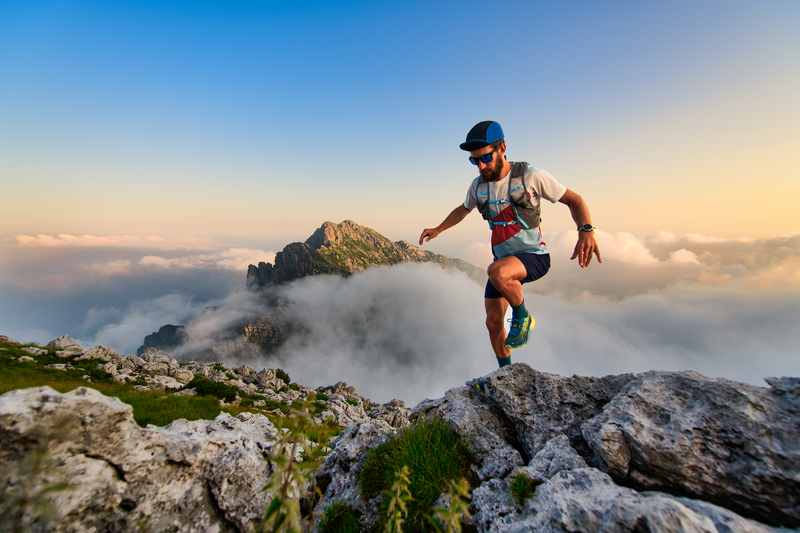
95% of researchers rate our articles as excellent or good
Learn more about the work of our research integrity team to safeguard the quality of each article we publish.
Find out more
REVIEW article
Front. Oncol. , 01 August 2022
Sec. Gastrointestinal Cancers: Colorectal Cancer
Volume 12 - 2022 | https://doi.org/10.3389/fonc.2022.954329
This article is part of the Research Topic From Precancerous Lesions to Anal Cancer: Screening, Diagnosis and Treatment View all 9 articles
Metabolic reprogramming plays a critical role in colorectal cancer (CRC). It contributes to CRC by shaping metabolic phenotypes and causing uncontrolled proliferation of CRC cells. Glucose metabolic reprogramming is common in carcinogenesis and cancer progression. Growing evidence has implicated the modifying effects of non-coding RNAs (ncRNAs) in glucose metabolic reprogramming and chemoresistance in CRC. In this review, we have summarized currently published studies investigating the role of ncRNAs in glucose metabolic alterations and chemoresistance in CRC. Elucidating the interplay between ncRNAs and glucose metabolic reprogramming provides insight into exploring novel biomarkers for the diagnosis and prognosis prediction of CRC.
Colorectal cancer (CRC) is one of the most common digestive cancers, with increasing incidence and mortality in young adults in particular (1, 2). CRC is the secondly most common cancer worldwide with the incidence and mortality of 12% and 7%, respectively. Identifying novel biomarkers for the early diagnosis and treatment of CRC is very important. Targeted therapy for CRC has been promoted with progress in the high-throughput sequencing technology. Glucose metabolic reprogramming is a hallmark of cancer, which plays a critical role during carcinogenesis (3). The glucose metabolic pathways primarily include aerobic glycolysis, gluconeogenesis, and pentose phosphate pathway (PPP). Aerobic glycolysis is called Warburg effect, which is common in most aggressive cancer cells. It has been well documented that abnormal aerobic glycolysis is closely related to cancer growth and survival (4). Besides, disorders of glucose metabolic reprogramming in immune cells can lead to microenvironment imbalance and affect anti-tumor immunity (5). Research in metabolomics has suggested those key molecules underlying the metabolic mechanisms would serve as optimal approaches for CRC diagnosis and prognosis prediction. Blocking the glucose metabolic reprogramming in immune cells can help to defend against cancer.
A growing number of studies have implicated that noncoding RNAs (ncRNAs) are involved in tumor initiation and progression, primarily including circular RNA (circRNA), long noncoding RNA (lncRNA) and microRNA (miRNA) (6, 7). The ncRNAs regulatory networks are essential for tumorigenesis, tumor invasion and metastasis. Some ncRNAs act as oncogenic drivers, while some other ncRNAs function as tumor suppressors. They can regulate numerous molecular targets through RNA-RNA or RNA-protein interactions. Increasing evidence has supported that ncRNAs participate in the glucose metabolic reprogramming of cancer by targeting metabolism-associated with genes, such as glucose transporter 1 (GLUT1), hypoxia-induced factor-1a (HIF-1a), and glycogen synthase 1 (GYS1). Some ncRNAs may also influence the chemoresistance to CRC. Accumulated studies have investigated the crucial role of ncRNAs in regulating glucose metabolism and chemoresistance in CRC in the past few years (8–10). The identification of ncRNA-based glucose metabolism regulatory networks is currently emerging as a promising approach in the field of early screening and targeted therapy of CRC. In this review, we aim to elucidate the molecular targets regulated by ncRNAs that might be involved in the glucose metabolic reprogramming and chemoresistance of CRC.
It has been well documented that abnormal glucose metabolism is one of the leading causes for CRC development (11). Usually, normal tissues acquire energy through the aerobic oxidative phosphorylation but undergo anaerobic glycolysis under hypoxia. However, the main way for acquiring energy of cancer cells is aerobic glycolysis even under the condition of adequate oxygen. Aerobic glycolysis is typical in the metabolic reprogramming of CRC. Abnormal glucose metabolism in CRC cells is primarily attributed to the mitochondrial dysfunction, abundant activation of key enzymes involved in glycolysis, altered isozyme profiles and dysregulated glucose metabolic signaling pathways (12, 13).
Accumulated studies have demonstrated that miRNAs play important roles in CRC by transcriptionally regulating specific mRNAs (14). They are single-stranded noncoding small RNAs with about 22 nucleotides. MiRNAs confer their biological effects by specifically complementary recognition of the miRNA response elements to the 3’ untranslated region (3’UTR) of mRNAs. Many miRNAs have been implicated in regulating cancer metabolic reprogramming. With advance in high-throughput technology, some miRNAs have been demonstrated to confer effects on the metabolic interactions between CRC cells and gut microbiota, suggesting the critical role of miRNAs in mediating tumor-microbiota metabolic interplays (15). To the best of our knowledge, butyrate produced by the gut microbiota provides approximately 70% of energy needs for the colonic epithelial cells. Aberrant expression of miRNAs can influence glucose metabolism mediated by butyrate in cancer cells through the gut-brain axis (16–18). Nonetheless, little is known of the potential interactions between miRNAs and gut microbiota in CRC glucose metabolic reprogramming. Current progress in the role of miRNAs in the glucose metabolism of CRC has been summarized in Table 1. The association between miRNAs and gut microbiota-mediated glucose metabolism in CRC has also been elucidated in the following context.
