- 1Department of Biochemistry and Molecular Biology, Mayo Clinic, Jacksonville, FL, United States
- 2Genomics and Molecular Medicine Unit, CSIR-Institute of Genomics and Integrative Biology, Mathura Road, New Delhi, India
- 3Division of Medical Oncology, Mayo Clinic, Rochester, MN, United States
- 4Division of Hematology and Medical Oncology, Mayo Clinic, Jacksonville, FL, United States
- 5Department of Quantitative Health Sciences, Mayo Clinic Scottsdale, AZ, United States
- 6Integrative and Functional Biology Unit, CSIR- Institute of Genomics and Integrative Biology, New Delhi, India
- 7Academy of Scientific and Innovative Research, CSIR- Institute of Genomics and Integrative Biology, New Delhi, India
Objective: The objective of this study is to evaluate the expression of different nicotinic acetylcholine receptors (nAChRs), programmed death ligand-1 (PD-L1), and dopamine receptor D2 (DRD2) as prognostic factors in lung cancer and any correlation among them. Since all of the above genes are typically upregulated in response to smoking, we hypothesized that a correlation might exist between DRD2, PD-L1, and nAChR expression in NSCLC patients with a smoking history and a prediction model may be developed to assess the clinical outcome.
Methods: We retrospectively analyzed samples from 46 patients with primary lung adenocarcinoma who underwent surgical resection at Mayo Clinic Rochester from June 2000 to October 2008. The expression of PD-L1, DRD2, CHRNA5, CHRNA7, and CHRNA9 were analyzed by quantitative PCR and correlated amongst themselves and with age, stage and grade, smoking status, overall survival (OS), and relapse-free survival (RFS).
Results: Only PD-L1 showed a statistically significant increase in expression in patients older than 65. All the above genes showed higher expression in stage IIIB than IIIA, but none reached statistical significance. Interestingly, we did not observe significant differences among never, former, and current smokers, but patients with pack years greater than 30 showed significantly higher expression of CHRNA9. We observed a strong positive correlation between PD-L1/DRD2, PD-L1/CHRNA5, and CHRNA5/CHRNA7 and a weak positive correlation between DRD2/CHRNA5 and DRD2/CHRNA7. Older age was independently associated with poor OS, whereas lower CHRNA7 expression was independently associated with better OS.
Conclusions: We observed strong positive correlations among PD-L1, DRD2, and some of the nAChRs. We investigated their prognostic significance in lung cancer patients and found CHRNA7 to be an independent prognostic factor. Overall, the results obtained from this preliminary study warrant a large cohort-based analysis that may ultimately lead to potential patient-specific stratification biomarkers predicting cancer-treatment outcomes.
Introduction
Lung cancer is the leading cause of cancer-associated mortalities irrespective of gender in the United States and worldwide (1, 2). Approximately 85% of lung cancer falls under non-small-cell lung cancer (NSCLC), while the rest is categorized as small-cell lung cancer (SCLC) (3). Tobacco smoking is the primary risk factor for lung cancer; SCLC is exclusively seen among smokers, whereas approximately 80-90% of NSCLC is smoking-related (4, 5). Traditionally, nicotine has been considered addictive only and not carcinogenic. However, studies have shown that nicotine promotes proliferation, migration, invasion, and survival in vitro and tumor growth and metastasis in vivo in cancers of different origins, including lung cancer (6–15). The primary underlying mechanism behind the tumor-promoting activity of nicotine is exerted through the upregulation and activation of nicotinic acetylcholine receptors (nAChR) (10, 16–18). Acetylcholine (Ach) is the endogenous ligand of nAChRs, but nicotine can displace Ach due to its higher affinity towards these receptors, thereby activating downstream tumor-promoting signaling cascades (19–21).
The nAChRs consist of five subunits spanning the plasma membrane and are organized symmetrically around a central ion pore (22, 23). They act as ligand-gated ion channels facilitating calcium flux and release of neurotransmitters in neuronal cells and neuromuscular junctions or growth factors such as VEGF in epithelial and endothelial cells (23–25). To date, 17 nAChR subunits (α1-α10, β1-β4, δ, ϵ, and γ) have been identified in vertebrates, although only a few of them (α3-α7, α9, β2, and β4) have been studied in the context of cancer (26).
Dopamine receptors (DRD1–5) are typically observed in the brain (27, 28), but have also been shown to express in the tumor microenvironment, where they play vital roles in tumorigenesis and cancer progression (29–34). We initially unveiled that DRD2 is essential for dopamine-mediated selective inhibition of VPF/VEGF-induced vascular permeability and angiogenesis (35). DRD2 is implicated in lung cancer (36–39), and DRD2 agonists demonstrate significant growth inhibition in both NSCLC and SCLC (29, 31, 40, 41). Interestingly, some DRD2 variant genotypes have been associated with a higher affinity to smoking and a lower likelihood of smoking cessation, which has been proposed to be a probable cause behind the familial aggregation of smoking-related cancers (42, 43). DRD2 genetic polymorphisms have been associated with reduced bioavailability of dopamine and a higher risk of NSCLC among smokers (36). We also showed that DRD2 expression in tumors of lung cancer patients demonstrates a positive correlation with the extent of cigarette smoke exposure and the histological grade of the tumor (29). Several studies suggested potential cross-talk between DRD2 with nAChRs within the brain (44–49), but no conclusive evidence of their interaction in cancer has been observed till now.
The interaction of programmed cell death protein-1 (PD-1) with its ligands, namely programmed cell death ligand-1 and -2 (PD-L1, PD-L2), act as immune checkpoints by reducing functionality of effector T-cells in peripheral tissues, and preventing them from attacking the host cells during inflammatory response (50, 51). Cancer cells hijack this mechanism to evade immune surveillance and induce immune suppression by expressing PD-L1 or PD-L2 on their surface (52, 53). Immune checkpoint inhibition via antibodies against PD-1 or PD-L1 demonstrated improved therapeutic response in several types of cancer, including NSCLC (54–58). Although patient stratification based on PD-L1 expression improved the response rate compared to non-stratified patients (59–61), a response is not always dictated by their expression (62–66). Interestingly, recent studies demonstrated a strong correlation between PD-L1 expression and smoking status in NSCLC patients, where smokers with higher pack-years demonstrated a higher intensity of PD-L1 expression (67–69). However, the correlation between PD-L1 and nAChRs have not been well studied in NSCLC patients.
The primary goal of the present study is to determine whether the expression pattern of PD-L1, DRD2, and the genes encoding different nAChR subunits in NSCLC are affected by the age, stage, grade, and smoking status and whether they could explain the variability of the influence of tobacco smoking in response to therapy and survival in NSCLC. Towards this end, we have used real-time quantitative polymerase chain reaction (qPCR) to examine normalized mRNA expression levels of the above genes in surgical tumor samples from 46 NSCLC patients and examined their correlation with the said parameters as well as with every other gene in our study.
Materials and methods
Sample collection
We collected flash-frozen samples of lung tumor tissues surgically removed from 46 patients with primary lung adenocarcinoma. The patients were admitted to and underwent surgery at Mayo Clinic Rochester from June 2000 to October 2008. Detailed demographic analyses of the patients are provided in Table 1. To avoid the potential confounding impact of the treatment, none of the selected patients received neoadjuvant treatment before the surgery, although some of the patients were treated with chemotherapy or radiotherapy or both post-surgeries. Tumor grading was abstracted from chest pathologists’ diagnosis documented in Mayo Clinic medical records and categorized as well-differentiated, moderately differentiated, poorly differentiated and undifferentiated. Tumor staging was based on the TNM staging system 7th edition (70). The Mayo Clinic Institutional Review Board reviewed the study protocol, and all patients signed written informed consent forms.
Total RNA isolation from tumor tissues
Total RNA was isolated from the flash frozen lung tumor tissues using Allprep DNA/RNA Mini Kit (Qiagen), as per manufacturer’s protocol. Briefly, five 10 µm sections of tumor tissue were homogenized in RLT plus buffer supplemented with 1% β-mercaptoethanol. The lysed mixture was centrifuged at 13000 rpm for 10 minutes to precipitate any remaining tissue debris. The clear supernatant was carefully collected, transferred to the AllPrep DNA spin column, and centrifuged for 30 s at 13,000 rpm. The flow-through was collected for RNA isolation, and the AllPrep DNA spin column was used for DNA isolation to be used elsewhere. For RNA isolation, flow-through was mixed with an equal volume of 70% ethanol, and the mixture was filtered through an RNAeasy column by centrifugation at 10000 rpm for 30 seconds. The flow-through was discarded, and the column was further washed using two changes of 700 µL RW wash buffer, and once with 500 µL RPE wash buffer. Finally, the column-bound RNA was eluted using 30 µL RNAse free water. The eluted RNA was stored at -80°C until further use.
