- 1Department of Dermatology, Miguel Servet University Hospital, Instituto Investigación Sanitaria (IIS), Zaragoza, Aragón, Spain
- 2Department of Biology, Universidad Autónoma de Madrid, Madrid, Spain
- 3Department of Experminetal Dermatology and Skin Biology, Instituto Ramón y Cajal de Investigaciones Sanitarias, IRYCIS, Madrid, Spain
- 4Servicio de Dermatología, Hospital General de Ciudad Real, Ciudad Real, Spain
- 5Department of Medicine and Medical Specialties, Universidad de Alcalá, Madrid, Spain
Non-melanoma skin cancer has recently seen an increase in prevalence, and it is estimated that this grow will continue in the coming years. In this sense, the importance of therapy effectiveness has increased, especially photodynamic therapy. Photodynamic therapy has attracted much attention as a minimally invasive, selective and repeatable approach for skin cancer treatment and prevention. Although its high efficiency, this strategy has also faced problems related to tumor resistance, where the tumor microenvironment has gained a well-deserved role in recent years. Tumor microenvironment denotes a wide variety of elements, such as cancer-associated fibroblasts, immune cells, endothelial cells or the extracellular matrix, where their interaction and the secretion of a wide diversity of cytokines. Therefore, the need of designing new strategies targeting elements of the tumor microenvironment to overcome the observed resistance has become evident. To this end, in this review we focus on the role of cancer-associated fibroblasts and tumor-associated macrophages in the resistance to photodynamic therapy. We are also exploring new approaches consisting in the combination of new and old drugs targeting these cells with photodynamic therapy to enhance treatment outcomes of non-melanoma skin cancer.
Introduction
According to the Global Cancer Observatory, non-melanoma skin cancer (NMSC) has an annual incidence of 5.8% of the world population. In addition, in the last few years it has been observed an increase in its prevalence (between 3% and 7%) and it is estimated that it will continue in the coming years. The most common types include basal cell carcinoma (BCC) and squamous cell carcinoma (SCC) (1–3). The standard treatment options for NMSC include surgery, radiotherapy, chemotherapy as well as their combination (4, 5). However, within non-invasive treatments, photodynamic therapy (PDT) stands out, since it has become one of the therapeutic modalities that has grown the most in recent years, presenting numerous advantages over other treatments. PDT is a light-based therapeutic modality that involves administration of a tumor-localizing photosensitizing agent, which may require metabolic synthesis, followed by its activation with light of a specific wavelength. The mechanisms of action depend on the generation of singlet oxygen (1O2), through the excitation of the photosensitizer (PS), which transfers its excitation energy to the molecular oxygen in tumor tissues via triplet state. The necrotic, autophagy or apoptotic destruction of the tumor cells is induced by cytotoxic singlet oxygen and other secondary molecules such as reactive oxygen species (ROS) (4, 6) (Figure 1).
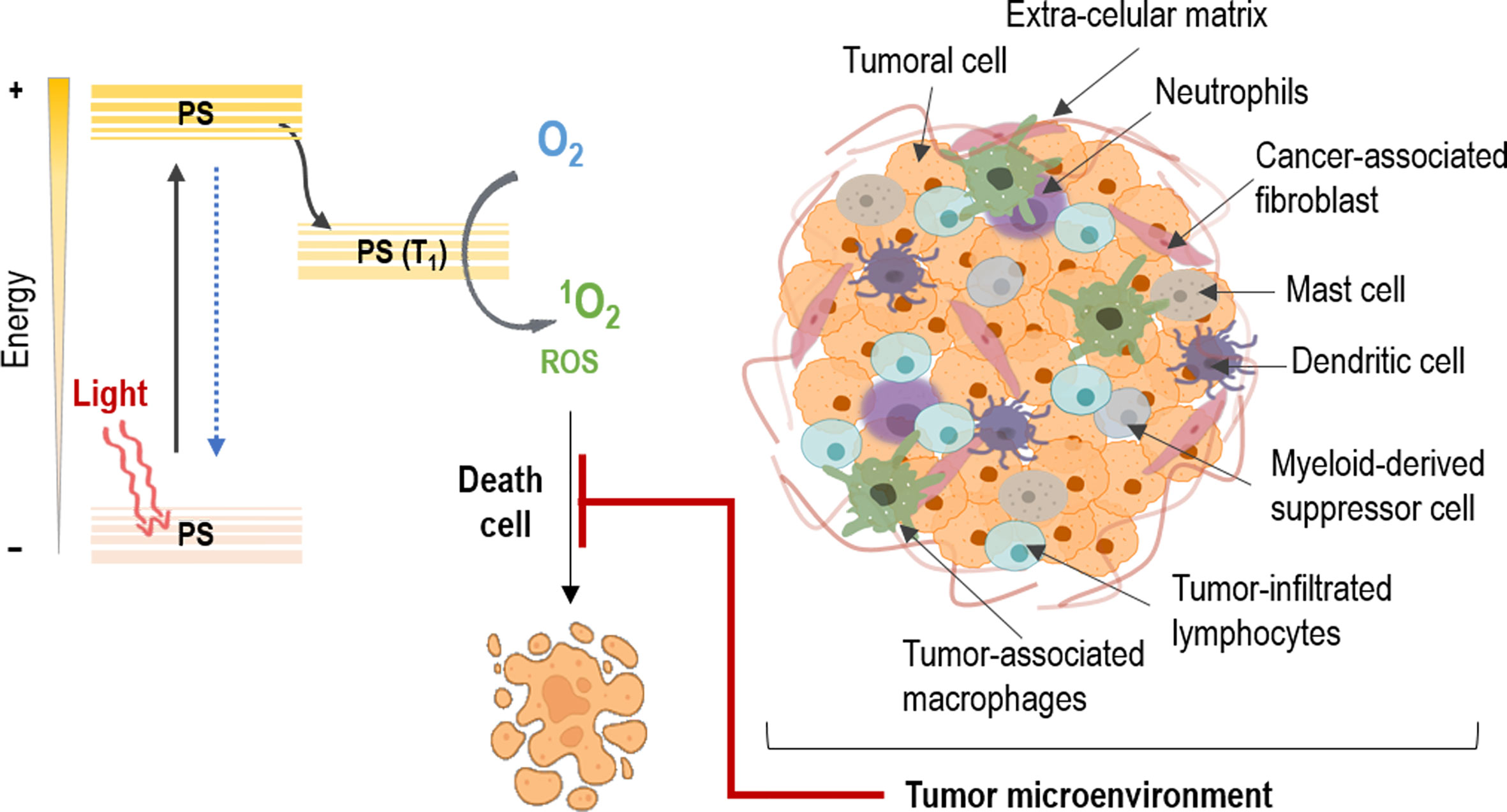
Figure 1 Mechanism of action on tumors in photodynamic therapy. The photosensitizer (PS) absorbs light and an electron moves to the first short-lived excited singlet state. This is followed by intersystem crossing, in which the excited electron changes its spin and produces a longer-lived triplet state. The PS triplet transfers energy to oxygen producing reactive singlet oxygen (1O2) and reactive oxygen species (ROS), which kill tumor cells. However, the tumor microenvironment protects the cancer cells against PDT through the interaction of its different constituents.
Moving on to other issues, despite the fact that the concept of tumor microenvironment (TME) has existed for more than a hundred years now, it has not been until recently that it has gained prominence (7). In this sense, tumors are not only constituted by cancer cells, but for a more complex intricate of diverse components (8). These TME constituents have been proved to be implicated in cancer cell survival, tumor development and therapeutic efficacy. Thereby, this tumoral stroma is composed by different cell types that fulfills their own role in these processes (9). Among them, fibroblasts, immune cells and endothelial cells are immersed in extracellular components, interacting with tumor cells. Moreover, a vascular network keeps nourished this amalgam of cells, where their crosstalk results in environment mediated drug resistance (10). These interactions proved to be involved in the effect of resistance of many tumors against different therapeutic strategies, specifically in PDT, are the object of the present review (Figure 1).
Tumor microenvironment components
Cancer-associated fibroblasts and cytokines
Fibroblasts are the main cell type present in the dermis. Because the dermis provides strength and flexibility to the skin, some of the principal functions of dermal fibroblasts are directly related to these abilities. Among others, wound healing and deposition of collagen and elastic fibers of the extracellular matrix (ECM) in connective tissue can be pointed out (11–13).
In the TME, normal fibroblasts suffer an activation process, acquiring specific characteristics and expressing differential markers such as vimentin, smooth muscle actin alpha (α-sma) and fibroblast activation protein (FAP) (14) (Figure 2). At this point, they receive the name of cancer-associated fibroblasts (CAFs) (15). Nevertheless, CAF populations have turned out to be heterogeneous, coexisting within the same tumor (such as inflammatory or myofibroblastic phenotypes) (16, 17). Because of this diversity, recent investigations have focused their efforts to study the broad amount of cytokines implicated in these changes (such as transforming growth factor beta (TGFβ), Interleukin-1 (IL-1) or integrins), as they are not only morphological, but also functional (18–21).
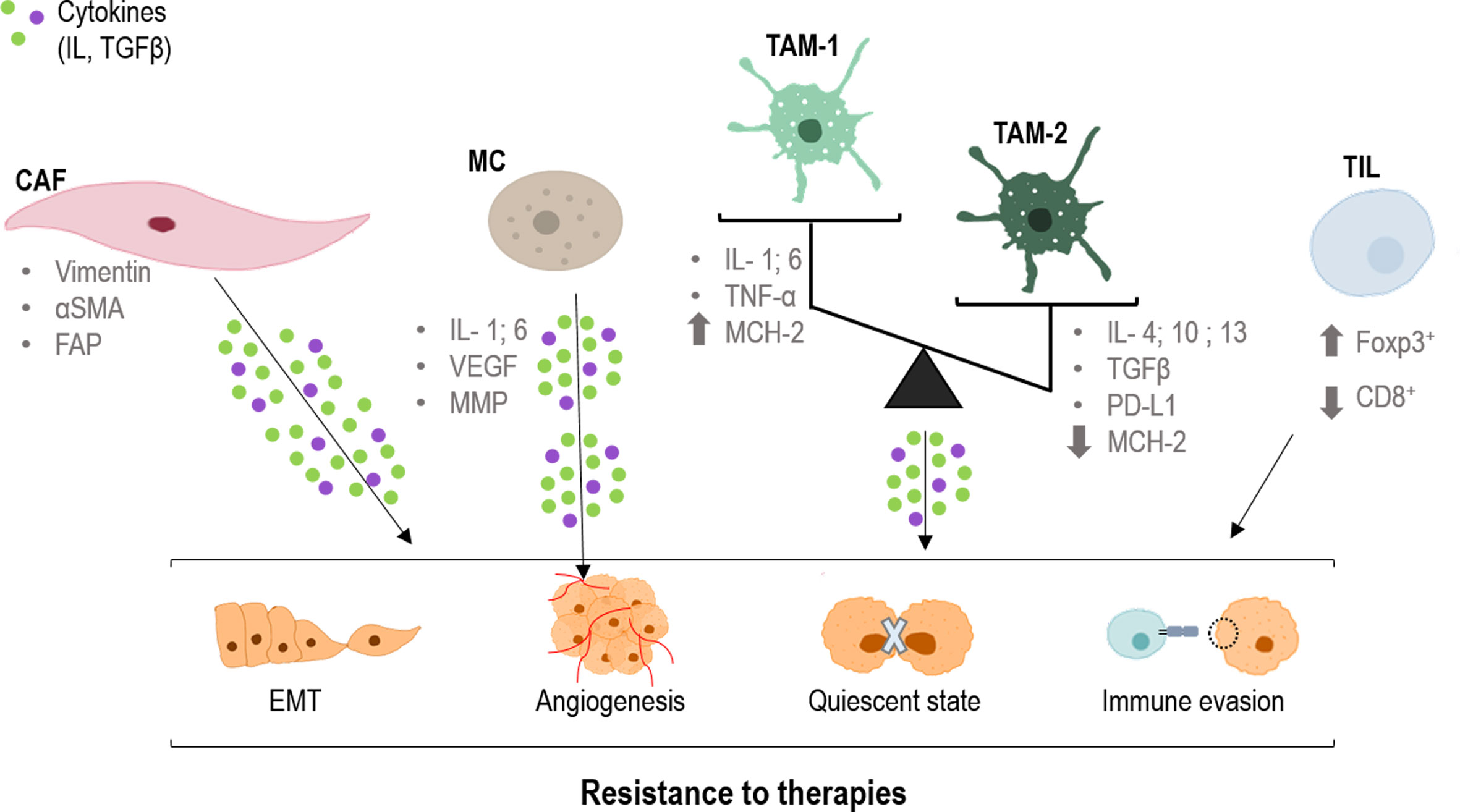
Figure 2 Role of CAFs, TAMs and TILs in the resistance to therapies. CAFs (cancer-associated fibroblast), TAMs (tumor-associated macrophages) and TILs (tumor infiltrated lymphocytes) produce cytokines that are taken up by tumor cells, producing changes in them. Epithelial mesenchymal transition (EMT), angiogenesis, quiescence and immune evasion, all factors that favor resistance to different treatments. CD8, cluster of differentiation 8; FAP, fibroblast activation protein; Foxp3, forkhead box P3; IL, interleukin; MCH-2, major histocompatibility complex 2; MMP, matrix metalloproteinases; PD-L1, programmed death-ligand 1; TGFβ, tumor growth factor; TNFα, tumor necrosis factor; VEGF, endothelial growth factor; α-SMA, smooth muscle actin alpha.