There are many glucose metabolism-associated miRNAs dysregulated in CRC, such as miR-4999-5p, miR-181d, and miR-24 (19–21) (Table 1). Increased expression of miR-4999-5p has been demonstrated in CRC, which can also modulate the glucose metabolic reprogramming of CRC cells by targeting the critical molecule of mTOR signaling pathway, namely, AMP-Activated Protein Kinase (PRKAA2) (19). It promotes glycolysis in CRC cells (19). MiR-26a overexpression can increase the production of pyruvate but decrease the generation of acetyl coenzyme A by suppressing pyruvate dehydrogenase protein X component (PDHX) in HCT116 cells, which suggests the critical of miR-26a in reprograming glucose metabolism in CRC (22). Jin F and the colleagues have illustrated that HIF-1α-induced miR-23a∼27a∼24 cluster promoted the progression of CRC by reprogramming glucose metabolism from oxidative phosphorylation to glycolysis (20). MiRNAs of miR-124, miR-137 and miR-340 have been documented to regulate Warburg effect by alternatively splicing pyruvate kinase isozyme (PKM) gene and controlling the ratio of PKM1/PKM2 in CRC cells (29). Another study by Qiu Z et al. have reported that miR-124 reduced PPP by regulating phosphoribosyl pyrophosphate synthetase 1 (PRPS1) and ribose-5-phosphate isomerase-A (RPIA) in CRC (33). Taken together, these findings have suggested the pivotal role of miRNAs in regulating glucose metabolism in CRC. MiRNAs exert biological effects on the glucose metabolism of CRC cells by regulating different genes. It must be mentioned that the targeted genes participating in the glucose metabolic reprogramming of CRC are diverse and complicated. The same miRNA may have different targets, while some different miRNAs may simultaneously target the same gene.
There are a couple of key enzymes and transporters regulating cancer cells glycolysis, such as GLUT1, PKM2, pyruvate dehydrogenase kinase isoform 2 (PDK2), and Lactate dehydrogenase A (LDHA). A number of miRNAs are implicated in targeting the key enzymes or transporters involved in cancer cells glycolysis (Table 1 and Figure 1). Zhao J et al. have reported that miR-143 downregulated the expression of GLUT1 and inhibited glucose uptake in CRC cells (23). Besides, miR-328 is also involved in regulating the Warburg effect by targeting GLUT1 in CRC cells (28). PKM2 is another key enzyme involved in glycolysis. MiR-339-5p has been well documented to restrain CRC cells glycolysis and growth by downregulating PKM2 (27). Similarly, the study by Fu R and the colleagues have found that hnRNPA1 was a direct target of miR-206, which suppressed PKM2 expression and attenuated Warburg effect of CRC cells (35). Moreover, the upregulation of miR-142-5p inhibits the intake of oxygen but facilitates aerobic glycolysis of CRC cells by targeting the key enzyme of succinate dehydrogenase-B(SDHB) (30). Elevated glucose consumption and lactate generation is found in miR-142-5p-treated CRC cells (30). LDHA is identified as the targeted gene of miR-374a, which refines the aerobic glycolysis of CRC cells (31). Apart from the above-mentioned key enzymes and transporters in glycolysis, hexokinase 2 (HK2) is a critical rate-limiting enzyme for glycolysis. It has been demonstrated to be the direct target of miR-143, miR-4458, and miR-98 in CRC cells (32, 34). Suppression of miR-143 contributes to the shift towards aerobic glycolysis in CRC via targeting HK2 (32). MiR-4458 is demonstrated to prevent from glycolysis and lactate production by directly regulating HK2, which thus inhibits the progression of CRC (40). Accordingly, those aberrantly expressed miRNAs and targeted molecules involved in glycolysis would serve as helpful targets for CRC.
Figure 1 NcRNAs regulate key enzymes/transporters involved in glucose metabolism of CRC through complicated signaling pathways.
In the last decade, some exosomes-delivering miRNAs have been well documented to participate in regulating glucose metabolism in CRC by targeting key metabolic genes of HIF-1α and PGK1, such as exosomal miR-6869-5p, miR-8075, miR-5787, and miR-548c-5p (45). Let-7a is demonstrated to be enriched in extracellular vesicles (EVs) derived from CRC cells (42). The EVs-derived let-7a can promote the mitochondrial oxidative phosphorylation via downregulating SNAP23 in CRC cells (42). Tao L et al. have reported that exosomal miR-101-3p acts as an oncomiR in CRC, which promoted glycolysis and influenced metabolic homeostasis by targeting homeodomain-interacting protein kinase (HIPK3) in CRC cells (44). However, how those miRNAs being encapsulated in EVs and transferred to cancer cells remains largely unknown. More future studies are encouraged to elucidate the underlying molecular mechanism of EVs-delivering miRNAs in regulating glucose metabolic reprogramming in CRC.
Most interestingly, certain miRNAs are involved in regulating the chemoresistance and glucose metabolism in CRC. MiR-149-3p has been found to promote 5-Fluorouracil (5-FU)-induced CRC cells apoptosis and inhibit the glucose metabolism by targeting PDK2 (24). MiR-488 is obviously decreased in metastatic/recurrent CRC, which can also refine the chemoresistance and glycolysis of CRC by targeting a key enzyme involved in glucose metabolism, namely, phosphofructokinase-2/fructose-2,6-bisphosphatase 3 (PFKFB3) (25). The study by Park GB et al. has shown the evidence that miR-125b-5p could significantly inhibit lactate generation and the chemoresistance of CRC cells to oxaliplatin and 5-fluorouracil (26). PKM2 is a critical enzyme for glycolysis, which has been elaborated to be targeted by miR-122 involved in regulating glucose metabolism of CRC cells (37). Furthermore, miR-122 can inhibit glycolysis and serve as a useful therapeutic strategy overcoming 5-FU chemoresistance in CRC (37). Apart from miR-122, miR-34a is also implicated in regulating the sensitivity to 5-FU in CRC by refining glycolysis, suggesting the critical role of miRNAs during interactions between chemoresistance and glycolysis (38). Taken together, some miRNAs can function as therapeutic targets in patients with chemoresistant CRC, including miR-149-3p, miR-122 and miR-34a. Nevertheless, the possible regulatory mechanism in mediating chemoresistance and glucose metabolism balance in CRC needs to be investigated in the future.
