- 1Mayo Clinic Alix School of Medicine, Scottsdale, AZ, United States
- 2Division of Hematology and Oncology, Mayo Clinic, Phoenix, AZ, United States
The therapeutic landscape for lymphomas is quite diverse and includes active surveillance, chemotherapy, immunotherapy, radiation therapy, and even stem cell transplant. Advances in the field have led to the development of targeted therapies, agents that specifically act against a specific component within the critical molecular pathway involved in tumorigenesis. There are currently numerous targeted therapies that are currently Food and Drug Administration (FDA) approved to treat certain lymphoproliferative disorders. Of many, some of the targeted agents include rituximab, brentuximab vedotin, polatuzumab vedotin, nivolumab, pembrolizumab, mogamulizumab, vemurafenib, crizotinib, ibrutinib, cerdulatinib, idelalisib, copanlisib, venetoclax, tazemetostat, and chimeric antigen receptor (CAR) T-cells. Although these agents have shown strong efficacy in treating lymphoproliferative disorders, the complex biology of the tumors have allowed for the malignant cells to develop various mechanisms of resistance to the targeted therapies. Some of the mechanisms of resistance include downregulation of the target, antigen escape, increased PD-L1 expression and T-cell exhaustion, mutations altering the signaling pathway, and agent binding site mutations. In this manuscript, we discuss and highlight the mechanism of action of the above listed agents as well as the different mechanisms of resistance to these agents as seen in lymphoproliferative disorders.
Introduction
Lymphomas are a group of malignancies characterized by the uncontrolled proliferation of either mature B-lymphocytes or T-lymphocytes. Lymphomas can be further classified as Hodgkin or non-Hodgkin based on the presence or absence of Reed-Sternberg cells, respectively. The treatment for lymphomas includes active surveillance, chemotherapy, immunotherapy, radiation therapy, and even stem cell transplant. In terms of chemotherapy, for Hodgkin lymphoma (HL), the front-line therapy has been ABVD (doxorubicin, bleomycin, vinblastine, dacarbazine), but despite great overall survival rates, around 30-40% of patients relapse within the first two years after treatment (1). On the other hand, for non-Hodgkin lymphoma (NHL), R-CHOP (rituximab, cyclophosphamide, doxorubicin, vincristine, and prednisone) has been the front-line therapy, and despite complete responses, around 40-50% of patients develop refractory/relapsed (R/R) disease (2). It should be noted that it took decades of trial and error of trying to improve upon the backbone of ABVD and CHOP to eventually develop the brentuximab vedotin + AVD (3) and rituximab + CHOP treatment regimens that are now part of the first-line therapies. One such example of the trial-and-error process is that after a pivotal trial found unacceptable levels of pulmonary toxicity with brentuximab vedotin + ABVD, bleomycin was removed from the regimen (4). In addition, after many trials, polatuzumab vedotin was also found to significantly improve the R-CHP treatment regimen in the first-line treatment setting for NHLs (5, 6). To address high R/R rates, technological advances have led to the development of targeted therapies against driver molecular aberrations that have emerged as highly effective treatment options in patients whose malignancies harbor the allotted target (7–9). A targeted therapy can be defined as an agent that targets a critical molecular pathway involved in tumorigenesis (10). Furthermore, advances facilitating rapid genomic profiling have allowed for the formation of hypotheses regarding which patients may benefit more from a targeted therapy based on their genetic subtype (11). However, many cancers have strategically developed means to outsmart the highly precise medicines to confer resistance. Thus, we will discuss the mechanisms of escape to various targeted therapies noted in lymphoproliferative disorders.
Targeted therapies
Rituximab
A chimeric monoclonal antibody, rituximab targets the CD20 antigen expressed on lymphocytes and induces cell lysis upon binding antibody-dependent cellular cytotoxicity (ADCC) and complement-dependent cytotoxicity (CDC). Rituximab is used in a variety of disorders, and when it comes to malignancies, it is mainly used to treat NHLs and chronic lymphocytic leukemia. Currently, the FDA has approved rituximab for use in treating NHLs, chronic lymphocytic leukemia (CLL), rheumatoid arthritis, granulomatosis with polyangiitis, and microscopic polyangiitis. Mechanisms of resistance to rituximab are not completely understood, since the therapy relies on the host immune system to mount an immune response, and thus, host factors can also significantly impact efficacy (12). However, three main mechanisms are postulated. The first is that tumor cells have developed ways to block CDC; analysis of rituximab-resistant cell lines has shown that these cells express high levels of membrane complement regulatory proteins (mCRP) – such as CD46, CD55, and CD59 – and these inhibitory proteins block the activation of the complement cascade (13). This theory has been supported by preclinical studies displaying that neutralizing mCRPs with antibodies lead to increased effectiveness of rituximab (14). Next, though rituximab inhibits B-cell lymphoma 2 (Bcl-2) expression to promote cell apoptosis, a study found that prolonged exposure to rituximab led to the downregulation of pro-apoptotic proteins Bcl-2 antagonist/killer (BAK) and Bcl-2 associated X (BAX), conferring resistance (15). Finally, the most supported mechanism of resistance is downregulation of CD20, the target antigen. Studies have identified C-terminal deletions in the CD20 gene as well as decreased expression of CD20 mRNA in cells found to be CD20 negative after rituximab exposure (16, 17). Since rituximab is utilized throughout many phases of lymphoma treatment – first-line, maintenance, and salvage – studies are being conducted to develop strategies for navigating these mechanisms of resistance.
Brentuximab vedotin
A chimeric antibody-drug conjugate, brentuximab vedotin targets the CD30 antigen expressed on lymphocytes to trigger cell death. It has been FDA-approved to treat classical HL and systemic anaplastic large cell lymphoma. Additionally, brentuximab vedotin is a part of the front-line therapy for HL and T-cell NHL (18, 19). CD30 is expressed on activated lymphocytes thus it is an attractive target for therapies (20). Upon binding, the drug is internalized into the cell and subsequently releases the potent microtubule inhibitor monomethyl auristatin E (MMAE) to inhibit cell differentiation and induce apoptosis (20). An in vitro study analyzing brentuximab vedotin resistant cells found that CD30 expression was not significantly lowered in these cells (21). Instead, the resistant cells upregulated the expression of the multi-drug resistance (MDR1) gene and its subsequent product, P-glycoprotein, to confer resistance (21). Additionally, the cells displayed decreased intracellular accumulation of MMAE and increased efflux of MMAE, allowing the cells to avoid death (21). This mechanism of resistance has been further supported by a phase 1 study evaluating the effects of two broad multi-drug resistance modifiers, cyclosporine A and verapamil, on brentuximab vedotin resistance in patients with brentuximab vedotin-resistant HL (22). This study found that inhibiting MDR1 restored sensitivity to brentuximab vedotin, increased intracellular MMAE levels, and improved overall brentuximab vedotin activity (22).
Polatuzumab vedotin
Polatuzumab vedotin is another antibody-drug conjugate that targets the CD79b antigen expressed on lymphocytes, and it has been FDA-approved for treating R/R diffuse large B-cell lymphoma (DLBCL). When antigens bind to the B-cell receptor (BCR), the ligand-receptor complex gets internalized into the cell so that the antigen can be presented on major histocompatibility complex (MHC) class II molecules on the B-cell surface. This process relies heavily on the proper functioning of CD79, a heterodimer of CD79a and CD79b, and within this, CD79b is the dominant player (23). Since CD79b is expressed on most cells of B-cell lymphomas and leukemias, it serves as a prime target for therapies such as polatuzumab vedotin (23). Upon binding to CD79b, polatuzumab vedotin induces cell death in a similar manner to brentuximab vedotin (23). Though polatuzumab vedotin is currently approved (in combination with bendamustine plus rituximab) for use in the R/R setting for DLBCL (24), the POLARIX study found that among 879 patients, the risk of disease progression, relapse, or death was lower in the group treated with polatuzumab vedotin + R-CHP when compared to the group treated with standard R-CHOP – highlighting that polatuzumab vedotin + R-CHP may soon emerge as a part of first-line therapy for DLBCL (5). POLARIX was a confirmatory phase 3 trial based on the positive toxicity profile seen regarding the use of Polatuzumab in the phase 1-2b study in patients with previously untreated DLBCL (25). Utilizing flow cytometry to analyze CD79b cell-surface expression, one study identified that a minimal threshold of 6.82 geometric mean fluorescence intensity units for CD79b expression must be present for anti-CD79b ADCC to be effective (25). Therefore, the primary mechanism of resistance to polatuzumab vedotin is downregulation of CD79b expression (25). However, the ROMULUS phase 2 clinical trial identified resistance to MMAE as another mechanism in patients with R/R diffuse large B-cell lymphoma (DLBCL) and follicular lymphoma (FL) (26). This trial compared polatuzumab vedotin to pinatuzumab vedotin – another antibody-drug conjugate targeted at CD22 (26). In this trial, six patients who had no response originally switched to the other antibody-drug conjugate, and none of these patients responded to the other drug – highlighting that their malignancies had developed resistance to MMAE, not the respective CD drug targets (26). Strategies to overcome resistance to polatuzumab vedotin are being devised and studied (27), and indeed many combinatorial approaches are under development in clinical trials including PolaR-ICE (rituximab, ifosfamide, carboplatin, and etoposide) (NCT04665765), polatuzumab vedotin + GemOX (gemcitabine and oxaliplatin) (NCT04182204), and polatuzumab vedotin + mosenutuzumab (NCT03671018) (28).
Nivolumab
Nivolumab is a monoclonal antibody that binds to and blocks the programmed death receptor-1 (PD-1). It has been FDA-approved to treat classical Hodgkin lymphoma (cHL), melanoma, non-small cell lung cancer, malignant pleural mesothelioma, renal cell carcinoma, squamous cell carcinoma of the head and neck, urothelial carcinoma, colorectal cancer, hepatocellular carcinoma, esophageal cancer, and gastric cancer. Also known as CD279, PD-1 is a checkpoint protein on T-cells and B-cells that binds to programmed death-ligand 1 and 2 (PD-L1 and PD-L2) on other cells of the body to prevent immune cells from attacking other cells in the body (29). Malignant cells express high levels of PD-L1 to help shield them from an immune system response (29). Additionally, the genes for PD-L1 and PD-L2 are located on chromosome 9p24.1, and amplification of 9p24.1 was found to be associated with increased expression of PD-L1 in HL (30). Therefore, blocking the interaction between PD-1 and PD-L1 enhances the immune system’s anti-tumor response and delays tumor growth (29). Mechanisms of resistance to immune checkpoint inhibition involve inadequate T-cell attraction and activation in addition to impaired T-cell effector functions. In cHL, the Hodgkin Reed-Sternberg (HRS) cells produce vascular endothelial growth factor (VEGF) which induces regulatory T-cell proliferation and increases the expression of inhibitory receptors, including PD-1 (31). This, in turn, leads to T-cell exhaustion (31). Thus, a tumor microenvironment with a higher proportion of regulatory T-cells and inhibitory receptors can alter the efficiency of PD-1 blockade therapy (31). Next, tumor cells can have absent or aberrant HLA expression which compromises antigen presentation and affects immune checkpoint inhibition efficacy (32). In fact, in around 70% of cHL cases, HLA class I surface expression is lost (32). Tumor cells can resist PD-1 blockade therapy by increasing the production of indoleamine 2,3-dioxygenase (IDO), the initial and rate-limiting enzyme involved in the degradation of tryptophan (33). Finally, HRS cells have decreased levels of adenosine deaminase, the enzyme involved degrading the purine adenosine (34). This increases levels of adenosine in cHL cells which activates the alternative degradation pathway involving CD32, CD203a, and CD73 (35). However, increased CD73 expression has been shown to directly reduce the effectiveness of PD-1 blockade therapy (36). Thus, increased adenosine levels in cHL cells confers resistance to immune checkpoint inhibitors such as nivolumab. Strategies to overcome resistance to nivolumab are currently being developed and studied (37), and certainly many combinatorial approaches are under development in clinical trial including nivolumab + AVD (NCT03907488) and brentuximab + nivolumab with or without ipilimumab (NCT01896999) (38).
