal stromal cells and released in exosomes that inhibit in vitro tumor growth: a new approach for drug delivery. J Control Release (2014) 192:262–70. doi: 10.1016/j.jconrel.2014.07.042
155. Yim N, Ryu SW, Choi K, Lee KR, Lee S, Choi H, et al. Exosome engineering forefficient intracellular delivery of soluble proteins usingoptically reversible protein-protein interaction module. Nat Commun (2016) 7:12277. doi: 10.1038/ncomms12277
156. Saari HLisitsyna ERautaniemi KRojalin TNiemi LNivaro O. FLIM reveals alternative EV-mediated cellular up-take pathways of paclitaxel. J Control Release (2018) 284:133ş143. doi: 10.1016/j.jconrel.2018.06.015
157. Yu M, Gai C, Li Z, Ding D, Zheng J, Zhang W, et al. Targeted exosome-encapsulated erastin induced ferroptosis in triple negative breast cancer cells. Cancer Sci (2019) 110(10). doi: 10.1111/cas.14181
158. Yang T, Martin P, Fogarity B, Brown A, Schurman K, Phipps R, et al. Exosome delivered anticancer drugs across the blood-brain barrier for brain cancer therapy in danio rerio. Pharm Res (2015) 32(6):2003–14. doi: 10.1007/s11095-014-1593-y
159. Winkler F. The brain microenvironment: friend or foe for metastatic tumor cells? Neuro-oncology (2014) 16(12):1565–6. doi: 10.1093/neuonc/nou308
160. O'Brien ER, Clare H, Sibson NR. The role of astrocytes in CNS tumors: pre-clinical models and novel imaging approaches. Front Cell Neurosci (2013) 7:40. doi: 10.3389/fncel.2013.00040
161. McDermott R, Gabik ian P, Sar vaiya P, Ulasov I, Lesniak MS. MicroRNA s in brain metastases: big things come in small packages. J Mol Med (Berl) (2013) 91(1):5–13. doi: 10.1007/s00109-012-0971-3
162. Li I, Nabet BY. Exosomes in the tumor microenvironment as mediators of cancer therapy resistance. Mol Cancer (2019) 18:32. doi: 10.1186/s12943-019-0975-5
163. Sandúa A, Alegre E, González Á. Exosomes in lung cancer: Actors and heralds of tumor development. Cancers (Basel). (2021) 13(17):4330. doi: 10.3390/cancers13174330
164. Oliveira FD, Castanho MARB, Neves V. Exosomes and brain metastases: A review on their role and potential applications. Int J Mol Sci (2021) 22(19):10899. doi: 10.3390/ijms221910899
165. Catelan S, Olioso D, Santangelo A, Scapoli C, Tamanini A, Pinna G, et al. miRNAs in serum exosomes for differential diagnosis of brain metastases. Cancers (Basel). (2022) 14(14):3493. doi: 10.3390/cancers14143493
166. Sweeney MD, Kisler K, Montagne A, Toga AW, Zlokovic BV. The role of brain vasculature in neurodegenerative disorders. Nat Neurosci (2018) 21(10):1318–31. doi: 10.1038/s41593-018-0234-x
Keywords: brain tumor, metastases, tumor cell, tumor microenvironment, exosomes
Citation: Aili Y, Maimaitiming N, Qin H, Ji W, Fan G, Wang Z and Wang Y (2022) Tumor microenvironment and exosomes in brain metastasis: Molecular mechanisms and clinical application. Front. Oncol. 12:983878. doi: 10.3389/fonc.2022.983878
Received: 01 July 2022; Accepted: 28 September 2022;
Published: 20 October 2022.
Edited by:
Nadège Bellance, Université de Bordeaux, FranceReviewed by:
Beth Coyle, University of Nottingham, United KingdomRafael Roesler, Federal University of Rio Grande do Sul, Brazil
Copyright © 2022 Aili, Maimaitiming, Qin, Ji, Fan, Wang and Wang. This is an open-access article distributed under the terms of the Creative Commons Attribution License (CC BY). The use, distribution or reproduction in other forums is permitted, provided the original author(s) and the copyright owner(s) are credited and that the original publication in this journal is cited, in accordance with accepted academic practice. No use, distribution or reproduction is permitted which does not comply with these terms.
*Correspondence: Zengliang Wang, d3psM25nQDEyNi5jb20=; Yongxin Wang, eGpkd3l4MjAwMEBzb2h1LmNvbQ==
†These authors have contributed equally to this work