Currently identified lncRNAs associated with glucose metabolism in CRC have been summarized in Table 2. As shown in Figure 1, some lncRNAs exert effects through RNA-protein or RNA-polypeptide interactions, while some lncRNAs regulate targeted genes via lncRNA-miRNA-mRNA competitive endogenous RNA (ceRNA) regulatory networks (46–50). A recent study has supported that lncRNA ZEB2-AS1 promoted glycolysis and regulated the expression of transforming growth factor β-activated kinase-binding protein 3 (TAB3) by adsorbing miR-188 (51). A LINC00265/miR-216b-5p/TRIM44 axis has been assured in promoting glycolysis and lactate production in CRC (52). LncRNA RAD51-AS1 is documented to bind with miR-29b and facilitate the expression of c-3p/NDRG2, which thus inhibits the glycolysis of CRC cells (53). Tang J et al. have found that lncRNA GLCC1 could enhance aerobic glycolysis by stabilizing transcriptional factor c-Myc and interacting with HSP90 chaperon (8). Similar effect of lncRNA LINRIS on aerobic glycolysis in CRC cells has been illustrated in the study by Wang Y et al. (54). LINRIS is identified to be upregulated in CRC tissues and plays an oncogenic role in CRC by promoting aerobic glycolysis via LINRIS-IGF2BP2-MYC axis (54). However, another study has suggested lncRNA MEG3 refined the aerobic glycolysis by depredating c-Myc in CRC cells (55). The transcription factor Yin Yang 1 (YY1) is demonstrated to regulate the expression of lncRNA MIR31HG, which can also forms a positive feedback via upregulating YY1 and sponging miR-361-3p (56). MIR31HG promotes the growth, glycolysis and lung metastasis of CRC cells (56). A recent study has suggested that transcription factor HIF-1a could increase the expression of lncRNA PTTG3P and contribute to glycolysis and M2 macrophage polarization in CRC (57). Accordingly, lncRNAs may serve as regulators for tumor-associated transcriptional factors of c-Myc and YY1, which can also facilitate the expression of certain lncRNAs via transcriptional activation loop.
To the best of our knowledge, N6-methyladenine (m6A) modulators contribute to CRC. It has been shown that some lncRNAs can be regulated by m6A modulators. However, little is known about the mechanism of m6A reader in regulating glycolysis in CRC. IMP2, namely IGF2BP2, is a m6A reader. LncRNA ZFAS1 is found to augment the hydrolysis of adenosine triphosphate (ATP) and glycolysis by recruiting Obg-like ATPase 1 (OLA1) in CRC, which can be stabilized by IMP2 in an m6A-dependent manner (47). More studies are warranted to explore the precise effects of certain lncRNAs on altering m6A and glycolysis of CRC cells.
Most interestingly, the recent studly by Hong J et al. has implicated that lncRNA ENO1-IT1 was involved in promoting Fusobacterium nucleatum (F. nucleatum)-mediated glycolysis and oncogenesis via targeting histone modification-associated gene enolase1-intronic transcript 1 (ENO1) in CRC (58), suggesting a complicated interaction between microbiome and glycometabolic lncRNA. Targeting ENO1-IT1 may be useful for CRC patients with increased F. nucleatum in gut. More future studies are warranted to elucidate the potential association between gut microbiota and glucose-associated ncRNAs in regulating glucose metabolic reprogramming in CRC.
LncRNA RAD51-AS1 has been shown to hamper glucose consumption and lactate production by inhibiting the key glycolysis enzyme HK2 and GLUT1 in CRC cells (53). Similar findings have been demonstrated in other studies published previously (59–61). LncRNA UCA1 is found to promote glycolysis via upregulating HK2 and LDHA in CRC cells (59). LncRNA HULC has been demonstrated to bind LDHA and PKM2 and thus promote aerobic glycolysis (74). Yan T, et al. (60) have reported that lncSLCC1 was upregulated in CRC and promoted glycolysis by transcriptionally activating HK2 (60). Another well documented lncRNA interacting with HK2 is lncRNA FGD5-AS1, which has been illustrated to promote glycolysis through the miR-330-3p-HK2 signaling network (67). Moreover, a lncRNA DANCR-miR-125b-5p-HK2 axis has been well established in colon cancer cells, which can promote aerobic glycolysis (75). Additionally, MCF2L-AS1 is found to be enriched in tissues of CRC, which enhances the glycolysis of CRC cells via MCF2L-AS1/miR-874-3p/FOXM1 ceRNA axis and upregulates GLUT1 and LDHA (63). It has been elaborated that lncARSR can sponge miR-34a-5p and promote hexokinase 1(HK1)-mediated glycolysis in CRC (62). Besides, high level of lncARSR predicts poor survival of CRC (62). Similarly, the study by Zhao S, et al. has shown the evidence that lncRNA MIR17HG facilitated HK1 expression by acting as a ceRNA for miR-138-5p (65). MIR17HG promotes glycolysis and the liver metastasis of CRC (65). Furthermore, LncRNA SPRY4-IT1 has been demonstrated to enhance CRC cell growth and glycolysis by promoting phosphoinositide-dependent kinase 1 (PDK1) expression (70). Similar altering effect of lncRNA MAFG-AS1 has been illustrated to promote PDK1 expression in CRC (72). Taken together, lncRNAs participate in CRC glycolysis primarily by regulating the key glycolysis-associated enzymes of HK1, HK2, PDK1, and crucial transporter GLUT1 (Figure 1). Elucidating the underlying mechanism and targets of lncRNAs in regulating glycolysis is helpful for exploring more effective strategies for the diagnosis and treatment of CRC.
HIF-1a is a key transcriptional factor for hypoxia-induced glycolysis in cancer, which is differentially regulated by diverse lncRNAs. LINC00525 is documented to activate HIF-1α, increase the expression of ubiquitin-conjugating enzyme E2Q family member 1 (UBE2Q1), and enhance hypoxia-enhanced glycolysis through miR-338-3p/UBE2Q1/β-catenin axis in CRC (46). Similarly, lnc-RP11-536 K7.3 plays an oncogenic role in CRC by promoting the angiogenesis, glycolysis, and chemo-resistance in CRC through the SOX2/USP7/HIF-1α signaling pathway (66). The study by Yu Z et al. has reported that miR-20b-5p was bound with lncRNA COL4A2-AS1, which facilitated the glycolysis of CRC cells by activating HIF-1α (68). Accordingly, HIF-1a-dependent lnRNAs serve as promising approaches for CRC treatment by controlling glucose metabolic balance.
As a key enzyme in glycolysis, PKM2 has been demonstrated to be regulated by several lncRNAs. For instance, lncRNA XIST facilitates glycolysis of CRC cells by upregulating PKM2 through XIST/miR-137/PKM2 axis (69). Similarly, lncRNA SNHG6 plays a critical role in the glucose metabolism of CRC, which specifically splices PKM mRNA, increases PKM2/PKM1 ratio and promotes the glycolysis in CRC (71). FEZF1-AS1 has been shown to promote the pyruvate kinase activity and aerobic glycolysis by targeting PKM2 in CRC cells (73). Taken together, lncRNA-PKM2 axis is critical in regulating CRC glycolysis.
