- 1Department of Biology, Faculty of Science II, Lebanese University, Beirut, Lebanon
- 2Université Claude Bernard Lyon 1, Lyon, France
- 3INSERM, Unit 1033, LYOS, Lyon, France
- 4Department of Chemistry and Biochemistry, Laboratory of Cancer Biology and Molecular Immunology, Faculty of Science I, Lebanese University, Hadat, Lebanon
- 5Department of Anatomy, Cell Biology and Physiology, Faculty of Medicine, American University of Beirut, Beirut, Lebanon
- 6Department of Pediatric and Adolescent Medicine, American University of Beirut Medical Center, Beirut, Lebanon
- 7Aix-Marseille University, INSERM 1263, INRAE 1260, C2VN, Marseille, France
Rhabdomyosarcoma (RMS) is a soft tissue sarcoma of skeletal muscle differentiation, with a predominant occurrence in children and adolescents. One of the major challenges facing treatment success is the presence of metastatic disease at the time of diagnosis, commonly associated with the more aggressive fusion-positive subtype. Non-coding RNA (ncRNA) can regulate gene transcription and translation, and their dysregulation has been associated with cancer development and progression. MicroRNA (miRNA) are short non-coding nucleic acid sequences involved in the regulation of gene expression that act by targeting messenger RNA (mRNA), and their aberrant expression has been associated with both RMS initiation and progression. Other ncRNA including long non-coding RNA (lncRNA), circular RNA (circRNA) and ribosomal RNA (rRNA) have also been associated with RMS revealing important mechanistic roles in RMS biology, but these studies are still limited and require further investigation. In this review, we discuss the established roles of ncRNA in RMS differentiation, growth and progression, highlighting their potential use in RMS prognosis, as therapeutic agents or as targets of treatment.
Introduction
Rhabdomyosarcoma (RMS) is a pediatric cancer currently classified into four histological subtypes with embryonal (ERMS) and alveolar (ARMS) being the most common. While ERMS represents 60% of RMS cases, it is less clinically aggressive than ARMS (1, 2). The latter is often characterized by the presence of a fusion oncoprotein, namely paired box 3-forkhead Box O1 (PAX3-FOXO1) or PAX7-FOXO1 that exhibit a more potent transcriptional activation of PAX3/7 targets and are expressed at higher levels compared to wild-type PAX3 and PAX7 (2–4). Presence of these fusion oncoproteins, and more particularly PAX3-FOXO1, is also associated with disease aggressiveness, tumor invasion and metastasis (5–8). Since the absence of a fusion oncoprotein in certain ARMS cases makes them indistinguishable from ERMS in terms of prognosis and overall survival, a better classification of RMS tumors is based on fusion status rather than histology, dividing it into fusion-positive (FP-)RMS or fusion-negative (FN-) RMS (2, 9).
While FP-RMS is associated with chromosomal translocations that encode altered transcription factors, FN-RMS is notably associated with loss-of-heterozygosity (LOH) in the chromosomal region 11p15.5 which harbors tumor suppressive IGF2, H19, and CDKN1C genes, chromosomal gains and losses, TP53 mutations, and increased expression of the HRAS oncogene (10, 11). Concerning the latter, mutations in the proto-oncogenes encoding RAS family members are one of the most commonly occurring mutations in FN-RMS where findings reveal that gain-of-function mutations in HRAS are a common molecular event that occurs during ERMS development (12). Furthermore, alterations in NRAS, KRAS and HRAS genes were mostly found in ERMS compared to fusion-negative ARMS tumors whereas FP-RMS tumors lacked these mutations (13). Despite histological and molecular differences between FP-RMS and FN-RMS, both subtypes exhibit defective or incomplete differentiation (14). FP- and FN-RMS cells consistently express myogenic markers, but they fail to complete differentiation into mature skeletal muscle cells due to mechanisms that are starting to be elucidated, such as an impaired transactivation function of MyoD, a master transcription factor associated with muscle cell differentiation (15).
Moreover, dysregulated expression of non-coding RNA (ncRNA) including microRNA (miRNA), long non-coding RNA (lncRNA), circular RNA (circRNA) and ribosomal RNA (rRNA), is associated with incomplete myogenic differentiation and enhanced proliferation of RMS cells (16–19). Figure 1 represents a brief and general overview of the different classes of ncRNA that are implicated to date in RMS biology, and their biogenesis within the cell.
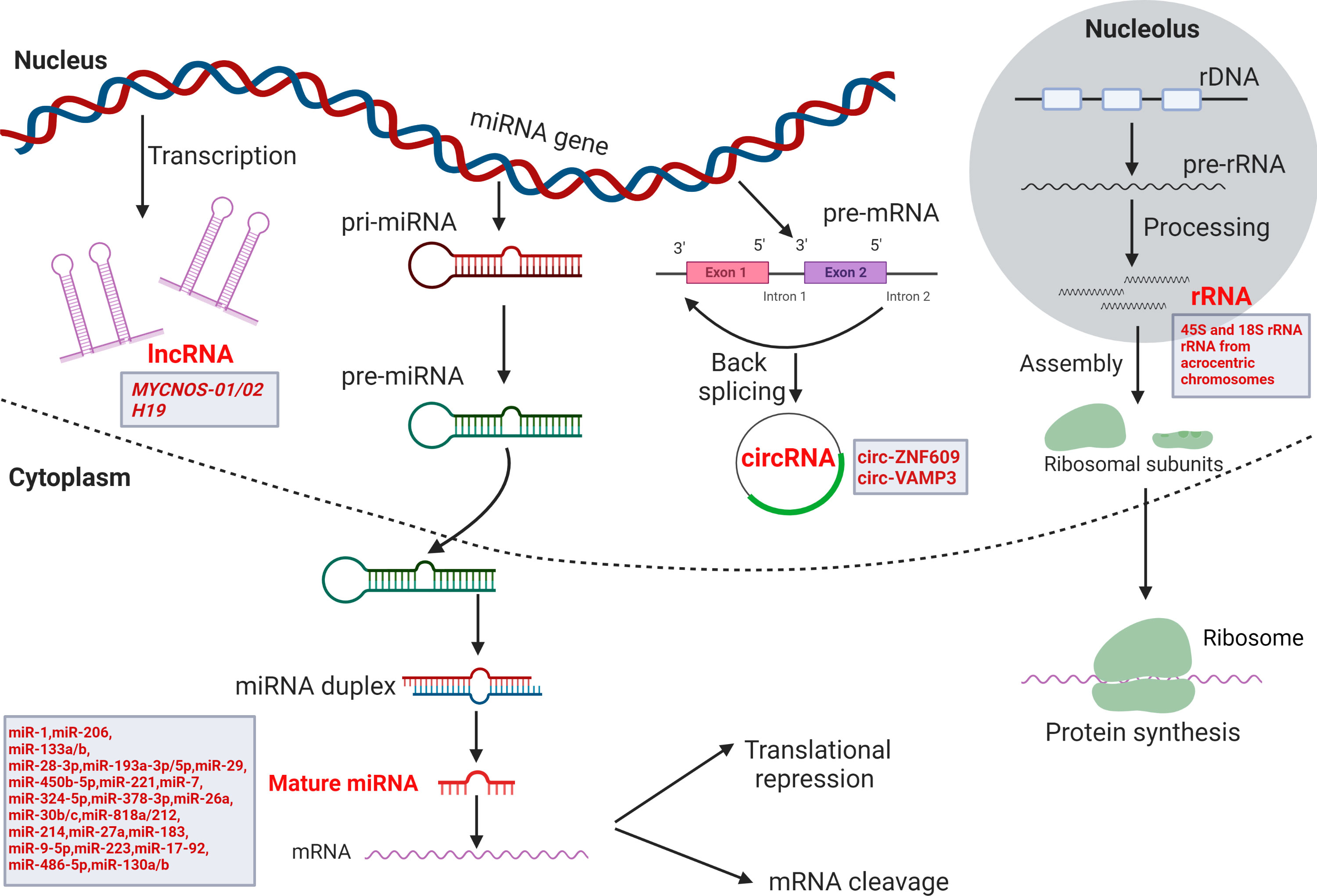
Figure 1 The different types of ncRNA that are implicated in RMS progression and metastasis. From left to right: lncRNA can be transcribed from different regions of DNA including enhancers and promotors; miRNA are transcribed first as pri-miRNA sequences which are subsequently processed into pre-miRNA and transported into the cytoplasm to complete maturation and bind to target mRNA for silencing or degradation; a pre-mRNA can be back-spliced to form a circRNA; within the nucleolus, rDNA can encode rRNA that regulate protein synthesis within the ribosomal complex. The ncRNA implicated to date in RMS biology and progression are represented within boxes. Created BioRender.com.
MiRNA are short ncRNA, with an average length of 22 nucleotides, which regulate cellular pathways and processes, including those involved in cancer growth and progression. The term oncomiR applies to a miRNA that inhibits the expression of tumor suppressive genes and further promotes cell growth and survival; thus, tumor-suppressive miRNA are those whose expression in cancer cells and the tumor microenvironment is often down-regulated to escape anti-cancer mechanisms (20, 21). Several miRNA have dual roles in cancer depending on the type of tumor (22). MiRNA biogenesis begins in the nucleus where a gene is first encoded into a primary transcript (pri-miRNA) which is processed by Drosha, an RNAse III enzyme, associated with the RNA-binding protein Pasha into a precursor pre-miRNA (Figure 1). Within the cytoplasm, the pre-miRNA is further processed by the Dicer enzyme into a functionally active mature miRNA (23) (Figure 1). MiRNA can downregulate target expression levels based on the degree of complementarity to the target mRNA, mostly between the seed region of the miRNA and the 3’ untranslated region (3’UTR) of the target mRNA, which can subsequently result in target degradation (24, 25). On the other hand, when only partial complementarity exists, the miRNA can repress the translation of mRNA, reducing only the protein levels of their target (26, 27). Some miRNA are expressed in a tissue-specific manner indicating a role in cellular differentiation and an association with certain diseases (28). A miRNA can act individually in targeting gene expression and producing important effects, but a concordance in the upregulation or downregulation of different miRNA under specific conditions reveals that multiple miRNA can regulate levels of several transcripts in a cooperative manner leading to augmented outcomes (29–31). In cancer, many miRNA are either enriched or downregulated with the majority of miRNA genes showing decreased expression compared to normal tissue suggesting that most miRNA act by inhibiting tumorigenesis (32, 33). In fact, rapidly emerging evidence suggests that miRNA loss-of-function contributes to cancer growth and progression highlighting the potential of miRNA replacement therapies as treatment strategies (34).
While miRNA modulate the expression of their target mRNA, lncRNA act by regulating gene expression at both the transcriptional and post-transcriptional levels, and some alter post-translational protein function by modulating protein phosphorylation, acetylation or glycosylation (35, 36). They are larger than 200 nucleotides and modulate a myriad of functions in many cellular processes (36). Furthermore, many lncRNA have been shown to interact with chromatin-modifying complexes and can organize chromatin structure into active or inactive domains; this is one way by which nuclear-enriched lncRNA regulate myogenesis (37). In fact, certain lncRNA can regulate the binding of transcription factors to myogenic loci including MyoD (38). They play significant roles in either promoting or suppressing tumor growth and may therefore also act as potential biomarkers with prognostic significance (39). LncRNA may also indirectly regulate mRNA expression by regulating the activity of other ncRNA, they may compete with miRNA in mRNA binding and interestingly, emerging evidence shows that they may also act as miRNA precursors (40, 41).
Also associated with various aspects of skeletal muscle development are circRNA. For instance, circLMO7, which was found to have the most downregulated expression in adult muscle relative to embryonic muscle tissue based on a circRNA expression analysis, is able to sustain myoblast survival and inhibit their differentiation (42). CircRNA are eukaryotic transcripts that result from non-canonical back-splicing causing exon circularization and a very stable structure with no 5′ and 3′ free termini (Figure 1). Their function remains largely undefined but have been shown to bind to miRNA, possibly modulating their function (43). Studies have focused on their role in regulating cell cycle progression and contributing to cancer cell proliferation (43).
While the aforementioned are small ncRNA, rRNA are much larger in size and are essential for ribosome function, protein synthesis and overall cell survival (44). They are mostly transcribed in the nucleoli and along with proteins constitute the ribosomal subunits that control protein synthesis (Figure 1). While ribosome biogenesis is considered a house-keeping process beginning in the nucleolus, studies have shown that it can be modulated in a cell type-specific manner. Cancer cells, for example, can alter rRNA synthesis rate as they demand higher ribosome activity and increased protein synthesis (45). In skeletal muscle, rRNA and ribosomes can regulate gene expression and contribute to myogenesis by promoting the biogenesis of myogenic markers. In one study, DEAD-Box RNA (DDX27) helicase, which is required for skeletal muscle growth, was demonstrated to regulate myogenesis by regulating rRNA maturation (46).
Here we review the identified roles of different ncRNA that may be associated with RMS tumor progression and the development of metastasis and those that aid in better prognosis of RMS. We also highlight the potential use of those ncRNA in therapeutic strategies against RMS including pro-differentiation therapies.
MiRNA implicated in RMS
MiRNA can regulate RMS progression and may serve as clinical biomarkers. They can act by directly regulating myogenic-regulatory factors thereby affecting muscle differentiation (47). Table 1 summarizes the different miRNA described below and that are implicated in RMS differentiation, tumor progression and prognosis.
Tumor suppressive MiRNA
Muscle-specific miRNA or myo-miRNA implicated in RMS
Muscle-specific miRNA, expressed in both cardiac and skeletal muscle, referred to as myo-miRNA or myomiR, regulate normal skeletal muscle differentiation by promoting myogenesis (47). They seem to be essential in muscle differentiation as evidenced by the fact that their dysregulated expression inhibits proper skeletal muscle growth and offers diagnostic potential in skeletal muscle dystrophies (81, 82). Their expression is regulated by myogenic transcription factors essential for skeletal muscle differentiation including MYOD1, MYOG, MYF5, MYF6 and MEF2 (83). Canonical myomiR currently include the miR-1 family (84). This latter can be divided into two groups based on the seed region: miR-1/miR-206 and miR-133a/b (81). MyomiR miR-1 and miR-206 differ by 3 nucleotides outside the seed region and miR-206 is the only known myomiR that is specific to skeletal muscle tissue (51, 85). Later members of the myomiR family include miR-208a, miR-208b, miR-499 and miR-486 (86). miR-486 is considered as “muscle-enriched” since its expression is not limited to muscle tissue, but it is nonetheless considered by some studies as a myomiR (83). miR-208a (which is cardiac specific) and miR-499 serum levels can be associated with myocardial damage in cardiovascular disease (87). The role of miR-1, miR-206 and miR-133 in skeletal muscle differentiation and RMS biology is described below.