Related to these functional changes, the crosstalk between different CAF populations and tumoral cells has been long associated with resistance to therapies in different cancer types. In this sense, chemotherapy resistance (with compounds such as cisplatin, tamoxifen or gemcitabine) is the most studied one (22–25). One of the main mediators related to these resistance effects is TGFβ. This cytokine, in combination with many other molecules, plays a key role in CAF heterogeneity (26–30). In this sense, TGFβ modulates tumor progression and therapy response through CAF activation status, shape and invasiveness (31–33).
Within tumor progression, CAFs promote migration and invasion of tumor cells through a well-known process: the epithelial-mesenchymal transition (EMT) (34). In this regard, CAFs secrete a variety of activators that initiate the TGFβ cascade in epithelial tumor cells leading to a change in morphology and response, acquiring quasi-mesenchymal characteristics (35–40). On the other hand, not only CAFs modulate EMT but also tumor cells themselves through the expression of EMT factors that interact with the TGFβ signaling, such as FOXF2 or SOX9 (41–43) (Figure 2). Thus, the presence of these ones and similar markers constitutes an indubitable signal of malignant progression in several types of carcinomas (44, 45). Moreover, TGFβ secreted by CAFs also participates in the formation of vascular-like channels not only by endothelial cells, but also by tumor cells (46) (Figure 2).
On the other hand and as stated before, CAFs are able to modulate the response of tumoral cells to different therapies, generating resistance effects (47–53). Specifically, the main mechanism that prompts this phenomenon is the TGFβ-SMAD pathway, which can be modulated by different MAPKs (19, 54–58) More extensively, translocation of the SMAD complex to the nucleus targets specific genes such as p21 and p15, which are two potent inhibitors of the cyclin dependent kinases (CDKs), main regulators of the cell cycle (59–63). Thus, this provokes cell cycle arrest in the G1 phase as a consequence of the dysregulation of the G1/S checkpoint (57, 61, 64, 65). Consequently, this cell cycle arrest induces a dormant or quiescent state of tumoral cells which prevents their effective response to therapy, constituting an underlying mechanism of therapy resistance and tumor recurrence (66) (Figure 2). Moreover, p21 also stimulates the glutathione metabolism, which triggers an antioxidant response related to a drop in ROS levels (main effector in most conventional therapies) (53, 67). In this sense, the combination of therapies with compounds able to interrupt the TGFβ pathway could abrogate this cell cycle arrest, and hence, the resistance effect (66, 68).
Tumor associated macrophages
Macrophages are one of the principal types of immune cells in innate immunity. They display different roles in pathogen phagocytosis antigen presentation and tissue regeneration. Despite its multiple beneficial functions, in a carcinogenic environment its recruitment could be detrimental. Tumoral context could induce a polarization in these cells, towards M1 antitumoral macrophages or towards M2 protumoral macrophages (69). These specific macrophages are defined as tumor associated macrophages (TAMs), which interact, modulate and influence tumor progression, invasion and metastasis. The main difference between them two is that antitumor agents act by releasing cytokines that promote adaptive immunity (IL-1, IL-6, TNF-α, NO, etc.) and have a high expression of MCH-2 (major histocompatibility complex 2) molecules, allowing the presentation of tumor antigens to cells of the immune system. In contrast, pro-oncogenic macrophages act by releasing anti-inflammatory cytokines (IL-4, IL-13, IL-10, TGFβ etc.), overexpressing PD-L1 (Programmed death-ligand 1), and expressing few MCH-2 molecules (70) (Figure 2).
Tumor associated macrophages influence tumor progression through secretion of cytokines such as IL-10, INF-γ and CCL5. These cytokines trigger the activation of JAK1/STAT1/NF-κB/Notch1, JAK/STAT3 β-catenin/STAT3 and PI3K/AKT signaling pathways, modulating tumor progression, stemness and metastasis (58, 71, 72) (Figure 2). However, TAMs do not always contribute to tumor progression. The balance between M1 and M2 polarization of them is critical to predict the prognosis of the patient (73–75).
Apart from being predictor of poor prognostic, TAMs are related to poor response to chemotherapy generating a resistance effect in colorectal cancer and glioblastoma models (76, 77) and to immune checkpoint inhibitors in prostate cancer (78). The modulation of this kind of cells may lead to a better response and tumor remission. However, it is hard to understand how they produce this effect. The blockade of molecules as PD-L1 and Stat6 with different antibodies leads to TAMs reprogramming, enhancing antitumor activity (79–81). mTOR inhibitors like Rapamycin and Metformin show the ability to modulate TAMs, boosting other therapies and controlling tumor progression (82, 83). Other compounds such as Chloroquine and its derivatives are, as well, modulators of TAMs polarization through an antitumoral phenotype, sensitizing tumor cells to chemotherapy (84, 85).
Other immune cells present in the tumor microenvironment
Lymphocytes are the principal type of cell in adaptive immunity, they recognize specific antigens and produce a specific immune response. These cells are subdivided according to the expression of different markers such as CD3 (cluster of differentiation 3), CD25, CD4, CD8 or Foxp3 (forkhead box P3) exerting different responses against the pathogens (86). In a tumoral context, those tumor-interacting lymphocytes are defined as tumor infiltrated lymphocytes (TILs). TILs can modulate the development of the tumor depending on its features, which could be affected by the tumor at the same time. Specifically, high levels of cytotoxic CD8+ TILs infiltration tend to present antitumor activity and better outcomes for the patient (71, 87, 88). On the contrary, high levels of Foxp3+ regulatory T cells are strictly correlated with immunosuppression and pro-tumor activity triggering poor outcomes (89–91) (Figure 2).
B lymphocytes could also be part of the TME. It has been demonstrated that its presence is related with a higher CD8+ cells infiltration and, therefore, with a better prognosis (92, 93). However, this B cell appearance is not always beneficial, since these cells can also adopt a regulatory phenotype under the influence of myeloid-derived suppressor cells (MDSCs). Once with the regulatory phenotype, those cells produce cytokines with immunosuppressive effect, depleting CD8+ cells antitumor activity (94).
Myeloid-derived suppressor cells comprises a heterogeneous group of cells which have in common its derivation from immature myeloid cells and play an important role in cancer development, progression, invasion and setting of the pre-metastatic niche in different types of cancer (95). The protumoral activity derived from MDSCs is driven by several molecules present in the tumor as the macrophage migration inhibitory factor, B7 homolog 3 protein, also known as CD276, or signal transducer and activator of transcription 3 (STAT3) (96, 97). MDSCs could be modulated by different molecules and drugs in order to suppress their effects. Metformin, STAT 1 or the blockade of IL-6 and NLRP3 (NLR family pyrin domain containing 3) inflammasome can improve the clinical outcome and the prognosis of the patient (98–100).
Mast cell (MC) infiltration has been reported in a wide range of human and animal tumors particularly malignant melanoma and breast and colorectal cancer. The consequences of their presence in the TME remain unclear. Within the tumor, MC interactions occur with infiltrated immune cells, tumor cells, and ECM through direct cell-to-cell interactions or release of a broad range of mediators capable of remodeling the TME. MCs actively contribute to angiogenesis and induce neovascularization by releasing the classical proangiogenic factors including VEGF (vascular endothelial growth factor), FGF-2 (fibroblast growth factor), and IL-6, and nonclassical proangiogenic factors mainly proteases including tryptase and chymase (Figure 2). MCs may support tumor invasiveness by releasing a broad range of matrix metalloproteinases (MMPs) (101). 5-ALA-mediated PDT was associated with degranulation of MC and angiogenesis in oral premalignat lesions induced in rats (102).
Finally, the neutrophils also are associated with tumor progression. They are capable of establishing a pro-tumor microenvironment, being correlated to poor prognosis in some tumors such as lung cancer (103–105).
Extracellular matrix
The extracellular matrix is a key factor in carcinogenesis, which offers structural and biochemical support for cellular components lowing it to influence cell communication, adhesion and proliferation (106). It consists of a network of macromolecules, including collagen, fibronectin, laminin and glycosaminoglycans (107, 108). Alteration in ECM components may be the basis for the tumor progression. For example, the laminin receptor expression plays an important role in SCC progression (109, 110); the loss of type IV collagen correlates with the poorly differentiated SCC (111) and fibronectin mediates cellular interactions with the ECM and it is important in cell migration (112). Matrix metalloproteinases (MMPs) are critical molecules for the EMT process because they degrade cell adhesion molecules and cell-ECM interactions, which are produced by both tumor and immune cells (106, 107). Finally, the ECM is also modulated by the action of CAFs through the secretion of soluble factors such as cytokines (113).
It should be noted that there is an interconnection between the different components of the EMT. TAMs are capable of producing differential responses in TILs depending on their polarization (114, 115). These TAMs could also be influenced by regulatory T cells, promoting a protumoral phenotype via repression of CD8+ secreted INF-γ (116). Also, CAFs have demonstrated marked effects on macrophages, attracting them and polarizing them to protumoral TAMs (117–119). In addition, CAFs, TAMs and TILs are providers of pro-angiogenic factors such as VEGF for the formation of a complex vascular network to meet the metabolic and nutritional needs of tumors (120–122).
