- 1Laboratorio de Plasticidad Metabólica y Bioenergética, Programa de Farmacología Clínica y Molecular, Instituto de Ciencias Biomédicas, Facultad de Medicina, Universidad de Chile, Santiago, Chile
- 2Network for Snake Venom Research and Drug Discovery, Santiago, Chile
- 3Interdisciplinary Group on Mitochondrial Targeting and Bioenergetics (MIBI), Talca, Chile
- 4Laboratorio de Biología Molecular, Facultad de Ciencias Biológicas, Universidad Nacional Mayor de San Marcos, Ciudad Universitaria, Lima, Peru
- 5Laboratory of Biochemistry of Proteins from Animal Venoms, Research and Development Center, Ezequiel Dias Foundation, Belo Horizonte, Brazil
- 6Laboratorio de Productos Bioactivos, Instituto de Química de Recursos Naturales, Universidad de Talca, Talca, Chile
Beyond the role of mitochondria in apoptosis initiation/execution, some mitochondrial adaptations support the metastasis and chemoresistance of cancer cells. This highlights mitochondria as a promising target for new anticancer strategies. Emergent evidence suggests that some snake venom toxins, both proteins with enzymatic and non-enzymatic activities, act on the mitochondrial metabolism of cancer cells, exhibiting unique and novel mechanisms that are not yet fully understood. Currently, six toxin classes (L-amino acid oxidases, thrombin-like enzymes, secreted phospholipases A2, three-finger toxins, cysteine-rich secreted proteins, and snake C-type lectin) that alter the mitochondrial bioenergetics have been described. These toxins act through Complex IV activity inhibition, OXPHOS uncoupling, ROS-mediated permeabilization of inner mitochondrial membrane (IMM), IMM reorganization by cardiolipin interaction, and mitochondrial fragmentation with selective migrastatic and cytotoxic effects on cancer cells. Notably, selective internalization and direct action of snake venom toxins on tumor mitochondria can be mediated by cell surface proteins overexpressed in cancer cells (e.g. nucleolin and heparan sulfate proteoglycans) or facilitated by the elevated Δψm of cancer cells compared to that non-tumor cells. In this latter case, selective mitochondrial accumulation, in a Δψm-dependent manner, of compounds linked to cationic snake peptides may be explored as a new anti-cancer drug delivery system. This review analyzes the effect of snake venom toxins on mitochondrial bioenergetics of cancer cells, whose mechanisms of action may offer the opportunity to develop new anticancer drugs based on toxin scaffolds.
Introduction
Energy requirements of cancer cells vary significantly from normal cells; they exhibit different metabolic phenotypes with dynamic contributions of both oxidative phosphorylation (OXPHOS) and glycolysis (1) in a process known as metabolic plasticity (2). Notably, a switch to mitochondrial metabolism correlates with metastatic abilities (3, 4) and chemoresistance using alternative energy substrates such as lactate and glutamine (5, 6). Beyond the role of mitochondria in apoptosis initiation/execution, several mitochondrial adaptations support the migration and invasion of cancer cells such as enhanced Complex I activity-dependent reactive oxygen species (ROS) production (3, 4, 7), mitochondrial localization in focal adhesions for local ATP synthesis required for actin cytoskeleton reorganization (8, 9), and high tricarboxylic acid (TCA) cycle activity (10, 11). All these characteristics are associated with more invasiveness and motility (12) and highlight mitochondria as a promising target for metastasis treatment (13).
Snake venoms are a natural source of active proteins, also known as toxins, which exhibit enzymatic or non-enzymatic activities, and are useful molecular scaffolds to develop agents with high affinity and selectivity on cancer cell models in vitro and in vivo (14–17). Currently, at least nine classes of snake toxins are recognized by suppressing the cancer hallmarks such as ROS-dependent DNA damage, blockage of extracellular matrix-integrin signaling, disruption of cytoskeleton network, inhibition of growth factor-dependent signaling, and induction of OXPHOS dysfunction (13, 18–20). Notably, emergent evidence suggests that several of these toxins act on the mitochondrial metabolism of cancer cells (21–24), exhibiting unique and novel mechanisms that are not yet fully understood. This offers the opportunity to develop new anticancer drugs based on toxin scaffolds. This review analyzes the effect of snake venom toxins on mitochondrial bioenergetics in cancer cells.
Snake Toxins That Target Mitochondrial Bioenergetics in Cancer Cells
Six classes of snake venom toxins with effects on mitochondrial bioenergetics of cancer cells have been studied (Table 1). Although the anti-cancer mechanisms of action are not yet fully understood, different determinants may influence the induction of mitochondrial dysfunction such as the site of toxin-cell (e.g. extra or intra-cellular) interaction, physical-chemistry toxin characteristics (e.g. positive charge) to facilitate mitochondrial uptake, and grade of intervention of the mitochondrial metabolism (e.g. permeabilization of the mitochondrial inner membrane, OXPHOS uncoupling, inhibition of respiration, between others). Below, these toxins are discussed in detail (Figure 1).
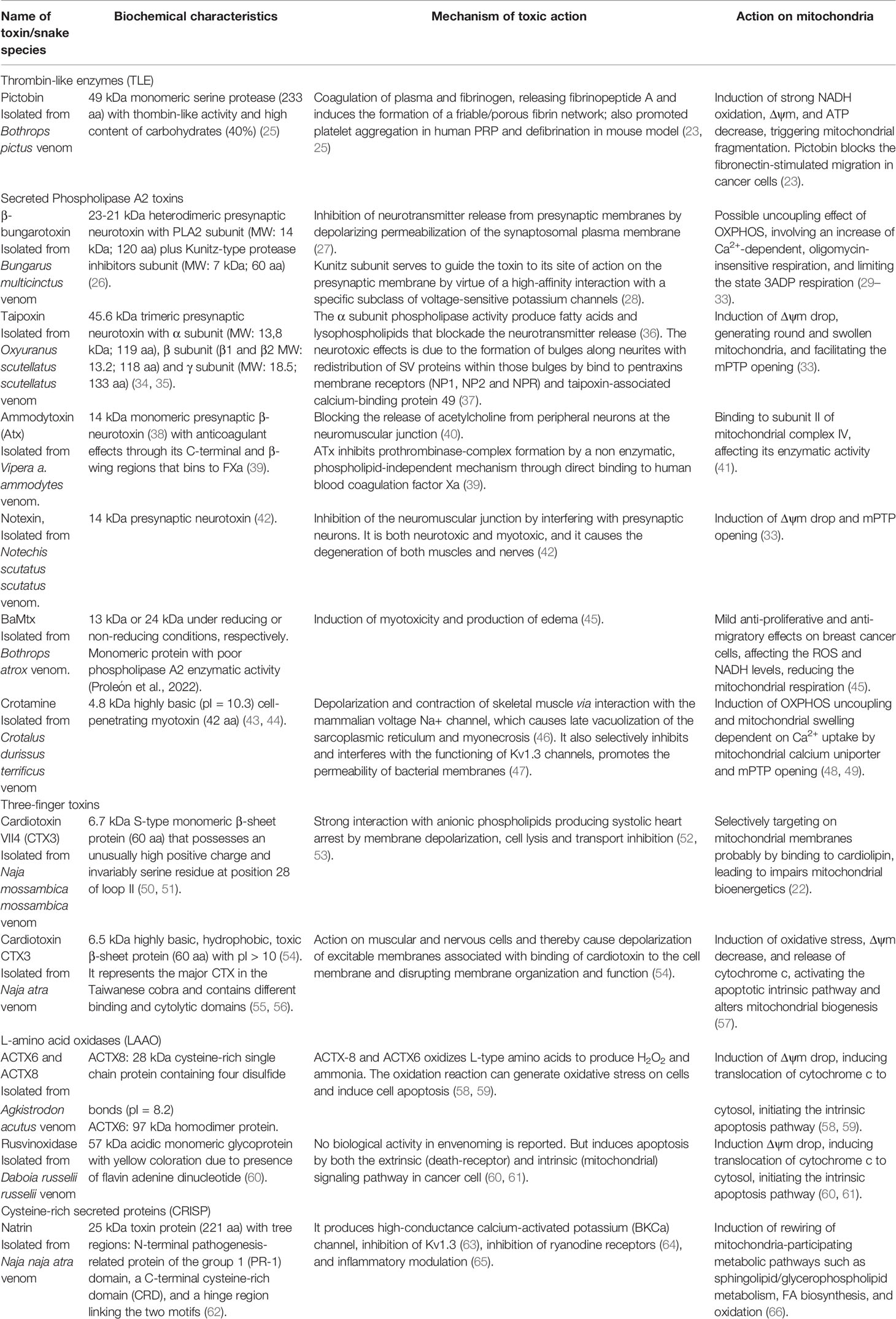
Table 1 Toxicological mechanisms of snake venom toxins with effects on mitochondrial bioenergetics of cancer cells.