MiR-1 and miR-206
While the upregulated expression of myomiR, particularly miR-1 and miR-206, in RMS cell lines relative to other sarcoma subtypes supports the myogenic origin of RMS, their downregulated expression in RMS tumors compared to normal skeletal muscle tissue suggests that they may be important tumor-suppressive agents in RMS (31, 47) (Figure 2). In fact, both miR-1 and miR-206 levels are downregulated in primary ARMS and ERMS tumors relative to normal skeletal muscle (47). This may be secondary to the downregulation in myogenic factors, such as MyoD which normally activates miR-1 and miR-206 expression, but is itself inactivated in RMS (88). Of note, in comparison studies, normal skeletal muscle would not be the ideal control for RMS as the former displays complete myogenic differentiation; a better control would therefore be skeletal myoblasts or satellite cells. In turn, miR-1 levels are higher in FP-RMS compared to FN-RMS which may be due to elevated myogenic factor levels in FP-RMS (52). Additionally, the ectopic upregulation of miR-1 mediates cell cycle arrest in FN-ERMS only, associated with the upregulation of myogenin (MyoG) (60). As for miR-206 expression in the two fusion subtypes, analysis of a large number of primary RMS samples revealed that low miR-206 levels correlate with poor overall survival, advanced stage and metastatic disease upon diagnosis in FN-RMS and expressing it in both FN-RMS and FP-RMS proved to have therapeutic potential (52). On the other hand, while miR-206 levels were downregulated in RMS tissues, plasma levels of circulating miR-206 were found to be upregulated in RMS patients, namely ARMS, compared to both healthy donors and non-RMS patients with other tumor types suggesting its potential use as prognostic biomarker in serum samples of RMS patients (53, 89). While in this study localization of serum miR-206 is not specified, this could be a mechanism of cellular release and disposal of tumor suppressive miRNA which can be mediated via extracellular vesicles, including exosomes. In fact, tumor cells may maintain their oncogenic properties by exosomal release of tumor suppressive miRNA that becomes elevated in patient sera (90).
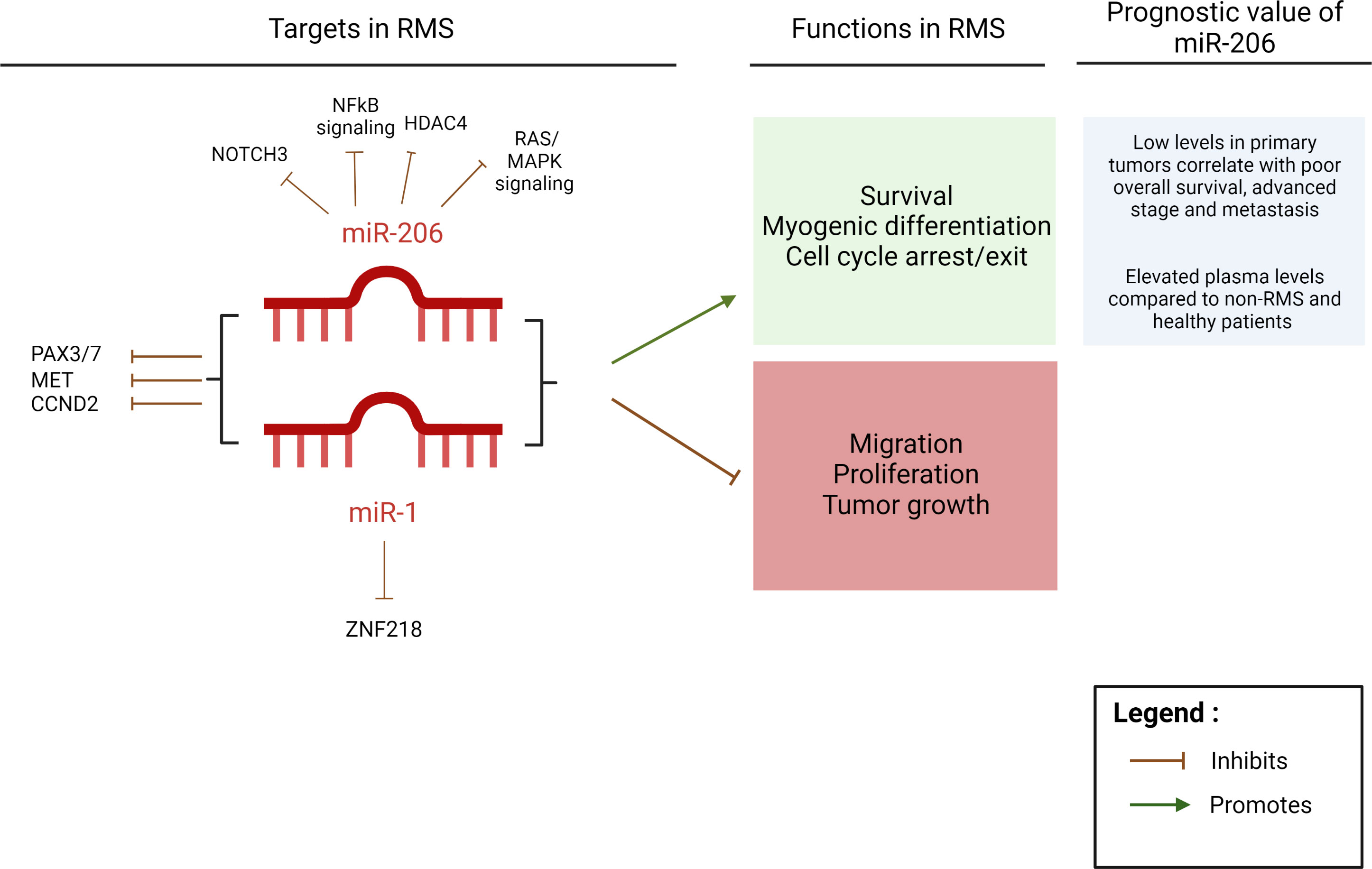
Figure 2 miR-1 and miR-206 targets and functions in RMS. Schematic diagram of miR-1 and miR-206 common and unique targets that have been demonstrated in RMS, and their functions and the prognostic value of miR-206 in RMS. Created with BioRender.com.
In addition to myogenic factors, myo-miR expression can be regulated by SNAIL proteins. For example, MiR-206 can promote myogenic differentiation in ARMS cells upon silencing of SNAIL family zinc finger 1 (54). SNAIL is a transcription factor with established roles in regulating RMS growth and differentiation (54, 91). SNAIL expression levels are higher in ARMS tumors compared to ERMS, which is associated with worse prognosis and its levels correlate with those of PAX3/7-FOXO1 (92). One of the mechanisms of action of SNAIL is regulating the expression of miRNA. In one study, silencing SNAIL was able to induce myogenic differentiation in the FP-RMS cell line Rh30 through the upregulation of myogenic factors which then upregulate miR-206 (54). The regulation of myogenic differentiation was found to be owed to miR-206 since transfection of Rh30 cells with the miR-206 precursor supported this differentiation whereas miR-206 inhibition reversed the effect induced by SNAIL knockdown (54).
MiR-1/miR-206 expression may also be regulated by PAX transcription factors. This is demonstrated by the fact that in FP-RMS Rh30 cell line, subclones lacking the expression of transcription factor PAX7 displayed decreased proliferation and migration accompanied with enhanced expression of several myomiR including miR-1-3p, miR-133a-3p, miR-133b, and miR-206 compared to PAX7-positive cells (93). SiRNA-mediated silencing of PAX7 in the latter led to increased expression of these myomiR. This suggests that PAX7 could downregulate myomiR expression levels in ARMS and contribute to its progression, which requires further study.
Both miR-1 and miR-206, sharing the same seed region sequence, were found to target PAX3 in FN-RMS but not FP-RMS which is mostly likely due to the loss of the PAX3 3’UTR fragment upon fusion with FOXO1 (31). Mir-1 and mir-206 were also found to target Pax7 in mouse skeletal muscle satellite cells and the inhibition of these miRNA not only stabilizes Pax7 expression but also inhibits myogenic differentiation and promotes cell proliferation (48). Pax3 and Pax7 transcription factors are known to be critical in activating the myogenic program whereby in their absence, muscle progenitor cells fail to enter myogenesis and complete differentiation into mature skeletal muscle fibers (94). However, their aberrant or sustained expression may prevent terminal differentiation of cells or contribute to tumorigenesis (48, 55, 95, 96). Moreover, using gene and proteomic profiling, low levels of PAX7 transcript and protein were found to be necessary for miR-206-mediated differentiation and cell cycle exit of FN-RMS cells only, which may be due to the absence of miR-206 binding sites in the fusion oncoprotein of FP-RMS cells (55). In addition to PAX3/7, key targets of miR-206 included NOTCH3 and cyclin D2 (CCND2). However, since miR-206 is able to promote differentiation and cell cycle exit in both FN-RMS and FP-RMS subtypes, this indicates that miR-206 role in RMS tumorigenesis is not only acting through the regulation of PAX7 (55). Based on these studies, one can conclude that while miR-206 is capable of promoting RMS myogenic differentiation by different mechanisms of action, the upregulation of PAX7 in FN-RMS and the fusion of PAX7 with FOXO1 in FP-RMS are mechanisms by which RMS cells could evade miR-206-mediated differentiation and inhibition of cell cycle progression, therefore contributing to the aggressive behavior of this subtype. In turn, downregulating PAX7 expression levels promotes the expression of myomiR including miR-206 revealing an intricate interplay between genetic drivers of RMS tumorigenesis and myomiR function.
Significance of miR-1 and miR-206 in RMS has been attributed to the high levels of MET receptor, whose mRNA is a potential target of both miRNA in RMS tumors (49, 51). C-Met is a tyrosine kinase receptor and a transcriptional target of PAX3 (97). It is physiologically downregulated during myogenic differentiation but is overexpressed in both FN-RMS and FP-RMS, where its expression is upregulated by PAX3-FOXO1, and promotes metastatic behavior of tumor cells in both subtypes (97, 98). In fact, shRNA-mediated knockdown of MET, following induction with doxycycline, resulted in marked reduction of RMS xenograft tumor size in mice (97). Moreover, MET protein enhances RMS motility and metastatic propensity by activating downstream ERK signaling pathway (99). Its protein levels inversely correlated with miR-1 and miR-206 levels in RMS cells and the re-introduction of miR-1/206 into ERMS cells induced a downregulation of c-Met expression levels, which was found to have two target sites for miR-1/206 binding as revealed by bioinformatics analysis, as well as a significant reduction in the levels of phosphorylated ERK1/2 and FAK (49). miR-1/206 ectopic expression in RD cells (an ERMS cell line), in turn, decreased RMS proliferation and migration in vitro and tumor growth in vivo (49). Additionally, miR-206 re-expression in RMS induced myogenic differentiation and switched the neoplastic phenotype into one resembling skeletal muscle. This miRNA caused a reduction in Met levels to a level comparable to that of differentiating murine satellite cells, accompanied by an enhanced myogenic differentiation of both FP-RMS and FN-RMS cells (51).
Both miR-1 and miR-206 have binding sites in the 3’UTR region of CCND2 and ectopic expression of either miRNA was able to downregulate CCND2 levels in RMS cells (31). CCND2 was shown to be a key regulator in promoting myogenic differentiation of muscle progenitor cells in dystrophin-deficient mice (100). RMS cells exhibit elevated expression of cell cycle regulator genes including CCND2 compared to normal skeletal myocytes (101). While not demonstrated in this study, this suggests that miR-1/206-mediated silencing of CCND2 could help reverse the incomplete myogenic differentiation of RMS cells (50).
MiR-1 has also been shown to inhibit ZNF281, a zinc finger transcription factor implicated in modulating cellular stemness, enhancing epithelial mesenchymal transition (EMT) in colon cancer cells (50, 102). ZNF281 can also promote differentiation of osteoblasts and neuronal cells but it inhibits the differentiation of muscle cells promoted by miR-1 (50, 103). In RMS patients, where miR-1 expression is low, ZNF281 transcript and protein expression levels are significantly high compared to their normal skeletal and smooth muscle tissue counterparts. It was therefore suggested that ZNF281 can be considered a marker of proliferative and dedifferentiation state of RMS and that its expression is partly regulated by miR-1 (50).
Generally, miR-206 seems to affect pathways that regulate RMS development, including the RAS/MAPK and NFκB signaling pathways as well as regulating myogenic transcription factors and epigenetic regulators (47). High miR-206 expression in FN-RMS was associated with a downregulation in interleukin-4 (IL-4) expression levels (52). IL-4 promoted RMS survival and proliferation while inhibiting MyoG expression (56).
MiR-206 targets also include HDAC4, a histone deacetylase enzyme that epigenetically inhibits gene expression by modulating the acetylation status of lysine residues on histone proteins thereby modifying chromatin structure (57, 104, 105). HDAC4 cellular localization has been shown to be important in regulating muscle differentiation (106). Oncomine database analysis shows its enrichment in RMS tissue compared to normal tissue and other sarcoma types (58, 107). It is mainly localized within the nucleus, in part due to Heme Oxygenase-1 (HO-1), which is an oxidative stress-response factor that promotes RMS progression by elevating downstream HGF and SDF-1 dependent pathways which stimulate myoblasts proliferation and inhibit their differentiation (58). RMS cells with elevated HO-1 produce less reactive oxygen species resulting in miR-206 repression. HO-1 inhibition, on the other hand, inhibits growth of RMS tumors by enhancing miR-206-mediated myogenic differentiation (58). HO-1 inhibition in RMS cells, promotes removal of HDAC4 from the nucleus, resulting in an upregulation of miR-206 and enhanced differentiation further revealing the tumor-suppressive effect of miR-206 in RMS (58).
MiR-133
MiR-133 has been reported to impact myogenic differentiation but whether it promotes or inhibits differentiation is still a matter of debate and seems to be tissue-specific (83). To begin with, miR-133 was found to promote myoblast proliferation and acts conversely to miR-1 by inhibiting myocyte differentiation (108). In contrast, other studies showed that miR-133 members, miR-133a and miR-133b, inhibit myoblast proliferation while promoting myogenic differentiation in C2C12 cells by inhibiting ERK signaling (109, 110).
In both ERMS and ARMS, miR-133a levels, as those of miR-1, are reduced while their targets mRNA are upregulated (60). Furthermore, the reintroduction of either miRNA mimics into ERMS RD cells line appears to inhibit RMS growth. This was accompanied with a downregulation of several genes, all of which had isoforms that are highly expressed in striated skeletal and cardiac muscle. Additionally, the list of the top 50-downregulated targets for both miR-1 and miR-133a included the epigenetic regulators HDAC4 and SMARCD1 (60). The latter has been shown to regulate important players in muscle differentiation (111, 112). However, while ectopic expression of miR-1 promoted the expression of myogenic markers indicating a role in promoting myoblast differentiation, miR-133a did not (60). These effects observed in FN-ERMS were not as evident in FP-RMS cells whereby ectopic expression of miR-1/133a in Rh30 cells, a FP-RMS cell line, did not inhibit cell growth. The reason behind this has yet to be determined but may be due to the substantial levels of these myomiR in FP-RMS compared to FN-RMS or due to the presence of the fusion oncogene PAX3-FOXO1 which may escape the miR-1/133a-mediated inhibition of cell growth.