Accumulating studies have suggested the critical role of lncRNAs in chemoresistance in cancer. Some lncRNAs have also been implicated to regulate the glucose metabolism and chemoresistance in CRC. The study by Li Q et al. have identified an oncogenic gene lnc-RP11-536 K7.3, which enhanced the glycolysis and chemoresistance to oxaliplatin in CRC via SOX2/USP7/HIF-1α signaling axis (66). Pyruvate dehydrogenase kinase 4 (PDK4) is a crucial enzyme for glucose metabolism. LncRNA HCG11 has been validated to facilitate 5-FU resistance by sponging miR-144-3p and upregulating PDK4 in CRC (64). Similar to HCG11, lncRNA FGD5-AS1 promotes glycolysis and 5-FU resistance of CRC cells by acting as a ceRNA for miR-330-3p (67). Besides, lncRNA UCA1 is documented to contribute to paclitaxel (Taxol)-resistance and promote glycolysis by facilitating the expression of HK2 and LDHA in CRC (59). Moreover, lncRNA XIST is demonstrated to promoting 5-FU/cisplatin-resistance and glycolysis in CRC by increasing the ratio of PKM2/PKM1, while miR-137 mimics can alleviate the facilitating effect of XIST (69). Accordingly, lncRNAs involved in regulating CRC glycolysis and chemoresistance will serve as novel anticancer strategies for CRC in the future.
Table 3 has shown the aberrantly expressed circRNAs in CRC. Some circRNAs function as onco-circRNAs, while some others act as cancer-suppressors. CircTADA2A has been reported to inhibit the cell cycle, glycolysis of CRCs but significantly promote CRC cells apoptosis (76). CircNOX4 has been identified as an oncogenic circRNA in CRC by enhancing the glycolysis and controlling the expression of CDC28 protein kinase regulatory subunit 1B(CKS1B) in CRC cells through the circNOX4/miR-485-5p/CSK1B axis (77). Knockdown of circ_0000231 can inhibit glycolysis and the growth of CRC cells by sponging miR-502-5p, which binds to myosin VI (MYO6) (78). A recent published study has suggested that circPLCE1 could also function as a ceRNA binding with miR-485-5p and expedite epithelial mesenchymal transformation (EMT) and glycolysis of CRC cells (79). Besides, circPLCE1 is capable of promoting tumor-associated macrophage (TAM) polarization towards M2 via upregulating γ-Actin Gene (ACTG1) but inhibiting miR-485-5p in CRC (79). Furthermore, silencing of circ-RNF121 represses the growth and glycolysis of CRC cells, which can act as a sponge for miR-1224-5p and target forkhead box M1 (FOXM1) (80). Most importantly, circ-RNF121 can be packaged into exosomes and thus contributes to intercellular communications and regulates glycolysis in CRC (80). Apart from circ-RNF121, exosomes-delivering ciRS-122 is involved in promoting the glycolysis of CRC cells through ciRS-122/miR-122/PKM2 ceRNA network (81). Accordingly, circRNA is capable of acting as a sponge of specific miRNA and thus participates in the Warburg effect by regulating glycolysis-associated genes in CRC (Figure 1). Some circRNAs can be delivered by exosomes and mediate glucose metabolic reprogramming in CRC, including circ-RNF121 and ciRS-122 (Figure 1).
The study by Zhang Z et al. has demonstrated that circDENND4C was upregulated in CRC, which promoted the proliferation, migration, and glycolysis of CRC cells by acting as a ceRNA for miR-760 and regulating GLUT1 (82). Besides, 6-phosphofructo-2-kinase/fructose-2,6-bisphosphatase isotype 3 (PFKFB3) is a pivotal enzyme for glucose metabolism. Gao Y and the colleagues have found circSAMD4A could facilitate the expression of PFKFB3 and promote glycolysis by sponging miR-545-3p (83). Moreover, PKM2 is another crucial enzyme for glycolysis in cancer. Exosomes from oxaliplatin-resistant CRC cells can deliver ciRS-122 to oxaliplatin-sensitive cells, which thereby upregulates PKM2 expression and promotes the glycolysis and drug resistance of CRC cells (81). Li Q et al. have reported that circACC1 played a critical role during the metabolic reprogramming of CRC cells by regulating adenosine monophosphate-activated protein kinase (AMPK) (84). CircACC1 enhances both fatty acid β-oxidation and glycolysis in CRC cells by activating AMPK (84). Taken together, those key enzymes or transporters targetedly regulated by circRNAs have suggested novel markers for CRC diagnosis and treatment by controlling glucose metabolism.
Some dysregulated circRNAs participate in regulating chemoresistance and glycolysis in CRC, such as circSAMD4A (83) and ciRS-122 (81). CircSAMD4A contributes to 5-Fu resistance via targeting miR-545-3p/PFKFB3 and regulating glycolysis of CRC cells, while knockdown of circSAMD4A improves the sensitivity of 5-Fu (83). Exosomes-derived ciRS-122 is capable of promoting glycolysis and making chemosensitive-CRC cells transform into chemoresistant-CRC cells via miR-122/PKM2 axis (81). Accumulating studies have implicated the important role of circRNA in regulating immune metabolic reprogramming and immune microenvironment balance in carcinogenesis (85). CircPLCE1 has been documented to promote TAM polarization towards M2 through miR-485-5p/ACTG1 axis in CRC, which plays a critical role in regulating CRC immune microenvironment balance (79). All these findings have provided a promising circRNA-targeted therapy for CRC by shaping cancer glucose metabolism, immune microenvironment balance and cancer cells chemoresistance.
In conclusion, ncRNA-based glucose metabolic reprogramming and chemoresistance have provided promising prospects for CRC. Elucidating the interaction and possible mechanism between ncRNAs and metabolic reprogramming has shed some insights into understanding the pathogenesis and drug resistance mechanisms of CRC. Novel biomarkers for the diagnosis, chemoresistance intervention and prognosis prediction of CRC can be investigated in more future studies. Most importantly, it is urgent to search for sufficient evidence supporting the practical clinical applications of ncRNAs in CRC.
SY, DX and ZG designed the manuscript. SW, WD, JC, SY and XW performed literature research and drafted the article. MC, DX and ZG revised the review. All authors have read and approved the final article. All authors contributed to the article and approved the submitted version.
This study is funded by National Natural Science Foundation, China (82003042, 82171790, and 81870237), and Natural Science Foundation of Shandong Province, China (ZR2020KC001).
The authors declare that the research was conducted in the absence of any commercial or financial relationships that could be construed as a potential conflict of interest.
All claims expressed in this article are solely those of the authors and do not necessarily represent those of their affiliated organizations, or those of the publisher, the editors and the reviewers. Any product that may be evaluated in this article, or claim that may be made by its manufacturer, is not guaranteed or endorsed by the publisher.