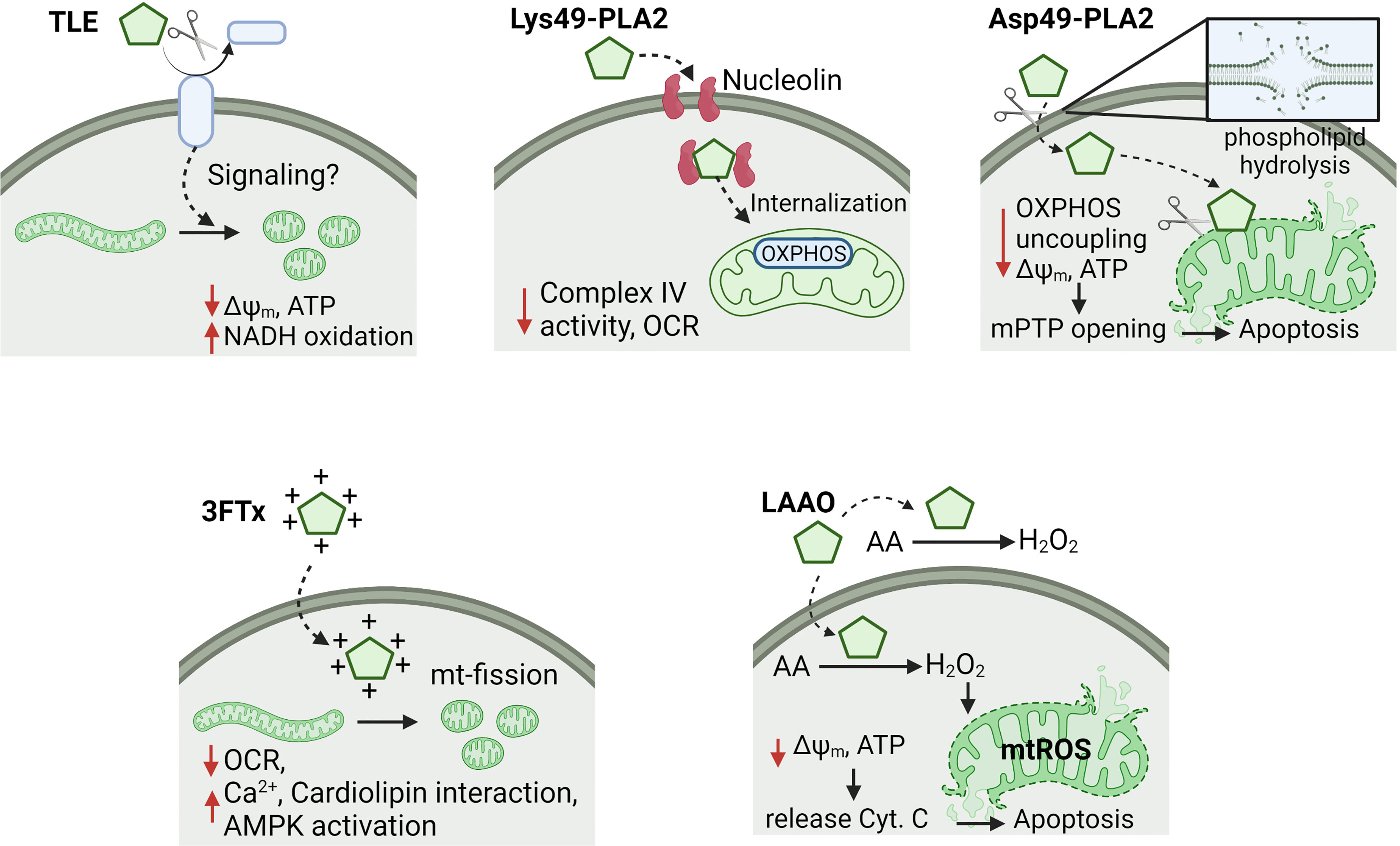
Figure 1 Effects of snake toxins on mitochondrial bioenergetics in cancer cells. It is represented the mechanisms of action of thrombin-like enzymes (TLE), secreted Lys49- and Asp49-phospholipases A2 (PLA2), three-finger toxins (3FTx) and L-amino acid oxidases (LAAO). Some toxins affect the oxidative phosphorylation (OXPHOS) by uncoupling or Complex IV inhibition, which decreases the mitochondrial membrane potential (Δψm) and ATP levels. Moreover, other toxins produce mitochondrial fragmentation (e.g. TLE and 3FTx) or permeabilization of inner mitochondrial membrane (e.g. Asp49-PLA2 and LAAO), triggering apoptosis. Cysteine-rich secreted proteins, and snake C-type lectin toxins were excluded of this figure due to reduced information of a putative mechanism of action on mitochondria. OCR, oxygen consumption rate; Cyt. C, cytochrome C; mPTP, mitochondrial permeability transition pore; mtROS, mitochondrial ROS; AA, amino acids; mt-fission, mitochondrial fission.
Thrombin-Like Enzymes
Thrombin-like enzymes (TLEs) are serine proteases belonging to family S1 (chymotrypsin), subfamily A of the trypsin-like group (67). Its active site (His57, Asp102, Ser195) catalytically releases fibrinopeptide A or B from Aα or Bβ fibrinogen’s chain cleavage, respectively, but rarely both fibrinopeptides, as thrombin (68). The molecular mass of TLEs varies between 26 and 67 kDa, depending on the content of its carbohydrates (N- or O-linked) (69, 70). The tertiary structure shows a β/β hydrolase type fold with variants in the shape of certain regions (mainly loops) that attribute particular functional mechanisms (71). TLEs have only up to 33% homology with thrombin and differ with it in 1) preference for fibrinogen chain cleavage; 2) absence of allosteric modulators; 3) insensitivity to antithrombin III, hirudin, and heparin, and 4) do not activate another coagulation factor (72). TLEs produce a coagulating effect in vitro; however, in vivo, form atypical fibrin meshes that are quickly degraded by secondary fibrinogenolytic processes, therefore, endogenous fibrinogen decreases considerably, making the blood uncoagulable (73). Additionally, TLEs produce alterations in the nervous system (74), complement system (75), muscle (76), and potassium channel blocking (77).
Pictobin is a 41-kDa glycoproteinase that coagulates plasma and fibrinogen, releasing fibrinopeptide A and promoting the formation of a friable/porous fibrin network (23). Platelets and cancer cells can interact between them or with extracellular cues to modify their reactivity or metastatic ability, respectively (13, 78). In this context, there is experimental evidence that pictobin triggers mitochondrial dysfunction in cancer cells. This toxin inhibits the fibronectin-dependent migration in lung and breast cancer cells and produces strong NADH oxidation, Δψm depolarization, ATP decrease and fragmentation of the mitochondrial network at non-cytotoxic concentrations (23). Currently, the molecular mechanism of mitochondrial dysfunction remains unknown; however, a probable intracellular signaling initiated in the plasma membrane by cleavage of some receptor in cancer cells (Figure 1), like to thrombin in platelets, may be occurring (79, 80). This may represent a novel mechanism of new anti-metastatic approaches by interfering with extracellular cues-metabolism communication (13).
Secreted Phospholipase A2 Toxins
Secreted phospholipases A2 present in some snake venoms (svPLA2s) are Ca+2-dependent enzymes that catalyze the hydrolysis of phospholipids at sn-2 positions, producing lysophospholipids and free fatty acids. Structurally, these toxins have three major α-helices and two antiparallel β-sheets linked by disulfide bonds. The residues His48, Asp49, Tyr52, and Asp99 make up the active site, coordinating Ca2+ by a hydrogen bond (81). Remarkably, svPLA2 group IIA is subdivided into two types according to their evolutionary pathway: classical PLA2s and PLA2 homologs (or PLA2-like proteins). The latter group exhibits substitutions in the catalytic domain (Asp49Lys) and the calcium-binding loop (Tyr28Asn) (82, 83), lacking phospholipase activity. Usually, they are also referred to as Lys49-PLA2s (K49-PLA2s). Notably, this svPLA2 diversity correlates with several biological actions, such as presynaptic/postsynaptic neurotoxicity, myotoxicity, cardiotoxicity (84), platelet aggregation inhibition, and edema-inducing effects (85). Moreover, antiviral effects against Dengue virus (86), antibacterial (87), antiparasitic 21), and antitumoral (88) activities have been also reported for svPLA2. A selected svPLA2 set interferes with mitochondrial bioenergetics, affecting the migration, proliferation, and viability of cancer cells, as is discussed below.
In human neuroblastoma SK-N-SH cells, notexin produces an increase in intracellular Ca2+ and ROS levels, activating p38 MAPK/ATF-2 and JNK/c-Jun signaling pathways. These, in turn, trigger the modulating expression of Bcl-2 family proteins, which produces Δψm drop, mitochondrial permeability transition pore (mPTP) opening, and consequently, intrinsic apoptosis pathway (89).
Taipoxin, notexin, and β-bungarotoxin selectively affect the mitochondria of nerve terminals (32), lacking the effects on Ca2+ transport into liver mitochondria (90). These neurotoxins induce the Ca2+ accumulation within nerve terminals, and bind the mitochondria, producing Δψm drop, generating round and swollen mitochondria, and facilitating the mPTP opening (33). Interestingly, lysophospholipid-fatty acid (FA) mixtures (two products generated by PLA2 activity) recapitulate the mitochondrial effects of these neurotoxins, suggesting a relevant role of local lipid changes in synaptic vesicle release during paralysis of the neuromuscular junction (91). Early studies on the effect of β-bungarotoxin on mitochondrial bioenergetics have suggested an uncoupling effect of OXPHOS (29–31, 90), involving an increase of Ca2+-dependent, oligomycin-insensitive respiration in synaptosomes (31). The FA released by PLA2 action at the plasma membrane, which diffuses across the cytosol and interacts with the mitochondrial inner membrane, apparently is a mechanism involved in this OXPHOS uncoupling (31). Moreover, a toxin-induced limitation in the maximal rate of electron transport (in presence of oxidable substrates such as pyruvate, glutamate, succinate, and TMPD plus ascorbate) can be observed in toxin-treated tissues, limiting the state 3ADP respiration (30). Notably, β-bungarotoxin is also a known inhibitor of α7 nicotinic acetylcholine receptors (α7nAChR) (92, 93); however, if inhibition of these receptors is involved in the observed effect on the mitochondrial bioenergetics remains currently elusive. In non-small cell lung carcinomas (NSCLC) cells under nicotine stimulation, β-bungarotoxin reduces the proliferation in vitro and tumor growth in vivo (94). Moreover, β-bungarotoxin reduces the invasion in vitro of primary cultures of cancer cells derived from 27 NSCLC tissue samples (95).
Ammodytoxin (Atx), a β-neurotoxin, is uptake intracellularly during a retrograde transport from the cell surface by binding to protein disulfide isomerase (PDI). The Atx-binding site on human PDI is located on an extensive area on its interfacial binding surface between domains b and b´ like the known binding sites of endogenous PLA2 such as the mammalian GIB, GIIA, GV, and GX PLA2s (96). In neuron cytosol, Atx binds to calmodulin and 14-3-3 proteins (97–99), which may be involved in the passage of Atx from the cytosol to nucleus and mitochondria (100). For decades R25, a 25KDa-putative mitochondrial protein, was considered as the receptor for Atx in neuronal mitochondria (101, 102). Recently, the R25 identity was revealed as a part of the subunit II of cytochrome c oxidoreductase, which is encoded by the mitochondrial MT-CO2 gene (41). In rat adrenal pheochromocytoma cells, Atx and its catalytically inactive mutant Atx(D49S) colocalize with mitochondria at 5 min of incubation and inhibit the Complex IV activity, suggesting that the PLA2 activity is dispensable for the Atx-Complex IV interaction 41). Remarkably, the subunit II of Complex IV is positioned to face the mitochondrial intermembrane space, being accessible to Atx arriving from the cytosol. The effects on mitochondrial bioenergetics and metabolic signaling pathways triggered by Complex IV inhibition by Atx still remain unknown.
