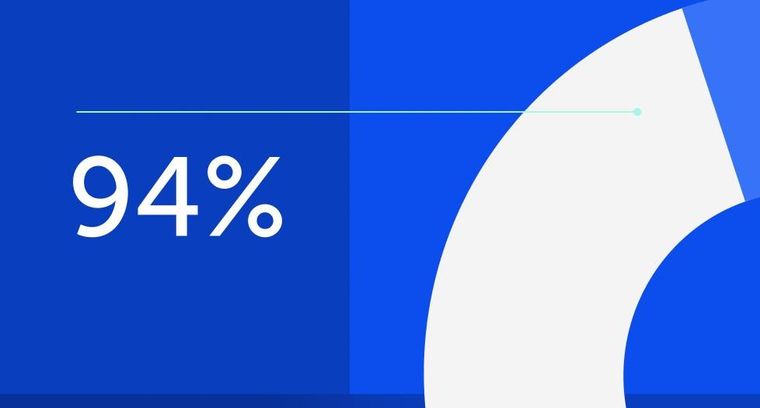
94% of researchers rate our articles as excellent or good
Learn more about the work of our research integrity team to safeguard the quality of each article we publish.
Find out more
MINI REVIEW article
Front. Oncol., 04 October 2022
Sec. Cancer Metabolism
Volume 12 - 2022 | https://doi.org/10.3389/fonc.2022.979683
This article is part of the Research TopicTargeting Metabolism of Cancer Cells and Host to Overcome Drug Resistance: Preclinical and Clinical StudiesView all 8 articles
Neuroblastoma is a pediatric cancer of neural crest cells. It develops most frequently in nerve cells around the adrenal gland, although other locations are possible. Neuroblastomas rely on glycolysis as a source of energy and metabolites, and the enzymes that catalyze glycolysis are potential therapeutic targets for neuroblastoma. Furthermore, glycolysis provides a protective function against DNA damage, and there is evidence that glycolysis inhibitors may improve outcomes from other cancer treatments. This mini-review will focus on glyceraldehyde 3-phosphate dehydrogenase (GAPDH), one of the central enzymes in glycolysis. GAPDH has a key role in metabolism, catalyzing the sixth step in glycolysis and generating NADH. GAPDH also has a surprisingly diverse number of localizations, including the nucleus, where it performs multiple functions, and the plasma membrane. One membrane-associated function of GAPDH is stimulating glucose uptake, consistent with a role for GAPDH in energy and metabolite production. The plasma membrane localization of GAPDH and its role in glucose uptake have been verified in neuroblastoma. Membrane-associated GAPDH also participates in iron uptake, although this has not been tested in neuroblastoma. Finally, GAPDH activates autophagy through a nuclear complex with Sirtuin. This review will discuss these activities and their potential role in cancer metabolism, treatment and drug resistance.
Neuroblastoma is a cancer of neural crest cells and is the most common tumor before the age of one. Although neuroblastoma accounts for 5% of pediatric cancers, it results in 9% of pediatric cancer deaths. Neuroblastoma is a remarkably heterogeneous disease in which some patients undergo spontaneous remission, while other patients endure disease progression that is largely refractory to treatment. Neuroblastomas arise from the neural crest sympathoadrenal lineage that would normally form sympathetic ganglia.
Disease progression is associated with amplification of the MYCN transcription factor in 20-30% of neuroblastomas (1). N-MYC is a basic helix-loop-helix protein that drives the expression of a number of genes associated with survival and proliferation (2), including genes that are required for glycolysis (3, 4). This review will focus on the role of the GAPDH protein in metabolism, nutrient transport and survival in neuroblastoma.
Non-malignant human cells release energy via oxidative phosphorylation, which is highly efficient, and use other less efficient pathways like glycolysis under specific stress conditions. However, cancer cells prefer to utilize glycolysis, producing lactate and pyruvate, even when oxygen is present. This metabolic preference, called the Warburg Effect (5), is a central property of cancer cells and is the target of a number of emerging therapeutic approaches. In neuroblastoma, the activity of succinate dehydrogenase is significantly reduced (6), reflecting the shift to glycolysis.
Glycolysis is not as efficient in producing energy as oxidative phosphorylation, producing a profound requirement for glucose uptake that has been a mainstay of cancer imaging for decades. Although glycolysis is not particularly efficient in generating ATP, it produces metabolic intermediates that include precursors of nucleotides, amino acids and lipids that are needed in rapidly proliferating cells, and these metabolites have been reviewed (7, 8). Cancer cells preferentially utilize anaerobic glycolysis, generating lactate, and rely on glutamine metabolism for metabolites (9). Some of the key glycolytic genes are regulated by pathways that are frequently altered in neuroblastoma (3), as are pathways associated with glutaminolysis (10, 11).
Importantly, tumor metabolism increases chemo- and radio-resistance (12, 13). A number of studies suggest that inhibition of glycolysis is effective against multiple types of cancer (14–19). Neuroblastomas have high levels of glucose uptake, high lactic acid production and low oxygen consumption (3), and there is evidence that glycolysis plays an important role in clinical responses in the disease. Doxorubicin is a standard chemotherapeutic agent used in the treatment of intermediate and high-risk neuroblastoma. When doxorubicin-resistant neuroblastoma cells were treated with both a glycolysis inhibitor and doxorubicin, cell viability was significantly decreased compared to either treatment alone, suggesting that glycolysis may increase chemoresistance (20).
One mechanism of chemoresistance involves P-glycoprotein, a drug efflux pump that relies on ATP. Decreased chemoresistance in the presence of a glycolysis inhibitor could possibly be explained by compromised P-glycoprotein activity due to ATP depletion following glycolysis inhibition (20). However, several studies suggested that the role and expression of P-glycoprotein in neuroblastoma cells remain to be fully elucidated (21, 22).
GAPDH catalyzes the fifth step in glycolysis, the conversion of glyceraldehyde 3-phosphate into 1,3-biphosphoglycerate (23), a reaction that generates NADH- a “high energy” compound that subsequently generates ATP. GAPDH is best known for this reaction and is often used as a “housekeeping” gene or loading control in expression analyses, belying its complex role in growth and survival. GAPDH contains two domains- for NAD+ binding and the catalytic domain. At the junction, cysteine 149 is a site for post-translational modification and is required for multiple GAPDH functions.
