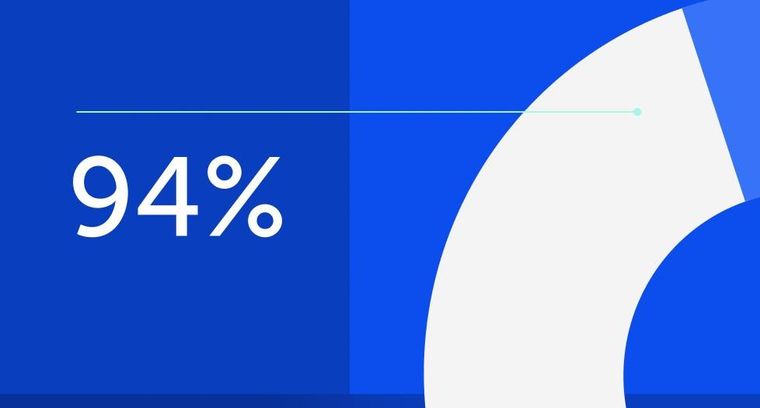
94% of researchers rate our articles as excellent or good
Learn more about the work of our research integrity team to safeguard the quality of each article we publish.
Find out more
REVIEW article
Front. Oncol., 01 August 2022
Sec. Cancer Metabolism
Volume 12 - 2022 | https://doi.org/10.3389/fonc.2022.941618
This article is part of the Research TopicFrom Metabolism Reprogramming to Cancer Cell Fate: Ferroptosis, Apoptosis, Pyroptosis and SenescenceView all 6 articles
Ferroptosis refers to iron-dependent, specialized, and regulated-necrosis mediated by lipid peroxidation, which is closely related to a variety of diseases, including cancer. Tumor cells undergo extensive changes in lipid metabolism, including lipid peroxidation and ferroptosis. Changes in lipid metabolism are critical for the regulation of ferroptosis and thus have important roles in cancer therapy. In this review, we introduce the characteristics of ferroptosis and briefly analyze the links between several metabolic mechanisms and ferroptosis. The effects of lipid peroxides, several signaling pathways, and the molecules and pathways involved in lipid metabolism on ferroptosis were extensively analyzed. Finally, our review highlights some ferroptosis-based treatments and presents some methods and examples of how these treatments can be combined with other treatments.
The discovery of ferroptosis stemmed from the identification of systematic xCT in 1980 (1). It was not until 2012 that Dixon al. formally proposed the concept of ferroptosis (2). And ferroptosis is morphologically, biochemically and genetically different from other regulated cell death (RCD).
The morphology of ferroptosis cells is significantly different from other RCD. Macroscopically, ferroptosis cells show swelling of the cytoplasm as well as organelles, deformation of the plasma membrane, and decreased chromatin condensation similar to necrosis (3, 4). Microscopically, ferroptosis cells show abnormalities in the mitochondrial structure, such as mitochondrial outer membrane rupture, an increase in mitochondrial membrane density, and reduced or even disappearance of the mitochondrial cristae.
Abnormal iron and lipid metabolism in ferroptosis is obvious, iron is a key factor in lipid oxidation and ferroptosis, and abnormalities in its input, secretion, distribution, and storage can affect ferroptosis (5, 6). Mitochondria is an important organelle involved in the production of ROS (7), and lipid peroxides are type of ROS that directly induce ferroptosis (8). Normal cellular biological processes require the participation of ROS, but excessive ROS will destroy cellular components and lead to cell death (9). Two signaling axes, Xc-/GSH/GPX4 and FSP1/DHODH/CoQ10 protect cells from ferroptosis. Specific regulatory factors and mechanisms are detailed below (Figure 1).
Figure 1 The regulatory substances and mechanisms of ferroptosis. Ferroptosis is driven by the accumulation of lipid peroxides. Iron metabolism, PUFA synthesis, and peroxidation promote the production of PUFA peroxides. AA and AdA are the key substances in the synthesis of PUFA. MUFA (such as OA) and Lipid droplets inhibit ferroptosis to a certain extent by affecting the synthesis of PUFA. NADPH has been shown to prevent lipid damage and counteract ferroptosis. System Xc-/GSH/GPX4 axis and FSP1/DHODH/CoQ10 axis can inhibit ferroptosis through antioxidant effects. The synthesis process of cholesterol is closely related to these two antioxidant axes, and IPP and FPP are the key substances.
Current research shows that ferroptosis is inextricably linked to amino acid, glucose, iron, and lipid metabolism. Glutamate and glutamine involved in the amino acid pathway regulate ferroptosis (10). Cysteine input through system Xc- belongs to the amino acid pathway, and the transsulfurization pathway allows cells to convert methionine to cysteine. The mitochondrial TCA cycle and electron transport chain can promote cysteine deprivation-induced ferroptosis, the process involving amino acid metabolism, lipid metabolism, and glucose metabolism (11).
Excessive accumulation of lipid peroxides is an important link in the process of ferroptosis, and substrates and enzymes involved in lipid peroxidation can regulate ferroptosis.
Ferroptosis is accompanied by the accumulation of lipid peroxides. Lipidomics analysis showed that PEs containing AA or epinephrine AdA in the membrane phospholipid family are susceptible to lipid peroxidation (12). Acyl-CoA synthetase long-chain family member 4 (ACSL4) and lysophosphatidylcholine acyltransferase 3 (LPCAT3) can acylate AA/AdA to membrane phospholipids, affect the properties of PUFA, and play important regulatory roles in ferroptosis (13, 14).
ACSL4 is an indispensable enzyme for lipid metabolism that catalyzes the binding of free long-chain fatty acids to CoA and preferentially recognizes arachidonic acid, and acylates it (15), after which LPCAT3 catalyzes the acylated AA into PL (14). The inhibition of these two enzymes can block the accumulation of lipid peroxides during ferroptosis, thus inhibiting ferroptosis to a certain extent. In addition, FSP1 catalyzes the conversion of ubiquinone to ubiquinol and prevents lipid peroxidation in the cell membranes (16).
Lipid peroxidation can be divided into enzymatic and non-enzymatic reactions (17), which the latter mainly refer to the entry of iron into cells through transferrin, followed by the Fenton reaction with hydrogen peroxide where excess oxygen free radicals are generated (18). Lipid oxidases involved in enzymatic reactions are divided into three types, namely lipoxygenase (LOX), cytochrome P450 oxidoreductase (POR), and cyclooxygenase (COX). The production of ROS requires the participation of these enzymes, furthermore, these enzymes are involved in ferroptosis (19). PUFA substrates are oxidized in the U-shaped fatty acid-binding channels of LOXs (20), the LOX gene family consists of six members: ALOX3, ALOX5, ALOX12, ALOX12B, ALOX15, and ALOX15B (21). TP53 is a tumor suppressor gene that suppresses the expression of SLC7A11 to deplete GSH, thus inducing ferroptosis (22). Different members of TP53 can induce ferroptosis via different ALOX (21, 23). Cytochrome P450 oxidoreductase (POR) can use electrons derived from the donor NADPH and provide them to downstream effectors (such as CYP and cytochrome p50) to reduce them. This causes the peroxidation of membrane polyunsaturated phospholipids which can lead to ferroptosis (24). COX-2 is upregulated during ferroptosis, however, inhibition of COX-2 does not modulate ferroptosis.