CRC, colorectal cancer; ncRNAs, non-coding RNAs; PPP, pentose phosphate pathway; circRNA, circular RNA; lncRNA, long noncoding RNA; miRNA, microRNA; GLUT1, glucose transporter 1; HIF-1a, hypoxia-induced factor-1a; GYS1,glycogen synthase; PDHX, pyruvate dehydrogenase protein X component; 3’UTR,3’ untranslated region; PKM, pyruvate kinase isozyme; HK1, hexokinase 1; HK2, hexokinase 2; SDHB, succinate dehydrogenase-B; PRKAA2, AMP-Activated Protein Kinase; GLUT1, glucose transporter 1; PDK2, pyruvate dehydrogenase kinase isoform 2; LDHA, Lactate dehydrogenase A; EVs, extracellular vesicles; HIPK3, homeodomain-interacting protein kinase; ceRNA, competitive endogenous RNA; TAB3, transforming growth factor β-activated kinase-binding protein 3; YY1, Yin Yang 1; UBE2Q1, ubiquitin-conjugating enzyme E2Q family member 1; ATP, adenosine triphosphate; OLA1, glycolysis by recruiting Obg-like ATPase 1; AMPK, adenosine monophosphate-activated protein kinase; TAM, tumor-associated macrophage; ACTG1, γ-Actin Gene; FOXM1, forkhead box M1.
1. Siegel RL, Miller KD, Goding Sauer A, Fedewa SA, Butterly LF, Anderson JC, et al. Colorectal cancer statistics, 2020. CA Cancer J Clin (2020) 70:145–64. doi: 10.3322/caac.21601
2. Miller KD, Fidler-Benaoudia M, Keegan TH, Hipp HS, Jemal A, Siegel RL. Cancer statistics for adolescents and young adults, 2020. CA Cancer J Clin (2020) 70:443–59. doi: 10.3322/caac.21637
3. Shen Y, Sun M, Zhu J, Wei M, Li H, Zhao P, et al. Tissue metabolic profiling reveals major metabolic alteration in colorectal cancer. Mol Omics. (2021) 17:464–71. doi: 10.1039/d1mo00022e
4. Nannini G, Meoni G, Amedei A, Tenori L. Metabolomics profile in gastrointestinal cancers: Update and future perspectives. World J Gastroenterol (2020) 26:2514–32. doi: 10.3748/wjg.v26.i20.2514
5. Shang S, Ji X, Zhang L, Chen J, Li C, Shi R, et al. Macrophage ABHD5 suppresses NFkappaB-dependent matrix metalloproteinase expression and cancer metastasis. Cancer Res (2019) 79:5513–26. doi: 10.1158/0008-5472.CAN-19-1059
6. Jung G, Hernandez-Illan E, Moreira L, Balaguer F, Goel A. Epigenetics of colorectal cancer: biomarker and therapeutic potential. Nat Rev Gastroenterol Hepatol (2020) 17:111–30. doi: 10.1038/s41575-019-0230-y
7. Chan JJ, Tay Y. Noncoding RNA:RNA regulatory networks in cancer. Int J Mol Sci (2018) 19:1310. doi: 10.3390/ijms19051310
8. Tang J, Yan T, Bao Y, Shen C, Yu C, Zhu X, et al. LncRNA GLCC1 promotes colorectal carcinogenesis and glucose metabolism by stabilizing c-myc. Nat Commun (2019) 10:3499. doi: 10.1038/s41467-019-11447-8
9. Li W, Lu Y, Ye C, Ouyang M. The regulatory network of MicroRNA in the metabolism of colorectal cancer. J Cancer. (2021) 12:7454–64. doi: 10.7150/jca.61618
10. Chu J, Fang X, Sun Z, Gai L, Dai W, Li H, et al. Non-coding RNAs regulate the resistance to anti-EGFR therapy in colorectal cancer. Front Oncol (2021) 11:801319. doi: 10.3389/fonc.2021.801319
11. Deng F, Zhou R, Lin C, Yang S, Wang H, Li W, et al. Tumor-secreted dickkopf2 accelerates aerobic glycolysis and promotes angiogenesis in colorectal cancer. Theranostics. (2019) 9:1001–14. doi: 10.7150/thno.30056
12. Song L, Dong N, Li Z. P,p’-dichlorodiphenyltrichloroethane promotes aerobic glycolysis via reactive oxygen species-mediated extracellular signal-regulated kinase/M2 isoform of pyruvate kinase (PKM2) signaling in colorectal cancer cells. Environ Toxicol (2020) 35:333–45. doi: 10.1002/tox.22869
13. Shi T, Ma Y, Cao L, Zhan S, Xu Y, Fu F, et al. B7-H3 promotes aerobic glycolysis and chemoresistance in colorectal cancer cells by regulating HK2. Cell Death Dis (2019) 10:308. doi: 10.1038/s41419-019-1549-6
14. Wang H. MicroRNAs and apoptosis in colorectal cancer. Int J Mol Sci (2020) 21:5353. doi: 10.3390/ijms21155353
15. Yuan C, Subramanian S. microRNA-mediated tumor-microbiota metabolic interactions in colorectal cancer. DNA Cell Biol (2019) 38:281–5. doi: 10.1089/dna.2018.4579
16. Siddiqui MT, Cresci GAM. The immunomodulatory functions of butyrate. J Inflammation Res (2021) 14:6025–41. doi: 10.2147/JIR.S300989
17. Guz M, Jeleniewicz W, Malm A, Korona-Glowniak I. A crosstalk between diet, microbiome and microRNA in epigenetic regulation of colorectal cancer. Nutrients. (2021) 13:2428. doi: 10.3390/nu13072428
18. Han R, Sun Q, Wu J, Zheng P, Zhao G. Sodium butyrate upregulates miR-203 expression to exert anti-proliferation effect on colorectal cancer cells. Cell Physiol Biochem (2016) 39:1919–29. doi: 10.1159/000447889
19. Zhang Q, Hong Z, Zhu J, Zeng C, Tang Z, Wang W, et al. miR-4999-5p predicts colorectal cancer survival outcome and reprograms glucose metabolism by targeting PRKAA2. Onco Targets Ther (2020) 13:1199–210. doi: 10.2147/OTT.S234666
20. Jin F, Yang R, Wei Y, Wang D, Zhu Y, Wang X, et al. HIF-1alpha-induced miR-23a approximately 27a approximately 24 cluster promotes colorectal cancer progression via reprogramming metabolism. Cancer Lett (2019) 440-441:211–22. doi: 10.1016/j.canlet.2018.10.025
21. Guo X, Zhu Y, Hong X, Zhang M, Qiu X, Wang Z, et al. miR-181d and c-myc-mediated inhibition of CRY2 and FBXL3 reprograms metabolism in colorectal cancer. Cell Death Dis (2017) 8:e2958. doi: 10.1038/cddis.2017.300
22. Chen B, Liu Y, Jin X, Lu W, Liu J, Xia Z, et al. MicroRNA-26a regulates glucose metabolism by direct targeting PDHX in colorectal cancer cells. BMC Cancer. (2014) 14:443. doi: 10.1186/1471-2407-14-443