While GAPDH is generally localized to the cytoplasm, it’s sub-cellular localization can change, particularly when modified by nitrosylation, oxidation and phosphorylation (23). This review will focus on three areas as they pertain to neuroblastoma: (i) the role of GAPDH in glycolysis, (ii) GAPDH functions in plasma membrane nutrient transport, and (iii) GAPDH nuclear functions as they pertain to DNA repair and the control of apoptosis and autophagy. Other functions of GAPDH, including RNA binding and tRNA nuclear export have been reviewed elsewhere (23).
A subset of GAPDH has been identified at the plasma membrane (23), and we detected plasma membrane GAPDH in neuroblastoma cells by membrane labeling (24). In other cell types, GAPDH at the plasma membrane catalyzes membrane fusion (25) and acts as a receptor for iron carrier proteins (26, 27). The role of the former in neuroblastoma is unclear. However, iron is increasingly linked to neuroblastoma, because there is an elevated requirement for iron in the disease (28, 29), and because there is an emerging link between MYCN and ferroptosis- a type of cell death mediated by iron (30). However, it is unclear whether GAPDH-mediated iron uptake has any role in ferroptosis in neuroblastoma. Membrane GAPDH also acts as a plasminogen receptor in infiltrating macrophages (31), and it is intriguing to consider that cancer cells might utilize this function to promote invasion. However, this idea is purely speculative.
In neuroblastoma cells, GAPDH contributes to glucose uptake (24). This function resembles earlier findings in which GAPDH physically associates with GLUT1 in erythrocyte membranes (32) (Figure 1). Similarly, GAPDH associates with the intracellular surface of GLUT4 in rat L6 myotubes (33), and GAPDH expression is required for insulin-induced glucose transport. Glucose uptake is important in neuroblastoma, being used in imaging (34), and GLUT1 is a predictor of poor survival (35, 36). Furthermore, GLUT1 is essential for neuroblastoma cell survival in culture (36–38).
Figure 1 Diagram depicting subcellular localizations of GAPDH associated with glycolysis (cytoplasm), DNA repair (nucleus), autophagy (nucleus) and increased glucose transport (plasma membrane). Likely binding partners are also shown. Details of the model are described in Aim 1.
One model suggests that GAPDH binds to microtubules and localizes to secretory vesicles (39). The GAPDH-Pro234Ser mutant, which was identified by a chemical mutagenesis screen, inhibits vesicle transport in normal kidney cells (40). In this assay, GAPDH also bound to the AKT serine-threonine kinase- a key signaling protein in cancer cell survival. This assay utilized a purified form of wild-type or mutant GAPDH added directly to cells (40), suggesting an uncharacterized uptake pathway for the protein. It is intriguing to speculate that GAPDH’s putative function in binding AKT and vesicle trafficking might be related to the transport of metabolic proteins such as GLUT1 and GLUT4, but this function is uncharacterized in cancer cells. This model is supported by a role for GAPDH in vesicle biogenesis (41), vesicular glycolysis (42, 43), and inhibiting vesicle transport (44) but the relevance of these findings for neuroblastoma is not known.
Upon modification, GAPDH can change binding partners and translocate to the nucleus, where it regulates multiple functions, including DNA repair. A number of modifications can cause GAPDH to translocate to the nucleus. These include phosphorylation of GAPDH-Ser122 by AMPK, AMP-dependent protein kinase (45). In contrast, AKT2 phosphorylation prevents nuclear translocation of GAPDH (46). The many chemical modifications of cysteine that alter GAPDH localization and alter its function have been reviewed recently (47). There are interactions between phosphorylation and other modifications, for example that AMPK phosphorylation can override nitrosylation (48), so that GAPDH-Ser122Ala mutants do not translocate to the nucleus (48), even if the protein undergoes other modifications.
In addition, GAPDH associates with the acetyltransferase p300/CBP (cyclic adenosine monophosphate response element/CREB-binding protein), which acetylates GAPDH on multiple lysine residues, which are required for translocation of a GAPDH-SIAH1 (seven in absentia homolog 1 E3 ubiquitin ligase) complex (49) to the nucleus (50). A number of studies of non-malignant tissues suggest that the transit of GAPDH to the nucleus after DNA damage promotes apoptosis (51–53). If GAPDH promotes apoptosis, then GAPDH inhibitors might suppress apoptosis in non-malignant cells while promoting cell death in cancer cells- a potential therapeutic window. Indeed, binding of GAPDH to the cytoplasmic protein GOSPEL (GAPDH competitor of SIAH protein enhances life/Rab interacting lysosomal protein like 1) competes with SIAH for binding to GAPDH and prevents NMDA-glutamate excitotoxicity (54). However, in some settings, nuclear GAPDH can perform repair or metabolic functions, and these are described next.
Nuclear GAPDH contributes to DNA repair by binding DNA directly and enzymatically modifying DNA. Uracil is sometimes mis-incorporated into DNA as dUMP or is created through deamination of cytosine, and uracil-DNA glycosylase activity eliminates uracil from DNA and initiates the base excision repair pathway. GAPDH is a uracil-DNA glycosylase, and GAPDH also interacts with the repair protein APE1 [apurinic/apyrimidinic endodeoxyribonuclease 1 (55)], an endonuclease that creates a nick in the DNA phosphodiesterase backbone following the removal of a damaged base by a DNA glycosylase. GAPDH requires Cys152 for APE1 binding (55), and the GAPDH inhibitor koningic acid blocks active site cysteines of GAPDH, disrupting the GAPDH-APE1 interaction (55). As a result, koningic acid enhances the toxicity of H2O2-mediated oxidative damage in smooth muscle cells (55). Koningic acid was isolated from three different fungi from soil (56) and inhibits GAPDH (57) with resulting activity towards a variety of cancer cell types (58–60), including neuroblastoma (61). In some cases, koningic acid reverses drug resistance in cancer (62).
In addition to APE1 (apurinic/apyrimidinic endo-deoxyribonuclease 1), GAPDH also associates with the DNA repair protein PARP1, polyADP ribose polymerase (55, 63, 64), which is critical for single-strand break repair and double-strand repair (65). PARP can ADP-ribosylate and inactivate GAPDH, contributing to GAPDH depletion and in some cases, cell death (64, 66–68). Thus, mutants disrupting this interaction can elevate DNA damage and cell death through a failure in repair (55) or can inflict damage through uncontrolled PARP activity (64). Interestingly, some natural products have PARP inhibitory activity and may partially reverse this pathway (69).