Compared with normal cells, the lipid metabolism of tumor cells is abnormal, and lipid metabolism is widespread in the process of ferroptosis. Fatty acid synthesis, fatty acid transport, the mevalonate pathway for cholesterol synthesis, and several major signaling pathways involved in lipid metabolism regulate ferroptosis.
PUFA play a unique and important role in regulating cell death, AA and AdA are the most critical substrate in ferroptosis. Hypermethylation of the promoter region results in low expression of ELOVL5 and FADS1 in intestinal-type gastric cancer (GC) cells, which are resistant to ferroptosis, and after supplementation with AA, the cells become ferroptosis-sensitive (25).
Treatment of cells with exogenous MUFA inhibits ferroptosis, and this protective effect is dependent on the involvement of ACSL3 (26). The mechanism is associated with the inhibition of ROS accumulation in the plasma membrane by MUFA, which competes with PUFA for binding to phospholipids. Increasing the ratio of saturated fatty acids (SFA) to monounsaturated fatty acids (MUFA) in cells, leads to lipotoxicity and apoptosis (27). The mechanism by which MUFA inhibits ferroptosis is distinct from its mechanism of inhibiting lipotoxicity by directing SFA into the triglyceride pool to form lipid droplets, which ultimately protects cells from lipotoxicity (28).
Normal cells rely on glucose as their primary energy source, and excess glucose is used for de novo lipogenesis. SFA and MUFA can be produced through this pathway, while PUFA level is dependent on dietary intake (29), glucose in cancer cells is used for anabolism rather than for generating oxidative energy (30). Approximately 95% of the fatty acids in tumor cells are derived from endogenous synthesis (31, 32). De novo synthesized fatty acids are stored in neutral lipids (stored in the LD) and phospholipids (in the membrane), and lipid synthesis is exuberant in cancer cells. Lipid droplets act as potential scavengers of ROS and promote de novo lipid synthesis in cancer cells (33, 34), the presence of lipid droplets inhibits cell ferroptosis to a certain extent.
In addition to de novo synthesis of fatty acids, fatty acid transporters such as fatty acid translocase (FAT/CD36), fatty acid transporter (FATP), and fatty acid-binding protein (FABP) can transport extracellular fatty acids into the cells (35). Similar to normal cells, cancer cells utilize exogenous lipids when de novo synthesis is inhibited (36).
The cluster of differentiation 36 (CD36) can be involved in fatty acid intake, and increased CD36 expression can lead to tumor metastasis. Blocking CD36 can inhibit tumor growth and metastasis in prostate cancer models (37). Fatty acid taken via CD36 is mostly stored rather than used for fatty acid oxidation, which may promote ferroptosis in CD36-overexpressing tumor cells (38). On the other hand, CD36 can suppress ferroptosis by exporting AA (39).
Besides CD36, FATP2 is also responsible for mediating fatty acid uptake. Selective inhibition of FATP2 can delay tumor progression, while loss of FATP2 leads to impaired uptake of AA, making cells resistant to ferroptosis (40). FATP2 expression was reduced in GC cells, but the cells enhanced their ability to synthesize fatty acids through de novo fatty acid synthesis so that AA deficiency had minimal effect, allowing cells to remain sensitive to ferroptosis (25).
Cholesterol can be oxidized and is an important component of the cell membrane structure and lipoprotein, studies have shown that cholesterol and ferroptosis have many links (41, 42). In addition to de novo synthesis of FAs and cholesterol by liver and adipocytes, cholesterol can also be synthesized via the mevalonate pathway, in which hydroxymethylglutaryl-CoA reductase (HMGCR) is the rate-limiting enzyme (43). Many enzymes associated with de novo synthesis and/or mevalonate pathway synthesis are thought to inhibit tumor growth (36, 44). The mevalonate pathway also affects selenoprotein biosynthesis (45), and GPX4 is a form of selenoprotein, thus, so the mevalonate pathway is related to ferroptosis. Statins have been used clinically for cholesterol-lowering therapy since their development (46), and they were later found that patients taking statins were resistant to some tumors, such as breast cancer (47). Statins can inhibit HMG-CoA reductase (HMGCR), resulting in the inactivation of GPX4 and induction of ferroptosis in mesenchymal cancer cells (48). FIN56 is a class III FIN that degrades GPX4 and activates squalene synthase (SQS), which can lead to the exhaustion of coenzyme Q10 (CoQ10), leading to ferroptosis (49), moreover, its mechanism of action involves the mevalonate pathway.
In addition to GPX4 and FSP1, there are also several signaling pathways involved in lipid metabolism that can regulate ferroptosis: the Hippo pathway involved in cell proliferation and regulation of cell size, the AMPK signaling involved in energy metabolism, and the HIF pathway involved in hypoxic conditions (50, 51). The regulatory mechanisms of these three pathways and their involvement in lipid metabolism are summarized in Table 1.
Table 1 This table introduces the three pathways that regulate ferroptosis, and some of their specific mechanisms, and states whether lipid metabolism processes are involved.
RCD includes but is not limited to autophagy, pyroptosis, necroptosis, and ferroptosis. Signal transduction in RCDs can be artificially regulated and promote tumor cell death (60). The mechanisms of RCDs are different (61–63). Mechanistically, some drugs and compounds involved in ferroptosis function by regulating iron metabolism, lipid peroxidation, or both pathways. We summarize some ferroptosis-based treatments (Table 2 and Figure 2).
Table 2 This table summarizes examples of radiation therapy, chemotherapy, immunotherapy, and other combination of treatments, and briefly describes the mechanism.
Figure 2 Mechanisms by which some treatments affect ferroptosis and tumors. Radiation therapy can generate ROS, induce lipid peroxidation, upregulate ASCL4, promote PUFA synthesis, provide a substrate for lipid peroxidation, deplete GSH and affect GPX4 to affect ferroptosis. IFN-γ in immunotherapy can affect system Xc-, which further affects ferroptosis through the GPX4 pathway. PD-L1 can directly inhibit tumors. Nanotherapy can deliver iron and chemotherapeutic drugs to cells to achieve better therapeutic effects. The impact of the immune microenvironment on ferroptosis and tumors cannot be ignored.
There is widespread dysregulation of iron metabolism in well-known cancer types, such as breast cancer, pancreatic cancer, lymphoma, hepatocellular carcinoma, glioblastoma, prostate cancer, and colorectal cancer (77). Regulation of ferroptosis via iron metabolism is a reliable approach for the treatment of different cancer types. Artesunate enhances the lysosomal activity and increases the lysosomal iron concentration in cells (78). Furthermore, it regulates the mRNA expression levels of iron-related genes (79), the expression of NCOA4 to promote ferritin phagocytosis to increase the cellular iron concentration, and other processes which can induce ferroptosis (80). Artesunate regulates iron metabolism through the above-mentioned mechanisms and induces ferroptosis in pancreatic cancer cells (81). Artesunate also inhibits Burkitt lymphoma by inducing ferroptosis (82). Dihydroartemisinin increases the autophagic degradation of ferritin by accumulating in lysosomes, increasing the cellular iron content, and inducing ferroptosis (78, 83), thereby triggering ferroptosis in glioma cells (84). Cisplatin, which is involved in ferritin phagocytosis and leads to an increase in cellular free iron levels, also induces ferroptosis (85). In a cisplatin-resistant head and neck cancer cell model, the treatment effect of artesunate was poor, and the inhibition of the NRF2-ARE pathway increased the sensitivity of artesunate and reversed the ferroptosis resistance of the model (86). Changes in iron metabolism were also involved in this process.