The tumor microenviroment as mediator of photodynamic resistance in non-melanoma skin cancer – Therapeutic opportunities
Photodynamic therapy not only targets neoplastic cells and tumor blood vessels, but also activates the immune system to induce inflammation and immune response to tumor cells (123). Although the intrinsic cellular mechanisms of resistance to PDT have been characterized, the emerging importance of the TME and the inflammatory/immune antitumor response is currently under investigation (123, 124). The specific role of CAFs and TAMs as an extrinsic mechanism of PDT resistance is attracting the attention for of researchers and is summarized in Table 1. Furthermore, although PDT has been extensively evaluated, both preclinically and clinically for the treatment of cancer cells, it has not been fully examined the concept of leveraging PDT to attack the tumor stroma in order to counteract therapeutic resistance induced by stromal signalling (149).
CAFs and PDT
Studies on the role of fibroblasts in the effectiveness of PDT in neoplastic cells are infrequent, and most of them have been evaluated in pancreatic cancer. Glidden et al. observed greater resistance to PDT when pancreatic ductal adenocarcinoma cells were co-cultured with normal fibroblasts for 7 days in 3D models (150), while Celli et al. did not observe effects from normal fibroblasts in the effectiveness of PDT in pancreatic cancer cells (149). Also, Chen et al. did not appreciate significant differences using conditioned culture medium of CAFs in 2D and 3D cultures (151). The role of CAF-derived TGFβ-1 as an extrinsic factor has been identified in different cancers, including cutaneous squamous cell carcinoma (cSCC) and basal cell carcinoma (BCC), the two main types of NMSC, in which it can induce resistance to different therapies (47, 132–134). Recently, our group investigated the role of TGFβ-1 in the resistance process to PDT in NMSC cells, showed that the TGFβ-1 secreted by CAFs of SCC is capable of conferring resistance to PDT with methyl aminolevulinate (MAL) through the induction of a quiescence state, postulating TGFβ-1 as a target for PDT optimization (47) (Figure 3A).
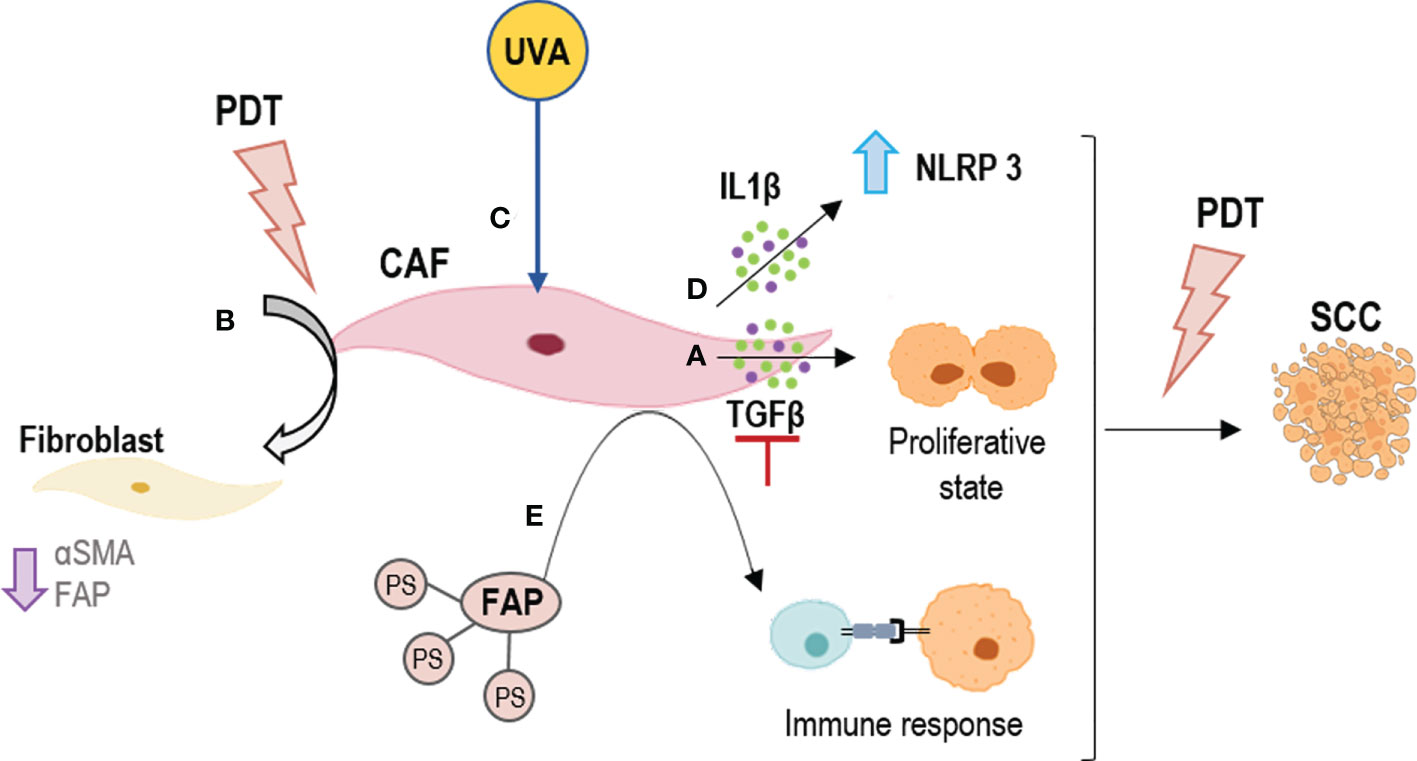
Figure 3 Strategies for outcome PDT resistance generated by CAFs. (A) To inhibit TGFβ secretion; (B) To revert the CAFs phenotype by PDT; (C) To boost PDT at dermal level by UVA light; (D) To activate NLRP3 inflammasomes by PDT to induce an inflammation response by IL1β; (E) To direct PDT to CAFs using their components as targets, such as FAPs. FAP, fibroblast activation protein; IL1β, Interleukin 1β; NLRP3, NLR family pyrin domain containing 3; TGFβ, tumor growth factor beta.
Furthermore, although the selectivity of PDT for tumor cells has been widely reported, the effect of ALA-PDT on CAFs is not well understood. However, it has been shown that alterations in the TME, such as the emergence of CAFs, are necessary for tumor progression to invasive and metastatic SCC, stages resistant to PDT (135). Li et al. indicated the effect of PDT with 5-aminolevulinic (ALA) on SCC-CAFs, showing a revers of the activation of CAFs (a reduction in α-SMA, FAP expression and migratory capacity of these cell types) (138) (Figure 3B). An interesting field of study is the effect of CAFs on genodermatoses that predispose to skin cancer such as Gorlin-Golz syndrome (GS) and Xeroderma pigmentosum (XP). Zamarron et al. investigated the overexpression of α-SMA and vinculin in fibroblast from GS and XP patients, observing an increase in the expression of both markers as compared to healthy fibroblasts and thus considering that primary fibroblasts obtained from these patients could be potential CAFs (152). PDT is an interesting treatment option in these two genodermatoses that achieves high clearance rates and excellent cosmetic outcomes probably due to the susceptibility of GS and XP fibroblasts to PDT (153). The fact that these fibroblasts behave as CAFs is likely contributing to malignancy in this genodermatoses. On the other hand, since they are highly susceptible to MAL-PDT, it suggests that this approach could not only be relevant to treat the epithelial component of tumors or premalignant lesions but also the activated stromal cells. One mechanism that Zamarron et al. have proposed to improve the efficacy of PDT is a priming response elicited by UVA light (the one with the capacity to reach the dermis) that may enhance the effect of MAL-PDT (152) (Figure 3C).
On the other hand, there are several investigations showing that CAFs are mediators of inflammation in squamous cell carcinogenesis through the IL1β secretion. This cytokine has a dual role of tumor promotion and suppression. In this sense, Nie et al. preliminarily demonstrated that the NLRP3 inflammasome mediating IL1β production in CAFs contributes to the PDT effect with ALA on SCC (136) (Figure 3D). Thus, modulating the inflammatory response and knowing well the expression of cytokines produced by CAFs could be a mechanism to avoid tumor resistance to PDT.
Besides the different mechanisms previously described to combat extrinsic resistance to PDT induced by the tumor stroma, other possible strategies have been investigated. Among the different mechanisms proposed, highlights the use of nanomedicine. Li et al. found that a single chain viable fragment (scFv, sequence specific to FAP) and the PS ZnF16Pc-loaded ferritin nanoparticles (scFv-Z@FRT) can mediate efficient and selective PDT, leading to eradication of CAFs in tumors (140) (Figure 3E). This strategy has proven to be a new and safe CAF-targeted therapy and a novel way to modulate TME in order to enhance immunity against cancer. This is due to CAFs regulate C-X-C motif chemokine ligand 12 (CXCL12) secretion and ECM deposition, preventing physical contact between T cells and cancer cells (137, 141).. Finally, another strategy to combat resistance to PDT is the use of drugs that have a synergistic and adjuvant effect, such as the combination of PDT and the multi-kinase inhibitor cabozantinib for extensive desmoplasia in pancreatic ductal adenocarcinoma, which frequently associates with treatment resistance. Blocking EMT phosphorylation with adjuvant cabozantinib caused a significant improvement in PDT efficacy with benzoporphyrin derivative, most notably by elevating spheroid necrosis at low radiant exposures (154). Even drugs with other therapeutic purposes such as metformin with promising effects in SCC cells resistant to MAL PDT, could increase the response to this treatment (139). Since metformin inhibits TGFβ-1-mediated EMT in breast cancer (155) and in carcinoma cells of the cervix through the inhibition of mTOR/p70s6k signalling (156). In non-small cell lung cancer, a recent study has shown that metformin exerts antitumor effects such as inhibition of proliferation and invasion as well as control of EMT through inhibition of NF-κB (Nuclear factor kappa-light-chain-enhancer of activated B cells) (157). Finally, CAFs have been reported to play a role in inhibiting apoptosis, among other factors (158). However, to date, there are no data on the part of these proteins and PDT resistance.
TAMs and PDT
Tumor associated macrophages also play complex but principal roles in the outcomes of PDT in malignant lesions Haga clic aquí para escribir texto.. The infiltration of M2 type TAMs to the neoplasm is associated with an increased risk of metastasis and tumor progression, due to the induction of angiogenesis and cell proliferation (159). The plasticity of TAMs can be used from a therapeutic point of view to generate more antitumor macrophages and destroy the neoplasm (70). TAMs have been shown to have a great capacity for the uptake of systemically administered PSs. Chan et al. used flow cytometry analysis and cell sorting to determine the content of a photosensitizer in the cellular fraction of a mouse colorectal carcinoma. Upon separating tumor-derived populations of high and low PS content, they identified macrophages among the cells with a high content (144). Korbelik et al. even showed that the amount of PS was greater in macrophages than in cells from the tumoral parenchyma (145). Macrophages M2 are selectively destroyed with PDT, and during the ensuing inflammatory reaction they are replaced with newly invading macrophages of M1 phenotype. Macrophages M1 have a principal role in the effect of PDT since they mediate in the removal of killed cancer cells and in the processing/presenting tumor antigens to T lymphocytes (160). The behavior of macrophages treated with PDT has been the subject of various studies. Macrophages treated with low doses of PDT seem to be activated and have greater activity, while those treated with higher doses lose their functionality (142). In addition, the macrophages treated with PDT generate a high amount of TNF-α, which entails the cytotoxic effect associated to this cytokine (146). Other mediators that are generated during PDT in the presence of TAMs and neoplastic cells are complement proteins (C3, C5 and C9), pentraxin, sphingolipids or toll-like receptors (TLR2, TLR4 and C3aR). All these molecules opsonize damaged tumor cells and facilitate phagocytosis by M1 TAMs (143).