Cancer cells activate numerous signaling pathways, particularly the mTOR (mammalian target of rapamycin) kinase, to inhibit autophagy (70). Numerous signaling, transport and proteolytic steps are in turn controlled by autophagy (71). In glioblastoma, GAPDH is included in an autophagy-related group of seven genes that served as a prognostic indicator (72). But autophagy has a complex role in neuroblastoma, as it does in other cancer types. Rapamycin inhibits mTOR and induces autophagy, and rapamycin induces growth arrest in neuroblastoma cells (73), suggesting a growth inhibitory role for autophagy in neuroblastoma. However, proteins that activate autophagy correlate with poor prognosis (70).
One possible explanation is that autophagy inhibits proliferation in the absence of cell stress but is necessary for cell survival under stressful or damaging conditions. Indeed, autophagy is associated with resistance to some types of chemotherapy [vincristine, doxorubicin and cisplatin (70)], endoplasmic reticulum stress (74), rotenone (75) and retinoid/apigenin treatment (76) in neuroblastoma. In contrast, autophagy uenhances or is required for cell death in neuroblastoma cells treated with the ribonuclease Onconase (77) the Akt inhibitor MK-2206 (78) and agents inducing oxidative damage (79). There are numerous additional examples of autophagy enhancing or inhibiting cell death in neuroblastoma, and some have been recently reviewed (63). Thus, the cell survival function of autophagy in neuroblastoma is dependent on the type of stress and precise experimental conditions (i.e. media components, time of treatment and experimental endpoints).
Given its role in energy metabolism, it is not surprising that GAPDH contributes to autophagy. Under low glucose conditions, ATP decreases and AMP increases, activating AMPK, which phosphorylates GAPDH on serine 122, triggering the translocation of GAPDH to the nucleus (48). There, GAPDH activates SIRT1/sirtuin 1, an NAD-dependent protein deacetylase, displacing a SIRT1 inhibitor and forming an active complex. Activated SIRT1, in turn, deacetylates LC3, a key marker of autophagy (80), increasing LC3 puncta formation in the cytoplasm, promoting autophagy (48), shown in Figure 1. These experiments were performed in mouse embryonic fibroblasts and HEK293 human embryonic kidney cells. In human pancreatic cancer cells, GAPDH nuclear translocation was inhibited by an arginine-273-histidine mutated form of the p53 tumor suppressor protein, favoring glycolysis and cell survival (63).
One of the key questions is how the different pathways directed by GAPDH might intersect in neuroblastoma. There are clues from the literature, although each has caveats. First, there are numerous gene expression studies in neuroblastoma using GAPDH as a loading control for other genes (81), in spite of concerns over utilizing GAPDH as a loading control (82). Other studies directly addressed the function of GAPDH in neuroblastoma. In cultured NG108-15 neuroblastoma-glioma hybrid cells, GAPDH inhibition for 24 hours efficiently induced apoptosis (61). Conversely, GAPDH was detected on the surface of Neuro2A and B103 neuroblastoma cells, and extracellular GAPDH increased neurite outgrowth in cultured neurons (83), although its role in neuroblastoma growth and survival were not determined.
A number of investigators have linked chemically modified forms of GAPDH to increased cell death (66, 84). Other studies support a role for nuclear GAPDH in the progression of apoptosis due to multiple stimuli in neuroblastoma (85–92). Aggregation of GAPDH into multimers also contributes to nuclear translocation and apoptosis (93, 94). Finally, secreted GAPDH may also contribute to cell death (95).
SHSY5Y cells are a widely used human model system that were derived from a 4-year-old girl with neuroblastoma. In studies with SHSY5Y cells, GAPDH inhibition with CGP3466B or heptilidic acid did not inhibit the formation of LC3-II (24), the cleaved and lipidated form of LC3 (80), but suppressed the degradation of the autophagosome target p62/SQSTM1, suggesting that GAPDH is not needed for the signaling pathways that initiate autophagy but is needed autophagic degradation in neuroblastoma. This is different from the GAPDH function in activating SIRT1 to promote LC3-II production (48), perhaps due to differences in glucose in the media. A separate study by Dodson, et al. in SHSY5Y cells after koningic acid (another name for heptilidic acid) treatment found minimal changes in LC3A-II and a slight increase in p62/SQSTM1 levels (96). But the conditions in the two studies were different. Craven, et al. used serum-free media for treatment (with high levels of autophagy), whereas Dodson, et al. used differentiated cells grown in 10% serum (with low levels of autophagy) and retinoic acid. It may be that high levels of autophagy detected in low serum were necessary to detect the effects of GAPDH inhibition. In the study by Dodson, et al., short-term (2 hour) GAPDH inhibition did not affect cell viability (96), and sensitivity to damaging agents such as chemotherapy was not measured. In that system, autophagy is required for viability when glycolysis is disrupted, underscoring a close and complex relationship between the pathways (96).
In a recent study, Ping, et al. used SHSY5Y cells in a co-culture system with E. coli expressing the α-synuclein, a soluble protein that regulates vesicle trafficking and neurotransmitter release. In this setting, GAPDH suppressed autophagy and promoted reactive oxygen species generation and cell death (97). However, this E. coli co-culture system expressing α-synuclein is not directly comparable to other systems. Finally, other investigators suggest an essential role for glycolysis in cell survival in SHSY5Y and SK-N-SH cells (98), based on the ability of ascorbate to induce cell death while suppressing glycolysis and inhibiting GAPDH activity. However, ascorbate also induced high levels of oxidative damage, suggesting that the effects of ascorbate were not attributable to a single mechanism.
GAPDH is a multi-functional protein with separate and antagonistic roles in cancer cell survival. While GAPDH-mediated glycolysis and DNA repair promote tumor cell survival, particularly in the presence of damage, GAPDH also has pro-apoptotic functions in response to different stimuli. Some of these functions are driven by changes in GAPDH cellular localization, and some GAPDH inhibitors are capable of blocking specific localizations. One factor that is less clear is how the function of GAPDH within the nucleus, for example, is directed towards repair versus pro-apoptotic functions or complexes that promote autophagy. Presumably, the availability of binding sites on partner proteins within the nucleus plays an important role, and this may be dynamic, depending on the quantity of any damage or metabolic stress that cells are experiencing.