The two signal axes mentioned earlier, system Xc-/GSH/GPX4 axis and FSP1/DHODH/CoQ10 axis, as well as transcription factor NERF2, p53 gene, and three types of lipid oxidase: COX, cytochrome POR, and LOX, can serve as targets for regulating lipid peroxidation. In terms of regulating the lipid peroxidation pathway, gemcitabine is the main treatment drug for Pancreatic ductal adenocarcinoma (PDAC), which leads to the accumulation of ROS and induces ferroptosis when it exerts its therapeutic effect (87). Inhibition of the HSPA5-GPX4 pathway can induce and provoke ferroptosis in PDAC cells and enhance the anticancer effect of gemcitabine (88). Sorafenib can directly inhibit the function of system Xc- and activate the NRF2-SLC7A11 signaling pathway. The use of the NRF2 inhibitor trigonelline in a sorafenib-treated mouse model was found to promote ferroptosis and enhance the tumor suppressor effect of sorafenib (68). In a glioblastoma multiforme (GBM) model, temozolomide (TMZ) induces SLC7A11 expression by activating NRF2 and ATF4, which partially inhibits ferroptosis (89).
Radiation therapy can induce various types of DNA damage in cells (90) and produce highly reactive OH radicals and other ROS by stimulating oxidase activity (91, 92), making it capable of inducing ferroptosis. The ionizing radiation produced by radiotherapy induces ferroptosis in three ways (93): 1. Production of excess ROS to induce lipid peroxidation. 2. Up-regulation of ACSL4 expression which further promotes PUFA-PL biosynthesis. 3. GSH consumption inhibits the protective effects of GPX4 on ferroptosis. The combination of radiation therapy and ferroptosis inducers such as erastin and sulfasalazine (SAS) for class I FIN, RSL3 and ML162 for class II FIN, and FIN56 for class III FIN increases tumor sensitivity to radiation therapy. Studies have shown that these ferroptosis inducers combined with radiation therapy increase the sensitivity of non-small cell lung cancer cells to radiation therapy and achieve better therapeutic effects than conventional radiotherapy (64). Recent studies have shown that the combination of class I FIN and radiotherapy for SLC7A11 has a better effect, while class II and class III FIN have poor pharmacokinetic effects and are prone to cytotoxicity in actually combined therapy, and the overall therapeutic effect is not as good as that of class I FIN in combination with radiation therapy (92).
At present, based on iron and lipid metabolism, two important factors related to ferroptosis, some effective drugs and methods for reversing tumor resistance have been discovered. Inhibition of ferroptosis-related genes and pathways in tumor cells is thought to be one of the reasons for drug resistance (94, 95).
Regarding iron metabolism, initial studies have shown that TFR is associated with tumor drug resistance, and down-regulation of TFR can reverse tumor drug resistance (96). Ferritin levels in multiple myeloma cells are thought to be directly related to bortezomib resistance, iron supplementation in multiple myeloma cell lines increases cell death, and ferritin reduction allows tumor cells to overcome bortezomib resistance (65). After targeted down-regulation of the ferritin L subunit by microRNA miR-133a in cisplatin and doxorubicin-resistant breast cancer cells, the cells showed increased sensitivity to these drugs (66).
Cells with high expression of GSH and the light chain subunit xCT in system Xc- exhibit resistance to drugs and radiation via lipid metabolism-related pathways (97, 98). The drug resistance of tumor cells is believed to originate from its dependence on GPX4, and inhibition of GPX4 can induce ferroptosis and attenuate the drug resistance of tumor cells (99). FSP1 acts as an oxidoreductase in the plasma membrane to reduce CoQ and inhibit lipid peroxidation and ferroptosis, a pathway thought to be parallel to the GSH/GPX4 axis (16), this pathway also shows the potential of anti-tumor drug resistance (100): in a mouse model established using ferroptosis-resistant H460 lung cancer cell xenografts, the inhibition of FSP1 resulted in significant tumor suppression in both GPX4 KO and GPX4 KO/FSP1 KO groups (16).
The role of ferroptosis in regulating tumor immunity is bidirectional. It not only kills tumors, but also upregulates immune checkpoints and the production of immunosuppressive mediators can promote tumor growth. Interferon-gamma (IFN-γ) released from CD8+ T cells downregulates the expression of SLC3A2 and SLC7A11 in system Xc-, leading to ferroptosis in mouse model tumor cells (69). Another study reported that IFN-γ secreted by CD8+ lymphocytes upregulates PD-L1 in ovarian cancer cells, promoting tumor growth (101). Therefore, it is speculated to imagine that the combination of immune checkpoint inhibitors and ferroptosis therapy may have a notable effect. TAMs are M2 polarized macrophages that secrete vascular endothelial growth factor (VEGF) to promote tumor growth (102). IFN-γ inhibits TAM differentiation in the tumor microenvironment and converts TAMs to M1 macrophages (103, 104), M1 macrophages are more resistant to ferroptosis than M2 macrophages, and studies have shown that nitric oxide synthase (iNOS)/NO regulates macrophage ferroptosis resistance, and decreased levels of iNOS result in decreased ferroptosis resistance in M1 cells, whereas NO donors increase ferroptosis resistance in M2 cells (105). Photodynamic therapy can increase tumor lymphocyte infiltration and recruit immune cells to secrete IFN-γ and enhance ferroptosis (70), which involves complex regulation of the immune microenvironment. The combination of ferroptosis and immunotherapy has broad prospects in the future, but the complexity of the immune microenvironment of tumor cells, the positive and negative effects of ferroptosis in tumor regulation, and the toxicity of ferroptosis-inducing agents on organisms require further in-depth experiments and inquiry.
Ferroptosis-based nanotherapeutics are currently under development, nanomaterials are generally divided into iron-based and non-iron-based nanomaterials. Iron-containing nanoparticulate materials can release iron to the tumor site and trigger the Fenton reaction to generate ROS, which induces ferroptosis (71). Chemotherapeutic drugs or ferroptosis inducers can be loaded into iron-based nanoparticles, such as sorafenib and cisplatin, to work more effectively (72, 73). Non-iron-based nanomaterials are iron-free in the first place, and they can function by carrying ferroptosis-inducing agents, carrying lipids, carrying non-coding RNAs, etc (106). PUFA can be supplemented by nanoparticle drugs, which can modulate lipid peroxidation and induce ferroptosis in tumor cells (74, 75). Furthermore, erastin has poor water solubility and is nephrotoxic, folic acid-labeled erastin-loaded exosomes were used in triple-negative breast cancer cells to avoid nephrotoxicity, and the treatment produced a good therapeutic effect (76). Tumor cell secretion of exosomal PD-L1 suppresses T cell activity, blocks immune checkpoints, and may lead to resistance to therapy (107). Nanounits constructed from exosome inhibitors and ferroptosis inducers can link the immunogenic advantage of exosome inhibition with ferroptosis, this is a new and effective immunotherapy strategy (106).
