- 1Department of Pharmacy, Shaoxing People’s Hospital, Shaoxing Hospital, Zhejiang University School of Medicine, Shaoxing, China
- 2Department of Orthopedics, Zhoushan Guanghua Hospital, Zhoushan, China
- 3College of Medicine, Shaoxing University, Shaoxing, China
- 4Department of Hepatobiliary Surgery, Shanghai Oriental Hepatobiliary Hospital, Shanghai, China
- 5Department of Gastroenterology, Zhoushan Hospital of Traditional Chinese Medicine Affiliated to Zhejiang Chinese Medical University, Zhoushan, China
- 6Medical Research Center, Zhoushan Hospital of Traditional Chinese Medicine Affiliated to Zhejiang Chinese Medical University, Zhoushan, China
New evidence suggests that the clinical success of chemotherapy is not merely due to tumor cell toxicity but also arises from the restoration of immunosurveillance, which has been immensely neglected in previous preclinical and clinical researches. There is an urgent need for novel insights into molecular mechanisms and regimens that uplift the efficacy of immunotherapy since only a minority of cancer patients are responsive to immune checkpoint inhibitors (ICIs). Recent findings on combination therapy of chemotherapy and ICIs have shown promising results. This strategy increases tumor recognition and elimination by the host immune system while reducing immunosuppression by the tumor microenvironment. Currently, several preclinical studies are investigating molecular mechanisms that give rise to the immunomodulation by chemotherapeutic agents and exploit them in combination therapy with ICIs in order to achieve a synergistic clinical activity. In this review, we summarize studies that exhibit the capacity of conventional chemotherapeutics to elicit anti-tumor immune responses, thereby facilitating anti-tumor activities of the ICIs. In conclusion, combining chemotherapeutics with ICIs appears to be a promising approach for improving cancer treatment outcomes.
Introduction
The link between host immunity and cancer development was unclear until recently. Now it is well documented that cancer development is associated with host immunity. Immunosurveillance is a monitoring process by which immune cells detect and destroy malignant cells (1). Over time, tumor cells evolve to escape immunosurveillance, resulting in tumor establishment (Figure 1). Following tumor establishment, cancer cells employ more immunosuppressive mechanisms to escape anti-tumor immune responses (1). Major mechanisms that these cells use for immunosuppression are 1. Binding to effector cells using inhibitory receptors called immune-checkpoints (ICs) such as cytotoxic T-lymphocyte-associated protein 4 (CTLA-4) and programmed cell death protein-1 (PD-1) (Figure 2) 2. Secretion of anti-inflammatory cytokines like IL-10 and 3. Suppression of effector cells upon depletion of essential metabolic substrates like tryptophan and arginine (2, 3). So the accumulation of immunosuppressive cells and increased levels of immunosuppressive molecules such as PD-1 ligand (PD-L1) and indoleamine 2,3-dioxygenase 1 (IDO1) are directly associated with poor prognosis and unfavorable disease outcomes in patients with cancer (4–6).
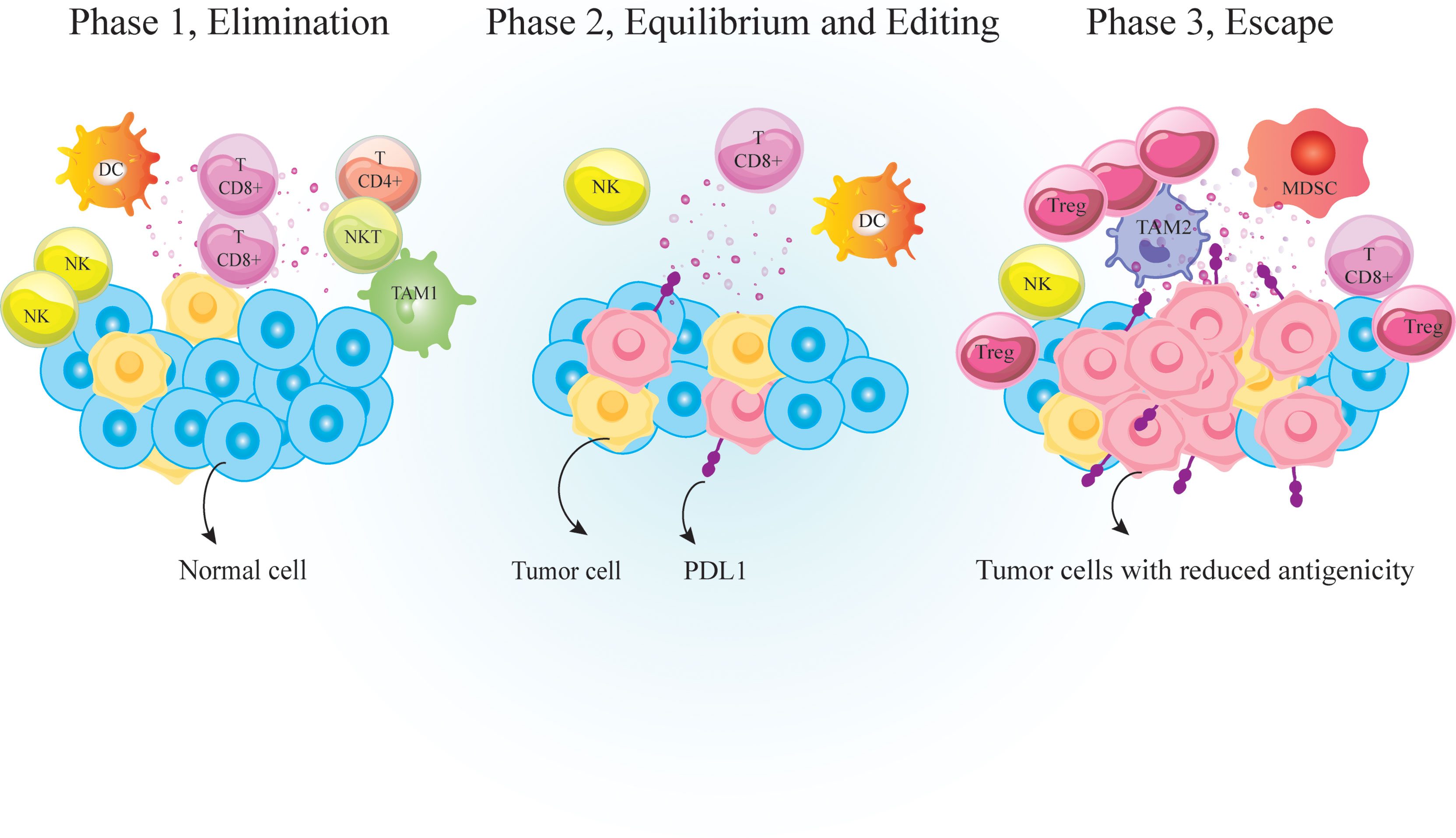
Figure 1 Immunosurveilance and cancer immunoediting. Immunosurveillance is a monitoring process by which cells of the immune system detect and destroy virally infected or malignant cells. It is consisted of three major phases; 1. Elimination phase that eradicate neoplastically transformed cells; 2. Equilibrium phase that occurs upon incomplete eradication of malignant cells so a temporary state of equilibrium develops between the growing tumor and the immune cells; And 3. Escape phase during which variants of tumor cells resist, avoid or suppress the anti-tumor activity of the host immune cells to the point that the immune system is no longer able to restrain tumor growth. PD-1, Programmed cell death 1; TCD4+, helper T cell; TCD8+, cytotoxic T lymphocyte, DC, dendritic cells; MDSC, myeloid-derived suppressor cell; NK, natural killer; TAM, tumor associated macrophages; Treg, regulatory T-cell.
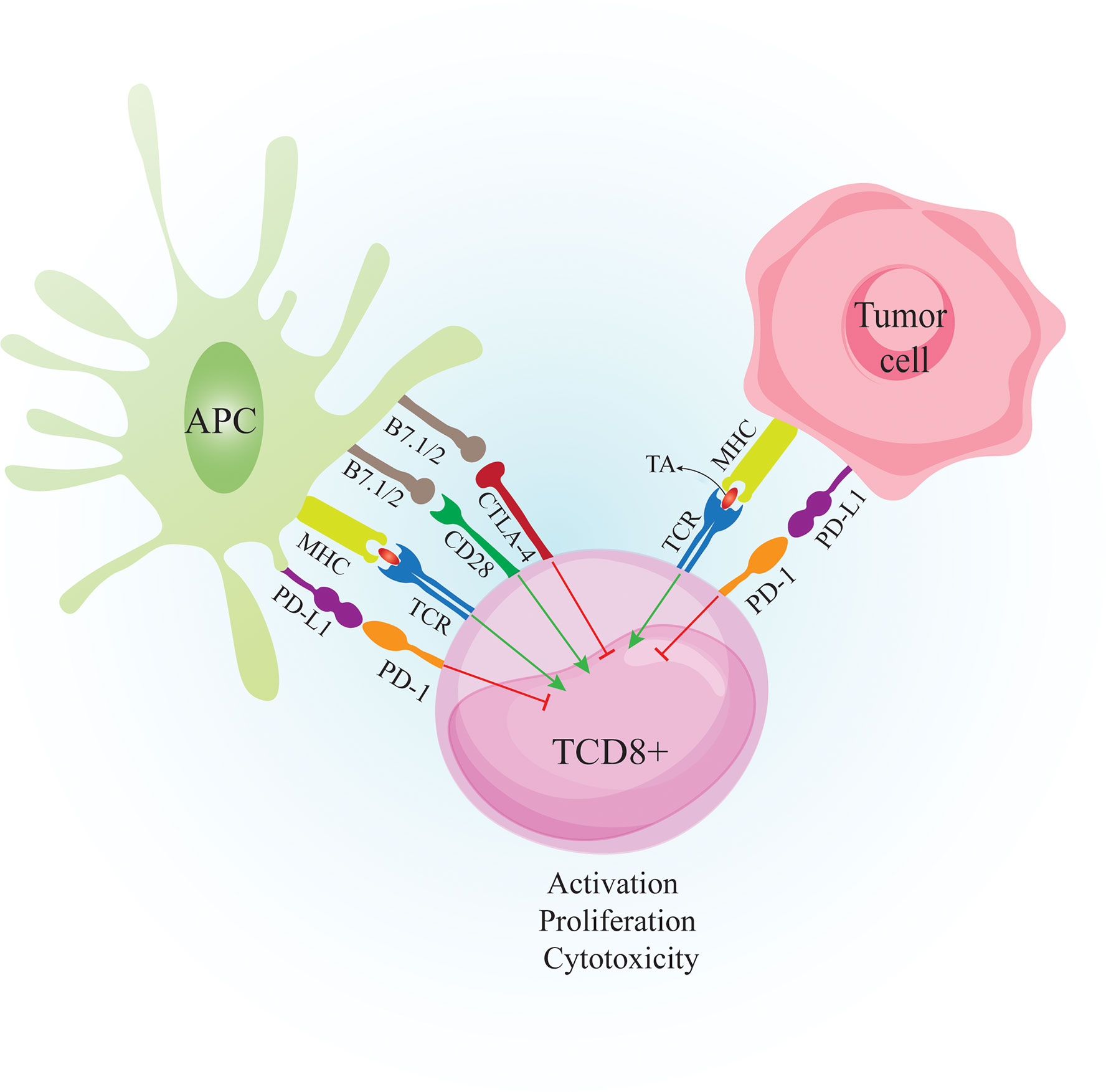
Figure 2 Crosstalk between CTL, APC and Tumor cell. Tumor cells are recognized by the immune system when tumor peptides are presented via APC to CD8+ T cells. APC also provide costimulatory molecules such as B7.1/2 to bind CD28 on T cells. The primed CD8+ T cells can recognize tumor antigens and destroy tumor cells by secretion of perforin and granzyme B However, tumor cells express inhibitory molecules such as PD-L1 to bind PD-1 on activated T cells to suppress anti-tumor responses. Tumor cells also induce PD-L1 expression on APCs to further suppress the immune responses. CTLA-4 is expressed on T cells following activation. Binding CTLA-4 on T cells to B7.1/2 on APCs causes inhibition of T cell activity. APC: antigen presenting cell. CTL, cytotoxic T lymphocyte; CD28, cluster of differentiation 2; CTLA-4, cytotoxic T-lymphocyte-associated protein 4; MHC-I, major histocompatibility complex class I; TA, tumor antigen; TCR, T cell receptor; PD-1, Programmed cell death 1; PD-L1, Programmed cell death-ligand 1;.
Accordingly, immunotherapeutic approaches such as blocking the ICs and reducing immunosuppressive molecules and cells could enhance the anti-tumor immune responses (7). IC inhibitors (ICIs) such as anti-PD-1 or anti-PDL1 antibodies have shown promising results in the treatment of various cancers like unresectable or metastatic melanoma, renal cancer, metastatic non-small cell lung cancer (NSCLC), and more recently, Hodgkin’s lymphoma and urothelial carcinoma (8–14).
Nevertheless, a significant proportion of patients with breast or prostate cancer show resistance to ICIs, mostly due to the immunosuppressive tumor microenvironment (TME) or the lack of immune checkpoints expression by tumor cells (15–18). Moreover, patients who initially have responded well to ICIs therapy can develop resistance as the disease progresses after a period of time. Therefore, it is essential to prevent resistance phenomena and enhance the anti-tumor activity of monoclonal antibody therapy by using combination therapies. Some studies on combination therapies are ongoing (19–26). Despite the primary belief that conventional chemotherapy is merely immunosuppressive, recent findings unveiled the immunostimulatory properties of chemotherapy. Utilizing chemotherapy leads to the release of antigens through cytotoxic cell death activity, stimulating immune responses and improving the activity of PD-1/PD-L1 blocking agents. In addition, chemotherapy may positively impact the leukocyte composition of infiltrated cells (26–29). Several ongoing clinical trials combine ICIs monoclonal antibodies with various chemotherapies (25–28, 30). This review aimed to discuss the different immunological effects of combining ICIs and chemotherapy.
ICIs resistance mechanisms and strategies to avoid them
Resistance to immunotherapy drugs can be primary, as seen in non-responders, or acquired, which occurs after some time in patients. Also, resistance can emerge intrinsically or extrinsically. The former happens when tumor cells directly interfere with processes involved in immune recognition, gene expression, and cell signaling. Extrinsic resistance happens externally to tumor cells via T-cell activation processes (31).