Quantitative Polymerase Chain Reaction
Total RNA obtained from the above step was transcribed into complementary DNA (cDNA) using SuperScript™ III First-Strand Synthesis System (Invitrogen) following the manufacturer’s protocol. Briefly, 1 µg of total RNA from each sample was mixed with 50 µM oligo[dT]20 primer and 10 mM dNTP mix. The mixture was incubated at 65°C for 5 min followed by 1 min at 4°C. Next, 10 µl of cDNA synthesis mix was added to each RNA/primer mixture and was incubated at 50°C for 50 min, and the reaction was terminated by heating at 85°C for 5 min followed by cooling the sample at 4°C. Finally, 1 µL of the cDNA was amplified using probe-specific primers (Table 2), and Power SYBR Green mastermix in an ABI 7500 real-time PCR system using the following protocol: 1x 10 min at 95°C, 40x (30 sec at 95°C, 1 min at 60°C) and hold at 4°C. ΔCt values were calculated by subtracting Ct values for β-actin from each sample’s respective genes.
Statistical analysis
We used R (version 3.6.0) and Graphpad Prism (version 9) for data analyses and presentation. We did not perform sample size calculation for this retrospective study due to its descriptive nature. Categorical data were summarized as frequency counts and percentages, and were compared using Fisher’s exact test. Continuous data were summarized as mean, standard deviation, or median and interquartile ranges (IQR), compared using the Wilcoxson rank-sum test. Time‐to‐event data were summarized using the Kaplan‐Meier method and compared using log‐rank tests. We used Cox proportional hazards model for multivariable analyses of potential prognostic indicators with p<0.05 in univariate analysis. All Cox proportional hazards regression results are presented as hazard ratios (HRs), 95% confidence intervals (95% CI) for the HR, and corresponding p values. The proportionality assumption was assessed graphically using log (−log) plots and quantitatively using the Z statistic. All statistical tests were two-sided. P<0.05 was considered statistically significant.
Results
Patient characteristics
Forty-six NSCLC patients were included in the study and their demographic and clinical characteristics are summarized in Table 1. The median age of the patients was 65. Patients were grouped according to their gender, smoking status, vital status, recurrence, tumor stage, histologic grade, and treatment post-surgery. Ever smokers were those who were not clearly identified as current or former smokers at the time of lung cancer diagnosis.
PD-L1, DRD2, and nAChR expression and selected patient characteristics
The expression of PD-L1, DRD2 and three nAChR genes encoding α5, α7, and α9 nAChR respectively (CHRNA5, CHRNA7, and CHRNA9) were analyzed against selected patient characteristics such as age, stage, smoking status, and pack-years (Figures 1A–D). For the graphical presentation, the patients were divided based on their median age of diagnosis, which was 65 years. Interestingly patients with an age of diagnosis greater than or equal to 65 showed a statistically significant (p = 0.022) lower mean ΔCt value (and hence a higher mean expression) for PD-L1 than patients with an age of diagnosis less than 65 (Figure 1A). DRD2, CHRNA5, CHRNA7, or CHRNA9 did not show any significant difference between these patient cohorts. Stage IIIB patients showed lower mean ΔCt values or higher mean expressions than stage IIIA patients for each of the genes; however only PD-L1 (p = 0.0556) and CHRNA9 (p=0.0596) were close to reaching statistical significance (Figure 1B). Similarly, we did not observe a significant difference in expressions among never, former, and current smokers (Figure 1C). However, patients with pack-years greater than or equal to 30 showed a highly significant lower mean ΔCt value or higher mean expression of CHRNA9 than patients with PY less than 30 (p = 0.0061) (Figure 1D).
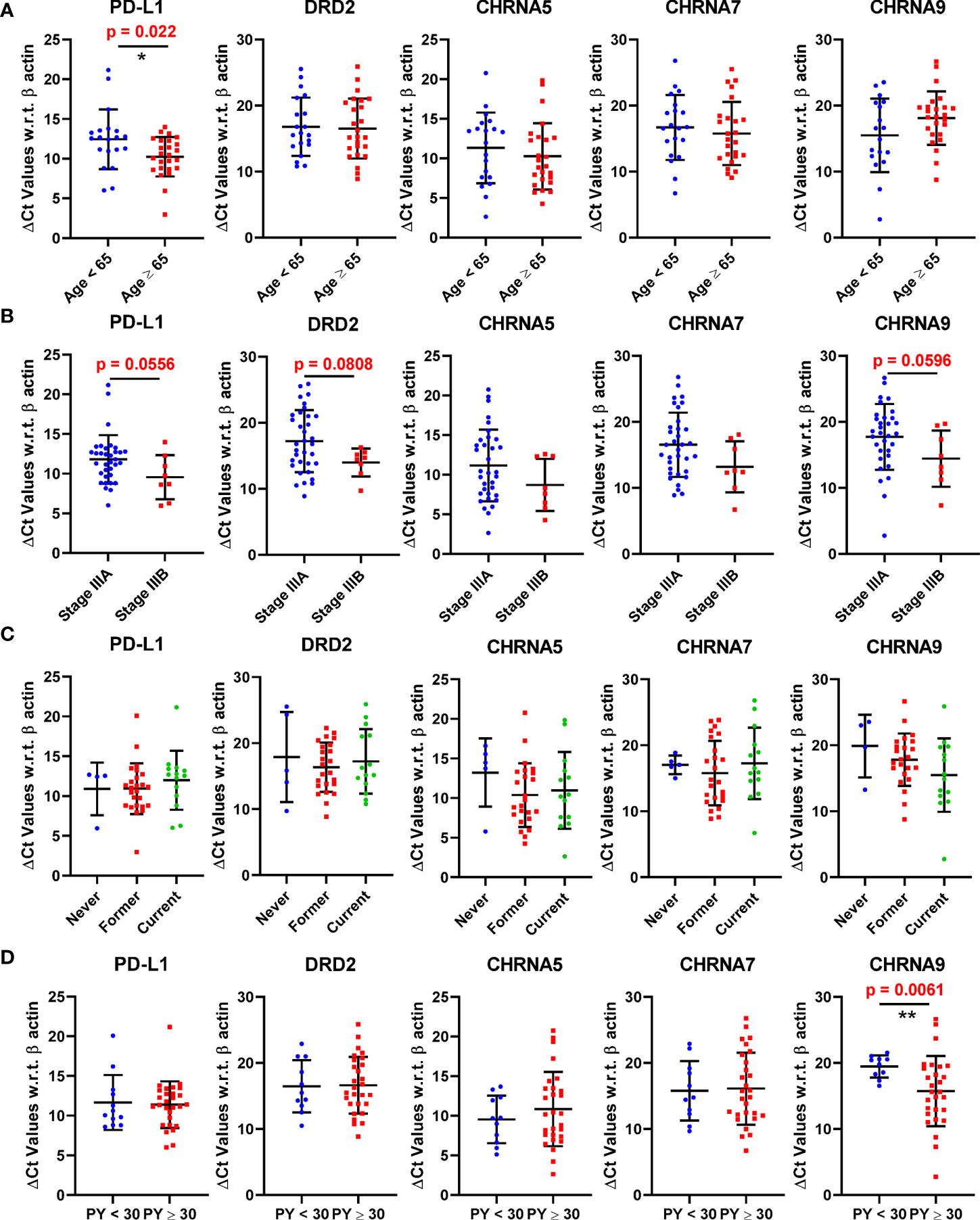
Figure 1 Expression of PD-L1, DRD2, CHRNA5, CHRNA7, and CHRNA9 mRNAs represented as ΔCt values in patients stratified based on age (A), Stage (B), Smoking status (C), and pack years (D). A lower ΔCt value means the gene expression is higher. * and ** denote p<0.05 and p<0.01 respectively.