There is a wide range of metabolic abnormalities associated with ferroptosis. This abnormal regulation of metabolic pathways occurs in many diseases, especially cancer. Researchers have discovered some regulatory substances, pathways, genes, enzymes, and other components involved in ferroptosis. The presence of these target sites makes it possible to achieve disease treatment by regulating ferroptosis. In this review, we summarize and present the link between lipid metabolism and ferroptosis, with a focus on treatments that modulate ferroptosis by regulating lipid metabolism, and in combination with other treatments to achieve tumor therapy. Although the roles of many related pathways and factors have been demonstrated and validated in different cancer models, there are still some issues that have not yet been resolved.
Cancer treatment by regulating ferroptosis has been confirmed and applied in experimental processes and clinical environments. However, there are indeed differences in the effects of in vitro experiments and in vivo treatment. The side effects of some treatment methods also limit their practical application. For different types of cancer, targeted therapy should be selected according to their characteristics. Combining ferroptosis-based treatment with other treatment methods to promote strengths and avoid weaknesses appears to be a better treatment strategy in the future.
XC and GW contributed to conception and design of the manuscript. YS wrote the first draft of the manuscript. YS, ZX and TH modified the manuscript. All authors contributed to the manuscript and approved the submitted version.
The PhD Start-up Fund of Liaoning Province from GW (2021-BS-209, Liaoning Province, 30000 CNY). Dalian Youth Science and Technology Star from GW (2021RQ010, Dalian, 100000CNY); Liaoning Provincial Department of Education(LJKZ0860).
The authors declare that the research was conducted in the absence of any commercial or financial relationships that could be construed as a potential conflict of interest.
All claims expressed in this article are solely those of the authors and do not necessarily represent those of their affiliated organizations, or those of the publisher, the editors and the reviewers. Any product that may be evaluated in this article, or claim that may be made by its manufacturer, is not guaranteed or endorsed by the publisher.
AA, trioxyarachidonic acid; ACC, acetyl-CoA carboxylase; ACSL4, long-chain family member 4; ACSL3, acyl-CoA synthetase long-chain family member 3; AdA, adrenal acid; ALOX, Arachidonate lipoxygenase; AMPK, 5’adenosine monophosphate-activated protein kinase; ATP, adenosine triphosphate; BECN1, beclin 1; CD36, cluster of differentiation 36; CN-A, Cotylenin A; CoQ10, Coenzyme Q10; COX, cyclooxygenase; cPLA2, cytoplasmic phospholipase A2; CYP, Cytochrome P450; DHODH, dihydroorotate dehydrogenase; DPP4, dipeptidyl peptidase 4; ECM, extracellular matrix; ELOVL5, elongase of very-long fatty acid 5; EMP1, epithelial membrane protein 1; EMT, epithelial-mesenchymal transition; FABP: fatty acid binding protein; FADS1: fatty acid desaturase 1; FAT: fatty acid translocase; FATP2: fatty acid transporter 2; Fer-1, ferrostatin-1; FIN, ferroptosis inducers; FPP, farnesyl pyrophosphate FSP1; ferroptosis suppressor protein1; GBM, glioblastoma multiforme; GC, gastric cancer; GPX4, glutathione peroxidase 4; GSH, γ-L-glutamyl-L-cysteinylglycine; GSSG, glutathione disulfide; HIF, hypoxia-inducible factor; HILPDA, hypoxia-inducible, lipid droplet-associated protein; HSPA5, heat shock protein family A member 5 IFN-γ, Interferon gamma; IPP, isopentenyl pyrophosphate; KO, knock out; LD, lipid droplets; LOX, lipoxygenases; LPA, Lysophosphatidic Acid; LPCAT3, lysophosphatidylcholine acyltransferase 3; ML162, molecular libraries 162; MUFA, monounsaturated fatty acids; NADPH, nicotinamide adenine dinucleotide phosphate; NCOA4, nuclear receptor coactivator 4; NOS, nitric oxide synthase NADPH oxidase 4; NRF2, nuclear factor erythroid 2-related factor 2; PE, phosphatidylethanolamine; PDAC, pancreatic ductal adenocarcinoma; PD-L1, Programmed cell death ligand 1; PEITC, phenethyl isothiocyanate; PLINs, perilipins; PMN-MDSC, polymorphonuclear myeloid-derived suppressor cell; POR, cytochrome; PRRs, pattern recognition receptors P450 oxidoreductase; RCD, regulated cell death; ROS: reactive oxygen species; PUFA, polyunsaturated fatty acid; RSL3, Ras selective lethal 3; RSL5, Ras selective lethal 5; SAS, sulfasalazine; SFA, saturated fatty acids; SLC3A2, Solute carrier family 3 member 2; SLC7A11, Solute carrier family 7 member 11; SQS, squalene synthase; STAT3, signal transducer and activator of transcription 3; TAM, Tyro3, Axl, and Mer; TAZ, transcriptional co-activator with PDZ-binding motif; TCA, tricarboxylic acid cycle; TCRs, T cell receptors; TFR1, ferrotransferrin receptor 1; TMZ, temozolomide; TNFR, tumor necrosis factor receptor; TP53, tumor protein 53; TRF, transferrin; YAP, yes-associated protein 1; VEGF, vascular endothelial growth factor.