Pembrolizumab
Like nivolumab, pembrolizumab is another monoclonal antibody that binds to and blocks PD-L1 (39). It has been FDA-approved for cHL, primary mediastinal large B-cell lymphoma, melanoma, non-small cell lung cancer, small cell lung cancer, head and neck squamous cell cancer, urothelial carcinoma, colorectal cancer, gastric cancer, esophageal cancer, cervical cancer, hepatocellular carcinoma, Merkel cell carcinoma, renal cell carcinoma, endometrial carcinoma, cutaneous squamous cell carcinoma, and triple-negative breast cancer. Mechanisms of resistance to pembrolizumab are similar to those outlined for nivolumab. Currently, studies have identified ways to circumvent resistance to immune checkpoint inhibition in solid tumors (40, 41); however, studies are being conducted to develop strategies to overcome resistance in lymphoproliferative disorders (42, 43).
Mogamulizumab
A monoclonal antibody, mogamulizumab targets the C-C chemokine receptor 4 (CCR4) to inhibit this signal transduction pathway. This, subsequently, prevents the chemokine-mediated migration and proliferation of T-cells (44). Since CCR4 is expressed on almost all T-cells in cutaneous or peripheral T-cell lymphomas or leukemias, mogamulizumab has emerged as an attractive therapeutic option (44). It is currently FDA-approved for use in treating R/R mycosis fungoides and Sézary syndrome. A study on 19 patients with either mycosis fungoides or Sézary syndrome found that though all patients had T-cells with CCR4 expression prior to starting treatment, all of them had to discontinue mogamulizumab due to lack or loss of response to therapy (45). After stopping treatment, in 57% of patients, CCR4 expression was no longer detected by immunohistochemistry (45). Targeted DNA-sequencing of these samples found that loss of CCR4 expression occurred both with and without genomic alterations in the CCR4 gene (45). Additionally, the study identified that none of the patients that experienced a loss of CCR4 expression benefitted from a second course of mogamulizumab (45). It should also be noted that this study also identified a subset of patients with high CCR4 expression and an undetermined mechanism of resistance to mogamulizumab (45). Further investigations are currently underway to better understand these mechanisms of resistance and devise strategies to overcome them. Many combinatorial approaches are under development in clinical trials including mogamulizumab plus magrolimab (NCT04541017) and mogamulizumab plus natural killer cells (NCT04848064) (46).
Vemurafenib
A small-molecule kinase inhibitor, vemurafenib inhibits the BRAF serine/threonine protein kinase with the V600E or V600K mutation. Cells with this aberrant molecule have unregulated cell growth through the mitogen activated protein kinase (MAPK) pathway (47, 48). Thus, this targeted therapy has been effectively used for treating melanoma and hairy cell leukemia (HCL). However, it is currently FDA-approved for unresectable or metastatic melanoma with the BRAF V600E mutation as detected by an FDA-approved test. Furthermore, it is also FDA-approved for patients with Erdheim-Chester disease, which is a rare histiocytic disorder (49, 50), that carry the BRAF V600E mutation (51). Initial studies on vemurafenib in HCL found a missense mutation in insulin receptor substrate 1 (IRS1) in addition to the BRAF V600E mutation that induced the MAPK pathway through activation of extracellular signal-regulated kinase (ERK) (52). By allowing BRAF to be bypassed to activate ERK, the IRS1 mutation conferred resistance to treatment (52). Additionally, the study identified a mutation in the Kirsten rat sarcoma (KRAS) gene, a protein involved in the RAS/MAPK pathway, that also mediated resistance to vemurafenib (52). Thus, this study outlined both ERK dependent and independent mechanisms of resistance to vemurafenib in HCL (52). Another study also found IRS1 and KRAS mutations in vemurafenib-resistant HCL cell lines, however, this study also found loss-of-function mutations in the neurofibromatosis 1 and 2 genes (NF1 and NF2) which contributed to the lack of response (53). To address these mechanisms of resistance, a study was conducted on a 74-year-old patient with vemurafenib-resistant HCL with many resistance-conferring mutations (including KRAS) present in the cell lines (54). MEK is a protein kinase upstream of ERK in the MAPK pathway, and with the addition of the MEK inhibitor cobimetinib to vemurafenib, the patient responded to such combination therapy (54). The bone marrow showed suppression of ERK activity (54). At 12 month follow up, the patient showed continued response and remained asymptomatic – highlighting MEK inhibition as a potential option for navigating resistance to vemurafenib (54).
Crizotinib
A tyrosine kinase receptor inhibitor, crizotinib specifically targets anaplastic lymphoma kinase (ALK), hepatocyte growth factor receptor (HGFR, c-MET), and Recepteur d’Origine Nantais (RON). It is currently FDA-approved for treating metastatic non-small cell lung cancer with ALK or ROS-1 positivity per an FDA-approved test, ALK-positive anaplastic large cell lymphoma, and ALK-positive inflammatory myofibroblastic tumors. In non-small cell lung cancer, studies found a chromosomal rearrangement creating a gene fusion product that resulted in a constitutively active ALK protein as the oncologic driver (55). Other studies on anaplastic large cell lymphoma, the most common T-cell NHL in children, found that tumor progression was primarily driven by a fusion product between ALK and mainly nucleophosmin 1 (NPM1) called NPM-ALK (56). For these reasons, crizotinib has emerged as an effective therapy for these malignancies. In cases where crizotinib resistance developed, studies identified ALK mutations conferring resistance; however, studies are being conducted to see if this can be overcome by using newer-generation ALK inhibitors such as alectinib, ceritinib, brigatinib, and lorlatinib (57). In other cases where crizotinib resistance developed and ALK mutations were not identified, a study used genome-wide clustered regularly interspaced short palindromic repeats (CRISPR) analysis to look for overexpressed genes that could be conferring resistance (58). This study found that in around 30% of crizotinib-resistant cell lines, the IL10RA gene for the IL-10 signaling pathway was overexpressed in cells both with and without ALK mutations (58). Through further investigation, this study identified how the IL-10 pathway ultimately activated signal transducer and activator of transcription 3 (STAT3), a molecule that promotes cell survival (58). Furthermore, STAT3 was found to bind to the promoter for IL10RA and upregulate its expression – ultimately creating a feedback loop that bypasses NPM-ALK and promotes cell survival through increased STAT3 activity (58). However, the authors of this study did note that further investigation of this mechanism of resistance is needed (58). Strategies to overcome resistance have been identified in non-small cell lung cancer, but studies are needed to develop these strategies in lymphoproliferative disorders (59).
Ibrutinib
Constitutive B-cell receptor signaling pathway activation has been implicated in numerous B-cell malignancies. One enzyme in this pathway is Bruton tyrosine kinase (BTK), and this enzyme plays a crucial role in modulating cytokine and integrin expression for B-cell trafficking and proliferation (60). Thus, ibrutinib was developed to specifically inhibit BTK (although other enzymes are indirectly affected too) and provide a therapeutic effect in malignancies such as CLL, mantle cell lymphoma (MCL), DLBCL, and Waldenström’s macroglobulinemia (WM) (60). It is currently FDA-approved for use in treating MCL, CLL, small lymphocytic lymphoma (SLL), WM, MZL, and chronic graft versus host disease. For understanding mechanisms of resistance, early studies utilized whole-exome sequencing to compare baseline and relapse genomes of patients with CLL who had been treated with ibrutinib (61). One study concluded that resistance developed due to the BTKC481S mutation in the binding site on BTK for ibrutinib (61). This study also identified a mutation in the 1-phosphatidylinositol-4,5-bisphosphate phosphodiesterase gamma-2 enzyme (PLCG2) – an enzyme that is further downstream of BTK in the B-cell signaling pathway; however its implication in resistance development was not entirely clear (61). Further investigations identified that this mutation did in fact contribute to ibrutinib-resistance in both CLL and WM (62, 63). It should be noted that within each malignancy, other genetic mutations have been identified conferring resistance to ibrutinib; however, the BTK and PLCG2 mutations are the most common in patients with CLL. BTK and PLCG2 mutations conferring resistance to ibrutinib have also been documented in MZL (64). In MCL specifically, studies have identified sustained distal B-cell receptor signaling pathway activation through the classical and alternative NFkB pathways as a mechanism underlying primary resistance to ibrutinib (65). In WM, BTK and PLCG2 mutations have been identified as mechanisms of resistance (66), however, responses to ibrutinib are also highly dependent on whether patients have the CXCR4WHIM mutation that confers resistance to ibrutinib (67, 68). Table 1 summarizes the mutated genes that lead to resistance to ibrutinib resistance in lymphoproliferative disorders. In terms of molecular changes that contribute to resistance, studies have found that resistant DLBCL lines overexpress CD79B while resistant MCL lines overexpress MYC (70, 71). Increased expression of XPO1 and loss of TRAIL-induced apoptosis has been identified as a mechanism of resistance to ibrutinib in CLL (84, 85), while deletions on chromosomes 6q and 8p have been identified in WM (86, 87). Hence, not every lymphoproliferative disorder will display the same mechanism of resistance when exposed to BTK inhibitors. Resistance to ibrutinib has been overcome through the development of second-generation BTK inhibitors that target BTK with much more specificity compared to ibrutinib. Table 1 also summarizes therapeutical strategies to circumvent resistance.
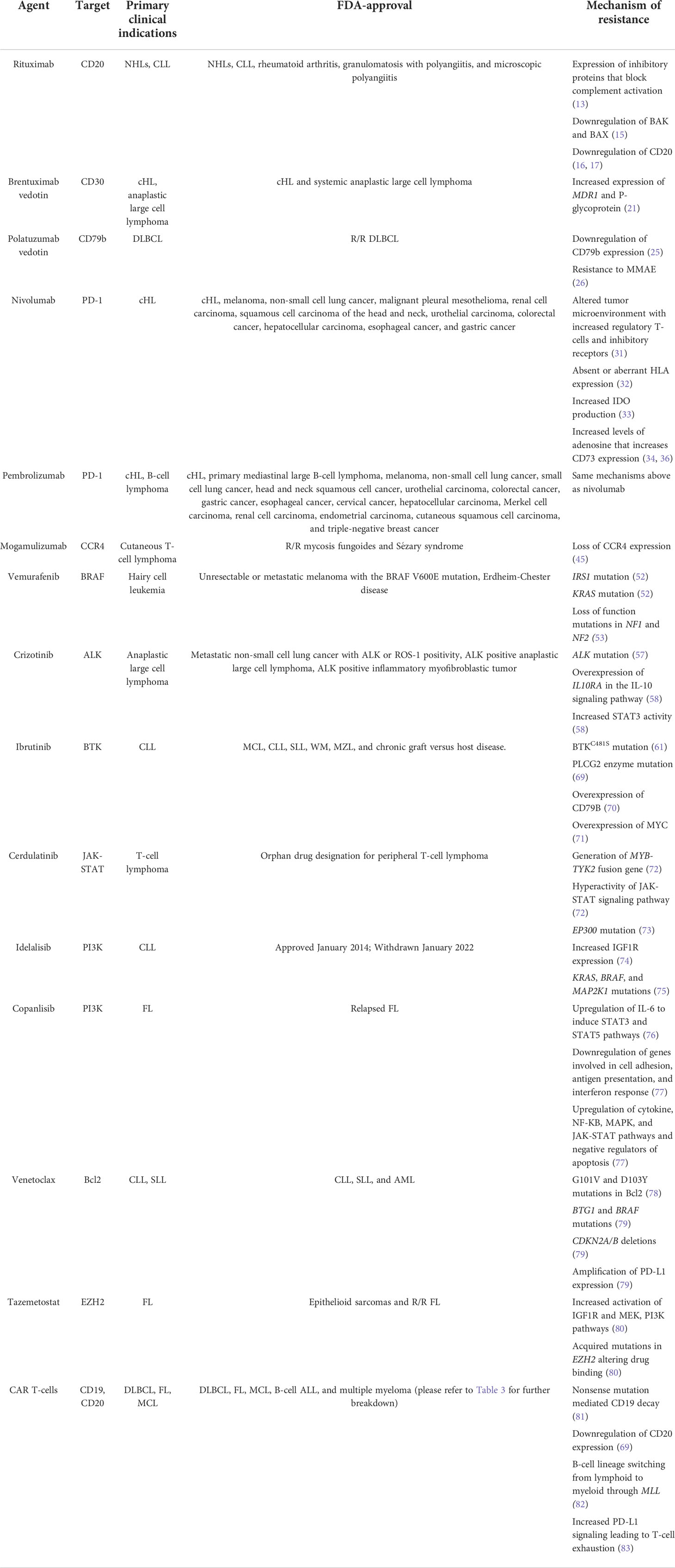
Table 1 Summary table of the mechanisms of resistance to targeted therapies in lymphoproliferative disorders.