Crotamine, a PLA2 with positively-charged 42 amino acids, 24) presents both cationic and hydrophobic regions that allow the interaction with negatively charged phospholipids (44). It is a cell-penetrating myotoxin that enters tumor cells by binding to cell surface heparan sulfate proteoglycans (HSPGs), triggering its endocytosis (103). This characteristic confers it the ability to cross lipid bilayers of cellular membranes and transport cargo into cells. Elegant examples of crotamine as a nanocarrier for non-viral delivery of DNA have been reported (103–105). In cancer cells, crotamine produces a rapid increase of the intracellular Ca2+ from the endoplasmic reticulum (ER) and lysosomes, Δψm depolarization, and in vivo, it induces selective penetration in proliferating cancer cells and consequently, apoptosis without toxic effect on non-tumoral tissues (48). On the other hand, crotamine promotes OXPHOS uncoupling and mitochondrial swelling dependent on Ca2+ uptake by mitochondrial calcium uniporter and mPTP opening (49). Since ER-mitochondria Ca2+ transfer is essential for cancer cell bioenergetics (106), metastasis (107), and chemoresistance (108), the anti-cancer effects of crotamine may rely on the disruption of ER-mitochondria axis and activation of Ca2+-dependent cytosolic proteases.
BaMtx is a 13 kDa Lys49-PLA2 homologue with high myotoxic activity 45). This effect is attributed to cationic residues in the conserved C-terminal region (109), which electrostatically interacts with negatively charged residues in the cellular membrane collapsing the membrane organization (110). In another Lys49-PLA2 toxins, this favors the penetration and disorganizes bilayers, leading to a Ca2+ influx that promotes selective necrosis of myotubes (111). This mechanism could be the basis for the mild anti-proliferative and anti-migratory effects showed BaMtx on breast cancer cells. A possible mechanism dependent on mitochondrial bioenergetics was recently hypothesized (45). BaMtx may increase a fast Ca2+ influx, producing oxidative stress that reduces the electron donor NADH for Complex I activity, reducing basal mitochondrial respiration and consequently the precursors required for G1/S-cell cycle transition as have been described for proliferating cancer cells (112, 113). For Lys49-PLA2, cellular uptake mediated by nucleolin is an essential step for the biological effect in several cell lines. Nucleolin is a protein located in the nucleolus, cytoplasm, and on the cell membrane, participating in DNA and RNA metabolism, ribosome biogenesis, and cytokinesis (114). Interestingly, nucleolin is overexpressed and partially localized on the cell surface of cancer cells (115), becoming it a preferential target for the delivery of anticancer agents that target surface nucleolin for potential effective and nontoxic cancer therapy. Mt-II, a Lys49-PLA2 isolated from Bothrops asper, interacts with the central RRM and the C-terminal R/F-GG domains of nucleolin at the cell surface and consequently occurring the internalization (116). This mechanism may explain the selective effect of PLA2s on tumor mitochondria and highlight their potential anticancer as Proleón et al. (45) have reported (Figure 1).
Three-Finger Toxins
Snake venom three-finger toxins (3FTxs) are present in the venoms of elapids (cobras, kraits, and mambas), hydrophiids (sea snakes), and some colubrids (117). The 3FTxs are characterized by three finger-like loops rich in β-strands and emerging from a dense, globular core reticulated by four highly conserved disulfide bridges (118). Commonly, they produce neuromuscular junction blocking by inhibition of nicotinic and muscarinic acetylcholine receptors or inhibition of enzyme acetylcholinesterase (119); however, other targets have been described for 3FTxs such as mitochondria in cancer cells.
Basic three-fingered S-type cardiotoxin from Naja mossambica mossambica venom translocates to mitochondria to promote apoptosis by inducing mitochondrial dysfunction in neuroblastoma cells (22). This toxin has a high net positive charge and produces fragmentation of the mitochondrial network, mitochondrial mass reduction, a decrease of the basal mitochondrial respiration and spare capacity, promoting mitochondrial permeabilization by electrostatic interaction with cardiolipin, the main phospholipid of the inner mitochondrial membrane (22). Another cardiotoxin, CTX3 from Naja atra, targets mitochondria to induce oxidative stress, Δψm decrease, and release of cytochrome c, activating the intrinsic apoptosis pathway (57). Recently, it has been described that CTX3 induces activation of the main sensor of cellular energy status, AMP-activated protein kinase (AMPK) (120, 121), which has an essential role in mitochondrial and lysosomal biogenesis (122–124). CTX3-induced mitochondrial dysfunction produces an increase of intracellular Ca2+, triggering autophagy and apoptosis by a Ca2+/protein phosphatase 2A (PP2A)/AMPK axis in leukemia cells (121).
L-Amino Acid Oxidases
L-amino acid oxidases (LAAOs) are homodimeric flavoenzymes with covalently linked-flavin adenine dinucleotides (FADs) that catalyze the oxidative deamination of L-amino acids to α-keto acids, producing ammonia and locally an excess of hydrogen peroxide (H2O2) in the vicinity of cells triggering oxidative stress and apoptosis (125, 126). LAAOs are present in the venom of Viperidae and Elapidae snake species and exhibit antimicrobial effects (127) and inhibition and activation of platelet aggregation (128–130). In cancer cells, LAAOs produce cell death through H2O2 accumulation-induced oxidative stress, which causes damage to DNA and cell membranes (131). Although the cytotoxic mechanisms are not fully understood, extensive studies have shown that LAAOs (e.g. Actx6, Actx8, and MipLAAO), decrease Δψm, inducing translocation of cytochrome c to cytosol, initiating the intrinsic apoptosis pathway (58, 60, 132, 133). Interestingly, rusvinoxidase purified from the venom of Daboia russelii russelii is a LAAO that induces apoptosis in MCF7 breast cancer cell line in a manner independent of its enzymatic activity. It produces Δψm drop accompanied by an increased ROS production, glutathione depletion, and catalase activity, producing release of cytochrome c, increase of Bak pro-apoptotic protein, and decrease anti-apoptotic proteins Bcl-XL and heat-shock proteins (HSP-90 and HSP-70) (60). Cancer cells with high catalase activity are resistant to toxin-induced death (61). This evidence suggests that intracellular ROS production is an essential step for triggering mitochondria-dependent apoptosis. In vivo experiments, rusvinoxidase is non-toxic in mice, indicating that it may be useful as a model for the development of peptide-based anticancer drugs.
Cysteine-Rich Secreted Proteins
The Cysteine-rich secreted proteins (CRISP) are proteins highly conserved that exhibit a common secondary structure that includes 16 conserved cysteine residues (134). In mammalians, CRISPs are associated with reproduction, cancer, and immune responses. They are also present in venomous glands of non-mammalian animals (e.g. snakes, spiders, scorpions, lizards, and cone snails) (134). Some snake venom CRISP (svCRISP) toxins inhibit depolarization-induced contraction in the arterial smooth muscle of rat tail, interacting with several ion channels, such as L-type Ca2+ channels (135), voltage-gated K+ channels, and ryanodine receptors (62, 64, 136). The biological effects of svCRISPs involve pro-inflammation and recruiting of neutrophils in vivo (65, 137), myotoxicity (138), antimicrobial activity (139), and increased trans-epithelial permeability (140). Natrin, a svCRISP of 221 amino acids and a molecular mass of 25-kDa, induces changes in metabolites such as phytosphingosine, 3-O-sulfogalactosylceramide, ganglioside, glycerophospholipids such as PC and PE, FAs such as lauric and palmitic acids (66). Although the natrin effect on cellular metabolism is not fully understood, these findings suggest that the natrin-induced apoptosis on cancer cells involves a rewiring of mitochondria-participating metabolic pathways such as sphingolipid/glycerophospholipid metabolism, FA biosynthesis, and oxidation (66). Future studies could explore the therapeutic potential of this metabolic phenotype induced by natrin in cancer cells.
Snake C-Type Lectin
C-type lectins are proteins that recognize and bind to specific carbohydrate domains on the cell membrane, and they are grouped into classical Ca2+- and sugar-binding lectins and the non-sugar-binding snake venom C-type lectin-related proteins (SV-CLRPs). These toxins target different coagulation factors and platelet receptors that participate in hemostasis, thrombus formation, inflammation, and metastasis with promising biomedical projections (141). Several cancer cell types under metastatic dissemination interact with platelets via podoplanin-C-type lectin-like receptor 2 (CLEC-2). CLEC-2 is a transmembrane receptor that upon activation, homodimerizes and initiates Syk-dependent signaling of collagen receptor GPVI, platelet integrin αIIbβ3, promoting platelet aggregation and thrombus formation (142–144). Interestingly, some C-type lectin from snake venom such as rhodocytin (also termed aggretin) purified from the venom of Calloselasma rhodostoma bind to CTLD domain of CLEC-2. This binding is favored by four arginine residues on its lateral face of CLEC-2 (145) and reduces the metastasis promoted by platelet-cancer cell interactions (146). Similarly, the rhodocytin α-chain C-terminus inhibits the platelet aggregation and metastatic abilities of hepatocarcinoma cells (147). Although mitochondrial metabolism is relevant in platelet aggregation (148), the effect of snake C-type lectins on platelet bioenergetics is currently unknown. Remarkably, some lights on the potential effect of snake C-type lectins on mitochondria of cancer cells can be found in the action of BlL toxin. BlL is a dimeric galactose-binding C-type lectin purified from Bothrops leucurus snake venom (149) with antibacterial and anticancer effects (150). It induces dose-dependent necrotic cell death in B16-F10 melanoma cells, without affecting the viability of keratinocytes (151). BlL increases the intracellular Ca2+ levels, and mitochondrial superoxide, and decreases Δψm, suggesting the induction of cyclosporine A-sensitive Ca2+-induced mPTP opening in cancer cells (150, 151).
Future Perspective and Conclusion
Emergent evidence suggests that several snake venom active components/toxins target the mitochondrial bioenergetics with selective migrastatic and cytotoxic effects on cancer cells. Since some of them additionally disturb platelet function, these toxins also offer novel opportunities for promising lead compounds that reduce platelet-cancer cell interaction and venous thromboembolism often seen observed in cancer. The site of toxin-cell interaction, the physical-chemistry characteristics of toxins, and the type of action on mitochondria (e.g. permeabilization of inner mitochondrial membrane, respiratory complex inhibition, cardiolipin interaction, and uncoupling of OXPHOS) are determinants for anticancer effects. Since cancer cells can exhibit elevated Δψm compared with non-tumor cells, selective accumulation of compounds linked to cationic snake peptides within mitochondria in a Δψm-dependent manner may be explored for a new anti-cancer drug delivery system. Despite the above, evaluation of efficacy, potency and safety are challenges that will need to be established for future in vivo applications.