Altogether, myo-miRs appear to be mostly tumor suppressive and act mainly by regulating myogenesis and promoting muscle cell differentiation. Their downregulation in RMS compared to normal skeletal muscle suggests their potential utilization in pro-differentiation therapy.
Non myo-miRNA implicated in RMS
MiR-28-3p and miR-193
In the context of SNAIL regulation of miRNA expression, analysis of the miRNA transcriptome in Rh30 cells revealed that SNAIL silencing can modulate miRNA levels (113). For example, SNAIL inhibition led to a downregulation of miR-28-3p and miR-193a-3p expression levels suggesting that SNAIL, which is upregulated in RMS tumors, leads to a downregulation of tumor-suppressive miRNA (113). Overexpression of these miRNA in Rh30 cells led to a potent inhibition of cell migration and a decrease in cell adhesion to endothelial cells (61). On another note, miR-193 family members act as tumor suppressors in many tumor types including colon cancer and osteosarcoma (114, 115). In another study, sixty myogenesis-related differentially expressed miRNA were identified in cells isolated from skeletal muscle biopsies, among which were miR-28-3p and miR-193a-5p that were shown to act as regulators of both ERMS and ARMS development and progression by downregulating proliferation, migration, invasion and contributing to cell cycle arrest (62). They were also able to induce the expression of myogenic transcription factors thereby promoting differentiation of RMS cells and myoblasts. Simultaneous overexpression of both miRNA diminished tumor growth in an in vivo model of RMS. Interestingly, miR-193a-5p overexpression in ARMS cells resulted in an upregulation of the myogenic-related miR-206 expression (62). Furthermore, miR-28-3p was found to indirectly downregulate EZR/ezrin in RMS, a key regulator of tumor metastasis (61, 113).
MiR-29
MiR-29 family of miRNA, which includes miR-29a, miR-29b and miR-29c, while not specific to muscle tissue, is highly implicated in myogenesis (57, 63). They are all significantly downregulated in both FP-RMS and FN-RMS where they have been regarded as tumor-suppressive by promoting myogenic differentiation (31). MiR-29 expression levels are downregulated in RMS cells due to an upregulation in the NFκB-YY1 pathway which epigenetically silences miR-29 expression and blocks myoblast differentiation both in vitro and in vivo (63). NFκB enhances YY1 expression in RMS which then interacts with enhancer of Zeste homolog 2 (EZH2), a methyltransferase enzyme. This interaction downregulates mir-29 family members and inhibits myogenic differentiation (63). On the other hand, reconstitution of mir-29 exerts a negative feedback on YY1, inhibits RMS growth and induces myogenic differentiation in an RMS xenograft mouse model (63). Furthermore, inducing mir-29b expression resulted in elevated levels of differentiation markers in RMS cells and a phenotypic shift from a round to an elongated appearance (63). Additionally, overexpression of mir-29 using mimics in RMS cells inhibited proliferation, migration and invasion (64).
Moreover, in human RMS tumors, miR-29 expression levels were inversely correlated to those of cell cycle regulators CCND2 and E2F7 (31). Indeed, in transfected RMS cells, ectopic miR-29 expression did not only downregulate the aforementioned cell cycle regulators but also activated the expression of myogenic differentiation genes including MyoG and alpha-actin compared to non-transfected control. This was accompanied by an inhibition of proliferation of ERMS cells. In FP-ARMS cells, miR-29-mediated inhibition of proliferation was less prominent compared to FN-ERMS cells (31). Of note, while ectopic expression of all miR-29 members downregulated CCND2 transcript levels, individual miR-29 family members exhibited cell-line specific regulation of CCDN2 protein levels. For example, miR-29a downregulated CCND2 protein levels in ERMS JR1 cells only. Similarly, all miR-29 family members were able to directly target 3’UTR of E2F7 in HEK293 cells, but its levels were decreased upon ectopic expression of miR-29a in FN-ERMS JR1 cells and FP-ARMS Rh30 cells while there was no effect on E2F7 expression in FN-ERMS RD cells. Similarly, for E2F7 protein levels, they were significantly downregulated in both JR1 and Rh30 cells by miR-29a, but no effect was observed in RD cells (31). Altogether, this indicated regulation of CCDN2 and E2F7 gene expression and protein levels by miR-29 family members in a cell-line specific manner. In the C2C12 mouse myogenic cell line, both mir-29 and mir-206 were demonstrated to not only inhibit HDAC4 by directly targeting the 3’UTR of its transcript, but also inhibit the TGF-β-mediated upregulation of HDAC4 by targeting Smad3, a transducer of TGF-β signaling (57). Both TGF-β and Smad3 have been characterized as potent inhibitors of myogenic differentiation, and HDAC4 suppresses the activity of myogenic transcription factor MEF2 (57, 116–118). As such, these miRNA act against the inhibitory effect of TGF-β on myogenic differentiation. Furthermore, RMS cells express high levels of TGF-β and Smad4 associated with a downregulation in miR-29 and miR-206 levels which further supports the idea that these miRNA promote muscle cell differentiation by suppressing TGF-β signaling (57).
Another mechanism by which miR-29 exerts tumor suppressive effects in RMS is through targeting guanine nucleotide exchange factor T mRNA (ARHGEF25/GEFT), whose overexpression correlates with poorer outcomes (65). GEFT was shown to promote RMS cell survival and proliferation, migration, invasion and EMT thereby enhancing RMS metastatic propensity (64, 119). GEFT was verified as miR-29 target and its inhibition suppresses RMS proliferation and motility (64).
MiR-450b-5p
Comprehensive microarray analysis on RMS cell lines and tissues identified a novel set of TGF-β1-related miRNA including miR-450b-5p as significantly expressed in TGF-β1 knocked-down RMS cells and TGF-β1-low-expression RMS tissues relative to control (66). In addition to its role in inhibiting myogenic differentiation, TGF-β1 is a known promoter of tumor cell metastasis and invasion in a variety of cancer types by modulating miRNA content (120). In RMS, TGF-β1 functions by suppressing miR-450b-5p, whereas miR-450b-5p significantly inhibits RMS growth and promotes the upregulation of MyoD in vitro and in vivo (66). Moreover, bioinformatics analysis revealed ecto-NOX disulfide-thiol exchanger 2 (ENOX2) and PAX9 as candidate mRNA targets of miR-450-5p. Their expression inversely correlated with that of miR-450-5p in RMS cells, and their knockdown promoted MyoD expression as well. Altogether, TGF-β1 inhibits miR-450-5p in RMS which is associated with an upregulation in its downstream targets and repression of myogenic differentiation (66).
MiR-221
MiR-221 is a tumor suppressive miRNA that is negatively regulated by PAX3-FOXO1 in FP-RMS (67). It functions partially through targeting CCND2, CDK6, and ERBB3 mRNA. Its ectopic overexpression in FP-RMS induced a pro-apoptotic effect, reduced cell viability, migration and invasion in FP-RMS (67).
MiR-7 and miR-324-5p
The overexpression of miR-7 and miR-324-5p inhibited cell proliferation and reduced tumor growth and metastasis in an in vivo RMS model (68). Additionally, miR-7 alone contributed to a decrease in cell invasion in vitro. Both miRNA have been described as tumor suppressive in several types of cancer and are regulators of alpha-9 integrin mRNA (ITGA9) in RMS (68, 121–124). Integrins mediate cell adhesion to the extracellular matrix thereby generating bidirectional signals mainly mediated by adapter proteins (125). ITGA9 has been shown to contribute to disease aggressiveness and metastasis in cancer (126). Furthermore, its inhibition is able to reduce motility and invasiveness in RMS cells (127).
MiR-378-3p
MiR-378 family can target insulin-like growth factor receptor 1 (IGF1R) mRNA which is upregulated in both FN-RMS and FP-RMS and whose deregulated expression is associated with muscle diseases (69, 128). Ectopic overexpression of miR-378a-3p in Rh30 and RD cells suppressed IGF1R expression and downregulated IGF1R/AKT signaling (69). On a functional level, this was associated with a decrease in migration of RMS cells, an increase in apoptosis and cell cycle arrest and enhanced differentiation. Specifically, upregulation of miR-378a-3p in RMS cells using miRNA mimics led to a slight increase in muscle differentiation markers MyoD1 and MyHC, and a downregulation of myogenic-repressor MyoR and early-myogenic factor Myf5. More organized actin filaments were observed in miR-378a-3p-transfected cells resembling differentiated skeletal muscle. Altogether, miR-378-3p was able to modulate myogenic regulatory factors thereby promoting RMS differentiation. With that, treatment of RMS cells with 5-aza-2′-deoxycytidine was able to induce apoptosis, cell arrest at G2 phase and a decrease in migration associated with an upregulation in miR-378-3p levels (69).
MiR-26a and miR-30b/30c
Comparing circulating serum levels of known tumor suppressive miRNA in RMS patients to healthy donors, miR-26a and miR-30b/30c were found lower in RMS patients (59). Furthermore, low levels of circulating miR-26a significantly correlated with progressive disease but this requires further study to determine whether circulating miR-26a could act as a biomarker in RMS patients (59).
MiR-181a/212
A promyogenic miRNA cocktail (PMC) can contribute to myogenic epigenetic memory thereby influencing cell fate (70, 129). Treatment of murine FN-RMS with selected PMC increased expression of MyoG, a key transcription factor that promotes myogenic differentiation (130). In silico analysis identified miR-181a/212 as the key combination required for the observed pro-myogenic effect (70). In vitro studies showed that this combination increases the fusion index of MyHC-positive myotubes in FN-RMS cells while reducing their migration and proliferation capacity. Moreover, in a syngeneic murine transplant model of FN-RMS cell line pretreated with this selected miRNA cocktail in order to demonstrate whether treatment with PMC can promote myogenic commitment of FN-RMS and reduce its tumorigenicity, the mice injected with miRNA-pretreated cells showed a reduction in tumor size indicating a decreased proliferation of FN-RMS cells, and an improvement in functional activity of the mice (70).
MiR-214
Evidence such as the downregulated levels of miR-214 in RMS cells and the inhibition of tumor growth mediated by its ectopic expression in RD cells, revealed that miR-214 is a tumor suppressive miRNA in RMS (71). Its downregulation is accompanied with an upregulation in N-Ras protein and transcript levels in murine embryonic fibroblasts, which acts as a proto-oncogene promoting tumor progression, and is demonstrated to be a target of miR-214. On the other hand, its ectopic expression was also able to induce differentiation and apoptosis while inhibiting colony formation and growth of xenografts in vivo (71).
MiR-27a
MiR-27a is another tumor suppressive miRNA in RMS that can target PAX3-FOXO1 by binding to PAX3 mRNA as evidenced in mouse and human cells and can target both PAX3 and PAX7 mRNA in muscle cells (72). Indeed, pharmacological inhibition of HDAC3 decreased the activity of the chromatin remodeling enzyme SMARCA4 which is a chromatin remodeling enzyme. In turn, pharmacological or siRNA-mediated inhibition of HDAC3 or SMARCA4 promoted miR-27a expression. Re-expression of the latter decreased PAX3-FOXO1 in ARMS cells and reduced tumor growth, suggesting that miR-27a is tumor suppressive and part of the HDAC3-SMARCA4-miR27a axis (72).
While the above miRNA are tumor-suppressive whose dysregulated expression in RMS cells contributes to tumor growth and progression, miRNA discussed in the section below have been shown to promote RMS progression by regulating either tumor growth or motility or by correlating with advanced stage and poor prognosis in RMS patients, thereby acting as oncomiR.
OncomiR implicated in RMS
Certain miRNA contribute to RMS metastasis, and can distinguish between FP-RMS and FN-RMS, raising their potential use as biomarkers of disease aggressiveness and possibly targets in RMS treatment strategies.
MiR-27a
Analysis of the miRNA expression profile of FP-RMS and FN-RMS cell lines revealed 16 miRNA whose expression discriminates between FP and FN cell lines (73). One such miRNA is miR-27a which is upregulated in the more aggressive FP-RMS cell lines. miR-27a promotes proliferation and tumor progression in a variety of cancer types, suggesting an oncogenic role (131–136). Specifically, miR-27a was shown to promote tumor cell proliferation in RMS which was attributed to its role in targeting the retinoic acid receptors: retinoic acid alpha receptor (RARA) and retinoic X receptor alpha (RXRA) (73).
MiR-183
miR-183 is significantly overexpressed in RMS where it can specifically target EGR1 mRNA as validated in vitro (74). Its knockdown in different cancer cell lines, including RMS, leads to a deregulation of miR-183–EGR1–PTEN network by upregulating EGR1 and PTEN mRNA levels, as well as EGR1 protein levels, both of which are tumor suppressive. While not studied in RMS particularly, miR-183 knockdown resulted in reduced cell migration through an EGR1-based mechanism. Therefore, miR-183 could be oncogenic in RMS, contributing to enhanced migration which is yet to be elucidated (74).
MiR-9-5p
Another oncogenic miRNA in RMS is miR-9-5p, which has been associated with metastasis (75). In fact, miR-9-5p levels correlated with poor outcome, enhanced migration and metastasis in RMS patients. Particularly, its level of expression was modulated by the fusion oncoprotein PAX3-FOXO1 whereby reduction in the expression of the latter led to a decrease in miR-9-5p expression. This was attributed to PAX3-FOXO1-mediated regulation of its transcriptional target MYCN expression which in turn modulates that of miR-9-5p (75, 76).
MiR-223
In both adolescent and young adult RMS patients, miR-223 was overexpressed and associated with an upregulation of both EMT and inflammation in an age-related manner, possibly contributing to RMS aggressiveness (77).
MiR-17-92 cluster
Certain cases of ARMS, mostly FP-RMS, exhibit an amplification in a chromosomal region containing the miR-17-92 cluster encoding several miRNA including miR-17, miR-19a/b, miR-20a, and miR-92 (78). While the majority of these cases were associated with an elevated expression of the miRNA, this upregulation was also found, to a lesser extent, in the absence of the chromosomal amplification. The upregulation of these miRNA correlated to poorer outcomes relative to non-amplified cases in neuroblastoma and its correlation with survival outcomes in RMS remains to be studied (137).
MiR 130a/b
In a recent study, RNA-seq analysis for RMS of the head and neck revealed that PPARG, a critical gene in the PPAR-signaling pathway was downregulated and that miR-130a/b was highly expressed compared to normal tissue; this was further confirmed by western blot and qRT-PCR analysis, respectively (80). Bioinformatics analysis using the TARGET database revealed an interaction between miR-130a/b and PPARG and a negative correlation between their expression levels. This was confirmed in vitro in RD cells using antagomir to downregulate miR-130a/b levels which was associated with a significant reduction in RD cell proliferation. Treating RD cells with rosiglitazone maleate, a PPARG agonist, reduced proliferation and the combination of miR-130a/b antagomirs with rosiglitazone maleate significantly suppressed proliferation compared to RD cells treated with the agonist alone (80). Altogether, this indicates that miR-130a/b promotes RMS cell proliferation by targeting PPARG expression which requires further investigation.