The use of nanoparticles has become a strategy for the treatment of neoplasms. The development nanoparticles-based PDT against TAMs opens up new and more selective therapeutic pathways that can avoid resistance (161). TAMs express several receptors, including scavenger receptor A (SR-A), which can bind a variety of polyanionic ligands. Therefore, the conjugation of a PS with a polyanionic compound can act more selectively on them (162) (Figure 4A). Furthermore, alginate is a natural anionic polymer that has been combined with a PS known as phthalocyanine (1-[4-(2-aminoethyl) phenoxy]zinc(II) phthalocyanine) in a 0.1% gel presentation. The result is a low-cost photosensitizing molecule that is effective in vivo and in vitro against tumors of murine lines. Side effects were minimal and local, and the effectiveness over TAMs was high (147).
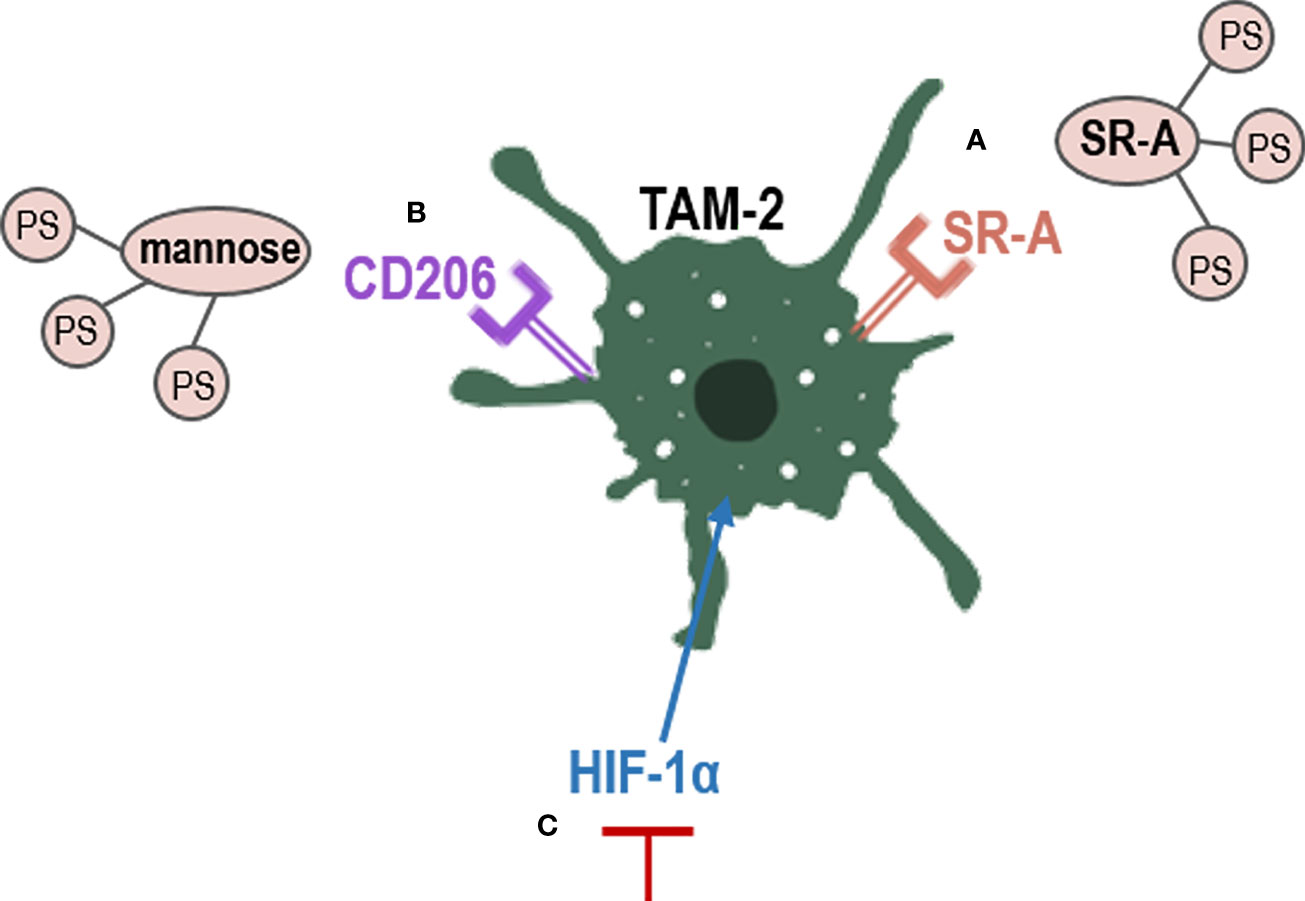
Figure 4 Strategies to eliminate TAM-2 by PDT. PS conjugation with TAMs receptors, such as (A) SR-A or (B) Manose receptors; (C) inhibition of HIF-1α, which promotes TAM-2. CD206, mannose receptor; HIF-1α, hypoxia factor 1; SR-A, scavenger receptor A; TAM-2, tumor associated macrophages 2.
Tumor associated macrophages overexpress CD206, a mannose receptor that is crucial for the role of macrophages in engulf, invasion and degradation of organism by endocytosis and phagocytosis (163). The use of mannose-conjugated chlorin (m-chlorin, photosensitizing substance) to target mannose receptors in TAMs has also been studied (Figure 4B). This PDT approach is a targeted therapy of TME TAMs to avoid their resistance, which induced a greater cell death in TAMs M2 (with mannose receptor) than TAMS M1 in cancer cells from the digestive tract (148). Finally, another strategy used to reduce TAMs-induced resistance is the use of curcumin as PS. Curcumin is a natural bioactive compound isolated from the rhizomes of Curcuma longa L., that exhibits great anti-tumor activity through the reduction of the levels of hypoxia factor 1 (HIF-1α) generated during PDT. HIF-1α promotes the M2 phenotype of macrophages and tumor survival, thus limiting the effectiveness of PDT (128) (Figure 4C). In the TME, uncontrolled cell proliferation avoid the ability to satisfy the oxygen demand from the preexisting blood vessels. Hypoxia has been found to directly regulate the expression of not only macrophages but also of other immune cells such as MDSCs (123, 127). In PDT, molecular oxygen is necessary, as a microenvironment of hypoxia could lead to treatment failure and drug resistance (126). Therefore, multiple nanomedicine-based strategies are being developed to circumvent hypoxia, such as hemoglobin oxygen carriers and cellular respiration inhibitions (129–131).
Conclusion
Cancer not only has a malignant epithelial component but also a stromal with various components, such as fibroblasts, endothelial and inflammatory cells, which form an appropriate TME to promote tumorigenesis, progression, and metastasis. TME components have been found to influence the processes of resistance to various treatments, and photodynamic therapy is not spared. Several studies have linked resistance to this treatment to the presence of cancer and/or macrophages-associated fibroblasts, the two major components of the tumor microenvironment. To date, the importance of TGFβ-1 in the resistance process to PDT in NMSC cells has been demonstrated, as CAFs induce tumor progression and tumor promotion by IL1β after PDT, avoiding physical contact between T cells and cancer cells by CXCL12 after PDT, or showing how high dose of PDT suppress macrophages activity.
Although different mechanisms to prevent resistance to PDT have already been studied (Table 1), more strategies are needed to target this component to inhibit the tumor growth and prevent resistance to PDT in NMSC. In this sense, strategies based on nanomedicine to enhance PDT, as well as new photosensitizers or nano-sized photosensitizer, and the use of combined treatments could contribute to the development of future perspectives. Furthermore, the mechanism by which photodynamic therapy may produce an inflammatory response that favors tumor remission and thus future recurrences should be extensively studied.
Author contributions
SG, YG, and AJ conceived the idea. PC, MM, MG-R, MA-B, JN-M, JS, and TG-C contributed to the preparation of manuscript and critically modified. MM and TG-C contributed in the preparation of figures. All authors contributed to the article and approved the submitted version.
Funding
The work was supported by Spanish grants from Instituto de Salud Carlos III MINECO and Feder Funds (PI18/00858; PI18/00708; PI21/00953 and PI21/00315).
Conflict of interest
The authors declare that the research was conducted in the absence of any commercial or financial relationships that could be construed as a potential conflict of interest.
Publisher’s note
All claims expressed in this article are solely those of the authors and do not necessarily represent those of their affiliated organizations, or those of the publisher, the editors and the reviewers. Any product that may be evaluated in this article, or claim that may be made by its manufacturer, is not guaranteed or endorsed by the publisher.