Several factors in response or resistance to ICIs therapy are related to tumor immunogenicity, TME, antigen presentation, and classic oncologic pathways.
The mechanisms underlying resistance to ICIs are not fully elucidated. However, defects in neoantigen and antigen presentation, mutations in inflammatory signaling pathways, Overexpression of other ICs, and overcoming immunosuppressive mechanisms in the TME can lead to ICI resistance (32).
The defect in antigen presentation is one mechanism of tumor cells to evade immune responses (33). It can stem from DC dysfunction, deficiency in MHC machinery, and decreased T-cell priming. Mutations in B2M (β2-microglobulin gene) lead to MHC-I loss (34). Some acquired ICI-resistant patients showed loss of B2M (35–37). However, not all mutations of B2M cause ICI resistance (32), as it has been reported that some B2M-mutated CRC patients were still ICI sensitive (38).
Co-expression of inhibitory receptors such as Lymphocyte-activation gene (LAG-3), T-cell immunoglobulin and mucin domain-3 (TIM-3), T cell immunoreceptor with Ig and ITIM domains (TIGIT), V-domain Ig suppressor of T cell activation (VISTA) (39), and B-/T-lymphocytes attenuator (BTLA), alteration in the balance of tumor-infiltrating lymphocytes (TILs) in favor of myeloid-derived suppressor cells (MDSCs) and regulatory T cells (Tregs), and increased production of indoleamine 2,3-dioxygenase (IDO) and adenosine are another circumstances that lead to ICI resistance (14, 31).
Neoantigens-specific T cells are principal anti-tumor effector cells that could express ICs (40, 41). Accordingly, loss of neoantigen expression by tumor cells may lead to immune evasion and ICI resistance (42, 43). Neoantigen-specific IC expressing T cell clones might be eliminated by the selective pressure that exists in the TME, resulting in the outgrowth of IC negative clones (44).
Other prominent factors that cause resistance to ICIs are mutations in JAK1/2, IFNGR1/2, and IRF1 (45), aberrant WNT/β-catenin signaling, and loss of tumor suppressor genes (46). Such signalings are required to express ICs on cells (47). Hence, defects in signaling can cause loss of ICs and ICI resistance. However, patients carrying heterozygous mutations still have active signaling pathways and IC expression, making them ICI sensitive (48).
The rational for ICIs and chemotherapy combination therapy
There are different therapeutic strategies to bypass this resistance to ICIs. Since T lymphocytes are the most effective and crucial components in immune defense against tumor development, any strategy that increases tumor immunogenicity and T cell priming that subsequently activates this type of cell can help defeat tumor resistance. Chemotherapy consists of a large group of molecules that target and destroy proliferating cells. Chemotherapy mainly affects growing cancer cells but may also affect normal proliferating immune cells causing myelosuppression and leukocytopenia. Hence, chemotherapy was long thought to be solely an immunosuppressive treatment modality. However, according to recent studies, there are forms of chemotherapy that demonstrate immune-stimulatory effects (49, 50). Chemotherapy increases tumor response to ICIs therapy by increasing the release of tumor antigens upon cancer cell death, resulting in enhanced T cell priming (29). In addition, chemotherapy contributes to the depletion of the MDSC and Treg population in the tumor site (27). Also, radiation therapy positively affects the ICIs treatment outcome in a similar manner. It increases antigen presentation and promotes an inflamed TME (51). Other strategies available for this purpose that have shown enhanced tumor regression are combining ICI with targeted therapy, cytokine/chemokine inhibitors, and immune-stimulatory agent therapy (31).
Given the complex network of signaling and regulation of immune responses against the tumor, it seems impossible to define a specific immunologic biomarker in order to select patients who would benefit the most from this approach. One of the strategies that help in choosing ICIs therapy alone or in combination with chemotherapy in a cancer patient is related to the immune status of TME and their “hot” or “cold” immunologic contexts. Immunogenic or hot TME comprises infiltrating T cells, inflammatory cytokines, and PD-L1. Contrarily, those lacking these features are called non-immunogenic or cold TMEs (52–55). Patients displaying hot TMEs are excellent candidates for receiving ICI therapy alone, while patients whose tumors are non-immunologic would benefit from the synergistic effect of combination therapies (54). In these patients, chemotherapy boosts immune responses against tumor cells by increasing the immunogenicity of the growing tumor, while IC blockade prolongs this effect by providing a long-lasting immune response resulting in fast tumor regression (56).
Immunomodulatory effects of chemotherapy
Conventional chemotherapy may exert anti-tumor immune responses by “on-target” effect, which directly increases immunogenicity of targeted cancer cells, or through “off-target” effect on different immune cell populations, leading to alteration of the whole-body physiology favoring anti-cancer immunosurveillance. Common action mechanisms of chemotherapeutic agents are 1. Marked lymphodepletion or so-called ‘reset’ of the immune system (with major adverse effects) and near-to-complete reconstitution of the host’s immunological repertoire (57), 2. Decrease immunosuppressive cells, including M2-like tumor-associated macrophages (TAMs), MDSCs, and Tregs, by providing an inflammatory condition (17, 58, 59), or 3. Activation of effector cells such as cytotoxic T cells (CTLs) (60), DCs (61),, and M1-like TAMS (62) (Figure 3).
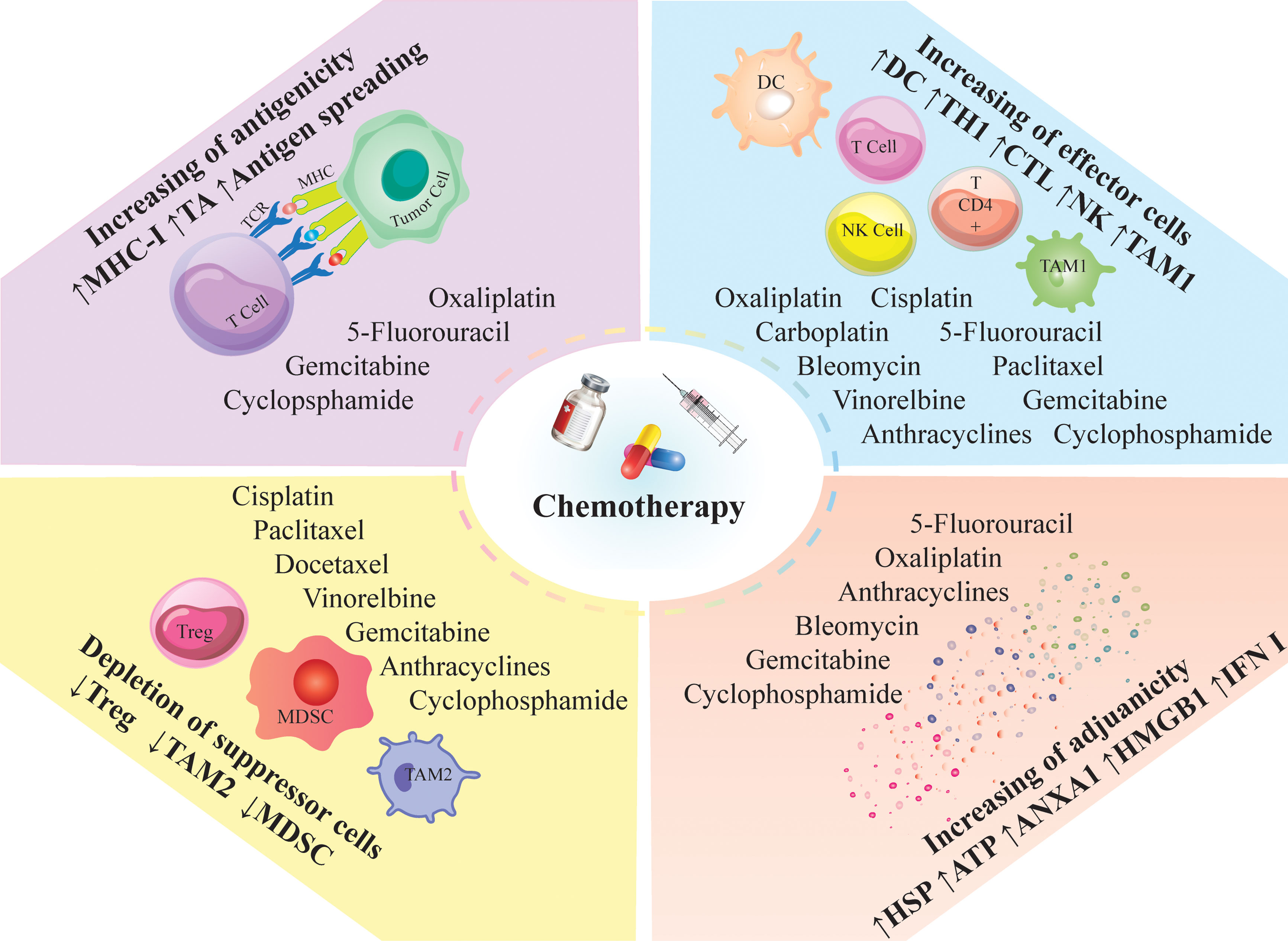
Figure 3 Immunomodulatory effects of chemotherapy; Chemotherapeutic agents can modulate the anti-tumor immune responses; They induce immunologic death and trigger the release of tumor antigens and neoantigens via antigen spreading; Besides antigen spreading, they can enhance the release of DAMPs and inflammatory mediators; The induced inflammation can recruit proinflammatory cells into the tumor milieu and decrease the immunosuppressor cells; Such inflammatory conditions; ANXA1, annexin A1; CTL, cytotoxic T lymphocyte; DC, dendritic cells; HMGB1, high mobility group box 1; HSP, heat shock protein; IFN, interferon; MDSC, myeloid derived suppressor cell; MHC-I, major histocompatibility complex class I; NK, natural killer; TAM, tumor associated macrophages; TA, tumor antigen; TCR, T cell receptor; Th, T helper cell; Treg, regulatory T-cell; DAMPs; Damage-associated molecular patterns.
An efficient anti-tumor immune response relies on the combination of two fundamental factors. First, tumor cells must express antigens for T cell recognition and activation (immunogenicity). Then, cancer cells should recruit adjuvant-like danger signals such as damage-associated molecular patterns (DAMPs) or pathogen-associated molecular patterns (PAMPs) to boost the immunogenicity (adjuvanticity) (63, 64). Conventional chemotherapies improve the immunogenicity and adjuvanticity of cancer cells by inducing cellular death and stress (Figure 3) (65).
Immunogenic cell death (ICD), a form of regulated cell death, occurs as a part of the so-called “integrated stress response” and emerges from the activation of unsuccessful cytoprotective pathways such as phosphorylation of eukaryotic translation initiation factor 2 subunit alpha (EIF2S1, also known as eIF2α) and autophagy. Activation of autophagy during ICD leads to the lysosomal secretion of ATP, which promotes purinergic receptors to stimulate the recruitment of DC precursors to the tumor site and accelerates inflammatory reactions after inflammasome activation (66–68). On the other hand, endoplasmic reticulum (ER) stress favors the translocation of ER chaperones such as heat shock protein family A (HSP70) member 1A (HSPA1A, also known as HSP70) and calreticulin to the cell surface. Exposure of these chaperones on the cell surface is a signal for the phagocytic uptake of the tumor cell by immature DCs (69). Accumulation of nuclear or mitochondrial DNA of dying cancer cells in extracellular spaces or the cytosol as a result of responding to chemotherapy elicits immune signaling via Toll-like receptor 9 (TLR9), TLR3, and GMP-AMP synthase (CGAS) that eventually results in the production of type I interferons (IFNs) (70, 71). Also, dying tumor cells release a number of nuclear and cytoplasmic proteins like high mobility group box 1 (HMGB1) and annexin A1 (ANXA1). HMGB1 facilitates the interaction of DCs with dead cell debris via binding to formyl peptide receptor 1 (FPR1), while ANXA1 promotes DC maturation upon binding to TLR4 (72). These observations represent the importance of adjuvanticity for chemotherapy to exert clinically efficient anti-tumor immunity. Preclinical studies also support this notion by demonstrating the positive prognostic value of the increased DAMP expression and ICD-associated stress responses in the host (73).
However, little is known about the ability of chemotherapy to increase tumor antigenicity. Major histocompatibility complex (MHC) class I upregulation driven by IFN signaling, accumulation of genetic and/or epigenetic defects, and transcriptomic perturbations like modifications in the expression of non-coding RNA are possible mechanisms by which chemotherapy improves antigenicity (74, 75). Supporting this notion, gemcitabine, a chemotherapeutic agent, has been shown to activate stress response pathways that ultimately result in the upregulation of β2 microglobulin (structural component of MHC I) (76).
Mechanisms of action of chemotherapeutic agents
Alkylating and platinum based anti-cancer drugs
Alkylating agents can stop protein synthesis by inhibiting the transcription of DNA into RNA. Anti-tumor alkylating agents covalently bind to the double strand of DNA and form platinum–DNA crosslinks. These inter- and intra-strand crosslinks disrupt DNA replication and transcription and ultimately induce apoptosis in cancer cells. In addition, platinum agents can exert their anti-tumor efficacy by modulating the host’s immune system. Cisplatin, oxaliplatin, and carboplatin are renowned platinum derivatives. Moreover, cisplatin is the most studied derivative regarding immunomodulatory effects (77–79). Oxaliplatin and cisplatin increase type I IFNs and IFN-γ signaling, leading to the upregulation of co-inhibitory ligands such as PD-L1 (80). Similarly, it has been observed that chemotherapy consisting of platinum-containing agents and 5-fluorouracil (5-FU) induces PD-L1 expression as a consequence of enhanced CD8+ CTL infiltration (81). Also, the same effect has been reported in patients with esophageal squamous cell carcinomas who received cisplatin plus 5-FU (82).
Platinum agents decrease STAT6 signaling through dephosphorylation of STAT6. Loss of STAT6 phosphorylation leads to the downregulation of PD-L2 on tumor cells and DCs and, in turn, increases tumor recognition by T lymphocytes (83, 84). Also, platinum agents can stimulate CTL-mediated attack (83). A combination of cisplatin and vinorelbine in patients with NSCLC made tumor cells more responsive to MHC-guided perforin and granzyme-B mediated CTL attacks, mainly associated with the MHC I upregulation (85). Besides this mechanism of action, it was reported that combining cisplatin with vinorelbine may increase the ratio of effector CD4+ to Tregs (86). However, cisplatin is unable to stimulate calreticulin release from ER, thereby not able to completely trigger ICD (87). In the same context, carboplatin is inefficient in inducing ICD, resulting in the partial release of HMGB1 and calreticulin. Conversely, platinum compounds play an important role in recruiting and activating DCs to tumor sites by inducing the release of ATP from dying cells (83). However, further investigations are required to elucidate the exact effect of various chemotherapeutic compounds on the immune response.