23. Zhao J, Chen Y, Liu F, Yin M. Overexpression of miRNA-143 inhibits colon cancer cell proliferation by inhibiting glucose uptake. Arch Med Res (2018) 49:497–503. doi: 10.1016/j.arcmed.2018.12.009
24. Liang Y, Hou L, Li L, Li L, Zhu L, Wang Y, et al. Dichloroacetate restores colorectal cancer chemosensitivity through the p53/miR-149-3p/PDK2-mediated glucose metabolic pathway. Oncogene. (2020) 39:469–85. doi: 10.1038/s41388-019-1035-8
25. Deng X, Li D, Ke X, Wang Q, Yan S, Xue Y, et al. Mir-488 alleviates chemoresistance and glycolysis of colorectal cancer by targeting PFKFB3. J Clin Lab Anal (2021) 35:e23578. doi: 10.1002/jcla.23578
26. Park GB, Jeong JY, Kim D. GLUT5 regulation by AKT1/3-miR-125b-5p downregulation induces migratory activity and drug resistance in TLR-modified colorectal cancer cells. Carcinogenesis. (2020) 41:1329–40. doi: 10.1093/carcin/bgaa074
27. Wu H, Cui M, Li C, Li H, Dai Y, Cui K, et al. Kaempferol reverses aerobic glycolysis via miR-339-5p-Mediated PKM alternative splicing in colon cancer cells. J Agric Food Chem (2021) 69:3060–8. doi: 10.1021/acs.jafc.0c07640
28. Santasusagna S, Moreno I, Navarro A, Munoz C, Martinez F, Hernandez R, et al. miR-328 mediates a metabolic shift in colon cancer cells by targeting SLC2A1/GLUT1. Clin Transl Oncol (2018) 20:1161–7. doi: 10.1007/s12094-018-1836-1
29. Sun Y, Zhao X, Zhou Y, Hu Y. miR-124, miR-137 and miR-340 regulate colorectal cancer growth via inhibition of the warburg effect. Oncol Rep (2012) 28:1346–52. doi: 10.3892/or.2012.1958
30. Liu S, Xiao Z, Ai F, Liu F, Chen X, Cao K, et al. miR-142-5p promotes development of colorectal cancer through targeting SDHB and facilitating generation of aerobic glycolysis. BioMed Pharmacother. (2017) 92:1119–27. doi: 10.1016/j.biopha.2017.05.134
31. Wang J, Wang H, Liu A, Fang C, Hao J, Wang Z. Lactate dehydrogenase a negatively regulated by miRNAs promotes aerobic glycolysis and is increased in colorectal cancer. Oncotarget. (2015) 6:19456–68. doi: 10.18632/oncotarget.3318
32. Gregersen LH, Jacobsen A, Frankel LB, Wen J, Krogh A, Lund AH. MicroRNA-143 down-regulates hexokinase 2 in colon cancer cells. BMC Cancer. (2012) 12:232. doi: 10.1186/1471-2407-12-232
33. Qiu Z, Guo W, Wang Q, Chen Z, Huang S, Zhao F, et al. MicroRNA-124 reduces the pentose phosphate pathway and proliferation by targeting PRPS1 and RPIA mRNAs in human colorectal cancer cells. Gastroenterology. (2015) 149:1587–98.e11. doi: 10.1053/j.gastro.2015.07.050
34. Zhu W, Huang Y, Pan Q, Xiang P, Xie N, Yu H. MicroRNA-98 suppress warburg effect by targeting HK2 in colon cancer cells. Dig Dis Sci (2017) 62:660–8. doi: 10.1007/s10620-016-4418-5
35. Fu R, Yang P, Amin S, Li Z. A novel miR-206/hnRNPA1/PKM2 axis reshapes the warburg effect to suppress colon cancer growth. Biochem Biophys Res Commun (2020) 531:465–71. doi: 10.1016/j.bbrc.2020.08.019
36. Liu Y, Tang W, Ren L, Liu T, Yang M, Wei Y, et al. Activation of miR-500a-3p/CDK6 axis suppresses aerobic glycolysis and colorectal cancer progression. J Transl Med (2022) 20:106. doi: 10.1186/s12967-022-03308-8
37. He J, Xie G, Tong J, Peng Y, Huang H, Li J, et al. Overexpression of microRNA-122 re-sensitizes 5-FU-resistant colon cancer cells to 5-FU through the inhibition of PKM2 in vitro and in vivo. Cell Biochem Biophys (2014) 70:1343–50. doi: 10.1007/s12013-014-0062-x
38. Li X, Zhao H, Zhou X, Song L. Inhibition of lactate dehydrogenase a by microRNA-34a resensitizes colon cancer cells to 5-fluorouracil. Mol Med Rep (2015) 11:577–82. doi: 10.3892/mmr.2014.2726
39. Babaei-Jadidi R, Kashfi H, Alelwani W, Karimi Bakhtiari A, Kattan SW, Mansouri OA, et al. Anti-miR-135/SPOCK1 axis antagonizes the influence of metabolism on drug response in intestinal/colon tumour organoids. Oncogenesis. (2022) 11:4. doi: 10.1038/s41389-021-00376-1
40. Qin Y, Cheng C, Lu H, Wang Y. miR-4458 suppresses glycolysis and lactate production by directly targeting hexokinase2 in colon cancer cells. Biochem Biophys Res Commun (2016) 469:37–43. doi: 10.1016/j.bbrc.2015.11.066
41. Barisciano G, Colangelo T, Rosato V, Muccillo L, Taddei ML, Ippolito L, et al. miR-27a is a master regulator of metabolic reprogramming and chemoresistance in colorectal cancer. Br J Cancer. (2020) 122:1354–66. doi: 10.1038/s41416-020-0773-2
42. Liu YD, Zhuang XP, Cai DL, Cao C, Gu QS, Liu XN, et al. Let-7a regulates EV secretion and mitochondrial oxidative phosphorylation by targeting SNAP23 in colorectal cancer. J Exp Clin Cancer Res (2021) 40:31. doi: 10.1186/s13046-020-01813-6
43. Jiang M, Xu B, Li X, Shang Y, Chu Y, Wang W, et al. O-GlcNAcylation promotes colorectal cancer metastasis via the miR-101-O-GlcNAc/EZH2 regulatory feedback circuit. Oncogene. (2019) 38:301–16. doi: 10.1038/s41388-018-0435-5
44. Tao L, Xu C, Shen W, Tan J, Li L, Fan M, et al. HIPK3 inhibition by exosomal hsa-miR-101-3p is related to metabolic reprogramming in colorectal cancer. Front Oncol (2021) 11:758336. doi: 10.3389/fonc.2021.758336
45. Yan S, Han B, Gao S, Wang X, Wang Z, Wang F, et al. Exosome-encapsulated microRNAs as circulating biomarkers for colorectal cancer. Oncotarget. (2017) 8:60149–58. doi: 10.18632/oncotarget.18557
46. Meng F, Luo X, Li C, Wang G. LncRNA LINC00525 activates HIF-1alpha through miR-338-3p / UBE2Q1 / beta-catenin axis to regulate the warburg effect in colorectal cancer. Bioengineered. (2022) 13:2554–67. doi: 10.1080/21655979.2021.2018538