Correlation among PD-L1, DRD2, and nAChR expression
PD-L1 expression showed strong positive correlation with DRD2 (p = 0.0034) and CHRNA5 (p = 0.0012) and was close to reaching statistical significance with CHRNA7 (p = 0.0581) (Figures 2A–C). In addition to PD-L1, DRD2 also showed positive correlation with CHRNA5 (p = 0.0194) and CHRNA7 (p = 0.0288) (Figures 2D, E). Furthermore, CHRNA5 and CHRNA7 showed strong positive correlation (p = 0.0094) (Figure 2F). We also checked the association between the above genes in The Cancer Genome Atlas Lung Adenocarcinoma (TCGA-LUAD) database using the TIMER portal (https://cistrome.shinyapps.io/timer/). Interestingly, there we found statistically significant correlation between CHRNA5/CHRNA9 (p = 1.02e-06), CHRNA5/PD-L1 (p = 3.64e-03), CHRNA7/PD-L1 (p = 3.72e-05), CHRNA7/DRD2 (p = 2.09e-05), and CHRNA9/DRD2 (p = 4.11e-05) (Supplementary Figure 1). However, it is to be noted that expression of these genes may be affected by the stage, population, or treatment which may significantly vary between our study and TCGA-LUAD database. But more importantly, the notion that the expression of the nAChRs, DRD2, and PD-L1 might have some level of correlation was certainly substantiated from these data.
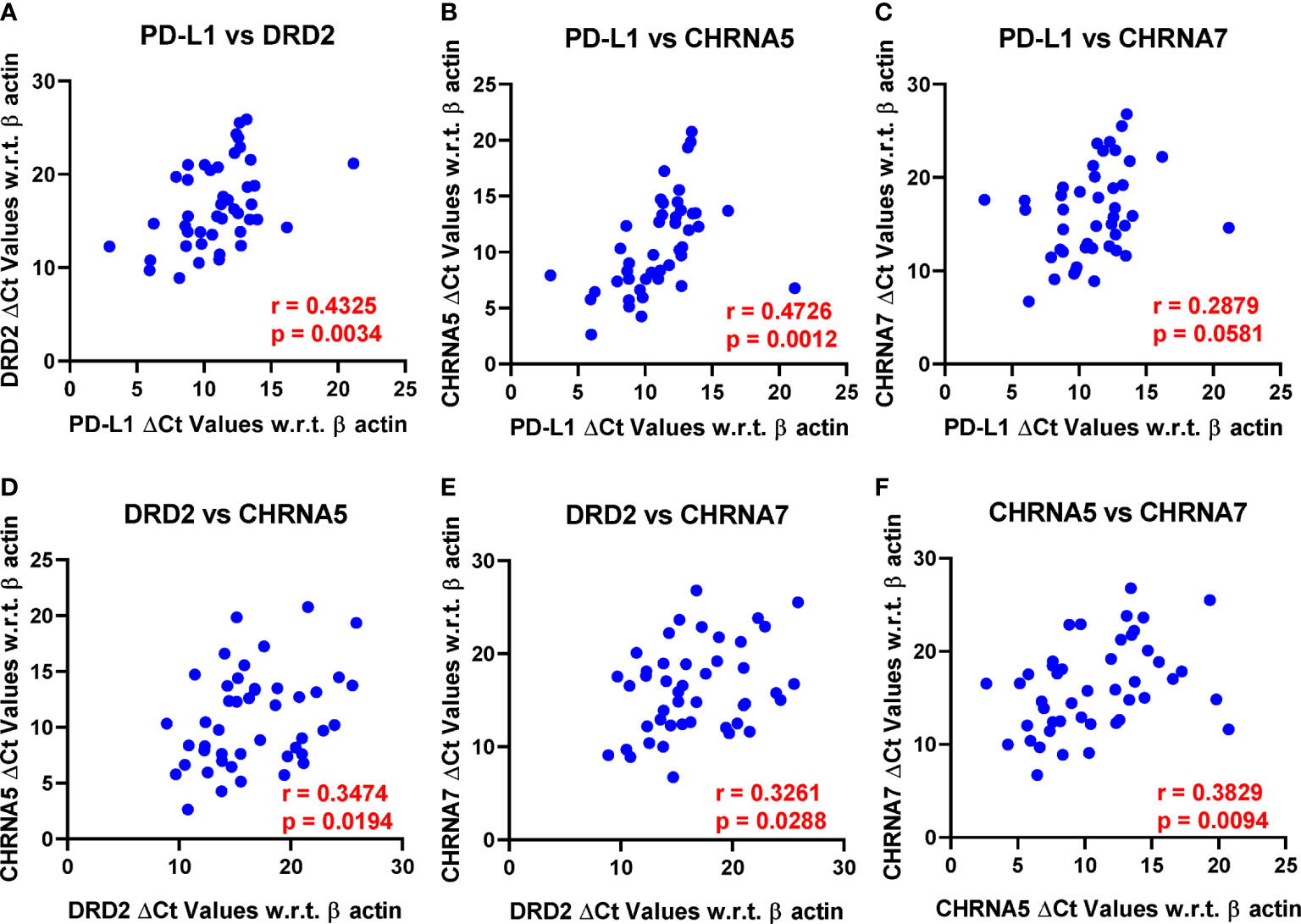
Figure 2 Pearson’s correlation analysis was performed to analyze the correlation between PD-L1, DRD2, CHRNA5, CHRNA7, and CHRNA9 mRNA expression represented as ΔCt values. Only those reaching or close to reaching statistical significance were included. r, Pearson’s correlation coefficient. (A) PD-L1 vs DRD2, (B) PD-L1 vs CHRNA5, (C) PD-L1 vs CHRNA7, (D) DRD2 vs CHRNA5, (E) DRD2 vs CHRNA7, and (F) CHRNA5 vs CHRNA7.
Prognostic values of PD-L1, DRD2, and nAChR expression
We further evaluated the association of PD-L1, DRD2, and nAChR expression with Overall survival (OS) and Relapse-free survival (RFS). OS was defined as the time interval between the date of diagnosis and the date of death or last follow-up (censored). RFS was defined as the time interval between the date of surgical resection and the date of recurrence, or the date of death or last follow-up if no recurrence occurred (censored). We excluded three extreme outliers (one in CHRNA5 and two in CHRNA9) from the analyses. We excluded patients with stage IIB and IV disease (n=3) from the survival analysis. Due to the small sample size, we regrouped treatment categories for survival analysis to avoid overfitting. Ever smokers were combined with current and former smokers for survival analysis. Here, we treated age and expressions of the genes as continuous variables instead of dichotomized for the analyses since it provides a higher statistical power. As shown in Table 3, older age was associated with poor OS (HR 1.05, 95% CI 1.00-1.09, p=0.038); lower DRD2 expression (HR 0.91, 95% CI 0.84-1.00, p=0.040) and lower CHRNA7 expression (HR 0.92, 95% CI 0.85-0.99, p=0.037) were associated with better OS. Lower CHRNA9 expression seemed to be associated with better OS, but did not reach statistical significance (HR 0.95, 95% CI 0.89-1.01, p=0.093). Based on model selection criteria and the significant correlation between DRD2 and CHRNA7, we include age and CHRNA7 for multivariable survival analysis. Older age was independently associated with poor OS (HR 1.05, 95% CI 1.01-1.10, p=0.014); lower CHRNA7 expression was independently associated with better OS (HR 0.90, 95% CI 0.82-0.98, p=0.017). Lower CHRNA7 expression seemed to be associated with better RFS, but did not reach statistical significance (HR 0.94, 95% CI 0.87-1.01, p=0.08).