Cyclophosphamide was the first chemotherapeutic agent reported to have immunomodulatory effects, if used at a certain dosage, by selective depletion of the Treg population. This observation initiated the investigation of the probable immunomodulatory effects of other agents (88). Furthermore, cyclophosphamide is able to trigger DCs homeostasis. Supporting this notion, mouse models treated with intraperitoneal cyclophosphamide showed tumor cell death with tumor infiltration and engulfment of apoptotic tumor cells by DCs, and ultimately cross-priming of CD8+ T cells by DCs (89). Other in vivo experiments have demonstrated that a single dose of cyclophosphamide converts the immune profile from TH2 to TH1 cytokines, increasing interleukin (IL)-2 and IFN-γ production and decreasing IL-10 and TGF-β production (90–92). Mouse Tregs appear to be less sensitive to cytotoxic effects of cyclophosphamide compared with human Tregs. Nevertheless, systemic cyclophosphamide mediates IL-12 and interferon regulatory factor 1 (IRF1)-dependent response resulting in increased TH1 polarization and Treg depletion (93). It is unclear whether a similar pathway is operational in humans. Another mechanism of anti-tumor efficacy of cyclophosphamide is inducing ICD (94).
The combination of cyclophosphamide and oxaliplatin in NSCLC patients significantly increased nuclear HMGB1 staining in tumor nodules; Also, the oxaliplatin cyclophosphamide combination was able to control tumor growth (95). It is important to mention that the dosage of cyclophosphamide used in combination therapies is crucial: high dosages may induce myelosuppression, while metronomic low dosages improve the immune system (96, 97). Low-dose cyclophosphamide has been observed to deplete both circulating and tumor-infiltrating Treg cells through ICD-independent mechanisms (98, 99). Of note, cyclophosphamide depletes both Treg cells and TH1 cells at first; however, TH1 cells are able to recover faster than Tregs after discontinuation of the treatment (100).
Topoisomerase inhibitors
Topoisomerase I and II are normal enzymes of mammalian cells that cut and repair DNA strands in DNA replication and cell division processes. Their activity significantly increases in rapidly dividing cancer cells. Topoisomerases represent an appropriate non-selective anti-cancer drug. Camptothecin is an active topoisomerase poison, and its derivatives, irinotecan and topotecan, serve as topoisomerase I inhibitors (101). According to preclinical findings, camptothecin derivatives enhance tumor recognition by T cells. Also, topoisomerase I inhibitors increase the expression of TP53INP1 and Melan-A/MART-1. Upon overexpression of these antigens, tumor recognition by T lymphocytes is increased, and consequently, T cell-mediated killing of cancer cells is improved (102, 103). Another study demonstrated upregulation of HMGB1, HSP70, and other DAMPs after treatment with irinotecan (104). MHC I and Fas expression are upregulated in tumor cells affected by topotecan treatment, making them more sensitive to killing by effector T cells (105, 106).
Anthracyclines are inhibitors of topoisomerase II and have shown to be effective in the selective depletion of immunosuppressive cells. Doxorubicin, epirubicin, and idarubicin are derivates of anthracyclines with immunosuppressive abilities (82). Clinical results in patients with breast cancer demonstrated that intraperitoneal administration of 5mg/kg doxorubicin leads to in vivo reduction of MDSCs and subsequent increase of CD4+ and CD8+ T cells, as well as other effector elements including IFN-γ, granzyme B and perforin (107). Besides their immunosuppressive capabilities, anthracycline has the capacity to elicit ICD by increasing the expression of DMAPs, including HMGB1, HSP70, and calreticulin (72, 108). However, inducing ICD requires higher doses than cytotoxic dose (109).
Other immunomodulatory effects of anthracyclines have been studied, and it appears that they can induce immune responses in a similar manner that viral pathogens initiate immune responses (71). Doxorubicin acts by damaging DNA and cell membrane by generating free oxygen radicals. It demonstrated limited clinical efficacy in NSCLC treatment mainly due to the rapid upregulation of NF-KB signaling in response to therapy and acquiring resistance in the host immune system (110). Doxorubicin can also exert anti-tumor activity by upregulating STAT1 signaling (78, 87). Epirubicin disrupts Treg-mediated suppression of CD8+ T cells by blocking the interaction between NF-KB subunit p65 and Foxp3 in vitro (111). A few studies have reported potential negative outcomes of anthracyclines. Daunorubicin has been observed to induce cell death in both resting and activated peripheral blood cells, which is considered a negative factor for ICI combination (112, 113).
Antimitotic agents
Anti-microtubule agents exert anti-neoplastic effects by disrupting microtubules. The most widely used anti-microtubule agents are Taxanes: Docetaxel and paclitaxel (114). Taxanes are generally known for depleting neutrophils and lymphocytes, especially neutrophils (115). Increased neutrophil to lymphocyte ratio favors ICIs treatment (116). CD3+, CD4+, CD8+, CD56+, and CD45RO+ cells are lymphocytes affected by taxane administration (117), some of which are related to ICI responses (118).
Taxanes improve the upregulation of proinflammatory cytokines such as IL-2, IL-6, IFN-γ, and GM-CSF after six cycles of standard treatment (119). The effect of taxane agents on the cytotoxicity of T cells is controversial. While some studies report impaired T cell-mediated cytotoxicity upon treatment with paclitaxel (120), others have shown increased NK and lymphokine-activated killer cell activity (119, 121). Moreover, both agents have immunomodulatory effects on specific immune cell subsets (79). At certain dosages, paclitaxel and docetaxel induce DC maturation by increasing the expression of MHC II (122). Also, it reduces the Treg population through upregulation of Fas receptors and increasing apoptosis while improving CD4+ and CD8+ T cells (123). Similarly, docetaxel exerts its anti-tumor activity by selective depletion of Tregs and reduction of MDSCs at the tumor site via activation of STAT3 signaling (56, 124).
Administration of a chemotherapeutic cocktail containing doxorubicin and cyclophosphamide contributes to the repolarization of the TME compartment from an M2-like phenotype to an M1-like phenotype (125). A similar finding was observed upon administrating paclitaxel which is reported to act as a TLR4 agonist (62). According to preclinical data, paclitaxel supports the upregulation of co-inhibitory ligands like PD-L1 by tumor-infiltrating myeloid cells or malignant cells as a consequence of type I IFN or IFN-γ signaling (126, 127). Therefore, upregulation of PD-L1 upon chemotherapy is used as a biomarker to classify and select patients who would benefit from anti-PD-1 or anti-PD-L1 antibodies alone or in combination with chemotherapy (128).
One of the concerns regarding the administration of chemotherapeutic agents is adverse effects like neuropathic pain and neuroinflammation. According to recent preclinical findings, gut microbiota can modulate neuroinflammation induced by taxanes (129, 130). Thereby, modulating gut microbiota by using prebiotics or probiotics may enhance the efficacy of chemotherapy and help manage the toxicities of these agents (131).
Antimetabolites
Antimetabolites are chemotherapeutic agents with ‘cytotoxic’ effects on cells by mimicking the molecules, such as genetic materials and enzymes that tumor cells need for growth. Thereby, tumor cells uptake and use these antimetabolites instead of normal cell materials (132).
Gemcitabine is an antimetabolite and pyrimidine analog that disrupts RNA and DNA synthesis (133). It modulates anti-cancer responses by selectively suppressing MDSCs (134) while increasing the expression of tumor antigens and making tumor cells more recognizable to the immune system. In a trial on patients with pancreatic cancer, the standard dosage of gemcitabine resulted in Treg depletion that lasted for two weeks (135). 5FU is extensively used as an anti-cancer drug. Since 1957, it has been important in treating cancers like colon cancer and breast cancer (128, 129). 5-FU has a structure similar to the pyrimidine molecules of DNA and RNA and is a uracil analog. It interferes with nucleoside metabolism and can be incorporated into RNA and DNA, leading to cytotoxicity and cell death (130). A standard dosage of 5FU exerts stimulatory effects by supporting antigen uptake of DCs. In an in vitro study, a gastric cancer cell line pre-treated with 5FU showed higher IL-12 production than the control. This subsequently increased the cytotoxicity of T cells generated by DCs compared to the control (136). Both gemcitabine and 5FU activate the NLRP3 inflammasome in MDSCs, leading to IL-1β secretion and ultimately immunosuppression by TH17 (137). In CT26 tumor-bearing mice, 5FU eliminated MDSCs without significantly affecting T, B, or NK cells (138).
Methotrexate is a folate derivative that can inhibit a number of enzymes involved in nucleotide synthesis culminating in the suppression of inflammation and prevention of cell division. High-dose methotrexate suppresses bone marrow, while low-dose methotrexate has been reported with immune-stimulating properties (139). According to an in vitro study, low-dose methotrexate supported DC maturation by upregulating CD40, CD80, and CD83. Consequently, DCs stimulated T cell proliferation and ultimately exerted a proper anti-tumor immune response (140).
Pemetrexed is another antimetabolite that inhibits enzymes involved in the folate pathway, including thymidylate synthase, dihydrofolate reductase, and glycinamide ribonucleotide formyltransferase (141–143). Despite the fact that the folate pathway is critical for T cell activation, several studies have reported improved T cell infiltration and antigen presentation in tumor sites upon pemetrexed administration (144). In addition, pemetrexed selectively activates CD45RO+ memory T cells and IFN-γ-producing NK cells (84).
Clinical trials of ICIs and chemotherapy combination therapy
Immunotherapy, particularly ICIs that target PD-1, PD-L1, and CTLA-4, is being widely used and becoming the predominant treatment modality in patients resistant to conventional therapies. ICIs generate moderate-to-severe side effects that require immunosuppressive drugs and active clinical management in some patients (145). Therefore, extensive research has been done in order to develop a proper combination therapy of chemotherapy and ICIs therapy to achieve early (with chemotherapy) and long-lasting (with ICIs) disease control while yielding superior overall survival and minimum risk of adverse effects.
Noteworthy, besides the augmenting effects of chemotherapy on ICI responses, ICIs can also enhance the clinical efficacy of chemotherapy in a reciprocal way (146). Chemotherapy increases the infiltration of immune cells in the tumor. However, a significant proportion of the infiltrated immune cells express ICs over time, limiting their functions (7, 63). Hence, adding ICIs to chemotherapy could augment the responses to chemotherapy (146).
Table 1 demonstrates trials using ICI-chemo combination therapy. In a phase II clinical trial in NSCLC patients, KEYNOTE-021, the efficacy of combining anti-PD-1 (pembrolizumab) with chemotherapy was investigated. It was observed that the combination of anti-PD-1 with carboplatin and pemetrexed culminated in a higher response rate than chemotherapy alone (180). However, adding immunomodulation to chemotherapy did not lead to enhanced toxicity (180, 181). An updated analysis confirmed improved response rate and progression-free survival of this combination therapy which led to its accelerated FDA approval for treatment of metastatic NSCLS patients. Interestingly, the enhanced response rate of 80% was associated with higher tumor PD-L1 expression (≥50%).
Subsequently, the KEYNOTE-189 phase III trial evaluated the efficacy of adding anti-PD-L1 therapy to chemotherapy in non-squamous NSCLC (182). Results indicated improved overall survival and progression-free survival compared to chemotherapy alone, independently of the tumor PD-L1 status. This culminated in full FDA approval for the combination approach of pembrolizumab and chemotherapy in treating NSCLC patients. Nevertheless, these suggestions are subject to change upon approval of nivolumab and ipilimumab for patients with PD-L1- positive tumors (PD-L1 ≥1%) and in combination with chemotherapy regardless of PD-L1 expression (183, 184). A phase III trial (KEYNOTE-407) is ongoing to evaluate whether this combination can also benefit patients with squamous NSCLC. Overall, adding ICI to conventional chemotherapy has modified the standards for treating metastatic lung cancer patients (182).
Despite extensive research on combining ICIs with chemotherapy, only a few trials attempted to systematically determine the most effective chemo-ICIs immunotherapy. The TONIC trial is the only trial to assess the possibility of sensitizing patients with metastatic breast cancer after administrating a PD-1 inhibitor and a chemotherapy agent. Five therapeutic options were tested: no treatment, radiotherapy, cisplatin, cyclophosphamide, or doxorubicin. Doxorubicin appeared to have the strongest sensitizing effect (185). It should be noted that this was a small-scale trial aiming to compare the efficacy of a few induction regimens, including systemic chemotherapy or local radiotherapy treatment in metastatic cancer (186). Thus, the results of this particular should not be generalized to other malignancies and interpreted as definite evidence regarding the superiority of doxorubicin over other induction therapies. Other studies have also used PD-1 inhibitors in combination with chemotherapy. Camrelizumab is a high-affinity, fully humanized, anti-PD-1 IgG4 type monoclonal antibody that blocks the binding of PD-1 to its ligands (187).
Fang et al. have published initial data for a nonrandomized single-arm phase I trial investigating camrelizumab as a first-line treatment for patients with metastatic nasopharyngeal carcinoma. The study investigated gemcitabine and cisplatin combined with camrelizumab (followed by maintenance camrelizumab). More of interest, the combination of camrelizumab with gemcitabine and cisplatin had good clinical efficacy with 20 (ORR 91%) out of 22 patients achieving an overall response after a median follow-up of 10.2 months (188).
In phase III clinical trial (PACIFIC trial) in patients with unresectable NSCLC, anti-PD-L1 antibody durvalumab was administered with chemotherapy plus radiotherapy. Improved treatment outcomes were observed with remarkable improvement in overall survival compared to placebo (median time to death or development of distant metastases 28.3 versus 16.2 months) (183). The same results were achieved in a trial in cohorts of patients with metastatic NSCLC who received anti-PD-L1-antibody atezolizumab combined with paclitaxel, bevacizumab, and carboplatin (184). In addition, both durvalumab and atezolizumab were found to be efficient in monotherapies in patients with metastatic NSCLC (189, 190). This supports the notion that the positive therapeutic performance of these combination therapies may be simply related to the effect of anti-PD-1 monoclonal antibodies.