1. Bannai S, Kitamura E. Transport interaction of l-cystine and l-glutamate in human diploid fibroblasts in culture. J Biol Chem (1980) 255:2372–6. doi: 10.1016/S0021-9258(19)85901-X
2. Dixon SJ, Lemberg KM, Lamprecht MR, Skouta R, Zaitsev EM, Gleason CE, et al. Ferroptosis: an iron-dependent form of nonapoptotic cell death. Cell (2012) 149:1060–72. doi: 10.1016/j.cell.2012.03.042
3. Vanden Berghe T, Linkermann A, Jouan-Lanhouet S, Walczak H, Vandenabeele P. Regulated necrosis: the expanding network of non-apoptotic cell death pathways. Nat Rev Mol Cell Biol (2014) 15:135–47. doi: 10.1038/nrm3737
4. Newton K, Manning G. Necroptosis and inflammation. Annu Rev Biochem (2016) 85:743–63. doi: 10.1146/annurev-biochem-060815-014830
5. Xie Y, Hou W, Song X, Yu Y, Huang J, Sun X, et al. Ferroptosis: process and function. Cell Death Differ (2016) 23:369–79. doi: 10.1038/cdd.2015.158
6. Feng H, Schorpp K, Jin J, Yozwiak CE, Hoffstrom BG, Decker AM, et al. Transferrin receptor is a specific ferroptosis marker. Cell Rep (2020) 30:3411–23.e3417. doi: 10.1016/j.celrep.2020.02.049
7. Sullivan LB, Chandel NS. Mitochondrial reactive oxygen species and cancer. Cancer Metab (2014) 2:17. doi: 10.1186/2049-3002-2-17
8. Wiernicki B, Dubois H, Tyurina YY, Hassannia B, Bayir H, Kagan VE, et al. Excessive phospholipid peroxidation distinguishes ferroptosis from other cell death modes including pyroptosis. Cell Death Dis (2020) 11:922. doi: 10.1038/s41419-020-03118-0
9. Dixon SJ, Stockwell BR. The role of iron and reactive oxygen species in cell death. Nat Chem Biol (2014) 10:9–17. doi: 10.1038/nchembio.1416
10. Stockwell BR, Friedmann Angeli JP, Bayir H, Bush AI, Conrad M, Dixon SJ, et al. Ferroptosis: A regulated cell death nexus linking metabolism, redox biology, and disease. Cell (2017) 171:273–85. doi: 10.1016/j.cell.2017.09.021
11. Gao M, Yi J, Zhu J, Minikes AM, Monian P, Thompson CB, et al. Role of mitochondria in ferroptosis. Mol Cell (2019) 73:354–63.e353. doi: 10.1016/j.molcel.2018.10.042
12. Kagan VE, Mao G, Qu F, Angeli JP, Doll S, Croix CS, et al. Oxidized arachidonic and adrenic PEs navigate cells to ferroptosis. Nat Chem Biol (2017) 13:81–90. doi: 10.1038/nchembio.2238
13. Kuch EM, Vellaramkalayil R, Zhang I, Lehnen D, Brugger B, Sreemmel W, et al. Differentially localized acyl-CoA synthetase 4 isoenzymes mediate the metabolic channeling of fatty acids towards phosphatidylinositol. Biochim Biophys Acta (2014) 1841:227–39. doi: 10.1016/j.bbalip.2013.10.018
14. Dixon SJ, Winter GE, Musavi LS, Lee ED, Snijder B, Rebsamen M, et al. Human haploid cell genetics reveals roles for lipid metabolism genes in nonapoptotic cell death. ACS Chem Biol (2015) 10:1604–9. doi: 10.1021/acschembio.5b00245
15. Kuwata H, Hara S. Role of acyl-CoA synthetase ACSL4 in arachidonic acid metabolism. Prostaglandins Other Lipid Mediat (2019) 144:106363. doi: 10.1016/j.prostaglandins.2019.106363
16. Bersuker K, Hendricks JM, Li Z, Magtanong L, Ford B, Tang PH, et al. The CoQ oxidoreductase FSP1 acts parallel to GPX4 to inhibit ferroptosis. Nature (2019) 575:688–92. doi: 10.1038/s41586-019-1705-2
17. Yang WS, Kim KJ, Gaschler MM, Patel M. Shchepinov MS and Stockwell BR. peroxidation of polyunsaturated fatty acids by lipoxygenases drives ferroptosis. Proc Natl Acad Sci USA (2016) 113:E4966–4975. doi: 10.1073/pnas.1603244113
18. Tang Z, Huang Z, Huang Y, Chen Y, Huang M, Liu H, et al. Ferroptosis: The silver lining of cancer therapy. Front Cell Dev Biol (2021) 9:765859. doi: 10.3389/fcell.2021.765859
19. Shintoku R, Takigawa Y, Yamada K, Kubota C, Yoshimoto Y, Takeuchi T, et al. Lipoxygenase-mediated generation of lipid peroxides enhances ferroptosis induced by erastin and RSL3. Cancer Sci (2017) 108:2187–94. doi: 10.1111/cas.13380
20. Newcomer ME, Brash AR. The structural basis for specificity in lipoxygenase catalysis. Protein Sci (2015) 24:298–309. doi: 10.1002/pro.2626
21. Tang D, Chen X, Kang R, Kroemer G. Ferroptosis: molecular mechanisms and health implications. Cell Res (2021) 31:107–25. doi: 10.1038/s41422-020-00441-1
22. Koppula P, Zhuang L, Gan B. Cystine transporter SLC7A11/xCT in cancer: ferroptosis, nutrient dependency, and cancer therapy. Protein Cell (2021) 12:599–620. doi: 10.1007/s13238-020-00789-5
23. Chu B, Kon N, Chen D, Li T, Liu T, Jiang L, et al. ALOX12 is required for p53-mediated tumour suppression through a distinct ferroptosis pathway. Nat Cell Biol (2019) 21:579–91. doi: 10.1038/s41556-019-0305-6
24. Zou Y, Li H, Graham ET, Deik AA, Eaton JK, Wang W, et al. Cytochrome P450 oxidoreductase contributes to phospholipid peroxidation in ferroptosis. Nat Chem Biol (2020) 16:302–9. doi: 10.1038/s41589-020-0472-6
25. Lee JY, Nam M, Son HY, Hyun K, Jang SY, Kim JW, et al. Polyunsaturated fatty acid biosynthesis pathway determines ferroptosis sensitivity in gastric cancer. Proc Natl Acad Sci USA (2020) 117:32433–42. doi: 10.1073/pnas.2006828117
26. Magtanong L, Ko PJ, To M, Cao JY, Forcina GC, Tarangelo A, et al. Exogenous monounsaturated fatty acids promote a ferroptosis-resistant cell state. Cell Chem Biol (2019) 26:420–32.e429. doi: 10.1016/j.chembiol.2018.11.016
27. Garbarino J, Sturley SL. Saturated with fat: new perspectives on lipotoxicity. Curr Opin Clin Nutr Metab Care (2009) 12:110–6. doi: 10.1097/MCO.0b013e32832182ee
28. Listenberger LL, Han X, Lewis SE, Cases S, Farese RV Jr., Ory DS, et al. Triglyceride accumulation protects against fatty acid-induced lipotoxicity. Proc Natl Acad Sci USA (2003) 100:3077–82. doi: 10.1073/pnas.0630588100
29. Lee JY, Kim WK, Bae KH, Lee SC, Lee EW. Lipid metabolism and ferroptosis. Biol (Basel) (2021) 10(3):184. doi: 10.3390/biology10030184
30. Carracedo A, Cantley LC, Pandolfi PP. Cancer metabolism: fatty acid oxidation in the limelight. Nat Rev Cancer (2013) 13:227–32. doi: 10.1038/nrc3483