One limitation to the use of GAPDH inhibitors for treating neuroblastoma is the possibility that some inhibitors that block GAPDH activity in glycolysis and glucose uptake may also inhibit apoptosis by suppressing a pro-apoptotic nuclear form of GAPDH. These would be contra-indicated when combined with chemotherapy. If these competing roles can be selectively inhibited, GAPDH has potential as a therapeutic target in neuroblastoma, particularly in combining GAPDH glycolytic, autophagy or DNA repair inhibitors with standard chemotherapy. For example, an ideal GAPDH inhibitor may inhibit the cytoplasmic and plasma membrane functions of GAPDH while leaving the pro-apoptotic nuclear function undisturbed. Other ideal inhibitors might selectively target nuclear GAPDH functions promoting autophagy or DNA repair while minimally inhibiting GAPDH pro-apoptotic activity. However, research in this area, including therapeutic development, is needed in order to advance GAPDH inhibitors as therapeutic approaches for neuroblastoma.
KC, AP, and OB completed this paper as part of a Master’s thesis project (KC) or as a research project for the Honor’s College at the University of Kentucky (AP and OB). Each author contributed literature searches, article critiques and original text to the paper. RC assembled the manuscript, directed the research and wrote the majority of the paper. All authors read and approved the final manuscript.
This research was funded in part by a University of Kentucky Department of Pharmacology and Nutritional Sciences Department Reinvestment Fund Award.
The authors declare that the research was conducted in the absence of any commercial or financial relationships that could be construed as a potential conflict of interest.
All claims expressed in this article are solely those of the authors and do not necessarily represent those of their affiliated organizations, or those of the publisher, the editors and the reviewers. Any product that may be evaluated in this article, or claim that may be made by its manufacturer, is not guaranteed or endorsed by the publisher.
1. Brodeur GM, Seeger RC, Schwab M, Varmus HE, Bishop JM. Amplification of n-myc in untreated human neuroblastomas correlates with advanced disease stage. Science (1984) 224(4653):1121–4. doi: 10.1126/science.6719137
2. Liu Z, Chen SS, Clarke S, Veschi V, Thiele CJ. Targeting MYCN in pediatric and adult cancers. Front Oncol (2020) 10:623679. doi: 10.3389/fonc.2020.623679
3. Aminzadeh S, Vidali S, Sperl W, Kofler B, Feichtinger RG. Energy metabolism in neuroblastoma and wilms tumor. Transl Pediatr (2015) 4(1):20–32. doi: 10.3978/j.issn.2224-4336.2015.01.04
4. Oliynyk G, Ruiz-Perez MV, Sainero-Alcolado L, Dzieran J, Zirath H, Gallart-Ayala H, et al. MYCN-enhanced oxidative and glycolytic metabolism reveals vulnerabilities for targeting neuroblastoma. iScience (2019) 21:188–204. doi: 10.1016/j.isci.2019.10.020
5. Liberti MV, Locasale JW. The warburg effect: How does it benefit cancer cells? Trends Biochem Sci (2016) 41(3):211–8. doi: 10.1016/j.tibs.2015.12.001
6. Feichtinger RG, Zimmermann F, Mayr JA, Neureiter D, Hauser-Kronberger C, Schilling FH, et al. Low aerobic mitochondrial energy metabolism in poorly- or undifferentiated neuroblastoma. BMC Cancer (2010) 10:149. doi: 10.1186/1471-2407-10-149
7. Soga T. Cancer metabolism: key players in metabolic reprogramming. Cancer Sci (2013) 104(3):275–81. doi: 10.1111/cas.12085
8. Yaku K, Okabe K, Hikosaka K, Nakagawa T. NAD metabolism in cancer therapeutics. Front Oncol (2018) 8:622. doi: 10.3389/fonc.2018.00622
9. Xiang Y, Stine ZE, Xia J, Lu Y, O’Connor RS, Altman BJ, et al. Targeted inhibition of tumor-specific glutaminase diminishes cell-autonomous tumorigenesis. J Clin Invest (2015) 125(6):2293–306. doi: 10.1172/JCI75836
10. Xiao D, Ren P, Su H, Yue M, Xiu R, Hu Y, et al. Myc promotes glutaminolysis in human neuroblastoma through direct activation of glutaminase 2. Oncotarget (2015) 6(38):40655–66. doi: 10.18632/oncotarget.5821
11. Kvamme E, Nissen-Meyer LS, Roberg BA, Torgner IA. Novel form of phosphate activated glutaminase in cultured astrocytes and human neuroblastoma cells, PAG in brain pathology and localization in the mitochondria. Neurochem Res (2008) 33(7):1341–5. doi: 10.1007/s11064-008-9589-9
12. Xu W, Wang S, Chen Q, Zhang Y, Ni P, Wu X, et al. TXNL1-XRCC1 pathway regulates cisplatin-induced cell death and contributes to resistance in human gastric cancer. Cell Death Dis (2014) 5:e1055. doi: 10.1038/cddis.2014.27
13. Lin J, Xia L, Liang J, Han Y, Wang H, Oyang L, et al. The roles of glucose metabolic reprogramming in chemo- and radio-resistance. J Exp Clin Cancer Res (2019) 38(1):218. doi: 10.1186/s13046-019-1214-z
14. Liberti MV, Dai Z, Wardell SE, Baccile JA, Liu X, Gao X, et al. A predictive model for selective targeting of the warburg effect through GAPDH inhibition with a natural product. Cell Metab (2017) 26(4):648–59.e8. doi: 10.1016/j.cmet.2017.08.017
15. Natarajan SR, Ponnusamy L, Manoharan R. MARK2/4 promotes warburg effect and cell growth in non-small cell lung carcinoma through the AMPKalpha1/mTOR/HIF-1alpha signaling pathway. Biochim Biophys Acta Mol Cell Res (2022) 1869(7):119242. doi: 10.1016/j.bbamcr.2022.119242