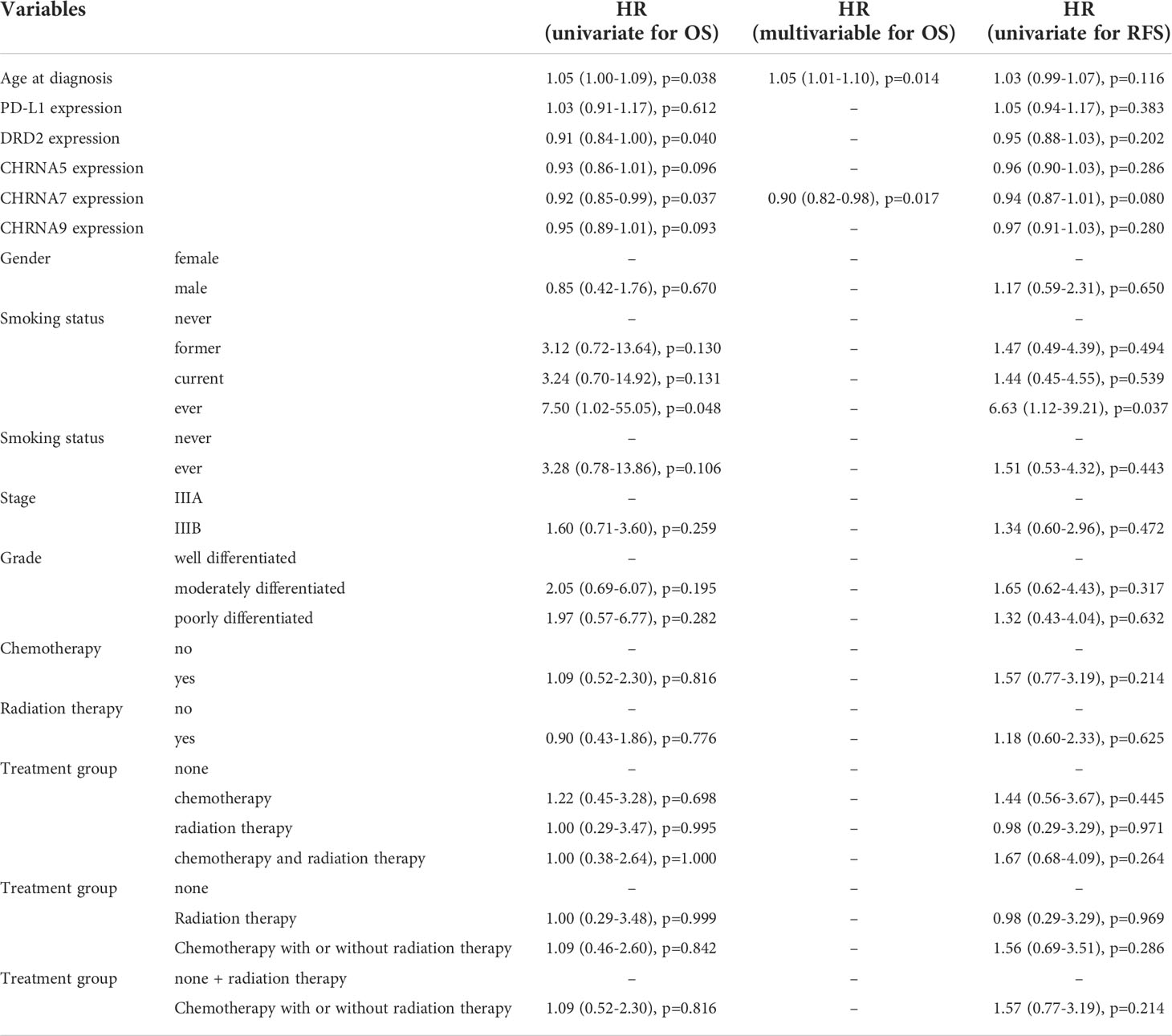
Table 3 Prognostic values of biomarkers, clinical factors, histopathological factors, and treatment in overall survival (OS) and relapse-free survival (RFS).
Discussion
The implications of different nAChR expression and their polymorphisms in lung cancer cell proliferation, apoptosis, angiogenesis, and invasion have been previously reported by several groups (18, 71–76). Among them, homomeric α7 nAChR (composed of five identical α7 subunits expressed from the CHRNA7 gene) is the most widely implicated in nicotine-mediated proliferation, angiogenesis, and metastasis in NSCLC (77–81). Additionally, both α5 and α9 nAChRs (encoded by CHRNA5 and CHRNA9 genes, respectively) have been associated with NSCLC (77, 82–85). A recent study using lung adenocarcinoma (ADC) patient samples revealed that α5-nAChR expression is correlated with the clinicopathological parameters such as T and N stages but not with age or sex (82). Another study with lung squamous cell carcinoma (SQCC) and ADC showed that α5-nAChR expression is significantly higher in tumors than in adjacent normal lung tissue (86). Interestingly α7-nAChR was significantly higher in SQCC than normal tissue but not in ADC in this study. However, another study had shown that α7-nAChR expression was significantly higher in both SQCC and ADC (87). Both α5- and α7-nAChR were significantly associated with unfavorable prognosis in ADC, but only α7-nAChR showed a significant correlation with prognosis in SQCC. Unfortunately, several important factors, including treatment modalities such as chemotherapy and radiation therapy, were missing in these studies, making the conclusion challenging to interpret.
The correlation of nAChRs and PD-L1 has been shown by in vitro studies in several human cell lines, including bronchial epithelial cells, HepG2 cells, melanoma and breast cancer cells (88–91). Furthermore, an in vitro study showed that chronic nicotine exposure could increase α1-nAChR and PD-L1 expressions in a lung adenocarcinoma cell line (92). Another recent study showed that coexpression of α5-nAChR and PD-L1 are associated with a worse prognosis in patients with lung adenocarcinoma (93). However, the correlation between PD-L1 and DRD2 has not been well-investigated in context to human cancer except for one recent study where Paliperidone (a DRD2 antagonist) reduced PD-L1 expression in glioblastoma cells and increased survival in a mouse model of glioblastoma (94).
Nicotine typically induces the release of dopamine in the brain via activation of the nAChR receptors in the central nervous system, but the released dopamine cannot cross blood-brain barrier (71). Interestingly, dopamine can also be synthesized in the peripheral nerves and released into circulation in response to stress, exercise, or hypovolemia (95). Nonetheless, a plausible role of nicotine or nAChRs in peripheral dopamine synthesis may not be ruled out, especially since correlative analyses from our data as well as TCGA database showed positive correlation between some of the nAChRs and DRD2 in lung adenocarcinoma patients (Figure 2 and Supplementary Figure 1).
Nicotinic acetylcholine receptors such as a5, a7, or a9 have been shown to express in various immune cells where they regulate the secretion of immunomodulatory cytokines and immune response (96). Interestingly, nicotine-mediated activation of both a5 and a9 nAChRs have been shown to upregulate PD-L1 expression in cancer cells via STAT3 signaling pathways (90, 93), so a positive correlation of nAChRs with PD-L1 may be expected (Supplementary Figure 2). Surprisingly, the nAChRs correlated with DRD2 or PD-L1 are different between our study and TCGA database, but the difference may be attributed to the difference in stages, patient population, or tumor-infiltrating immune cells. For instance, differential tumor infiltration of immune cells having varied expression of nAChRs or DRD2 can affect their correlation in the tumor samples.
DRD2 is also expressed on the surface of a variety of immune cells and has been implicated in the regulation of immune cell activity (97). DRD2 antagonism has been reported to induce immune cell proliferation and activation in preclinical studies, whereas DRD2 activation suppressed the function of natural killer cells. DRD2 stimulation has also been shown to inhibit proliferation and cytokine production in activated T cells. DRD2 antagonists could also induce M1-polarization of macrophages and decrease PD-L1 expression in cancer cells via inhibition of ERK and STAT3 signaling pathways (94, 98). Consequently, a positive correlation between DRD2 and PD-L1 may not be improbable after all (Supplementary Figure 2).
Our study demonstrated positive correlations among PD-L1, DRD2, and nAChR in tumor samples from 46 NSCLC patients at our institution. Our study indicates a possible underlying mechanism that DRD2 and nAChR involved pathways may affect the tumor immune microenvironment, leading to the expression of PD-L1. It remains unknown whether DRD2 and nAChR share the same signaling pathways or play a synergetic role in tumorigenesis and therefore requires further investigation. In addition, given the increased roles of anti-PD-1/anti-PD-L1 immunotherapies in early-stage and advanced-stage NSCLC, our data imply that DRD2 and nAChR might be potential molecular biomarkers along with PD-L1 to guide treatment decisions in NSCLC patients in the future. Furthermore, targeting nAChR or DRD2 may be a potential therapeutic strategy to alter PD-1/PD-L1 pathway that can benefit those NSCLC patients who are refractory to immunotherapy.
We found that lower expressions of DRD2 and CHRNA7 are associated with a slightly better OS. Previous studies showed that a high expression of CHRNA7 is associated with an unfavorable prognosis in NSCLC (45). Of note, most patients studied in this cohort are stage I and II. We observed a similar result in our cohort of stage III NSCLC patients, in which lower CHRNA7 expression is independently associated with better OS, although the difference is small (HR = 0.90). Whether similar trend can be observed in stage IV NSCLC patients and this is associated with any clinical significance needs to be further evaluated. We did not observe statistically significant differences in RFS regarding the expression of each gene tested. This may be due to the small size of our cohort. Whether DRD2 or nAChR affects or has predictive value of the response to systemic therapy, such as chemotherapy or immunotherapy, remains unknown and warrants further investigation.