Cerdulatinib
In the pathogenesis of B-cell malignancies, the Janus kinase and Signal Transducer and Activator of Transcription (JAK-STAT) pathway produces an active STAT3 molecule that promotes cell survival even in a hostile tumor microenvironment (88). Studies showed that inactivating either JAK or STAT3 decreased cell proliferation and increased apoptosis, and this provided the rationale for developing cerdulatinib – a small-molecule ATP-competitive inhibitor of SYK, JAK1, JAK2, JAK3, and TYK2 (88). This therapy has FDA orphan drug designation for treating peripheral T-cell lymphoma. It should be noted that studies have highlighted that cerdulatinib can overcome ibrutinib-resistance in R/R CLL (89). Preliminary data has also shown the drug’s efficacy in treating small lymphoplasmacytic lymphoma (SLL), FL, DLBCL, ALL, and peripheral T-cell lymphoma (PTCL). In vitro studies have modeled several mechanisms of resistance to cerdulatinib in ALL (72). The first was that long-term exposure to the drug facilitated the generation of the MYB-TYK2 fusion gene that conferred resistance (72). Next, resistant cells with the MYB-TYK2 fusion protein displayed hyperactivation of the JAK/STAT signaling pathway, leading to no response to the drug (72). However, withdrawing the drug for a brief period did re-sensitize the cells to treatment (72). In a phase 1 trial, eight patients with R/R CLL were given cerdulatinib, and two patients were found to have disease progression with treatment (73). These patients were found to have mutations in BTK, TP53, and EP300. Furthermore, it was proposed that the mutation in EP300, a gene encoding a histone acetyltransferase, was the mostly likely mechanism of resistance of cerdulatinib (73). Strategies to overcome resistance to cerdulatinib are highly awaited. For example, there was a phase 2 trial combining cerdulatinib with or without rituximab in patients with lymphoma (73).
Idelalisib
In many cancers, the phosphoinositide 3-kinase (PI3K) signal transduction pathway is highly active, which is why developing agents targeting PI3K was previously attractive (90). However, a challenge that arises is that four distinct PI3K isoforms exist with partially overlapping functions and differing toxic effects (90). One such agent is idelalisib, a selective inhibitor of the delta isoform of PI3K, which has shown strong efficacy in treating B-cell malignancies with an acceptable side-effect profile (90). This drug was previously FDA-approved for treating CLL, FL, and SLL (90). However, there was a voluntary withdrawal of the indication for SLL and FL in 2022 (91). Table 2 illustrates select PI3K inhibitors, their clinical indications, and FDA-approval status. In vitro studies evaluating idelalisib resistance in CLL found that it is associated with increased expression of the insulin-like growth factor 1 receptor (IGF1R) (74). Furthermore, this study also found that cells became re-sensitized to treatment when an IGF1R inhibitor was utilized (74). Another study found that CLL cells became resistant to idelalisib with increased and constitutive MAPK pathway activation, and this allowed for communication between the PI3K and MAPK pathways that circumvented PI3K inhibition (75). This study also identified that increased MAPK pathway activation was associated with the acquisition of mutations in KRAS, BRAF, and MAP2K1 (75).
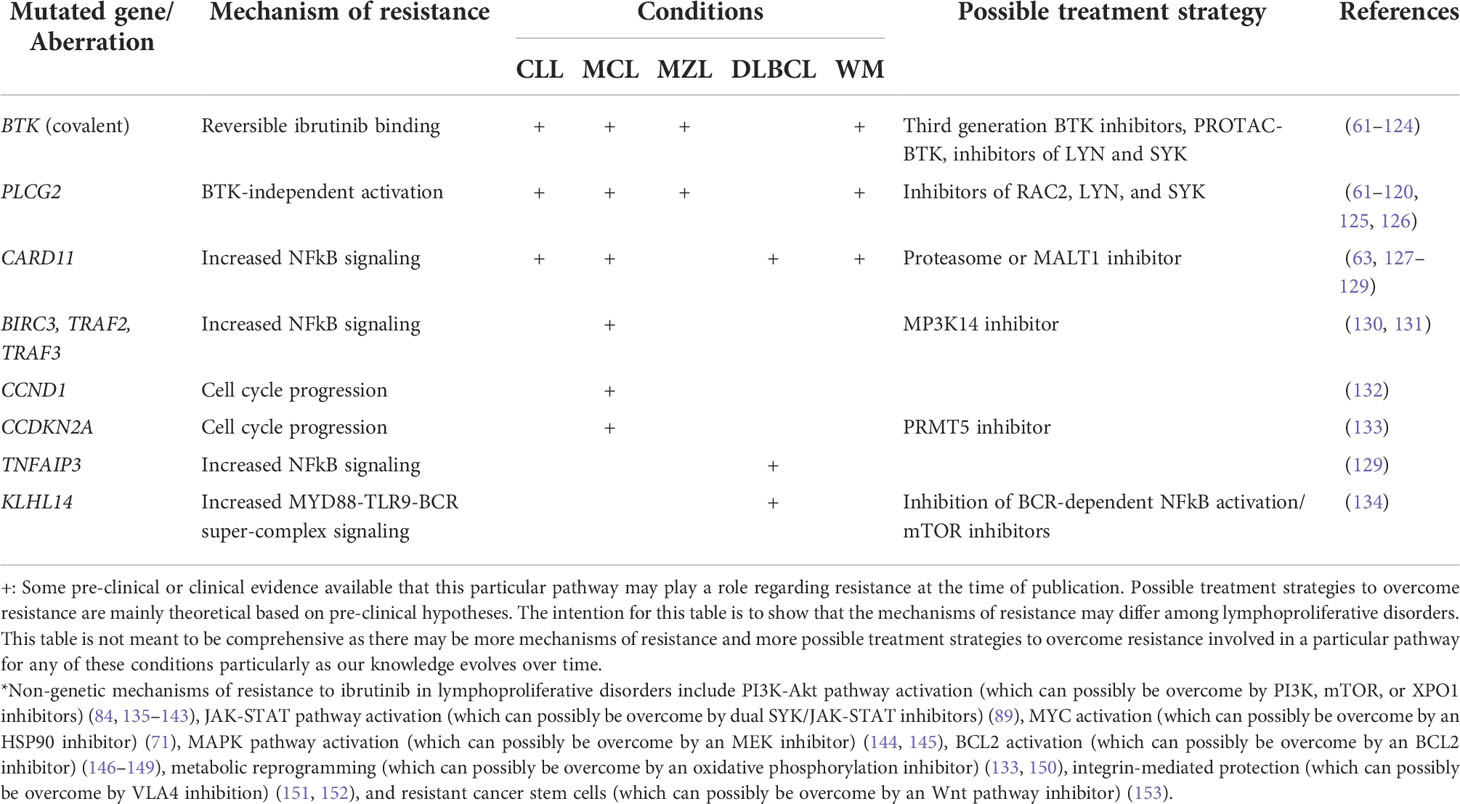
Table 2 Summary of mechanisms of resistance to ibrutinib in lymphoproliferative disorders and strategies to overcome resistance.
Copanlisib
Similar to idelalisib, copanlisib is a highly selective and potent intravenous PI3K inhibitor, yet it is unique because it can target multiple isoforms of PI3K, making it a pan-PI3K inhibitor (92, 93). For example, its unique affinity to the alpha isoform of PI3K (which is present in the pancreas) explains some of its toxicities including hyperglycemia (94). Furthermore, the intravenous route of administration as well as intermittent dosing schedule of the drug have been suggested to portray a more favorable tolerability profile compared to oral PI3K inhibitors (95). Nevertheless, the intravenous administration can also be cumbersome for patients that live far from a cancer center. It has been FDA-approved for relapsed FL. For mechanisms of resistance, a study on B-cell lymphoma resistant cells identified upregulation of IL-6, and IL-6 was able to independently activate STAT3 or STAT5 pathways to confer resistance to PI3K inhibition (76), thus the STAT pathway may be a relevant mechanism of resistance for some lymphoproliferative disorders (96). In resistant MZL cells, gene expression profiling showed upregulation of cytokine, NF-KB, MAPK, and JAK-STAT signaling pathways as well as the negative regulators of apoptosis (77), CD44 and JUN, as a mechanism underlying resistance (77). Furthermore, the cells showed decreased expression of genes involved in cell adhesion (ITGA4, ITGB1), antigen presentation, and interferon response (PARP12, GBP6) (77). This study also used flow cytometry to identify increased CXCR4 surface expression on resistant cells, and subsequently, the addition of a CXCR4 inhibitor overcame resistance to copanlisib (77).
Venetoclax
Venetoclax is an inhibitor of B-cell lymphoma 2 (Bcl2), a pro-survival molecule that regulates the intrinsic apoptosis pathway. This drug is currently FDA-approved to treat CLL, SLL, and acute myeloid leukemia (AML). By binding to Bcl2, venetoclax enables the Bim and BH3 proteins to activate the pro-apoptotic molecules, Bax and Bak. Activation of these molecules commits the cell to apoptosis through the intrinsic mitochondrial pathway and prohibits further cell proliferation. However, malignant cells have developed many mechanisms of resistance to the drug. Some studies have identified mutations in the BH3 binding groove of Bcl2 that led to a protein conformation change hindering the ability of venetoclax to bind to Bcl2 and ultimately conferring resistance (97). Additionally, G101V and D103Y mutations in Bcl2 were identified which also interfere with the drug binding to Bcl2 (78). Other studies looking at patients with R/R CLL identified many genetics aberrations in cancer-related genes that conferred resistance to treatment. These included: mutations of BTG1 and BRAF, deletions in CDKN2A/B, and amplification of PD-L1 expression – suggesting multiple mechanisms of resistance (79). Combination treatment strategies have been developed to improve the clinical efficacy and studies have shown improved response rates with venetoclax in combination with various agents including cytarabine, ibrutinib, rituximab, or bendamustine (98). Additional studies are currently being conducted to develop optimal combination regimens (98).
Tazemetostat
Enhancer of zeste homolog 2 (EZH2) is a part of the polycomb group gene (PcG) family, and this is a group of epigenetic regulators that represses transcription (99). Aberrant EZH2 expression and signaling has been implicated in the pathogenesis of various cancers, which led to the development of the EZH2 inhibitor, tazemetostat (99). This agent is FDA-approved to treat epithelioid sarcomas and R/R FL. Although the agent is FDA approved for FL, we are still trying to elucidate the mechanisms of resistance to tazemetostat in FL (80, 100). For example, it has been described that resistance to EZH2 inhibitors in DLBCL occurs due to the activation of survival pathways and acquired EZH2 mutations that prevent drug binding (80). Resistant DLBCL cells have been found to display increased activation of IGF1R as well as the MEK and PI3K pathways, conferring resistance to EZH2 inhibition (80). Additionally, this study identified acquired mutations in the gene for EZH2 that included EZH2Y641F, EZH2C663Y, EZH2E720G, and EZH2Y726F (80). These mutations prevented drug binding to the EZH2 mutants which decreased the effectiveness of treatment (80). Strategies to overcome resistance to tazemetostat are highly awaited in lymphoproliferative disorders.