16. Noble RA, Thomas H, Zhao Y, Herendi L, Howarth R, Dragoni I, et al. Simultaneous targeting of glycolysis and oxidative phosphorylation as a therapeutic strategy to treat diffuse large b-cell lymphoma. Br J Cancer (2022) 127:937–47. doi: 10.1038/s41416-022-01848-w
17. Kumar V, Singh P, Gupta SK, Ali V, Jyotirmayee, Verma M. Alterations in cellular metabolisms after imatinib therapy: a review. Med Oncol (2022) 39(5):95. doi: 10.1007/s12032-022-01699-8
18. Zhou Y, Guo Y, Tam KY. Targeting glucose metabolism to develop anticancer treatments and therapeutic patents. Expert Opin Ther Pat. (2022) 32(4):441–53. doi: 10.1080/13543776.2022.2027912
19. Wang Y, Wang Y, Ren Y, Zhang Q, Yi P, Cheng C. Metabolic modulation of immune checkpoints and novel therapeutic strategies in cancer. Semin Cancer Biol (2022). doi: 10.1016/j.semcancer.2022.02.010
20. Bean JF, Qiu YY, Yu S, Clark S, Chu F, Madonna MB. Glycolysis inhibition and its effect in doxorubicin resistance in neuroblastoma. J Pediatr Surg (2014) 49(6):981–4. doi: 10.1016/j.jpedsurg.2014.01.037
21. Dhooge CR, De Moerloose BM, Benoit YC, Van Roy N, Philippe, Laureys GG. Expression of the MDR1 gene product p-glycoprotein in childhood neuroblastoma. Cancer (1997) 80(7):1250–7. doi: 10.1002/(SICI)1097-0142(19971001)80:7<1250::AID-CNCR8>3.0.CO;2-O
22. Kutlik MT, Ayhan A, Gogus S, Yalcin B, Caglar M, Buyukpamukcu M. Glutathione s-transferase and p-glycoprotein expressions in neuroblastoma. Pediatr Hematol Oncol (2002) 19(5):337–45. doi: 10.1080/08880010290057354
23. Sirover MA. Structural analysis of glyceraldehyde-3-phosphate dehydrogenase functional diversity. Int J Biochem Cell Biol (2014) 57:20–6. doi: 10.1016/j.biocel.2014.09.026
24. Craven RJ, Frazier HN, Thibault O. Dependence of glucose transport on autophagy and GAPDH activity. Brain Res (2022) 1776:147747. doi: 10.1016/j.brainres.2021.147747
25. Glaser PE, Gross RW. Rapid plasmenylethanolamine-selective fusion of membrane bilayers catalyzed by an isoform of glyceraldehyde-3-phosphate dehydrogenase: discrimination between glycolytic and fusogenic roles of individual isoforms. Biochemistry (1995) 34(38):12193–203. doi: 10.1021/bi00038a013
26. Kumar S, Sheokand N, Mhadeshwar MA, Raje CI, Raje M. Characterization of glyceraldehyde-3-phosphate dehydrogenase as a novel transferrin receptor. Int J Biochem Cell Biol (2012) 44(1):189–99. doi: 10.1016/j.biocel.2011.10.016
27. Rawat P, Kumar S, Sheokand N, Raje CI, Raje M. The multifunctional glycolytic protein glyceraldehyde-3-phosphate dehydrogenase (GAPDH) is a novel macrophage lactoferrin receptor. Biochem Cell Biol (2012) 90(3):329–38. doi: 10.1139/o11-058
28. Wijesinghe TP, Dharmasivam M, Dai CC, Richardson DR. Innovative therapies for neuroblastoma: The surprisingly potent role of iron chelation in up-regulating metastasis and tumor suppressors and down-regulating the key oncogene, n-myc. Pharmacol Res (2021) 173:105889. doi: 10.1016/j.phrs.2021.105889
29. Floros KV, Cai J, Jacob S, Kurupi R, Fairchild CK, Shende M, et al. MYCN-amplified neuroblastoma is addicted to iron and vulnerable to inhibition of the system xc-/Glutathione axis. Cancer Res (2021) 81(7):1896–908. doi: 10.1158/0008-5472.CAN-20-1641
30. Alborzinia H, Florez AF, Kreth S, Bruckner LM, Yildiz U, Gartlgruber M, et al. MYCN mediates cysteine addiction and sensitizes neuroblastoma to ferroptosis. Nat Cancer (2022) 3(4):471–85. doi: 10.1038/s43018-022-00355-4
31. Chauhan AS, Kumar M, Chaudhary S, Patidar A, Dhiman A, Sheokand N, et al. Moonlighting glycolytic protein glyceraldehyde-3-phosphate dehydrogenase (GAPDH): an evolutionarily conserved plasminogen receptor on mammalian cells. FASEB J (2017) 31(6):2638–48. doi: 10.1096/fj.201600982R
32. Heard KS, Diguette M, Heard AC, Carruthers A. Membrane-bound glyceraldehyde-3-phosphate dehydrogenase and multiphasic erythrocyte sugar transport. Exp Physiol (1998) 83(2):195–202. doi: 10.1113/expphysiol.1998.sp004103
33. Zaid H, Talior-Volodarsky I, Antonescu C, Liu Z, Klip A. GAPDH binds GLUT4 reciprocally to hexokinase-II and regulates glucose transport activity. Biochem J (2009) 419(2):475–84. doi: 10.1042/BJ20081319
34. Freebody J, Wegner EA, Rossleigh MA. 2-deoxy-2-((18)F)fluoro-D-glucose positron emission tomography/computed tomography imaging in paediatric oncology. World J Radiol (2014) 6(10):741–55. doi: 10.4329/wjr.v6.i10.741
35. Ramani P, Headford A, May MT. GLUT1 protein expression correlates with unfavourable histologic category and high risk in patients with neuroblastic tumours. Virchows Arch (2013) 462(2):203–9. doi: 10.1007/s00428-012-1370-4
36. Matsushita K, Uchida K, Saigusa S, Ide S, Hashimoto K, Koike Y, et al. Glycolysis inhibitors as a potential therapeutic option to treat aggressive neuroblastoma expressing GLUT1. J Pediatr Surg (2012) 47(7):1323–30. doi: 10.1016/j.jpedsurg.2011.12.007
37. Peng Y, Xing SN, Tang HY, Wang CD, Yi FP, Liu GL, et al. Influence of glucose transporter 1 activity inhibition on neuroblastoma in vitro. Gene (2019) 689:11–7. doi: 10.1016/j.gene.2018.12.010
38. Noguchi C, Kamitori K, Hossain A, Hoshikawa H, Katagi A, Dong Y, et al. D-allose inhibits cancer cell growth by reducing GLUT1 expression. Tohoku J Exp Med (2016) 238(2):131–41. doi: 10.1620/tjem.238.131