Exosomal miRNA
MiRNA enrichment within exosomes and their subsequent delivery into recipient cells suggests possible roles in modulating the tumor microenvironment to promote tumor growth. Exosomes are small extracellular vesicles which carry and deliver nucleic acid including non-coding RNA and protein cargos to recipient cells. They have been implicated in cancer progression in variety of tumor types where they essentially enhance recipient cell migration, invasion, anchorage-independent growth, and promote angiogenesis and immunomodulation (79, 138–142). miRNA enriched in ERMS-derived exosomes were represented in pathways implicated in cancer cell cycle biology, while those enriched in ARMS-derived exosomes targeted proteins implicated in cancer biology, metastasis, and stemness (138). Of note, while certain miRNA are enriched in exosomes consistent with their enrichment in the donor cells, others are only enriched in exosomes revealing selective sorting of these miRNA which may be independent of their level of expression in the donor cells (138, 143). Furthermore, 2 miRNA, miR-1246 and miR-1268, were commonly enriched in exosomes of all studied RMS cell lines. Bioinformatic pathway analysis revealed that they are related to tumorigenesis through regulating pathways including Wnt pathway, EGFR pathway, angiogenesis and apoptosis (138).
MiR-486-5p is implicated in several cancers where it either acts as a tumor suppressor or an oncomiR depending on tumor type and cellular context (67, 144–146). In RMS, miR-486-5p is enriched in both cells and exosomes, more particularly in FP-RMS (67). PAX3-FOXO1 upregulates the transcription of miR-486-5p which then promotes FP-RMS proliferation, colony formation and invasion. In fact, miR-486-5p inhibition reduced FP-RMS cell survival and decreased tumor growth in xenografted mice (67). Upregulating the expression of mir-486-5p in C2C12-derived exosomes, by transfecting C2C12 mouse myoblasts with either mir-486-5p or PAX3-FOXO1 expressing vectors, enhanced recipient fibroblast proliferation, migration, invasion and anchorage-independent growth (79). Additionally, analysis of a small series of human serum samples showed that miR-486-5p seems to be enriched in exosomes of RMS patients (79). However, specific effectors and downstream targets of miR-486-5p in RMS are yet to be determined.
Other oncomiRNA
Utilization of the NanoString digital profiling technology in different prognostic groups of ERMS and spindle-cell sclerosing RMS (SRMS) patients revealed significant upregulation of either oncomiR or tumor suppressive miRNA (147). Of note, SRMS is biologically heterogeneous and like ERMS, it harbors a variety of genetic mutations that can result in different clinical outcomes. This study revealed differential expression of miRNA between the different prognostic groups. For example, miR-612, miR-3144-3p, miR-548y, miR-302d-3p, miR-421, miR-548y and miR-548ar-5p were significantly overexpressed in tumors with adverse/poor prognosis compared to those with favorable prognosis (147). These may be interesting miRNA players in RMS development and progression, but their specific roles in RMS have not been determined.
Therefore, oncomiR are important players in RMS progression and a better understanding of their roles and targets in RMS could pave the way for future treatment strategies that target these miRNAs or utilizes their expression levels for monitoring disease progression and treatment success.
Long non-coding RNA implicated in RMS
LncRNA have been shown to play a role in regulating skeletal muscle differentiation. For instance, SYISL (SYNPO2-intron sense-overlapping), an abundant and intron-encoded lncRNA, was identified in muscle where it promotes myoblast proliferation and fusion (148) (Figure 3). However, it was demonstrated to inhibit myogenic differentiation. It acts by indirectly inhibiting MyoG, muscle creatine kinase (MCK), and myosin heavy chain 4 (Myh4) through the recruitment of EZH2 to their promoters. In fact, studies have shown that EZH2 interacts with various regulatory lncRNA and plays important roles in myogenesis (149). Similarly, lncRNA Neat1 was shown to promote myogenic C2C12 division but inhibit their differentiation and fusion by recruiting Ezh2 to p21 and to muscle-specific Myog and Myh4 genes promoters (150) (Figure 3). Additionally, some lncRNA act as precursors of miRNA involved in myogenic differentiation. For example, the lncRNA Linc-MD1 generates miR-206 and miR-133b indicating a role in muscle differentiation and a possible implication in RMS differentiation (41) (Figure 3). While these findings are general to skeletal muscle, determining the role of these lncRNA in RMS biology is worthy of examination.
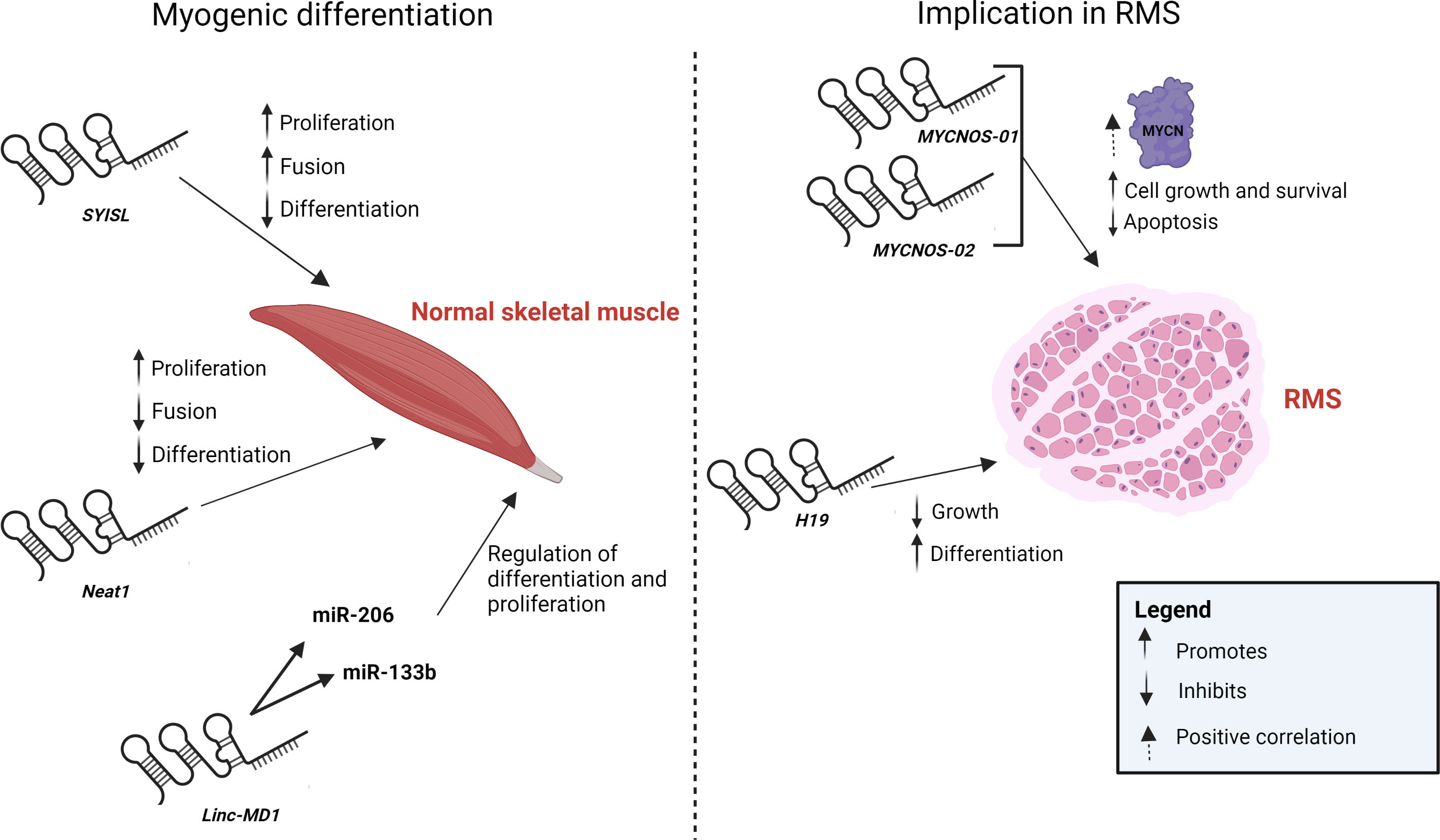
Figure 3 LncRNA implication in skeletal muscle myogenesis and RMS. Schematic diagram of lncRNA SYISL, Neat1 and Linc-MD1 implicated in skeletal muscle differentiation and proliferation (left panel) and lncRNA MYCNOS-01, MYCNOS-02 and H19 implicated in RMS proliferation biology (right panel). Created with BioRender.com.
In RMS, overexpression of the oncogene MYCN contributes to cell growth in ARMS where its transcription is driven by PAX3-FOXO1 (17, 76). The lncRNA MYCNOS-01 and MYCNOS-02 on the antisense strand of MYCN were shown to upregulate MYCN protein levels in RMS (Figure 3). However, MYCNOS-01 did not affect mRNA levels and was presumed either to be acting as a cis-antisense lncRNA on its sense partner MYCN, or it may be interacting with a miRNA that targets MYCN thereby upregulating MYCN protein expression levels (17). In all cases, regulation of MYCN via MYCNOS-01, whose levels are upregulated in RMS cells harboring MYCN genomic amplification, was found to play a role in cell growth whereby silencing MYCNOS-01 resulted in a reduction of MYCN protein levels in RMS cells, that in turn, specifically reduced cell viability of RMS cells having amplified MYCN expression (17). Similarly, a significant correlation exists between levels of MYCNOS-02 and MYCN expression in the studied RMS samples and cell lines, however, knockdown of MYCNOS-02 did not have consistent effects on MYCN expression. Decreasing MYCNOS-02 expression, on the other hand, significantly downregulated cell survival but only in RMS cell lines with amplified MYCN expression, while promoting apoptosis. Altogether, the positive regulation of MYCN by MYCNOS-01 and MYCNOS-02 likely contributes to RMS cell growth (17).
H19 lncRNA was among the first lncRNA whose function was described in various biological contexts. Expression of the H19 gene is significantly suppressed in RMS tissue, and to a higher extent in ERMS, compared to normal muscle (151) (Figure 3). It is characterized by parental imprinting and a preferential loss of the maternal allele in RMS tissue and, as such, a loss-of-function which likely contributes to RMS development. H19 has dual roles in cancer where it can promote tumorigenesis but can also act as a tumor suppressive lncRNA through different mechanisms of action (152–155). It is a precursor of miR-675, a miRNA embedded within its first exon, where its reactivation in RMS cells was shown to aid in the inhibition of RMS growth by upregulating miR-675 expression and promoting muscle cell differentiation (156, 157).
Circular RNA implicated in RMS
Some circRNA were shown to regulate myoblast differentiation. For instance, circLMO7 possesses the functional ability to act as a competing endogenous RNA for miR-378a-3p, which is involved in bovine muscle development; its overexpression stimulates proliferation and inhibits differentiation of primary bovine myoblasts (42). On the other hand, circFUT10 promotes myoblast differentiation but reduces their proliferation rate (158). This suggests that circRNA could be interesting players in myogenesis and in RMS differentiation which requires investigation.
Two circRNA have been described to be implicated in RMS (Figure 4). Circ-ZNF609 has been shown to be upregulated in both ERMS and ARMS human biopsies (18). Its knockdown in the ERMS-derived cell line, but not in ARMS, induced cell cycle arrest at the G1/S transition, resulting in a strong reduction in p-Akt and pRb phosphorylation levels (18). This reveals a potential role for circ-ZNF609 in promoting RMS growth. Of note, high levels of circ-ZNF609 have been associated with tumor progression and poor prognosis in breast cancer (159). It was also shown to regulate myoblast proliferation (160). Its mouse homolog has been shown to inhibit myogenic differentiation by sponging miR-194-5p (161).
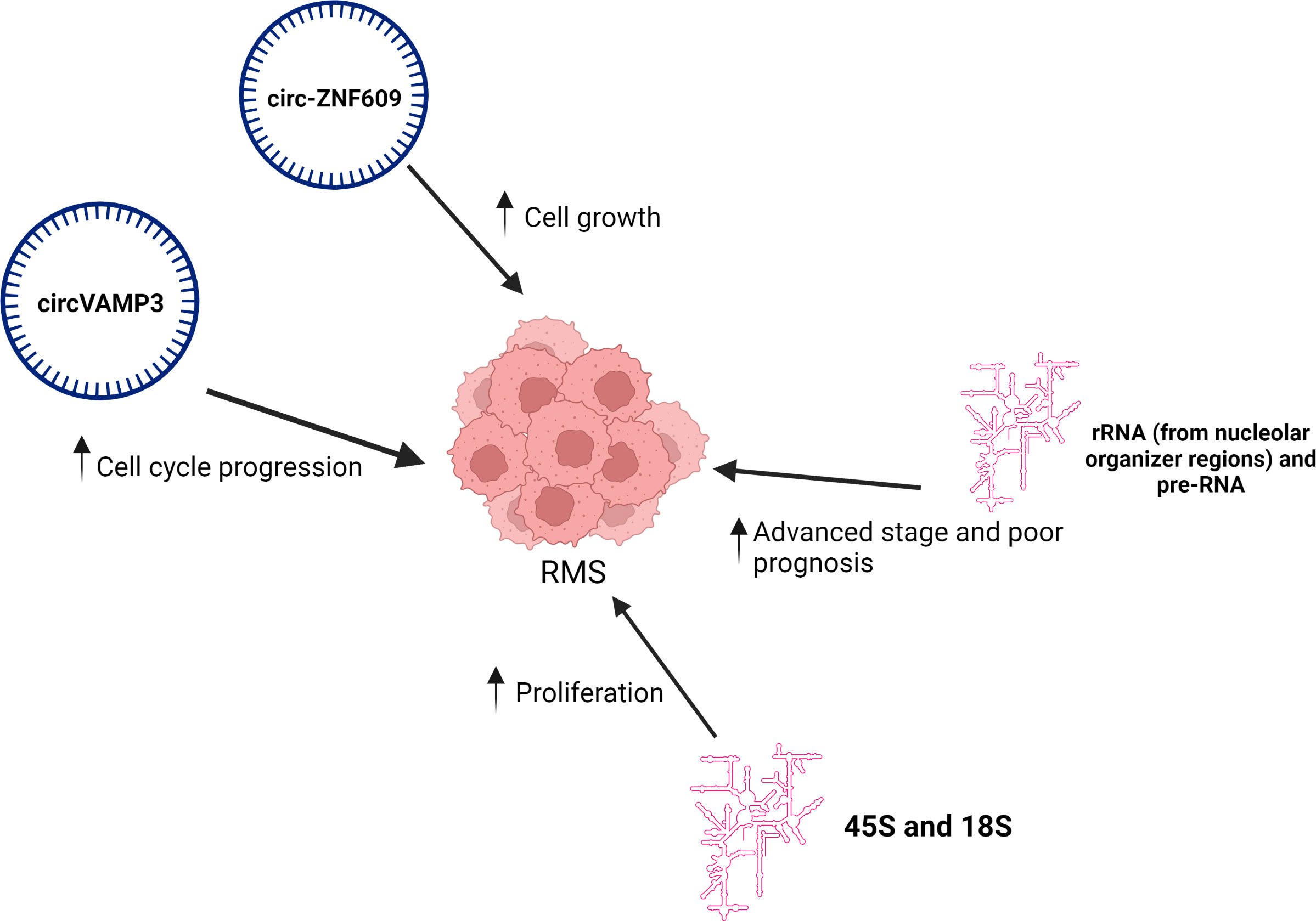
Figure 4 CircRNA and rRNA implicated in RMS biology. Schematic representation of circ-ZNF609 and circVAMP3 upregulating RMS cell growth and proliferation, respectively; rRNA from nucleolar organizer regions and pre-RNA correlated with advanced stage and poor prognosis of RMS; as well as ribosomal subunits 45S and 18S promoting RMS proliferation. Created with BioRender.com.