CAR T-cells
CAR T-cell therapy has emerged as the breakthrough treatment for numerous hematological malignancies. The basic principle behind this autologous therapy is genetically engineering and modifying a person’s T-cells to display a tumor antigen-binding receptor that directs the T-cells to mount a response against tumor cells (101). A CAR construct is a genetically engineered antigen receptor that binds to a target antigen (101). The CAR construct, of the 3 FDA approved constructs currently in the market for lymphoma, is made to target cluster of differentiation (CD) molecules that are expressed on malignant cells (101). For example, in numerous B-cell malignancies, CD19 is a primary target since this is highly expressed throughout all stages of B-cell development and differentiation (101). CAR T-cell therapy has shown great efficacy in treating DLBCL, FL, MCL, B-cell ALL, and multiple myeloma. In fact, studies have corroborated CAR T-cell therapy efficacy and toxicity with standard of care products in real-world investigations (102). Additionally, CAR T-cells are FDA-approved in multiple lymphoproliferative disorders including DLBCL (103–105), MCL (106–108), and FL (109). Table 3 summarizes the currently available CAR T-cell therapies and their FDA-approved clinical indications as of July 31, 2022. Seeing as how effective CAR T-cell therapy has been in the R/R setting for lymphoproliferative disorders, studies are currently being conducted to investigate incorporating CAR T-cell therapy in earlier lines of therapy (110, 111). The main toxicities associated with CAR T-cell therapy are cytokine release syndrome (CRS) and immune effector cell-associated neurotoxicity syndrome (ICANS). Studies have shown that certain therapies utilized prior to CAR T-cell therapy – including bridging and prophylaxis – may influence toxicity profiles and outcomes, hence we need to choose prior therapies carefully (112–116). To minimize the occurrences of CRS and ICANS, studies are investigating combinatorial approaches with the hope that these approaches could potentially be used to decrease toxicity and increase efficacy (117). As a cautionary note, not all combinations will serve as effective therapies; for example efficacy outcomes and peak CAR T-cell levels seem to be similar between patients treated with axicabtagene ciloleucel plus atezolizumab (an immune checkpoint inhibitor) as part of the ZUMA-6 trial compared to historical outcomes as part of the ZUMA-1 trial for axicabtagene ciloleucel alone (118). These malignancies have developed resistance to therapy through alteration of the CD19 marker itself through mechanisms such as frameshift mutations leading to nonsense mutation mediated CD19 decay (81). Other studies found that resistance to anti-CD20 CAR T-cell therapy arose from the tumor cells downregulating the expression of CD20 (69). In B-cell ALL, studies found that the tumor cells switch from B-cell lineage to myeloid lineage after CAR T-cell therapy through a mixed-lineage leukemia (MLL) gene rearrangement on chromosome 11q23 (82). Finally, in almost all B-cell malignancies, studies have identified T-cell exhaustion as a contributing factor to the poor persistence of CAR T-cells after infusion. Furthermore, studies have found that enhanced PD-L1 pathway signaling directly contributes to T-cell exhaustion (83). In fact, increased PD-L1 signaling downregulates CD28 co-domain signaling – a signal that is essential for the proper activation of CAR T-cells after the CAR molecule binds to the antigen on tumor cells (83). Thus, PD-L1 interferes with the proliferation and cytotoxicity of T-cells, conferring resistance to therapy (83). Strategies to overcome resistance are being developed and studied – including the addition of small molecules and monoclonal antibodies (102).
Conclusion
Targeted therapies in lymphoproliferative disorders have made great breakthroughs in treating aggressive malignancies. However, tumor cells continually develop new strategies for survival, and thus mechanisms of resistance to even the most specific agents. We have discussed the currently understood mechanisms of resistance to the most utilized targeted agents in lymphoproliferative diseases, and this has been summarized in Table 4. We also have discussed the general common themes regarding mechanisms of resistance to targeted agents, and we illustrated this in Figure 1. We eagerly await further studies that identify methods to re-sensitize tumor cells to treatment to increase response rates.
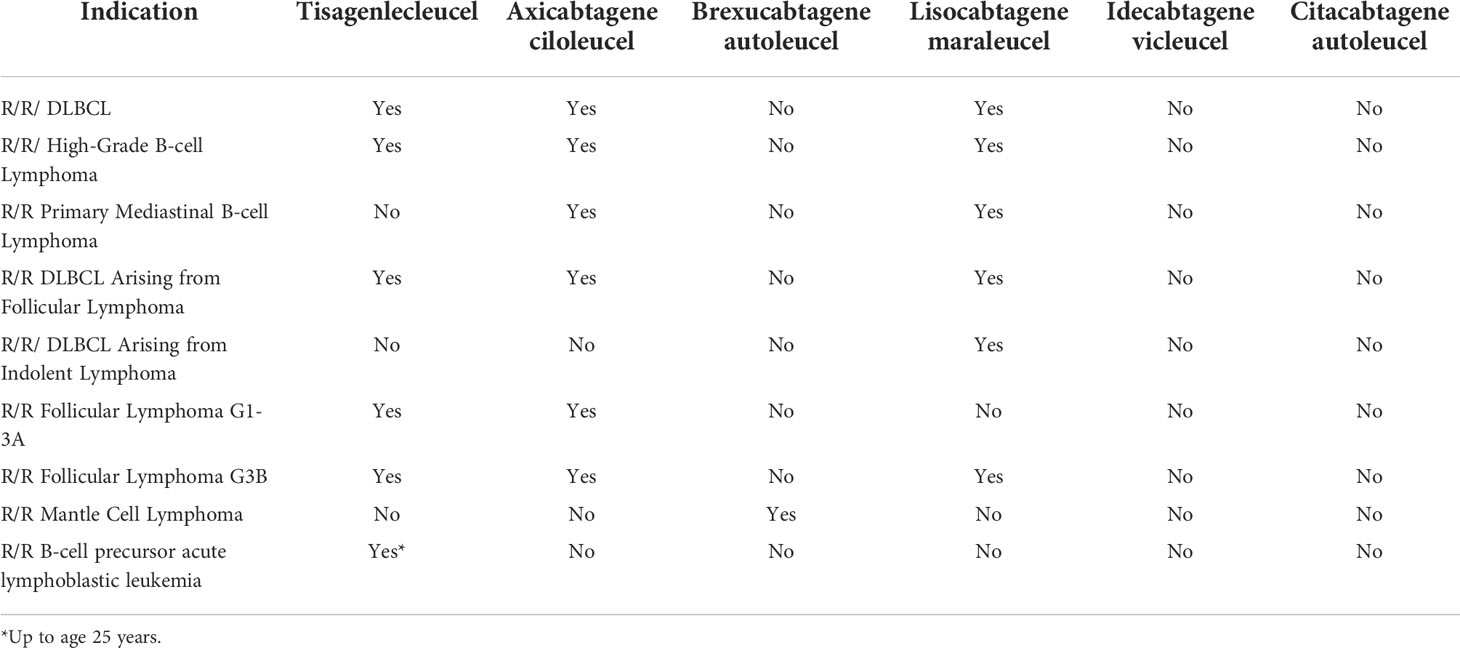
Table 4 Summary table of the currently available CAR T-cell therapies and their FDA-approved clinical indications as of July 31, 2022.
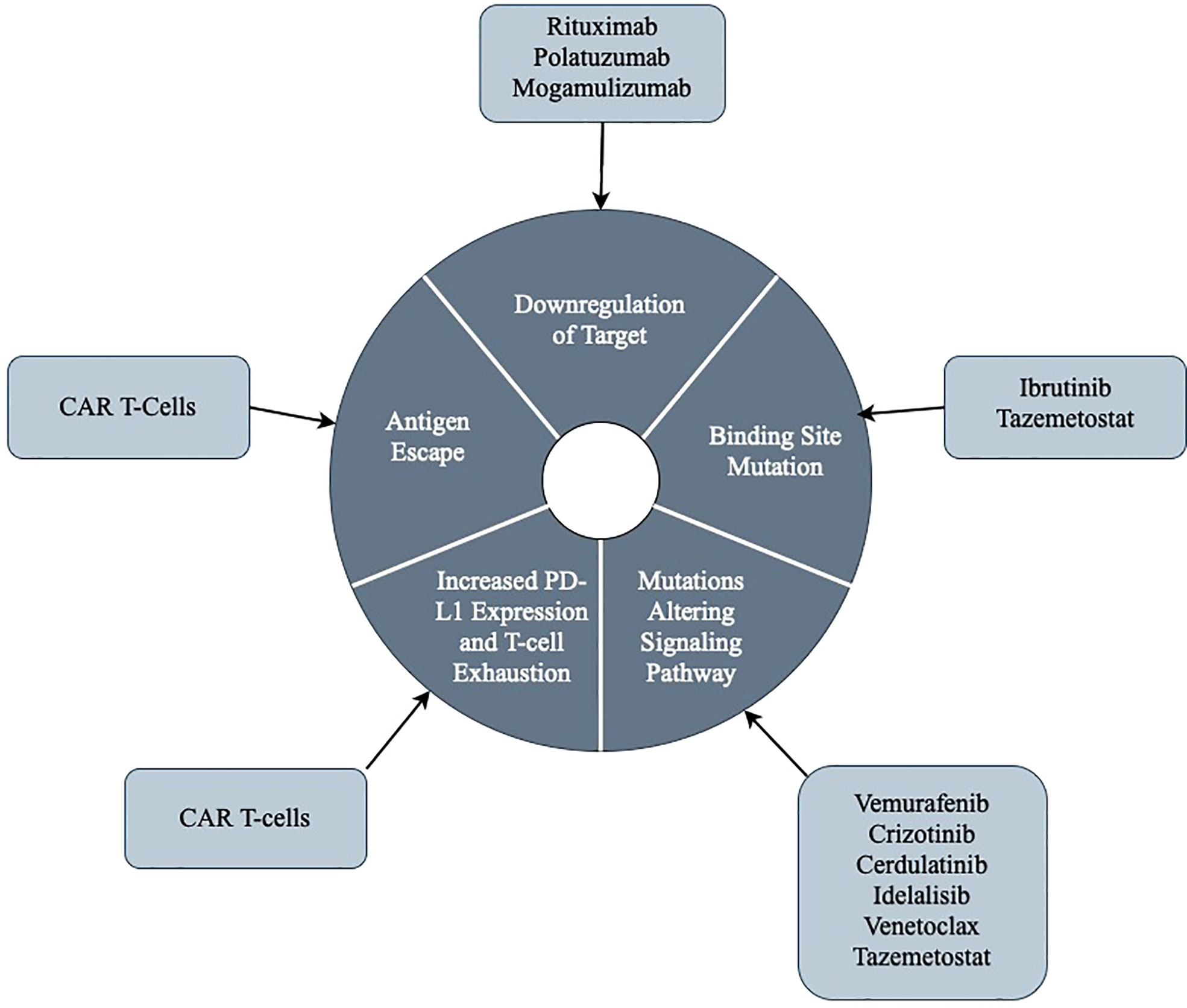
Figure 1 An illustrative summary of the general themes regarding mechanisms of resistance to targeted therapies.
Author contributions
AD and JM contributed to the writing of the manuscript. All authors contributed to the article and approved the submitted version.
Conflict of interest
JM: consulting – Pharmacyclics/Abbvie, Bayer, Gilead/Kite Pharma, Pfizer, Janssen, Juno/Celgene, BMS, Kyowa, Alexion, Fosunkite, Innovent, Seattle Genetics, Debiopharm, Karyopharm, Genmab, ADC Therapeutics, Epizyme, Beigene, Servier, Novartis, Morphosys/Incyte, Mei pharma, Zodiac; research funding – Bayer, Gilead/Kite Pharma, Celgene, Merck, Portola, Incyte, Genentech, Pharmacyclics, Seattle Genetics, Janssen, Millennium. Honoraria from Targeted Oncology, OncView, Curio, Kyowa, Physicians’ Education Resource, Dava, Global clinical insights, MJH, Shanghai Youyao, and Seattle Genetics; speaker’s bureau – Gilead/Kite Pharma, Kyowa, Bayer, Pharmacyclics/Janssen, Seattle Genetics, Acrotech/Aurobindo, Beigene, Verastem, AstraZeneca, Celgene/BMS, Genentech/Roche.