Glossary
References
1. Apalla Z, Lallas A, Sotiriou E, Lazaridou E, Ioannides D. Epidemiological trends in skin cancer. Dermatol Pract Concept (2017) 7:1–6. doi: 10.5826/dpc.0702a01
2. Ferlay J, Colombet M, Soerjomataram I, Mathers C, Parkin DM, Piñeros M, et al. Estimating the global cancer incidence and mortality in 2018: GLOBOCAN sources and methods. Int J Cancer (2019) 144:1941–53. doi: 10.1002/ijc.31937
3. Ríos L, Nagore E, López JL, Redondo P, Martí RM, Fernández-de-Misa R, et al. Melanoma characteristics at diagnosis from the Spanish national cutaneous melanoma registry: 15 years of experience. Actas Dermosifiliogr (2013) 104:789–99. doi: 10.1016/j.ad.2013.02.003
4. Juarranz Á, Jaén P, Sanz-Rodríguez F, Cuevas J, González S. Photodynamic therapy of cancer. basic principles and applications. Clin Transl Oncol (2008) 10:148–54. doi: 10.1007/s12094-008-0172-2
5. Shelton ME, Adamson AS. Review and update on evidence-based surgical treatment recommendations for nonmelanoma skin cancer. Dermatol Clin (2019) 37:425–33. doi: 10.1016/j.det.2019.05.002
6. Solban N, Ortel B, Pogue B, Hasan T. Optical imaging and photodynamic therapy. Ernst Schering Res Found Workshop (2005) 49:229–58. doi: 10.1007/3-540-26809-x_12
7. Wei R, Liu S, Zhang S, Min L, Zhu S. Cellular and extracellular components in tumor microenvironment and their application in early diagnosis of cancers. Anal Cell Pathol (2020) 2020:1–13. doi: 10.1155/2020/6283796
8. Farc O, Cristea V. An overview of the tumor microenvironment, from cells to complex networks (Review). Exp Ther Med (2020) 21:96. doi: 10.3892/etm.2020.9528
9. Baghban R, Roshangar L, Jahanban-Esfahlan R, Seidi K, Ebrahimi-Kalan A, Jaymand M, et al. Tumor microenvironment complexity and therapeutic implications at a glance. Cell Commun Signal (2020) 18:59. doi: 10.1186/s12964-020-0530-4
10. Wu T, Dai Y. Tumor microenvironment and therapeutic response. Cancer Lett (2017) 387:61–8. doi: 10.1016/j.canlet.2016.01.043
11. Thulabandu V, Chen D, Atit RP. Dermal fibroblast in cutaneous development and healing. WIREs Developm Biol (2018) 7:1–6. doi: 10.1002/wdev.307
12. Driskell RR, Watt FM. Understanding fibroblast heterogeneity in the skin. Trends Cell Biol (2015) 25:92–9. doi: 10.1016/j.tcb.2014.10.001
13. Addis R, Cruciani S, Santaniello S, Bellu E, Sarais G, Ventura C, et al. Fibroblast proliferation and migration in wound healing by phytochemicals: Evidence for a novel synergic outcome. Int J Med Sci (2020) 17:1030–42. doi: 10.7150/ijms.43986
14. Phan SH. Biology of fibroblasts and myofibroblasts. Proc Am Thorac Soc (2008) 5:334–7. doi: 10.1513/pats.200708-146DR
15. Ringuette Goulet C, Bernard G, Tremblay S, Chabaud S, Bolduc S, Pouliot F. Exosomes induce fibroblast differentiation into cancer-associated fibroblasts through TGFβ signaling. Mol Cancer Res (2018) 16:1196–204. doi: 10.1158/1541-7786.MCR-17-0784
16. Su S, Chen J, Yao H, Liu J, Yu S, Lao L, et al. CD10+GPR77+ cancer-associated fibroblasts promote cancer formation and chemoresistance by sustaining cancer stemness. Cell (2018) 172:841–56. doi: 10.1016/j.cell.2018.01.009
17. Yang X, Lin Y, Shi Y, Li B, Liu W, Yin W, et al. FAP promotes immunosuppression by cancer-associated fibroblasts in the tumor microenvironment via STAT3–CCL2 signaling. Cancer Res (2016) 76:4124–35. doi: 10.1158/0008-5472.CAN-15-2973
18. Smithmyer ME, Spohn JB, Kloxin AM. Probing fibroblast activation in response to extracellular cues with whole protein- or peptide-functionalized step-growth hydrogels. ACS Biomat Sci Eng (2018) 4:3304–16. doi: 10.1021/acsbiomaterials.8b00491
19. Itoh S. The transcriptional co-activator P/CAF potentiates TGF-beta/Smad signaling. Nucleic Acids Res (2000) 28:4291–8. doi: 10.1093/nar/28.21.4291
20. Grauel AL, Nguyen B, Ruddy D, Laszewski T, Schwartz S, Chang J, et al. TGFβ-blockade uncovers stromal plasticity in tumors by revealing the existence of a subset of interferon-licensed fibroblasts. Nat Commun (2020) 11:6315. doi: 10.1038/s41467-020-19920-5
21. Biffi G, Oni TE, Spielman B, Hao Y, Elyada E, Park Y, et al. IL1-induced JAK/STAT signaling is antagonized by TGFβ to shape CAF heterogeneity in pancreatic ductal adenocarcinoma. Cancer Discov (2019) 9:282–301. doi: 10.1158/2159-8290.CD-18-0710
22. Wei L, Ye H, Li G, Lu Y, Zhou Q, Zheng S, et al. Cancer-associated fibroblasts promote progression and gemcitabine resistance via the SDF-1/SATB-1 pathway in pancreatic cancer. Cell Death Dis (2018) 9:1065. doi: 10.1038/s41419-018-1104-x
23. Gao Y, Li X, Zeng C, Liu C, Hao Q, Li W, et al. CD63 + cancer-associated fibroblasts confer tamoxifen resistance to breast cancer cells through exosomal miR-22. Adv Sci (2020) 7:2002518. doi: 10.1002/advs.202002518
24. Ren J, Ding L, Zhang D, Shi G, Xu Q, Shen S, et al. Carcinoma-associated fibroblasts promote the stemness and chemoresistance of colorectal cancer by transferring exosomal lncRNA H19. Theranostics (2018) 8:3932–48. doi: 10.7150/thno.25541
25. Wang L, Li X, Ren Y, Geng H, Zhang Q, Cao L, et al. Cancer-associated fibroblasts contribute to cisplatin resistance by modulating ANXA 3 in lung cancer cells. Cancer Sci (2019) 110:1609–20. doi: 10.1111/cas.13998
26. Shangguan L, Ti X, Krause U, Hai B, Zhao Y, Yang Z, et al. Inhibition of TGF-β/Smad signaling by BAMBI blocks differentiation of human mesenchymal stem cells to carcinoma-associated fibroblasts and abolishes their protumor effects. Stem Cells (2012) 30:2810–9. doi: 10.1002/stem.1251
27. Melling GE, Flannery SE, Abidin SA, Clemmens H, Prajapati P, Hinsley EE, et al. A miRNA-145/TGF-β1 negative feedback loop regulates the cancer-associated fibroblast phenotype. Carcinogenesis (2018) 39:798–807. doi: 10.1093/carcin/bgy032
28. Kojima Y, Acar A, Eaton EN, Mellody KT, Scheel C, Ben-Porath I, et al. Autocrine TGF- and stromal cell-derived factor-1 (SDF-1) signaling drives the evolution of tumor-promoting mammary stromal myofibroblasts. Proc Nat Acad Sci (2010) 107:20009–14. doi: 10.1073/pnas.1013805107
29. Ishimoto T, Miyake K, Nandi T, Yashiro M, Onishi N, Huang KK, et al. Activation of transforming growth factor beta 1 signaling in gastric cancer-associated fibroblasts increases their motility, via expression of rhomboid 5 homolog 2, and ability to induce invasiveness of gastric cancer cells. Gastroenterology (2017) 153:191–204.e16. doi: 10.1053/j.gastro.2017.03.046
30. Bordignon P, Bottoni G, Xu X, Popescu AS, Truan Z, Guenova E, et al. Dualism of FGF and TGF-β signaling in heterogeneous cancer-associated fibroblast activation with ETV1 as a critical determinant. Cell Rep (2019) 28:2358–2372.e6. doi: 10.1016/j.celrep.2019.07.092
31. Stylianou A, Gkretsi V, Stylianopoulos T. Transforming growth factor-β modulates pancreatic cancer associated fibroblasts cell shape, stiffness and invasion. Biochim Biophys Acta Gen Subj (2018) 1862:1537–46. doi: 10.1016/j.bbagen.2018.02.009
32. Franco OE, Jiang M, Strand DW, Peacock J, Fernandez S, Jackson RS, et al. Altered TGF-β signaling in a subpopulation of human stromal cells promotes prostatic carcinogenesis. Cancer Res (2011) 71:1272–81. doi: 10.1158/0008-5472.CAN-10-3142
33. Tang X, Tu G, Yang G, Wang X, Kang L, Yang L, et al. Autocrine TGF-β1/miR-200s/miR-221/DNMT3B regulatory loop maintains CAF status to fuel breast cancer cell proliferation. Cancer Lett (2019) 452:79–89. doi: 10.1016/j.canlet.2019.02.044
34. Ghahremanifard P, Chanda A, Bonni S, Bose P. TGF-β mediated immune evasion in cancer–spotlight on cancer-associated fibroblasts. Cancers (Basel) (2020) 12:3650. doi: 10.3390/cancers12123650
35. Du X, Xu Q, Pan D, Xu D, Niu B, Hong W, et al. HIC-5 in cancer-associated fibroblasts contributes to esophageal squamous cell carcinoma progression. Cell Death Dis (2019) 10:873. doi: 10.1038/s41419-019-2114-z
36. Xiao L, Zhu H, Shu J, Gong D, Zheng D, Gao J. Overexpression of TGF-β1 and SDF-1 in cervical cancer-associated fibroblasts promotes cell growth, invasion and migration. Arch Gynecol Bstet (2022) 305:179–92. doi: 10.1007/s00404-021-06137-0
37. Miyazaki K, Togo S, Okamoto R, Idiris A, Kumagai H, Miyagi Y. Collective cancer cell invasion in contact with fibroblasts through integrin-α5β1/fibronectin interaction in collagen matrix. Cancer Sci (2020) 111:4381–92. doi: 10.1111/cas.14664
38. Yeung T-L, Leung CS, Wong K-K, Samimi G, Thompson MS, Liu J, et al. TGF-β modulates ovarian cancer invasion by upregulating CAF-derived versican in the tumor microenvironment. Cancer Res (2013) 73:5016–28. doi: 10.1158/0008-5472.CAN-13-0023
39. Tan H-X, Gong W-Z, Zhou K, Xiao Z-G, Hou F-T, Huang T, et al. CXCR4/TGF-β1 mediated hepatic stellate cells differentiation into carcinoma-associated fibroblasts and promoted liver metastasis of colon cancer. Cancer Biol Ther (2020) 21:258–68. doi: 10.1080/15384047.2019.1685157
40. Liu J, Chen S, Wang W, Ning B-F, Chen F, Shen W, et al. Cancer-associated fibroblasts promote hepatocellular carcinoma metastasis through chemokine-activated hedgehog and TGF-β pathways. Cancer Lett (2016) 379:49–59. doi: 10.1016/j.canlet.2016.05.022
41. Haga K, Yamazaki M, Maruyama S, Kawaharada M, Suzuki A, Hoshikawa E, et al. Crosstalk between oral squamous cell carcinoma cells and cancer-associated fibroblasts via the TGF-β/SOX9 axis in cancer progression. Transl Oncol (2021) 14:101236. doi: 10.1016/j.tranon.2021.101236
42. Mezawa Y, Daigo Y, Takano A, Miyagi Y, Yokose T, Yamashita T, et al. CD26 expression is attenuated by TGF-β and SDF-1 autocrine signaling on stromal myofibroblasts in human breast cancers. Cancer Med (2019) 8:3936–48. doi: 10.1002/cam4.2249
43. Lu J-T, Tan C-C, Wu X-R, He R, Zhang X, Wang Q-S, et al. FOXF2 deficiency accelerates the visceral metastasis of basal-like breast cancer by unrestrictedly increasing TGF-β and miR-182-5p. Cell Death Different (2020) 27:2973–87. doi: 10.1038/s41418-020-0555-7
44. Casey TM, Eneman J, Crocker A, White J, Tessitore J, Stanley M, et al. Cancer associated fibroblasts stimulated by transforming growth factor beta1 (TGF-β1) increase invasion rate of tumor cells: a population study. Breast Cancer Res Treat (2008) 110:39–49. doi: 10.1007/s10549-007-9684-7