Author Contributions
FU designed and outlined the structure and contents of the review. FU, DV-R, EF, and RA-M contributed to the literature review, discussion, and writing of the manuscript. All the authors contributed equally to the draft revisions and final approval of the version to be published.
Funding
This work was funded by Agencia Nacional de Investigación y Desarrollo (ANID-Chile) FONDECYT grants #1221874 (RA-M), #11201322 (FU), VID-University of Chile #UI-024/20 (FU), International collaboration Project ANID #Redbio0027 (FU, RA-M, DV-R, ES), Anillo grant ACT210097 (RA-M, FU), Fondo Nacional de Desarrollo Científico, Tecnológico y de Innovación Tecnológica (FONDECYT-Perú) #079-2021-FONDECYT (DV-R), Fundação de Amparo a Pesquisa do Estado de Minas Gerais (FAPEMIG-Brazil), Grant #APQ-01724-18 (ES), Conselho Nacional de Desenvolvimento Científico e Tecnológico (CNPq-Brazil), and Grant #309823/2021-8 (ES).
Conflict of Interest
The authors declare that the research was conducted in the absence of any commercial or financial relationships that could be construed as a potential conflict of interest.
Publisher’s Note
All claims expressed in this article are solely those of the authors and do not necessarily represent those of their affiliated organizations, or those of the publisher, the editors and the reviewers. Any product that may be evaluated in this article, or claim that may be made by its manufacturer, is not guaranteed or endorsed by the publisher.
References
1. Zheng J. Energy Metabolism of Cancer: Glycolysis Versus Oxidative Phosphorylation (Review). Oncol Lett (2012) 4(6):1151–7. doi: 10.3892/ol.2012.928
2. Lehuédé C, Dupuy F, Rabinovitch R, Jones R, Siegel P. Metabolic Plasticity as a Determinant of Tumor Growth and Metastasis. Cancer Res (2016) 76(18):5201–8. doi: 10.1158/0008-5472.CAN-16-0266
3. LeBleu V, O'Connell J, Gonzalez Herrera K, Wikman H, Pantel K, Haigis M, et al. PGC-1α Mediates Mitochondrial Biogenesis and Oxidative Phosphorylation in Cancer Cells to Promote Metastasis. Nat Cell Biol (2014) 10(992-1003):1–15. doi: 10.1038/ncb3039
4. Porporato P, Payen V, Pérez-Escuredo J, De Saedeleer C, Danhier P, Copetti T, et al. A Mitochondrial Switch Promotes Tumor Metastasis. Cell Rep (2014) 8(3):754–66. doi: 10.1016/j.celrep.2014.06.043
5. Park S, Chang C, Safi R, Liu X, Baldi R, Jasper J, et al. Errα-Regulated Lactate Metabolism Contributes to Resistance to Targeted Therapies in Breast Cancer. Cell Rep (2016) 15(2):323–35. doi: 10.1016/j.celrep.2016.03.026
6. Fiorillo M, Sotgia F, Sisci D, Cappello A, Lisanti M. Mitochondrial “Power” Drives Tamoxifen Resistance: NQO1 and GCLC are New Therapeutic Targets in Breast Cancer. Oncotarget (2017) 8:20309–27. doi: 10.18632/oncotarget.15852
7. Mao W, Xiong G, Wu Y, Wang C, St Clair D, Li JD, et al. Rorα Suppresses Cancer-Associated Inflammation by Repressing Respiratory Complex I-Dependent ROS Generation. Int J Mol Sci (2021) 22(19):10665. doi: 10.3390/ijms221910665
8. Caino M, Chae Y, Vaira V, Ferrero S, Nosotti M, Martin N, et al. Metabolic Stress Regulates Cytoskeletal Dynamics and Metastasis of Cancer Cells. J Clin Invest. (2013) 123(7):2907–20. doi: 10.1172/JCI67841
9. Caino MC, Seo JH, Aguinaldo A, Wait E, Bryant KG, Kossenkov AV, et al. A Neuronal Network of Mitochondrial Dynamics Regulates Metastasis. Nat Commun (2016) 7:13730. doi: 10.1038/ncomms13730
10. Wynn ML, Yates JA, Evans CR, Van Wassenhove LD, Wu ZF, Bridges S, et al. RhoC GTPase Is a Potent Regulator of Glutamine Metabolism and N-Acetylaspartate Production in Inflammatory Breast Cancer Cells. J Biol Chem (2016) 291(26):13715–29. doi: 10.1074/jbc.M115.703959
11. Bertero T, Oldham WM, Grasset EM, Bourget I, Boulter E, Pisano S, et al. Tumor-Stroma Mechanics Coordinate Amino Acid Availability to Sustain Tumor Growth and Malignancy. Cell Metab (2019) 29(1):124–40.e110. doi: 10.1016/j.cmet.2018.09.012
12. Tang S, Wang X, Shen Q, Yang X, Yu C, Cai C, et al. Mitochondrial Ca²+ Uniporter is Critical for Store-Operated Ca²+ Entry-Dependent Breast Cancer Cell Migration. Biochem Biophys Res Commun (2015) 458(1):186–93. doi: 10.1016/j.bbrc.2015.01.092
13. Urra FA, Fuentes-Retamal S, Palominos C, Rodríguez-Lucart YA, López-Torres C, Araya-Maturana R. Extracellular Matrix Signals as Drivers of Mitochondrial Bioenergetics and Metabolic Plasticity of Cancer Cells During Metastasis. Front Cell Dev Biol (2021) 9:751301. doi: 10.3389/fcell.2021.751301
14. Guimarães DO, Lopes DS, Azevedo FV, Gimenes SN, Silva MA, Achê DC, et al. In Vitro Antitumor and Antiangiogenic Effects of Bothropoidin, a Metalloproteinase From Bothrops Pauloensis Snake Venom. Int J Biol Macromol (2017) 97:770–7. doi: 10.1016/j.ijbiomac.2017.01.064
15. Li L, Huang J, Lin Y. Snake Venoms in Cancer Therapy: Past, Present and Future. Toxins (Basel) (2018) 10(9):346. doi: 10.3390/toxins10090346
16. Uzair B, Atlas N, Malik SB, Jamil N, Ojuolape ST, Rehman MU, et al. Snake Venom as an Effective Tool Against Colorectal Cancer. Protein Pept Lett (2018) 25(7):626–32. doi: 10.2174/0929866525666180614112935
17. Colella F, Scillitani G, Pierri CL. Sweet as Honey, Bitter as Bile: Mitochondriotoxic Peptides and Other Therapeutic Proteins Isolated From Animal Tissues, for Dealing With Mitochondrial Apoptosis. Toxicology (2021) 447:152612. doi: 10.1016/j.tox.2020.152612
18. Dhananjaya BL, Sivashankari PR. Snake Venom Derived Molecules in Tumor Angiogenesis and its Application in Cancer Therapy; an Overview. Curr Top Med Chem (2015) 15(7):649–57. doi: 10.2174/1568026615666150225113402
19. Urra FA, Araya-Maturana R. Targeting Metastasis With Snake Toxins: Molecular Mechanisms. Toxins (Basel) (2017) 9(12):390. doi: 10.3390/toxins9120390
20. Urra FA, Araya-Maturana R. Putting the Brakes on Tumorigenesis With Snake Venom Toxins: New Molecular Insights for Cancer Drug Discovery. Semin Cancer Biol (2022) 80:195–204. doi: 10.1016/j.semcancer.2020.05.006
21. Stábeli RG, Amui SF, Sant'Ana CD, Pires MG, Nomizo A, Monteiro MC, et al. Bothrops Moojeni Myotoxin-II, a Lys49-Phospholipase A2 Homologue: An Example of Function Versatility of Snake Venom Proteins. Comp Biochem Physiol C Toxicol Pharmacol (2006) 142(3-4):371–81. doi: 10.1016/j.cbpc.2005.11.020
22. Zhang B, Li F, Chen Z, Shrivastava IH, Gasanoff ES, Dagda RK. Naja Mossambica Mossambica Cobra Cardiotoxin Targets Mitochondria to Disrupt Mitochondrial Membrane Structure and Function. Toxins (Basel) (2019) 11(3):152. doi: 10.3390/toxins11030152
23. Vivas-Ruiz DE, Sandoval GA, Gonzalez-Kozlova E, Zarria-Romero J, Lazo F, Rodríguez E, et al. Fibrinogen-Clotting Enzyme, Pictobin, From Bothrops Pictus Snake Venom. Structural and Functional Characterization. Int J Biol Macromol (2020) 153:779–95. doi: 10.1016/j.ijbiomac.2020.03.055
24. Rádis-Baptista G, Kerkis I. Crotamine, a Small Basic Polypeptide Myotoxin From Rattlesnake Venom With Cell-Penetrating Properties. Curr Pharm Des (2011) 17(38):4351–61. doi: 10.2174/138161211798999429
25. Vivas-Ruiz D, Sandoval GA, Lazo F, Rodríguez E, Yarlequé A, Flores-Sánchez E. Characterization of Thrombin Like Enzyme from Bothrops Pictus Venom. Rev Peru Med Exp Salud Publica (2015) 32(4):652–8. doi: 10.17843/rpmesp.2015.324.1754
26. Kwong PD, McDonald NQ, Sigler PB, Hendrickson WA. Structure of Beta 2-Bungarotoxin: Potassium Channel Binding by Kunitz Modules and Targeted Phospholipase Action. Structure (1995) 3(10):1109–19. doi: 10.1016/s0969-2126(01)00246-5