The remaining author declares that the research was conducted in the absence of any commercial or financial relationships that could be construed as a potential conflict of interest.
Publisher’s note
All claims expressed in this article are solely those of the authors and do not necessarily represent those of their affiliated organizations, or those of the publisher, the editors and the reviewers. Any product that may be evaluated in this article, or claim that may be made by its manufacturer, is not guaranteed or endorsed by the publisher.
References
1. Lapuz C, Enjeti AK, O'Brien PC, Capp AL, Holliday EG, Gupta SA. Outcomes and relapse patterns following chemotherapy in advanced Hodgkin lymphoma in the positron emission tomography era. Blood Lymphat Cancer (2018) 8:13–20. doi: 10.2147/BLCTT.S160404
2. Crump M, Sattva S, Neelapu SS, Farooq U, Van Den Neste E, Kuruvilla J, et al. Outcomes in refractory diffuse large b-cell lymphoma: results from the international SCHOLAR-1 study. Blood (2017) 130(16):1800–8. doi: 10.1182/blood-2017-03-769620
3. Ansell SM, Radford J, Connors JM, Długosz-Danecka M, Kim W-S, Gallamini A, et al. Overall survival with brentuximab vedotin in stage III or IV hodgkin’s lymphoma. New Engl J Med (2022) 387(4):310–20. doi: 10.1056/NEJMoa2206125
4. Younes A, Connors JM, Park SI, Fanale M, O'Meara MM, Hunder NN, et al. Brentuximab vedotin combined with ABVD or AVD for patients with newly diagnosed hodgkin's lymphoma: a phase 1, open-label, dose-escalation study. Lancet Oncol (2013) 14(13):1348–56. doi: 10.1016/S1470-2045(13)70501-1
5. Tilly H, Morschhauser F, Sehn LH, Friedberg JW, Trněný M, Sharman JP, et al. Polatuzumab vedotin in previously untreated diffuse Large b-cell lymphoma. N Engl J Med (2021) 386(4):351–63. doi: 10.1056/nejmoa2115304
6. Tilly H, Morschhauser F, Bartlett NL, Mehta A, Salles G, Haioun C, et al. Polatuzumab vedotin in combination with immunochemotherapy in patients with previously untreated diffuse large b-cell lymphoma: an open-label, non-randomised, phase 1b-2 study. Lancet Oncol (2019) 20(7):998–1010. doi: 10.1016/S1470-2045(19)30091-9
7. Munoz J, Swanton C, Kurzrock R. Molecular profiling and the reclassification of cancer: Divide and conquer. Am Soc Clin Oncol Educ Book (2013) 33):127–34. doi: 10.14694/EdBook_AM.2013.33.127
8. Munoz J, Schlette E, Kurzrock R. Rapid response to vemurafenib in a heavily pretreated patient with hairy cell leukemia and a BRAF mutation. J Clin Oncol (2013) 31(20):e351–2. doi: 10.1200/JCO.2012.45.7739
9. Munoz J, Kurzrock R. Targeted therapy in rare cancers–adopting the orphans. Nat Rev Clin Oncol (2012) 9(11):631–42. doi: 10.1038/nrclinonc.2012.160
10. Green MR. Targeting targeted therapy. N Engl J Med (2004) 350(21):2191–3. doi: 10.1056/NEJMe048101
11. Schmitz R, Wright GW, Huang DW, Johnson CA, Phelan JD, Wang JQ, et al. Genetics and pathogenesis of diffuse Large b-cell lymphoma. N Engl J Med (2018) 378(15):1396–407. doi: 10.1056/NEJMoa1801445
12. Rezvani AR, Maloney DG. Rituximab resistance. Best Pract Res Clin Haematol (2011) 24(2):203–16. doi: 10.1016/j.beha.2011.02.009
13. Takei K, Yamazaki T, Sawada U, Ishizuka H, Aizawa S. Analysis of changes in CD20, CD55, and CD59 expression on established rituximab-resistant b-lymphoma cell lines. Leuk Res (2006) 30(5):625–31. doi: 10.1016/j.leukres.2005.09.008
14. Golay J, Lazzari M, Facchinetti V, Bernasconi S, Borleri G, Barbui T, et al. CD20 levels determine the in vitro susceptibility to rituximab and complement of b-cell chronic lymphocytic leukemia: further regulation by CD55 and CD59. Blood (2001) 98(12):3383–9. doi: 10.1182/blood.V98.12.3383
15. Olejniczak SH, Hernandez-Ilizaliturri FJ, Clements JL, Czuczman MS. Acquired resistance to rituximab is associated with chemotherapy resistance resulting from decreased bax and bak expression. Clin Cancer Res (2008) 14(5):1550–60. doi: 10.1158/1078-0432.CCR-07-1255
16. Terui Y, Mishima Y, Sugimura N, Kojima K, Sakurai T, Mishima Y, et al. Identification of CD20 c-terminal deletion mutations associated with loss of CD20 expression in non-hodgkin's lymphoma. Clin Cancer Res (2009) 15(7):2523–30. doi: 10.1158/1078-0432.CCR-08-1403
17. Hiraga J, Tomita A, Sugimoto T, Shimada K, Ito M, Nakamura S, et al. Down-regulation of CD20 expression in b-cell lymphoma cells after treatment with rituximab-containing combination chemotherapies: its prevalence and clinical significance. Blood (2009) 113(20):4885–93. doi: 10.1182/blood-2008-08-175208
18. Straus DJ, Dlugosz-Danecka M, Alekseev S, Illes A, Picardi M, Lech-Maranda E, et al. Brentuximab vedotin with chemotherapy for stage III/IV classical Hodgkin lymphoma: 3-year update of the ECHELON-1 study. Blood (2020) 135(10):735–42. doi: 10.1182/blood.2019003127
19. Horwitz S, O'Connor OA, Pro B, Illidge T, Fanale M, Advani R, et al. Brentuximab vedotin with chemotherapy for CD30-positive peripheral T-cell lymphoma (ECHELON-2): a global, double-blind, randomised, phase 3 trial. Lancet. (2019) 393(10168):229–40. doi: 10.1016/S0140-6736(18)32984-2
20. Donato EM, Fernandez-Zarzoso M, Hueso JA, de la Rubia J. Brentuximab vedotin in Hodgkin lymphoma and anaplastic large-cell lymphoma: an evidence-based review. Onco Targets Ther (2018) 11:4583–90. doi: 10.2147/OTT.S141053
21. Chen R, Hou J, Newman E, Kim Y, Donohue C, Liu X, et al. CD30 downregulation, MMAE resistance, and MDR1 upregulation are all associated with resistance to brentuximab vedotin. Mol Cancer Ther (2015) 14(6):1376–84. doi: 10.1158/1535-7163.MCT-15-0036
22. Chen R, Herrera AF, Hou J, Chen L, Wu J, Guo Y, et al. Inhibition of MDR1 overcomes resistance to brentuximab vedotin in Hodgkin lymphoma. Clin Cancer Res (2020) 26(5):1034–44. doi: 10.1158/1078-0432.CCR-19-1768
23. Sawalha Y, Maddocks K. Profile of polatuzumab vedotin in the treatment of patients with Relapsed/Refractory non-Hodgkin lymphoma: A brief report on the emerging clinical data. Onco Targets Ther (2020) 13:5123–33. doi: 10.2147/OTT.S219449
24. Sehn LH, Herrera AF, Flowers CR, Kamdar MK, McMillan A, Hertzberg M, et al. Polatuzumab vedotin in relapsed or refractory diffuse Large b-cell lymphoma. J Clin Oncol (2020) 38(2):155–65. doi: 10.1200/JCO.19.00172
25. Dornan D, Bennett F, Chen Y, Dennis M, Eaton D, Elkins K, et al. Therapeutic potential of an anti-CD79b antibody-drug conjugate, anti-CD79b-vc-MMAE, for the treatment of non-Hodgkin lymphoma. Blood (2009) 114(13):2721–9. doi: 10.1182/blood-2009-02-205500
26. Morschhauser F, Flinn IW, Advani R, Sehn LH, Diefenbach C, Kolibaba K, et al. Polatuzumab vedotin or pinatuzumab vedotin plus rituximab in patients with relapsed or refractory non-Hodgkin lymphoma: final results from a phase 2 randomised study (ROMULUS). Lancet Haematol (2019) 6(5):e254–e65. doi: 10.1016/S2352-3026(19)30026-2
27. Garcia-Alonso S, Ocana A, Pandiella A. Resistance to antibody-drug conjugates. Cancer Res (2018) 78(9):2159–65. doi: 10.1158/0008-5472.CAN-17-3671
28. Clinical trials using polatuzumab vedotin. In: NIH National cancer institute. Available at: https://www.cancer.gov/about-cancer/treatment/clinical-trials/intervention/polatuzumab-vedotin.
29. Guo L, Zhang H, Chen B. Nivolumab as programmed death-1 (PD-1) inhibitor for targeted immunotherapy in tumor. J Cancer (2017) 8(3):410–6. doi: 10.7150/jca.17144
30. Green MR, Monti S, Rodig SJ, Juszczynski P, Currie T, O'Donnell E, et al. Integrative analysis reveals selective 9p24.1 amplification, increased PD-1 ligand expression, and further induction via JAK2 in nodular sclerosing Hodgkin lymphoma and primary mediastinal large b-cell lymphoma. Blood (2010) 116(17):3268–77. doi: 10.1182/blood-2010-05-282780
31. Voron T, Colussi O, Marcheteau E, Pernot S, Nizard M, Pointet AL, et al. VEGF-a modulates expression of inhibitory checkpoints on CD8+ T cells in tumors. J Exp Med (2015) 212(2):139–48. doi: 10.1084/jem.20140559
32. Nijland M, Veenstra RN, Visser L, Xu C, Kushekhar K, van Imhoff GW, et al. HLA dependent immune escape mechanisms in b-cell lymphomas: Implications for immune checkpoint inhibitor therapy? Oncoimmunology (2017) 6(4):e1295202. doi: 10.1080/2162402X.2017.1295202
33. Spranger S, Spaapen RM, Zha Y, Williams J, Meng Y, Ha TT, et al. Up-regulation of PD-L1, IDO, and t(regs) in the melanoma tumor microenvironment is driven by CD8(+) T cells. Sci Transl Med (2013) 5(200):200ra116. doi: 10.1126/scitranslmed.3006504
34. Tiacci E, Doring C, Brune V, van Noesel CJ, Klapper W, Mechtersheimer G, et al. Analyzing primary Hodgkin and reed-sternberg cells to capture the molecular and cellular pathogenesis of classical Hodgkin lymphoma. Blood (2012) 120(23):4609–20. doi: 10.1182/blood-2012-05-428896
35. Horenstein AL, Chillemi A, Zaccarello G, Bruzzone S, Quarona V, Zito A, et al. A CD38/CD203a/CD73 ectoenzymatic pathway independent of CD39 drives a novel adenosinergic loop in human T lymphocytes. Oncoimmunology (2013) 2(9):e26246. doi: 10.4161/onci.26246
36. Beavis PA, Milenkovski N, Henderson MA, John LB, Allard B, Loi S, et al. Adenosine receptor 2A blockade increases the efficacy of anti-PD-1 through enhanced antitumor T-cell responses. Cancer Immunol Res (2015) 3(5):506–17. doi: 10.1158/2326-6066.CIR-14-0211
37. Nowicki TS, Hu-Lieskovan S, Ribas A. Mechanisms of resistance to PD-1 and PD-L1 blockade. Cancer J (2018) 24(1):47–53. doi: 10.1097/PPO.0000000000000303
38. Brentuximab vedotin and nivolumab with or without ipilimumab in treating patients with relapsed or refractory Hodgkin lymphoma. In: NIH National cancer institute. Available at: https://www.cancer.gov/about-cancer/treatment/clinical-trials/search/v?id=NCI-2013-01275&r=1.