47. Lu S, Han L, Hu X, Sun T, Xu D, Li Y, et al. N6-methyladenosine reader IMP2 stabilizes the ZFAS1/OLA1 axis and activates the warburg effect: implication in colorectal cancer. J Hematol Oncol (2021) 14:188. doi: 10.1186/s13045-021-01204-0
48. Zheng Y, Wang Y, Liu Y, Xie L, Ge J, Yu G, et al. N6-methyladenosine modification of PTTG3P contributes to colorectal cancer proliferation via YAP1. Front Oncol (2021) 11:669731. doi: 10.3389/fonc.2021.669731
49. Liu Y, Peng H, Shen Y, Da R, Tian A, Guo X. Downregulation of long noncoding RNA myocardial infarction associated transcript suppresses cell proliferation, migration, invasion, and glycolysis by regulation of miR-488-3p/IGF1R pathway in colorectal cancer. Cancer Biother Radiopharm (2020). doi: 10.1089/cbr.2020.3671
50. Guo X, Zhang Y, Liu L, Yang W, Zhang Q. HNF1A-AS1 regulates cell migration, invasion and glycolysis via modulating miR-124/MYO6 in colorectal cancer cells. Onco Targets Ther (2020) 13:1507–18. doi: 10.2147/OTT.S231249
51. Li YL, Zhang XX, Yao JN, Gao B, Gao SL, Wang CF, et al. ZEB2-AS1 regulates the expression of TAB3 and promotes the development of colon cancer by adsorbing microRNA-188. Eur Rev Med Pharmacol Sci (2020) 24:4180–9. doi: 10.26355/eurrev_202004_20998
52. Sun S, Li W, Ma X, Luan H. Long noncoding RNA LINC00265 promotes glycolysis and lactate production of colorectal cancer through regulating of miR-216b-5p/TRIM44 axis. Digestion. (2020) 101:391–400. doi: 10.1159/000500195
53. Li C, Wang P, Du J, Chen J, Liu W, Ye K. LncRNA RAD51-AS1/miR-29b/c-3p/NDRG2 crosstalk repressed proliferation, invasion and glycolysis of colorectal cancer. IUBMB Life (2021) 73:286–98. doi: 10.1002/iub.2427
54. Wang Y, Lu JH, Wu QN, Jin Y, Wang DS, Chen YX, et al. LncRNA LINRIS stabilizes IGF2BP2 and promotes the aerobic glycolysis in colorectal cancer. Mol Cancer. (2019) 18:174. doi: 10.1186/s12943-019-1105-0
55. Zuo S, Wu L, Wang Y, Yuan X. Long non-coding RNA MEG3 activated by vitamin d suppresses glycolysis in colorectal cancer via promoting c-myc degradation. Front Oncol (2020) 10:274. doi: 10.3389/fonc.2020.00274
56. Guo T, Liu D, Peng S, Wang M, Li Y. A positive feedback loop of lncRNA MIR31HG-miR-361-3p -YY1 accelerates colorectal cancer progression through modulating proliferation, angiogenesis, and glycolysis. Front Oncol (2021) 11:684984. doi: 10.3389/fonc.2021.684984
57. Wang Y, Yu G, Liu Y, Xie L, Ge J, Zhao G, et al. Hypoxia-induced PTTG3P contributes to colorectal cancer glycolysis and M2 phenotype of macrophage. Biosci Rep (2021) 41:BSR20210764. doi: 10.1042/BSR20210764
58. Hong J, Guo F, Lu SY, Shen C, Ma D, Zhang X, et al. F. nucleatum targets lncRNA ENO1-IT1 to promote glycolysis and oncogenesis in colorectal cancer. Gut. (2021) 70:2123–37. doi: 10.1136/gutjnl-2020-322780
59. Shi H, Li K, Feng J, Zhang X. Overexpression of long non-coding RNA urothelial carcinoma associated 1 causes paclitaxel (Taxol) resistance in colorectal cancer cells by promoting glycolysis. J Chemother (2021) 33:409–19. doi: 10.1080/1120009X.2021.1906032
60. Yan T, Shen C, Jiang P, Yu C, Guo F, Tian X, et al. Risk SNP-induced lncRNA-SLCC1 drives colorectal cancer through activating glycolysis signaling. Signal Transduct Target Ther (2021) 6:70. doi: 10.1038/s41392-020-00446-7
61. Chen C, Wei M, Wang C, Sun D, Liu P, Zhong X, et al. Long noncoding RNA KCNQ1OT1 promotes colorectal carcinogenesis by enhancing aerobic glycolysis via hexokinase-2. Aging (Albany NY). (2020) 12:11685–97. doi: 10.18632/aging.103334
62. Li S, Zhu K, Liu L, Gu J, Niu H, Guo J. lncARSR sponges miR-34a-5p to promote colorectal cancer invasion and metastasis via hexokinase-1-mediated glycolysis. Cancer Sci (2020) 111:3938–52. doi: 10.1111/cas.14617
63. Zhang Z, Yang W, Li N, Chen X, Ma F, Yang J, et al. LncRNA MCF2L-AS1 aggravates proliferation, invasion and glycolysis of colorectal cancer cells via the crosstalk with miR-874-3p/FOXM1 signaling axis. Carcinogenesis. (2021) 42:263–71. doi: 10.1093/carcin/bgaa093
64. Cui Z, Wang Q, Deng MH, Han QL. LncRNA HCG11 promotes 5-FU resistance of colon cancer cells through reprogramming glucose metabolism by targeting the miR-144-3p-PDK4 axis. Cancer biomark (2022) 34:41–53. doi: 10.3233/CBM-210212
65. Zhao S, Guan B, Mi Y, Shi D, Wei P, Gu Y, et al. LncRNA MIR17HG promotes colorectal cancer liver metastasis by mediating a glycolysis-associated positive feedback circuit. Oncogene. (2021) 40:4709–24. doi: 10.1038/s41388-021-01859-6
66. Li Q, Sun H, Luo D, Gan L, Mo S, Dai W, et al. Lnc-RP11-536 K7.3/SOX2/HIF-1alpha signaling axis regulates oxaliplatin resistance in patient-derived colorectal cancer organoids. J Exp Clin Cancer Res (2021) 40:348. doi: 10.1186/s13046-021-02143-x
67. Gao SJ, Ren SN, Liu YT, Yan HW, Chen XB. Targeting EGFR sensitizes 5-fu-resistant colon cancer cells through modification of the lncRNA-FGD5-AS1-miR-330-3p-Hexokinase 2 axis. Mol Ther Oncolytics. (2021) 23:14–25. doi: 10.1016/j.omto.2021.06.012
68. Yu Z, Wang Y, Deng J, Liu D, Zhang L, Shao H, et al. Long non-coding RNA COL4A2-AS1 facilitates cell proliferation and glycolysis of colorectal cancer cells via miR-20b-5p/hypoxia inducible factor 1 alpha subunit axis. Bioengineered. (2021) 12:6251–63. doi: 10.1080/21655979.2021.1969833
69. Zheng H, Zhang M, Ke X, Deng X, Li D, Wang Q, et al. LncRNA XIST/miR-137 axis strengthens chemo-resistance and glycolysis of colorectal cancer cells by hindering transformation from PKM2 to PKM1. Cancer biomark (2021) 30:395–406. doi: 10.3233/CBM-201740