A negative point of current clinical trials that use combination therapy is that the majority of them administer chemotherapy and ICIs concomitantly at full doses. A study evaluated three different regimens using the combination of ipilimumab and gemcitabine in non-immunogenic mouse models (191). Gemcitabine was used 15 days prior to anti-CTLA-4, concurrently, and three days after anti-CTLA-4. The results showed synergistic effects in the concomitant regimen and removing the first dosage of gemcitabine significantly reduced anti-tumor effects. Similar results were found in another in vivo study that combined cyclophosphamide and anti-CTLA-4 (192). Administration of cyclophosphamide one day prior to anti-CTLA-4 improved immunological anti-tumor responses. However, when the orders were reversed, the anti-tumor effects of anti-CTLA-4 were decreased, and CD8+ T cells underwent massive apoptosis. These findings prove that chemotherapy accentuates the anti-tumor effects of ICI therapy. Nevertheless, only a few studies have addressed the optimal dosage or sequence of administration of chemotherapeutic agents. Preclinical data shows that these parameters may affect treatment outcomes.
It has been reported that the induction phase of chemotherapy may optimize TME for the following ICIs therapy. A study in metastatic triple-negative breast cancer patients evaluated induction therapy with different types of chemotherapy. In the induction phase, low dosages of cisplatin, doxorubicin, and cyclophosphamide were administered for two weeks. In a cohort receiving this regimen, the response rate appeared to be higher than nivolumab alone (193). By far, favorable response rates have been observed upon induction of doxorubicin and cisplatin. Biomarker analysis also supported the notion that treatment with these two agents culminates in the upregulation of immunological pathways related to anti-PD-1, which ultimately facilitates the nivolumab anti-tumor effect. In addition, inducing these two compounds increased TILs in TME (194).
Another phase II clinical trial investigating the performance of ipilimumab with paclitaxel and carboplatin was conducted in NSCLC patients. Three different regimens were evaluated: Administration of chemotherapy prior to ipilimumab, concomitant regimen, and the control group receiving placebo and chemotherapy. Similar to previous trials, results indicated the importance of the chemotherapy induction phase (173). Thus far, most of these trials provided inconclusive preclinical evidence regarding the optimal dosage and sequel of administration. Therefore, a large multi-center trial is needed to determine the optimal combinations of ICIs and immunogenic chemotherapy for cancer treatment.
Dose and time optimization for ICIs and chemotherapy combination therapy
It should be noted that chemotherapy might impose immunosuppressive effects based on dose. High doses of cytotoxic chemotherapy have myelosuppression effects, leading to immunosuppression and ICI resistance (146). Moreover, high-dose chemotherapy causes off-target effects and toxicity. However, it has been implied that the doses for induction of immunologic cell death are generally higher than the cytotoxic doses of chemotherapy (109). So, optimum doses of chemotherapy are required to enhance the ICIs response. Interestingly, a study showed that daily prescription of 100 mg oral cyclophosphamide decreased Treg proportion without significant effects on other immune cells. However, doubling this dose depleted all lymphocytes (97). This study concluded that the metronomic doses of cyclophosphamide could decrease Tregs and spare effector T and NK cells (97).
Besides the treatment dose, treatment time is also critical in achieving the best outcome. It has been reported that a single standard dose of gemtacibine increased the infiltration of CD8+ T cells into the tumor and upregulated the PD-L1 expression in pancreatic and ovarian tumors (127, 135). However, this effect is observed within the first week and not in the second week after treatment (127). So, administration of ICIs within the first week of treatment with gemtacibine might result in favorable responses. Accordingly, similar studies on mice showed that ipilimumab had a synergistic effect with gemtacibine or cyclophosphamide only when administered concomitantly (191, 192). Interestingly, anti-tumor immune responses are yielded when chemotherapy is administered one day before the ICI. However, reversing the order caused significant apoptosis in CD8+ T cells and reduced the ICIs effects (192). These findings indicate the necessitate to optimize the dose and timing of treatment.
Patient-derived organoid (PDO) platforms are promising models to optimize the dose and time of treatment ex vivo (195). Advanced PDOs, such as air-liquid interface (ALI) models that contain both tumor and immune cells in a 3D interaction resembling tumor milieu, can be used in further investigations to determine the optimum dose and time and also the ideal combination of chemo-immunotherapy (196, 197).
Combination of chemotherapy with other immunotherapies
According to the systemic and local changes that chemotherapy makes, it can synergize the effects of many immunotherapies. Adoptive cell therapy is an immunotherapeutic approach that uses the patient’s or another donor’s immune cells to fight cancer (18). Tumor-infiltrating lymphocytes (TILs) are immune cells infiltrating into the TME (17). The frequency of TILs in the TME is a prognostic factor in many tumors (198). Isolation, expansion, activation, and re-administration of TILs to the patients is an old way of immunotherapy in immunogenic tumors (17). However, in non-immunogenic or cold tumors with a low frequency of TILs, TIL therapy might not significantly improve the survival (17). In this condition, chemotherapy might heat up the TME, leading to a higher infiltration rate of TILs (199). So, it can potentiate TIL therapy in cold tumors (199). More recently, CAR-T cell therapy has been introduced and used as a promising anti-tumor modality (200). CAR-T cells are genetically modified T cells that harbor the chimeric antigen receptors comprising the extracellular domain of B cell receptor (BCR) and signaling domains of T cell receptors (TCRs) and co-stimulatory receptors (200). They can be activated independent of MHC and produce many cytokines or release cytotoxic molecules (200). Chemotherapy can enhance the susceptibility of tumor cells to cytotoxic mediators of T cells such as granzyme B (201). In the mouse model of breast cancer, combining doxorubicin and T cell therapy had synergistic effects beyond the effects of each treatment alone (202). Further clinical trials in the era of combining chemotherapy and adoptive cell therapy are warranted.
Chemotherapy is also beneficial for improving the effects of cancer vaccines, especially in cold tumors (203–205). In a mouse model of melanoma (B16), it has been shown that the addition of ICI (anti-PD-1) to the cancer peptide vaccine did not further inhibit the tumor growth and was not able to improve the survival (203). However, combining metronomic chemotherapy with the vaccine resulted in tumor growth inhibition in half of the mice (203). Notably, adding ICI to this combination delayed tumor growth in all mice and inhibited tumor growth in two-thirds of mice (203). This synergistic effect of chemotherapy with cancer vaccines might be through the decrease of Tregs and inhibition of tumor angiogenesis (204). These findings suggest that multi-aspect combination therapy should be employed in advanced and resistant tumors.
Oncolytic viruses (OVs) are wild-type or engineered viruses with anti-tumor capabilities that is able to impose direct cytotoxic or heat up the TME via upregulation of the inflammatory responses (206). Similar to chemotherapy, OVs can induce ICD, releasing neoantigens and inflammatory mediators that potentiate the immunotherapies (207). However, chemotherapy and OVs can be combined to maximize the inflammatory and cytotoxic responses against tumor cells (207). There are many clinical trials evaluating the safety and efficacy of chemo-OV combination therapy that are comprehensively reviewed elsewhere (207).
Chemotherapy can be combined with many other immunotherapies and targeted therapies, such as monoclonal antibodies, bispecific antibodies, nanobodies, DC vaccines, NK/CAR-NK cells, etc., which their safety and efficacy of these combinations are under investigation.
Perspectives and conclusions
According to several clinical trials, chemotherapeutic agents can exert immunostimulatory effects by activating effector cells and/or inhibiting immunosuppressive cells or elevating immunogenicity and enhancing T-cell infiltration. However, more research is required to achieve the best and most efficacious combination therapy. In order to do so, it is suggested that future researches focus on the following suggestions: 1. Preclinical studies that evaluate drug efficacy should be compatible as much as possible with the clinical situation. Thereby, results obtained from animal models can also be utilized for human malignancies. 2. Until now, most studies assessed the immunomodulatory effects of chemotherapy in peripheral blood and not TME. Further studies investigating the effect of chemotherapy on TME are required. 3. In addition to chemotherapy, ICD can also be induced as a result of pathological conditions. Therefore, it is crucial to differentiate between chemotherapy-induced ICD and the type of ICD caused by normal physiological processes and other pathological conditions. 4. Identifying tumor-associated markers specific to each tumor and each cancer patient may help design better combination therapies in the future. 5. Furthermore, understanding the toxicity patterns of these treatment regimens in preclinical studies would provide us with more knowledge of how to prevent and manage them in clinical studies. In conclusion, various clinical and preclinical findings indicate that combination therapy can result in a more efficient, long-lasting anti-tumor immune response.
Author contributions
WL, conception, design, and inviting co-authors to participate. LZ, CZ, SZ, and XC, writing original manuscript draft. WL, JL, and FX, review and editing of manuscript critically for important intellectual content and provided comments and feedback for the scientific contents of the manuscript. All authors contributed to the article and approved the submitted version.
Funding
This work was supported by the Project of Shaoxing Medical Key Discipline Construction Plan (2019SZD06 to LZ) and the Project of Health and Family Planning Commission of Zhejiang Province (2021KY1139, and 2018KY831 to LZ), Shaoxing Medical and Health Science and Technology Plan Project (2020A13026 to LZ).
Conflict of interest
The authors declare that the research was conducted in the absence of any commercial or financial relationships that could be construed as a potential conflict of interest.
Publisher’s note
All claims expressed in this article are solely those of the authors and do not necessarily represent those of their affiliated organizations, or those of the publisher, the editors and the reviewers. Any product that may be evaluated in this article, or claim that may be made by its manufacturer, is not guaranteed or endorsed by the publisher.
References
1. Finn OJ. A believer’s overview of cancer immunosurveillance and immunotherapy. J Immunol (2018) 200(2):385–91. doi: 10.4049/jimmunol.1701302
2. Rabinovich GA, Gabrilovich D, Sotomayor EM. Immunosuppressive strategies that are mediated by tumor cells. Annu Rev Immunol (2007) 25:267–96. doi: 10.1146/annurev.immunol.25.022106.141609
3. Salami F, Fekrvand S, Yazdani R, Shahkarami S, Azizi G, Bagheri Y, et al. Evaluation of expression of LRBA and CTLA-4 proteins in common variable immunodeficiency patients. Immunol investigations (2022) 51(2):381–94. doi: 10.1080/08820139.2020.1833029
4. Fridman WH, Pagès F, Sautès-Fridman C, Galon J. The immune contexture in human tumours: impact on clinical outcome. Nat Rev Cancer (2012) 12(4):298–306. doi: 10.1038/nrc3245
5. Galluzzi L, Senovilla L, Zitvogel L, Kroemer G. The secret ally: immunostimulation by anticancer drugs. Nat Rev Drug Discovery (2012) 11(3):215–33. doi: 10.1038/nrd3626
6. Azizi G, Jamee M, Yazdani R, Bagheri Y, Fayyaz F, Jadidi-Niaragh F, et al. CTLA-4 expression in CD4+ T cells from patients with LRBA deficiency and common variable immunodeficiency with no known monogenic disease. J Investig Allergol Clin Immunol (2018) 28(6):422–4. doi: 10.18176/jiaci.0302
7. Kazemi MH, Najafi A, Karami J, Ghazizadeh F, Yousefi H, Falak R, et al. Immune and metabolic checkpoints blockade: Dual wielding against tumors. Int Immunopharmacol (2021) 94:107461. doi: 10.1016/j.intimp.2021.107461
8. Raedler LA. Opdivo (Nivolumab): Second PD-1 inhibitor receives FDA approval for unresectable or metastatic melanoma. Am Health Drug Benefits (2015) 8(Spec Feature):180–3.