We did not observe a significant difference in PD-L1, DRD2, and nAChR expressions among never, former, and current smokers. Nevertheless, patients with more pack-year (>30) smoking history have significantly higher CHRNA9 expression, but not CHRNA5 or CHRNA7 expression. This indicates a possible unique smoking-related upregulating mechanism on CHRNA9 gene expression, which requires further elucidation.
Although our study suggests a probable connection of DRD2 with nAChR and PD-L1 in lung cancer for the first time, there are several limitations in our study. First, we only examined gene expression at the mRNA level by using qPCR due to the limited availability of tumor tissues. Whether the observed trends hold true at the protein level needs further validation. Additionally, immunohistochemistry data would have been potentially useful in determining the spatial expression of these markers in the tumor microenvironment. This is important since it would delineate the effect of different tumor-infiltrating immune cell populations with varied nAChR, DRD2 or PD-L1 expression on the prognosis. Second, our cohort has a relatively small size. This limits the statistical power to detect the difference in targeted gene expression between subgroups, such as smoking status. Furthermore, our study only includes localized NSCLC patients who received surgery. It is important to extend our analysis to a large-scale study that includes patients who are not surgical candidates or at stage IV to evaluate DRD2 and nAChR’s role in systemic therapy.
Conclusion
In conclusion, we investigated the expression pattern and prognostic value of PD-L1, DRD2, and three nAChR family members in NSCLC using surgical samples obtained from 46 patients. We found strong positive correlations between PD-L1/DRD2, PD-L1/CHRNA5, and CHRNA5/CHRNA7 and a weak positive correlation between DRD2/CHRNA5 and DRD2/CHRNA7 at the mRNA level. CHRNA7 was an independent prognostic factor in surgically resected stage III NSCLC patients. Further studies using large-scale cohorts including patients at different stages and receiving various treatments are warranted.
Data availability statement
The original contributions presented in the study are included in the article/Supplementary Material. Further inquiries can be directed to the corresponding author.
Ethics statement
The studies involving human participants were reviewed and approved by The Mayo Clinic Institutional Review Board. The patients/participants provided their written informed consent to participate in this study.
Author contributions
DM and SC conceived the idea and supervised overall research. PY and AM arranged the patient sample collection. KP designed the experiments, analyzed, and interpreted the data. TH performed the experiments. HX and PY performed statistical analyses. KP, TH, HX, and SL wrote the original manuscript. DM, SC, PY and YL revised the article. All authors contributed to the article and approved the submitted version.
Funding
This work was supported by NIH grants CA150190, CA78383, Florida Department of Health Grant #20K02, and Cancer Research Chair Fund #3J (DM), the DBT/Wellcome Trust India Alliance Fellowship [grant number IA/S/18/2/504021] awarded to SC, and CSIR-Mayo Clinic Collaboration for Translation Research Program for support to TH.
Acknowledgments
The authors thank Dr. Jin Jen for providing critical resources.
Conflict of interest
The authors declare that the research was conducted in the absence of any commercial or financial relationships that could be construed as a potential conflict of interest.
Publisher’s note
All claims expressed in this article are solely those of the authors and do not necessarily represent those of their affiliated organizations, or those of the publisher, the editors and the reviewers. Any product that may be evaluated in this article, or claim that may be made by its manufacturer, is not guaranteed or endorsed by the publisher.
Supplementary material
The Supplementary Material for this article can be found online at: https://www.frontiersin.org/articles/10.3389/fonc.2022.959500/full#supplementary-material
Supplementary Figure 1 | Pearson’s correlation analysis was performed to analyze the correlation between PD-L1 (CD274), DRD2, CHRNA5, CHRNA7, and CHRNA9 mRNA expression from TCGA-LUAD database.
Supplementary Figure 2 | A schematic diagram depicting plausible connection between nAChR, DRD2, and PD-L1. Both nAChR and DRD2 activation by their respective ligands induce PD-L1 expression via STAT3 signaling mechanism. However, it is not clear how nAChRs are involved in peripheral DRD2 signaling, although they are known to be involved in dopamine synthesis in the brain. Created with BioRender.com.
References
1. Siegel RL, Miller KD, Jemal A. Cancer statistics, 2020. CA: A Cancer J Clin (2020) 70(1):7–30. doi: 10.3322/caac.21590
2. Bray F, Ferlay J, Soerjomataram I, Siegel RL, Torre LA, Jemal A. Global cancer statistics 2018: Globocan estimates of incidence and mortality worldwide for 36 cancers in 185 countries. CA: A Cancer J Clin (2018) 68(6):394–424. doi: 10.3322/caac.21492
3. Inamura K. Lung cancer: Understanding its molecular pathology and the 2015 who classification. Front Oncol (2017) 7:193. doi: 10.3389/fonc.2017.00193
4. Pelosof LC, Ahn C, Madrigales A, Cox J, Minna JD, Schiller JH. Rate of never smokers in non small cell lung cancer (Nsclc) patients. J Clin Oncol (2015) 33(15_suppl):e19007–e. doi: 10.1200/jco.2015.33.15_suppl.e19007
6. Dasgupta P, Rizwani W, Pillai S, Kinkade R, Kovacs M, Rastogi S, et al. Nicotine induces cell proliferation, invasion and epithelial-mesenchymal transition in a variety of human cancer cell lines. Int J Cancer (2009) 124(1):36–45. doi: 10.1002/ijc.23894
7. Heeschen C, Jang JJ, Weis M, Pathak A, Kaji S, Hu RS, et al. Nicotine stimulates angiogenesis and promotes tumor growth and atherosclerosis. Nat Med (2001) 7(7):833–9. doi: 10.1038/89961
8. Trevino JG, Pillai S, Kunigal S, Singh S, Fulp WJ, Centeno BA, et al. Nicotine induces inhibitor of differentiation-1 in a src-dependent pathway promoting metastasis and chemoresistance in pancreatic adenocarcinoma. Neoplasia (2012) 14(12):1102–14. doi: 10.1593/neo.121044
9. Momi N, Ponnusamy MP, Kaur S, Rachagani S, Kunigal SS, Chellappan S, et al. Nicotine/Cigarette smoke promotes metastasis of pancreatic cancer through Alpha7nachr-mediated Muc4 upregulation. Oncogene (2013) 32(11):1384–95. doi: 10.1038/onc.2012.163
10. Schaal C, Chellappan SP. Nicotine-mediated cell proliferation and tumor progression in smoking-related cancers. Mol Cancer Res (2014) 12(1):14–23. doi: 10.1158/1541-7786.MCR-13-0541
11. Calderon LE, Liu S, Arnold N, Breakall B, Rollins J, Ndinguri M. Bromoenol lactone attenuates nicotine-induced breast cancer cell proliferation and migration. PloS One (2015) 10(11):e0143277. doi: 10.1371/journal.pone.0143277
12. Yuge K, Kikuchi E, Hagiwara M, Yasumizu Y, Tanaka N, Kosaka T, et al. Nicotine induces tumor growth and chemoresistance through activation of the Pi3k/Akt/Mtor pathway in bladder cancer. Mol Cancer Ther (2015) 14(9):2112–20. doi: 10.1158/1535-7163.MCT-15-0140