39. Tisdale EJ. Glyceraldehyde-3-phosphate dehydrogenase is required for vesicular transport in the early secretory pathway. J Biol Chem (2001) 276(4):2480–6. doi: 10.1074/jbc.M007567200
40. Tisdale EJ, Talati NK, Artalejo CR, Shisheva A. GAPDH binds akt to facilitate cargo transport in the early secretory pathway. Exp Cell Res (2016) 349(2):310–9. doi: 10.1016/j.yexcr.2016.10.025
41. Dar GH, Mendes CC, Kuan WL, Speciale AA, Conceicao M, Gorgens A, et al. GAPDH controls extracellular vesicle biogenesis and enhances the therapeutic potential of EV mediated siRNA delivery to the brain. Nat Commun (2021) 12(1):6666. doi: 10.1038/s41467-021-27056-3
42. Zala D, Hinckelmann MV, Yu H, Lyra da Cunha MM, Liot G, Cordelieres FP, et al. Vesicular glycolysis provides on-board energy for fast axonal transport. Cell (2013) 152(3):479–91. doi: 10.1016/j.cell.2012.12.029
43. Hinckelmann MV, Virlogeux A, Niehage C, Poujol C, Choquet D, Hoflack B, et al. Self-propelling vesicles define glycolysis as the minimal energy machinery for neuronal transport. Nat Commun (2016) 7:13233. doi: 10.1038/ncomms13233
44. Yang JS, Hsu JW, Park SY, Li J, Oldham WM, Beznoussenko GV, et al. GAPDH inhibits intracellular pathways during starvation for cellular energy homeostasis. Nature (2018) 561(7722):263–7. doi: 10.1038/s41586-018-0475-6
45. Kwon HJ, Rhim JH, Jang IS, Kim GE, Park SC, Yeo EJ. Activation of AMP-activated protein kinase stimulates the nuclear localization of glyceraldehyde 3-phosphate dehydrogenase in human diploid fibroblasts. Exp Mol Med (2010) 42(4):254–69. doi: 10.3858/emm.2010.42.4.025
46. Huang Q, Lan F, Zheng Z, Xie F, Han J, Dong L, et al. Akt2 kinase suppresses glyceraldehyde-3-phosphate dehydrogenase (GAPDH)-mediated apoptosis in ovarian cancer cells via phosphorylating GAPDH at threonine 237 and decreasing its nuclear translocation. J Biol Chem (2011) 286(49):42211–20. doi: 10.1074/jbc.M111.296905
47. Tossounian MA, Zhang B, Gout I. The writers, readers, and erasers in redox regulation of GAPDH. Antioxidants (Basel) (2020) 9(12):1288. doi: 10.3390/antiox9121288
48. Chang C, Su H, Zhang D, Wang Y, Shen Q, Liu B, et al. AMPK-dependent phosphorylation of GAPDH triggers Sirt1 activation and is necessary for autophagy upon glucose starvation. Mol Cell (2015) 60(6):930–40. doi: 10.1016/j.molcel.2015.10.037
49. Hara MR, Agrawal N, Kim SF, Cascio MB, Fujimuro M, Ozeki Y, et al. S-nitrosylated GAPDH initiates apoptotic cell death by nuclear translocation following Siah1 binding. Nat Cell Biol (2005) 7(7):665–74. doi: 10.1038/ncb1268
50. Ventura M, Mateo F, Serratosa J, Salaet I, Carujo S, Bachs O, et al. Nuclear translocation of glyceraldehyde-3-phosphate dehydrogenase is regulated by acetylation. Int J Biochem Cell Biol (2010) 42(10):1672–80. doi: 10.1016/j.biocel.2010.06.014
51. Tarze A, Deniaud A, Le Bras M, Maillier E, Molle D, Larochette N, et al. GAPDH, a novel regulator of the pro-apoptotic mitochondrial membrane permeabilization. Oncogene (2007) 26(18):2606–20. doi: 10.1038/sj.onc.1210074
52. Chuang DM, Hough C, Senatorov VV. Glyceraldehyde-3-phosphate dehydrogenase, apoptosis, and neurodegenerative diseases. Annu Rev Pharmacol Toxicol (2005) 45:269–90. doi: 10.1146/annurev.pharmtox.45.120403.095902
53. Sen N, Hara MR, Kornberg MD, Cascio MB, Bae BI, Shahani N, et al. Nitric oxide-induced nuclear GAPDH activates p300/CBP and mediates apoptosis. Nat Cell Biol (2008) 10(7):866–73. doi: 10.1038/ncb1747
54. Sen N, Hara MR, Ahmad AS, Cascio MB, Kamiya A, Ehmsen JT, et al. GOSPEL: a neuroprotective protein that binds to GAPDH upon s-nitrosylation. Neuron (2009) 63(1):81–91. doi: 10.1016/j.neuron.2009.05.024
55. Hou X, Snarski P, Higashi Y, Yoshida T, Jurkevich A, Delafontaine P, et al. Nuclear complex of glyceraldehyde-3-phosphate dehydrogenase and DNA repair enzyme apurinic/apyrimidinic endonuclease I protect smooth muscle cells against oxidant-induced cell death. FASEB J (2017) 31(7):3179–92. doi: 10.1096/fj.201601082R
56. Itoh Y, Kodama K, Furuya K, Takahashi S, Haneishi T, Takiguchi Y, et al. A new sesquiterpene antibiotic, heptelidic acid producing organisms, fermentation, isolation and characterization. J Antibiot (Tokyo) (1980) 33(5):468–73. doi: 10.7164/antibiotics.33.468
57. Endo A, Hasumi K, Sakai K, Kanbe T. Specific inhibition of glyceraldehyde-3-phosphate dehydrogenase by koningic acid (heptelidic acid). J Antibiot (Tokyo) (1985) 38(7):920–5. doi: 10.7164/antibiotics.38.920
58. Kawashima J, Ito F, Kato T, Niwano M, Koshino H, Uramoto M. Antitumor activity of heptelidic acid chlorohydrin. J Antibiot (Tokyo) (1994) 47(12):1562–3. doi: 10.7164/antibiotics.47.1562
59. Kim JH, Lee CH. Heptelidic acid, a sesquiterpene lactone, inhibits etoposide-induced apoptosis in human leukemia U937 cells. J Microbiol Biotechnol (2009) 19(8):787–91. doi: 10.4014/jmb.0810.585
60. Konishi H, Isozaki S, Kashima S, Moriichi K, Ichikawa S, Yamamoto K, et al. Probiotic aspergillus oryzae produces anti-tumor mediator and exerts anti-tumor effects in pancreatic cancer through the p38 MAPK signaling pathway. Sci Rep (2021) 11(1):11070. doi: 10.1038/s41598-021-90707-4
61. Nakazawa M, Uehara T, Nomura Y. Koningic acid (a potent glyceraldehyde-3-phosphate dehydrogenase inhibitor)-induced fragmentation and condensation of DNA in NG108-15 cells. J Neurochem (1997) 68(6):2493–9. doi: 10.1046/j.1471-4159.1997.68062493.x