Another circRNA, circVAMP3, is significantly overexpressed in ARMS Rh4 cells compared to both normal myoblasts and the ERMS RD cell line (162). It was detected as both the circular and linear isoforms with the former being particularly more interesting to study in Rh4 cells because its upregulation was more prominent. Its depletion by siRNA technology, by targeting a unique site that distinguishes it from its linear counterpart, in the ARMS cell line impaired cell cycle progression leading to a small but significant increase in the percentage of cells in G2. CircVAMP3 knockdown was shown to act through the alteration of the AKT-related pathway, and several AKT-pathway related genes were significantly deregulated such as AKT1 levels that were downregulated in siRNA-treated (si-circVAMP3) Rh4 cells compared to those treated with scrambled control. Furthermore, levels of the downstream inhibitors of the G2/M transition, the WEE1 and CDKN1A factors increased upon circVAMP3 knock-down (162).
Ribosomal RNA implicated in RMS
An amplification of rRNA synthesis has been identified as a hallmark of cancer cell growth (163). While studies are still limited, certain rRNA have been implicated in RMS biology (Figure 3). A multifunctional protein and an indirect inhibitor of skeletal muscle differentiation, known as Prohibitin 2 (PHB2) was found to play an important role in ERMS RD cell line progression (19, 164). One of its methods of action was the regulation of rRNA; this was evidenced by the down-regulation of 45S and 18S rRNA synthesis following PHB2 knockdown. The exact mechanism behind this was a decrease in c-Myc occupancy at the ribosomal DNA (rDNA) promoter and an increase in MyoD molecules that were bound to it instead; altogether indicating that c-Myc-mediated rRNA synthesis is essential for ERMS cell proliferation (19). Moreover, comparative expressed sequence hybridization (CESH) suggested an increase in rRNA synthesized from the nucleolar organizer regions located on acrocentric chromosomes in RMS samples (165). This, in turn, correlated with poor prognosis, specifically in ARMS. In addition, pre-rRNA expression significantly correlated with tumor stage indicating that pre-rRNA could serve as a useful prognostic marker in ARMS.
Therapeutic strategies and future implications
Extensive research has demonstrated the role of ncRNA in regulating myoblast differentiation and proliferation. In this review, we discussed how the dysregulated expression of ncRNA is associated with the incomplete myogenic differentiation that characterizes RMS cells as well as their enhanced proliferation and metastatic propensity. This indicates a potential use of ncRNA either in disease diagnosis and prognosis, as pro-differentiation agents, or as therapeutic targets in RMS treatment. In fact, to date, several ncRNA therapeutics in cancer and other diseases including the use of anti-miR and siRNA, have entered phase II or III of clinical development, though not yet in RMS (166). For example, Miravirsen is an anti-miR-122 that has entered phase II clinical trials against Hepatitis C viral infection and the antisense oligonucleotide Prexigebersen has also entered phase II clinical trials in acute and chronic myeloid leukemia patients (166).
Clinical trials investigating miRNA as biomarkers include those that monitor miRNA levels in patients receiving FDA-approved drug treatment. The advantage of using miRNA as biomarkers is their ubiquitous expression in different body fluids including serum and saliva (167). Differentially expressed miRNA between FP-RMS and FN-RMS may serve as biomarkers that distinguish between the two subtypes, as well as for prognostication. For example, low miR-206 expression correlated with poor overall survival in metastatic RMS cases lacking the PAX3-FOXO1 fusion protein (52). Their circulation and detection in body fluids is especially important in allowing their use in monitoring disease progression and treatment success. We mention, for example, how comparing serum levels of circulating miR-26a and miR-30b/30c distinguishes between RMS patients and healthy donors as these miRNA are detected at lower levels in RMS patients (59). This indicates that these miRNA have a potential role in patient diagnosis. In fact, studies suggest that ncRNA, particularly the expression signature of several ncRNA seems to be promising in cancer diagnosis and prognosis (168).
Pro-differentiation therapy has been clinically established in cancer and the role of myomiR in promoting skeletal myoblast differentiation suggests that they may be essential players in this kind of therapy (60, 169). Ectopic expression of cell-type specific miRNA can shift the mRNA expression profile to one resembling the tissue or cell types in which the miRNA are originally enriched (51, 170). For example, pre-miR-1 and pre-miR-206 induced myogenic differentiation and switched the mRNA expression profile in ERMS (RD18) and ARMS (RH4) mice xenografts into one resembling mature skeletal muscle cell (51). One way to enhance the expression of a certain miRNA is through the use of miRNA mimics, synthetic versions of endogenous miRNA that can restore the regulation of gene expression (171). Hanna et al., demonstrated that the knockdown of a single or a subset of miR-206 targets was insufficient in promoting differentiation of RMS cells compared to the treatment with miR-206 mimics which can both promote differentiation and cell cycle exit suggesting that because a miRNA can have hundreds of targets, miRNA replacement therapy may be more valuable than therapies that target single molecules or pathways (55). Interestingly, expression of miRNA may be dysregulated in cancer due to aberrant epigenetic events including global hypermethylation observed in cancer cells (172). With that, studies have shown that hypomethylating agents, in addition to histone deacytelase inhibitors, can activate miRNA expression in different types of cancer. For example, treatment of bladder cancer cells with the prodrug 5-aza-2- deoxycytidine (Decitabine), a methyltransferase inhibitor/hypomethylating agent, and 4-phenylbutyrate induced high expression of miR-127 with tumor suppressive role in bladder cancer cells (172).
Small molecules comprise another approach to regulate ncRNA. The advantages of small molecules in RNA therapeutics are their low molecular weights, oral administration, and permeability that allows them to easily pass the cell membrane (173). Their synthesis is also more cost-effective compared to synthetic oligos. They can act by interacting with proteins involved in ncRNA synthesis thereby regulating ncRNA expression (173). For example, enoxacin is a small molecule identified to enhance miR-125a expression by acting at the level of pre- and pri-miRNA (174). However, in addition to problems with delivery and efficacy, the fact that miRNA influence the expression of thousands of genes may also pose a problem due to possible off-target effects. Therefore, the use of miRNA to promote myogenic differentiation requires extensive understanding of their mechanisms of action in regulating myogenesis and ensuring that their ectopic expression does not exceed physiological levels (169, 175).
Targeting ncRNA expression, on the other hand, involves RNA interference (RNAi) therapy which includes the inhibition of oncomiR by utilizing synthetic anti-miR also known as blockmir or antagomir. These bind to complimentary miRNA and inhibit their activity or mask the binding site of certain miRNA targets, respectively (171). Therefore, targeting oncomiR in RMS may help to reduce proliferation, migration and/or invasion. Small molecule inhibitors can also be used to target miRNA expression. For example, Targaprimir-96 is a miRNA-processing inhibitor which can bind to pri-miR-96 and inhibit its processing into mature oncogenic miR-96 (176). However, the best miRNA targets for RMS treatment have to be identified and one method is through collection of biopsy samples from a large number of patients and at different stages of disease progression to determine key miRNA and ideal targets for treatment (177). CircRNA therapeutics also includes regulating circRNA expression through their targeting, for example by the use of gold nanoparticles conjugated with siRNA, or through overexpression of tumor-suppressive circRNA (178). This has shown to downregulate cancer progression and enhance response to chemotherapeutic treatment. LncRNA targeting includes inhibition of lncRNA transcription, modulation of genomic loci that encode lncRNA or post-transcriptional inhibition (166). These strategies have yet to be explored in RMS biology.
Altogether, correction of dysregulated ncRNA could be a promising intervention that can be adjuvant to existing standard treatment strategies against RMS which is needed to enhance survival rates, but the efficient delivery and ensuring safety of the drugs that target ncRNA should be improved and studies are needed to establish their efficacy in RMS.
Conclusion
miRNA and other ncRNA are important players in RMS tumorigenesis. The specific role of miRNA in muscle growth and differentiation whereby dysregulation, even in small amounts, in their expression can determine muscle differentiation reveals important mechanistic roles of these miRNA in RMS biology. We have summarized the evidence to date showing that the dysregulation of miRNA is crucial in RMS and how these miRNA may serve in important prognostic roles and in ncRNA-based therapeutic strategies in RMS patients. However, sufficient studies that include large patient cohorts, RMS serum specimens, and clinical samples revealing the diagnostic and prognostic potential of miRNA in RMS are still lacking. Furthermore, lncRNA, circRNA and rRNA are emerging key players in cancer growth and progression, but these are largely understudied in RMS biology thereby necessitating further investigation.
Author contributions
FR, RS and SG drafted and refined the manuscript. NH, PC and PAC reviewed and critically read the manuscript. All authors contributed and approved the submitted version.
Funding
This work was funded by a grant from the Lebanese University, a grant from Ligue Contre le Cancer (Rhone) and a grant from Aix-Marseille University.
Conflict of interest
The authors declare that the research was conducted in the absence of any commercial or financial relationships that could be construed as a potential conflict of interest.
Publisher’s note
All claims expressed in this article are solely those of the authors and do not necessarily represent those of their affiliated organizations, or those of the publisher, the editors and the reviewers. Any product that may be evaluated in this article, or claim that may be made by its manufacturer, is not guaranteed or endorsed by the publisher.
References
1. Rudzinski ER, Anderson JR, Hawkins DS, Skapek SX, Parham DM, Teot LA. The world health organization classification of skeletal muscle tumors in pediatric rhabdomyosarcoma a report from the children’s oncology group. Arch Pathol Lab Med (2015) 139:1281–7. doi: 10.5858/arpa.2014-0475-OA
2. Parham DM, Barr FG. Classification of rhabdomyosarcoma and its molecular basis. Adv Anat Pathol (2013) 20:387–97. doi: 10.1097/PAP.0b013e3182a92d0d
3. Bennicelli JL, Edwards RH, Barr FG. Mechanism for transcriptional gain of function resulting from chromosomal translocation in alveolar rhabdomyosarcoma. Proc Natl Acad Sci U.S.A. (1996) 93:5455–9. doi: 10.1073/pnas.93.11.5455
4. Bennicelli JL, Advani S, Schäfer BW, Barr FG. PAX3 and PAX7 exhibit conserved cis-acting transcription repression domains and utilize a common gain of function mechanism in alveolar rhabdomyosarcoma. Oncogene (1999) 18:4348–56. doi: 10.1038/sj.onc.1202812
5. Barr FG. Gene fusions involving PAX and FOX family members in alveolar rhabdomyosarcoma. Oncogene (2001) 20:5736–46. doi: 10.1038/sj.onc.1204599
6. Linardic CM. PAX3-FOXO1 fusion gene in rhabdomyosarcoma. Cancer Lett (2008) 270:10–8. doi: 10.1016/j.canlet.2008.03.035