13. Khalil AA, Jameson MJ, Broaddus WC, Lin PS, Chung TD. Nicotine enhances proliferation, migration, and radioresistance of human malignant glioma cells through egfr activation. Brain Tumor Pathol (2013) 30(2):73–83. doi: 10.1007/s10014-012-0101-5
14. Nieh S, Jao SW, Yang CY, Lin YS, Tseng YH, Liu CL, et al. Regulation of tumor progression Via the snail-rkip signaling pathway by nicotine exposure in head and neck squamous cell carcinoma. Head Neck (2015) 37(12):1712–21. doi: 10.1002/hed.23820
15. Davis R, Rizwani W, Banerjee S, Kovacs M, Haura E, Coppola D, et al. Nicotine promotes tumor growth and metastasis in mouse models of lung cancer. PloS One (2009) 4(10):e7524. doi: 10.1371/journal.pone.0007524
16. Improgo MR, Soll LG, Tapper AR, Gardner PD. Nicotinic acetylcholine receptors mediate lung cancer growth. Front Physiol (2013) 4:251. doi: 10.3389/fphys.2013.00251
17. Carlisle DL, Liu X, Hopkins TM, Swick MC, Dhir R, Siegfried JM. Nicotine activates cell-signaling pathways through muscle-type and neuronal nicotinic acetylcholine receptors in non-small cell lung cancer cells. Pulm Pharmacol Ther (2007) 20(6):629–41. doi: 10.1016/j.pupt.2006.07.001
18. Schuller HM. Is cancer triggered by altered signalling of nicotinic acetylcholine receptors? Nat Rev Cancer (2009) 9(3):195–205. doi: 10.1038/nrc2590
19. Heeschen C, Weis M, Aicher A, Dimmeler S, Cooke JP. A novel angiogenic pathway mediated by non-neuronal nicotinic acetylcholine receptors. J Clin Invest (2002) 110(4):527–36. doi: 10.1172/JCI14676
20. Wang Y, Pereira EF, Maus AD, Ostlie NS, Navaneetham D, Lei S, et al. Human bronchial epithelial and endothelial cells express Alpha7 nicotinic acetylcholine receptors. Mol Pharmacol (2001) 60(6):1201–9. doi: 10.1124/mol.60.6.1201
21. Singh S, Pillai S, Chellappan S. Nicotinic acetylcholine receptor signaling in tumor growth and metastasis. J Oncol (2011) 2011:456743. doi: 10.1155/2011/456743
22. Itier V, Bertrand D. Neuronal nicotinic receptors: From protein structure to function. FEBS Lett (2001) 504(3):118–25. doi: 10.1016/s0014-5793(01)02702-8
23. Albuquerque EX, Pereira EF, Alkondon M, Rogers SW. Mammalian nicotinic acetylcholine receptors: From structure to function. Physiol Rev (2009) 89(1):73–120. doi: 10.1152/physrev.00015.2008
24. Dani JA. Neuronal nicotinic acetylcholine receptor structure and function and response to nicotine. Int Rev Neurobiol (2015) 124:3–19. doi: 10.1016/bs.irn.2015.07.001
25. Zhao Y. The oncogenic functions of nicotinic acetylcholine receptors. J Oncol (2016) 2016:1–9. doi: 10.1155/2016/9650481
26. Dang N, Meng X, Song H. Nicotinic acetylcholine receptors and cancer. Biomed Rep (2016) 4(5):515–8. doi: 10.3892/br.2016.625
27. Jaber M, Robinson SW, Missale C, Caron MG. Dopamine receptors and brain function. Neuropharmacology (1996) 35(11):1503–19. doi: 10.1016/s0028-3908(96)00100-1
28. Levey AI, Hersch SM, Rye DB, Sunahara RK, Niznik HB, Kitt CA, et al. Localization of D1 and D2 dopamine receptors in brain with subtype-specific antibodies. Proc Natl Acad Sci (1993) 90(19):8861–5. doi: 10.1073/pnas.90.19.8861
29. Hoeppner LH, Wang Y, Sharma A, Javeed N, Van Keulen VP, Wang E, et al. Dopamine D2 receptor agonists inhibit lung cancer progression by reducing angiogenesis and tumor infiltrating myeloid derived suppressor cells. Mol Oncol (2015) 9(1):270–81. doi: 10.1016/j.molonc.2014.08.008
30. Borcherding DC, Tong W, Hugo ER, Barnard DF, Fox S, LaSance K, et al. Expression and therapeutic targeting of dopamine receptor-1 (D1r) in breast cancer. Oncogene (2015) 35(24):3103–13. doi: 10.1038/onc.2015.369
31. Roy S, Lu K, Nayak MK, Bhuniya A, Ghosh T, Kundu S, et al. Activation of D2dopamine receptors in Cd133+Ve cancer stem cells in non-small cell lung carcinoma inhibits proliferation, clonogenic ability, and invasiveness of these cells. J Biol Chem (2017) 292(2):435–45. doi: 10.1074/jbc.M116.748970
32. Pornour M, Ahangari G, Hejazi SH, Ahmadkhaniha HR, Akbari ME. Dopamine receptor gene (Drd1-Drd5) expression changes as stress factors associated with breast cancer. Asian Pacific J Cancer Prev (2015) 15(23):10339–43. doi: 10.7314/apjcp.2014.15.23.10339
33. Robles AI, Yang P, Jen J, McClary AC, Calhoun K, Bowman ED, et al. A Drd1 polymorphism predisposes to lung cancer among those exposed to secondhand smoke during childhood. Cancer Prev Res (2014) 7(12):1210–8. doi: 10.1158/1940-6207.Capr-14-0158
34. Wang X, Wang Z-B, Luo C, Mao X-Y, Li X, Yin J-Y, et al. The prospective value of dopamine receptors on bio-behavior of tumor. J Cancer (2019) 10(7):1622–32. doi: 10.7150/jca.27780
35. Basu S, Nagy JA, Pal S, Vasile E, Eckelhoefer IA, Bliss VS, et al. The neurotransmitter dopamine inhibits angiogenesis induced by vascular permeability Factor/Vascular endothelial growth factor. Nat Med (2001) 7(5):569–74. doi: 10.1038/87895
36. Campa D, Zienolddiny S, Lind H, Ryberg D, Skaug V, Canzian F, et al. Polymorphisms of dopamine Receptor/Transporter genes and risk of non-small cell lung cancer. Lung Cancer (2007) 56(1):17–23. doi: 10.1016/j.lungcan.2006.11.007
37. Cherubini E, Di Napoli A, Noto A, Osman GA, Esposito MC, Mariotta S, et al. Genetic and functional analysis of polymorphisms in the human dopamine receptor and transporter genes in small cell lung cancer. J Cell Physiol (2016) 231(2):345–56. doi: 10.1002/jcp.25079
38. Wu X-Y, Zhang C-X, Deng L-C, Xiao J, Yuan X, Zhang B, et al. Overexpressed D2 dopamine receptor inhibits non-small cell lung cancer progression through inhibiting nf-Kb signaling pathway. Cell Physiol Biochem (2018) 48(6):2258–72. doi: 10.1159/000492644
39. Ferone D, Arvigo M, Semino C, Jaquet P, Saveanu A, Taylor JE, et al. Somatostatin and dopamine receptor expression in lung carcinoma cells and effects of chimeric somatostatin-dopamine molecules on cell proliferation. Am J Physiology-Endocrinology Metab (2005) 289(6):E1044–E50. doi: 10.1152/ajpendo.00209.2005
40. Senogles SE. D2 dopamine receptor-mediated antiproliferation in a small cell lung cancer cell line, nci-H69. Anti-Cancer Drugs (2007) 18(7):801–7. doi: 10.1097/CAD.0b013e3280b10d36
41. Sheikhpour M, Ahangari G, Sadeghizadeh M, Deezagi A. A novel report of apoptosis in human lung carcinoma cells using selective agonist of D2-like dopamine receptors: A new approach for the treatment of human non-small cell lung cancer. Int J Immunopathology Pharmacol (2013) 26(2):393–402. doi: 10.1177/039463201302600212
42. Styn MA, Nukui T, Romkes M, Perkins K, Land SR, Weissfeld JL. The impact of genetic variation in Drd2 and Slc6a3 on smoking cessation in a cohort of participants 1 year after enrollment in a lung cancer screening study. Am J Med Genet B Neuropsychiatr Genet (2009) 150B(2):254–61. doi: 10.1002/ajmg.b.30801
43. Wu X, Hudmon KS, Detry MA, Chamberlain RM, Spitz MR. D2 dopamine receptor gene polymorphisms among African-americans and Mexican-americans: A lung cancer case-control study. Cancer Epidemiol Biomarkers Prev (2000) 9(10):1021–6.