31. Vazquez-Martin A, Colomer R, Brunet J, Lupu R, Menendez JA. Overexpression of fatty acid synthase gene activates HER1/HER2 tyrosine kinase receptors in human breast epithelial cells. Cell Prolif (2008) 41:59–85. doi: 10.1111/j.1365-2184.2007.00498.x
32. Rohrig F, Schulze A. The multifaceted roles of fatty acid synthesis in cancer. Nat Rev Cancer (2016) 16:732–49. doi: 10.1038/nrc.2016.89
33. Koizume S, Miyagi Y. Lipid droplets: A key cellular organelle associated with cancer cell survival under normoxia and hypoxia. Int J Mol Sci (2016) 17(9):1430. doi: 10.3390/ijms17091430
34. Rysman E, Brusselmans K, Scheys K, Timmermans L, Derua R, Munck S, et al. De novo lipogenesis protects cancer cells from free radicals and chemotherapeutics by promoting membrane lipid saturation. Cancer Res (2010) 70:8117–26. doi: 10.1158/0008-5472.CAN-09-3871
35. Abumrad N, Coburn C, Ibrahimi A. Membrane proteins implicated in long-chain fatty acid uptake by mammalian cells: CD36, FATP and FABPm. Biochim Biophys Acta (1999) 1441:4–13. doi: 10.1016/s1388-1981(99)00137-7
36. Fernandez LP, de Cedron MG, de Molina AR. Alterations of lipid metabolism in cancer: Implications in prognosis and treatment. Front Oncol (2020) 10:577420. doi: 10.3389/fonc.2020.577420
37. Pascual G, Avgustinova A, Mejetta S, Martin M, Castellanos A, Attolini CS, et al. Targeting metastasis-initiating cells through the fatty acid receptor CD36. Nature (2017) 541:41–5. doi: 10.1038/nature20791
38. Watt MJ, Clark AK, Selth LA, Haynes VR, Lister N, Rebello R, et al. Suppressing fatty acid uptake has therapeutic effects in preclinical models of prostate cancer. Sci Transl Med (2019) 11(478). doi: 10.1126/scitranslmed.aau5758
39. Kuda O, Jenkins CM, Skinner JR, Moon SH, Su X, Gross RW, et al. CD36 protein is involved in store-operated calcium flux, phospholipase A2 activation, and production of prostaglandin E2. J Biol Chem (2011) 286:17785–95. doi: 10.1074/jbc.M111.232975
40. Veglia F, Tyurin VA, Blasi M, De Leo A, Kossenkov AV, Donthireddy L, et al. Fatty acid transport protein 2 reprograms neutrophils in cancer. Nature (2019) 569:73–8. doi: 10.1038/s41586-019-1118-2
41. Zielinski ZA, Pratt DA. Cholesterol autoxidation revisited: Debunking the dogma associated with the most vilified of lipids. J Am Chem Soc (2016) 138:6932–5. doi: 10.1021/jacs.6b03344
42. Simons K, Toomre D. Lipid rafts and signal transduction. Nat Rev Mol Cell Biol (2000) 1:31–9. doi: 10.1038/35036052
43. Clendening JW, Pandyra A, Boutros PC, El Ghamrasni S, Khosravi F, Trentin GA, et al. Dysregulation of the mevalonate pathway promotes transformation. Proc Natl Acad Sci USA (2010) 107:15051–6. doi: 10.1073/pnas.0910258107
44. Santos CR, Schulze A. Lipid metabolism in cancer. FEBS J (2012) 279:2610–23. doi: 10.1111/j.1742-4658.2012.08644.x
45. Bulteau AL, Chavatte L. Update on selenoprotein biosynthesis. Antioxid Redox Signal (2015) 23:775–94. doi: 10.1089/ars.2015.6391
46. Endo A. The discovery and development of HMG-CoA reductase inhibitors. J Lipid Res (1992) 33:1569–82. doi: 10.1016/S0022-2275(20)41379-3
47. Cauley JA, McTiernan A, Rodabough RJ, LaCroix A, Bauer DC, Margolis KL, et al. Statin use and breast cancer: prospective results from the women's health initiative. J Natl Cancer Inst (2006) 98:700–7. doi: 10.1093/jnci/djj188
48. Viswanathan VS, Ryan MJ, Dhruv HD, Gill S, Eichhoff OM, Seashore-Ludlow B, et al. Dependency of a therapy-resistant state of cancer cells on a lipid peroxidase pathway. Nature (2017) 547:453–7. doi: 10.1038/nature23007
49. Shimada K, Skouta R, Kaplan A, Yang WS, Hayano M, Dixon SJ, et al. Global survey of cell death mechanisms reveals metabolic regulation of ferroptosis. Nat Chem Biol (2016) 12:497–503. doi: 10.1038/nchembio.2079
50. Jiang X, Stockwell BR, Conrad M. Ferroptosis: mechanisms, biology and role in disease. Nat Rev Mol Cell Biol (2021) 22:266–82. doi: 10.1038/s41580-020-00324-8
51. Zhao S, Li P, Wu W, Wang Q, Qian B, Li X, et al. Roles of ferroptosis in urologic malignancies. Cancer Cell Int (2021) 21:676. doi: 10.1186/s12935-021-02264-5
52. Yang WH, Chi JT. Hippo pathway effectors YAP/TAZ as novel determinants of ferroptosis. Mol Cell Oncol (2020) 7:1699375. doi: 10.1080/23723556.2019.1699375
53. Yang WH, Ding CC, Sun T, Rupprecht G, Lin CC, Hsu D, et al. The hippo pathway effector TAZ regulates ferroptosis in renal cell carcinoma. Cell Rep (2019) 28:2501–8.e2504. doi: 10.1016/j.celrep.2019.07.107
54. Wu J, Minikes AM, Gao M, Bian H, Li Y, Stockwell BR, et al. Intercellular interaction dictates cancer cell ferroptosis via NF2-YAP signalling. Nature (2019) 572:402–6. doi: 10.1038/s41586-019-1426-6
55. Dietrich C, Hofmann TG. Ferroptosis meets cell-cell contacts. Cells (2021) 10(9):2462. doi: 10.3390/cells10092462
56. Lee H, Zandkarimi F, Zhang Y, Meena JK, Kim J, Zhuang L, et al. Energy-stress-mediated AMPK activation inhibits ferroptosis. Nat Cell Biol (2020) 22:225–34. doi: 10.1038/s41556-020-0461-8
57. Song X, Zhu S, Chen P, Hou W, Wen Q, Liu J, et al. AMPK-mediated BECN1 phosphorylation promotes ferroptosis by directly blocking system xc(-) activity. Curr Biol (2018) 28:2388–99.e2385. doi: 10.1016/j.cub.2018.05.094
58. Miess H, Dankworth B, Gouw AM, Rosenfeldt M, Schmitz W, Jiang M, et al. The glutathione redox system is essential to prevent ferroptosis caused by impaired lipid metabolism in clear cell renal cell carcinoma. Oncogene (2018) 37:5435–50. doi: 10.1038/s41388-018-0315-z
59. Zou Y, Palte MJ, Deik AA, Li H, Eaton JK, Wang W, et al. A GPX4-dependent cancer cell state underlies the clear-cell morphology and confers sensitivity to ferroptosis. Nat Commun (2019) 10:1617. doi: 10.1038/s41467-019-09277-9
60. Qi X, Li Q, Che X, Wang Q, Wu G. Application of regulatory cell death in cancer: Based on targeted therapy and immunotherapy. Front Immunol (2022) 13:837293. doi: 10.3389/fimmu.2022.837293