7. Anderson J, Ramsay A, Gould S, Pritchard-Jones K. PAX3-FKHR induces morphological change and enhances cellular proliferation and invasion in rhabdomyosarcoma. Am J Pathol (2001) 159:1089–96. doi: 10.1016/S0002-9440(10)61784-1
8. Rosenberg AR, Skapek SX, Hawkins DS. The inconvenience of convenience cohorts: Rhabdomyosarcoma and the PAX-FOXO1 biomarker. Cancer Epidemiol Biomarkers Prev (2012) 21:1012–8. doi: 10.1158/1055-9965.EPI-12-0207
9. Williamson D, Missiaglia E, De Reyniès A, Pierron G, Thuille B, Palenzuela G, et al. Fusion gene-negative alveolar rhabdomyosarcoma is clinically and molecularly indistinguishable from embryonal rhabdomyosarcoma. J Clin Oncol (2010) 28:2151–8. doi: 10.1200/JCO.2009.26.3814
10. Xia SJ, Pressey JG, Barr FG. Molecular pathogenesis of rhabdomyosarcoma. Cancer Biol Ther (2002) 1:97–104. doi: 10.4161/cbt.51
11. Huh W, Egas Bejar D. Rhabdomyosarcoma in adolescent and young adult patients: current perspectives. Adolesc Health Med Ther (2014) 5:115–25. doi: 10.2147/ahmt.s44582
12. Martinelli S, McDowell HP, Delle Vigne S, Kokai G, Uccini S, Tartaglia M, et al. RAS signaling dysregulation in human embryonal rhabdomyosarcoma. Genes Chromosom Cancer (2009) 48:975–82. doi: 10.1002/gcc.20702
13. Shern JF, Chen L, Chmielecki J, Wei JS, Patidar R, Rosenberg M, et al. Comprehensive genomic analysis of rhabdomyosarcoma reveals a landscape of alterations affecting a common genetic axis in fusion-positive and fusion-negative tumors. Cancer Discovery (2014) 4:216–31. doi: 10.1158/2159-8290.CD-13-0639
14. Saab R, Spunt SL, Skapek SX. Myogenesis and rhabdomyosarcoma: The Jekyll and Hyde of skeletal muscle. Curr Top Dev Biol (2011) 94:197–234. doi: 10.1016/B978-0-12-380916-2.00007-3
15. Keller C, Guttridge DC. Mechanisms of impaired differentiation in rhabdomyosarcoma. FEBS J (2013) 280:4323–34. doi: 10.1111/febs.12421
16. Gasparini P, Ferrari A, Casanova M, Limido F, Massimino M, Sozzi G, et al. MiRNAs as players in rhabdomyosarcoma development. Int J Mol Sci (2019) 20:5818. doi: 10.3390/ijms20225818
17. O’Brien EM, Selfe JL, Martins AS, Walters ZS, Shipley JM. The long non-coding RNA MYCNOS-01 regulates MYCN protein levels and affects growth of MYCN-amplified rhabdomyosarcoma and neuroblastoma cells. BMC Cancer (2018) 18:217. doi: 10.1186/s12885-018-4129-8
18. Rossi F, Legnini I, Megiorni F, Colantoni A, Santini T, Morlando M, et al. Circ-ZNF609 regulates G1-s progression in rhabdomyosarcoma. Oncogene (2019) 38:3843–54. doi: 10.1038/s41388-019-0699-4
19. Zhou Z, Ai H, Li K, Yao X, Zhu W, Liu L, et al. Prohibitin 2 localizes in nucleolus to regulate ribosomal RNA transcription and facilitate cell proliferation in RD cells. Sci Rep (2018) 8:1479. doi: 10.1038/s41598-018-19917-7
20. Esquela-Kerscher A. Slack FJ oncomirs - MicroRNAs with a role in cancer. Nat Rev Cancer (2006) 6:259–69. doi: 10.1038/nrc1840
21. Ciesla M, Skrzypek K, Kozakowska M, Loboda A, Jozkowicz A, Dulak J. MicroRNAs as biomarkers of disease onset. Anal Bioanal Chem (2011) 401:2051–61. doi: 10.1007/s00216-011-5001-8
22. Otmani K, Lewalle P. Tumor suppressor miRNA in cancer cells and the tumor microenvironment: Mechanism of deregulation and clinical implications. Front Oncol (2021) 11:708765. doi: 10.3389/fonc.2021.708765
23. Lee Y, Jeon K, Lee JT, Kim S, Kim VN. MicroRNA maturation: Stepwise processing and subcellular localization. EMBO J (2002) 21:4663–70. doi: 10.1093/emboj/cdf476
24. Gebert LFR, MacRae IJ. Regulation of microRNA function in animals. Nat Rev Mol Cell Biol (2019) 20:21–37. doi: 10.1038/s41580-018-0045-7
25. Treiber T, Treiber N, Meister G. Regulation of microRNA biogenesis and its crosstalk with other cellular pathways. Nat Rev Mol Cell Biol (2019) 20:5–20. doi: 10.1038/s41580-018-0059-1
26. O’Brien J, Hayder H, Zayed Y, Peng C. Overview of microRNA biogenesis, mechanisms of actions, and circulation. Front Endocrinol (Lausanne) (2018) 9:402. doi: 10.3389/fendo.2018.00402
27. Iwakawa HO, Tomari Y. The functions of MicroRNAs: mRNA decay and translational repression. Trends Cell Biol (2015) 25:651–65. doi: 10.1016/j.tcb.2015.07.011
28. Guo Z, Maki M, Ding R, Yang Y, Zhang B, Xiong L. Genome-wide survey of tissue-specific microRNA and transcription factor regulatory networks in 12 tissues. Sci Rep (2014) 4:1–9. doi: 10.1038/srep05150
29. Mavrakis KJ, van der Meulen J, Wolfe AL, Liu X, Mets E, Taghon T, et al. A cooperative microRNA-tumor suppressor gene network in acute T-cell lymphoblastic leukemia (T-ALL). Nat Genet (2011) 43:673–8. doi: 10.1038/ng.858
30. Na YJ, Kim JH. Understanding cooperativity of microRNAs via microRNA association networks. BMC Genomics (2013) 14 Suppl 5:S17. doi: 10.1186/1471-2164-14-S5-S17
31. Li L, Sarver AL, Alamgir S, Subramanian S. Downregulation of microRNAs miR-1, -206 and -29 stabilizes PAX3 and CCND2 expression in rhabdomyosarcoma. Lab Investig (2012) 92:571–83. doi: 10.1038/labinvest.2012.10
32. Calin GA, Sevignani C, Dumitru CD, Hyslop T, Noch E, Yendamuri S, et al. Human microRNA genes are frequently located at fragile sites and genomic regions involved in cancers. Proc Natl Acad Sci U.S.A. (2004) 101:2999–3004. doi: 10.1073/pnas.0307323101
33. Kumar MS, Lu J, Mercer KL, Golub TR, Jacks T. Impaired microRNA processing enhances cellular transformation and tumorigenesis. Nat Genet (2007) 39:673–7. doi: 10.1038/ng2003
34. Kara G, Calin GA, Ozpolat B. RNAi-based therapeutics and tumor targeted delivery in cancer. Adv Drug Delivery Rev (2022) 182:114113. doi: 10.1016/j.addr.2022.114113
35. Dykes IM, Emanueli C. Transcriptional and post-transcriptional gene regulation by long non-coding RNA. Genomics Proteomics Bioinforma (2017) 15:177–86. doi: 10.1016/j.gpb.2016.12.005
36. Zhang X, Wang W, Zhu W, Dong J, Cheng Y, Yin Z, et al. Mechanisms and functions of long non-coding RNAs at multiple regulatory levels. Int J Mol Sci (2019) 20:5573. doi: 10.3390/ijms20225573
37. Mishra K, Kanduri C. Understanding long noncoding RNA and chromatin interactions: What we know so far. Non-coding RNA (2019) 5:54. doi: 10.3390/ncrna5040054
38. Martone J, Mariani D, Desideri F, Ballarino M. Non-coding RNAs shaping muscle. Front Cell Dev Biol (2020) 7:394. doi: 10.3389/fcell.2019.00394
39. Khawar MB, Hamid SE, Jan T, Abbasi MH, Idnan M, Sheikh N. Diagnostic, prognostic and therapeutic potential of long noncoding RNAs in cancer. Mol Biol Rep (2022) 49:2311–9. doi: 10.1007/s11033-022-07180-z
40. Paraskevopoulou MD, Hatzigeorgiou AG. “Analyzing MiRNA–LncRNA interactions BT - long non-coding RNAs: Methods and protocols.,”. Feng Y, Zhang L, editors. New York, NY: Springer New York (2016) p. 271–86. doi: 10.1007/978-1-4939-3378-5_21
41. Yoon J-H, Abdelmohsen K, Gorospe M. Functional interactions among microRNAs and long noncoding RNAs. Semin Cell Dev Biol (2014) 34:9–14. doi: 10.1016/j.semcdb.2014.05.015
42. Wei X, Li H, Yang J, Hao D, Dong D, Huang Y, et al. Circular RNA profiling reveals an abundant circLMO7 that regulates myoblasts differentiation and survival by sponging miR-378a-3p. Cell Death Dis (2017) 8:e3153. doi: 10.1038/cddis.2017.541
43. Vo JN, Cieslik M, Zhang Y, Shukla S, Xiao L, Zhang Y, et al. The landscape of circular RNA in cancer. Cell (2019) 176:869–881.e13. doi: 10.1016/j.cell.2018.12.021
44. Noller HF, Green R, Heilek G, Hoffarth V, Hüttenhofer A, Joseph S, et al. Structure and function of ribosomal RNA. Biochem Cell Biol (1995) 73:997–1009. doi: 10.1139/o95-107
45. Gaviraghi M, Vivori C, Tonon G. How cancer exploits ribosomal RNA biogenesis: A journey beyond the boundaries of rRNA transcription. Cells (2019) 8:1098. doi: 10.3390/cells8091098
46. Bennett AH, O’Donohue MF, Gundry SR, Chan AT, Widrick J, Draper I, et al. RNA Helicase, DDX27 regulates skeletal muscle growth and regeneration by modulation of translational processes. PLoS Genet (2018) 14:e1007226. doi: 10.1371/journal.pgen.1007226
47. Cieśla M, Dulak J, Józkowicz A. MicroRNAs and epigenetic mechanisms of rhabdomyosarcoma development. Int J Biochem Cell Biol (2014) 53:482–92. doi: 10.1016/j.biocel.2014.05.003
48. Chen JF, Tao Y, Li J, Deng Z, Yan Z, Xiao X, et al. microRNA-1 and microRNA-206 regulate skeletal muscle satellite cell proliferation and differentiation by repressing Pax7. J Cell Biol (2010) 190:867–79. doi: 10.1083/jcb.200911036
49. Yan D, Da DX, Chen X, Wang L, Lu C, Wang J, et al. MicroRNA-1/206 targets c-met and inhibits rhabdomyosarcoma development. J Biol Chem (2009) 284:29596–604. doi: 10.1074/jbc.M109.020511
50. Nicolai S, Pieraccioli M, Smirnov A, Pitolli C, Anemona L, Mauriello A, et al. ZNF281/Zfp281 is a target of miR-1 and counteracts muscle differentiation. Mol Oncol (2020) 14:294–308. doi: 10.1002/1878-0261.12605
51. Taulli R, Bersani F, Foglizzo V, Linari A, Vigna E, Ladanyi M, et al. The muscle-specific microRNA miR-206 blocks human rhabdomyosarcoma growth in xenotransplanted mice by promoting myogenic differentiation. J Clin Invest (2009) 119:2366–78. doi: 10.1172/JCI38075
52. Missiaglia E, Shepherd CJ, Patel S, Thway K, Pierron G, Pritchard-Jones K, et al. MicroRNA-206 expression levels correlate with clinical behaviour of rhabdomyosarcomas. Br J Cancer (2010) 102:1769–77. doi: 10.1038/sj.bjc.6605684
53. Miyachi M, Tsuchiya K, Yoshida H, Yagyu S, Kikuchi K, Misawa A, et al. Circulating muscle-specific microRNA, miR-206, as a potential diagnostic marker for rhabdomyosarcoma. Biochem Biophys Res Commun (2010) 400:89–93. doi: 10.1016/j.bbrc.2010.08.015
54. Skrzypek K, Kusienicka A, Trzyna E, Szewczyk B, Ulman A, Konieczny P, et al. SNAIL is a key regulator of alveolar rhabdomyosarcoma tumor growth and differentiation through repression of MYF5 and MYOD function. Cell Death Dis (2018) 9:643. doi: 10.1038/s41419-018-0693-8
55. Hanna JA, Garcia MR, Go JC, Finkelstein D, Kodali K, Pagala V, et al. PAX7 is a required target for microRNA-206-induced differentiation of fusion-negative rhabdomyosarcoma. Cell Death Dis (2016) 7:e2256. doi: 10.1038/cddis.2016.159
56. Nanni P, Nicoletti G, Palladini A, Astolfi A, Rinella P, Croci S, et al. Opposing control of rhabdomyosarcoma growth and differentiation by myogenin and interleukin 4. Mol Cancer Ther (2009) 8:754–61. doi: 10.1158/1535-7163.MCT-08-0678
57. Winbanks CE, Wang B, Beyer C, Koh P, White L, Kantharidis P, et al. TGF-β regulates miR-206 and miR-29 to control myogenic differentiation through regulation of HDAC4. J Biol Chem (2011) 286:13805–14. doi: 10.1074/jbc.M110.192625
58. Ciesla M, Marona P, Kozakowska M, Jez M, Seczynska M, Loboda A, et al. Heme oxygenase-1 controls an HDAC4-MIR-206 pathway of oxidative stress in rhabdomyosarcoma. Cancer Res (2016) 76:5707–18. doi: 10.1158/0008-5472.CAN-15-1883
59. Tombolan L, Millino C, Pacchioni B, Cattelan M, Zin A, Bonvini P, et al. Circulating miR-26a as potential prognostic biomarkers in pediatric rhabdomyosarcoma. Front Genet (2020) 11:606274. doi: 10.3389/fgene.2020.606274
60. Rao PK, Missiaglia E, Shields L, Hyde G, Yuan B, Shepherd CJ, et al. Distinct roles for miR-1 and miR-133a in the proliferation and differentiation of rhabdomyosarcoma cells. FASEB J (2010) 24:3427–37. doi: 10.1096/fj.09-150698
61. Fröse J, Chen MB, Hebron KE, Reinhardt F, Hajal C, Zijlstra A, et al. Epithelial-mesenchymal transition induces podocalyxin to promote extravasation via ezrin signaling. Cell Rep (2018) 24:962–72. doi: 10.1016/j.celrep.2018.06.092
62. Skrzypek K, Nieszporek A, Badyra B, Lasota M, Majka M. Enhancement of myogenic differentiation and inhibition of rhabdomyosarcoma progression by miR-28-3p and miR-193a-5p regulated by SNAIL. Mol Ther - Nucleic Acids (2021) 24:888–904. doi: 10.1016/j.omtn.2021.04.013
63. Wang H, Garzon R, Sun H, Ladner KJ, Singh R, Dahlman J, et al. NF-κB-YY1-miR-29 regulatory circuitry in skeletal myogenesis and rhabdomyosarcoma. Cancer Cell (2008) 14:369–81. doi: 10.1016/j.ccr.2008.10.006
64. Wang Y, Zhang L, Pang Y, Song L, Shang H, Li Z, et al. MicroRNA-29 family inhibits rhabdomyosarcoma formation and progression by regulating GEFT function. Am J Transl Res (2020) 12(3):1136–54eCollection 2020.
65. Sun C, Liu C, Li S, Li H, Wang Y, Xie Y, et al. Overexpression of GEFT, a rho family guanine nucleotide exchange factor, predicts poor prognosis in patients with rhabdomyosarcoma. Int J Clin Exp Pathol (2014) 7:1606–15.