27. Rugolo M, Dolly JO, Nicholls DG. The Mechanism of Action of Beta-Bungarotoxin at the Presynaptic Plasma Membrane. Biochem J (1986) 233(2):519–23. doi: 10.1042/bj2330519
28. Petersen M, Penner R, Pierau FK, Dreyer F. Beta-Bungarotoxin Inhibits a non-Inactivating Potassium Current in Guinea Pig Dorsal Root Ganglion Neurones. Neurosci Lett (1986) 68(1):141–5. doi: 10.1016/0304-3940(86)90244-2
29. Howard BD. Effects of β-Bungarotoxin on Mitochondrial Respiration are Caused by Associated Phospholipase a Activity. Biochem Biophys Res Commun (1975) 67(1):58–65. doi: 10.1016/0006-291X(75)90282-X
30. Wernicke JF, Vanker AD, Howard BD. The Mechanism of Action of β-Bungarotoxin. J Neurochemistry (1975) 25(4):483–96. doi: 10.1111/j.1471-4159.1975.tb04354.x
31. Nicholls D, Snelling R, Dolly O. Bioenergetic Actions of Beta-Bungarotoxin, Dendrotoxin and Bee-Venom Phospholipase A2 on Guinea-Pig Synaptosomes. Biochem J (1985) 229(3):653–62. doi: 10.1042/bj2290653
32. Rigoni M, Pizzo P, Schiavo G, Weston AE, Zatti G, Caccin P, et al. Calcium Influx and Mitochondrial Alterations at Synapses Exposed to Snake Neurotoxins or Their Phospholipid Hydrolysis Products. J Biol Chem (2007) 282(15):11238–45. doi: 10.1074/jbc.M610176200
33. Rigoni M, Paoli M, Milanesi E, Caccin P, Rasola A, Bernardi P, et al. Snake Phospholipase A2 Neurotoxins Enter Neurons, Bind Specifically to Mitochondria, and Open Their Transition Pores. J Biol Chem (2008) 283(49):34013–20. doi: 10.1074/jbc.M803243200
34. Fohlman J, Eaker D, Karlsoon E, Thesleff S. Taipoxin, an Extremely Potent Presynaptic Neurotoxin From the Venom of the Australian Snake Taipan (Oxyuranus s. Scutellatus). Isolation, Characterization, Quaternary Structure and Pharmacological Properties. Eur J Biochem (1976) 68(2):457–69. doi: 10.1111/j.1432-1033.1976.tb10833.x
35. Cendron L, Mičetić I, Polverino de Laureto P, Paoli M. Structural Analysis of Trimeric Phospholipase A2 Neurotoxin From the Australian Taipan Snake Venom. FEBS J (2012) 279(17):3121–35. doi: 10.1111/j.1742-4658.2012.08691.x
36. Bonanomi D, Pennuto M, Rigoni M, Rossetto O, Montecucco C, Valtorta F. Taipoxin Induces Synaptic Vesicle Exocytosis and Disrupts the Interaction of Synaptophysin I With Vamp2. Mol Pharmacol (2005) 67(6):1901–8. doi: 10.1124/mol.104.005678
37. Kirkpatrick LL, Matzuk MM, Dodds DC, Perin MS. Biochemical Interactions of the Neuronal Pentraxins. Neuronal Pentraxin (NP) Receptor Binds to Taipoxin and Taipoxin-Associated Calcium-Binding Protein 49 via NP1 and NP2. J Biol Chem (2000) 275(23):17786–92. doi: 10.1074/jbc.M002254200
38. Ritonja A, Gubensek F. Ammodytoxin A, a Highly Lethal Phospholipase A2 From Vipera Ammodytes Ammodytes Venom. Biochim Biophys Acta (1985) 828(3):306–12. doi: 10.1016/0167-4838(85)90312-7
39. Prijatelj P, Charnay M, Ivanovski G, Jenko Z, Pungercar J, Krizaj I, et al. The C-Terminal and Beta-Wing Regions of Ammodytoxin A, a Neurotoxic Phospholipase A2 From Vipera Ammodytes Ammodytes, are Critical for Binding to Factor Xa and for Anticoagulant Effect. Biochimie (2006) 88(1):69–76. doi: 10.1016/j.biochi.2005.06.015
40. Lee CY, Tsai MC, Chen YM, Ritonja A, Gubensek F. Mode of Neuromuscular Blocking Action of Toxic Phospholipases A2 From Vipera Ammodytes Venom. Arch Int Pharmacodyn Ther (1984) 268(2):313–24.
41. Šribar J, Kovačič L, Oberčkal J, Ivanušec A, Petan T, Fox JW, et al. The Neurotoxic Secreted Phospholipase A(2) From the Vipera a. Ammodytes Venom Targets Cytochrome C Oxidase in Neuronal Mitochondria. Sci Rep (2019) 9(1):283. doi: 10.1038/s41598-018-36461-6
42. Harris JB, MacDonell CA. Phospholipase A2 Activity of Notexin and its Role in Muscle Damage. Toxicon (1981) 19(3):419–30. doi: 10.1016/0041-0101(81)90046-5
43. Goncalves JM, Polson A. The Electrophoretic Analysis of Snake Venoms. Arch Biochem (1947) 13(2):253–9.
44. Coronado MA, Gabdulkhakov A, Georgieva D, Sankaran B, Murakami MT, Arni RK, et al. Structure of the Polypeptide Crotamine From the Brazilian Rattlesnake Crotalus Durissus Terrificus. Acta Crystallogr D Biol Crystallogr (2013) 69(Pt 10):1958–64. doi: 10.1107/s0907444913018003
45. Proleón A, Torrejón D, Urra FA, Lazo F, López-Torres C, Fuentes-Retamal S, et al. Functional, Immunological Characterization, and Anticancer Activity of BaMtx: A New Lys49- PLA2 Homologue Isolated From the Venom of Peruvian Bothrops Atrox Snake (Serpentes: Viperidae). Int J Biol Macromolecules (2022) 206:990–1002. doi: 10.1016/j.ijbiomac.2022.03.111
46. Chang CC, Tseng KH. Effect of Crotamine, a Toxin of South American Rattlesnake Venom, on the Sodium Channel of Murine Skeletal Muscle. Br J Pharmacol (1978) 63(3):551–9. doi: 10.1111/j.1476-5381.1978.tb07811.x
47. Oguiura N, Boni-Mitake M, Affonso R, Zhang G. In Vitro Antibacterial and Hemolytic Activities of Crotamine, a Small Basic Myotoxin From Rattlesnake Crotalus Durissus. J Antibiot (Tokyo) (2011) 64(4):327–31. doi: 10.1038/ja.2011.10
48. Nascimento FD, Sancey L, Pereira A, Rome C, Oliveira V, Oliveira EB, et al. The Natural Cell-Penetrating Peptide Crotamine Targets Tumor Tissue In Vivo and Triggers a Lethal Calcium-Dependent Pathway in Cultured Cells. Mol Pharm (2012) 9(2):211–21. doi: 10.1021/mp2000605
49. Batista da Cunha D, Pupo Silvestrini AV, Gomes da Silva AC, Maria de Paula Estevam D, Pollettini FL, de Oliveira Navarro J, et al. Mechanistic Insights Into Functional Characteristics of Native Crotamine. Toxicon (2018) 146:1–12. doi: 10.1016/j.toxicon.2018.03.007
50. Rees B, Bilwes A, Samama JP, Moras D. Cardiotoxin VII4 From Naja Mossambica Mossambica. The Refined Crystal Structure. J Mol Biol (1990) 214(1):281–97. doi: 10.1016/0022-2836(90)90161-e
51. Gorai B, Karthikeyan M, Sivaraman T. Putative Membrane Lytic Sites of P-Type and S-Type Cardiotoxins From Snake Venoms as Probed by All-Atom Molecular Dynamics Simulations. J Mol Model (2016) 22(10):238. doi: 10.1007/s00894-016-3113-y
52. Chien KY, Chiang CM, Hseu YC, Vyas AA, Rule GS, Wu W. Two Distinct Types of Cardiotoxin as Revealed by the Structure and Activity Relationship of Their Interaction With Zwitterionic Phospholipid Dispersions. J Biol Chem (1994) 269(20):14473–83. doi: 10.1039/c3cc42394h
53. Piszkiewicz S, Kirkbride EA, Doreng-Stearns N, Henderson BR, Lenker MA, Tang E, et al. Molecularly-Imprinted Nanoparticles That Recognize Naja Mossambica Cytotoxins: Binding Studies and Biological Effects. Chem Commun (Camb) (2013) 49(53):5954–6. doi: 10.1039/c3cc42394h
54. Yu C, Bhaskaran R, Yang CC. Structures in Solution of Toxins From Taiwan Cobra Venom, Naja Naja Atra, Derived From NMR Spectra. J Toxicology: Toxin Rev (1994) 13(3):291–315. doi: 10.3109/15569549409089966
55. Su SH, Su SJ, Lin SR, Chang KL. Cardiotoxin-III Selectively Enhances Activation-Induced Apoptosis of Human CD8+ T Lymphocytes. Toxicol Appl Pharmacol (2003) 193(1):97–105. doi: 10.1016/s0041-008x(03)00327-2
56. Lee SC, Lin CC, Wang CH, Wu PL, Huang HW, Chang CI, et al. Endocytotic Routes of Cobra Cardiotoxins Depend on Spatial Distribution of Positively Charged and Hydrophobic Domains to Target Distinct Types of Sulfated Glycoconjugates on Cell Surface. J Biol Chem (2014) 289(29):20170–81. doi: 10.1074/jbc.M114.557157
57. Chen KC, Chiou YL, Kao PH, Lin SR, Chang LS. Taiwan Cobra Cardiotoxins Induce Apoptotic Death of Human Neuroblastoma SK-N-SH Cells Mediated by Reactive Oxygen Species Generation and Mitochondrial Depolarization. Toxicon (2008) 51(4):624–34. doi: 10.1016/j.toxicon.2007.11.024
58. Zhang L, Wei LJ. ACTX-8, a Cytotoxic L-Amino Acid Oxidase Isolated From Agkistrodon Acutus Snake Venom, Induces Apoptosis in Hela Cervical Cancer Cells. Life Sci (2007) 80(13):1189–97. doi: 10.1016/j.lfs.2006.12.024