9. Kazandjian D, Suzman DL, Blumenthal G, Mushti S, He K, Libeg M, et al. FDA Approval summary: Nivolumab for the treatment of metastatic non-small cell lung cancer with progression on or after platinum-based chemotherapy. Oncologist (2016) 21(5):634–42. doi: 10.1634/theoncologist.2015-0507
10. Powles T, Eder JP, Fine GD, Braiteh FS, Loriot Y, Cruz C, et al. MPDL3280A (anti-PD-L1) treatment leads to clinical activity in metastatic bladder cancer. Nat (2014) 515(7528):558–62. doi: 10.1038/nature13904
11. Wolchok JD, Kluger H, Callahan MK, Postow MA, Rizvi NA, Lesokhin AM, et al. Nivolumab plus ipilimumab in advanced melanoma. N Engl J Med (2013) 369(2):122–33. doi: 10.1056/NEJMoa1302369
12. Galanina N, Kline J, Bishop MR. Emerging role of checkpoint blockade therapy in lymphoma. Ther Adv Hematol (2017) 8(2):81–90. doi: 10.1177/2040620716673787
13. Lee CH, Motzer RJ. Immune checkpoint therapy in renal cell carcinoma. Cancer J (2016) 22(2):92–5. doi: 10.1097/PPO.0000000000000177
14. Hajifathali A, Parkhideh S, Kazemi MH, Chegeni R, Roshandel E, Gholizadeh M. Immune checkpoints in hematologic malignancies: What made the immune cells and clinicians exhausted! J Cell Physiol (2020) 235(12):9080–97. doi: 10.1002/jcp.29769
15. Shin D, Garcia-Diaz A, Zaretsky J, Escuin-Ordinas H, Hu-Lieskovan S, Palaskas NJ, et al. Innate resistance of PD-1 blockade through loss of function mutations in JAK resulting in inability to express PD-L1 upon interferon exposure. J Immunother Cancer (2015) 3(Suppl 2):311. doi: 10.1186/2051-1426-3-S2-P311
16. Sharma P, Hu-Lieskovan S, Wargo JA, Ribas A. Primary, adaptive, and acquired resistance to cancer immunotherapy. Cell (2017) 168(4):707–23. doi: 10.1016/j.cell.2017.01.017
17. Kazemi MH, Barough MS, Ghanavatinejad A, Momeni-Varposhti Z, Khorrami S, Sadeghi B, et al. Decrease of tumor-infiltrating regulatory T cells using pentoxifylline: An ex vivo analysis in triple-negative breast cancer mouse model. Iranian J Allergy Asthma Immunol (2022) 21:1–11. doi: 10.18502/ijaai.v21i2.9224
18. Barzaman K, Moradi-Kalbolandi S, Hosseinzadeh A, Kazemi MH, Khorramdelazad H, Safari E, et al. Breast cancer immunotherapy: Current and novel approaches. Int Immunopharmacol (2021) 98:107886. doi: 10.1016/j.intimp.2021.107886
19. Swart M, Verbrugge I, Beltman JB. Combination approaches with immune-checkpoint blockade in cancer therapy. Front Oncol (2016) 6:233. doi: 10.3389/fonc.2016.00233
20. Harris SJ, Brown J, Lopez J, Yap TA. Immuno-oncology combinations: raising the tail of the survival curve. Cancer Biol Med (2016) 13(2):171–93. doi: 10.20892/j.issn.2095-3941.2016.0015
21. Bendell JC, Powderly JD, Lieu CH, Eckhardt SG, Hurwitz H, Hochster HS, et al. Safety and efficacy of MPDL3280A (anti-PDL1) in combination with bevacizumab (bev) and/or FOLFOX in patients (pts) with metastatic colorectal cancer (mCRC). J Clin Oncol (2015) 33(3_suppl):704–. doi: 10.1200/jco.2015.33.3_suppl.704
22. Amin A, Plimack ER, Infante JR, Ernstoff MS, Rini BI, McDermott DF, et al. Nivolumab (anti-PD-1; BMS-936558, ONO-4538) in combination with sunitinib or pazopanib in patients (pts) with metastatic renal cell carcinoma (mRCC). J Clin Oncol (2014) 32(15_suppl):5010–. doi: 10.1200/jco.2014.32.15_suppl.5010
23. Ribas A, Butler M, Lutzky J, Lawrence DP, Robert C, Miller W, et al. Phase I study combining anti-PD-L1 (MEDI4736) with BRAF (dabrafenib) and/or MEK (trametinib) inhibitors in advanced melanoma. J Clin Oncol (2015) 33(15_suppl):3003–. doi: 10.1200/jco.2015.33.15_suppl.3003
24. Antonia SJ, Brahmer JR, Gettinger S, Chow LQ, Juergens R, Shepherd FA, et al. Nivolumab (Anti-PD-1; BMS-936558, ONO-4538) in combination with platinum-based doublet chemotherapy (PT-DC) in advanced non-small cell lung cancer (NSCLC): Metastatic non-small cell lung cancer. Int J Radiat Oncol Biol Physics (2014) 90(5):S2. doi: 10.1016/j.ijrobp.2014.08.024
25. Pfirschke C, Engblom C, Rickelt S, Cortez-Retamozo V, Garris C, Pucci F, et al. Immunogenic chemotherapy sensitizes tumors to checkpoint blockade therapy. Immun (2016) 44(2):343–54. doi: 10.1016/j.immuni.2015.11.024
26. Grasselly C, Denis M, Bourguignon A, Talhi N, Mathe D, Tourette A, et al. The antitumor activity of combinations of cytotoxic chemotherapy and immune checkpoint inhibitors is model-dependent. Front Immunol (2018) 9:2100. doi: 10.3389/fimmu.2018.02100
27. van der Most RG, Currie AJ, Mahendran S, Prosser A, Darabi A, Robinson BW, et al. Tumor eradication after cyclophosphamide depends on concurrent depletion of regulatory T cells: a role for cycling TNFR2-expressing effector-suppressor T cells in limiting effective chemotherapy. Cancer Immunol Immunother (2009) 58(8):1219–28. doi: 10.1007/s00262-008-0628-9
28. Zitvogel L, Galluzzi L, Smyth MJ, Kroemer G. Mechanism of action of conventional and targeted anticancer therapies: reinstating immunosurveillance. Immun (2013) 39(1):74–88. doi: 10.1016/j.immuni.2013.06.014
29. Zitvogel L, Kepp O, Kroemer G. Immune parameters affecting the efficacy of chemotherapeutic regimens. Nat Rev Clin Oncol (2011) 8(3):151–60. doi: 10.1038/nrclinonc.2010.223
30. Cui S. Immunogenic chemotherapy sensitizes renal cancer to immune checkpoint blockade therapy in preclinical models. Med Sci Monit (2017) 23:3360–6. doi: 10.12659/MSM.902426
31. Fares CM, Van Allen EM, Drake CG, Allison JP, Hu-Lieskovan S. Mechanisms of resistance to immune checkpoint blockade: Why does checkpoint inhibitor immunotherapy not work for all patients? Am Soc Clin Oncol Educ Book (2019) 39:147–64. doi: 10.1200/EDBK_240837
32. Schoenfeld AJ, Hellmann MD. Acquired resistance to immune checkpoint inhibitors. Cancer Cell (2020) 37(4):443–55. doi: 10.1016/j.ccell.2020.03.017
33. Restifo NP, Marincola FM, Kawakami Y, Taubenberger J, Yannelli JR, Rosenberg SA. Loss of functional beta2-microglobulin in metastatic melanomas from five patients receiving immunotherapy. J Natl Cancer InsT (1996) 88(2):100–8. doi: 10.1093/jnci/88.2.100
34. Hulpke S, Tampé R. The MHC I loading complex: a multitasking machinery in adaptive immunity. Trends Biochem Sci (2013) 38(8):412–20. doi: 10.1016/j.tibs.2013.06.003
35. Gettinger S, Choi J, Hastings K, Truini A, Datar I, Sowell R, et al. Impaired HLA class I antigen processing and presentation as a mechanism of acquired resistance to immune checkpoint inhibitors in lung CancerAntigen-processing defects and resistance to PD-1 blockade. Cancer discovery (2017) 7(12):1420–35. doi: 10.1158/2159-8290.CD-17-0593
36. Le DT, Durham JN, Smith KN, Wang H, Bartlett BR, Aulakh LK, et al. Mismatch repair deficiency predicts response of solid tumors to PD-1 blockade. Sci (2017) 357(6349):409–13. doi: 10.1126/science.aan6733
37. Zaretsky JM, Garcia-Diaz A, Shin DS, Escuin-Ordinas H, Hugo W, Hu-Lieskovan S, et al. Mutations associated with acquired resistance to PD-1 blockade in melanoma. New Engl J Med (2016) 375(9):819–29. doi: 10.1056/NEJMoa1604958
38. Middha S, Yaeger R, Shia J, Stadler ZK, King S, Guercio S, et al. Majority of B2M-mutant and-deficient colorectal carcinomas achieve clinical benefit from immune checkpoint inhibitor therapy and are microsatellite instability-high. JCO Precis Oncol (2019) 3:1–14. doi: 10.1200/PO.18.00321
39. Shayan G, Srivastava R, Li J, Schmitt N, Kane LP, Ferris RL. Adaptive resistance to anti-PD1 therapy by Tim-3 upregulation is mediated by the PI3K-akt pathway in head and neck cancer. Oncoimmunol (2017) 6(1):e1261779. doi: 10.1080/2162402X.2016.1261779
40. McGranahan N, Furness AJ, Rosenthal R, Ramskov S, Lyngaa R, Saini SK, et al. Clonal neoantigens elicit T cell immunoreactivity and sensitivity to immune checkpoint blockade. Sci (2016) 351(6280):1463–9. doi: 10.1126/science.aaf1490
41. Rizvi NA, Hellmann MD, Snyder A, Kvistborg P, Makarov V, Havel JJ, et al. Mutational landscape determines sensitivity to PD-1 blockade in non–small cell lung cancer. Sci (2015) 348(6230):124–8. doi: 10.1126/science.aaa1348
42. Anagnostou V, Smith KN, Forde PM, Niknafs N, Bhattacharya R, White J, et al. Evolution of neoantigen landscape during immune checkpoint blockade in non-small cell lung cancer. Cancer Discovery (2017) 7(3):264–76. doi: 10.1158/2159-8290.CD-16-0828
43. Rosenthal R, Cadieux EL, Salgado R, Bakir MA, Moore DA, Hiley CT, et al. Neoantigen-directed immune escape in lung cancer evolution. Nat (2019) 567(7749):479–85. doi: 10.1038/s41586-019-1032-7
44. Verdegaal EM, De Miranda NF, Visser M, Harryvan T, Van Buuren MM, Andersen RS, et al. Neoantigen landscape dynamics during human melanoma–T cell interactions. Nat (2016) 536(7614):91–5. doi: 10.1038/nature18945
45. Shin DS, Zaretsky JM, Escuin-Ordinas H, Garcia-Diaz A, Hu-Lieskovan S, Kalbasi A, et al. Primary resistance to PD-1 blockade mediated by <em<JAK1/2</em< mutations. Cancer Discov (2017) 7(2):188. doi: 10.1158/2159-8290.CD-16-1223
46. Takeda K, Nakayama M, Hayakawa Y, Kojima Y, Ikeda H, Imai N, et al. IFN-γ is required for cytotoxic T cell-dependent cancer genome immunoediting. Nat Commun (2017) 8(1):14607. doi: 10.1038/ncomms14607
47. Bach EA, Aguet M, Schreiber RD. The IFNγ receptor: a paradigm for cytokine receptor signaling. Annu Rev Immunol (1997) 15(1):563–91. doi: 10.1146/annurev.immunol.15.1.563
48. Sucker A, Zhao F, Pieper N, Heeke C, Maltaner R, Stadtler N, et al. Acquired IFNγ resistance impairs anti-tumor immunity and gives rise to T-cell-resistant melanoma lesions. Nat Commun (2017) 8(1):1–15. doi: 10.1038/ncomms15440
49. Rivera Vargas T, Apetoh L. Can immunogenic chemotherapies relieve cancer cell resistance to immune checkpoint inhibitors? Front Immunol (1181) 2019:10. doi: 10.3389/fimmu.2019.01181
50. Heinhuis KM, Ros W, Kok M, Steeghs N, Beijnen JH, Schellens JHM. Enhancing antitumor response by combining immune checkpoint inhibitors with chemotherapy in solid tumors. Ann Oncol (2019) 30(2):219–35. doi: 10.1093/annonc/mdy551
51. Wang Y, Deng W, Li N, Neri S, Sharma A, Jiang W, et al. Combining immunotherapy and radiotherapy for cancer treatment: Current challenges and future directions. Front Pharmacol (2018) 9:185. doi: 10.3389/fphar.2018.00185
52. Gibney GT, Weiner LM, Atkins MB. Predictive biomarkers for checkpoint inhibitor-based immunotherapy. Lancet Oncol (2016) 17(12):e542–e51. doi: 10.1016/S1470-2045(16)30406-5
53. Seo J-S, Kim A, Shin J-Y, Kim YT. Comprehensive analysis of the tumor immune micro-environment in non-small cell lung cancer for efficacy of checkpoint inhibitor. Sci Rep (2018) 8(1):14576. doi: 10.1038/s41598-018-32855-8
54. Sharma P, Allison JP. The future of immune checkpoint therapy. Sci (2015) 348(6230):56. doi: 10.1126/science.aaa8172
55. Taube JM, Klein A, Brahmer JR, Xu H, Pan X, Kim JH, et al. Association of PD-1, PD-1 ligands, and other features of the tumor immune microenvironment with response to anti–PD-1 therapy. Clin Cancer Res (2014) 20(19):5064. doi: 10.1158/1078-0432.CCR-13-3271
56. Champiat S, Ileana E, Giaccone G, Besse B, Mountzios G, Eggermont A, et al. Incorporating immune-checkpoint inhibitors into systemic therapy of NSCLC. J Thorac Oncol (2014) 9(2):144–53. doi: 10.1097/JTO.0000000000000074
57. Ghosh A, Politikos I, Perales MA. Stop and go: hematopoietic cell transplantation in the era of chimeric antigen receptor T cells and checkpoint inhibitors. Curr Opin Oncol (2017) 29(6):474–83. doi: 10.1097/CCO.0000000000000408
58. Otsubo D, Yamashita K, Fujita M, Nishi M, Kimura Y, Hasegawa H, et al. Early-phase treatment by low-dose 5-fluorouracil or primary tumor resection inhibits MDSC-mediated lung metastasis formation. Anticancer Res (2015) 35(8):4425–31.