66. Sun MM, Li JF, Guo LL, Xiao HT, Dong L, Wang F, et al. TGF-β1 suppression of microRNA-450b-5p expression: A novel mechanism for blocking myogenic differentiation of rhabdomyosarcoma. Oncogene (2014) 33(16):2075–86. doi: 10.1038/onc.2013.165
67. Hanna JA, Garcia MR, Lardennois A, Leavey PJ, Maglic D, Fagnan A, et al. PAX3-FOXO1 drives miR-486-5p and represses miR-221 contributing to pathogenesis of alveolar rhabdomyosarcoma. Oncogene (2018) 37(15):1991–2007. doi: 10.1038/s41388-017-0081-3
68. Molist C, Navarro N, Giralt I, Zarzosa P, Gallo-Oller G, Pons G, et al. miRNA-7 and miRNA-324-5p regulate alpha9-integrin expression and exert anti-oncogenic effects in rhabdomyosarcoma. Cancer Lett (2020) 477:49–59. doi: 10.1016/j.canlet.2020.02.035
69. Megiorni F, Cialfi S, McDowell HP, Felsani A, Camero S, Guffanti A, et al. Deep sequencing the microRNA profile in rhabdomyosarcoma reveals down-regulation of miR-378 family members. BMC Cancer (2014) 14:880. doi: 10.1186/1471-2407-14-880
70. Pozzo E, Giarratana N, Sassi G, Elmastas M, Killian T, Wang CC, et al. Upregulation of miR181a/miR212 improves myogenic commitment in murine fusion-negative rhabdomyosarcoma. Front Physiol (2021) 12:701354. doi: 10.3389/fphys.2021.701354
71. Huang HJ, Liu J, Hua H, Li S, Zhao J, Yue S, et al. MiR-214 and n-ras regulatory loop suppresses rhabdomyosarcoma cell growth and xenograft tumorigenesis. Oncotarget (2014) 5:2161–75. doi: 10.18632/oncotarget.1855
72. Bharathy N, Berlow NE, Wang E, Abraham J, Settelmeyer TP, Hooper JE, et al. The HDAC3-SMARCA4-miR-27a axis promotes expression of the PAX3:FOXO1 fusion oncogene in rhabdomyosarcoma. Sci Signal (2018) 11:eaau7632. doi: 10.1126/scisignal.aau7632
73. Tombolan L, Zampini M, Casara S, Boldrin E, Zin A, Bisogno G, et al. MicroRNA-27a contributes to rhabdomyosarcoma cell proliferation by suppressing RARA and RXRA. PLoS One (2015) 10:e0125171. doi: 10.1371/journal.pone.0125171
74. Sarver AL, Li H, Subramanian S. MicroRNA miR-183 functions as an oncogene by targeting the transcription factor EGR1 and promoting tumor cell migration. Cancer Res (2010) 70:9570–80. doi: 10.1158/0008-5472.CAN-10-2074
75. Missiaglia E, Shepherd CJ, Aladowicz E, Olmos D, Selfe J, Pierron G, et al. MicroRNA and gene co-expression networks characterize biological and clinical behavior of rhabdomyosarcomas. Cancer Lett (2017) 385:251–60. doi: 10.1016/j.canlet.2016.10.011
76. Mercado GE, Xia SJ, Zhang C, Ahn H, Gustafson DM, Laé M, et al. Identification of PAX3-FKHR-regulated genes differentially expressed between alveolar and embryonal rhabdomyosarcoma: Focus on MYCN as a biologically relevant target. Genes Chromosom Cancer (2008) 47:510–20. doi: 10.1002/gcc.20554
77. Gasparini P, Fortunato O, De Cecco L, Casanova M, Iannó MF, Carenzo A, et al. Age-related alterations in immune contexture are associated with aggressiveness in rhabdomyosarcoma. Cancers (Basel) (2019) 11:1380. doi: 10.3390/cancers11091380
78. Reichek JL, Duan F, Smith LM, Gustafson DM, O’Connor RS, Zhang C, et al. Genomic and clinical analysis of amplification of the 13q31 chromosomal region in alveolar rhabdomyosarcoma: A report from the children’s oncology group. Clin Cancer Res (2011) 17:1463–73. doi: 10.1158/1078-0432.CCR-10-0091
79. Ghamloush F, Ghayad SE, Rammal G, Fahs A, Ayoub AJ, Merabi Z, et al. The PAX3-FOXO1 oncogene alters exosome miRNA content and leads to paracrine effects mediated by exosomal miR-486. Sci Rep (2019) 9:14242. doi: 10.1038/s41598-019-50592-4
80. Pan Y, Li J, Lou S, Chen W, Lin Y, Shen N, et al. Down-regulated miR-130a/b attenuates rhabdomyosarcoma proliferation via PPARG. Front Mol Biosci (2022) 8:766887. doi: 10.3389/fmolb.2021.766887
81. Alexander MS, Kunkel LM. Skeletal muscle MicroRNAs: Their diagnostic and therapeutic potential in human muscle diseases. J Neuromuscul Dis (2015) 2:1–11. doi: 10.3233/JND-140058
82. Siracusa J, Koulmann N, Banzet S. Circulating myomiRs: a new class of biomarkers to monitor skeletal muscle in physiology and medicine. J Cachexia Sarcopenia Muscle (2018) 9:20–7. doi: 10.1002/jcsm.12227
83. Horak M, Novak J, Bienertova-Vasku J. Muscle-specific microRNAs in skeletal muscle development. Dev Biol (2016) 410:1–13. doi: 10.1016/j.ydbio.2015.12.013
84. Mitchelson KR. Roles of the canonical myomiRs miR-1, -133 and -206 in cell development and disease. World J Biol Chem (2015) 6:162. doi: 10.4331/wjbc.v6.i3.162
85. McCarthy JJ. MicroRNA-206: The skeletal muscle-specific myomiR. Biochim Biophys Acta - Gene Regul Mech (2008) 1779:682–91. doi: 10.1016/j.bbagrm.2008.03.001
86. Kirby TJ, Chaillou T, McCarthy JJ. The role of microRNAs in skeletal muscle health and disease. Front Biosci - Landmark (2015) 20:37–77. doi: 10.2741/4298
87. Corsten MF, Dennert R, Jochems S, Kuznetsova T, Devaux Y, Hofstra L, et al. Circulating MicroRNA-208b and MicroRNA-499 reflect myocardial damage in cardiovascular disease. Circ Cardiovasc Genet (2010) 3:499–506. doi: 10.1161/CIRCGENETICS.110.957415
88. Rao PK, Kumar RM, Farkhondeh M, Baskerville S, Lodish HF. Myogenic factors that regulate expression of muscle-specific microRNAs. Proc Natl Acad Sci U.S.A. (2006) 102:1769–77. doi: 10.1073/pnas.0602831103
89. Miyachi M, Tsuchiya K, Nitta Y, Ouchi K, Yoshida H, Kikuchi K, et al. Diagnostic and prognostic role of circulating miR-206 in rhabdomyosarcoma patients. J Clin Oncol (2015) 33:10038. doi: 10.1200/jco.2015.33.15_suppl.10038
90. Rashed MH, Kanlikilicer P, Rodriguez-Aguayo C, Pichler M, Bayraktar R, Bayraktar E, et al. Exosomal miR-940 maintains SRC-mediated oncogenic activity in cancer cells: A possible role for exosomal disposal of tumor suppressor miRNAs. Oncotarget (2017) 8:20145–64. doi: 10.18632/oncotarget.15525
91. Ulman A, Skrzypek K, Konieczny P, Mussolino C, Cathomen T, Majka M. Genome editing of the SNAI1 gene in rhabdomyosarcoma: A novel model for studies of its role. Cells (2020) 9:1–16. doi: 10.3390/cells9051095
92. Püsküllüoglu M, Lukasiewicz E, Miekus K, Jarocha D, Majka M. Differential expression of Snail1 transcription factor and Snail1-related genes in alveolar and embryonal rhabdomyosarcoma subtypes. Folia Histochem Cytobiol (2010) 48:671–7. doi: 10.2478/v10042-010-0046-7
93. Skrzypek K, Adamek G, Kot M, Badyra B, Majka M. Progression and differentiation of alveolar rhabdomyosarcoma is regulated by PAX7 transcription factor–significance of tumor subclones. Cells (2021) 10:1–25. doi: 10.3390/cells10081870
94. Relaix F, Montarras D, Zaffran S, Gayraud-Morel B, Rocancourt D, Tajbakhsh S, et al. Pax3 and Pax7 have distinct and overlapping functions in adult muscle progenitor cells. J Cell Biol (2006) 172:91–102. doi: 10.1083/jcb.200508044
95. Arasu A, Murugan S, Essa MM, Velusamy T, Guillemin GJ. PAX3: A molecule with oncogenic or tumor suppressor function is involved in cancer. BioMed Res Int (2018) 2018:1–12. doi: 10.1155/2018/1095459
96. Blake J, Ziman MR. Aberrant PAX3 and PAX7 expression. a link to the metastatic potential of embryonal rhabdomyosarcoma and cutaneous malignant melanoma? Histol Histopathol (2003) 18:529–39. doi: 10.14670/HH-18.529
97. Taulli R, Scuoppo C, Bersani F, Accornero P, Forni PE, Miretti S, et al. Validation of met as a therapeutic target in alveolar and embryonal rhabdomyosarcoma. Cancer Res (2006) 66:4742–9. doi: 10.1158/0008-5472.CAN-05-4292
98. Kikuchi K, Tsuchiya K, Otabe O, Gotoh T, Tamura S, Katsumi Y, et al. Effects of PAX3-FKHR on malignant phenotypes in alveolar rhabdomyosarcoma. Biochem Biophys Res Commun (2008) 365:568–74. doi: 10.1016/j.bbrc.2007.11.017
99. Saini M, Verma A, Mathew SJ. SPRY2 is a novel MET interactor that regulates metastatic potential and differentiation in rhabdomyosarcoma. Cell Death Dis (2018) 9:237. doi: 10.1038/s41419-018-0261-2
100. Khanjyan MV, Yang J, Kayali R, Caldwell T, Bertoni C. A high-content, high-throughput siRNA screen identifies cyclin D2 as a potent regulator of muscle progenitor cell fusion and a target to enhance muscle regeneration. Hum Mol Genet (2013) 22:3283–95. doi: 10.1093/hmg/ddt184
101. Xu L, Zheng Y, Liu J, Rakheja D, Singleterry S, Laetsch TW, et al. Integrative Bayesian analysis identifies rhabdomyosarcoma disease genes. Cell Rep (2018) 24:238–51. doi: 10.1016/j.celrep.2018.06.006
102. Hahn S, Jackstadt R, Siemens H, Hünten S, Hermeking H. SNAIL and miR-34a feed-forward regulation of ZNF281/ZBP99 promotes epithelial-mesenchymal transition. EMBO J (2013) 32:3079–95. doi: 10.1038/emboj.2013.236
103. Pieraccioli M, Nicolai S, Pitolli C, Agostini M, Antonov A, Malewicz M, et al. ZNF281 inhibits neuronal differentiation and is a prognostic marker for neuroblastoma. Proc Natl Acad Sci U.S.A. (2018) 115:7356–61. doi: 10.1073/pnas.1801435115
104. Winbanks CE, Beyer C, Hagg A, Qian H, Sepulveda PV, Gregorevic P. miR-206 represses hypertrophy of myogenic cells but not muscle fibers via inhibition of HDAC4. PLoS One (2013) 8:1–13. doi: 10.1371/journal.pone.0073589
105. Delcuve GP, Khan DH, Davie JR. Roles of histone deacetylases in epigenetic regulation: Emerging paradigms from studies with inhibitors. Clin Epigenet (2012) 4:5. doi: 10.1186/1868-7083-4-5
106. Miska EA, Langley E, Wolf D, Karlsson C, Pines J, Kouzarides T. Differential localization of HDAC4 orchestrates muscle differentiation. Nucleic Acids Res (2001) 29:3439–47. doi: 10.1093/nar/29.16.3439
107. Baird K, Davis S, Antonescu CR, Harper UL, Walker RL, Chen Y, et al. Gene expression profiling of human sarcomas: Insights into sarcoma biology. Cancer Res (2005) 65:9226–35. doi: 10.1158/0008-5472.CAN-05-1699
108. Chen JF, Mandel EM, Thomson JM, Wu Q, Callis TE, Hammond SM, et al. The role of microRNA-1 and microRNA-133 in skeletal muscle proliferation and differentiation. Nat Genet (2006) 38:228–33. doi: 10.1038/ng1725
109. Hak KK, Yong SL, Sivaprasad U, Malhotra A, Dutta A. Muscle-specific microRNA miR-206 promotes muscle differentiation. J Cell Biol (2006) 174:677–87. doi: 10.1083/jcb.200603008
110. Feng Y, Niu LL, Wei W, Zhang WY, Li XY, Cao JH, et al. A feedback circuit between miR-133 and the ERK1/2 pathway involving an exquisite mechanism for regulating myoblast proliferation and differentiation. Cell Death Dis (2013) 4:e934. doi: 10.1038/cddis.2013.462
111. de la Serna IL, Carlson KA, Anthony N. Imbalzano. Mammalian SWI/SNF complexes promote MyoD-mediatedmuscle differentiation. Nat Genet (2001) 27:187–90. doi: 10.1038/84826
112. Tang H, Macpherson P, Marvin M, Meadows E, Klein WH, Yang XJ, et al. A histone deacetylase 4/Myogenin positive feedback loop coordinates denervation-dependent gene induction and suppression. Mol Biol Cell (2009) 20:1120. doi: 10.1091/MBC.E08-07-0759
113. Skrzypek K, Kot M, Konieczny P, Nieszporek A, Kusienicka A, Lasota M, et al. SNAIL promotes metastatic behavior of rhabdomyosarcoma by increasing EZRIN and AKT expression and regulating microRNA networks. Cancers (Basel) (2020) 12:1–25. doi: 10.3390/cancers12071870
114. Shirafkan N, Shomali N, Kazemi T, Shanehbandi D, Ghasabi M, Baghbani E, et al. microRNA-193a-5p inhibits migration of human HT-29 colon cancer cells via suppression of metastasis pathway. J Cell Biochem (2019) 120:8775–83. doi: 10.1002/jcb.28164
115. Pu Y, Zhao F, Cai W, Meng X, Li Y, Cai S. MiR-193a-3p and miR-193a-5p suppress the metastasis of human osteosarcoma cells by down-regulating Rab27B and SRR, respectively. Clin Exp Metastasis (2016) 33:359–72. doi: 10.1007/s10585-016-9783-0
116. Liu D, Black BL, Derynck R. TGF-β inhibits muscle differentiation through functional repression of myogenic transcription factors by Smad3. Genes Dev (2001) 15:2950–66. doi: 10.1101/gad.925901
117. Lu J, McKinsey TA, Zhang CL, Olson EN. Regulation of skeletal myogenesis by association of the MEF2 transcription factor with class II histone deacetylases. Mol Cell (2000) 6:233–44. doi: 10.1016/s1097-2765(00)00025-3
118. McKinsey TA, Zhang CL, Lu J, Olson EN. Signal-dependent nuclear export of a histone deacetylase regulates muscle differentiation. Nature (2000) 408:106–11. doi: 10.1038/35040593
119. Liu C, Zhang L, Cui W, Du J, Li Z, Pang Y, et al. Epigenetically upregulated GEFT-derived invasion and metastasis of rhabdomyosarcoma via epithelial mesenchymal transition promoted by the Rac1/Cdc42-PAK signalling pathway. EBioMedicine (2019) 50:122–34. doi: 10.1016/j.ebiom.2019.10.060
120. Yang P, Zhang Y, Markowitz GJ, Guo X, Wang XF. TGF-β-regulated microRNAs and their function in cancer biology. Methods Mol Biol (2016) 1344:325–39. doi: 10.1007/978-1-4939-2966-5_21
121. Cao L, Xie B, Yang X, Liang H, Jiang X, Zhang D, et al. MIR-324-5p suppresses hepatocellular carcinoma cell invasion by counteracting ECM degradation through post-transcriptionally downregulating ETS1 and SP1. PLoS One (2015) 10:1–16. doi: 10.1371/journal.pone.0133074
122. Babae N, Bourajjaj M, Liu Y, Van Beijnum JR, Cerisoli F, Scaria PV, et al. Systemic miRNA-7 delivery inhibits tumor angiogenesis and growth in murine xenograft glioblastoma. Oncotarget (2014) 5:6687–700. doi: 10.18632/oncotarget.2235
123. Cui YX, Bradbury R, Flamini V, Wu B, Jordan N, Jiang WG. MicroRNA-7 suppresses the homing and migration potential of human endothelial cells to highly metastatic human breast cancer cells. Br J Cancer (2017) 117:89–101. doi: 10.1038/bjc.2017.156
124. Tang B, Xu A, Xu J, Huang H, Chen L, Su Y, et al. MicroRNA-324-5p regulates stemness, pathogenesis and sensitivity to bortezomib in multiple myeloma cells by targeting hedgehog signaling. Int J Cancer (2018) 142:109–20. doi: 10.1002/ijc.31041
125. Hamidi H, Ivaska J. Every step of the way: Integrins in cancer progression and metastasis. Nat Rev Cancer (2018) 18:533–48. doi: 10.1038/s41568-018-0038-z
126. Høye AM, Couchman JR, Wewer UM, Fukami K, Yoneda A. The newcomer in the integrin family: Integrin α9 in biology and cancer. Adv Biol Regul (2012) 52:326–39. doi: 10.1016/j.jbior.2012.03.004
127. Masià A, Almazán-Moga A, Velasco P, Reventós J, Torán N, Sánchez De Toledo J, et al. Notch-mediated induction of n-cadherin and α9-integrin confers higher invasive phenotype on rhabdomyosarcoma cells. Br J Cancer (2012) 107:1374–83. doi: 10.1038/bjc.2012.411
128. Werner H, Maor S. The insulin-like growth factor-I receptor gene: a downstream target for oncogene and tumor suppressor action. Trends Endocrinol Metab (2006) 17:236–42. doi: 10.1016/j.tem.2006.06.007
129. Giacomazzi G, Holvoet B, Trenson S, Caluwé E, Kravic B, Grosemans H, et al. MicroRNAs promote skeletal muscle differentiation of mesodermal iPSC-derived progenitors. Nat Commun (2017) 8:1–14. doi: 10.1038/s41467-017-01359-w
130. Asfour HA, Allouh MZ, Said RS. Myogenic regulatory factors: The orchestrators of myogenesis after 30 years of discovery. Exp Biol Med (2018) 243:118–28. doi: 10.1177/1535370217749494
131. Liu T, Tang H, Lang Y, Liu M, Li X. MicroRNA-27a functions as an oncogene in gastric adenocarcinoma by targeting prohibitin. Cancer Lett (2009) 273:233–42. doi: 10.1016/j.canlet.2008.08.003
132. Mi S, Lu J, Sun M, Li Z, Zhang H, Neilly MB, et al. MicroRNA expression signatures accurately discriminate acute lymphoblastic leukemia from acute myeloid leukemia. Proc Natl Acad Sci U.S.A. (2007) 104:19971–6. doi: 10.1073/pnas.0709313104
133. Fulci V, Chiaretti S, Goldoni M, Azzalin G, Carucci N, Tavolaro S, et al. Quantitative technologies establish a novel microRNA profile of chronic lymphocytic leukemia. Blood (2007) 109:4944–51. doi: 10.1182/blood-2006-12-062398
134. Mertens-Talcott SU, Chintharlapalli S, Li X, Safe S. The oncogenic microRNA-27a targets genes that regulate specificity protein transcription factors and the G2-m checkpoint in MDA-MB-231 breast cancer cells. Cancer Res (2007) 67:11001–11. doi: 10.1158/0008-5472.CAN-07-2416
135. Huang D, Wang H, Liu R, Li H, Ge S, Bai M, et al. MiRNA27a is a biomarker for predicting chemosensitivity and prognosis in metastatic or recurrent gastric cancer. J Cell Biochem (2014) 115:549–56. doi: 10.1002/jcb.24689
136. Hao L, Wang J. miR-27a acts as an oncogene to regulate endometrial cancer progression by targeting USP46. Int J Clin Exp Pathol (2021) 14:720–5.