59. Zhang L, Wu WT. Isolation and Characterization of ACTX-6: A Cytotoxic L-Amino Acid Oxidase From Agkistrodon Acutus Snake Venom. Nat Prod Res (2008) 22(6):554–63. doi: 10.1080/14786410701592679
60. Mukherjee AK, Saviola AJ, Burns PD, Mackessy SP. Apoptosis Induction in Human Breast Cancer (MCF-7) Cells by a Novel Venom L-Amino Acid Oxidase (Rusvinoxidase) is Independent of its Enzymatic Activity and is Accompanied by Caspase-7 Activation and Reactive Oxygen Species Production. Apoptosis (2015) 20(10):1358–72. doi: 10.1007/s10495-015-1157-6
61. Mukherjee AK, Saviola AJ, Mackessy SP. Cellular Mechanism of Resistance of Human Colorectal Adenocarcinoma Cells Against Apoptosis-Induction by Russell's Viper Venom L-Amino Acid Oxidase (Rusvinoxidase). Biochimie (2018) 150:8–15. doi: 10.1016/j.biochi.2018.04.017
62. Wang F, Li H, Liu MN, Song H, Han HM, Wang QL, et al. Structural and Functional Analysis of Natrin, a Venom Protein That Targets Various Ion Channels. Biochem Biophys Res Commun (2006) 351(2):443–8. doi: 10.1016/j.bbrc.2006.10.067
63. Wang J, Shen B, Guo M, Lou X, Duan Y, Cheng XP, et al. Blocking Effect and Crystal Structure of Natrin Toxin, a Cysteine-Rich Secretory Protein From Naja Atra Venom That Targets the BKCa Channel. Biochemistry (2005) 44(30):10145–52. doi: 10.1021/bi050614m
64. Zhou Q, Wang QL, Meng X, Shu Y, Jiang T, Wagenknecht T, et al. Structural and Functional Characterization of Ryanodine Receptor-Natrin Toxin Interaction. Biophys J (2008) 95(9):4289–99. doi: 10.1529/biophysj.108.137224
65. Wang Y-L, Kuo J-H, Lee S-C, Liu J-S, Hsieh Y-C, Shih Y-T, et al. Cobra CRISP Functions as an Inflammatory Modulator via a Novel Zn2+- and Heparan Sulfate-Dependent Transcriptional Regulation of Endothelial Cell Adhesion Molecules. J Biol Chem (2010) 285(48):37872–83. doi: 10.1074/jbc.M110.146290
66. Lu S, Lu R, Song H, Wu J, Liu X, Zhou X, et al. Metabolomic Study of Natrin-Induced Apoptosis in SMMC-7721 Hepatocellular Carcinoma Cells by Ultra-Performance Liquid Chromatography-Quadrupole/Time-Of-Flight Mass Spectrometry. Int J Biol Macromol (2019) 124:1264–73. doi: 10.1016/j.ijbiomac.2018.11.060
67. Serrano SM. The Long Road of Research on Snake Venom Serine Proteinases. Toxicon (2013) 62:19–26. doi: 10.1016/j.toxicon.2012.09.003
68. Castro HC, Zingali RB, Albuquerque MG, Pujol-Luz M, Rodrigues CR. Snake Venom Thrombin-Like Enzymes: From Reptilase to Now. Cell Mol Life Sci (2004) 61(7-8):843–56. doi: 10.1007/s00018-003-3325-z
69. Vivas-Ruiz DE, Sandoval GA, Mendoza J, Inga RR, Gontijo S, Richardson M, et al. Coagulant Thrombin-Like Enzyme (Barnettobin) From Bothrops Barnetti Venom: Molecular Sequence Analysis of its cDNA and Biochemical Properties. Biochimie (2013) 95(7):1476–86. doi: 10.1016/j.biochi.2013.03.015
70. Pradniwat P, Rojnuckarin P. Snake Venom Thrombin-Like Enzymes. Toxin Rev (2014) 33(1-2):16–22. doi: 10.3109/15569543.2013.852109
71. Serrano SM, Maroun RC. Snake Venom Serine Proteinases: Sequence Homology vs. Substrate Specificity, a Paradox to be Solved. Toxicon (2005) 45(8):1115–32. doi: 10.1016/j.toxicon.2005.02.020
72. Bode W. Structure and Interaction Modes of Thrombin. Blood Cells Molecules Dis (2006) 36(2):122–30. doi: 10.1016/j.bcmd.2005.12.027
73. Stocker K, Fischer H, Meier J. Thrombin-Like Snake Venom Proteinases. Toxicon (1982) 20(1):265–73. doi: 10.1016/0041-0101(82)90225-2
74. Wu W, Kuang P, Jiang S, Yang J, Sui N, Chen A, et al. Effect of Batroxobin on Expression of C-Jun in Left Temporal Ischemic Rats With Spatial Learning and Memory Disorder. J Tradit Chin Med (2000) 20(2):147–51. doi: 10.1046/j.1365-2567.2002.01490.x
75. Yamamoto C, Tsuru D, Oda-Ueda N, Ohno M, Hattori S, Kim S-T. Flavoxobin, a Serine Protease From Trimeresurus Flavoviridis (Habu Snake) Venom, Independently Cleaves Arg726-Ser727 of Human C3 and Acts as a Novel, Heterologous C3 Convertase. Immunology (2002) 107(1):111–7. doi: 10.1046/j.1365-2567.2002.01490.x
76. Costa Jde O, Fonseca KC, Mamede CC, Beletti ME, Santos-Filho NA, Soares AM, et al. Bhalternin: Functional and Structural Characterization of a New Thrombin-Like Enzyme From Bothrops Alternatus Snake Venom. Toxicon (2010) 55(7):1365–77. doi: 10.1016/j.toxicon.2010.02.014
77. Boldrini-França J, Pinheiro-Junior EL, Peigneur S, Pucca MB, Cerni FA, Borges RJ, et al. Beyond Hemostasis: A Snake Venom Serine Protease With Potassium Channel Blocking and Potential Antitumor Activities. Sci Rep (2020) 10(1):4476. doi: 10.1038/s41598-020-61258-x
78. Obermann WMJ, Brockhaus K, Eble JA. Platelets, Constant and Cooperative Companions of Sessile and Disseminating Tumor Cells, Crucially Contribute to the Tumor Microenvironment. Front Cell Dev Biol (2021) 9:674553. doi: 10.3389/fcell.2021.674553
79. Ravi S, Chacko B, Sawada H, Kramer PA, Johnson MS, Benavides GA, et al. Metabolic Plasticity in Resting and Thrombin Activated Platelets. PloS One (2015) 10(4):e0123597. doi: 10.1371/journal.pone.0123597
80. Corona de la Peña N, Gutiérrez-Aguilar M, Hernández-Reséndiz I, Marín-Hernández Á., Rodríguez-Enríquez S. Glycoprotein Ib Activation by Thrombin Stimulates the Energy Metabolism in Human Platelets. PloS One (2017) 12(8):e0182374. doi: 10.1371/journal.pone.0182374
81. Magro AJ, Fernandes CA, dos Santos JI, Fontes MR. Influence of Quaternary Conformation on the Biological Activities of the Asp49-Phospholipases A2s From Snake Venoms. Protein Pept Lett (2009) 16(8):852–9. doi: 10.2174/092986609788923301
82. Kini RM. Excitement Ahead: Structure, Function and Mechanism of Snake Venom Phospholipase A2 Enzymes. Toxicon (2003) 42(8):827–40. doi: 10.1016/j.toxicon.2003.11.002
83. Higuchi DA, Barbosa CM, Bincoletto C, Chagas JR, Magalhaes A, Richardson M, et al. Purification and Partial Characterization of Two Phospholipases A2 From Bothrops Leucurus (White-Tailed-Jararaca) Snake Venom. Biochimie (2007) 89(3):319–28. doi: 10.1016/j.biochi.2006.10.010
84. López-Dávila AJ, Weber N, Kraft T, Matinmehr F, Arias-Hidalgo M, Fernández J, et al. Cytotoxicity of Snake Venom Lys49 PLA2-Like Myotoxin on Rat Cardiomyocytes Ex Vivo Does Not Involve a Direct Action on the Contractile Apparatus. Sci Rep (2021) 11(1):19452. doi: 10.1038/s41598-021-98594-5
85. Mamede CCN, de Sousa Simamoto BB, da Cunha Pereira DF, de Oliveira Costa J, Ribeiro MSM, de Oliveira F. Edema, Hyperalgesia and Myonecrosis Induced by Brazilian Bothropic Venoms: Overview of the Last Decade. Toxicon (2020) 187:10–8. doi: 10.1016/j.toxicon.2020.08.016
86. Cecilio AB, Caldas S, Oliveira RA, Santos AS, Richardson M, Naumann GB, et al. Molecular Characterization of Lys49 and Asp49 Phospholipases A2from Snake Venom and Their Antiviral Activities Against Dengue Virus. Toxins (Basel) (2013) 5(10):1780–98. doi: 10.3390/toxins5101780
87. Páramo L, Lomonte B, Pizarro-Cerdá J, Bengoechea JA, Gorvel JP, Moreno E. Bactericidal Activity of Lys49 and Asp49 Myotoxic Phospholipases A2 From Bothrops Asper Snake Venom–Synthetic Lys49 Myotoxin II-(115-129)-Peptide Identifies its Bactericidal Region. Eur J Biochem (1998) 253(2):452–61. doi: 10.1046/j.1432-1327.1998.2530452.x
88. Gebrim LC, Marcussi S, Menaldo DL, de Menezes CS, Nomizo A, Hamaguchi A, et al. Antitumor Effects of Snake Venom Chemically Modified Lys49 Phospholipase A2-Like BthTX-I and a Synthetic Peptide Derived From its C-Terminal Region. Biologicals (2009) 37(4):222–9. doi: 10.1016/j.biologicals.2009.01.010