45. Yu Y, Xiao C-H, Tan L-D, Wang Q-S, Li X-Q, Feng Y-M. Cancer-associated fibroblasts induce epithelial–mesenchymal transition of breast cancer cells through paracrine TGF-β signalling. Br J Cancer (2014) 110:724–32. doi: 10.1038/bjc.2013.768
46. Yang J, Lu Y, Lin Y-Y, Zheng Z-Y, Fang J-H, He S, et al. Vascular mimicry formation is promoted by paracrine TGF-β and SDF1 of cancer-associated fibroblasts and inhibited by miR-101 in hepatocellular carcinoma. Cancer Lett (2016) 383:18–27. doi: 10.1016/j.canlet.2016.09.012
47. Gallego-Rentero M, Gutiérrez-Pérez M, Fernández-Guarino M, Mascaraque M, Portillo-Esnaola M, Gilaberte Y, et al. TGFβ1 secreted by cancer-associated fibroblasts as an inductor of resistance to photodynamic therapy in squamous cell carcinoma cells. Cancers (Basel) (2021) 13:5613. doi: 10.3390/cancers13225613
48. Steins A, Ebbing EA, Creemers A, Zalm AP, Jibodh RA, Waasdorp C, et al. Chemoradiation induces epithelial-to-mesenchymal transition in esophageal adenocarcinoma. Int J Cancer (2019) 145:2792–803. doi: 10.1002/ijc.32364
49. Shan G, Gu J, Zhou D, Li L, Cheng W, Wang Y, et al. Cancer-associated fibroblast-secreted exosomal miR-423-5p promotes chemotherapy resistance in prostate cancer by targeting GREM2 through the TGF-β signaling pathway. Exp Mol Med (2020) 52:1809–22. doi: 10.1038/s12276-020-0431-z
50. Yegodayev KM, Novoplansky O, Golden A, Prasad M, Levin L, Jagadeeshan S, et al. TGF-Beta-Activated cancer-associated fibroblasts limit cetuximab efficacy in preclinical models of head and neck cancer. Cancers (Basel) (2020) 12:339. doi: 10.3390/cancers12020339
51. Katsuno Y, Meyer DS, Zhang Z, Shokat KM, Akhurst RJ, Miyazono K, et al. Chronic TGF-β exposure drives stabilized EMT, tumor stemness, and cancer drug resistance with vulnerability to bitopic mTOR inhibition. Sci Signal (2019) 12:aau8544. doi: 10.1126/scisignal.aau8544
52. Xu X, Zhang L, He X, Zhang P, Sun C, Xu X, et al. TGF-β plays a vital role in triple-negative breast cancer (TNBC) drug-resistance through regulating stemness, EMT and apoptosis. Biochem Biophys Res Commun (2018) 502:160–5. doi: 10.1016/j.bbrc.2018.05.139
53. Brown JA, Yonekubo Y, Hanson N, Sastre-Perona A, Basin A, Rytlewski JA, et al. TGF-β-Induced quiescence mediates chemoresistance of tumor-propagating cells in squamous cell carcinoma. Cell Stem Cell (2017) 21:650–64. doi: 10.1016/j.stem.2017.10.001
54. Li Q, Zhang D, Wang Y, Sun P, Hou X, Larner J, et al. MiR-21/Smad 7 signaling determines TGF-β1-induced CAF formation. Sci Rep (2013) 3:2038. doi: 10.1038/srep02038
55. Meng W, Xia Q, Wu L, Chen S, He X, Zhang L, et al. Downregulation of TGF-beta receptor types II and III in oral squamous cell carcinoma and oral carcinoma-associated fibroblasts. BMC Cancer (2011) 11:88. doi: 10.1186/1471-2407-11-88
56. Busch S, Acar A, Magnusson Y, Gregersson P, Rydén L, Landberg G. TGF-beta receptor type-2 expression in cancer-associated fibroblasts regulates breast cancer cell growth and survival and is a prognostic marker in pre-menopausal breast cancer. Oncogene (2015) 34:27–38. doi: 10.1038/onc.2013.527
57. Zhao J, Li G, Wei J, Dang S, Yu X, Ding L, et al. Ellagic acid induces cell cycle arrest and apoptosis via the TGF β1/Smad3 signaling pathway in human colon cancer HCT 116 cells. Oncol Rep (2020) 44:768–76. doi: 10.3892/or.2020.7617
58. Yang H-L, Thiyagarajan V, Shen P-C, Mathew DC, Lin K-Y, Liao J-W, et al. Anti-EMT properties of CoQ0 attributed to PI3K/AKT/NFKB/MMP-9 signaling pathway through ROS-mediated apoptosis. J Exp Clin Cancer Res (2019) 38:186. doi: 10.1186/s13046-019-1196-x
59. Patel S, Tang J, Overstreet JM, Anorga S, Lian F, Arnouk A, et al. Rac-GTPase promotes fibrotic TGF-β1 signaling and chronic kidney disease via EGFR, p53, and Hippo/YAP/TAZ pathways. FASEB J (2019) 33:9797–810. doi: 10.1096/fj.201802489RR
60. Nataraj SE, Blain SW. A cyclin d-CDK6 dimer helps to reshuffle cyclin-dependent kinase inhibitors (CKI) to overcome TGF-beta-mediated arrest and maintain CDK2 activity. Cell Cycle (2021) 20:808–18. doi: 10.1080/15384101.2021.1909261
61. Kim S-C, Kang J-I, Hyun J-W, Kang J-H, Koh Y-S, Kim Y-H, et al. Methylhonokiol protects HaCaT cells from TGF-β1-Induced cell cycle arrest by regulating canonical and non-canonical pathways of TGF-β signaling. Biomol Therap (2017) 25:417–26. doi: 10.4062/biomolther.2016.003
62. Melzer C, von der Ohe J, Hass R, Ungefroren H. TGF-β-Dependent growth arrest and cell migration in benign and malignant breast epithelial cells are antagonistically controlled by Rac1 and Rac1b. Int J Mol Sci (2017) 18:1574. doi: 10.3390/ijms18071574
63. Chatterjee A, Mukhopadhyay S, Tung K, Patel D, Foster DA. Rapamycin-induced G1 cell cycle arrest employs both TGF-β and Rb pathways. Cancer Lett (2015) 360:134–40. doi: 10.1016/j.canlet.2015.01.043
64. Ren Y, Geng R, Lu Q, Tan X, Rao R, Zhou H, et al. Involvement of TGF-β and ROS in G1 cell cycle arrest induced by titanium dioxide nanoparticles under UVA irradiation in a 3D spheroid model. Int J Nanom (2020) 15:1997–2010. doi: 10.2147/IJN.S238145
65. Kim S, Seo Y, Chowdhury T, Yu HJ, Lee CE, Kim K-M, et al. Inhibition of MUC1 exerts cell-cycle arrest and telomerase suppression in glioblastoma cells. Sci Rep (2020) 10:18238. doi: 10.1038/s41598-020-75457-z
66. Huang T, Song X, Xu D, Tiek D, Goenka A, Wu B, et al. Stem cell programs in cancer initiation, progression, and therapy resistance. Theranostics (2020) 10:8721–43. doi: 10.7150/thno.41648
67. Bui AT, Laurent F, Havard M, Dautry F, Tchénio T. SMAD signaling and redox imbalance cooperate to induce prostate cancer cell dormancy. Cell Cycle (2015) 14:1218–31. doi: 10.1080/15384101.2015.1014145
68. de Streel G, Bertrand C, Chalon N, Liénart S, Bricard O, Lecomte S, et al. Selective inhibition of TGF-β1 produced by GARP-expressing tregs overcomes resistance to PD-1/PD-L1 blockade in cancer. Nat Commun (2020) 11:4545. doi: 10.1038/s41467-020-17811-3
69. Mehla K, Singh PK. Metabolic regulation of macrophage polarization in cancer. Trends Cancer (2019) 5:822–34. doi: 10.1016/j.trecan.2019.10.007
70. Anfray, Ummarino, Andón, Allavena. Current strategies to target tumor-Associated-Macrophages to improve anti-tumor immune responses. Cells (2019) 9:46. doi: 10.3390/cells9010046
71. Zhang X, Zeng Y, Qu Q, Zhu J, Liu Z, Ning W, et al. PD-L1 induced by IFN-γ from tumor-associated macrophages via the JAK/STAT3 and PI3K/AKT signaling pathways promoted progression of lung cancer. Int J Clin Oncol (2017) 22:1026–33. doi: 10.1007/s10147-017-1161-7
72. Huang R, Wang S, Wang N, Zheng Y, Zhou J, Yang B, et al. CCL5 derived from tumor-associated macrophages promotes prostate cancer stem cells and metastasis via activating β-catenin/STAT3 signaling. Cell Death Dis (2020) 11:234. doi: 10.1038/s41419-020-2435-y
73. Bao X, Shi R, Zhao T, Wang Y, Anastasov N, Rosemann M, et al. Integrated analysis of single-cell RNA-seq and bulk RNA-seq unravels tumour heterogeneity plus M2-like tumour-associated macrophage infiltration and aggressiveness in TNBC. Cancer Immunol Immunoth (2021) 70:189–202. doi: 10.1007/s00262-020-02669-7
74. Agarbati S, Mascitti M, Paolucci E, Togni L, Santarelli A, Rubini C, et al. Prognostic relevance of macrophage phenotypes in high-grade oral tongue squamous cell carcinomas. App Immunohistochem Mol Morphol (2021) 29:359–65. doi: 10.1097/PAI.0000000000000867
75. Dumars C, Ngyuen J-M, Gaultier A, Lanel R, Corradini N, Gouin F, et al. Dysregulation of macrophage polarization is associated with the metastatic process in osteosarcoma. Oncotarget (2016) 7:78343–54. doi: 10.18632/oncotarget.13055
76. Perrotta C, Cervia D, di Renzo I, Moscheni C, Bassi MT, Campana L, et al. Nitric oxide generated by tumor-associated macrophages is responsible for cancer resistance to cisplatin and correlated with syntaxin 4 and acid sphingomyelinase inhibition. Front Immunol (2018) 9:1186. doi: 10.3389/fimmu.2018.01186
77. Yin Y, Yao S, Hu Y, Feng Y, Li M, Bian Z, et al. The immune-microenvironment confers chemoresistance of colorectal cancer through macrophage-derived IL6. Clin Cancer Res (2017) 23:7375–87. doi: 10.1158/1078-0432.CCR-17-1283
78. JiaWei Z, ChunXia D, CunDong L, Yang L, JianKun Y, HaiFeng D, et al. M2 subtype tumor associated macrophages (M2-TAMs) infiltration predicts poor response rate of immune checkpoint inhibitors treatment for prostate cancer. Ann Med (2021) 53:730–40. doi: 10.1080/07853890.2021.1924396
79. Hartley GP, Chow L, Ammons DT, Wheat WH, Dow SW. Programmed cell death ligand 1 (PD-L1) signaling regulates macrophage proliferation and activation. Cancer Immunol Res (2018) 6:1260–73. doi: 10.1158/2326-6066.CIR-17-0537
80. Xiao H, Guo Y, Li B, Li X, Wang Y, Han S, et al. M2-like tumor-associated macrophage-targeted codelivery of STAT6 inhibitor and IKKβ siRNA induces M2-to-M1 repolarization for cancer immunotherapy with low immune side effects. ACS Cent Sci (2020) 6:1208–22. doi: 10.1021/acscentsci.9b01235
81. Binnemars-Postma K, Bansal R, Storm G, Prakash J. Targeting the Stat6 pathway in tumor-associated macrophages reduces tumor growth and metastatic niche formation in breast cancer. FASEB J (2018) 32:969–78. doi: 10.1096/fj.201700629R
82. Chen B, Gao A, Tu B, Wang Y, Yu X, Wang Y, et al. Metabolic modulation via mTOR pathway and anti-angiogenesis remodels tumor microenvironment using PD-L1-targeting codelivery. Biomaterials (2020) 255:120187. doi: 10.1016/j.biomaterials.2020.120187
83. Wei Z, Zhang X, Yong T, Bie N, Zhan G, Li X, et al. Boosting anti-PD-1 therapy with metformin-loaded macrophage-derived microparticles. Nat Commun (2021) 12:440. doi: 10.1038/s41467-020-20723-x
84. Chen D, Xie J, Fiskesund R, Dong W, Liang X, Lv J, et al. Chloroquine modulates antitumor immune response by resetting tumor-associated macrophages toward M1 phenotype. Nat Commun (2018) 9:873. doi: 10.1038/s41467-018-03225-9
85. Li Y, Cao F, Li M, Li P, Yu Y, Xiang L, et al. Hydroxychloroquine induced lung cancer suppression by enhancing chemo-sensitization and promoting the transition of M2-TAMs to M1-like macrophages. J Exp Clin Cancer Res (2018) 37:259. doi: 10.1186/s13046-018-0938-5