61. Guo JY, Chen HY, Mathew R, Fan J, Strohecker AM, Karsli-Uzunbas G, et al. Activated ras requires autophagy to maintain oxidative metabolism and tumorigenesis. Genes Dev (2011) 25:460–70. doi: 10.1101/gad.2016311
62. Nystrom S, Antoine DJ, Lundback P, Lock JG, Nita AF, Hogstrand K, et al. TLR activation regulates damage-associated molecular pattern isoforms released during pyroptosis. EMBO J (2013) 32:86–99. doi: 10.1038/emboj.2012.328
63. Gong Y, Fan Z, Luo G, Yang C, Huang Q, Fan K, et al. The role of necroptosis in cancer biology and therapy. Mol Cancer (2019) 18:100. doi: 10.1186/s12943-019-1029-8
64. Lei G, Zhang Y, Koppula P, Liu X, Zhang J, Lin SH, et al. The role of ferroptosis in ionizing radiation-induced cell death and tumor suppression. Cell Res (2020) 30:146–62. doi: 10.1038/s41422-019-0263-3
65. Campanella A, Santambrogio P, Fontana F, Frenquelli M, Cenci S, Marcatti M, et al. Iron increases the susceptibility of multiple myeloma cells to bortezomib. Haematologica (2013) 98:971–9. doi: 10.3324/haematol.2012.074872
66. Chekhun VF, Lukyanova NY, Burlaka CA, Bezdenezhnykh NA, Shpyleva SI, Tryndyak VP, et al. Iron metabolism disturbances in the MCF-7 human breast cancer cells with acquired resistance to doxorubicin and cisplatin. Int J Oncol (2013) 43:1481–6. doi: 10.3892/ijo.2013.2063
67. Bjarnadottir O, Romero Q, Bendahl PO, Jirstrom K, Ryden L, Loman N, et al. Targeting HMG-CoA reductase with statins in a window-of-opportunity breast cancer trial. Breast Cancer Res Treat (2013) 138:499–508. doi: 10.1007/s10549-013-2473-6
68. Sun X, Ou Z, Chen R, Niu X, Chen D, Kang R, et al. Activation of the p62-Keap1-NRF2 pathway protects against ferroptosis in hepatocellular carcinoma cells. Hepatology (2016) 63:173–84. doi: 10.1002/hep.28251
69. Wang W, Green M, Choi JE, Gijon M, Kennedy PD, Johnson JK, et al. CD8(+) T cells regulate tumour ferroptosis during cancer immunotherapy. Nature (2019) 569:270–4. doi: 10.1038/s41586-019-1170-y
70. Xu T, Ma Y, Yuan Q, Hu H, Hu X, Qian Z, et al. Enhanced ferroptosis by oxygen-boosted phototherapy based on a 2-in-1 nanoplatform of ferrous hemoglobin for tumor synergistic therapy. ACS Nano (2020) 14:3414–25. doi: 10.1021/acsnano.9b09426
71. Manivasagan P, Joe A, Han HW, Thambi T, Selvaraj M, Chidambaram K, et al. Recent advances in multifunctional nanomaterials for photothermal-enhanced fenton-based chemodynamic tumor therapy. Mater Today Bio (2022) 13:100197. doi: 10.1016/j.mtbio.2021.100197
72. Zhang L, Gong F, Zhang F, Ma J, Zhang P and Shen J. Targeted therapy for human hepatic carcinoma cells using folate-functionalized polymeric micelles loaded with superparamagnetic iron oxide and sorafenib in vitro. Int J Nanomedicine (2013) 8:1517–24. doi: 10.2147/IJN.S43263
73. Ma P, Xiao H, Yu C, Liu J, Cheng Z, Song H, et al. Enhanced cisplatin chemotherapy by iron oxide nanocarrier-mediated generation of highly toxic reactive oxygen species. Nano Lett (2017) 17:928–37. doi: 10.1021/acs.nanolett.6b04269
74. Yang J, Gong Y, Sontag DP, Corbin I, Minuk GY. Effects of low-density lipoprotein docosahexaenoic acid nanoparticles on cancer stem cells isolated from human hepatoma cell lines. Mol Biol Rep (2018) 45:1023–36. doi: 10.1007/s11033-018-4252-2
75. Zhou Z, Song J, Tian R, Yang Z, Yu G, Lin L, et al. Activatable singlet oxygen generation from lipid hydroperoxide nanoparticles for cancer therapy. Angew Chem Int Ed Engl (2017) 56:6492–6. doi: 10.1002/anie.201701181
76. Yu M, Gai C, Li Z, Ding D, Zheng J, Zhang W, et al. Targeted exosome-encapsulated erastin induced ferroptosis in triple negative breast cancer cells. Cancer Sci (2019) 110:3173–82. doi: 10.1111/cas.14181
77. Manz DH, Blanchette NL, Paul BT, Torti FM, Torti SV. Iron and cancer: recent insights. Ann N Y Acad Sci (2016) 1368:149–61. doi: 10.1111/nyas.13008
78. Yang ND, Tan SH, Ng S, Shi Y, Zhou J, Tan KS, et al. Artesunate induces cell death in human cancer cells via enhancing lysosomal function and lysosomal degradation of ferritin. J Biol Chem (2014) 289:33425–41. doi: 10.1074/jbc.M114.564567
79. Ooko E, Saeed ME, Kadioglu O, Sarvi S, Colak M, Elmasaoudi K, et al. Artemisinin derivatives induce iron-dependent cell death (ferroptosis) in tumor cells. Phytomedicine (2015) 22:1045–54. doi: 10.1016/j.phymed.2015.08.002
80. Kong Z, Liu R, Cheng Y. Artesunate alleviates liver fibrosis by regulating ferroptosis signaling pathway. BioMed Pharmacother (2019) 109:2043–53. doi: 10.1016/j.biopha.2018.11.030
81. Eling N, Reuter L, Hazin J, Hamacher-Brady A, Brady NR. Identification of artesunate as a specific activator of ferroptosis in pancreatic cancer cells. Oncoscience (2015) 2:517–32. doi: 10.18632/oncoscience.160
82. Wang N, Zeng GZ, Yin JL, Bian ZX. Artesunate activates the ATF4-CHOP-CHAC1 pathway and affects ferroptosis in burkitt's lymphoma. Biochem Biophys Res Commun (2019) 519:533–9. doi: 10.1016/j.bbrc.2019.09.023
83. Torii S, Shintoku R, Kubota C, Yaegashi M, Torii R, Sasaki M, et al. An essential role for functional lysosomes in ferroptosis of cancer cells. Biochem J (2016) 473:769–77. doi: 10.1042/BJ20150658
84. Chen Y, Li N, Wang H, Wang N, Peng H, Wang J, et al. Amentoflavone suppresses cell proliferation and induces cell death through triggering autophagy-dependent ferroptosis in human glioma. Life Sci (2020) 247:117425. doi: 10.1016/j.lfs.2020.117425
85. Zhang X, Sui S, Wang L, Li H, Zhang L, Xu S, et al. Inhibition of tumor propellant glutathione peroxidase 4 induces ferroptosis in cancer cells and enhances anticancer effect of cisplatin. J Cell Physiol (2020) 235:3425–37. doi: 10.1002/jcp.29232
86. Roh JL, Kim EH, Jang H, Shin D. Nrf2 inhibition reverses the resistance of cisplatin-resistant head and neck cancer cells to artesunate-induced ferroptosis. Redox Biol (2017) 11:254–62. doi: 10.1016/j.redox.2016.12.010