62. Peng X, Gong F, Chen Y, Jiang Y, Liu J, Yu M, et al. Autophagy promotes paclitaxel resistance of cervical cancer cells: involvement of warburg effect activated hypoxia-induced factor 1-alpha-mediated signaling. Cell Death Dis (2014) 5:e1367. doi: 10.1038/cddis.2014.297
63. Butera G, Mullappilly N, Masetto F, Palmieri M, Scupoli MT, Pacchiana R, et al. Regulation of autophagy by nuclear GAPDH and its aggregates in cancer and neurodegenerative disorders. Int J Mol Sci (2019) 20(9):2062–79. doi: 10.3390/ijms20092062
64. Nakajima H, Kubo T, Ihara H, Hikida T, Danjo T, Nakatsuji M, et al. Nuclear-translocated glyceraldehyde-3-phosphate dehydrogenase promotes Poly(ADP-ribose) polymerase-1 activation during Oxidative/Nitrosative stress in stroke. J Biol Chem (2015) 290(23):14493–503. doi: 10.1074/jbc.M114.635607
65. Jannetti SA, Zeglis BM, Zalutsky MR, Reiner T. Poly(ADP-Ribose)Polymerase (PARP) inhibitors and radiation therapy. Front Pharmacol (2020) 11:170. doi: 10.3389/fphar.2020.00170
66. Kamoshima W, Kitamura Y, Nomura Y, Taniguchi T. Possible involvement of ADP-ribosylation of particular enzymes in cell death induced by nitric oxide-donors in human neuroblastoma cells. Neurochem Int (1997) 30(3):305–11. doi: 10.1016/S0197-0186(96)00091-5
67. Du X, Matsumura T, Edelstein D, Rossetti L, Zsengeller Z, Szabo C, et al. Inhibition of GAPDH activity by poly(ADP-ribose) polymerase activates three major pathways of hyperglycemic damage in endothelial cells. J Clin Invest. (2003) 112(7):1049–57. doi: 10.1172/JCI18127
68. Devalaraja-Narashimha K, Padanilam BJ. PARP-1 inhibits glycolysis in ischemic kidneys. J Am Soc Nephrol (2009) 20(1):95–103. doi: 10.1681/ASN.2008030325
69. Nie F, Yan J, Ling Y, Liu Z, Fu C, Li X, et al. Effect of shuangdan mingmu capsule, a Chinese herbal formula, on oxidative stress-induced apoptosis of pericytes through PARP/GAPDH pathway. BMC Complement Med Ther (2021) 21(1):118. doi: 10.1186/s12906-021-03238-w
70. Belounis A, Nyalendo C, Le Gall R, Imbriglio TV, Mahma M, Teira P, et al. Autophagy is associated with chemoresistance in neuroblastoma. BMC Cancer. (2016) 16(1):891. doi: 10.1186/s12885-016-2906-9
71. Alvarez-Meythaler JG, Garcia-Mayea Y, Mir C, Kondoh H, ME LL. Autophagy takes center stage as a possible cancer hallmark. Front Oncol (2020) 10:586069. doi: 10.3389/fonc.2020.586069
72. Wang D, Jiang Y, Wang T, Wang Z, Zou F. Identification of a novel autophagy-related prognostic signature and small molecule drugs for glioblastoma by bioinformatics. BMC Med Genomics (2022) 15(1):111. doi: 10.1186/s12920-022-01261-5
73. Lin X, Han L, Weng J, Wang K, Chen T. Rapamycin inhibits proliferation and induces autophagy in human neuroblastoma cells. Biosci Rep (2018) 38(6):1822–30. doi: 10.1042/BSR20181822
74. Ogata M, Hino S, Saito A, Morikawa K, Kondo S, Kanemoto S, et al. Autophagy is activated for cell survival after endoplasmic reticulum stress. Mol Cell Biol (2006) 26(24):9220–31. doi: 10.1128/MCB.01453-06
75. Xiong N, Jia M, Chen C, Xiong J, Zhang Z, Huang J, et al. Potential autophagy enhancers attenuate rotenone-induced toxicity in SH-SY5Y. Neuroscience (2011) 199:292–302. doi: 10.1016/j.neuroscience.2011.10.031
76. Mohan N, Banik NL, Ray SK. Combination of n-(4-hydroxyphenyl) retinamide and apigenin suppressed starvation-induced autophagy and promoted apoptosis in malignant neuroblastoma cells. Neurosci Lett (2011) 502(1):24–9. doi: 10.1016/j.neulet.2011.07.016
77. Michaelis M, Cinatl J, Anand P, Rothweiler F, Kotchetkov R, von Deimling A, et al. Onconase induces caspase-independent cell death in chemoresistant neuroblastoma cells. Cancer Lett (2007) 250(1):107–16. doi: 10.1016/j.canlet.2006.09.018
78. Dong Y, Gong W, Hua Z, Chen B, Zhao G, Liu Z, et al. Combination of rapamycin and MK-2206 induced cell death via autophagy and necroptosis in MYCN-amplified neuroblastoma cell lines. Front Pharmacol (2020) 11:31. doi: 10.3389/fphar.2020.00031
79. Castino R, Fiorentino I, Cagnin M, Giovia A, Isidoro C. Chelation of lysosomal iron protects dopaminergic SH-SY5Y neuroblastoma cells from hydrogen peroxide toxicity by precluding autophagy and akt dephosphorylation. Toxicol Sci (2011) 123(2):523–41. doi: 10.1093/toxsci/kfr179
80. Tanida I, Ueno T, Kominami E. LC3 and autophagy. Methods Mol Biol (2008) 445:77–88. doi: 10.1007/978-1-59745-157-4_4
81. Tanaka S, Tajiri T, Noguchi S, Shono K, Ihara K, Hara T, et al. Clinical significance of a highly sensitive analysis for gene dosage and the expression level of MYCN in neuroblastoma. J Pediatr Surg (2004) 39(1):63–8. doi: 10.1016/j.jpedsurg.2003.09.015
82. Barber RD, Harmer DW, Coleman RA, Clark BJ. GAPDH as a housekeeping gene: analysis of GAPDH mRNA expression in a panel of 72 human tissues. Physiol Genomics (2005) 21(3):389–95. doi: 10.1152/physiolgenomics.00025.2005
83. Makhina T, Loers G, Schulze C, Ueberle B, Schachner M, Kleene R. Extracellular GAPDH binds to L1 and enhances neurite outgrowth. Mol Cell Neurosci (2009) 41(2):206–18. doi: 10.1016/j.mcn.2009.02.010
84. Kumar R, Kumari R, Kumar S, Jangir DK, Maiti TK. Extracellular alpha-synuclein disrupts membrane nanostructure and promotes s-Nitrosylation-Induced neuronal cell death. Biomacromolecules (2018) 19(4):1118–29. doi: 10.1021/acs.biomac.7b01727