89. Chen KC, Liu WH, Kao PH, Chang LS. Calcium-Stimulated Mitogen-Activated Protein Kinase Activation Elicits Bcl-xL Downregulation and Bak Upregulation in Notexin-Treated Human Neuroblastoma SK-N-SH Cells. J Cell Physiol (2010) 222(1):177–86. doi: 10.1002/jcp.21934
90. Ng RH, Howard BD. Mitochondria and Sarcoplasmic Reticulum as Model Targets for Neurotoxic and Myotoxic Phospholipases A2. Proc Natl Acad Sci (1980) 77(3):1346–50. doi: 10.1073/pnas.77.3.1346
91. Rigoni M, Caccin P, Gschmeissner S, Koster G, Postle AD, Rossetto O, et al. Equivalent Effects of Snake PLA2 Neurotoxins and Lysophospholipid-Fatty Acid Mixtures. Science (2005) 310(5754):1678–80. doi: 10.1126/science.1120640
92. Chan J, Quik M. A Role for the Nicotinic Alpha-Bungarotoxin Receptor in Neurite Outgrowth in PC12 Cells. Neuroscience (1993) 56(2):441–51. doi: 10.1016/0306-4522(93)90344-f
93. King JR, Ullah A, Bak E, Jafri MS, Kabbani N. Ionotropic and Metabotropic Mechanisms of Allosteric Modulation of α7 Nicotinic Receptor Intracellular Calcium. Mol Pharmacol (2018) 93(6):601–11. doi: 10.1124/mol.117.111401
94. Zhang C, Yu P, Zhu L, Zhao Q, Lu X, Bo S. Blockade of α7 Nicotinic Acetylcholine Receptors Inhibit Nicotine-Induced Tumor Growth and Vimentin Expression in non-Small Cell Lung Cancer Through MEK/ERK Signaling Way. Oncol Rep (2017) 38(6):3309–18. doi: 10.3892/or.2017.6014
95. Medjber K, Freidja ML, Grelet S, Lorenzato M, Maouche K, Nawrocki-Raby B, et al. Role of Nicotinic Acetylcholine Receptors in Cell Proliferation and Tumour Invasion in Broncho-Pulmonary Carcinomas. Lung Cancer (2015) 87(3):258–64. doi: 10.1016/j.lungcan.2015.01.001
96. Oberčkal J, Kovačič L, Šribar J, Leonardi A, Dolinar K, Janež PA, et al. On the Role of Protein Disulfide Isomerase in the Retrograde Cell Transport of Secreted Phospholipases A2. PloS One (2015) 10(3):e0120692. doi: 10.1371/journal.pone.0120692
97. Prijatelj P, Sribar J, Ivanovski G, Krizaj I, Gubensek F, Pungercar J. Identification of a Novel Binding Site for Calmodulin in Ammodytoxin A, a Neurotoxic Group IIA Phospholipase A2. Eur J Biochem (2003) 270(14):3018–25. doi: 10.1046/j.1432-1033.2003.03679.x
98. Petrovic U, Sribar J, Paris A, Rupnik M, Krzan M, Vardjan N, et al. Ammodytoxin, a Neurotoxic Secreted Phospholipase A(2), can Act in the Cytosol of the Nerve Cell. Biochem Biophys Res Commun (2004) 324(3):981–5. doi: 10.1016/j.bbrc.2004.09.144
99. Praznikar ZJ, Kovacic L, Rowan EG, Romih R, Rusmini P, Poletti A, et al. A Presynaptically Toxic Secreted Phospholipase A2 is Internalized Into Motoneuron-Like Cells Where it is Rapidly Translocated Into the Cytosol. Biochim Biophys Acta (2008) 1783(6):1129–39. doi: 10.1016/j.bbamcr.2008.01.011
100. Logonder U, Jenko-Praznikar Z, Scott-Davey T, Pungercar J, Krizaj I, Harris JB. Ultrastructural Evidence for the Uptake of a Neurotoxic Snake Venom Phospholipase A2 Into Mammalian Motor Nerve Terminals. Exp Neurol (2009) 219(2):591–4. doi: 10.1016/j.expneurol.2009.07.017
101. Vučemilo N, Čopič A, Gubenšek F, Križaj I. Identification of a New High-Affinity Binding Protein for Neurotoxic Phospholipases A2. Biochem Biophys Res Commun (1998) 251(1):209–12. doi: 10.1006/bbrc.1998.9427
102. Čopič A, Vučemilo N, Gubenšek F, Križaj I. Identification and Purification of a Novel Receptor for Secretory Phospholipase A2 in Porcine Cerebral Cortex*. J Biol Chem (1999) 274(37):26315–20. doi: 10.1074/jbc.274.37.26315
103. Campeiro JD, Dam W, Monte GG, Porta LC, Oliveira LCG, Nering MB, et al. Long Term Safety of Targeted Internalization of Cell Penetrating Peptide Crotamine Into Renal Proximal Tubular Epithelial Cells In Vivo. Sci Rep (2019) 9(1):3312. doi: 10.1038/s41598-019-39842-7
104. Kerkis I, Hayashi MAF, Prieto da Silva ARB, Pereira A, De Sá Júnior PL, Zaharenko AJ, et al. State of the Art in the Studies on Crotamine, a Cell Penetrating Peptide From South American Rattlesnake. BioMed Res Int (2014) 2014:675985. doi: 10.1155/2014/675985
105. Dal Mas C, Pinheiro DA, Campeiro JD, Mattei B, Oliveira V, Oliveira EB, et al. Biophysical and Biological Properties of Small Linear Peptides Derived From Crotamine, a Cationic Antimicrobial/Antitumoral Toxin With Cell Penetrating and Cargo Delivery Abilities. Biochim Biophys Acta (BBA) - Biomembranes (2017) 1859(12):2340–9. doi: 10.1016/j.bbamem.2017.09.006
106. Cardenas C, Lovy A, Silva-Pavez E, Urra F, Mizzoni C, Ahumada-Castro U, et al. Cancer Cells With Defective Oxidative Phosphorylation Require Endoplasmic Reticulum-To-Mitochondria Ca(2+) Transfer for Survival. Sci Signal (2020) 13(640):eaay1212. doi: 10.1126/scisignal.aay1212
107. Doghman-Bouguerra M, Lalli E. ER-Mitochondria Interactions: Both Strength and Weakness Within Cancer Cells. Biochim Biophys Acta Mol Cell Res (2019) 1866(4):650–62. doi: 10.1016/j.bbamcr.2019.01.009
108. Kerkhofs M, Bittremieux M, Morciano G, Giorgi C, Pinton P, Parys JB, et al. Emerging Molecular Mechanisms in Chemotherapy: Ca(2+) Signaling at the Mitochondria-Associated Endoplasmic Reticulum Membranes. Cell Death Dis (2018) 9(3):334. doi: 10.1038/s41419-017-0179-0
109. Chioato L, Ward RJ. Mapping Structural Determinants of Biological Activities in Snake Venom Phospholipases A2 by Sequence Analysis and Site Directed Mutagenesis. Toxicon (2003) 42(8):869–83. doi: 10.1016/j.toxicon.2003.11.027
110. Zambelli VO, Chioato L, Gutierrez VP, Ward RJ, Cury Y. Structural Determinants of the Hyperalgesic Activity of Myotoxic Lys49-Phospholipase a(2). J Venom Anim Toxins Incl Trop Dis (2017) 23:7. doi: 10.1186/s40409-017-0099-6
111. Cintra-Francischinelli M, Pizzo P, Rodrigues-Simioni L, Ponce-Soto LA, Rossetto O, Lomonte B, et al. Calcium Imaging of Muscle Cells Treated With Snake Myotoxins Reveals Toxin Synergism and Presence of Acceptors. Cell Mol Life Sci (2009) 66(10):1718–28. doi: 10.1007/s00018-009-9053-2
112. Birsoy K, Wang T, Chen WW, Freinkman E, Abu-Remaileh M, Sabatini DM. An Essential Role of the Mitochondrial Electron Transport Chain in Cell Proliferation Is to Enable Aspartate Synthesis. Cell (2015) 162(3):540–51. doi: 10.1016/j.cell.2015.07.016
113. Sullivan LB, Gui DY, Hosios AM, Bush LN, Freinkman E, Vander Heiden MG. Supporting Aspartate Biosynthesis Is an Essential Function of Respiration in Proliferating Cells. Cell (2015) 162(3):552–63. doi: 10.1016/j.cell.2015.07.017
114. Brignole C, Bensa V, Fonseca NA, Del Zotto G, Bruno S, Cruz AF, et al. Cell Surface Nucleolin Represents a Novel Cellular Target for Neuroblastoma Therapy. J Exp Clin Cancer Res (2021) 40(1):180. doi: 10.1186/s13046-021-01993-9
115. Hovanessian AG, Soundaramourty C, Khoury DE, Nondier I, Svab J, Krust B. Surface Expressed Nucleolin Is Constantly Induced in Tumor Cells to Mediate Calcium-Dependent Ligand Internalization. PloS One (2010) 5(12):e15787. doi: 10.1371/journal.pone.0015787
116. Massimino ML, Simonato M, Spolaore B, Franchin C, Arrigoni G, Marin O, et al. Cell Surface Nucleolin Interacts With and Internalizes Bothrops Asper Lys49 Phospholipase A2 and Mediates its Toxic Activity. Sci Rep (2018) 8(1):10619. doi: 10.1038/s41598-018-28846-4
117. Utkin YN. Three-Finger Toxins, a Deadly Weapon of Elapid Venom–Milestones of Discovery. Toxicon (2013) 62:50–5. doi: 10.1016/j.toxicon.2012.09.007
118. Kini RM, Koh CY. Snake Venom Three-Finger Toxins and Their Potential in Drug Development Targeting Cardiovascular Diseases. Biochem Pharmacol (2020) 181:114105. doi: 10.1016/j.bcp.2020.114105
119. Rowan EG, Harvey AL. Snake Toxins From Mamba Venoms: Unique Tools for the Physiologist. Acta Chim Slov (2011) 58(4):689–92.