137. Rota R, Ciarapica R, Giordano A, Miele L, Locatelli F. MicroRNAs in rhabdomyosarcoma: Pathogenetic implications and translational potentiality. Mol Cancer (2011) 10:120. doi: 10.1186/1476-4598-10-120
138. Ghayad SE, Rammal G, Ghamloush F, Basma H, Nasr R, Diab-Assaf M, et al. Exosomes derived from embryonal and alveolar rhabdomyosarcoma carry differential miRNA cargo and promote invasion of recipient fibroblasts. Sci Rep (2016) 6:37088. doi: 10.1038/srep37088
139. Rammal G, Fahs A, Kobeissy F, Mechref Y, Zhao J, Zhu R, et al. Proteomic profiling of rhabdomyosarcoma-derived exosomes yield insights into their functional role in paracrine signaling. J Proteome Res (2019) 18:3567–79. doi: 10.1021/acs.jproteome.9b00157
140. Miller IV, Raposo G, Welsch U, Prazeres da Costa O, Thiel U, Lebar M, et al. First identification of ewing’s sarcoma-derived extracellular vesicles and exploration of their biological and potential diagnostic implications. Biol Cell (2013) 105:289–303. doi: 10.1111/boc.201200086
141. Kahlert C, Kalluri R. Exosomes in tumor microenvironment influence cancer progression and metastasis. J Mol Med (2013) 91:431–7. doi: 10.1007/s00109-013-1020-6
142. Schwarzenbach H, Gahan PB. Exosomes in immune regulation. Non-coding RNA (2021) 7:1–32. doi: 10.3390/ncrna7010004
143. Temoche-Diaz MM, Shurtleff MJ, Nottingham RM, Yao J, Fadadu RP, Lambowitz AM, et al. Distinct mechanisms of microrna sorting into cancer cell-derived extracellular vesicle subtypes. Elife (2019) 8:e47544. doi: 10.7554/eLife.47544
144. Song L, Lin C, Gong H, Wang C, Liu L, Wu J, et al. MiR-486 sustains NF-κB activity by disrupting multiple NF-κB-negative feedback loops. Cell Res (2013) 31:1138. doi: 10.1038/cr.2012.174
145. Tian F, Wang J, Ouyang T, Lu N, Lu J, Shen Y, et al. MiR-486-5p serves as a good biomarker in nonsmall cell lung cancer and suppresses cell growth with the involvement of a target PIK3R1. Front Genet (2019) 10:688. doi: 10.3389/fgene.2019.00688
146. Shaham L, Vendramini E, Ge Y, Goren Y, Birger Y, Tijssen MR, et al. MicroRNA-486-5p is an erythroid oncomiR of the myeloid leukemias of down syndrome. Blood (2015) 125:1292–301. doi: 10.1182/blood-2014-06-581892
147. Ahmed AA, Farooqi MS, Habeebu SS, Gonzalez E, Flatt TG, Wilson AL, et al. NanoString digital molecular profiling of protein and microRNA in rhabdomyosarcoma. Cancers (Basel) (2022) 14:522. doi: 10.3390/cancers14030522
148. Jin JJ, Lv W, Xia P, Xu ZY, Zheng AD, Wang XJ, et al. Long noncoding RNA SYISL regulates myogenesis by interacting with polycomb repressive complex 2. Proc Natl Acad Sci U.S.A. (2018) 115:E9802–11. doi: 10.1073/pnas.1801471115
149. Zhou L, Sun K, Zhao Y, Zhang S, Wang X, Li Y, et al. Linc-YY1 promotes myogenic differentiation and muscle regeneration through an interaction with the transcription factor YY1. Nat Commun (2015) 6:10026. doi: 10.1038/ncomms10026
150. Wang S, Zuo H, Jin J, Lv W, Xu Z, Fan Y, et al. Long noncoding RNA Neat1 modulates myogenesis by recruiting Ezh2. Cell Death Dis (2019) 10:505. doi: 10.1038/s41419-019-1742-7
151. Casola S, Pedone PV, Cavazzana AO, Basso G, Luksch R, D’Amore ESG, et al. Expression and parental imprinting of the H19 gene in human rhabdomyosarcoma. Oncogene (1997) 14:1503–10. doi: 10.1038/sj.onc.1200956
152. Yoshimizu T, Miroglio A, Ripoche MA, Gabory A, Vernucci M, Riccio A, et al. The H19 locus acts in vivo as a tumor suppressor. Proc Natl Acad Sci U.S.A. (2008) 105:12417–22. doi: 10.1073/pnas.0801540105
153. Zhang Y, Liu YT, Tang H, Xie WQ, Yao H, Gu WT, et al. Exosome-transmitted lncRNA H19 inhibits the growth of pituitary adenoma. J Clin Endocrinol Metab (2019) 104:6345–56. doi: 10.1210/jc.2019-00536
154. Ghafouri-Fard S, Khoshbakht T, Hussen BM, Taheri M, Samadian M. A review on the role of miR-1246 in the pathoetiology of different cancers. Front Mol Biosci (2022) 8:771835. doi: 10.3389/fmolb.2021.771835
155. Schultheiss CS, Laggai S, Czepukojc B, Hussein UK, List M, Barghash A, et al. The long non-coding RNA H19 suppresses carcinogenesis and chemoresistance in hepatocellular carcinoma. Cell Stress (2017) 1:37–54. doi: 10.15698/cst2017.10.105
156. Tarnowski M, Tkacz M, Czerewaty M, Poniewierska-Baran A, Grymuła K, Ratajczak MZ. 5-azacytidine inhibits human rhabdomyosarcoma cell growth by downregulating insulin-like growth factor 2 expression and reactivating the H19 gene product miR-675, which negatively affects insulin-like growth factors and insulin signaling. Int J Oncol (2015) 46:2241–50. doi: 10.3892/ijo.2015.2906
157. Dey BK, Pfeifer K, Dutta A. The H19 long noncoding RNA gives rise to microRNAs miR-675-3p and miR-675-5p to promote skeletal muscle differentiation and regeneration. Genes Dev (2014) 28:491–501. doi: 10.1101/gad.234419.113
158. Li H, Yang J, Wei X, Song C, Dong D, Huang Y, et al. CircFUT10 reduces proliferation and facilitates differentiation of myoblasts by sponging miR-133a. J Cell Physiol (2018) 233:4643–51. doi: 10.1002/jcp.26230
159. Wang S, Xue X, Wang R, Li X, Li Q, Wang Y, et al. CircZNF609 promotes breast cancer cell growth, migration, and invasion by elevating p70S6K1 via sponging miR-145-5p. Cancer Manag Res (2018) 10:3881–90. doi: 10.2147/CMAR.S174778
160. Legnini I, Di Timoteo G, Rossi F, Morlando M, Briganti F, Sthandier O, et al. Circ-ZNF609 is a circular RNA that can be translated and functions in myogenesis. Mol Cell (2017) 66:22–37.e9. doi: 10.1016/j.molcel.2017.02.017
161. Wang YH, Li ML, Wang YH, Liu J, Zhang ML, Fang XT, et al. A Zfp609 circular RNA regulates myoblast differentiation by sponging miR-194-5p. Int J Biol Macromol (2019) 121:1308–13. doi: 10.1016/j.ijbiomac.2018.09.039
162. Rossi F, Centrón-Broco A, Dattilo D, Di Timoteo G, Guarnacci M, Colantoni A, et al. CircVAMP3: A circRNA with a role in alveolar rhabdomyosarcoma cell cycle progression. Genes (Basel) (2021) 12:985. doi: 10.3390/genes12070985
163. Nguyen LXT, Raval A, Garcia JS, Mitchell BS. Regulation of ribosomal gene expression in cancer. J Cell Physiol (2015) 230:1181–8. doi: 10.1002/jcp.24854
164. Sun L, Liu L, Yang XJ, Wu Z. Akt binds prohibitin 2 and relieves its repression of MyoD and muscle differentiation. J Cell Sci (2004) 117:3021–9. doi: 10.1242/jcs.01142
165. Williamson D, Lu YJ, Fang C, Pritchard-Jones K, Shipley J. Nascent pre-rRNA overexpression correlates with an adverse prognosis in alveolar rhabdomyosarcoma. Genes Chromosom Cancer (2006) 45:839–45. doi: 10.1002/gcc.20347
166. Winkle M, El-Daly SM, Fabbri M, Calin GA. Noncoding RNA therapeutics — challenges and potential solutions. Nat Rev Drug Discovery (2021) 20:629–51. doi: 10.1038/s41573-021-00219-z
167. Hanna J, Hossain GS, Kocerha J. The potential for microRNA therapeutics and clinical research. Front Genet (2019) 10:478. doi: 10.3389/fgene.2019.00478
168. Zhou JG, Huang Z, Slaby O, Navarro A. Editorial: The role of ncRNAs in solid tumors prognosis: From laboratory to clinical utility. Front Oncol (2021) 10:631316. doi: 10.3389/fonc.2020.631316
169. Mishra PJ, Merlino G. MicroRNA reexpression as differentiation therapy in cancer. J Clin Invest (2009) 119:2119–23. doi: 10.1172/JCI40107
170. Lim LP, Lau NC, Garrett-Engele P, Grimson A, Schelter JM, Castle J, et al. Microarray analysis shows that some microRNAs downregulate large numbers of-target mRNAs. Nature (2005) 33:769–73. doi: 10.1038/nature03315
171. Setten RL, Rossi JJ, Han Sp. The current state and future directions of RNAi-based therapeutics. Nat Rev Drug Discovery (2019) 18:421–46. doi: 10.1038/s41573-019-0017-4
172. Havelange V, Heaphy CEA, Garzon R. MicroRNAs in the diagnosis, prognosis and treatment of cancer. Oncol Rev (2008) 2:203–13. doi: 10.1007/s12156-008-0076-y
173. Connelly CM, Deiters A. Identification of inhibitors of microRNA function from small molecule screens. Methods Mol Biol (2014) 1095:147–56. doi: 10.1007/978-1-62703-703-7_12
174. Shan G, Li Y, Zhang J, Li W, Szulwach KE, Duan R, et al. A small molecule enhances RNA interference and promotes microRNA processing. Nat Biotechnol (2008) 26:933–40. doi: 10.1038/nbt.1481
175. Wurdinger T, Costa FF. Molecular therapy in the microRNA era. Pharmacogenom J (2007) 7:297–304. doi: 10.1038/sj.tpj.6500429
176. Nguyen LD, Chau RK, Krichevsky AM. Small molecule drugs targeting non-coding RNAs as treatments for alzheimer’s disease and related dementias. Genes (Basel) (2021) 12:2005. doi: 10.3390/genes12122005
177. Rupaimoole R, Slack FJ. MicroRNA therapeutics: Towards a new era for the management of cancer and other diseases. Nat Rev Drug Discovery (2017) 16:203–21. doi: 10.1038/nrd.2016.246
Keywords: rhabdomyosarcoma, non-coding RNAs, miRNA, lncRNA, circRNA, rRNA, prognosis, therapeutic targets
Citation: Ramadan F, Saab R, Hussein N, Clézardin P, Cohen PA and Ghayad SE (2022) Non-coding RNA in rhabdomyosarcoma progression and metastasis. Front. Oncol. 12:971174. doi: 10.3389/fonc.2022.971174
Received: 16 June 2022; Accepted: 25 July 2022;
Published: 11 August 2022.
Edited by:
Dong-Hua Yang, St. John’s University, United StatesReviewed by:
Zoë Walters, University of Southampton, United KingdomBishwanath Chatterjee, National Institutes of Health (NIH), United States
Copyright © 2022 Ramadan, Saab, Hussein, Clézardin, Cohen and Ghayad. This is an open-access article distributed under the terms of the Creative Commons Attribution License (CC BY). The use, distribution or reproduction in other forums is permitted, provided the original author(s) and the copyright owner(s) are credited and that the original publication in this journal is cited, in accordance with accepted academic practice. No use, distribution or reproduction is permitted which does not comply with these terms.
*Correspondence: Sandra E. Ghayad, c2FuZHJhLmdoYXlhZEB1bml2LWFtdS5mcg==