85. Dastoor Z, Dreyer JL. Potential role of nuclear translocation of glyceraldehyde-3-phosphate dehydrogenase in apoptosis and oxidative stress. J Cell Sci (2001) 114(Pt 9):1643–53. doi: 10.1242/jcs.114.9.1643
86. Maruyama W, Oya-Ito T, Shamoto-Nagai M, Osawa T, Naoi M. Glyceraldehyde-3-phospate dehydrogenase is translocated into nuclei through golgi apparatus during apoptosis induced by 6-hydroxydopamine in human dopaminergic SH-SY5Y cells. Neurosci Lett (2002) 321(1-2):29–32. doi: 10.1016/S0304-3940(01)02490-9
87. Puttonen KA, Lehtonen S, Raasmaja A, Mannisto PT. A prolyl oligopeptidase inhibitor, z-Pro-Prolinal, inhibits glyceraldehyde-3-phosphate dehydrogenase translocation and production of reactive oxygen species in CV1-p cells exposed to 6-hydroxydopamine. Toxicol In Vitro (2006) 20(8):1446–54. doi: 10.1016/j.tiv.2006.07.001
88. Ou XM, Stockmeier CA, Meltzer HY, Overholser JC, Jurjus GJ, Dieter L, et al. A novel role for glyceraldehyde-3-phosphate dehydrogenase and monoamine oxidase b cascade in ethanol-induced cellular damage. Biol Psychiatry (2010) 67(9):855–63. doi: 10.1016/j.biopsych.2009.10.032
89. Leisner TM, Moran C, Holly SP, Parise LV. CIB1 prevents nuclear GAPDH accumulation and non-apoptotic tumor cell death via AKT and ERK signaling. Oncogene (2013) 32(34):4017–27. doi: 10.1038/onc.2012.408
90. Fang M, Jin A, Zhao Y, Liu X. Homocysteine induces glyceraldehyde-3-phosphate dehydrogenase acetylation and apoptosis in the neuroblastoma cell line Neuro2a. Braz J Med Biol Res (2016) 49(2):e4543. doi: 10.1590/1414-431X20154543
91. Sakaguchi M, Nishiuchi R, Bando M, Yamada Y, Kondo R, Mitsumori M, et al. Prolyl oligopeptidase participates in the cytosine arabinoside-induced nuclear translocation of glyceraldehyde 3-phosphate dehydrogenase in a human neuroblastoma cell line. Biochem Biophys Res Commun (2021) 572:65–71. doi: 10.1016/j.bbrc.2021.07.094
92. Lazarev VF, Nikotina AD, Semenyuk PI, Evstafyeva DB, Mikhaylova ER, Muronetz VI, et al. Small molecules preventing GAPDH aggregation are therapeutically applicable in cell and rat models of oxidative stress. Free Radic Biol Med (2016) 92:29–38. doi: 10.1016/j.freeradbiomed.2015.12.025
93. Nakajima H, Amano W, Kubo T, Fukuhara A, Ihara H, Azuma YT, et al. Glyceraldehyde-3-phosphate dehydrogenase aggregate formation participates in oxidative stress-induced cell death. J Biol Chem (2009) 284(49):34331–41. doi: 10.1074/jbc.M109.027698
94. Nakajima H, Amano W, Fukuhara A, Kubo T, Misaki S, Azuma YT, et al. An aggregate-prone mutant of human glyceraldehyde-3-phosphate dehydrogenase augments oxidative stress-induced cell death in SH-SY5Y cells. Biochem Biophys Res Commun (2009) 390(3):1066–71. doi: 10.1016/j.bbrc.2009.10.118
95. Lazarev VF, Dutysheva EA, Komarova EY, Mikhaylova ER, Guzhova IV, Margulis BA. GAPDH-targeted therapy - a new approach for secondary damage after traumatic brain injury on rats. Biochem Biophys Res Commun (2018) 501(4):1003–8. doi: 10.1016/j.bbrc.2018.05.099
96. Dodson M, Liang Q, Johnson MS, Redmann M, Fineberg N, Darley-Usmar VM, et al. Inhibition of glycolysis attenuates 4-hydroxynonenal-dependent autophagy and exacerbates apoptosis in differentiated SH-SY5Y neuroblastoma cells. Autophagy (2013) 9(12):1996–2008. doi: 10.4161/auto.26094
97. Ping Z, Fan H, Wen C, Ji Z, Liang S. GAPDH siRNA regulates SH-SY5Y cell apoptosis induced by exogenous alpha-synuclein protein. Neuroscience (2021) 469:91–102. doi: 10.1016/j.neuroscience.2021.06.035
Keywords: neuroblastoma, metabolism, glycolysis, glucose, autophagy
Citation: Cornett K, Puderbaugh A, Back O and Craven R (2022) GAPDH in neuroblastoma: Functions in metabolism and survival. Front. Oncol. 12:979683. doi: 10.3389/fonc.2022.979683
Received: 27 June 2022; Accepted: 20 September 2022;
Published: 04 October 2022.
Edited by:
Hanchen Xu, Longhua Hospital Shanghai University of Traditional Chinese Medicine, ChinaReviewed by:
Helene Launay, UMR7281 Bioénergétique et Ingénierie des Protéines, (CNRS), FranceCopyright © 2022 Cornett, Puderbaugh, Back and Craven. This is an open-access article distributed under the terms of the Creative Commons Attribution License (CC BY). The use, distribution or reproduction in other forums is permitted, provided the original author(s) and the copyright owner(s) are credited and that the original publication in this journal is cited, in accordance with accepted academic practice. No use, distribution or reproduction is permitted which does not comply with these terms.
*Correspondence: Rolf Craven, cm9sZi5jcmF2ZW5AdWt5LmVkdQ==
†These authors have contributed equally to this work and share first authorship
Disclaimer: All claims expressed in this article are solely those of the authors and do not necessarily represent those of their affiliated organizations, or those of the publisher, the editors and the reviewers. Any product that may be evaluated in this article or claim that may be made by its manufacturer is not guaranteed or endorsed by the publisher.
Research integrity at Frontiers
Learn more about the work of our research integrity team to safeguard the quality of each article we publish.