39. Kwok G, Yau TC, Chiu JW, Tse E, Kwong YL. Pembrolizumab (Keytruda). Hum Vaccin Immunother (2016) 12(11):2777–89. doi: 10.1080/21645515.2016.1199310
40. Puccini A, Battaglin F, Iaia ML, Lenz HJ, Salem ME. Overcoming resistance to anti-PD1 and anti-PD-L1 treatment in gastrointestinal malignancies. J Immunother Cancer (2020) 8(1). doi: 10.1136/jitc-2019-000404
41. Dos Santos LV, Abrahao CM, William WN Jr. Overcoming resistance to immune checkpoint inhibitors in head and neck squamous cell carcinomas. Front Oncol (2021) 11:596290. doi: 10.3389/fonc.2021.596290
42. Fessler J, Matson V, Gajewski TF. Exploring the emerging role of the microbiome in cancer immunotherapy. J Immunother Cancer (2019) 7(1):108. doi: 10.1186/s40425-019-0574-4
43. Flood BA, Higgs EF, Li S, Luke JJ, Gajewski TF. STING pathway agonism as a cancer therapeutic. Immunol Rev (2019) 290(1):24–38. doi: 10.1111/imr.12765
44. Duvic M, Evans M, Wang C. Mogamulizumab for the treatment of cutaneous T-cell lymphoma: recent advances and clinical potential. Ther Adv Hematol (2016) 7(3):171–4. doi: 10.1177/2040620716636541
45. Beygi S, Fernandez-Pol S, Duran G, Kim YH, Khodadoust MS. Resistance to mogamulizumab is associated with loss of CCR4 in cutaneous T cell lymphoma. Blood (2021) 138(Supplement 1):1325–. doi: 10.1182/blood-2021-153177
46. Clinical trials using mogamulizumab. In: NIH National cancer institute. Available at: https://www.cancer.gov/about-cancer/treatment/clinical-trials/intervention/mogamulizumab?redirect=true.
47. Sharma A, Shah SR, Illum H, Dowell J. Vemurafenib: targeted inhibition of mutated BRAF for treatment of advanced melanoma and its potential in other malignancies. Drugs (2012) 72(17):2207–22. doi: 10.2165/11640870-000000000-00000
48. Turski ML, Vidwans SJ, Janku F, Garrido-Laguna I, Munoz J, Schwab R, et al. Genomically driven tumors and actionability across histologies: BRAF-mutant cancers as a paradigm. Mol Cancer Ther (2016) 15(4):533–47. doi: 10.1158/1535-7163.MCT-15-0643
49. Munoz J, Janku F, Cohen PR, Kurzrock R. Erdheim-Chester disease: characteristics and management. Mayo Clin Proc (2014) 89(7):985–96. doi: 10.1016/j.mayocp.2014.01.023
50. Janku F, Munoz J, Subbiah V, Kurzrock R. A tale of two histiocytic disorders. Oncologist. (2013) 18(1):2–4. doi: 10.1634/theoncologist.2012-0440
51. Diamond EL, Subbiah V, Lockhart AC, Blay JY, Puzanov I, Chau I, et al. Vemurafenib for BRAF V600-mutant erdheim-Chester disease and langerhans cell histiocytosis: Analysis of data from the histology-independent, phase 2, open-label VE-BASKET study. JAMA Oncol (2018) 4(3):384–8. doi: 10.1001/jamaoncol.2017.5029
52. Park JH, Chung SS, Durham B, Chung YR, Scott S, Getta B, et al. Diverse mechanisms of vemurafenib resistance in BRAF-mutant hairy cell leukemia. Blood (2015) 126(23):449–. doi: 10.1182/blood.V126.23.449.449
53. Durham BH, Getta B, Dietrich S, Taylor J, Won H, Bogenberger JM, et al. Genomic analysis of hairy cell leukemia identifies novel recurrent genetic alterations. Blood. (2017) 130(14):1644–8. doi: 10.1182/blood-2017-01-765107
54. Caeser R, Collord G, Yao W-Q, Chen Z, Vassiliou GS, Beer PA, et al. Targeting MEK in vemurafenib-resistant hairy cell leukemia. Leukemia (2019) 33(2):541–5. doi: 10.1038/s41375-018-0270-2
55. Morris SW, Kirstein MN, Valentine MB, Dittmer KG, Shapiro DN, Saltman DL, et al. Fusion of a kinase gene, ALK, to a nucleolar protein gene, NPM, in non-hodgkin's lymphoma. Science (1994) 263(5151):1281–4. doi: 10.1126/science.8122112
56. Larose H, Burke GAA, Lowe EJ, Turner SD. From bench to bedside: the past, present and future of therapy for systemic paediatric ALCL, ALK. Br J Haematol (2019) 185(6):1043–54. doi: 10.1111/bjh.15763
57. Camidge DR. Next-generation ALK inhibitors: is the median the message? Lancet Respir Med (2020) 8(1):5–7. doi: 10.1016/S2213-2600(19)30362-5
58. Hu G, Feldman AL. Drivers of crizotinib resistance in ALK+ ALCL. Blood (2020) 136(14):1573–5. doi: 10.3390%2Fcancers13236003
59. Muller IB, De Langen AJ, Honeywell RJ, Giovannetti E, Peters GJ. Overcoming crizotinib resistance in ALK-rearranged NSCLC with the second-generation ALK-inhibitor ceritinib. Expert Rev Anticancer Ther (2016) 16(2):147–57. doi: 10.1586/14737140.2016.1131612
60. George B, Chowdhury SM, Hart A, Sircar A, Singh SK, Nath UK, et al. Ibrutinib resistance mechanisms and treatment strategies for b-cell lymphomas. Cancers (Basel) (2020) 12(5). doi: 10.3390/cancers12051328
61. Woyach JA, Furman RR, Liu T-M, Ozer HG, Zapatka M, Ruppert AS, et al. Resistance mechanisms for the bruton's tyrosine kinase inhibitor ibrutinib. N Engl J Med (2014) 370(24):2286–94. doi: 10.1056/NEJMoa1400029
62. Lampson BL, Brown JR. Are BTK and PLCG2 mutations necessary and sufficient for ibrutinib resistance in chronic lymphocytic leukemia? Expert Rev Hematol (2018) 11(3):185–94. doi: 10.1080/17474086.2018.1435268
63. Xu L, Tsakmaklis N, Yang G, Chen JG, Liu X, Demos M, et al. Acquired mutations associated with ibrutinib resistance in waldenstrom macroglobulinemia. Blood (2017) 129(18):2519–25. doi: 10.1182/blood-2017-01-761726
64. Epperla N, Shana'ah AY, Jones D, Christian BA, Ayyappan S, Maddocks K, et al. Resistance mechanism for ibrutinib in marginal zone lymphoma. Blood Adv (2019) 3(4):500–2. doi: 10.1182/bloodadvances.2018029058
65. Hershkovitz-Rokah O, Pulver D, Lenz G, Shpilberg O. Ibrutinib resistance in mantle cell lymphoma: clinical, molecular and treatment aspects. Br J Haematol (2018) 181(3):306–19. doi: 10.1111/bjh.15108
66. Jimenez C, Chan GG, Xu L, Tsakmaklis N, Chen JG, Demos M, et al. Genomic analysis of ibrutinib resistance in waldenstrom macroglobulinemia. Blood (2018) 132(Supplement 1):1372. doi: 10.1182/blood-2018-99-118819
67. Treon SP, Tripsas CK, Meid K, Warren D, Varma G, Green R, et al. Ibrutinib in previously treated waldenstrom's macroglobulinemia. N Engl J Med (2015) 372(15):1430–40. doi: 10.1056/NEJMoa1501548
68. Deshpande A, Munoz J. Zanubrutinib in treating waldenstrom macroglobulinemia, the last shall be the first. Ther Clin Risk Manage (2022) 18:657–68. doi: 10.2147/TCRM.S338655
69. Watanabe K, Terakura S, Martens AC, van Meerten T, Uchiyama S, Imai M, et al. Target antigen density governs the efficacy of anti-CD20-CD28-CD3 zeta chimeric antigen receptor-modified effector CD8+ T cells. J Immunol (2015) 194(3):911–20. doi: 10.4049/jimmunol.1402346
70. Kim JH, Kim WS, Ryu K, Kim SJ, Park C. CD79B limits response of diffuse large b cell lymphoma to ibrutinib. Leuk Lymphoma (2016) 57(6):1413–22. doi: 10.3109/10428194.2015.1113276
71. Lee J, Zhang LL, Wu W, Guo H, Li Y, Sukhanova M, et al. Activation of MYC, a bona fide client of HSP90, contributes to intrinsic ibrutinib resistance in mantle cell lymphoma. Blood Adv (2018) 2(16):2039–51. doi: 10.1182/bloodadvances.2018016048
72. Tavakoli Shirazi P, Eadie LN, Page EC, Heatley SL, Bruning JB, White DL. Constitutive JAK/STAT signaling is the primary mechanism of resistance to JAKi in TYK2-rearranged acute lymphoblastic leukemia. Cancer Letters (2021) 512:28–37. doi: 10.1016/j.canlet.2021.04.027
73. Hamlin PA, Patel MR, Stevens D, Hess BT, Munoz J, Feldman TA, et al. Phase 2a study of the dual SYK/JAK inhibitor cerdulatinib (ALXN2075) as monotherapy or in combination with rituximab in patients with Relapsed/Refractory follicular lymphoma. Blood (2021) 138(Supplement 1):2423–. doi: 10.1182/blood-2021-148313
74. Scheffold A, Jebaraj BMC, Tausch E, Bloehdorn J, Ghia P, Yahiaoui A, et al. IGF1R as druggable target mediating PI3K-delta inhibitor resistance in a murine model of chronic lymphocytic leukemia. Blood (2019) 134(6):534–47. doi: 10.1182/blood.2018881029
75. Murali I, Kasar S, McWilliams EM, Itchaki G, Tyekucheva S, Livitz D, et al. Activating MAPK pathway mutations mediate primary resistance to PI3K inhibitors in chronic lymphocytic leukemia (CLL). Blood (2018) 132(Supplement 1):587–. doi: 10.1182/blood-2018-99-115304
76. Kim JH, Kim WS, Park C. Interleukin-6 mediates resistance to PI3K-pathway-targeted therapy in lymphoma. BMC Cancer (2019) 19(1):936. doi: 10.1186/s12885-019-6057-7
77. Arribas AJ, Napoli S, Cascione L, Gaudio E, Bordone-Pittau R, Barreca M, et al. Abstract PO-46: Mechanisms of resistance to the PI3K inhibitor copanlisib in marginal zone lymphoma. Blood Cancer Discov (2020) 1(3_Supplement):PO–46. doi: 10.1158/2643-3249.LYMPHOMA20-PO-46
78. Tausch E, Close W, Dolnik A, Bloehdorn J, Chyla B, Bullinger L, et al. Venetoclax resistance and acquired BCL2 mutations in chronic lymphocytic leukemia. Haematologica (2019) 104(9):e434–e7. doi: 10.3324/haematol.2019.222588
79. Herling CD, Abedpour N, Weiss J, Schmitt A, Jachimowicz RD, Merkel O, et al. Clonal dynamics towards the development of venetoclax resistance in chronic lymphocytic leukemia. Nat Commun (2018) 9(1):727. doi: 10.1038/s41467-018-03170-7
80. Bisserier M, Wajapeyee N. Mechanisms of resistance to EZH2 inhibitors in diffuse large b-cell lymphomas. Blood. (2018) 131(19):2125–37. doi: 10.1182/blood-2017-08-804344
81. Sotillo E, Barrett DM, Black KL, Bagashev A, Oldridge D, Wu G, et al. Convergence of acquired mutations and alternative splicing of CD19 enables resistance to CART-19 immunotherapy. Cancer Discov (2015) 5(12):1282–95. doi: 10.1158/2159-8290.CD-15-1020