120. Lin SC, Hardie DG. AMPK: Sensing Glucose as Well as Cellular Energy Status. Cell Metab (2018) 27(2):299–313. doi: 10.1016/j.cmet.2017.10.009
121. Chiou JT, Shi YJ, Wang LJ, Huang CH, Lee YC, Chang LS. Naja Atra Cardiotoxin 3 Elicits Autophagy and Apoptosis in U937 Human Leukemia Cells Through the Ca(2+)/PP2A/AMPK Axis. Toxins (Basel) (2019) 11(9):527. doi: 10.3390/toxins11090527
122. Fernández-Mosquera L, Diogo CV, Yambire KF, Santos GL, Luna Sánchez M, Bénit P, et al. Acute and Chronic Mitochondrial Respiratory Chain Deficiency Differentially Regulate Lysosomal Biogenesis. Sci Rep (2017) 7:45076. doi: 10.1038/srep45076
123. Fernandez-Mosquera L, Yambire KF, Couto R, Pereyra L, Pabis K, Ponsford AH, et al. Mitochondrial Respiratory Chain Deficiency Inhibits Lysosomal Hydrolysis. Autophagy (2019) 15(9):1572–91. doi: 10.1080/15548627.2019.1586256
124. Vara-Ciruelos D, Russell FM, Hardie DG. The Strange Case of AMPK and Cancer: Dr Jekyll or Mr Hyde? (†). Open Biol (2019) 9(7):190099. doi: 10.1098/rsob.190099
125. Samel M, Vija H, Rönnholm G, Siigur J, Kalkkinen N, Siigur E. Isolation and Characterization of an Apoptotic and Platelet Aggregation Inhibiting L-Amino Acid Oxidase From Vipera Berus Berus (Common Viper) Venom. Biochim Biophys Acta (2006) 1764(4):707–14. doi: 10.1016/j.bbapap.2006.01.021
126. Hiu JJ, Yap MKK. Cytotoxicity of Snake Venom Enzymatic Toxins: Phospholipase A2 and L-Amino Acid Oxidase. Biochem Soc Trans (2020) 48(2):719–31. doi: 10.1042/bst20200110
127. Salama WH, Ibrahim NM, El Hakim AE, Bassuiny RI, Mohamed MM, Mousa FM, et al. L-Amino Acid Oxidase From Cerastes Vipera Snake Venom: Isolation, Characterization and Biological Effects on Bacteria and Tumor Cell Lines. Toxicon (2018) 150:270–9. doi: 10.1016/j.toxicon.2018.06.064
128. Naumann GB, Silva LF, Silva L, Faria G, Richardson M, Evangelista K, et al. Cytotoxicity and Inhibition of Platelet Aggregation Caused by an L-Amino Acid Oxidase From Bothrops Leucurus Venom. Biochim Biophys Acta (2011) 1810(7):683–94. doi: 10.1016/j.bbagen.2011.04.003
129. Lazo F, Vivas-Ruiz DE, Sandoval GA, Rodríguez EF, Kozlova EEG, Costal-Oliveira F, et al. Biochemical, Biological and Molecular Characterization of an L-Amino Acid Oxidase (LAAO) Purified From Bothrops Pictus Peruvian Snake Venom. Toxicon (2017) 139:74–86. doi: 10.1016/j.toxicon.2017.10.001
130. Ullah A. Structure-Function Studies and Mechanism of Action of Snake Venom L-Amino Acid Oxidases. Front Pharmacol (2020) 11:110. doi: 10.3389/fphar.2020.00110
131. Tan KK, Bay BH, Gopalakrishnakone P. L-Amino Acid Oxidase From Snake Venom and its Anticancer Potential. Toxicon (2018) 144:7–13. doi: 10.1016/j.toxicon.2018.01.015
132. Zhang L, Cui L. A Cytotoxin Isolated From Agkistrodon Acutus Snake Venom Induces Apoptosis via Fas Pathway in A549 Cells. Toxicol In Vitro (2007) 21(6):1095–103. doi: 10.1016/j.tiv.2007.04.008
133. Bedoya-Medina J, Mendivil-Perez M, Rey-Suarez P, Jimenez-Del-Rio M, Núñez V, Velez-Pardo C. L-Amino Acid Oxidase Isolated From Micrurus Mipartitus Snake Venom (MipLAAO) Specifically Induces Apoptosis in Acute Lymphoblastic Leukemia Cells Mostly via Oxidative Stress-Dependent Signaling Mechanism. Int J Biol Macromolecules (2019) 134:1052–62. doi: 10.1016/j.ijbiomac.2019.05.174
134. Tadokoro T, Modahl CM, Maenaka K, Aoki-Shioi N. Cysteine-Rich Secretory Proteins (CRISPs) From Venomous Snakes: An Overview of the Functional Diversity in A Large and Underappreciated Superfamily. Toxins (Basel) (2020) 12(3):175. doi: 10.3390/toxins12030175
135. Yamazaki Y, Brown RL, Morita T. Purification and Cloning of Toxins From Elapid Venoms That Target Cyclic Nucleotide-Gated Ion Channels. Biochemistry (2002) 41(38):11331–7. doi: 10.1021/bi026132h
136. Yamazaki Y, Morita T. Structure and Function of Snake Venom Cysteine-Rich Secretory Proteins. Toxicon (2004) 44(3):227–31. doi: 10.1016/j.toxicon.2004.05.023
137. Lodovicho ME, Costa TR, Bernardes CP, Menaldo DL, Zoccal KF, Carone SE, et al. Investigating Possible Biological Targets of Bj-CRP, the First Cysteine-Rich Secretory Protein (CRISP) Isolated From Bothrops Jararaca Snake Venom. Toxicol Lett (2017) 265:156–69. doi: 10.1016/j.toxlet.2016.12.003
138. Peichoto ME, Mackessy SP, Teibler P, Tavares FL, Burckhardt PL, Breno MC, et al. Purification and Characterization of a Cysteine-Rich Secretory Protein From Philodryas Patagoniensis Snake Venom. Comp Biochem Physiol Part C: Toxicol Pharmacol (2009) 150(1):79–84. doi: 10.1016/j.cbpc.2009.03.002
139. Badari JC, Díaz-Roa A, Teixeira Rocha MM, Mendonça RZ, Silva Junior PI.da. Patagonin-CRISP: Antimicrobial Activity and Source of Antimicrobial Molecules in Duvernoy’s Gland Secretion (Philodryas Patagoniensis Snake). Front Pharmacol (2021) 11:586705. doi: 10.3389/fphar.2020.586705
140. Suntravat M, Cromer WE, Marquez J, Galan JA, Zawieja DC, Davies P, et al. The Isolation and Characterization of a New Snake Venom Cysteine-Rich Secretory Protein (Svcrisp) From the Venom of the Southern Pacific Rattlesnake and its Effect on Vascular Permeability. Toxicon (2019) 165:22–30. doi: 10.1016/j.toxicon.2019.04.006
141. Eble JA. Structurally Robust and Functionally Highly Versatile-C-Type Lectin (-Related) Proteins in Snake Venoms. Toxins (Basel) (2019) 11(3):136. doi: 10.3390/toxins11030136
142. Martins Lima A, Martins Cavaco AC, Fraga-Silva RA, Eble JA, Stergiopulos N. From Patients to Platelets and Back Again: Pharmacological Approaches to Glycoprotein VI, a Thrilling Antithrombotic Target With Minor Bleeding Risks. Thromb Haemost (2019) 119(11):1720–39. doi: 10.1055/s-0039-1695770
143. Dunster JL, Unsworth AJ, Bye AP, Haining EJ, Sowa MA, Di Y, et al. Interspecies Differences in Protein Expression do Not Impact the Spatiotemporal Regulation of Glycoprotein VI Mediated Activation. J Thromb Haemostasis (2020) 18(2):485–96. doi: 10.1111/jth.14673
144. Sanchez EF, Alvarenga VG, Oliveira LS, Oliveira DL, Estevao-Costa MI, Flores-Ortiz R, et al. A Fibrinolytic Snake Venom Metalloproteinase, Mutalysin-II, With Antiplatelet Activity and Targeting Capability Toward Glycoprotein Gpibα and Glycoprotein GPVI. Biochimie (2021) 184:1–7. doi: 10.1016/j.biochi.2021.01.016
145. Nagae M, Morita-Matsumoto K, Kato M, Kaneko MK, Kato Y, Yamaguchi Y. A Platform of C-Type Lectin-Like Receptor CLEC-2 for Binding O-Glycosylated Podoplanin and Nonglycosylated Rhodocytin. Structure (London Engl 1993) (2014) 22(12):1711–21. doi: 10.1016/j.str.2014.09.009
146. Sasaki T, Shirai T, Tsukiji N, Otake S, Tamura S, Ichikawa J, et al. Functional Characterization of Recombinant Snake Venom Rhodocytin: Rhodocytin Mutant Blocks CLEC-2/Podoplanin-Dependent Platelet Aggregation and Lung Metastasis. J Thromb Haemost (2018) 16(5):960–72. doi: 10.1111/jth.13987
147. Chang CH, Chung CH, Hsu CC, Peng HC, Huang TF. Inhibitory Effects of Polypeptides Derived From a Snake Venom C-Type Lectin, Aggretin, on Tumor Cell-Induced Platelet Aggregation. J Thromb Haemost (2014) 12(4):540–9. doi: 10.1111/jth.12519
148. Fuentes E, Araya-Maturana R, Urra FA. Regulation of Mitochondrial Function as a Promising Target in Platelet Activation-Related Diseases. Free Radic Biol Med (2019) 136:172–82. doi: 10.1016/j.freeradbiomed.2019.01.007
149. Nunes E, de Souza MAA, Vaz AF.d.M, Santana GM.d.S, Gomes FS, Coelho LCBB, et al. Purification of a Lectin With Antibacterial Activity From Bothrops Leucurus Snake Venom. Comp Biochem Physiol Part B: Biochem Mol Biol (2011) 159(1):57–63. doi: 10.1016/j.cbpb.2011.02.001
150. Nunes ES, Souza MA, Vaz AF, Silva TG, Aguiar JS, Batista AM, et al. Cytotoxic Effect and Apoptosis Induction by Bothrops Leucurus Venom Lectin on Tumor Cell Lines. Toxicon (2012) 59(7-8):667–71. doi: 10.1016/j.toxicon.2012.03.002
151. Aranda-Souza MA, Rossato FA, Costa RA, Figueira TR, Castilho RF, Guarniere MC, et al. A Lectin From Bothrops Leucurus Snake Venom Raises Cytosolic Calcium Levels and Promotes B16-F10 Melanoma Necrotic Cell Death via Mitochondrial Permeability Transition. Toxicon (2014) 82:97–103. doi: 10.1016/j.toxicon.2014.02.018
Keywords: OXPHOS (oxidative phosphorylation), electron transport chain, snake venom, cardiolipin, mitochondrial dysfunction, migrastatics, anticancer compounds
Citation: Urra FA, Vivas-Ruiz DE, Sanchez EF and Araya-Maturana R (2022) An Emergent Role for Mitochondrial Bioenergetics in the Action of Snake Venom Toxins on Cancer Cells. Front. Oncol. 12:938749. doi: 10.3389/fonc.2022.938749
Received: 08 May 2022; Accepted: 14 June 2022;
Published: 18 July 2022.
Edited by:
Gavin P. McStay, Staffordshire University, United KingdomReviewed by:
Fiorella Tonello, National Research Council (CNR), ItalyCopyright © 2022 Urra, Vivas-Ruiz, Sanchez and Araya-Maturana. This is an open-access article distributed under the terms of the Creative Commons Attribution License (CC BY). The use, distribution or reproduction in other forums is permitted, provided the original author(s) and the copyright owner(s) are credited and that the original publication in this journal is cited, in accordance with accepted academic practice. No use, distribution or reproduction is permitted which does not comply with these terms.
*Correspondence: Félix A. Urra, ZmVsaXh1cnJhZkB1LnVjaGlsZS5jbA==