59. Dimeloe S, Frick C, Fischer M, Gubser PM, Razik L, Bantug GR, et al. Human regulatory T cells lack the cyclophosphamide-extruding transporter ABCB1 and are more susceptible to cyclophosphamide-induced apoptosis. Eur J Immunol (2014) 44(12):3614–20. doi: 10.1002/eji.201444879
60. Schaer DA, Geeganage S, Amaladas N, Lu ZH, Rasmussen ER, Sonyi A, et al. The folate pathway inhibitor pemetrexed pleiotropically enhances effects of cancer immunotherapy. Clin Cancer Res (2019) 25(23):7175–88. doi: 10.1158/1078-0432.CCR-19-0433
61. Ma Y, Mattarollo SR, Adjemian S, Yang H, Aymeric L, Hannani D, et al. CCL2/CCR2-dependent recruitment of functional antigen-presenting cells into tumors upon chemotherapy. Cancer Res (2014) 74(2):436. doi: 10.1158/0008-5472.CAN-13-1265
62. Wanderley CW, Colón DF, Luiz JPM, Oliveira FF, Viacava PR, Leite CA, et al. Paclitaxel reduces tumor growth by reprogramming tumor-associated macrophages to an M1 profile in a TLR4-dependent manner. Cancer Res (2018) 78(20):5891. doi: 10.1158/0008-5472.CAN-17-3480
63. Galluzzi L, Humeau J, Buqué A, Zitvogel L, Kroemer G. Immunostimulation with chemotherapy in the era of immune checkpoint inhibitors. Nat Rev Clin Oncol (2020) 17:725–41. doi: 10.1038/s41571-020-0413-z
64. Bakhshaei P, Kazemi MH, Golara M, Abdolmaleki S, Khosravi-Eghbal R, Khoshnoodi J, et al. Investigation of the cellular immune response to recombinant fragments of filamentous hemagglutinin and pertactin of bordetella pertussis in BALB/c mice. J Interferon Cytokine Res (2018) 38(4):161–70. doi: 10.1089/jir.2017.0060
65. Galluzzi L, Yamazaki T, Kroemer G. Linking cellular stress responses to systemic homeostasis. Nat Rev Mol Cell Biol (2018) 19(11):731–45. doi: 10.1038/s41580-018-0068-0
66. Ma Y, Adjemian S, Mattarollo Stephen R, Yamazaki T, Aymeric L, Yang H, et al. Anticancer chemotherapy-induced intratumoral recruitment and differentiation of antigen-presenting cells. Immun (2013) 38(4):729–41. doi: 10.1016/j.immuni.2013.03.003
67. Ghalamfarsa G, Kazemi MH, Raoofi Mohseni S, Masjedi A, Hojjat-Farsangi M, Azizi G, et al. CD73 as a potential opportunity for cancer immunotherapy. Expert Opin Ther targets (2019) 23(2):127–42. doi: 10.1080/14728222.2019.1559829
68. Kazemi MH, Raoofi Mohseni S, Hojjat-Farsangi M, Anvari E, Ghalamfarsa G, Mohammadi H, et al. Adenosine and adenosine receptors in the immunopathogenesis and treatment of cancer. J Cell Physiol (2018) 233(3):2032–57. doi: 10.1002/jcp.25873
69. Kasikova L, Hensler M, Truxova I, Skapa P, Laco J, Belicova L, et al. Calreticulin exposure correlates with robust adaptive antitumor immunity and favorable prognosis in ovarian carcinoma patients. J ImmunoTher Cancer (2019) 7(1):312. doi: 10.1186/s40425-019-0781-z
70. Wang Z, Chen J, Hu J, Zhang H, Xu F, He W, et al. cGAS/STING axis mediates a topoisomerase II inhibitor-induced tumor immunogenicity. J Clin Invest (2019) 129(11):4850–62. doi: 10.1172/JCI127471
71. Sistigu A, Yamazaki T, Vacchelli E, Chaba K, Enot DP, Adam J, et al. Cancer cell-autonomous contribution of type I interferon signaling to the efficacy of chemotherapy. Nat Med (2014) 20(11):1301–9. doi: 10.1038/nm.3708
72. Galluzzi L, Buqué A, Kepp O, Zitvogel L, Kroemer G. Immunogenic cell death in cancer and infectious disease. Nat Rev Immunol (2017) 17(2):97–111. doi: 10.1038/nri.2016.107
73. Fucikova J, Moserova I, Urbanova L, Bezu L, Kepp O, Cremer I, et al. Prognostic and predictive value of DAMPs and DAMP-associated processes in cancer. Front Immunol (2015) 6:402. doi: 10.3389/fimmu.2015.00402
74. Melief CJM, Kessler JH. Novel insights into the HLA class I immunopeptidome and T-cell immunosurveillance. Genome Med (2017) 9(1):44. doi: 10.1186/s13073-017-0439-8
75. Roshandel E, Noorazar L, Farhadihosseinabadi B, Mehdizadeh M, Kazemi MH, Parkhideh S. PI3 kinase signaling pathway in hematopoietic cancers: A glance in miRNA's role. J Clin Lab analysis (2021) 35(4):e23725. doi: 10.1002/jcla.23725
76. Gravett AM, Trautwein N, Stevanović S, Dalgleish AG, Copier J. Gemcitabine alters the proteasome composition and immunopeptidome of tumour cells. Oncoimmunol (2018) 7(6):e1438107. doi: 10.1080/2162402X.2018.1438107
77. Goss GD, Tsvetkova E. Drug resistance and its significance for treatment decisions in non-small-cell lung cancer. Curr Oncol (1969) 19(0):S45–51. doi: 10.3747/co.19.1113
78. Wang Y-J, Fletcher R, Yu J, Zhang L. Immunogenic effects of chemotherapy-induced tumor cell death. Genes Diseases (2018) 5(3):194–203. doi: 10.1016/j.gendis.2018.05.003
79. Zitvogel L, Apetoh L, Ghiringhelli F, Kroemer G. Immunological aspects of cancer chemotherapy. Nat Rev Immunol (2008) 8(1):59–73. doi: 10.1038/nri2216
80. Park SJ, Ye W, Xiao R, Silvin C, Padget M, Hodge JW, et al. Cisplatin and oxaliplatin induce similar immunogenic changes in preclinical models of head and neck cancer. Oral Oncol (2019) 95:127–35. doi: 10.1016/j.oraloncology.2019.06.016
81. Leduc C, Adam J, Louvet E, Sourisseau T, Dorvault N, Bernard M, et al. TPF induction chemotherapy increases PD-L1 expression in tumour cells and immune cells in head and neck squamous cell carcinoma. ESMO Open (2018) 3(1):e000257.
82. Fukuoka E, Yamashita K, Tanaka T, Sawada R, Sugita Y, Arimoto A, et al. Neoadjuvant chemotherapy increases PD-L1 expression and CD8(+) tumor-infiltrating lymphocytes in esophageal squamous cell carcinoma. Anticancer Res (2019) 39(8):4539–48. doi: 10.21873/anticanres.13631
83. Hato SV, Khong A, de Vries IJM, Lesterhuis WJ. Molecular pathways: The immunogenic effects of platinum-based chemotherapeutics. Clin Cancer Res (2014) 20(11):2831. doi: 10.1158/1078-0432.CCR-13-3141
84. Mathew M, Enzler T, Shu CA, Rizvi NA. Combining chemotherapy with PD-1 blockade in NSCLC. Pharmacol Ther (2018) 186:130–7. doi: 10.1016/j.pharmthera.2018.01.003
85. de Biasi AR, Villena-Vargas J, Adusumilli PS. Cisplatin-induced antitumor immunomodulation: A review of preclinical and clinical evidence. Clin Cancer Res (2014) 20(21):5384. doi: 10.1158/1078-0432.CCR-14-1298
86. Roselli M, Cereda V, di Bari MG, Formica V, Spila A, Jochems C, et al. Effects of conventional therapeutic interventions on the number and function of regulatory T cells. Oncoimmunol (2013) 2(10):e27025. doi: 10.4161/onci.27025
87. Wu J, Waxman DJ. Immunogenic chemotherapy: Dose and schedule dependence and combination with immunotherapy. Cancer Letters (2018) 419:210–21. doi: 10.1016/j.canlet.2018.01.050
88. Lutsiak ME, Semnani RT, De Pascalis R, Kashmiri SV, Schlom J, Sabzevari H. Inhibition of CD4(+)25+ T regulatory cell function implicated in enhanced immune response by low-dose cyclophosphamide. Blood (2005) 105(7):2862–8. doi: 10.1182/blood-2004-06-2410
89. Schiavoni G, Sistigu A, Valentini M, Mattei F, Sestili P, Spadaro F, et al. Cyclophosphamide synergizes with type I interferons through systemic dendritic cell reactivation and induction of immunogenic tumor apoptosis. Cancer Res (2011) 71(3):768–78. doi: 10.1158/0008-5472.CAN-10-2788
90. Matar P, Rozados VR, Gervasoni SI, Scharovsky GO. Th2/Th1 switch induced by a single low dose of cyclophosphamide in a rat metastatic lymphoma model. Cancer Immunol Immunother (2002) 50(11):588–96. doi: 10.1007/s00262-001-0237-3
91. Bagheri Y, Babaha F, Falak R, Yazdani R, Azizi G, Sadri M, et al. IL-10 induces TGF-β secretion, TGF-β receptor II upregulation, and IgA secretion in b cells. Eur Cytokine Network (2019) 30(3):107–13. doi: 10.1684/ecn.2019.0434
92. Azizi G, Bagheri Y, Yazdani R, Zaki-Dizaji M, Jamee M, Jadidi-Niaragh F, et al. The profile of IL-4, IL-5, IL-10 and GATA3 in patients with LRBA deficiency and CVID with no known monogenic disease: Association with disease severity. Allergol Immunopathol (Madr) (2019) 47(2):172–8. doi: 10.1016/j.aller.2018.06.003
93. Buccione C, Fragale A, Polverino F, Ziccheddu G, Aricò E, Belardelli F, et al. Role of interferon regulatory factor 1 in governing treg depletion, Th1 polarization, inflammasome activation and antitumor efficacy of cyclophosphamide. Int J Cancer (2018) 142(5):976–87. doi: 10.1002/ijc.31083
94. Pol J, Vacchelli E, Aranda F, Castoldi F, Eggermont A, Cremer I, et al. Trial watch: Immunogenic cell death inducers for anticancer chemotherapy. Oncoimmunol (2015) 4(4):e1008866. doi: 10.1080/2162402X.2015.1008866
95. Garris CS, Arlauckas SP, Kohler RH, Trefny MP, Garren S, Piot C, et al. Successful anti-PD-1 cancer immunotherapy requires T cell-dendritic cell crosstalk involving the cytokines IFN-γ and IL-12. Immun (2018) 49(6):1148–61.e7. doi: 10.1016/j.immuni.2018.09.024
96. Wu J, Jordan M, Waxman DJ. Metronomic cyclophosphamide activation of anti-tumor immunity: tumor model, mouse host, and drug schedule dependence of gene responses and their upstream regulators. BMC Cancer (2016) 16:623. doi: 10.1186/s12885-016-2597-2
97. Ghiringhelli F, Menard C, Puig PE, Ladoire S, Roux S, Martin F, et al. Metronomic cyclophosphamide regimen selectively depletes CD4+CD25+ regulatory T cells and restores T and NK effector functions in end stage cancer patients. Cancer Immunol Immunother (2007) 56(5):641–8. doi: 10.1007/s00262-006-0225-8
98. Tongu M, Harashima N, Monma H, Inao T, Yamada T, Kawauchi H, et al. Metronomic chemotherapy with low-dose cyclophosphamide plus gemcitabine can induce anti-tumor T cell immunity in vivo. Cancer Immunol Immunother (2013) 62(2):383–91. doi: 10.1007/s00262-012-1343-0
99. Barbon CM, Yang M, Wands GD, Ramesh R, Slusher BS, Hedley ML, et al. Consecutive low doses of cyclophosphamide preferentially target tregs and potentiate T cell responses induced by DNA PLG microparticle immunization. Cell Immunol (2010) 262(2):150–61. doi: 10.1016/j.cellimm.2010.02.007
100. Moschella F, Valentini M, Aricò E, Macchia I, Sestili P, D'Urso MT, et al. Unraveling cancer chemoimmunotherapy mechanisms by gene and protein expression profiling of responses to cyclophosphamide. Cancer Res (2011) 71(10):3528–39. doi: 10.1158/0008-5472.CAN-10-4523
101. Dehshahri A, Ashrafizadeh M, Ghasemipour Afshar E, Pardakhty A, Mandegary A, Mohammadinejad R, et al. Topoisomerase inhibitors: Pharmacology and emerging nanoscale delivery systems. Pharmacol Res (2020) 151:104551. doi: 10.1016/j.phrs.2019.104551
102. Haggerty TJ, Dunn IS, Rose LB, Newton EE, Martin S, Riley JL, et al. Topoisomerase inhibitors modulate expression of melanocytic antigens and enhance T cell recognition of tumor cells. Cancer Immunol Immunother (2011) 60(1):133–44. doi: 10.1007/s00262-010-0926-x
103. McKenzie JA, Mbofung RM, Malu S, Zhang M, Ashkin E, Devi S, et al. The effect of topoisomerase I inhibitors on the efficacy of T-Cell-Based cancer immunotherapy. J Natl Cancer Inst (2018) 110(7):777–86. doi: 10.1093/jnci/djx257
104. Frey B, Stache C, Rubner Y, Werthmöller N, Schulz K, Sieber R, et al. Combined treatment of human colorectal tumor cell lines with chemotherapeutic agents and ionizing irradiation can in vitro induce tumor cell death forms with immunogenic potential. J Immunotoxicol (2012) 9(3):301–13. doi: 10.3109/1547691X.2012.693547
105. Alagkiozidis I, Facciabene A, Tsiatas M, Carpenito C, Benencia F, Adams S, et al. Time-dependent cytotoxic drugs selectively cooperate with IL-18 for cancer chemo-immunotherapy. J Trans Med (2011) 9(1):77. doi: 10.1186/1479-5876-9-77
106. Wan S, Pestka S, Jubin RG, Lyu YL, Tsai YC, Liu LF. Chemotherapeutics and radiation stimulate MHC class I expression through elevated interferon-beta signaling in breast cancer cells. PloS One (2012) 7(3):e32542. doi: 10.1371/journal.pone.0032542
107. Alizadeh D, Trad M, Hanke NT, Larmonier CB, Janikashvili N, Bonnotte B, et al. Doxorubicin eliminates myeloid-derived suppressor cells and enhances the efficacy of adoptive T-cell transfer in breast cancer. Cancer Res (2014) 74(1):104–18. doi: 10.1158/0008-5472.CAN-13-1545
108. Martins I, Tesniere A, Kepp O, Michaud M, Schlemmer F, Senovilla L, et al. Chemotherapy induces ATP release from tumor cells. Cell Cycle (2009) 8(22):3723–8. doi: 10.4161/cc.8.22.10026
109. Showalter A, Limaye A, Oyer JL, Igarashi R, Kittipatarin C, Copik AJ, et al. Cytokines in immunogenic cell death: Applications for cancer immunotherapy. Cytokine (2017) 97:123–32. doi: 10.1016/j.cyto.2017.05.024
110. Mi J, Zhang X, Rabbani ZN, Liu Y, Reddy SK, Su Z, et al. RNA Aptamer-targeted inhibition of NF-kappa B suppresses non-small cell lung cancer resistance to doxorubicin. Mol Ther (2008) 16(1):66–73. doi: 10.1038/sj.mt.6300320
111. Kashima H, Momose F, Umehara H, Miyoshi N, Ogo N, Muraoka D, et al. Epirubicin, identified using a novel luciferase reporter assay for Foxp3 inhibitors, inhibits regulatory T cell activity. PloS One (2016) 11(6):e0156643. doi: 10.1371/journal.pone.0156643
112. Murta EFC, de Andrade JM, Falcio RP, Bighetti S. Lymphocyte subpopulations in patients with advanced breast cancer submitted to neoadjuvant chemotherapy. Tumori J (2000) 86(5):403–7. doi: 10.1177/030089160008600507
113. Ferraro C, Quemeneur L, Fournel S, Prigent AF, Revillard JP, Bonnefoy-Berard N. The topoisomerase inhibitors camptothecin and etoposide induce a CD95-independent apoptosis of activated peripheral lymphocytes. Cell Death Differ (2000) 7(2):197–206. doi: 10.1038/sj.cdd.4400595
114. Calderoni A, Cerny T. Taxanes in lung cancer: a review with focus on the European experience. Crit Rev Oncol/Hematol (2001) 38(2):105–27. doi: 10.1016/S1040-8428(00)00121-9
115. Quartino AL, Friberg LE, Karlsson MO. A simultaneous analysis of the time-course of leukocytes and neutrophils following docetaxel administration using a semi-mechanistic myelosuppression model. Invest New Drugs (2012) 30(2):833–45. doi: 10.1007/s10637-010-9603-3
116. Cassidy MR, Wolchok RE, Zheng J, Panageas KS, Wolchok JD, Coit D, et al. Neutrophil to lymphocyte ratio is associated with outcome during ipilimumab treatment. EBioMedicine (2017) 18:56–61. doi: 10.1016/j.ebiom.2017.03.029
117. Kotsakis A, Sarra E, Peraki M, Koukourakis M, Apostolaki S, Souglakos J, et al. Docetaxel-induced lymphopenia in patients with solid tumors: a prospective phenotypic analysis. Cancer (2000) 89(6):1380–6. doi: 10.1002/1097-0142(20000915)89:6<1380::AID-CNCR23>3.0.CO;2-R
118. Tietze JK, Angelova D, Heppt MV, Reinholz M, Murphy WJ, Spannagl M, et al. The proportion of circulating CD45RO(+)CD8(+) memory T cells is correlated with clinical response in melanoma patients treated with ipilimumab. Eur J Cancer (2017) 75:268–79. doi: 10.1016/j.ejca.2016.12.031
119. Tsavaris N, Kosmas C, Vadiaka M, Kanelopoulos P, Boulamatsis D. Immune changes in patients with advanced breast cancer undergoing chemotherapy with taxanes. Br J Cancer (2002) 87(1):21–7. doi: 10.1038/sj.bjc.6600347
120. Chuang LT, Lotzová E, Heath J, Cook KR, Munkarah A, Morris M, et al. Alteration of lymphocyte microtubule assembly, cytotoxicity, and activation by the anticancer drug taxol. Cancer Res (1994) 54(5):1286–91.