82. Gardner R, Wu D, Cherian S, Fang M, Hanafi LA, Finney O, et al. Acquisition of a CD19-negative myeloid phenotype allows immune escape of MLL-rearranged b-ALL from CD19 CAR-t-cell therapy. Blood (2016) 127(20):2406–10. doi: 10.1182/blood-2015-08-665547
83. Jiang Y, Chen M, Nie H, Yuan Y. PD-1 and PD-L1 in cancer immunotherapy: clinical implications and future considerations. Hum Vaccin Immunother (2019) 15(5):1111–22. doi: 10.1080/21645515.2019.1571892
84. Cosson A, Chapiro E, Bougacha N, Lambert J, Herbi L, Cung HA, et al. Gain in the short arm of chromosome 2 (2p+) induces gene overexpression and drug resistance in chronic lymphocytic leukemia: analysis of the central role of XPO1. Leukemia (2017) 31(7):1625–9. doi: 10.1038/leu.2017.100
85. Burger JA, Landau DA, Taylor-Weiner A, Bozic I, Zhang H, Sarosiek K, et al. Clonal evolution in patients with chronic lymphocytic leukaemia developing resistance to BTK inhibition. Nat Commun (2016) 7:11589. doi: 10.1038/ncomms11589
86. Jimenez C, Chan GG, Xu L, Tsakmaklis N, Kofides A, Demos MG, et al. Genomic evolution of ibrutinib-resistant clones in waldenstrom macroglobulinaemia. Br J Haematol (2020) 189(6):1165–70. doi: 10.1111/bjh.16463
87. Guerrera ML, Tsakmaklis N, Xu L, Yang G, Demos M, Kofides A, et al. MYD88 mutated and wild-type waldenstrom's macroglobulinemia: characterization of chromosome 6q gene losses and their mutual exclusivity with mutations in CXCR4. Haematologica (2018) 103(9):e408–e11. doi: 10.3324/haematol.2018.190181
88. Ma J, Xing W, Coffey G, Dresser K, Lu K, Guo A, et al. Cerdulatinib, a novel dual SYK/JAK kinase inhibitor, has broad anti-tumor activity in both ABC and GCB types of diffuse large b cell lymphoma. Oncotarget (2015) 6(41):43881–96. doi: 10.18632/oncotarget.6316
89. Guo A, Lu P, Coffey G, Conley P, Pandey A, Wang YL. Dual SYK/JAK inhibition overcomes ibrutinib resistance in chronic lymphocytic leukemia: Cerdulatinib, but not ibrutinib, induces apoptosis of tumor cells protected by the microenvironment. Oncotarget (2017) 8(8):12953–67. doi: 10.18632/oncotarget.14588
90. Fruman DA, Cantley LC. Idelalisib–a PI3Kdelta inhibitor for b-cell cancers. N Engl J Med (2014) 370(11):1061–2. doi: 10.1056/NEJMe1400055
91. Richardson NC, Kasamon Y, Pazdur R, Gormley N. The saga of PI3K inhibitors in haematological malignancies: survival is the ultimate safety endpoint. Lancet Oncol (2022) 23(5):563–6. doi: 10.1016/S1470-2045(22)00200-5
92. Munoz J, Follows GA, Nastoupil LJ. Copanlisib for the treatment of malignant lymphoma: Clinical experience and future perspectives. Target Oncol (2021) 16(3):295–308. doi: 10.1007/s11523-021-00802-9
93. Dreyling M, Santoro A, Mollica L, Leppä S, Follows G, Lenz G, et al. Long-term safety and efficacy of the PI3K inhibitor copanlisib in patients with relapsed or refractory indolent lymphoma: 2-year follow-up of the CHRONOS-1 study. Am J Hematol (2020) 95(4):362–71. doi: 10.1002/ajh.25711
94. Cheson BD, O'Brien S, Ewer MS, Goncalves MD, Farooki A, Lenz G, et al. Optimal management of adverse events from copanlisib in the treatment of patients with non-Hodgkin lymphomas. Clin Lymphoma Myeloma Leuk (2019) 19(3):135–41. doi: 10.1016/j.clml.2018.11.021
95. Zinzani PL, Santoro A, Mollica L, Leppä S, Follows GA, Lenz G, et al. Copanlisib, a PI3K inhibitor, demonstrates a favorable long-term safety profile in a pooled analysis of patients with hematologic malignancies. Blood (2019) 134(Supplement_1):4009–. doi: 10.1182/blood-2019-131779
96. Munoz J, Dhillon N, Janku F, Watowich SS, Hong DS. STAT3 inhibitors: finding a home in lymphoma and leukemia. Oncologist (2014) 19(5):536–44. doi: 10.1634/theoncologist.2013-0407
97. Yue X, Chen Q, He J. Combination strategies to overcome resistance to the BCL2 inhibitor venetoclax in hematologic malignancies.Cancer Cell Int (2020) 20(1):524. doi: 10.1186/s12935-020-01614-z
98. Yue X, Chen Q, He J. Combination strategies to overcome resistance to the BCL2 inhibitor venetoclax in hematologic malignancies. Cancer Cell Int (2020) 20(1):524. doi: 10.1186/s12935-020-01614-z
99. Duan R, Du W, Guo W. EZH2: a novel target for cancer treatment. J Hematol Oncol (2020) 13(1):104. doi: 10.1186/s13045-020-00937-8
100. Julia E, Salles G. EZH2 inhibition by tazemetostat: mechanisms of action, safety and efficacy in relapsed/refractory follicular lymphoma. Future Oncol (2021) 17(17):2127–40. doi: 10.2217/fon-2020-1244
101. Porter DL, Levine BL, Kalos M, Bagg A, June CH. Chimeric antigen receptor-modified T cells in chronic lymphoid leukemia. N Engl J Med (2011) 365(8):725–33. doi: 10.1056/NEJMoa1103849
102. Nastoupil LJ, Jain MD, Feng L, Spiegel JY, Ghobadi A, Lin Y, et al. Standard-of-Care axicabtagene ciloleucel for relapsed or refractory Large b-cell lymphoma: Results from the US lymphoma CAR T consortium. J Clin Oncol (2020) 38(27):3119–28. doi: 10.1200/JCO.19.02104
103. Neelapu SS, Locke FL, Bartlett NL, Lekakis LJ, Miklos DB, Jacobson CA, et al. Axicabtagene ciloleucel CAR T-cell therapy in refractory Large b-cell lymphoma. N Engl J Med (2017) 377(26):2531–44. doi: 10.1056/NEJMoa1707447
104. Locke FL, Ghobadi A, Jacobson CA, Miklos DB, Lekakis LJ, Oluwole OO, et al. Long-term safety and activity of axicabtagene ciloleucel in refractory large b-cell lymphoma (ZUMA-1): a single-arm, multicentre, phase 1-2 trial. Lancet Oncol (2019) 20(1):31–42. doi: 10.1016/S1470-2045(18)30864-7
105. Neelapu SS, Jacobson CA, Oluwole OO, Munoz J, Deol A, Miklos DB, et al. Outcomes of older patients in ZUMA-1, a pivotal study of axicabtagene ciloleucel in refractory large b-cell lymphoma. Blood. (2020) 135(23):2106–9. doi: 10.1182/blood.2019004162
106. Wang M, Munoz J, Goy A, Locke FL, Jacobson CA, Hill BT, et al. KTE-X19 CAR T-cell therapy in relapsed or refractory mantle-cell lymphoma. N Engl J Med (2020) 382(14):1331–42. doi: 10.1056/NEJMoa1914347
107. Wang M, Munoz J, Goy A, Locke FL, Jacobson CA, Hill BT, et al. Three-year follow-up of KTE-X19 in patients with Relapsed/Refractory mantle cell lymphoma, including high-risk subgroups, in the ZUMA-2 study. J Clin Oncol (2022), JCO2102370. doi: 10.1200/JCO.21.02370
108. Deshpande A, Wang Y, Munoz J, Jain P. Brexucabtagene autoleucel: a breakthrough in the treatment of mantle cell lymphoma. Drugs Today (Barc) (2022) 58(6):283–98. doi: 10.1358/dot.2022.58.6.3378055
109. Jacobson CA, Chavez JC, Sehgal AR, William BM, Munoz J, Salles G, et al. Axicabtagene ciloleucel in relapsed or refractory indolent non-Hodgkin lymphoma (ZUMA-5): a single-arm, multicentre, phase 2 trial. Lancet Oncol (2022) 23(1):91–103. doi: 10.1016/S1470-2045(21)00591-X
110. Neelapu SS, Dickinson M, Munoz J, Ulrickson ML, Thieblemont C, Oluwole OO, et al. Axicabtagene ciloleucel as first-line therapy in high-risk large b-cell lymphoma: the phase 2 ZUMA-12 trial. Nat Med (2022) 28(4):735–42. doi: 10.1038/s41591-022-01731-4
111. Locke FL, Miklos DB, Jacobson CA, Perales MA, Kersten MJ, Oluwole OO, et al. Axicabtagene ciloleucel as second-line therapy for Large b-cell lymphoma. N Engl J Med (2022) 386(7):640–54. doi: 10.1056/NEJMoa2116133
112. Iqbal M, Bansal R, Yassine F, Gandhi S, Rosenthal A, Moustafa MA, et al. Impact of rituximab and corticosteroids on late cytopenias post chimeric antigen receptor T-cell therapy. Transplant Cell Ther (2022). doi: 10.1016/j.jtct.2022.07.009
113. Jacobs MT, Jain MD, Gao F, Nastoupil LJ, Spiegel JY, Lin Y, et al. Severity of cytokine release syndrome influences outcome after axicabtagene ciloleucel for Large b cell lymphoma: Results from the US lymphoma CAR-T consortium. Clin Lymphoma Myeloma Leuk (2022). doi: 10.1016/j.clml.2022.05.004
114. Saifi O, Breen WG, Lester SC, Rule WG, Stish B, Rosenthal A, et al. Does bridging radiation therapy affect the pattern of failure after CAR T-cell therapy in non-Hodgkin lymphoma? Radiother Oncol (2022) 166:171–9. doi: 10.1016/j.radonc.2021.11.031
115. Oluwole OO, Bouabdallah K, Munoz J, De Guibert S, Vose JM, Bartlett NL, et al. Prophylactic corticosteroid use in patients receiving axicabtagene ciloleucel for large b-cell lymphoma. Br J Haematol (2021) 194(4):690–700. doi: 10.1111/bjh.17527
116. Deshpande A, Rule W, Rosenthal A. Radiation and chimeric antigen receptor T-cell therapy in b-cell non-Hodgkin lymphomas. Curr Treat Options Oncol (2022) 23(1):89–98. doi: 10.1007/s11864-021-00935-z
117. Munoz JL, Wang Y, Jain P, Wang M. BTK inhibitors and CAR T-cell therapy in treating mantle cell lymphoma-finding a dancing partner. Curr Oncol Rep (2022). doi: 10.1007/s11912-022-01286-0
118. Jacobson CA, Westin JR, Miklos DB, Herrera AF, Lee J, Seng J, et al. Abstract CT055: Phase 1/2 primary analysis of ZUMA-6: Axicabtagene ciloleucel (Axi-cel) in combination with atezolizumab (Atezo) for the treatment of patients (Pts) with refractory diffuse large b cell lymphoma (DLBCL). Cancer Res (2020) 80(16_Supplement):CT055–CT. doi: 10.1158/1538-7445.AM2020-CT055
119. Furman RR, Cheng S, Lu P, Setty M, Perez AR, Guo A, et al. Ibrutinib resistance in chronic lymphocytic leukemia. N Engl J Med (2014) 370(24):2352–4. doi: 10.1056/NEJMc1402716
120. Chiron D, Di Liberto M, Martin P, Huang X, Sharman J, Blecua P, et al. Cell-cycle reprogramming for PI3K inhibition overrides a relapse-specific C481S BTK mutation revealed by longitudinal functional genomics in mantle cell lymphoma. Cancer Discov (2014) 4(9):1022–35. doi: 10.1158/2159-8290.CD-14-0098