86. Abbas AK, Lichtman AH, Pillai S. Cellular and molecular immunology e-book. Elsevier (2014) 1:1–50.
87. Woolaver RA, Wang X, Krinsky AL, Waschke BC, Chen SMY, Popolizio V, et al. Differences in TCR repertoire and T cell activation underlie the divergent outcomes of antitumor immune responses in tumor-eradicating versus tumor-progressing hosts. J Immunother Cancer (2021) 9:e001615. doi: 10.1136/jitc-2020-001615
88. Gurin D, Slavik M, Hermanova M, Selingerova I, Kazda T, Hendrych M, et al. The tumor immune microenvironment and its implications for clinical outcome in patients with oropharyngeal squamous cell carcinoma. J Oral Pathol Med (2020) 49:886–96. doi: 10.1111/jop.13055
89. Qiao B, Huang J, Mei Z, Lam AK, Zhao J, Ying L. Analysis of immune microenvironment by multiplex immunohistochemistry staining in different oral diseases and oral squamous cell carcinoma. Front Oncol (2020) 10:555757. doi: 10.3389/fonc.2020.555757
90. Zhang S, Bai W, Tong X, Bu P, Xu J, Xi Y. Correlation between tumor microenvironment associated factors and the efficacy and prognosis of neoadjuvant therapy for rectal cancer. Oncol Lett (2018) 17:10621070. doi: 10.3892/ol.2018.9682
91. Li W, Xu M, Li Y, Huang Z, Zhou J, Zhao Q, et al. Comprehensive analysis of the association between tumor glycolysis and immune/inflammation function in breast cancer. J Transl Med (2020) 18:92. doi: 10.1186/s12967-020-02267-2
92. Hladíková K, Koucký V, Bouček J, Laco J, Grega M, Hodek M, et al. Tumor-infiltrating b cells affect the progression of oropharyngeal squamous cell carcinoma via cell-to-cell interactions with CD8+ T cells. J Immunother Cancer (2019) 7:261. doi: 10.1186/s40425-019-0726-6
93. Sato J, Kitano S, Motoi N, Ino Y, Yamamoto N, Watanabe S, et al. CD20+ tumor-infiltrating immune cells and CD204+ M2 macrophages are associated with prognosis in thymic carcinoma. Cancer Sci (2020) 111:1921–32. doi: 10.1111/cas.14409
94. Lee-Chang C, Rashidi A, Miska J, Zhang P, Pituch KC, Hou D, et al. Myeloid-derived suppressive cells promote b cell–mediated immunosuppression via transfer of PD-L1 in glioblastoma. Cancer Immunol Res (2019) 7:1928–43. doi: 10.1158/2326-6066.CIR-19-0240
95. Pang X, Fan H, Tang Y, Wang S, Cao M, Wang H, et al. Myeloid derived suppressor cells contribute to the malignant progression of oral squamous cell carcinoma. PloS One (2020) 15:e0229089. doi: 10.1371/journal.pone.0229089
96. Zhou Y, Zhang G, Zhang W, Wei X, Hou J, Huang Y. B7-H3 promotes prostate cancer progression in mice by antagonizing myeloid-derived suppressor cell apoptosis. Technol Cancer Res Treat (2020) 19:153303382097164. doi: 10.1177/1533033820971649
97. Trovato R, Fiore A, Sartori S, Canè S, Giugno R, Cascione L, et al. Immunosuppression by monocytic myeloid-derived suppressor cells in patients with pancreatic ductal carcinoma is orchestrated by STAT3. J Immunother Cancer (2019) 7:255. doi: 10.1186/s40425-019-0734-6
98. Lu F, Zhao Y, Pang Y, Ji M, Sun Y, Wang H, et al. NLRP3 inflammasome upregulates PD-L1 expression and contributes to immune suppression in lymphoma. Cancer Lett (2021) 497:178–89. doi: 10.1016/j.canlet.2020.10.024
99. Caetano MS, Zhang H, Cumpian AM, Gong L, Unver N, Ostrin EJ, et al. IL6 blockade reprograms the lung tumor microenvironment to limit the development and progression of k-ras–mutant lung cancer. Cancer Res (2016) 76:3189–99. doi: 10.1158/0008-5472.CAN-15-2840
100. Uehara T, Eikawa S, Nishida M, Kunisada Y, Yoshida A, Fujiwara T, et al. Metformin induces CD11b+-cell-mediated growth inhibition of an osteosarcoma: implications for metabolic reprogramming of myeloid cells and anti-tumor effects. Int Immunol (2019) 31:187–98. doi: 10.1093/intimm/dxy079
101. Komi DEA, Redegeld FA. Role of mast cells in shaping the tumor microenvironment. Clin Rev Allergy Immunol (2020) 58:313–25. doi: 10.1007/s12016-019-08753-w
102. Rosin FC, Barcessat AR, Borges GG, Corrêa L, J Photochem Photobiol B. Effect of 5-ALA-mediated photodynamic therapy on mast cell and microvessels densities present in oral premalignant lesions induced in rats. J Photochem Photobiol B (2015) 153:429–34. doi: 10.1016/j.jphotobiol.2015.10.027
103. Zhang C, Tang B, Hu J, Fang X, Bian H, Han J, et al. Neutrophils correlate with hypoxia microenvironment and promote progression of non-small-cell lung cancer. Bioengineered (2021) 12:8872–84. doi: 10.1080/21655979.2021.1987820
104. Faget J, Groeneveld S, Boivin G, Sankar M, Zangger N, Garcia M, et al. Neutrophils and snail orchestrate the establishment of a pro-tumor microenvironment in lung cancer. Cell Rep (2017) 21:3190–204. doi: 10.1016/j.celrep.2017.11.052
105. Liu K, Zhao K, Wang L, Sun E. The prognostic values of tumor-infiltrating neutrophils, lymphocytes and neutrophil/lymphocyte rates in bladder urothelial cancer. Pathol Res Pract (2018) 214:1074–80. doi: 10.1016/j.prp.2018.05.010
106. Walker C, Mojares E, del Río Hernández A. Role of extracellular matrix in development and cancer progression. Int J Mol Sci (2018) 19:3028. doi: 10.3390/ijms19103028
107. Xiong G-F, Xu R. Function of cancer cell-derived extracellular matrix in tumor progression. J Cancer Met Treat (2016) 2:357. doi: 10.20517/2394-4722.2016.08
108. Lu P, Weaver VM, Werb Z. The extracellular matrix: A dynamic niche in cancer progression. J Cell Biol (2012) 196:395–406. doi: 10.1083/jcb.201102147
109. Zhang K, Kramer RH. Laminin 5 deposition promotes keratinocyte motility. Exp Cell Res (1996) 227:309–22. doi: 10.1006/excr.1996.0280
110. Janes SM, Watt FM. New roles for integrins in squamous-cell carcinoma. Nat Rev Cancer (2006) 6:175–83. doi: 10.1038/nrc1817
111. Agarwal P, Ballabh R. Expression of type IV collagen in different histological grades of oral squamous cell carcinoma: An immunohistochemical study. J Cancer Res Ther (2013) 9:272. doi: 10.4103/0973-1482.113382
112. Scanlon CS, van Tubergen EA, Inglehart RC, D’Silva NJ. Biomarkers of epithelial-mesenchymal transition in squamous cell carcinoma. J Dent Res (2013) 92:114–21. doi: 10.1177/0022034512467352
113. Linares J, Marín-Jiménez JA, Badia-Ramentol J, Calon A. Determinants and functions of CAFs secretome during cancer progression and therapy. Front Cell Dev Biol (2021) 8:621070. doi: 10.3389/fcell.2020.621070
114. Kuroda H, Jamiyan T, Yamaguchi R, Kakumoto A, Abe A, Harada O, et al. Tumor microenvironment in triple-negative breast cancer: the correlation of tumor-associated macrophages and tumor-infiltrating lymphocytes. Clin Transl Oncol (2021) 23:2513–25. doi: 10.1007/s12094-021-02652-3
115. Petty AJ, Dai R, Lapalombella R, Baiocchi RA, Benson DM, Li Z, et al. Hedgehog-induced PD-L1 on tumor-associated macrophages is critical for suppression of tumor-infiltrating CD8+ T cell function. JCI Insight (2021) 6:e146707. doi: 10.1172/jci.insight.146707
116. Liu C, Chikina M, Deshpande R, v. MA, Wang T, Tabib T, et al. Treg cells promote the SREBP1-dependent metabolic fitness of tumor-promoting macrophages via repression of CD8+ T cell-derived interferon-γ. Immunity (2019) 51:381–97. doi: 10.1016/j.immuni.2019.06.017
117. Gok Yavuz B, Gunaydin G, Gedik ME, Kosemehmetoglu K, Karakoc D, Ozgur F, et al. Cancer associated fibroblasts sculpt tumour microenvironment by recruiting monocytes and inducing immunosuppressive PD-1+ TAMs. Sci Rep (2019) 9:3172. doi: 10.1038/s41598-019-39553-z
118. Takahashi H, Sakakura K, Kudo T, Toyoda M, Kaira K, Oyama T, et al. Cancer-associated fibroblasts promote an immunosuppressive microenvironment through the induction and accumulation of protumoral macrophages. Oncotarget (2017) 8:8633–47. doi: 10.18632/oncotarget.14374
119. Stadler M, Pudelko K, Biermeier A, Walterskirchen N, Gaigneaux A, Weindorfer C, et al. Stromal fibroblasts shape the myeloid phenotype in normal colon and colorectal cancer and induce CD163 and CCL2 expression in macrophages. Cancer Lett (2021) 520:184–200. doi: 10.1016/j.canlet.2021.07.006
120. Pietras K, Östman A. Hallmarks of cancer: Interactions with the tumor stroma. Exp Cell Res (2010) 316:1324–31. doi: 10.1016/j.yexcr.2010.02.045
121. Larionova I, Kazakova E, Gerashchenko T, Kzhyshkowska J. New angiogenic regulators produced by TAMs: Perspective for targeting tumor angiogenesis. Cancers (Basel) (2021) 13:3253. doi: 10.3390/cancers13133253
122. König L, Mairinger FD, Hoffmann O, Bittner A-K, Schmid KW, Kimmig R, et al. Dissimilar patterns of tumor-infiltrating immune cells at the invasive tumor front and tumor center are associated with response to neoadjuvant chemotherapy in primary breast cancer. BMC Cancer (2019) 19:120. doi: 10.1186/s12885-019-5320-2
123. Zhang M, Zhao Y, Ma H, Sun Y, Cao J. How to improve photodynamic therapy-induced antitumor immunity for cancer treatment? Theranostics (2022) 12:4629. doi: 10.7150/thno.72465
124. Sorrin AJ, Kemal Ruhi M, Ferlic NA, Karimnia V, Polacheck WJ, Celli JP, et al. Photodynamic therapy and the biophysics of the tumor microenvironment. Photochem Photobiol (2020) 96:232–59. doi: 10.1111/php.13209
125. Wan Y, Fu LH, Li C, Lin J, Huang P. Conquering the hypoxia limitation for photodynamic therapy. Adv Mat (2021) 33:2103978. doi: 10.1002/adma.202103978
126. Jahanban-Esfahlan R, de la Guardia M, Ahmadi D, Yousefi B. Modulating tumor hypoxia by nanomedicine for effective cancer therapy. J Cell Physiol (2018) 233:2019–31. doi: 10.1002/jcp.25859
127. Kumar V, Gabrilovich DI. Hypoxia-inducible factors in regulation of immune responses in tumor microenvironment. Immunology (2014) 143:512–9. doi: 10.1111/imm.12380
128. Fu X, He Y, Li M, Huang Z, Najafi M. Targeting of the tumor microenvironment by curcumin. BioFactors (2021) 47:914–32. doi: 10.1002/biof.1776