44. Markett S, Reuter M, Montag C, Weber B. The dopamine D2 receptor gene Drd2 and the nicotinic acetylcholine receptor gene Chrna4 interact on striatal Gray matter volume: Evidence from a genetic imaging study. NeuroImage (2013) 64:167–72. doi: 10.1016/j.neuroimage.2012.08.059
45. Rypma B, Di Giorgio A, Smith RM, Fazio L, D'Ambrosio E, Gelao B, et al. Drd2/Chrna5 interaction on prefrontal biology and physiology during working memory. PloS One (2014) 9(5):e95997. doi: 10.1371/journal.pone.0095997
46. Gozen O, Nesil T, Kanit L, Koylu EO, Pogun S. Nicotinic cholinergic and dopaminergic receptor mrna expression in Male and female rats with high or low preference for nicotine. Am J Drug Alcohol Abuse (2016) 42(5):556–66. doi: 10.1080/00952990.2016.1198799
47. Robinson JD, Versace F, Lam CY, Minnix JA, Engelmann JM, Cui Y, et al. The Chrna3 Rs578776 variant is associated with an intrinsic reward sensitivity deficit in smokers. Front Psychiatry (2013) 4:114. doi: 10.3389/fpsyt.2013.00114
48. Toyoda H. Interaction of nicotinic acetylcholine receptors with dopamine receptors in synaptic plasticity of the mouse insular cortex. Synapse (2019) 73(7):e22094. doi: 10.1002/syn.22094
49. Zhao-Shea R, Cohen BN, Just H, McClure-Begley T, Whiteaker P, Grady SR, et al. Dopamine D2-receptor activation elicits akinesia, rigidity, catalepsy, and tremor in mice expressing hypersensitive {Alpha}4 nicotinic receptors via a cholinergic-dependent mechanism. FASEB J (2010) 24(1):49–57. doi: 10.1096/fj.09-137034
50. Freeman GJ, Long AJ, Iwai Y, Bourque K, Chernova T, Nishimura H, et al. Engagement of the pd-1 immunoinhibitory receptor by a novel B7 family member leads to negative regulation of lymphocyte activation. J Exp Med (2000) 192(7):1027–34. doi: 10.1084/jem.192.7.1027
51. Keir ME, Liang SC, Guleria I, Latchman YE, Qipo A, Albacker LA, et al. Tissue expression of pd-L1 mediates peripheral T cell tolerance. J Exp Med (2006) 203(4):883–95. doi: 10.1084/jem.20051776
52. Dong H, Strome SE, Salomao DR, Tamura H, Hirano F, Flies DB, et al. Tumor-associated B7-H1 promotes T-cell apoptosis: A potential mechanism of immune evasion. Nat Med (2002) 8(8):793–800. doi: 10.1038/nm730
53. Dong H, Chen L. B7-H1 pathway and its role in the evasion of tumor immunity. J Mol Med (Berl) (2003) 81(5):281–7. doi: 10.1007/s00109-003-0430-2
54. Brahmer JR, Drake CG, Wollner I, Powderly JD, Picus J, Sharfman WH, et al. Phase I study of single-agent anti-programmed death-1 (Mdx-1106) in refractory solid tumors: Safety, clinical activity, pharmacodynamics, and immunologic correlates. J Clin Oncol (2010) 28(19):3167–75. doi: 10.1200/JCO.2009.26.7609
55. Topalian SL, Hodi FS, Brahmer JR, Gettinger SN, Smith DC, McDermott DF, et al. Safety, activity, and immune correlates of anti-Pd-1 antibody in cancer. N Engl J Med (2012) 366(26):2443–54. doi: 10.1056/NEJMoa1200690
56. Brahmer J, Reckamp KL, Baas P, Crino L, Eberhardt WE, Poddubskaya E, et al. Nivolumab versus docetaxel in advanced squamous-cell non-Small-Cell lung cancer. N Engl J Med (2015) 373(2):123–35. doi: 10.1056/NEJMoa1504627
57. Borghaei H, Paz-Ares L, Horn L, Spigel DR, Steins M, Ready NE, et al. Nivolumab versus docetaxel in advanced nonsquamous non-Small-Cell lung cancer. N Engl J Med (2015) 373(17):1627–39. doi: 10.1056/NEJMoa1507643
58. Herbst RS, Baas P, Kim DW, Felip E, Perez-Gracia JL, Han JY, et al. Pembrolizumab versus docetaxel for previously treated, pd-L1-Positive, advanced non-Small-Cell lung cancer (Keynote-010): A randomised controlled trial. Lancet (2016) 387(10027):1540–50. doi: 10.1016/S0140-6736(15)01281-7
59. Cho JH. Immunotherapy for non-Small-Cell lung cancer: Current status and future obstacles. Immune Network (2017) 17(6):378–91. doi: 10.4110/in.2017.17.6.378
60. Reck M, Rodríguez-Abreu D, Robinson AG, Hui R, Csőszi T, Fülöp A, et al. Pembrolizumab versus chemotherapy for Pd-L1–positive non–Small-Cell lung cancer. New Engl J Med (2016) 375(19):1823–33. doi: 10.1056/NEJMoa1606774
61. Shukuya T, Carbone DP. Predictive markers for the efficacy of anti–Pd-1/Pd-L1 antibodies in lung cancer. J Thorac Oncol (2016) 11(7):976–88. doi: 10.1016/j.jtho.2016.02.015
62. Mino-Kenudson M, Mino-Kenudson M. Programmed cell death ligand-1 (Pd-L1) expression by immunohistochemistry: Could it be predictive and/or prognostic in non-small cell lung cancer? Cancer Biol Med (2016) 13(2):157–70. doi: 10.20892/j.issn.2095-3941.2016.0009
63. Kerr KM, Tsao M-S, Nicholson AG, Yatabe Y, Wistuba II, Hirsch FR. Programmed death-ligand 1 immunohistochemistry in lung cancer: In what state is this art? J Thorac Oncol (2015) 10(7):985–9. doi: 10.1097/jto.0000000000000526
64. Cho JH, Sorensen SF, Choi Y-L, Feng Y, Kim T-E, Choi H, et al. Programmed death ligand 1 expression in paired non–small cell lung cancer tumor samples. Clin Lung Cancer (2017) 18(6):e473–e9. doi: 10.1016/j.cllc.2017.04.008
65. McLaughlin J, Han G, Schalper KA, Carvajal-Hausdorf D, Pelekanou V, Rehman J, et al. Quantitative assessment of the heterogeneity of pd-L1 expression in non–Small-Cell lung cancer. JAMA Oncol (2016) 2(1):46–54. doi: 10.1001/jamaoncol.2015.3638
66. Deb S, Nakamura S, Hayashi K, Imaoka Y, Kitamura Y, Akazawa Y, et al. Intratumoral heterogeneity of programmed cell death ligand-1 expression is common in lung cancer. PloS One (2017) 12(10):e0186192. doi: 10.1371/journal.pone.0186192
67. Calles A, Liao X, Sholl LM, Rodig SJ, Freeman GJ, Butaney M, et al. Expression of pd-1 and its ligands, pd-L1 and pd-L2, in smokers and never smokers with kras-mutant lung cancer. J Thorac Oncol (2015) 10(12):1726–35. doi: 10.1097/JTO.0000000000000687
68. D'Incecco A, Andreozzi M, Ludovini V, Rossi E, Capodanno A, Landi L, et al. Pd-1 and pd-L1 expression in molecularly selected non-Small-Cell lung cancer patients. Br J Cancer (2015) 112(1):95–102. doi: 10.1038/bjc.2014.555
69. Sterlacci W, Fiegl M, Droeser RA, Tzankov A. Expression of pd-L1 identifies a subgroup of more aggressive non-small cell carcinomas of the lung. Pathobiology (2016) 83(5):267–75. doi: 10.1159/000444804
70. Mountain CF. Revisions in the international system for staging lung cancer. Chest (1997) 111(6):1710–7. doi: 10.1378/chest.111.6.1710
71. Benowitz NL. Pharmacology of nicotine: Addiction, smoking-induced disease, and therapeutics. Annu Rev Pharmacol Toxicol (2009) 49:57–71. doi: 10.1146/annurev.pharmtox.48.113006.094742
72. Lambrechts D, Buysschaert I, Zanen P, Coolen J, Lays N, Cuppens H, et al. The 15q24/25 susceptibility variant for lung cancer and chronic obstructive pulmonary disease is associated with emphysema. Am J Respir Crit Care Med (2010) 181(5):486–93. doi: 10.1164/rccm.200909-1364OC
73. Saccone NL, Culverhouse RC, Schwantes-An TH, Cannon DS, Chen X, Cichon S, et al. Multiple independent loci at chromosome 15q25.1 affect smoking quantity: A meta-analysis and comparison with lung cancer and copd. PloS Genet (2010) 6(8):e1001053. doi: 10.1371/journal.pgen.1001053
74. Spitz MR, Amos CI, Dong Q, Lin J, Wu X. The Chrna5-A3 region on chromosome 15q24-25.1 is a risk factor both for nicotine dependence and for lung cancer. J Natl Cancer Inst (2008) 100(21):1552–6. doi: 10.1093/jnci/djn363