87. Yang J, Xu J, Zhang B, Tan Z, Meng Q, Hua J, et al. Ferroptosis: At the crossroad of gemcitabine resistance and tumorigenesis in pancreatic cancer. Int J Mol Sci (2021) 22(20):10944. doi: 10.3390/ijms222010944
88. Zhu S, Zhang Q, Sun X, Zeh HJ 3rd, Lotze MT, Kang R, et al. HSPA5 regulates ferroptotic cell death in cancer cells. Cancer Res (2017) 77:2064–77. doi: 10.1158/0008-5472.CAN-16-1979
89. Chen L, Li X, Liu L, Yu B, Xue Y, Liu Y. Erastin sensitizes glioblastoma cells to temozolomide by restraining xCT and cystathionine-gamma-lyase function. Oncol Rep (2015) 33:1465–74. doi: 10.3892/or.2015.3712
90. Baidoo KE, Yong K, Brechbiel MW. Molecular pathways: targeted alpha-particle radiation therapy. Clin Cancer Res (2013) 19:530–7. doi: 10.1158/1078-0432.CCR-12-0298
91. Azzam EI, Jay-Gerin JP, Pain D. Ionizing radiation-induced metabolic oxidative stress and prolonged cell injury. Cancer Lett (2012) 327:48–60. doi: 10.1016/j.canlet.2011.12.012
92. Lei G, Mao C, Yan Y, Zhuang L, Gan B. Ferroptosis, radiotherapy, and combination therapeutic strategies. Protein Cell (2021) 12:836–57. doi: 10.1007/s13238-021-00841-y
93. Ye LF, Chaudhary KR, Zandkarimi F, Harken AD, Kinslow CJ, Upadhyayula PS, et al. Radiation-induced lipid peroxidation triggers ferroptosis and synergizes with ferroptosis inducers. ACS Chem Biol (2020) 15:469–84. doi: 10.1021/acschembio.9b00939
94. Loignon M, Miao W, Hu L, Bier A, Bismar TA, Scrivens PJ, et al. Cul3 overexpression depletes Nrf2 in breast cancer and is associated with sensitivity to carcinogens, to oxidative stress, and to chemotherapy. Mol Cancer Ther (2009) 8:2432–40. doi: 10.1158/1535-7163.MCT-08-1186
95. Sleire L, Skeie BS, Netland IA, Forde HE, Dodoo E, Selheim F, et al. Drug repurposing: sulfasalazine sensitizes gliomas to gamma knife radiosurgery by blocking cystine uptake through system xc-, leading to glutathione depletion. Oncogene (2015) 34:5951–9. doi: 10.1038/onc.2015.60
96. Barabas K, Faulk WP. Transferrin receptors associate with drug resistance in cancer cells. Biochem Biophys Res Commun (1993) 197:702–8. doi: 10.1006/bbrc.1993.2536
97. Britten RA, Green JA, Warenius HM. Cellular glutathione (GSH) and glutathione s-transferase (GST) activity in human ovarian tumor biopsies following exposure to alkylating agents. Int J Radiat Oncol Biol Phys (1992) 24:527–31. doi: 10.1016/0360-3016(92)91069-y
98. Habib E, Linher-Melville K, Lin HX, Singh G. Expression of xCT and activity of system xc(-) are regulated by NRF2 in human breast cancer cells in response to oxidative stress. Redox Biol (2015) 5:33–42. doi: 10.1016/j.redox.2015.03.003
99. Hangauer MJ, Viswanathan VS, Ryan MJ, Bole D, Eaton JK, Matov A, et al. Drug-tolerant persister cancer cells are vulnerable to GPX4 inhibition. Nature (2017) 551:247–50. doi: 10.1038/nature24297
100. Doll S, Freitas FP, Shah R, Aldrovandi M, da Silva MC, Ingold I, et al. FSP1 is a glutathione-independent ferroptosis suppressor. Nature (2019) 575:693–8. doi: 10.1038/s41586-019-1707-0
101. Abiko K, Matsumura N, Hamanishi J, Horikawa N, Murakami R, Yamaguchi K, et al. IFN-gamma from lymphocytes induces PD-L1 expression and promotes progression of ovarian cancer. Br J Cancer (2015) 112:1501–9. doi: 10.1038/bjc.2015.101
102. Wang FQ, Chen G, Zhu JY, Zhang W, Ren JG, Liu H, et al. M2-polarised macrophages in infantile haemangiomas: correlation with promoted angiogenesis. J Clin Pathol (2013) 66:1058–64. doi: 10.1136/jclinpath-2012-201286
103. Jeong SK, Yang K, Park YS, Choi YJ, Oh SJ, Lee CW, et al. Interferon gamma induced by resveratrol analog, HS-1793, reverses the properties of tumor associated macrophages. Int Immunopharmacol (2014) 22:303–10. doi: 10.1016/j.intimp.2014.07.004
104. Ni L, Lu J. Interferon gamma in cancer immunotherapy. Cancer Med (2018) 7:4509–16. doi: 10.1002/cam4.1700
105. Wu Y, Yu C, Luo M, Cen C, Qiu J, Zhang S, et al. Ferroptosis in cancer treatment: Another way to Rome. Front Oncol (2020) 10:571127. doi: 10.3389/fonc.2020.571127
106. Luo L, Wang H, Tian W, Li X, Zhu Z, Huang R, et al. Targeting ferroptosis-based cancer therapy using nanomaterials: strategies and applications. Theranostics (2021) 11:9937–52. doi: 10.7150/thno.65480
Keywords: lipid metabolism, ferroptosis, tumor, tumor therapy, lipid peroxidation
Citation: Sun Y, Xue Z, Huang T, Che X and Wu G (2022) Lipid metabolism in ferroptosis and ferroptosis-based cancer therapy. Front. Oncol. 12:941618. doi: 10.3389/fonc.2022.941618
Received: 11 May 2022; Accepted: 08 July 2022;
Published: 01 August 2022.
Edited by:
Cai Yu, Fudan University, ChinaReviewed by:
Hao Liu, Eli Lilly, United StatesCopyright © 2022 Sun, Xue, Huang, Che and Wu. This is an open-access article distributed under the terms of the Creative Commons Attribution License (CC BY). The use, distribution or reproduction in other forums is permitted, provided the original author(s) and the copyright owner(s) are credited and that the original publication in this journal is cited, in accordance with accepted academic practice. No use, distribution or reproduction is permitted which does not comply with these terms.
*Correspondence: Guangzhen Wu, d3VndWFuZzA2MTNAaG90bWFpbC5jb20=; Xiangyu Che, ZGFsaWFuY2hleGlhbmd5dUAxNjMuY29t; Tao Huang, NDEzMzg1OEBxcS5jb20=
†These authors share first authorship
Disclaimer: All claims expressed in this article are solely those of the authors and do not necessarily represent those of their affiliated organizations, or those of the publisher, the editors and the reviewers. Any product that may be evaluated in this article or claim that may be made by its manufacturer is not guaranteed or endorsed by the publisher.
Research integrity at Frontiers
Learn more about the work of our research integrity team to safeguard the quality of each article we publish.