121. Tong AW, Seamour B, Lawson JM, Ordonez G, Vukelja S, Hyman W, et al. Cellular immune profile of patients with advanced cancer before and after taxane treatment. Am J Clin Oncol (2000) 23(5):463–72. doi: 10.1097/00000421-200010000-00007
122. John J, Ismail M, Riley C, Askham J, Morgan R, Melcher A, et al. Differential effects of paclitaxel on dendritic cell function. BMC Immunol (2010) 11(1):14. doi: 10.1186/1471-2172-11-14
123. Zhang L, Dermawan K, Jin M, Liu R, Zheng H, Xu L, et al. Differential impairment of regulatory T cells rather than effector T cells by paclitaxel-based chemotherapy. Clin Immunol (2008) 129(2):219–29. doi: 10.1016/j.clim.2008.07.013
124. Kodumudi KN, Woan K, Gilvary DL, Sahakian E, Wei S, Djeu JY. A novel chemoimmunomodulating property of docetaxel: Suppression of myeloid-derived suppressor cells in tumor bearers. Clin Cancer Res (2010) 16(18):4583. doi: 10.1158/1078-0432.CCR-10-0733
125. Buhtoiarov IN, Sondel PM, Wigginton JM, Buhtoiarova TN, Yanke EM, Mahvi DA, et al. Anti-tumour synergy of cytotoxic chemotherapy and anti-CD40 plus CpG-ODN immunotherapy through repolarization of tumour-associated macrophages. Immunol (2011) 132(2):226–39. doi: 10.1111/j.1365-2567.2010.03357.x
126. Liu P, Zhao L, Pol J, Levesque S, Petrazzuolo A, Pfirschke C, et al. Crizotinib-induced immunogenic cell death in non-small cell lung cancer. Nat Commun (2019) 10(1):1486. doi: 10.1038/s41467-019-09415-3
127. Peng J, Hamanishi J, Matsumura N, Abiko K, Murat K, Baba T, et al. Chemotherapy induces programmed cell death-ligand 1 overexpression via the nuclear factor-κB to foster an immunosuppressive tumor microenvironment in ovarian cancer. Cancer Res (2015) 75(23):5034–45. doi: 10.1158/0008-5472.CAN-14-3098
128. Grigg C, Rizvi NA. PD-L1 biomarker testing for non-small cell lung cancer: truth or fiction? J ImmunoTher Cancer (2016) 4(1):48. doi: 10.1186/s40425-016-0153-x
129. Loman BR, Jordan KR, Haynes B, Bailey MT, Pyter LM. Chemotherapy-induced neuroinflammation is associated with disrupted colonic and bacterial homeostasis in female mice. Sci Rep (2019) 9(1):16490. doi: 10.1038/s41598-019-52893-0
130. Ramakrishna C, Corleto J, Ruegger PM, Logan GD, Peacock BB, Mendonca S, et al. Dominant role of the gut microbiota in chemotherapy induced neuropathic pain. Sci Rep (2019) 9(1):20324. doi: 10.1038/s41598-019-56832-x
131. Alexander JL, Wilson ID, Teare J, Marchesi JR, Nicholson JK, Kinross JM. Gut microbiota modulation of chemotherapy efficacy and toxicity. Nat Rev Gastroenterol Hepatol (2017) 14(6):356–65. doi: 10.1038/nrgastro.2017.20
132. Lee YT, Tan YJ, Oon CE. Molecular targeted therapy: Treating cancer with specificity. Eur J Pharmacol (2018) 834:188–96. doi: 10.1016/j.ejphar.2018.07.034
133. Ciccolini J, Serdjebi C, Peters GJ, Giovannetti E. Pharmacokinetics and pharmacogenetics of gemcitabine as a mainstay in adult and pediatric oncology: an EORTC-PAMM perspective. Cancer Chemother Pharmacol (2016) 78(1):1–12. doi: 10.1007/s00280-016-3003-0
134. Weir GM, Liwski RS, Mansour M. Immune modulation by chemotherapy or immunotherapy to enhance cancer vaccines. Cancers (Basel) (2011) 3(3):3114–42. doi: 10.3390/cancers3033114
135. Homma Y, Taniguchi K, Nakazawa M, Matsuyama R, Mori R, Takeda K, et al. Changes in the immune cell population and cell proliferation in peripheral blood after gemcitabine-based chemotherapy for pancreatic cancer. Clin Trans Oncol (2014) 16(3):330–5. doi: 10.1007/s12094-013-1079-0
136. Galetto A, Buttiglieri S, Forno S, Moro F, Mussa A, Matera L. Drug- and cell-mediated antitumor cytotoxicities modulate cross-presentation of tumor antigens by myeloid dendritic cells. Anticancer Drugs (2003) 14(10):833–43. doi: 10.1097/00001813-200311000-00010
137. Bruchard M, Mignot G, Derangère V, Chalmin F, Chevriaux A, Végran F, et al. Chemotherapy-triggered cathepsin b release in myeloid-derived suppressor cells activates the Nlrp3 inflammasome and promotes tumor growth. Nat Med (2013) 19(1):57–64. doi: 10.1038/nm.2999
138. Vincent J, Mignot G, Chalmin F, Ladoire S, Bruchard M, Chevriaux A, et al. 5-fluorouracil selectively kills tumor-associated myeloid-derived suppressor cells resulting in enhanced T cell-dependent antitumor immunity. Cancer Res (2010) 70(8):3052–61. doi: 10.1158/0008-5472.CAN-09-3690
139. Grosflam J, Weinblatt ME. Methotrexate: mechanism of action, pharmacokinetics, clinical indications, and toxicity. Curr Opin Rheumatol (1991) 3(3):363–8. doi: 10.1097/00002281-199106000-00006
140. Kaneno R, Shurin GV, Tourkova IL, Shurin MR. Chemomodulation of human dendritic cell function by antineoplastic agents in low noncytotoxic concentrations. J Trans Med (2009) 7(1):58. doi: 10.1186/1479-5876-7-58
141. Assaraf YG. Molecular basis of antifolate resistance. Cancer Metastasis Rev (2007) 26(1):153–81. doi: 10.1007/s10555-007-9049-z
142. Gonen N, Assaraf YG. Antifolates in cancer therapy: Structure, activity and mechanisms of drug resistance. Drug Resistance Updates (2012) 15(4):183–210. doi: 10.1016/j.drup.2012.07.002
143. Tomasini P, Barlesi F, Mascaux C, Greillier L. Pemetrexed for advanced stage nonsquamous non-small cell lung cancer: latest evidence about its extended use and outcomes. Ther Adv Med Oncol (2016) 8(3):198–208. doi: 10.1177/1758834016644155
144. Novosiadly R, Schaer D, Amaladas N, Rasmussen E, Lu ZH, Sonyi A, et al. Abstract 4549: Pemetrexed enhances anti-tumor efficacy of PD1 pathway blockade by promoting intra tumor immune response via immunogenic tumor cell death and T cell intrinsic mechanisms. Cancer Res (2018) 78(13 Supplement):4549. doi: 10.1158/1538-7445.AM2018-4549
145. Boutros C, Tarhini A, Routier E, Lambotte O, Ladurie FL, Carbonnel F, et al. Safety profiles of anti-CTLA-4 and anti-PD-1 antibodies alone and in combination. Nat Rev Clin Oncol (2016) 13(8):473–86. doi: 10.1038/nrclinonc.2016.58
146. Yan Y, Kumar AB, Finnes H, Markovic SN, Park S, Dronca RS, et al. Combining immune checkpoint inhibitors with conventional cancer therapy. Front Immunol (2018) 9:1739. doi: 10.3389/fimmu.2018.01739
147. Weiss GJ, Waypa J, Blaydorn L, Coats J, McGahey K, Sangal A, et al. A phase ib study of pembrolizumab plus chemotherapy in patients with advanced cancer (PembroPlus). Br J Cancer (2017) 117(1):33–40. doi: 10.1038/bjc.2017.145
148. Obeid E, Zhou C, Macfarlane A, Alpaugh RK, McAleer C, Dulaimi E, et al. Combining chemotherapy and programmed death 1 (PD-1) blockade to induce a T-cell response in patients with metastatic triple negative breast cancer (mTNBC). J Clin Oncol (2017) 35(15_suppl):11563–. doi: 10.1200/JCO.2017.35.15_suppl.11563
149. Tolaney SM, Kalinsky K, Kaklamani V, Savulsky C, Olivo M, Aktan G, et al. Abstract PD6-13: Phase 1b/2 study to evaluate, triplenegative emicwpipwm, Supplement):PD6-13–PD6-13. Cancer Res (2018) 78(4_Supplement). doi: 10.1158/1538-7445.SABCS17-PD6-13
150. Page DB, Kim IK, Sanchez K, Moxon N, Mellinger SL, Conlin AK, et al. Safety and efficacy of pembrolizumab (pembro) plus. JCO (2018) 36(15_suppl):1033. doi: 10.1200/JCO.2018.36.15_suppl.1033
151. Bhatti S HJ, Lehn C, et al. Safety and efficacy study of pembrolizumab (MK-3475) in combination with chemotherapy as neoadjuvant treatment for participants with triple. NBCTM–KjPO (2016). Available at: https://clinicaltrials.gov/ct2/show/NCT02622074
152. Garrido-Castro AC, Barry WT, Traina TA, Wesolowski R, Tung NM, Keenan T, et al. A randomized phase II trial of carboplatin with. TJCO (2018) 36(15_suppl):TPS1118–TPS1118. doi: 10.1200/JCO.2018.36.15_suppl.TPS1118
153. Landry CA, Guziel J, Ru M, Shapiro CL, Fasano J, Bhardwaj AS, et al. A phase ib study evaluating the safety and tolerability of, breast dicweipwH-nm. JCO (2018) 36(15_suppl):TPS3116–TPS3116. doi: 10.1200/JCO.2018.36.15_suppl.TPS3116
154. Loibl S, Untch M, Burchardi N, Huober J, Blohmer JU, Grischke EM, et al. Randomized phase II neoadjuvant study, containing gtitaodtat-a. TA (2018) 154:145114.