70. Liu S, Huang F, Ye Q, Li Y, Chen J, Huang H. SPRY4-IT1 promotes survival of colorectal cancer cells through regulating PDK1-mediated glycolysis. Anim Cells Syst (Seoul). (2020) 24:220–7. doi: 10.1080/19768354.2020.1784274
71. Lan Z, Yao X, Sun K, Li A, Liu S, Wang X. The interaction between lncRNA SNHG6 and hnRNPA1 contributes to the growth of colorectal cancer by enhancing aerobic glycolysis through the regulation of alternative splicing of PKM. Front Oncol (2020) 10:363. doi: 10.3389/fonc.2020.00363
72. Cui S, Yang X, Zhang L, Zhao Y, Yan W. LncRNA MAFG-AS1 promotes the progression of colorectal cancer by sponging miR-147b and activation of NDUFA4. Biochem Biophys Res Commun (2018) 506:251–8. doi: 10.1016/j.bbrc.2018.10.112
73. Bian Z, Zhang J, Li M, Feng Y, Wang X, Zhang J, et al. LncRNA-FEZF1-AS1 promotes tumor proliferation and metastasis in colorectal cancer by regulating PKM2 signaling. Clin Cancer Res (2018) 24:4808–19. doi: 10.1158/1078-0432.CCR-17-2967
74. Wang C, Li Y, Yan S, Wang H, Shao X, Xiao M, et al. Interactome analysis reveals that lncRNA HULC promotes aerobic glycolysis through LDHA and PKM2. Nat Commun (2020) 11:3162. doi: 10.1038/s41467-020-16966-3
75. Shi H, Li K, Feng J, Liu G, Feng Y, Zhang X. LncRNA-DANCR interferes with miR-125b-5p/HK2 axis to desensitize colon cancer cells to cisplatin vis activating anaerobic glycolysis. Front Oncol (2020) 10:1034. doi: 10.3389/fonc.2020.01034
76. Li Z, Yao H, Wang S, Li G, Gu X. CircTADA2A suppresses the progression of colorectal cancer via miR-374a-3p/KLF14 axis. J Exp Clin Cancer Res (2020) 39:160. doi: 10.1186/s13046-020-01642-7
77. Wang X, Tao G, Huang D, Liang S, Zheng D. Circular RNA NOX4 promotes the development of colorectal cancer via the microRNA4855p/CKS1B axis. Oncol Rep (2020) 44:2009–20. doi: 10.3892/or.2020.7758
78. Liu Y, Li H, Ye X, Ji A, Fu X, Wu H, et al. Hsa_circ_0000231 knockdown inhibits the glycolysis and progression of colorectal cancer cells by regulating miR-502-5p/MYO6 axis. World J Surg Oncol (2020) 18:255. doi: 10.1186/s12957-020-02033-0
79. Yi B, Dai K, Yan Z, Yin Z. Circular RNA PLCE1 promotes epithelial mesenchymal transformation, glycolysis in colorectal cancer and M2 polarization of tumor-associated macrophages. Bioengineered. (2022) 13:6243–56. doi: 10.1080/21655979.2021.2003929
80. Jiang Z, Hu H, Hu W, Hou Z, Liu W, Yu Z, et al. Circ-RNF121 regulates tumor progression and glucose metabolism by miR-1224-5p/FOXM1 axis in colorectal cancer. Cancer Cell Int (2021) 21:596. doi: 10.1186/s12935-021-02290-3
81. Wang X, Zhang H, Yang H, Bai M, Ning T, Deng T, et al. Exosome-delivered circRNA promotes glycolysis to induce chemoresistance through the miR-122-PKM2 axis in colorectal cancer. Mol Oncol (2020) 14:539–55. doi: 10.1002/1878-0261.12629
82. Zhang ZJ, Zhang YH, Qin XJ, Wang YX, Fu J. Circular RNA circDENND4C facilitates proliferation, migration and glycolysis of colorectal cancer cells through miR-760/GLUT1 axis. Eur Rev Med Pharmacol Sci (2020) 24:2387–400. doi: 10.26355/eurrev_202003_20506
83. Gao Y, Liu C, Xu X, Wang Y, Jiang Y. Circular RNA sterile alpha motif domain containing 4A contributes to cell 5-fluorouracil resistance in colorectal cancer by regulating the miR-545-3p/6-phosphofructo-2-kinase/fructose-2,6-bisphosphataseisotype 3 axis. Anticancer Drugs (2022) 33:553–63. doi: 10.1097/CAD.0000000000001285
84. Li Q, Wang Y, Wu S, Zhou Z, Ding X, Shi R, et al. CircACC1 regulates assembly and activation of AMPK complex under metabolic stress. Cell Metab (2019) 30:157–73.e7. doi: 10.1016/j.cmet.2019.05.009
Keywords: Circular RNA, colorectal cancer, glucose metabolism, long noncoding RNA, metabolic reprogramming, microRNA
Citation: Yan S, Wang S, Wang X, Dai W, Chu J, Cheng M, Guo Z and Xu D (2022) Emerging role of non-coding RNAs in glucose metabolic reprogramming and chemoresistance in colorectal cancer. Front. Oncol. 12:954329. doi: 10.3389/fonc.2022.954329
Received: 27 May 2022; Accepted: 11 July 2022;
Published: 01 August 2022.
Edited by:
Gaetano Gallo, Sapienza University of Rome, ItalyReviewed by:
Shuohui Dong, Shandong University, ChinaCopyright © 2022 Yan, Wang, Wang, Dai, Chu, Cheng, Guo and Xu. This is an open-access article distributed under the terms of the Creative Commons Attribution License (CC BY). The use, distribution or reproduction in other forums is permitted, provided the original author(s) and the copyright owner(s) are credited and that the original publication in this journal is cited, in accordance with accepted academic practice. No use, distribution or reproduction is permitted which does not comply with these terms.
*Correspondence: Zhiliang Guo, ZHJ6bGd1b0AxNjMuY29t ; Donghua Xu, eHVkaEB3Zm1jLmVkdS5jbg==
†These authors have contributed equally to this work
Disclaimer: All claims expressed in this article are solely those of the authors and do not necessarily represent those of their affiliated organizations, or those of the publisher, the editors and the reviewers. Any product that may be evaluated in this article or claim that may be made by its manufacturer is not guaranteed or endorsed by the publisher.
Research integrity at Frontiers
Learn more about the work of our research integrity team to safeguard the quality of each article we publish.