129. Sun Y, Zhao D, Wang G, Wang Y, Cao L, Sun J, et al. Recent progress of hypoxia-modulated multifunctional nanomedicines to enhance photodynamic therapy: opportunities, challenges, and future development. Acta Pharm Sin B (2020) 10:1382–96. doi: 10.1016/j.apsb.2020.01.004
130. Zhao M, Yang X, Fu H, Chen C, Zhang Y, Wu Z, et al. Immune/hypoxic tumor microenvironment regulation-enhanced photodynamic treatment realized by pH-responsive phase transition-targeting nanobubbles. ACS Appl Mat Interfaces (2021) 13:32763–79. doi: 10.1021/acsami.1c07323
131. Guo X, Qu J, Zhu C, Li W, Luo L, Yang J, et al. Synchronous delivery of oxygen and photosensitizer for alleviation of hypoxia tumor microenvironment and dramatically enhanced photodynamic therapy. Drug Delivery (2018) 25:585–99. doi: 10.1080/10717544.2018.1435751
132. Guo S, Deng C-X. Effect of stromal cells in tumor microenvironment on metastasis initiation. Int J Biol Sci (2018) 14:2083–93. doi: 10.7150/ijbs.25720
133. Omland SH, Wettergren EE, Mollerup S, Asplund M, Mourier T, Hansen AJ, et al. Cancer associated fibroblasts (CAFs) are activated in cutaneous basal cell carcinoma and in the peritumoral skin. BMC Cancer (2017) 17:1–9. doi: 10.1186/s12885-017-3663-0
134. Peltanova B, Raudenska M, Masarik M. Effect of tumor microenvironment on pathogenesis of the head and neck squamous cell carcinoma: a systematic review. Mol Cancer (2019) 18:63. doi: 10.1186/s12943-019-0983-5
135. Nissinen L, Farshchian M, Riihilä P, Kähäri VM. New perspectives on role of tumor microenvironment in progression of cutaneous squamous cell carcinoma. Cell Tissue Res (2016) 365:691–702. doi: 10.1007/s00441-016-2457-z
136. Nie S, Wang X, Wang H. NLRP3 inflammasome mediated interleukin-1β production in cancer-associated fibroblast contributes to ALA-PDT for cutaneous squamous cell carcinoma. Cancer Manag Res (2019) 11:10257–67. doi: 10.2147/CMAR.S226356
137. Zhen Z, Tang W, Wang M, Zhou S, Wang H, Wu Z, et al. Protein nanocage mediated fibroblast-activation protein targeted photoimmunotherapy to enhance cytotoxic T cell infiltration and tumor control. Nano Lett (2017) 17:862–9. doi: 10.1021/acs.nanolett.6b04150
138. Li S, Wang P, Zhang G, Ji J, Lv T, Wang X, et al. The effect of ALA-PDT on reversing the activation of cancer-associated fibroblasts in cutaneous squamous cell carcinoma. Photodiagnosis Photod Ther (2019) 27:234–40. doi: 10.1016/j.pdpdt.2019.05.043
139. Mascaraque-Checa M, Gallego-Rentero M, Nicolás-Morala J, Portillo-Esnaola M, Cuezva JM, González S, et al. Metformin overcomes metabolic reprogramming-induced resistance of skin squamous cell carcinoma to photodynamic therapy. Mol Metab (2022) 60:101496. doi: 10.1016/j.molmet.2022.101496
140. Li L, Zhou S, Lv N, Zhen Z, Liu T, Gao S, et al. Photosensitizer-encapsulated ferritins mediate photodynamic therapy against cancer-associated fibroblasts and improve tumor accumulation of nanoparticles. Mol Pharm (2018) 15:3595–9. doi: 10.1021/acs.molpharmaceut.8b00419
141. Yao H, Xu K, Zhou J, Zhou L, Wei S. A tumor microenvironment destroyer for efficient cancer suppression. ACS Biom Sci Engin (2020) 6:450–62. doi: 10.1021/acsbiomaterials.9b01544
142. Marshall JF, Chan W-S, Hart IR. Effect of photodynamic therapy on anti-tumor immune defenses: comparison of the photosensitizers hematoporphyrin derivative and chloro-aluminum sulfonated phthalocyanine. Photochem Photobiol (1989) 49:627–32. doi: 10.1111/j.1751-1097.1989.tb08434.x
143. Korbelik M. Complement upregulation in photodynamic therapy-treated tumors: Role of toll-like receptor pathway and NFκB. Cancer Lett (2009) 281:232–8. doi: 10.1016/j.canlet.2009.02.049
144. Chan WS, Marshall JF, Lam GY, Hart IR. Tissue uptake, distribution, and potency of the photoactivatable dye chloroaluminum sulfonated phthalocyanine in mice bearing transplantable tumors. Cancer Res (1988) 48:3040–4.
145. Korbelik M, Krosl G. Photofrin accumulation in malignant and host cell populations of various tumours. Br J Cancer (1996) 73:506–13. doi: 10.1038/bjc.1996.88
146. Evans S, Matthews W, Perry R, Fraker D, Norton J, Pass HI. Effect of photodynamic therapy on tumor necrosis factor production by murine macrophages. J Nat Cancer Inst (1990) 82:34–9. doi: 10.1093/jnci/82.1.34
147. Tang F, Xie W, Li S, Hu Q, Zheng B, Ke M, et al. Alginate-zinc (II) phthalocyanine conjugates: Synthesis, characterization and tumor-associated macrophages-targeted photodynamic therapy. Carbohydr Polym (2020) 240:116239. doi: 10.1016/j.carbpol.2020.116239
148. Hayashi N, Kataoka H, Yano S, Tanaka M, Moriwaki K, Akashi H, et al. A novel photodynamic therapy targeting cancer cells and tumor-associated macrophages. Mol Cancer Therap (2015) 14:452–60. doi: 10.1158/1535-7163.MCT-14-0348
149. Celli JP, Spring BQ, Rizvi I, Evans CL, Samkoe KS, Verma S, et al. Imaging and photodynamic therapy: Mechanisms, monitoring, and optimization. Chem Rev (2010) 110:2795–838. doi: 10.1021/cr900300p
150. Glidden MD, Massodi I, Rizvi I, Celli JP, Hasan T. Probing tumor-stroma interactions and response to photodynamic therapy in a 3D pancreatic cancer-fibroblast co-culture model. Kessel DH, Hasan T, editors (2012), p. 821006. doi: 10.1117/12.910924.
151. Chen Y-C, Lou X, Zhang Z, Ingram P, Yoon E. High-throughput cancer cell sphere formation for characterizing the efficacy of photo dynamic therapy in 3D cell cultures. Sci Rep (2015) 5:12175. doi: 10.1038/srep12175
152. Zamarrón A, García M, del RíM, Larcher F, Juarranz Á. Effects of photodynamic therapy on dermal fibroblasts from xeroderma pigmentosum and gorlin-goltz syndrome patients. Oncotarget (2017) 8:77385–99. doi: 10.18632/oncotarget.20485
153. Basset-Seguin N, Bissonnette R, Girard C, Haedersdal M, Lear JT, Paul C, et al. Consensus recommendations for the treatment of basal cell carcinomas in gorlin syndrome with topical methylaminolaevulinate-photodynamic therapy. J Eur Acad Dermatol Venereol (2014) 28:626–32. doi: 10.1111/jdv.12150
154. Broekgaarden M, Alkhateeb A, Bano S, Bulin A-L, Obaid G, Rizvi I, et al. Cabozantinib inhibits photodynamic therapy-induced auto- and paracrine MET signaling in heterotypic pancreatic microtumors. Cancers (Basel) (2020) 12:1401. doi: 10.3390/cancers12061401
155. Leonel C, Borin TF, de Carvalho Ferreira L, Moschetta MG, Bajgelman MC, Viloria-Petit AM, et al. Inhibition of epithelial-mesenchymal transition and metastasis by combined TGFbeta knockdown and metformin treatment in a canine mammary cancer xenograft model. J Mammary Gland Biol Neoplasia (2017) 22:27–41. doi: 10.1007/s10911-016-9370-7
156. Cheng K, Hao M. Metformin inhibits TGF-β1-Induced epithelial-to-Mesenchymal transition via PKM2 relative-mTOR/p70s6k signaling pathway in cervical carcinoma cells. Int J Mol Sc (2016) 17:2000. doi: 10.3390/ijms17122000
157. Sekino N, Kano M, Matsumoto Y, Sakata H, Akutsu Y, Hanari N, et al. Antitumor effects of metformin are a result of inhibiting nuclear factor kappa b nuclear translocation in esophageal squamous cell carcinoma. Cancer Sci (2018) 109:1066–74. doi: 10.1111/cas.13523
158. Wu F, Yang J, Liu J, Wang Y, Mu J, Zeng Q, et al. Signaling pathways in cancer-associated fibroblasts and targeted therapy for cancer. Sig Transduct Target Ther (2021) 6:1–35. doi: 10.1038/s41392-021-00641-0
159. Mittal A, Wang M, Vidyarthi A, Yanez D, Pizzurro G, Thakral D, et al. Topical arginase inhibition decreases growth of cutaneous squamous cell carcinoma. Sci Rep (2021) 11:10731. doi: 10.1038/s41598-021-90200-y
160. Korbelik M, Hamblin MR. The impact of macrophage-cancer cell interaction on the efficacy of photodynamic therapy. Photochem Photobiol Sci (2015) 14:1403–9. doi: 10.1039/C4PP00451E
161. Wen AM, Lee KL, Cao P, Pangilinan K, Carpenter BL, Lam P, et al. Utilizing viral Nanoparticle/Dendron hybrid conjugates in photodynamic therapy for dual delivery to macrophages and cancer cells. Bioconjugate Chem (2016) 27:1227–35. doi: 10.1021/acs.bioconjchem.6b00075
162. Neyen C, Plüddemann A, Mukhopadhyay S, Maniati E, Bossard M, Gordon S, et al. Macrophage scavenger receptor a promotes tumor progression in murine models of ovarian and pancreatic cancer. J Immunol (2013) 190:3798–805. doi: 10.4049/jimmunol.1203194
Keywords: tumor microenvironment, non-melanoma skin cancer, resistance, photodynamic therapy, cancer-asssociated fiboroblasts, immune cells, mast cell
Citation: Cerro PA, Mascaraque M, Gallego-Rentero M, Almenara-Blasco M, Nicolás-Morala J, Santiago JL, González S, Gracia-Cazaña T, Juarranz Á and Gilaberte Y (2022) Tumor microenvironment in non-melanoma skin cancer resistance to photodynamic therapy. Front. Oncol. 12:970279. doi: 10.3389/fonc.2022.970279
Received: 16 June 2022; Accepted: 07 October 2022;
Published: 21 October 2022.
Edited by:
Yong Sang Song, Seoul National University, South KoreaReviewed by:
Blassan George, University of Johannesburg, South AfricaJulita Kulbacka, Wroclaw Medical University, Poland
Copyright © 2022 Cerro, Mascaraque, Gallego-Rentero, Almenara-Blasco, Nicolás-Morala, Santiago, González, Gracia-Cazaña, Juarranz and Gilaberte. This is an open-access article distributed under the terms of the Creative Commons Attribution License (CC BY). The use, distribution or reproduction in other forums is permitted, provided the original author(s) and the copyright owner(s) are credited and that the original publication in this journal is cited, in accordance with accepted academic practice. No use, distribution or reproduction is permitted which does not comply with these terms.
*Correspondence: Ángeles Juarranz, angeles.juarranz@uam.es; Yolanda Gilaberte, ygilaberte@gmail.com
†These authors have contributed equally to this work and share first authorship