75. Tournier JM, Birembaut P. Nicotinic acetylcholine receptors and predisposition to lung cancer. Curr Opin Oncol (2011) 23(1):83–7. doi: 10.1097/CCO.0b013e3283412ea1
76. Truong T, Hung RJ, Amos CI, Wu X, Bickeboller H, Rosenberger A, et al. Replication of lung cancer susceptibility loci at chromosomes 15q25, 5p15, and 6p21: A pooled analysis from the international lung cancer consortium. J Natl Cancer Inst (2010) 102(13):959–71. doi: 10.1093/jnci/djq178
77. Mucchietto V, Fasoli F, Pucci S, Moretti M, Benfante R, Maroli A, et al. A9- and A7-containing receptors mediate the pro-proliferative effects of nicotine in the A549 adenocarcinoma cell line. Br J Pharmacol (2018) 175(11):1957–72. doi: 10.1111/bph.13954
78. Schuller HM, Jull BA, Sheppard BJ, Plummer HK. Interaction of tobacco-specific toxicants with the neuronal A7 nicotinic acetylcholine receptor and its associated mitogenic signal transduction pathway: Potential role in lung carcinogenesis and pediatric lung disorders. Eur J Pharmacol (2000) 393(1-3):265–77. doi: 10.1016/s0014-2999(00)00094-7
79. Grozio A, Paleari L, Catassi A, Servent D, Cilli M, Piccardi F, et al. Natural agents targeting the A7-Nicotinic-Receptor in nsclc: A promising prospective in anti-cancer drug development. Int J Cancer (2007) 122(8):1911–5. doi: 10.1002/ijc.23298
80. Al-Wadei HAN, Al-Wadei MH, Masi T, Schuller HM. Chronic exposure to estrogen and the tobacco carcinogen nnk cooperatively modulates nicotinic receptors in small airway epithelial cells. Lung Cancer (2010) 69(1):33–9. doi: 10.1016/j.lungcan.2009.09.011
81. Yang L, Lu X, Qiu F, Fang W, Zhang L, Huang D, et al. Duplicated copy of Chrna7 increases risk and worsens prognosis of copd and lung cancer. Eur J Hum Genet (2014) 23(8):1019–24. doi: 10.1038/ejhg.2014.229
82. Sun H-J, Jia Y-F, Ma X-L. Alpha5 nicotinic acetylcholine receptor contributes to nicotine-induced lung cancer development and progression. Front Pharmacol (2017) 8:573. doi: 10.3389/fphar.2017.00573
83. Zhang Y, Jia Y, Li P, li H, Xiao D, Wang Y, et al. Reciprocal activation of A5-nachr and Stat3 in nicotine-induced human lung cancer cell proliferation. J Genet Genomics (2017) 44(7):355–62. doi: 10.1016/j.jgg.2017.03.003
84. Sun Y, Li J, Zheng C, Zhou B. Study on polymorphisms in Chrna5/Chrna3/Chrnb4 gene cluster and the associated with the risk of non-small cell lung cancer. Oncotarget (2017) 9(2):2435–44. doi: 10.18632/oncotarget.23459
85. Wang Y, Zhang Y, Gu C, Bao W, Bao Y. Neuronal acetylcholine receptor subunit alpha-9 (Chrna9) polymorphisms are associated with nsclc risk in a Chinese population. Med Oncol (2014) 31(5):932. doi: 10.1007/s12032-014-0932-5
86. Bordas A, Cedillo JL, Arnalich F, Esteban-Rodriguez I, Guerra-Pastrián L, de Castro J, et al. Expression patterns for nicotinic acetylcholine receptor subunit genes in smoking-related lung cancers. Oncotarget (2017) 8(40):67878–90. doi: 10.18632/oncotarget.18948
87. Ma G, Ji D, Qu X, Liu S, Yang X, Wang G, et al. Mining and validating the expression pattern and prognostic value of acetylcholine receptors in non-small cell lung cancer. Medicine (2019) 98(20):e15555. doi: 10.1097/md.0000000000015555
88. Kwok H-H, Gao B, Chan K-H, Ip MS-M, Minna JD, Lam DC-L. Nicotinic acetylcholine receptor subunit A7 mediates cigarette smoke-induced pd-L1 expression in human bronchial epithelial cells. Cancers (2021) 13(21):5345. doi: 10.3390/cancers13215345
89. Afrashteh Nour M, Kheradmand F, Rasmi Y, Asadzadeh Z, Doustvandi MA, Jafarlou M, et al. Nicotinic acetylcholine receptor subunit alpha-7 mediates pd-L1 and ctla-4 expression in Hepg2 cells. ImmunoAnalysis (2021) 1(1):10. doi: 10.34172/ia.2021.10
90. Nguyen HD, Liao Y-C, Ho Y-S, Chen L-C, Chang H-W, Cheng T-C, et al. The A9 nicotinic acetylcholine receptor mediates nicotine-induced pd-L1 expression and regulates melanoma cell proliferation and migration. Cancers (2019) 11(12):1991. doi: 10.3390/cancers11121991
91. Chellappan S, Murayama MA, Takada E, Takai K, Arimitsu N, Shimizu J, et al. Nicotine treatment regulates pd-L1 and pd-L2 expression Via inhibition of akt pathway in Her2-type breast cancer cells. PloS One (2022) 17(1):e0260838. doi: 10.1371/journal.pone.0260838
92. Yeo CD, Kim IK, Ban WH, Kang HS, Kim JW, Kim SJ, et al. Chronic nicotine exposure affects programmed death-ligand 1 expression and sensitivity to epidermal growth factor receptor-tyrosine kinase inhibitor in lung cancer. Trans Cancer Res (2019) 8(S4):S378–S88. doi: 10.21037/tcr.2019.05.02
93. Zhu P, Kang G, Jiao Y, Gui C, Fan H, Li X, et al. The A5-Nachr/Pd-L1 axis facilitates lung adenocarcinoma cell migration and invasion. Hum Cell (2022) 35(4):1207–18. doi: 10.1007/s13577-022-00709-1
94. Liu Y-S, Huang B-R, Lin C-J, Shen C-K, Lai S-W, Chen C-W, et al. Paliperidone inhibits glioblastoma growth in mouse brain tumor model and reduces pd-L1 expression. Cancers (2021) 13(17):4357. doi: 10.3390/cancers13174357
95. Rubí B, Maechler P. Minireview: New roles for peripheral dopamine on metabolic control and tumor growth: Let’s seek the balance. Endocrinology (2010) 151(12):5570–81. doi: 10.1210/en.2010-0745
96. Fujii T, Mashimo M, Moriwaki Y, Misawa H, Ono S, Horiguchi K, et al. Expression and function of the cholinergic system in immune cells. Front Immunol (2017) 8:1085. doi: 10.3389/fimmu.2017.01085
97. Zhang X, Liu Q, Liao Q, Zhao Y. Potential roles of peripheral dopamine in tumor immunity. J Cancer (2017) 8(15):2966–73. doi: 10.7150/jca.20850
Keywords: lung cancer, NSCLC, smoking, nAChR, DRD2, PD-L1
Citation: Pal K, Hussain T, Xie H, Li S, Yang P, Mansfield A, Lou Y, Chowdhury S and Mukhopadhyay D (2022) Expression, correlation, and prognostic significance of different nicotinic acetylcholine receptors, programed death ligand 1, and dopamine receptor D2 in lung adenocarcinoma. Front. Oncol. 12:959500. doi: 10.3389/fonc.2022.959500
Received: 01 June 2022; Accepted: 21 July 2022;
Published: 22 August 2022.
Edited by:
Khalil Hajiasgharzadeh, Tabriz University of Medical Sciences, IranReviewed by:
Alireza Mani, University College London, United KingdomNarges Dastmalchi, University College of Nabi Akram, Iran
Behzad Baradaran, Tabriz University of Medical Sciences, Iran
Copyright © 2022 Pal, Hussain, Xie, Li, Yang, Mansfield, Lou, Chowdhury and Mukhopadhyay. This is an open-access article distributed under the terms of the Creative Commons Attribution License (CC BY). The use, distribution or reproduction in other forums is permitted, provided the original author(s) and the copyright owner(s) are credited and that the original publication in this journal is cited, in accordance with accepted academic practice. No use, distribution or reproduction is permitted which does not comply with these terms.
*Correspondence: Debabrata Mukhopadhyay, bXVraG9wYWRoeWF5LmRlYmFicmF0YUBtYXlvLmVkdQ==
†Present address: Tabish Hussain, The University of Texas MD Anderson Cancer Center, TX, United States
Hao Xie, Division of Medical Oncology, Moffitt Cancer Center, Tampa, FL, United States
‡These authors have contributed equally to this work
§These authors share senior authorship