121. Byrd JC, Smith S, Wagner-Johnston N, Sharman J, Chen AI, Advani R, et al. First-in-human phase 1 study of the BTK inhibitor GDC-0853 in relapsed or refractory b-cell NHL and CLL. Oncotarget (2018) 9(16):13023–35. doi: 10.18632/oncotarget.24310
122. Reiff SD, Mantel R, Smith LL, Greene JT, Muhowski EM, Fabian CA, et al. The BTK inhibitor ARQ 531 targets ibrutinib-resistant CLL and Richter transformation. Cancer Discov (2018) 8(10):1300–15. doi: 10.1158/2159-8290.CD-17-1409
123. Sun Y, Ding N, Song Y, Yang Z, Liu W, Zhu J, et al. Degradation of bruton's tyrosine kinase mutants by PROTACs for potential treatment of ibrutinib-resistant non-Hodgkin lymphomas. Leukemia (2019) 33(8):2105–10. doi: 10.1038/s41375-019-0440-x
124. Sun Y, Zhao X, Ding N, Gao H, Wu Y, Yang Y, et al. PROTAC-induced BTK degradation as a novel therapy for mutated BTK C481S induced ibrutinib-resistant b-cell malignancies. Cell Res (2018) 28(7):779–81. doi: 10.1038/s41422-018-0055-1
125. Liu TM, Woyach JA, Zhong Y, Lozanski A, Lozanski G, Dong S, et al. Hypermorphic mutation of phospholipase c, gamma2 acquired in ibrutinib-resistant CLL confers BTK independency upon b-cell receptor activation. Blood. (2015) 126(1):61–8. doi: 10.1182/blood-2015-02-626846
126. Walliser C, Hermkes E, Schade A, Wiese S, Deinzer J, Zapatka M, et al. The phospholipase Cgamma2 mutants R665W and L845F identified in ibrutinib-resistant chronic lymphocytic leukemia patients are hypersensitive to the rho GTPase Rac2 protein. J Biol Chem (2016) 291(42):22136–48. doi: 10.1074/jbc.M116.746842
127. Kanagal-Shamanna R, Jain P, Patel KP, Routbort M, Bueso-Ramos C, Alhalouli T, et al. Targeted multigene deep sequencing of bruton tyrosine kinase inhibitor-resistant chronic lymphocytic leukemia with disease progression and Richter transformation. Cancer (2019) 125(4):559–74. doi: 10.1002/cncr.31831
128. Wu C, de Miranda NF, Chen L, Wasik AM, Mansouri L, Jurczak W, et al. Genetic heterogeneity in primary and relapsed mantle cell lymphomas: Impact of recurrent CARD11 mutations. Oncotarget (2016) 7(25):38180–90. doi: 10.18632/oncotarget.9500
129. Wilson WH, Young RM, Schmitz R, Yang Y, Pittaluga S, Wright G, et al. Targeting b cell receptor signaling with ibrutinib in diffuse large b cell lymphoma. Nat Med (2015) 21(8):922–6. doi: 10.1038/nm.3884
130. Lenz G, Balasubramanian S, Goldberg J, Rizo A, Schaffer M, Phelps C, et al. Sequence variants in patients with primary and acquired resistance to ibrutinib in the phase 3 MCL3001 (RAY) trial. J Clin Oncol (2016) 34(15_suppl):7570. doi: 10.1200/JCO.2016.34.15_suppl.7570
131. Rahal R, Frick M, Romero R, Korn JM, Kridel R, Chan FC, et al. Pharmacological and genomic profiling identifies NF-kappaB-targeted treatment strategies for mantle cell lymphoma. Nat Med (2014) 20(1):87–92. doi: 10.1038/nm.3435
132. Mohanty A, Sandoval N, Das M, Pillai R, Chen L, Chen RW, et al. CCND1 mutations increase protein stability and promote ibrutinib resistance in mantle cell lymphoma. Oncotarget (2016) 7(45):73558–72. doi: 10.18632/oncotarget.12434
133. Zhang L, Yao Y, Zhang S, Liu Y, Guo H, Ahmed M, et al. Metabolic reprogramming toward oxidative phosphorylation identifies a therapeutic target for mantle cell lymphoma. Sci Transl Med (2019) 11(491). doi: 10.1126/scitranslmed.aau1167
134. Choi J, Phelan JD, Wright GW, Haupl B, Huang DW, Shaffer AL 3rd, et al. Regulation of b cell receptor-dependent NF-kappaB signaling by the tumor suppressor KLHL14. Proc Natl Acad Sci USA (2020) 117(11):6092–102. doi: 10.1073/pnas.1921187117
135. Phelan JD, Young RM, Webster DE, Roulland S, Wright GW, Kasbekar M, et al. A multiprotein supercomplex controlling oncogenic signalling in lymphoma. Nature (2018) 560(7718):387–91. doi: 10.1038/s41586-018-0290-0
136. Kapoor I, Li Y, Sharma A, Zhu H, Bodo J, Xu W, et al. Resistance to BTK inhibition by ibrutinib can be overcome by preventing FOXO3a nuclear export and PI3K/AKT activation in b-cell lymphoid malignancies. Cell Death Dis (2019) 10(12):924. doi: 10.1038/s41419-019-2158-0
137. Zhao X, Lwin T, Silva A, Shah B, Tao J, Fang B, et al. Unification of de novo and acquired ibrutinib resistance in mantle cell lymphoma. Nat Commun (2017) 8:14920. doi: 10.1038/ncomms14920
138. Guan J, Huang D, Yakimchuk K, Okret S. p110alpha inhibition overcomes stromal cell-mediated ibrutinib resistance in mantle cell lymphoma. Mol Cancer Ther (2018) 17(5):1090–100. doi: 10.1158/1535-7163.MCT-17-0784
139. de Rooij MF, Kuil A, Kater AP, Kersten MJ, Pals ST, Spaargaren M. Ibrutinib and idelalisib synergistically target BCR-controlled adhesion in MCL and CLL: a rationale for combination therapy. Blood (2015) 125(14):2306–9. doi: 10.1182/blood-2014-12-619163
140. Davids MS, Kim HT, Nicotra A, Savell A, Francoeur K, Hellman JM, et al. Umbralisib in combination with ibrutinib in patients with relapsed or refractory chronic lymphocytic leukaemia or mantle cell lymphoma: a multicentre phase 1-1b study. Lancet Haematol (2019) 6(1):e38–47. doi: 10.1016/S2352-3026(18)30196-0
141. Hing ZA, Fung HY, Ranganathan P, Mitchell S, El-Gamal D, Woyach JA, et al. Next-generation XPO1 inhibitor shows improved efficacy and in vivo tolerability in hematological malignancies. Leukemia (2016) 30(12):2364–72. doi: 10.1038/leu.2016.136
142. Hing ZA, Mantel R, Beckwith KA, Guinn D, Williams E, Smith LL, et al. Selinexor is effective in acquired resistance to ibrutinib and synergizes with ibrutinib in chronic lymphocytic leukemia. Blood (2015) 125(20):3128–32. doi: 10.1182/blood-2015-01-621391
143. Ming M, Wu W, Xie B, Sukhanova M, Wang W, Kadri S, et al. XPO1 inhibitor selinexor overcomes intrinsic ibrutinib resistance in mantle cell lymphoma via nuclear retention of IkappaB. Mol Cancer Ther (2018) 17(12):2564–74. doi: 10.1158/1535-7163.MCT-17-0789-ATR
144. Lukas M, Velten B, Sellner L, Tomska K, Huellein J, Walther T, et al. Survey of ex vivo drug combination effects in chronic lymphocytic leukemia reveals synergistic drug effects and genetic dependencies. Leukemia. (2020) 34(11):2934–50. doi: 10.1038/s41375-020-0846-5
145. Gaudio E, Tarantelli C, Kwee I, Barassi C, Bernasconi E, Rinaldi A, et al. Combination of the MEK inhibitor pimasertib with BTK or PI3K-delta inhibitors is active in preclinical models of aggressive lymphomas. Ann Oncol (2016) 27(6):1123–8. doi: 10.1093/annonc/mdw131
146. Hillmen P, Rawstron AC, Brock K, Munoz-Vicente S, Yates FJ, Bishop R, et al. Ibrutinib plus venetoclax in Relapsed/Refractory chronic lymphocytic leukemia: The CLARITY study. J Clin Oncol (2019) 37(30):2722–9. doi: 10.1200/JCO.19.00894
147. Jain N, Keating M, Thompson P, Ferrajoli A, Burger J, Borthakur G, et al. Ibrutinib and venetoclax for first-line treatment of CLL. N Engl J Med (2019) 380(22):2095–103. doi: 10.1056/NEJMoa1900574
148. Cervantes-Gomez F, Lamothe B, Woyach JA, Wierda WG, Keating MJ, Balakrishnan K, et al. Pharmacological and protein profiling suggests venetoclax (ABT-199) as optimal partner with ibrutinib in chronic lymphocytic leukemia. Clin Cancer Res (2015) 21(16):3705–15. doi: 10.1158/1078-0432.CCR-14-2809
149. Kater AP, Seymour JF, Hillmen P, Eichhorst B, Langerak AW, Owen C, et al. Fixed duration of venetoclax-rituximab in Relapsed/Refractory chronic lymphocytic leukemia eradicates minimal residual disease and prolongs survival: Post-treatment follow-up of the MURANO phase III study. J Clin Oncol (2019) 37(4):269–77. doi: 10.1200/JCO.18.01580
150. Galicia-Vazquez G, Aloyz R. Ibrutinib resistance is reduced by an inhibitor of fatty acid oxidation in primary CLL lymphocytes. Front Oncol (2018) 8:411. doi: 10.3389/fonc.2018.00411
151. Burger M, Hartmann T, Krome M, Rawluk J, Tamamura H, Fujii N, et al. Small peptide inhibitors of the CXCR4 chemokine receptor (CD184) antagonize the activation, migration, and antiapoptotic responses of CXCL12 in chronic lymphocytic leukemia b cells. Blood (2005) 106(5):1824–30. doi: 10.1182/blood-2004-12-4918
152. Mraz M, Zent CS, Church AK, Jelinek DF, Wu X, Pospisilova S, et al. Bone marrow stromal cells protect lymphoma b-cells from rituximab-induced apoptosis and targeting integrin alpha-4-beta-1 (VLA-4) with natalizumab can overcome this resistance. Br J Haematol (2011) 155(1):53–64. doi: 10.1111/j.1365-2141.2011.08794.x
Keywords: lymphoma, targeted therapy, resistance, mechanism of action, CAR T-cells, tazemetostat, cerdulatinib
Citation: Deshpande A and Munoz J (2022) Targeted and cellular therapies in lymphoma: Mechanisms of escape and innovative strategies. Front. Oncol. 12:948513. doi: 10.3389/fonc.2022.948513
Received: 19 May 2022; Accepted: 08 August 2022;
Published: 12 September 2022.
Edited by:
Bartosz Puła, Institute of Hematology and Transfusiology (IHT), PolandReviewed by:
Steven Park, Wake Forest University, United StatesNarendranath Epperla, The Ohio State University, United States
Copyright © 2022 Deshpande and Munoz. This is an open-access article distributed under the terms of the Creative Commons Attribution License (CC BY). The use, distribution or reproduction in other forums is permitted, provided the original author(s) and the copyright owner(s) are credited and that the original publication in this journal is cited, in accordance with accepted academic practice. No use, distribution or reproduction is permitted which does not comply with these terms.
*Correspondence: Anagha Deshpande, RGVzaHBhbmRlLmFuYWdoYUBtYXlvLmVkdQ==