155. Adams S, Diamond JR, Hamilton EP, Pohlmann PR, Tolaney SM, Molinero L, et al. Phase ib trial of atezolizumab in combination with. Clin J (2016) 34(15_suppl):1009. O. doi: 10.1200/JCO.2016.34.15_suppl.1009
156. Ozaki Y, Matsumoto K, Takahashi M, Mukohara T, Futamura M, Masuda N, et al. Phase II study of a combination therapy of, nivolumab bapipwH-nmb, 33, cancer as a first-line treatment. JCO (2018) 36(15_suppl):TPS1110–TPS1110. doi: 10.1200/JCO.2018.36.15_suppl.TPS1110
157. Nishina T, Hironaka S, Kadowaki S, Yamanaka T, Nakajima TE. An investigator initiated multicenter phase I/II study, of paclitaxel rwnats-ltipw. JCO (2018) 36(15_suppl):TPS4131–TPS4131. doi: 10.1200/JCO.2018.36.15_suppl.TPS4131
158. Bang Y-J, Muro K, Fuchs CS, Golan T, Geva R, Hara H, et al. KEYNOTE-059 cohort 2: Safety and efficacy of, treatment ppp-f-Facff-lL. JCO (2017) 35(15):4012. doi: 10.1200/JCO.2017.35.15_suppl.4012
160. Fujimoto N, Aoe K, Kozuki T, Oze I, Kato K, Kishimoto T, et al. A phase II trial of first-line combination chemotherapy, with cisplatin p, and nivolumab for unresectable malignant pleural. CLC (2018) 19(5):e705–7. doi: 10.1016/j.cllc.2018.05.001
161. Hersh EM, O’Day SJ, Powderly J, Khan KD, Pavlick AC, Cranmer LD, et al. A phase II multicenter study of ipilimumab with or. New ID (2011) 29(3):489–98. doi: 10.1007/s10637-009-9376-8
162. Robert C, Thomas L, Bondarenko I, O'Day S, Weber J, Garbe C, et al. Ipilimumab plus dacarbazine for previously. NEJM (2011) 364(26):2517–26. doi: 10.1056/NEJMoa1104621
163. Vera Aguilera J, Paludo J, Bangalore A, Failing J, McWilliams RR, Kottschade LA, et al. Chemoimmunotherapy combination after PD-1. Oncol JC (2018) 36(15_suppl):9558. doi: 10.1200/JCO.2018.36.15_suppl.9558
164. Paz-Ares L, Luft A, Vicente D, Tafreshi A, Gümüş M, Mazières J, et al. Pembrolizumab plus chemotherapy for squamous non. NEJM (2018) 379(21):2040–51. doi: 10.1056/NEJMoa1810865
165. Sistigu A, Viaud S, Chaput N, Bracci L, Proietti E, Zitvogel L. Immunomodulatory effects of cyclophosphamide and. SI (2011) 33(4):369–83. doi: 10.1007/s00281-011-0245-0
166. Gandhi L, Rodriguez-Abreu D, Gadgeel S, Esteban E, Felip E, Angelis FD, et al. Pembrolizumab plus chemotherapy in. NEJM (2018) 378(22):2078–92. doi: 10.1056/NEJMoa1801005
167. Langer CJ, Gadgeel SM, Borghaei H, Papadimitrakopoulou VA, Patnaik A, Powell SF, et al. Carboplatin and pemetrexed with or without, pembrolizumab for advanced n-sn-s-clcar, phase. LO (2016) 17(11):1497–508. doi: 10.1016/S1470-2045(16)30498-3
168. Papadimitrakopoulou V, Gadgeel SM, Borghaei H, Gandhi L, Patnaik A, Powell SF, et al. First-line carboplatin and pemetrexed, updated CwowppfanN. JCO (2017) 35(15_suppl):9094. doi: 10.1200/JCO.2017.35.15_suppl.9094
169. Evans NR, Cowan S, Solomides C, Hooper DC, Harshyne L, Lu-Yao GL, et al. Nivolumab plus cisplatin/pemetrexed or. NJCO (2018) 36(15_suppl):TPS8582–TPS8582. doi: 10.1200/JCO.2018.36.15_suppl.TPS8582
170. Rizvi NA, Hellmann M, Brahmer JR, Juergens RA, Borghaei H, Gettinger S, et al. Nivolumab in combination with platinum-based. JCO (2016) 34(25):2969–79. doi: 10.1200/JCO.2016.66.9861
171. Kanda S, Goto K, Shiraishi H, Kubo E, Tanaka A, Utsumi H, Sunami K, et al. Safety and efficacy of nivolumab and standard. AO (2016) 27(12):2242–50. doi: 10.1093/annonc/mdw416
172. Govindan R, Szczesna A, Ahn MJ, Schneider CP, Gonzalez Mella PF, Barlesi F, et al. Phase III trial of ipilimumab combined with paclitaxel and carboplatin in advanced squamous non-Small-Cell lung cancer. J Clin Oncol (2017) 35(30):3449–57. doi: 10.1200/JCO.2016.71.7629
173. Lynch TJ, Bondarenko I, Luft A, Serwatowski P, Barlesi F, Chacko R, et al. Ipilimumab in combination with paclitaxel and carboplatin as first-line treatment in stage IIIB/IV non-small-cell lung cancer: results from a randomized, double-blind, multicenter phase II study. J Clin Oncol (2012) 30(17):2046–54. doi: 10.1200/JCO.2011.38.4032
174. Reck M, Socinski M, Cappuzzo F, Orlandi F, Stroyakovskii D, Nogami N, Rodríguez-Abreu D, et al. Primary PFS and safety analyses of a randomized, phase III study of carboplatin + paclitaxel +/– bevacizumab wowai. IAO (2017) 28(0):mdx760.002. doi: 10.1093/annonc/mdx760.002
175. Horn L, Mansfield AS, Szczęsna A, Havel L, Krzakowski M, Hochmair MJ, et al. First-line atezolizumab plus chemotherapy in extensive-stage small-cell lung cancer. New Engl J Med (2018) 379(23):2220–9. doi: 10.1056/NEJMoa1809064
176. Liu SV, Camidge DR, Gettinger SN, Giaccone G, Heist RS, Hodi FS, et al. Long-term survival follow-up of atezolizumab in combination with platinum-based doublet chemotherapy in patients with advanced non–small-cell lung cancer. Eur J Cancer (2018) 101:114–22. doi: 10.1016/j.ejca.2018.06.033
177. Chawla S, Sankhala K, Ravicz J, Kang G, Liu S, Stumpf N, et al. Clinical experience with combination chemo-. Clin JO (2018) 36(15_suppl):e23568–8. doi: 10.1200/JCO.2018.36.15_suppl.e23568
178. Galsky MD, Wang H, Hahn NM, Twardowski P, Pal SK, Albany C, et al. Phase 2 trial of gemcitabine, cisplatin, plus ipilimumab in patients with metastatic urothelial cancer and impact of DNA damage response gene mutations on outcomes. Eur Urol (2018) 73(5):751–9. doi: 10.1016/j.eururo.2017.12.001
179. Parikh M, Pan CX, Beckett LA, Li Y, Robles DA, Aujla PK, et al. Pembrolizumab combined with either docetaxel or gemcitabine in patients with advanced or metastatic platinum-refractory urothelial cancer: Results from a phase I study. Clin Genitourin Cancer (2018) 16(6):421–8.e1. doi: 10.1016/j.clgc.2018.07.004
180. Langer CJ, Gadgeel SM, Borghaei H, Papadimitrakopoulou VA, Patnaik A, Powell SF, et al. Carboplatin and pemetrexed with or without pembrolizumab for advanced, non-squamous non-small-cell lung cancer: a randomised, phase 2 cohort of the open-label KEYNOTE-021 study. Lancet Oncol (2016) 17(11):1497–508. doi: 10.1016/S1470-2045(16)30498-3
181. Borghaei H, Langer CJ, Gadgeel S, Papadimitrakopoulou VA, Patnaik A, Powell SF, et al. 24-month overall survival from KEYNOTE-021 cohort G: Pemetrexed and carboplatin with or without pembrolizumab as first-line therapy for advanced nonsquamous non-small cell lung cancer. J Thorac Oncol (2019) 14(1):124–9. doi: 10.1016/j.jtho.2018.08.004
182. Gandhi L, Rodríguez-Abreu D, Gadgeel S, Esteban E, Felip E, De Angelis F, et al. Pembrolizumab plus chemotherapy in metastatic non-Small-Cell lung cancer. N Engl J Med (2018) 378(22):2078–92. doi: 10.1056/NEJMoa1801005
183. Antonia SJ, Villegas A, Daniel D, Vicente D, Murakami S, Hui R, et al. Durvalumab after chemoradiotherapy in stage III non–Small-Cell lung cancer. New Engl J Med (2017) 377(20):1919–29. doi: 10.1056/NEJMoa1709937
184. Socinski MA, Jotte RM, Cappuzzo F, Orlandi F, Stroyakovskiy D, Nogami N, et al. Atezolizumab for first-line treatment of metastatic nonsquamous NSCLC. New Engl J Med (2018) 378(24):2288–301. doi: 10.1056/NEJMoa1716948
185. Voorwerk L, Slagter M, Horlings HM, Sikorska K, van de Vijver KK, de Maaker M, et al. Immune induction strategies in metastatic triple-negative breast cancer to enhance the sensitivity to PD-1 blockade: the TONIC trial. Nat Med (2019) 25(6):920–8. doi: 10.1038/s41591-019-0432-4
186. Demaria S, Romano E, Brackstone M, Formenti SC. Immune induction strategies to enhance responses to PD-1 blockade: lessons from the TONIC trial. J ImmunoTher Cancer (2019) 7(1):318. doi: 10.1186/s40425-019-0783-x
187. Markham A, Keam SJ. Camrelizumab: first global approval. Drugs (2019) 79(12):1355–61. doi: 10.1007/s40265-019-01167-0
188. Fang W, Yang Y, Ma Y, Hong S, Lin L, He X, et al. Camrelizumab (SHR-1210) alone or in combination with gemcitabine plus cisplatin for nasopharyngeal carcinoma: results from two single-arm, phase 1 trials. Lancet Oncol (2018) 19(10):1338–50. doi: 10.1016/S1470-2045(18)30495-9
189. Peters S, Gettinger S, Johnson ML, Jänne PA, Garassino MC, Christoph D, et al. Phase II trial of atezolizumab as first-line or subsequent therapy for patients with programmed death-ligand 1-selected advanced non-Small-Cell lung cancer (BIRCH). J Clin Oncol (2017) 35(24):2781–9. doi: 10.1200/JCO.2016.71.9476
190. Garassino MC, Cho BC, Kim JH, Mazières J, Vansteenkiste J, Lena H, et al. Durvalumab as third-line or later treatment for advanced non-small-cell lung cancer (ATLANTIC): an open-label, single-arm, phase 2 study. Lancet Oncol (2018) 19(4):521–36. doi: 10.1016/S1470-2045(18)30144-X
191. Lesterhuis WJ, Salmons J, Nowak AK, Rozali EN, Khong A, Dick IM, et al. Synergistic effect of CTLA-4 blockade and cancer chemotherapy in the induction of anti-tumor immunity. PloS One (2013) 8(4):e61895. doi: 10.1371/journal.pone.0061895
192. Iida Y, Harashima N, Motoshima T, Komohara Y, Eto M, Harada M. Contrasting effects of cyclophosphamide on anti-CTL-associated protein 4 blockade therapy in two mouse tumor models. Cancer Sci (2017) 108(10):1974–84. doi: 10.1111/cas.13337
193. Kok M, Voorwerk L, Horlings H, Sikorska K, van der Vijver K, Slagter M, et al. Adaptive phase II randomized trial of nivolumab after induction treatment in triple negative breast cancer (TONIC trial): Final response data stage I and first translational data. J Clin Oncol (2018) 36(15_suppl):1012–. doi: 10.1200/JCO.2018.36.15_suppl.1012
194. Gray A, de la Luz Garcia-Hernandez M, van West M, Kanodia S, Hubby B, Kast WM. Prostate cancer immunotherapy yields superior long-term survival in TRAMP mice when administered at an early stage of carcinogenesis prior to the establishment of tumor-associated immunosuppression at later stages. Vaccine (2009) 27 Suppl 6:G52–9. doi: 10.1016/j.vaccine.2009.09.106
195. Pasch CA, Favreau PF, Yueh AE, Babiarz CP, Gillette AA, Sharick JT, et al. Patient-derived cancer organoid cultures to predict sensitivity to chemotherapy and radiation. Clin Cancer Res (2019) 25(17):5376–87. doi: 10.1158/1078-0432.CCR-18-3590
196. Jenkins RW, Aref AR, Lizotte PH, Ivanova E, Stinson S, Zhou CW, et al. Ex vivo profiling of PD-1 blockade using organotypic tumor SpheroidsEx vivo profiling of immune checkpoint blockade. Cancer discovery (2018) 8(2):196–215. doi: 10.1158/2159-8290.CD-17-0833
197. Yuki K, Cheng N, Nakano M, Kuo CJ. Organoid models of tumor immunology. Trends Immunol (2020) 41(8):652–64. doi: 10.1016/j.it.2020.06.010
198. Nelson MA, Ngamcherdtrakul W, Luoh S-W, Yantasee W. Prognostic and therapeutic role of tumor-infiltrating lymphocyte subtypes in breast cancer. Cancer Metastasis Rev (2021) 40(2):519–36. doi: 10.1007/s10555-021-09968-0
199. Pocaterra A, Catucci M, Mondino A. Adoptive T cell therapy of solid tumors: time to team up with immunogenic chemo/radiotherapy. Curr Opin Immunol (2022) 74:53–9. doi: 10.1016/j.coi.2021.10.004
200. Truong NT, Gargett T, Brown MP, Ebert LM. Effects of chemotherapy agents on circulating leukocyte populations: Potential implications for the success of CAR-T cell therapies. Cancers (2021) 13(9):2225. doi: 10.3390/cancers13092225
201. Ramakrishnan R, Assudani D, Nagaraj S, Hunter T, Cho H-I, Antonia S, et al. Chemotherapy enhances tumor cell susceptibility to CTL-mediated killing during cancer immunotherapy in mice. J Clin Invest (2010) 120(4):1111–24. doi: 10.1172/JCI40269
202. Alizadeh D, Trad M, Hanke NT, Larmonier CB, Janikashvili N, Bonnotte B, et al. Doxorubicin eliminates myeloid-derived suppressor cells and enhances the efficacy of adoptive T-cell transfer in breast CancerDoxorubicin eliminates MDSC. Cancer Res (2014) 74(1):104–18. doi: 10.1158/0008-5472.CAN-13-1545
203. Petrizzo A, Mauriello A, Luciano A, Rea D, Barbieri A, Arra C, et al. Inhibition of tumor growth by cancer vaccine combined with metronomic chemotherapy and anti-PD-1 in a pre-clinical setting. Oncotarget (2018) 9(3):3576. doi: 10.18632/oncotarget.23181
204. Chen C-A, Ho C-M, Chang M-C, Sun W-Z, Chen Y-L, Chiang Y-C, et al. Metronomic chemotherapy enhances antitumor effects of cancer vaccine by depleting regulatory T lymphocytes and inhibiting tumor angiogenesis. Mol Ther (2010) 18(6):1233–43. doi: 10.1038/mt.2010.34
205. Tagliamonte M, Petrizzo A, Napolitano M, Luciano A, Arra C, Maiolino P, et al. Novel metronomic chemotherapy and cancer vaccine combinatorial strategy for hepatocellular carcinoma in a mouse model. Cancer Immunol Immunother (2015) 64(10):1305–14. doi: 10.1007/s00262-015-1698-0
206. Kazemi MH, Dehaghi BK, Roshandel E, Parkhideh S, Mehdizadeh M, Salimi M, et al. Oncolytic virotherapy in hematopoietic stem cell transplantation. Hum Immunol (2021) 82(9):640–8. doi: 10.1016/j.humimm.2021.05.007
Keywords: combination therapy, chemotherapy, immune checkpoint inhibitors, tumor microenvironment, cancer treatment
Citation: Zhang L, Zhou C, Zhang S, Chen X, Liu J, Xu F and Liang W (2022) Chemotherapy reinforces anti-tumor immune response and enhances clinical efficacy of immune checkpoint inhibitors. Front. Oncol. 12:939249. doi: 10.3389/fonc.2022.939249
Received: 08 May 2022; Accepted: 04 July 2022;
Published: 08 August 2022.
Edited by:
Eyad Elkord, University of Salford, United KingdomReviewed by:
Leticia Rocha-Zavaleta, National Autonomous University of Mexico, MexicoAnubhab Mukherjee, Esperer Onco Nutrition Pvt. Ltd., India
Copyright © 2022 Zhang, Zhou, Zhang, Chen, Liu, Xu and Liang. This is an open-access article distributed under the terms of the Creative Commons Attribution License (CC BY). The use, distribution or reproduction in other forums is permitted, provided the original author(s) and the copyright owner(s) are credited and that the original publication in this journal is cited, in accordance with accepted academic practice. No use, distribution or reproduction is permitted which does not comply with these terms.
*Correspondence: Fangming Xu, ZmFybXh1QDEyNi5jb20=; Wenqing Liang, bGlhbmd3cUB1c3guZWR1LmNu
†These authors have contributed equally to this work